- 1Division of Neonatology, Pediatric Intensive Care & Neuropediatrics, Department of Pediatrics and Adolescent Medicine, Medical University of Vienna, Vienna, Austria
- 2Precision Vaccines Program, Division of Infectious Diseases, Department of Medicine, Boston Children's Hospital, Boston, MA, United States
- 3Harvard Medical School, Boston, MA, United States
- 4Department of Pediatrics, Maastricht University Medical Centre (MUMC+), Maastricht, Netherlands
- 5School for Oncology and Developmental Biology (GROW), Maastricht University, Maastricht, Netherlands
- 6Department of Surgery, Research Labs & Core Facility Flow Cytometry, Medical University of Vienna, Vienna, Austria
- 7Broad Institute of MIT and Harvard, Boston, MA, United States
Despite continued advances in neonatal medicine, sepsis remains a leading cause of death worldwide in neonatal intensive care units. The clinical presentation of sepsis in neonates varies markedly from that in older children and adults, and distinct acute inflammatory responses results in age-specific inflammatory and protective immune response to infection. This review first provides an overview of the neonatal immune system, then covers current mainstream, and experimental preventive and adjuvant therapies in neonatal sepsis. We also discuss how the distinct physiology of the perinatal period shapes early life immune responses and review strategies to reduce neonatal sepsis-related morbidity and mortality. A summary of studies that characterize immune ontogeny and neonatal sepsis is presented, followed by discussion of clinical trials assessing interventions such as breast milk, lactoferrin, probiotics, and pentoxifylline. Finally, we critically appraise future treatment options such as stem cell therapy, other antimicrobial protein and peptides, and targeting of pattern recognition receptors in an effort to prevent and/or treat sepsis in this highly vulnerable neonatal population.
Introduction
Despite advances in neonatal care leading to improved survival rates and reduced complications in preterm infants (1), there has been little improvement in the prophylaxis, treatment, and adverse neurodevelopmental outcomes associated with neonatal sepsis over the last three decades (2–5). The incidence of neonatal sepsis is inversely correlated with gestational age (GA) and birth weight (BW). While ~20% of very low BW (<1,500 g; VLBW) infants suffer from one or more systemic infections during their hospital stay (6, 7), the rate may reach up to 60% in the most immature infants (8). Inflammatory conditions such as neonatal sepsis and necrotizing enterocolitis (NEC) are associated with persistently high morbidity and mortality rates in these infants (9, 10).
Definition and Clinical Course of Neonatal Sepsis
While a consensus definition of pediatric sepsis exists, defined as a systemic inflammatory response syndrome (SIRS) in the presence of suspected or proven infection (11), no such consensus definition has yet been published for neonatal sepsis (12). Although a positive blood culture defines bacteremia and has been included in some proposed definitions of neonatal sepsis, such an approach does not take into account that in most cases of neonatal sepsis clinical signs are not associated with positive blood cultures (13). Recent studies included a combination of laboratory tests, clinical findings and/or the duration of antimicrobial treatment (≥5 days), reflecting complexity and heterogeneity in neonatal sepsis (3). Thus, in clinical practice, diagnosis of neonatal sepsis is complicated by the absence of a consensus definition, non-specific symptoms, and low sensitivity of the low volume bacterial blood cultures typically obtained (12). Furthermore, established diagnostic tests to predict severity and to guide treatment are lacking (3). Complete blood counts, including immature to total neutrophil ratios, C-reactive protein (CRP), interleukin (IL)-6 or CXCL-8 (IL-8), and procalcitonin (PCT) have some clinical utility (14). Cell surface markers on circulating cells, such as soluble CD14, CD64, and HLA-DR, offer some diagnostic value (15–17). However, much remains to be learned regarding optimal diagnostics and research in this area, including the application of systems biology approaches for biomarker discovery (18).
Early empiric treatment with antibiotics is essential for neonatal bacterial sepsis. Rapid clinical deterioration, however, may still ensue even if antibiotic treatment is started promptly. Possible life-threatening complications include the development of disseminated intra-vascular coagulation, pulmonary hypertension, congestive heart failure and shock (19). These complications can result from phases of excessive inflammation as well as immunosuppression (20–22). Until recently, the immunological basis of sepsis was thought to be a biphasic process with an initial hyperinflammatory phase followed by a later anti-inflammatory phase manifesting as functional immune suppression (23). However, genome-wide transcription profiling in human sepsis of term neonates, children, and adults demonstrates that phases of pro- and anti-inflammatory mechanisms occur during variable times over a sepsis episode and that patients may cycle through each phase multiple times during the course of sepsis (22, 24–28).
Immunological Risk Factors for Neonatal Sepsis
Little is known regarding the sepsis phases in preterm human neonates, but recent findings indicate that both gestational and postnatal age are significant factors affecting immune responses during the critical window of immune adaptation (20, 29, 30). During this window, pathogen-associated molecular patterns (PAMPs) and damage-associated molecular patterns (DAMPs) are potent inducers of inflammation and might shape immune responses early in life (31). Stimulation of pattern recognition receptors (PRRs) in human preterm blood by exogenous PAMPs induces T helper (Th) and anti-inflammatory profiles with impaired Th1 and pro-inflammatory cytokines (32). In this context, innate immunity in newborns has often been alluded to as “impaired,” “defective,” or “immature.” However, it is more accurately described as “distinct” since the fetus/preterm neonate is well-equipped for life in utero and the term newborn immune system appropriately mediates the transition from in utero to ex utero life. For maintenance of tolerance to maternal antigens and to avoid inflammation-triggered preterm delivery, neonatal immune responses are in general T helper (Th)-2 and Th-17 cell biased (33). However, such polarization also corresponds to GA-dependent susceptibility to invasive infections (32, 34). In addition, decreased complement-mediated/phagocytic activity (35, 36), reduced absolute neutrophil counts and functions (37), as well as altered phenotype and function of professional antigen-presenting cells (APCs) (38–40) in response to most Toll-like-receptor agonists (TLRAs) have been described. Moreover, the distinct composition of neonatal plasma, including high concentrations of adenosine (41–44), prostaglandins (45), placental hormones such as cortisol (46), estradiol, and progesterone (47), inhibits the production of Th1 cytokines. In addition, adenosine may also contribute to impaired neutrophil responses by inhibiting neutrophil-endothelial adhesion molecules (48). In contrast, high perinatal levels of cytokines such as the migration inhibitory factor (MIF) (49) and d-dopachrome tautomerase (DDT, also known as MIF-2) (50) counter regulate the activity of adenosine and prostaglandin E2 and together with interleukin-18 (51) further shape immune responses early in life. Taken together, a tightly regulated distinct balance of pro- and anti-inflammatory mediators in neonates shapes early life innate immune responses.
Of note, with respect to human in vitro assays, whereas responses to most pure TLRAs are reduced in early life, live organisms such as Group B streptococcus (GBS) may signal robust inflammatory responses including TNF production (52). GBS signals in part via TLR8 (53), a TLR pathway that recognizes microbial viability (54). GBS in human newborn blood potentially acts via vita-PAMPs, that is a subset of PAMPs expressed specifically by live microorganism (55), and TLR8 that detects bacterial RNA (52, 54, 56) and might therefore contribute to an exaggerated inflammation in bacterial neonatal sepsis in vivo (20). In addition, pro-inflammatory IL-1β responses are impaired in cord blood of preterm infants, but are restored to adult levels during the neonatal period, indicating rapid maturation of these responses after birth (57). Systemic inflammation characterized by high concentrations of proinflammatory cytokines are associated with poor long-term outcomes, and preterm infants are especially vulnerable to inflammatory injuries (10).
A non-inflammatory profile with mature immunoregulatory capacities is acquired in an age—dependent manner and a delayed adjustment of regulatory signaling pathways might in part explain the preterm infants' susceptibility to inflammatory conditions. Neonatal cord blood of term neonates contains extremely high concentrations of alarmins that act as endogenous DAMPs (58). In utero, alarmins induce an “endotoxin-like state” by altering MyD88-dependent pro-inflammatory gene programs with corresponding low Th1 responses (30). After birth, alarmin-levels decrease and regulatory TIR-domain-containing adapter-inducing interferon-β (TRIF)-dependent genes gradually increase (30, 58). A disruption of this critical sequence of transient alarmin programming and subsequent reprogramming of regulatory pathways, as occurs in preterm birth, increase the risk of hyperinflammation and neonatal sepsis (30). Of note, alarmins are increased in intra-amniotic infection/inflammation (59) and histologic chorioamnionitis correlates with a decreased risk of LOS (60) indicating that perinatal inflammation may enhance the immunoregulatory and/or functional maturation of the preterm immune system.
Other counter-inflammatory mechanisms, such as increased numbers of regulatory T cells (61, 62), and myeloid-derived suppressor cells (63, 64), as well as impaired phagocytosis-induced cell death (65, 66) might further affect the outcome of neonatal sepsis. However, the role of these mechanisms in neonatal sepsis has not yet been fully delineated, mostly because of limited access to neonatal peripheral blood samples and difficulties in performing longitudinal studies to investigate neonatal immune ontogeny.
Epidemiological Risk Factors of Neonatal Sepsis
In addition to GA-specific immunological characteristics, sex (67), genetic predisposition (68, 69), the evolving microbiome/ microbial colonization (70), and underlying medical conditions shape immune responses and impact the risk of developing neonatal sepsis (4, 71). Given the number of cellular and molecular factors involved, emerging systems biology offer new avenues to monitor functional immune development in the near future (72–74).
The high susceptibility of preterm infants to invasive infections and associated poor long-term outcomes, have prompted the exploration of alternative therapies for neonatal sepsis. A number of interventions have been evaluated, and some such as oral lactoferrin have demonstrated promise (75), but beyond antibiotics and supportive care, there is presently no approved drug for the treatment or prevention of sepsis in preterm or term neonates (25, 76). This review summarizes past and present immunomodulatory concepts and outlines novel potential targets for the prevention and treatment of neonatal sepsis. A literature search for published meta-analyses, randomized controlled trials (RCTs), systematic reviews, individual clinical studies and emerging work from animal models was performed. A list of current approaches discussed in this article is presented in Table 1 (treatment) and Table 2 (prevention); the corresponding literature search strategy is outlined in Supplementary Figure 1.
Prior Studies That Have Not Demonstrated Clinical Benefit
Immunoglobulins
Preterm neonates born prior to 32 weeks gestation have low levels of passively acquired antibodies, and endogenous immunoglobulin (Ig) synthesis does not begin until 24 weeks of life (93, 94). Immunoglobulins provide opsonic activity, activate complement, and promote antibody-dependent cytotoxicity (95). Given these biological effects and the observation of decreased Ig levels in severe sepsis, several clinical studies have investigated the use of intravenous immunoglobulins (IVIG) to prevent and treat neonatal sepsis (78, 96–98).
A 2015 Cochrane Review, evaluating 9 IVIG studies and including a total of 3,973 infants showed no reduction of in-hospital mortality or in the combined outcome of death or major disability at 2 years of age in preterm infants with suspected or proven infection (Table 1) (78). Additionally, IgM-enriched IVIG preparations, which may provide higher opsonization activity and complement activation compared to IgG, did not significantly reduce mortality during hospital stay in infants with suspected sepsis (n = 66) (78). Based on these findings, routine administration of IVIG or IgM-enriched IVIG to prevent mortality in infants with suspected or proven neonatal infection cannot be recommended.
Given that >50% of cases of late-onset sepsis (LOS) in VLBW are caused by Staphylococcus spp., various type-specific antibodies targeted at different antigenic markers of Staphylococcus have been developed and studied in RCTs (82, 83). A 2009 Cochrane Review evaluated the effects of two anti-staphylococcal immunoglobulins, INH-A21 (pooled generic anti-staphylococcal immunoglobulin) and Altastaph (human polyclonal immunoglobulin against capsular polysaccharide antigens type 5 and 8), on the prevention of LOS in infants ≤ 32 weeks (82). No significant reduction in the risk of infection or mortality was identified (Table 2). A third anti-staphylococcal immunoglobulin, pagibaximab (anti-lipoteichoic acid monoclonal antibody) was studied, but again no significant reduction in sepsis or mortality was found (83, 84)(Table 2).
The reasons for these failed trials have not yet been elucidated but one might speculate that distinct immune responses in the preterm infant such as reduced complement or leukocyte activity may play a role or that the correct type or combination of antibodies has not yet been found (83). Future studies need to identify antibodies that (i) target optimal epitopes, (ii) have optimal bioactivity and/or (iii) can be targeted to carefully defined populations that are most likely to benefit from them. Studies of protective neutralizing antibodies against neonatal pathogens are ongoing (99). Prior to human studies, such investigations should evaluate the activity of potential immunomodulating products in an age-specific manner including in age-specific human in vitro platforms as well as in preterm animal models.
Glutamine
Endogenous glutamine, a conditionally essential amino acid, is insufficiently biosynthesized in states of metabolic stress. Accordingly the supplementation with glutamine improved clinical outcomes in critically ill adults (100). Glutamine is abundant in human milk, but levels in formula are much lower and it is not routinely supplemented in parenteral nutrition solutions for neonates.
Despite its potential role in metabolic stress, a systematic review of 12 RCTs including 2,877 VLBW did not find any effect of preventive glutamine supplementation on mortality or major neonatal morbidities (Table 1) (91). It remains unknown, whether glutamine supplementation may be beneficial in the recovery of critically ill infants, in particular, after gastrointestinal inflammatory processes such as NEC.
Antioxidants: Selenium, Melatonin, and Vitamin A
Preterm neonates are at increased risk of oxidative stress due to lower basal levels of plasma antioxidants and metal-binding proteins (ceruloplasmin, transferrin), reduced activity of antioxidant enzymes, and higher potential for exposure to reactive oxygen species (101–103). A 2003 Cochrane review including 297 preterm neonates (<32 weeks of gestation and/or BW ≤ 2,000 g) showed a significant reduction of sepsis episodes associated with prophylactic selenium supplementation but no difference in survival (Table 2) (92). A more recent RCT confirmed these findings (104); an updated meta-analysis is pending. Thus, selenium supplementation in preterm infants might reduce the incidence of sepsis, but does not affect overall mortality.
Melatonin has antioxidant, anti-inflammatory and anti-apoptotic properties that may improve neonatal sepsis outcome, in particular in mitochondrial injury (105). Three small single-center studies investigated the use of melatonin as adjuvant therapy in neonatal sepsis and results indicate a beneficial effect of melatonin (106–108). However, so far no follow-up RCTs have been conducted, thus, firm conclusions here are precluded.
No reduction of neonatal sepsis in vitamin A-treated patients has been demonstrated so far (109), and a recent meta-analysis could not demonstrate a significant reduction of mortality in term neonates, who were supplemented with vitamin A (110). Further results of on-going clinical trials investigating the effect of vitamin A for the treatment of sepsis and NEC are still pending (111).
Granulocyte and Granulocyte-Macrophage Colony Stimulating Factors
Myeloid colony stimulating factors (CSFs), including granulocyte-macrophage CSF (GM-CSF; CSF-2) and granulocyte CSF (G-CSF; CSF-3), stimulate innate immune function, improve myelopoiesis, and limit apoptosis. (112). The clearance of apoptotic cells is essential for the resolution of inflammation and phagocytosis of apoptotic granulocytes is diminished in neonates compared to adults (113). Neonates rapidly deplete their small neutrophil pool when septic, resulting in neutropenia and Gram negative sepsis was partially reversed by administration of G-CSF in preterm infants (114, 115). Thus, a number of clinical trials investigated the effect of G-CSF and GM-SCF in the prevention and treatment of neonatal sepsis over the last decades. A 2003 Cochrane Review of seven treatment and three prophylaxis studies however, demonstrated no significant survival advantage at 14 days from the start of therapy (79) (Table 1). Of note, a subgroup analysis of 97 infants, who in addition to systemic infection, had clinically significant neutropenia at trial entry, did show a significant reduction in mortality (79). Three prophylactic studies, on the other hand, did not demonstrate significantly reduced mortality in neonates receiving GM-CSF (79). The authors concluded that due to the small sample size, there was insufficient evidence to support the introduction of either G-CSF or GM-CSF into neonatal practice, either as treatment of established systemic infection to reduce resulting mortality, or as prophylaxis to prevent systemic infection in high-risk neonates (79).
A study from 2009 investigating 280 neonates ≤ 31 weeks' gestation demonstrated that even higher doses of postnatal prophylactic GM-CSF (10 μg/kg per day administrated subcutaneously on 5 consecutive days) did not reduce sepsis or improve survival or short-term outcomes (85). When this study was pooled with the three previously published small RCTs, no significant effects of prophylactic GM-CSF on mortality or sepsis incidence were observed (79, 85) (Table 2).
In summary, available data do not support the use of G- or GM-CSF for prophylaxis of infections in neonates. This might in part be explained by a hyporesponsiveness of neonatal granulocytes to G- or GM-CSF induced anti-apoptotic effects compared to adults (116). Preterm neonates with moderate (<1,700/μL) or severe (<500/μL) neutropenia and systemic infection, however, might benefit from adjuvant treatment with G-CSF or GM-CSF, respectively (117). Optimal timing of administration and monitoring of G- or GM-CSF levels will be crucial to maximize the beneficial aspects of these cytokines in these infants.
Granulocyte Transfusions
Granulocytes of term and preterm neonates exhibit quantitative and qualitative differences compared to those of adults, which may contribute to the neonates' higher risk for developing bacterial infections (114). Treatment of neonatal sepsis with granulocyte transfusions was thus investigated to determine whether it might enhance quality and quantity of neutrophils thereby leading to improved outcome. However, no significant difference in mortality was found in infants with sepsis and neutropenia who received granulocyte transfusions when compared to placebo (Table 1) (80). Of importance, potentially severe side effects have been reported: fluid overload, transmission of blood-borne infection, graft-vs.-host disease, pulmonary complications secondary to leukocyte aggregation and sequestration and sensitization to donor erythrocyte and leukocytes (80, 101). Thus, the application of granulocyte transfusions cannot be recommended due to insufficient evidence of safety and efficacy in preterm infants.
Exchange Transfusions
Exchange transfusion may remove toxic bacterial products and potentially harmful circulating inflammatory mediators, including cytokines, in an effort to improve perfusion and tissue oxygenation, replace clotting factors, and enhance humoral immune responses.
One retrospective and one prospective single center study investigated the effect of exchange transfusions in a cohort of 101 and 83 preterm infants, respectively. In these cohorts of preterm infants with severe sepsis/septic shock no significant reduction in mortality rates was found (118, 119). Nevertheless, a trend in mortality reduction was reported (119) and another study found a statistically significant protective effect, after controlling for potential confounding factors significantly associated with death (GA, serum lactate, inotropic drugs, oligo/anuria) (118). Although hypoglycemia, electrolyte disturbances, hemodynamic instability, and thrombosis are potential complications of exchange transfusion, the authors reported no side effects (120). Thus, based on the current evidence and safety data, no firm conclusion on the recommendation of exchange transfusions for the treatment of neonatal sepsis can be made.
Recombinant Activated Human Protein C
Recombinant human activated protein C (rhAPC) possesses a broad spectrum of activity including modulation of coagulation, inflammation, and apoptosis (121). However, results among adults and children demonstrated lack of efficacy and an increased risk of bleeding associated with higher mortality rates (122, 123). Consequently, rhAPC was withdrawn from the market before any randomized trials were performed in preterm neonates, and in 2012 a clear recommendation against the treatment with rhAPC for neonatal sepsis was proclaimed (124).
Current Interventions With Clinical Evidence of Benefit
Human Milk
Human milk contains multiple distinct bioactive molecules, including antimicrobial proteins and peptides (APPs), that protect against infection and contribute to immune maturation, and healthy microbial colonization (Figure 1C) (140). Ethical limitations preclude RCTs on the topic but feeding preterm infants with their own mother's milk (MOM) offers an impressive array of benefits, including decreased rates of LOS, NEC and retinopathy of prematurity, lower rates of re-hospitalizations in the first year of life, and improved neurodevelopmental outcomes (141–144).
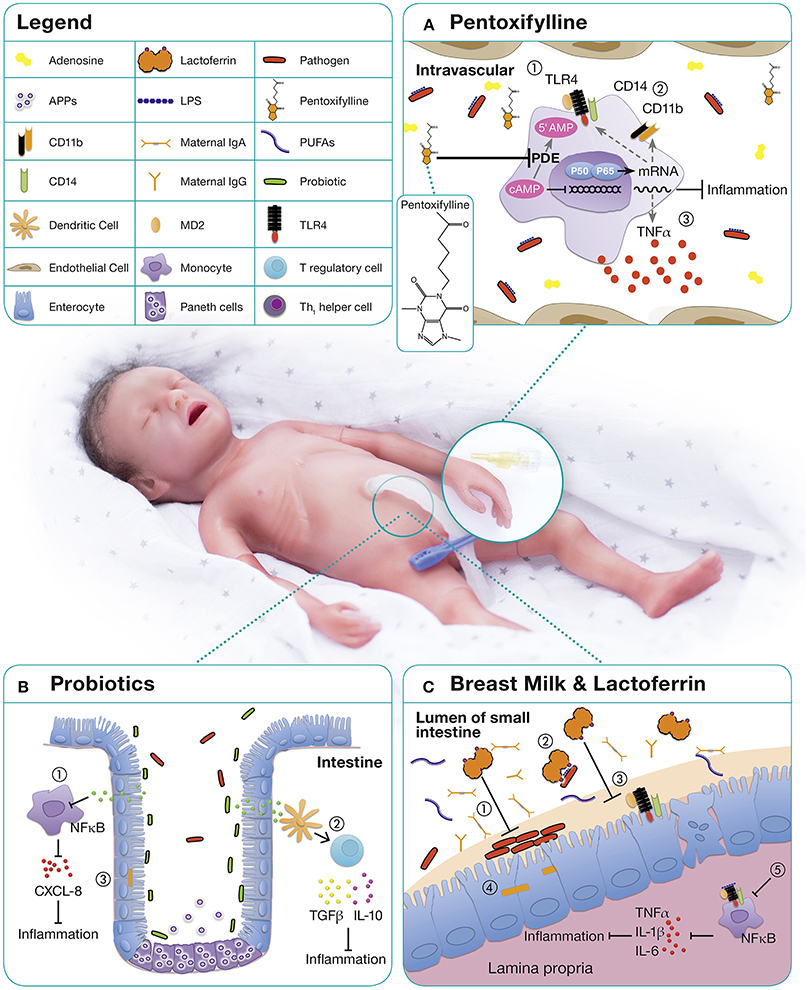
Figure 1. Immunomodulatory approaches for the treatment and prevention of neonatal sepsis. (A) PTX, a phosphodiesterase inhibitor, mediates most of its functions by enhanced cyclic AMP (cAMP) due to a reduced degradation of cAMP (125, 126). Relatively high concentrations of adenosine are present in neonatal blood plasma and neonatal mononuclear cells demonstrate increased sensitivity to the cAMP-mediated inhibitory effects of adenosine (127, 128). As immunomodulatory properties of PTX are mediated via adenosine-dependent pathways, adenosine and PTX in combination, lead to a profound inhibitory effect on pro-inflammatory cytokine production (129). On neonatal APCs, PTX demonstrates anti-inflammatory properties by (1) down-regulating TLR4 expression and signaling, (2) downregulation of surface molecules such as CD14 and CD11b, and (3) inhibition of inflammatory cytokine production (70). (B) The microbiome of premature infants has a smaller proportion of beneficial bacteria and higher numbers of pathogenic bacteria compared to term infants, likely owing to higher frequencies of cesarean sections, antibiotic use, exposure to the hospital environment, and artificial feeding (130). The administration of probiotics up-regulates local and systemic immunity by (1) decreasing proinflammatory cytokines, (2) increasing the production of anti-inflammatory cytokines, and (3) decreasing the permeability of the gut to bacteria and toxins (131). (C) Human milk contains a range of distinct bioactive molecules that protect against infection and inflammation including immunoglobulins, long-chain PUFAs, and LF. Among them, the antimicrobial and immunomodulatory effects of lactoferrin are best studied: (1) Inhibition of bacterial adhesion and biofilm formation (132–134), (2) binding of endotoxins from intestinal pathogens (135), (3) blocking of receptors essential for epithelial invasion of microbes (136) thereby (4) prevention of bacterial translocation (137), (5) promotion of anergic/anti-inflammatory effects in LPS or LTA stimulated macrophages by TLR expression and pathway interference (138, 139).
The term “human milk feeding” is frequently used to encompass both MOM and donor human milk (DHM), implying that the multiple beneficial outcomes attributed to MOM can be generalized to DHM (145, 146). This assumption, however, may only be partially correct due to differences in milk composition and processing. Preterm mothers' milk shows great variation in total protein levels and inverse correlation with lactation and daily milk volume (147). This demand-adapted regulation of protein intake, including APPs is hampered in DHM, because GA of donors' infants and infants receiving their milk might be mismatched or DHM might be pooled. More importantly, pasteurization of DHM destroys or significantly decreases the concentration of many of the protective elements in human milk, including lysozymes, secretory IgA, growth factors, lactoferrin, antioxidants, and microbiota (145, 146, 148–150). Nevertheless, improved outcomes of infants fed DHM may be primarily to avoiding potentially injurious effects of formula feeding (145, 146, 151). A meta-analysis showed that feeding with formula compared with DHM results in a higher risk of developing NEC (86). However, feeding DHM instead of formula did not significantly affect mortality or rate of invasive infection (Table 2) (86).
Prebiotics
A wide range of prebiotic components (substrates that are selectively utilized by host microorganisms conferring a health benefit) and antimicrobial and anti-inflammatory factors are delivered by breast milk (141–143). These provide passive protection to the neonate and stimulate maturation of host intestinal defenses, which are particularly relevant for premature infants (144). Prebiotic components of human milk promote the growth of a physiologic, probiotic flora including Bifidobacteria spp. and Lactobacilli spp. in the colon. Although there is currently no evidence regarding the effectiveness of the isolated application of prebiotics in preventing nosocomial sepsis in preterm infants (152), combinations of probiotics and synbiotics, i.e., a synergistic combination of probiotics and prebiotics, may be beneficial for prevention of LOS, as described below (153).
Probiotics
In healthy term infants the gut is colonized with maternal probiotic bacteria including Lactobacilla spp. and Bifidobacteria spp., which upregulate local and systemic immunity, increase anti-inflammatory cytokines, and decrease the permeability of the gut to bacteria and toxins (Figure 1B). Treatment with antibiotics and delayed enteral feeding are common in preterm infants and contribute to the development of sepsis and NEC (154). Probiotics, live non-pathogenic microorganisms, might confer a benefit to neonatal host immunity. By altering host epithelial and immunological responses (155), they may reduce several neonatal inflammatory conditions (156).
A 2014 Cochrane review of 24 randomized or quasi-RCTs evaluated the effect of probiotics in the prevention of NEC and LOS in preterm infants <37 weeks GA or <2,500 g birth weight, or both (157). Enteral probiotic supplementation significantly reduced the incidence of severe NEC ≥ stage II and mortality but initially, no evidence of significant reduction of LOS was found (157). Recent meta-analyses, including 37 RCTs (n = 9,416) have demonstrated a significant protective effect of probiotics against LOS and all cause mortality (87, 88, 158) (Table 2). The optimal composition of probiotics remains to be determined, but there is evidence that probiotics consisting of multiple strains are more effective than single-strain probiotics in preventing mortality and NEC (90).
Side effects of probiotic treatment are rare and most clinical studies did not report significant adverse effects. However, occasional cases of sepsis caused by administered probiotic species have been reported (159).
In summary, growing evidence suggests that the preventive use of probiotics reduces the risk for neonatal sepsis in preterm infants. Further studies are necessary to optimize formulation, composition, standardization and optimal dosing of probiotics.
Synbiotics
The potential benefit of synbiotics, a combination of probiotics and prebiotics is currently being investigated and so far one large RCT has been published. This recent RCT of an oral synbiotics preparation (Lactobacillus plantarum plus fructo-oligosaccharide) enrolled 4,556 infants >2,000 g or 35 weeks of gestation, in rural India and found a significant reduction of the primary outcome sepsis and death in the treatment arm (risk ratio 0.60, 95% confidence interval 0.48–0.74) (160). Preterm infants represent a major challenge in resource-poor settings where NEC and sepsis carry greater risks of death (161). The risk–benefit ratio of prophylactic probiotics or synbiotics might differ between healthcare settings. Results indicate an equal or even more pronounced beneficial effect in neonates from developing countries and support further RCTs in both, high and low resource settings.
Lactoferrin
Lactoferrin is the major whey protein in mature human milk and is present in even higher concentrations in colostrum (153). This multifunctional, 80 kDa iron-binding glycoprotein is part of the innate immune system and possesses a broad range of antimicrobial, immunostimulatory, anti-inflammatory, and anti-apoptotic properties (Figure 1C) (162).
Lactoferrin directly interacts with TLR4 and CD14 and demonstrates bacteriostatic activity through its high affinity for iron its ability to directly bind LPS (163, 164). In addition to its function as an antimicrobial protein, lactoferrin has also demonstrated immunoregulatory properties in vitro and in vivo (131, 165, 166). These studies indicate a potential role of lactoferrin as an immune-sensor to maintain immune homeostasis with an immunosuppressive effect on inflammatory monocytes/macrophages of preterm neonates (30, 131, 167).
Bovine lactoferrin (bLF) is only 69% homologous to human lactoferrin (hLF), but both serve similar biological functions (168). In RCTs the oral supplementation with bLF was associated with a decreased incidence of LOS caused by bacteria and invasive fungal infections in preterm infants (169). A recent Cochrane Review including six RCTs demonstrated that oral bLF supplementation with or without probiotics decreased LOS and NEC stage II or III but not mortality (Table 2) (75). Of note, to date no adverse effects regarding the use of bLF in human infants have been reported (75). Talactoferrin, a recombinant hLF, has been tested in a phase I study in preterm neonates (170). In contrast to bLF, talactoferrin does not need to be pasteurized and might therefore differ in function and effectiveness. Studies comparing talactoferrin and bLF remain to be done. Given the common use of probiotics, potential interactions between oral probiotics and bLF/talactoferrin should be tested (171).
Thus, optimum dosing regimens, type of lactoferrin (human or bovine), and effects on long-term outcomes still need to be defined, but lactoferrin might prove to be an effective agent in helping to prevent LOS in very preterm infants.
Zinc
Preterm infants are born with low zinc (Zn) stores and a diminished capacity for Zn absorption and retention. Zn supplementation decreases oxidative stress markers and limits pro-inflammatory cytokine production by targeting Nuclear Factor Kappa B (NF-κB) (172, 173). A RCT in 352 infants aged 7–120 days with probable serious bacterial infection showed that Zn supplementation significantly reduced treatment failure, defined as need to change antibiotics, need for intensive care, or death (174). In a RCT high doses of Zn (9.7–10.7 mg/day) reduced mortality in preterm infants (24–32 weeks) without signs of infection at initial inclusion (175). Two RCTs have evaluated the effects of enteral Zn supplementation in preterm infants (≥32 weeks) with suspected sepsis (176, 177) and one of them (177) showed a significant reduction in mortality (178). A Cochrane review of the effects of enteral Zn supplementation in preterm neonates morbidity and mortality is currently pending (179).
Pentoxifylline
Pentoxifylline (PTX), a non-specific phosphodiesterase inhibitor with immunomodulatory properties (Figure 1A), may be beneficial in preterm neonates with sepsis and NEC. PTX inhibits the production of TLR—and inflammasome-mediated inflammatory cytokines and the expression of LPS-stimulated surface markers in vitro (127, 128). PTX's effects are more pronounced in neonatal immune cells than in adults and it suppresses pro-inflammatory cytokines to a greater degree than anti-inflammatory cytokines (127, 128). This corresponds to the observation of limited clinical benefit in adult sepsis, but promising results from RCTs in neonates. Moreover, PTX's anti-inflammatory effects on PRR signaling are distinct from those of other anti-inflammatory agents such as dexamethasone and azithromycin with which PTX can act in synergy (180).
A meta-analysis of six RCTs encompassing 416 infants concluded that PTX, when used as an adjunct therapy to antibiotics in neonatal sepsis, might decrease mortality (Table 1) (77). Statistical subgroup analyses of four of these studies revealed lower mortality in preterm infants, infants with confirmed sepsis, and infants with Gram-negative sepsis. However, according to the authors, the overall quality of evidence was low (77). A recent double-blind RCT of 120 preterm infants with LOS demonstrated several beneficial adjuvant effects of PTX including a reduced length of hospital stay (p = 0.04), duration of respiratory support (p = 0.02) and less need for vasopressors (p = 0.01) but was not powered for clinical outcomes (181). Of note, no adverse effects of PTX were reported. Further in vivo studies, both in animal sepsis models and in human clinical trials, may help to define the optimal timing and dosing of PTX as well as its efficacy in improving short—and long-term outcomes following neonatal inflammation. Initiated in 2016, a randomized, placebo controlled multi-center study with a cohort size of 900 very preterm infants with LOS or NEC, is currently investigating PTX's effect on disability-free survival (Australian New Zealand Clinical Trials Registry 12616000405415).
Future Concepts With Potential Benefits
Although certain measures have indicated benefit, there remains an urgent and unmet need for novel, safe and efficacious strategies to reduce the huge burden of sepsis-related morbidity in the preterm population. We herein discuss new approaches with potential future applications that have not yet been tested beyond phase 1 clinical trials.
Maternal Immunization
Maternal immunization boosts the concentration of vaccine-specific IgG antibodies in the mother and increases antibody concentration in the infant at birth. Currently three vaccines have specific recommendations for routine use in pregnancy: tetanus, influenza and pertussis (182). Future prospects include potential development of maternal vaccines against GBS (183), cytomegalovirus (CMV) (184) and respiratory syncytial virus (RSV) (185).
In term infants, maternal vaccination may provide protection until the period of maximum susceptibility or risk has passed or until the infant has completed the routine immunizations. This benefit may be reduced in preterm infants due to reduced transplacental antibody transport before the third trimester with resulting lower antibody-concentrations compared to term infants (186). While much remains to be learned regarding the optimal timing, safety and efficacy of maternal vaccines as well as their potential effect on subsequent infant responses to vaccines (187), maternal immunization is an important strategy to substantially reduce morbidity and mortality from infectious diseases after birth.
Antimicrobial Proteins and Peptides (APPs)
Both in vitro and in vivo data support the hypothesis that APPs are important contributors to intrinsic mucosal immunity. Alterations in the level of APP expression or biologic activity can predispose the organism to microbial infection (188). Primarily released by neutrophils, monocytes, and macrophages, APPs are also produced within the skin and at mucosal surfaces by epithelial cells and thus are present within body fluids, including saliva, airway surface liquid, and breast milk (189). Circulating and intracellular levels of APPs are relatively low in early life, especially in preterm infants, potentially lessening protective immunity (190). APPs expressed in neonates include α- and β-defensins, cathelicidins, bactericidal/permeability-increasing protein (BPI), and lactoferrin (191). While some studies argue for lactoferrin in the prevention of LOS in VLBW infants (192), preclinical data also support antibacterial and anti-endotoxin properties of therapeutic APPs. For example, the recombinant bactericidal/permeability-increasing protein (rBPI21) has demonstrated beneficial effects in children with severe meningococcal sepsis (193, 194). Further research on APPs for prevention and treatment of neonatal sepsis is ongoing and has recently been reviewed in detail (190). Synthetic peptides with combined antimicrobial and immunomodulatory properties, such as clavanin-MO, an adenosine monophosphate (AMP) isolated from Styela clava, and Innate defense regulator (IDR)-1018, derived from bovine bactenecin, represent a promising approach to treat invasive infections of various bacterial strains, including multidrug-resistant hospital isolates (195, 196). Of the APPs lactoferrin (Lf) has demonstrated benefit as oral agent in the prevention of neonatal sepsis (75). Regarding other APPs, although the results of preclinical studies are encouraging, to our knowledge neonatal clinical trials have not yet been conducted.
Innate Immune Stimulants
Exposure to non-pathogenic components that augment the innate immune responses to prevent neonatal sepsis is promising, but has not yet been thoroughly investigated. Possible candidates are PRR- agonists; among them TLRAs are most extensively studied. In newborn mice, pre-treatment with TLRAs was associated with increased cytokine responses to subsequent polymicrobial infection, induced via intraperitoneal injection of a cecal slurry, with enhanced recruitment of phagocytes and reduced mortality (197). Although Th1-polarizing TLR- responses are diminished in preterm neonates compared to term neonates and adults (38, 39, 198), Th1 cytokine responses to TLR7/8 agonists such as R848 reach adult levels (37, 199). Whether any benefits confer to human neonates from TLRAs currently used as stand-alone agents (e.g., imiquimod cream, TLR7A) or as components of adjuvanted vaccines in clinical and preclinical trials in adults (200) needs to be carefully investigated in preclinical studies.
Most recently a beneficial effect of subcutaneously administered aluminum salts (alum) in the prevention of neonatal polymicrobial sepsis in mice was demonstrated (201). Alum, the most widely used adjuvant in human vaccines, does not activate TLRs but, rather, promotes caspase-1 activation and IL-1β production via the NACHT, LRR and PYD domains-containing protein 3 (NLRP3)-inflammasome (202).
Live attenuated vaccines such as Bacille Calmette–Guérin (BCG) provide inherent PRR-activating activity that may contribute to enhanced immune responses and a decreased susceptibility to invasive non-TB infections in developing countries, where BCG is routinely administered (54, 203). BCG may exert its beneficial potential through heterologous, “non-specific” effects. This heterologous innate immune protection may be due to “trained immunity,” the phenomenon whereby innate activation results in a heightened state of innate responses to a broad range of pathogens and thus broad protection, via innate memory as has been reviewed elsewhere (204). Of note, the combined administration of BCG plus the alum-adjuvanted hepatitis B vaccine (HBV) demonstrates age-dependent synergistic enhancement of IL-1ß, production potentially enhancing both innate and adaptive immune responses (205).
Thus, results from animal and human in vitro studies are indicative for a beneficial effect of innate immune stimulants in the prevention of neonatal sepsis and should be further investigated.
Stem Cells
Mesenchymal stromal cells (MSCs) are non-hematopoietic, multipotent stromal precursor cells that can be isolated from the placenta, cord blood, and Wharton's Jelly (206, 207). MSCs are capable of modulating immune responses (208) by both cell-to-cell contact and through the release of soluble paracrine factors including nitric oxide, indoleamine 2,3-dioxygenase, PGE2, TGF-β, and IL-10 (209, 210). MSCs may improve bacterial clearance by various mechanisms, including enhancement of phagocytic activity of APCs and up-regulation of TLR-2 and TLR-4, and β-defensin 2 secretion (211, 212). A comprehensive review of 18 preclinical studies published between 2009 and 2015 demonstrated that MSC therapy in animal models of sepsis significantly reduced the overall odds of death (OR 0.27, 95% CI 0.18–0.40) (213). A clinical trial of infusion of MSCs to adults demonstrated a significant increase in survival rate (214, 215). Further adult clinical trials are on-going, but to our knowledge neonatal sepsis trials have not yet been conducted (216). However, several studies currently investigate the use of umbilical cord blood derived-MSCs for the prevention and treatment of bronchopulmonary dysplasia (BPD) in human preterm infants at risk, after phase 1 trials showed an acceptable safety profile (217).
Of note, the abundance of hematopoietic stem and progenitor cells (HSPCs) in preterm compared to term cord blood, may contribute to the beneficial effects of delayed cord clamping in very preterm infants (218, 219). A recent systemic review of 2,834 preterm infants found high-quality evidence for reduced hospital mortality, but no clear evidence for a reduction of LOS in infants with delayed cord clamping (220).
Inflammasome Inhibitors
The inflammasome is a newly identified group of PRRs and specific blockage of these by small-molecule inflammasome inhibitors is a promising approach in different inflammatory conditions, including microbe-induced inflammation (221, 222). Numerous inflammasomes have been described so far; among them the (NLRP3) has been best characterized. Interactions among the three proteins of the NLRP3 inflammasome (NLRP3 protein, adapter protein apoptosis-associated speck-like protein (ASC) and procaspase-1) tightly regulate inflammasome function to ensure immune activity only when appropriate. In vivo neutralization of the NLRP3 inflammasome with an orally available small molecule inhibitor decreased inflammasome dependent cytokine secretion (IL-1β /IL-18) in a murine Staph. aureus infection, and improved the bacterial clearance through improved acidification of the phagosome (223). Newborn neonatal caspase-1/11 knockout mice, showed improved survival following septic challenge compared with wild-type mice (224). This effect was independent of NLRP3 activation (224). Despite promising results from clinical trials in adults treated with inflammasome inhibitors for inflammatory diseases other than sepsis (225, 226), more information is needed regarding the mode of inflammasome action in neonates to inform potential targeted therapeutic inhibition in this distinct age group.
Antibiotics With Anti-inflammatory Properties
Inflammation in sepsis is usually triggered by microbial components, hypoxia, arterial hypotension, and reperfusion. Some antimicrobial agents, such as β-lactam antibiotics, may exacerbate inflammation through the lysis of bacteria (227).
In contrast to proinflammatory effects of certain antibiotics that lyse bacteria, several protein synthesis inhibiting antibiotics exhibit anti-inflammatory activities. Macrolides, rifampicin, and tetracycline demonstrate anti-inflammatory and immunomodulatory properties with a potential application in systemic inflammation (228–231). Rifampicin and tetracycline are contraindicated in the neonatal period; the use of macrolides for other indications, namely, Ureaplasma spp. infection and the prevention of BPD in preterm neonates, however, has been studied (232, 233). Prophylactic azithromycin significantly reduced BPD and the composite outcome of BPD/death in preterm infants (232).
The macrolide azithromycin, specifically inhibits IL-1α and IL-1β secretion and non-canonical inflammasome activation upon TLR agonist, including LPS, stimulation in vitro (180) and in vivo (234). A decrease in IL-1β-mediated inflammation has been attributed to destabilizing mRNA levels for NALP3, a key inflammasome component (235). In a murine sepsis model, mortality was lower along with decreased sepsis scores when mice were treated with a combination of ampicillin and azithromycin instead of ampicillin alone (236). In adults, azithromycin was associated with more ICU-free days in severe sepsis patients with and without pneumonia (237), however questions on the applicability of these results in neonates remain including the consideration of side effects (238–240).
None of these new approaches has been tested in human neonates thus far and the potential in vitro and in vivo effects of new therapies need to be fully explored before any clinical studies in neonates can be performed. In addition to possibly beneficial single agent such as PTX, future studies will evaluate if a multimodal approach including a combination of immunomodulatory agents may prevent or mitigate neonatal sepsis and associated long term- morbidities in preterm infants.
Conclusion
Administration of human milk is a key approach in preventing neonatal sepsis. The use of probiotics and lactoferrin might be effective but more evidence is needed to confirm preliminary observations and to optimize formulation, composition, and dosing of these agents. In certain settings/populations, vaccination with BCG is associated with a reduction in neonatal sepsis via heterologous (“non-specific”) effects possibly related to trained immunity. With respect to treatment of neonatal sepsis, PTX holds promise, but larger studies with long-term outcome data are still pending. Several other immunotherapies evaluated for the prophylaxis of neonatal sepsis including IVIG, myeloid CSFs and granulocyte transfusions have failed to demonstrate benefit. As we look to the future, APPs, PRR-agonists, stem cell therapy and inhibitors of the inflammasome might offer new therapeutic or preventive avenues in neonatal sepsis with preclinical and clinical studies yet to be done.
The successful development of new prophylactic and treatment options should take into account age-specific immune responses. Timing of therapy and dosage may determine whether immunomodulatory agents induce a protective immune response or whether the same approach causes potentially harmful interference with the developing immune system.
Author Contributions
SS conducted the literature review and drafted the paper. EV designed the tables and assisted in drafting. BK, AS, OL, and AB contributed to critical revision of the article, assisted in drafting, and suggested additional references for inclusion in the final version. OL edited the final manuscript. All authors approved the final manuscript as submitted.
Funding
OL's laboratory is supported by U.S. National Institutes of Health (NIH) grants 1R01AI100135-01, and 3R01AI067353-05S1, the National Institutes of Allergy and Infectious Diseases (NIAID), NIH, Department of Health and Human Services, NIH UO1 award Molecular Mechanisms of Combination Adjuvants (1U01AI124284-01), Adjuvant Discovery Program Contract No. HHSN272201400052C as well as Global Health (OPPGH5284) and Grand Challenges Explorations (OPP1035192) awards from the Bill & Melinda Gates Foundation and an internal Boston Children's Hospital award to the Precision Vaccines Program. SS is supported by a Max Kade Fellowship awarded by the Austrian Academy of Science.
Conflict of Interest Statement
In addition to its major funding via the National Institutes of Health, the Levy Lab has periodically received sponsored research support from companies that develop anti-infectives, adjuvants and/or adjuvanted vaccines such as Johnson & Johnson (Janssen Pharmaceuticals), MedImmune, Shire, and 3M Drug Delivery Systems. OL is an inventor on a licensed patent on use of the antimicrobial protein rBPI21 to mitigate radiation injury and on several patent applications regarding vaccine adjuvants.
The remaining authors declare that the research was conducted in the absence of any commercial or financial relationships that could be construed as a potential conflict of interest.
Acknowledgments
We thank Tobias Strunk, Ludwig Gortner, and David Dowling for helpful discussions and suggestions. We thank Scott Lapinski for professional assistance with the literature research, Karen Pepper for proof reading and Jens-Christian Schwindt for providing a picture of a premature baby simulator. The graphical support by Sheila Ehm is gratefully appreciated. SS thanks the Max Kade Foundation for supporting her fellowship at Boston Children's Hospital.
Supplementary Material
The Supplementary Material for this article can be found online at: https://www.frontiersin.org/articles/10.3389/fped.2018.00199/full#supplementary-material
References
1. Soll RF. Progress in the care of extremely preterm infants. JAMA (2015) 314:1007–8. doi: 10.1001/jama.2015.10911
2. Stoll BJ, Hansen NI, Adams-Chapman I, Fanaroff AA, Hintz SR, Vohr B, Higgins RD. National Institute of child health and human development neonatal research network. neurodevelopmental and growth impairment among extremely low-birth-weight infants with neonatal infection. JAMA (2004) 292:2357. doi: 10.1001/jama.292.19.2357
3. Wynn JL, Wong HR, Shanley TP, Bizzarro MJ, Saiman L, Polin RA. Time for a neonatal-specific consensus definition for sepsis. Pediatr Crit Care Med. (2014) 15:523–8. doi: 10.1097/PCC.0000000000000157
4. Camacho-Gonzalez A, Spearman PW, Stoll BJ. Neonatal infectious diseases: evaluation of neonatal sepsis. Pediatr Clin North Am. (2013) 60:367–89. doi: 10.1016/j.pcl.2012.12.003
5. Hornik CP, Fort P, Clark RH, Watt K, Benjamin DK, Smith PB, et al. Early and late onset sepsis in very-low-birth-weight infants from a large group of neonatal intensive care units. Early Hum Dev. (2012) 88 (Suppl. 2):S69–74. doi: 10.1016/S0378-3782(12)70019-1
6. Stoll BJ, Hansen N, Fanaroff AA, Wright LL, Carlo WA, Ehrenkranz RA, et al. Late-onset sepsis in very low birth weight neonates: the experience of the NICHD Neonatal Research Network. Pediatrics (2002) 110:285–91. doi: 10.1542/peds.110.2.285
7. Stoll BJ, Hansen NI, Higgins RD, Fanaroff AA, Duara S, Goldberg R. Very low birth weight preterm infants with early onset neonatal sepsis: the predominance of gram-negative infections continues in the National Institute of Child Health and Human Development Neonatal Research Network, 2002-2003. Pediatr Infect Dis J. (2005) 24:635–9. doi: 10.1097/01.inf.0000168749.82105.64
8. Stoll BJ, Hansen NI, Bell EF, Shankaran S, Laptook AR, Walsh MC, et al. Neonatal Outcomes of Extremely Preterm Infants From the NICHD Neonatal Research Network. Pediatrics (2010) 126:443–56. doi: 10.1542/peds.2009-2959
9. Adams-Chapman I, Stoll BJ. Neonatal infection and long-term neurodevelopmental outcome in the preterm infant. Curr Opin Infect Dis. (2006) 19:290–7. doi: 10.1097/01.qco.0000224825.57976.87
10. Strunk T, Inder T, Wang X, Burgner D, Mallard C, Levy O. Infection-induced inflammation and cerebral injury in preterm infants. Lancet Infect Dis. (2014) 14:751–62. doi: 10.1016/S1473-3099(14)70710-8
11. Goldstein B, Giroir B, Randolph A, International Consensus Conference on Pediatric Sepsis. International pediatric sepsis consensus conference: definitions for sepsis and organ dysfunction in pediatrics*. Pediatr Crit Care Med. (2005) 6:2–8. doi: 10.1097/01.PCC.0000149131.72248.E6
12. Wynn JL. Defining neonatal sepsis. Curr Opin Pediatr. (2016) 28:135–40. doi: 10.1097/MOP.0000000000000315
13. Fanaroff AA, Korones SB, Wright LL, Verter J, Poland RL, Bauer CR, et al. Incidence, presenting features, risk factors and significance of late onset septicemia in very low birth weight infants. The National Institute of Child Health and Human Development Neonatal Research Network. Pediatr Infect Dis J. (1998) 17:593–8.
14. Shane AL, Sánchez PJ, Stoll BJ. Neonatal sepsis. Lancet (2017) 390:1770–80. doi: 10.1016/S0140-6736(17)31002-4
15. Gilfillan M, Bhandari V. Biomarkers for the diagnosis of neonatal sepsis and necrotizing enterocolitis: Clinical practice guidelines. Early Hum Dev. (2017) 105:25–33. doi: 10.1016/j.earlhumdev.2016.12.002
16. Juskewitch JE, Abraham RS, League SC, Jenkins SM, Smith CY, Enders FT, et al. Monocyte HLA-DR expression and neutrophil CD64 expression as biomarkers of infection in critically ill neonates and infants. Pediatr Res. (2015) 78:683–90. doi: 10.1038/pr.2015.164
17. Topcuoglu S, Arslanbuga C, Gursoy T, Aktas A, Karatekin G, Uluhan R, et al. Role of presepsin in the diagnosis of late-onset neonatal sepsis in preterm infants. J Matern Neonatal Med. (2015) 29:1–6. doi: 10.3109/14767058.2015.1064885
18. Dickinson P, Smith CL, Forster T, Craigon M, Ross AJ, Khondoker MR, et al. Whole blood gene expression profiling of neonates with confirmed bacterial sepsis. Genomics data (2015) 3:41–8. doi: 10.1016/j.gdata.2014.11.003
19. Wynn JL, Wong HR. Pathophysiology and treatment of septic shock in neonates. Clin Perinatol. (2010) 37:439–79. doi: 10.1016/j.clp.2010.04.002
20. Zhao J, Kim KD, Yang X, Auh S, Fu Y-X, Tang H. Hyper innate responses in neonates lead to increased morbidity and mortality after infection. Proc Natl Acad Sci USA. (2008) 105:7528–33. doi: 10.1073/pnas.0800152105
21. Boomer JS, To K, Chang KC, Takasu O, Osborne DF, Walton AH, et al. Immunosuppression in patients who die of sepsis and multiple organ failure. JAMA (2011) 306:2594–605. doi: 10.1001/jama.2011.1829
22. Raymond SL, López MC, Baker HV, Larson SD, Efron PA, Sweeney TE, et al. Unique transcriptomic response to sepsis is observed among patients of different age groups. PLoS ONE (2017) 12:e0184159. doi: 10.1371/journal.pone.0184159
23. Tang BMP, McLean AS, Dawes IW, Huang SJ, Lin RCY. Gene-expression profiling of peripheral blood mononuclear cells in sepsis*. Crit Care Med. (2009) 37:882–8. doi: 10.1097/CCM.0b013e31819b52fd
24. Wynn JL, Cvijanovich NZ, Allen GL, Thomas NJ, Freishtat RJ, Anas N, et al. The influence of developmental age on the early transcriptomic response of children with septic shock. Mol Med. (2011) 17:1146–56. doi: 10.2119/molmed.2011.00169
25. Boomer JS, Green JM, Hotchkiss RS. The changing immune system in sepsis: is individualized immuno-modulatory therapy the answer? Virulence (2014) 5:45–56. doi: 10.4161/viru.26516
26. Maslove DM, Wong HR. Gene expression profiling in sepsis: timing, tissue, and translational considerations. Trends Mol Med. (2014) 20:204–13. doi: 10.1016/j.molmed.2014.01.006
27. Hotchkiss RS, Monneret G, Payen D. Immunosuppression in sepsis: a novel understanding of the disorder and a new therapeutic approach. Lancet Infect Dis. (2013) 13:260–8. doi: 10.1016/S1473-3099(13)70001-X
28. Tang BM, Huang SJ, McLean AS. Genome-wide transcription profiling of human sepsis: a systematic review. Crit Care (2010) 14:R237. doi: 10.1186/cc9392
29. Wynn JL, Guthrie SO, Wong HR, Lahni P, Ungaro R, Lopez MC, et al. Postnatal age is a critical determinant of the neonatal host response to sepsis. Mol Med. (2015) 21:496–504. doi: 10.2119/molmed.2015.00064
30. Ulas T, Pirr S, Fehlhaber B, Bickes MS, Loof TG, Vogl T, et al. S100-alarmin-induced innate immune programming protects newborn infants from sepsis. Nat Immunol. (2017) 18:622–32. doi: 10.1038/ni.3745
31. Bianchi ME. DAMPs, PAMPs and alarmins: all we need to know about danger. J Leukoc Biol. (2006) 81:1–5. doi: 10.1189/jlb.0306164
32. Kollmann TR, Levy O, Montgomery RR, Goriely S. Innate immune function by Toll-like receptors: distinct responses in newborns and the elderly. Immunity (2012) 37:771–83. doi: 10.1016/j.immuni.2012.10.014
33. van Well GTJ, Daalderop LA, Wolfs T, Kramer BW. Human perinatal immunity in physiological conditions and during infection. Mol Cell Pediatr. (2017) 4:4. doi: 10.1186/s40348-017-0070-1
34. Dowling DJ, Levy O. Ontogeny of early life immunity. Trends Immunol. (2014) 35:299–310. doi: 10.1016/j.it.2014.04.007
35. Zilow EP, Hauck W, Linderkamp O, Zilow G. Alternative pathway activation of the complement system in preterm infants with early onset infection. Pediatr Res. (1997) 41:334–9. doi: 10.1203/00006450-199703000-00005
36. Bektas S, Goetze B, Speer CP. Decreased adherence, chemotaxis and phagocytic activities of neutrophils from preterm neonates. Acta Paediatr Scand. (1990) 79:1031–8.
37. Levy O. Innate immunity of the newborn: basic mechanisms and clinical correlates. Nat Rev Immunol. (2007) 7:379–90. doi: 10.1038/nri2075
38. Förster-Waldl E, Sadeghi K, Tamandl D, Gerhold B, Hallwirth U, Rohrmeister K, et al. Monocyte toll-like receptor 4 expression and LPS-induced cytokine production increase during gestational aging. Pediatr Res. (2005) 58:121–4. doi: 10.1203/01.PDR.0000163397.53466.0F
39. Schüller SS, Sadeghi K, Wisgrill L, Dangl A, Diesner SC, Prusa AR, et al. Preterm neonates display altered plasmacytoid dendritic cell function and morphology. J Leukoc Biol. (2013) 93:781–8. doi: 10.1189/jlb.1011525
40. Burl S, Townend J, Njie-Jobe J, Cox M, Adetifa UJ, Touray E, et al. Age-dependent maturation of Toll-like receptor-mediated cytokine responses in Gambian infants. PLoS ONE (2011) 6:e18185. doi: 10.1371/journal.pone.0018185
41. Belderbos ME, Levy O, Meyaard L, Bont L. Plasma-mediated immune suppression: a neonatal perspective. Pediatr Allergy Immunol. (2013) 24:102–13. doi: 10.1111/pai.12023
42. Power Coombs MR, Belderbos ME, Gallington LC, Bont L, Levy O. Adenosine modulates Toll-like receptor function: basic mechanisms and translational opportunities. Expert Rev Anti Infect Ther. (2011) 9:261–9. doi: 10.1586/eri.10.158
43. Haskó G, Kuhel DG, Chen JF, Schwarzschild MA, Deitch EA, Mabley JG, et al. Adenosine inhibits IL-12 and TNF-[alpha] production via adenosine A2a receptor-dependent and independent mechanisms. FASEB J. (2000) 14:2065–74. doi: 10.1096/fj.99-0508com
44. Haskó G, Cronstein BN. Adenosine: an endogenous regulator of innate immunity. Trends Immunol. (2004) 25:33–9. doi: 10.1016/j.it.2003.11.003
45. Mitchell MD, Lucas A, Etches PC, Brunt JD, Turnbull AC. Plasma prostaglandin levels during early neonatal life following term and pre-term delivery. Prostaglandins (1978) 16:319–26.
46. Kauppila A, Koivisto M, Pukka M, Tuimala R. Umbilical cord and neonatal cortisol levels. Effect of gestational and neonatal factors. Obstet Gynecol. (1978) 52:666–72.
47. Giannoni E, Guignard L, Knaup Reymond M, Perreau M, Roth-Kleiner M, Calandra T, et al. Estradiol and progesterone strongly inhibit the innate immune response of mononuclear cells in newborns. Infect Immun. (2011) 79:2690–8. doi: 10.1128/IAI.00076-11
48. Pettengill M, Robson S, Tresenriter M, Millán JL, Usheva A, Bingham T, et al. Soluble ecto-5′-nucleotidase (5′-NT), alkaline phosphatase, and adenosine deaminase (ADA1) activities in neonatal blood favor elevated extracellular adenosine. J Biol Chem. (2013) 288:27315–26. doi: 10.1074/jbc.M113.484212
49. Roger T, Schneider A, Weier M, Sweep FCGJ, Le Roy D, Bernhagen J, et al. High expression levels of macrophage migration inhibitory factor sustain the innate immune responses of neonates. Proc Natl Acad Sci USA. (2016) 113:E997–1005. doi: 10.1073/pnas.1514018113
50. Roger T, Schlapbach LJ, Schneider A, Weier M, Wellmann S, Marquis P, et al. Plasma Levels of macrophage migration inhibitory factor and d-dopachrome tautomerase show a highly specific profile in early life. Front Immunol. (2017) 8:26. doi: 10.3389/fimmu.2017.00026
51. Wynn JL, Wilson CS, Hawiger J, Scumpia PO, Marshall AF, Liu J-H, et al. Targeting IL-17A attenuates neonatal sepsis mortality induced by IL-18. Proc Natl Acad Sci USA. (2016) 113:E2627–35. doi: 10.1073/pnas.1515793113
52. Levy O, Jean-Jacques RM, Cywes C, Sisson RB, Zarember KA, Godowski PJ, et al. Critical role of the complement system in group B streptococcus-induced tumor necrosis factor alpha release. Infect Immun. (2003) 71:6344–53. doi: 10.1128/iai.71.11.6344-6353.2003
53. Ehrnström B, Beckwith KS, Yurchenko M, Moen SH, Kojen JF, Lentini G, et al. Toll-Like receptor 8 is a major sensor of group bstreptococcusbut notescherichia coliin human primary monocytes and macrophages. Front Immunol. (2017) 8:1243. doi: 10.3389/fimmu.2017.01243
54. Ugolini M, Gerhard J, Burkert S, Jensen KJ, Georg P, Ebner F, et al. Recognition of microbial viability via TLR8 drives TFH cell differentiation and vaccine responses. Nat Immunol. (2018) 19:386–96. doi: 10.1038/s41590-018-0068-4
55. Moretti J, Blander JM. Detection of a vita-PAMP STINGs cells into reticulophagy. Autophagy (2018). doi: 10.1080/15548627.2018.1441471. [Epub ahead of print].
56. Blander JM, Sander LE. Beyond pattern recognition: five immune checkpoints for scaling the microbial threat. Nat Rev Immunol. (2012) 12:215–25. doi: 10.1038/nri3167
57. Sharma AA, Jen R, Kan B, Sharma A, Marchant E, Tang A, et al. Impaired NLRP3 inflammasome activity during fetal development regulates IL-1β production in human monocytes. Eur J Immunol. (2015) 45:238–49. doi: 10.1002/eji.201444707
58. Austermann J, Friesenhagen J, Fassl SK, Petersen B, Ortkras T, Burgmann J, et al. Alarmins MRP8 and MRP14 induce stress tolerance in phagocytes under sterile inflammatory conditions. Cell Rep. (2014) 9:2112–23. doi: 10.1016/j.celrep.2014.11.020
59. Romero R, Chaiworapongsa T, Alpay Savasan Z, Xu Y, Hussein Y, Dong Z, et al. Damage-associated molecular patterns (DAMPs) in preterm labor with intact membranes and preterm PROM: a study of the alarmin HMGB1. J Matern Neonatal Med. (2011) 24:1444–55. doi: 10.3109/14767058.2011.591460
60. Strunk T, Doherty D, Jacques MBiostat A, Simmer K, Richmond P, Kohan R, et al. Histologic chorioamnionitis is associated with reduced risk of late-onset sepsis in preterm infants. Pediatrics (2012) 129:e134–41. doi: 10.1542/peds.2010-3493
61. Scheible KM, Emo J, Yang H, Holden-Wiltse J, Straw A, Huyck H, et al. Developmentally determined reduction in CD31 during gestation is associated with CD8+ T cell effector differentiation in preterm infants. Clin Immunol. (2015) 161:65–74. doi: 10.1016/j.clim.2015.07.003
62. Luciano AA, Arbona-Ramirez IM, Ruiz R, Llorens-Bonilla BJ, Martinez-Lopez DG, Funderburg N, et al. Alterations in regulatory t cell subpopulations seen in preterm infants. PLoS ONE (2014) 9:e95867. doi: 10.1371/journal.pone.0095867
63. Gervassi A, Lejarcegui N, Dross S, Jacobson A, Itaya G, Kidzeru E, et al. Myeloid derived suppressor cells are present at high frequency in neonates and suppress in vitro t cell responses. PLoS ONE (2014) 9:e107816. doi: 10.1371/journal.pone.0107816
64. Rieber N, Gille C, Köstlin N, Schäfer I, Spring B, Ost M, et al. Neutrophilic myeloid-derived suppressor cells in cord blood modulate innate and adaptive immune responses. Clin Exp Immunol. (2013) 174:45–52. doi: 10.1111/cei.12143
65. Dreschers S, Saupp P, Hornef M, Prehn A, Platen C, Morschhäuser J, et al. Reduced PICD in monocytes mounts altered neonate immune response to Candida albicans. PLoS ONE (2016) 11:e0166648. doi: 10.1371/journal.pone.0166648
66. Gille C, Leiber A, Spring B, Kempf VAJ, Loeffler J, Poets CF, et al. Diminished Phagocytosis-Induced Cell Death (PICD) in Neonatal Monocytes upon Infection with Escherichia coli. Pediatr Res. (2008) 63:33–8. doi: 10.1203/PDR.0b013e31815b8e9f
67. O'Driscoll DN, Greene CM, Molloy EJ. Immune function? A missing link in the gender disparity in preterm neonatal outcomes. Expert Rev Clin Immunol. (2017) 13:1061–71. doi: 10.1080/1744666X.2017.1386555
68. Sampath V, Mulrooney N, Patel AL, Cohen J, Zhang L, Garland J, et al. A potential role for the NOD1 Variant (rs6958571) in Gram-positive blood stream infection in ELBW infants. Neonatology (2017) 112:354–8. doi: 10.1159/000477433
69. Esposito S, Zampiero A, Pugni L, Tabano S, Pelucchi C, Ghirardi B, et al. Genetic polymorphisms and sepsis in premature neonates. PLoS ONE (2014) 9:e101248. doi: 10.1371/journal.pone.0101248
70. Amenyogbe N, Kollmann TR, Ben-Othman R. Early-life host–microbiome interphase: the key frontier for immune development. Front Pediatr. (2017) 5:111. doi: 10.3389/fped.2017.00111
71. Gerdes JS. Diagnosis and management of bacterial infections in the neonate. Pediatr Clin North Am. (2004) 51:939–59. doi: 10.1016/j.pcl.2004.03.009
72. Smith CL, Dickinson P, Forster T, Craigon M, Ross A, Khondoker MR, et al. Identification of a human neonatal immune-metabolic network associated with bacterial infection. Nat Commun. (2014) 5:4649. doi: 10.1038/ncomms5649
73. Fragiadakis GK, Baca QJ, Gherardini PF, Ganio EA, Gaudilliere DK, Tingle M, et al. Mapping the fetomaternal peripheral immune system at term pregnancy. J Immunol. (2016) 197:4482–92. doi: 10.4049/jimmunol.1601195
74. Amenyogbe N, Levy O, Kollmann TR. Systems vaccinology: a promise for the young and the poor. Philos Trans R Soc Lond B Biol Sci. (2015) 370:20140340. doi: 10.1098/rstb.2014.0340
75. Pammi M, Suresh G. Enteral lactoferrin supplementation for prevention of sepsis and necrotizing enterocolitis in preterm infants. Cochrane Database Syst Rev. (2017) 6:CD007137. doi: 10.1002/14651858.CD007137.pub5
77. Pammi M, Haque KN. Pentoxifylline for treatment of sepsis and necrotizing enterocolitis in neonates. Cochrane Database Syst Rev. (2015) 3:CD004205. doi: 10.1002/14651858.CD004205.pub3
78. Ohlsson A, Lacy JB. Intravenous immunoglobulin for suspected or proven infection in neonates. Cochrane Database Syst Rev. (2015) 3:CD001239. doi: 10.1002/14651858.CD001239.pub5
79. Carr R, Modi N, Doré C. G-CSF and GM-CSF for treating or preventing neonatal infections. Cochrane database Syst Rev. (2003) CD003066. doi: 10.1002/14651858.CD003066
80. Pammi M, Brocklehurst P. Granulocyte transfusions for neonates with confirmed or suspected sepsis and neutropenia. Cochrane Database Syst Rev. (2011) CD003956. doi: 10.1002/14651858.CD003956.pub2
81. Ohlsson A, Lacy JB. Intravenous immunoglobulin for preventing infection in preterm and/or low birth weight infants. In: Ohlsson A, editor. Cochrane Database of Systematic Reviews (Chichester: John Wiley & Sons, Ltd), (2013) CD000361. doi: 10.1002/14651858.CD000361.pub2
82. Shah PS, Kaufman DA. Antistaphylococcal immunoglobulins to prevent staphylococcal infection in very low birth weight infants. Cochrane Database Syst Rev. (2009) CD006449. doi: 10.1002/14651858.CD006449.pub2
83. Patel M, Kaufman DA. Anti-lipoteichoic acid monoclonal antibody (pagibaximab) studies for the prevention of staphylococcal bloodstream infections in preterm infants. Expert Opin Biol Ther. (2015) 15:595–600. doi: 10.1517/14712598.2015.1019857
84. Weisman LE, Thackray HM, Steinhorn RH, Walsh WF, Lassiter HA, Dhanireddy R, et al. A randomized study of a monoclonal antibody (pagibaximab) to prevent staphylococcal sepsis. Pediatrics (2011) 128:271–9. doi: 10.1542/peds.2010-3081
85. Carr R, Brocklehurst P, Doré CJ, Modi N. Granulocyte-macrophage colony stimulating factor administered as prophylaxis for reduction of sepsis in extremely preterm, small for gestational age neonates (the PROGRAMS trial): a single-blind, multicentre, randomised controlled trial. Lancet (2009) 373:226–33. doi: 10.1016/S0140-6736(09)60071-4
86. Quigley M, McGuire W. Formula versus donor breast milk for feeding preterm or low birth weight infants. Coch Database Syst Rev. (2014) CD002971. doi: 10.1002/14651858.CD002971.pub3
87. Dermyshi E, Wang Y, Yan C, Hong W, Qiu G, Gong X, et al. The “Golden Age” of probiotics: a systematic review and meta-analysis of randomized and observational studies in preterm infants. Neonatology (2017) 112:9–23. doi: 10.1159/000454668
88. Rao SC, Athalye-Jape GK, Deshpande GC, Simmer KN, Patole SK. Probiotic supplementation and late-onset sepsis in preterm infants: a meta-analysis. Pediatrics (2016) 137:e20153684. doi: 10.1542/peds.2015-3684
89. Agrawal S, Rao S, Patole S. Probiotic supplementation for preventing invasive fungal infections in preterm neonates–a systematic review and meta-analysis. Mycoses (2015) 58:642–51. doi: 10.1111/myc.12368
90. Chang H-Y, Chen J-H, Chang J-H, Lin H-C, Lin C-Y, Peng C-C. Multiple strains probiotics appear to be the most effective probiotics in the prevention of necrotizing enterocolitis and mortality: an updated meta-analysis. PLoS ONE (2017) 12:e0171579. doi: 10.1371/journal.pone.0171579
91. Moe-Byrne T, Brown JV, McGuire W. Glutamine supplementation to prevent morbidity and mortality in preterm infants. Cochrane Database Syst Rev. (2016) 4:CD001457. doi: 10.1002/14651858.CD001457.pub6
92. Darlow BA, Austin NC. Selenium supplementation to prevent short-term morbidity in preterm neonates. Cochrane Database Syst Rev. (2003) CD003312. doi: 10.1002/14651858.CD003312
93. Kaufman D, Fairchild KD. Clinical microbiology of bacterial and fungal sepsis in very-low-birth-weight infants. Clin Microbiol Rev. (2004) 17:638–80. doi: 10.1128/CMR.17.3.638-680.2004
94. van den Berg JP, Westerbeek EAM, van der Klis FRM, Berbers GAM, van Elburg RM. Transplacental transport of IgG antibodies to preterm infants: a review of the literature. Early Hum Dev. (2011) 87:67–72. doi: 10.1016/j.earlhumdev.2010.11.003
95. Shankar-Hari M, Spencer J, Sewell WA, Rowan KM, Singer M. Bench-to-bedside review: Immunoglobulin therapy for sepsis - biological plausibility from a critical care perspective. Crit Care (2012) 16:206. doi: 10.1186/cc10597
96. Benjamin DK, Schelonka R, White R, Holley HP, Bifano E, Cummings J, et al. A blinded, randomized, multicenter study of an intravenous Staphylococcus aureus immune globulin. J Perinatol. (2006) 26:290–5. doi: 10.1038/sj.jp.7211496
97. Baker CJ, Melish ME, Hall RT, Casto DT, Vasan U, Givner LB. Intravenous immune globulin for the prevention of nosocomial infection in low-birth-weight neonates. N Engl J Med. (1992) 327:213–9. doi: 10.1056/NEJM199207233270401
98. INIS Collaborative Group, Brocklehurst P, Farrell B, King A, Juszczak E, Darlow B, Haque K, et al. Treatment of neonatal sepsis with intravenous immune globulin. N Engl J Med. (2011) 365:1201–11. doi: 10.1056/NEJMoa1100441
99. Sause WE, Buckley PT, Strohl WR, Lynch AS, Torres VJ. Antibody-based biologics and their promise to combat Staphylococcus aureus infections. Trends Pharmacol Sci. (2016) 37:231–41. doi: 10.1016/j.tips.2015.11.008
100. Tao K-M, Li X-Q, Yang L-Q, Yu W-F, Lu Z-J, Sun Y-M, et al. Glutamine supplementation for critically ill adults. Cochrane Database Syst Rev. (2014) CD010050. doi: 10.1002/14651858.CD010050.pub2
101. Tarnow-Mordi W, Isaacs D, Dutta S. Adjunctive immunologic interventions in neonatal sepsis. Clin Perinatol. (2010) 37:481–99. doi: 10.1016/j.clp.2009.12.002
102. Saugstad OD. Oxidative stress in the newborn–a 30-year perspective. Biol Neonate (2005) 88:228–36. doi: 10.1159/000087586
103. Belik J, González-Luis GE, Perez-Vizcaino F, Villamor E. Isoprostanes in fetal and neonatal health and disease. Free Radic Biol Med. (2010) 48:177–88. doi: 10.1016/j.freeradbiomed.2009.10.043
104. Aggarwal R, Gathwala G, Yadav S, Kumar P. Selenium supplementation for prevention of late-onset sepsis in very low birth weight preterm neonates. J Trop Pediatr. (2016) 62:185–93. doi: 10.1093/tropej/fmv096
105. D'Angelo G, Marseglia L, Reiter RJ, Buonocore G, Gitto E. Melatonin and neonatal sepsis: a promising antioxidant adjuvant agent. Am J Perinatol. (2017) 34:1382–8. doi: 10.1055/s-0037-1604244
106. El Frargy M, El-sharkawy HM, Attia GF. Use of melatonin as an adjuvant therapy in neonatal sepsis. J Neonatal Perinatal Med. (2015) 8:227–32. doi: 10.3233/NPM-15814072
107. Gitto E, Karbownik M, Reiter RJ, Tan DX, Cuzzocrea S, Chiurazzi P, et al. Effects of melatonin treatment in septic newborns. Pediatr Res. (2001) 50:756–60. doi: 10.1203/00006450-200112000-00021
108. El-Gendy FM, El-Hawy MA, Hassan MG. Beneficial effect of melatonin in the treatment of neonatal sepsis. J Matern Fetal Neonatal Med. (2017) 31:2299–303. doi: 10.1080/14767058.2017.1342794
109. Uberos J, Miras-Baldo M, Jerez-Calero A, Narbona-López E. Effectiveness of vitamin a in the prevention of complications of prematurity. Pediatr Neonatol. (2014) 55:358–62. doi: 10.1016/j.pedneo.2013.12.002
110. Haider BA, Sharma R, Bhutta ZA. Neonatal vitamin A supplementation for the prevention of mortality and morbidity in term neonates in low and middle income countries. Cochrane Database Syst Rev. (2017) 2:CD006980. doi: 10.1002/14651858.CD006980.pub3
111. Effect of Vitamin A in the Treatment of Neonatal Sepsis and Necrotizing Enterocolitis - Full Text View - ClinicalTrials.gov. Available online at: https://clinicaltrials.gov/ct2/show/NCT00707785 [Accessed April 4, 2017]
112. Mathias B, Szpila BE, Moore FA, Efron PA, Moldawer LL. A review of GM-CSF Therapy in Sepsis. Medicine (Baltimore) (2015) 94:e2044. doi: 10.1097/MD.0000000000002044
113. Gille C, Steffen F, Lauber K, Keppeler H, Leiber A, Spring B, et al. Clearance of apoptotic neutrophils is diminished in cord blood monocytes and does not lead to reduced il-8 production. Pediatr Res. (2009) 66:507–12. doi: 10.1203/PDR.0b013e3181b9b470
114. Melvan JN, Bagby GJ, Welsh DA, Nelson S, Zhang P. Neonatal sepsis and neutrophil insufficiencies. Int Rev Immunol. (2010) 29:315–48. doi: 10.3109/08830181003792803
115. Christensen RD, Rothstein G. Exhaustion of mature marrow neutrophils in neonates with sepsis. J Pediatr. (1980) 96:316–8.
116. Molloy EJ, O'Neill AJ, Grantham JJ, Sheridan-Pereira M, Fitzpatrick JM, Webb DW, et al. Granulocyte colony-stimulating factor and granulocyte-macrophage colony-stimulating factor have differential effects on neonatal and adult neutrophil survival and function. Pediatr Res. (2005) 57:806–12. doi: 10.1203/01.PDR.0000156500.13600.B5
117. Castagnola E, Dufour C. Role of G-CSF GM-CSF in the management of infections in preterm newborns: an update. Early Hum Dev. (2014) 90:S15–17. doi: 10.1016/S0378-3782(14)50005-9
118. Pugni L, Ronchi A, Bizzarri B, Consonni D, Pietrasanta C, Ghirardi B, et al. Exchange transfusion in the treatment of neonatal septic shock: a ten-year experience in a neonatal intensive care unit. Int J Mol Sci. (2016) 17:695. doi: 10.3390/ijms17050695
119. Aradhya AS, Sundaram V, Kumar P, Ganapathy SM, Jain A, Rawat A. Double volume exchange transfusion in severe neonatal sepsis. Indian J Pediatr. (2016) 83:107–13. doi: 10.1007/s12098-015-1841-0
120. Jackson JC. Adverse events associated with exchange transfusion in healthy and ill newborns. Pediatrics (1997) 99:E7.
121. Sarangi PP, Lee H, Kim M. Activated protein C action in inflammation. Br J Haematol. (2010) 148:817–33. doi: 10.1111/j.1365-2141.2009.08020.x
122. Thachil J, Toh CH, Levi M, Watson HG. The withdrawal of Activated Protein C from the use in patients with severe sepsis and DIC [Amendment to the BCSH guideline on disseminated intravascular coagulation]. Br J Haematol. (2012) 157:493–4. doi: 10.1111/j.1365-2141.2011.09019.x
123. Nadel S, Goldstein B, Williams MD, Dalton H, Peters M, Macias WL, et al. Drotrecogin alfa (activated) in children with severe sepsis: a multicentre phase III randomised controlled trial. Lancet (2007) 369:836–43. doi: 10.1016/S0140-6736(07)60411-5
124. Kylat RI, Ohlsson A. Recombinant human activated protein C for severe sepsis in neonates. In: Kylat RI editor. Cochrane Database of Systematic Reviews (Chichester: John Wiley & Sons, Ltd), (2012) CD005385. doi: 10.1002/14651858.CD005385.pub3
125. Caccavo D, Pellegrino NM, Altamura M, Rigon A, Amati L, Amoroso A, et al. Antimicrobial and immunoregulatory functions of lactoferrin and its potential therapeutic application. J Endotoxin Res. (2002) 8:403–17. doi: 10.1179/096805102125001000
126. Elass E, Masson M, Mazurier J, Legrand D. Lactoferrin inhibits the lipopolysaccharide-induced expression and proteoglycan-binding ability of interleukin-8 in human endothelial cells. Infect Immun. (2002) 70:1860–6. doi: 10.1128/iai.70.4.1860-1866.2002
127. Schüller SS, Wisgrill L, Herndl E, Spittler A, Förster-Waldl E, Sadeghi K, et al. Pentoxifylline modulates LPS-induced hyperinflammation in monocytes of preterm infants in vitro. Pediatr Res. (2017) 82:215–25. doi: 10.1038/pr.2017.41
128. Speer EM, Dowling DJ, Ozog LS, Xu J, Yang J, Kennady G, et al. Pentoxifylline inhibits TLR- and inflammasome-mediated in vitro inflammatory cytokine production in human blood with greater efficacy and potency in newborns. Pediatr Res. (2017) 81:806–16. doi: 10.1038/pr.2017.6
129. Anaya JM, Espinoza LR. Phosphodiesterase inhibitor pentoxifylline: an antiinflammatory/immunomodulatory drug potentially useful in some rheumatic diseases. J Rheumatol. (1995) 22:595–9.
130. Sherman MP. New concepts of microbial translocation in the neonatal intestine: mechanisms and prevention. Clin Perinatol. (2010) 37:565–79. doi: 10.1016/j.clp.2010.05.006
131. Wisgrill L, Wessely I, Spittler A, Förster-Waldl E, Berger A, Sadeghi K. Human lactoferrin attenuates the proinflammatory response of neonatal monocyte-derived macrophages. Clin Exp Immunol. (2018) 192:315–24. doi: 10.1111/cei.13108
132. Kallapur SG, Nitsos I, Moss TJM, Polglase GR, Pillow JJ, Cheah F-C, et al. IL-1 mediates pulmonary and systemic inflammatory responses to chorioamnionitis induced by lipopolysaccharide. Am J Respir Crit Care Med. (2009) 179:955–61. doi: 10.1164/rccm.200811-1728OC
133. Nold MF, Mangan NE, Rudloff I, Cho SX, Shariatian N, Samarasinghe TD, et al. Interleukin-1 receptor antagonist prevents murine bronchopulmonary dysplasia induced by perinatal inflammation and hyperoxia. Proc Natl Acad Sci USA. (2013) 110:14384–9. doi: 10.1073/pnas.1306859110
134. Rudloff I, Cho SX, Bui CB, McLean C, Veldman A, Berger PJ, et al. Refining anti-inflammatory therapy strategies for bronchopulmonary dysplasia. J Cell Mol Med. (2017) 21:1128–38. doi: 10.1111/jcmm.13044
135. Liao J, Kapadia VS, Brown LS, Cheong N, Longoria C, Mija D, et al. The NLRP3 inflammasome is critically involved in the development of bronchopulmonary dysplasia. Nat Commun. (2015) 6:8977. doi: 10.1038/ncomms9977
136. Singh PK, Parsek MR, Greenberg EP, Welsh MJ. A component of innate immunity prevents bacterial biofilm development. Nature (2002) 417:552–5. doi: 10.1038/417552a
137. Elass-Rochard E, Legrand D, Salmon V, Roseanu A, Trif M, Tobias PS, et al. Lactoferrin inhibits the endotoxin interaction with CD14 by competition with the lipopolysaccharide-binding protein. Infect Immun (1998) 66:486–91.
138. Kawasaki Y, Tazume S, Shimizu K, Matsuzawa H, Dosako S, Isoda H, et al. Inhibitory effects of bovine lactoferrin on the adherence of enterotoxigenic Escherichia coli to host cells. Biosci Biotechnol Biochem. (2000) 64:348–54.
139. Ochoa TJ, Noguera-Obenza M, Ebel F, Guzman CA, Gomez HF, Cleary TG. Lactoferrin impairs type III secretory system function in enteropathogenic Escherichia coli. Infect Immun. (2003) 71:5149–55.
140. Ballard O, Morrow AL. Human milk composition: nutrients and bioactive factors. Pediatr Clin North Am. (2013) 60:49–74. doi: 10.1016/j.pcl.2012.10.002
141. Schanler RJ, Shulman RJ, Lau C. Feeding strategies for premature infants: beneficial outcomes of feeding fortified human milk versus preterm formula. Pediatrics (1999) 103:1150–7.
142. de Silva A, Jones PW, Spencer SA. Does human milk reduce infection rates in preterm infants? A systematic review. Arch Dis Child (2004) 89:F509–13. doi: 10.1136/adc.2003.045682
143. Cacho NT, Lawrence RM. Innate immunity and breast milk. Front Immunol. (2017) 8:584. doi: 10.3389/fimmu.2017.00584
144. Underwood MA. Human milk for the premature infant. Pediatr Clin North Am. (2013) 60:189–207. doi: 10.1016/j.pcl.2012.09.008
145. Cacho NT, Parker LA, Neu J. Necrotizing enterocolitis and human milk feeding: a systematic review. Clin Perinatol. (2017) 44:49–67. doi: 10.1016/j.clp.2016.11.009
146. Meier P, Patel A, Esquerra-Zwiers A. Donor human milk update: evidence, mechanisms, and priorities for research and practice. J Pediatr. (2017) 180:15–21. doi: 10.1016/j.jpeds.2016.09.027
147. Kreissl A, Zwiauer V, Repa A, Binder C, Thanhaeuser M, Jilma B, et al. Human milk analyser shows that the lactation period affects protein levels in preterm breastmilk. Acta Paediatr. (2016) 105:635–40. doi: 10.1111/apa.13348
148. Gormaz M, Torres-Cuevas I, Cernada M, Kuligowski J, Cubells E, Escobar J, et al. Role of human milk in oxidative stress associated with prematurity. J Pediatr Biochem. (2013) 3:169–77. doi: 10.3233/JPB-130090
149. Peila C, Moro GE, Bertino E, Cavallarin L, Giribaldi M, Giuliani F, et al. The effect of holder pasteurization on nutrients and biologically-active components in donor human milk: a review. Nutrients (2016) 8:477. doi: 10.3390/nu8080477
150. Daniels B, Schmidt S, King T, Israel-Ballard K, Amundson Mansen K, Coutsoudis A. The effect of simulated flash-heat pasteurization on immune components of human milk. Nutrients (2017) 9:178. doi: 10.3390/nu9020178
151. Villamor-Martínez E, Pierro M, Cavallaro G, Mosca F, Kramer BW, Villamor E. Donor human milk protects against bronchopulmonary dysplasia: a systematic review and meta-analysis. Nutrients (2018) 10:238. doi: 10.3390/nu10020238
152. Gibson GR, Hutkins R, Sanders ME, Prescott SL, Reimer RA, Salminen SJ, et al. Expert consensus document: the international scientific association for probiotics and prebiotics (ISAPP) consensus statement on the definition and scope of prebiotics. Nat Rev Gastroenterol Hepatol. (2017) 14:491–502. doi: 10.1038/nrgastro.2017.75
153. Jakaitis BM, Denning PW. Human breast milk and the gastrointestinal innate immune system. Clin Perinatol. (2014) 41:423–35. doi: 10.1016/j.clp.2014.02.011
154. Nair V, Soraisham AS. Probiotics and prebiotics: role in prevention of nosocomial sepsis in preterm infants. Int J Pediatr. (2013) 2013:874726. doi: 10.1155/2013/874726
155. Sanders ME, Guarner F, Guerrant R, Holt PR, Quigley EM, Sartor RB, et al. An update on the use and investigation of probiotics in health and disease. Gut (2013) 62:787–96. doi: 10.1136/gutjnl-2012-302504
156. Madan JC, Salari RC, Saxena D, Davidson L, O'Toole GA, Moore JH, Sogin ML, et al. Gut microbial colonisation in premature neonates predicts neonatal sepsis. Arch Dis Child Fetal Neonatal Ed. (2012) 97:F456–62. doi: 10.1136/fetalneonatal-2011-301373
157. AlFaleh K, Anabrees J. Probiotics for prevention of necrotizing enterocolitis in preterm infants. Cochrane Database Syst Rev. (2014) CD005496. doi: 10.1002/14651858.CD005496.pub4
158. Deshpande G, Jape G, Rao S, Patole S. Benefits of probiotics in preterm neonates in low-income and medium-income countries: a systematic review of randomised controlled trials. BMJ Open (2017) 7:e017638. doi: 10.1136/bmjopen-2017-017638
159. Dani C, Coviello CC, Corsini II, Arena F, Antonelli A, Rossolini GM. Lactobacillus sepsis and probiotic therapy in newborns: two new cases and literature review. AJP Rep. (2016) 6:e25–9. doi: 10.1055/s-0035-1566312
160. Panigrahi P, Parida S, Nanda NC, Satpathy R, Pradhan L, Chandel DS, et al. A randomized synbiotic trial to prevent sepsis among infants in rural India. Nature (2017) 548:407–12. doi: 10.1038/nature23480
161. Embleton ND, Zalewski S, Berrington JE. Probiotics for prevention of necrotizing enterocolitis and sepsis in preterm infants. Curr Opin Infect Dis. (2016) 29:256–61. doi: 10.1097/QCO.0000000000000269
162. Kruzel ML, Zimecki M, Actor JK. Lactoferrin in a context of inflammation-induced pathology. Front Immunol. (2017) 8:1438. doi: 10.3389/fimmu.2017.01438
163. Elass-Rochard E, Roseanu A, Legrand D, Trif M, Salmon V, Motas C, et al. Lactoferrin-lipopolysaccharide interaction: involvement of the 28-34 loop region of human lactoferrin in the high-affinity binding to Escherichia coli 055B5 lipopolysaccharide. Biochem J. (1995) 312 (Pt 3):839–45.
164. Curran CS, Demick KP, Mansfield JM. Lactoferrin activates macrophages via TLR4-dependent and -independent signaling pathways. Cell Immunol. (2006) 242:23–30. doi: 10.1016/j.cellimm.2006.08.006
165. Zimecki M, Właszczyk A, Zagulski T, Kübler A. Lactoferrin lowers serum interleukin 6 and tumor necrosis factor alpha levels in mice subjected to surgery. Arch Immunol Ther Exp. (Warsz) (1998) 46:97–104.
166. Zimecki M, Właszczyk A, Wojciechowski R, Dawiskiba J, Kruzel M. Lactoferrin regulates the immune responses in post-surgical patients. Arch Immunol Ther Exp. (Warsz) (2001) 49:325–33.
167. Kruzel ML, Actor JK, Boldogh I, Zimecki M. Lactoferrin in health and disease. Postepy Hig Med Dosw (Online) (2007) 61:261–7.
168. Pierce A, Colavizza D, Benaissa M, Maes P, Tartar A, Montreuil J, et al. Molecular cloning and sequence analysis of bovine lactotransferrin. Eur J Biochem. (1991) 196:177–84.
169. Manzoni P, Rinaldi M, Cattani S, Pugni L, Romeo MG, Messner H, et al. Bovine lactoferrin supplementation for prevention of late-onset sepsis in very low-birth-weight neonates<subtitle>a randomized trial</subtitle> JAMA (2009) 302:1421. doi: 10.1001/jama.2009.1403
170. Sherman MP, Adamkin DH, Niklas V, Radmacher P, Sherman J, Wertheimer F, et al. Randomized controlled trial of talactoferrin oral solution in preterm infants. J Pediatr. (2016) 175:68–73.e3. doi: 10.1016/j.jpeds.2016.04.084
171. Strunk T, Hibbert J, Doherty D, Granland C, Trend S, Simmer K, et al. Probiotics and antimicrobial protein and peptide levels in preterm infants. Acta Paediatr. (2017) 106:1747–53. doi: 10.1111/apa.13826
172. Gammoh NZ, Rink L. Zinc in Infection and Inflammation. Nutrients (2017) 9:624. doi: 10.3390/nu9060624
173. Prasad AS. Zinc: mechanisms of host defense. J Nutr. (2007) 137:1345–9. doi: 10.1093/jn/137.5.1345
174. Bhatnagar S, Wadhwa N, Aneja S, Lodha R, Kabra SK, Natchu UCM, et al. Zinc as adjunct treatment in infants aged between 7 and 120 days with probable serious bacterial infection: a randomised, double-blind, placebo-controlled trial. Lancet (2012) 379:2072–8. doi: 10.1016/S0140-6736(12)60477-2
175. Terrin G, Berni Canani R, Passariello A, Messina F, Conti MG, Caoci S, Smaldore A, et al. Zinc supplementation reduces morbidity and mortality in very-low-birth-weight preterm neonates: a hospital-based randomized, placebo-controlled trial in an industrialized country. Am J Clin Nutr. (2013) 98:1468–74. doi: 10.3945/ajcn.112.054478
176. Mehta K, Bhatta NK, Majhi S, Shrivastava MK, Singh RR. Oral zinc supplementation for reducing mortality in probable neonatal sepsis: a double blind randomized placebo controlled trial. Indian Pediatr. (2013) 50:390–3. doi: 10.1007/s13312-013-0120-2
177. Banupriya N, Bhat BV, Benet BD, Catherine C, Sridhar MG, Parija SC. Short term oral zinc supplementation among babies with neonatal sepsis for reducing mortality and improving outcome - a double-blind randomized controlled trial. Indian J Pediatr. (2018) 85:5–9. doi: 10.1007/s12098-017-2444-8
178. Tang Z, Wei Z, Wen F, Wu Y. Efficacy of zinc supplementation for neonatal sepsis: a systematic review and meta-analysis. J Matern Fetal Neonatal Med. (2017). doi: 10.1080/14767058.2017.1402001. [Epub ahead of print].
179. Staub E, Evers K, Askie LM. Enteral zinc supplementation for prevention of morbidity and mortality in preterm neonates (Protocol). Coch Database Syst Rev. (2017) CD012797. doi: 10.1002/14651858.CD012797
180. Speer EM, Dowling DJ, Xu J, Ozog LS, Mathew JA, Chander A, et al. Pentoxifylline, dexamethasone and azithromycin demonstrate distinct age-dependent and synergistic inhibition of TLR- and inflammasome-mediated cytokine production in human newborn and adult blood in vitro. PLoS ONE (2018) 13:e0196352. doi: 10.1371/journal.pone.0196352
181. Shabaan AE, Nasef N, Shouman B, Nour I, Mesbah A, Abdel-Hady H. Pentoxifylline therapy for late-onset sepsis in preterm infants: a randomized controlled trial. Pediatr Infect Dis J. (2015) 34:e143-8. doi: 10.1097/INF.0000000000000698
182. Jones CE, Calvert A, Le Doare K. Vaccination in pregnancy—recent developments. Pediatr Infect Dis J. (2018) 37:191–3. doi: 10.1097/INF.0000000000001822
183. Heath PT, Culley FJ, Jones CE, Kampmann B, Le Doare K, Nunes MC, et al. Group B streptococcus and respiratory syncytial virus immunisation during pregnancy: a landscape analysis. Lancet Infect Dis. (2017) 17:e223–4. doi: 10.1016/S1473-3099(17)30232-3
184. Krause PR, Bialek SR, Boppana SB, Griffiths PD, Laughlin CA, Ljungman P, et al. Priorities for CMV vaccine development. Vaccine (2013) 32:4–10. doi: 10.1016/j.vaccine.2013.09.042
185. Munoz FM, Piedra PA, Glezen WP. Safety and immunogenicity of respiratory syncytial virus purified fusion protein-2 vaccine in pregnant women. Vaccine (2003) 21:3465–7. doi: 10.1016/s0264-410x(03)00352-9
186. van den Berg JP, Westerbeek EAM, Smits GP, van der Klis FRM, Berbers GAM, van Elburg RM. Lower transplacental antibody transport for measles, mumps, rubella and varicella zoster in very preterm infants. PLoS ONE (2014) 9:e94714. doi: 10.1371/journal.pone.0094714
187. Marchant A, Sadarangani M, Garand M, Dauby N, Verhasselt V, Pereira L, et al. Maternal immunisation: collaborating with mother nature. Lancet Infect Dis. (2017) 17:e197–208. doi: 10.1016/S1473-3099(17)30229-3
188. Martin L, van Meegern A, Doemming S, Schuerholz T. Antimicrobial peptides in human sepsis. Front Immunol. (2015) 6:404. doi: 10.3389/fimmu.2015.00404
189. Huttner KM, Bevins CL. Antimicrobial peptides as mediators of epithelial host defense. Pediatr Res. (1999) 45:785–94. doi: 10.1203/00006450-199906000-00001
190. Battersby AJ, Khara J, Wright VJ, Levy O, Kampmann B. Antimicrobial proteins and peptides in early life: ontogeny and translational opportunities. Front Immunol. (2016) 7:309. doi: 10.3389/fimmu.2016.00309
191. Kai-Larsen Y, Gudmundsson GH, Agerberth B. A review of the innate immune defence of the human foetus and newborn, with the emphasis on antimicrobial peptides. Acta Paediatr. (2014) 103:1000–8. doi: 10.1111/apa.12700
192. Pammi M, Abrams SA. Oral lactoferrin for the prevention of sepsis and necrotizing enterocolitis in preterm infants. In: Pammi M editor. Cochrane Database of Systematic Reviews (Chichester: John Wiley & Sons, Ltd) (2015) CD007137. doi: 10.1002/14651858.CD007137.pub4
193. Levin M, Quint PA, Goldstein B, Barton P, Bradley JS, Shemie SD, et al. Recombinant bactericidal/permeability-increasing protein (rBPI21) as adjunctive treatment for children with severe meningococcal sepsis: a randomised trial. rBPI21 Meningococcal Sepsis Study Group. Lancet (2000) 356:961–7. doi: 10.1016/s0140-6736(00)02712-4
194. Giroir BP, Scannon PJ, Levin M. Bactericidal/permeability-increasing protein–lessons learned from the phase III, randomized, clinical trial of rBPI21 for adjunctive treatment of children with severe meningococcemia. Crit Care Med. (2001) 29:S130–5. doi: 10.1097/00003246-200107001-00039
195. Silva ON, de la Fuente-Núñez C, Haney EF, Fensterseifer ICM, Ribeiro SM, Porto WF, et al. An anti-infective synthetic peptide with dual antimicrobial and immunomodulatory activities. Sci Rep. (2016) 6:35465. doi: 10.1038/srep35465
196. Mansour SC, de la Fuente-Núñez C, Hancock REW. Peptide IDR-1018: modulating the immune system and targeting bacterial biofilms to treat antibiotic-resistant bacterial infections. J Pept Sci. (2015) 21:323–9. doi: 10.1002/psc.2708
197. Wynn JL, Scumpia PO, Winfield RD, Delano MJ, Kelly-Scumpia K, Barker T, et al. Defective innate immunity predisposes murine neonates to poor sepsis outcome but is reversed by TLR agonists. Blood (2008) 112:1750–8. doi: 10.1182/blood-2008-01-130500
198. Sharma AA, Jen R, Brant R, Ladd M, Huang Q, Skoll A, et al. Hierarchical maturation of innate immune defences in very preterm neonates. Neonatology (2014) 106:1–9. doi: 10.1159/000358550
199. Schüller S, Wisgrill L, Sadeghi K, Gindl E, Helmer H, Husslein P, et al. The TLR-specific Adjuvants R-848 and CpG-B endorse the immunological reaction of neonatal antigen presenting cells. Pediatr Res. (2016) 80:311–8. doi: 10.1038/pr.2016.71
200. Savva A, Roger T. Targeting toll-like receptors: promising therapeutic strategies for the management of sepsis-associated pathology and infectious diseases. Front Immunol. (2013) 4:387. doi: 10.3389/fimmu.2013.00387
201. Rincon JC, Cuenca AL, Raymond SL, Mathias B, Nacionales DC, Ungaro R, et al. Adjuvant pretreatment with alum protects neonatal mice in sepsis through myeloid cell activation. Clin Exp Immunol. (2017) 191:268–78. doi: 10.1111/cei.13072
202. Li H, Willingham SB, Ting JP-Y, Re F. Cutting edge: inflammasome activation by alum and alum's adjuvant effect are mediated by NLRP3. J Immunol. (2008) 181:17–21. doi: 10.4049/jimmunol.181.1.17
203. Aaby P, Roth A, Ravn H, Napirna BM, Rodrigues A, Lisse IM, et al. Randomized trial of BCG vaccination at birth to low-birth-weight children: beneficial nonspecific effects in the neonatal period? J Infect Dis. (2011) 204:245–52. doi: 10.1093/infdis/jir240
204. Levy O, Netea MG. Innate immune memory: implications for development of pediatric immunomodulatory agents and adjuvanted vaccines. Pediatr Res. (2014) 75:184–8. doi: 10.1038/pr.2013.214
205. Scheid A, Borriello F, Pietrasanta C, Christou H, Diray-Arce J, Pettengill MA, et al. Adjuvant effect of bacille calmette–guérin on hepatitis b vaccine immunogenicity in the preterm and term newborn. Front Immunol. (2018) 9:29. doi: 10.3389/fimmu.2018.00029
206. Chamberlain G, Fox J, Ashton B, Middleton J. Concise review: mesenchymal stem cells: their phenotype, differentiation capacity, immunological features, and potential for homing. Stem Cells (2007) 25:2739–49. doi: 10.1634/stemcells.2007-0197
207. Cóndor JM, Rodrigues CE, Sousa Moreira R de, Canale D, Volpini RA, Shimizu MHM, et al. Treatment with human wharton's jelly-derived mesenchymal stem cells attenuates sepsis-induced kidney injury, liver injury, and endothelial dysfunction. Stem Cells Transl Med. (2016) 5:1048–57. doi: 10.5966/sctm.2015-0138
208. Le Blanc K, Tammik L, Sundberg B, Haynesworth SE, Ringdén O. Mesenchymal stem cells inhibit and stimulate mixed lymphocyte cultures and mitogenic responses independently of the major histocompatibility complex. Scand J Immunol. (2003) 57:11–20. doi: 10.1046/j.1365-3083.2003.01176.x
209. Ren G, Zhang L, Zhao X, Xu G, Zhang Y, Roberts AI, et al. Mesenchymal stem cell-mediated immunosuppression occurs via concerted action of chemokines and nitric oxide. Cell Stem Cell (2008) 2:141–50. doi: 10.1016/j.stem.2007.11.014
210. English K, Ryan JM, Tobin L, Murphy MJ, Barry FP, Mahon BP. Cell contact, prostaglandin E(2) and transforming growth factor beta 1 play non-redundant roles in human mesenchymal stem cell induction of CD4+CD25(High) forkhead box P3+ regulatory T cells. Clin Exp Immunol. (2009) 156:149–60. doi: 10.1111/j.1365-2249.2009.03874.x
211. Krasnodembskaya A, Samarani G, Song Y, Zhuo H, Su X, Lee J-W, et al. Human mesenchymal stem cells reduce mortality and bacteremia in gram-negative sepsis in mice in part by enhancing the phagocytic activity of blood monocytes. Am J Physiol Lung Cell Mol Physiol. (2012) 302:L1003–13. doi: 10.1152/ajplung.00180.2011
212. Sung DK, Chang YS, Sung SI, Yoo HS, Ahn SY, Park WS. Antibacterial effect of mesenchymal stem cells against Escherichia coli is mediated by secretion of beta- defensin- 2 via toll- like receptor 4 signalling. Cell Microbiol. (2016) 18:424–36. doi: 10.1111/cmi.12522
213. Lalu MM, Sullivan KJ, Mei SH, Moher D, Straus A, Fergusson DA, et al. Evaluating mesenchymal stem cell therapy for sepsis with preclinical meta-analyses prior to initiating a first-in-human trial. Elife (2016) 5:e17850. doi: 10.7554/eLife.17850
214. Johnson CL, Soeder Y, Dahlke MH. Concise review: mesenchymal stromal cell-based approaches for the treatment of acute respiratory distress and sepsis syndromes. Stem Cells Transl Med. (2017) 6:1141–51. doi: 10.1002/sctm.16-0415
215. Galstian GM, Parovichnikova EN, Makarova PM, Kuzmina LA, Troitskaya VV, Gemdzhian E, et al. The results of the russian clinical trial of mesenchymal stromal cells (MSCs) in severe neutropenic patients (pts) with septic shock (SS) (RUMCESS trial). Blood (2015) 126:2220.
216. Cellular Immunotherapy for Septic Shock: A Phase I Trial - Full Text View - ClinicalTrials.gov. Available online at: https://clinicaltrials.gov/ct2/show/NCT02421484 [Accessed April 17, 2017]
217. Chang YS, Ahn SY, Yoo HS, Sung SI, Choi SJ, Oh W Il, et al. Mesenchymal stem cells for bronchopulmonary dysplasia: phase 1 dose-escalation clinical trial. J Pediatr. (2014) 164:966–72.e6. doi: 10.1016/j.jpeds.2013.12.011
218. Mercer JS, Vohr BR, McGrath MM, Padbury JF, Wallach M, Oh W. Delayed cord clamping in very preterm infants reduces the incidence of intraventricular hemorrhage and late-onset sepsis: a randomized, controlled trial. Pediatrics (2006) 117:1235–42. doi: 10.1542/peds.2005-1706
219. Wisgrill L, Schüller S, Bammer M, Berger A, Pollak A, Radke TF, et al. Hematopoietic stem cells in neonates: any differences between very preterm and term neonates? PLoS ONE (2014) 9:e106717. doi: 10.1371/journal.pone.0106717
220. Fogarty M, Osborn DA, Askie L, Seidler AL, Hunter K, Lui K, et al. Delayed vs early umbilical cord clamping for preterm infants: a systematic review and meta-analysis. Am J Obstet Gynecol. (2018) 218:1–18. doi: 10.1016/j.ajog.2017.10.231
221. Coll RC, Robertson AAB, Chae JJ, Higgins SC, Muñoz-Planillo R, Inserra MC, et al. A small-molecule inhibitor of the NLRP3 inflammasome for the treatment of inflammatory diseases. Nat Med. (2015) 21:248–55. doi: 10.1038/nm.3806
222. Shao B-Z, Xu Z-Q, Han B-Z, Su D-F, Liu C. NLRP3 inflammasome and its inhibitors: a review. Front Pharmacol. (2015) 6:262. doi: 10.3389/fphar.2015.00262
223. Cohen TS, Boland ML, Boland BB, Takahashi V, Tovchigrechko A, Lee Y, et al. S. aureus evades macrophage killing through NLRP3-dependent effects on mitochondrial trafficking. Cell Rep. (2018) 22:2431–41. doi: 10.1016/j.celrep.2018.02.027
224. Gentile LF, Cuenca AL, Cuenca AG, Nacionales DC, Ungaro R, Efron PA, et al. Improved emergency myelopoiesis and survival in neonatal sepsis by caspase-1/11 ablation. Immunology (2015) 145:300–11. doi: 10.1111/imm.12450
225. Fenini G, Contassot E, French LE. Potential of IL-1, IL-18 and Inflammasome Inhibition for the Treatment of Inflammatory Skin Diseases. Front Pharmacol. (2017) 8:278. doi: 10.3389/fphar.2017.00278
226. Ridker PM, Everett BM, Thuren T, MacFadyen JG, Chang WH, Ballantyne C, et al. Antiinflammatory therapy with canakinumab for atherosclerotic disease. N Engl J Med. (2017) 377:1119–31. doi: 10.1056/NEJMoa1707914
227. Orman KL, English BK. Effects of antibiotic class on the macrophage inflammatory response to Streptococcus pneumoniae. J Infect Dis. (2000) 182:1561–5. doi: 10.1086/315861
228. von Seth M, Sjölin J, Larsson A, Eriksson M, Hillered L, Lipcsey M. Effects of tigecycline and doxycycline on inflammation and hemodynamics in porcine endotoxemia. Shock (2015) 43:604–11. doi: 10.1097/SHK.0000000000000351
229. Krakauer T, Buckley M. Doxycycline is anti-inflammatory and inhibits staphylococcal exotoxin-induced cytokines and chemokines. Antimicrob Agents Chemother (2003) 47:3630–3. doi: 10.1128/aac.47.11.3630-3633.2003
230. Bi W, Zhu L, Jing X, Zeng Z, Liang Y, Xu A, et al. Rifampicin improves neuronal apoptosis in LPS-stimulated co-cultured BV2 cells through inhibition of the TLR-4 pathway. Mol Med Rep. (2014) 10:1793–9. doi: 10.3892/mmr.2014.2480
231. Altenburg J, de Graaff CS, van der Werf TS, Boersma WG. Immunomodulatory effects of macrolide antibiotics – part 1: biological mechanisms. Respiration (2011) 81:67–74. doi: 10.1159/000320319
232. Nair V, Loganathan P, Soraisham AS. Azithromycin and other macrolides for prevention of bronchopulmonary dysplasia: a systematic review and meta-analysis. Neonatology (2014) 106:337–47. doi: 10.1159/000363493
233. Kallapur SG, Kramer BW, Jobe AH. Ureaplasma and BPD. Semin Perinatol. (2013) 37:94–101. doi: 10.1053/j.semperi.2013.01.005
234. Gualdoni GA, Lingscheid T, Schmetterer KG, Hennig A, Steinberger P, Zlabinger GJ. Azithromycin inhibits IL-1 secretion and non-canonical inflammasome activation. Sci Rep. (2015) 5:12016. doi: 10.1038/srep12016
235. Lendermon EA, Coon TA, Bednash JS, Weathington NM, McDyer JF, Mallampalli RK. Azithromycin decreases NALP3 mRNA stability in monocytes to limit inflammasome-dependent inflammation. Respir Res. (2017) 18:131. doi: 10.1186/s12931-017-0608-8
236. Upadhyay K, Hiregoudar B, Meals E, English BK, Talati AJ. Combination therapy with ampicillin and azithromycin improved outcomes in a mouse model of group B streptococcal sepsis. PLoS ONE (2017) 12:e0182023. doi: 10.1371/journal.pone.0182023
237. Afshar M, Foster CL, Layden JE, Burnham EL. Azithromycin use and outcomes in severe sepsis patients with and without pneumonia. J Crit Care (2016) 32:120–5. doi: 10.1016/j.jcrc.2015.12.010
238. Ruiz VE, Battaglia T, Kurtz ZD, Bijnens L, Ou A, Engstrand I, et al. A single early-in-life macrolide course has lasting effects on murine microbial network topology and immunity. Nat Commun. (2017) 8:518. doi: 10.1038/s41467-017-00531-6
239. Murchison L, De Coppi P, Eaton S. Post-natal erythromycin exposure and risk of infantile hypertrophic pyloric stenosis: a systematic review and meta-analysis. Pediatr Surg Int. (2016) 32:1147–52. doi: 10.1007/s00383-016-3971-5
Keywords: neonatal sepsis, preterm infant, adjuvant sepsis therapy, immunomodulation, pentoxifylline, lactoferrin, probiotics, human milk
Citation: Schüller SS, Kramer BW, Villamor E, Spittler A, Berger A and Levy O (2018) Immunomodulation to Prevent or Treat Neonatal Sepsis: Past, Present, and Future. Front. Pediatr. 6:199. doi: 10.3389/fped.2018.00199
Received: 30 April 2018; Accepted: 25 June 2018;
Published: 19 July 2018.
Edited by:
Eric Giannoni, Centre Hospitalier Universitaire Vaudois (CHUV), SwitzerlandReviewed by:
Alessandro Borghesi, Policlinico San Matteo Fondazione (IRCCS), ItalyMartin Stocker, Luzerner Kantonsspital, Switzerland
Copyright © 2018 Schüller, Kramer, Villamor, Spittler, Berger and Levy. This is an open-access article distributed under the terms of the Creative Commons Attribution License (CC BY). The use, distribution or reproduction in other forums is permitted, provided the original author(s) and the copyright owner(s) are credited and that the original publication in this journal is cited, in accordance with accepted academic practice. No use, distribution or reproduction is permitted which does not comply with these terms.
*Correspondence: Simone S. Schüller, c2ltb25lLnNjaHVlbGxlckBtZWR1bml3aWVuLmFjLmF0
Ofer Levy, b2Zlci5sZXZ5QGNoaWxkcmVucy5oYXJ2YXJkLmVkdQ==