- 1Department of Pediatrics-Critical Care, University of Colorado Denver, Denver, CO, USA
- 2Cardiovascular Pulmonary Research, University of Colorado Denver, Denver, CO, USA
- 3Department of Bioengineering, University of Colorado Denver, Denver, CO, USA
- 4Linda Crnic Institute for Down Syndrome, University of Colorado Denver, Denver, CO, USA
Pediatric lung diseases remain a costly worldwide health burden. For many children with end-stage lung disease, lung transplantation remains the only therapeutic option. Due to the limited number of lungs available for transplantation, alternatives to lung transplant are desperately needed. Recently, major improvements in tissue engineering have resulted in newer technology and methodology to develop viable bioengineered lungs. These include critical advances in lung cell biology, stem cell biology, lung extracellular matrix, microfabrication techniques, and orthotopic transplantation of bioartificial lungs. The goal of this short review is to engage the reader’s interest with regard to these emerging concepts and to stimulate their interest to learn more. We review the existing state of the art of lung tissue engineering, and point to emerging paradigms and platforms in the field. Finally, we summarize the challenges and unmet needs that remain to be overcome.
Introduction
The Study of Lung Disease Continues to Be Transformed by Biotechnology and Bioengineering
The lung is a highly complex organ that accomplishes a great variety of physiological tasks. For many chronic parenchymal and vascular lung diseases, lung transplantation, first performed in 1963 (1), becomes the last treatment option (2). Despite the progressive increase in the number of lung transplants, the number of lungs suitable for transplantation has decreased (2, 3). Mirroring this, lung transplantation for pediatric lung diseases, especially cystic fibrosis and idiopathic pulmonary hypertension, is a viable therapeutic option (4). Since the first pediatric lung transplant in 1987, the number of lung transplants performed in children is around 100 per year (5). This small number does not reflect a small clinical need, but rather is an indication of the complexities of lung transplant such as special surgical challenges related to size matching, availability of suitable donor lungs, medical center expertise, and immune system issues (6, 7). Realization of the dream of bioengineering lungs suitable for transplant in children would potentially overcome most, if not all, of these collective barriers. New tissue and cell-based techniques to study the lung, combined with the incorporation of bioengineering approaches, have the potential to increase our understanding of its complex biology, improve current therapies to obviate the need for transplant, and possibly increase the number of lungs available for transplant. Herein, we provide a short review of newer technologies and paradigms driving this exciting endeavor, with the goal to stimulate the reader toward further in-depth reading on the subject.
Emerging Paradigms
In recent years, a number of research themes have emerged with regard to chronic lung disease. For example, the details of how the type of lung injury affects the downstream disease pathogenesis are much more concrete. The ability to replicate the aspects of lung pathology as close to the human condition as possible obviously relies on a deep understanding of the disease process. Pulmonary hypertension (8), lung cancer (9), chronic obstructive pulmonary disease (10), acute respiratory distress (11), and interstitial lung disease (12) are lung disorders that have been modeled extensively using in vivo systems, and some have utilized sophisticated in vitro systems [well-summarized in Ref. (13)]. Again, the extent to which any of these in vitro systems faithfully recapitulates the aspects of human lung disease (especially in children), or the pathobiology of animal models of lung disease, depends largely on the nature of the injury: infection (lipopolysaccharide), physical injury (hemodynamic), chemical injury (bleomycin), and hypersensitivity reactions (ovalbumin) have all been investigated (13).
In addition to the nature of the lung injury, the roles of lung endogenous and exogenous stem cells in disease processes are being increasingly investigated using in vitro lung modeling systems. In a recent review (14), Weiss summarized the proceedings from the 2011 Vermont Stem Cell Conference, and the reader is urged to consult this review for a glossary of stem cell terminology. The lung appears to be populated with endogenous stem and progenitor cells, from the trachea to the distal airways and alveoli. With regard to the lung vasculature, the precise identity and tissue location(s) of putative endogenous lung vascular stem cells have remained elusive. However, endothelial progenitor cells (circulating bone marrow-derived), fibrocytes, and mesenchymal stem cells have all been identified as having immunomodulatory and/or tissue remodeling properties in certain contexts in both the airways and the pulmonary vasculature (15). As the human lung is comprised of over 40 cell types (15), it is critically important to understand how the lung utilizes endogenous and non-lung stem or progenitor cells to maintain homeostasis and respond appropriately to injury. Indeed, the steady-state lung is extraordinarily quiescent with respect to cell proliferation, and much of what we appreciate about lung stem or progenitor cells derives from animal models of lung injury (16). Deriving stem and progenitor cells for the purpose of lung-directed therapies seems a much closer reality with the advent of strategies to differentiate embryonic stem cells and inducible pluripotent stem cells into functional lung cell lineages (17, 18).
There is an increasing awareness that cells themselves (“seeds”) represent only a portion of what makes up the collective identity of a tissue. Indeed, the functions of the extracellular matrix (ECM, “soil”) with respect to control of lung homeostasis and its impact on both endogenous and non-lung resident cells in terms of their positional and morphogenetic guidance cues are coming into sharper focus. For example, seeding of fibroblasts onto decellularized fibrotic lung results in differentiation into myofibroblasts compared to seeding on decellularized control lungs (19). Thus, the biochemical composition and the architecture of the ECM combine to provide cellular cues important for phenotypic designation, localization/addressing, and function (20). In 2008, a decellularized trachea re-seeded with primary autologous cells was transplanted to replace a small length of airway and did not require immunosuppression to remain patent (21). Numerous approaches to decellularize rodent, pig, and human lungs, as well as the information gleaned regarding the impact of ECM components on the performance of the resulting scaffolds have been extensively reviewed (15, 22, 23). Collectively, these data point to the overall feasibility of using decellularized lungs as scaffolds for tissue engineering. This could be especially important in children, given the range of lung sizes required to treat the pediatric transplant patient. These studies also have highlighted the importance of increasing our understanding of how decellularization procedures impact the lung in terms of biomechanics, ECM composition and architecture, as well as its ability to facilitate cell seeding (22).
Emerging Platforms
Tissue engineering is a multidisciplinary process by which life sciences, materials science, and engineering principles can be married together with transplant science to achieve the goal of restoring organ and/or tissue function (24). When considering engineering any part of the lung, most approaches involve either cell therapy or utilize cell-matrix constructs (23). A smaller number of studies have focused on the development and testing of tissue-engineered scaffolds and/or cell culture devices. Regardless of approach, consideration must be given to the cell types involved (stem cells, sources of cells, etc.), the nature of the scaffolding, and the means by which the cell-matrix construct is sustained [summarized nicely in Ref. (23)]. The size of the model system (diffusion distances for gas exchange), as well as the methods used to evaluate and validate its performance, is additional critical considerations (13). Figure 1 summarizes the large variety of scientific disciplines that converge on the path toward bioengineering a lung.
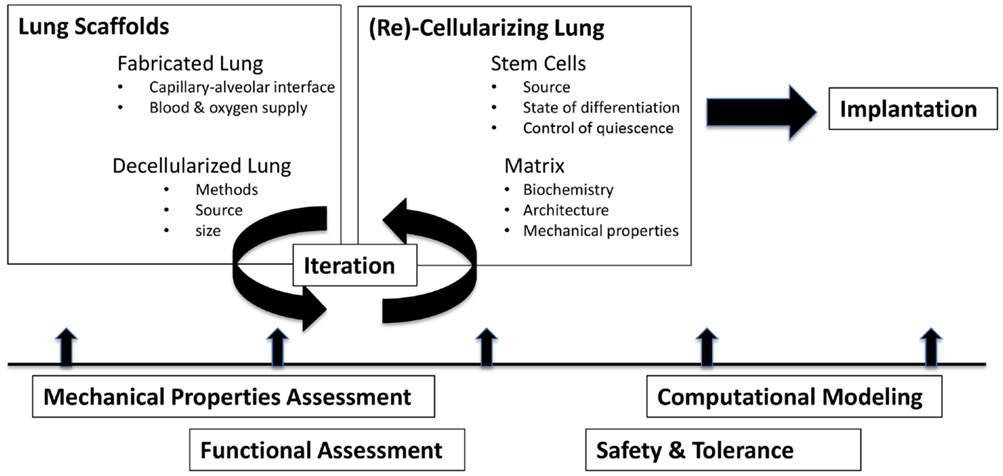
Figure 1. Challenges in bioengineering lungs to treat pediatric lung disease. Ideally, lung scaffolds of appropriate size, architecture, and complexity are prepared and then seeded with cells and/or matrix. In each stage of development, assessments of function, safety, mechanics, and efficacy each contribute to an iterative process of refinement.
Lung Scaffolds
It is generally accepted that decellularized lung scaffolds likely provide more appropriate organotypic and inductive signals by virtue of the ECM architecture (15). The methodology of decellularization (25), the amount of residual cellular or biochemical (DNA, detergent) material in the scaffold (26, 27), and the nature of the residual ECM proteins and proteoglycans (and their regional differences in the lung) (28, 29) are all important questions under intense investigation. With regard to the developing lung of a child, there may be age-specific cues in the ECM that are presently unappreciated. In addition, the use of cadaveric lungs as potential scaffolds, as well as the possibility of using animal-derived scaffolds (pig, sheep) is all avenues of active investigation. Several methods of decellularizing the lung are widely used, originating from some of the earliest efforts to decellularize rat lung (30). Regardless of technique or solution used, the goal is to remove as much cellular material and debris as possible, while maintaining as much biochemical and architectural character as possible. The use of perfusion-decellularization (31), as a means to achieve this goal, has gained prominence in recent years. Detergent-based solutions, such as sodium deoxycholate, sodium dodecyl sulfate, triton x-100, and CHAPS, can be perfused into cannulated airways and blood vessels of the lung (25). Depending on the detergents used, the duration of the procedure, and the choice of perfusion route(s), lung cells are effectively removed with minimal compromise to the vessels, airways, and lung interstitium [tabulated nicely by Wagner et al. (15)]. Due to the wide variety of approaches used, there is considerable variability in the results reported and difficulty in reproducibility and consistency of findings in the literature. Thus, direct comparison of results is problematic (32). In addition to the decellularization protocols themselves, methods of assessing the extent of decellularization also vary substantially. At present, there is no consensus regarding acceptable levels of residual cellular material, often measured by DNA content (33). Furthermore, depending on method, the ECM is altered to a greater or lesser extent during decellularization procedures as evidenced by variable collagen, elastin, and proteoglycan staining (34) and by changes in stiffness (35). Curiously, despite differences in ECM content and character, decellularized lung scaffolds all seem to accommodate reseeding with cells (36). Future studies will need to rigorously standardize decellularization techniques to move beyond methodologies based primarily on anecdotal experience.
Fabricated Devices
In addition to scaffold derived from in vivo sources, alternative platforms are continuously being developed. Significant challenges in design arise owing to the complexity of the lung architecture as well as the high dynamic range of functions that lung tissue accomplishes. A bioengineered lung would need to provide surface area for gas exchange over a thin non-porous but gas permeable membrane. To supply blood and oxygen to such an interface and to match ventilation with perfusion would require an even more highly complex device. Microfabrication techniques have been used to produce “organs on a chip” (37), including the lung. By layering a group of individual vascular networks with gas exchange compartments composed of polydimethylsiloxane (PDMS), a large surface area to volume ratio can be achieved (38). PDMS is an ideal material for this application given its non-porosity to water-based fluids, its permeability to gases, and its capacity to be 3-D printed and formed into microscale structures (39). Extracorporeal lung assist devices utilize such technology to provide oxygenator support for cardiac surgery and as lung assist devices for preterm infants with respiratory failure (40). In one study, a variety of blood flow rates and layering designs were tested and the resulting hydraulic resistances, oxygen transfer rates, and shear forces were calculated (38). Although scaling up to physiologically relevant sizes is accompanied by technological barriers (38), these devices have demonstrated proof of principle with regard to studying the alveolar-capillary unit (41), gas exchange (42), the effect of mechanical stretch during breathing cycles (43), and vascular networks (44, 45). Scaling up of such devices into more durable and portable designs would potentially provide continuous gas exchange support. Such biologically inspired technologies will undoubtedly continue to yield key insights into the structural and mechanical aspects of lung biology that influence interstitial fluid flow, immune-cell trafficking, and repair processes in response to injury such as edema (46). However, fabricated devices for lung oxygenation would need to fulfill several additional design criteria that are largely obviated by use of decellularized/recellularized lungs: (1) provide appropriate surface area-volume ratios, (2) conduct blood to gas exchange interfaces while avoiding thrombus, inappropriate shear force, (3) operate using room air without a pump, (4) work with the right ventricular output without need for external blood pump (47).
Validation and Evaluation of Performance
Equally as important as the design of new methods and devices is the need to validate their performance. Determination of the mechanical properties of bioengineered scaffolds and the regions of the lung they are intended to mimic using atomic force microscopy (AFM) (29, 35, 48) and microstretching techniques (49) will continue to be critical to moving forward (20). Indeed, comparative measurement of the mechanical properties of diseased tissues compared to controls is becoming increasingly important and informative. AFM is a technique that can be used to assess mechanical properties of microscale regions of lung tissue. A small tip mounted on a cantilever spring and connected to a piezoelectric positioner, laser and position-sensitive detector is applied over a surface, akin to a phonograph needle moving over a vinyl record. The vertical motion of the tip can be measured as force and magnitude as the tip is moved over the surface of the lung. The resulting force–motion data at each location, combined with the extent of the tissue deformation at the probe tip can be used to calculate the elastic modulus (from the slope of stress-strain curves). AFM data derived from bleomycin-induced pulmonary fibrosis in decellularized mouse lungs indicated a twofold increase in stiffness compared to controls but with widespread inhomogeneity (48). Although AFM is powerful for measuring material stiffness of lung tissue, it requires unique expertise, is not suitable for whole lung analyses, and is not a high-throughput technique. Estimations of mechanical properties of lung such as stiffness can be inferred by techniques that combine stretching lung tissue with microscopic examination of cells and matrix components (49). By examination of how matrix components such as collagen and elastin fibrils orient during stretch, the mechanical properties of lung tissue can be estimated. The aspect ratios of cells in the lung region being stretched can also be informative of tissue mechanical properties (50). Regardless of the techniques used to measure or estimate the mechanical properties of intact and decellularized normal and diseased lungs, further refinement of both design and data validation will be increasingly informed by mathematical modeling (51–53).
Collectively, the evaluation of the engineered tissue will require rigorous assessment of the function of cells and the scaffold as a system (13). The reader is directed to two excellent reviews that summarize our current knowledge of tissue-engineered models of normal and diseased lung (13, 54). In addition to function, the safety (tolerance, immunogenicity, etc.) of implanted bioengineered lungs requires careful evaluation.
Conclusion: Challenges and Unmet Needs
The need to better treat end-stage of both adult and pediatric lung disease using newer methods and twenty-first-century technology cannot be overstated. In the United States, ~30 million people live with end-stage lung disease (55), for which lung transplantation often is the only effective treatment option. However, less than ~2,000 lung transplants are performed annually (55). The situation for children with end-stage lung disease, especially cystic fibrosis and idiopathic pulmonary artery hypertension, is even more complex (5). Recent efforts to transplant bioengineered lungs into animal models have met with tantalizing success (55, 56), but currently are not yet translatable to the clinic and no studies have yet focused on a pediatric setting. In this mini-review, we have focused on the lung, but regardless of the organ, the overarching goals for bioengineering are essentially the same and have been reviewed (22, 25). The obvious challenges to successful optimization of lung physiology such as gas exchange, nervous system innervation, ventilation-perfusion matching, and air conduction from trachea to alveolar spaces represent a huge dynamic range of physiologic and morphologic complexity. The lungs in a developing child represent additional challenges. As new technologies and approaches emerge, the bottlenecks of reproducibility and high throughput will need to be overcome. Further barriers to progress related to implantation of recellularized scaffold (57) and the potential for untoward immunogenicity (58, 59) are daunting. Finally, improving the reproducibility of the methodologies to generate decellularized scaffolds, as well as reducing intra-assay and inter-assay variability is important and worthy goals. Over the past 20 years, The National Institutes of Health, the National Science Foundation (NSF), and private foundations have dramatically increased funding for tissue engineering (search of NSF website, April 2015; http://www.nsf.gov/pubs/2004/nsf0450/inst_supp.htm). In parallel, there has been significant growth (~threefold from 2008 to 2011) in sales generated by tissue engineering and stem cell industries, estimated in 2011 to be $3.5 billion annually (60). Such dynamic economic forces will undoubtedly help the field overcome many of the daunting obstacles and deliver on past speculations made as far back as 1998: “In the next 10 years, a veritable body shop of spare parts will wend its way from labs to patients.” (61). Indeed, bioengineering the lung holds the promise to profoundly change the lives of children with intractable lung disease, from basic science, to increased efficacy of drug screens and their targets, to successful implantation of replacement lung tissue.
Conflict of Interest Statement
The authors declare that the research was conducted in the absence of any commercial or financial relationships that could be construed as a potential conflict of interest.
Acknowledgments
Both authors contributed equally to this review. The authors have declared no conflict of interest. MEY is funded by a seed grant from the Linda Crnic Institute for Down Syndrome.
Abbreviations
ECM, extracellular matrix.
References
1. Hardy JD, Webb WR, Dalton ML Jr, Walker GR Jr. Lung homotransplantation in man. JAMA (1963) 186:1065–74. doi:10.1001/jama.1963.63710120001010
2. Whitson BA, Hayes D Jr. Indications and outcomes in adult lung transplantation. J Thorac Dis (2014) 6:1018–23. doi:10.3978/j.issn.2072-1439.2014.07.04
3. Yusen RD, Christie JD, Edwards LB, Kucheryavaya AY, Benden C, Dipchand AI, et al. The registry of the international society for heart and lung transplantation: thirtieth adult lung and heart-lung transplant report – 2013; focus theme: age. J Heart Lung Transplant (2013) 32:965–78. doi:10.1016/j.healun.2013.08.007
4. Takatsuki S, Ivy DD. Current challenges in pediatric pulmonary hypertension. Semin Respir Crit Care Med (2013) 34:627–44. doi:10.1055/s-0033-1356461
5. Kirkby S, Hayes D Jr. Pediatric lung transplantation: indications and outcomes. J Thorac Dis (2014) 6:1024–31. doi:10.3978/j.issn.2072-1439.2014.04.27
6. Goldstein BS, Sweet SC, Mao J, Huddleston CB, Grady RM. Lung transplantation in children with idiopathic pulmonary arterial hypertension: an 18-year experience. J Heart Lung Transplant (2011) 30:1148–52. doi:10.1016/j.healun.2011.04.009
7. Gruber S, Eiwegger T, Nachbaur E, Tiringer K, Aigner C, Jaksch P, et al. Lung transplantation in children and young adults: a 20-year single-centre experience. Eur Respir J (2012) 40:462–9. doi:10.1183/09031936.00092211
8. Colvin KL, Yeager ME. Animal models of pulmonary hypertension: matching disease mechanisms to etiology of the human disease. J Pulm Respir Med (2014) 4(4):198.
9. Hayes SA, Hudson AL, Clarke SJ, Molloy MP, Howell VM. From mice to men: GEMMs as trial patients for new NSCLC therapies. Semin Cell Dev Biol (2014) 27:118–27. doi:10.1016/j.semcdb.2014.04.002
10. Fricker M, Deane A, Hansbro PM. Animal models of chronic obstructive pulmonary disease. Expert Opin Drug Discov (2014) 9:629–45. doi:10.1517/17460441.2014.909805
11. Fard N, Saffari A, Emami G, Hofer S, Kauczor HU, Mehrabi A. Acute respiratory distress syndrome induction by pulmonary ischemia-reperfusion injury in large animal models. J Surg Res (2014) 189:274–84. doi:10.1016/j.jss.2014.02.034
12. Moore BB, Lawson WE, Oury TD, Sisson TH, Raghavendran K, Hogaboam CM. Animal models of fibrotic lung disease. Am J Respir Cell Mol Biol (2013) 49:167–79. doi:10.1165/rcmb.2013-0094TR
13. Nichols JE, Niles JA, Vega SP, Argueta LB, Eastaway A, Cortiella J. Modeling the lung: design and development of tissue engineered macro- and micro-physiologic lung models for research use. Exp Biol Med (2014) 239:1135–69. doi:10.1177/1535370214536679
14. Weiss DJ. Stem cells, cell therapies, and bioengineering in lung biology and diseases. Comprehensive review of the recent literature 2010-2012. Ann Am Thorac Soc (2013) 10:S45–97. doi:10.1513/AnnalsATS.201304-090AW
15. Wagner DE, Bonvillain RW, Jensen T, Girard ED, Bunnell BA, Finck CM, et al. Can stem cells be used to generate new lungs? Ex vivo lung bioengineering with decellularized whole lung scaffolds. Respirology (2013) 18:895–911. doi:10.1111/resp.12102
16. Kotton DN, Morrisey EE. Lung regeneration: mechanisms, applications and emerging stem cell populations. Nat Med (2014) 20:822–32. doi:10.1038/nm.3642
17. Longmire TA, Ikonomou L, Hawkins F, Christodoulou C, Cao Y, Jean JC, et al. Efficient derivation of purified lung and thyroid progenitors from embryonic stem cells. Cell Stem Cell (2012) 10:398–411. doi:10.1016/j.stem.2012.01.019
18. Christodoulou C, Longmire TA, Shen SS, Bourdon A, Sommer CA, Gadue P, et al. Mouse ES and iPS cells can form similar definitive endoderm despite differences in imprinted genes. J Clin Invest (2011) 121:2313–25. doi:10.1172/JCI43853
19. Booth AJ, Hadley R, Cornett AM, Dreffs AA, Matthes SA, Tsui JL, et al. Acellular normal and fibrotic human lung matrices as a culture system for in vitro investigation. Am J Respir Crit Care Med (2012) 186:866–76. doi:10.1164/rccm.201204-0754OC
20. Suki B. Assessing the functional mechanical properties of bioengineered organs with emphasis on the lung. J Cell Physiol (2014) 229:1134–40. doi:10.1002/jcp.24600
21. Macchiarini P, Jungebluth P, Go T, Asnaghi MA, Rees LE, Cogan TA, et al. Clinical transplantation of a tissue-engineered airway. Lancet (2008) 372:2023–30. doi:10.1016/S0140-6736(08)61598-6
22. Calle EA, Ghaedi M, Sundaram S, Sivarapatna A, Tseng MK, Niklason LE. Strategies for whole lung tissue engineering. IEEE Trans Biomed Eng (2014) 61:1482–96. doi:10.1109/TBME.2014.2314261
23. Fishman JM, Lowdell M, Birchall MA. Stem cell-based organ replacements-airway and lung tissue engineering. Semin Pediatr Surg (2014) 23:119–26. doi:10.1053/j.sempedsurg.2014.04.002
25. Tapias LF, Ott HC. Decellularized scaffolds as a platform for bioengineered organs. Curr Opin Organ Transplant (2014) 19:145–52. doi:10.1097/MOT.0000000000000051
26. Wagner DE, Bonenfant NR, Sokocevic D, DeSarno MJ, Borg ZD, Parsons CS, et al. Three-dimensional scaffolds of acellular human and porcine lungs for high throughput studies of lung disease and regeneration. Biomaterials (2014) 35:2664–79. doi:10.1016/j.biomaterials.2013.11.078
27. Cebotari S, Tudorache I, Jaekel T, Hilfiker A, Dorfman S, Ternes W, et al. Detergent decellularization of heart valves for tissue engineering: toxicological effects of residual detergents on human endothelial cells. Artif Organs (2010) 34:206–10. doi:10.1111/j.1525-1594.2009.00796.x
28. Lin YM, Zhang A, Rippon HJ, Bismarck A, Bishop AE. Tissue engineering of lung: the effect of extracellular matrix on the differentiation of embryonic stem cells to pneumocytes. Tissue Eng Part A (2010) 16:1515–26. doi:10.1089/ten.TEA.2009.0232
29. Luque T, Melo E, Garreta E, Cortiella J, Nichols J, Farre R, et al. Local micromechanical properties of decellularized lung scaffolds measured with atomic force microscopy. Acta Biomater (2013) 9:6852–9. doi:10.1016/j.actbio.2013.02.044
30. Lwebuga-Mukasa JS, Ingbar DH, Madri JA. Repopulation of a human alveolar matrix by adult rat type II pneumocytes in vitro. A novel system for type II pneumocyte culture. Exp Cell Res (1986) 162:423–35. doi:10.1016/0014-4827(86)90347-2
31. Ott HC, Matthiesen TS, Goh SK, Black LD, Kren SM, Netoff TI, et al. Perfusion-decellularized matrix: using nature’s platform to engineer a bioartificial heart. Nat Med (2008) 14:213–21. doi:10.1038/nm1684
32. He M, Callanan A. Comparison of methods for whole-organ decellularization in tissue engineering of bioartificial organs. Tissue Eng Part B Rev (2013) 19:194–208. doi:10.1089/ten.TEB.2012.0340
33. Jensen T, Roszell B, Zang F, Girard E, Matson A, Thrall R, et al. A rapid lung de-cellularization protocol supports embryonic stem cell differentiation in vitro and following implantation. Tissue Eng Part C Methods (2012) 18:632–46. doi:10.1089/ten.TEC.2011.0584
34. Price AP, England KA, Matson AM, Blazar BR, Panoskaltsis-Mortari A. Development of a decellularized lung bioreactor system for bioengineering the lung: the matrix reloaded. Tissue Eng Part A (2010) 16:2581–91. doi:10.1089/ten.TEA.2009.0659
35. Melo E, Garreta E, Luque T, Cortiella J, Nichols J, Navajas D, et al. Effects of the decellularization method on the local stiffness of acellular lungs. Tissue Eng Part C Methods (2014) 20:412–22. doi:10.1089/ten.TEC.2013.0325
36. Wallis JM, Borg ZD, Daly AB, Deng B, Ballif BA, Allen GB, et al. Comparative assessment of detergent-based protocols for mouse lung de-cellularization and re-cellularization. Tissue Eng Part C Methods (2012) 18:420–32. doi:10.1089/ten.TEC.2011.0567
37. Selimovic S, Dokmeci MR, Khademhosseini A. Organs-on-a-chip for drug discovery. Curr Opin Pharmacol (2013) 13:829–33. doi:10.1016/j.coph.2013.06.005
38. Kniazeva T, Epshteyn AA, Hsiao JC, Kim ES, Kolachalama VB, Charest JL, et al. Performance and scaling effects in a multilayer microfluidic extracorporeal lung oxygenation device. Lab Chip (2012) 12:1686–95. doi:10.1039/c2lc21156d
39. Kamei K, Mashimo Y, Koyama Y, Fockenberg C, Nakashima M, Nakajima M, et al. 3D printing of soft lithography mold for rapid production of polydimethylsiloxane-based microfluidic devices for cell stimulation with concentration gradients. Biomed Microdevices (2015) 17:9928. doi:10.1007/s10544-015-9928-y
40. Wu WI, Rochow N, Chan E, Fusch G, Manan A, Nagpal D, et al. Lung assist device: development of microfluidic oxygenators for preterm infants with respiratory failure. Lab Chip (2013) 13:2641–50. doi:10.1039/c3lc41417e
41. Huh D, Matthews BD, Mammoto A, Montoya-Zavala M, Hsin HY, Ingber DE. Reconstituting organ-level lung functions on a chip. Science (2010) 328:1662–8. doi:10.1126/science.1188302
42. Kniazeva T, Hsiao JC, Charest JL, Borenstein JT. A microfluidic respiratory assist device with high gas permeance for artificial lung applications. Biomed Microdevices (2011) 13:315–23. doi:10.1007/s10544-010-9495-1
43. Douville NJ, Zamankhan P, Tung YC, Li R, Vaughan BL, Tai CF, et al. Combination of fluid and solid mechanical stresses contribute to cell death and detachment in a microfluidic alveolar model. Lab Chip (2011) 11:609–19. doi:10.1039/c0lc00251h
44. Hoganson DM, Pryor HI II, Spool ID, Burns OH, Gilmore JR, Vacanti JP. Principles of biomimetic vascular network design applied to a tissue-engineered liver scaffold. Tissue Eng Part A (2010) 16:1469–77. doi:10.1089/ten.TEA.2009.0118
45. Hoganson DM, Pryor HI II, Vacanti JP. Tissue engineering and organ structure: a vascularized approach to liver and lung. Pediatr Res (2008) 63:520–6. doi:10.1203/01.pdr.0000305879.38476.0c
46. Huh D, Leslie DC, Matthews BD, Fraser JP, Jurek S, Hamilton GA, et al. A human disease model of drug toxicity-induced pulmonary edema in a lung-on-a-chip microdevice. Sci Transl Med (2012) 4:159ra147. doi:10.1126/scitranslmed.3004249
47. Potkay JA. The promise of microfluidic artificial lungs. Lab Chip (2014) 14:4122–38. doi:10.1039/c4lc00828f
48. Melo E, Cardenes N, Garreta E, Luque T, Rojas M, Navajas D, et al. Inhomogeneity of local stiffness in the extracellular matrix scaffold of fibrotic mouse lungs. J Mech Behav Biomed Mater (2014) 37:186–95. doi:10.1016/j.jmbbm.2014.05.019
49. Kononov S, Brewer K, Sakai H, Cavalcante FS, Sabayanagam CR, Ingenito EP, et al. Roles of mechanical forces and collagen failure in the development of elastase-induced emphysema. Am J Respir Crit Care Med (2001) 164:1920–6. doi:10.1164/ajrccm.164.10.2101083
50. Bartolak-Suki E, LaPrad AS, Harvey BC, Suki B, Lutchen KR. Tidal stretches differently regulate the contractile and cytoskeletal elements in intact airways. PLoS One (2014) 9:e94828. doi:10.1371/journal.pone.0094828
51. Glynn P, Unudurthi SD, Hund TJ. Mathematical modeling of physiological systems: an essential tool for discovery. Life Sci (2014) 111:1–5. doi:10.1016/j.lfs.2014.07.005
52. Tewari SG, Bugenhagen SM, Wang Z, Schreier DA, Carlson BE, Chesler NC, et al. Analysis of cardiovascular dynamics in pulmonary hypertensive C57BL6/J mice. Front Physiol (2013) 4:355. doi:10.3389/fphys.2013.00355
53. Cavalcante FS, Ito S, Brewer K, Sakai H, Alencar AM, Almeida MP, et al. Mechanical interactions between collagen and proteoglycans: implications for the stability of lung tissue. J Appl Physiol (2005) 98:672–9. doi:10.1152/japplphysiol.00619.2004
54. Ben-Tal A, Tawhai MH. Integrative approaches for modeling regulation and function of the respiratory system. Wiley Interdiscip Rev Syst Biol Med (2013) 5:687–99. doi:10.1002/wsbm.1244
55. Song JJ, Kim SS, Liu Z, Madsen JC, Mathisen DJ, Vacanti JP, et al. Enhanced in vivo function of bioartificial lungs in rats. Ann Thorac Surg (2011) 92:998–1005; discussion 1005–6. doi:10.1016/j.athoracsur.2011.05.018
56. Ott HC, Clippinger B, Conrad C, Schuetz C, Pomerantseva I, Ikonomou L, et al. Regeneration and orthotopic transplantation of a bioartificial lung. Nat Med (2010) 16:927–33. doi:10.1038/nm.2193
57. Petersen TH, Calle EA, Zhao L, Lee EJ, Gui L, Raredon MB, et al. Tissue-engineered lungs for in vivo implantation. Science (2010) 329:538–41. doi:10.1126/science.1189345
58. Badylak SF, Gilbert TW. Immune response to biologic scaffold materials. Semin Immunol (2008) 20:109–16. doi:10.1016/j.smim.2007.11.003
59. Shaw AS, Filbert EL. Scaffold proteins and immune-cell signalling. Nat Rev Immunol (2009) 9:47–56. doi:10.1038/nri2473
60. Jaklenec A, Stamp A, Deweerd E, Sherwin A, Langer R. Progress in the tissue engineering and stem cell industry “are we there yet?” Tissue Eng Part B Rev (2012) 18:155–66. doi:10.1089/ten.TEB.2011.0553
Keywords: pediatric, bioengineering, lung, extracellular matrix, stem/progenitor cell, scaffold
Citation: Colvin KL and Yeager ME (2015) Applying biotechnology and bioengineering to pediatric lung disease: emerging paradigms and platforms. Front. Pediatr. 3:45. doi: 10.3389/fped.2015.00045
Received: 25 November 2014; Accepted: 08 May 2015;
Published: 09 June 2015
Edited by:
Philip Keith Pattemore, University of Otago, New ZealandReviewed by:
Hui-leng Tan, Royal Brompton & Harefield NHS Foundation Trust, UKGeoffrey Mark Shaw, Canterbury District Health Board, New Zealand
Copyright: © 2015 Colvin and Yeager. This is an open-access article distributed under the terms of the Creative Commons Attribution License (CC BY). The use, distribution or reproduction in other forums is permitted, provided the original author(s) or licensor are credited and that the original publication in this journal is cited, in accordance with accepted academic practice. No use, distribution or reproduction is permitted which does not comply with these terms.
*Correspondence: Michael E. Yeager, 12700 E. 19th Avenue, Box C218, Aurora, CO 80045, USA,bWljaGFlbC55ZWFnZXJAdWNkZW52ZXIuZWR1