- 1Department of Pediatrics, Aarhus University Hospital, Aarhus, Denmark
- 2Department of Cardiothoracic and Vascular Surgery, Aarhus University Hospital, Aarhus, Denmark
- 3Comparative Medicine Lab, Aarhus University Hospital, Aarhus, Denmark
- 4The MR Research Centre, Aarhus University Hospital, Aarhus, Denmark
Introduction: Hypoxic ischemic encephalopathy (HIE) is a major cause of death and disability in children worldwide. Apart from supportive care, the only established treatment for HIE is therapeutic hypothermia (TH). As TH is only partly neuroprotective, there is a need for additional therapies. Intermittent periods of limb ischemia, called remote ischemic postconditioning (RIPC), have been shown to be neuroprotective after HIE in rats and piglets. However, it is unknown whether RIPC adds to the effect of TH. We tested the neuroprotective effect of RIPC with TH compared to TH alone using magnetic resonance imaging and spectroscopy (MRI/MRS) in a piglet HIE model.
Methods: Thirty-two male and female piglets were subjected to 45-min global hypoxia-ischemia (HI). Twenty-six animals were randomized to TH or RIPC plus TH; six animals received supportive care only. TH was induced through whole-body cooling. RIPC was induced 1 h after HI by four cycles of 5 min of ischemia and 5 min of reperfusion in both hind limbs. Primary outcome was Lac/NAA ratio at 24 h measured by MRS. Secondary outcomes were NAA/Cr, diffusion-weighted imaging (DWI), arterial spin labeling, aEGG score, and blood oxygen dependent (BOLD) signal measured by MRI/MRS at 6, 12, and 24 h after the hypoxic-ischemic insult.
Results: All groups were subjected to a comparable but mild insult. No difference was found between the two intervention groups in Lac/NAA ratio, NAA/Cr ratio, DWI, arterial spin labeling, or BOLD signal. NAA/Cr ratio at 24 h was higher in the two intervention groups compared to supportive care only. There was no difference in aEEG score between the three groups.
Conclusion: Treatment with RIPC resulted in no additional neuroprotection when combined with TH. However, insult severity was mild and only evaluated at 24 h after HI with a short MRS echo time. In future studies more subtle neurological effects may be detected with increased MRS echo time and post mortem investigations, such as brain histology. Thus, the possible neuroprotective effect of RIPC needs further evaluation.
Introduction
Neonatal hypoxic ischemic encephalopathy (HIE) is a major cause of death and impairment in children (1). Treatment with therapeutic hypothermia (TH) has improved outcome in neonates with moderate to severe HIE, but morbidity and mortality remain high (2). Treatment with TH is limited by a narrow therapeutic time window and further limited to tertiary centers due to technical requirements and the need for specially trained staff. Accordingly, there is a need for neuroprotective strategies that can be combined with TH to improve outcome.
In 1986, Murry et al. showed in dogs that infarction size after coronary occlusion was reduced if the acute myocardial injury was preceded by short ischemic periods—preconditioning (3). Through short periods ischemia applied to a hind limp after the insult, the similar tissue protective effect was later demonstrated in a stroke model in adult rats—remote ischemic postconditioning (RIPC) (4). The tissue protective mechanism of RIPC remains to be fully elucidated together with the optimal timing, number, and duration of remote ischemic cycles. In a review of RIPC for cardio and neuro protection, clinical studies used 5-min cycles of RIPC (5, 6). RIPC has been proposed as a novel neuroprotective intervention for HIE and proven to be neuroprotective in both smaller and larger animal models (7). RIPC reduced infarct volume in rat pups compared to untreated controls (8). Another study in rat pups found improved long-term motor sensory deficits in animals treated with RIPC 24 h after the insult (9). When applied in a larger animal model of HIE, Ezzati et al. found reduced white matter Lac/NAA ratio, higher levels of whole brain ATP, and reduced histological white matter damage with 10 min cycles of RIPC (10). RIPC has also been shown to reduce nitrosative stress in piglets with HIE (11). We have recently shown reduced Lac/NAA ratio in the basal ganglia in piglets treated with 5-min cycles of RIPC compared to piglets who received supportive care only (12). RIPC is a low-tech, readily available intervention and therefore holds potential as a novel neuroprotectant for neonates with HIE. However, it is unknown whether RIPC in combination with TH will improve neuroprotection beyond that of TH alone (13).
We therefore investigated whether there is an added neuroprotective effect of combining RIPC with TH compared to TH alone using magnetic resonance imaging and spectroscopy (MRI/MRS).
Materials and Methods
The study was approved by the Danish Animal Experiments Inspectorate (Permission nr. 2016-15-0201-01052). This study is reported in accordance with the ARRIVE guidelines (ARRIVE checklist in Supplementary Material 1) (14). Details on this piglet model of HIE have previously been given (12, 15).
Anesthesia
Newborn Danish Landrace piglets (<12 h old) were used in this study. Animals were transported directly from the farm to the experimental facilities. Piglets were anesthetized using inhalation of 2–4% sevoflurane. Peripheral intravenous access was acquired through an ear vein. A bolus of propofol 10 mg/kg, fentanyl 30 μg/kg, and rocuronium 1 mg/kg was given and the piglet was intubated and ventilated. Anesthesia was maintained through an infusion of propofol 4–10 mg/kg/h and fentanyl 5–12 μg/kg/h. Anesthetics were reduced to the lowest relevant dose to minimize any possible effect on the aEEG and neurological outcomes. The ventilator was adjusted to an end-tidal CO2 of 4.5–5.5 kPa. Under sterile conditions, umbilical venous and arterial catheters were placed. SatO2%, heart rate, mean arterial blood pressure (MABP), core temperature, and electrocardiogram were continuously recorded and downloaded to a computer (Datex Ohmeda S/5 Collect, Finland). Core temperature was measured through a rectal thermometer placed ~5 cm into the rectum. A single-channel amplitude-integrated electroencephalogram (aEEG) was recorded continuously (Natus Medical Incorporated, CA, USA). Two electrodes were placed on the left and the right side, one behind each eye (approximately equivalent to the parietal 3 and 4 electrodes used in a human neonate). A reference electrode was placed at the base of the snout and a ground electrode on the most caudal part of the head. Piglets received i.v. gentamicin 5 mg/kg once every 24 h and ampicillin 30 mg/kg every 12 h. Blood glucose and electrolytes were monitored and kept within the normal range through infusion of 5–10 ml/kg/h of NeoKNaG (Na+: 15 mmol/L, K+: 10 mmol/L, Cl−: 25 mmol/L, glucose: 505 mmol/L). We aimed to keep MABP >40 mmHg. If hypotension occurred, anesthetics were reduced to the minimum relevant dose and the following treatment given: first, a bolus of saline (10 ml/kg) was administered, followed by infusion of noradrenaline (0.25–1.5 μg/kg/min) and/or dopamine (5–15 μg/kg/min) and/or adrenalin (0.1–1.5 μg/kg/min) and/or dobutamine (2–20 μg/kg/min) (16). For refractory hypotension a bolus of hydrocortisone 2.5 mg/kg was administered.
Hypoxic-Ischemic Insult
During a 45-min period, piglets were ventilated at an FiO2 of 2–10% to mimic the generalized hypoxia neonates may experience during birth. To ensure maximal survival combined with a clinically relevant insult, FiO2 was titrated to a target aEEG (<7μV) combined with a target MABP (<70% of baseline MABP) for at least 5 min. FiO2 was briefly increased if HR <80 min−1 or aEEG <3 μV. After 45 min of hypoxia the piglets were resuscitated at an FiO2 of 21%. If needed, FiO2 was increased to keep SatO2 > 90%.
Therapeutic Hypothermia
TH was achieved through whole-body cooling with a target temperature of 33.5–34.0°C. TH was induced by active cooling with 5°C water bags directly placed on the piglet until target temperature was reached and then maintained through passive cooling with ambient air. TH was commenced 90 min after HI and continued for 24 h.
Remote Ischemic Postconditioning
Sixty minutes after the HI insult, RIPC was induced by four conditioning cycles of 5 min of ischemia and 5 min of reperfusion on both hind limbs. Total occlusion of blood flow was induced by two plastic strips around the proximal part of the hind limbs, and absence/presence of blood flow was verified by ultrasound with Doppler. 5-min periods of ischemia/reperfusion have been found cardio- and neuroprotective in adults (5, 6). In accordance with this, we have previously found reduced brain Lac/NAA ratios in piglets treated with 5 min of RIPC compared to untreated controls (12). Thus, to ensure comparability, 5 min of ischemia/reperfusion were chosen for this study.
Magnetic Resonance Imaging and Spectroscopy (MRI/MRS)
MRI/MRS was performed with a 3 T MR scanner using a knee transmit/receive coil (Skyra, Siemens, Erlangen, Germany). Axial and coronal T2-weighted images were acquired [fast spin echo, repetition and echo time (TR/TE) 6,430/74 ms, slice thickness 2 mm, matrix 320 × 240, field of view (FOV) 160 × 160 mm2]. Diffusion-weighted images (DWI) were acquired (single-shot EPI, TR/TE 3,300/108 msec, slice thickness 3 mm, matrix 196 × 190, FOV 213 × 206 mm2, b-value 800 s/mm2) and apparent diffusion coefficient (ADC) values were calculated in a region of interest (ROI) in the right thalamus. Perfusion-weighted images were acquired using an arterial spin labeling (ASL) sequence (PICORE Q2T, single-shot EPI, TR/TE 3,200/25.6 ms, slice thickness 5 mm, 84 × 84 matrix, FOV 144 × 144 mm2), and whole brain perfusion was calculated in three slices and averaged. Due to low signal-to-noise ratio, ASL data may be negative; all negative values were manually removed before final analysis. A multiecho gradient echo (MGRE) sequence was used for obtaining T2* maps (11 echoes TR/TE 431/3.67–49 ms, slice thickness 4 mm, 192 × 126 matrix, FOV 180 × 118 mm2). ROIs were drawn on the thalamus in two slices and values were averaged. The researcher performing the MRI data analysis was blinded to treatment allocation in the two intervention groups, but not to the group receiving supportive care only. Images were analyzed with Horos software (Annapolis, MD, USA) version 3.3.5. A representative image is provided in Supplementary Material 2. Single voxel proton MRS (PRESS, TR/TE 2,000/135 ms, voxel size 8 × 8 × 8 mm3, 1,024 sample points, spectral width (SW) 1,200 Hz, 128 averages) was acquired in the right side of thalamus, subcortical right-side white matter at the centrum semiovale level, and frontal and occipital cortex. N-acetylaspartate (NAA, 2.02 ppm), lactate (Lac, 1.33 ppm), choline (Cho, 3.2 ppm), and creatine (Cr, 3.02 ppm) were identified. Spectroscopy data were analyzed with LCModel (Stephen Provencher, Oakville, ON, Canada) version 6.3-1L, and Lac/NAA and NAA/Cr ratios were calculated. Thalamic MRS was acquired in two planes and averaged. An image illustrating voxel location and graph can be found in Supplementary Material 2.
Electroencephalographic Analysis
Amplitude integrated EEG (aEEG) recordings were obtained continuously throughout the study. During transport and acquisition of MRI/MRS, electrodes were removed and recordings were paused. aEEG was recorded for all animals during the HI insult. Due to equipment limitations, aEEG was recorded in only half of the animals during the observation period after the HI insult. aEEG recordings were analyzed and scored from 0 to 4 depending on severity as previously described (17, 18). aEEG recordings (0; flat trace, 1; continuous low voltage, 2; burst suppression, 3; discontinuous normal voltage, and 4; normal voltage) were analyzed for each hour after the HI insult and then averaged at 1, 6, 12, 18, and 24 h. Animals were selected randomly for continuous aEEG recording, and the researchers calculating the aEEG score were blinded to the intervention.
Experimental Protocol
A total of 26 piglets were subjected to 45 min of global hypoxia-ischemia (HI) and randomized to RIPC + TH (RIPC + TH group) or TH alone (TH group). The experimental protocol was carried out in two piglets from the same litter on each experimental day. After the HI insult one piglet was randomized to one of the intervention groups, while the other was automatically allocated to the other. Six piglets served as controls and were subjected to the HI insult, received supportive care only, and were kept normothermic (NT group). Animals were observed for 24 h and MRI/MRS was performed at 6, 12, and 24 h of observation. After 24 h, piglets were euthanized by a lethal pentobarbital (80 mg/kg) injection.
Statistics
Based on the data from Zhou et al., a reduction in infarction size from 31 to 22% in RIPC treated animals, and assuming an alpha level of 5 and 90% power, we estimated that 11 animals were sufficient to detect an effect in this study (8). With an expected mortality of 10%, 13 animals were enrolled in each treatment group. Statistical analysis was performed using GraphPad Prism® v8 software. Use of inotropes was registered hourly as infusion rate and then averaged for the 24-h observation period. Number of animals receiving inotropes was reported, the average infusion rate was calculated in the animals that received inotropes. Non-parametric MRS data were log transformed (y=log(y+(1/6)). MRI/MRS and EEG data were tested by mixed-effect model analysis with assumed sphericity and randomly missing values, corrected for multiple comparisons and post-tested with Tukeys test. Demographic- and insult-severity data, inotrope infusion, blood-gas values, and vital parameters were compared with one-way ANOVA for parametric data and Kruskal-Wallis test for non-parametric data. A two-sided p-value < 0.05 was considered statistically significant. Demographic- and insult-severity data, inotrope infusion, blood-gas values, and vital parameters are presented as median with interquartile range (IQR). MRI/MRS data are presented as scatter plots with superimposed median and interquartile range.
Results
Insult Severity and Survival
Four animals in the TH group, three animals in the RIPC + TH group, and one animal in the NT group died after the HI insult. All died from refractory hypotension except for the one death in the NT group which was caused by mechanical ventilator failure. Thus, nine animals in the TH group, ten animals in the RIPC+TH group, and five animals in the NT group completed the whole study (Table 1). All three groups received a comparable insult with regard to duration of aEEG depression, hypotension, and metabolic acidosis (Tables 2, 3). The two intervention groups received more dopamine than the NT group (Table 2). One animal in the TH and two animals in the TH + RIPC group received a bolus of hydrocortisone. One animal in the NT group received infusion with adrenaline (0.01 μg/kg/min) and dobutamine (0.42 μg/kg/min). Temperature from before to 1 h after the insult was slightly increased but within normal range (Table 3). TH was successfully induced in both intervention groups and resulted in a decreased heart rate (Table 3).
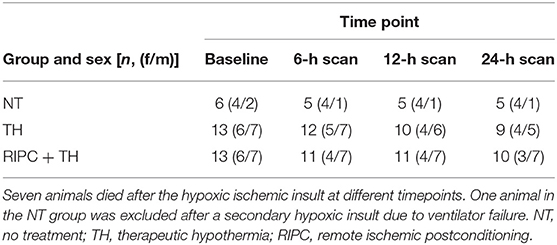
Table 1. Survival and gender distribution in the three groups and number of animals who were scanned at the three time points.
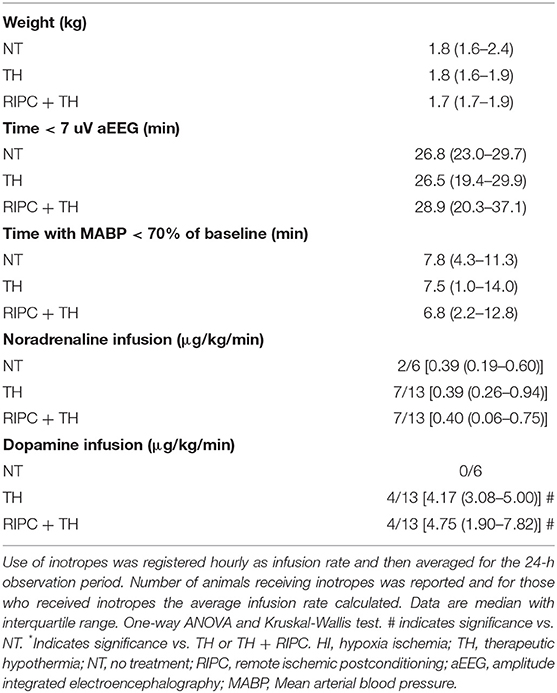
Table 2. Demographic- and insult-severity data for piglets subjected to a HI insult and subsequently treated with TH, TH + RIPC, or supportive care only.
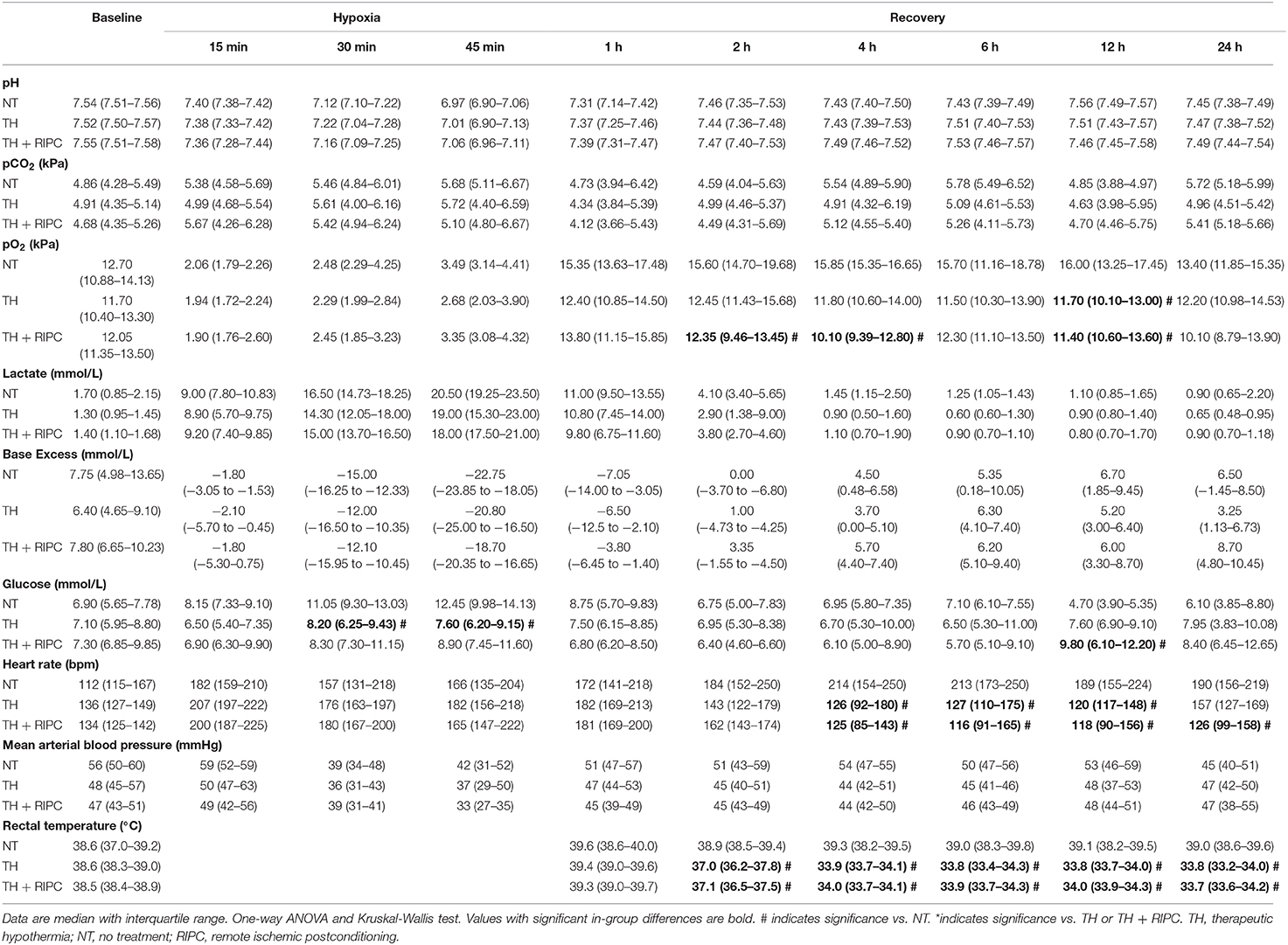
Table 3. Arterial blood-gas values and vital parameters at baseline, during hypoxia, and in recovery in piglets subjected to a hypoxic-ischemic insult and subsequently treated with TH, TH + RIPC, or supportive care only.
Magnetic Resonance Spectroscopy
MRS showed no difference between the three groups with regards to Lac/NAA ratio at any time point (Figure 1). MRS showed decreased NAA/Cr ratio in the occipital cortex and thalamus 24 h after the insult in the NT group compared to the TH and TH + RIPC group (Figure 2) and the NAA/Cr ratio in the occipital cortex was lower in the TH + RIPC group compared to the TH group (Figure 2). NAA/Cr ratio was stable over time in the two intervention groups, while the NAA/Cr ratio decreased with time in the NT group (Figure 2).
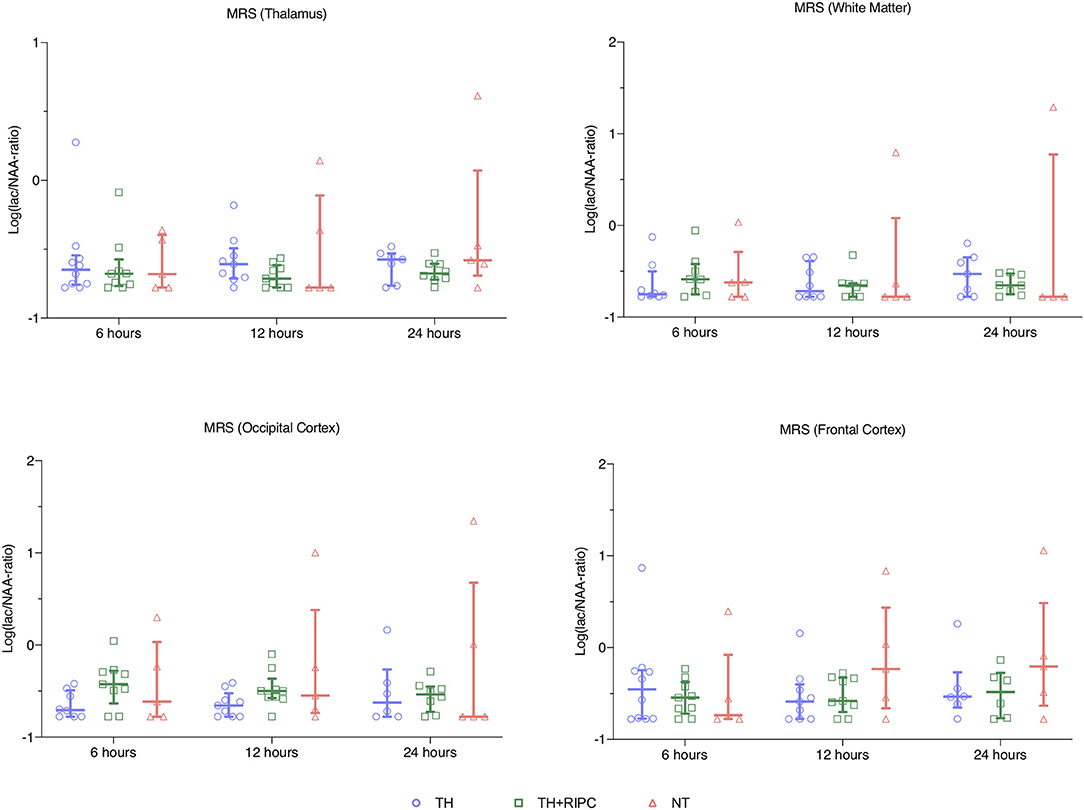
Figure 1. Magnetic resonance spectroscopy in piglets subjected to a HI insult and treated with therapeutic hypothermia (TH), TH and remote ischemic postconditioning (TH + RIPC), or supportive care only (NT). Scans were performed at 6, 12, and 24 h after the insult. Lac, lactate; NAA, N-acetylaspartate. Data are scatter plots with superimposed median and interquartile range. Mixed-effect model analysis, *p < 0.05.
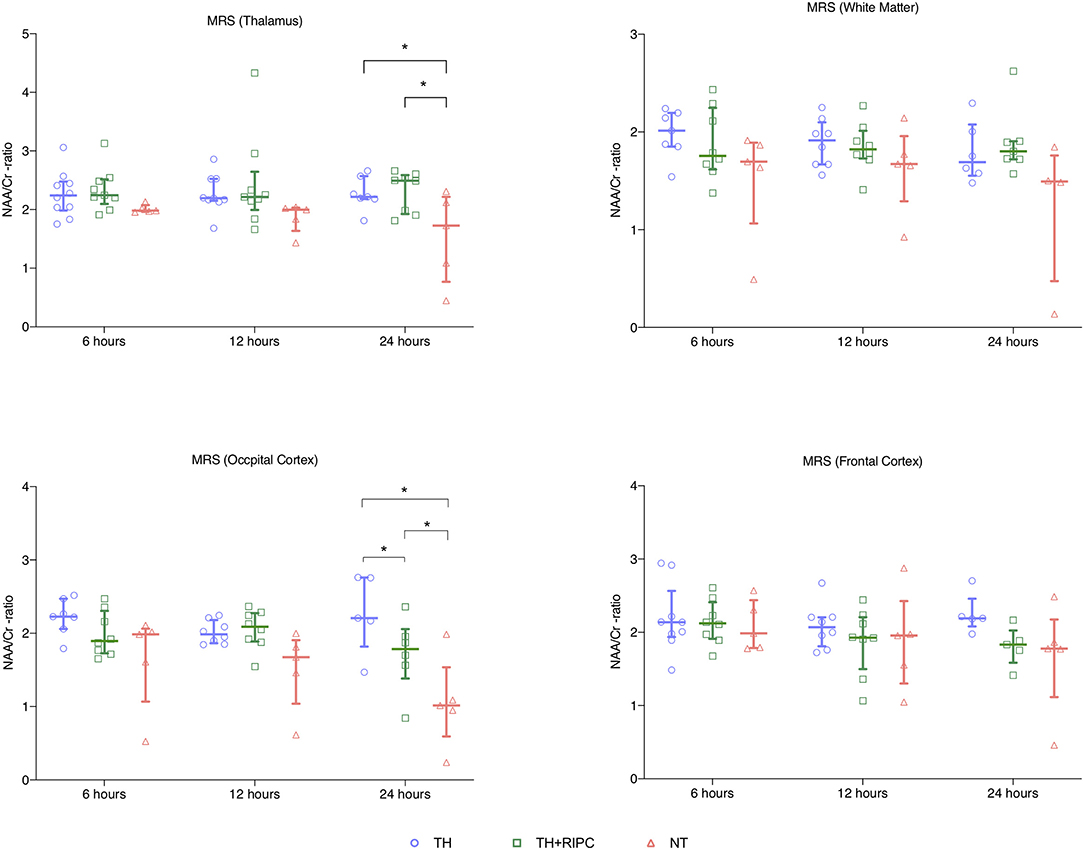
Figure 2. Magnetic resonance spectroscopy in piglets subjected to a HI insult and treated with therapeutic hypothermia (TH), TH and remote ischemic postconditioning (TH + RIPC), or supportive care only (NT). Scans were performed at 6, 12, and 24 h after the insult. NAA, N-acetyl aspartate; Cr, creatinine. Data are scatter plots with superimposed median and interquartile range. Mixed-effect model analysis, *p < 0.05.
Magnetic Resonance Imaging
Cerebral edema measured by DWI showed no difference between the three groups (Figure 3). Although not statistically significant, animals in the NT group had lower cerebral oxygenation 6 and 12 h after the HI insult as assessed from by BOLD measurements (Figure 3). There was no difference between the two intervention groups with regard to cerebral oxygenation. After a quality check of the ASL data one scan was removed from the TH+RIPC group and two from the NT group. CBF measured by ASL was similar in the three groups (Figure 3).
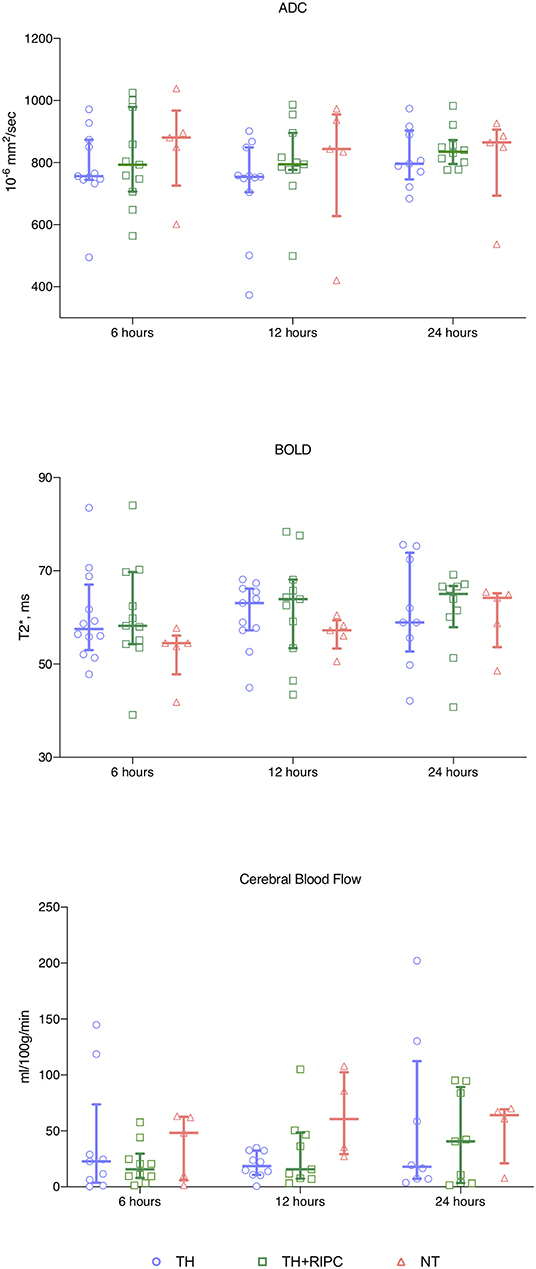
Figure 3. Magnetic resonance imaging in piglets subjected to a HI insult and treated with therapeutic hypothermia (TH), TH and remote ischemic postconditioning (TH + RIPC), or supportive care only (NT). Scans were performed at 6, 12, and 24 h after the insult. ADC, apparent diffusion imaging; BOLD, blood oxygenation level dependent. Data are scatter plots with superimposed median and interquartile range. Mixed-effect model analysis, *p < 0.05.
aEEG Analysis
aEEG was available for three animals in the NT group, six animals in the TH group, and seven animals in the TH+RIPC group. aEEG data were missing during the first 6 h in two animals in the TH + RIPC group. Early EEG recovery (continuous aEEG reached by the 2nd hour) was seen in two of the three animals in the NT group, four of the six animals in the TH group, and two of the five in the TH+RIPC group. Seizures and brief rhythmic discharges were detected in 0 out of three animals in the NT group, two of the six animals in the TH group, and three of the seven animals in the TH + RIPC group. There was no overall difference in aEEG score between the three groups (Figure 4).
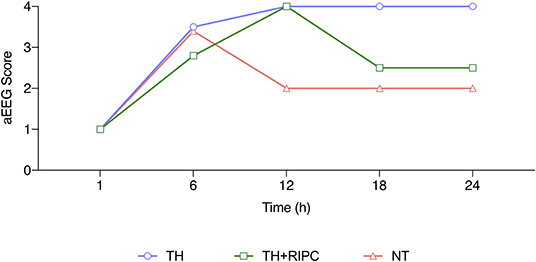
Figure 4. aEEG score for piglets subjected to a HI insult and treated with therapeutic hypothermia (TH), TH and remote ischemic postconditioning (TH + RIPC), or supportive care only (NT). aEEG were scored every hour and averaged for 6, 12, 18, and 24 h. Data are median. Mixed-effect model analysis. #TH vs. NT, *TH vs. TH + RIPC, §NT vs. TH + RIPC.
Discussion
This is the first study to assess RIPC in addition to TH in neonatal HIE. Overall, adding RIPC to TH resulted in no additional neuroprotective effect. When comparing duration of aEEG suppression and duration of hypotension from this study with data from a similar study in piglets, the insult severity acquired in this study should be regarded as mild rather than severe (19). Indeed, most of our animals presented with rapid recovery and normalization of aEEG after HI (Figure 4). To ensure survival and substantial neural damage, titration of the insult is essential as too extensive HI results in death and too little HI results in absence of damage (20). Insult titration also ensures that the animal is subjected not only to a period of hypoxia but also to a predetermined period of hypotension. Hypotension combined with hypoxia has been shown to be essential to produce a more severe insult (19). This is in accordance with our own observations in a previous study where severely damaged animals had aEEG suppression of at least 40 min combined with a period of hypotension (12). The importance of cerebral hypoperfusion in the pathology of brain injury is further underlined by other studies in which universal hypoxia was combined with carotid clamping (21). It would therefore be relevant in future studies to investigate the neuroprotective effect of RIPC when combined with TH after a severe insult.
Despite the mild insult, the NT group showed a lower NAA/Cr ratio at 24 h than was seen in the other groups. N-acetylaspartate is an amino acid present in healthy neurons and oligodendrocytes-type 2 astrocyte progenitor cells (22, 23), and a reduction in NAA after a HI insult is due to neural damage and reduction in progenitor cells. Peak-area ratio of NAA/Cr and [NAA] measured by MRS has high prognostic accuracy for neonates with HIE (24, 25). This is in accordance with our results as peak-area ratio of NAA/Cr was reduced as early 24 h after the insult in the NT group. This finding underline one of the possible neuroprotective mechanisms of TH.
Cerebral edema measured by diffusion weighted imaging (DWI) has been proposed as a biomarker of brain injury in neonates with HIE (26). Compared to MRS, DWI has been shown to underestimate the extent of damage when acquired on day 1 (27). McKinstry et al. found that edema measured by diffusion tensor imaging also underestimates the neural damage when performed on day 1 compared to images acquired on day 2–4 (28). In keeping with this, in our study we found no difference between the three groups on ADC maps acquired within the first 24 h after the HI insult.
BOLD measured by MRI is based on the paramagnetic properties of deoxyhemoglobin. Increased concentration of deoxyhemoglobin will result in decreased T2* relaxation time and signal loss (29). In this study, BOLD measurements were performed in the thalamus after the HI insult and compared local differences in oxygenation. Although not statistically significant, animals in the NT group had lower T2* values after 6 and 12 h and reached the same levels as did the TH and TH+RICP groups by 24 h. TH is known to reduce cerebral metabolism (30). The decreased T2* values could indicate an increased turnover of oxyhemoglobin to deoxyhemoglobin due to relatively high metabolism immediately after the HI insult. Increased oxygen consumption is coupled to regional changes in blood flow, and T2* values have been correlated to CBF measured by ASL (31). Surprisingly, change in blood flow was not demonstrated in our study as the NT group presented with CBF values comparable to the two intervention groups despite lower T2* values.
Several of the animals that received TH developed hypotension after the HI insult. Ethical standards for animal studies require pain relief and sedation. A side effect of propofol is reduced cardiac output and hypotension, which may be more severe when propofol is combined with opioids (32). In addition, drug metabolism may be impaired after HI due to liver and kidney failure and then be further reduced by TH because it alters drug metabolism (33, 34). Cardiac function might also be compromised due to TH (35). This was apparent in our study, as piglets in the two TH-treated groups required more inotropes and died from refractory hypotension more often than piglets that received supportive care only. The comparison between the two TH-treated groups should, however, still be valid. The increased mortality due to this complication is unlikely to influence the overall conclusion of this study because the majority of MRI/MRS data were similar between the two TH-treated groups.
Limitations
Piglets received intravenous infusion with fentanyl and propofol to ensure pain relief and sedation. One of the proposed mechanisms of RIPC is through opioid receptor activation (8). Since both intervention groups received fentanyl, the additional neuroprotective effect of RIPC may have been reduced.
Another limitation is spatial resolution of the MRI data. RIPC was shown to predominantly protect white matter measured by MRS in a previous piglet study (10). Due to limited spatial resolution in the MRI data we were unable to perform DWI, BOLD, and ADC measurements on white matter specifically.
An echo time (TE) of 135 ms was chosen as this is part of the MRS protocol used in our clinical MR Centre. A TE of 135 ms also allows for easy lactate-peak identification due to peak inversion. However, a prolonged TE of 288 ms will increase PRESS detection (36). Lactate peak identification with a TE of 135 ms in a 3T scanner is possibly further complicated by anomalous J-modulation (37). To ensure high spatial resolution, a 8 × 8 × 8 mm voxel was used despite the decrease in signal-to-noise ratio. However, the low signal-to-noise ratio and short TE may have obscured the detection of lactate peaks in some animals. Accordingly, in future studies an TE of 288 ms and a larger voxel size might identify more subtle differences.
We examined MRI/MRS 24 h after HI based on times-series pilot studies that showed significant lactate accumulation detectable early in normothermic piglets. This timing would coincide with the secondary energy failure phase. Clinical data on early MRI/MRS suggest that moderate-severe HIE can be identified at this time (38). However, TH augments and delays neuropathological processes and the possible protective effect of RIPC may not manifest until later as neurological injury after HI is a dynamic process that evolves over time (39). Thus, longer running studies are required to evaluate the full extent of neural damage. We opted to focus on outcome measures available in the clinical setting. However, histological analysis was not performed, but could contribute with valuable information on the more subtle neural damage present at this early stage.
Conclusion
We found no additional neuroprotective effect when RIPC was added to TH after mild HI. However, despite the mild trauma, the neuroprotective effect of TH was detectable by a reduction in peak-area ratio of NAA/Cr. Our results suggest that future studies may benefit from more severe insults, prolonged TE on MRS, less sedation, histological analysis, and outcomes measured at a later time point after HI.
Data Availability Statement
The datasets generated for this study are available on request to the corresponding author.
Ethics Statement
The animal study was reviewed and approved by the Danish Animal Experiments Inspectorate (Permission nr. 2016-15-0201-01052). This study is reported in accordance with the ARRIVE guidelines (ARRIVE checklist in Supplementary Material 1).
Author Contributions
TA, KK, VH, MP, SR, and TH designed the study. TA, MVP, MA, and HA undertook the experiments. TA, SR, LH, and MP performed data analysis. TA drafted the manuscript. All authors have critically reviewed the drafted manuscript and have approved the final manuscript and agree to be accountable for all aspects of the work.
Funding
This study was funded by The Lundbeck Foundation (grant no: R20 8-2015-3647), Aarhus University, The Ludvig and Sara Elsass Foundation (grant no: 19-3-0288), and Young Investigator START-UP Award by the European Society for Pediatric Research.
Conflict of Interest
The authors declare that the research was conducted in the absence of any commercial or financial relationships that could be construed as a potential conflict of interest.
Acknowledgments
The authors would like to acknowledge the staff at the animal facilities at Institute of Clinical Medicine at Aarhus University.
Supplementary Material
The Supplementary Material for this article can be found online at: https://www.frontiersin.org/articles/10.3389/fped.2020.00299/full#supplementary-material
References
1. Douglas-Escobar M, Weiss MD. Hypoxic-ischemic encephalopathy A review for the clinician. JAMA Pediatr. (2015) 169:397–403. doi: 10.1001/jamapediatrics.2014.3269
2. Jacobs SE, Berg M, Hunt R, Tarnow-Mordi WO, Inder TE, Davis PG. Cooling for newborns with hypoxic ischaemic encephalopathy. Cochrane Database Syst Rev. (2013) 2013:CD003311. doi: 10.1002/14651858.CD003311.pub3
3. Murry CE, Jennings RB, Reimer KA. Preconditioning with ischemia : injury delay of lethal cell ischemic myocardium. Circulation. (1986) 74:1224–136. doi: 10.1161/01.CIR.74.5.1124
4. Ren C, Yan Z, Wei D, Gao X, Chen X, Zhao H. Limb remote ischemic postconditioning protects against focal ischemia in rats. Brain Res. (2009) 1288:88–94. doi: 10.1016/j.brainres.2009.07.029
5. Zhao JJ, Xiao H, Zhao WB, Zhang XP, Xiang Y, Ye ZJ, et al. Remote ischemic postconditioning for ischemic stroke: a systematic review and meta-analysis of randomized controlled trials. Chin Med J. (2018) 131:956–65. doi: 10.4103/0366-6999.229892
6. Pickard JMJ, Bøtker HE, Crimi G, Davidson B, Davidson SM, Dutka D, et al. Remote ischemic conditioning: from experimental observation to clinical application: report from the 8th Biennial Hatter Cardiovascular Institute Workshop. Basic Res Cardiol. (2015) 110:453. doi: 10.1007/s00395-014-0453-6
7. Hassell KJ, Ezzati M, Alonso-Alconada D, Hausenloy DJ, Robertson NJ. New horizons for newborn brain protection: enhancing endogenous neuroprotection. Arch Dis Child Fetal Neonatal Ed. (2015) 100:F541–51. doi: 10.1136/archdischild-2014-306284
8. Zhou Y, Fathali N, Lekic T, Ostrowski RP, Chen C, Martin RD, et al. Remote limb ischemic postconditioning protects against neonatal hypoxic-ischemic brain injury in rat pups by the opioid receptor/akt pathway. Stroke. (2011) 42:439–44. doi: 10.1161/STROKEAHA.110.592162
9. Drunalini Perera PN, Hu Q, Tang J, Li L, Barnhart M, Doycheva DM, et al. Delayed remote ischemic postconditioning improves long term sensory motor deficits in a neonatal hypoxic ischemic rat model. PLoS ONE. (2014) 9:e90258. doi: 10.1371/journal.pone.0090258
10. Ezzati M, Bainbridge A, Broad KD, Kawano G, Oliver-Taylor A, Rocha-Ferreira E, et al. Immediate remote ischemic postconditioning after hypoxia ischemia in piglets protects cerebral white matter but not grey matter. J Cereb Blood Flow Metab. (2016) 36:1396–411. doi: 10.1177/0271678X15608862
11. Rocha-Ferreira E, Rudge B, Hughes MP, Rahim AA, Hristova M, Robertson NJ. Immediate remote ischemic postconditioning reduces brain nitrotyrosine formation in a piglet asphyxia model. Oxid Med Cell Longev. (2016) 2016:5763743. doi: 10.1155/2016/5763743
12. Kyng KJ, Kerrn-Jespersen S, Bennedsgaard K, Skajaa T, Pedersen M, Holm IE, et al. Short-term outcomes of remote ischemic postconditioning 1 h after perinatal hypoxia-ischemia in term piglets. Pediatr Res. (2020). doi: 10.1038/s41390-020-0878-6. [Epub ahead of print].
13. Adstamongkonkul D, Hess DC. Ischemic Conditioning and neonatal hypoxic ischemic encephalopathy: a literature review. Cond Med. (2017) 1:9–16.
14. Kilkenny C, Browne WJ, Cuthill IC, Emerson M, Altman DG. The ARRIVE guidelines checklist animal research : reporting in vivo experiments. Br J Pharmacol. (2010) 8:8–9. doi: 10.1371/journal.pbio.1000412
15. Kyng KJ, Skajaa T, Kerrn-Jespersen S, Andreassen CS, Bennedsgaard K, Henriksen TB. A piglet model of neonatal hypoxic-ischemic encephalopathy. J Vis Exp. (2015) e52454. doi: 10.3791/52454
16. Nachar RA, Booth EA, Friedlich P, Borzage M, Soleymani S, Wider MD, et al. Dose-dependent hemodynamic and metabolic effects of vasoactive medications in normotensive, anesthetized neonatal piglets. Pediatr Res. (2011) 70:473–9. doi: 10.1203/PDR.0b013e31822e178e
17. Hellström-Westas L, Rosen I, de Vries LS, Greisen G. Amplitude-integrated EEG classification and interpretation in preterm and term infants. Neoreviews. (2006) 7:e76–87. doi: 10.1542/neo.7-2-e76
18. Robertson NJ, Martinello K, Lingam I, Avdic-Belltheus A, Meehan C, Alonso-Alconada D, et al. Melatonin as an adjunct to therapeutic hypothermia in a piglet model of neonatal encephalopathy: a translational study. Neurobiol Dis. (2019) 121:240–51. doi: 10.1016/j.nbd.2018.10.004
19. Foster KA, Colditz PB, Lingwood BE, Burke C, Dunster KR, Roberts MS. An improved survival model of hypoxia / ischaemia in the piglet suitable for neuroprotection studies. Brain Res. (2001) 919:122–31. doi: 10.1016/S0006-8993(01)03011-6
20. Björkman ST, Foster KA, O'driscoll SM, Healy GN, Lingwood BE, Burke C, et al. Hypoxic/Ischemic models in newborn piglet: comparison of constant FiO2 versus variable FiO2 delivery. Brain Res. (2006) 1100:110–7. doi: 10.1016/j.brainres.2006.04.119
21. Munkeby BH, De Lange C, Emblem KE, Bjornerud A, Kro GAB, Andresen J, et al. A piglet model for detection of hypoxic-ischemic brain injury with magnetic resonance imaging. Acta Radiol. (2008) 49:1049–57. doi: 10.1080/02841850802334224
22. Urenjak J, Williams SR, Gadian DG, Noble M. Proton nuclear magnetic resonance spectroscopy unambiguously identifies different neural cell types. J Neurosci. (1993) 13:981–9. doi: 10.1523/JNEUROSCI.13-03-00981.1993
23. Urenjak J, Williams SR, Gadian DG, Noble M. Specific expression of N-acetylaspartate in neurons, oligodendrocyte-type-2 astrocyte progenitors, and immature oligodendrocytes in vitro. J Neurochem. (1992) 59:55–61. doi: 10.1111/j.1471-4159.1992.tb08875.x
24. Cheong JLY, Cady EB, Penrice J, Wyatt JS, Cox IJ, Robertson NJ. Proton MR spectroscopy in neonates with perinatal cerebral hypoxic-ischemic injury: metabolite peak-area ratios, relaxation times, and absolute concentrations. Am J Neuroradiol. (2006) 27:1546–54.
25. Lally PJ, Montaldo P, Oliveira V, Soe A, Swamy R, Bassett P, et al. Magnetic resonance spectroscopy assessment of brain injury after moderate hypothermia in neonatal encephalopathy: a prospective multicentre cohort study. Lancet Neurol. (2019) 18:35–45. doi: 10.1016/S1474-4422(18)30325-9
26. van Laerhoven H, de Haan TR, Offringa M, Post B, van der Lee JH. Prognostic tests in term neonates with hypoxic-ischemic encephalopathy: a systematic review. Pediatrics. (2013) 131:88–98. doi: 10.1542/peds.2012-1297
27. Barkovich AJ, Westmark KD, Bedi HS, Partridge JC, Ferriero DM, Vigneron DB. Proton spectroscopy and diffusion imaging on the first day of life after perinatal asphyxia: preliminary report. Am J Neuroradiol. (2001) 22:1786–94.
28. McKinstry RC, Miller JH, Snyder AZ, Mathur A, Schefft GL, Almli CR, et al. A prospective, longitudinal diffusion tensor imaging study of brain injury in newborns. Neurology. (2002) 59:824–33. doi: 10.1212/WNL.59.6.824
29. Thulborn KR, Waterton JC, Matthews PM, Radda GK. Oxygenation dependence of the transverse relaxation time of water protons in whole blood at high field. Biochim Biophys Acta. (1982) 714:265–70. doi: 10.1016/0304-4165(82)90333-6
30. Laptook AR, Corbett RJT, Sterett R, Garcia D, Tollefsbol G. Quantitative relationship between brain temperature and energy utilization rate measured in vivo using 31P and 1H magnetic resonance spectroscopy. Pediatr Res. (1995) 38:919–25. doi: 10.1203/00006450-199512000-00015
31. Christen T, Schmiedeskamp H, Straka M, Bammer R, Zaharchuk G. Measuring brain oxygenation in humans using a multiparametric quantitative blood oxygenation level dependent MRI approach. Magn Reson Med. (2012) 68:905–11. doi: 10.1002/mrm.23283
32. Skues MA, Prys-Roberts C. The pharmacology of propofol. J Clin Anesth. (1989) 1:387–400. doi: 10.1016/0952-8180(89)90080-9
33. Shah P, Riphagen S, Beyene J, Perlman M. Multiorgan dysfunction in infants with post-asphyxial hypoxic-ischaemic encephalopathy. Arch Dis Child Fetal Neonatal Ed. (2004) 89:F152–5. doi: 10.1136/adc.2002.023093
34. van den Broek MPH, Groenendaal F, Egberts ACG, Rademaker CMA. Effects of hypothermia on pharmacokinetics and pharmacodynamics. Clin Pharmacokinet. (2010) 49:277–94. doi: 10.2165/11319360-000000000-00000
35. Giesinger RE, Bailey LJ, Deshpande P, McNamara PJ. Hypoxic-ischemic encephalopathy and therapeutic hypothermia: the hemodynamic perspective. J Pediatr. (2017) 180:22–30.e2. doi: 10.1016/j.jpeds.2016.09.009
36. Robertson NJ, Thayyil S, Cady BE, Raivich G. Magnetic resonance spectroscopy biomarkers in term perinatal asphyxial encephalopathy: from neuropathological correlates to future clinical applications. Curr Pediatr Rev. (2014) 10:37–47. doi: 10.2174/157339631001140408120613
37. Lange T, Dydak U, Roberts TPL, Rowley HA, Bjeljac M, Boesiger P. Pitfalls in lactate measurements at 3T. Am J Neuroradiol. (2006) 27:895–901.
38. Lucke AM, Shetty AN, Hagan JL, Walton A, Stafford TD, Chu ZD, et al. Early proton magnetic resonance spectroscopy during and after therapeutic hypothermia in perinatal hypoxic–ischemic encephalopathy. Pediatr Radiol. (2019) 49:941–50. doi: 10.1007/s00247-019-04383-8
Keywords: neonatal encephalopathy, neuroprotection, remote ishemic postconditioning, piglet model, magnetic resonance imaging, magnetic resonance spectroscopy
Citation: Andelius TCK, Pedersen MV, Andersen HB, Andersen M, Hjortdal VE, Pedersen M, Ringgaard S, Hansen LH, Henriksen TB and Kyng KJ (2020) No Added Neuroprotective Effect of Remote Ischemic Postconditioning and Therapeutic Hypothermia After Mild Hypoxia-Ischemia in a Piglet Model. Front. Pediatr. 8:299. doi: 10.3389/fped.2020.00299
Received: 31 January 2020; Accepted: 11 May 2020;
Published: 26 June 2020.
Edited by:
Julie Wixey, The University of Queensland, AustraliaReviewed by:
Ivo Bendix, Essen University Hospital, GermanyNicola Jayne Robertson, University College London, United Kingdom
Copyright © 2020 Andelius, Pedersen, Andersen, Andersen, Hjortdal, Pedersen, Ringgaard, Hansen, Henriksen and Kyng. This is an open-access article distributed under the terms of the Creative Commons Attribution License (CC BY). The use, distribution or reproduction in other forums is permitted, provided the original author(s) and the copyright owner(s) are credited and that the original publication in this journal is cited, in accordance with accepted academic practice. No use, distribution or reproduction is permitted which does not comply with these terms.
*Correspondence: Ted C. K. Andelius, ted.andelius@clin.au.dk
†ORCID: Ted C. K. Andelius orcid.org/0000-0003-4438-4686