- 1Department of Maternal and Child Health and Urological Sciences, Policlinico Umberto I, Sapienza University of Rome, Rome, Italy
- 2Department of Experimental Medicine, Sapienza University of Rome, Rome, Italy
- 3Department of Molecular Medicine, Sapienza University of Rome, Rome, Italy
- 4Pediatric Immunopathology and Allergology Unit, University of Rome Tor Vergata, Policlinico Tor Vergata, Rome, Italy
- 5Ph.D. Program in Immunology, Molecular Medicine and Applied Biotechnology, University of Rome Tor Vergata, Rome, Italy
- 6Department of Clinical Immunology, Royal Free Hospital, London, United Kingdom
- 7Infection, Immunity & Inflammation Department, Institute of Child Health, University College London (UCL), London, United Kingdom
- 8Diagnostic Immunology Research Unit, Multimodal Medicine Research Area, Bambino Gesù Children's Hospital, IRCCS, Rome, Italy
- 9Microbiology and Diagnostic Immunology Unit, Bambino Gesù Children's Hospital, IRCCS, Rome, Italy
- 10Department Saint Camillus International University of Health and Medical Sciences, Rome, Italy
With birth, the newborn is transferred from a quasi-sterile environment to the outside world. At this time, the neonatal immune system is inexperienced and continuously subject to a process of development as it encounters different antigenic stimuli after birth. It is initially characterized by a bias toward T helper 2 phenotype, reduced T helper 1, and cytotoxic responses to microbial stimuli, low levels of memory, and effector T and B cells and a high production of suppressive T regulatory cells. The aim of this setting, during fetal life, is to maintain an anti-inflammatory state and immune-tolerance. Maternal antibodies are transferred during pregnancy through the placenta and, in the first weeks of life of the newborn, they represent a powerful tool for protection. Thus, optimization of vaccination in pregnancy represents an important strategy to reduce the burden of neonatal infections and sepsis. Beneficial effects of maternal immunization are universally recognized, although the optimal timing of vaccination in pregnancy remains to be defined. Interestingly, the dynamic exchange that takes place at the fetal-maternal interface allows the transfer not only of antibodies, but also of maternal antigen presenting cells, probably in order to stimulate the developing fetal immune system in a harmless way. There are still controversial effects related to maternal immunization including the so called “immunology blunting,” i.e., a dampened antibody production following infant's vaccination in those infants who received placentally transferred maternal immunity. However, clinical relevance of this phenomenon is still not clear. This review will provide an overview of the evolution of the immune system in early life and discuss the benefits of maternal vaccination. Current maternal vaccination policies and their rationale will be summarized on the road to promising approaches to enhance immunity in the neonate.
Introduction
Despite significant advances in child survival in the last few decades, infectious diseases continue to be among the main causes of morbidity and mortality, especially in the neonatal period (1). Newborns are at increased risk of infections because their distinct immune system is not always able to mount an efficient protective immune response against pathogens (2).
Extended vaccination programs worldwide have significantly improved child survival by preventing infections such as polio, pertussis, smallpox, and measles (3, 4). However, when it comes to neonatal immunization, there are just a few vaccines licensed for administration in the first days of life (5). Several factors might affect programming of the immune system in early life and immune response might differ at neonatal and later ages. Thus, scarce knowledge and awareness of risks and benefits contribute to low neonatal immunization (6).
Maternal immunization has been recognized and recommended as a public health strategy to protect the mother, fetus, and infant from infections. Maternally derived pathogen specific antibodies represent a tool to protect the vulnerable infants until their immune system can adequately respond to vaccinations or infections (7). In fact, maternal antibodies are passively transferred throughout the placenta and later in colostrum and breast milk, ready to combat infections in early life (8). Optimal concentration of transplacentally transferred maternal antibodies, and the exact timing of maternal immunization, are still a matter of debate (9, 10). An open issue remains the so-called “immunology blunting,” i.e., the phenomenon by which maternal Immunoglobulin G (IgG) antibodies may dampen the response of the child to vaccination (11). On the other hand, it has been suggested that the mother may also pass immune cells to the child by placental transfer. Maternal cells may help the development of the fetal and neonatal immune system (12).
Herein, we will review some aspects of maternal, fetal and neonatal immune systems in the context of maternal immunization and highlight the current status of vaccination in pregnancy. Taken together, these data provide a framework to update our current understanding and to open new vaccine avenues in the field of immunization in pregnancy and the young infant.
Search Strategy
To retrieve information for this review, a PubMed based research was conducted using the medical subject heading database terms “vaccination” OR “immunization” AND “pregnancy.” In addition, maternal, fetal, immune system, placenta, neonate were used as search terms. We also included the currently allowed, contraindicated and in development vaccines for pregnant women, as well as the recent evidences on COVID-19. Filters for humans, any publication date and articles in English language were applied. For this narrative review, evidence was included from randomized clinical trials, original research and observational studies, case series, position statements, systematic reviews, meta-analysis studies, and selected reviews addressing the covered questions or cross-references from these publications.
Maternal and Fetal Immune System
Maternal Immune System and the Maternal-Fetal Interface
Early in pregnancy, the maternal immune system undergoes a timely regulated remodeling to allow the implantation, preservation, and growth of the semi-allogenic fetus while protecting against pathogens (13–15). Maternal and fetal immune systems establish a cooperative status (16) depending on a delicate balance between anatomic, endocrine, metabolic, and microbiome factors (17). Of note, in pregnant women, the ability to mount an adequate antibody response and immunologic memory following an infection or vaccination is not affected (18–20).
Main changes in the maternal immune system can be summarized into three phases: the 1st trimester requires a strong proinflammatory state to guarantee the implant of the blastocyst in the uterus. This process implies the break of the epithelial lining of the uterus, damage of the endometrial tissue and a rearrangement of the endothelium and vascular smooth muscle of the maternal blood vessels to establish an adequate placental–fetal blood supply (21). All these activities require an inflammatory environment to secure the adequate repair of the uterine epithelium and the removal of cellular debris; in the 2nd and 3rd trimester, the anti-inflammatory response prevails, allowing tolerance of the semi-allogenic fetus, its rapid growth and development. Finally, the switch to a new proinflammatory state favors the cascade of events that synergistically lead to parturition (22, 23). Specific cell populations mediate the transition from the pro- to the anti-inflammatory state and viceversa. First, T lymphocytes take part through a shift from a type 1 T helper lymphocytes (Th1) response, oriented toward cell-mediated immunity, toward a type 2 T helper lymphocytes (Th2) response, which favors humoral immunity by stimulating B cells to increase the production of maternal antibodies (24). Th2 lymphocyes are fundamental for the mother-child immunological tolerance up to the time of delivery, suppressing cytotoxic T cells activity (25). Moreover, T regulatory cells (Tregs), a sub-type of CD4+ T cells that express CD25, increase in pregnancy and contribute to the maintenance of immunological tolerance to the fetus by actively suppressing self-reactive lymphocytes (26, 27).
In pregnancy, the maternal immune barrier to infections enlists a combination of signals and immune modulators originating from the fetoplacental dyad (28), including the placenta and decidua. In fact, the placenta is an active site of innate immune response capable of reacting to pathogens by the release of antimicrobial peptides and cytokines (13, 16). Nevertheless, a second important site of innate immune responses is localized at the decidual layer. The maternal-fetal interface represents the direct contact between the embryo and the mother and it is populated by fetal trophoblast cells, maternal decidual stromal cells (DSCs), and decidual immune cells (DICs) (29). The decidua is an active site of chemokine synthesis throughout gestation, attracting neutrophils, natural killer cells, dendritic cells, and macrophages (17). However, the transfer of innate inflammatory mediators from the mother to the fetus is less well-understood (16). Finally, early in the second trimester, the maternal immune system protects the fetus by passive transfer of maternal antibodies (IgG) and maternal immune cells across the placenta (30).
Fetal and Neonatal Immune System
Recent technological advances have provided critical information in the field of early life human immunology with its timely and spacely development (31). The human immune system starts developing after the first 2–3 weeks of fetal life, when hematopoiesis and generation of pluripotent and self-renewing hematopoietic stem cells (HSC) begin in the yolk sac and the aorta-gonad- mesonephros region of the embryo (32). Subsequently, HSC migrate to the fetal liver, representing the major hematopoietic site during fetal life, as localization into the bone marrow occurs by week 20 (33). T cell differentiation and maturation occur in the thymus. During embryogenesis, immune cells also inhabit peripheral organs, including skin, intestine, kidney, and lung, and participate in the respective organ environment (34).
Innate immune cells are the first to emerge: granulocytes, NK cells and lymphocyte precursors are detected in the fetal circulation between gestational week (GW) 8–10 (35). From GW 8, HSCs migrate to the thymic rudiment and, by GW 16–20, mature T cells are released into the peripheral blood (36). Lymphoid precursor cells develop into B-lymphocytes with subsequent functional maturation in secondary lymphoid tissue (e.g., lymph nodes and spleen) toward the end of the first trimester (37). Thus, during the second trimester, immune cells, predominantly lymphocytes, grow in number, mature, and differentiate. Toward the end of the third trimester, the fetal immune system is functional and capable of producing a response (38).
Diverse immune cell types develop and mature at various gestational stages, which is necessary to establish feto-maternal tolerance and functional responses according to specific demands. The fetal and neonatal T-cell response is shifted toward the suppression of cytotoxicity: with a strong Th2 polarization, a dominant anti-inflammatory cytokine profile (39) and Tregs dominating the fetal circulation, suppressing reactivity to non-inherited maternal antigens (40).
At birth, the infant's response to Toll-like receptor (TLR) (i.e., innate immune response) is characterized by high production of IL-10, IL-6 and IL-23, which induce IL-17-producing helper T cells (Th17 cells) (41). The predominance of a Th17-like pattern, combined with considerable IL-10 production, may contribute to diminished T helper type 1 (Th1) responses, resulting in greater susceptibility to intracellular infections and diminished vaccine responses during infancy (42).
Since delivery, exposure to antigens and environmental challenges during infancy and childhood will promote further adaptation and expansion of the immune system (43). The exposure to the antigen rich world during the first weeks after birth leads to a massive increase in lymphocytes in a way that is largely independent of gestational age (GA) at birth. B and T cells are abundant at birth, followed by a gradual decrease over the first years of life until adulthood. As expected, the newborn presents with a lower number of memory B cells compared to older ones, reflecting the lack of exposure to foreign antigens during fetal life (33). The frequency of switched and non-switched memory B cells increases slowly with age and reaches adult levels in children 10–15 years of age (44).
Briefly, the human immune system follows a precise developmental trajectory with extraordinary plasticity in response to the different demands. However, after antigen exposure, specific protective immunity takes time; thus maternal immunization represents a great opportunity to reduce the risk of disease in the mother, fetus, and infant (30).
How Maternal Vaccination Impacts the Immune System in Early Life
Transfer of Maternal Antibodies Through the Placenta and Breast Milk
Immunization of the mother during pregnancy increases vaccine-specific antibodies to ensure effective protection not only of the mother, but also the offspring in first months of life (19) (Figure 1A). The concentration of maternal antibodies in the serum of the neonate determines the effectiveness of protection. Understanding the mechanisms by which IgGs are transferred across the placenta is important to develop optimal maternal and infant immunization strategies and make decisions about the timing of vaccination in pregnancy (45, 46).
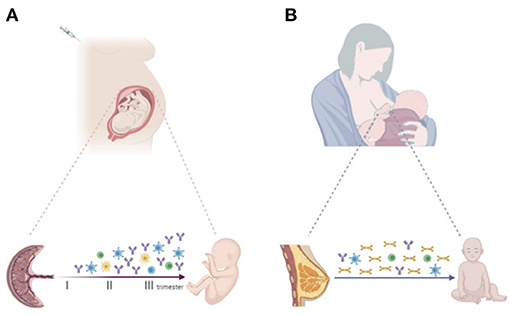
Figure 1. (A) Progressive increase of vaccine-specific antibody production and transplacental transfer after maternal immunization during pregnancy. Maternal vaccination may also impact the transfer of maternal immune cells to the offspring, priming the developing fetal immune system. (B) Breastfeeding enables the transfer of vaccine-specific maternal antibodies, mainly sIgA, and immune cells secreted through colostrum and breast milk to the infant. Created with BioRender.com.
The transfer mechanisms depend on various factors, including the syncytiotrophoblast and the capillary barrier. IgGs are selectively transported from the maternal blood to the fetus via neonatal Fc receptor (FcRn) expressed in syncytiotrophoblast of the placenta (47). FcRn is a major histocompatibility complex (MHC) class I-related Fc receptor involved in the bidirectional transcytosis of IgG and IgG immune complexes across various human epithelia. It binds the Fc fragment of Ig antibodies at acidic pH (48). In maternal blood, with a physiological pH of 7.4, IgG cannot bind the FcRn at the apical side of the syncytiotrophoblasts and need to be transported by endocytosis. In the acid environment of endosomes, FcRn-IgG complexes are then carried to the fetal side of the syncytiotrophoblast, where IgG is released upon exposure to normal pH (30). IgG subsequently crosses the villous stroma and fetal capillary endothelium and enters fetal circulation, although this mechanism is not fully understood (49).
Several factors affect the IgG transfer from the mother to the fetal circulation, i.e., the amount of FcRn expressed by syncytiotrophoblasts and total maternal IgG levels. Indeed, it was observed that when the amount of maternal IgG reaches a level of 15 g/L, FcRn is saturated and maternal antibodies stop to be transferred (50).
Placental transfer of IgG also depends on gestational age. The process starts during the first trimester of pregnancy and increases exponentially over time (51). Most of the IgG transfer occurs after 28 weeks of gestation (52). As pregnancy progresses and the placenta grows in cell mass, the expression of FcRn might increase with relatively higher antibody transport rate (53). Although in preterm infants, the reduced period of gestation leads to a lower and less efficient IgG placental transfer, several studies support the efficacy of early maternal vaccination in reducing the risk of infections at birth (54, 55). Recent UK guidance recommended pertussis immunization from 20 GW to optimize protection of preterm infants (56).
Different specificities of IgG antibodies are passively transferred to the newborn, depending on maternal immunological experience, and on the type of vaccine administered in pregnancy (57). The transfer efficacy through the placenta depends on the IgG subtype affinity for the FcRn receptor (58). IgG1 is the subtype of antibodies transferred most efficiently to the fetus, followed by IgG4, IgG3, and IgG2 (52). This differential ability of immunoglobulin to be transferred is important in the context of vaccination in pregnancy. While IgG1 and smaller amounts of IgG3 and IgG4 are induced predominantly by vaccines containing protein antigens, such as tetanus, dipheteria, pertussis (Tdap), IgG2 with some IgG1, are induced predominantly by vaccines containing polysaccharide antigens, such as those against Haemophilus influenzae type b or Neisseria meningitidis (59, 60).
Previous maternal infections are another factor that can impact the specific and amount of maternal IgG antibodies to be transported across the placenta (45), as reported in mothers infected by human immunodeficiency virus-1 (HIV-1) or malaria (61–63). To provide greater protection to the infant, specific immunization programs should be developed in childbearing age or in pregnant women with chronic infections (60).
Breastfeeding represents another mechanism by which the mother protects the neonate against pathogens in early life. Indeed, maternal secretory IgA (sIgA) and, to a lesser extent IgG and IgM, are secreted in the colostrum and breast milk and seed the luminal tract of the newborn, conferring an immunological benefit (64). In particular, sIgA provides defense at the mucosal level against pathogens of the gastrointestinal and respiratory tract through direct neutralization, inactivation, and prevention of adherence to epithelial cells of toxins and other virulence factors (65).
Several studies on the protective role of breast milk in women vaccinated during pregnancy identified a high amount of vaccine-specific sIgA in the breast milk samples up to several weeks postpartum (66). Not surprisingly, a lower incidence of respiratory illness with fever episodes in young infants of influenza-vaccinated mothers was also reported (67).
Of note, different maternal immune cell populations, including dendritic cells, macrophages, natural killers (NK), memory B and T cells are also detected in breast milk. It has been suggested that these cells may modulate the developing neonatal and infant immune system (68) (Figure 1B).
Blunting Effect of the Maternal Immune System
Despite evidences of the protective role of maternal IgG antibodies, there are concerns about their possible interference with the infant antibody response to the vaccines administered in the first months of life. It has been suggested that the presence of maternal antibodies might result in a decreased antibody production by the infant after vaccination and, consequently, in decreased protection for the child (11). This phenomenon, called “blunting,” has been associated not only to the antibodies transferred by the mother to the fetus after vaccination, but also to those generated in response to natural infection before or during pregnancy. Immune blunting has been observed in several studies regarding tetanus, diphtheria, pertussis, influenza, measles, and mumps maternal vaccination (69–71), but results are still controversial (72–74), etiopathogenesis and clinical relevance are still to be clarified (50, 75). Indeed, low antibody levels post-vaccination do not necessarily imply a low degree of protection (76). Children born to immunized mothers and vaccinated against diphtheria, tetanus, Polio, Hep B, and Hib had lower antibodies levels than children of non-vaccinated mothers. However, specific antibodies reached the seroprotective levels for all antigens (73, 77). In addition, no evidence of a clinically significant effect of blunting has been found in the United States (US) and the United Kingdom (UK), where maternal Tdap vaccination has been implemented since 2011 (US) and 2012 (UK) (78, 79).
Protection induced by vaccines may be related not only to antibody concentration, but also to their functional characteristics, such as avidity and neutralization potential, and to the generation of memory B cells, all aspects not extensively assessed yet (77). Finally, the effect of maternal antibodies on cellular immune responses after infant vaccination is another interesting aspect to consider. A recent review reporting five studies on humans concluded that pre-existing maternal antibodies do not affect infants' cellular immune response after primary vaccination (70). Specific T cell responses have been observed after immunization in the presence of maternal antibodies (71, 80). Also, maternal antibodies could prime the infant immune system to develop a stronger and long life cellular response, the so-called “prime-boost” effect (81, 82).
Transfer of Maternal Cells Through the Placenta
The fetal immune system can be primed and responds to foreign antigens during pregnancy. Increasing evidence suggests that maternal infection or vaccination during gestation may shape and train the fetal immune system even in the absence of fetal infection, affecting infants' immune responses to pathogens and vaccines during the extrauterine life (83) (Figure 1A).
The exact mechanisms by which the fetal immune system can be primed by antigen in the absence of fetal infection remain unclear. A possible explanation is the vertical transmission of a low level of antigens from the mother to the fetus. A second hypothesis is that maternal cells carrying the antigen or antigen-loaded microvesicles cross the placental barrier (83, 84). Actually, maternal cells are found in human fetal tissues from the second trimester of pregnancy onwards (85, 86). This natural phenomenon is referred to as microchimerism (Mc). It is defined as the presence in an organism of a small number of cells or amount of DNA deriving from a genetically different individual. The Mc origins from bi-directional cell exchange during pregnancy (87).
Among the accepted functions of Mc, that is worth mentioning is promoting feto-maternal tolerance and improving the outcome of future pregnancies. Feto-maternal tolerance is the process that allows fetal and maternal cells and tissues to avoid immune rejection and coexist. Fetal immune components, exposed to maternal tissues, tolerate foreign non-inherited maternal antigens (NIMAs). On the other hand, mothers during pregnancy, encompass genetically foreign paternal antigens expressed by the developing fetus. This coincides with a bidirectional transfer across the placenta of cells that seed both in maternal and fetal tissues. The presence of maternal microchimeric cells in the fetus favors the generation of fetal Tregs, which are generated in utero against NIMAs expressed by maternal cells (85) and assure fetal tolerance suppressing fetal T cells functions.
Besides promoting tolerance, Mc might be crucial to prepare the fetal immune system to face the pathogens after birth. Despite the predominant tolerogenic context, the existence of fetal memory T cells has been reported in the fetal spleen (88) and gut (89), as well as in the cord blood (90). The generation of memory T cells requires fetal exposure to foreign antigens presented by antigen-presenting cells (APC). It has been supposed that maternal APC loaded with antigen may pass the placenta and prime the fetal immune system.
Fetally memory T cells have been also found in uninfected children born to mothers infected with human immunodeficiency virus (HIV) (91), hepatitis B virus (HBV) (92), hepatitis C virus (HCV) (93), or plasmodium (94). In endemic regions, it has been observed that maternal infection by helminths, including filariasis (95), schistosomiasis (96), onchocerciasis (97), and ascariasis (98) was associated with fetal lymphocytic responses and the consequent production of specific immunoglobulins. If fetal immune cells respond to antigens of infectious agents carried by the mother, it seems reasonable that the fetal immune system may also respond to maternal antigen exposure to pathogens. Interestingly, maternal tetanus toxoid immunization led to IgM anti-tetanus antibodies in the blood of children before neonatal immunization with tetanus vaccine, showing that vaccine antigen is available and that it activates the fetal immune system (99). Of note, influenza virus-specific T cells were detected in cord blood cells of neonates whose mothers received vaccination during pregnancy (100).
The exposure to antigens mediated by maternal cells in utero might affect the development of fetal innate immune cells as well, such as macrophages and monocytes. Not surprisingly, fetal programming of monocytes is functionally different from the adult one. It has been demonstrated that in fetal monocytes stimulation with IFN-γ, IL-6, or IL-4 generate distinct JAK/STAT signaling responses, thus triggering innate responses and antimicrobial activities rather than promoting a strong adaptive and potentially harmful inflammatory response (101). Fetal monocytes can mount a more primitive, but potentially protective, innate antimicrobial response, which may also optimize the fetus and newborn's chance of successfully combating a microbial invader.
These observations add important information to understand the potential of vaccination in pregnancy including the ability to modulate the development of the fetal innate immune system (see paragraph “Future directions” on live-attenuated vaccines).
The timing of maternal vaccination can influence the transplacental transfer of vaccine antigens and the subsequent fetal immune response. In other words, the gestational age when the vaccine is administered may have an impact on priming. A study on allergen exposure in pregnancy suggested that T-cell priming with inhalant and food allergens may occur starting from 22 weeks' gestation (102).
Fetal exposure to pathogens-related antigens during pregnancy might lead to acquired protective immune responses in the offspring. However, there is still concern that it may also cause immune tolerance, increasing infants' susceptibility to both homologous and unrelated pathogens (103, 104). Non-replicating modified vaccinia Ankara was associated with reduced responses to unrelated pathogens, indicating some degree of induced tolerance (102); Diphteria-Tetanus-Pertussis (DTP) has also been shown to induce immune tolerance to unrelated antigens 3 months after vaccination, with immuno-tolerance partly restored by concurrent or subsequent BCG vaccination (105). T cell anergy and a rise in Tregs might be responsible for immune tolerance (106). Tregs have been shown to suppress antigen-specific immune responses to malaria in infants born to mothers with infection during pregnancy (107).
Maternal Vaccination in Clinical Practice
Current Recommendations
Influenza Vaccine During Pregnancy and Breastfeeding
Pregnant women have a higher risk than normal population to develop severe and life-threatening complications due to influenza (Flu) virus infections (25, 108), with an increased incidence of cardiorespiratory involvement. This could be partially explained by the physiological cardiopulmonary adaptation during pregnancy due to the welcoming fetus. Moreover, immunological alterations, with reduction of T-helper 1 cell-mediated cytotoxic T activity and B cell expansion, could also play a role (109).
Flu infection is also associated with increased risk of poor pregnancy outcomes with a higher incidence of stillbirth, preterm birth and low birth weight. During the 2009 H1N1 influenza pandemic, as reported by Callaghan et al. the pregnancy-related mortality ratio for confirmed or probable H1N1 pandemic was equal to 2.2 per 100.000 live births (110, 111). During the same period, an increased prevalence of intensive care unit requests, low Apgar scores, fetal demises, preterm births, neonatal deaths, and low birth weights has been associated with the H1N1 pandemic (112). Moreover, infants younger than 6 months of age are at risk of severe complications during flu infection and for them, an effective flu vaccine is not yet available. In fact, due to the low immunogenicity of available influenza vaccine preparations, none of these vaccines is licensed for them (25).
Under this scenario, it is not surprising that the interest for flu vaccine during pregnancy has always been high. The usage of inactivated influenza vaccines (IIV) has been reported in the US since the 1950s, with an optimal spectrum of safety. Firstly, licensed in 1997 for pregnant women in the second or third trimester, IIV has then been recommended in 2004 for all pregnant women during flu season (113). Following data of 2009 H1N1 influenza pandemic, pregnant women have been identified as one of the highest priority groups under the immunization program and, since 2012, the recommendation to use IIV has been extended by the World Health Organization (WHO) to all pregnant women, regardless of their time of gestation (114). Similarly, both the Advisory Committee on Immunization Practices (ACIP) and the American College of Obstetricians and Gynecologists (ACOG) recommend IIV for all pregnant women at any time of gestation, as well as for all women planning to get pregnant or in the postpartum phase during flu season (115–117).
Conversely, Live Attenuated Influenza Vaccine (LAIV) is contraindicated during pregnancy and data on the use of recombinant influenza vaccine (RIV) in pregnant women are limited.
Worldwide, the efficacy of the flu vaccine for both the mother and fetus has been demonstrated. In a large Norwegian study including more than 117.000 pregnant women, a significant decrease in flu diagnosis and a relevant—although not statistically significant—reduction of fetal death risk has been reported (118). Another study, conducted on 2.310 South African pregnant women, 194 of them affected by human immunodeficiency virus (HIV) infection, has shown a decrease of confirmed flu infections in HIV and non-HIV infected women (119).
No association between IIV and adverse pregnancy events, such as congenital malformations, spontaneous abortion or preterm birth, has been reported (120). Conversely, flu vaccine administration in pregnant women has been associated with a lower rate of pregnancy-related adverse events such as preterm birth, low birthweight or fetal death (118, 121).
Thus, flu vaccine administration in pregnancy and related, specific maternal antibodies, also protects the newborns during the first 6 months of life, with an estimated reduction of 72% of laboratory-confirmed influenza hospitalizations at that age (122, 123). Another study conducted by Benowitz et al. has shown that maternal immunization against flu reduces of 91.5% influenza-related hospitalizations in children < 6 months of age (124).
Finally, IIV could be administered at postpartum or while breastfeeding in women not previously vaccinated during pregnancy (120).
Tdap (Tetanus, Diphtheria, Pertussis) Vaccine During Pregnancy
Nowadays, tetanus and diphtheria are rare in industrialized countries thanks to the routine immunization programs. Due to reduced hygiene conditions, these diseases are common in low and middle-income countries, particularly in pregnant women and their offspring. The possibility to eradicate tetanus is currently unrealistic because of its intrinsic characteristics: high spread in the environment, high resistance to antimicrobial measures, and possible transmission by an open wound.
Unvaccinated pregnant women and their newborns—when unprotected by passive immunity—are at higher risk of severe complications due to tetanus disease. Also, infants born from unvaccinated mothers are unprotected against tetanus during the first months of life (25). The use of unsterile instruments and poor hygiene conditions are the main risk factors (111).
This underlines the importance of preventive measures such as vaccination programs combined with improvement of hygiene conditions and pre, peri, and postnatal care and post-exposure prophylaxis in unsafe conditions (125).
Since 1989, due to severe epidemiologic data—with 787.000 newborns death of neonatal tetanus in 1988 and an estimated annual global mortality of 6.7 per 1.000 live births—WHO promoted the Maternal and Neonatal Tetanus Elimination (MNTE) program, to reduce maternal and neonatal tetanus (MNT) so that it would no longer be a public health problem.
The MNTE initiative involves the implementation of both maternal anti-tetanus immunization and hygiene conditions during pre, peri and postnatal care practices. In 2015, thanks to MNTE efforts, a decrease of 96% in neonatal tetanus were reached, with 34.000 related deaths in newborns were reported during that year. Further progress has been made with an even more significant global reduction of deaths due to neonatal tetanus, equal to 25.000 cases in 2018. Data relative to the first seven months of 2019 have shown that neonatal tetanus has yet to be eliminated in 12 countries (126).
In order to reach a long-term immunity for tetanus, vaccine booster doses are required (125, 127). According to WHO recommendation, in previously unvaccinated pregnant women or if the immunization status is unknown, two doses of a tetanus toxoid-containing vaccine (TT-CV) are recommended, the second dose being 1 month after the first dose and at least 2 weeks before childbirth. A third dose should be administered 6 months after the second one to protect for at least 5 years. For these women who have received the first tetanus vaccine during pregnancy, two further doses should be administered after the third dose, in two subsequent years, or during two subsequent pregnancies. Conversely, in women with a previous history of 1–4 doses of tetanus vaccine administration, only one dose of TT-CV should be given (128).
While tetanus and diphtheria are uncommon in industrialized countries, pertussis is still globally endemic. Infants in the first month of life are at higher risk of severe complications, including pneumonia, apnea, encephalopathy, and multiorgan failure due to pertussis infection. Pertussis could also be fatal, mainly at this early age (109). Despite the effectiveness of the pertussis vaccine—licensed in the 1950'—in reducing morbidity and mortality, several outbreaks have occurred in different countries (129, 130).
During the 2010 pertussis outbreak in California, more than 9.000 cases have been reported, with 10 deaths, all in infants < 2 months of age, 9 of which were healthy before the infection (131). Another pertussis resurgence occurred in 2012 in the United Kingdom (UK), resulting in the death of 14 infants (25).
To reduce the burden of whooping cough in infants, both in developing and industrialized countries, WHO recommended immunization program in pregnant women. Of note, the pertussis vaccine is not administered as a mono-component but only in combination with tetanus and diphtheria.
In their statements, ACIP and WHO recommended providing one dose of Tdap to all pregnant women at each pregnancy, regardless of previous vaccine history (132). Tdap should be administered at 27–36 weeks of gestation, although it could be given at any time during pregnancy. Women who were not vaccinated during pregnancy should receive Tdap vaccine postpartum.
The choice of the last trimester as the best timing option for Tdap immunization depends on the highest concentration of maternal specific IgG and on the most efficient placental transport after 34 weeks of pregnancy as previously mentioned (109).
The safety of Tdap vaccination during pregnancy has been demonstrated across different studies. Among a cohort of 123.494 pregnant women, of whom 21% have been vaccinated with Tdap, there was no association between Tdap vaccine and adverse birth events such as preterm delivery and low birth weight (133).
Additional Vaccines in Special Circumstances
Additional vaccines can be considered to protect against infectious diseases in the case of traveling to at-risk areas or close positive contacts. Anti-hepatitis A and B, pneumococcus and meningococcus vaccines are an example of this condition (25). In the same way, further vaccines such as those against tick-borne or Japanese encephalitis should be administered if the risk of these infections is high and/or in endemic areas during disease re-surgence (6, 25).
Vaccine Contraindications
Live attenuated vaccines (LAV), including measles, mumps, rubella and varicella vaccine (MMR or MMRV), are generally contraindicated in pregnant women, as a precautionary measure, because of the theoretical risk of perinatal infection. LAV are burdened by the risk of transplacental vaccine-virus/bacterial infection. Furthermore, data regarding LAV safety during pregnancy are limited due to the exclusion of pregnant women from clinical trials (134).
However, data from accidental vaccination with MMRV in pregnant women have shown that the risk of adverse pregnancy-related events is rare (109). Evidence regarding other LAVs is lacking (135).
Live Attenuated Rubella, Measles, Mumps, and Varicella Vaccines
Rubella virus infection during pregnancy can lead to congenital rubella syndrome (CRS) as the virus has the ability to cross the placenta and infect the fetus. The risk to develop CRS is higher if rubella infection occurs in the first 12 weeks of gestation. Live attenuated rubella vaccines, licensed since the late 1960s and available for childhood immunization program in several countries, alone or in combination with measles, mumps and varicella vaccines, have lead to a notable reduction in the CRS cases (134).
As with the other LAVs, rubella vaccines are contraindicated in pregnant women, either alone or in combination with measles, mumps, and varicella.
Although wild-type rubella infection can be burdened by a higher risk of teratogenicity and intrauterine infection, inadvertent rubella vaccination during pregnancy or before conception has not been associated with a higher risk of adverse pregnancy outcomes such as congenital malformations, spontaneous abortion, prematurity, neonatal death, and low birth weight (136–138).
Of note, no CRS cases have been reported in a large study conducted in six Latin American countries between 2001 and 2008 on more than 2,800 pregnant women accidentally immunized with rubella vaccine, although anti-rubella specific IgM were detected in the cord blood of 3.5% of the newborns (139). The same is true for other studies in which no cases of CRS have been detected following accidental rubella immunization (140, 141). In a systematic review recently published by Mangtani et al. the maximum theoretic risk of CRS following accidental vaccination in pregnant women has been estimated to be 0.099% (134). Despite these data, vertical transmission of this attenuated vaccine virus has been detected (142) and some infants can develop a completely asymptomatic vaccine-virus infection (134).
Specific studies regarding the safety of mumps and measles vaccines in pregnant women are lacking, yet no evidence of a higher risk of congenital malformations and spontaneous abortion has been demonstrated (134). Similarly, no case of congenital varicella syndrome has been reported in a study conducted on 981 women inadvertently exposed to varicella vaccine (143).
At any rate, data from accidental vaccination during pregnancy is not sufficient to justify their use in pregnant women so that current guidelines contraindicate any live vaccine during pregnancy.
Table 1 summarizes maternal vaccines currently recommended, contraindicated and allowed in special circumstances during pregnancy.
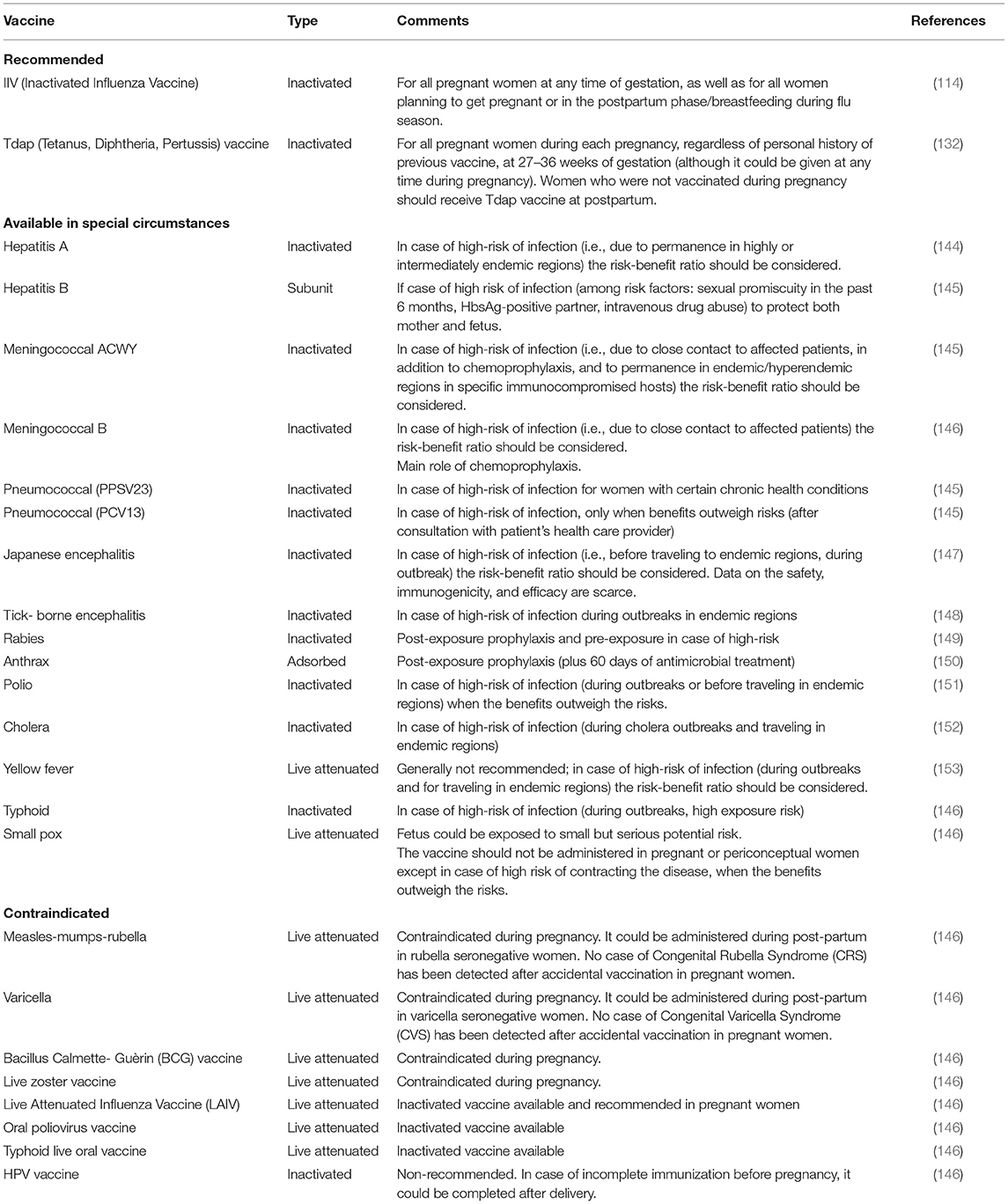
Table 1. Vaccines recommended, contraindicated, and available in special circumstances during pregnancy.
COVID-19 Vaccination in Pregnancy
SARS-CoV-2 pandemic is the major global health emergency of our century.
The infection might lead to COVID-19 disease, described mainly by upper and lower respiratory symptoms, fever and in some cases by a systemic inflammatory syndrome, with high mortality (154). Pregnant women are also susceptible to the infection with a higher risk of preterm labor and pregnancy related complications (155). Little is known on the long-term consequences of the perinatal infection in the newborn.
As COVID-19 vaccination programs have been adopted by the states to deal with the health emergency, many concerns are rising regarding the safety of the licensed COVID-19 vaccines for pregnant women.
Two mRNA based-technology vaccines Pfizer/BioNTech and Moderna, and two viral vector vaccine-type, AstraZeneca and Johnson & Johnson, have been approved by most countries worldwide including European Union, United States and United Kingdom. Others are under rolling review.
Current recommendations suggest that pregnant women should be vaccinated when the benefits outweigh the potential risks (156). Thus, pregnant workers on the frontline and those with pre-existing conditions should receive the vaccine. About 20,000 pregnant women have been vaccinated for COVID-19 in USA and a smaller number in the UK (157, 158). The data is encouraging, as no major adverse effects have raised. Moreover, one case study described specific maternal IgG anti-spike protein in a newborn whose mother was previously vaccinated (159).
While awaiting more data about the risks and benefits of the vaccine during pregnancy, these encouraging studies suggest that maternal vaccination could provide newborns with some level of protection against infection.
Future Directions
Maternal Vaccines in Development
Beyond maternal vaccines currently available, several new vaccines are in development to protect against neonatal pathogens, including respiratory syncytial virus (RSV) and group B streptococcus (GBS).
Respiratory Syncytial Virus (RSV) Vaccination
RSV is the main cause of viral lower respiratory tract infections in children, especially in the first 2 years of age, leading to significant morbidity and mortality worldwide (160). It causes bronchiolitis and pneumonia and frequently results in hospitalization and recurrent wheezing or asthma (161).
While it is widely accepted that an effective vaccine against RSV would have a major impact on child health globally, few data are available on the incidence and impact of maternal RSV infection during pregnancy. A few observational studies have shown that RSV can cause acute upper and lower respiratory tract disease in pregnant women, particularly in the third trimester of gestation (162). However, being a respiratory virus, it may act like other viral respiratory infections in pregnancy, causing consistent morbidity of the mother (163). Therefore, using an effective RSV vaccine in pregnancy could potentially benefit both the mother and the baby.
The success and efficacy of passive immunization with palivizumab in infants, which bind to antigenic site II on the RSV F protein, has led many vaccine developers to focus on this as the primary immunogen (8). Several candidate vaccines are currently under clinical development (164). A phase 3, randomized, placebo-controlled trial investigated the safety and efficacy of RSV-F nanoparticle alum-adjuvanted vaccine. This trial is the largest study so far to evaluate a vaccine primarily designed for use in pregnant women (165). It has been conducted in 11 countries and involved 4,636 healthy pregnant women at 28–36 weeks 0 days of gestation (ClinicalTrials.gov identifier: NCT02624947). The results from this trial showed that maternal RSV F vaccine had an overall adverse event profile similar to placebo. Unfortunately, the primary endpoint of RSV-associated medically significant lower respiratory tract infection up to the first 90 days of life in infants was not met. However, a possible efficacy against RSV LRTI with severe hypoxemia and RSV LRTI with hospitalization was detected (48%vaccine efficacy, 95% CI: 8.2–75 and 44% vaccine efficacy, 95%CI: 19.6–61.5) (166).
At the moment, considering the high risk of RSV infection in neonates born prematurely, a combined approach with maternal immunization followed by administration of passive antibodies for protection against RSV in early life remains the optimal strategy for preterm infants at risk (167).
Group B Streptococcus (GBS) Vaccination GBS
GBS is an important cause of neonatal pneumonia, meningitis, and sepsis. The most common current strategy to reduce early-onset neonatal sepsis is screening for GBS in pregnant women and administration of intrapartum antibiotics to those who are colonized (168). However, the incidence of late-onset disease is not substantially reduced (169).
In addition, the identification and treatment of women at risk is often difficult in settings where access to diagnostic testing and intravenous antibiotics during labor is limited, as in many low and middle-income countries (170).
Thus, immunization of pregnant women with a GBS vaccine represents a potential strategy to protect newborns from invasive GBS disease through passive immunity.
Recently, the WHO developed a document titled “Group B Streptococcus Vaccine Development Technology Roadmap,” proposing priority activities for research, development and testing of GBS vaccines, to facilitate and accelerate vaccine licensure and guarantee global availability (171). One important goal of this effort is to conduct a pivotal efficacy trial with a vaccine that includes the most relevant GBS serotypes and specific, measurable, priority endpoints, such as the prevention of stillbirth, neonatal deaths, and late-onset GBS disease and its complications (167).
Several studies have been conducted in pregnant women, with monovalent (serotype III) and trivalent (serotypes Ia, Ib, III) conjugate vaccines against GBS. These vaccines demonstrated safety and tolerability and efficient transplacental antibody transfer to the infant. However, for the moment, none have yet entered Phase 3 development (8, 167).
The Potential of Live-Attenuated Vaccines in Pregnancy
Live-attenuated vaccines (BCG, measles, mumps, rubella, smallpox, chickenpox, yellow fever) generally have the advantage of single-dose, rapid induction and durable immunity compared to inactivated vaccines. Epidemiological studies have also suggested that live vaccines extend protective effects toward unrelated pathogens (172). This unexpected pathogen-agnostic protection has been demonstrated for the measles, oral polio vaccine (OPV), BCG, and smallpox vaccines (3, 173–176). Heterologous immunity and trained immunity might favor the occurrence of an agnostic immunity that broadens beyond the specific immune response to vaccine antigens (177).
However, LAV are currently contraindicated during pregnancy because of the potential risk of causing infection and subsequent transplacental transmission of the pathogen to the developing fetus, recent systematic review and meta-analysis on maternal immunization safety with LAV mostly demonstrated no or very low risk of adverse pregnancy outcomes (135). Randomized clinical trials (RCTs) and non-RCTs studies should be performed to investigate the potential of vaccination with live vaccines in pregnancy as a tool to protect the mother and the newborn from infectious diseases.
Challenges to Be Met With Maternal Vaccines
Although national and international health authorities have defined tetanus, pertussis, and flu maternal immunization schedules, vaccine uptake remains unsatisfactory. In fact, apart from few studies in the UK and US reporting a Tdap and flu uptake between 40 and 70% (refer to these studies), in most European countries vaccine coverage rarely reaches >10% (165). Several studies have investigated potential factors affecting pregnant women's awareness and behavior on seasonal flu and pertussis vaccine compliances (178). Different cultural, social, economic, and structural barriers need to be overcome (45, 179). Within our Italian project called VaxInPerMam, at Tor Vergata University Hospital, ~1,000 childbearing Italian women have been surveyed to evaluate flu and pertussis vaccine attitude and uptake. Less than 40% of women knew maternal immunization recommendations, and, mainly due to safety concerns, <10% would receive pertussis and flu vaccines (manuscript in preparation). Misinformation represents a key factor affecting vaccine uptake. Specific intervention programs directed at both the healthcare provider and the mother-to-be are required to overcome barriers and prejudices (180).
Conclusions
Maternal immunological experience protects the child in the early postnatal period when the risk of infection is highest. Vertically transferred maternal antibodies of IgG isotype exert protection in the early postnatal period when the neonate has not yet been able to produce its own antibodies. Maternal IgA and IgG antibodies are also transferred through breastfeeding and increased levels of both isotypes can be detected in breastmilk after vaccination during pregnancy. Moreover, neonatal immune cells may be stimulated by maternal cells and antigens transferred in utero, with evidence of both activating and tolerogenic impacts on fetal immune components.
Maternal vaccination has already been shown protect the newborn from severe infections and currently represents the best defense option against various pathogens. The vaccines currently administered to pregnant women have excellent safety profiles. Strategies to overcome vaccine hesitancy include education on the importance of immunization in pregnancy through specific public awareness campaigns. Healthcare providers' recommendations are essential to increase vaccination coverage during pregnancy and for further advancement of a maternal immunization programs. Finally, a better understanding of the mother-child immunological link and of early life immunological steps will sustain further vaccine research and development to combat the surge of infectious diseases with benefit of the entire population.
Author Contributions
BC, MC, MS, VM, and MD contributed to the conception and design of the work. BC, MC, and MS wrote the manuscript. GT, RE, RC, AF, EP, GB, MDC, AZ, VM, and MD revised it carefully for important intellectual content. All authors contributed to the manuscript revision, read, and approved the submitted version.
Conflict of Interest
The authors declare that the research was conducted in the absence of any commercial or financial relationships that could be construed as a potential conflict of interest.
References
1. Popescu CR, Cavanagh MMM, Tembo B, Chiume M, Lufesi N, Goldfarb DM, et al. Neonatal sepsis in low-income countries: epidemiology, diagnosis and prevention. Exp Rev Anti Infect Ther. (2020) 18:443–52. doi: 10.1080/14787210.2020.1732818
2. Dowling DJ, Levy O. Ontogeny of early life immunity. Trends Immunol. (2014) 35:299–310. doi: 10.1016/j.it.2014.04.007
3. Aaby P, Martins CL, Garly M-L, Andersen A, Fisker AB, Claesson MH, et al. Measles vaccination in the presence or absence of maternal measles antibody: impact on child survival. Clin Infect Dis. (2014) 59:484–92. doi: 10.1093/cid/ciu354
4. Gans H, Yasukawa L, Rinki M, DeHovitz R, Forghani B, Beeler J, Audet S, et al. Immune responses to measles and mumps vaccination of infants at 6, 9, and 12 months. J Infect Dis. (2001) 184:817–26. doi: 10.1086/323346
5. Whittaker E, Goldblatt D, McIntyre P, Levy O. Neonatal immunization: rationale, current state, and future prospects. Front Immunol. (2018) 9:532. doi: 10.3389/fimmu.2018.00532
6. Saso A, Kampmann B. Vaccine responses in newborns. Semin Immunopathol. (2017) 39:627–42. doi: 10.1007/s00281-017-0654-9
7. Marshall H, McMillan M, Andrews RM, Macartney K, Edwards K. Vaccines in pregnancy: the dual benefit for pregnant women and infants. Hum Vaccin Immunother. (2016) 12:848–56. doi: 10.1080/21645515.2015.1127485
8. Perrett KP, Nolan TM. Immunization during pregnancy: impact on the infant. Paediatr Drugs. (2017) 19:313–24. doi: 10.1007/s40272-017-0231-7
9. Albrecht M, Arck PC. Vertically transferred immunity in neonates: mothers, mechanisms and mediators. Front Immunol. (2020) 11:555. doi: 10.3389/fimmu.2020.00555
10. Psarris A, Sindos M, Daskalakis G, Chondrogianni ME, Panayiotou S, Antsaklis P, et al. Immunizations during pregnancy: how, when and why. Eur J Obstet Gynecol Reprod Biol. (2019) 240:29–35. doi: 10.1016/j.ejogrb.2019.06.019
11. Bento AI, Rohani P. Forecasting epidemiological consequences of maternal immunization. Clin Infect Dis. (2016) 63:S205–12. doi: 10.1093/cid/ciw557
12. Wilcox CR, Jones CE. Beyond passive immunity: is there priming of the fetal immune system following vaccination in pregnancy and what are the potential clinical implications? Front Immunol. (2018) 9:1548. doi: 10.3389/fimmu.2018.01548
13. Mor G, Aldo P, Alvero AB. The unique immunological and microbial aspects of pregnancy. Nat Rev Immunol. (2017) 17:469–82. doi: 10.1038/nri.2017.64
14. Nguyen TG, Ward CM, Morris JM. To B or not to B cells-mediate a healthy start to life. Clin Exp Immunol. (2013) 171:124–34. doi: 10.1111/cei.12001
15. Muzzio D, Zenclussen AC, Jensen F. The role of B cells in pregnancy: the good and the bad. Am J Reprod Immunol. (2013) 69:408–12. doi: 10.1111/aji.12079
16. Yockey LJ, Iwasaki A. Interferons and proinflammatory cytokines in pregnancy and fetal development. Immunity. (2018) 49:397–412. doi: 10.1016/j.immuni.2018.07.017
17. Olmos-Ortiz A, Flores-Espinosa P, Mancilla-Herrera I, Vega-Sánchez R, Díaz L, Zaga-Clavellina V. Innate immune cells and toll-like receptor-dependent responses at the maternal-fetal interface. Int J Mol Sci. (2019) 20:E3654. doi: 10.3390/ijms20153654
18. Mor G, Cardenas I. The immune system in pregnancy: a unique complexity. Am J Reprod Immunol. (2010) 63:425–33. doi: 10.1111/j.1600-0897.2010.00836.x
19. Kachikis A, Eckert LO, Englund J. Who's the target: mother or baby? Viral Immunol. (2018) 31:184–94. doi: 10.1089/vim.2017.0135
20. Zenclussen AC. Adaptive immune responses during pregnancy. Am J Reprod Immunol. (2013) 69:291–303. doi: 10.1111/aji.12097
21. Osol G, Mandala M. Maternal uterine vascular remodeling during pregnancy. Physiology. (2009) 24:58–71. doi: 10.1152/physiol.00033.2008
22. Dekel N, Gnainsky Y, Granot I, Racicot K, Mor G. The role of inflammation for a successful implantation. Am J Reprod Immunol. (2014) 72:141–7. doi: 10.1111/aji.12266
23. Mor G, Cardenas I, Abrahams V, Guller S. Inflammation and pregnancy: the role of the immune system at the implantation site. Ann N Y Acad Sci. (2011) 1221:80–7. doi: 10.1111/j.1749-6632.2010.05938.x
24. Erlebacher A. Immunology of the maternal-fetal interface. Annu Rev Immunol. (2013) 31:387–411. doi: 10.1146/annurev-immunol-032712-100003
25. Vojtek I, Dieussaert I, Doherty TM, Franck V, Hanssens L, Miller J, et al. Maternal immunization: where are we now and how to move forward? Ann Med. (2018) 50:193–208. doi: 10.1080/07853890.2017.1421320
26. Somerset DA, Zheng Y, Kilby MD, Sansom DM, Drayson MT. Normal human pregnancy is associated with an elevation in the immune suppressive CD25+ CD4+ regulatory T-cell subset. Immunology. (2004) 112:38–43. doi: 10.1111/j.1365-2567.2004.01869.x
27. Zhang Y-H, Sun H-X. Immune checkpoint molecules in pregnancy: focus on regulatory T cells. Eur J Immunol. (2020) 50:160–9. doi: 10.1002/eji.201948382
28. Racicot K, Kwon J-Y, Aldo P, Silasi M, Mor G. Understanding the complexity of the immune system during pregnancy. Am J Reprod Immunol. (2014) 72:107–16. doi: 10.1111/aji.12289
29. Chang R-Q, Zhou W-J, Li D-J, Li M-Q. Innate lymphoid cells at the maternal-fetal interface in human pregnancy. Int J Biol Sci. (2020) 16:957–69. doi: 10.7150/ijbs.38264
30. Palmeira P, Quinello C, Silveira-Lessa AL, Zago CA, Carneiro-Sampaio M. IgG placental transfer in healthy and pathological pregnancies. Clin Dev Immunol. (2012) 2012:985646. doi: 10.1155/2012/985646
31. Park J-E, Jardine L, Gottgens B, Teichmann SA, Haniffa M. Prenatal development of human immunity. Science. (2020) 368:600–3. doi: 10.1126/science.aaz9330
32. Tavian M, Biasch K, Sinka L, Vallet J, Péault B. Embryonic origin of human hematopoiesis. Int J Dev Biol. (2010) 54:1061–5. doi: 10.1387/ijdb.103097mt
33. Ygberg S, Nilsson A. The developing immune system - from foetus to toddler. Acta Paediatr. (2012) 101:120–7. doi: 10.1111/j.1651-2227.2011.02494.x
34. Rijkers GT, Niers T, de Jager W, Janssens P, Gaiser K, Wiertsema S, et al. Development of The Immune System In The Foetal and Perinatal Period. In: Smit Sibinga TH, Luban N, editors. Neonatology and Blood Transfusion: Proceedings of the Twenty-Eighth International Symposium on Blood Transfusion, Groningen, NL Organized by the Sanquin Division Blood Bank Noord Nederland Developments in Hematology and Immunology. Boston, MA: Springer US (2005). p. 25–30.
35. Gollwitzer ES, Marsland BJ. Impact of early-life exposures on immune maturation and susceptibility to disease. Trends Immunol. (2015) 36:684–96. doi: 10.1016/j.it.2015.09.009
36. Germain RN. T-cell development and the CD4-CD8 lineage decision. Nat Rev Immunol. (2002) 2:309–22. doi: 10.1038/nri798
37. LeBien TW, Tedder TF. B lymphocytes: how they develop and function. Blood. (2008) 112:1570–80. doi: 10.1182/blood-2008-02-078071
38. Rechavi E, Somech R. Maturation of the immune system in the fetus and the implications for congenital CMV. Best Pract Res Clin Obstet Gynaecol. (2019) 60:35–41. doi: 10.1016/j.bpobgyn.2019.03.002
39. Goenka A, Kollmann TR. Development of immunity in early life. J Infect. (2015) 71(Suppl 1):S112–20. doi: 10.1016/j.jinf.2015.04.027
40. Mold JE, Venkatasubrahmanyam S, Burt TD, Michaëlsson J, Rivera JM, Galkina SA, et al. Fetal and adult hematopoietic stem cells give rise to distinct T cell lineages in humans. Science. (2010) 330:1695–9. doi: 10.1126/science.1196509
41. Levy O. Innate immunity of the newborn: basic mechanisms and clinical correlates. Nat Rev Immunol. (2007) 7:379–90. doi: 10.1038/nri2075
42. PrabhuDas M, Adkins B, Gans H, King C, Levy O, Ramilo O, Siegrist C-A. Challenges in infant immunity: implications for responses to infection and vaccines. Nat Immunol. (2011) 12:189–94. doi: 10.1038/ni0311-189
43. Pagenkemper M, Diemert A. Monitoring fetal immune development in human pregnancies: current concepts and future goals. J Reprod Immunol. (2014) 104–105:49–53. doi: 10.1016/j.jri.2014.06.001
44. Aranburu A, Piano Mortari E, Baban A, Giorda E, Cascioli S, Marcellini V, et al. Human B-cell memory is shaped by age- and tissue-specific T-independent and GC-dependent events. Eur J Immunol. (2017) 47:327–44. doi: 10.1002/eji.201646642
45. Bergin N, Murtagh J, Philip RK. Maternal vaccination as an essential component of life-course immunization and its contribution to preventive neonatology. Int J Environ Res Public Health. (2018) 15:847. doi: 10.3390/ijerph15050847
46. Calvert A, Jones CE. Placental transfer of antibody and its relationship to vaccination in pregnancy. Curr Opin Infect Dis. (2017) 30:268–73. doi: 10.1097/QCO.0000000000000372
47. Simister NE. Placental transport of immunoglobulin G. Vaccine. (2003) 21:3365–9. doi: 10.1016/S0264-410X(03)00334-7
48. Wilcox CR, Holder B, Jones CE. Factors affecting the fcrn-mediated transplacental transfer of antibodies and implications for vaccination in pregnancy. Front Immunol. (2017) 8:1294. doi: 10.3389/fimmu.2017.01294
49. Fouda GG, Martinez DR, Swamy GK, Permar SR. The impact of IgG transplacental transfer on early life immunity. Immunohorizons. (2018) 2:14–25. doi: 10.4049/immunohorizons.1700057
50. Niewiesk S. Maternal antibodies: clinical significance, mechanism of interference with immune responses, and possible vaccination strategies. Front Immunol. (2014) 5:446. doi: 10.3389/fimmu.2014.00446
51. Bright NA, Ockleford CD. Cytotrophoblast cells: a barrier to maternofetal transmission of passive immunity. J Histochem Cytochem. (1995) 43:933–44. doi: 10.1177/43.9.7642966
52. Malek A, Sager R, Kuhn P, Nicolaides KH, Schneider H. Evolution of maternofetal transport of immunoglobulins during human pregnancy. Am J Reprod Immunol. (1996) 36:248–55. doi: 10.1111/j.1600-0897.1996.tb00172.x
53. Lozano NA, Lozano A, Marini V, Saranz RJ, Blumberg RS, Baker K, et al. Expression of FcRn receptor in placental tissue and its relationship with IgG levels in term and preterm newborns. Am J Reprod Immunol. (2018) 80:e12972. doi: 10.1111/aji.12972
54. Katz J, Englund JA, Steinhoff MC, Khatry SK, Shrestha L, Kuypers J, et al. Impact of timing of influenza vaccination in pregnancy on transplacental antibody transfer, influenza incidence, and birth outcomes: a randomized trial in Rural Nepal. Clin Infect Dis. (2018) 67:334–40. doi: 10.1093/cid/ciy090
55. Kent A, Ladhani SN, Andrews NJ, Matheson M, England A, Miller E, et al. Pertussis antibody concentrations in infants born prematurely to mothers vaccinated in pregnancy. Pediatrics. (2016) 138:e20153854. doi: 10.1542/peds.2015-3854
56. Byrne L, Campbell H, Andrews N, Ribeiro S, Amirthalingam G. Hospitalisation of preterm infants with pertussis in the context of a maternal vaccination programme in England. Arch Dis Child. (2018) 103:224–9. doi: 10.1136/archdischild-2016-311802
57. Post AL, Li SH, Berry M, Itell H, Martinez DR, Xie G, et al. Efficiency of placental transfer of vaccine-elicited antibodies relative to prenatal Tdap vaccination status. Vaccine. (2020) 38:4869–76. doi: 10.1016/j.vaccine.2020.05.036
58. Mimoun A, Delignat S, Peyron I, Daventure V, Lecerf M, Dimitrov JD, et al. Relevance of the materno-fetal interface for the induction of antigen-specific immune tolerance. Front Immunol. (2020) 11:810. doi: 10.3389/fimmu.2020.00810
59. Vidarsson G, Dekkers G, Rispens T. IgG subclasses and allotypes: from structure to effector functions. Front Immunol. (2014) 5:520. doi: 10.3389/fimmu.2014.00520
60. Faucette AN, Unger BL, Gonik B, Chen K. Maternal vaccination: moving the science forward. Hum Reprod Update. (2015) 21:119–35. doi: 10.1093/humupd/dmu041
61. Cumberland P, Shulman CE, Maple PAC, Bulmer JN, Dorman EK, Kawuondo K, et al. Maternal HIV infection and placental malaria reduce transplacental antibody transfer and tetanus antibody levels in newborns in Kenya. J Infect Dis. (2007) 196:550–7. doi: 10.1086/519845
62. de Moraes-Pinto MI, Verhoeff F, Chimsuku L, Milligan PJ, Wesumperuma L, Broadhead RL, et al. Placental antibody transfer: influence of maternal HIV infection and placental malaria. Arch Dis Child Fetal Neonatal Ed. (1998) 79:F202–5. doi: 10.1136/fn.79.3.F202
63. Nunes MC, Cutland CL, Dighero B, Bate J, Jones S, Hugo A, et al. Kinetics of hemagglutination-inhibiting antibodies following maternal influenza vaccination among mothers with and those without HIV infection and their infants. J Infect Dis. (2015) 212:1976–87. doi: 10.1093/infdis/jiv339
64. Hanson LA. Breastfeeding provides passive and likely long-lasting active immunity. Ann Allergy Asthma Immunol. (1998) 81:523–33; quiz 533–534, 537. doi: 10.1016/S1081-1206(10)62704-4
65. Andreas NJ, Kampmann B, Mehring Le-Doare K. Human breast milk: a review on its composition and bioactivity. Early Hum Dev. (2015) 91:629–35. doi: 10.1016/j.earlhumdev.2015.08.013
66. Maertens K, De Schutter S, Braeckman T, Baerts L, Van Damme P, De Meester I, et al. Breastfeeding after maternal immunisation during pregnancy: providing immunological protection to the newborn: a review. Vaccine. (2014) 32:1786–92. doi: 10.1016/j.vaccine.2014.01.083
67. Schlaudecker EP, Steinhoff MC, Omer SB, McNeal MM, Roy E, Arifeen SE, et al. IgA and neutralizing antibodies to influenza a virus in human milk: a randomized trial of antenatal influenza immunization. PLoS ONE. (2013) 8:e70867. doi: 10.1371/journal.pone.0070867
68. Molès J-P, Tuaillon E, Kankasa C, Bedin A-S, Nagot N, Marchant A, et al. Breastfeeding-related maternal microchimerism. Nat Rev Immunol. (2017) 17:729–21. doi: 10.1038/nri.2017.115
69. Voysey M, Kelly DF, Fanshawe TR, Sadarangani M, O'Brien KL, Perera R, et al. The influence of maternally derived antibody and infant age at vaccination on infant vaccine responses: an individual participant meta-analysis. JAMA Pediatr. (2017) 171:637–46. doi: 10.1001/jamapediatrics.2017.0638
70. Orije MRP, Maertens K, Corbière V, Wanlapakorn N, Van Damme P, Leuridan E, et al. The effect of maternal antibodies on the cellular immune response after infant vaccination: a review. Vaccine. (2020) 38:20–8. doi: 10.1016/j.vaccine.2019.10.025
71. Gans H, DeHovitz R, Forghani B, Beeler J, Maldonado Y, Arvin AM. Measles and mumps vaccination as a model to investigate the developing immune system: passive and active immunity during the first year of life. Vaccine. (2003) 21:3398–405. doi: 10.1016/S0264-410X(03)00341-4
72. Jones C, Pollock L, Barnett SM, Battersby A, Kampmann B. The relationship between concentration of specific antibody at birth and subsequent response to primary immunization. Vaccine. (2014) 32:996–1002. doi: 10.1016/j.vaccine.2013.11.104
73. Zimmermann P, Perrett KP, Messina NL, Donath S, Ritz N, van der Klis FRM, et al. The effect of maternal immunisation during pregnancy on infant vaccine responses. EClinicalMedicine. (2019) 13:21–30. doi: 10.1016/j.eclinm.2019.06.010
74. Njie-Jobe J, Nyamweya S, Miles DJC, van der Sande M, Zaman S, Touray E, et al. Immunological impact of an additional early measles vaccine in Gambian children: responses to a boost at 3 years. Vaccine. (2012) 30:2543–50. doi: 10.1016/j.vaccine.2012.01.083
75. Siegrist C-A, Aspinall R. B-cell responses to vaccination at the extremes of age. Nat Rev Immunol. (2009) 9:185–94. doi: 10.1038/nri2508
76. Kollmann TR, Kampmann B, Mazmanian SK, Marchant A, Levy O. Protecting the newborn and young infant from infectious diseases: lessons from immune ontogeny. Immunity. (2017) 46:350–63. doi: 10.1016/j.immuni.2017.03.009
77. Kandeil W, Savic M, Ceregido MA, Guignard A, Kuznetsova A, Mukherjee P. Immune interference. (blunting) in the context of maternal immunization with Tdap-containing vaccines: is it a class effect? Exp Rev Vaccines. (2020) 19:341–52. doi: 10.1080/14760584.2020.1749597
78. Amirthalingam G, Campbell H, Ribeiro S, Fry NK, Ramsay M, Miller E, et al. Sustained effectiveness of the maternal pertussis immunization program in England 3 years following introduction. Clin Infect Dis. (2016) 63:S236–43. doi: 10.1093/cid/ciw559
79. Becker-Dreps S, Butler AM, McGrath LJ, Boggess KA, Weber DJ, Li D, et al. Effectiveness of prenatal tetanus, diphtheria, acellular pertussis vaccination in the prevention of infant pertussis in the U.S. Am J Prev Med. (2018) 55:159–66. doi: 10.1016/j.amepre.2018.04.013
80. Gans HA, Yasukawa LL, Alderson A, Rinki M, DeHovitz R, Beeler J, et al. Humoral and cell-mediated immune responses to an early 2-dose measles vaccination regimen in the United States. J Infect Dis. (2004) 190:83–90. doi: 10.1086/421032
81. Gans HA, Yasukawa LL, Sung P, Sullivan B, DeHovitz R, Audet S, et al. Measles humoral and cell-mediated immunity in children aged 5-10 years after primary measles immunization administered at 6 or 9 months of age. J Infect Dis. (2013) 207:574–82. doi: 10.1093/infdis/jis719
82. Bertley FMN, Ibrahim SA, Libman M, Ward BJ. Measles vaccination in the presence of maternal antibodies primes for a balanced humoral and cellular response to revaccination. Vaccine. (2004) 23:444–9. doi: 10.1016/j.vaccine.2004.06.021
83. Jones CE, Hesseling AC, Tena-Coki NG, Scriba TJ, Chegou NN, Kidd M, et al. The impact of HIV exposure and maternal Mycobacterium tuberculosis infection on infant immune responses to bacille Calmette-Guérin vaccination. AIDS. (2015) 29:155–65. doi: 10.1097/QAD.0000000000000536
84. Miles DJC, Gadama L, Gumbi A, Nyalo F, Makanani B, Heyderman RS. Human immunodeficiency virus. (HIV) infection during pregnancy induces CD4 T-cell differentiation and modulates responses to Bacille Calmette-Guérin. (BCG) vaccine in HIV-uninfected infants. Immunology. (2010) 129:446–54. doi: 10.1111/j.1365-2567.2009.03186.x
85. Mold JE, Michaëlsson J, Burt TD, Muench MO, Beckerman KP, Busch MP, et al. Maternal alloantigens promote the development of tolerogenic fetal regulatory T cells in utero. Science. (2008) 322:1562–5. doi: 10.1126/science.1164511
86. Jonsson AM, Uzunel M, Götherström C, Papadogiannakis N, Westgren M. Maternal microchimerism in human fetal tissues. Am J Obstet Gynecol. (2008) 198:325.e1–6. doi: 10.1016/j.ajog.2007.09.047
87. Paul WE. Self/Nonself-immune recognition and signaling: a new journal tackles a problem at the center of immunological science. Self Nonself . (2010) 1:2–3. doi: 10.4161/self.1.1.10682
88. Sprent J, Surh CD. Normal T cell homeostasis: the conversion of naive cells into memory-phenotype cells. Nat Immunol. (2011) 12:478–84. doi: 10.1038/ni.2018
89. Bunders MJ, van der Loos CM, Klarenbeek PL, van Hamme JL, Boer K, Wilde JCH, et al. Memory CD4(+)CCR5(+) T cells are abundantly present in the gut of newborn infants to facilitate mother-to-child transmission of HIV-1. Blood. (2012) 120:4383–90. doi: 10.1182/blood-2012-06-437566
90. Crespo M, Martinez DG, Cerissi A, Rivera-Reyes B, Bernstein HB, Lederman MM, et al. Neonatal T-cell maturation and homing receptor responses to Toll-like receptor ligands differ from those of adult naive T cells: relationship to prematurity. Pediatr Res. (2012) 71:136–43. doi: 10.1038/pr.2011.26
91. Rowland-Jones SL, Nixon DF, Aldhous MC, Gotch F, Ariyoshi K, Hallam N, et al. HIV-specific cytotoxic T-cell activity in an HIV-exposed but uninfected infant. Lancet. (1993) 341:860–1. doi: 10.1016/0140-6736(93)93063-7
92. Koumbi L, Bertoletti A, Anastasiadou V, Machaira M, Goh W, Papadopoulos NG, et al. Hepatitis B-specific T helper cell responses in uninfected infants born to HBsAg+/HBeAg- mothers. Cell Mol Immunol. (2010) 7:454–8. doi: 10.1038/cmi.2010.34
93. Babik JM, Cohan D, Monto A, Hartigan-O'Connor DJ, McCune JM. The human fetal immune response to hepatitis C virus exposure in utero. J Infect Dis. (2011) 203:196–206. doi: 10.1093/infdis/jiq044
94. Metenou S, Suguitan AL, Long C, Leke RGF, Taylor DW. Fetal immune responses to Plasmodium falciparum antigens in a malaria-endemic region of Cameroon. J Immunol. (2007) 178:2770–7. doi: 10.4049/jimmunol.178.5.2770
95. Achary KG, Bal MS, Mandal NN, Satapathy AK. Increased IgG antibody responses to excretory/secretory antigens in neonates born from mothers infected with filarial nematodes. J Helminthol. (2017) 91:752–6. doi: 10.1017/S0022149X16000778
96. Malhotra I, Ouma J, Wamachi A, Kioko J, Mungai P, Omollo A, et al. In utero exposure to helminth and mycobacterial antigens generates cytokine responses similar to that observed in adults. J Clin Invest. (1997) 99:1759–66. doi: 10.1172/JCI119340
97. Soboslay PT, Geiger SM, Drabner B, Banla M, Batchassi E, Kowu LA, et al. Prenatal immune priming in onchocerciasis-onchocerca volvulus-specific cellular responsiveness and cytokine production in newborns from infected mothers. Clin Exp Immunol. (1999) 117:130–7. doi: 10.1046/j.1365-2249.1999.00906.x
98. Guadalupe I, Mitre E, Benitez S, Chico ME, Nutman TB, Cooper PJ. Evidence for in utero sensitization to Ascaris lumbricoides in newborns of mothers with ascariasis. J Infect Dis. (2009) 199:1846–50. doi: 10.1086/599214
99. Gill TJ, Repetti CF, Metlay LA, Rabin BS, Taylor FH, Thompson DS, et al. Transplacental immunization of the human fetus to tetanus by immunization of the mother. J Clin Invest. (1983) 72:987–96. doi: 10.1172/JCI111071
100. Rastogi D, Wang C, Mao X, Lendor C, Rothman PB, Miller RL. Antigen-specific immune responses to influenza vaccine in utero. J Clin Invest. (2007) 117:1637–46. doi: 10.1172/JCI29466
101. Krow-Lucal ER, Kim CC, Burt TD, McCune JM. Distinct functional programming of human fetal and adult monocytes. Blood. (2014) 123:1897–904. doi: 10.1182/blood-2013-11-536094
102. Jones AC, Miles EA, Warner JO, Colwell BM, Bryant TN, Warner JA. Fetal peripheral blood mononuclear cell proliferative responses to mitogenic and allergenic stimuli during gestation. Pediatr Allergy Immunol. (1996) 7:109–16. doi: 10.1111/j.1399-3038.1996.tb00117.x
103. Dauby N, Goetghebuer T, Kollmann TR, Levy J, Marchant A. Uninfected but not unaffected: chronic maternal infections during pregnancy, fetal immunity, and susceptibility to postnatal infections. Lancet Infect Dis. (2012) 12:330–40. doi: 10.1016/S1473-3099(11)70341-3
104. May K, Grube M, Malhotra I, Long CA, Singh S, Mandaliya K, et al. Antibody-dependent transplacental transfer of malaria blood-stage antigen using a human ex vivo placental perfusion model. PLoS ONE. (2009) 4:e7986. doi: 10.1371/journal.pone.0007986
105. Blok BA, de Bree LCJ, Diavatopoulos DA, Langereis JD, Joosten LAB, Aaby P, et al. Interacting, Nonspecific, immunological effects of bacille calmette-Guérin and Tetanus-diphtheria-pertussis inactivated polio vaccinations: an explorative, randomized trial. Clin Infect Dis. (2020) 70:455–63. doi: 10.1093/cid/ciz246
106. Fathman CG, Lineberry NB. Molecular mechanisms of CD4+ T-cell anergy. Nat Rev Immunol. (2007) 7:599–609. doi: 10.1038/nri2131
107. Brustoski K, Moller U, Kramer M, Hartgers FC, Kremsner PG, Krzych U, et al. Reduced cord blood immune effector-cell responsiveness mediated by CD4+ cells induced in utero as a consequence of placental Plasmodium falciparum infection. J Infect Dis. (2006) 193:146–54. doi: 10.1086/498578
108. Mertz D, Geraci J, Winkup J, Gessner BD, Ortiz JR, Loeb M. Pregnancy as a risk factor for severe outcomes from influenza virus infection: a systematic review and meta-analysis of observational studies. Vaccine. (2017) 35:521–8. doi: 10.1016/j.vaccine.2016.12.012
109. Müller-Schulte E, Gärtner BC. Vaccinations during pregnancy: a call to sting into action. Future Microbiol. (2019) 14:995–1006. doi: 10.2217/fmb-2019-0101
110. Callaghan WM, Creanga AA, Jamieson DJ. Pregnancy-related mortality resulting from influenza in the United States during the 2009-2010 pandemic. Obstet Gynecol. (2015) 126:486–90. doi: 10.1097/AOG.0000000000000996
111. Fortner KB, Nieuwoudt C, Reeder CF, Swamy GK. Infections in pregnancy and the role of vaccines. Obstet Gynecol Clin North Am. (2018) 45:369–88. doi: 10.1016/j.ogc.2018.01.006
112. Newsome K, Alverson CJ, Williams J, McIntyre AF, Fine AD, Wasserman C, et al. Outcomes of infants born to women with influenza A(H1N1)pdm09. Birth Defects Res. (2019) 111:88–95. doi: 10.1002/bdr2.1445
113. Mak TK, Mangtani P, Leese J, Watson JM, Pfeifer D. Influenza vaccination in pregnancy: current evidence and selected national policies. Lancet Infect Dis. (2008) 8:44–52. doi: 10.1016/S1473-3099(07)70311-0
114. Vaccines against influenza WHO position paper – November 2012. Wkly Epidemiol Rec. (2012) 87:461–76.
115. Grohskopf LA, Alyanak E, Broder KR, Walter EB, Fry AM, Jernigan DB. Prevention and control of seasonal influenza with vaccines: recommendations of the advisory committee on immunization practices-United States, 2019–20 influenza season. MMWR Recomm Rep. (2019) 68:1–21. doi: 10.15585/mmwr.rr6803a1
116. ACOG Committee Opinion No. 732: influenza vaccination during pregnancy. Obstet Gynecol. (2018) 131:e109–14. doi: 10.1097/AOG.0000000000002588
117. ACOG Committee Opinion No. 741: maternal immunization. Obstet Gynecol. (2018) 131:e214–7. doi: 10.1097/AOG.0000000000002662
118. Håberg SE, Trogstad L, Gunnes N, Wilcox AJ, Gjessing HK, Samuelsen SO, et al. Risk of fetal death after pandemic influenza virus infection or vaccination. N Engl J Med. (2013) 368:333–40. doi: 10.1056/NEJMoa1207210
119. Madhi SA, Cutland CL, Kuwanda L, Weinberg A, Hugo A, Jones S, et al. Influenza vaccination of pregnant women and protection of their infants. N Engl J Med. (2014) 371:918–31. doi: 10.1056/NEJMoa1401480
120. Committee on infectious diseases. Recommendations for prevention and control of influenza in children, 2019-2020. Pediatrics. (2019) 144:e20192478. doi: 10.1542/peds.2019-2478
121. Källén B, Olausson PO. Vaccination against H1N1 influenza with Pandemrix(®) during pregnancy and delivery outcome: a Swedish register study. BJOG. (2012) 119:1583–90. doi: 10.1111/j.1471-0528.2012.03470.x
122. Misra RS, Nayak JL. The importance of vaccinating children and pregnant women against influenza virus infection. Pathogens. (2019) 8:265. doi: 10.3390/pathogens8040265
123. Thompson MG, Kwong JC, Regan AK, Katz MA, Drews SJ, Azziz-Baumgartner E, et al. Influenza vaccine effectiveness in preventing influenza-associated hospitalizations during pregnancy: a multi-country retrospective test negative design study, 2010–2016. Clin Infect Dis. (2019) 68:1444–53. doi: 10.1093/cid/ciy737
124. Benowitz I, Esposito DB, Gracey KD, Shapiro ED, Vázquez M. Influenza vaccine given to pregnant women reduces hospitalization due to influenza in their infants. Clin Infect Dis. (2010) 51:1355–61. doi: 10.1086/657309
125. Thwaites CL, Beeching NJ, Newton CR. Maternal and neonatal tetanus. Lancet. (2015) 385:362–70. doi: 10.1016/S0140-6736(14)60236-1
126. WHO. Maternal and Neonatal Tetanus Elimination (MNTE). WHO. Available online at: http://www.who.int/immunization/diseases/MNTE_initiative/en/ (accessed at: November 10, 2020).
128. Topic Page | RHL. Available online at: https://extranet.who.int/rhl/topics/preconception-pregnancy-childbirth-and-postpartum-care/antenatal-care (accessed at: November 23, 2020).
129. Domenech de Cellès M, Magpantay FMG, King AA, Rohani P. The pertussis enigma: reconciling epidemiology, immunology and evolution. Proc Biol Sci. (2016) 283:20152309. doi: 10.1098/rspb.2015.2309
130. Burns DL, Meade BD, Messionnier NE. Pertussis resurgence: perspectives from the Working Group Meeting on pertussis on the causes, possible paths forward, and gaps in our knowledge. J Infect Dis. (2014) 209(Suppl 1):S32–35. doi: 10.1093/infdis/jit491
131. Winter K, Harriman K, Zipprich J, Schechter R, Talarico J, Watt J, e4t al. California pertussis epidemic, 2010. J Pediatr. (2012) 161:1091–6. doi: 10.1016/j.jpeds.2012.05.041
132. Havers FP, Moro PL, Hunter P, Hariri S, Bernstein H. Use of tetanus toxoid, reduced diphtheria toxoid, and acellular pertussis vaccines: updated recommendations of the advisory committee on immunization practices-United States, 2019. MMWR Morb Mortal Wkly Rep. (2020) 69:77–83. doi: 10.15585/mmwr.mm6903a5
133. Kharbanda EO, Vazquez-Benitez G, Lipkind HS, Klein NP, Cheetham TC, Naleway A, et al. Evaluation of the association of maternal pertussis vaccination with obstetric events and birth outcomes. JAMA. (2014) 312:1897–904. doi: 10.1001/jama.2014.14825
134. Mangtani P, Evans SJW, Lange B, Oberle D, Smith J, Drechsel-Baeuerle U, et al. Safety profile of rubella vaccine administered to pregnant women: a systematic review of pregnancy related adverse events following immunisation, including congenital rubella syndrome and congenital rubella infection in the foetus or infant. Vaccine. (2020) 38:963–78. doi: 10.1016/j.vaccine.2019.11.070
135. Laris-González A, Bernal-Serrano D, Jarde A, Kampmann B. Safety of administering live vaccines during pregnancy: a systematic review and meta-analysis of pregnancy outcomes. Vaccines. (2020) 8:124. doi: 10.3390/vaccines8010124
136. Keller-Stanislawski B, Englund JA, Kang G, Mangtani P, Neuzil K, Nohynek H, et al. Safety of immunization during pregnancy: a review of the evidence of selected inactivated and live attenuated vaccines. Vaccine. (2014) 32:7057–64. doi: 10.1016/j.vaccine.2014.09.052
137. Badilla X, Morice A, Avila-Aguero ML, Saenz E, Cerda I, Reef S, et al. Fetal risk associated with rubella vaccination during pregnancy. Pediatr Infect Dis J. (2007) 26:830–5. doi: 10.1097/INF.0b013e318124a9f4
138. Sato HK, Sanajotta AT, Moraes JC, Andrade JQ, Duarte G, Cervi MC, et al. Rubella vaccination of unknowingly pregnant women: the São Paulo experience, 2001. J Infect Dis. (2011) 204(Suppl 2):S737–44. doi: 10.1093/infdis/jir419
139. Castillo-Solórzano C, Reef SE, Morice A, Vascones N, Chevez AE, Castalia-Soares R, et al. Rubella vaccination of unknowingly pregnant women during mass campaigns for rubella and congenital rubella syndrome elimination, the Americas 2001-2008. J Infect Dis. (2011) 204(Suppl 2):S713–7. doi: 10.1093/infdis/jir489
140. Bar-Oz B, Levichek Z, Moretti ME, Mah C, Andreou S, Koren G. Pregnancy outcome following rubella vaccination: a prospective controlled study. Am J Med Genet A. (2004) 130A:52–4. doi: 10.1002/ajmg.a.30225
141. Pardon F, Vilariño M, Barbero P, Garcia G, Outon E, Gil C, et al. Rubella vaccination of unknowingly pregnant women during 2006 mass campaign in Argentina. J Infect Dis. (2011) 204(Suppl 2):S745–7. doi: 10.1093/infdis/jir442
142. Hofmann J, Kortung M, Pustowoit B, Faber R, Piskazeck U, Liebert UG. Persistent fetal rubella vaccine virus infection following inadvertent vaccination during early pregnancy. J Med Virol. (2000) 61:155–8. doi: 10.1002/(SICI)1096-9071(200005)61:1<155::AID-JMV25>3.0.CO;2-O
143. Wilson E, Goss MA, Marin M, Shields KE, Seward JF, Rasmussen SA, et al. Varicella vaccine exposure during pregnancy: data from 10 years of the pregnancy registry. J Infect Dis. (2008) 197(Suppl 2):S178–84. doi: 10.1086/522136
145. Kim DK. Advisory committee on immunization practices recommended immunization schedule for adults aged 19 years or older — United States, 2016. MMWR Morb Mortal Wkly Rep. (2016) 65:88–90. doi: 10.15585/mmwr.mm6504a5
146. Pregnancy Guidelines and Recommendations by Vaccine|CDC (2020). Available online at: https://www.cdc.gov/vaccines/pregnancy/hcp-toolkit/guidelines.html
147. WHO. Japanese Encephalitis Vaccines: WHO position paper, February 2015–recommendations. Vaccine. (2016) 34:302–3. doi: 10.1016/j.vaccine.2015.07.057
148. Who Publication null. Vaccines against tick-borne encephalitis: WHO position paper–recommendations. Vaccine. (2011) 29:8769–70. doi: 10.1016/j.vaccine.2011.07.024
149. Manning SE, Rupprecht CE, Fishbein D, Hanlon CA, Lumlertdacha B, Guerra M, et al. Human rabies prevention–United States, 2008: recommendations of the advisory committee on immunization practices. MMWR Recomm Rep. (2008) 57:1–28.
150. Wright JG, Quinn CP, Shadomy S, Messonnier N, Centers for Disease Control and Prevention (CDC). Use of anthrax vaccine in the United States: recommendations of the Advisory Committee on Immunization Practices. (ACIP), 2009. MMWR Recomm Rep. (2010) 59:1–30.
151. Contraindications Precautions for Polio Vaccination | CDC. (2019). Available online at: https://www.cdc.gov/vaccines/vpd/polio/hcp/contraindications-precautions.html
153. WHO. Vaccines and vaccination against yellow fever: WHO Position Paper, June 2013–recommendations. Vaccine. (2015) 33:76–7. doi: 10.1016/j.vaccine.2014.05.040
154. Hu B, Guo H, Zhou P, Shi ZL. Characteristics of SARS-CoV-2 and COVID-19. Nat Rev Microbiol. (2021) 19:141–54. doi: 10.1038/s41579-020-00459-7
155. Verma S, Bradshaw C, Auyeung NSF, Lumba R, Farkas JS, Sweeney NB, et al. Outcomes of maternal-newborn dyads after maternal SARS-CoV-2. Pediatrics. (2020) 146:e2020005637. doi: 10.1542/peds.2020-005637
156. CDC. Vaccination Considerations for People Pregnant or Breastfeeding. Centers for Disease Control and Prevention. (2021). Available online at: https://www.cdc.gov/coronavirus/2019-ncov/vaccines/recommendations/pregnancy.html
157. Press Briefing by White House COVID-19 Response Team and Public Health Officials. The White House. (2021). Available online at: https://www.whitehouse.gov/briefing-room/press-briefings/2021/02/10/press-briefing-by-white-house-covid-19-response-team-and-public-health-officials-3/
158. The safety of COVID-19 vaccines when given in pregnancy. GOVUK. Available online at: https://www.gov.uk/government/publications/safety-of-covid-19-vaccines-when-given-in-pregnancy/the-safety-of-covid-19-vaccines-when-given-in-pregnancy
159. Newborn Antibodies to SARS-CoV-2 Detected in Cord Blood After Maternal Vaccination. Available online at: https://www.medrxiv.org/content/10.1101/2021.02.03.21250579v1
160. Borchers AT, Chang C, Gershwin ME, Gershwin LJ. Respiratory syncytial virus—a comprehensive review. Clin Rev Allergy Immunol. (2013) 45:331–79. doi: 10.1007/s12016-013-8368-9
161. Nenna R, Ferrara M, Nicolai A, Pierangeli A, Scagnolari C, Papoff P, et al. Viral load in infants hospitalized for respiratory syncytial virus bronchiolitis correlates with recurrent wheezing at thirty-six-month follow-up. Pediatr Infect Dis J. (2015) 34:1131–2. doi: 10.1097/INF.0000000000000825
162. Swamy GK, Beigi RH. Maternal benefits of immunization during pregnancy. Vaccine. (2015) 33:6436–40. doi: 10.1016/j.vaccine.2015.08.035
163. Saso A, Kampmann B. Vaccination against respiratory syncytial virus in pregnancy: a suitable tool to combat global infant morbidity and mortality? Lancet Infect Dis. (2016) 16:e153–63. doi: 10.1016/S1473-3099(16)00119-5
164. Blanco JCG, Boukhvalova MS, Morrison TG, Vogel SN. A multifaceted approach to RSV vaccination. Hum Vaccin Immunother. (2018) 14:1734–45. doi: 10.1080/21645515.2018.1472183
165. Abu-Raya B, Maertens K, Edwards KM, Omer SB, Englund JA, Flanagan KL, et al. Global perspectives on immunization during pregnancy and priorities for future research and development: an international consensus statement. Front Immunol. (2020) 11:1282. doi: 10.3389/fimmu.2020.01282
166. Madhi SA, Polack FP, Piedra PA, Munoz FM, Trenholme AA, Simões EAF, et al. Respiratory syncytial virus vaccination during pregnancy and effects in infants. N England J Med. (2020) 383:426–39. doi: 10.1056/NEJMoa1908380
167. Munoz FM. Current challenges and achievements in maternal immunization research. Front Immunol. (2018) 9:436. doi: 10.3389/fimmu.2018.00436
168. Giles ML, Krishnaswamy S, Wallace EM. Maternal immunisation: what have been the gains? Where are the gaps? What does the future hold? F1000Res. (2018) 7:F1000 Faculty Rev-1733. doi: 10.12688/f1000research.15475.1
169. Puopolo KM, Lynfield R, Cummings JJ, Committee on Fetus And Newborn Committee on Infectious Diseases. Management of infants at risk for group B streptococcal disease. Pediatrics. (2019) 144:e20191881. doi: 10.1542/peds.2019-1881
170. Kobayashi M, Vekemans J, Baker CJ, Ratner AJ, Le Doare K, Schrag SJ. Group B Streptococcus vaccine development: present status and future considerations, with emphasis on perspectives for low and middle income countries. F1000Res. (2016) 5:2355. doi: 10.12688/f1000research.9363.1
171. WHO. Group B Streptococcus Vaccine Development Technology Roadmap. Priority activities for development, testing, licensure and global availability of Group B streptococcus vaccines.
172. Goodridge HS, Ahmed SS, Curtis N, Kollmann TR, Levy O, Netea MG, et al. Harnessing the beneficial heterologous effects of vaccination. Nat Rev Immunol. (2016) 16:392–400. doi: 10.1038/nri.2016.43
173. Mina MJ, Metcalf CJE, de Swart RL, Osterhaus ADME, Grenfell BT. Long-term measles-induced immunomodulation increases overall childhood infectious disease mortality. Science. (2015) 348:694–9. doi: 10.1126/science.aaa3662
174. Lund N, Andersen A, Hansen ASK, Jepsen FS, Barbosa A, Biering-Sørensen S, et al. The effect of oral polio vaccine at birth on infant mortality: a randomized trial. Clin Infect Dis. (2015) 61:1504–11. doi: 10.1093/cid/civ617
175. Biering-Sørensen S, Aaby P, Napirna BM, Roth A, Ravn H, Rodrigues A, et al. Small randomized trial among low-birth-weight children receiving bacillus Calmette-Guérin vaccination at first health center contact. Pediatr Infect Dis J. (2012) 31:306–8. doi: 10.1097/INF.0b013e3182458289
176. Rieckmann A, Villumsen M, Sørup S, Haugaard LK, Ravn H, Roth A, et al. Vaccinations against smallpox and tuberculosis are associated with better long-term survival: a Danish case-cohort study 1971-2010. Int J Epidemiol. (2017) 46:695–705. doi: 10.1093/ije/dyw120
177. Kleinnijenhuis J, Quintin J, Preijers F, Joosten LAB, Ifrim DC, Saeed S, et al. Bacille Calmette-Guerin induces NOD2-dependent nonspecific protection from reinfection via epigenetic reprogramming of monocytes. Proc Natl Acad Sci USA. (2012) 109:17537–542. doi: 10.1073/pnas.1202870109
178. Kochhar S, Edwards KM, Ropero Alvarez AM, Moro PL, Ortiz JR. Introduction of new vaccines for immunization in pregnancy - programmatic, regulatory, safety and ethical considerations. Vaccine. (2019) 37:3267–77. doi: 10.1016/j.vaccine.2019.04.075
179. Wilson RJ, Paterson P, Jarrett C, Larson HJ. Understanding factors influencing vaccination acceptance during pregnancy globally: a literature review. Vaccine. (2015) 33:6420–9. doi: 10.1016/j.vaccine.2015.08.046
Keywords: maternal immunization, vaccination, pregnancy, immune system, neonate
Citation: Cinicola B, Conti MG, Terrin G, Sgrulletti M, Elfeky R, Carsetti R, Fernandez Salinas A, Piano Mortari E, Brindisi G, De Curtis M, Zicari AM, Moschese V and Duse M (2021) The Protective Role of Maternal Immunization in Early Life. Front. Pediatr. 9:638871. doi: 10.3389/fped.2021.638871
Received: 07 December 2020; Accepted: 26 March 2021;
Published: 28 April 2021.
Edited by:
Vassiliki Papaevangelou, National and Kapodistrian University of Athens, GreeceReviewed by:
Rashmi Ranjan Das, All India Institute of Medical Sciences, IndiaLovro Lamot, University of Zagreb, Croatia
Copyright © 2021 Cinicola, Conti, Terrin, Sgrulletti, Elfeky, Carsetti, Fernandez Salinas, Piano Mortari, Brindisi, De Curtis, Zicari, Moschese and Duse. This is an open-access article distributed under the terms of the Creative Commons Attribution License (CC BY). The use, distribution or reproduction in other forums is permitted, provided the original author(s) and the copyright owner(s) are credited and that the original publication in this journal is cited, in accordance with accepted academic practice. No use, distribution or reproduction is permitted which does not comply with these terms.
*Correspondence: Bianca Cinicola, YmlhbmNhY2luaWNvbGFAZ21haWwuY29t
†These authors share first authorship
‡These authors share senior authorship