- 1Intensive Care and Department of Pediatric Surgery, Erasmus University Medical Center – Sophia Children's Hospital, Rotterdam, Netherlands
- 2Department of Intensive Care, Erasmus University Medical Center, Rotterdam, Netherlands
- 3Department of Translational Physiology, Amsterdam University Medical Center, Amsterdam, Netherlands
Assuring adequate tissue oxygenation in the critically ill, but still developing child is challenging. Conventional hemodynamic monitoring techniques fall short in assessing tissue oxygenation as these are directed at the macrocirculation and indirect surrogates of tissue oxygenation. The introduction of handheld vital microscopy (HVM) has allowed for the direct visualization of the microcirculation and with this has offered insight into tissue oxygenation on a microcirculatory level. Since its introduction, technical improvements have been made to HVM, to both hardware and software, and guidelines have been developed through expert consensus on image assessment and analysis. Using HVM, the microcirculation of the skin, the buccal mucosa, and the sublingual mucosa of healthy and (critically) ill neonates and children have been visualized and investigated. Yet, integration of HVM in hemodynamic monitoring has been limited due to technical shortcomings. Only superficial microcirculatory beds can be visualized, inter-observer and intra-observer variabilities are not accounted for and image analysis happens offline and is semi-automated and time-consuming. More importantly, patients need to be cooperative or fully sedated to prevent pressure and movement artifacts, which is often not the case in children. Despite these shortcomings, observational research with HVM in neonates and children has revealed the following: (1) age-related developmental changes in the microcirculation, (2) loss of hemodynamic coherence, i.e., microcirculatory disturbances in the presence of a normal macrocirculation and, (3) microcirculatory disturbances which were independently associated with increased mortality risk. Although these observations underline the importance of microcirculatory monitoring, several steps have to be taken before integration in the decision process during critical care can happen. These steps include technological innovations to ease the use of HVM in the pediatric age group, measuring additional functional parameters of microvascular blood flow and integrated automated analysis software. As a next step, reference values for microcirculatory parameters need to be established, while also accounting for developmental changes. Finally, studies on microcirculatory guided therapies are necessary to assess whether the integration of microcirculatory monitoring will actually improve patient outcome. Nevertheless, HVM remains a promising, non-invasive tool to help physicians assure tissue oxygenation in the critically ill child.
Introduction
Hemodynamic monitoring plays a pivotal role in assessing an existing imbalance between oxygen delivery and oxygen consumption and guiding therapy to recover such an imbalance in critically ill neonates and children. Conventional hemodynamic monitoring techniques are aimed at the macrocirculation, i.e., the heart and larger blood vessels, and therefore do not offer insight into oxygen delivery on a cellular level (1–3). A well-functioning macrocirculation alone is no guarantee for adequate oxygen delivery at the cellular level, as it remains unclear whether the microcirculation, comprising smaller arterioles, capillaries, and venules, performs equally well to provide sufficient oxygen delivery.
With the introduction of handheld vital microscopy (HVM) techniques almost 20 years ago, it became possible to assess the microcirculation in a non-invasive manner at the patients' bedside. Microcirculatory research since then has revealed loss of hemodynamic coherence, microcirculatory disturbances in the presence of normal macrocirculatory hemodynamics (4). Loss of hemodynamic coherence can occur either when the recovery of macrocirculatory hemodynamics is insufficient to also recover microcirculatory disturbances or when manipulation of macrocirculatory hemodynamics causes microcirculatory disturbances. In adults with septic shock, Hernandez et al. showed that dobutamine administration improved global hemodynamic parameters, while it failed to improve the sublingual microcirculation and other peripheral perfusion parameters (5). Comparable signs of loss of hemodynamic coherence have also been found in children. In neonates with congenital diaphragmatic hernia, catecholaminergic drugs improved macrocirculatory hemodynamic parameters including mean arterial pressure and heart rate, but could not recruit the microcirculation (6).
These found microcirculatory disturbances have shown to be associated with higher mortality risk (7–9). To illustrate, a study on a mixed group of critically ill adult patients showed the microcirculation to be an independent predictor of ICU mortality, even when corrected for the Acute Physiology, Age, Chronic Health Evaluation (APACHE) II score which includes macrocirculatory parameters (7). Similarly, the microcirculation of pediatric patients with septic shock on the first day could better predict mortality than the Pediatric Risk of Mortality (PRISM) score which also includes macrocirculatory parameters (8). Pediatric patients who underwent therapeutic hypothermia post cardiac arrest but did not survive also showed persistent microcirculatory disturbances, while global hemodynamics did not differ between survivors and non-survivors (9).
Despite the continuous improvement of and the extensive research with HVM, we are still a few steps away from routinely applying microcirculatory monitoring in clinical care. The latest expert consensus on the assessment of the sublingual microcirculation has included important research questions for future observational studies and studies for guiding interventions, but these questions were not specified for neonates and children (10).
This review provides an overview of the different generations of HVM and a summary of studies on the application of HVM in neonates and children. We also discuss important research objectives for future microcirculatory research in these populations and the issues that need to be addressed before clinical application of HVM and integration in current hemodynamic monitoring in neonatal and pediatric critical care is possible.
Microcirculatory Monitoring
Evolution of Handheld Vital Microscopy Techniques
Over the years, HVM has become an important tool for animal and human research on the microcirculation. Using HVM, microvascular beds of different types of mucosa and solid organ surfaces can be visualized directly up to a depth of ~1 mm, in real-time, and non-invasively at the patient's bedside. In neonates and children, microcirculatory imaging can be acquired from the buccal and sublingual mucosa (11, 12). In neonates, transcutaneous measurements (e.g., upper inner arm, axilla, ear conch, fossa triangularis) are also possible (13). Figure 1 shows examples of images of the buccal, sublingual, and cutaneous microcirculation assessed with HVM.
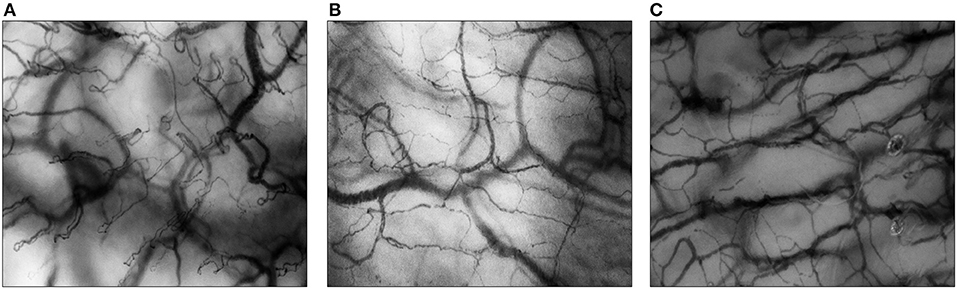
Figure 1. Microcirculatory imaging of different surfaces. All images are assessed with Cytocam-IDF imaging. (A) The buccal microcirculation of a neonatal patient; (B) the sublingual microcirculation of a pediatric patient; (C) the cutaneous microcirculation (upper inner arm) of a preterm neonate.
In the late nineties, Groner et al. introduced the first generation of HVM, the orthogonal polarization spectral (OPS) imaging device (14). The OPS imaging device illuminates tissue through a light source in a handheld probe. While some light is absorbed by hemoglobin, other light is reflected. By disposing of reflected light by the tissue and having scattered (depolarized) light pass through the system, high-contrast images can be acquired of flowing dark red blood cells (RBC) against a light gray background. In 2007, Goedhart et al. introduced the sidestream dark field (SDF) imaging device, a comparable handheld probe with an integrated pulsating green light source (15). The reflected green light is lead through the center of the probe with a magnifying lens system up to a computer to create images with higher contrast and better quality than that of its predecessor. Finally, in 2015 Aykut et al. introduced the third and latest generation microscope, the incident dark field illumination (IDF) imaging device (16). The device has a lightweight pen-like probe incorporated with IDF and higher resolution lenses. The lenses project images on to a computer with a better density sensor and improved control of illumination and image acquisition. These alterations result in a larger field of view and larger image size with higher resolution than those of its predecessors (17–20). The IDF imaging device provides images of higher quality and accuracy and therefore shows higher vessel densities (17). More details on the technical aspects of the currently available microscopes can be found in the review by Massey et al. (20).
Image Acquisition and Analysis
In 2007, De Backer et al. published the first expert consensus paper addressing the requirements for optimal image acquisition and the different parameters necessary for interpretation (21). More recently, Ince et al. published an updated consensus paper specifically discussing the assessment of the sublingual microcirculation in critically ill (adult) patients (10). Currently, there are no special considerations for assessment in pediatric and neonatal patients. Only a single manual for the assessment of microcirculatory imaging has been published, describing measurements of the cutaneous microcirculation in (pre)term neonates (22). Extensive training for both image acquisition and analysis by experienced users is necessary to assure adequate quality of images and reliability of results. Before image acquisition, a clean protective cap is placed on the probe. The surface area is cleaned from any saliva with gauze, if applicable, as excessive saliva can reduce the visibility of vessels and should therefore be removed wherever possible. For transcutaneous measurements, gel, oil or saline is applied on the tip of the probe. The probe is gently placed on the surface area. The focus is adjusted to the degree that single RBC's are visible in the capillaries. Artifacts caused by excessive saliva, air bubbles or pressure should be avoided. For a minimum of 4 s each, motion-free measurements are made of at least three different areas.
After image acquisition image analysis is possible through real-time visual evaluation, offline manual analysis, and offline semi-automated analysis. Massey et al. developed an image quality score to ensure that only imaging of sufficient quality is considered for analysis (23). The three clips with the best quality are selected for image analysis of a single time point. Image analysis provides several quantitative and qualitative parameters for microcirculatory function as described elsewhere and summarized below (10). The average of the three selected clips (of three different spots) is calculated to assess the value of a single time point.
Total vessel density (TVD, mm/mm2) quantifies the total vessel area visible in the frame. Before the semi-automated assessment of vessel density was possible, the Backer score (n/mm) was calculated as an estimation of TVD (24). Proportion of perfused vessels (PPV, %) gives the number of perfused vessels per total number of visible vessels in the frame. Perfused vessel density (PVD, mm/mm2), in former studies often referred to as functional vessel density (FVD, cm/cm2) or functional capillary density (FCD, cm/cm2) for small vessels, describes the functional vessel area visible in the frame, through the multiplication of TVD and PPV. TVD and PVD can be used to assess alterations in diffusive properties of the microcirculation as these parameters are surrogates for the distance oxygen has to cover from capillary to cell. As for flow properties, quality, heterogeneity, and velocity of flow can be determined. Microcirculatory flow index (MFI) is a semi-qualitative score to describe the quality of flow through the microvascular vessels. Figure 2 shows two examples of continuous flow and intermittent flow, respectively. The MFI can be assessed in real-time (25, 26). To account for variability between measured areas, the heterogeneity index (HI) can be calculated for PPV and MFI through the equation (highest value–lowest value)/mean value. HI can help determine the presence of abnormalities in flow distribution. The increase of heterogeneity of flow can be a sign of functional shunting. To assess the velocity of flow, RBC velocities can be measured using space-time diagrams (STD). Recently, Uz et al. developed a method to also count leukocytes and assess leukocyte velocity in microcirculatory imaging using STD (27). Fabian-Jessing et al. also developed a method for assessing and quantifying leukocyte rolling and adherence (28). Finally, to assess early markers of vascular wall damage endothelial glycocalyx layer dimensions can be estimated through the width of flowing RBC's (29).
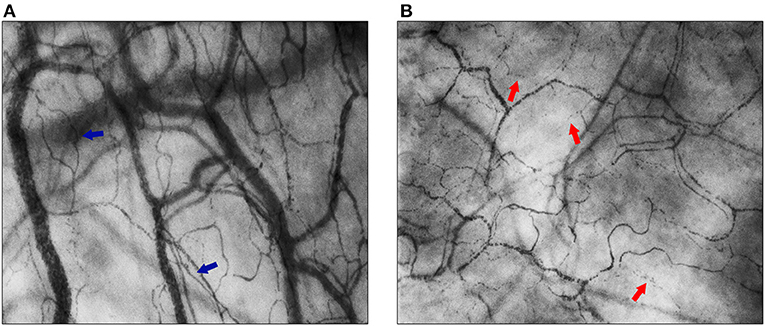
Figure 2. Flow quality of the sublingual microcirculation. In both images, the sublingual microcirculation of neonatal patients is visualized, assessed with Cytocam-IDF imaging. (A) Blue arrows point toward capillaries with normal flow (MFI = 3); (B) red arrows point toward capillaries with intermittent flow (MFI = 1).
Currently available analysis software is semi-automated and therefore time-consuming. Automated analysis software has been developed, but these are not yet validated for use in clinical practice (30–34). Currently, online automated analysis software is being developed to obtain the above mentioned and additional parameters more easily and rapidly.
Limitations
Despite technological advancements and the extensive application of HVM in research, there are still several limitations to their use. The microcirculation can only be visualized if the epithelial layer of the area of interest is thin. Also, pressure and movement artifacts are not rare. Although its use is validated in children and neonates, the acquisition is complicated as children need to be sufficiently sedated or fully cooperative to attain good quality imaging (13, 35, 36). Gonzalez et al. performed an observational study in the pediatric ICU, where sublingual microcirculatory monitoring was only possible in 17% of the admitted patients in the study period (37). These patients were sedated and/or more severely ill. As for microcirculatory measurements of the skin, these are performed in the first few weeks of life in both preterm and term neonates. Thickening of skin with age and the presence of hair interferes with optimal visualization of the cutaneous microcirculation. There is no exact limit for age for the assessment of the cutaneous microcirculation, as this has not been investigated. Van Elteren et al. has looked into the difference in transcutaneous microcirculation of preterm and term neonates and stated that depth of focus depended on postnatal age rather than gestational age (22). In both groups, it was more difficult to assess images on day 28. For image analysis, only offline semi-automated analysis software is currently available and existing automated software programs are not yet validated. Therefore actual bedside evaluation of the microcirculation is not possible, although MFI could be assessed at the patient's bedside through eyeballing (25, 26, 38). Because the semi-automated analysis is currently the gold standard for image analysis, inter-observer, and intra-observer variabilities need to be addressed. Lima et al. found a moderate agreement of assessing microcirculatory disturbances between real-time assessment and offline analysis (39). As for the offline analysis of SDF imaging with semi-automated software, van den Berg et al. found that vessel density of the buccal microcirculation was highly reproducible, while vessel density of the cutaneous microcirculation was not and showed high variabilities (35). It remains unclear how great inter-observer and intra-observer variabilities are for the sublingual microcirculation and if these variabilities differ with the use of different generations of HVM.
Currently, reference values for microcirculatory parameters for neonates and children are lacking. Only two small observational studies have been performed in healthy term neonates and no studies have been performed in healthy children (35, 36). There is insufficient knowledge of intra-individual heterogeneity, the natural fluctuation of measured variables and the correlation between different microvascular beds under healthy and pathological conditions (40). It is also important to address developmental changes through aging and the differences in values of microcirculatory variables derived from the different techniques and from different microvascular beds (17). This creates a challenge for comparing research findings, especially for comparing measurements or using reference values made with newer, more sensitive techniques. Results derived from older techniques are not obsolete as the vast majority of these studies evaluate change over time, change after intervention, or differences between groups. However, all studies conducted in children (as described in the following paragraph) have been observational.
Microcirculation Research in Neonates and Children
Since its introduction, HVM has also found its way in neonatal and pediatric research, although only in rather small observational studies. Table 1 gives a summary of the microcirculatory studies performed with HVM in preterm and term neonates and Table 2 gives a summary of the studies performed in pediatric patients. Genzel-Boroviczény et al. were the first to use OPS imaging on the cutaneous microcirculation of term and preterm neonates (13). The skin of the inner upper arm was the most feasible area; here the skin is thin, without hair and measurements are less prone to movement artifacts caused by breathing. Later on, both SDF imaging and IDF imaging were used on the cutaneous and buccal microcirculation (17, 35, 36). While buccal FVD values were highly reproducible, cutaneous FVD values were not. As for pediatric patients, either the buccal or the sublingual microcirculation have been areas of interest as apparent from Table 2.
Microcirculatory imaging has been used to assess physiological changes in the microcirculation, in particular developmental changes. Studies with all three generations of HVM in term and preterm neonates showed that the FVD of both the cutaneous and buccal microcirculation and the TVD of the cutaneous microcirculation decreased in the first 4 weeks of life (17, 42, 45, 51). Along the same lines, studies showed that preterm neonates had higher TVD values than term neonates. The parameters of the two microcirculatory beds did not correlate (17). Gassmann et al. observed term neonates born at high altitude with IDF imaging and found higher TVD in the cutaneous microcirculation of these neonates than of those born at sea level (52). Schwepcke et al. investigated the cutaneous microcirculation of both hypotensive as normotensive preterm neonates with OPS imaging and found higher FVD values in the first group (49). Twelve hours after birth these differences had disappeared.
HVM also offers the opportunity to examine how the microcirculation reacts under different circumstances including hypercapnia and administration of inhaled nitric oxide. Puchwein-Schwepcke et al. looked at the effect of hypercapnia on the cutaneous microcirculation of preterm neonates with extremely low birth weight with OPS imaging, as a sub analysis of a RCT (55). High PCO2 levels affected the microcirculation by reducing FVD and causing a shift to more functional large vessels than small vessels. Top et al. investigated the effect of inhaled nitric oxide on the buccal microcirculation in children with hypoxemic respiratory failure with OPS imaging. FVD was found to increase with inhaled nitric oxide (57).
Others have used HVM to assess pathophysiological changes in the microcirculation during disease. To illustrate, microcirculatory disturbances have been found in patients with an infection and/or sepsis, with persistently declined FVD and impaired microcirculatory blood flow. Weidlich et al. demonstrated with OPS imaging that FVD declined in preterm neonates with an infection over several days, before clinical suspicion occurred (43). Puchwein-Schwepcke et al. found comparable results in term neonates with SDF imaging on the upper ear conch, as patients with an infection treated with antibiotics showed lower FVD and, in addition, a higher proportion of hyperdynamic flow compared to controls without an infection (54). In contrast, Alba-Alejandre observed different microcirculatory alterations in both the ear conch and the upper arm of term neonates with a mild to moderate infection (48). These patients showed less perfused vessels with continuous flow than patients without an infection, while FVD did not differ between the two groups. Top et al. looked at the buccal microcirculation in 18 children with septic shock with OPS imaging (8). FVD was lower in non-survivors than in survivors. Also, in survivors FVD improved on the second day, while FVD did not change in non-survivors. These findings demonstrated that persistent microcirculatory disturbances irrespective of macrocirculatory hemodynamics could be a sign of worse outcome. Similarly, Paize et al. investigated the sublingual microcirculation of 20 children with severe meningococcal disease with SDF imaging. They found all microcirculatory parameters to be lower in these children than in healthy controls (58). Parallel to clinical recovery, microcirculatory parameters restored to values comparable to those of controls.
HVM can also be applied to assess the effect of different therapies on the microcirculation and the predictive value of persistent microcirculatory impairment. Buijs et al. looked at the effect of therapeutic hypothermia (TH) on the buccal microcirculation in children after cardiac arrest with SDF imaging (9). During TH the microcirculation was impaired, while after TH the microcirculation improved rapidly. Severely altered microcirculation at the start of TH was also associated with mortality. Similar findings were shown in the study with SDF imaging on the effect of TH on the cutaneous microcirculation in neonates with hypoxic-ischemic encephalopathy (50). Buijs et al. monitored the buccal microcirculation of term neonates with congenital diaphragmatic hernia receiving catecholaminergic drugs with SDF imaging (6). Dopamine, norepinephrine, and epinephrine improved macrocirculatory hemodynamics but did not alter microcirculatory function. Impaired microcirculation despite therapeutic efforts was predictive of poor outcome, irrespective of macrocirculatory hemodynamics.
Genzel-Boroviczeny et al. demonstrated the effects of blood transfusion on the cutaneous microcirculation of anemic preterm neonates with OPS imaging (41). After a blood transfusion, FVD increased, while systemic hemodynamics were unaltered. Along the same lines, Schinagl et al. looked at the effect of RBC transfusion on the buccal microcirculation of anemic children with SDF imaging (62). Anemic children showed lower TVD values and higher RBC velocities than controls. After a transfusion, TVD increased and RBC velocities decreased, although still not to values of controls. TVD was highly correlated to hemoglobin levels. It is possible RBC velocities increase to compensate for the decreased oxygen delivering capacity of RBC with anemia. As blood viscosity decrease and arteries dilate, the peripheral resistance is lowered, probably the reason for RBC velocities to increase (65). This would explain why RBC velocities decrease after a transfusion, as these hemodynamic compensating mechanisms are no longer necessary when the oxygen delivering capacity has improved.
Following research findings in adults, the effect of cardiopulmonary bypass on the microcirculation has also been assessed in children. Nussbaum et al. looked at the effect of cardiac surgery on cardiopulmonary bypass on the cutaneous microcirculation with SDF imaging (61). After cardiac surgery, glycocalyx thickness was reduced but recovered to baseline values after 1 week. MFI and PVD also declined postoperatively. In a similar population, Scolletta et al. looked at the sublingual microcirculation, also comparing cyanotic and a-cyanotic heart defects with SDF imaging (63). The sublingual microcirculation was not altered over time, in contrast to previous findings in cutaneous microcirculation. Cyanotic heart defects, however, did seem to affect TVD and PPV. Top et al. attempted to assess the effect of extracorporeal membrane oxygenation (ECMO), another type of cardiopulmonary bypass applied in critical care. They looked into the buccal microcirculation of neonates with severe respiratory failure treated with veno-arterial ECMO with OPS imaging (11). Before the start of ECMO treatment, FVD values were lower than controls and after ECMO treatment FVD values increased. In a similar study population, Top et al. showed that FVD is preserved after starting ECMO treatment, while FVD deteriorated in neonates who were only ventilated (47).
Future Perspectives
Research with HVM has revealed the added value of microcirculatory monitoring as part of hemodynamic monitoring to determine if oxygen delivery is sufficient to meet metabolic demands, as adequate microcirculatory blood supply is one of the prerequisites for meeting metabolic demands. However, clinical application is not possible before technical improvements have been made and further research has been conducted on microcirculatory guided therapy. This is especially vital for children as most of the research has been conducted in human adults and only rather small observational studies have been performed in children. In this final part, we have summarized important considerations from the previously mentioned expert consensus with special focus on those which are important for neonatal and pediatric patients (10).
Technological Innovations
One of the biggest challenges of microcirculatory monitoring in children is acquiring imaging of sufficient quality due to movement and pressure artifacts and lack of cooperation. Currently, HVM measurements are limited to deeply sedated and critically ill patients. Technological innovations are necessary to ease the use in children and thus to have full access to the potential of HVM. Hardware improvements should include pressure recognition and quantification, artifact recognition and warning signaling, and the possibility to perform single spot measurements over a longer period of time, e.g., through hands-free measurements. New software should be integrated with RBC velocity measurements throughout the entire frame, automated assessment of MFI, automated image stabilization and quality assessment and, most importantly, automated analysis software. Real-time monitoring will only be possible if automated analysis software is integrated in the software of the device. Finally, additional parameters are necessary to fully assess microcirculatory function. As MFI is only a qualitative measure for the convective capacity (i.e., blood flow) of the microcirculation, a quantitative measure is needed to assess the actual oxygen-carrying capacity of the microcirculation. This will be possible through the measurement of capillary hematocrit, as proposed by Ince et al.: tube hematocrit (the hematocrit of capillary blood at a single moment in time) and discharge hematocrit (the hematocrit flowing through capillaries per unit of time) (10).
Future Research Objectives
In order to study microcirculatory disturbances and attempt to correct these disturbances, first reference and critical values have to be established. For this research objective, we also need to account for differences between different microcirculatory beds and between different generations of HVM and for developmental changes in the microcirculation after birth and through childhood. Also, expert consensus for assessment of the buccal and cutaneous microcirculation in neonates and children would be preferable as no such guidelines exist.
After reference values are established research could take steps toward microcirculatory guided therapy. We need to assess how different microcirculatory beds correlate to one another under pathological conditions. For example, in adult septic patients Edul et al. demonstrated the dissociation between the sublingual and intestinal microcirculation in response to fluid resuscitation (66). While responsiveness of the sublingual microcirculation seemed to be dependent on cardiac output, the intestinal microcirculation responded irrespective of systemic hemodynamics. It cannot be excluded that organ-specific reactivity also plays a role. Therefore, under a variety of clinical conditions, such as laparotomy and thoracotomy, individual organs should be measured alongside the sublingual microcirculation and compared with the latter. Also, we have to establish when loss of hemodynamic coherence occurs in critically ill children as to account for differences between macrocirculatory and microcirculatory disturbances that need to be recovered. Then, it has to be clear when recovery of macrocirculatory hemodynamics is sufficient to recover microcirculatory disturbances and when we need to take additional steps to recover the microcirculation. To illustrate, Dubin et al. demonstrated in adult septic shock patients that while norepinephrine improved macrocirculatory hemodynamics, it did not alter sublingual microcirculatory parameters (67). Buijs et al. made similar observations in neonates with congenital diaphragmatic hernia (6). Holmgaard et al. showed that different levels of mean arterial pressure did not alter the sublingual microcirculation in adults during cardiopulmonary bypass (68).
The next step would be to assess which therapeutic strategies could recover microcirculatory disturbances in children. In adult research few studies have already looked into therapeutic strategies. In septic patients, Dubin et al. also found that microcirculatory monitoring could help distinguish which type of fluid, crystalloid or colloid, would be more effective in recovering microcirculatory blood flow during early goal-directed therapy (69). Spronk et al. performed a small observational study in adult septic shock patients and found that infusion of nitroglycerin might help resolve microcirculatory disturbances as MFI improved (70). Following this finding, Boerma et al. performed an RCT in a similar population, but here infusion of nitroglycerin was unsuccessful in the recruitment of the sublingual microcirculation (71). For therapeutic strategies to be effective, target ranges need to be established for relevant microcirculatory parameters. Pranskunas et al. underlined this by demonstrating that microcirculatory monitoring could help assess which (adult) patients were eligible for fluid therapy (72). Patients with MFI <2.6 were fluid responsive, as MFI increased after fluid resuscitation, while in patients with MFI > 2.6 MFI was unaltered. Van der Voort et al. tested an actual therapeutic strategy incorporated with a microcirculatory target in a pilot study with adults with septic shock (73). Recruitment of the microcirculation in this resuscitation strategy did not resolve organ dysfunction quicker than standard therapy, although the study itself had some flaws in its design. However, this study shows a potential way of looking into whether actively recovering microcirculatory disturbances could help improve patient outcome. Finally, one should also take intra-individual variability in therapy response into account when looking at the effectiveness of therapeutic strategies.
Although some observational studies have pointed toward the existing association between microcirculatory disturbances and outcome, strong evidence for the predictive value of the microcirculation is lacking for children. It has yet to be established that recovery of microcirculatory disturbances will indeed improve patient outcome since there are only two small negative trials, of which one also failed to recover microcirculatory disturbances (71, 73). Based on these trials in adults, there is currently no evidence to support the clinical use of microcirculatory monitoring with HVM in children. However, there might be in the future, since larger trials to evaluate the microcirculation, similar to for example trials on high-frequency oscillatory ventilation or inhaled nitric oxide for acute respiratory distress syndrome, have not yet been carried out (74, 75). A good example to follow would be the study on lactate-guided therapy in critically ill adults performed by Jansen et al. (76). To perform similar trials with microcirculatory monitoring, international research collaborations are necessary as single centers will have insufficient numbers of patients to prove any effect of therapeutic interventions on the microcirculation of children. Nevertheless, we should also be realistic regarding the number of patients and consider the feasibility of such trials. To illustrate, to prove the effect of ECMO treatment in pediatric acute respiratory distress syndrome in an RCT, one would need more than 1,000 patients per treatment arm (77, 78).
Summary
Integrating microcirculatory monitoring in routine hemodynamic monitoring may be important for the assurance of adequate tissue oxygenation as it offers additional insight into oxygen delivery. HVM is a promising tool for assessing otherwise unnoticed disturbed oxygen delivery on a microcirculatory level. Before application in neonatal and pediatric critical care is possible, however, technological advancements and extensive research on microcirculatory guided therapy are necessary.
Author Contributions
ÖE and JK wrote the draft of the manuscript. All authors contributed to manuscript revision, read, and approved the submitted version.
Funding
ÖE was supported by a grant from the Stichting Sophia Kinderziekenhuis Fonds (grant number S17-06). The Stichting Sophia Kinderziekenhuis Fonds had no role in the preparation or publishing of this manuscript.
Conflict of Interest Statement
CI has developed SDF imaging and is listed as an inventor on related patents commercialized by MicroVision Medical under a license from the Academic Medical Center in Amsterdam, the Netherlands. He has been a consultant for MicroVision Medical in the past, but has not been involved with this company for over 5 years now and holds no shares. Braedius Medical, a company owned by a relative of CI, has developed and designed a handheld microscope called CytoCam-IDF imaging. CI has no financial relationship with Braedius Medical of any sort, i.e., never owned shares, or received consultancy or speaker fees from Braedius Medical.
The remaining authors declare that the research was conducted in the absence of any commercial or financial relationships that could be construed as a potential conflict of interest.
Abbreviations
APACHE II score, acute physiology, age, chronic health evaluation II score; ECMO, extracorporeal membrane oxygenation; FCD, functional capillary density; FVD, functional vessel density; HI, heterogeneity index; HVM, handheld vital microscopy; MFI, microcirculatory flow index; OPS, orthogonal polarization spectral; PPV, proportion of perfused vessels; PRISM score, pediatric risk of mortality score; PVD, perfused vessel density; RBC, red blood cell; SDF, sidestream dark field; STD, space-time diagram; TH, therapeutic hypothermia; IDF, incident dark field illumination; TVD, total vessel density.
References
1. de Boode WP. Clinical monitoring of systemic hemodynamics in critically ill newborns. Early Hum Dev. (2010) 86:137–41. doi: 10.1016/j.earlhumdev.2010.01.031
2. Vrancken SL, van Heijst AF, de Boode WP. Neonatal hemodynamics: from developmental physiology to comprehensive monitoring. Front Pediatr. (2018) 6:87. doi: 10.3389/fped.2018.00087
3. Bronicki RA. Hemodynamic monitoring. Pediatr Crit Care Med. (2016) 17(8 Suppl 1):S207–14. doi: 10.1097/PCC.0000000000000779
4. Ince C. Hemodynamic coherence and the rationale for monitoring the microcirculation. Crit Care. (2015) 19 Suppl 3:S8. doi: 10.1186/cc14726
5. Hernandez G, Bruhn A, Luengo C, Regueira T, Kattan E, Fuentealba A, et al. Effects of dobutamine on systemic, regional and microcirculatory perfusion parameters in septic shock: a randomized, placebo-controlled, double-blind, crossover study. Intensive Care Med. (2013) 39:1435–43. doi: 10.1007/s00134-013-2982-0
6. Buijs EA, Reiss IK, Kraemer U, Andrinopoulou ER, Zwiers AJ, Ince C, et al. Increasing mean arterial blood pressure and heart rate with catecholaminergic drugs does not improve the microcirculation in children with congenital diaphragmatic hernia: a prospective cohort study. Pediatr Crit Care Med. (2014) 15:343–54. doi: 10.1097/PCC.0000000000000105
7. Scorcella C, Damiani E, Domizi R, Pierantozzi S, Tondi S, Carsetti A, et al. MicroDAIMON study: Microcirculatory DAIly MONitoring in critically ill patients: a prospective observational study. Ann Intensive Care. (2018) 8:64. doi: 10.1186/s13613-018-0411-9
8. Top AP, Ince C, de Meij N, van Dijk M, Tibboel D. Persistent low microcirculatory vessel density in nonsurvivors of sepsis in pediatric intensive care. Crit Care Med. (2011) 39:8–13. doi: 10.1097/CCM.0b013e3181fb7994
9. Buijs EA, Verboom EM, Top AP, Andrinopoulou ER, Buysse CM, Ince C, et al. Early microcirculatory impairment during therapeutic hypothermia is associated with poor outcome in post-cardiac arrest children: a prospective observational cohort study. Resuscitation. (2014) 85:397–404. doi: 10.1016/j.resuscitation.2013.10.024
10. Ince C, Boerma EC, Cecconi M, De Backer D, Shapiro NI, Duranteau J, et al. Second consensus on the assessment of sublingual microcirculation in critically ill patients: results from a task force of the European Society of Intensive Care Medicine. Intensive Care Med. (2018) 44:281–99. doi: 10.1007/s00134-018-5070-7
11. Top AP, Ince C, van Dijk M, Tibboel D. Changes in buccal microcirculation following extracorporeal membrane oxygenation in term neonates with severe respiratory failure. Crit Care Med. (2009) 37:1121–4. doi: 10.1097/CCM.0b013e3181962a5f
12. Tytgat SH, van der Zee DC, Ince C, Milstein DM. Carbon dioxide gas pneumoperitoneum induces minimal microcirculatory changes in neonates during laparoscopic pyloromyotomy. Surg Endosc. (2013) 27:3465–73. doi: 10.1007/s00464-013-2927-2
13. Genzel-Boroviczeny O, Strotgen J, Harris AG, Messmer K, Christ F. Orthogonal polarization spectral imaging (OPS): a novel method to measure the microcirculation in term and preterm infants transcutaneously. Pediatr Res. (2002) 51:386–91. doi: 10.1203/00006450-200203000-00019
14. Groner W, Winkelman JW, Harris AG, Ince C, Bouma GJ, Messmer K, et al. Orthogonal polarization spectral imaging: a new method for study of the microcirculation. Nat Med. (1999) 5:1209–12. doi: 10.1038/13529
15. Goedhart PT, Khalilzada M, Bezemer R, Merza J, Ince C. Sidestream Dark Field (SDF) imaging: a novel stroboscopic LED ring-based imaging modality for clinical assessment of the microcirculation. Opt Express. (2007) 15:15101–14. doi: 10.1364/OE.15.015101
16. Aykut G, Veenstra G, Scorcella C, Ince C, Boerma C. Cytocam-IDF (incident dark field illumination) imaging for bedside monitoring of the microcirculation. Intensive Care Med Exp. (2015) 3:40. doi: 10.1186/s40635-015-0040-7
17. van Elteren HA, Ince C, Tibboel D, Reiss IK, de Jonge RC. Cutaneous microcirculation in preterm neonates: comparison between sidestream dark field (SDF) and incident dark field (IDF) imaging. J Clin Monit Comput. (2015) 29:543–8. doi: 10.1007/s10877-015-9708-5
18. Lehmann C, Sardinha J, Mukhtar AM. Sidestream versus incident dark field imaging: how to compare two different technologies to study the microcirculation? J Clin Monit Comput. (2015) 29:539–40. doi: 10.1007/s10877-015-9740-5
19. Gilbert-Kawai E, Coppel J, Bountziouka V, Ince C, Martin D, Caudwell Xtreme E, et al. A comparison of the quality of image acquisition between the incident dark field and sidestream dark field video-microscopes. BMC Med Imaging. (2016) 16:10. doi: 10.1186/s12880-015-0078-8
20. Massey MJ, Shapiro NI. A guide to human in vivo microcirculatory flow image analysis. Crit Care. (2016) 20:35. doi: 10.1186/s13054-016-1213-9
21. De Backer D, Hollenberg S, Boerma C, Goedhart P, Buchele G, Ospina-Tascon G, et al. How to evaluate the microcirculation: report of a round table conference. Crit Care. (2007) 11:R101. doi: 10.1186/cc6118
22. van Elteren H, Reiss IK, de Jonge RC. Transcutaneous microcirculatory imaging in preterm neonates. J Vis Exp. (2015) 106:e53562. doi: 10.3791/53562
23. Massey MJ, Larochelle E, Najarro G, Karmacharla A, Arnold R, Trzeciak S, et al. The microcirculation image quality score: development and preliminary evaluation of a proposed approach to grading quality of image acquisition for bedside videomicroscopy. J Crit Care. (2013) 28:913–7. doi: 10.1016/j.jcrc.2013.06.015
24. De Backer D, Creteur J, Preiser JC, Dubois MJ, Vincent JL. Microvascular blood flow is altered in patients with sepsis. Am J Respir Crit Care Med. (2002) 166:98–104. doi: 10.1164/rccm.200109-016OC
25. Arnold RC, Parrillo JE, Phillip Dellinger R, Chansky ME, Shapiro NI, Lundy DJ, et al. Point-of-care assessment of microvascular blood flow in critically ill patients. Intensive Care Med. (2009) 35:1761–6. doi: 10.1007/s00134-009-1517-1
26. Naumann DN, Mellis C, Husheer SL, Hopkins P, Bishop J, Midwinter MJ, et al. Real-time point of care microcirculatory assessment of shock: design, rationale and application of the point of care microcirculation (POEM) tool. Crit Care. (2016) 20:310. doi: 10.1186/s13054-016-1492-1
27. Uz Z, van Gulik TM, Aydemirli MD, Guerci P, Ince Y, Cuppen D, et al. Identification and quantification of human microcirculatory leukocytes using handheld video microscopes at the bedside. J Appl Physiol. (2018) 124:1550–7. doi: 10.1152/japplphysiol.00962.2017
28. Fabian-Jessing BK, Massey MJ, Filbin MR, Hou PC, Wang HE, Kirkegaard H, et al. In vivo quantification of rolling and adhered leukocytes in human sepsis. Crit Care. (2018) 22:240. doi: 10.1186/s13054-018-2173-z
29. Nieuwdorp M, Meuwese MC, Mooij HL, Ince C, Broekhuizen LN, Kastelein JJ, et al. Measuring endothelial glycocalyx dimensions in humans: a potential novel tool to monitor vascular vulnerability. J Appl Physiol. (2008) 104:845–52. doi: 10.1152/japplphysiol.00440.2007
30. Bezemer R, Dobbe JG, Bartels SA, Boerma EC, Elbers PW, Heger M, et al. Rapid automatic assessment of microvascular density in sidestream dark field images. Med Biol Eng Comput. (2011) 49:1269–78. doi: 10.1007/s11517-011-0824-1
31. Demir SU, Hakimzadeh R, Hargraves RH, Ward KR, Myer EV, Najarian K. An automated method for analysis of microcirculation videos for accurate assessment of tissue perfusion. BMC Med Imaging. (2012) 12:37. doi: 10.1186/1471-2342-12-37
32. Arnemann PH, Hessler M, Kampmeier T, Morelli A, Van Aken HK, Westphal M, et al. Comparison of an automatic analysis and a manual analysis of conjunctival microcirculation in a sheep model of haemorrhagic shock. Intensive Care Med Exp. (2016) 4:37. doi: 10.1186/s40635-016-0110-5
33. Carsetti A, Aya HD, Pierantozzi S, Bazurro S, Donati A, Rhodes A, et al. Ability and efficiency of an automatic analysis software to measure microvascular parameters. J Clin Monit Comput. (2017) 31:669–76. doi: 10.1007/s10877-016-9928-3
34. Sorelli M, Ince C, Bocchi L. Particle tracking for the assessment of microcirculatory perfusion. Physiol Meas. (2017) 38:358–73. doi: 10.1088/1361-6579/aa56ab
35. van den Berg VJ, van Elteren HA, Buijs EA, Ince C, Tibboel D, Reiss IK, et al. Reproducibility of microvascular vessel density analysis in Sidestream dark-field-derived images of healthy term newborns. Microcirculation. (2015) 22:37–43. doi: 10.1111/micc.12163
36. Wright IM, Latter JL, Dyson RM, Levi CR, Clifton VL. Videomicroscopy as a tool for investigation of the microcirculation in the newborn. Physiol Rep. (2016) 4:e12941. doi: 10.14814/phy2.12941
37. Gonzalez R, Lopez J, Urbano J, Solana MJ, Fernandez SN, Santiago MJ, et al. Evaluation of sublingual microcirculation in a paediatric intensive care unit: prospective observational study about its feasibility and utility. BMC Pediatr. (2017) 17:75. doi: 10.1186/s12887-017-0837-5
38. Boerma EC, Mathura KR, van der Voort PH, Spronk PE, Ince C. Quantifying bedside-derived imaging of microcirculatory abnormalities in septic patients: a prospective validation study. Crit Care. (2005) 9:R601–6. doi: 10.1186/cc3809
39. Lima A, Lopez A, van Genderen ME, Hurtado FJ, Angulo M, Grignola JC, et al. Interrater reliability and diagnostic performance of subjective evaluation of sublingual microcirculation images by physicians and nurses: a multicenter observational study. Shock. (2015) 44:239–44. doi: 10.1097/SHK.0000000000000401
40. Henzler D, Scheffler M, Westheider A, Kohler T. Microcirculation measurements: barriers for use in clinical routine. Clin Hemorheol Microcirc. (2017) 67:505–9. doi: 10.3233/CH-179229
41. Genzel-Boroviczeny O, Christ F, Glas V. Blood transfusion increases functional capillary density in the skin of anemic preterm infants. Pediatr Res. (2004) 56:751–5. doi: 10.1203/01.PDR.0000141982.38959.10
42. Kroth J, Weidlich K, Hiedl S, Nussbaum C, Christ F, Genzel-boroviczeny O. Functional vessel density in the first month of life in preterm neonates. Pediatr Res. (2008) 64:567–71. doi: 10.1203/PDR.0b013e318184134e
43. Weidlich K, Kroth J, Nussbaum C, Hiedl S, Bauer A, Christ F, et al. Changes in microcirculation as early markers for infection in preterm infants–an observational prospective study. Pediatr Res. (2009) 66:461–5. doi: 10.1203/PDR.0b013e3181b3b1f6
44. Hiedl S, Schwepcke A, Weber F, Genzel-Boroviczeny O. Microcirculation in preterm infants: profound effects of patent ductus arteriosus. J Pediatr. (2010) 156:191–6. doi: 10.1016/j.jpeds.2009.08.034
45. Top AP, van Dijk M, van Velzen JE, Ince C, Tibboel D. Functional capillary density decreases after the first week of life in term neonates. Neonatology. (2011) 99:73–7. doi: 10.1159/000316945
46. Ergenekon E, Hirfanoglu IM, Turan O, Beken S, Gucuyener K, Atalay Y. Partial exchange transfusion results in increased cerebral oxygenation and faster peripheral microcirculation in newborns with polycythemia. Acta Paediatr. (2011) 100:1432–6. doi: 10.1111/j.1651-2227.2011.02358.x
47. Top AP, Buijs EA, Schouwenberg PH, van Dijk M, Tibboel D, Ince C. The microcirculation is unchanged in neonates with severe respiratory failure after the initiation of ECMO treatment. Crit Care Res Pract. (2012) 2012:372956. doi: 10.1155/2012/372956
48. Alba-Alejandre I, Hiedl S, Genzel-Boroviczeny O. Microcirculatory changes in term newborns with suspected infection: an observational prospective study. Int J Pediatr. (2013) 2013:768784. doi: 10.1155/2013/768784
49. Schwepcke A, Weber FD, Mormanova Z, Cepissak B, Genzel-Boroviczeny O. Microcirculatory mechanisms in postnatal hypotension affecting premature infants. Pediatr Res. (2013) 74:186–90. doi: 10.1038/pr.2013.78
50. Ergenekon E, Hirfanoglu I, Beken S, Turan O, Kulali F, Koc E, et al. Peripheral microcirculation is affected during therapeutic hypothermia in newborns. Arch Dis Child Fetal Neonatal Ed. (2013) 98:F155–7. doi: 10.1136/archdischild-2012-301647
51. van Elteren HA, de Jonge RC, van Rosmalen J, Ince C, Reiss IK. Adaptation of the cutaneous microcirculation in preterm neonates. Microcirculation. (2016) 23:468–74. doi: 10.1111/micc.12295
52. Gassmann NN, van Elteren HA, Goos TG, Morales CR, Rivera-Ch M, Martin DS, et al. Pregnancy at high altitude in the Andes leads to increased total vessel density in healthy newborns. J Appl Physiol. (2016) 121:709–15. doi: 10.1152/japplphysiol.00561.2016
53. Kulali F, Ergenekon E, Aktas S, Kazanci E, Unal S, Hirfanoglu I, et al. Impact of mode of delivery on skin microcirculation in term healthy newborns within the first day of life. J Matern Fetal Neonatal Med. (2017) 30:673–7. doi: 10.1080/14767058.2016.1182977
54. Puchwein-Schwepcke A, Preusser D, Nussbaum C, Genzel-Boroviczeny O. Screening for infection - an analysis of the microcirculation in term newborns. J Neonatol Clin Pediatr. (2017) 4:7. doi: 10.24966/NCP-878X/100020
55. Puchwein-Schwepcke AF, Schottmayer K, Mormanova Z, Dreyhaupt J, Genzel-Boroviczeny O, Thome UH. Permissive hypercapnia results in decreased functional vessel density in the skin of extremely low birth weight infants. Front Pediatr. (2018) 6:52. doi: 10.3389/fped.2018.00052
56. Top AP. Microcirculation in Critically Ill Children (Doctoral dissertation) (2011) 35–41. Retrieved from: http://hdl.handle.net/1765/51630
57. Top AP, Ince C, Schouwenberg PH, Tibboel D. Inhaled nitric oxide improves systemic microcirculation in infants with hypoxemic respiratory failure. Pediatr Crit Care Med. (2011) 12:e271–4. doi: 10.1097/PCC.0b013e31820ac0b3
58. Paize F, Sarginson R, Makwana N, Baines PB, Thomson AP, Sinha I, et al. Changes in the sublingual microcirculation and endothelial adhesion molecules during the course of severe meningococcal disease treated in the paediatric intensive care unit. Intensive Care Med. (2012) 38:863–71. doi: 10.1007/s00134-012-2476-5
59. Buijs EA. Critically Ill Children and the Microcirculation: Go with the Flow? (Doctoral dissertation) (2014) 57–78. Retrieved from: http://hdl.handle.net/1765/51532
60. Nussbaum C, Cavalcanti Fernandes Heringa A, Mormanova Z, Puchwein-Schwepcke AF, Bechtold-Dalla Pozza S, Genzel-Boroviczeny O. Early microvascular changes with loss of the glycocalyx in children with type 1 diabetes. J Pediatr. (2014) 164:584–9.e1. doi: 10.1016/j.jpeds.2013.11.016
61. Nussbaum C, Haberer A, Tiefenthaller A, Januszewska K, Chappell D, Brettner F, et al. Perturbation of the microvascular glycocalyx and perfusion in infants after cardiopulmonary bypass. J Thorac Cardiovasc Surg. (2015) 150:1474–81.e1. doi: 10.1016/j.jtcvs.2015.08.050
62. Schinagl CM, Mormanova ZH, Puchwein-Schwepcke A, Schmid I, Genzel-Boroviczeny O. The effect of red blood cell transfusion on the microcirculation of anemic children. Eur J Pediatr. (2016) 175:793–8. doi: 10.1007/s00431-016-2704-z
63. Scolletta S, Marianello D, Isgro G, Dapoto A, Terranova V, Franchi F, et al. Microcirculatory changes in children undergoing cardiac surgery: a prospective observational study. Br J Anaesth. (2016) 117:206–13. doi: 10.1093/bja/aew187
64. Riedijk MA, Milstein DMJ. Imaging sublingual microcirculatory perfusion in pediatric patients receiving procedural sedation with propofol: a pilot study. Microcirculation. (2018) 25:e12484. doi: 10.1111/micc.12484
65. Metivier F, Marchais SJ, Guerin AP, Pannier B, London GM. Pathophysiology of anaemia: focus on the heart and blood vessels. Nephrol Dial Transplant. (2000) 15 (Suppl 3):14–8. doi: 10.1093/oxfordjournals.ndt.a027970
66. Edul VK, Ince C, Navarro N, Previgliano L, Risso-Vazquez A, Rubatto Birri PN, et al. Dissociation between sublingual and gut microcirculation in the response to a fluid challenge in postoperative patients with abdominal sepsis. Ann Intensive Care. (2014) 4:39. doi: 10.1186/s13613-014-0039-3
67. Dubin A, Pozo MO, Casabella CA, Palizas F Jr, Murias G, Moseinco MC, et al. Increasing arterial blood pressure with norepinephrine does not improve microcirculatory blood flow: a prospective study. Crit Care. (2009) 13:R92. doi: 10.1186/cc7922
68. Holmgaard F, Vedel AG, Ravn HB, Nilsson JC, Rasmussen LS. Impact of mean arterial pressure on sublingual microcirculation during cardiopulmonary bypass-Secondary outcome from a randomized clinical trial. Microcirculation. (2018) 25:e12459. doi: 10.1111/micc.12459
69. Dubin A, Pozo MO, Casabella CA, Murias G, Palizas F Jr, Moseinco MC, et al. Comparison of 6% hydroxyethyl starch 130/0.4 and saline solution for resuscitation of the microcirculation during the early goal-directed therapy of septic patients. J Crit Care. (2010) 25:659.e1–8. doi: 10.1016/j.jcrc.2010.04.007
70. Spronk PE, Ince C, Gardien MJ, Mathura KR, Oudemans-van Straaten HM, Zandstra DF. Nitroglycerin in septic shock after intravascular volume resuscitation. Lancet. (2002) 360:1395–6. doi: 10.1016/S0140-6736(02)11393-6
71. Boerma EC, Koopmans M, Konijn A, Kaiferova K, Bakker AJ, van Roon EN, et al. Effects of nitroglycerin on sublingual microcirculatory blood flow in patients with severe sepsis/septic shock after a strict resuscitation protocol: a double-blind randomized placebo controlled trial. Crit Care Med. (2010) 38:93–100. doi: 10.1097/CCM.0b013e3181b02fc1
72. Pranskunas A, Koopmans M, Koetsier PM, Pilvinis V, Boerma EC. Microcirculatory blood flow as a tool to select ICU patients eligible for fluid therapy. Intensive Care Med. (2013) 39:612–9. doi: 10.1007/s00134-012-2793-8
73. van der Voort PH, van Zanten M, Bosman RJ, van Stijn I, Wester JP, van Raalte R, et al. Testing a conceptual model on early opening of the microcirculation in severe sepsis and septic shock: a randomised controlled pilot study. Eur J Anaesthesiol. (2015) 32:189–98. doi: 10.1097/EJA.0000000000000126
74. Sud S, Sud M, Friedrich JO, Wunsch H, Meade MO, Ferguson ND, et al. High-frequency oscillatory ventilation versus conventional ventilation for acute respiratory distress syndrome. Cochrane Database Syst Rev. (2016) 4:CD004085. doi: 10.1002/14651858.CD004085.pub4
75. Karam O, Gebistorf F, Wetterslev J, Afshari A. The effect of inhaled nitric oxide in acute respiratory distress syndrome in children and adults: a Cochrane Systematic Review with trial sequential analysis. Anaesthesia. (2017) 72:106–17. doi: 10.1111/anae.13628
76. Jansen TC, van Bommel J, Schoonderbeek FJ, Sleeswijk Visser SJ, van der Klooster JM, Lima AP, et al. Early lactate-guided therapy in intensive care unit patients: a multicenter, open-label, randomized controlled trial. Am J Respir Crit Care Med. (2010) 182:752–61. doi: 10.1164/rccm.200912-1918OC
77. Barbaro RP, Xu Y, Borasino S, Truemper EJ, Watson RS, Thiagarajan RR, et al. Does extracorporeal membrane oxygenation improve survival in pediatric acute respiratory failure? Am J Respir Crit Care Med. (2018) 197:1177–86. doi: 10.1164/rccm.201709-1893OC
Keywords: microcirculation, hemodynamic monitoring, neonates, pediatrics, critical care
Citation: Erdem Ö, Ince C, Tibboel D and Kuiper JW (2019) Assessing the Microcirculation With Handheld Vital Microscopy in Critically Ill Neonates and Children: Evolution of the Technique and Its Potential for Critical Care. Front. Pediatr. 7:273. doi: 10.3389/fped.2019.00273
Received: 04 February 2019; Accepted: 17 June 2019;
Published: 09 July 2019.
Edited by:
Hitesh Singh Sandhu, University of Tennessee Health Science Center (UTHSC), United StatesReviewed by:
Oliver Karam, Children's Hospital of Richmond at VCU, United StatesDavid N. Naumann, University of Birmingham, United Kingdom
Copyright © 2019 Erdem, Ince, Tibboel and Kuiper. This is an open-access article distributed under the terms of the Creative Commons Attribution License (CC BY). The use, distribution or reproduction in other forums is permitted, provided the original author(s) and the copyright owner(s) are credited and that the original publication in this journal is cited, in accordance with accepted academic practice. No use, distribution or reproduction is permitted which does not comply with these terms.
*Correspondence: Jan Willem Kuiper, ai5rdWlwZXJAZXJhc211c21jLm5s