- 1Center for Human Genetics, Bioscientia, Ingelheim, Germany
- 2Department of Medicine, University Hospital Freiburg, Freiburg, Germany
Autosomal recessive polycystic kidney disease (ARPKD) is a hepatorenal fibrocystic disorder that is characterized by enlarged kidneys with progressive loss of renal function and biliary duct dilatation and congenital hepatic fibrosis that leads to portal hypertension in some patients. Mutations in the PKHD1 gene are the primary cause of ARPKD; however, the disease is genetically not as homogeneous as long thought and mutations in several other cystogenes can phenocopy ARPKD. The family history usually is negative, both for recessive, but also often for dominant disease genes due to de novo arisen mutations or recessive inheritance of variants in genes that usually follow dominant patterns such as the main ADPKD genes PKD1 and PKD2. Considerable progress has been made in the understanding of polycystic kidney disease (PKD). A reduced dosage of disease proteins leads to the disruption of signaling pathways underlying key mechanisms involved in cellular homeostasis, which may help to explain the accelerated and severe clinical progression of disease course in some PKD patients. A comprehensive knowledge of disease-causing genes is essential for counseling and to avoid genetic misdiagnosis, which is particularly important in the prenatal setting (e.g., preimplantation genetic diagnosis/PGD). For ARPKD, there is a strong demand for early and reliable prenatal diagnosis, which is only feasible by molecular genetic analysis. A clear genetic diagnosis is helpful for many families and improves the clinical management of patients. Unnecessary and invasive measures can be avoided and renal and extrarenal comorbidities early be detected in the clinical course. The increasing number of genes that have to be considered benefit from the advances of next-generation sequencing (NGS) which allows simultaneous analysis of a large group of genes in a single test at relatively low cost and has become the mainstay for genetic diagnosis. The broad phenotypic and genetic heterogeneity of cystic and polycystic kidney diseases make NGS a particularly powerful approach for these indications. Interpretation of genetic data becomes the challenge and requires deep clinical understanding.
Polycystic Kidney Disease and Ciliary Dysfunction
Polycystic kidney disease (PKD) is a clinically and genetically heterogeneous group of disorders with phenotypes ranging from manifestation in utero to clinically silent disease well into adulthood. PKD can be inherited in a dominant (ADPKD) and recessive (ARPKD) way. In addition to ADPKD and ARPKD, cystic kidney disease is a common feature of syndromic ciliopathies. Patients with ciliopathies represent a significant proportion of all patients with end stage renal disease (ESRD). In addition to fibrocystic renal changes, patients often present with extrarenal manifestations (1–7). Cystic kidneys require careful clinical workup to identify the underlying genetic disorder, especially in children. In recent years, our understanding of the mechanistic basis of polycystic kidney disease has increased substantially. It has been shown that PKD-associated proteins co-localize in multimeric complexes at distinct subcellular epithelial sites. Ultrastructural analysis reveals that PKD-associated proteins localize to the primary cilium and suggests that dysfunctional primary cilia may be the source of the underlying etymology of cystic kidney disease (1–4, 8). Cilia are microtubule-based organelles that project from the surface of most quiescent vertebrate cells, and function in chemo- and mechanosensation and fluid transport. The ciliary axoneme is anchored in the cell by the centriole-derived basal body and is ensheathed by the ciliary membrane. Located between the basal body and axoneme, the transition zone (TZ) forms a diffusion barrier that modulates the selective passage of proteins to and from the cilium (9–12). The insights gained in recent years have led to approval of Tolvaptan (Jinarc/Samsca) for ADPKD in many countries and also to the establishment of multiple clinical trials evaluating emerging therapeutic strategies that may have the potential for rational personalized therapies in future years (13).
An accurate diagnosis is essential both in the management of patients with cystic kidneys and in counseling their families. When an effort is made to classify the wide array of different entities with renal cysts, knowledge about the family history, the clinical phenotype, location and morphology of the cysts, and any possible extrarenal manifestations should help in making a diagnosis. Conventional heritable cystic kidney diseases include ADPKD and ARPKD, as well as nephronophthisis (NPHP), medullary cystic kidney disease, and glomerulocystic kidney disease. Other rare ciliopathies that may affect multiple tissues need to be taken into consideration.
Prevalence and Recurrence Risk
ARPKD occurs in about 1 in 20,000 live births among Caucasians. This corresponds to a carrier frequency of approximately 1:70 in non-isolated populations (14–16). The exact incidence is unknown since published studies vary in the cohorts of patients examined (e.g., autopsied patients vs. moderately affected patients followed by pediatricians), and some severely affected babies may die perinatally without a definitive diagnosis. Isolated or inbred populations with many consanguineous marriages may have much higher prevalence, e.g., Kääriäinen reported an incidence of 1:8,000 in Finland (17). Notably, among children with polycystic kidneys, the total number of patients with early-onset ADPKD may be comparable to those of children with ARPKD.
Given its autosomal recessive mode of inheritance, the recurrence risk for subsequent pregnancies of parents of an affected child is 25%. Males and females are equally affected. As indicated by formal genetics, unaffected siblings harbor a two-thirds risk of being a carrier for ARPKD. By definition, heterozygous carriers do not show any clinical disease manifestations, however may have an increased risk for polycystic liver disease (PLD). Healthy siblings, other relatives, and patients themselves seeking genetic counseling for their own family planning can usually be reassured of a low risk for offspring with ARPKD when neither the partner is related with the index family nor a case of ARPKD is known in the partner’s pedigree. At an assumed heterozygosity rate of 1:70, the estimated risk is 1:140 for offspring of patients, 1:420 for offspring of patients’ healthy siblings, and 1:560 for offspring of patients’ healthy uncles/aunts.
Clinical Course
Clinically, ARPKD typically presents much earlier and is more severe than ADPKD. The majority of cases are identified at birth or late in pregnancy. Affected fetuses display a “Potter” phenotype with massively enlarged kidneys, pulmonary hypoplasia, a characteristic facies, and contracted limbs with club feet. Approximately 30–50% of affected neonates die shortly after birth from respiratory insufficiency due to pulmonary hypoplasia and thoracic compression by the excessively enlarged kidneys. However, the clinical spectrum is often more variable than generally presumed. While the majority of cases are identified at birth or late in pregnancy, there have been some reported cases of elderly people with ARPKD being only moderately affected (18, 19) (Figure 1).
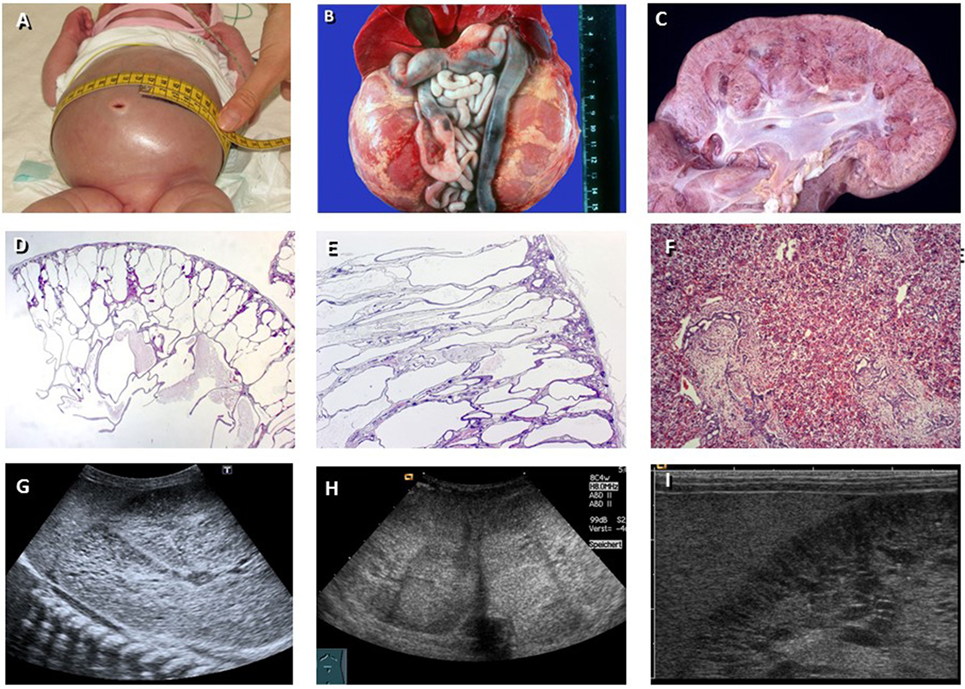
Figure 1. Autosomal recessive polycystic kidney disease (ARPKD). (A) Baby with distended abdomen due to voluminous kidneys that lead to respiratory problems. (B) Abdominal situs of a perinatally demised ARPKD patient with symmetrically enlarged kidneys that maintain their reniform configuration. (C) Cross section of an ARPKD kidney with cortical extension of fusiform and cylindrical spaces arranged radially throughout the renal parenchyma from medulla to cortex. (D,E) Microscopically, fusiform dilations of renal collecting ducts and distal tubuli lined by columnar or cuboidal epithelium. These dilated collecting ducts run perpendicular to the renal capsule. (F) Obligatory hepatobiliary changes in ARPKD known as ductal plate malformation characterized by dysgenesis of the hepatic portal triad with hyperplastic biliary ducts and congenital hepatic fibrosis. (G–I) Renal ultrasound of young children with ARPKD demonstrating enlarged echogenic kidneys with fusiform dilations of collecting ducts and distal tubules arranged radially throughout the renal parenchyma from medulla to cortex.
Ultrasound scans typically show bilaterally enlarged hyperechoic kidneys with poor corticomedullary differentiation, retained reniform contour, and multiple tiny cysts confined to distal tubules and collecting ducts (Figure 1). With advancing clinical course, the kidney structure might increasingly resemble the pattern observed in ADPKD with renal cysts that vary considerably in size and appearance, often also accompanied by some degree of interstitial fibrosis (20). Arterial hypertension, often difficult to control despite multi-drug treatment, often develops during the first months of life and affects up to 80% of children with ARPKD. Close monitoring of blood pressure is essential as hypertension can exacerbate already deteriorating renal function (19).
In addition to renal manifestations and other comorbidities which characterize the early stages of ARPKD, patients necessarily develop some form of histologic liver involvement explaining why the entity is also entitled “Polycystic Kidney and Hepatic Disease 1 (PKHD1).” These histological changes are a result of defective remodeling of the ductal plate leading to biliary duct ectasia and hepatic fibrosis collectively known as ductal plate malformation (DPM) (7, 21) (Figure 1F). DPM is also a common feature of other ciliopathies. Biliary anomalies may develop at any stage of the physiologic involution-remodeling process, and the timing or stage of development determines the resulting clinical and histological phenotype (22).
Outside of the portal tracts, the remaining structures of the liver develop normally in ARPKD. In contrast to similar diseases, such as HNF1β, enzyme levels are typically within normal ranges, with the exception of cholestasis parameters, which may be elevated. In older patients, hepatobiliary complications are more significant causes of morbidity. DPM leads to progressive fibrosis of the liver resulting in portal hypertension. This can lead to a number of complications such as hypersplenism and esophageal varices (15, 19). ARPKD is one of the two major indications for combined liver and kidney transplantation during childhood. The best timing and strategy for combined transplantation is still a matter of debate and usually requires individualized decision-making on a case by case basis. Moreover, there is some preliminary evidence that adult ARPKD patients beyond the age of 40 years may have a slightly increased risk to develop hepatic tumors, especially cholangiocarcinoma (21, 23). While a preponderance of ARPKD patients show a consistent disease progression, individual patients may present with atypical phenotypes, such as exclusive or predominant phenotypes of either the liver or kidneys. Accordingly, in certain cases of isolated congenital hepatic fibrosis (CHF) (Caroli’s disease), PKHD1 has been demonstrated to be the causative gene (24). Similarly, in mouse models for Pkhd1, the liver phenotype is stronger than renal involvement (25–28). Overall, ARPKD is associated with a significant portion of liver and kidney associated morbidity in children, resulting in diminished life expectancy.
PKHD1 Gene and Its Encoded Protein Polyductin/Fibrocystin
In 2002, PKHD1 was identified as the main gene for ARPKD (29, 30). PKHD1 is among the largest disease genes in the human genome, extending over a genomic segment of at least 470 kb and including a minimum of 86 exons. Both PKHD1 and its murine ortholog undergo a complex and extensive pattern of alternative splicing, generating transcripts highly variable in size. In accordance with the disease phenotype, the gene is highly expressed in fetal and adult kidney and at lower levels in liver (30, 31). Weak expression is present in other tissues too, including pancreas and arterial wall. The longest PKHD1 transcript contains 67 exons encoding a protein of 4,074 amino acids.
The predicted full-length protein (termed fibrocystin or polyductin) represents a novel integral membrane protein with a signal peptide at the amino terminus of its extensive, highly glycosylated extracellular domain, a single transmembrane (TM)-spanning segment, and a short cytoplasmic C-terminal tail containing potential protein kinase A phosphorylation sites (Figure 2). Fibrocystin localizes to the ciliary membrane where it is thought to facilitate ciliary targeting signals through an 18 amino acid cytoplasmic tail (32). In line with its proposed role as a ciliary-localized membrane protein, an 18-residue motif in the cytoplasmic tail serves as a ciliary targeting signal (32). The ~3,860 amino acid extracellular portion contains several IPT and IPT-like domains similar to those found in some cell surface receptors and transcription factors. Between the IPT domains and the TM segment, multiple PbH1 repeats are present, a motif also found in polysaccharidases that may bind to carbohydrate moieties such as glycoproteins on the cell surface and/or in the basement membrane. The structural characteristics of PKHD1 and the histological defects found in human ARPKD patients suggest that fibrocystin may be involved in regulating cell–cell adhesion and proliferation. Additionally, its predicted functional domains, membrane localization, and TM characteristics suggest that it may act as a membrane-bound receptor (30).
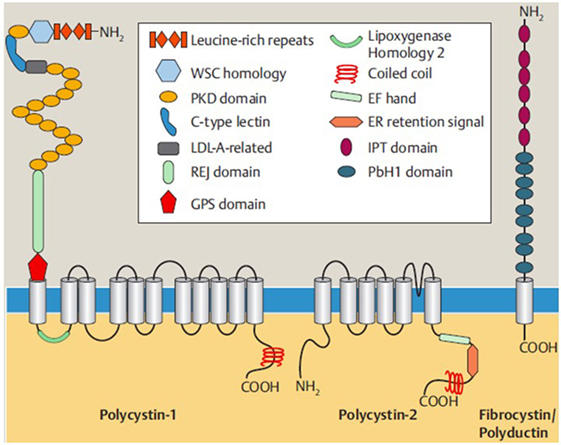
Figure 2. Shown are the structures of polycystin-1, polycystin-2, and fibrocystin/polyductin, the main proteins for ADPKD and ARPKD that form a protein network in polycystic kidney disease and interact with each other. Polycystin-1 and polycystin-2 are ciliary multipass transmembrane proteins, while fibrocystin/polyductin represents a type 1-membrane protein, each with an intracellular C-terminal end. The proteins’ functions are still not fully understood, but roles, e.g., as mechanoreceptor involved in cell cycle regulation, planar cell polarity, and cell–cell and cell–matrix interactions have been discussed. Polycystin-2 (also known as TRPP2) is a member of the TRP (transient receptor potential) channel family and a non-selective cation channel important for Ca2+-homeostasis, etc. Both for polycystin-1 and for fibrocystin/polyductin, processed isoforms in the cytoplasma and the nucleus have been described that underlie regulated intramembrane proteolysis and may serve as transcription factors [from Bergmann (33); 9:151–180].
Like polycystin-1 and polycystin-2, fibrocystin is localized to the primary cilium and basal body. During renal development, fibrocystin localizes to the apical aspect of nephronic precursors and becomes concentrated at the basal body during the early stages of ciliogenesis (12, 34–37). This spatio-temporal subcellular localization in conjunction with its interaction with polycystin-2 and CAML suggest that fibrocystin might be involved in microtubule organization and/or in mechano- or chemosensation, key functions of the primary cilia (38).
In addition, there is preliminary evidence of fibrocystin isoproteins which may be secreted in exosomes and undergo posttranslational processing (12, 34–37, 39, 40). However, the number of alternative PKHD1 transcripts that are actually translated into protein and exert biological function(s) is as yet unknown. In order to fully understand the role that individual isoforms play in the maintenance of renal and hepatobiliary function, we will need to improve our understanding of fibrocystin in the pathophysiology of ARPKD. The relatively even distribution of described mutations along the entire length of PKHD1 suggests that the full transcript is required for proper fibrocystin function. It could be hypothesized that a certain amount of full-length functional protein is required for normal function. Alternatively, it could be the case that mutations disrupt the relative abundance of different functional isoforms of fibrocystin, which are otherwise maintained in strict stoichiometric ratios by splicing patterns.
PKHD1 Mutation Spectrum
The considerable size of PKHD1, its presumably complex splicing pattern, and our limited understanding of the protein’s function(s) present challenges to DNA-based diagnostic testing.
Further requirements for investigation are set by the extensive allelic heterogeneity with a high number of missense mutations and private mutations in non-isolate populations (19, 41–46). Thus, we decided to set up a locus-specific database for PKHD1 that helps in the assessment of changes. Homozygous or compound heterozygous mutations in PKHD1 are found. In approximately 80% of ARPKD patients, ranging from individuals with perinatal demise to moderately affected adults (19, 45–48). At least one mutation is even found in more than 95% of families screened. One reason for missing mutations may have been the limited sensitivity of the screening methods (e.g., SSCP, DHPLC) used in earlier studies. Moreover, silent exonic changes and intronic sequence variations may also have an effect on PKHD1 splicing, e.g., by affecting splice enhancer or silencer sites (49). Functional and mRNA studies are usually needed to prove a possible pathogenic effect of such changes (50). Mutations may also be “hidden” in non-coding regulatory elements making them difficult to detect. In addition, the PKHD1 gene might also be susceptible to genomic rearrangements (51). However, in patients with only one PKHD1 variant or without any detectable PKHD1 mutation, differential diagnoses of ARPKD should be considered. With the advance of next-generation sequencing (NGS) based testing options, many patients primarily diagnosed as ARPKD have been re-classified. It is also becoming increasingly apparent that the characteristics of ARPKD can be mimicked by mutations in a number of other genes (52). Moreover, ARPKD is not as homogeneous as widely proposed and evidence for genetic heterogeneity has been found in a subset of families.
The most common PKHD1 mutation is c.107C>T (p.Thr36Met) in exon 3 that accounts for approximately 15–20% of mutated alleles (43). It is under debate whether this incidence is a result of a common ancestral mutation or a result of separate selective pressures. Given its frequency in certain populations, such as Central European populations, the former hypothesis cannot be dismissed. However, analysis of a multitude of unrelated families from varying ethnic groups from various haplotypes identified c.107C>T as a mutational “hotspot,” suggesting that factors outside of common ancestry likely account for at least some of the incidence of this mutation (19).
Overall, marked allelic heterogeneity characterizes the mutational spectrum in PKHD1 with the majority of variants unique to single families in “non-isolate” populations. Given the size of the PKHD1 gene and relative absence of mutational hotspots, conventional PKHD1 molecular testing by Sanger sequencing is time-consuming and cumbersome (53, 54). NGS streamlines this process and makes molecular genetic diagnostics much more time- and cost-efficient (52). Moreover, as a number of genes need to be considered for PKD, NGS represents a powerful approach and allows simultaneous analysis of many genes in a single test at relatively low cost.
Genotype–Phenotype Correlations
Establishing genotype–phenotype correlations for PKHD1 has proved challenging. The high incidence of compound heterozygotes as well as multiple alleles makes correlations difficult to define consistently. In light of these challenges, genotype–phenotype correlations focus on types of mutations instead of the specific site of the mutation (42). Typically patients with at least two truncating mutations are severely affected and display perinatally or neonatal mortality. Patients with at least one missense mutation tend to be less severely affected and are more likely to survive the neonatal period. However, this is not always the case as some missense mutations can be as severe as truncating mutations. No significant clinical differences could be observed between patients with two missense mutations and those patients harboring a truncating mutation in trans; thus, the milder mutation obviously defines the phenotype (19).
Loss of function probably explains the uniformly early demise of patients carrying two severe truncating alleles. A critical amount of the full-length fibrocystin protein seems to be required for normal function that obviously cannot be compensated by alternative isoforms, which might be generated by re-initiation of translation at a downstream ATG codon. In contrast, missense mutations and small in-frame deletions may have more variable effects on protein function. Phenotypic diversity also reflects the variable extent to which different PKHD1 missense mutations might compromise the function and/or abundance of the mutant protein. While some missense mutations might result in a relatively negligible effect on overall protein function leading to a milder disease course, others might result in loss of function in certain variants. Recent evidence suggests that complex transcriptional profiles may further play a role in defining the patient’s phenotype (54, 55).
While related individuals typically share a similar clinical course, about 20% of pedigrees display significant variability regarding peri/neonatal mortality between siblings with one sibling surviving to early childhood or longer (56). In families with at least one neonatal survivor, a higher proportion of 20 out of 48 sibships (42%) was observed (19). Controlling for family size, the risk for perinatal demise of any additional affected children was 37%. This is concerning from the standpoint of genetic counseling as the rate in this cohort is representative of patients in pediatric nephrology units as a whole. It is worth noting that the categorization of ARPKD as “mild,” “moderate,” and “severe” is an oversimplification. Neonatal survival is one of the principle determinates of the severity of ARPKD. Patients surviving past the neonatal period typically have much better prognosis. However, neonatal survival is influenced by a number of factors, including the extent of pulmonary hypoplasia and resultant lung disease, the availability and quality of intensive care facilities as well as awareness of risk of ARPKD by parents. Given the large degree of variability in the clinical course between siblings, it is clear that predicting the degree to which further children might be affected cannot be based on PKHD1 genotype alone. Identification of potential modifying factors will be one of the principle goals of future research. Understanding the degree to which such modifiers play a role in ARPKD will be particularly important regarding hypomorphic missense mutations and may explain the variable clinical course associated with such cases. Conversely given the comparatively consistent clinical course in the case of null alleles modifying factors are likely to be less relevant.
Differential Diagnosis
DZIP1L-Related Polycystic Kidney Disease
Recently, mutations in DZIP1L have been described in patients with a moderate clinical course of ARPKD. DZIP1L encodes a ciliary TZ protein (DZIP1L, DAZ interacting protein 1-like) that in line with the other PKD proteins localizes to centrioles and the distal end of basal bodies. DZIP1L was demonstrated to interact with septin2 (SEPT2), a protein implicated in maintenance of the periciliary diffusion barrier at the ciliary TZ. Consistent with a defect in the diffusion barrier, the ciliary membrane translocation of the ADPKD proteins, polycystin-1 and -2, has been shown to be compromised in DZIP1L mutant cells suggesting a requirement for DZIP1L in regulating TZ integrity.
The DZIP1L gene is quite easy to analyze and spans only about 53 kb of genomic DNA with 16 exons (ATG start codon in exon 2) that encode a protein of 767 amino acids. Mutations in DZIP1L are a considerably rarer cause of ARPKD than PKHD1 mutations, maybe due in part to the larger size of PKHD1 relative to DZIP1L. Furthermore, our limited data suggest that the region encoding the N-terminus of DZIP1L may be more susceptible to mutations, a concept supported by in silico data that predict pathogenicity scores to decay toward the C-terminus.
The clinical course of patients and siblings with DZIP1L mutations described so far were invariably moderate. While the clinical manifestations in all of those patients were first detected prenatally or during early childhood, none showed perinatal demise. So far, the data suggest that the type or location of DZIP1L mutation does not determine the severity of the clinical course. Patients bearing missense mutations on both parental alleles displayed a comparable phenotype to patients with two truncating DZIP1L alleles. Although we have not screened large numbers of embryonically lethal patients, in view of homozygous truncating mutations in two of our patients we hypothesize that it is unlikely that DZIP1L mutations play a major role in this cohort of most severely affected patients.
Early and Severe Autosomal Dominant Polycystic Kidney Disease (ADPKD)
Typically, ADPKD symptoms do not become apparent before adulthood, notably, amongst a small proportion (2–5%) of ADPKD patients symptoms can manifest during childhood or even prenatally. While there is significant phenotypic variability within the same family, families in which one child displays an early manifestation of ADPKD have a high recurrence of early onset in further children suggesting a common familial background modifier that leads to early disease expression (57). Early disease manifestation in ADPKD is the most important differential diagnosis of ARPKD (Figure 3). Parental renal ultrasound is a must and should be performed in every child with cystic kidney disease of unknown origin. However, the family history often is unremarkable mainly because of two reasons: first, some alleles are incompletely penetrant (hypomorphic) and inherited in a recessive manner (58) (Figure 4). Second, a considerable proportion (about 15–20%) of all mutations in ADPKD are thought to arise de novo (Figure 5).
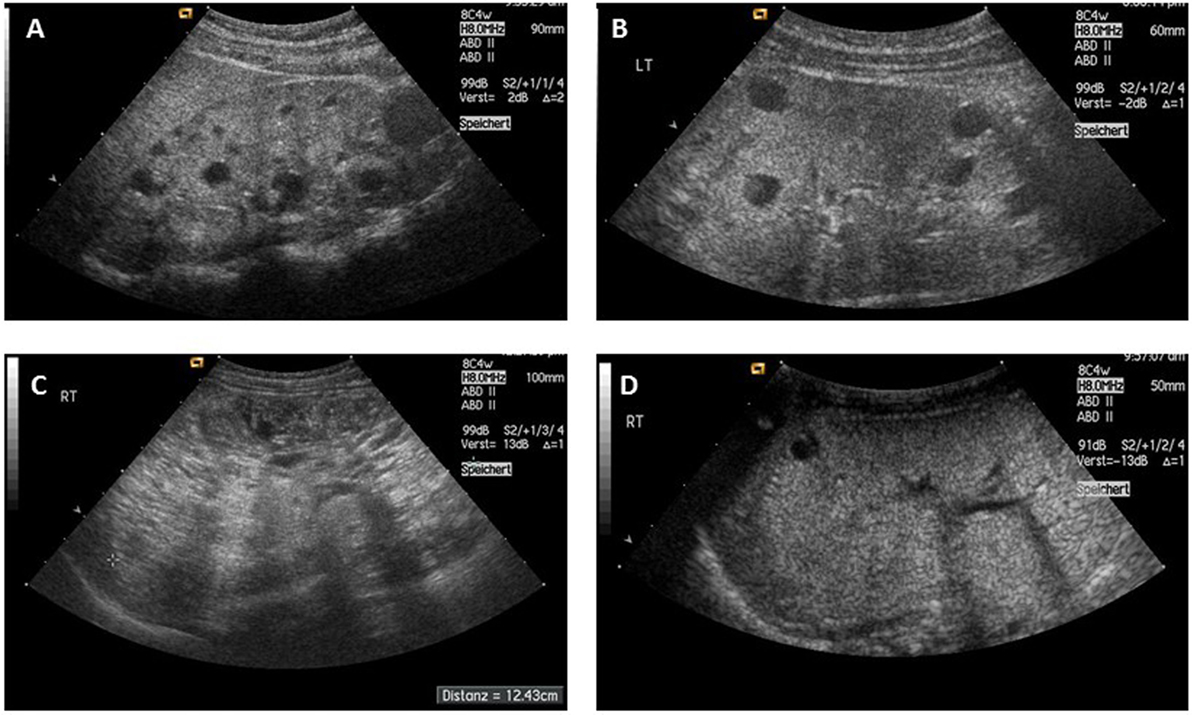
Figure 3. (A,B) Typical sonographic picture of ADPKD in a 10-month-old girl (A) and an 11-year-old boy (B). (C) ADPKD resembling ARPKD in a 3-year-old boy with enlarged echogenic kidneys and small sized cysts. (D) Three-week-old girl with previous oligohydramnion, arterial hypertension, and small cysts in massively enlarged kidneys with a calculated total kidney volume of about 100 ml (normal age-related value <40 ml). Her mother’s phenotype resembled ADPKD, but the family was shown to carry an HNF1β germline mutation [from Bergmann (5)].
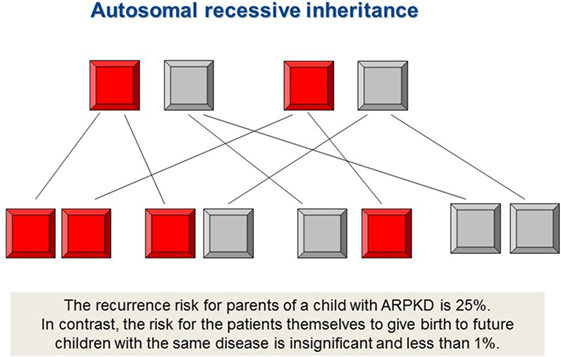
Figure 4. Autosomal recessive inheritance. PKHD1 mutations and most ciliopathies are inherited in a recessive mode, however, importantly also mutations in dominant disease genes such as PKD1 and PKD2 can follow autosomal recessive traits. The family history usually is negative. Both, father and mother, are healthy and typically carry an “unfavorable” recessive disease allele in heterozygous state which is not sufficient to manifest the disease, however. By definition, both disease alleles need to be mutated in affected individuals with autosomal recessive disease. The recurrence risk for parents of a child with ARPKD is 25%. In contrast, the risk of patients to give birth to children with the same disease is insignificant and less than 1% in case the patient’s partner does not originate from the same family (consanguineous marriage), is not equally affected and the disease is not present in the partner’s pedigree.
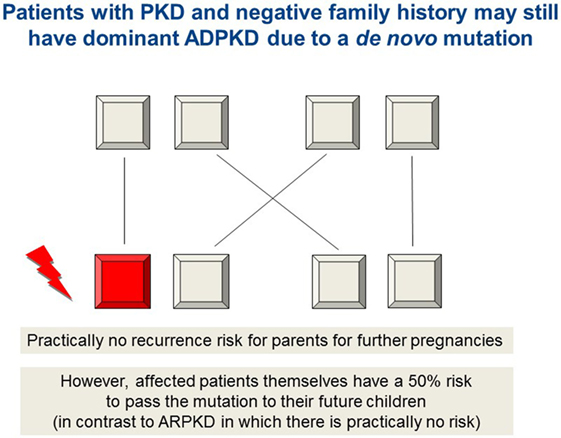
Figure 5. Autosomal dominant inheritance due to a de novo mutation in PKD1, PKD2, or HNF1β, for example, which may mimic ARPKD. As in autosomal recessive inheritance, the family history is unremarkable and both parents are healthy. For the rest of the family as well as for parents and patients, it is of major importance to know if they are afflicted by a recessive or a dominant disease due to a de novo arisen mutation. The latter means that there is practically no recurrence risk for future children neither for the parents nor for other family members. In contrast, the affected patients themselves bear a 50% risk to pass the (dominant) mutation to their own future children (in contrast to ARPKD in which there is practically no risk for the patients to have own children affected by the disease).
The majority of ADPKD patients carries a germline mutation in PKD1 encoding polycystin-1, whereas about 15–20% harbor a mutation in PKD2 leading to alterations of the polycystin-2 protein (38). PKD2 is usually significantly milder than PKD1 with development of ESRD at a later age and a lower prevalence of arterial hypertension and urinary tract infections. Patients with PKD1 develop ESRD earlier than patients with PKD2. The median age of ESRD onset in PKD1 patients is ~20 years earlier than PKD2 (58.1 and 79.9 years, respectively). Additionally, PKD1 patients present with more severe cysts than PKD2; however, this is likely due to development of cysts at an earlier age rather than an increase in cyst growth (59). Analysis of over 500 families with ADPKD revealed that patients with truncating PKD1 mutations develop ESRD an average of 12 years earlier than those with non-truncating mutations in this gene (55.6 vs. 67.9 years) (60).
Recently, other genes such as GANAB have been described to be mutated in a subset of patients with ADPKD (61). ADPKD has a particularly high amount of allelic heterogeneity. A significant proportion of mutations are private and the majority of known PKD1 and PKD2 mutations are predicted to be truncating mutations (70 and 80%, respectively).
Polycystin-1 and polycystin-2 are TM proteins that interact with each other through their C-terminal tails containing coiled-coil motifs (Figure 2). Polycystin-2 is a divalent cation channel involved in cellular Ca2+ signaling (62). Polycystin-1 forms a complex with Polycystin-2 and, when bound to a as yet unidentified ligand, facilitates conformational changes in Polycystin-2 opening the Ca2+ channel. The polycystin complex is also hypothesized to be activated by mechanical strain. There is also data from functional analysis and mutations that suggest fibrocystin may also be part of the polycystin complex (63–66).
Screening for PKD1 mutations is confounded by the six pseudogenes adjacent to the PKD1 locus. Whether or not these pseudogenes function as junk DNA is a matter of debate. Some studies suggest that PKD1 pseudogenes have a functional role in regulating gene expression, perhaps by acting as decoys for micro RNAs (67). In recent years, the increased sophistication of targeted NGS approaches has allowed mutational analysis of even highly confounding loci such as PKD1 (68).
It has become clear that ADPKD is in fact a systemic disorder displaying high co-morbidity in a number of organs including the liver, pancreas, arachnoid membrane, and heart. In ADPKD patients, PLD is common and notably there appears to be evidence for a dosage sensitive network suggesting both genetic and functional overlaps between PKD and PLD (69). A broad range of cardiovascular comorbidities may also arise. Approximately 8% of ADPKD develop intracerebral aneurysms. There is also a high prevalence of cardiac valve disease in ADPKD patients with 25% of patients developing valve defects, though this is usually subclinical.
Early and Severe PKD due to TSC2-PKD1 Microdeletion
ADPKD can be mimicked by tuberous sclerosis (TSC) and von Hippel–Lindau (VHL) syndrome (Figure 6). TSC is caused by an autosomal dominant germline mutation in either TSC1 or TSC2 and can affect a broad range of organs with a birth incidence of approximately 1:6,000 (70). Renal manifestations are the leading cause of death in adult patients (71); cystic kidney disease occurs in 50%, angiomyolipomas are diagnosed in even 80% of patients. Tumors (mainly non-cancerous) may develop, principally in the heart and brain. Patients harboring a deletion encompassing both the PKD1 and TSC2 gene develop an early and severe onset of PKD.
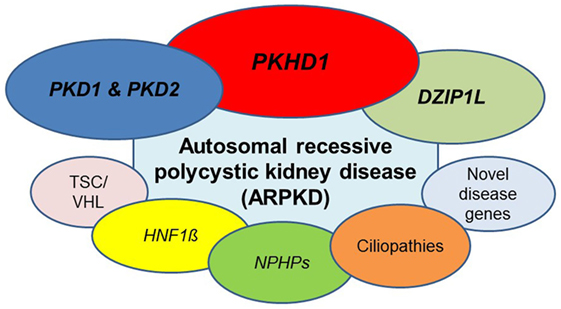
Figure 6. ARPKD can be caused by mutations in different genes. Beyond doubt, the main gene mutated is PKHD1, however, the phenotype is genetically not as homogeneous as often thought. A number of other recessive and dominant genes need to be considered. Most important are dominant and recessive mutations in PKD1 and PKD2, the two genes mainly mutated in patients with adult-onset ADPKD. Other entities that may have to be discussed are HNF1β, novel genes for ARPKD such as DZIP1L and genes that typically cause other cystic kidney diseases and ciliopathies (see main text for details).
Von Hippel–Lindau is an autosomal dominant disorder caused by mutations of the VHL gene. Causative mutations deactivate VHL which is a tumor suppressor gene, this leads to the development of hemangioblastoma in a number of CNS tissues. The CNS tumors are often accompanied by renal tumors in the form of renal clear cell carcinoma (72). VHL patients also have an increased risk of renal and pancreatic cysts.
The phenotypic overlap between TSC, VHL, and PKD patients suggests there is some functional relationship between the causative genes/proteins. From a functional standpoint, the common thread between these three entities appears to be the primary cilium and more specifically the mTOR signaling network (73). Polycystin-1 and the TSC1/TSC2 tumor suppressor complex both act to suppress the activity of mTOR. This leads to G1-cell cycle arrest resulting in apoptosis. Interestingly, it has also been demonstrated that the TSC2 protein tuberin traffics polycystin-1 to the plasma membrane (74), which may be the underlying molecular basis for the apparent genetic interaction.
HNF1β-Related Disease
ARPKD and ADPKD are subject to a large number of phenocopies (Figures 3 and 6). One such example is HNF1β (HNF1β/TCF2 gene). Mutations in HNF1β can result in a broad range of phenotypes, many of which are common in other cystic kidney diseases and ciliopathies and as such plays a critical role in the diagnostics of cystic kidney disease. HNF1β mutations are commonly designated as renal cyst and diabetes (75) syndrome. However, other characteristics include abnormalities of the genital tract, endocrine/exocrine insufficiency, hypomagnesemia, and an increase in liver enzymes.
HNF1β is inherited autosomal dominantly. As is often the case for transcription factors, there is a high degree of variability in penetrance and expressivity as well as a high frequency of spontaneous mutations which need to be considered when evaluating family history. Around 30–50% of HNF1β patients possess de novo mutations. Additionally, approximately 50% of patients have large deletions that remove around 1.4 Mb of genomic DNA, which includes HNF1β and several other genes on the 17q12 chromosome. Surprisingly, many patients carrying the microdeletion do not show a more severe phenotype than patients harboring an HNF1β point mutation. However, there is now convincing evidence that patients with a large genomic rearrangement in 17q12 are much more frequently affected by cognitive impairment, seizures, and other neurodevelopmental disorders (e.g., schizophrenia and autism spectrum disorders) (76, 77).
Overall, HNF1β mutations are thought to constitute the main cause of prenatally diagnosed bilateral hyperechogenic kidneys (78). While many of these fetuses display normal-sized kidneys often with bilateral cortical cysts and normal amniotic fluid volume, HNF1β patients may also show Potter’s sequence with oligo-/anhydramnios and massively enlarged polycystic kidneys (>+3 SD) that mimic ARPKD. In accordance, mice with an Hnf1β mutation showed a PKD-like phenotype with diminished Pkhd1 expression (79). Given the role of HNF1β as a master regulator of a number of cystic kidney disease genes including PKHD1 and PKD2, there is a credible genetic cause which might explain the resemblance between HNF1β and ARPKD/ADPKD patients.
Nephronophthisis
Nephronophthisis is the general term for a heterogeneous collection of autosomal recessive cystic kidney diseases characterized by tubulointerstitial cysts and small or normal size kidneys. The size of the kidneys is usually sufficient to differentiate NPHP from ARPKD; however, in some cases, NPHP diseases can present with ARPKD-like enlarged kidneys or even Potter-like characteristics. NPHP disease genes have been shown to be involved in some common functional networks. Recently, we identified ANKS6 as a core component of the cystoprotein module, which links NEK8 (NPHP9) to INVS (NPHP2) and NPHP3 (80).
Characterization of the NPHP proteins has yielded considerable gains in the fundamental mechanisms involved in cystogenesis and other cilia-associated disorders. NPHP mutations are a significant cause of ESRD in patients under 25. The most common form of NPHP is juvenile NPHP which is characterized initially by defects in urinary concentration as well as anemia, polyuria, and polydipsia (81, 82). The most common mutations are homozygous deletions of NPHP1, which represent about 20–40% of all cases with juvenile NPHP. NPHP patients have normal–small size kidneys and develop cysts at the corticomedullary boundary. However, patients do not normally display cysts until they have already developed advanced chronic kidney disease. Similarly arterial hypertension does not become clinically relevant until late in the disease course. From a histological standpoint there is significant tubulointerstitial fibrosis as well as disintegration and thickening of the basement membrane (83). Similar to many cystic kidney diseases and ciliopathies, NPHP genes are largely pleiotropic and can result in a number of extrarenal manifestations (84).
Medullary cystic kidney disease is caused by mutations in MUC1 and UMOD. Rudimentarily, it can be described as the autosomal dominant form of NPHP. Typically, it has a later onset of ESRD than the recessive forms.
Mutations in Other Ciliary Genes May Mimic ARPKD
In rare instances, especially in the prenatal setting and during early childhood, polycystic kidney disease may be mimicked by mutations in genes that typically cause other, usually more complex ciliopathies such as Bardet–Biedl, Joubert, and Meckel syndrome (MKS).
Bardet–Biedl syndrome (BBS) is usually characterized by obesity, hypogonadism, retinal degeneration, polydactyly, mental retardation, and renal malformations. Various additional features such as hearing loss, diabetes mellitus, and other metabolic defects have also been described. Renal disease is a major cause of morbidity and mortality in BBS and can be heterogeneous in phenotype, but often presents with features of ARPKD or ADPKD with enlarged, hyperechogenic kidneys, and loss of corticomedullary differentiation. Currently, more than 20 BBS genes have been identified and ALMS1, the causative gene for Alstrom syndrome which has significant phenotypic overlap with BBS.
At the more severe end of the ciliopathy spectrum are MKS and Joubert syndrome (JS/JBTS). Like ARPKD, MKS and JBTS both affect multiple systems. However, unlike the classical cystic kidney diseases, MKS and JBTS are characterized by severe, early-onset developmental disorders rather than degenerative disorders. Consistent with many ciliopathies, they are highly heterogeneous and typically present with a host of extrarenal manifestations including hepatobiliary DPM, occipital meningoencephalocele (MKS), and postaxial polydactyly. Prognosis of patients varies considerably. Survival past the neonatal period is quite rare in MKS with a significant portion of patients dying in utero. MKS and JBTS also display very pronounced defects in brain development leading to mental retardation among other neural defects. The “molar tooth sign” is typical for JBTS and an indicator of underlying malformation of the mid- and hindbrain (85). MKS and JBTS patients may also display a range of characteristics common to many ciliopathies such as liver fibrosis, polydactyly and cystic kidneys as well as feeding and respiratory issues.
Genetic Testing
In the past, strategies for genetic testing were mainly based on time- and cost-intensive single-gene testing. The advances in NGS now allow simultaneous analysis of a large group of genes in a single test at relatively low cost. It can be expected that performing single-gene testing will soon be the exception rather than the rule, especially for genetically heterogeneous disorders with a broad phenotypic spectrum such as cystic kidney disease.
At the moment, targeted NGS panel testing might be the most efficient diagnostic approach. Whatever primary strategy is chosen, it is important to be aware of the strengths and pitfalls of each method (68). Close interdisciplinary collaborations between pediatricians and clinical geneticists may prove beneficial. For polycystic kidney disease, the testing approach should be able to detect copy-number variations (CNVs) such as heterozygous deletions (e.g., in HNF1B) and to cover even complex genomic regions such as the PKD1 gene. It needs to be clear that the workflow in NGS, especially with regard to complex genomic regions is much more sophisticated than for conventional approaches and requires specific expertise and infrastructure. While genetic testing evolves rapidly, there are still some limitations to consider. A significant problem many physicians in different parts of the world face is the cost of genetic testing. Although costs are decreasing due to the advances of NGS, they are still comparably high and the reason why genetics is still not used in many healthcare systems on a broader range for children with kidney diseases. Another major challenge that need to be tackled in future years in terms of personalized health efforts is a better and more precise prediction of the individual clinical course from genotype and other in vitro data.
Nevertheless, despite all obstacles, it is widely recommended to offer genetic testing to every family facing early-onset bilateral cystic kidney disease and to discuss the medical and ethical implications in an interdisciplinary manner. For example, genetic testing may:
– lead to earlier diagnosis and avoid a “diagnostic odyssey” and unnecessary diagnostic measures (e.g., renal or liver biopsy in patients with hyperechogenic kidneys).
– establish a definite diagnosis (“relevance to finally give the disease a name”), which represents an underestimated issue many patients and families find psychologically helpful.
– point to renal and extrarenal comorbidities which may otherwise have taken longer to diagnose and which may benefit from early detection and disease monitoring.
– highlight possible future complications which allows focused screening and better prevention.
– enable informed genetic counseling and concise information on recurrence risk for future children or other family members.
In addition, in the future, genetic diagnosis may provide guidance for personalized medical management on a more gene-specific basis (“precision medicine”). The specific genotype of a patient is particularly important when deciding to include patients in clinical trials and when choosing future treatment options. For example, individuals with hypomorphic PKD1/PKD2 alleles are likely to have a slower disease progression and may in fact never develop ESRD. In such cases, the benefit of including the patient in clinical trials for a new treatment may not outweigh the risk.
Though there is significant overlap between ARPKD and ADPKD, there are a number of complications associated with each disease, respectively, which are relevant for proper clinical management. ADPKD patients rarely develop hepatobiliary complications like CHF or DPM. Patients with ARPKD on the other hand will almost certainly develop some complications due to CHF and DPM over time. In a similar vein, ADPKD patients require special considerations for cardiovascular comorbidities, in particular, intracerebral aneurysms (ICAs) (86) which do not typically occur in ARPKD. While general screening for ICAs is usually not recommended, it might be good to know both from the patient’s as well as from the doctor’s side whether there is a positive family history which can lead to an increased risk (87–89). Typically aneurysm rupture is quite rare in children and young adults, so screening is usually not necessary until patients reach the age of 20 years.
Many cystic kidney diseases and ciliopathies display a significant overlap of phenotypes. However, many individual diseases have particular complications that require specific clinical management. Because of the phenotypical similarity of many diseases, genomic screening is now standard practice to delineate underlying causative mutations. One example that demonstrates importance of delineating genetic causation are NPHP mutations. As mentioned previously in the review, NPHP is characterized by a broad range of symptoms and can, in some cases, mimic ARPKD. In addition to the characteristics it shares with ARPKD, NPHP mutations may often lead to retinitis pigmentosa, which is not a feature of ARPKD. Unfortunately, there is no cure for RP currently and ultimately visual impairment is inevitable. In such cases, knowing the genetic cause of the disease allows the patient and their family to prepare for such difficulties later in life. For example, pre-emptively learning braille can ease the progressive transition into visual impairment and is strongly linked to both academic and employment success. Conversely, if definitive mutations are found in other genes then the patient and their family can be assured that visual impairment is not part of the disease spectrum.
Another such example is Alstrom syndrome, which shares many common features with BBS such as retinal dystrophy and obesity. However, Alstrom syndrome patients typically have normal intelligence and do not develop polydactyly, whereas they tend to have much more severe sensorineural hearing loss as well as development of early-onset type 2 diabetes mellitus. Additionally, Alstrom syndrome patients often develop a number of cardiac, pulmonary and hepatic complications that require ongoing clinical management.
Genetic Counseling
It is only possible to discuss in detail clinical course, disease spectrum, and recurrence risk if the nature of the patient’s genotype is known. Understandably, it is extremely important for parents who already have an affected child to know if they carry a 50% (as for autosomal dominantly inherited disorders), 25% (as for recessive diseases), or practically no recurrence risk (Figures 4 and 5). The latter holds true when the mutation in the patient arose de novo. A 100% guarantee cannot be given because of theoretically possible germline mosaicism in one of the parents; thus, the term “practically no recurrence risk” is usually used. In HNF1β, 30–50% of mutations arise de novo, while this number is thought to be 15–20% in ADPKD. In cases with a de novo mutation, patients carry a 50% recurrence risk for their own offspring, whereas as said it is practically zero for the parents and the rest of the family which means a huge relief for them (Figure 5). Genetic diagnosis is also pertinent to family members in the case of X-linked disorders. Genetic counseling is common for female siblings of an affected patient who may wish to know if they are a carrier or not and what the potential risk for their own children is.
With recent improvements to clinical management of PKD, many affected children who survive the neonatal period are likely to reach adulthood and may opt to have children themselves. In such cases, it is imperative for proper genetic counseling to know whether the patients’ causative mutations are dominant or recessive. Patients with dominant mutations will have a 50% recurrence risk, whereas those with recessive mutations will practically have no risk for affected children (risk <1%) except in rather unlikely cases where their partner has the same mutation in their pedigree, either through a carrier or an affected family member. For male patients, it may be worthwhile to determine if mutations are autosomal or X-linked, as there is no risk of father-son recurrence.
Prenatal Diagnosis
Due to the early and severe manifestations of ARPKD and the high recurrence risk (25%), it is common for parents of children with ARPKD to undergo early screening by ultrasound prenatally or early postnatally. This allows for early diagnosis as well as guiding future family planning. However, examination by ultrasound may not be sufficient to detect a number of features of ARPKD such as kidney enlargement and increased echogenicity. As such, definitive prenatal diagnosis is often only reliably attainable through genetic screening. Typically prenatal diagnosis is only offered to families with a known risk of ARPKD as the procedure requires chorionic villus sampling or amniocentesis which are invasive. Another option, especially for couples with particularly high recurrence risk, is preimplantation genetic diagnosis (PGD). This process requires a significant amount of time investment as well as coordination and planning. In the future, non-invasive prenatal testing will likely become more prominent as the technology develops.
Needless to say, in the case of prenatal testing, medical and ethical implications require careful review by all attending physicians and the parents. These tests should be embedded in an ethical framework based on the principles of beneficence and respect for autonomy. Beneficence-based clinical judgment should be evidence-based, i.e., on the basis of the best available evidence, clinical strategies should be identified that are reliably expected to result in the greatest balance of benefits (i.e., the protection and promotion of health-related interests) over clinical harms (i.e., impairments of those interests) (8). Respect for autonomy and the patient’s perspective is another key issue in the context of perinatal genetics. The physician is obliged to respect parents’ values and beliefs, to respect their perspective on their interests, and to implement only those clinical strategies authorized as the result of the informed consent process (8).
What have We Learned During Recent Years and What is on the Horizon?
Considerable progress has been made in the understanding of polycystic kidney diseases, including ARPKD. However, there are still more questions than answers and we wonder about phenomena such as significantly variable disease expression even within the same family. Stochastic, epigenetic, and environmental factors can be expected to modify the phenotype. While standard Mendelian genetics will explain the expressivity of disease in many patients, a significant portion will have more complex genotypes often leading to more severe expression of disease (2). Factors such as second-site modifying alleles may have an epistatic effect and contribute to increased severity. Additionally, hypomorphic accessory mutations may lead to the reduction in the overall dose of function proteins and disrupt so called “dosage sensitive network” leading to a more severe clinical outcome.
Given the considerable heterogeneity, prevalence of phenocopies and overall similarity between many cystic kidney diseases and ciliopathies the possible causative gene (or genes) may number in hundreds (Figure 6). In order to analyze all the potential disease genes in a given case, clinicians now routinely utilize NGS techniques. In addition, NGS also allows for the identification of features such as CNVs, unlike Sanger sequencing. Identification of deletions/duplications is vital as they represent about 5% of the mutational spectrum for some genes. In some case such as HNF1β deletions represent large proportion (around 50%) of all causative mutations.
Overall, the high-throughput targeted analysis of patients’ genomes allows for accurate genetic diagnosis of disease and helps to avoid misdiagnosis. Additionally, increased usage of NGS allows clinicians and researchers to better interpretation identified variants. For the time being, targeted NGS panel approaches are considered as the method of choice for cystic and polycystic kidney diseases considerably increasing the detection rates. However, genetic technologies are evolving rapidly and whole-genome sequencing (WGS) offers advantages over other methodologies and will likely be used in greater volumes for diagnostic purposes. In future years, WGS may eventually even serve as a universal first line test for any disorder with suspected genetic origin. However, currently a one-size-fits-all approach is definitely not the best approach. Next-generation sequencing (NGS) has revolutionized genetic diagnostics and already became a commodity that helps to provide rapidly growing insight into the discussed issues. Interpretation of data becomes the challenge and bench and bedside benefit from digitized medicine and smart bioinformatics algorithms embedded in a multidisciplinary setting.
Author Contributions
The author confirms being the sole contributor of this work and approved it for publication.
Conflict of Interest Statement
The author declares that the research was conducted in the absence of any commercial or financial relationships that could be construed as a potential conflict of interest.
Acknowledgments
I would like to cordially thank John Devane for editorial assistance. The author is an employee of Bioscientia/Sonic Healthcare and holds a part-time faculty appointment at the University of Freiburg. His research lab receives support from the Deutsche Forschungsgemeinschaft (DFG) Collaborative Research Centre (SFB) KIDGEM 1140 and the Federal Ministry of Education and Research (BMBF, 01GM1515C).
References
1. Fliegauf M, Benzing T, Omran H. When cilia go bad: cilia defects and ciliopathies. Nat Rev Mol Cell Biol (2007) 8:880–93. doi:10.1038/nrm2278
2. Gerdes JM, Davis EE, Katsanis N. The vertebrate primary cilium in development, homeostasis, and disease. Cell (2009) 137:32–45. doi:10.1016/j.cell.2009.03.023
3. Nigg EA, Raff JW. Centrioles, centrosomes, and cilia in health and disease. Cell (2009) 139:134. doi:10.1016/j.cell.2009.10.036
4. Hildebrandt F, Benzing T, Katsanis N. Ciliopathies. N Engl J Med (2011) 364:1533–43. doi:10.1056/NEJMra1010172
5. Bergmann C. ARPKD and early manifestations of ADPKD: the original polycystic kidney disease and phenocopies. Pediatr Nephrol (2015) 30(1):15–30. doi:10.1007/s00467-013-2706-2
6. Sweeney WE Jr, Avner ED. Diagnosis and management of childhood polycystic kidney disease. Pediatr Nephrol (2011) 26:675–92. doi:10.1007/s00467-010-1656-1
7. Drenth JP, Chrispijn M, Bergmann C. Congenital fibrocystic liver diseases. Best Pract Res Clin Gastroenterol (2010) 24:573–84. doi:10.1016/j.bpg.2010.08.007
8. Bergmann C, Weiskirchen R. It’s not all in the cilium, but on the road to it: genetic interaction network in polycystic kidney and liver diseases and how trafficking and quality control matter. J Hepatol (2012) 56:1201–3. doi:10.1016/j.jhep.2011.10.014
9. Jensen VL, Li C, Bowie RV, Clarke L, Mohan S, Blacque OE, et al. Formation of the transition zone by Mks5/Rpgrip1L establishes a ciliary zone of exclusion (CIZE) that compartmentalises ciliary signalling proteins and controls PIP2 ciliary abundance. EMBO J (2015) 34:2537–56. doi:10.15252/embj.201488044
10. Reiter JF, Blacque OE, Leroux MR. The base of the cilium: roles for transition fibres and the transition zone in ciliary formation, maintenance and compartmentalization. EMBO Rep (2012) 13:608–18. doi:10.1038/embor.2012.73
11. Guay-Woodford LM. Autosomal recessive polycystic kidney disease: the prototype of the hepato-renal fibrocystic diseases. J Pediatr Genet (2014) 3:89–101. doi:10.3233/PGE-14092
12. Wang S, Luo Y, Wilson PD, Witman GB, Zhou J. The autosomal recessive polycystic kidney disease protein is localized to primary cilia, with concentration in the basal body area. J Am Soc Nephrol (2004) 15:592–602. doi:10.1097/01.ASN.0000113793.12558.1D
13. Liebau MC, Bergmann C. In: Geary DF, Schaefer F, editors. Polycystic Kidney Disease: ADPKD and ARPKD. Pediatric Kidney Disease. Berlin, Heidelberg: Springer (2016).
14. Bergmann C, Küpper F, Dornia C, Schneider F, Senderek J, Zerres K. Algorithm for efficient PKHD1 mutation screening in autosomal recessive polycystic kidney disease (ARPKD). Hum Mutat (2005) 25:225–31. doi:10.1002/humu.20145
15. Guay-Woodford LM, Desmond RA. Autosomal recessive polycystic kidney disease: the clinical experience in North America. Pediatrics (2003) 111:1072–80. doi:10.1542/peds.111.5.1072
16. Gunay-Aygun M, Avner ED, Bacallao RL, Choyke PL, Flynn JT, Germino GG, et al. Autosomal recessive polycystic kidney disease and congenital hepatic fibrosis: summary statement of a first National Institutes of Health/Office of Rare Diseases Conference. J Pediatr (2006) 149:159–64. doi:10.1016/j.jpeds.2006.03.014
17. Kääriäinen H. Polycystic kidney disease in children: a genetic and epidemiological study of 82 Finnish patients. J Med Genet (1987) 24:474–81. doi:10.1136/jmg.24.8.474
18. Adeva M, El-Youssef M, Rossetti S, Kamath PS, Kubly V, Consugar MB, et al. Clinical and molecular characterization defines a broadened spectrum of autosomal recessive polycystic kidney disease (ARPKD). Medicine (Baltimore) (2006) 85(1):1–21. doi:10.1097/01.md.0000200165.90373.9a
19. Bergmann C, Senderek J, Windelen E. Clinical consequences of PKHD1 mutations in 164 patients with autosomal recessive polycystic kidney disease (ARPKD). Kidney Int (2005) 67:829–48. doi:10.1111/j.1523-1755.2005.00148.x
20. Avni FE, Guissard G, Hall M, Janssen F, DeMaertelaer V, Rypens F. Hereditary polycystic kidney diseases in children: changing sonographic patterns through childhood. Pediatr Radiol (2002) 32:169–74. doi:10.1007/s00247-001-0624-0
21. Turkbey B, Ocak I, Daryanani K. Autosomal recessive polycystic kidney disease and congenital hepatic fibrosis (ARPKD/CHF). Pediatr Radiol (2009) 39:100–11. doi:10.1007/s00247-008-1064-x
22. Desmet VJ. Ludwig symposium on biliary disorders-part I. Pathogenesis of ductal plate abnormalities. Mayo Clin Proc (1998) 73:80–9. doi:10.4065/73.1.80
23. Telega G, Cronin D, Avner ED. New approaches to the autosomal recessive polycystic kidney disease patient with dual kidney-liver complications. Pediatr Transplant (2013) 17:328–35. doi:10.1111/petr.12076
24. Rossetti S, Torra R, Coto E, Consugar M, Kubly V, Malaga S, et al. A complete mutation screen of PKHD1 in autosomal recessive polycystic kidney pedigrees. Kidney Int (2003) 64:391–403. doi:10.1046/j.1523-1755.2003.00111.x
25. Gallagher AR, Esquivel EL, Briere TS, Tian X, Mitobe M, Menezes LF, et al. Biliary and pancreatic dysgenesis in mice harboring a mutation in Pkhd1. Am J Pathol (2008) 172:417–29. doi:10.2353/ajpath.2008.070381
26. Garcia-Gonzalez MA, Menezes LF, Piontek KB, Kaimori J, Huso DL, Watnick T, et al. Genetic interaction studies link autosomal dominant and recessive polycystic kidney disease in a common pathway. Hum Mol Genet (2007) 16:1940–50. doi:10.1093/hmg/ddm141
27. Moser M, Matthiesen S, Kirfel J. A mouse model for cystic biliary dysgenesis in autosomal recessive polycystic kidney disease (ARPKD). Hepatology (2005) 41:1113–21. doi:10.1002/hep.20655
28. Woollard JR, Punyashtiti R, Richardson S, Masyuk TV, Whelan S, Huang BQ, et al. A mouse model of autosomal recessive polycystic kidney disease with biliary duct and proximal tubule dilatation. Kidney Int (2007) 72:328–36. doi:10.1038/sj.ki.5002294
29. Ward CJ, Hogan MC, Rossetti S, Walker D, Sneddon T, Wang X, et al. The gene mutated in autosomal recessive polycystic kidney disease encodes a large, receptor-like protein. Nat Genet (2002) 30:259–69. doi:10.1038/ng833
30. Onuchic LF, Furu L, Nagasawa Y, Hou X, Eggermann T, Ren Z, et al. PKHD1, the polycystic kidney and hepatic disease 1 gene, encodes a novel large protein containing multiple immunoglobulin-like plexin-transcription-factor domains and parallel beta-helix 1 repeats. Am J Hum Genet (2002) 70:1305–17. doi:10.1086/340448
31. Nagasawa Y, Matthiesen S, Onuchic LF, Hou X, Bergmann C, Esquivel E, et al. Identification and characterization of Pkhd1, the mouse orthologue of the human ARPKD gene. J Am Soc Nephrol (2002) 13:2246–58. doi:10.1097/01.ASN.0000030392.19694.9D
32. Follit JA, Li L, Vucica Y, Pazour GJ. The cytoplasmic tail of fibrocystin contains a ciliary targeting sequence. J Cell Biol (2010) 188:21–8. doi:10.1083/jcb.200910096
33. Bergmann C. Zystische Nierenerkrankungen und Ziliopathien. Pädiatrie up2date (2014) 9:151–80. doi:10.1055/s-0034-1365722
34. Masyuk TV, Huang BQ, Ward CJ, Masyuk AI, Yuan D, Splinter PL, et al. Defects in cholangiocyte fibrocystin expression and ciliary structure in the PCK rat. Gastroenterology (2003) 125:1303–10. doi:10.1016/j.gastro.2003.09.001
35. Menezes LF, Cai Y, Nagasawa Y, Silva AM, Watkins ML, Da Silva AM, et al. Polyductin, the PKHD1 gene product, comprises isoforms expressed in plasma membrane, primary cilium, and cytoplasm. Kidney Int (2004) 66:1345–55. doi:10.1111/j.1523-1755.2004.00844.x
36. Ward CJ, Yuan D, Masyuk TV, Wang X, Punyashthiti R, Whelan S, et al. Cellular and subcellular localization of the ARPKD protein; fibrocystin is expressed on primary cilia. Hum Mol Genet (2003) 12:2703–10. doi:10.1093/hmg/ddg274
37. Zhang MZ, Mai W, Li C, Cho SY, Hao C, Moeckel G, et al. PKHD1 protein encoded by the gene for autosomal recessive polycystic kidney disease associates with basal bodies and primary cilia in renal epithelial cells. Proc Natl Acad Sci U S A (2004) 101:2311–6. doi:10.1073/pnas.0400073101
38. Harris PC, Torres VE. Polycystic kidney disease. Annu Rev Med (2009) 60:321–37. doi:10.1146/annurev.med.60.101707.125712
39. Hiesberger T, Gourley E, Erickson A, Koulen P, Ward CJ, Masyuk TV, et al. Proteolytic cleavage and nuclear translocation of fibrocystin is regulated by intracellular Ca2+ and activation of protein kinase C. J Biol Chem (2006) 281:34357–64. doi:10.1074/jbc.M606740200
40. Kaimori JY, Nagasawa Y, Menezes LF, Garcia-Gonzalez MA, Deng J, Imai E, et al. Polyductin undergoes notch-like processing and regulated release from primary cilia. Hum Mol Genet (2007) 16:942–56. doi:10.1093/hmg/ddm039
41. Rossetti S, Chauveau D, Walker D, Saggar-Malik A, Winearls CG, Torres VE, et al. A complete mutation screen of the ADPKD genes by DHPLC. Kidney Int (2002) 61:1588–99. doi:10.1046/j.1523-1755.2002.00326.x
42. Bergmann C, Senderek J, Sedlacek B, Pegiazoglou I, Puglia P, Eggermann T, et al. Spectrum of mutations in the gene for autosomal recessive polycystic kidney disease (ARPKD/PKHD1). J Am Soc Nephrol (2003) 14:76–89. doi:10.1097/01.ASN.0000039578.55705.6E
43. Bergmann C, Senderek J, Küpper F, Schneider F, Dornia C, Windelen E, et al. PKHD1 mutations in autosomal recessive polycystic kidney disease (ARPKD). Hum Mutat (2004) 23:453–63.
44. Bergmann C, Senderek J, Schneider F, Dornia C, Küpper F, Eggermann T, et al. PKHD1 mutations in families requesting prenatal diagnosis for autosomal recessive polycystic kidney disease (ARPKD). Hum Mutat (2004) 23:487–95. doi:10.1002/humu.20019
45. Furu L, Onuchic LF, Gharavi A, Hou X, Esquivel EL, Nagasawa Y, et al. Milder presentation of recessive polycystic kidney disease requires presence of amino acid substitution mutations. J Am Soc Nephrol (2003) 14:2004–14. doi:10.1097/01.ASN.0000078805.87038.05
46. Losekoot M, Haarloo C, Ruivenkamp C, White SJ, Breuning MH, Peters DJ. Analysis of missense variants in the PKHD1-gene in patients with autosomal recessive polycystic kidney disease (ARPKD). Hum Genet (2005) 118:185–206. doi:10.1007/s00439-005-0027-7
47. Gunay-Aygun M, Tuchman M, Font-Montgomery E, Lukose L, Edwards H, Garcia A, et al. PKHD1 sequence variations in 78 children and adults with autosomal recessive polycystic kidney disease and congenital hepatic fibrosis. Mol Genet Metab (2010) 99:160–73. doi:10.1016/j.ymgme.2009.10.010
48. Gunay-Aygun M, Font-Montgomery E, Lukose L, Tuchmann M, Graf J, Bryant JC, et al. Correlation of kidney function, volume and imaging findings, and PKHD1 mutations in 73 patients with autosomal recessive polycystic kidney disease. Clin J Am Soc Nephrol (2010) 5:972–84. doi:10.2215/CJN.07141009
49. Baralle D, Baralle M. Splicing in action: assessing disease causing sequence changes. J Med Genet (2005) 42:737–48. doi:10.1136/jmg.2004.029538
50. Bergmann C, Frank V, Küpper F, Schmidt C, Senderek J, Zerres K. Functional analysis of PKHD1 splicing in autosomal recessive polycystic kidney disease. J Hum Genet (2006) 51:788–93. doi:10.1007/s10038-006-0022-4
51. Bergmann C, Küpper F, Schmitt CP, Vester U, Neuhaus TJ, Senderek J, et al. Multi-exon deletions of the PKHD1 gene cause autosomal recessive polycystic kidney disease (ARPKD). J Med Genet (2005) 42:e63. doi:10.1136/jmg.2005.032318
52. Bergmann C. Autosomal Recessive Polycystic Kidney Disease. Oxford: Oxford University Press (2014).
53. Krall P, Pineda C, Ruiz P, Ejarque L, Vendrell T, Camacho JA, et al. Cost-effective PKHD1 genetic testing for autosomal recessive polycystic kidney disease. Pediatr Nephrol (2013) 29(2):223–34. doi:10.1007/s00467-013-2657-7
54. Boddu R, Yang C, O’Connor AK, Hendrickson RC, Boone B, Cui X, et al. Intragenic motifs regulate the transcriptional complexity of Pkhd1/PKHD1. J Mol Med (2014) 92:1045–56. doi:10.1007/s00109-014-1185-7
55. Frank V, Zerres K, Bergmann C. Transcriptional complexity in autosomal recessive polycystic kidney disease. Clin J Am Soc Nephrol (2014) 9:1729–36. doi:10.2215/CJN.00920114
56. Deget F, Rudnik-Schoneborn S, Zerres K. Course of autosomal recessive polycystic kidney disease (ARPKD) in siblings: a clinical comparison of 20 sibships. Clin Genet (1995) 47:248–53. doi:10.1111/j.1399-0004.1995.tb04305.x
57. Torres VE, Harris PC, Pirson Y. Autosomal dominant polycystic kidney disease. Lancet (2007) 369:1287–301. doi:10.1016/S0140-6736(07)60601-1
58. Lu H, Galeano MCR, Ott E, Kaeslin G, Kausalya PJ, Kramer C, et al. Mutations in DZIP1L, which encodes a ciliary-transition zone protein, cause autosomal recessive polycystic kidney disease. Nat Genet (2017) 49:1025–34. doi:10.1038/ng.3871
59. Harris PC, Bae KT, Rossetti S, Torries VE, Grantham JJ, Chapman AB. Cyst number but not the rate of cystic growth is associated with the mutated gene in autosomal dominant polycystic kidney disease. J Am Soc Nephrol (2006) 17:3013–9. doi:10.1681/ASN.2006080835
60. Cornec-Le Gall E, Audrézet MP, Chen JM, Hourmant M, Morin MP, Perrichot R, et al. Type of PKD1 mutation influences renal outcome in ADPKD. J Am Soc Nephrol (2013) 24:1006–13. doi:10.1681/ASN.2012070650
61. Porath B, Gainullin VG, Cornec-Le Gall E, Dillinger EK, Heyer CM, Hopp K, et al. Mutations in GANAB, encoding the glucosidase iialpha subunit, cause autosomal-dominant polycystic kidney and liver disease. Am J Hum Genet (2016) 98:1193–207. doi:10.1016/j.ajhg.2016.05.004
62. Torres VE, Harris PC. Autosomal dominant polycystic kidney disease: the last 3 years. Kidney Int (2009) 76:149–68. doi:10.1038/ki.2009.128
63. Bergmann C, von Bothmer J, Ortiz Brüchle N, Venghaus A, Frank V, Fehrenbach H, et al. Mutations in multiple PKD genes may explain early and severe polycystic kidney disease. J Am Soc Nephrol (2011) 22:2047–56. doi:10.1681/ASN.2010101080
64. Kim I, Fu Y, Hui K, Moeckel G, Mai W, Li C, et al. Fibrocystin/polyductin modulates renal tubular formation by regulating polycystin-2 expression and function. J Am Soc Nephrol (2008) 19:455–68. doi:10.1681/ASN.2007070770
65. Wang S, Zhang J, Nauli SM, Li X, Starremans PG, Luo Y, et al. Fibrocystin/polyductin, found in the same protein complex with polycystin-2, regulates calcium responses in kidney epithelia. Mol Cell Biol (2007) 27:3241–52. doi:10.1128/MCB.00072-07
66. Wu Y, Dai XQ, Li Q, Chen CX, Mai W, Hussain Z, et al. Kinesin-2 mediates physical and functional interactions between polycystin-2 and fibrocystin. Hum Mol Genet (2006) 15:3280–92. doi:10.1093/hmg/ddl404
67. Poliseno L, Salmena L, Zhang J, Carver B, Haveman WJ, Pandolfi PP. A coding-independent function of gene and pseudogene mRNAs regulates tumour biology. Nature (2010) 465:1033–8. doi:10.1038/nature09144
68. Eisenberger T, Decker C, Hiersche M, Hamann RC, Decker E, Neuber S, et al. An efficient and comprehensive strategy for genetic diagnostics of polycystic kidney disease. PLoS One (2015) 10:e0116680. doi:10.1371/journal.pone.0116680
69. Fedeles SV, Tian X, Gallagher AR, Mitobe M, Nishio S, Lee SH, et al. A genetic interaction network of five genes for human polycystic kidney and liver diseases defines polycystin-1 as the central determinant of cyst formation. Nat Genet (2011) 43:639–47. doi:10.1038/ng.860
70. Crino PB, Nathanson KL, Henske EP. The tuberous sclerosis complex. N Engl J Med (2006) 355:1345–56. doi:10.1056/NEJMra055323
71. Shepherd CW, Gomez MR, Lie JT, Crowson CS. Causes of death in patients with tuberous sclerosis. Mayo Clin Proc (1991) 66:792–6. doi:10.1016/S0025-6196(12)61196-3
72. Kaelin WG Jr. The von Hippel-Lindau tumour suppressor protein: O2 sensing and cancer. Nat Rev Cancer (2008) 8:865–73. doi:10.1038/nrc2502
73. Huber TB, Walz G, Kuehn EW. mTOR and rapamycin in the kidney: signaling and therapeutic implications beyond immunosuppression. Kidney Int (2010) 79:502–11. doi:10.1038/ki.2010.457
74. Shillingford JM, Murcia NS, Larson CH, Low SH, Hedgepeth R, Brown N, et al. The mTOR pathway is regulated by polycystin-1, and its inhibition reverses renal cystogenesis in polycystic kidney disease. Proc Natl Acad Sci U S A (2006) 103:5466–71. doi:10.1073/pnas.0509694103
75. Verhave JC, Bech AP, Wetzels JF, Nijenhuis T. Hepatocyte nuclear factor 1beta-associated kidney disease: more than renal cysts and diabetes. J Am Soc Nephrol (2016) 27:345–53. doi:10.1681/ASN.2015050544
76. Moreno-De-Luca D, SGENE ConsortiumMulle JG, Simons Simplex Collection Genetics ConsortiumKaminsky EB, Sanders SJ, et al. Deletion 17q12 is a recurrent copy number variant that confers high risk of autism and schizophrenia. Am J Hum Genet (2010) 87:618–30. doi:10.1016/j.ajhg.2010.10.004
77. Nagamani SC, Erez A, Shen J, Li C, Roeder E, Cox S, et al. Clinical spectrum associated with recurrent genomic rearrangements in chromosome 17q12. Eur J Hum Genet (2010) 18:278–84. doi:10.1038/ejhg.2009.174
78. Decramer S, Parant O, Beaufils S, Clauin S, Guillou C, Kessler S, et al. Anomalies of the TCF2 gene are the main cause of fetal bilateral hyperechogenic kidneys. J Am Soc Nephrol (2007) 18:923–33. doi:10.1681/ASN.2006091057
79. Hiesberger T, Bai Y, Shao X, McNally BT, Sinclair AM, Tian X, et al. Mutation of hepatocyte nuclear factor-1beta inhibits Pkhd1 gene expression and produces renal cysts in mice. J Clin Invest (2004) 113:814–25. doi:10.1172/JCI200420083
80. Hoff S, Halbritter J, Epting D, Frank V, Nguyen TM, van Reeuwijk J, et al. ANKS6 is a central component of a nephronophthisis module linking NEK8 to INVS and NPHP3. Nat Genet (2013) 45:951–6. doi:10.1038/ng.2681
81. Hildebrandt F, Zhou W. Nephronophthisis-associated ciliopathies. J Am Soc Nephrol (2007) 18:1855–71. doi:10.1681/ASN.2006121344
82. Salomon R, Saunier S, Niaudet P. Nephronophthisis. Pediatr Nephrol (2009) 24:2333–44. doi:10.1007/s00467-008-0840-z
83. Omran H. Nephronophthis and medullary cystic kidney disease. In: Geary DF, Schaefer F, editors. Comprehensive Pediatric Nephrology. Amsterdam: Elsevier (2008). p. 143–54.
84. Bergmann C, Fliegauf M, Brüchle NO, Frank V, Olbrich H, Kirschner J, et al. Loss of nephrocystin-3 function can cause embryonic lethality, Meckel-Gruber-like syndrome, situs inversus, and renal-hepatic-pancreatic dysplasia. Am J Hum Genet (2008) 82:959–70. doi:10.1016/j.ajhg.2008.02.017
85. Parisi MA. Clinical and molecular features of Joubert syndrome and related disorders. Am J Med Genet C Semin Med Genet (2009) 151C:326–40. doi:10.1002/ajmg.c.30229
86. Xu HW, Yu SQ, Mei CL, Li MH. Screening for intracranial aneurysm in 355 patients with autosomal-dominant polycystic kidney disease. Stroke (2011) 42:204–6. doi:10.1161/STROKEAHA.110.578740
87. Pirson Y, Chauveau D, Torres V. Management of cerebral aneurysms in autosomal dominant polycystic kidney disease. J Am Soc Nephrol (2002) 13:269–76.
88. Huston J III, Torres VE, Sulivan PP, Offord KP, Wiebers DO. Value of magnetic resonance angiography for the detection of intracranial aneurysms in autosomal dominant polycystic kidney disease. J Am Soc Nephrol (1993) 3:1871–7.
Keywords: autosomal recessive polycystic kidney disease (ARPKD), ADPKD, PKHD1, DZIP1L, PKD1/PKD2, ciliopathies, HNF1β/TCF2, nephronophthisis (NPHP)
Citation: Bergmann C (2018) Genetics of Autosomal Recessive Polycystic Kidney Disease and Its Differential Diagnoses. Front. Pediatr. 5:221. doi: 10.3389/fped.2017.00221
Received: 10 January 2017; Accepted: 02 October 2017;
Published: 09 February 2018
Edited by:
Katherine MacRae Dell, Case Western Reserve University, United StatesReviewed by:
Hesham Safouh, Cairo University, EgyptR. Morrison Hurley, University of British Columbia, Canada
Copyright: © 2018 Bergmann. This is an open-access article distributed under the terms of the Creative Commons Attribution License (CC BY). The use, distribution or reproduction in other forums is permitted, provided the original author(s) and the copyright owner are credited and that the original publication in this journal is cited, in accordance with accepted academic practice. No use, distribution or reproduction is permitted which does not comply with these terms.
*Correspondence: Carsten Bergmann, Y2Fyc3Rlbi5iZXJnbWFubiYjeDAwMDQwO2Jpb3NjaWVudGlhLmRl, Y2Fyc3Rlbi5iZXJnbWFubiYjeDAwMDQwO3VuaWtsaW5pay1mcmVpYnVyZy5kZQ==