- 1Department of Biomedical Engineering, School of Engineering, Virginia Commonwealth University, Richmond, VA, USA
- 2Department of Internal Medicine, Division of Pulmonary Disease and Critical Care Medicine, School of Medicine, Virginia Commonwealth University, Richmond, VA, USA
The field of stem cell biology, cell therapy, and regenerative medicine has expanded almost exponentially, in the last decade. Clinical trials are evaluating the potential therapeutic use of stem cells in many adult and pediatric lung diseases with vascular component, such as bronchopulmonary dysplasia (BPD), chronic obstructive pulmonary disease (COPD), idiopathic pulmonary fibrosis (IPF), or pulmonary arterial hypertension (PAH). Extensive research activity is exploring the lung resident and circulating progenitor cells and their contribution to vascular complications of chronic lung diseases, and researchers hope to use resident or circulating stem/progenitor cells to treat chronic lung diseases and their vascular complications. It is becoming more and more clear that progress in mechanobiology will help to understand the various influences of physical forces and extracellular matrix composition on the phenotype and features of the progenitor cells and stem cells. The current review provides an overview of current concepts in the field.
Introduction
Chronic lung diseases are among the most vicious killers in children and adults. With a growing body of evidence based on ongoing research efforts, we are beginning more and more to understand the true importance of stem and progenitor cells: that these cells are suitable for use in cell therapies and in regenerative medicine, but even more that lung resident and even circulating or bone marrow (BM)-derived stem progenitor cells may be important culprits in many disease processes itself (1, 2).
There are a limited number of stem and progenitor cell populations that are currently of interest in vascular remodeling and cell therapy in the lung. These cells and their putative net effect on pulmonary vascular remodeling (promoting vs. inhibitory) are given in Figure 1. Here, we provide a short introduction on the most important features of these cells that are pertinent to lung diseases with vascular complications. References to more detailed articles on these cells are provided in the bibliography of this article.
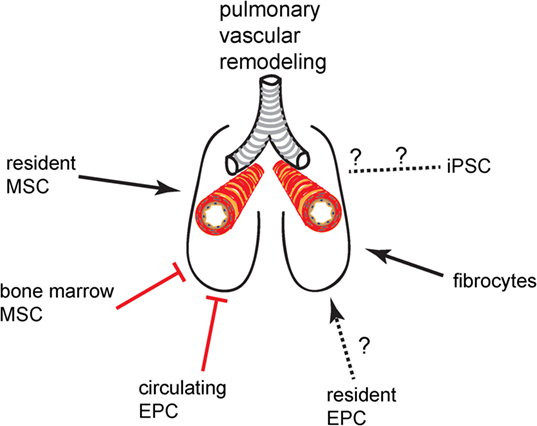
Figure 1. Promoting or inhibitory effect of progenitor cell and stem cell populations on pulmonary vascular remodeling.
Mesenchymal Stem Cells
Mesenchymal stem cells (MSCs) are among the best investigated stem cell populations (3). BM-derived MSCs are the most frequently studied MSCs. The international society for cell therapies has set minimum requirements for the definition of MSC: positive for surface markers CD73, CD90, and CD105 and negative for CD14, CD34, and CD45 (4). In addition, MSCs also need to be able to generate adipocytes, osteoblasts, and chondroblasts in lineage differentiation assays, in vitro (4). This criterion shows the multipotency of these cells, meaning that MSCs are able to give rise to several cell types within the mesenchymal lineage. MSCs have a typical fibroblast-like morphology when cultured in vitro and grow in colony-forming units-fibroblast-like (CFU-F) when seeded in limiting dilution (5). Because of their lack of human leukocyte antigen (HLA) expression, the immune system is largely blind toward MSC, leading to what has been called the “immune-privileged status” of MSC (6). This immune-privileged status has made the MSC an ideal candidate cell for transplantation approaches, as HLA incompatibility is not an issue with MSC. BM-MSCs have shown great promise for cell therapy in various animal models of lung disease and undergone testing for safety and efficacy in several patient groups of lung diseases as will be outlined in the following paragraphs.
In addition to BM-MSCs, lung resident MSCs or mesenchymal stromal progenitors have raised attention due to their potential contribution to several disease processes (7–12). These cells are less clearly defined in their phenotype, although authors use many of the criteria that define BM-MSC.
Bronchopulmonary Dysplasia
Preterm infants who are treated for postnatal respiratory distress are at risk to develop bronchopulmonary dysplasia (BPD) as a consequence of mechanical ventilation and oxygen therapy (13). BPD pathology shows elements of inflammation, abnormal alveolarization, fibrosis, and pathological vascular remodeling (14). The histopathological correlates of the vascular remodeling are dysmorphic capillaries in the interior of thickened alveolar septa, as well as periarteriolar thickening, degeneration of elastic laminae, and increased thickness of the vascular smooth muscle layer (15, 16). There is a mixed bag kind of literature found for the role of MSC in BPD, and it is not clear whether MSCs are friend or foe in BPD (17). In one study, the presence of MSCs in tracheal aspirate predicted the development of BPD and was associated with increased mortality (18). In animal models, BM-MSCs successfully improved neonatal lung injury and arrested alveolar growth (19, 20). It is even more interesting that MSCs rely on their secretome for their beneficial effect, which supports the concept that MSCs protect cells not by direct replacement of cells but rather by paracrine effects (21).
Chronic Obstructive Pulmonary Disease
Patients with chronic obstructive pulmonary disease (COPD) present with a diverse variety of possible phenotype ranging from emphysema to chronic obstructive bronchitis, all characterized by irreversible airflow limitation. The lung vasculature is also affected by a chronic inflammatory response in the lung: This notion is supported by the findings that, for example, the pulmonary arteries show adventitial infiltrates with CD8+ T lymphocytes and cigarette smoke promotes accumulation of neutrophil granulocytes in the lung capillaries (22, 23). Pathological findings in the lung vessels of patients with COPD include increased wall thickness, changes in the composition of the extracellular matrix (ECM) causing stiffening of the blood vessel wall, and increased vascularization of the bronchial wall (23–25). Because COPD belongs to the top killers, efforts are underway to evaluate the role of MSC in the natural course of the disease and as a means for regenerative medicine to revert the severe tissue destruction found in the lungs of COPD patients. Some concepts suggest that aging of BM-MSCs could contribute to the development of COPD, e.g., through stem cell depletion (26). Transplantation of BM-MSCs is being considered for therapy of patients with COPD: a recent study has demonstrated the safety of giving BM-MSCs to patients with COPD (27).
Pulmonary Arterial Hypertension
The changes in the pulmonary arteries in pulmonary arterial hypertension (PAH) range from thickening of the smooth muscle layer and distal extension of a smooth muscle layer to non-muscularized precapillary arterioles to complex multicellular concentric and plexiform lesions (28–31). The literature regarding MSC in pulmonary vascular disease are ambivalent: multipotent MSCs are found in vascular lesions of patients with thromboembolic pulmonary hypertension (PH) (12) and pericytes, mesenchymal vascular cells that are a source of MSCs in the lung, contribute to pulmonary artery remodeling in PAH (11). On the other hand, several studies have demonstrated that MSC transplantation reduces PAH in experimental animal models (32–37). There is a potential difference between BM-derived MSCs that are used for cell transplantation and therapy and lung resident MSCs that may be part of the remodeling process in the vasculature. Future investigations are not only important to answer this question but may also yield a better understanding of the interaction between lung resident and circulating BM-derived cell populations in PAH pathobiology.
Idiopathic Pulmonary Fibrosis
The understanding of the disease pathobiology has come a long way in idiopathic pulmonary fibrosis (IPF). In its humble beginnings, IPF was understood as a disease of dysregulated fibroblast proliferation and activity (38, 39). Pathobiological concepts circled around the so-called “fibroblastic foci,” areas of active fibroblast growth and deposition of ECM with typical sub-epithelial localization. With the onset of finer methods and a better understanding for cell plasticity, the concept that IPF likely starts from repetitive microinjuries to the alveolar epithelium, causing epithelial apoptosis and activation of myofibroblasts arose (40). We have shown that endothelial cell (EC) apoptosis also occurs in areas of active fibrosis both in IPF and in experimental PF (41, 42). Other studies have also found heterogeneous remodeling of the lung vasculature in IPF, with reduced vascular density and aberrant capillaries in regions of active fibrosis and elevated vascular density in border areas adjacent to fibrotic areas (43–45). Based on data showing that PH in IPF reduces survival, it is likely that lung vascular remodeling is more than just a bystander in this devastating disease (41). Various sources of the myofibroblasts, which are the cornerstones of fibrogenesis, have been discussed over time, including resident fibroblasts, epithelial cells via epithelial-to-mesenchymal transition (EMT), circulating mesenchymal progenitors (“fibrocytes”), endothelial cells via endothelial-to-mesenchymal (EndMT) transition, and pericytes (9, 10, 46–56). Recent work has demonstrated that resident mesenchymal cells, particularly pericytes, give rise to the vast majority of myofibroblasts (9, 10, 56). This is quite interesting because at least a fraction of the pericytes likely originates from multipotent mesenchymal progenitor/stem cells (57, 58). Hence, these results suggest that lung-resident MSCs contribute to the disease process. It is interesting that current therapeutic trials evaluate BM-derived MSCs as potential therapeutic target and that the results so far are promising. These studies show, so far, that it is safe to treat IPF patients with BM-MSCs, an outcome that may be counterintuitive at first (59–61). But the results make sense if MSCs are seen as anti-inflammatory cells that support repair processes mainly via paracrine secretion, as suggested by preclinical studies, yielding exciting results by transferring only exosomes of MSCs (62, 63).
Fibrocytes
Fibrocytes have been discovered as a population of hematopoietic cells with ability to produce collagen and to differentiate to myofibroblasts (64). Although shown to accumulate in multiple organ systems during injury repair and fibrosis, fibrocytes have received particular attention in the field of chronic lung diseases, because of a possible contribution of fibrocytes to disease development and progression: such diseases include ILD of the adult, such as IPF, where high levels of circulating fibrocytes have been demonstrated to indicate a poor prognosis (48). In addition, histological evidence for fibrocytes is present in lung tissue from IPF patients (46). Homing of fibrocytes to fibrotic lung tissue depends upon CXC chemokine ligand 12 (CXCL12), a finding that provides an interesting option to block tissue accumulation of fibrocytes as a potential therapeutic avenue (65). Fibrocytes may also represent a target of therapy in PAH, as evidenced by reduced fibrocyte accumulation as a response to treprostinil therapy in an animal model (66).
Endothelial Progenitor Cells
Different cell entities have been summarized under the term “EPC”: EPC have been recently shown as resident cells in the lung circulation and initially as circulating cells (67–71). There are two main ways to identify circulating EPC: first, EPC can be detected within blood mononuclear cells by flow cytometry with specific surface markers (72). The most frequently used marker combination is CD34+ CD133+ VEGFR2+. The identity of these cells as “true” EPC has been controversial for quite a while (68, 73). Second, EPC can be detected by growth: depending on culture principles, early outgrowth CFU-Hills have been distinguished from late outgrowth endothelial colony-forming cells (ECFC) (71, 74, 75). Today, the consensus is that CFU-Hills are rather mononuclear cells with endothelial markers that exert protective effects on endothelial cells largely in a paracrine fashion (76), whereas ECFC are “true” EPC with the ability to replace damaged EC by engraftment (74). Different results have been obtained in preclinical studies for PAH, largely depending on the type of cell that the investigators used: Whereas endogenous EPC seem to be functionally impaired with higher than control proliferation rate but lacking ability for angiogenesis (72), therapy with genetically modified EPC has been successful for treatment in animal models of PAH (77). A clinical trial to determine whether genetically modified EPC have the same therapeutic benefit on PAH patients failed to provide the expected results (78).
In addition to circulating EPC, there is evidence for several EPC populations in the adult lung: Alphonse et al. have shown that ECFC can be isolated from the lung periphery and that these ECFC are functionally impaired in hyperoxia lung injury (79, 80). There is also evidence for EPC with high ability for self-renewal in the adult murine lung (81). These cells have been named “vascular endothelial stem cells” and represent EPC that are on the very top of the lung EC hierarchy. The finding of these endothelial stem cells in the blood vessels of the lung is consistent with previous reports of systemic blood vessels harboring a complete hierarchy of EPC (82). EPC at various levels of self-renewal and differentiation could indeed provide a basis for extensive vascular regeneration. On the other hand, because of the high ability to grow, some of these EPC or endothelial stem cells may also contribute to generate the apoptosis-resistant EC that are found in the complex vascular lesions of PAH patients (83). Evidence for mono- and polyclonal EC proliferation in complex lesions of pulmonary arteries in PAH indeed suggests a contribution of EPC or endothelial stem cells to the development of plexiform lesions in PAH (84). Much work needs to be done to characterize the EC/EPC/endothelial stem cell hierarchy in the lung and to identify the potential of EPC/EC stem cells for regenerative medicine or disease pathobiology.
Induced Pluripotent Stem Cells
Adult stem cells are mostly understood as multipotent cells, meaning that adult stem cells can give rise to multiple cell types within a given lineage (85). The classical example for a multipotent stem cell is the MSC with the ability to produce cells of multiple skeletal cell types, such as osteoblasts, chrondroblasts, adipocytes, and muscle cells (3, 86). In contrast, during embryonic development, there is an early population of stem cells that have the ability to make cell lineages from all three germinal layers (87). This ability is called pluripotency, and the cells are described as embryonic stem cells. No single marker or transcription factor can define pluripotency, but instead a panel of functional assays in combination with activity of several transcription factors, e.g., Oct-4, Nanog, Klf4, and others, can help to identify pluripotent cells (87–90). In recent years, a variety of strategies have been shown to use a mature cell, such as fibroblasts, and induce pluripotency in such a cell. The strategies range from simultaneous overexpression of several transcription factors to chemical stimulation methods (88–94). So why is it an advantage to generate such iPSC? First, pluripotent cells are very powerful cells that can give rise to almost any cell lineage in the body and, therefore, their value for regenerative medicine may be skyrocketing. But human embryonic stem cells, which are the “natural” pluripotent stem cells, are highly restricted and difficult to obtain. Hence, iPSC would provide a much easier accessible and less regulated source of pluripotent stem cells. Second, iPSC allow generating pluripotent stem cells from tissues such as skin of patients and, therefore, providing a patient-specific and genetically identical source of cells. Such approaches will likely be very useful in the dawning age of “personalized medicine.” Recent work in the vascular field has demonstrated that iPSC can give rise to different vascular lineages, including EC (95, 96). The ability to generate genetically identical EC from, e.g., skin fibroblasts will provide a unique tool for regenerative medicine, including seeding of decellularized lung matrices with genetically engineered but patient-specific lung cells as prerequisite for using these recellularized lungs as transplant organs (97). Despite all the enthusiasm and hopes that flare up with such exciting and groundbreaking progress, these so powerful iPSC also need to be careful evaluated for adverse effects when transplanted to patients and seeded in organ matrices to generate future transplant organs: because iPSC can give rise to so many different lineages and have a high self-renewal potential, iPSC harbor the danger of giving rise to produce genetic and epigenetic changes, immunogenicity, and tumor cells (98). Even the possibility of such adverse effect of iPSC therapy needs to be carefully considered, and the risks have to be carefully weighted against the clear and exciting potential to benefit the patient by using iPSC.
Role of Biomechanics and Stem Cell Mechanobiology in Vascular Remodeling
As science progresses with a better understanding of the role of the various stem or progenitor cell types in contribution to lung disease, repair, or regeneration, the mechanical microenvironment has emerged as a major player. Mechanical signal transduction through focal adhesions, the glycocalyx, mechanically activated ion channels, primary cilia, and force across cell–cell junctions may all play a role in the contribution of stem cells to pulmonary vascular remodeling (99). The dynamic mechanical environment of the lung lends itself to study the effects of mechanobiology in normal physiology and pathology of the lung microvasculature. The mechanical environment is essential to stem cell behavior during lung development and during pathological vascular remodeling events, which occur in BPD, IPF, COPD, and PAH.
During development of the pulmonary microvasculature, the fetal lung undergoes hydrostatic pressure between 1–2 mmHg, which maintains the lung in a distended state (100). Fetal breathing movements cause cyclic mechanical loading to the developing alveoli and microvasculature causing shear stress (101). Following the development of the primitive circulatory system, blood velocity and shear stress is critical for embryonic vascular patterning, remodeling, and proper development (102). Lack of proper mechanotransduction at any stage in the developmental process can lead to structural abnormalities and an underdeveloped lung.
Bronchopulmonary dysplasia is characterized by impaired alveolarization and vascularization and often occurs following mechanical ventilation. The impaired structure of the lung microvasculature leads to abnormal fluid flow and reduced gas exchange. High air flow from mechanical ventilation can increase lung injury, leading to the development of BPD (103).
Idiopathic pulmonary fibrosis is associated with progressive fibrotic scarring and stiffening of the lung ECM (104). This scarring is also associated with loss of compliance. The overall stiffness of the alveolar septa is increased by two- to threefold as measured by atomic force microscopy (105). IPF is also associated with increased vascular remodeling and may be associated with increased PH.
In PAH, pulmonary vascular remodeling is influenced by both hemodynamic and ECM changes. By its very nature, the remodeling in PAH causes increases in the pulmonary vasculature pressure as shown by animal models of severe PAH (106). But increased pulmonary artery pressure, e.g., by vasoconstriction, may also contribute to remodeling of pulmonary arteries and even foster the development of complex lesions in pulmonary arteries (107, 108). Furthermore, the ECM changes that occur in remodeling lend themselves to increased substrate stiffness (109, 110).
Chronic obstructive pulmonary disease also causes changes in lung mechanics and vascular remodeling. A reduction of vascularity in the alveolar septa occurs in emphysema (111). Loss of alveoli causes lung hyperinflation and overdistension of the remaining alveoli and capillaries. Furthermore, pulmonary arterial stiffness and blood pressure are increased in COPD patients and are not reduced with pulmonary rehabilitation (112).
These altered mechanical environments and their effects on stem cell contribution to pulmonary vascular remodeling warrants further study. The general physiological response of stem cells to mechanical signaling has been studied greatly in vitro, with in vivo studies largely lacking. Furthermore, specific studies of the mechanical environment on stem cells residing in the lung vasculature are especially absent. Information may be gleaned by understanding stem and progenitor cell response to general mechanical conditions. We will briefly review the state of the science of stem cell response to each of three mechanical conditions: shear stress, cyclic strain, and substrate stiffness as they may pertain to pulmonary vascular remodeling and stem cell-based therapies. These mechanical loading conditions and their effect on cell signaling and phenotype are summarized in Table 1.
Shear Stress
Whether in vitro or in vivo, cells generate force and are often exposed to force, and both can influence stem cell fates (126). Exposed forces can be axial or shear in nature, as one would find in fluid flow (115). Embryonic stem cells and BM-derived EPC have been shown to differentiate into endothelial cells (113). In fact, under flow conditions, EPC can form functional blood vessels from single c-kit+ cells (81). Human EPC demonstrate increased proliferation and VEGF-receptors, endothelial cadherin, and tyrosine-kinase-1 under laminar flow conditions up to 2.5 dyn/cm2 (115). Furthermore, under laminar flow conditions, artery phenotypes of Ephrin B2, Notch 1/3, Hey 1/2, and activin receptor-like kinase increased in EPCs (114). The altered signaling found in these studies under fluid shear stress emphasize the importance to examine stem cell response under dynamic mechanical conditions, such as those found in the diseased lung.
Cyclic Mechanical Strain
A body of work has been examined wherein microvascular endothelial cells exposed to cyclic mechanical loading have been shown to contribute to damage and inflammation (124, 125). However, there has been little work, to date, examining the role of stem cells in this process. Under cyclic strain, EPC may differentiate into smooth muscle cells, since it has been shown that platelet-derived growth factor-BB can induce EPC differentiation into smooth muscle cells (127). BM-derived progenitor cells exposed to cyclic stretch increased α-smooth muscle actin and h1-calponin (118).
Altered Substrate Stiffness
Vascular remodeling alters ECM composition and stiffness. It is well known that naive MSCs can be induced to specific lineages using soluble induction factors in conjunction with tissue-specific substrate stiffness (128). Mechanical stiffness has been shown to increase differentiation of BM-derived mononuclear cells into endothelial progenitor cells (129). Tube morphogenesis from EPC required the combined effects of stiffness and VEGF (116). Differentiation of EPC based on stiffness alone has not been well established. One group looking at ECFC found different cell adhesion attributes based on stiffness but found no phenotypic changes associated with stiffness (117). However, this experiment utilized media that may have prevented differentiation. Transdifferentiation, or lineage deprogramming, can also occur through alterations of the substrate stiffness. In epithelial cells, spontaneous EMT can occur on stiffer substrates (119). In murine breast cancer, stiff substrates can induce EMT while softer substrates induce apoptosis (120). For endothelial cells, TGF-β1 and TGF-β2 play the most important role to induce EndMT, but stiffness can significantly impact EndMT, with softer substrates almost inhibiting the effects of TGF-β (122, 123). Substrate stiffness does play a major role in cellular adhesion, proliferation, morphology, and migration (130). While forces may induce or prohibit differentiation, the effects are much more significant with growth factor support. Further studies are necessary to relate these in vitro studies to the in vivo effects of vascular remodeling in vivo.
In conclusion, stem cell contribution to vascular remodeling in lung pathologies will be dependent on the mechanical environment. These changes will play a role in cellular differentiation and matrix deposition, all contributing to the pathological process. In stem cell therapy approaches, these effects will need to be harnessed to promote positive tissue remodeling and regeneration.
Author Contributions
Conception of work: RH, PL, LF. Drafting or revising the manuscript: RH, PL, LF. Final approval: RH, PL, LF. Agreement to be accountable for all aspects of the work: RH, PL, LF.
Conflict of Interest Statement
The authors declare that the research was conducted in the absence of any commercial or financial relationships that could be construed as a potential conflict of interest.
Funding
The work was funded by the American Heart Association (13SDG16360018).
References
1. Stewart DJ, Mei SH. Cell-based therapies for lung vascular diseases: lessons for the future. Proc Am Thorac Soc (2011) 8(6):535–40. doi: 10.1513/pats.201105-035MW
2. Farkas L, Kolb M. Vascular repair and regeneration as a therapeutic target for pulmonary arterial hypertension. Respiration (2013) 85(5):355–64. doi:10.1159/000350177
3. Keating A. Mesenchymal stromal cells: new directions. Cell Stem Cell (2012) 10(6):709–16. doi:10.1016/j.stem.2012.05.015
4. Dominici M, Le Blanc K, Mueller I, Slaper-Cortenbach I, Marini F, Krause D, et al. Minimal criteria for defining multipotent mesenchymal stromal cells. The International Society for Cellular Therapy position statement. Cytotherapy (2006) 8:315–7. doi:10.1080/14653240600855905
5. Lv FJ, Tuan RS, Cheung KM, Leung VY. Concise review: the surface markers and identity of human mesenchymal stem cells. Stem Cells (2014) 32(6):1408–19. doi:10.1002/stem.1681
6. Le Blanc K, Mougiakakos D. Multipotent mesenchymal stromal cells and the innate immune system. Nat Rev Immunol (2012) 12(5):383–96. doi:10.1038/nri3209
7. Chow K, Fessel JP, Kaoriihida S, Schmidt EP, Gaskill C, Alvarez D, et al. Dysfunctional resident lung mesenchymal stem cells contribute to pulmonary microvascular remodeling. Pulm Circ (2013) 3(1):31–49. doi:10.4103/2045-8932.109912
8. Lama VN, Smith L, Badri L, Flint A, Andrei A-C, Murray S, et al. Evidence for tissue-resident mesenchymal stem cells in human adult lung from studies of transplanted allografts. J Clin Invest (2007) 117(4):989–96. doi:10.1172/jci29713
9. Rock JR, Barkauskas CE, Cronce MJ, Xue Y, Harris JR, Liang J, et al. Multiple stromal populations contribute to pulmonary fibrosis without evidence for epithelial to mesenchymal transition. Proc Natl Acad Sci U S A (2011) 108(52):E1475–83. doi:10.1073/pnas.1117988108
10. Hung C, Linn G, Chow YH, Kobayashi A, Mittelsteadt K, Altemeier WA, et al. Role of lung pericytes and resident fibroblasts in the pathogenesis of pulmonary fibrosis. Am J Respir Crit Care Med (2013) 188(7):820–30. doi:10.1164/rccm.201212-2297OC
11. Ricard N, Tu L, Le Hiress M, Huertas A, Phan C, Thuillet R, et al. Increased pericyte coverage mediated by endothelial-derived fibroblast growth factor-2 and interleukin-6 is a source of smooth muscle-like cells in pulmonary hypertension. Circulation (2014) 129(15):1586–97. doi:10.1161/CIRCULATIONAHA.113.007469
12. Firth AL, Yao W, Ogawa A, Madani MM, Lin GY, Yuan JX-J. Multipotent mesenchymal progenitor cells are present in endarterectomized tissues from patients with chronic thromboembolic pulmonary hypertension. Am J Physiol Cell Physiol (2010) 298(5):C1217–25. doi:10.1152/ajpcell.00416.2009
13. Mourani PM, Abman SH. Pulmonary hypertension and vascular abnormalities in bronchopulmonary dysplasia. Clin Perinatol (2015) 42(4):839–55. doi:10.1016/j.clp.2015.08.010
14. Bhatt AJ, Pryhuber GS, Huyck H, Watkins RH, Metlay LA, Maniscalco WM. Disrupted pulmonary vasculature and decreased vascular endothelial growth factor, Flt-1, and TIE-2 in human infants dying with bronchopulmonary dysplasia. Am J Respir Crit Care Med (2001) 164(10 Pt 1):1971–80. doi:10.1164/ajrccm.164.10.2101140
15. Northway WH Jr, Rosan RC, Porter DY. Pulmonary disease following respirator therapy of hyaline-membrane disease. Bronchopulmonary dysplasia. N Engl J Med (1967) 276(7):357–68. doi:10.1056/nejm196702162760701
16. Bonikos DS, Bensch KG, Northway WH Jr, Edwards DK. Bronchopulmonary dysplasia: the pulmonary pathologic sequel of necrotizing bronchiolitis and pulmonary fibrosis. Hum Pathol (1976) 7(6):643–66. doi:10.1016/S0046-8177(76)80077-9
17. Pierro M, Thebaud B. Mesenchymal stem cells in chronic lung disease: culprit or savior? Am J Physiol Lung Cell Mol Physiol (2010) 298(6):L732–4. doi:10.1152/ajplung.00099.2010
18. Popova AP, Bozyk PD, Bentley JK, Linn MJ, Goldsmith AM, Schumacher RE, et al. Isolation of tracheal aspirate mesenchymal stromal cells predicts bronchopulmonary dysplasia. Pediatrics (2010) 126(5):e1127–33. doi:10.1542/peds.2009-3445
19. Abman SH, Matthay MA. Mesenchymal stem cells for the prevention of bronchopulmonary dysplasia: delivering the secretome. Am J Respir Crit Care Med (2009) 180(11):1039–41. doi:10.1164/rccm.200909-1330ED
20. Aslam M, Baveja R, Liang OD, Fernandez-Gonzalez A, Lee C, Mitsialis SA, et al. Bone marrow stromal cells attenuate lung injury in a murine model of neonatal chronic lung disease. Am J Respir Crit Care Med (2009) 180(11):1122–30. doi:10.1164/rccm.200902-0242OC
21. van Haaften T, Byrne R, Bonnet S, Rochefort GY, Akabutu J, Bouchentouf M, et al. Airway delivery of mesenchymal stem cells prevents arrested alveolar growth in neonatal lung injury in rats. Am J Respir Crit Care Med (2009) 180(11):1131–42. doi:10.1164/rccm.200902-0179OC
22. Peinado VI, BarberÁ JA, Abate P, RamÍRez J, Roca J, Santos S, et al. Inflammatory reaction in pulmonary muscular arteries of patients with mild chronic obstructive pulmonary disease. Am J Respir Crit Care Med (1999) 159(5):1605–11. doi:10.1164/ajrccm.159.5.9807059
23. Harkness LM, Kanabar V, Sharma HS, Westergren-Thorsson G, Larsson-Callerfelt A-K. Pulmonary vascular changes in asthma and COPD. Pulm Pharmacol Ther (2014) 29(2):144–55. doi:10.1016/j.pupt.2014.09.003
24. Kranenburg AR, de Boer WI, Alagappan VKT, Sterk PJ, Sharma HS. Enhanced bronchial expression of vascular endothelial growth factor and receptors (Flk-1 and Flt-1) in patients with chronic obstructive pulmonary disease. Thorax (2005) 60(2):106–13. doi:10.1136/thx.2004.023986
25. Zanini A, Chetta A, Saetta M, Baraldo S, Castagnetti C, Nicolini G, et al. Bronchial vascular remodelling in patients with COPD and its relationship with inhaled steroid treatment. Thorax (2009) 64(12):1019–24. doi:10.1136/thx.2009.114629
26. Mora AL, Rojas M. Adult stem cells for chronic lung diseases. Respirology (2013) 18(7):1041–6. doi:10.1111/resp.12112
27. Weiss DJ, Casaburi R, Flannery R, LeRoux-Williams M, Tashkin DP. A placebo-controlled, randomized trial of mesenchymal stem cells in COPD. Chest (2013) 143(6):1590–8. doi:10.1378/chest.12-2094
28. Rabinovitch M. Molecular pathogenesis of pulmonary arterial hypertension. J Clin Invest (2012) 122(12):4306–13. doi:10.1172/JCI60658
29. Stacher E, Graham BB, Hunt JM, Gandjeva A, Groshong SD, McLaughlin VV, et al. Modern age pathology of pulmonary arterial hypertension. Am J Respir Crit Care Med (2012) 186(3):261–72. doi:10.1164/rccm.201201-0164OC
30. Cool CD, Kennedy D, Voelkel NF, Tuder RM. Pathogenesis and evolution of plexiform lesions in pulmonary hypertension associated with scleroderma and human immunodeficiency virus infection. Hum Pathol (1997) 28(4):434–42. doi:10.1016/S0046-8177(97)90032-0
31. Cool CD, Stewart JS, Werahera P, Miller GJ, Williams RL, Voelkel NF, et al. Three-dimensional reconstruction of pulmonary arteries in plexiform pulmonary hypertension using cell-specific markers. Evidence for a dynamic and heterogeneous process of pulmonary endothelial cell growth. Am J Pathol (1999) 155(2):411–9. doi:10.1016/S0002-9440(10)65137-1
32. Jiang L, Song XH, Liu P, Zeng CL, Huang ZS, Zhu LJ, et al. Platelet-mediated mesenchymal stem cells homing to the lung reduces monocrotaline-induced rat pulmonary hypertension. Cell Transplant (2012) 21(7):1463–75. doi:10.3727/096368912x640529
33. Liang OD, Mitsialis SA, Chang MS, Vergadi E, Lee C, Aslam M, et al. Mesenchymal stromal cells expressing heme oxygenase-1 reverse pulmonary hypertension. Stem Cells (2011) 29(1):99–107. doi:10.1002/stem.548
34. Luan Y, Zhang X, Kong F, Cheng GH, Qi TG, Zhang ZH. Mesenchymal stem cell prevention of vascular remodeling in high flow-induced pulmonary hypertension through a paracrine mechanism. Int Immunopharmacol (2012) 14(4):432–7. doi:10.1016/j.intimp.2012.08.001
35. Umar S, de Visser YP, Steendijk P, Schutte CI, Laghmani el H, Wagenaar GT, et al. Allogenic stem cell therapy improves right ventricular function by improving lung pathology in rats with pulmonary hypertension. Am J Physiol Heart Circ Physiol (2009) 297(5):H1606–16. doi:10.1152/ajpheart.00590.2009
36. Zhang Y, Liao S, Yang M, Liang X, Poon MW, Wong CY, et al. Improved cell survival and paracrine capacity of human embryonic stem cell-derived mesenchymal stem cells promote therapeutic potential for pulmonary arterial hypertension. Cell Transplant (2012) 21(10):2225–39. doi:10.3727/096368912x653020
37. Zhang ZH, Lu Y, Luan Y, Zhao JJ. Effect of bone marrow mesenchymal stem cells on experimental pulmonary arterial hypertension. Exp Ther Med (2012) 4(5):839–43. doi:10.3892/etm.2012.691
38. Calabrese F, Giacometti C, Rea F, Loy M, Valente M. Idiopathic interstitial pneumonias: primum movens: epithelial, endothelial or whatever. Sarcoidosis Vasc Diffuse Lung Dis (2005) 22(Suppl 1):S15–23.
39. Selman M, King TE, Pardo A. Idiopathic pulmonary fibrosis: prevailing and evolving hypotheses about its pathogenesis and implications for therapy. Ann Intern Med (2001) 134(2):136–51. doi:10.7326/0003-4819-134-2-200101160-00015
40. Selman M, Pardo A. Role of epithelial cells in idiopathic pulmonary fibrosis: from innocent targets to serial killers. Proc Am Thorac Soc (2006) 3(4):364–72. doi:10.1513/pats.200601-003TK
41. Farkas L, Gauldie J, Voelkel NF, Kolb M. Pulmonary hypertension and idiopathic pulmonary fibrosis: a tale of angiogenesis, apoptosis, and growth factors. Am J Respir Cell Mol Biol (2011) 45(1):1–15. doi:10.1165/rcmb.2010-0365TR
42. Farkas L, Farkas D, Ask K, Moller A, Gauldie J, Margetts P, et al. VEGF ameliorates pulmonary hypertension through inhibition of endothelial apoptosis in experimental lung fibrosis in rats. J Clin Invest (2009) 119(5):1298–311. doi:10.1172/JCI36136
43. Ebina M, Shimizukawa M, Shibata N, Kimura Y, Suzuki T, Endo M, et al. Heterogeneous increase in CD34-positive alveolar capillaries in idiopathic pulmonary fibrosis. Am J Respir Crit Care Med (2004) 169(11):1203–8. doi:10.1164/rccm.200308-1111OC
44. Renzoni EA, Walsh DA, Salmon M, Wells AU, Sestini P, Nicholson AG, et al. Interstitial vascularity in fibrosing alveolitis. Am J Respir Crit Care Med (2003) 167(3):438–43. doi:10.1164/rccm.200202-135OC
45. Hanumegowda C, Farkas L, Kolb M. Angiogenesis in pulmonary fibrosis: too much or not enough? Chest (2012) 142(1):200–7. doi:10.1378/chest.11-1962
46. Andersson-Sjöland A, de Alba CG, Nihlberg K, Becerril C, Ramírez R, Pardo A, et al. Fibrocytes are a potential source of lung fibroblasts in idiopathic pulmonary fibrosis. Int J Biochem Cell Biol (2008) 40(10):2129–40. doi:10.1016/j.biocel.2008.02.012
47. Mehrad B, Burdick MD, Zisman DA, Keane MP, Belperio JA, Strieter RM. Circulating peripheral blood fibrocytes in human fibrotic interstitial lung disease. Biochem Biophys Res Commun (2007) 353(1):104–8. doi:10.1016/j.bbrc.2006.11.149
48. Moeller A, Gilpin SE, Ask K, Cox G, Cook D, Gauldie J, et al. Circulating fibrocytes are an indicator of poor prognosis in idiopathic pulmonary fibrosis. Am J Respir Crit Care Med (2009) 179(7):588–94. doi:10.1164/rccm.200810-1534OC
49. Heise RL, Stober V, Cheluvaraju C, Hollingsworth JW, Garantziotis S. Mechanical stretch induces epithelial-mesenchymal transition in alveolar epithelia via hyaluronan activation of innate immunity. J Biol Chem (2011) 286(20):17435–44. doi:10.1074/jbc.M110.137273
50. Jain R, Shaul PW, Borok Z, Willis BC. Endothelin-1 induces alveolar EMT through ET-A-mediated production of transforming growth factor-{beta}1. Am J Respir Cell Mol Biol (2007) 37(1):38–47. doi:10.1165/rcmb.2006-0353OC
51. Kasai H, Allen JT, Mason RM, Kamimura T, Zhang Z. TGF-beta1 induces human alveolar epithelial to mesenchymal cell transition (EMT). Respir Res (2005) 6:56. doi:10.1186/1465-9921-6-56
52. Königshoff M, Kramer M, Balsara N, Wilhelm J, Amarie OV, Jahn A, et al. WNT1-inducible signaling protein-1 mediates pulmonary fibrosis in mice and is upregulated in humans with idiopathic pulmonary fibrosis. J Clin Invest (2009) 119(4):772–87. doi:10.1172/JCI33950
53. Willis BC, Liebler JM, Luby-Phelps K, Nicholson AG, Crandall ED, du Bois RM, et al. Induction of epithelial-mesenchymal transition in alveolar epithelial cells by transforming growth factor-beta1: potential role in idiopathic pulmonary fibrosis. Am J Pathol (2005) 166(5):1321–32. doi:10.1016/S0002-9440(10)62351-6
54. Piera-Velazquez S, Li Z, Jimenez SA. Role of endothelial-mesenchymal transition (EndoMT) in the pathogenesis of fibrotic disorders. Am J Pathol (2011) 179(3):1074–80. doi:10.1016/j.ajpath.2011.06.001
55. Hashimoto N, Phan SH, Imaizumi K, Matsuo M, Nakashima H, Kawabe T, et al. Endothelial-mesenchymal transition in bleomycin-induced pulmonary fibrosis. Am J Respir Cell Mol Biol (2010) 43(2):161–72. doi:10.1165/rcmb.2009-0031OC
56. Marriott S, Baskir RS, Gaskill C, Menon S, Carrier EJ, Williams J, et al. ABCG2pos lung mesenchymal stem cells are a novel pericyte subpopulation that contributes to fibrotic remodeling. Am J Physiol Cell Physiol (2014) 307(8):C684–98. doi:10.1152/ajpcell.00114.2014
57. Klein D, Meissner N, Kleff V, Jastrow H, Yamaguchi M, Ergun S, et al. Nestin(+) tissue-resident multipotent stem cells contribute to tumor progression by differentiating into pericytes and smooth muscle cells resulting in blood vessel remodeling. Front Oncol (2014) 4:169. doi:10.3389/fonc.2014.00169
58. Klein D, Weißhardt P, Kleff V, Jastrow H, Jakob HG, Ergün S. Vascular wall-resident CD44+ multipotent stem cells give rise to pericytes and smooth muscle cells and contribute to new vessel maturation. PLoS One (2011) 6(5):e20540. doi:10.1371/journal.pone.0020540
59. Zhao F, Zhang YF, Liu YG, Zhou JJ, Li ZK, Wu CG, et al. Therapeutic effects of bone marrow-derived mesenchymal stem cells engraftment on bleomycin-induced lung injury in rats. Transplant Proc (2008) 40(5):1700–5. doi:10.1016/j.transproceed.2008.01.080
60. Kumamoto M, Nishiwaki T, Matsuo N, Kimura H, Matsushima K. Minimally cultured bone marrow mesenchymal stem cells ameliorate fibrotic lung injury. Eur Respir J (2009) 34(3):740–8. doi:10.1183/09031936.00128508
61. Chambers DC, Enever D, Ilic N, Sparks L, Whitelaw K, Ayres J, et al. A phase 1b study of placenta-derived mesenchymal stromal cells in patients with idiopathic pulmonary fibrosis. Respirology (2014) 19(7):1013–8. doi:10.1111/resp.12343
62. Akram KM, Samad S, Spiteri MA, Forsyth NR. Mesenchymal stem cells promote alveolar epithelial cell wound repair in vitro through distinct migratory and paracrine mechanisms. Respir Res (2013) 14:9. doi:10.1186/1465-9921-14-9
63. Choi M, Ban T, Rhim T. Therapeutic use of stem cell transplantation for cell replacement or cytoprotective effect of microvesicle released from mesenchymal stem cell. Mol Cells (2014) 37(2):133–9. doi:10.14348/molcells.2014.2317
64. Bucala R, Spiegel LA, Chesney J, Hogan M, Cerami A. Circulating fibrocytes define a new leukocyte subpopulation that mediates tissue repair. Mol Med (1994) 1(1):71–81.
65. Phillips RJ, Burdick MD, Hong K, Lutz MA, Murray LA, Xue YY, et al. Circulating fibrocytes traffic to the lungs in response to CXCL12 and mediate fibrosis. J Clin Invest (2004) 114(3):438–46. doi:10.1172/JCI20997
66. Nikam VS, Schermuly RT, Dumitrascu R, Weissmann N, Kwapiszewska G, Morrell N, et al. Treprostinil inhibits the recruitment of bone marrow-derived circulating fibrocytes in chronic hypoxic pulmonary hypertension. Eur Respir J (2010) 36(6):1302–14. doi:10.1183/09031936.00028009
67. Alvarez DF, Huang L, King JA, ElZarrad MK, Yoder MC, Stevens T. Lung microvascular endothelium is enriched with progenitor cells that exhibit vasculogenic capacity. Am J Physiol Lung Cell Mol Physiol (2008) 294(3):L419–30. doi:10.1152/ajplung.00314.2007
68. Case J, Mead LE, Bessler WK, Prater D, White HA, Saadatzadeh MR, et al. Human CD34+AC133+VEGFR-2+ cells are not endothelial progenitor cells but distinct, primitive hematopoietic progenitors. Exp Hematol (2007) 35(7):1109–18. doi:10.1016/j.exphem.2007.04.002
69. Mao SZ, Ye X, Liu G, Song D, Liu SF. Resident endothelial cells and endothelial progenitor cells restore endothelial barrier function after inflammatory lung injury. Arterioscler Thromb Vasc Biol (2015) 35(7):1635–44. doi:10.1161/atvbaha.115.305519
70. Suzuki T, Suzuki S, Fujino N, Ota C, Yamada M, Suzuki T, et al. c-Kit immunoexpression delineates a putative endothelial progenitor cell population in developing human lungs. Am J Physiol Lung Cell Mol Physiol (2014) 306(9):L855–65. doi:10.1152/ajplung.00211.2013
71. Yoder MC, Mead LE, Prater D, Krier TR, Mroueh KN, Li F, et al. Redefining endothelial progenitor cells via clonal analysis and hematopoietic stem/progenitor cell principals. Blood (2007) 109(5):1801–9. doi:10.1182/blood-2006-08-043471
72. Toshner M, Voswinckel R, Southwood M, Al-Lamki R, Howard LS, Marchesan D, et al. Evidence of dysfunction of endothelial progenitors in pulmonary arterial hypertension. Am J Respir Crit Care Med (2009) 180(8):780–7. doi:10.1164/rccm.200810-1662OC
73. Timmermans F, Van Hauwermeiren F, De Smedt M, Raedt R, Plasschaert F, De Buyzere ML, et al. Endothelial outgrowth cells are not derived from CD133+ cells or CD45+ hematopoietic precursors. Arterioscler Thromb Vasc Biol (2007) 27(7):1572–9. doi:10.1161/atvbaha.107.144972
74. Ingram DA, Mead LE, Tanaka H, Meade V, Fenoglio A, Mortell K, et al. Identification of a novel hierarchy of endothelial progenitor cells using human peripheral and umbilical cord blood. Blood (2004) 104(9):2752–60. doi:10.1182/blood-2004-04-1396
75. Yoder MC, Ingram DA. The definition of EPCs and other bone marrow cells contributing to neoangiogenesis and tumor growth: is there common ground for understanding the roles of numerous marrow-derived cells in the neoangiogenic process? Biochim Biophys Acta (2009) 1796(1):50–4. doi:10.1016/j.bbcan.2009.04.002
76. Ormiston ML, Deng Y, Stewart DJ, Courtman DW. Innate immunity in the therapeutic actions of endothelial progenitor cells in pulmonary hypertension. Am J Respir Cell Mol Biol (2010) 43(5):546–54. doi:10.1165/rcmb.2009-0152OC
77. Zhao YD, Courtman DW, Deng Y, Kugathasan L, Zhang Q, Stewart DJ. Rescue of monocrotaline-induced pulmonary arterial hypertension using bone marrow-derived endothelial-like progenitor cells: efficacy of combined cell and eNOS gene therapy in established disease. Circ Res (2005) 96(4):442–50. doi:10.1161/01.RES.0000157672.70560.7b
78. Granton J, Langleben D, Kutryk MJ, Camack N, Galipeau J, Courtman D, et al. Endothelial NO-synthase gene-enhanced progenitor cell therapy for pulmonary arterial hypertension: the PHACeT trial. Circ Res (2015) 117(7):645–54. doi:10.1161/circresaha.114.305951
79. Alphonse RS, Vadivel A, Fung M, Shelley WC, Critser PJ, Ionescu L, et al. Existence, functional impairment, and lung repair potential of endothelial colony-forming cells in oxygen-induced arrested alveolar growth. Circulation (2014) 129(21):2144–57. doi:10.1161/CIRCULATIONAHA.114.009124
80. Alphonse RS, Vadivel A, Zong S, McConaghy S, Ohls R, Yoder MC, et al. The isolation and culture of endothelial colony-forming cells from human and rat lungs. Nat Protoc (2015) 10(11):1697–708. doi:10.1038/nprot.2015.107
81. Fang S, Wei J, Pentinmikko N, Leinonen H, Salven P. Generation of functional blood vessels from a single c-kit+ adult vascular endothelial stem cell. PLoS Biol (2012) 10(10):e1001407. doi:10.1371/journal.pbio.1001407
82. Ingram DA, Mead LE, Moore DB, Woodard W, Fenoglio A, Yoder MC. Vessel wall-derived endothelial cells rapidly proliferate because they contain a complete hierarchy of endothelial progenitor cells. Blood (2005) 105(7):2783–6. doi:10.1182/blood-2004-08-3057
83. Tuder RM, Groves B, Badesch DB, Voelkel NF. Exuberant endothelial cell growth and elements of inflammation are present in plexiform lesions of pulmonary hypertension. Am J Pathol (1994) 144(2):275–85.
84. Lee SD, Shroyer KR, Markham NE, Cool CD, Voelkel NF, Tuder RM. Monoclonal endothelial cell proliferation is present in primary but not secondary pulmonary hypertension. J Clin Invest (1998) 101(5):927–34. doi:10.1172/JCI1910
85. Kotton DN. Next-generation regeneration: the hope and hype of lung stem cell research. Am J Respir Crit Care Med (2012) 185(12):1255–60. doi:10.1164/rccm.201202-0228PP
86. Pittenger MF, Mackay AM, Beck SC, Jaiswal RK, Douglas R, Mosca JD, et al. Multilineage potential of adult human mesenchymal stem cells. Science (1999) 284(5411):143–7. doi:10.1126/science.284.5411.143
87. De Miguel MP, Fuentes-Julian S, Alcaina Y. Pluripotent stem cells: origin, maintenance and induction. Stem Cell Rev (2010) 6(4):633–49. doi:10.1007/s12015-010-9170-1
88. Li W, Wei W, Zhu S, Zhu J, Shi Y, Lin T, et al. Generation of rat and human induced pluripotent stem cells by combining genetic reprogramming and chemical inhibitors. Cell Stem Cell (2009) 4(1):16–9. doi:10.1016/j.stem.2008.11.014
89. O’Doherty R, Greiser U, Wang W. Nonviral methods for inducing pluripotency to cells. Biomed Res Int (2013) 2013:705902. doi:10.1155/2013/705902
90. Yamanaka S, Blau HM. Nuclear reprogramming to a pluripotent state by three approaches. Nature (2010) 465(7299):704–12. doi:10.1038/nature09229
91. Kadzik RS, Morrisey EE. Directing lung endoderm differentiation in pluripotent stem cells. Cell Stem Cell (2012) 10(4):355–61. doi:10.1016/j.stem.2012.03.013
92. Orlova VV, Mummery CL. What endothelial cells from patient iPSCs can tell us about aortic valve disease. Cell Stem Cell (2015) 16(5):455–7. doi:10.1016/j.stem.2015.04.006
93. Takase O, Yoshikawa M, Idei M, Hirahashi J, Fujita T, Takato T, et al. The role of NF-kappaB signaling in the maintenance of pluripotency of human induced pluripotent stem cells. PLoS One (2013) 8(2):e56399. doi:10.1371/journal.pone.0056399
94. Mathieu J, Zhang Z, Nelson A, Lamba DA, Reh TA, Ware C, et al. Hypoxia induces re-entry of committed cells into pluripotency. Stem Cells (2013) 31(9):1737–48. doi:10.1002/stem.1446
95. Prasain N, Lee MR, Vemula S, Meador JL, Yoshimoto M, Ferkowicz MJ, et al. Differentiation of human pluripotent stem cells to cells similar to cord-blood endothelial colony-forming cells. Nat Biotechnol (2014) 32(11):1151–7. doi:10.1038/nbt.3048
96. Treguer K, Heinrich EM, Ohtani K, Bonauer A, Dimmeler S. Role of the microRNA-17-92 cluster in the endothelial differentiation of stem cells. J Vasc Res (2012) 49(5):447–60. doi:10.1159/000339429
97. Ren X, Moser PT, Gilpin SE, Okamoto T, Wu T, Tapias LF, et al. Engineering pulmonary vasculature in decellularized rat and human lungs. Nat Biotechnol (2015) 33(10):1097–102. doi:10.1038/nbt.3354
98. Okano H, Nakamura M, Yoshida K, Okada Y, Tsuji O, Nori S, et al. Steps toward safe cell therapy using induced pluripotent stem cells. Circ Res (2013) 112(3):523–33. doi:10.1161/circresaha.111.256149
99. Jaalouk DE, Lammerding J. Mechanotransduction gone awry. Nat Rev Mol Cell Biol (2009) 10(1):63–73. doi:10.1038/nrm2597
100. Dickson KA, Harding R. Restoration of lung liquid volume following its acute alteration in fetal sheep. J Physiol (1987) 385:531–43. doi:10.1113/jphysiol.1987.sp016506
101. Hooper SB, Wallace MJ. Role of the physicochemical environment in lung development. Clin Exp Pharmacol Physiol (2006) 33(3):273–9. doi:10.1111/j.1440-1681.2006.04358.x
102. Stolberg S, McCloskey KE. Can shear stress direct stem cell fate? Biotechnol Prog (2009) 25(1):10–9. doi:10.1002/btpr.124
103. Bach KP, Kuschel CA, Hooper SB, Bertram J, McKnight S, Peachey SE, et al. High bias gas flows increase lung injury in the ventilated preterm lamb. PLoS One (2012) 7(10):e47044. doi:10.1371/journal.pone.0047044
104. Thannickal VJ, Henke CA, Horowitz JC, Noble PW, Roman J, Sime PJ, et al. Matrix biology of idiopathic pulmonary fibrosis: a workshop report of the national heart, lung, and blood institute. Am J Pathol (2014) 184(6):1643–51. doi:10.1016/j.ajpath.2014.02.003
105. Liu F, Tschumperlin DJ. Micro-mechanical characterization of lung tissue using atomic force microscopy. J Vis Exp (2011) (54):2911. doi:10.3791/2911
106. Stenmark KR, Meyrick B, Galie N, Mooi WJ, McMurtry IF. Animal models of pulmonary arterial hypertension: the hope for etiological discovery and pharmacological cure. Am J Physiol Lung Cell Mol Physiol (2009) 297(6):L1013–32. doi:10.1152/ajplung.00217.2009
107. Happe CM, De Raaf MA, Rol N, Schalij I, Vonk-Noordegraaf A, Westerhof N, et al. Pneumonectomy combined with SU5416 induces severe pulmonary hypertension in rats. Am J Physiol Lung Cell Mol Physiol (2016) 310(11):L1088–97. doi:10.1152/ajplung.00023.2016
108. Stenmark KR, Fagan KA, Frid MG. Hypoxia-induced pulmonary vascular remodeling: cellular and molecular mechanisms. Circ Res (2006) 99(7):675–91. doi:10.1161/01.RES.0000243584.45145.3f
109. Schafer M, Myers C, Brown RD, Frid MG, Tan W, Hunter K, et al. Pulmonary arterial stiffness: toward a new paradigm in pulmonary arterial hypertension pathophysiology and assessment. Curr Hypertens Rep (2016) 18(1):4. doi:10.1007/s11906-015-0609-2
110. Lee KM, Tsai KY, Wang N, Ingber DE. Extracellular matrix and pulmonary hypertension: control of vascular smooth muscle cell contractility. Am J Physiol (1998) 274(1 Pt 2):H76–82.
111. Brusasco V, Martinez F. Chronic obstructive pulmonary disease. Compr Physiol (2014) 4(1):1–31. doi:10.1002/cphy.c110037
112. Vanfleteren LEGW, Spruit MA, Groenen MTJ, Bruijnzeel PLB, Taib Z, Rutten EPA, et al. Arterial stiffness in patients with COPD: the role of systemic inflammation and the effects of pulmonary rehabilitation. Eur Respir J (2014) 43(5):1306–15. doi:10.1183/09031936.00169313
113. Ando J, Yamamoto K. Vascular mechanobiology. Circ J (2009) 73(11):1983–92. doi:10.1253/circj.CJ-09-0583
114. Obi S, Yamamoto K, Shimizu N, Kumagaya S, Masumura T, Sokabe T, et al. Fluid shear stress induces arterial differentiation of endothelial progenitor cells. J Appl Physiol (2009) 106(1):203–11. doi:10.1152/japplphysiol.00197.2008
115. Yamamoto K, Sokabe T, Watabe T, Miyazono K, Yamashita JK, Obi S, et al. Fluid shear stress induces differentiation of Flk-1-positive embryonic stem cells into vascular endothelial cells in vitro. Am J Physiol Heart Circ Physiol (2005) 288(4):H1915–24. doi:10.1152/ajpheart.00956.2004
116. Hanjaya-Putra D, Yee J, Ceci D, Truitt R, Yee D, Gerecht S. Vascular endothelial growth factor and substrate mechanics regulate in vitro tubulogenesis of endothelial progenitor cells. J Cell Mol Med (2010) 14(10):2436–47. doi:10.1111/j.1582-4934.2009.00981.x
117. Fioretta ES, Fledderus JO, Baaijens FPT, Bouten CVC. Influence of substrate stiffness on circulating progenitor cell fate. J Biomech (2012) 45(5):736–44. doi:10.1016/j.jbiomech.2011.11.013
118. Hamilton DW, Maul TM, Vorp DA. Characterization of the response of bone marrow-derived progenitor cells to cyclic strain: implications for vascular tissue-engineering applications. Tissue Eng (2004) 10(3–4):361–9. doi:10.1089/107632704323061726
119. Markowski MC, Brown AC, Barker TH. Directing epithelial to mesenchymal transition through engineered microenvironments displaying orthogonal adhesive and mechanical cues. J Biomed Mater Res (2012) 100(8):2119–27. doi:10.1002/jbm.a.34068
120. Leight JL, Wozniak MA, Chen S, Lynch ML, Chen CS. Matrix rigidity regulates a switch between TGF-β1-induced apoptosis and epithelial-mesenchymal transition. Mol Biol Cell (2012) 23(5):781–91. doi:10.1091/mbc.E11-06-0537
121. Yeh YT, Hur SS, Chang J, Wang KC, Chiu JJ, Li YS, et al. Matrix stiffness regulates endothelial cell proliferation through septin 9. PLoS One (2012) 7(10):e46889. doi:10.1371/journal.pone.0046889
122. Zhang H, Chang H, Wang L-M, Ren K-F, Martins MCL, Barbosa MA, et al. Effect of polyelectrolyte film stiffness on endothelial cells during endothelial-to-mesenchymal transition. Biomacromolecules (2015) 16(11):3584–93. doi:10.1021/acs.biomac.5b01057
123. Medici D, Potenta S, Kalluri R. Transforming growth factor-beta2 promotes Snail-mediated endothelial-mesenchymal transition through convergence of Smad-dependent and Smad-independent signalling. Biochem J (2011) 437(3):515–20. doi:10.1042/bj20101500
124. Gawlak G, Son S, Tian Y, O’Donnell JJ, Birukov KG, Birukova AA. Chronic high magnitude cyclic stretch stimulates EC inflammatory response via VEGF receptor 2 dependent mechanism. Am J Physiol Lung Cell Mol Physiol (2016) 310(11):L1062–70. doi:10.1152/ajplung.00317.2015
125. Tian Y, Gawlak G, O’Donnell JJ, Birukova AA, Birukov KG. Activation of VEGF receptor-2 mediates endothelial permeability caused by cyclic stretch. J Biol Chem (2016) 291(19):10032–45. doi:10.1074/jbc.M115.690487
126. Discher DE, Mooney DJ, Zandstra PW. Growth factors, matrices, and forces combine and control stem cells. Science (2009) 324(5935):1673–7. doi:10.1126/science.1171643
127. Guo S, Cheng Y, Ma Y, Yang X. Endothelial progenitor cells derived from CD34+ cells form cooperative vascular networks. Cell Physiol Biochem (2010) 26(4–5):679–88. doi:10.1159/000322335
128. Engler AJ, Sen S, Sweeney HL, Discher DE. Matrix elasticity directs stem cell lineage specification. Cell (2006) 126(4):677–89. doi:10.1016/j.cell.2006.06.044
129. Zhang S, Sun A, Ma H, Yao K, Zhou N, Shen L, et al. Infarcted myocardium-like stiffness contributes to endothelial progenitor lineage commitment of bone marrow mononuclear cells. J Cell Mol Med (2011) 15(10):2245–61. doi:10.1111/j.1582-4934.2010.01217.x
Keywords: stem cells, lung diseases, pulmonary circulation/physiology, bioengineering, mechanotransduction, cellular
Citation: Heise RL, Link PA and Farkas L (2016) From Here to There, Progenitor Cells and Stem Cells Are Everywhere in Lung Vascular Remodeling. Front. Pediatr. 4:80. doi: 10.3389/fped.2016.00080
Received: 29 April 2016; Accepted: 20 July 2016;
Published: 17 August 2016
Edited by:
Michael E. Yeager, University of Colorado Denver, USAReviewed by:
Yusei Ohshima, University of Fukui, JapanKostas N. Priftis, National and Kapodistrian University of Athens, Greece
Copyright: © 2016 Heise, Link and Farkas. This is an open-access article distributed under the terms of the Creative Commons Attribution License (CC BY). The use, distribution or reproduction in other forums is permitted, provided the original author(s) or licensor are credited and that the original publication in this journal is cited, in accordance with accepted academic practice. No use, distribution or reproduction is permitted which does not comply with these terms.
*Correspondence: Laszlo Farkas, laszlo.farkas@vcuhealth.org, lfarkasmd@gmail.com