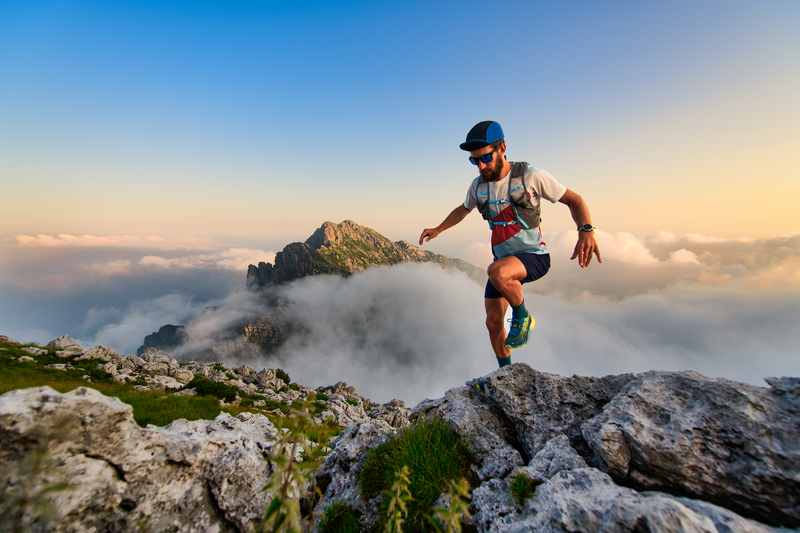
94% of researchers rate our articles as excellent or good
Learn more about the work of our research integrity team to safeguard the quality of each article we publish.
Find out more
MINI REVIEW article
Front. Pediatr. , 24 July 2018
Sec. Pediatric Immunology
Volume 6 - 2018 | https://doi.org/10.3389/fped.2018.00197
This article is part of the Research Topic Human Milk Composition and Health Outcomes in Children View all 13 articles
The incidence of pediatric asthma has increased substantially in recent decades, reaching a worldwide prevalence of 14%. This rapid increase may be attributed to the loss of “Old Friend” microbes from the human microbiota resulting in a less diverse and “dysbiotic” gut microbiota, which fails to optimally stimulate immune development during infancy. This hypothesis is supported by observations that the gut microbiota is different in infants who develop asthma later in life compared to those who remain healthy. Thus, early life exposures that influence gut microbiota play a crucial role in asthma development. Breastfeeding is one such exposure; it is generally considered protective against pediatric asthma, although conflicting results have been reported, potentially due to variations in milk composition between individuals and across populations. Human milk oligosaccharides (HMOs) and milk microbiota are two major milk components that influence the infant gut microbiota and hence, development of the immune system. Among their many immunomodulatory functions, HMOs exert a selective pressure within the infant gut microbial niche, preferentially promoting the proliferation of specific bacteria including Bifidobacteria. Milk is also a source of viable bacteria originating from the maternal gut and infant oral cavity. As such, breastmilk has prebiotic and probiotic properties that can modulate two of the main forces controlling the gut microbial community assembly, i.e., dispersal and selection. Here, we review the latest evidence, mechanisms and hypotheses for the synergistic and/or additive effects of milk microbiota and HMOs in protecting against pediatric asthma.
Breastfeeding has many established benefits for maternal and child health (1), including a potentially protective effect against pediatric asthma development. In a meta-analysis of 117 studies, Dogaru et al. found that breastfeeding was associated with a 22% reduced risk of asthma, with the strongest effects observed during early childhood (2). This association was not seen among adults in the population-based UK Biobank study (3); however, breastfeeding data were self-reported and did not account for duration or exclusivity. Several plausible mechanisms have been proposed to explain how breastfeeding might protect against asthma (4). For example, breastfeeding appears to mitigate the harmful effects of asthmogenic exposures including air pollution (5) and psychosocial stress (6). In addition, breastfeeding has been shown to support lung growth (7) and enhance lung function (8). Recently in the Canadian Healthy Infant Longitudinal Development (CHILD) Study, we reported a dose-dependent reduced risk of wheezing (9) and asthma (10) among breastfed children. These associations were stronger among infants fed at the breast compared to those receiving pumped breast milk, although both were superior to infant formula (10). This suggests that the act of suckling and/or skin-to-skin contact may contribute to the protective effect of breastfeeding. Alternatively or in addition, this finding could reflect a role for bioactive components in human milk, which may be altered during the pumping and storage process.
Breastfeeding also profoundly influences development of the infant oral and gut microbiota (11), which have been independently linked with asthma development (12, 13). Recent increases in asthma prevalence (14) have been attributed to a loss of diversity within the human microbiota due to the dramatic lifestyle changes in the last century (15). “Old Friend” and “missing microbe” hypotheses speculate that our increasingly hygienic lifestyle and overuse of antibiotics have resulted in the loss of specific bacteria from the modern day human microbiota (15, 16). Given the co-adaptation and co-evolution of the “ancient” microbiota with the human immune system, it is plausible that their loss could result in aberrant immune responses leading to allergic, autoimmune, and inflammatory diseases, including asthma (16). This hypothesis is supported by evidence that skin and nasal microbiota differ between adjacent but socioeconomically contrasting regions of Finnish and Russian Karelia with discordant prevalence of asthma and allergy (17). Consistent with this line of evidence, gut microbiota profiles have also been shown to differ between infants who do or do not develop asthma in the CHILD cohort and other longitudinal studies (12, 13). In addition, early life exposures that alter the microbiota (such as antibiotic use, cesarean delivery, and formula feeding) have been linked to asthma development (18).
Human milk oligosaccharides (HMOs) and microbiota are two major milk components that influence infant gut microbiota and hence, development of the immune system. As such, breastmilk has prebiotic and probiotic properties that can modulate two of the main forces controlling the gut microbial community assembly, i.e., dispersal (acquiring new bacterial species) and selection (achieving a permissive environment to facilitate sustainable colonization). If these processes are disrupted, the infant gut microbiota developmental trajectory will be altered, potentially leading to a suboptimal final composition. This could be one of the underlying mechanisms of predisposition to a range of chronic diseases including allergy and asthma (19) (Figure 1). Here, we review the latest evidence and hypothesized mechanisms for the synergistic and/or additive effects of HMOs and milk microbiota in protecting against pediatric asthma.
Figure 1. Hypothesized pathways of association between breastfeeding and lung health. Breastfeeding influences infant gut microbiota development and stability during the critical developmental period in early life via two of its main components: human milk oligosaccharides (HMOs) and milk microbiota. HMOs exert a selective pressure within the infant gut microbial niche, preferentially promoting the proliferation of specific bacteria including Bifidobacteria. Milk is also a source of viable bacteria originating from the maternal gut and infant oral cavity. HMO composition is influenced by maternal genetics, geography, and season while microbiota is affected by maternal weight status, mode of breastfeeding and infant sex (Table 1). Variations in HMOs and milk microbiota could modulate the effect of breastfeeding on the infant gut microbiota, which in turn shapes the infant immune system and could ultimately influence lung health and asthma development.
Breastfeeding affects both gut microbiota and immune system development (20, 21). Human milk functions as a bioactive food consisting of all essential nutrients plus immune components, hormones, HMOs, and microbiota, which serve crucial roles in early life metabolic and immune system homeostasis and development (22). HMOs and the microbiota are of particular interest because of their influence on the infant gut microbiota and potential long-term health importance (22) (Table 1).
Table 1. Evidence on factors influencing the composition of human milk oligosaccharides (HMOs) and milk microbiota and their association with pediatric asthma.
HMOs constitute the third largest component of human milk (31). These structurally diverse carbohydrates are synthesized by sequential addition of monosaccharides to lactose, and various α-glycosidic linkages of fucose and/or sialic acid to the core molecules (23, 36). More than 100 different HMOs have been identified, with the amount and composition varying substantially between women and over the course of lactation (25, 28, 37, 38). HMO fucosylation is regulated by enzymes encoded by the fucosyltransferase 2 (FUT2) and FUT3 genes, which determine secretor status and Lewis blood group status, respectively (23, 24). However, geographical variation in HMO composition suggests that non-genetic factors such as sociocultural and environmental factors may also play a role (24, 25). In the CHILD cohort we have observed that, beyond genetic FUT2 secretor status, HMO composition is associated with ethnicity, lactation stage, parity, geographic location, season of collection, and breastfeeding exclusivity (26).
Although still an emerging field of research, many biological functions have been attributed to HMOs (24, 31). The majority of ingested HMOs reach the lower intestinal tract where they can function as prebiotics, providing selective substrates for gut bacteria (30), as discussed below. Preliminary evidence also suggests that specific HMOs could directly modulate the immune response, with studies in pigs (39) and mice (40, 41) demonstrating direct effects on viral pathogens as well as host immune cells. In breastfed infants, approximately 1% of HMOs are absorbed into the peripheral circulation, potentially reaching all organs including the lungs (31), thus it is plausible that HMOs could affect lung mucosal immunity by interacting with airway epithelia, immune cells, potential pathogens or resident microbes, providing another mechanism for protecting against asthma.
While the mechanisms are not fully elucidated, there is some evidence that supplementation with prebiotics (42, 43) or HMOs (44) may be protective against allergy and asthma in animal models and human studies. A recent systematic review reported a reduction in asthma among high-risk infants given prebiotics, such as galacto-oligosaccharide (GOS) and fructo-oligosaccharide (FOS) (45). However, GOS and FOS are structurally distinct from HMOs, which have not been widely studied in relation to asthma, although a few studies have examined their association with allergic disease. One study found that infants receiving milk with low Lacto-N-fucopentaose III concentrations were more likely to develop cow's milk allergy (33), an effect that might be modulated by birth mode (29). In the CHILD cohort, we have observed that HMO composition (but not any individual HMO) is associated with the development of allergic sensitization during infancy (26). Associations with asthma during early childhood are currently being explored. Altogether, epidemiological and experimental studies support the prebiotic effects of HMOs and suggest a potential role in asthma development (Table 1), but further research is needed to confirm and characterize this relationship.
Culture-dependent and independent studies have confirmed the presence of bacteria in human milk (46). It is estimated that breastfed infants receive 104-106 bacteria per day (based on an average daily consumption of 800 mL of milk) with most isolated species belonging to the genera Staphylococcus, Streptococcus, Lactobacillus, and Bifidobacterium (47). Culture-independent (DNA sequencing-based) approaches in the CHILD cohort (34) and others (48) have recovered a higher diversity of bacteria in breast milk including lactic acid bacteria (Enterococcus and Lactococcus), oral-derived (Veillonella and Gemella), skin-associated (Cutibacterium and Staphylococcus), and environmental bacteria (Pseudomonas and Sphingomonas) with a high degree of inter-individual variability. While bacterial load appears to remain constant during milk maturation (49), it gradually decreases during one feed (50).
The milk microbiota is suggested to originate from the maternal gut, breast tissue, or infant oral cavity (51). Depending on the source of bacteria, different factors may contribute to shaping the milk microbiota (Table 1). For example, while maternal factors could influence the mother's gut bacteria, early life factors such as mode of delivery and mode of breastfeeding (directly at the breast vs. expressed and bottled breast milk) could potentially alter the exogenous bacteria derived from the infant (22). In the CHILD cohort, we have found that mode of breastfeeding was significantly associated with milk microbiota composition, with expressed milk feeding favoring depletion of Bifidobacteria and enrichment with potential pathogens and environmental bacteria (34). We also observed some sex-specific associations (e.g., maternal BMI associated with milk microbiota only if the infant is female) while other factors demonstrated a phylum-specific effect (e.g., maternal atopy associated with Actinobacteria richness) (34). As discussed below, the milk microbiota is suggested to provide a mechanism of vertical microbial transmission from the mother to the infant.
To the best of our knowledge, no study to date has directly linked milk microbiota with pediatric asthma and allergy. However, we have previously reported that indirect breastfeeding was associated with higher risk of pediatric asthma in the CHILD study (10), and we have also observed that mode of breastfeeding is consistently associated with milk microbiota composition in this cohort (34). It is plausible that the milk microbiota could play a role in asthma development, conceivably via the modulation of gut microbiota. Studies to date have been inconclusive regarding the use of commercial probiotics for preventing asthma (52); however, the natural milk microbiota is a complex community, and hence, may be more effective in this regard, as it has presumably evolved to optimally support infant gut microbiota and immune development.
Dietary exposures are among the most influential factors shaping the infant gut microbiota. Breastmilk not only provides nutrients to the infant, but is also a source of probiotics (milk microbiota) and prebiotics (HMOs) contributing to the establishment of the infant gut microbiota (11). The human gut microbiota develops through a complex process of stepwise successions beginning at birth (53). First colonizers of the infant gut are facultative anaerobes, including the Staphylococcus, Streptococcus, and Enterococcus genera, and the Enterobacteriaceae family. These pioneering bacteria reduce the redox potential and hence, create a favorable condition for the growth of obligate anaerobes such as the Bifidobacterium, Bacteroides, Clostridium, and Eubacterium genera (53). In contrast to the mature gut microbiota, the infant gut is dominated by Bifidobacterium species prior to weaning, including B. longum, B. breve, and B. bifidum (35, 54). HMOs are specifically utilized by Bacteroides and Bifidobacterium spp. (30) leading to dominance of these taxa in breastfed infants (55), with different Bifidobacterium species and strains demonstrating different preferences for specific HMOs (56). Furthermore, rates of absorption and excretion are different for different categories of HMOs (57), resulting in different amounts reaching the colon; further highlighting the nuanced impact of the HMO composition on the infant gut microbiota. HMOs could also indirectly affect the gut microbiota composition through their decoy activity that prevents pathogen colonization (31). Additionally, HMOs can modify host-microbe interactions by affecting epithelial cell turnover (32) and glycocalyx mucus formation (58).
In addition to the prebiotic role of HMOs, the viability of milk bacteria suggests that human milk could function as a probiotic shaping the infant gut microbiota by facilitating dispersal (22). Milk microbiota may provide pioneering species and impact the final gut microbiota composition (59). Human microbiota studies demonstrating strain similarities between maternal gut, milk, and infant gut support this hypothesis (54), and find that Bifidobacterium spp. constitute the majority of shared taxa between maternal milk and infant stool (35). It is plausible that human milk specifically enriches and protects “beneficial” bacteria as they are transported through the infant's acidic stomach environment to the lower intestinal tract. Milk could also function as an incubator of bacteria to increase their “dose,” thereby increasing the likelihood of successful colonization in the infant gut (60).
The precise role of milk microbiota and HMOs in shaping the infant gut microbiota and immune development is an active area of research with potential implications for asthma prevention. The gastrointestinal tract is the largest immune organ in the human body and it plays a crucial role in the education and subsequent maturation of immune system by (i) maintaining immunological tolerance to food components as well as the commensal microbiota, and (ii) acquiring the capacity to appropriately respond to pathogenic microbes (61, 62). Moreover, the immune system is essential in keeping the balance of bacterial communities and preventing dysbiosis (61, 63). This host-microbiota crosstalk is shaped early in life (63, 64). It has been hypothesized and experimentally demonstrated that alteration of the early gut microbiota can disrupt the microbially-mediated mechanisms of immunological tolerance, resulting in predisposition to allergic disorders including asthma (61, 65).
Development of the immune system begins in utero, and continues during infancy (18). At birth, the adaptive immune system is dominated by the T-helper cell type 2 (Th2) which will later shift to Th1 and Th17 phenotype (66, 67). Delayed or impaired Th2/Th1 transition during early infancy is associated with increased risk of atopic disorders, including asthma (18). It has been shown that the gut microbiota stimulates regulatory T cells that help monitor the Th1/Th2 balance (18, 61, 62). Furthermore, exposure to microbes or microbial products such as microbe-associated molecular patterns (MAMPs) or short chain fatty acids (SCFA) during the early infancy is essential for stimulating the infant's naïve and immature immune system (53, 67), gut-associated lymphoid tissue development (64, 66), and B cell maturation (68). Thus, early life microbiota disruption could lead to alterations in several immune parameters including regulatory T cells proliferation, Th17 response, and IgE response (53), all of which contribute to asthma development.
A growing body of evidence supports the role of gut microbiota in the development of allergic disorders (64, 69, 70). However, it is not clear whether the risk of asthma development is modulated by presence of specific bacteria, temporal succession patterns, or the overall taxonomic and functional diversity of the microbial community (53). Observational human studies have indicated that lower gut microbiota diversity early in life could be associated with higher risk of asthma at later time points (12). In contrast, gut microbiota diversity and overall community composition were not associated with atopic wheezing in the CHILD cohort, although lower relative abundances of specific bacterial genera including Faecalibacterium, Lachnospira, Veillonella, and Rothia were associated with this phenotype (13). These genera were further shown to reduce clinical features of asthma including respiratory tract neutrophil and lymphocyte infiltrations and histopathological alterations in a mouse model of ovalbumin-induced asthma (13). Similarly, other studies have found that higher relative abundances of specific taxa including Clostridium difficile and Clostridium neonatale in the infant gut microbiota are associated with an increased risk of asthma (18, 71–74). Despite the discrepancies between studies on the important microbial features potentially associated with asthma, they have consistently shown that gut microbiota status in infancy, more so than early childhood, is associated with an altered risk of asthma. Collectively, this evidence supports the existence of a “critical period” during which the gut microbiota shapes the infant immune landscape and thus susceptibility to asthma later in life (70, 75).
Breastfeeding profoundly influences the infant gut microbiota, and emerging evidence indicates that divergence from this evolutionarily conserved process can alter immune system maturation and influence asthma development. However, much remains to be discovered about the underlying mechanisms, including the prebiotic and probiotic properties of human milk. For example, we do not fully understand how HMOs and milk microbiota modulate the overall gut microbial community and whether this involves many or just a few key taxa. Moreover, there is tremendous variation in HMO utilization by different bacterial strains, and some HMOs are not utilized by the gut microbiota. The source and fate of bacteria in human milk is also uncertain. HMOs and milk microbiota may influence infant microbial communities at body sites other than the gut, including the airways and nasopharyngeal cavity, which may also play a role in asthma development. In addition to HMOs and microbiota, other milk components likely also impact the gut microbiota and/or the immune system, including cytokines, antimicrobial compounds, and immunoglobulins. These diverse components have largely been studied in isolation, yet they co-exist and interact in the mammary gland and the infant gut. New methods and integrated approaches will be required to disentangle the complex and dynamic interactions between HMOs, milk microbiota, and other milk components and their collective impact on the infant gut microbiota, immune function, and pediatric asthma. Understanding these processes will help define the role of breastfeeding and human milk in normal development, and could ultimately inform new microbiota-based strategies for asthma prevention.
All authors contributed to the conception and design of this review and wrote sections of the manuscript. SM and MA compiled and integrated the individual sections. All authors contributed to manuscript revision, read and approved the submitted version.
MA holds a Canada Research Chair in the Developmental Origins of Chronic Disease. SM holds a Research Manitoba Doctoral Studentship. Our human milk research program is supported by the Heart and Stroke Foundation and Canadian Lung Association Emerging Research Leaders Initiative, in partnership with the Canadian Respiratory Research Network and the Allergy, Genes and Environment Network of Centers of Excellence (AllerGen NCE), and by Research Manitoba. These entities had no role in the design and conduct of this review, or approval of the manuscript.
The authors declare that the research was conducted in the absence of any commercial or financial relationships that could be construed as a potential conflict of interest.
We have used the following Creative Commons icons from the Noun Project (https://thenounproject.com). Artists' names are in brackets: baby (James Bickerton), DNA (Lloyd Humphreys), gender symbols (Guilhem), globe (Ben Davis), increase (Gregor Cresnar), lungs (Brennan Novak), obesity and 1 month baby (Gan Khoon Lay), and seasons (Marie Van den Broeck).
1. Binns C, Lee M, Low WY. The long-term public health benefits of breastfeeding. Asia Pac J Public Health (2016) 28:7–14. doi: 10.1177/1010539515624964
2. Dogaru CM, Nyffenegger D, Pescatore AM, Spycher BD, Kuehni CE. Breastfeeding and childhood asthma: systematic review and meta-analysis. Am J Epidemiol. (2014) 179:1153–67. doi: 10.1093/aje/kwu072
3. Ek WE, Karlsson T, Hernándes CA, Rask-Andersen M, Johansson Å. Breast-feeding and risk of asthma, hay fever, and eczema. J Allergy Clin Immunol. (2018) 141:1157–9.e9. doi: 10.1016/j.jaci.2017.10.022
4. Oddy WH. Breastfeeding, Childhood Asthma, and Allergic Disease. Ann Nutr Metab. (2017) 70 (Suppl. 2):26–36. doi: 10.1159/000457920
5. Dong GH, Qian ZM, Liu MM, Wang D, Ren WH, Bawa S, et al. Breastfeeding as a modifier of the respiratory effects of air pollution in children. Epidemiology (2013) 24:387–94. doi: 10.1097/EDE.0b013e3182877eb8
6. Montgomery SM, Ehlin A, Sacker A. Breast feeding and resilience against psychosocial stress. Arch Dis Child. (2006) 91:990–4. doi: 10.1136/adc.2006.096826
7. Dogaru CM, Narayanan M, Spycher BD, Pescatore AM, Owers-Bradley J, Beardsmore CS, et al. Breastfeeding, lung volumes and alveolar size at school-age. BMJ Open Respir Res. (2015) 2:e000081. doi: 10.1136/bmjresp-2015-000081
8. Waidyatillake NT, Allen KJ, Lodge CJ, Dharmage SC, Abramson MJ, Simpson JA, et al. The impact of breastfeeding on lung development and function: a systematic review. Expert Rev Clin Immunol. (2013) 9:1253–65. doi: 10.1586/1744666X.2013.851005
9. Azad MB, Vehling L, Lu Z, Dai D, Subbarao P, Becker AB, et al. Breastfeeding, maternal asthma and wheezing in the first year of life: a longitudinal birth cohort study. Eur Respir J. (2017) 49:1602019. doi: 10.1183/13993003.02019-2016
10. Klopp A, Vehling L, Becker AB, Subbarao P, Mandhane PJ, Turvey SE, et al. Modes of infant feeding and the risk of childhood asthma: a prospective birth cohort study. J Pediatr. (2017) 190:192–9. doi: 10.1016/j.jpeds.2017.07.012
11. Bode L, McGuire M, Rodriguez JM, Geddes DT, Hassiotou F, Hartmann PE, et al. It's alive: microbes and cells in human milk and their potential benefits to mother and infant. Adv Nutr. (2014) 5:571–3. doi: 10.3945/an.114.006643
12. Abrahamsson TR, Jakobsson HE, Andersson AF, Bjorksten B, Engstrand L, Jenmalm MC. Low gut microbiota diversity in early infancy precedes asthma at school age. Clin Exp Allergy (2014) 44:842–50. doi: 10.1111/cea.12253
13. Arrieta MC, Stiemsma LT, Dimitriu PA, Thorson L, Russell S, Yurist-Doutsch S, et al. Early infancy microbial and metabolic alterations affect risk of childhood asthma. Sci Transl Med. (2015) 7:307ra152. doi: 10.1126/scitranslmed.aab2271
14. Subbarao P, Mandhane PJ, Sears MR. Asthma: epidemiology, etiology and risk factors. CMAJ (2009) 181:E181–90. doi: 10.1503/cmaj.080612
15. Blaser MJ, Falkow S. What are the consequences of the disappearing human microbiota? Nat Rev Microbiol. (2009) 7:887–94. doi: 10.1038/nrmicro2245
16. Rook GA. 99th Dahlem conference on infection, inflammation and chronic inflammatory disorders: darwinian medicine and the ‘hygiene’ or ‘old friends’ hypothesis. Clin Exp Immunol. (2010) 160:70–9. doi: 10.1111/j.1365-2249.2010.04133.x
17. Ruokolainen L, Paalanen L, Karkman A, Laatikainen T, von Hertzen L, Vlasoff T, et al. Significant disparities in allergy prevalence and microbiota between the young people in Finnish and Russian Karelia. Clin Exp Allergy (2017) 47:665–74. doi: 10.1111/cea.12895
18. Azad MB, Kozyrskyj AL. Perinatal programming of asthma: the role of gut microbiota. Clin Dev Immunol. (2012) 2012:932072. doi: 10.1155/2012/932072
19. Gomez-Gallego C, Garcia-Mantrana I, Salminen S, Collado MC. The human milk microbiome and factors influencing its composition and activity. Semin Fetal Neonatal Med. (2016) 21:400–5. doi: 10.1016/j.siny.2016.05.003
20. Andreas NJ, Kampmann B, Mehring Le-Doare K. Human breast milk: a review on its composition and bioactivity. Early Hum Dev. (2015) 91:629–35. doi: 10.1016/j.earlhumdev.2015.08.013
21. Ardeshir A, Narayan NR, Mendez-Lagares G, Lu D, Rauch M, Huang Y, et al. Breast-fed and bottle-fed infant rhesus macaques develop distinct gut microbiotas and immune systems. Sci Transl Med. (2014) 6:252ra120. doi: 10.1126/scitranslmed.3008791
22. Munblit D, Peroni DG, Boix-Amoros A, Hsu PS, Van't Land B, Gay MCL, et al. Human milk and allergic diseases: an unsolved puzzle. Nutrients (2017) 9:E894. doi: 10.3390/nu9080894
23. Kunz C, Rudloff S, Baier W, Klein N, Strobel S. Oligosaccharides in human milk: structural, functional, and metabolic aspects. Annu Rev Nutr. (2000) 20:699–722. doi: 10.1146/annurev.nutr.20.1.699
24. Smilowitz JT, Lebrilla CB, Mills DA, German JB, Freeman SL. Breast milk oligosaccharides: structure-function relationships in the neonate. Annu Rev Nutr. (2014) 34:143–69. doi: 10.1146/annurev-nutr-071813-105721
25. McGuire MK, Meehan CL, McGuire MA, Williams JE, Foster J, Sellen DW, et al. What's normal? Oligosaccharide concentrations and profiles in milk produced by healthy women vary geographically. Am J Clin Nutr. (2017) 105:1086–100. doi: 10.3945/ajcn.116.139980
26. Miliku K, Robertson B, Sharma AK, Subbarao P, Becker AB, Mandhane PJ, et al. Human milk oligosaccharide profiles and food sensitization among infants in the CHILD Study. Allergy (2018) doi: 10.1111/all.13476. [Epub ahead of print].
27. Davis JC, Lewis ZT, Krishnan S, Bernstein RM, Moore SE, Prentice AM, et al. Growth and morbidity of gambian infants are influenced by maternal milk oligosaccharides and infant gut microbiota. Sci Rep. (2017) 7:40466. doi: 10.1038/srep40466
28. Chaturvedi P, Warren CD, Altaye M, Morrow AL, Ruiz-Palacios GP, Newburg LK. D. S. Fucosylated human milk oligosaccharides vary between individuals and over the course of lactation. Glycobiology (2001) 11:365–72. doi: 10.1093/glycob/11.5.365
29. Sprenger N, Odenwald H, Kukkonen AK, Kuitunen M, Savilahti E, Kunz C. FUT2-dependent breast milk oligosaccharides and allergy at 2 and 5 years of age in infants with high hereditary allergy risk. Eur J Nutr. (2017) 56:1293–301. doi: 10.1007/s00394-016-1180-6
30. Marcobal A, Sonnenburg JL. Human milk oligosaccharide consumption by intestinal microbiota. Clin Microbiol Infect. (2012) 18(Suppl. 4):12–5. doi: 10.1111/j.1469-0691.2012.03863.x
31. Bode L. The functional biology of human milk oligosaccharides. Early Hum Dev. (2015) 91:619–22. doi: 10.1016/j.earlhumdev.2015.09.001
32. Kuntz S, Rudloff S, Kunz C. Oligosaccharides from human milk influence growth-related characteristics of intestinally transformed and non-transformed intestinal cells. Br J Nutr. (2008) 99:462–71. doi: 10.1017/S0007114507824068
33. Seppo AE, Autran CA, Bode L, Jarvinen KM. Human milk oligosaccharides and development of cow's milk allergy in infants. J Allergy Clin Immunol. (2017) 139:708–11.e5. doi: 10.1016/j.jaci.2016.08.031
34. Moossavi S, Khafipour E, Sepehri S, Robertson B, Bode L, Becker AB, et al. Maternal and early life factors influencing the human milk microbiota in the child cohort. In: Poster Session Presented at: Canadian Society of Microbiololists. Waterloo, ON (2017).
35. Biagi E, Quercia S, Aceti A, Beghetti I, Rampelli S, Turroni S, et al. The bacterial ecosystem of mother's milk and infant's mouth and gut. Front Microbiol. (2017) 8:1214. doi: 10.3389/fmicb.2017.01214
36. Boehm G, Stahl B. Oligosaccharides from milk. J Nutr. (2007) 137(3 Suppl. 2):847s−9s. doi: 10.1093/jn/137.3.847S
37. Xu G, Davis JC, Goonatilleke E, Smilowitz JT, German JB, Lebrilla CB. Absolute quantitation of human milk oligosaccharides reveals phenotypic variations during lactation. J Nutr. (2017) 147:117–24. doi: 10.3945/jn.116.238279
38. Bode L. Human milk oligosaccharides: every baby needs a sugar mama. Glycobiology (2012) 22:1147–62. doi: 10.1093/glycob/cws074
39. Comstock SS, Wang M, Hester SN, Li M, Donovan SM. Select human milk oligosaccharides directly modulate peripheral blood mononuclear cells isolated from 10-d-old pigs. Br J Nutr. (2014) 111:819–28. doi: 10.1017/S0007114513003267
40. Schijf MA, Kruijsen D, Bastiaans J, Coenjaerts FE, Garssen J, van Bleek GM, et al. Specific dietary oligosaccharides increase Th1 responses in a mouse respiratory syncytial virus infection model. J Virol. (2012) 86:11472–82. doi: 10.1128/JVI.06708-11
41. Pandey RP, Kim DH, Woo J, Song J, Jang SH, Kim JB, et al. Broad-spectrum neutralization of avian influenza viruses by sialylated human milk oligosaccharides: in vivo assessment of 3'-sialyllactose against H9N2 in chickens. Sci Rep. (2018) 8:2563. doi: 10.1038/s41598-018-20955-4
42. Vos AP, van Esch BC, Stahl B, M'Rabet L, Folkerts G, Nijkamp FP, et al. Dietary supplementation with specific oligosaccharide mixtures decreases parameters of allergic asthma in mice. Int Immunopharmacol. (2007) 7:1582–7. doi: 10.1016/j.intimp.2007.07.024
43. Arslanoglu S, Moro GE, Boehm G, Wienz F, Stahl B, Bertino E. Early neutral prebiotic oligosaccharide supplementation reduces the incidence of some allergic manifestations in the first 5 years of life. J Biol Regulators Homeost Agents (2012) 26(3 Suppl.):49–59. doi: 10.1088/2058-7058/26/08/11
44. Castillo-Courtade L, Han S, Lee S, Mian FM, Buck R, Forsythe P. Attenuation of food allergy symptoms following treatment with human milk oligosaccharides in a mouse model. Allergy (2015) 70:1091–102. doi: 10.1111/all.12650
45. Osborn DA, Sinn JK. Prebiotics in infants for prevention of allergy. Cochrane Database Syst Rev. (2013) CD006474. doi: 10.1002/14651858.CD006474
46. Fitzstevens JL, Smith KC, Hagadorn JI, Caimano MJ, Matson AP, Brownell EA. Systematic review of the human milk microbiota. Nutr Clin Pract. (2016) 32:354–64. doi: 10.1177/0884533616670150
47. Heikkila MP, Saris PEJ. Inhibition of Staphylococcus aureus by the commensal bacteria of human milk. J Appl Microbiol. (2003) 95:471–8. doi: 10.1046/j.1365-2672.2003.02002.x
48. McGuire MK, McGuire MA. Got bacteria? The astounding, yet not-so-surprising, microbiome of human milk. Curr Opin Biotechnol. (2017) 44:63–8. doi: 10.1016/j.copbio.2016.11.013
49. Collado MC, Laitinen K, Salminen S, Isolauri E. Maternal weight and excessive weight gain during pregnancy modify the immunomodulatory potential of breast milk. Pediatr Res. (2012) 72:77–85. doi: 10.1038/pr.2012.42
50. West PA, Hewitt JH, Murphy OM. The influence of methods of collection and storage on the bacteriology of human milk. J Appl Bacteriol. (1979) 46:269–77.
51. Fernandez L, Langa S, Martin V, Maldonado A, Jimenez E, Martin R, et al. The human milk microbiota: origin and potential roles in health and disease. Pharmacol Res. (2013) 69:1–10. doi: 10.1016/j.phrs.2012.09.001
52. Azad MB, Coneys JG, Kozyrskyj AL, Field CJ, Ramsey CD, Becker AB, et al. Probiotic supplementation during pregnancy or infancy for the prevention of asthma and wheeze: systematic review and meta-analysis. BMJ (2013) 347:f6471. doi: 10.1136/bmj.f6471
53. Laforest-Lapointe I, Arrieta MC. Patterns of early-life gut microbial colonization during human immune development: an ecological perspective. Front Immunol. (2017) 8:788. doi: 10.3389/fimmu.2017.00788
54. Asnicar F, Manara S, Zolfo M, Truong DT, Scholz M, Armanini F, et al. Studying vertical microbiome transmission from mothers to infants by strain-level metagenomic profiling. mSystems (2017) 2:e00164–16. doi: 10.1128/mSystems.00164-16
55. Wang M, Li M, Wu S, Lebrilla CB, Chapkin RS, Ivanov I, et al. Fecal microbiota composition of breast-fed infants is correlated with human milk oligosaccharides consumed. J Pediatr Gastroenterol Nutr. (2015) 60:825–33. doi: 10.1097/MPG.0000000000000752
56. Ruiz-Moyano S, Totten SM, Garrido DA, Smilowitz JT, German JB, Lebrilla CB, et al. Variation in consumption of human milk oligosaccharides by infant gut-associated strains of bifidobacterium breve. Appl Environ Microbiol. (2013) 79:6040–9. doi: 10.1128/AEM.01843-13
57. Davis JC, Totten SM, Huang JO, Nagshbandi S, Kirmiz N, Garrido DA, et al. Identification of oligosaccharides in feces of breast-fed infants and their correlation with the gut microbial community. Mol Cell Proteomics (2016) 15:2987–3002.
58. Angeloni S, Ridet JL, Kusy N, Gao H, Crevoisier F, Guinchard S, et al. Glycoprofiling with micro-arrays of glycoconjugates and lectins. Glycobiology (2005) 15:31–41. doi: 10.1093/glycob/cwh143
59. Fukami T. Historical contingency in community assembly: integrating niches, species pools, and priority effects. Annu Rev Ecol Evol Syst. (2015) 46:1–23. doi: 10.1146/annurev-ecolsys-110411-160340
60. Obadia B, Guvener ZT, Zhang V, Ceja-Navarro JA, Brodie EL, Ja WW, et al. Probabilistic Invasion Underlies Natural Gut Microbiome Stability. Curr Biol. (2017) 27:1999–2006 e8. doi: 10.1016/j.cub.2017.05.034
61. Li M, Wang M, Donovan SM. Early development of the gut microbiome and immune-mediated childhood disorders. Semin Reprod Med. (2014) 32:74–86. doi: 10.1055/s-0033-1361825
62. Kaplan JL, Shi HN, Walker WA. The role of microbes in developmental immunologic programming. Pediatr Res. (2011) 69:465–72. doi: 10.1203/PDR.0b013e318217638a
63. Wang M, Monaco MH, Donovan SM. Impact of early gut microbiota on immune and metabolic development and function. Semin Fetal Neonatal Med. (2016) 21:380–7. doi: 10.1016/j.siny.2016.04.004
64. Tanaka M, Nakayama J. Development of the gut microbiota in infancy and its impact on health in later life. Allergol Int. (2017) 66:515–22. doi: 10.1016/j.alit.2017.07.010
65. Huffnagle GB. The microbiota and allergies/asthma. PLoS Pathog. (2010) 6:e1000549. doi: 10.1371/journal.ppat.1000549
66. Arrieta MC, Stiemsma LT, Amenyogbe N, Brown EM, Finlay B. The intestinal microbiome in early life: health and disease. Front Immunol. (2014) 5:427. doi: 10.3389/fimmu.2014.00427
67. Gollwitzer ES, Marsland BJ. Impact of early-life exposures on immune maturation and susceptibility to disease. Trends Immunol. (2015) 36:684–96. doi: 10.1016/j.it.2015.09.009
68. Lundell AC, Bjornsson V, Ljung A, Ceder M, Johansen S, Lindhagen G, et al. Infant B cell memory differentiation and early gut bacterial colonization. J Immunol. (2012) 188:4315–22. doi: 10.4049/jimmunol.1103223
69. Fujimura KE, Lynch SV. Microbiota in allergy and asthma and the emerging relationship with the gut microbiome. Cell Host Microbe. (2015) 17:592–602. doi: 10.1016/j.chom.2015.04.007
70. Gensollen T, Blumberg RS. Correlation between early-life regulation of the immune system by microbiota and allergy development. J Allergy Clin Immunol. (2017) 139:1084–91. doi: 10.1016/j.jaci.2017.02.011
71. Penders J, Thijs C, van den Brandt PA, Kummeling I, Snijders B, Stelma F, et al. Gut microbiota composition and development of atopic manifestations in infancy: the KOALA Birth Cohort Study. Gut (2007) 56:661–7. doi: 10.1136/gut.2006.100164
72. Vael C, Vanheirstraeten L, Desager KN, Goossens H. Denaturing gradient gel electrophoresis of neonatal intestinal microbiota in relation to the development of asthma. BMC Microbiol. (2011) 11:68. doi: 10.1186/1471-2180-11-68
73. Stiemsma LT, Arrieta MC, Dimitriu PA, Cheng J, Thorson L, Lefebvre DL, et al. Shifts in Lachnospira and Clostridium sp. in the 3-month stool microbiome are associated with preschool age asthma. Clin Sci. (2016) 130:2199–207. doi: 10.1042/CS20160349
74. Kalliomaki M, Kirjavainen P, Eerola E, Kero P, Salminen S, Isolauri E. Distinct patterns of neonatal gut microflora in infants in whom atopy was and was not developing. J Allergy Clin Immunol. (2001) 107:129–34. doi: 10.1067/mai.2001.111237
Keywords: human milk, microbiota, human milk oligosaccharides, immune development, asthma, pediatrics, probiotic, prebiotic
Citation: Moossavi S, Miliku K, Sepehri S, Khafipour E and Azad MB (2018) The Prebiotic and Probiotic Properties of Human Milk: Implications for Infant Immune Development and Pediatric Asthma. Front. Pediatr. 6:197. doi: 10.3389/fped.2018.00197
Received: 01 May 2018; Accepted: 19 June 2018;
Published: 24 July 2018.
Edited by:
Daniel Munblit, I.M. Sechenov First Moscow State Medical University, RussiaReviewed by:
Yuying Liu, University of Texas Health Science Center at Houston, United StatesCopyright © 2018 Moossavi, Miliku, Sepehri, Khafipour and Azad. This is an open-access article distributed under the terms of the Creative Commons Attribution License (CC BY). The use, distribution or reproduction in other forums is permitted, provided the original author(s) and the copyright owner(s) are credited and that the original publication in this journal is cited, in accordance with accepted academic practice. No use, distribution or reproduction is permitted which does not comply with these terms.
*Correspondence: Meghan B. Azad, bWVnaGFuLmF6YWRAdW1hbml0b2JhLmNh
Disclaimer: All claims expressed in this article are solely those of the authors and do not necessarily represent those of their affiliated organizations, or those of the publisher, the editors and the reviewers. Any product that may be evaluated in this article or claim that may be made by its manufacturer is not guaranteed or endorsed by the publisher.
Research integrity at Frontiers
Learn more about the work of our research integrity team to safeguard the quality of each article we publish.