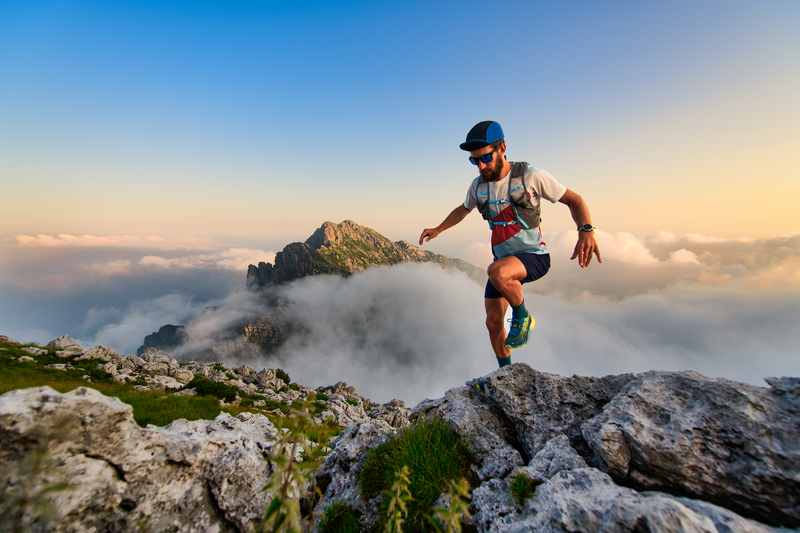
95% of researchers rate our articles as excellent or good
Learn more about the work of our research integrity team to safeguard the quality of each article we publish.
Find out more
MINI REVIEW article
Front. Pediatr. , 27 August 2019
Sec. Pediatric Immunology
Volume 7 - 2019 | https://doi.org/10.3389/fped.2019.00350
This article is part of the Research Topic Translational Insights into Pediatric Immune-Related Diseases View all 13 articles
The encounter between Mycobacterium tuberculosis (Mtb) and the host leads to a complex and multifaceted immune response possibly resulting in latent infection, tubercular disease or to the complete clearance of the pathogen. Macrophages and CD4+ T lymphocytes, together with granuloma formation, are traditionally considered the pillars of immune defense against Mtb and their role stands out clearly. However, there is no component of the immune system that does not take part in the response to this pathogen. On the other side, Mtb displays a complex artillery of immune-escaping mechanisms capable of responding in an equally varied manner. In addition, the role of each cellular line has become discussed and uncertain further than ever before. Each defense mechanism is based on a subtle balance that, if altered, can lean to one side to favor Mtb proliferation, resulting in disease progression and on the other to the host tissue damage by the immune system itself. Through a brief and complete overview of the role of each cell type involved in the Mtb response, we aimed to highlight the main literature reviews and the most relevant studies in order to facilitate the approach to such a complex and changeable topic. In conclusion, this narrative mini-review summarizes the various immunologic mechanisms which modulate the individual ability to fight Mtb infection taking in account the major host and pathogen determinants in the susceptibility to tuberculosis.
Tuberculosis (TB), caused by Mycobacterium tuberculosis (Mtb) infection, was among the top 10 causes of death worldwide in 2017 with about 1.5 million registered deceases (1). Mtb was responsible for approximately 10.0 million incident cases of TB disease with 10% of these occurring among children (1). One to five bacilli may suffice to transmit the infection by air (2). When inhaled, Mtb encounters a first line of defense consisting of airway epithelial cells (AECs) and “professional” phagocytes (neutrophils, monocytes and dendritic cells) (3, 4). If this first line succeeds in eliminating the Mtb rapidly, the infection aborts (5). Otherwise, phagocytes are infected and the Mtb reproduces inside the cells, initially causing few, if any, clinical manifestations (5). The establishment of the infection, the development of active TB (ATB) rather than latent TB infection (LTBI) and the eventual evolution of LTBI to ATB depends on the complex relation between bacterial and host factors.
The aim of this narrative minireview is to give a hint of the complexity of the above-mentioned determinants and to briefly summarize the major defense mechanisms of innate and adaptive immunity against Mtb outlining the role of the different cell populations and their complex interplay.
In order to perform a narrative review of the available literature, we searched the PubMed database from April 2014 through April 2019, using the following key words: “immune,” “immunity,” “tuberculosis,” “Mycobacterium tuberculosis.” Subsequently, for each topic, specific key words (“susceptibility,” “resistance,” “virulence,” “airway epithelial cell,” “macrophage,” “neutrophil,” “dendritic cell,” “natural killer,” “mast cell,” “complement,” “CD4,” “CD8,” “humoral,” “antibody,” and “granuloma”) were associated with the word “tuberculosis” in order to access proper specific literature. The search and the selection process were not systematic. Articles were limited to English language and full text availability, and they were excluded if they were redundant or not pertinent. References of all relevant articles were also evaluated, and studies published previously than 2014 were cited if considered relevant. Results were critically summarized in the following paragraphs: (1) “host and bacterial determinants in human tuberculosis,” (2) “innate immune response against Mycobacterium tuberculosis,” and (3) “adaptive immune response against Mycobacterium tuberculosis.”
Several epidemiological models of family members who have long shared the bedroom with subjects with ATB, sailors who lived in confined spaces with subjects with open TB and extensive case studies of South African miners and Norwegian or American students, have clearly demonstrated that 5 to 20% of those who meet subjects with ATB do not become infected (resilient individuals or resisters), or become infected only transiently and then get rid of the infection (early sterilization or early clearance) (6). An individual can be defined resilient if after close and prolonged contact with the index case shows simultaneous negativity of the skin reactivity test and of the IFN-γ release assay (IGRA) which persists for at least 1 year. Studies carried out on siblings have shown that Mtb resilience is more frequent between two siblings than between two unrelated subjects, suggesting the role of genetics in the development of Mtb resilience (7). Genome wide linkage analysis detected several loci like 2q21-2q24, 5p13-5q22, and the TST1 on 11p14 associated with the resilient phenotype (8, 9).
On the other side, the study of TB susceptibility, has shed light onto various components of immunity to mycobacteria in humans. Different genetic polymorphisms which modulate the host immune response in favor of TB infection and disease progression have been identified in human leukocyte antigens (HLA), toll like receptors (TLR), vitamin D receptors (VDR), cytokines with their receptors and many other functional immune components (10, 11). Moreover, mendelian susceptibilities to mycobacterial disease (MSMD) have been identified as clinical conditions with selective susceptibility to poorly virulent mycobacteria in the absence of patent immunodeficiency (12). Since 1996, 11 genes which underlie 21 different genetic disorders related to interferon (IFN)-γ immunity and responsible for MSMD have been identified (12).
Furthermore, transcriptomic studies have described a TB signature of neutrophil-driven IFN-inducible genes in ATB, including IFN-γ but also type I IFNs, reflecting disease extension and response to treatment and highlighting the previously under-appreciated role of IFNαβ signaling in TB pathogenesis (4, 13, 14).
Beyond host factors, bacterial virulence constitutes the other major player when evaluating the risk of TB infection. Virulence is not merely limited to bacterial strain or burden in respiratory secretion but takes into account the differential Mtb gene expression in the different phases of infection. Mtb lacks classical virulence factors such as toxins and its immune-escaping ability depends on the modulation of lipid metabolism, metal-transporter proteins, protease, proteins inhibiting the antimicrobial effectors of macrophages (MΦs) and many others (15).
The study of immune response in resilient and susceptible individuals, together with bacterial factors, has offered fundamental information for the understanding of TB immunology suggesting potential improvements in diagnostic and therapeutic approaches (Table 1).
The significance of innate immunity in the defense against Mtb stands out clearly as we consider the MSMD where a disruption of the innate axis leads to dramatic, life-threatening clinical presentation of TB (12).
MΦ, neutrophils, dendritic cells (DCs), natural killer cells (NK), mast cells and complement are the major players of innate immunity. On the other hand, AECs also contribute to the defense attempt against Mtb and could be considered as innate immunity components (Table 1).
AECs are the first cells to come in contact with Mtb. Beyond their major role as physical barriers, they display several immunological functions albeit being traditionally considered as “non-professional” immune cells. Through pattern recognition receptors (PRRs), AECs can perceive the presence of Mtb and consequently modulate the composition of the airways surface liquid improving its antimicrobial capacity (16). Moreover, PRRs activation leads to the production of inflammatory cytokines and to the activation of mucosal-associated invariant T cells stimulating IFN-γ and tumor necrosis factor (TNF)-α production (17).
MΦs are the first line of defense, but only if the ratio of forces lies clearly to their advantage and the intervention is immediate they can cancel the infection (5, 18). Otherwise, they favor its development because they become first a niche for the slow replication of the Mtb and then the sanctuary for the persistence of the infection inside the phagosome during the latent infection phase. Mtb expresses an extremely wide variety of virulence factors that counteract MΦs efforts in suppressing the pathogen. Among Mtb strategies we can include the inhibition of intracellular trafficking, the inhibition of autophagy, the acquisition of cytosol access, the induction of host cell death and the neutralization of toxic components as reactive oxygen species and toxic metals (19).
Whilst IFN-γ is a key element in the containment of Mtb within the MΦ, it is now widely recognized that performing this function requires the presence of vitamin D (63). Thanks to vitamin D, the macrophage increases phagosome maturation and the production of antimicrobial peptides through the maximal regulation of the hCAP-18 gene encoding for cathelicidin antimicrobial peptide which activates, in turn, the transcription of autophagy-related genes (20). The 6 kDa early secretory antigenic target (ESAT-6) protein family secretion (ESX) system is a sophisticated secretion system that Mtb uses to export proteins with immune-escaping activity. So that while the IFN-γ axis is struggling against the ESX-system to enhance phagolysosmal activity, vitamin D deficiency abets the Mtb replication (21).
Nitric oxide (NO) within macrophages plays a less important role in humans than that one observed in animal models (19). Although, in humans too, reactive oxygen species (ROS) play a well-documented role in the immune response to Mtb as highlighted by the discovery of TB susceptibility in patients displaying mutations in a catalytic subunit of NADPH-oxidase 2 involved in ROS production on phagolysosomal membrane (22, 23). Moreover, it is demonstrated that Mtb affects NADPH-oxidase activity through nucleoside diphosphate kinase (Npk) interaction with small GTPases involved in NADPH-oxidase assembly and functioning (24).
The fight unfolds inside the phagosome of the MΦ between the cell and the Mtb with metals as a battlefield of sorts (25). The MΦ delivers an overload of copper and zinc, which are toxic to Mtb at high concentrations. Mtb deploys a series of protection mechanisms that include controlling the capture of such metals, oxidation, and an increase in efflux (25). The up-regulation of ctpC gene encoding for the P-type ATPase which regulates the intra-bacterial levels of Zinc is a clear example of how Mtb manages to prevent heavy metal poisoning (26). As a counter-move, the MΦ then attempts to block the arrival of nutrients to the Mtb such as iron and manganese (25).
Neutrophil granulocytes are the most widely present cell population within BAL and sputum in patients with active TB (27). There is evidence of their role as defense mechanisms against Mtb. In particular, there is a clear inverse correlation between the number of neutrophilic granulocytes in the peripheral blood and the hazard of developing TB after contact with an infectious subject. Antimicrobial peptides and apoptotic neutrophils are phagocytized by MΦ and carry out an effective activity against Mtb inside these cells (28). This is possible thanks to the fusion, within the MΦ, of neutrophil granules with phagosomes containing Mtb (29). Furthermore, ETosis, extracellular traps (ET) formation, is a type of cell death that differently from apoptosis is characterized by DNA release, consequent MΦ activation and the formation of a DNA scaffold that incorporates pathogens and exposes them to antimicrobial molecules (64). The formation of neutrophils ETs, thus constitutes an improved killing strategy and a synergic alliance between phagocytes.
Moreover, as with many immune mechanisms, neutrophils do not only play a positive role, but can eventually constitute a negative element, causing tissue damage through production and subsequent release of their antimicrobial products (27). To this phenomenon, it must be added the potentially negative interaction with lymphocytes. Neutrophils express on their cell membrane the ligand 1 of cell death (programmed death ligand 1 or PD-L1), which interacts with the lymphocyte receptor for programmed death (programmed death receptor or PD-1), and determines, in the course of chronic infections, the loss of function and finally the death of lymphocytes (30).
Neutrophils with expressed PD-L1 are present in high proportion in patients with ATB.
DCs are functionally located in the middle between innate and adaptive immunity. These cells play a fundamental role in the immune defense system due to antigen presentation, co-stimulating activity and the large cytokine production capacity with activity on the lymphocytes cluster of differentiation (CD) 4 (32). DCs role in TB immunity is controversial. Present evidence is not sufficient to establish whether these cells strengthen cellular immunity or if their manipulation by the Mtb can be used as a tool to diminish specific T-cell response (31). DCs soon become a niche for the Mtb. CD209, also called DC-specific intercellular adhesion molecule 3-grabbing non-integrin receptor (DC-SIGN), represents the gateway of Mtb into the DC (31). CD209 is, under normal conditions, a receptor for CD54, the intercellular adhesion molecule 1 (ICAM1) present on endothelial cells where it favors DCs migration. CD209 is coupled with the lipoarabinomannan mannose (ManLAM) of the Mtb that penetrates into the cell. This penetration leads to a disruption of DCs activity by prompting the production of interleukin (IL)-10 and reducing the production of IL-12, thus causing a suppression of T lymphocytes activity (33, 34). The manipulation of the maturation of the DCs probably represents one of the winning strategies of Mbt that, by restraining the activity of DCs and, consequently, of T lymphocytes, allows the Mtb, whose speed of growth is relatively slow, to efficiently establish a bridgehead in the airways (35). Based on the above mentioned mechanism DC-SIGN has recently been proposed as a potential target for a vaccine purpose eventually able to enhance immunity against Mtb (36). On the other side, DC-SIGN may prevent tissue pathology by maintaining a balanced inflammatory state and thus promoting host protection (37).
It is certain that NK cells enter the immunological circuit of Mtb infection both in their CD56 diminished phenotype (preferential cytotoxic activity) and in CD56 bright phenotype (preferential cytokines secreting activity) (38). In several studies the percentage representation of NK cell is augmented in the peripheral blood of patients with ATB (65). There is a direct relationship between NK cell representation, clinical condition and response to therapy (38, 65). Nonetheless, it has not yet been ascertained exactly what the cause and the consequence is (38).
Several components of the Mbt wall are recognized and bound by the NKp44 receptor of NK cells (39). In addition, Mtb-infected NKs lyse and stimulate MΦs to produce IFN-γ and IL-22, which increase phagolysosomal fusion thus inhibiting Mtb replication and stimulate the production of additional IFN-γ by CD8+ lymphocytes. This effect is mediated by the IL-15 and IL-18 production by an infected MΦ. As a further infection control mechanism DCs favor the development of T lymphocytes with γδ receptor through TNF-α and IL-12 production (40).
The role of mast cells in Mtb infection is not well-known in humans (41). In mice, mast cells capture Mtb via CD48 and internalize it. This process ensues the development of a cytokine cascade, some of them with protective roles, including IL-12, IL-13, IL-6, CXLL2, CCL7, CCL2, TNF-α, and consequent neutrophils recall in the site of infection (41). Histamine's role is ambivalent in terms of Mtb clearance as on one hand it augments lung neutrophilia but on the other it seems to impair the efficient production of a T helper 1 (Th1) response (42). The presence of mast cell ETs containing Mtb in humans has not been proved. However, mast cells enclose a large number of mediators known to take part in the process (41).
The role of the complement cascade on the progression of the infection and Mtb disease is almost unknown (44). It is likely that the C5 and C7 components play a defensive role. However, it has been observed that a high expression of C1q correlates with a worse clinical condition, so as to be a marker between latent TB and active TB but still with unclear significance in terms of pathogenesis (43, 44).
The immune response of T lymphocytes begins at the moment that Mtb spreads inside the lymph nodes but its arousal lays in the early activation of the innate immune system. Inside the lymph nodes, T lymphocytes undergo a process of activation and expansion of the specific populations for the Mtb antigens. However, at this point, the largest part is done and the infection is now established. Cellular immune response can be evidenced 2–6 weeks after Mtb infection by the development of a delayed hypersensitivity response to intradermal injected tuberculin (DHT) or purified protein derivative. It is important to underline that protective response to TB does not relate with DHT positivity and disease can occur in those who mount adequate DHT response (66) (Table 1).
The in vivo human model of HIV-infected CD4+-depleted patients is the most striking evidence of the pivotal role of these cells in TB immunity. The process of maturation of the phagosome of MΦ is facilitated and increased by IFN-γ, the production of which is mostly dependent on the T lymphocytes CD4+ with a minor support of lymphocytes CD8+ and T lymphocytes with γδ receptor (45). Animal models of knockout mice for IFN-γ clearly show that these animals suffer a very severe course of Mtb infection exactly as it happens in humans with MSMD. It is well known that patients with mutations in genes encoding IFN-γ or its receptors undergo disseminated infection by BCG or other non-tuberculous components of the mycobacteria genus (12). IFN-γ production is modest in patients with active TB, but recovers with antitubercular treatment without reaching levels similar to those of uninfected subjects.
The optimal production of IFN-γ, as well as that of IL-17 (67), is linked to an equally optimal cooperation between DCs and T lymphocytes CD4+. In its defensive strategy, Mtb markedly interferes in the CD40-CD40 ligand binding, that is essential for the cooperation between both cell lines (46). The importance of IFN-γ production by CD4+ cells is particularly relevant at the early stages of Mtb infection as it is demonstrated that adequate IFN-γ levels can be obtained with 3 weeks of delay even in CD4-disrupted mice thanks to the compensation offered by other cell types like CD8+ (68).
Moreover, IFN-γ cannot control infection alone and it requires the association of other molecules such as IL-6, IL-1 and the TNF-α. The chemokines CCL5, CCL9, CXCL10, and CCL2 attract immunity cells at the site of infection and their production is stimulated by TNF-α and boosts the production of NO by MΦ (47).
Several studies, both in adult and pediatric patients, have demonstrated CD4+ percentage and absolute value reduction in the peripheral blood of patients with ATB suggesting both an augmented pooling in the site of infection but also eventually a primary role of TB in immune modifications related with the severity of infection (65).
A portion of T lymphocytes are Foxp3+ and perform a control function over the activity of other T lymphocytes in fact, they are defined as T regulators (Treg). It is only on a hypothetical level that we can imagine any positive role of this cell line on the disease progression limiting tissue damage by other immune cells; however, it has been ascertained that, by restraining the response of the T lymphocytes, the Tregs favor the infection development and persistence (48). Similarly, T lymphocytes CD4+ may deal more damage, or at least become irrelevant, rather than hinder the progress of the infection (49).
For a long time, it was considered that, unlike T lymphocytes CD4+, T lymphocytes CD8+ had no role in controlling the infection and Mtb disease. This concept stemmed from the modest availability of human models with T lymphocyte CD8+ defect, unlike the large human model of HIV infection. An activity against Mtb is conceivable considering that T lymphocytes CD8+ recognize Mtb antigens through class I molecules of the major histocompatibility complex (MHC), and produce IL-2, IFN-γ and TNF-α, which have a well-known role in controlling Mtb. Furthermore, T lymphocytes CD8+ exert a cytolytic action against Mtb by means of perforin and granulysin, albeit not by Fas (CD95) -Fas ligand (50, 51) interaction. This direct cell-to-cell contact determines the apoptosis of the Mtb-infected cell (especially MΦ) depriving Mtb from its natural growth environment and at the same time reducing its viability by unknown mechanism (52). On the other hand, lymphocytes CD8+ produce IL-10 and TGF-β which instead favor the development of the Mtb infection.
The role of humoral adaptive immunity in TB is extremely uncertain (53, 54). Complement-mediated opsonization does not alter Mtb survival. High levels of antibody titers correlate with more serious conditions of infection and disease, and passive immunization with antibodies does not confer protection (55). Patients with a defective antibody-production mechanism and/or B lymphocyte defect are not particularly at risk of TB infection.
The role of the crystallizable fragment or Fc in the constant portion of the immunoglobulin, which binds and activates various cell lines present in the granuloma (NK cells, monocytes, neutrophils) the low-affinity FcγRIIIb receptor and the high affinity FcγRIIa receptor have shown different functional profiles and glycosylation patterns in subjects with ATB rather than LTBI (54, 56).
The loss of FcγRIIIb activity and the increase of FcγRIIa-mediated inhibitory function (which correlates with a high IL10 production) are associated with a worse clinical profile and can distinguish ATB from LTBI and suggests a role of antibodies in the augmented phagolysosomal maturation and Mtb killing observed in LTBI patients (56).
Following the development of adaptive immunity, a complex and well-coordinated mechanism is established between both immunity mechanisms, i.e., innate and adaptive, which seal the Mtb inside granulomas (5, 58, 59). This mechanism develops in at least 90% of the infected subjects and leads to LTBI. During latent TB, which would be better described as non-replicating-persistence phase (in fact, Mtb works perfectly albeit in a different way than during active TB), the subject is generally positive for the tuberculin skin test and for the IGRA (69). Latent TB becomes active when, for the most various reasons, a condition of immunodepression develops. At this stage, the subject may become capable of transmitting the infection because the granuloma opens in the bronchial lumen and Mtb are expelled when coughing. At the beginning of the infection, Mtb demands an environment with inflammatory traits to develop the granuloma; subsequently, however, its survival is linked to an environment lacking or with low inflammation. This switch is caused by ESAT-6 (60), a well-known Mtb virulence factor involved in the ESX secretion system, to which it gives its name. ESAT-6 causes the transformation of MΦ from phenotype M1, which produces IL-6, IL-12 and TNF-α, into MΦ with phenotype M2, which is capable of stimulating production of IL-10 (60, 61, 70). As currently known, IL-6 and TNF-α favor inflammation, whilst IL-10 curbs it. Accordingly, the formation of the granuloma is triggered by the MΦ and then develops with multi-nucleated giant cells and MΦ with abundant presence of intracytoplasmic lipids, which lend these cells their frothy appearance. Around these cells, there is a ring of T lymphocytes although B lymphocytes, neutrophils and dendritic cells (CD) also participate in the formation of granuloma (4).
Inside the granuloma, cholesterol—and not glucose or glycerol—is the only carbon source. This leads to a lack of carbon and nutrients, hypoxia and a high concentration of nitric oxide (NO). The significance of cholesterol in the survival of Mtb inside the granuloma is evidenced by the negative role that statins play against Mtb (61, 70).
The debate remains open on whether the granuloma is purely protective for the host or if it promotes disease progression and tissue damage (4). This uncertainty depends on the extreme heterogeneity detected in granuloma morphology at the different stages of disease, on the role of inflammation, hypoxia and differential Mtb gene expression and lipid metabolism manipulation inside the granulomas of ATB and LTBI patients (62). The most likely answer is that an homeostatic interaction establishes and the granuloma becomes a well-suited shelter for both Mtb long-term survival and host protection (57).
The different cell lines of innate and adaptive immunity come into play at different times in the battle against Mtb in a clash in which the genetic susceptibility of the host and the virulence of the pathogen play decisive roles for the final outcome. The success of Mtb over thousands of years against man arises from its extraordinary ability to subvert the mechanisms that should eliminate it in the MΦ from the infection onset. At the onset of the infection, Mtb manages to perforate the phagosome in the MΦ through the ESX system and, therefore, to block its maturation via Npk, which inhibits lysosomal traffic and NADPH-oxidase activity (31). With various mechanisms, some of which also operate at the level of macrophage DNA, the Mbt prevents the activation of pathogen destruction systems, which are implemented through autophagy. The Mtb DNA manages to prevent the activation of the AIM2 inflammasome thus hindering the synthesis of IL-1β and IL-18 (31). Under normal conditions, IFN-γ stimulates the expression of MHC class II molecules on the MΦ. But Mtb, thanks to the prolonged activation of TLR2, succeeds in suppressing this mechanism. Even in cells that already express class II MHC molecules, Mtb manages to block the presentation of antigens by the action on ESCRT (endosomal sorting complexes required for transport) of its EsxG·EsxH protein (31). The “great manipulator” also interferes with the functions of DCs, neutrophils and all other components of the immune system.
In conclusion, having to deal with a micro-organism of great evasive abilities, immune mechanisms have only one way to go: to focus on a very rapid response at the onset of the infection.
Paraphrasing a famous aphorism by General Erwin Rommel about amphibious battles that is well-suited to TB, victory or defeat against Mtb is decided in the first moments of the infection (5). Exactly as it happened on the first day of the amphibious assault, the day that General Erwin Rommel defined as “the longest day.” When it comes to implementing new prevention and therapeutic approaches, a clear understanding of the interplay between the immune system and Mtb at a molecular level is the only way to unravel this millenary skein.
MdM wrote the main body of this minireview. LL, LG, and EC contributed with literature search and revisions.
This work received no fundings. The payment of fees for open access publication will be supported by the Dipartimento di Medicina Sperimentale e Clinica of the University of Florence.
The authors declare that the research was conducted in the absence of any commercial or financial relationships that could be construed as a potential conflict of interest.
The handling editor declared a past co-authorship with several of the authors MdM, LG, and EC.
1. WHO. Global Tuberculosis Report. (2018). Available online at: http://www.who.int/tb/publications/global_report/en/ (accessed June 11, 2019).
2. Tellier R, Li Y, Cowling BJ, Tang JW. Recognition of aerosol transmission of infectious agents: a commentary. BMC Infect Dis. (2019) 19:101. doi: 10.1186/s12879-019-3707-y
3. Middleton AM, Chadwick MV, Nicholson AG, Dewar A, Groger RK, Brown EJ, et al. Interaction of Mycobacterium tuberculosis with human respiratory mucosa. Tuberculosis. (2002) 82:69–78. doi: 10.1054/tube.2002.0324
4. O'Garra A, Redford PS, McNab FW, Bloom CI, Wilkinson RJ, Berry MPR. The immune response in tuberculosis. Annu Rev Immunol. (2013) 31:475–527. doi: 10.1146/annurev-immunol-032712-095939
5. de Martino M, Galli L, Chiappini E. Reflections on the immunology of tuberculosis: will we ever unravel the skein? BMC Infect Dis. (2014) 14 (Suppl. 1):S1. doi: 10.1186/1471-2334-14-S1-S1
6. Ringshausen FC, Nienhaus A, Schablon A, Schlösser S, Schultze-Werninghaus G, Rohde G. Predictors of persistently positive Mycobacterium-tuberculosis-specific interferon-gamma responses in the serial testing of health care workers. BMC Infect Dis. (2010) 10:220. doi: 10.1186/1471-2334-10-220
7. Casanova J-L, Abel L. Genetic dissection of immunity to mycobacteria: the human model. Annu Rev Immunol. (2002) 20:581–620. doi: 10.1146/annurev.immunol.20.081501.125851
8. Cobat A, Gallant CJ, Simkin L, Black GF, Stanley K, Hughes J, et al. Two loci control tuberculin skin test reactivity in an area hyperendemic for tuberculosis. J Exp Med. (2009) 206:2583–91. doi: 10.1084/jem.20090892
9. Stein CM, Zalwango S, Malone LL, Won S, Mayanja-Kizza H, Mugerwa RD, et al. Genome scan of M. tuberculosis infection and disease in Ugandans. PLoS ONE. (2008) 3:e4094. doi: 10.1371/journal.pone.0004094
10. Harishankar M, Selvaraj P, Bethunaickan R. Influence of genetic polymorphism towards pulmonary tuberculosis susceptibility. Front Med. (2018) 5:213. doi: 10.3389/fmed.2018.00213
11. van Tong H, Velavan TP, Thye T, Meyer CG. Human genetic factors in tuberculosis: an update. Trop Med Int Health. (2017) 22:1063–71. doi: 10.1111/tmi.12923
12. Rosain J, Kong X-F, Martinez-Barricarte R, Oleaga-Quintas C, Ramirez-Alejo N, Markle J, et al. Mendelian susceptibility to mycobacterial disease: 2014–2018 update. Immunol Cell Biol. (2019) 97:360–7. doi: 10.1111/imcb.12210
13. Berry MPR, Graham CM, McNab FW, Xu Z, Bloch SAA, Oni T, et al. An interferon-inducible neutrophil-driven blood transcriptional signature in human tuberculosis. Nature. (2010) 466:973–7. doi: 10.1038/nature09247
14. Blankley S, Graham CM, Turner J, Berry MPR, Bloom CI, Xu Z, et al. The transcriptional signature of active tuberculosis reflects symptom status in extra-pulmonary and pulmonary tuberculosis. PLoS ONE. (2016) 11:e0162220. doi: 10.1371/journal.pone.0162220
15. Forrellad MA, Klepp LI, Gioffré A, Sabio y García J, Morbidoni HR, Santangelo M de la P, et al. Virulence factors of the Mycobacterium tuberculosis complex. Virulence. (2013) 4:3–66. doi: 10.4161/viru.22329
16. Li Y, Wang Y, Liu X. The role of airway epithelial cells in response to mycobacteria infection. Clin Dev Immunol. (2012) 2012:791392. doi: 10.1155/2012/791392
17. Harriff MJ, Cansler ME, Toren KG, Canfield ET, Kwak S, Gold MC, et al. Human lung epithelial cells contain Mycobacterium tuberculosis in a late endosomal vacuole and are efficiently recognized by CD8+ T cells. PLoS ONE. (2014) 9:e97515. doi: 10.1371/journal.pone.0097515
18. Queval CJ, Brosch R, Simeone R. The macrophage: a disputed fortress in the battle against Mycobacterium tuberculosis. Front Microbiol. (2017) 8:2284. doi: 10.3389/fmicb.2017.02284
19. Lerner TR, Borel S, Gutierrez MG. The innate immune response in human tuberculosis. Cell Microbiol. (2015) 17:1277–85. doi: 10.1111/cmi.12480
20. Yuk J-M, Shin D-M, Lee H-M, Yang C-S, Jin HS, Kim K-K, et al. Vitamin D3 induces autophagy in human monocytes/macrophages via cathelicidin. Cell Host Microbe. (2009) 6:231–43. doi: 10.1016/j.chom.2009.08.004
21. Gröschel MI, Sayes F, Simeone R, Majlessi L, Brosch R. ESX secretion systems: mycobacterial evolution to counter host immunity. Nat Rev Microbiol. (2016) 14:677–91. doi: 10.1038/nrmicro.2016.131
22. Bustamante J, Arias AA, Vogt G, Picard C, Galicia LB, Prando C, et al. Germline CYBB mutations that selectively affect macrophages in kindreds with X-linked predisposition to tuberculous mycobacterial disease. Nat Immunol. (2011) 12:213–21. doi: 10.1038/ni.1992
23. Sia JK, Georgieva M, Rengarajan J. Innate immune defenses in human tuberculosis: an overview of the interactions between Mycobacterium tuberculosis and innate immune cells. J Immunol Res. (2015) 2015:747543. doi: 10.1155/2015/747543
24. Sun J, Singh V, Lau A, Stokes RW, Obregón-Henao A, Orme IM, et al. Mycobacterium tuberculosis nucleoside diphosphate kinase inactivates small GTPases leading to evasion of innate immunity. PLoS Pathog. (2013) 9:e1003499. doi: 10.1371/journal.ppat.1003499
25. Neyrolles O, Wolschendorf F, Mitra A, Niederweis M. Mycobacteria, metals, and the macrophage. Immunol Rev. (2015) 264:249–63. doi: 10.1111/imr.12265
26. Botella H, Peyron P, Levillain F, Poincloux R, Poquet Y, Brandli I, et al. Mycobacterial p(1)-type ATPases mediate resistance to zinc poisoning in human macrophages. Cell Host Microbe. (2011) 10:248–59. doi: 10.1016/j.chom.2011.08.006
27. Kroon EE, Coussens AK, Kinnear C, Orlova M, Möller M, Seeger A, et al. Neutrophils: innate effectors of TB resistance? Front Immunol. (2018) 9:2637.doi: 10.3389/fimmu.2018.02637
28. Lowe DM, Demaret J, Bangani N, Nakiwala JK, Goliath R, Wilkinson KA, et al. Differential effect of viable versus necrotic neutrophils on Mycobacterium tuberculosis growth and cytokine induction in whole blood. Front Immunol. (2018) 9:903. doi: 10.3389/fimmu.2018.00903
29. Tan BH, Meinken C, Bastian M, Bruns H, Legaspi A, Ochoa MT, et al. Macrophages acquire neutrophil granules for antimicrobial activity against intracellular pathogens. J Immunol. (2006) 177:1864–71. doi: 10.4049/jimmunol.177.3.1864
30. Zhang Y, Zhou Y, Lou J, Li J, Bo L, Zhu K, et al. PD-L1 blockade improves survival in experimental sepsis by inhibiting lymphocyte apoptosis and reversing monocyte dysfunction. Crit Care. (2010) 14:R220.doi: 10.1186/cc9354
31. Mihret A. The role of dendritic cells in Mycobacterium tuberculosis infection. Virulence. (2012) 3:654–9. doi: 10.4161/viru.22586
32. Khan N, Vidyarthi A, Pahari S, Agrewala JN. Distinct strategies employed by dendritic cells and macrophages in restricting Mycobacterium tuberculosis infection: different philosophies but same desire. Int Rev Immunol. (2016) 35:386–98. doi: 10.3109/08830185.2015.1015718
33. Wu T, Guo S, Wang J, Li L, Xu L, Liu P, et al. Interaction between mannosylated lipoarabinomannan and dendritic cell-specific intercellular adhesion molecule-3 grabbing nonintegrin influences dendritic cells maturation and T cell immunity. Cell Immunol. (2011) 272:94–101. doi: 10.1016/j.cellimm.2011.09.001
34. Balboa L, Romero MM, Yokobori N, Schierloh P, Geffner L, Basile JI, et al. Mycobacterium tuberculosis impairs dendritic cell response by altering CD1b, DC-SIGN and MR profile. Immunol Cell Biol. (2010) 88:716–26. doi: 10.1038/icb.2010.22
35. Georgieva M, Sia JK, Bizzell E, Madan-Lala R, Rengarajan J. Mycobacterium tuberculosis GroEL2 modulates dendritic cell responses. Infect Immun. (2018) 86:e00387-17. doi: 10.1128/IAI.00387-17
36. Velasquez LN, Stüve P, Gentilini MV, Swallow M, Bartel J, Lycke NY, et al. Targeting Mycobacterium tuberculosis antigens to dendritic cells via the DC-specific-ICAM3-grabbing-nonintegrin receptor induces strong T-helper 1 immune responses. Front Immunol. (2018) 9:471. doi: 10.3389/fimmu.2018.00471
37. Ehlers S. DC-SIGN and mannosylated surface structures of Mycobacterium tuberculosis: a deceptive liaison. Eur J Cell Biol. (2010) 89:95–101. doi: 10.1016/j.ejcb.2009.10.004
38. Esin S, Batoni G. Natural killer cells: a coherent model for their functional role in Mycobacterium tuberculosis infection. J Innate Immun. (2015) 7:11–24. doi: 10.1159/000363321
39. Arora P, Foster EL, Porcelli SA. CD1d and natural killer T cells in immunity to Mycobacterium tuberculosis. Adv Exp Med Biol. (2013) 783:199–223. doi: 10.1007/978-1-4614-6111-1_11
40. Zhang R, Zheng X, Li B, Wei H, Tian Z. Human NK cells positively regulate gammadelta T cells in response to Mycobacterium tuberculosis. J Immunol. (2006) 176:2610–6. doi: 10.4049/jimmunol.176.4.2610
41. Garcia-Rodriguez KM, Goenka A, Alonso-Rasgado MT, Hernández-Pando R, Bulfone-Paus S. The role of mast cells in tuberculosis: orchestrating innate immune crosstalk? Front Immunol. (2017) 8:1290. doi: 10.3389/fimmu.2017.01290
42. Carlos D, Fremond C, Samarina A, Vasseur V, Maillet I, Ramos SG, et al. Histamine plays an essential regulatory role in lung inflammation and protective immunity in the acute phase of Mycobacterium tuberculosis infection. Infect Immun. (2009) 77:5359–68. doi: 10.1128/IAI.01497-08
43. Lubbers R, Sutherland JS, Goletti D, de Paus RA, van Moorsel CHM, Veltkamp M, et al. Complement component C1q as serum biomarker to detect active tuberculosis. Front Immunol. (2018) 9:2427. doi: 10.3389/fimmu.2018.02427
44. Cai Y, Yang Q, Tang Y, Zhang M, Liu H, Zhang G, et al. Increased complement C1q level marks active disease in human tuberculosis. PLoS ONE. (2014) 9:e92340. doi: 10.1371/journal.pone.0092340
45. Cooper AM. Cell-mediated immune responses in tuberculosis. Annu Rev Immunol. (2009) 27:393–422. doi: 10.1146/annurev.immunol.021908.132703
46. Sia JK, Bizzell E, Madan-Lala R, Rengarajan J. Engaging the CD40-CD40L pathway augments T-helper cell responses and improves control of Mycobacterium tuberculosis infection. PLoS Pathog. (2017) 13:e1006530. doi: 10.1371/journal.ppat.1006530
47. Domingo-Gonzalez R, Prince O, Cooper A, Khader SA. Cytokines and chemokines in Mycobacterium tuberculosis infection. Microbiol Spectr. (2016) 4:TBTB2-0018-2016. doi: 10.1128/microbiolspec.TBTB2-0018-2016
48. Parkash O, Agrawal S, Madhan Kumar M. T regulatory cells: Achilles' heel of Mycobacterium tuberculosis infection? Immunol Res. (2015) 62:386–98. doi: 10.1007/s12026-015-8654-0
49. Sallin MA, Sakai S, Kauffman KD, Young HA, Zhu J, Barber DL. Th1 differentiation drives the accumulation of intravascular, non-protective CD4 T cells during tuberculosis. Cell Rep. (2017) 18:3091–104. doi: 10.1016/j.celrep.2017.03.007
50. Lin PL, Flynn JL. CD8 T cells and Mycobacterium tuberculosis infection. Semin Immunopathol. (2015) 37:239–49. doi: 10.1007/s00281-015-0490-8
51. Canaday DH, Wilkinson RJ, Li Q, Harding CV, Silver RF, Boom WH. CD4+ and CD8+ T cells kill intracellular Mycobacterium tuberculosis by a perforin and Fas/Fas ligand-independent mechanism. J Immunol. (2001) 167:2734–42. doi: 10.4049/jimmunol.167.5.2734
52. Oddo M, Renno T, Attinger A, Bakker T, MacDonald HR, Meylan PR. Fas ligand-induced apoptosis of infected human macrophages reduces the viability of intracellular Mycobacterium tuberculosis. J Immunol. (1998) 160:5448–54.
53. Kozakiewicz L, Phuah J, Flynn J, Chan J. The role of B cells and humoral immunity in Mycobacterium tuberculosis infection. Adv Exp Med Biol. (2013) 783:225–50. doi: 10.1007/978-1-4614-6111-1_12
54. Jacobs AJ, Mongkolsapaya J, Screaton GR, McShane H, Wilkinson RJ. Antibodies and tuberculosis. Tuberculosis. (2016) 101:102–3. doi: 10.1016/j.tube.2016.08.001
55. Glatman-Freedman A, Casadevall A. Serum therapy for tuberculosis revisited: reappraisal of the role of antibody-mediated immunity against Mycobacterium tuberculosis. Clin Microbiol Rev. (1998) 11:514–32.
56. Lu LL, Chung AW, Rosebrock TR, Ghebremichael M, Yu WH, Grace PS, et al. A functional role for antibodies in tuberculosis. Cell. (2016) 167:433-443.e14. doi: 10.1016/j.cell.2016.08.072
57. Ramakrishnan L. Revisiting the role of the granuloma in tuberculosis. Nat Rev Immunol. (2012) 12:352–66. doi: 10.1038/nri3211
58. Russell DG. Who puts the tubercle in tuberculosis? Nat Rev Microbiol. (2007) 5:39–47. doi: 10.1038/nrmicro1538
59. Reece ST, Kaufmann SHE. Floating between the poles of pathology and protection: can we pin down the granuloma in tuberculosis? Curr Opin Microbiol. (2012) 15:63–70. doi: 10.1016/j.mib.2011.10.006
60. Refai A, Gritli S, Barbouche M-R, Essafi M. Mycobacterium tuberculosis virulent factor ESAT-6 drives macrophage differentiation toward the pro-inflammatory M1 phenotype and subsequently switches it to the anti-inflammatory M2 phenotype. Front Cell Infect Microbiol. (2018) 8:327. doi: 10.3389/fcimb.2018.00327
61. Martinot AJ. Microbial offense vs host defense: who controls the TB granuloma? Vet Pathol. (2018) 55:14–26. doi: 10.1177/0300985817705177
62. Russell DG, Cardona P-J, Kim M-J, Allain S, Altare F. Foamy macrophages and the progression of the human tuberculosis granuloma. Nat Immunol. (2009) 10:943–8.
63. Gou X, Pan L, Tang F, Gao H, Xiao D. The association between vitamin D status and tuberculosis in children: a meta-analysis. Medicine. (2018) 97:e12179. doi: 10.1097/MD.0000000000012179
64. Guimarães-Costa AB, Nascimento MTC, Wardini AB, Pinto-da-Silva LH, Saraiva EM. ETosis: a microbicidal mechanism beyond cell death. J Parasitol Res. (2012) 2012:929743. doi: 10.1155/2012/929743
65. Venturini E, Lodi L, Francolino I, Ricci S, Chiappini E, de Martino M, et al. CD3, CD4, CD8, CD19 and CD16/CD56 positive cells in tuberculosis infection and disease: peculiar features in children. Int J Immunopathol Pharmacol. (2019) 33:2058738419840241. doi: 10.1177/2058738419840241
66. McKinney J, Jacobs W, Bloom B. Persisting problems in tuberculosis. In: Krause RM, editor. Emerging Infections. San Diego, CA: Academic Press (1998). p. 51–146.
67. Monin L, Griffiths KL, Slight S, Lin Y, Rangel-Moreno J, Khader SA. Immune requirements for protective Th17 recall responses to Mycobacterium tuberculosis challenge. Mucosal Immunol. (2015) 8:1099–109. doi: 10.1038/mi.2014.136
68. Caruso AM, Serbina N, Klein E, Triebold K, Bloom BR, Flynn JL. Mice deficient in CD4 T cells have only transiently diminished levels of IFN-gamma, yet succumb to tuberculosis. J Immunol. (1999) 162:5407–16.
69. Wayne LG. Dynamics of submerged growth of Mycobacterium tuberculosis under aerobic and microaerophilic conditions. Am Rev Respir Dis. (1976) 114:807–11.
Keywords: Mycobacterium tuberculosis, tuberculosis, children, immune response, immunity, macrophage, adaptive immunity, granuloma
Citation: de Martino M, Lodi L, Galli L and Chiappini E (2019) Immune Response to Mycobacterium tuberculosis: A Narrative Review. Front. Pediatr. 7:350. doi: 10.3389/fped.2019.00350
Received: 07 April 2019; Accepted: 06 August 2019;
Published: 27 August 2019.
Edited by:
Gian Luigi Marseglia, Policlinico San Matteo Fondazione (IRCCS), ItalyReviewed by:
Silvia Garazzino, University Hospital of the City of Health and Science of Turin, ItalyCopyright © 2019 de Martino, Lodi, Galli and Chiappini. This is an open-access article distributed under the terms of the Creative Commons Attribution License (CC BY). The use, distribution or reproduction in other forums is permitted, provided the original author(s) and the copyright owner(s) are credited and that the original publication in this journal is cited, in accordance with accepted academic practice. No use, distribution or reproduction is permitted which does not comply with these terms.
*Correspondence: Elena Chiappini, ZWxlbmEuY2hpYXBwaW5pQHVuaWZpLml0
Disclaimer: All claims expressed in this article are solely those of the authors and do not necessarily represent those of their affiliated organizations, or those of the publisher, the editors and the reviewers. Any product that may be evaluated in this article or claim that may be made by its manufacturer is not guaranteed or endorsed by the publisher.
Research integrity at Frontiers
Learn more about the work of our research integrity team to safeguard the quality of each article we publish.