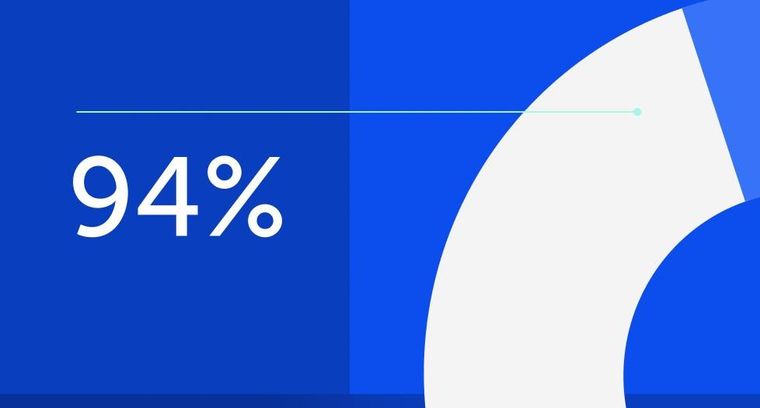
94% of researchers rate our articles as excellent or good
Learn more about the work of our research integrity team to safeguard the quality of each article we publish.
Find out more
REVIEW article
Front. Pediatr., 15 January 2021
Sec. Pediatric Gastroenterology, Hepatology and Nutrition
Volume 8 - 2020 | https://doi.org/10.3389/fped.2020.620189
This article is part of the Research TopicPediatric Inflammatory Bowel Disease: the Next FrontierView all 26 articles
Diet provides a safe and attractive alternative to available treatment options in a variety of diseases; however, research has only just begun to elucidate the role of diet in chronic diseases, such as the inflammatory bowel diseases (IBD). The chronic and highly debilitating IBDs, Crohn disease and ulcerative colitis, are hallmarked by intestinal inflammation, immune dysregulation, and dysbiosis; and evidence supports a role for genetics, microbiota, and the environment, including diet, in disease pathogenesis. This is true especially in children with IBD, where diet-based treatments have shown excellent results. One interesting group of dietary factors that readily links microbiota to gut health is dietary fibers. Fibers are not digested by human cells, but rather fermented by the gut microbes within the bowel. Evidence has been mounting over the last decade in support of the importance of dietary fibers in the maintenance of gut health and in IBD; however, more recent studies highlight the complexity of this interaction and importance of understanding the role of each individual dietary fiber subtype, especially during disease. There are roughly ten subtypes of dietary fibers described to date, categorized as soluble or insoluble, with varying chemical structures, and large differences in their fermentation profiles. Many studies to date have described the benefits of the byproducts of fermentation in healthy individuals and the potential health benefits in select disease models. However, there remains a void in our understanding of how each of these individual fibers affect human health in dysbiotic settings where appropriate fermentation may not be achieved. This review highlights the possibilities for better defining the role of individual dietary fibers for use in regulating inflammation in IBD.
The etiology of the chronic and severely debilitating Inflammatory Bowel Diseases (IBD), Crohn disease (CD), and ulcerative colitis (UC), remains poorly understood and incidence rates are increasing, especially in children (1–3). Risk factors associated with IBD include urban lifestyle, lack of greenspace (4), genetic factors, heightened hygiene, dietary factors, and changes in the microbiome (5–11). Gut microbes are critical to human health as they mediate key functions of metabolism and immunity (6, 12). It remains unclear whether the dysbiotic microbial communities associated with IBD are a cause or a consequence of the disease. However, both compositional changes and reduced microbial biodiversity are hallmarks of IBD and can even predict therapy failure in pediatric IBD (13). Alterations in gut microbial composition are often associated with more “proinflammatory” microbes, such as Proteobacteria phylum and Ruminococcus gnavus species, and decreased levels of butyrate-producing bacteria (e.g., Faecalibacterium prausnitzii), which correlated strongly in the presence of disease (10–12). Interestingly, dietary therapy is highly effective for pediatric CD, likely mediated by microbes, but is challenging to complete (14, 15). Increased incidence of IBD has been associated with a Western diet (16), and several studies have demonstrated the influence of diet on the gut microbiota (17–20). A number of fantastic reviews have been recently published (21, 22), illustrating the scientific evidence in support of the role that diet plays in the pathogenesis of IBD. Fibers have attracted specific attention and are generally considered beneficial to gut health. However, the reality is much more complex as some patients describe increased symptoms with fibers (23). Therefore, in the following review we will delve deeper, discussing the specific role of dietary fibers in health, and current evidence of fiber effects in IBD cell lines, animal models, and clinical trials, suggesting reasons why some IBD patients describe a sensitivity and worsening symptoms following fiber consumption.
The term dietary fiber, first coined by Howeler, describes a complex group of non-digestible components of cell walls (Figure 1) (24). The term has grown to include non-starch polysaccharides (e.g., cellulose, pectin), non-carbohydrate-based polymers (e.g., lignan), resistant oligosaccharides (e.g., fructooligosaccharides, galatooligosaccharides), and carbohydrates considered to be of animal origin (e.g., chitin) (25). These dietary fibers can be found in a variety of food sources (Figure 2) and structurally differ in their chain length, linkage type, sugar components, and ability to associate with other chemical compounds (Figure 1) (25). Unlike most dietary components, non-digestible dietary carbohydrates (fiber and resistant starch) can withstand the acidity of the stomach and do not undergo degradation in the human small intestine (26); instead they are fermented by the gut microbiota consortium within the large bowel (Figure 2) where one microbe starts the fermentation and others continue the fermentation process, thereby working together systematically. This microbial consortium role thus highlights the potential implications of dysbiosis, which could alter or even prevent fiber fermentation. The colon houses one of the most complex (over 1,000 species) and highest populated microbiomes in the human body (70% of all microbes in the body) (27–29). Diet has been shown to be a key factor influencing the composition and functions of intestinal microbes, and continues to be examined as a tool in shaping the gut microbiota (30, 31). Studies suggest that while the recommended daily fiber intake from the Institute of Medicine (IOM) ranges from 19–38 g per day, dependent on age and gender (32), typical western diets lack fibers, and typically include roughly 13–20 g of dietary fiber per day, from which the commensal microbes are reliant upon for a source of energy and carbon (33–35). Most of the dietary fibers we consume originate from plant cell walls making up fruits, vegetables, and whole grains (25, 36). Dietary fibers consist of a variety of linked monosaccharides creating a variety of diverse molecules with varying side chains and physical variations, including physical arrangement and solubility (25). While these dietary fibers can be classified in a number of ways (37–40), the most common method of classification for nutritional purposes in humans segregates dietary fibers as water-soluble or insoluble (40). Water solubility within the gastrointestinal tract is related to the degree of fermentation by gut microbes (41). Soluble dietary fibers can increase digesta viscosity, which in turn delays gastric emptying and nutrient release, thus reducing glycaemic response (40, 42, 43). Examples of soluble dietary fibers include pectin, arabinoxylan, β-glucans, inulin, fructo-oligosaccharides, galacto-oligosaccharides, and xyloglucans (36). Insoluble dietary fibers, such as cellulose and lignin, on the other hand, are considered to be less valuable to the gut microbes as their strong hydrogen-binding networks reduce their accessible surface area to allow for fermentation (44). The complex structures of these dietary fibers require specific enzymes, such as carbohydrate-active enzymes (CAZymes) found only in microorganisms for degradation and utilization (25, 45). There are over 120 CAZenzymes identified to date, which have been shown to cleave glycosidic linkages (25). A number of key enzymes are highlighted in Figure 1, including those that target the breakdown of arabinoxylan (46), β-fructans (47, 48), cellulose (48), pectin (48), and lignin (49).
Figure 1. Not all Fibers are born equal; Structure, classification, and chemical features of key dietary fibers with key enzymes highlighted in red.
A variety of different microbes, that are able to utilize the same dietary fibers, coexist within the human bowel, resulting in a competitive environment that drives a variety of outcomes, based on the utility of these fibers (50). These selective differences in the microbiome of individuals results in distinct bacterial responses to consumption of dietary fibers (51, 52). Studies have demonstrated that this is likely because fermentation of dietary fibers is dependent on microbial community composition, wherein key microbes must be present for fermentation by their partner organisms to take place (52–55).
The human gut microbiome consists of at least 7 key phyla, predominated by Firmicutes (60–90%), Bacteroidetes (8–28%), Proteobacteria (0.1–8%), and Actinobacteria (2.5–5%) (56, 57). Studies suggest that a number of Bacteroidetes are involved in the breakdown of larger polysaccharides into smaller sugars, which can be utilized by Firmicutes that act as cross-feeders for other microbes (44). These microbes utilize a variety of complex pathways in the breakdown of dietary fibers to byproducts essential for human health. Fiber fermentation produces byproducts, such as short chain fatty acids (SCFAs) (58–63). Enzymes such as polysaccharidases, glycosidases, proteases, and peptidases are utilized to break complex carbohydrates or fibers into their sugar and amino acid components before fermenting these smaller components into SCFA, carboxylic acids, CO2, and H2 (64, 65).
The primary SCFAs produced by fiber fermentation include acetate, butyrate, and propionate, which have been generally shown to benefit the host through many mechanisms, including providing energy for colonic mucosa cells, reducing inflammation in IBD, and promoting differentiation and apoptosis of colonic cancer cells (66–68). A recent review by Williams et al. provides an excellent overview of the health benefits of these byproducts in humans (36). The precise mechanisms underlying the coordinated multi-microbe breakdown of dietary fibers into their byproducts and subsequent uptake within the gut continues to be illuminated in various healthy host settings. Changes in fermentation processes carry the potential to regulate changes in the gut microenvironment (60), immune modulation (69), and altered energy metabolism (70).
Interestingly, fiber-rich diets are associated with healthy growth of specific commensal microorganisms (71–76), many of which are directly responsible for SCFA production, improved gut mucosal barrier, and preventing inflammation. The amount and rate of SCFA production is dependent on the species and amounts of microbiota present within the gut (77), further illustrating how an altered gut microbiome in the presence of IBD impacts the production and absorption of protective SCFAs.
Although there is consistent evidence to support the general concept that dietary fibers and their fermentation products are likely beneficial in the setting of IBD (78), it is important to recognize that much of this is an extrapolation of what we know from healthy state and that there are fundamental factors that would challenge this paradigm and potentially impact effects of fibers on IBD. First, fiber intake prior to developing IBD has been linked to risk of disease but having IBD has also been shown to result in changes within the patient's diet (79, 80). The same is true for microbes, which are obviously highly relevant to the impact of fiber on human health; they are thought to play a role in disease pathogenesis, but are clearly altered by having IBD (81). Surveys indicate that many patients (mostly with UC) tend to avoid consumption of dietary fibers (23). Some epidemiological studies, such as the EPIC-IBD cohort study, suggest there are no clear associations found to correlate development with IBD in relation to consumption of either total fiber or fiber from select sources (82). Other clinical studies suggest that long term intake of dietary fiber from fruits, and to a lesser degree, vegetables, reduces the risk of developing CD by up to 40%, with no effect on UC (83). These studies suggest that the protective effect of fiber for CD patients is mainly sourced from fruits and not associated at all with fiber intake sourced from whole grain or legumes (84–86). A restriction of dietary fiber consumption in “humanized” mice increases the consumption of colonic mucosa by colonic microbes, which has been suggested to contribute to reduced mucosa and inflammation in IBD (87). Many of the specific microbes affected by fiber-rich diets have been found to be less abundant in IBD (88–95), supporting the idea that fermentation of fibers may be altered in IBD patients. Additionally, animal studies have demonstrated that dietary fibers can inhibit IBD-associated inflammation (96–98), and clinical trials have shown that SCFA can prevent intestinal atrophy in IBD patients, allowing for tissue recovery (99).
Researchers continue to strive to understand the role that individual dietary fibers play in human health and in IBD patients to better appreciate how manipulating diet in these patients may help to improve clinical outcomes. Patients with ulcerative colitis showed a decrease in serum C-reactive protein (CRP) levels when given a fiber-based prebiotic, as well as a reduction in abdominal pain and cramping (100). A similar study by Fritsch et al. demonstrated a reduction of inflammatory markers and dysbiotic microbiome in patients with ulcerative colitis on a low-fat, high-fiber diet (101). Similarly, mice given a probiotic and fiber-based prebiotic displayed amelioration in disease activity and histological score compared to a prebiotic alone, and a reduction in serum CRP levels (102). Recent studies have shown that Institute of Cancer Research (ICR) mice fed a fiber-free diet displayed shortening of colon length, which was found to be an indicator for IBD, and reduced microbial diversity (103). This indicates that dietary fibers play an important role in mitigating disease severity in IBD patients.
Current studies further support the SCFA butyrate as an anti-inflammatory product of fermentation within the colon that may improve epithelial barrier integrity (104–107). However, it is important to also highlight that there remains contradictory evidence from studies that have shown that butyrate had no benefit in these patients (108, 109). Unfortunately, many clinicians continue to promote reduced fiber consumption, or fiber avoidance altogether, in IBD patients (particularly UC) while there is no evidence to support this as a relevant therapeutic intervention (110, 111). While it remains unclear exactly which factors modulate the health benefits associated with dietary fibers in IBD, results from studies performed to date suggest that modulating diet through prebiotic/probiotic therapies to promote select fiber fermentative microbes may aid in improving IBD patient symptoms.
Key features of the following dietary fibers are summarized in Table 1.
We will first present soluble fibers, followed by insoluble.
Arabinoxylan (AX) is a hemicellulose molecule composed of a β-(1,4)-linked xylose backbone containing arabinose side chains (112, 113). The detailed structure of AX is dependent on several factors, such as its source, enzymes used for hydrolysis, and methods of extraction (114). AX is a large component of dietary fiber found in cereal grains and other plant and animal food sources (115–117). The fermentation of AX by gut microbes such as Streptococcus and Bifidobacteria results in the production of SCFAs, including acetate, propionate, and butyrate (115, 118–121). Furthermore, downstream fungal microbes, such as Saccharomyces cerevisiae result in fermentation processes producing biomass and ethanol (122).
Due to the previously stated structural differences that can exist within AX molecules, it is important to consider the polymer side branching and source of AX fiber when discussing immunomodulatory properties of this fiber (123–125). Studies have shown that oral administration of corn-husk AX fiber has anti-inflammatory effects in mice, through the activation of an INF-γ dependent T helper 1-like immune response (126). AX can also stimulate COX-2 through TLR-4 upregulation, as well as contributing to a reduction in pro-inflammatory cytokines IL-8 and TNF-α in colon cancer cell lines (127). Prebiotic administration of long-chain AX resulted in decreased abundance of mucin-degrading microorganisms and led to a three-fold increase of cecal mucin levels in rats (128). This is especially important in the context of IBD as the protective mucin layer within the gut that aids in providing a healthy barrier against environmental threats, is greatly diminished in IBD patients (129).
An AX enriched food, germinated barley (GB), was used in a clinical trial, in which 59 UC patients in remission were divided into two groups; 37 individuals in the control group received a conventional drug for 1 year and the other 22 patients in the GB group received a conventional drug plus 20 g of GB daily. GB significantly ameliorated the disease activity index and reduced the recurrence rate compared to control group, and no significant side effects were observed (130). Neyrinck et al. reported that the abundance of Bacteroides and Roseburia spp., as well as Bifidobacteria, were increased with wheat AX supplementation, and that the gut barrier function was strengthened while serum pro-inflammatory markers were reduced (131). In another 8-week clinical trial, 19 UC patients with wheat bran AXs and resistant starch treatments were shown to shape the microbial community composition, which increased diversity within the Clostridium cluster XIVa compared to the control group (132). Clostridium cluster XIVa and Bifidobacteria have gained much attention recently due to their contribution to gut homeostasis, by preserving gut barrier functions and exerting immunomodulatory and anti-inflammatory properties (133).
β-glucans are a family of naturally occurring glucose polysaccharides that make up the cell wall of select bacteria and fungi, but are also found in dietary plant cells, such as oat and barley (134, 135). Interestingly, studies focused on the immune response to fungal infection indicate that β-glucan on the surface of fungal cells interacts with host immune cell receptors (e.g., Dectin-1), inducing a pro-inflammatory response (47–49). Dietary β-glucans differ from those found in fungal cell walls by level of solubility and downstream effects (136, 137). Dietary β-glucans have been shown to be fermented by microbes such as Lactobacilli, Enterococcus, and Bifidobacteria, forming a variable amount of SCFAs, depending on the source of fiber along with the microbial species involved (138, 139). Furthermore, production of the β-glucan receptor, Dectin-1, has been shown to be increased in dextran sodium sulfate (DSS)-induced colitis model mice (140). Inhibition of Dectin-1 has been shown to ameliorate colitis in this model (141). The DSS mouse model was recently utilized to examine inflammatory responses to a series of different glucan preparations (142). Findings from this study suggest that histology disease scores were reduced in response to fungal extracted glucans, which were associated with Dectin-1. Specifically, the glucans most effectively associated with reduction of IBD-like symptoms in DSS mice were those collected from the edible mushroom Pleurotus eryngii (142). Meanwhile other studies have demonstrated that oral administration of various other isolates of β-glucan in fact worsened intestinal inflammation in DSS-induced colitis models (143). These results highlight the importance of understanding the chemical structures of different dietary fibers and their direct interactions within the bowel to potentially aid in developing dietary recommendations to promote improved gut health in IBD patients.
The β-(2 → 1) linked fructose oligo- and poly-saccharides known as β-fructans (inulin and oligofructose/FOS, respectively), are commonly found in plant sources including chicory root, agave, and artichokes, while other sources (banana, wheat, onion, garlic) contain lesser amounts (144). Microbes such as Bifidobacteria, Lactobacillus, Streptococcus, Flavobacterium, and a variety of baking yeast have been shown to be responsible for fermentation of β-fructans (144, 145). Butyrate-producing bacteria have primarily been demonstrated to be positively affected by these fibers, resulting in a wide variety of health benefits (146–149). As a prebiotic, these fibers have demonstrated benefits for the treatment of intestinal inflammation in mouse models of IBD, as described in a recent series of review articles (150–153). Overall, DSS-induced IBD-like symptoms were reduced in mice fed with β-fructans, or relapse was prevented, through the mediation of antioxidative defense mechanisms, identified in more recent studies in animal models and cell lines (154, 155). β-fructans have also been shown to interact with carbohydrate receptors (GLP-1R), affecting reactive oxygen species (ROS) production and associated inflammation (144, 156–158). Interestingly, while inulin has been demonstrated to have positive effects on inflammation in select situations, a number of studies have also suggested inulin can exacerbate the severity of colitis in an IL10−/− and DSS-model of colitis (159), and promote HCC-progression in mice (160).
Galactooligosaccharides (GOS) are oligosaccharides composed of different galactosyl residues (from 2 to 9 units) and terminal glucose molecules linked by β-glycosidic bonds (161). They naturally are found at low concentrations in the milk of many animals, including humans and cows, but they also can be produced by chemical glycosylation or biocatalysis of lactose (162). In a human randomized, double-blind, placebo-controlled study, the effect of 6-weeks daily intake of 5 g GOS on intestinal barrier function and gut microbiome composition was evaluated in 114 obese individuals. The GOS showed a bifidogenic effect on the resident gut microbiota, and improvement on colonic permeability (163). Bifidobacteria have gained emerging attention recently because of their contribution to gut homeostasis, by preserving gut barrier functions and exerting immunomodulatory and anti-inflammatory properties (133). Thus, Bifidobacteria are generally considered to be health-promoting organisms and constitute one of the main groups of organisms targeted by prebiotics. A human study reported the positive effects of GOS with daily dosage 5 or 10 g on Bifidobacterium strains from these genera have been elucidated, while a lower dose of 2.5 g showed no significant effect (164). GOS has also been demonstrated to enhance F. prausnitzii levels (165). F. prausnitzii is a commensal bacterium with suggested anti-inflammatory effects, supported by gut microbiota analysis of CD patients and functional studies (166). A decrease of F. prausnitzii abundance in the gut is typically recognized as a signature of gut dysbiosis in CD, compared to healthy people (167). Furthermore, 44 patients with irritable bowel syndrome (IBS) were randomized to receive 3.5 g/day GOS, 7 g/day GOS, or 7 g/day placebo over 12 weeks. Results showed that GOS acted as a prebiotic via stimulating gut Bifidobacteria in IBS patients, and effectively alleviated IBS symptoms (168). Given that several important symptoms, including pain and diarrhea, overlap in IBD and IBS, these interventions may also provide references to use prebiotics for IBD treatment or adjuvant treatment, but this needs to be proven in studies. Together, recent evidence suggests that the prebiotic effects of GOS on the specific gut microbiota, such as Bifidobacterial consortia and F. prausnitzii with the resulting oligosaccharide degradation, might offer a potential mechanism to improve gut barrier function, and suppress inflammation.
Pectin is a complex polysaccharide found in the cell wall of fruits and vegetables (169). It is made up mostly of α-1,4-linked D-galacturonic acid residues with variable levels of esterification between plant species that results in different effects on fermentation, SCFA production, and effects on the immune profile (170, 171). A variety of microorganisms (e.g., Bacteroides, Prevetella, Bacillus, Agrobacterium, Pseudomonas, Ralstonia, Dickeya, and yeast) utilize enzymatic processes (e.g., isomerase) or oxidative pathways, in the almost complete fermentation of pectin within the colon, resulting in the production of a wide variety of SCFAs along with a number of gases, including CO2, H2, H2S, and CH4 (169, 172–177). Pectin directly inhibits the pro-inflammatory cytokine toll-like receptor (TLR)1&2 pathways and prevents ileitis in mice (53). In vitro studies have shown that pectin reduces the pro-inflammatory cytokine IL-1β and interacts with and down regulates TLR-4 signaling (178). Dietary pectin also moderates the production of pro-inflammatory cytokines and immunoglobulins, working to downregulate inflammatory response in the colon of mice (179). A recent study examining the variable effects of pectin side chain content on colitis induced C57BL/6 mice demonstrated that a diet high in pectin (orange pectin) ameliorated disease compared to low pectin (citrus pectin) or no-pectin diet supplementation (180).
Cellulose is the main structural component of plant cell walls and is composed of linear chains of β(1 → 4) linked glucose monomers (181, 182). While insoluble fibers are considered to be much less important for the production of healthy byproducts of fermentation due to the limitations in breaking down these components, microbes (e.g., Bacteroides, Clostridium, Fibrobacter, Ruminococcus, and anaerobic fungi) have been demonstrated to utilize cellulose for the production of intermediate byproducts of fermentation, such as succinate, along with a variety of SCFA byproducts, predominantly acetate (149, 183–185). This means that degradation of cellulose provides the opportunity for a variety of responses within the bowel; however, in the context of IBD it is important to highlight that the byproduct succinate has been associated with inflammation, suggesting one reason that may help explain why some patients experience sensitivity to dietary fibers (186, 187). Mice fed a high-cellulose diet were shown to have increased expression of Mt1 and Mt2 genes (188, 189), which have been found to play a protective role in inflammation (188, 190) and a colitis model through anti-apoptotic and immune-modulating effects (188, 191). Experimental studies in mice have demonstrated that dietary supplementation of cellulose contributes to substantial shifts in the gut microbiome, which were associated with transient trophic and anticolitic effects (192). A recent study has indicated that a high-cellulose diet maintains gut homeostasis and ameliorates gut inflammation by altering gut microbiota and metabolites in mice, and can be protective against colitis (188). In this study, low-cellulose diets were found to cause crypt atrophy, goblet cell depletion, and upregulation of pro-inflammatory genes in the colon (188). Additionally, dietary cellulose is thought to be associated with increased abundance of Akkermansia, which promote gut barrier function, and enhanced mucin secretion by goblet cells (188). Interestingly, cellulose has been found to improve survival in the murine model of sepsis through systemic anti-inflammatory effects. High-fiber cellulose diets were associated with a decrease in serum concentration of pro-inflammatory cytokines, reduced infiltration of neutrophils in the lungs, and decreased hepatic inflammation (193).
Lignan is a complex polymer containing ~40 oxygenated phenylpropane units that have undergone a dehydrogenative polymerization process and is a non-carbohydrate component of cell walls (182). Lignan can be found in many plants including grains and vegetables, and the highest concentration is found in flaxseed (194). Much like cellulose, the breakdown of lignan is complex and difficult to achieve. Certain wood-degrading fungi (e.g., Bjerkandera, Fomitopsis, and Schizophyllum) have been shown to utilize peroxidase, ligninase, and laccase enzymes in the degradation of lignin, producing ethanol (Figure 1) (195–197). Interestingly Rhodococcus, Pseudomonas, and Sphingobacterium are able to use the aromatic components of lignan during its breakdown process in order to produce high levels of lipids for biofuel production (198–201). These data suggest that there may also be some gut microbiota capable of some level of lignin fermentation processes. Lignan was found to contribute to decreased white blood cell counts and proinflammatory/profibrogenic cytokine levels, as well as decreased gene expression of cytokines and cytokine receptors in mice exposed to asbestos (202). A study conducted on hemodialysis patients showed that supplementation of flaxseed in the diet contributed to improved lipid abnormalities and reduced systemic inflammation (203).
These studies highlight the importance of studying the variability in response to specific dietary fibers (or similar molecular structures), while most studies continue to evaluate fiber diets in a holistic manner, lumping all dietary fibers into one.
Based on the studies published to date, there remains clear evidence for the importance of high intake of dietary fibers overall with no current evidence to support restriction of dietary fibers in patients without intestinal strictures or obstructions (21). However, the benefits of the specific types and sources of dietary fibers remain poorly understood, and the research paradigm has begun to shift toward examining the direct effects of individual fiber types in IBD. As many patients describe a sensitivity to dietary fibers (23), it seems wise to recommend that the increased source of dietary fiber come from those fibers with clear evidence to support anti-inflammatory or preventative effects as speculation remains surrounding the negative impact of certain fibers on patient health during IBD flares (110). A recent review of current literature by Levine and colleagues suggests that there is an acceptable amount of data to support recommended moderate to high amounts of fruit and vegetables in CD patient diets, while there remains insufficient evidence to support these recommendations in UC patients (21). Notably, the evidence we have discussed in the previous sections suggests that gut microbes more readily ferment soluble dietary fibers, and the SCFA, butyrate, seems to be the most beneficial byproduct studied to date, providing a source of carbon and energy for the colon epithelium (204). It could be said that specifically those fibers utilized for the production of increased butyrate should be included in greater quantities in the diet of IBD patients, while due to a lack a consistent evidence to support a beneficial effect in IBD patients, insoluble fiber consumption should perhaps be avoided or reduced, especially for those patients with active disease (21). Specifically, arabinoxylans, β-glucans, β-fructans, and pectins, which are all found in vegetables, fruits, and grains, have been shown to be valuable sources of SCFAs by butyrate-producing microbes (21). So what is the easiest means of increasing consumption of these dietary fibers while limiting insoluble fiber consumption? Food products such as bananas and broccolis, nuts, tomatoes, and carrots, mushrooms, and peeled apples and citrus fruits contain high levels of soluble dietary fibers while limiting insoluble fiber intake (205). It is important to note that the evidence in support of this includes very limited numbers of human studies, and a number of contradictory animal and in vitro studies, however, these recommendations may help certain individuals. Interindividual variability in microbial composition and function will also need to be considered, as further discussed below (118).
Some of these considerations require additional attention in the pediatric setting. First, the first-line therapy for pediatric-onset CD, as recommended by the revised ECCO-ESPGHAN guidelines, exclusive enteral nutrition (EEN) (206), is based on liquid diet that does not include fiber in most cases. Would selective addition of soluble fiber to these controlled diets further improve their effect? The Crohn disease exclusion diet (CDED) has utilized some of the principles discussed in this review in the design of the food-based diet (19). CDED has shown at least equal success to EEN, but even better tolerance, which is also coupled by some of the expected changes in microbial composition (20, 207). However, as many of the origins of IBD pathogenesis are likely related to early life events, including early commensal engraftment of the infant gut, it is possible that what we feed our microbes (i.e., fibers) may have an impact on developmental immune responses related to many chronic conditions, including IBD. This might explain the beneficial effect of breastfeeding (through human milk oligosaccharides, such as GOS), as well as other links to diet (208). Once the science linking diet through microbes to chronic disease is further developed, we would expect that better guidance on early life fiber exposure could help prevent IBD at a later age.
With an increasing focus on nutritional interventions, especially in children with Crohn disease, and the interest (but still limited supportive research) on specific use of probiotics and prebiotics in IBD, it is important that we broaden our understanding of how foods affect the bowel, especially in regards to the fiber fermentation processes that occur in the bowel. Results from hundreds of studies to date have demonstrated the ability of select individual microbes or whole microbe cultures, in rats, mice, rabbits, swine, poultry, and even select studies in humans, to manipulate fermentation of dietary fibers (209–213). But how do these in vitro and in vivo studies translate to the complex system of the human bowel? As this review highlighted, differences in microbial composition and dietary factors present can result in substantial differences in host inflammatory response (21). Taking into account that each select microorganism utilizes a variety of different environmental sources from the host diet and microenvironment for carbon and energy, along with the dysbiotic nature of the microbiome of IBD patients, there is a clear possibility that incomplete fermentation, potentially due to dysbiotic microbiome, can result in buildup of pro-inflammatory byproducts such as succinate (214). Evidence also suggests an alternative option; specifically, when macrophage cells are stimulated by lipopolysaccharide (LPS), such as that found on pathobionts, a metabolic shift occurs, resulting in accumulation of succinate (214, 215). This in turn promotes biological pathways responsible for the production of pro-inflammatory cytokines, such as interleukin (IL) 1β, suggesting possible avenues for future therapeutics (66, 216). However, with the right combination of microbes and environmental factors, each of these fiber subtypes have been shown to provide anti-inflammatory benefits as highlighted above. It is important to re-highlight here that the gut microbes work in consort and therefore, it is not just one microbe species performing these fermentative processes in isolation; this adds to the complexity of the gut environment.
The precise interaction of whole chain fibers with the human bowel remains under-studied and complete answers to many questions still remain. How does the dysbiotic nature of IBD affect fiber fermentation? And how does this relate to the sensitivity that patients experience to dietary fibers, especially during disease flare ups? While sequencing and culture techniques continue to improve our ability to examine these system interactions, there remains a gap in understanding how these factors all align when it comes to microbes, nutrition, and gut health. Considering it is the gut microbiome that is responsible for the fermentation of dietary fibers, and that there is a great deal of variability among individuals, let alone IBD patients, one of the keys to understanding the role of dietary fibers in IBD, is likely in understanding which microbes must be present and/or absent for the healthy fermentation and production of SCFA to occur (18, 213, 217–219). This idea highlights the importance of patient-specific medicine. In this instance, future therapies will likely begin with profiling the gut microbiota of individuals and identifying a specific combination of dietary recommendations, prebiotic, and probiotic therapies, directed toward re-establishing a healthy, balanced microbiome capable of providing the appropriate sources of carbon and energy via fermentation. We believe that it is imperative to advance our understanding of fiber fermentation within the IBD gut to aid in improving dietary guidelines and therapeutic options, in order to promote the growth of a healthy microbiota and increase beneficial SCFAs in patients.
HA structured the manuscripts draft. HA and DA constructed all figures. All authors contributed to writing and editing the manuscript.
Work in the Wine Lab was supported by grants from the W. Garfield Weston Foundation and the Canadian Institutes for Health Research (CIHR). HA was supported by a CIHR Post-doctoral Fellowship.
The authors declare that the research was conducted in the absence of any commercial or financial relationships that could be construed as a potential conflict of interest.
1. Benchimol EI, Mack DR, Nguyen GC, Snapper SB, Li W, Mojaverian N, et al. Incidence, outcomes, and health services burden of very early onset inflammatory bowel disease. Gastroenterology. (2014) 147:803–13. doi: 10.1053/j.gastro.2014.06.023
2. Kaplan GG. The global burden of IBD: from 2015 to 2025. Nat Rev Gastroenterol Hepatol. (2015) 12:720–7. doi: 10.1038/nrgastro.2015.150
3. Rocchi A, Benchimol EI, Bernstein CN, Bitton A, Feagan B, Panaccione R, et al. Inflammatory bowel disease: a Canadian burden of illness review. Can J Gastroenterol. (2012) 26:811–7. doi: 10.1155/2012/984575
4. Elten M, Benchimol EI, Fell DB, Kuenzig ME, Smith G, Kaplan GG, et al. Residential greenspace in childhood reduces risk of pediatric inflammatory bowel disease: a population-based cohort study. Am J Gastroenterol. (2020). doi: 10.14309/ajg.0000000000000990
5. Abu Freha N, Schwartz D, Elkrinawi J, Ben Yakov G, Abu Tailakh M, Munteanu D, et al. Inflammatory bowel disease among bedouin arabs in Southern Israel: urbanization and increasing prevalence rates. Eur J Gastroenterol Hepatol. (2015) 27:230–4. doi: 10.1097/MEG.0000000000000263
6. Ng SC. Emerging leadership lecture: inflammatory bowel disease in Asia: emergence of a “Western” disease. J Gastroenterol Hepatol. (2015) 30:440–5. doi: 10.1111/jgh.12859
7. Gasparetto M, Guariso G. Highlights in IBD epidemiology and its natural history in the paediatric age. Gastroenterol Res Pract. (2013) 2013:829040. doi: 10.1155/2013/829040
8. Benchimol EI, Mack DR, Guttmann A, Nguyen GC, To T, Mojaverian N, et al. Inflammatory bowel disease in immigrants to Canada and their children: a population-based cohort study. Am J Gastroenterol. (2015) 110:553–63. doi: 10.1038/ajg.2015.52
9. Wine E. Should we be treating the bugs instead of cytokines and T cells? Dig Dis. (2014) 32:403–9. doi: 10.1159/000358146
10. Aujnarain A, Mack DR, Benchimol EI. The role of the environment in the development of pediatric inflammatory bowel disease. Curr Gastroenterol Rep. (2013) 15:326. doi: 10.1007/s11894-013-0326-4
11. Levine A, Wine E. Effects of enteral nutrition on Crohn's disease: clues to the impact of diet on disease pathogenesis. Inflammat Bowel Dis. (2013) 19:1322–9. doi: 10.1097/MIB.0b013e3182802acc
12. Alkadhi S, Kunde D, Cheluvappa R, Randall-Demllo S, Eri R. The murine appendiceal microbiome is altered in spontaneous colitis and its pathological progression. Gut Pathog. (2014) 6:25. doi: 10.1186/1757-4749-6-25
13. Michail S, Durbin M, Turner D, Griffiths AM, Mack DR, Hyams J, et al. Alterations in the gut microbiome of children with severe ulcerative colitis. Inflammat Bowel Dis. (2012) 18:1799–808. doi: 10.1002/ibd.22860
14. Sigall-Boneh R, Levine A, Lomer M, Wierdsma N, Allan P, Fiorino G, et al. Research gaps in diet and nutrition in inflammatory bowel disease. A topical review by D-ECCO working group [dietitians of ECCO]. J Crohn's Colitis. (2017) 11:1407–19. doi: 10.1093/ecco-jcc/jjx109
15. Lawley M, Wu JW, Navas-Lopez VM, Huynh HQ, Carroll MW, Chen M, et al. Global variation in use of enteral nutrition for pediatric crohn disease. J Pediatr Gastroenterol Nutr. (2018) 67:e22–e9. doi: 10.1097/MPG.0000000000001946
16. Knight-Sepulveda K, Kais S, Santaolalla R, Abreu MT. Diet and inflammatory bowel disease. Gastroenterol Hepatol. (2015) 11:511–20.
17. Singh RK, Chang HW, Yan D, Lee KM, Ucmak D, Wong K, et al. Influence of diet on the gut microbiome and implications for human health. J Transl Med. (2017) 15:73. doi: 10.1186/s12967-017-1175-y
18. Zmora N, Suez J, Elinav E. You are what you eat: diet, health and the gut microbiota. Nat Rev Gastroenterol Hepatol. (2019) 16:35–56. doi: 10.1038/s41575-018-0061-2
19. Levine A, Sigall Boneh R, Wine E. Evolving role of diet in the pathogenesis and treatment of inflammatory bowel diseases. Gut. (2018) 67:1726–38. doi: 10.1136/gutjnl-2017-315866
20. Van Limbergen J, Wine E, Assa A, Sigall Boneh R, Shaoul R, Kori M, et al. Crohn's disease exclusion diet is equally effective but better tolerated than exclusive enteral nutrition for induction of remission in mild-to-moderate pediatric crohn's disease: a prospective randomized controlled trial. Gastroenterology. (2019) 156:S59. doi: 10.1016/S0016-5085(19)36933-1
21. Levine A, Rhodes JM, Lindsay JO, Abreu MT, Kamm MA, Gibson PR, et al. Dietary guidance from the international organization for the study of inflammatory bowel diseases. Clin Gastroenterol Hepatol. (2020) 18:1381–92. doi: 10.1016/j.cgh.2020.01.046
22. Castro F, de Souza HSP. Dietary composition and effects in inflammatory bowel disease. Nutrients. (2019) 11:1398. doi: 10.3390/nu11061398
23. Cohen AB, Lee D, Long MD, Kappelman MD, Martin CF, Sandler RS, et al. Dietary patterns and self-reported associations of diet with symptoms of inflammatory bowel disease. Dig Dis Sci. (2013) 58:1322–8. doi: 10.1007/s10620-012-2373-3
24. Howeler JF, Hewson AD. Dietary fiber and toxaemia of pregnancy. Med J Aust. (1957) 44:761–3. doi: 10.5694/j.1326-5377.1957.tb59828.x
25. Hamaker BR, Tuncil YE. A perspective on the complexity of dietary fiber structures and their potential effect on the gut microbiota. J Mol Biol. (2014) 426:3838–50. doi: 10.1016/j.jmb.2014.07.028
26. Valcheva R, Dieleman LA. Prebiotics: definition and protective mechanisms. Best Pract Res Clin Gastroenterol. (2016) 30:27–37. doi: 10.1016/j.bpg.2016.02.008
27. Hollister EB, Gao C, Versalovic J. Compositional and functional features of the gastrointestinal microbiome and their effects on human health. Gastroenterology. (2014) 146:1449–58. doi: 10.1053/j.gastro.2014.01.052
28. Hillman ET, Lu H, Yao T, Nakatsu CH. Microbial ecology along the gastrointestinal tract. Microbes Environ. (2017) 32:300–13. doi: 10.1264/jsme2.ME17017
29. Tuddenham S, Sears CL. The intestinal microbiome and health. Curr Opin Infect Dis. (2015) 28:464–70. doi: 10.1097/QCO.0000000000000196
30. Scott KP, Antoine JM, Midtvedt T, van Hemert S. Manipulating the gut microbiota to maintain health and treat disease. Microb Ecol Health Dis. (2015) 26:25877. doi: 10.3402/mehd.v26.25877
31. Li D, Wang P, Wang P, Hu X, Chen F. Targeting the gut microbiota by dietary nutrients: a new avenue for human health. Crit Rev Food Sci Nutr. (2019) 59:181–95. doi: 10.1080/10408398.2017.1363708
32. Agriculture UDo. What We Eat in America: Nutrient Intakes From Food by Gender Age. National Health Nutrition Examination Survey (NHANES) 2009-10. Hyattsville, MD: U.S. Department of Health & Human Services, National Center for Health Statistics (2010).
33. Garcia-Meseguer MJ, Delicado-Soria A, Serrano-Urrea R. Fiber patterns in young adults living in different environments (USA, Spain, and Tunisia). Anthropometric and lifestyle characteristics. Nutrients. (2017) 9:1030. doi: 10.3390/nu9091030
34. Hallfrisch J, Tobin JD, Muller DC, Andres R. Fiber intake, age, and other coronary risk factors in men of the Baltimore longitudinal Study (1959-1975). J Gerontol. (1988) 43:M64–8. doi: 10.1093/geronj/43.3.M64
35. Quagliani D, Felt-Gunderson P. Closing America's fiber intake gap: communication strategies from a food and fiber summit. Am J Lifestyle Med. (2017) 11:80–5. doi: 10.1177/1559827615588079
36. Williams BA, Grant LJ, Gidley MJ, Mikkelsen D. Gut fermentation of dietary fibers: physico-chemistry of plant cell walls and implications for health. Int J Mol Sci. (2017) 18:2203. doi: 10.3390/ijms18102203
37. Leclercq S, Matamoros S, Cani PD, Neyrinck AM, Jamar F, Starkel P, et al. Intestinal permeability, gut-bacterial dysbiosis, and behavioral markers of alcohol-dependence severity. Proc Natl Acad Sci USA. (2014) 111:E4485–93. doi: 10.1073/pnas.1415174111
38. Wu H, Tremaroli V, Backhed F. Linking microbiota to human diseases: a systems biology perspective. Trends Endocrinol Metab. (2015) 26:758–70. doi: 10.1016/j.tem.2015.09.011
39. Larsson E, Tremaroli V, Lee YS, Koren O, Nookaew I, Fricker A, et al. Analysis of gut microbial regulation of host gene expression along the length of the gut and regulation of gut microbial ecology through MyD88. Gut. (2012) 61:1124–31. doi: 10.1136/gutjnl-2011-301104
40. Mudgil D, Barak S. Composition, properties and health benefits of indigestible carbohydrate polymers as dietary fiber: a review. Int J Biol Macromol. (2013) 61:1–6. doi: 10.1016/j.ijbiomac.2013.06.044
41. Chawla R, Patil GR. Soluble dietary fiber. Comprehensive Rev Food Sci Food Saf. (2010) 9:178–96. doi: 10.1111/j.1541-4337.2009.00099.x
42. Andoh A, Bamba T, Sasaki M. Physiological and anti-inflammatory roles of dietary fiber and butyrate in intestinal functions. JPEN J Parenter Enteral Nutr. (1999) 23:S70–3. doi: 10.1177/014860719902300518
43. Dhital S, Dolan G, Stokes JR, Gidley MJ. Enzymatic hydrolysis of starch in the presence of cereal soluble fiber polysaccharides. Food Func. (2014) 5:579–86. doi: 10.1039/c3fo60506j
44. Cockburn DW, Koropatkin NM. Polysaccharide degradation by the intestinal microbiota and its influence on human health and disease. J Mol Biol. (2016) 428:3230–52. doi: 10.1016/j.jmb.2016.06.021
45. Grondin JM, Tamura K, Dejean G, Abbott DW, Brumer H. Polysaccharide utilization loci: fueling microbial communities. J Bacteriol. (2017) 199:e00860-16. doi: 10.1128/JB.00860-16
46. Sharma M, Soni R, Nazir A, Oberoi HS, Chadha BS. Evaluation of glycosyl hydrolases in the secretome of aspergillus fumigatus and saccharification of alkali-treated rice straw. Appl Biochem Biotechnol. (2011) 163:577–91. doi: 10.1007/s12010-010-9064-3
47. Obenland DM, Simmen U, Boller T, Wiemken A. Purification and characterization of three soluble invertases from barley (Hordeum vulgare L.) leaves. Plant Physiol. (1993) 101:1331–9. doi: 10.1104/pp.101.4.1331
48. BenoitChassaing ATG. Gut microbiome and metabolism. In: Press A, editor. Physiology of the Gastrointestinal Tract. 6th Edn. Elsevier (2018). p. 775–93.
49. Lombard V, Golaconda Ramulu H, Drula E, Coutinho PM, Henrissat B. The carbohydrate-active enzymes database (CAZy) in (2013). Nucleic Acids Res. (2014) 42:D490–5. doi: 10.1093/nar/gkt1178
50. Bauer MA, Kainz K, Carmona-Gutierrez D, Madeo F. Microbial wars: competition in ecological niches and within the microbiome. Microb Cell. (2018) 5:215–9. doi: 10.15698/mic2018.05.628
51. Johnson AJ, Vangay P, Al-Ghalith GA, Hillmann BM, Ward TL, Shields-Cutler RR, et al. Daily sampling reveals personalized diet-microbiome associations in humans. Cell Host Microbe. (2019) 25:789–802.e5. doi: 10.1016/j.chom.2019.05.005
52. Patnode ML, Beller ZW, Han ND, Cheng J, Peters SL, Terrapon N, et al. Interspecies competition impacts targeted manipulation of human gut bacteria by fiber-derived glycans. Cell. (2019) 179:59–73.e13. doi: 10.1016/j.cell.2019.08.011
53. Chen T, Long W, Zhang C, Liu S, Zhao L, Hamaker BR. Fiber-utilizing capacity varies in prevotella- versus bacteroides-dominated gut microbiota. Sci Rep. (2017) 7:2594. doi: 10.1038/s41598-017-02995-4
54. Human Microbiome Project C. Structure, function and diversity of the healthy human microbiome. Nature. (2012) 486:207–14. doi: 10.1038/nature11234
55. Jeffery IB, Claesson MJ, O'Toole PW, Shanahan F. Categorization of the gut microbiota: enterotypes or gradients? Nat Rev Microbiol. (2012) 10:591–2. doi: 10.1038/nrmicro2859
56. Wu GD, Bushmanc FD, Lewis JD. Diet, the human gut microbiota, and IBD. Anaerobe. (2013) 24:117–20. doi: 10.1016/j.anaerobe.2013.03.011
57. Peterson DA, Frank DN, Pace NR, Gordon JI. Metagenomic approaches for defining the pathogenesis of inflammatory bowel diseases. Cell Host Microbe. (2008) 3:417–27. doi: 10.1016/j.chom.2008.05.001
58. Cummings JH, Bingham SA. Dietary fiber, fermentation and large bowel cancer. Cancer Surveys. (1987) 6:601–21.
59. Cummings JH, Pomare EW, Branch WJ, Naylor CP, Macfarlane GT. Short chain fatty acids in human large intestine, portal, hepatic and venous blood. Gut. (1987) 28:1221–7. doi: 10.1136/gut.28.10.1221
60. Rios-Covian D, Ruas-Madiedo P, Margolles A, Gueimonde M, de Los Reyes-Gavilan CG, Salazar N. Intestinal short chain fatty acids and their link with diet and human health. Front Microbiol. (2016) 7:185. doi: 10.3389/fmicb.2016.00185
61. Pistollato F, Sumalla Cano S, Elio I, Masias Vergara M, Giampieri F, Battino M. Role of gut microbiota and nutrients in amyloid formation and pathogenesis of alzheimer disease. Nutr Rev. (2016) 74:624–34. doi: 10.1093/nutrit/nuw023
62. Lozupone CA, Stombaugh JI, Gordon JI, Jansson JK, Knight R. Diversity, stability and resilience of the human gut microbiota. Nature. (2012) 489:220–30. doi: 10.1038/nature11550
63. Sonnenburg ED, Sonnenburg JL. Starving our microbial self: the deleterious consequences of a diet deficient in microbiota-accessible carbohydrates. Cell Metab. (2014) 20:779–86. doi: 10.1016/j.cmet.2014.07.003
64. Salyers AA, J D'Elia AR. Solving the problem of how to eat something as big as yourself: diverse bacterial strategies for degrading polysaccharides. J Ind Microbiol. (1996) 17:470–6. doi: 10.1007/BF01574778
65. Macfarlane GT. Gibson GR. Carbohydrate fermentation, energy transduction and gas metabolism in the human large intestine. In: Mackie RI, editor. Ecology and Physiology of Gastrointestinal Microbes. New York, NY: Chapman and Hall (1996). p. 269–318.
66. Armstrong H, Bording-Jorgensen M, Dijk S, Wine E. The complex interplay between chronic inflammation, the microbiome, and cancer: understanding disease progression and what we can do to prevent it. Cancers. (2018) 10:83. doi: 10.3390/cancers10030083
67. Sokol H, Lay C, Seksik P, Tannock GW. Analysis of bacterial bowel communities of IBD patients: what has it revealed? Inflammat Bowel Dis. (2008) 14:858–67. doi: 10.1002/ibd.20392
68. Andoh A, Tsujikawa T, Fujiyama Y. Role of dietary fiber and short-chain fatty acids in the colon. Curr Pharm Des. (2003) 9:347–58. doi: 10.2174/1381612033391973
69. Smith PM, Howitt MR, Panikov N, Michaud M, Gallini CA, Bohlooly YM, et al. The microbial metabolites, short-chain fatty acids, regulate colonic treg cell homeostasis. Science. (2013) 341:569–73. doi: 10.1126/science.1241165
70. den Besten G, van Eunen K, Groen AK, Venema K, Reijngoud DJ, Bakker BM. The role of short-chain fatty acids in the interplay between diet, gut microbiota, and host energy metabolism. J Lipid Res. (2013) 54:2325–40. doi: 10.1194/jlr.R036012
71. Halmos EP, Christophersen CT, Bird AR, Shepherd SJ, Gibson PR, Muir JG. Diets that differ in their FODMAP content alter the colonic luminal microenvironment. Gut. (2015) 64:93–100. doi: 10.1136/gutjnl-2014-307264
72. Costabile A, Klinder A, Fava F, Napolitano A, Fogliano V, Leonard C, et al. Whole-grain wheat breakfast cereal has a prebiotic effect on the human gut microbiota: a double-blind, placebo-controlled, crossover study. Br J Nutr. (2008) 99:110–20. doi: 10.1017/S0007114507793923
73. Carvalho-Wells AL, Helmolz K, Nodet C, Molzer C, Leonard C, McKevith B, et al. Determination of the in vivo prebiotic potential of a maize-based whole grain breakfast cereal: a human feeding study. Br J Nutr. (2010) 104:1353–6. doi: 10.1017/S0007114510002084
74. Walker AW, Ince J, Duncan SH, Webster LM, Holtrop G, Ze X, et al. Dominant and diet-responsive groups of bacteria within the human colonic microbiota. ISME J. (2011) 5:220–30. doi: 10.1038/ismej.2010.118
75. Keim NL, Martin RJ. Dietary whole grain-microbiota interactions: insights into mechanisms for human health. Adv Nutr. (2014) 5:556–7. doi: 10.3945/an.114.006536
76. Leitch EC, Walker AW, Duncan SH, Holtrop G, Flint HJ. Selective colonization of insoluble substrates by human faecal bacteria. Environ Microbiol. (2007) 9:667–79. doi: 10.1111/j.1462-2920.2006.01186.x
77. Wong JM, de Souza R, Kendall CW, Emam A, Jenkins DJ. Colonic health: fermentation and short chain fatty acids. J Clin Gastroenterol. (2006) 40:235–43. doi: 10.1097/00004836-200603000-00015
78. Pituch-Zdanowska A, Banaszkiewicz A, Albrecht P. The role of dietary fiber in inflammatory bowel disease. Prz Gastroenterol. (2015) 10:135–41. doi: 10.5114/pg.2015.52753
79. Collaborators GBDIBD. The global, regional, and national burden of inflammatory bowel disease in 195 countries and territories, 1990-2017: a systematic analysis for the global burden of disease study 2017. Lancet Gastroenterol Hepatol. (2020) 5:17–30. doi: 10.1016/S2468-1253(19)30333-4
80. Yao CK. The low-fiber diet: contender in IBD, or has it had its time? Lancet Gastroenterol Hepatol. (2019) 4:339. doi: 10.1016/S2468-1253(19)30096-2
81. Armstrong H, Alipour M, Valcheva R, Bording-Jorgensen M, Jovel J, Zaidi D, et al. Host immunoglobulin G selectively identifies pathobionts in pediatric inflammatory bowel diseases. Microbiome. (2019) 7:1. doi: 10.1186/s40168-018-0604-3
82. Andersen V, Chan S, Luben R, Khaw KT, Olsen A, Tjonneland A, et al. Fiber intake and the development of inflammatory bowel disease: a European prospective multi-centre cohort study (EPIC-IBD). J Crohns Colitis. (2018) 12:129–36. doi: 10.1093/ecco-jcc/jjx136
83. Schreiner P, Martinho-Grueber M, Studerus D, Vavricka SR, Tilg H, Biedermann L, et al. Nutrition in inflammatory bowel disease. Digestion. (2020) 101(Suppl. 1):120–35. doi: 10.1159/000505368
84. Ananthakrishnan AN, Khalili H, Konijeti GG, Higuchi LM, de Silva P, Korzenik JR, et al. A prospective study of long-term intake of dietary fiber and risk of crohn's disease and ulcerative colitis. Gastroenterology. (2013) 145:970–7. doi: 10.1053/j.gastro.2013.07.050
85. Ananthakrishnan AN, Khalili H, Song M, Higuchi LM, Richter JM, Nimptsch K, et al. High school diet and risk of crohn's disease and ulcerative colitis. Inflammat Bowel Dis. (2015) 21:2311–9. doi: 10.1097/MIB.0000000000000501
86. Liu X, Wu Y, Li F, Zhang D. Dietary fiber intake reduces risk of inflammatory bowel disease: result from a meta-analysis. Nutr Res. (2015) 35:753–8. doi: 10.1016/j.nutres.2015.05.021
87. Desai MS, Seekatz AM, Koropatkin NM, Kamada N, Hickey CA, Wolter M, et al. A dietary fiber-deprived gut microbiota degrades the colonic mucus barrier and enhances pathogen susceptibility. Cell. (2016) 167:1339–53.e21. doi: 10.1016/j.cell.2016.10.043
88. Pinzone MR, Celesia BM, Di Rosa M, Cacopardo B, Nunnari G. Microbial translocation in chronic liver diseases. Int J Microbiol. (2012) 2012:694629. doi: 10.1155/2012/694629
89. Chen X, Fruehauf J, Goldsmith JD, Xu H, Katchar KK, Koon HW, et al. Saccharomyces boulardii inhibits EGF receptor signaling and intestinal tumor growth in Apc(min) mice. Gastroenterology. (2009) 137:914–23. doi: 10.1053/j.gastro.2009.05.050
90. Venturi A, Gionchetti P, Rizzello F, Johansson R, Zucconi E, Brigidi P, et al. Impact on the composition of the faecal flora by a new probiotic preparation: preliminary data on maintenance treatment of patients with ulcerative colitis. Aliment Pharmacol Ther. (1999) 13:1103–8. doi: 10.1046/j.1365-2036.1999.00560.x
91. Eloe-Fadrosh EA, Brady A, Crabtree J, Drabek EF, Ma B, Mahurkar A, et al. Functional dynamics of the gut microbiome in elderly people during probiotic consumption. Mbio. (2015) 6:e0023115. doi: 10.1128/mBio.00231-15
92. Barcenilla A, Pryde SE, Martin JC, Duncan SH, Stewart CS, Henderson C, et al. Phylogenetic relationships of butyrate-producing bacteria from the human gut. Appl Environ Microbiol. (2000) 66:1654–61. doi: 10.1128/AEM.66.4.1654-1661.2000
93. Schneider H, Simmering R, Hartmann L, Pforte H, Blaut M. Degradation of quercetin-3-glucoside in gnotobiotic rats associated with human intestinal bacteria. J Appl Microbiol. (2000) 89:1027–37. doi: 10.1046/j.1365-2672.2000.01209.x
94. Miquel S, Martin R, Rossi O, Bermudez-Humaran LG, Chatel JM, Sokol H, et al. Faecalibacterium prausnitzii and human intestinal health. Curr Opin Microbiol. (2013) 16:255–61. doi: 10.1016/j.mib.2013.06.003
95. Walters WA, Xu Z, Knight R. Meta-analyses of human gut microbes associated with obesity and IBD. FEBS Lett. (2014) 588:4223–33. doi: 10.1016/j.febslet.2014.09.039
96. Aldini R, Micucci M, Cevenini M, Fato R, Bergamini C, Nanni C, et al. Antiinflammatory effect of phytosterols in experimental murine colitis model: prevention, induction, remission study. PLoS ONE. (2014) 9:e108112. doi: 10.1371/journal.pone.0108112
97. Suchecka D, Harasym J, Wilczak J, Gromadzka-Ostrowska J. Hepato- and gastro- protective activity of purified oat 1-3, 1-4-beta-d-glucans of different molecular weight. Int J Biol Macromol. (2016) 91:1177–85. doi: 10.1016/j.ijbiomac.2016.06.062
98. Wilczak J, Blaszczyk K, Kamola D, Gajewska M, Harasym JP, Jalosinska M, et al. The effect of low or high molecular weight oat beta-glucans on the inflammatory and oxidative stress status in the colon of rats with LPS-induced enteritis. Food Func. (2015) 6:590–603. doi: 10.1039/C4FO00638K
99. Luceri C, Femia AP, Fazi M, Di Martino C, Zolfanelli F, Dolara P, et al. Effect of butyrate enemas on gene expression profiles and endoscopic/histopathological scores of diverted colorectal mucosa: a randomized trial. Dig Liver Dis. (2016) 48:27–33. doi: 10.1016/j.dld.2015.09.005
100. Faghfoori Z, Shakerhosseini R, Navai L, Somi MH, Nikniaz Z, Abadi A. Effects of an oral supplementation of germinated barley foodstuff on serum CRP level and clinical signs in patients with ulcerative colitis. Health Promot Perspect. (2014) 4:116–21. doi: 10.5681/hpp.2014.015
101. Fritsch J, Garces L, Quintero MA, Pignac-Kobinger J, Santander AM, Fernandez I, et al. Low-fat, high-fiber diet reduces markers of inflammation and dysbiosis and improves quality of life in patients with ulcerative colitis. Clin Gastroenterol Hepatol. (2020). doi: 10.1016/j.cgh.2020.05.026
102. Shinde T, Perera AP, Vemuri R, Gondalia SV, Karpe AV, Beale DJ, et al. Synbiotic supplementation containing whole plant sugar cane fiber and probiotic spores potentiates protective synergistic effects in mouse model of IBD. Nutrients. (2019) 11:818. doi: 10.3390/nu11040818
103. Kuda TY, Shikano A, Takei M, Takahashi H, Kimura B. Dietary and lifestyle disease indices and caecal microbiota in high fat diet, dietary fiber free diet, or DSS induced IBD models in ICR mice. J Func Foods. (2017) 35:605–14. doi: 10.1016/j.jff.2017.06.030
104. Couto MR, Goncalves P, Magro F, Martel F. Microbiota-derived butyrate regulates intestinal inflammation: focus on inflammatory bowel disease. Pharmacol Res. (2020) 159:104947. doi: 10.1016/j.phrs.2020.104947
105. Wang RX, Lee JS, Campbell EL, Colgan SP. Microbiota-derived butyrate dynamically regulates intestinal homeostasis through regulation of actin-associated protein synaptopodin. Proc Natl Acad Sci USA. (2020) 117:11648–57. doi: 10.1073/pnas.1917597117
106. Chen G, Ran X, Li B, Li Y, He D, Huang B, et al. Sodium butyrate inhibits inflammation and maintains epithelium barrier integrity in a TNBS-induced inflammatory bowel disease mice model. EBioMedicine. (2018) 30:317–25. doi: 10.1016/j.ebiom.2018.03.030
107. Geirnaert A, Calatayud M, Grootaert C, Laukens D, Devriese S, Smagghe G, et al. Butyrate-producing bacteria supplemented in vitro to crohn's disease patient microbiota increased butyrate production and enhanced intestinal epithelial barrier integrity. Sci Rep. (2017) 7:11450. doi: 10.1038/s41598-017-11734-8
108. Vancamelbeke M, Laeremans T, Vanhove W, Arnauts K, Ramalho AS, Farre R, et al. Butyrate does not protect against inflammation-induced loss of epithelial barrier function and cytokine production in primary cell monolayers from patients with ulcerative colitis. J Crohns Colitis. (2019) 13:1351–61. doi: 10.1093/ecco-jcc/jjz064
109. Ferrer-Picon E, Dotti I, Corraliza AM, Mayorgas A, Esteller M, Perales JC, et al. Intestinal inflammation modulates the epithelial response to butyrate in patients with inflammatory bowel disease. Inflammat Bowel Dis. (2020) 26:43–55. doi: 10.1093/ibd/izz119
110. Brown AC, Rampertab SD, Mullin GE. Existing dietary guidelines for crohn's disease and ulcerative colitis. Expert Rev Gastroenterol Hepatol. (2011) 5:411–25. doi: 10.1586/egh.11.29
111. Owczarek D, Rodacki T, Domagala-Rodacka R, Cibor D, Mach T. Diet and nutritional factors in inflammatory bowel diseases. World J Gastroenterol. (2016) 22:895–905. doi: 10.3748/wjg.v22.i3.895
112. Kiszonas A, Fuerst EP, Morris CF. Wheat arabinoxylan structure provides insight into function. Cereal Chem. (2013) 90:387–95. doi: 10.1094/CCHEM-02-13-0025-FI
113. Dornez E, Gebruers K, Delcour JA, Courtin C. Grain-associated xylanases: Occurrence, variability, and implications for cereal processing. Trends Food Sci. Technol. (2009) 20:495–510. doi: 10.1016/j.tifs.2009.05.004
114. Zhang ZS, Li W. Extraction and modification technology of arabinoxylans from cereal by-products: a critical review. Food Res Int. (2014) 65:423–36. doi: 10.1016/j.foodres.2014.05.068
115. Mendis M, Leclerc E, Simsek S. Arabinoxylans, gut microbiota and immunity. Carbohydr Polym. (2016) 139:159–66. doi: 10.1016/j.carbpol.2015.11.068
116. Lu Z, Walker KZ, Muir JG, Mascara, et al. Arabinoxylan fiber, a byproduct of wheat flour processing, reduces the postprandial glucose response in normoglycemic subjects. Am J Clin Nutr. (2000) 71:1123–8. doi: 10.1093/ajcn/71.5.1123
117. Henry R. A comparison of the non-starch carbohydrates in cereal grains. J Sci Food Agric. (1985) 36:1243–53. doi: 10.1002/jsfa.2740361207
118. Nguyen NK, Deehan EC, Zhang Z, Jin M, Baskota N, Perez-Munoz ME, et al. Gut microbiota modulation with long-chain corn bran arabinoxylan in adults with overweight and obesity is linked to an individualized temporal increase in fecal propionate. Microbiome. (2020) 8:118. doi: 10.1186/s40168-020-00887-w
119. Tremaroli V, Backhed F. Functional interactions between the gut microbiota and host metabolism. Nature. (2012) 489:242–9. doi: 10.1038/nature11552
120. Cooper-Bribiesca B, Navarro-Ocana A, Diaz-Ruiz G, Aguilar-Osorio G, Rodriguez-Sanoja R, Wacher C. Lactic acid fermentation of arabinoxylan from nejayote by Streptococcus infantarius ssp. infantarius 25124 isolated from pozol. Front. Microbiol. (2018) 9:3061. doi: 10.3389/fmicb.2018.03061
121. Pastell H, Westermann P, Meyer AS, Tuomainen P, Tenkanen M. In vitro fermentation of arabinoxylan-derived carbohydrates by bifidobacteria and mixed fecal microbiota. J Agric Food Chem. (2009) 57:8598–606. doi: 10.1021/jf901397b
122. Bettiga M, Bengtsson O, Hahn-Hagerdal B, Gorwa-Grauslund MF. Arabinose and xylose fermentation by recombinant saccharomyces cerevisiae expressing a fungal pentose utilization pathway. Microb Cell Fact. (2009) 8:40. doi: 10.1186/1475-2859-8-40
123. Adams EL, Rice PJ, Graves B, Ensley HE, Yu H, Brown GD, et al. Differential high-affinity interaction of dectin-1 with natural or synthetic glucans is dependent upon primary structure and is influenced by polymer chain length and side-chain branching. J Pharmacol Exp Ther. (2008) 325:115–23. doi: 10.1124/jpet.107.133124
124. Kawasaki T, Fujimi S, Lederer JA, Hubbard WJ, Choudhry MA, Schwacha MG, et al. Trauma-hemorrhage induces depressed splenic dendritic cell functions in mice. J Immunol. (2006) 177:4514–20. doi: 10.4049/jimmunol.177.7.4514
125. Zhang Z, Smith C, Li W, Ashworth J. Characterization of nitric oxide modulatory activities of alkaline-extracted and enzymatic-modified arabinoxylans from corn bran in cultured human monocytes. J Agric Food Chem. (2016) 64:8128–37. doi: 10.1021/acs.jafc.6b02896
126. Ogawa K, Takeuchi M, Nakamura N. Immunological effects of partially hydrolyzed arabinoxylan from corn husk in mice. Biosci Biotechnol Biochem. (2005) 69:19–25. doi: 10.1271/bbb.69.19
127. Mendis M, Leclerc E, Simsek S. Arabinoxylan hydrolyzates as immunomodulators in Caco-2 and HT-29 colon cancer cell lines. Food Func. (2017) 8:220–31. doi: 10.1039/C6FO00866F
128. Van den Abbeele P, Gerard P, Rabot S, Bruneau A, El Aidy S, Derrien M, et al. Arabinoxylans and inulin differentially modulate the mucosal and luminal gut microbiota and mucin-degradation in humanized rats. Environ Microbiol. (2011) 13:2667–80. doi: 10.1111/j.1462-2920.2011.02533.x
129. Sun J, Shen X, Li Y, Guo Z, Zhu W, Zuo L, et al. Therapeutic potential to modify the mucus barrier in inflammatory bowel disease. Nutrients. (2016) 8:44. doi: 10.3390/nu8010044
130. Kanauchi O, Mitsuyama K, Homma T, Takahama K, Fujiyama Y, Andoh A, et al. Treatment of ulcerative colitis patients by long-term administration of germinated barley foodstuff: multi-center open trial. Int J Mol Med. (2003) 12:701–4. doi: 10.3892/ijmm.12.5.701
131. Neyrinck AM, Possemiers S, Druart C, Van de Wiele T, De Backer F, Cani PD, et al. Prebiotic effects of wheat arabinoxylan related to the increase in bifidobacteria, roseburia and bacteroides/prevotella in diet-induced obese mice. PLoS ONE. (2011) 6:e20944. doi: 10.1371/journal.pone.0020944
132. James SL, Christophersen CT, Bird AR, Conlon MA, Rosella O, Gibson PR, et al. Abnormal fiber usage in UC in remission. Gut. (2015) 64:562–70. doi: 10.1136/gutjnl-2014-307198
133. Velasquez-Manoff M. Gut microbiome: the peacekeepers. Nature. (2015) 518:S3–11. doi: 10.1038/scientificamerican0315-S3
134. Akramiene D, Kondrotas A, Didziapetriene J, Kevelaitis E. Effects of beta-glucans on the immune system. Medicina. (2007) 43:597–606. doi: 10.3390/medicina43080076
135. El Khoury D, Cuda C, Luhovyy BL, Anderson GH. Beta glucan: health benefits in obesity and metabolic syndrome. J Nutr Metab. (2012) 2012:851362. doi: 10.1155/2012/851362
136. Vetvicka V, Větvičková J. Physiological effects of different types of beta-glucan. Biomed Pap Med Fac Univ Palacky Olomouc Czech Repub. (2007) 151:225–31. doi: 10.5507/bp.2007.038
137. Vetvicka V, Vetvickova J. B1, 3-glucan: silver bullet or hot air? Open Glycosci. (2010) 3:1–6. doi: 10.2174/1875398101003010001
138. Lam K, Keung H, Ko K, Kwan H, Cheung PC. In vitro fermentation of beta-glucans and other selected carbohydrates by infant fecal inoculum: An evaluation of their potential as prebiotics in infant formula. Bioact Carbohydr Diet Fiber. (2018) 14:20–4. doi: 10.1016/j.bcdf.2017.07.009
139. Zhao J, Cheung P. Fermentation of β-glucans derived from different sources by bifidobacteria: evaluation of their bifidogenic effect. J Agric Food Chem. (2011) 59:5986–92. doi: 10.1021/jf200621y
140. Sakisaka H, Takedatsu H, Mitsuyama K, Mochizuki S, Sakurai K, Sakisaka S, et al. Topical therapy with antisense tumor necrosis factor alpha using novel beta-glucan-based drug delivery system ameliorates intestinal inflammation. Int J Mol Sci. (2020) 21:683. doi: 10.3390/ijms21020683
141. Tang C, Kamiya T, Liu Y, Kadoki M, Kakuta S, Oshima K, et al. Inhibition of dectin-1 signaling ameliorates colitis by inducing lactobacillus-mediated regulatory T cell expansion in the intestine. Cell Host Microbe. (2015) 18:183–97. doi: 10.1016/j.chom.2015.07.003
142. Vetvicka V, Gover O, Hayby H, Danay O, Ezov N, Hadar Y, et al. Immunomodulating effects exerted by glucans extracted from the king oyster culinary-medicinal mushroom Pleurotus eryngii (Agaricomycetes) grown in substrates containing various concentrations of olive mill waste. Int J Med Mushrooms. (2019) 21:765–81. doi: 10.1615/IntJMedMushrooms.2019031549
143. Heinsbroek SE, Williams DL, Welting O, Meijer SL, Gordon S, de Jonge WJ. Orally delivered beta-glucans aggravate dextran sulfate sodium (DSS)-induced intestinal inflammation. Nutr Res. (2015) 35:1106–12. doi: 10.1016/j.nutres.2015.09.017
144. Mitmesser S, Combs M. Chapter 23 - Prebiotics: Inulin and Other Oligosaccharides. In: Floch MH, Ringel Y, Allan Walker W, editors. The Microbiota in Gastrointestinal Pathophysiology. Academic Press (2017). p. 201–8.
145. Fraberger V, Call LM, Domig KJ, D'Amico S. Applicability of yeast fermentation to reduce fructans and other FODMAPs. Nutrients. (2018) 10:1247. doi: 10.3390/nu10091247
146. Falony G, Lazidou K, Verschaeren A, Weckx S, Maes D, De Vuyst L. In vitro kinetic analysis of fermentation of prebiotic inulin-type fructans by bifidobacterium species reveals four different phenotypes. Appl Environ Microbiol. (2009) 75:454–61. doi: 10.1128/AEM.01488-08
147. Gibson GR, Roberfroid MB. Dietary modulation of the human colonic microbiota: introducing the concept of prebiotics. J Nutr. (1995) 125:1401–12. doi: 10.1093/jn/125.6.1401
148. Picard C, Fioramonti J, Francois A, Robinson T, Neant F, Matuchansky C. Review article: bifidobacteria as probiotic agents – physiological effects and clinical benefits. Aliment Pharmacol Ther. (2005) 22:495–512. doi: 10.1111/j.1365-2036.2005.02615.x
149. Flint HJ, Scott KP, Duncan SH, Louis P, Forano E. Microbial degradation of complex carbohydrates in the gut. Gut microbes. (2012) 3:289–306. doi: 10.4161/gmic.19897
150. Leenen CH, Dieleman LA. Inulin and oligofructose in chronic inflammatory bowel disease. J Nutr. (2007) 137:2572S−5S. doi: 10.1093/jn/137.11.2572S
151. Akram W, Garud N, Joshi R. Role of inulin as prebiotics on inflammatory bowel disease. Drug Discov Ther. (2019) 13:1–8. doi: 10.5582/ddt.2019.01000
152. Laurell A, Sjoberg K. Prebiotics and synbiotics in ulcerative colitis. Scand J Gastroenterol. (2017) 52:477–85. doi: 10.1080/00365521.2016.1263680
153. Guarner F. Prebiotics in inflammatory bowel diseases. Br J Nutr. (2007) 98 (Suppl. 1):S85–9. doi: 10.1017/S0007114507832958
154. Wang X, Guo R, Lv Y, Fu R. The regulatory role of Fos related antigen1 in inflammatory bowel disease. Mol Med Rep. (2018) 17:1979–85. doi: 10.3892/mmr.2017.8071
155. Kim KJ, Park JM, Lee JS, Kim YS, Kangwan N, Han YM, et al. Oligonol prevented the relapse of dextran sulfate sodium-ulcerative colitis through enhancing NRF2-mediated antioxidative defense mechanism. J Physiol Pharmacol. (2018) 69. doi: 10.26402/jpp.2018.3.03
156. Speert DP, Eftekhar F, Puterman ML. Nonopsonic phagocytosis of strains of pseudomonas aeruginosa from cystic fibrosis patients. Infect Immun. (1984) 43:1006–11. doi: 10.1128/IAI.43.3.1006-1011.1984
157. Sehgal G, Zhang K, Todd RF 3rd, Boxer LA, Petty HR. Lectin-like inhibition of immune complex receptor-mediated stimulation of neutrophils. Effects on cytosolic calcium release and superoxide production. J Immunol. (1993) 150:4571–80.
158. Seifert S, Watzl B. Inulin and oligofructose: review of experimental data on immune modulation. J Nutr. (2007) 137:2563S−7S. doi: 10.1093/jn/137.11.2563S
159. Miles JP, Zou J, Kumar MV, Pellizzon M, Ulman E, Ricci M, et al. Supplementation of low- and high-fat diets with fermentable fiber exacerbates severity of DSS-induced acute colitis. Inflammat Bowel Dis. (2017) 23:1133–43. doi: 10.1097/MIB.0000000000001155
160. Singh V, Yeoh BS, Chassaing B, Xiao X, Saha P, Aguilera Olvera R, et al. Dysregulated microbial fermentation of soluble fiber induces cholestatic liver cancer. Cell. (2018) 175:679–94.e22. doi: 10.1016/j.cell.2018.09.004
161. Illanes A, Guerrero C, Vera C, Wilson L, Conejeros R, Scott, et al. Chapter 1 lactose production and upgrading. In: Lactose-Derived Prebiotics. Elsevier (2016). p. 1–33.
162. Ackerman DL, Craft KM, Townsend SD. Infant food applications of complex carbohydrates: structure, synthesis, and function. Carbohydr Res. (2017) 437:16–27. doi: 10.1016/j.carres.2016.11.007
163. Krumbeck JA, Rasmussen HE, Hutkins RW, Clarke J, Shawron K, Keshavarzian A, et al. Probiotic bifidobacterium strains and galactooligosaccharides improve intestinal barrier function in obese adults but show no synergism when used together as synbiotics. Microbiome. (2018) 6:121. doi: 10.1186/s40168-018-0494-4
164. Davis LM, Martinez I, Walter J, Hutkins R. A dose dependent impact of prebiotic galactooligosaccharides on the intestinal microbiota of healthy adults. Int J Food Microbiol. (2010) 144:285–92. doi: 10.1016/j.ijfoodmicro.2010.10.007
165. Azcarate-Peril MA, Ritter AJ, Savaiano D, Monteagudo-Mera A, Anderson C, Magness ST, et al. Impact of short-chain galactooligosaccharides on the gut microbiome of lactose-intolerant individuals. Proc Natl Acad Sci USA. (2017) 114:E367–E75. doi: 10.1073/pnas.1606722113
166. Sokol H, Pigneur B, Watterlot L, Lakhdari O, Bermudez-Humaran LG, Gratadoux JJ, et al. Faecalibacterium prausnitzii is an anti-inflammatory commensal bacterium identified by gut microbiota analysis of Crohn disease patients. Proc Natl Acad Sci USA. (2008) 105:16731–6. doi: 10.1073/pnas.0804812105
167. Pascal V, Pozuelo M, Borruel N, Casellas F, Campos D, Santiago A, et al. A microbial signature for crohn's disease. Gut. (2017) 66:813–22. doi: 10.1136/gutjnl-2016-313235
168. Silk DB, Davis A, Vulevic J, Tzortzis G, Gibson GR. Clinical trial: the effects of a trans-galactooligosaccharide prebiotic on faecal microbiota and symptoms in irritable bowel syndrome. Aliment Pharmacol Ther. (2009) 29:508–18. doi: 10.1111/j.1365-2036.2008.03911.x
169. Dongowski G, Lorenz A, Anger H. Degradation of pectins with different degrees of esterification by bacteroides thetaiotaomicron isolated from human gut flora. Appl Environ Microbiol. (2000) 66:1321–7. doi: 10.1128/AEM.66.4.1321-1327.2000
170. Sahasrabudhe NM, Beukema M, Tian L, Troost B, Scholte J, Bruininx E, et al. Dietary fiber pectin directly blocks toll-like receptor 2-1 and prevents doxorubicin-induced ileitis. Front Immunol. (2018) 9:383. doi: 10.3389/fimmu.2018.00383
171. Tian L, Scholte J, Borewicz K, van den Bogert B, Smidt H, Scheurink AJ, et al. Effects of pectin supplementation on the fermentation patterns of different structural carbohydrates in rats. Mol Nutr Food Res. (2016) 60:2256–66. doi: 10.1002/mnfr.201600149
172. Luis AS, Briggs J, Zhang X, Farnell B, Ndeh D, Labourel A, et al. Dietary pectic glycans are degraded by coordinated enzyme pathways in human colonic bacteroides. Nat Microbiol. (2018) 3:210–9. doi: 10.1038/s41564-017-0079-1
173. Larsen N, Bussolo de Souza C, Krych L, Barbosa Cahu T, Wiese M, Kot W, et al. Potential of pectins to beneficially modulate the gut microbiota depends on their structural properties. Front Microbiol. (2019) 10:223. doi: 10.3389/fmicb.2019.00223
174. Kaur S, Gupta VK. Production of pectinolytic enzymes pectinase and pectin lyase by bacillus subtilis SAV-21 in solid state fermentation. Ann Microbiol. (2017) 67:333–42. doi: 10.1007/s13213-017-1264-4
175. Dittoe DK, Barabote RD, Rothrock MJ, Ricke SC. Assessment of a potential role of Dickeya dadantii DSM 18020 as a pectinase producer for utilization in poultry diets based on in silico analyses. Front Microbiol. (2020) 11:751. doi: 10.3389/fmicb.2020.00751
176. Benoit I, Coutinho PM, Schols HA, Gerlach JP, Henrissat B, de Vries RP. Degradation of different pectins by fungi: correlations and contrasts between the pectinolytic enzyme sets identified in genomes and the growth on pectins of different origin. BMC Genomics. (2012) 13:321. doi: 10.1186/1471-2164-13-321
177. Kuivanen J, Biz A, Richard P. Microbial hexuronate catabolism in biotechnology. AMB Express. (2019) 9:16. doi: 10.1186/s13568-019-0737-1
178. Salman H, Bergman M, Djaldetti M, Orlin J, Bessler H. Citrus pectin affects cytokine production by human peripheral blood mononuclear cells. Biomed Pharmacother. (2008) 62:579–82. doi: 10.1016/j.biopha.2008.07.058
179. Ye MB, Lim BO. Dietary pectin regulates the levels of inflammatory cytokines and immunoglobulins in interleukin-10 knockout mice. J Agric Food Chem. (2010) 58:11281–6. doi: 10.1021/jf103262s
180. Ishisono K, Mano T, Yabe T, Kitaguchi K. Dietary fiber pectin ameliorates experimental colitis in a neutral sugar side chain-dependent manner. Front. Immunol. (2019) 10:2979. doi: 10.3389/fimmu.2019.02979
181. Lattimer JM, Haub MD. Effects of dietary fiber and its components on metabolic health. Nutrients. (2010) 2:1266–89. doi: 10.3390/nu2121266
183. Murray WD. Symbiotic relationship of Bacteroides cellulosolvens and Clostridium saccharolyticum in cellulose fermentation. Appl Environ Microbiol. (1986) 51:710–4. doi: 10.1128/AEM.51.4.710-714.1986
184. Gokarn RR, Eiteman MA, Martin SA, Eriksson KE. Production of succinate from glucose, cellobiose, and various cellulosic materials by the ruminal anaerobic bacteria Fibrobacter succinogenes and Ruminococcus flavefaciens. Appl Biochem Biotechnol. (1997) 68:69–80. doi: 10.1007/BF02785981
185. Bernalier A, Fonty G, Bonnemoy F, Gouet P. Degradation and fermentation of cellulose by the rumen anaerobic fungi in axenic cultures or in association with cellulolytic bacteria. Curr Microbiol. (1992) 25:143–8. doi: 10.1007/BF01571022
186. Mills E, O'Neill LA. Succinate: a metabolic signal in inflammation. Trends Cell Biol. (2014) 24:313–20. doi: 10.1016/j.tcb.2013.11.008
187. Zaidi D, Wine E. Building fences: how A20 protects the intestinal mucosa in inflammatory bowel diseases. Dig Dis Sci. (2020) 65:1288–90. doi: 10.1007/s10620-019-05964-1
188. Kim Y, Hwang SW, Kim S, Lee YS, Kim TY, Lee SH, et al. Dietary cellulose prevents gut inflammation by modulating lipid metabolism and gut microbiota. Gut Microbes. (2020) 11:944–61. doi: 10.1080/19490976.2020.1730149
189. Davis SR, Cousins RJ. Metallothionein expression in animals: a physiological perspective on function. J Nutr. (2000) 130:1085–8. doi: 10.1093/jn/130.5.1085
190. Inoue K, Takano H, Shimada A, Satoh M. Metallothionein as an anti-inflammatory mediator. Mediators Inflamm. (2009) 2009:101659. doi: 10.1155/2009/101659
191. Waeytens A, De Vos M, Laukens D. Evidence for a potential role of metallothioneins in inflammatory bowel diseases. Mediators Inflamm. (2009) 2009:729172. doi: 10.1155/2009/729172
192. Nagy-Szakal D, Hollister EB, Luna RA, Szigeti R, Tatevian N, Smith CW, et al. Cellulose supplementation early in life ameliorates colitis in adult mice. PLoS ONE. (2013) 8:e56685. doi: 10.1371/journal.pone.0056685
193. Morowitz MJ, Di Caro V, Pang D, Cummings J, Firek B, Rogers MB, et al. Dietary supplementation with nonfermentable fiber alters the gut microbiota and confers protection in murine models of sepsis. Crit Care Med. (2017) 45:e516–e23. doi: 10.1097/CCM.0000000000002291
194. Yoder S, Lancaster SM, Hullar MAJ, Lampe, et al. Chapter 7 - Gut microbial metabolism of plant lignans: influence on human health. In: Press A, editor. Diet-Microbe Interactions in the Gut. Elsevier (2015). p. 103–17. doi: 10.1016/B978-0-12-407825-3.00007-1
195. Janusz G, Pawlik A, Sulej J, Swiderska-Burek U, Jarosz-Wilkolazka A, Paszczynski A. Lignin degradation: microorganisms, enzymes involved, genomes analysis and evolution. FEMS Microbiol Rev. (2017) 41:941–62. doi: 10.1093/femsre/fux049
196. Bugg TD, Ahmad M, Hardiman EM, Rahmanpour R. Pathways for degradation of lignin in bacteria and fungi. Nat Prod Rep. (2011) 28:1883–96. doi: 10.1039/c1np00042j
197. Horisawa S, Ando H, Ariga O, Sakuma Y. Direct ethanol production from cellulosic materials by consolidated biological processing using the wood rot fungus Schizophyllum commune. Bioresour Technol. (2015) 197:37–41. doi: 10.1016/j.biortech.2015.08.031
198. Lee S, Kang M, Bae JH, Sohn JH, Sung BH. Bacterial valorization of lignin: strains, enzymes, conversion pathways, biosensors, and perspectives. Front Bioeng Biotechnol. (2019) 7:209. doi: 10.3389/fbioe.2019.00209
199. Anthony WE, Carr RR, DeLorenzo DM, Campbell TP, Shang Z, Foston M, et al. Development of Rhodococcus opacus as a chassis for lignin valorization and bioproduction of high-value compounds. Biotechnol Biofuels. (2019) 12:192. doi: 10.1186/s13068-019-1535-3
200. Ponnusamy VK, Nguyen DD, Dharmaraja J, Shobana S, Banu JR, Saratale RG, et al. A review on lignin structure, pretreatments, fermentation reactions and biorefinery potential. Bioresour Technol. (2019) 271:462–72. doi: 10.1016/j.biortech.2018.09.070
201. Ravi K, Garcia-Hidalgo J, Gorwa-Grauslund MF, Liden G. Conversion of lignin model compounds by Pseudomonas putida KT2440 and isolates from compost. Appl Microbiol Biotechnol. (2017) 101:5059–70. doi: 10.1007/s00253-017-8211-y
202. Pietrofesa RA, Velalopoulou A, Arguiri E, Menges CW, Testa JR, Hwang WT, et al. Flaxseed lignans enriched in secoisolariciresinol diglucoside prevent acute asbestos-induced peritoneal inflammation in mice. Carcinogenesis. (2016) 37:177–87. doi: 10.1093/carcin/bgv174
203. Khalatbari Soltani S, Jamaluddin R, Tabibi H, Mohd Yusof BN, Atabak S, Loh SP, et al. Effects of flaxseed consumption on systemic inflammation and serum lipid profile in hemodialysis patients with lipid abnormalities. Hemodial Int. (2013) 17:275–81. doi: 10.1111/j.1542-4758.2012.00754.x
204. Roberts CL, Keita AV, Duncan SH, O'Kennedy N, Soderholm JD, Rhodes JM, et al. Translocation of crohn's disease Escherichia coli across M-cells: contrasting effects of soluble plant fibers and emulsifiers. Gut. (2010) 59:1331–9. doi: 10.1136/gut.2009.195370
205. Ross JK, English C, Perlmutter CA. Dietary fiber constituents of selected fruits and vegetables. J Am Diet Assoc. (1985) 85:1111–6.
206. van Rheenen PF, Aloi M, Assa A, Bronsky J, Escher JC, Fagerberg UL, et al. The medical management of paediatric crohn's disease: an ECCO-ESPGHAN guideline update. J Crohn's Colitis. (2020) doi: 10.1093/ecco-jcc/jjaa161
207. Levine A, Wine E, Assa A, Sigall Boneh R, Shaoul R, Kori M, et al. Crohn's disease exclusion diet plus partial enteral nutrition induces sustained remission in a randomized controlled trial. Gastroenterology. (2019) 157:440–50.e8. doi: 10.1053/j.gastro.2019.04.021
208. Xu L, Lochhead P, Ko Y, Claggett B, Leong RW, Ananthakrishnan AN. Systematic review with meta-analysis: breastfeeding and the risk of crohn's disease and ulcerative colitis. Aliment Pharm Ther. (2017) 46:780–9. doi: 10.1111/apt.14291
209. Li D, Chen H, Mao B, Yang Q, Zhao J, Gu Z, et al. Microbial biogeography and core microbiota of the rat digestive tract. Sci Rep. (2017) 8:45840. doi: 10.1038/srep45840
210. Koistinen VM, Karkkainen O, Borewicz K, Zarei I, Jokkala J, Micard V, et al. Contribution of gut microbiota to metabolism of dietary glycine betaine in mice and in vitro colonic fermentation. Microbiome. (2019) 7:103. doi: 10.1186/s40168-019-0718-2
211. Wu Z, Zhou H, Li F, Zhang N, Zhu Y. Effect of dietary fiber levels on bacterial composition with age in the cecum of meat rabbits. Microbiologyopen. (2019) 8:e00708. doi: 10.1002/mbo3.708
212. Jha R, Fouhse JM, Tiwari UP, Li L, Willing BP. Dietary fiber and intestinal health of monogastric animals. Front Vet Sci. (2019) 6:48. doi: 10.3389/fvets.2019.00048
213. Holscher HD. Dietary fiber and prebiotics and the gastrointestinal microbiota. Gut Microbes. (2017) 8:172–84. doi: 10.1080/19490976.2017.1290756
214. Tannahill GM, Curtis AM, Adamik J, Palsson-McDermott EM, McGettrick AF, Goel G, et al. Succinate is an inflammatory signal that induces IL-1beta through HIF-1alpha. Nature. (2013) 496:238–42. doi: 10.1038/nature11986
215. Demon D, Vande Walle L, Lamkanfi M. Sensing the enemy within: how macrophages detect intracellular gram-negative bacteria. Trends Biochem Sci. (2014) 39:574–6. doi: 10.1016/j.tibs.2014.10.006
216. Bording-Jorgensen M, Alipour M, Danesh G, Wine E. Inflammasome activation by ATP enhances citrobacter rodentium clearance through ROS generation. Cell Physiol Biochem. (2017) 41:193–204. doi: 10.1159/000455988
217. Kolodziejczyk AA, Zheng D, Elinav E. Diet-microbiota interactions and personalized nutrition. Nat Rev Microbiol. (2019) 17:742–53. doi: 10.1038/s41579-019-0256-8
218. Levy M, Kolodziejczyk AA, Thaiss CA, Elinav E. Dysbiosis and the immune system. Nat Rev Immunol. (2017) 17:219–32. doi: 10.1038/nri.2017.7
Keywords: ulcerative colitis, Crohn disease, IBD–inflammatory bowel diseases, pediatric IBD, dietary fiber
Citation: Armstrong H, Mander I, Zhang Z, Armstrong D and Wine E (2021) Not All Fibers Are Born Equal; Variable Response to Dietary Fiber Subtypes in IBD. Front. Pediatr. 8:620189. doi: 10.3389/fped.2020.620189
Received: 22 October 2020; Accepted: 10 December 2020;
Published: 15 January 2021.
Edited by:
André Hörning, University Hospital Erlangen, GermanyReviewed by:
Andrew T Gewirtz, Georgia State University, United StatesCopyright © 2021 Armstrong, Mander, Zhang, Armstrong and Wine. This is an open-access article distributed under the terms of the Creative Commons Attribution License (CC BY). The use, distribution or reproduction in other forums is permitted, provided the original author(s) and the copyright owner(s) are credited and that the original publication in this journal is cited, in accordance with accepted academic practice. No use, distribution or reproduction is permitted which does not comply with these terms.
*Correspondence: Heather Armstrong, aGFybXN0cm9AdWFsYmVydGEuY2E=
Disclaimer: All claims expressed in this article are solely those of the authors and do not necessarily represent those of their affiliated organizations, or those of the publisher, the editors and the reviewers. Any product that may be evaluated in this article or claim that may be made by its manufacturer is not guaranteed or endorsed by the publisher.
Research integrity at Frontiers
Learn more about the work of our research integrity team to safeguard the quality of each article we publish.