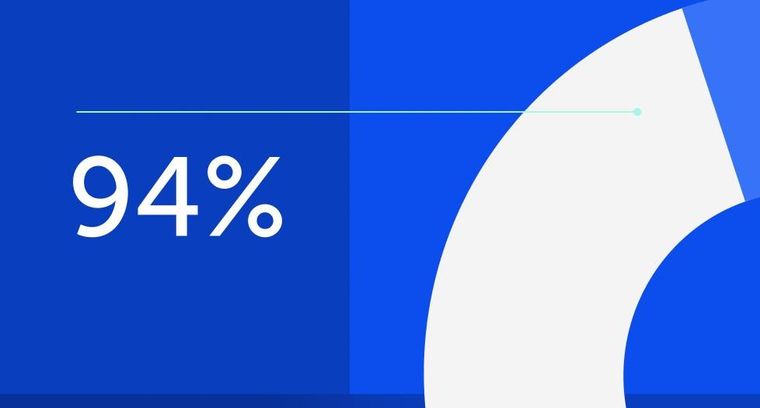
94% of researchers rate our articles as excellent or good
Learn more about the work of our research integrity team to safeguard the quality of each article we publish.
Find out more
ORIGINAL RESEARCH article
Front. Pediatr., 23 December 2020
Sec. Pediatric Urology
Volume 8 - 2020 | https://doi.org/10.3389/fped.2020.586287
Background and Objective: Mild hypospadias is a birth congenital condition characterized by the relocation of the male urethral meatus from its typical anatomical position near the tip of the glans penis, to a lower ventral position up to the brim of the glans corona, which can also be accompanied by foreskin ventral deficiency. For the most part, a limited number of cases have known etiology. We have followed a high-throughput proteomics approach to study the proteome in mild hypospadias patients.
Methods: Foreskin samples from patients with mild hypospadias were collected during urethroplasty, while control samples were collected during elective circumcision (n = 5/group). A high-throughput, quantitative proteomics approach based on multiplexed peptide stable isotope labeling (SIL) and liquid chromatography-tandem mass spectrometry (LC-MS/MS) analysis was used to ascertain protein abundance changes in hypospadias patients when compared to control samples.
Results: A total of 4,815 proteins were quantitated (2,522 with at least two unique peptides). One hundred and thirty-three proteins from patients with mild hypospadias showed significant abundance changes with respect to control samples, where 38 proteins were increased, and 95 proteins were decreased. Unbiased functional biological analysis revealed that both mitochondrial energy production and apoptotic signaling pathways were enriched in mild hypospadias.
Conclusions: This first comprehensive proteomics characterization of mild hypospadias shows molecular changes associated with essential cellular processes related to energy production and apoptosis. Further evaluation of the proteome may expand the search of novel candidates in the etiology of mild hypospadias and could also lead to the identification of biomarkers for this congenital urogenital condition.
Hypospadias is characterized by the localization of the urethral opening at any anatomical position on the ventral-medial side of the penis rather than near the tip of the glans. Its prevalence is ~21/10,000 live births (1–7). The most common type of this congenital urogenital condition is mild hypospadias (1st degree, anterior or Type I), comprising ~70% of cases, followed by moderate hypospadias (2nd degree, middle, or Type II) and severe hypospadias (3rd degree, posterior or Type III) with the remaining 30% (5, 8–11).
The formation of the male external genitalia is both a hormone-dependent and a hormone-independent multistep developmental event that spans embryonic and fetal periods. The androgen receptor (AR), activating transcription factor 3 (ATF3), estrogen receptor 1 and 2 (ESR1 and ESR2), steroid-5-alpha-reductase 2 (SRD5A2), hydroxysteroid (17-beta) dehydrogenase 3 (HSD17B3), hydroxy-delta-5-steroid dehydrogenase, 3 beta-and steroid delta isomerase 1 (HSD3B1), stAR-related lipid transfer domain containing 3 (STARD3), and mastermind like domain containing 1 (MAMLD1) are some of the genes that have been identified and associated with hypospadias (8, 12–25). In fact, molecular differences have been found at the gene, mRNA, and protein levels according to severity. Polymorphisms, mutations, and epigenetic changes in the AR, CYR61, HSD17B3, HSD3B1, MAMLD1, SRD5A2, and STARD3 have been associated to hypospadias risk in severe types of hypospadias that have not been found in mild hypospadias (26–30). Some examples of these include reduced AR gene methylation (31) and increased GGN or CAG repeat sequence of the AR gene [(32, 33); respectively]. Allele variations and single nucleotide polymorphisms (SNPs) of the SRD5A2 have also been associated with hypospadias risk (13, 27, 29, 34). Similarly, upregulation of ATF3 in preputial tissue from hypospadiac boys has been reported (30, 35–37). SNPs and haplotypes in ESR1 (17, 38, 39) and longer variants of (CA)n polymorphism in ESR2 (40) have been identified and associated with hypospadias. At the mRNA and protein level, significant changes as severity increases have been observed in AR, CYR61, ATF3, and CTGF, some of which are estrogen-responsive genes (30, 41). In terms of downstream products, we found lower concentrations of aspartate in moderate and severe hypospadias but not in mild hypospadias (42). This finding is of interest as aspartate modulates steroidogenesis in Leydig cells (43–45). In addition, it has been shown that aspartate increases testosterone, dihydrotestosterone (DHT), luteinizing hormone (LH), and gonadotropin-releasing hormone (GnRH) levels, as well as stAR mRNA and protein expression levels and AR protein expression levels (46–50).
Despite these associations with hormone-dependent pathways, the etiology of hypospadias remains elusive. In addition to steroidal signaling, the development of the male external genitalia involves cell migration, differentiation, and proliferation, patterning, and apoptosis during development, all of which demand high energy inputs. For instance, luminization of the glandular urethra is achieved through apoptosis in the solid epithelial cord at the tip of the glans (51). Therefore, we hypothesized that disruption of proteins with key cellular roles previously ascribed to embryonic and fetal development would be apparent in the proteome of mild hypospadias. We found that cellular energy and apoptotic processes are disrupted in mild forms of this congenital condition.
This study was approved by the Institutional Review Board (IRB) under the Human Research Subjects Protection Office (HRSPO) at the University of Puerto Rico, Medical Sciences Campus. Parents of children undergoing urethroplasty and boys scheduled for elective circumcision were recruited at the HIMA San Pablo Hospital, Caguas, Puerto Rico. Hypospadias was assessed by Marcos Raymond Pérez-Brayfield, MD (pediatric urologist, Faculty in Surgery, Urology Section, School of Medicine, UPR). Informed consent was obtained from legal parents or guardians. Foreskin samples were collected from boys with non-comorbid mild hypospadias (also known as 1st degree, anterior or Type I hypospadias: n = 5) and elective circumcision (n = 5) between the ages of 5–28 months of age.
Mild hypospadias cases were confirmed during urethroplasty and included two glandular, one coronal, and two subcoronal cases after penile degloving and chordee release whenever present. Table 1 shows the age of the patient and the location of the urethral meatus. Given that this study did not control for the age of the patient at the time of the urethroplasty procedure for mild hypospadias cases, the age distribution in this patient cohort was not normally distributed. Mann-Whitney test revealed a significant age difference between experimental groups (p = 0.02; see Discussion for further details). Samples were obtained from both lateral and dorsal inner foreskin tissue, which is mostly comprised of mucous membrane. Samples with suggestive clinical changes of balanitis xerotica obliterans (BXO) were excluded from the study.
One hundred milligrams of wet foreskin tissue were weighed and homogenized with a tissue tearor in 1.0 mL of Lysis Buffer (50 mM Tris, 10 mM DTT, and 1% SDS pH 6.8). Samples were incubated for 5 min at 97°C with shaking (600 rpm, Eppendorf Thermomixer), and thereafter incubated overnight in rotator at 4°C. Homogenates were centrifuged for 5 min at 13,000 rpm, and the supernatant was collected. Protein concentration in the protein extracts was measured using the RC DC Protein Assay Kit II (Bio-Rad, USA) following the manufacturer's instructions.
Protein extracts (100 μg) were concentrated on 30 K Filter-Aided Sample Preparation (FASP) filters (Expedeon). After washing with denaturing buffer (8 M urea in 100 mM Tris-HCl pH 8.5) at 10,000 rpm for 15 min, free thiol groups were alkylated by incubation with 50 mM iodoacetamide 30 min at room temperature in the dark. Then the filters were washed with denaturing buffer followed by washing with trypsin digestion buffer (50 mM ammonium bicarbonate pH 8.8). Protein samples were digested overnight at 37°C with sequencing grade trypsin (Promega, Madison, WI, USA) at 1:40 (w/w) trypsin: protein ratio in digestion buffer. The resulting tryptic peptides from each sample were recovered by centrifugation at 10,000 rpm for 5 min after the addition of 40 μl of trypsin digestion buffer, after which 50 μl of 500 mM NaCl was added and the filters centrifuged for 15 min at 10,000 rpm. Trifluoroacetic acid was added to a final concentration of 1%, and the peptides were desalted on C18 Oasis HLB extraction cartridges (Waters Corporation, Milford, MA, USA) and dried-down. Peptide labeling was performed using a 10-plex TMT kit (Tandem Mass Tag, Thermo Scientific, USA) according to the manufacturer's instructions, and desalted onto Oasis C18 cartridges before analysis by liquid chromatography-tandem mass spectrometry (LC-MS/MS).
LC-MS/MS was performed using a nano-HPLC Easy nLC 1,000 chromatograph coupled to a Q-Exactive hybrid quadrupole orbitrap mass spectrometer (Thermo Scientific). Peptides were loaded onto a C18 RP nano-precolumn (75 μ m I.D. and 2 cm, Acclaim PepMap 100, Thermo Scientific), and separated on an analytical C18 nano-column (75 μ m I.D. and 50 cm, Acclaim PepMap 100) using a continuous gradient consisting of 8–30% B for 60 min and 30–90% B for 2 min (B = 90% ACN, 0.1% formic acid) at 200 nL/min. The chromatographic run acquired an FT-resolution spectrum (140,000 resolution) in the 390-1,500 m/z range, followed by data-dependent MS/MS spectra of the 15 most intense parent ions. The normalized HCD collision energy was set to 30%, and the parent ion mass isolation width was 1.5 Da.
Peptides were identified from MS/MS spectra using the SEQUEST HT algorithm integrated with Proteome Discoverer 2.1 (Thermo Scientific). MS/MS scans were matched against a human protein database (UniProt 2017_12_19 Release). Parameters were selected as follows: two trypsin missed cleavages maximum, 800 ppm precursor mass tolerance and 0.02 Da fragment mass tolerance. Cys carbamidomethylation, N-terminal TMT labeling, and Lys TMT labeling were chosen as fixed modifications, while Met oxidation was considered a dynamic modification. For peptide false discovery rate (FDR) assessment using the refined method (52, 53), MS/MS spectra were searched against the corresponding inverted human database.
Quantitative information was extracted from the intensity of the TMT reporter ions in MS/MS spectra. Protein abundance changes were assessed based on the Weighted Scan-Peptide-Protein (WSPP) statistical model (54) under the SanXoT software package (55). This model provides the standardized variable Zq, which is defined as the mean-corrected log2-ratio expressed in units of standard deviation at the protein level. Thus, Zq values were calculated for each sample, both mild hypospadias, and control samples, with respect to a weighted average of control samples. The study design is depicted in Figure 1.
Figure 1. Proteomics study design. Foreskin samples were collected during scheduled urethroplasty from patients with mild hypospadias (n = 5) or from individuals submitted to a circumcision procedure (control group, n = 5). One-hundred milligrams of foreskin samples from each patient were homogenized and proteins extracted from tissue. A total of 100 μg of protein was processed per sample. Protein extracts were digested with trypsin using the FASP technology. Subsequently, peptide samples were labeled using a 10-plex TMT kit. Each sample was labeled with a specific TMT mass tag. After labeling, samples were pooled and separated into five fractions using high pH reversed-phase peptide fractionation. Peptides were resuspended in 0.1% formic acid and analyzed by LC-MS/MS. Upon fragmentation, the specific reporter ion released from each of the 10 mass tags shows in the low-mass region of the MS/MS spectrum. The intensity of this reporter ion was used to measure relative peptide abundance levels among samples, from which relative protein abundance between control and mild hypospadias cases was inferred (see text for details).
Statistical comparison between hypospadias and control group Zq values was performed by Student's t-test. A p-value < 0.05 was considered significant. The MetaboAnalyst (56) online platform was used with Zq values to perform multivariate statistical analyses, including principal component analysis (PCA) and partial least squares-discriminant analysis (PLS-DA).
The STRING database (http://string-db.org), version 11.0 (57), was used for functional enrichment analysis with proteins found significantly decreased (95 proteins) or increased (38 proteins) in the hypospadias group. A low protein-protein interaction (PPI) enrichment p-value indicates that the nodes are not random and that the observed number of edges is significant. A 5% FDR cutoff was used to evaluate significant functional category enrichment. Supplementary Table 1 details accession numbers, gene, and protein names for these proteins.
Cytochrome C was detected and quantified by enzyme-linked immunosorbent assay (ELISA; n = 10 controls and n = 8 mild hypospadias). Test results were submitted to the Mann-Whitney U-test statistical analysis. Human Cytochrome C Platinum ELISA (Thermo Fisher Scientific, Waltham, MA) was used for quantitative detection following the protocol provided by the manufacturer.
A multiplexed, quantitative proteomics approach based on 10-plex TMT labeling and LC-MS/MS analysis was employed to identify and quantify proteins of foreskin tissue from mild hypospadias and control samples (n = 5/group). A total of 2,522 proteins were identified and quantified with more than one unique peptide. Multivariate analyses revealed differences between the proteome of mild hypospadias samples when compared to control samples. Principal component analysis showed that control samples displayed a wide distribution, whereas samples from mild hypospadias patients were similar amongst each other. Components were explained by principal component (PC) 1 with a 29.2% variance and PC 2 with a 18.1% variance. Figure 2A depicts PC1 and PC2 components with 95% confidence ellipses for control and mild hypospadias samples. Furthermore, PLS-DA showed a marked separation between control and mild hypospadias groups. Partial least squares (PLS) 1 contributed 17.5%, whereas PLS2 carried 21.9% of the total variance. Figure 2B shows the separation between control and mild hypospadias groups with 95% confidence ellipses. One hundred thirty-three proteins were identified with significant changes in protein abundance in mild hypospadias samples in comparison to control samples at 1% FDR (available at PeptideAtlas repository). Specifically, 95 proteins were found decreased (Figure 3), and 38 proteins were increased (Figure 4) in mild hypospadias when compared to control samples. Supplementary Table 1 details accession numbers, gene, and protein names for these proteins.
Figure 2. Mild hypospadias protein profile differs from controls. Zq values from a total of 2,522 proteins identified with two or more peptides were subjected to PCA and PLS-DA. (A) Score plot for PC1 (29.2% variance explained) vs. PC2 (18.1% variance explained). (B) Score plot for PLS1 (17.5% variance explained) vs. PLS2 (21.9% variance explained). The ellipses illustrate 95% confidence region for these groups. n = 5/group.
Figure 3. Proteins found downregulated in the proteome from mild hypospadias patients. Ninety-five proteins were found decreased between control and mild hypospadias samples. Heatmaps depict protein abundance changes in terms of Zq across samples (C1-C5 for control and H1-H5 for mild hypospadias samples). The magnitude of abundance change [increased (red) or decreased (blue)] is shown according to the color scale on the right.
Figure 4. Proteins found upregulated in the proteome from mild hypospadias patients. Thirty-eight proteins were found increased between control and mild hypospadias samples. Heatmaps depict protein abundance changes in terms of Zq across samples (C1-C5 for control and H1-H5 for mild hypospadias samples). The magnitude of abundance change [increased (red) or decreased (blue)] is shown according to the color scale on the right.
We performed an unbiased functional enrichment analysis for proteins showing significantly decreased or increased abundances using STRING network analysis. Interacting proteins were found in both sets of proteins with a PPI enrichment p-value < 1.0e-16. For proteins with decreased levels, 257 Gene Ontology (GO) terms were found significantly enriched (Supplementary Table 2). For proteins with increased levels, 79 GO terms were found significantly enriched (Supplementary Table 3). Within the identified biological processes that were enriched, we observed pathways involving cellular energy processes and apoptotic signaling (Table 2).
Table 2. Enriched functional categories involving cellular energy processes and apoptotic signaling pathways.
CYCS is an essential protein in the mitochondrial electron transport chain that also plays key roles in apoptosis through the caspase-dependent apoptosis pathway. Given that biological processes related to cellular energy and apoptotic processes were found significantly enriched in this study, CYCS was selected for validation with ELISA. Significant differences between control and mild hypospadias samples were found (p = 0.0067). Specifically, CYCS was found in lower concentrations (ng/ml) in mild hypospadias (median = 8.86) when compared to control samples (median = 12.56; Figure 5).
Figure 5. Validation of cytochrome C by ELISA. Cytochrome C was found in significantly lower concentrations in mild hypospadias samples (median = 8.86 ng/ml) in comparison to controls (median = 12.56 ng/ml) samples in agreement with proteomics results. Dashed line represents the median value. Dotted lines represent quartiles, top: 75% percentile and bottom: 25% percentile. ** = p < 0.05. Controls, n = 10; Mild hypospadias, n = 8.
To the best of our knowledge, this is the first study that has evaluated the proteome in mild hypospadias, which is the most common type of this congenital urogenital condition accounting for nearly 70% of cases. We have analyzed human foreskin samples by using a multiplexed, quantitative proteomics approach based on SIL and LC-MS/MS that has allowed the identification of 2,522 proteins which were identified with two or more unique peptides. Significant differences in protein abundances were identified for 133 proteins. Specifically, 95 proteins were found with significantly decreased protein abundance and 38 with significantly increased protein abundance in mild hypospadias when compared to control samples. It is of interest that unbiased functional enrichment analysis revealed mitochondrial energy production and apoptotic signaling pathways as significantly enriched processes.
Mitochondrial dysfunction has been linked to congenital conditions such as spina bifida, cardiomyopathy, and inborn errors of metabolism (58–60). Our enrichment data, from known and predicted protein-protein interactions, revealed interactions between ADH1A, ADH1B, ATP5J, CYCS, DERA, FH, GPD2, HK1, NDUFAB1, NDUFA6, NNT, OGDH, PGK1, and SUCLA2 for generation of precursor metabolites and energy; CYCS, FH, GPD2, NDUFAB1, NDUFA6, NNT, OGDH, and SUCLA2 for cellular respiration; CYCS, GPD2, NDUFA6, and NDUFAB1 for respiratory electron transport chain; ATP1B1, ATP5J, CYCS, HK1, NDUFAB1, NDUFA6, OGDH, and PGK1 for ATP metabolic process; FH, NNT, OGDH, and SUCLA2 for tricarboxylic acid cycle; ATP5J, HK1, OGDH, and PGK1 for ATP biosynthetic process; HK1, OGDH, and PGK1 for glycolytic process; and GOT2, GPD2, and PGK1 for gluconeogenesis, among other which indirectly contribute to cellular energy processes such as glucose metabolic process, fatty acid metabolic process, and fumarate metabolic process.
Embryonic development demands high energy inputs to carry out essential processes such as cellular differentiation, cell migration, proliferation, and patterning processes. Early during the embryonic period, the major source of energy is glycolysis. As the embryo develops, cells produce energy from the three major processes of energy production in eukaryotic cells: glycolysis, Krebs cycle, and the electron transport chain (61). Thus, it is not surprising that the dysregulation of proteins involved in the production of cellular energy demands has been linked to congenital conditions, as shown here. For instance, PGK, NNT, SUCLA2, and FH have been linked to inherited disorders including, but not limited to, infantile encephalopathy, X-linked inherited disorders, myopathies, and neural tube defects (62–66). Programmed cell death is also an energy-dependent process, and, as reported here, the intrinsic apoptotic signaling pathway (67, 68) was disrupted in mild hypospadias samples. Taken together, our results may present a new molecular research strategy to study mild hypospadias, although further research on the involvement of mitochondrial dysfunction in this congenital condition may provide promising venues for future scientific inquiry.
Furthermore, apoptosis plays a key role during the development of the male genitalia (51, 69, 70). Previous studies have proposed that the suppression of apoptotic-related genes can give rise to hypospadias (71, 72). For instance, reduced apoptosis is observed in finasteride-induced hypospadiac rats through the MAPK/ERK signaling pathway (73). In addition, as confirmed by the presence of apoptotic cells, the luminization of the urethra in human embryo specimens is accomplished by apoptosis (51).
Of note, in this study, CYCS was found significantly downregulated in mild hypospadias samples. CYCS is an inner mitochondrial protein with key biological roles in mitochondrial electron transport and apoptosis. Cytochrome C assumes an apoptotic role when released into the cytosol. Suppression or activation of anti- and pro-apoptotic members of the Bcl-2 family leads to altered mitochondrial membrane permeability resulting in the release of CYCS into the cytosol. Once in the cytosol, CYCS binds to Apaf-1, which triggers the activation of caspase-9, which in turn accelerates apoptosis by the activation of other caspases (74). Given the major role of CYCS as a modulator of apoptosis, we analyzed additional samples by ELISA, which revealed a significant downregulation of CYCS in mild hypospadias as compared to control samples. It remains as a challenge for future experiments to determine the time course of such downregulation across development.
AIFM1, which has also been found downregulated in the hypospadias group, is a pro-apoptotic factor in the caspase-independent pathway, which, in response to apoptotic stimuli, is released from the mitochondrial intermembrane space into the cytosol and to the nucleus (74). Mutations of AIF can lead to severe mitochondrial dysfunction and have been associated with several disorders and neuropathogenesis (75–77). CUL3 and CUL4A, which were also downregulated, are part of the Cullin gene family, the largest ubiquitin ligase family in mammals, which are part of the core component of multiple cullin-RING-based E3 ubiquitin-protein ligase complexes that mediate the ubiquitination of target proteins and subsequent proteasomal degradation. These proteins participate in the extrinsic apoptotic pathway activated by the tumor necrosis factor (TNF) receptor family through the ubiquitination of caspase-8 (78–80). CUL4A-mediated protein degradation has been reported as essential for the maturation of primary spermatocytes in mouse testis (81). Taken together, the observed downregulation of AIFM1, CUL3, CUL4A, and CYCS in this study warrants further research.
There are a number of limitations to this study. As the first study of the proteome in hypospadias, it is necessary to replicate the results presented here with a larger patient cohort. Owing to the difficulties inherent to obtaining patient tissue samples, the high cost of proteomics analysis, and the limited accession to instruments, many clinical proteomics studies are based on relatively small patient cohorts [see, for example, (82–84)]. It is important to validate clinical proteomics results with larger patient cohorts and by using orthogonal techniques like ELISA and Western blot. The validation of Cytochrome C presented here is a first step in this direction. It must be noted, however, that in this study, the limited number of samples is compensated by the application of WSPP as a rigorous statistical model. Second, although the current state of knowledge on the proximal growth of the preputial lamina over the glans penis is most-likely an androgen-dependent effect that is completed during intrauterine development (85), this study cannot address whether the so-called “mini-puberty” in males (86, 87) contributes further to cytoarchitectural changes of foreskin samples so that some of the changes in protein levels observed here could be due, at least in part, to postnatal androgen-dependent effects in foreskin tissue up to 12 months of age. Important to note is that available evidence suggests that human penile growth is androgen-independent from ~12 months of age up to puberty (87). Given the age range of our patient cohort, we cannot address this question. Nevertheless, in humans, the effects of postnatal cytoarchitectural changes on foreskin protein expression will most likely remain as a vexata quaestio as it is unethical to time urethroplasty procedures to address this experimental conundrum. Related to this, the third limitation of this study is the significant age difference between experimental groups. Although it has been noted that histological and morphological changes occur in foreskin tissue during postnatal development (88), such as changes in vasculature, connective tissue, and nervous tissue, for example, we are of the opinion that it is unlikely that such structural changes during development heavily rely on the distinct cellular energy and apoptotic pathways reported here. The fourth limitation of this study is related to the histological origin of foreskin samples, which were mostly comprised of lateral and dorsal foreskin primarily due to the ventral deficiency that characterizes hypospadias. This study cannot rule out the possibility of under-or over-estimating the levels of protein expression because it is not possible to sample the circumferential prepuce in hypospadias. Of interest, the formation of the circumferential prepuce during intrauterine development is an androgen-independent event (89). In fact, future studies on the dependency of androgen signaling for the changes in protein expression levels observed here may provide valuable insights on the timing of embryological events that are affected in hypospadias.
The results reported in this study rely on a multi-step data analysis approach within a carefully designed statistical framework that allows for the identification of proteins, their relative abundances, and the quantitative analysis of whether such abundances significantly differ among experimental groups. It is important to underscore that pathways and protein-protein interactions reported here rely on existing protein databases. These are “predicted” pathways and interactions based on published data from a myriad of biological systems studied with a staggering diversity of experimental approaches. The ones discussed here are based on our own interpretation or previously linked to hypospadias or other congenital conditions.
In conclusion, this first line of proteomics assessment shows a differential proteome profile for mild hypospadias. Proteins related to cellular energy production and apoptotic pathways stand out in this proteomics signature. These pathways may facilitate the search for novel candidates in the etiology of mild hypospadias and may also constitute potential biomarkers for early detection of this congenital urogenital condition.
The datasets generated in this study can be found in online repositories. The names of the repository/repositories and accession number(s) can be found below: http://www.peptideatlas.org/PASS/PASS01602.
The studies involving human participants were reviewed and approved by Human Research Subjects Protection Office (HRSPO) at the University of Puerto Rico, Medical Sciences Campus. Written informed consent to participate in this study was provided by the participants' legal guardian/next of kin.
The study was designed by CP-R, MP-B, and JJ. MP-B performed all surgical procedures and collected all tissue samples. CP-R assisted in sample collection and conducted sample preparation for proteomics experiments under the guidance and expert advice of HS and conducted validation experiments. IJ, EC, RM, and JV developed statistical algorithms and submitted collected samples to proteomic analyses. EM-V assisted in sample preparation and performed statistical analysis for validation experiments. Final analyses, the depiction of data, and writing of the first draft were by CP-R and JJ. All authors participated in the drafting and approval of the final version of the manuscript.
This research was supported by the Research Initiative for Scientific Enhancement (MBRS-RISE) Program Award Number R25GM061838, by the National Institute on Minority Health and Health Disparities of the National Institutes of Health Award Number 8G12MD007600, by the Hispanic Alliance for Clinical and Translational Research of the National Institues of Health under award number U54GM133807-01A1, and by the Comprehensive Cancer Center of the UPR (a public corporation of the Government of Puerto Rico created in virtue of Law 230 of August 26, 2004, as amended). This study was also supported by competitive grants from the Spanish Ministry of Science, Innovation, and Universities (PGC2018-097019-B-I00), and the Carlos III Institute of Health-Fondo de Investigación Sanitaria grant PRB3 (IPT17/0019—ISCIII-SGEFI / ERDF, ProteoRed). The CNIC is supported by the Instituto de Salud Carlos III (ISCIII), the Ministerio de Ciencia, Innovación y Universidades (MCNU), and the Pro CNIC Foundation, and is a Severo Ochoa Center of Excellence (SEV-2015-0505). The content is entirely the responsibility of the authors and does not necessarily represent the official views of the NIH nor of the Comprehensive Cancer Center UPR. Infrastructure support was provided in part by the National Institute on Minority Health and Health Disparities RCMI Grant: U54MD007600 and the Comprehensive Cancer Center—Clinical Proteomics Laboratory and RCMI Proteomics CORE in San Juan, Puerto Rico, for research facilities and technical support.
The authors declare that the research was conducted in the absence of any commercial or financial relationships that could be construed as a potential conflict of interest.
We acknowledge the staff administration and the nursing and surgery teams of Hospital HIMA San Pablo in Caguas, Puerto Rico, for the opportunity to collect human tissue samples used in this study.
The Supplementary Material for this article can be found online at: https://www.frontiersin.org/articles/10.3389/fped.2020.586287/full#supplementary-material
1. Baskin LS. Hypospadias and genital development. In: Advances in Experimental Medicine and Biology. New York, NY: Kluwer Academic/Plenum Publishers (2004).
2. Bergman JE, Loane M, Vrijheid M, Pierini A, Nijman RJ, Addor MC, et al. Epidemiology of hypospadias in Europe: a registry-based study. World J Urol. (2015) 33:2159–67. doi: 10.1007/s00345-015-1507-6
3. Loane M, Dolk H, Kelly A, Teljeur C, Greenlees R, Densem J. Paper 4: EUROCAT statistical monitoring: identification and investigation of ten year trends of congenital anomalies in Europe. Birth Defects Res Part A Clin Mol Teratol. (2011) 91:S31–43. doi: 10.1002/bdra.20778
4. Mai CT, Isenburg J, Langlois PH, Alverson CJ, Gilboa SM, Rickard R, et al. Population-based birth defects data in the United States, 2008 to 2012: Presentation of state-specific data and descriptive brief on variability of prevalence. Birth Defects Res Part A Clin Mol Teratol. (2015) 103:972–93. doi: 10.1002/bdra.23461
5. Paulozzi LJ, Erickson JD, Jackson RJ. Hypospadias trends in two US surveillance systems. Pediatrics. (1997) 100:831–4. doi: 10.1542/peds.100.5.831
6. Toppari J, Kaleva M, Virtanen HE. Trends in the incidence of cryptorchidism and hypospadias, and methodological limitations of registry-based data. Hum Reprod Update. (2001) 7:282–6. doi: 10.1093/humupd/7.3.282
7. Yu X, Nassar N, Mastroiacovo P, Canfield M, Groisman B, Bermejo-Sánchez E, et al. Hypospadias prevalence and trends in international birth defect surveillance systems, 1980–2010. Eur Urol. (2019) 76:482–90. doi: 10.1016/j.eururo.2019.06.027
8. Baskin LS, Ebbers MB. Hypospadias: anatomy, etiology, and technique. J Pediatr Surg. (2006) 41:463–72. doi: 10.1016/j.jpedsurg.2005.11.059
9. Carmichael SL, Shaw GM, Nelson V, Selvin S, Torfs CP, Curry CJ. Hypospadias in California: trends and descriptive epidemiology. Epidemiology. (2003) 14:701–6. doi: 10.1097/01.ede.0000091603.43531.d0
10. Dolk H, Vrijheid M, Scott JE, Addor MC, Botting B, de Vigan C, et al. Toward the effective surveillance of hypospadias. Environ Health Perspect. (2004) 112:398–402. doi: 10.1289/ehp.6398
11. Nassar N, Bower C, Barker A. Increasing prevalence of hypospadias in Western Australia, 1980-2000. Arch Dis Child. (2007) 92:580–4. doi: 10.1136/adc.2006.112862
12. Beleza-Meireles A, Töhönen V, Söderhäll C, Schwentner C, Radmayr C, Kockum I, et al. Activating transcription factor 3: a hormone responsive gene in the etiology of hypospadias. Eur J Endocrinol. (2008) 158:729–39. doi: 10.1530/EJE-07-0793
13. Carmichael SL, Witte JS, Ma C, Lammer EJ, Shaw GM. Hypospadias and variants in genes related to sex hormone biosynthesis and metabolism. Andrology. (2014) 2:130–7. doi: 10.1111/j.2047-2927.2013.00165.x
14. Chen T, Li Q, Xu J, Ding K, Wang Y, Wang W, et al. Mutation screening of BMP4, BMP7, HOXA4 and HOXB6 genes in Chinese patients with hypospadias. Eur J Hum Genet. (2007) 15:23–8. doi: 10.1038/sj.ejhg.5201722
15. Bouty A, Ayers KL, Pask A, Heloury Y, Sinclair AH. The genetic and environmental factors underlying hypospadias. Sex Dev. (2015) 9:239–59. doi: 10.1159/000441988
16. Haraguchi R, Mo R, Hui C, Motoyama J, Makino S, Shiroishi T, et al. Unique functions of Sonic hedgehog signaling during external genitalia development. Development. (2001) 128:4241–50.
17. Choudhry S, Baskin LS, Lammer EJ, Witte JS, Dasgupta S, Ma C, et al. Genetic polymorphisms in ESR1 and ESR2 genes, and risk of hypospadias in a multiethnic study population. J Urol. (2015) 193:1625–31. doi: 10.1016/j.juro.2014.11.087
18. Geller F, Feenstra B, Carstensen L, Pers TH, van Rooij IA, Körberg IB, et al. Genome-wide association analyses identify variants in developmental genes associated with hypospadias. Nat Genet. (2014) 46:957–63. doi: 10.1038/ng.3063
19. Kalfa N, Philibert P, Sultan C. Is hypospadias a genetic, endocrine or environmental disease, or still an unexplained malformation? Int J Androl. (2008) 32:187–97. doi: 10.1111/j.1365-2605.2008.00899.x
20. Köhler B, Delezoide AL, Boizet-Bonhoure B, McPhaul MJ, Sultan C, Lumbroso S. Coexpression of Wilms' tumor suppressor 1 (WT1) and androgen receptor (AR) in the genital tract of human male embryos and regulation of AR promoter activity by WT1. J Mol Endocrinol. (2007) 38:547–54. doi: 10.1677/JME-06-0020
21. Morgan EA, Nguyen SB, Scott V, Stadler HS. Loss of Bmp7 and Fgf8 signaling in Hoxa13-mutant mice causes hypospadia. Development. (2003) 130:3095–109. doi: 10.1242/dev.00530
22. Perriton CL, Powles N, Chiang C, Maconochie MK, Cohn MJ. Sonic hedgehog signaling from the urethral epithelium controls external genital development. Dev Biol. (2002) 247:26–46. doi: 10.1006/dbio.2002.0668
23. Singh N, Gupta DK, Sharma S, Sahu DK, Mishra A, Yadav DK, et al. Single-nucleotide and copy-number variance related to severity of hypospadias. Pediatr Surg Int. (2018) 34:991–1008. doi: 10.1007/s00383-018-4330-5
24. Suzuki H, Matsushita S, Suzuki K, Yamada G. 5α-Dihydrotestosterone negatively regulates cell proliferation of the periurethral ventral mesenchyme during urethral tube formation in the murine male genital tubercle. Andrology. (2017) 5:146–52. doi: 10.1111/andr.12241
25. Utsch B, Albers N, Ludwig M. Genetic and molecular aspects of hypospadias. Eur J Pediatr Surg. (2004) 14:297–302. doi: 10.1055/s-2004-821275
26. Chen Y, Thai HT, Lundin J, Lagerstedt-Robinson K, Zhao S, Markljung E, et al. Mutational study of the MAMLD1-gene in hypospadias. Eur J Med Genet. (2010) 53:122–6. doi: 10.1016/j.ejmg.2010.03.005
27. Sata F, Kurahashi N, Ban S, Moriya K, Tanaka KD, Ishizuka M, et al. Genetic polymorphisms of 17 β-hydroxysteroid dehydrogenase 3 and the risk of hypospadias. J Sex Med. (2010) 7:2729–38. doi: 10.1111/j.1743-6109.2009.01641.x
28. van der Zanden LF, van Rooij IA, Feitz WF, Franke B, Knoers NV, Roeleveld N. Aetiology of hypospadias: a systematic review of genes and environment. Hum Reprod Update. (2012) 18:260–83. doi: 10.1093/humupd/dms002
29. Wang Y, Li Q, Xu J, Liu Q, Wang W, Lin Y, et al. Mutation analysis of five candidate genes in Chinese patients with hypospadias. Eur J Hum Genet. (2004) 12:706–12. doi: 10.1038/sj.ejhg.5201232
30. Wang Z, Liu BC, Lin GT, Lin CS, Lue TF, Willingham E, et al. Up-regulation of estrogen responsive genes in hypospadias: microarray analysis. J Urol. (2007) 177:1939–46. doi: 10.1016/j.juro.2007.01.014
31. Vottero A, Minari R, Viani I, Tassi F, Bonatti F, Neri TM, et al. Evidence for epigenetic abnormalities of the androgen receptor gene in foreskin from children with hypospadias. J Clin Endocrinol Metab. (2011) 96:E1953–62. doi: 10.1210/jc.2011-0511
32. Aschim EL, Nordenskjöld A, Giwercman A, Lundin KB, Ruhayel Y, Haugen TB, et al. Linkage between cryptorchidism, hypospadias, and GGN repeat length in the androgen receptor gene. J Clin Endocrinol Metab. (2004) 89:5105–9. doi: 10.1210/jc.2004-0293
33. Adamovic T, Nordenskjöld A. The CAG repeat polymorphism in the androgen receptor gene modifies the risk for hypospadias in Caucasians. BMC Med Genet. (2012) 13:109. doi: 10.1186/1471-2350-13-109
34. Samtani R, Bajpai M, Vashisht K, Ghosh PK, Saraswathy KN. Hypospadias risk and polymorphism in SRD5A2 and CYP17 genes: case-control study among Indian children. J Urol. (2011) 185:2334–9. doi: 10.1016/j.juro.2011.02.043
35. Gurbuz C, Demir S, Zemheri E, Canat L, Kilic M, Caskurlu T. Is activating transcription factor 3 upregulated in patients with hypospadias? Korean J Urol. (2010) 51:561–4. doi: 10.4111/kju.2010.51.8.561
36. Karabulut R, Turkyilmaz Z, Sonmez K, Kumas G, Ergun S, Ergun M, et al. Twenty-four genes are upregulated in patients with hypospadias. Balkan J Med Genet. (2013) 16:39–44. doi: 10.2478/bjmg-2013-0030
37. Liu B, Wang Z, Lin G, Agras K, Ebbers M, Willingham E, et al. Activating transcription factor 3 is upregulated in patients with hypospadias. Pediatr Res. (2005) 58:1280–3. doi: 10.1203/01.pdr.0000187796.28007.2d
38. Ban S, Sata F, Kurahashi N, Kasai S, Moriya K, Kakizaki H, et al. Genetic polymorphisms of ESR1 and ESR2 that may influence estrogen activity and the risk of hypospadias. Hum Reprod. (2008) 23:1466–71. doi: 10.1093/humrep/den098
39. Watanabe M, Yoshida R, Ueoka K, Aoki K, Sasagawa I, Hasegawa T, et al. Haplotype analysis of the estrogen receptor 1 gene in male genital and reproductive abnormalities. Hum Reprod. (2007) 22:1279–84. doi: 10.1093/humrep/del513
40. Beleza-Meireles A, Barbaro M, Wedell A, Töhönen V, Nordenskjöld A. Studies of a co-chaperone of the androgen receptor, FKBP52, as candidate for hypospadias. Reprod Biol Endocrinol. (2007) 5:8. doi: 10.1186/1477-7827-5-8
41. Qiao L, Tasian GE, Zhang H, Cao M, Ferretti M, Cunha GR, et al. Androgen receptor is overexpressed in boys with severe hypospadias, and ZEB1 regulates androgen receptor expression in human foreskin cells. Pediatr Res. (2012) 71:393–8. doi: 10.1038/pr.2011.49
42. Piñeyro-Ruiz C, Chorna NE, Pérez-Brayfield MR, Jorge JC. Severity-dependent profile of the metabolome in hypospadias. Front Pediatr. (2020) 8:202. doi: 10.3389/fped.2020.00202
43. Di Fiore MM, Santillo A, Chieffi Baccari G. Current knowledge of D-aspartate in glandular tissues. Amino Acids. (2014) 46:1805–18. doi: 10.1007/s00726-014-1759-2
44. Di Fiore MM, Santillo A, Falvo S, Longobardi S, Chieffi Baccari G. Molecular mechanisms elicited by d-aspartate in leydig cells and spermatogonia. Int J Mol Sci. (2016) 17:1127. doi: 10.3390/ijms17071127
45. Di Fiore MM, Boni R, Santillo A, Falvo S, Gallo A, Esposito S, et al. D-aspartic acid in vertebrate reproduction: animal models and experimental designs. Biomolecules. (2019) 9:445. doi: 10.3390/biom9090445
46. D'Aniello G, Tolino A, D'Aniello A, Errico F, Fisher GH, Di Fiore MM. The role of D-aspartic acid and N-methyl-D-aspartic acid in the regulation of prolactin release. Endocrinology. (2000) 141:3862–70. doi: 10.1210/endo.141.10.7706
47. D'Aniello G, Grieco N, Di Filippo MA, Cappiello F, Topo E, D'Aniello E, et al. Reproductive implication of D-aspartic acid in human pre-ovulatory follicular fluid. Hum Reprod. (2007) 22(12), 3178-3183. doi: 10.1093/humrep/dem328
48. Nagata Y, Homma H, Lee JA, Imai K. D-Aspartate stimulation of testosterone synthesis in rat Leydig cells. FEBS Lett. (1999) 444:160–4. doi: 10.1016/S0014-5793(99)00045-9
49. Nagata Y, Homma H, Matsumoto M, Imai K. Stimulation of steroidogenic acute regulatory protein (StAR) gene expression by D-aspartate in rat Leydig cells. FEBS Lett. (1999) 454:317–20. doi: 10.1016/S0014-5793(99)00840-6
50. Santillo A, Falvo S, Chieffi P, Burrone L, Chieffi Baccari G, Longobardi S, et al. D-aspartate affects NMDA receptor-extracellular signal-regulated kinase pathway and upregulates androgen receptor expression in the rat testis. Theriogenology. (2014) 81:744–51. doi: 10.1016/j.theriogenology.2013.12.009
51. van der Werff JF, Nievelstein RA, Brands E, Luijsterburg AJ, Vermeij-Keers C. Normal development of the male anterior urethra. Teratology. (2000) 61:172–83. doi: 10.1002/(SICI)1096-9926(200003)61:3<172::AID-TERA4>3.0.CO;2-B
52. Martínez-Bartolomé S, Navarro P, Martín-Maroto F, López-Ferrer D, Ramos-Fernández A, Villar M, et al. Properties of average score distributions of SEQUEST: the probability ratio method. Mol Cell Proteomics. (2008) 7:1135–45. doi: 10.1074/mcp.M700239-MCP200
53. Navarro P, Vázquez J. A refined method to calculate false discovery rates for peptide identification using decoy databases. J Proteome Res. (2009) 8:1792–6. doi: 10.1021/pr800362h
54. Navarro P, Trevisan-Herraz M, Bonzon-Kulichenko E, Núñez E, Martínez-Acedo P, Pérez-Hernández D, et al. General statistical framework for quantitative proteomics by stable isotope labeling. J Proteome Res. (2014) 13:1234–47. doi: 10.1021/pr4006958
55. Trevisan-Herraz M, Bagwan N, García-Marqués F, Rodriguez JM, Jorge I, Ezkurdia I, et al. SanXoT: a modular and versatile package for the quantitative analysis of high-throughput proteomics experiments. Bioinformatics. (2019) 35:1594–6. doi: 10.1093/bioinformatics/bty815
56. MetaboAnalyst—Statistical. Functional and Integrative Analysis of Metabolomics Data. Available online at: www.metaboanalyst.ca/ (accessed June 23, 2020).
57. Szklarczyk D, Gable AL, Lyon D, Junge A, Wyder S, Huerta-Cepas J, et al. STRING v11: protein-protein association networks with increased coverage, supporting functional discovery in genome-wide experimental datasets. Nucleic Acids Res. (2019) 47:D607–13. doi: 10.1093/nar/gky1131
58. Towbin JA, Jefferies JL. Cardiomyopathies due to left ventricular noncompaction, mitochondrial and storage diseases, and inborn errors of metabolism. Circ Res. (2017) 121:838–54. doi: 10.1161/CIRCRESAHA.117.310987
59. Vockley J. Metabolism as a complex genetic trait, a systems biology approach: implications for inborn errors of metabolism and clinical diseases. J Inherit Metab Dis. (2008) 31:619–29. doi: 10.1007/s10545-008-1005-8
60. Xue J, Gu H, Liu D, Ma W, Wei X, Zhao L, et al. Mitochondrial dysfunction is implicated in retinoic acid-induced spina bifida aperta in rat fetuses. Int J Dev Neurosci. (2018) 68:39–44. doi: 10.1016/j.ijdevneu.2018.04.003
61. Folmes CD, Terzic A. Metabolic determinants of embryonic development and stem cell fate. Reprod Fertil Dev. (2014) 27:82–8. doi: 10.1071/RD14383
62. Chiarelli LR, Morera SM, Bianchi P, Fermo E, Zanella A, Galizzi A, Valentini G. Molecular insights on pathogenic effects of mutations causing phosphoglycerate kinase deficiency. PLoS ONE. (2012) 7:e32065. doi: 10.1371/journal.pone.0032065
63. Coman D, Kranc KR, Christodoulou J. Fumarate hydratase deficiency. In: Adam MP, et al., editors. GeneReviews®. Seattle: University of Washington (2006).
64. Fujisawa Y, Napoli E, Wong S, Song G, Yamaguchi R, Matsui T, et al. Impact of a novel homozygous mutation in nicotinamide nucleotide transhydrogenase on mitochondrial DNA integrity in a case of familial glucocorticoid deficiency. BBA Clin. (2015) 3:70–8. doi: 10.1016/j.bbacli.2014.12.003
65. Landsverk ML, Zhang VW, Wong LC, Andersson HC. A SUCLG1 mutation in a patient with mitochondrial DNA depletion and congenital anomalies. Mol Genet Metab Rep. (2014) 1:451–4. doi: 10.1016/j.ymgmr.2014.09.007
66. Suazo J, Pardo R, Castillo S, Martin LM, Rojas F, Santos JL, et al. Family-based association study between SLC2A1, HK1, and LEPR polymorphisms with myelomeningocele in Chile. Reprod Sci. (2013) 20:1207–14. doi: 10.1177/1933719113477489
67. Elmore S. Apoptosis: a review of programmed cell death. Toxicol Pathol. (2007) 35:495–516. doi: 10.1080/01926230701320337
68. Igney FH, Krammer PH. Death and anti-death: tumour resistance to apoptosis. Nature reviews. Cancer. (2002) 2:277–88. doi: 10.1038/nrc776
69. Penington EC, Hutson JM. The urethral plate–does it grow into the genital tubercle or within it? BJU Int. (2002) 89:733–9. doi: 10.1046/j.1464-410X.2002.02656.x
70. Zhou Y, Liu X, Huang F, Liu Y, Cao X, Shen L, et al. Epithelial-mesenchymal transformation and apoptosis in rat urethra development. Pediatr Res. (2017) 82:1073–9. doi: 10.1038/pr.2017.185
71. Li X, Li J, Zhang Y, Zhou Y. Di-n-butyl phthalate induced hypospadias relates to autophagy in genital tubercle via the PI3K/Akt/mTOR pathway. J Occup Health. (2017) 59:8–16. doi: 10.1539/joh.16-0089-OA
72. van der Zanden LF, Galesloot TE, Feitz WF, Brouwers MM, Shi M, Knoers NV, et al. Exploration of gene-environment interactions, maternal effects and parent of origin effects in the etiology of hypospadias. J Urol. (2012) 188:2354–60. doi: 10.1016/j.juro.2012.08.033
73. An N, Peng J, He G, Fan X, Li F, Chen H. Involvement of activation of mitogen-activated protein kinase (MAPK)/extracellular signal-regulated kinase (ERK) signaling pathway in proliferation of urethral plate fibroblasts in finasteride-induced rat hypospadias. Med Sci Monit. (2018) 24:8984–92. doi: 10.12659/MSM.911271
74. Jiang X, Wang X. Cytochrome C-mediated apoptosis. Ann Rev Biochem. (2004) 73:87–106. doi: 10.1146/annurev.biochem.73.011303.073706
75. Bano D, Prehn J. Apoptosis-inducing factor (AIF) in physiology and disease: the tale of a repented natural born killer. EBioMedicine. (2018) 30:29–37. doi: 10.1016/j.ebiom.2018.03.016
76. Rinaldi C, Grunseich C, Sevrioukova IF, Schindler A, Horkayne-Szakaly I, Lamperti C, et al. (2012). Cowchock syndrome is associated with a mutation in apoptosis-inducing factor. Am J Hum Genet. (2018) 91:1095–102. doi: 10.1016/j.ajhg.2012.10.008
77. Zong L, Guan J, Ealy M, Zhang Q, Wang D, Wang H, et al. Mutations in apoptosis-inducing factor cause X-linked recessive auditory neuropathy spectrum disorder. J Med Genet. (2015) 52:523–31. doi: 10.1136/jmedgenet-2014-102961
78. Wertz IE, Dixit VM. Regulation of death receptor signaling by the ubiquitin system. Cell Death Differ. (2010) 17:14–24. doi: 10.1038/cdd.2009.168
79. Woo SM, Kwon TK. E3 ubiquitin ligases and deubiquitinases as modulators of TRAIL-mediated extrinsic apoptotic signaling pathway. BMB Rep. (2019) 52:119–26. doi: 10.5483/BMBRep.2019.52.2.011
80. Jin Z, Li Y, Pitti R, Lawrence D, Pham VC, Lill JR, et al. Cullin3-based polyubiquitination and p62-dependent aggregation of caspase-8 mediate extrinsic apoptosis signaling. Cell. (2009) 137:721–35. doi: 10.1016/j.cell.2009.03.015
81. Yin Y, Lin C, Kim ST, Roig I, Chen H, Liu L, et al. (2011). The E3 ubiquitin ligase Cullin 4A regulates meiotic progression in mouse spermatogenesis. Dev Biol. (2009) 356:51–62. doi: 10.1016/j.ydbio.2011.05.661
82. Zhang Q, Fillmore TL, Schepmoes AA, Clauss TR, Gritsenko MA, Mueller PW, et al. Serum proteomics reveals systemic dysregulation of innate immunity in type 1 diabetes. J Exp Med. (2013) 210:191–203. doi: 10.1084/jem.20111843
83. Kim SJ, Chae S, Kim H, Mun DG, Back S, Choi HY, et al. A protein profile of visceral adipose tissues linked to early pathogenesis of type 2 diabetes mellitus. Mol Cell Proteomics. (2014) 13:811–22. doi: 10.1074/mcp.M113.035501
84. Schiller HB, Fernandez IE, Burgstaller G, Schaab C, Scheltema RA, Schwarzmayr T, et al. Time- and compartment-resolved proteome profiling of the extracellular niche in lung injury and repair. Mol Syst Biol. (2015) 11:819. doi: 10.15252/msb.20156123
85. Cunha GR, Liu G, Sinclair A, Cao M, Glickman S, Cooke PS, et al. Androgen-independent events in penile development in humans and animals. Differentiation. (2020) 111:98–114. doi: 10.1016/j.diff.2019.07.005
86. Boas M, Boisen KA, Virtanen HF, Kaleva M, Suomi AM, Schmidt IM, et al. Postnatal penile length and growth rate correlate to serum testosterone levels: a longitudinal study of 1962 normal boys. Eur J Endocrinol. (2006) 154:125–9. doi: 10.1530/eje.1.02066
87. Wang YN, Zeng Q, Xiong F, Zeng Y. Male external genitalia growth curves and charts for children and adolescents aged 0 to 17 years in Chongqing, China. Asian J Androl. (2018) 20:567–71. doi: 10.4103/aja.aja_51_18
88. Dossanova A, Lozovoy V, Manekenova K, Lozovaya Y, Seidakhmetov M, Dossanov B, et al. Histological and morphological characteristics of the prepuce of penis skin structure in different age groups. J Pediatr Urol. (2018) 14:280.e1–e6. doi: 10.1016/j.jpurol.2018.02.022
Keywords: quantitative proteomics, etiology, proteomics, hypospadias, liquid chromatography-tandem mass spectrometry (LC-MS/MS)
Citation: Piñeyro-Ruiz C, Serrano H, Jorge I, Miranda-Valentin E, Pérez-Brayfield MR, Camafeita E, Mesa R, Vázquez J and Jorge JC (2020) A Proteomics Signature of Mild Hypospadias: A Pilot Study. Front. Pediatr. 8:586287. doi: 10.3389/fped.2020.586287
Received: 22 July 2020; Accepted: 24 November 2020;
Published: 23 December 2020.
Edited by:
Alexander Springer, Medical University of Vienna, AustriaReviewed by:
Andres Gomez Fraile, University Hospital October 12, SpainCopyright © 2020 Piñeyro-Ruiz, Serrano, Jorge, Miranda-Valentin, Pérez-Brayfield, Camafeita, Mesa, Vázquez and Jorge. This is an open-access article distributed under the terms of the Creative Commons Attribution License (CC BY). The use, distribution or reproduction in other forums is permitted, provided the original author(s) and the copyright owner(s) are credited and that the original publication in this journal is cited, in accordance with accepted academic practice. No use, distribution or reproduction is permitted which does not comply with these terms.
*Correspondence: Juan Carlos Jorge, anVhbi5qb3JnZUB1cHIuZWR1
Disclaimer: All claims expressed in this article are solely those of the authors and do not necessarily represent those of their affiliated organizations, or those of the publisher, the editors and the reviewers. Any product that may be evaluated in this article or claim that may be made by its manufacturer is not guaranteed or endorsed by the publisher.
Research integrity at Frontiers
Learn more about the work of our research integrity team to safeguard the quality of each article we publish.