- Department of Pharmacy Practice and Science, College of Pharmacy, University of Kentucky, Lexington, KY, United States
An iron-containing milk protein named lactoferrin (Lf) has demonstrated antiparasitic and immunomodulatory properties against a variety of human parasites. This protein has shown its capability to bind and transport iron molecules in the vicinity of the host–pathogen environment. The ability of parasites to sequester the iron molecule and to increase their pathogenicity and survival depends on the availability of iron sources. Lf protein has suggested a iron chelating effect on parasites iron and, hence, has shown its antiparasitic effect. Since the parasites have a complex life cycle and have developed drug resistance, vaccines and other treatments are a handful. Therefore, therapeutic research focusing on natural treatment regimens that target the parasite and are non-toxic to host cells is urgently needed. The antiparasitic efficacy of Lf protein has been extensively studied over the past 40 years using both in vitro and in vivo studies. This review article highlighted past important studies on Lf protein that revealed its potential antiparasitic activity against various intracellular and extracellular intestinal or blood-borne human parasites. This review article structures the role of Lf protein in its various forms, such as native, peptide, and nanoformulation, laying the groundwork for its function as an antiparasitic agent and its possible known mechanisms of action.
1 Introduction
Parasites are responsible for many human diseases worldwide. One of the most life-threatening parasitic infections is malaria, which was responsible for 247 million cases and more than 600,000 deaths in the year 2022 (WHO, 2022). Some other blood-borne parasitic infections, such as trypanosomiasis, leishmaniasis, toxoplasmosis, and filariasis, are also responsible for millions of cases each year and have a high disease burden (Drame et al., 2016; Theel and Pritt, 2016). Intestinal infections such as giardiasis, amoebiasis, cryptosporidiosis, and helminthic infection are more common in tropical and non-tropical regions (Suntaravitun and Dokmaikaw, 2018; Chen et al., 2022; Nath et al., 2022). The incidence rate is high in low income countries and areas of poor sanitation (Kamau et al., 2012; Hajare et al., 2021). Parasites cause zoonotic infections that transmit between vertebrates and invertebrate hosts and involve multiple life cycle stages. Interruption in transmission in different stages and hosts may lead to their mortality (Auld and Tinsley, 2015). Multiple life cycle stages in different hosts leads to treatment failure and drug resistance, which has been known in many parasites. Plasmodium has shown drug resistance to conventional drugs such as chloroquine, mefloquine, and artemisinin (Shibeshi et al., 2020). Artemisinin combination therapy (ACT) has also shown emerging resistance since 2008 in South Asian countries, which continues to spread (Kagoro et al., 2022). Leishmania parasite has also shown drug resistance towards pentavalent antimonial and pentamidines (Maltezou, 2010). Amphotericin B has been the second line of treatment against leishmaniasis and has shown effective results, but because of its numerous off-target toxicities, its usage is limited (Laniado-Laborín and Cabrales-Vargas, 2009). There are multiple drugs or secondary metabolites used to target human and animal protozoan and helminthic parasites to control the spread of disease (Velázquez-Antunez et al., 2023). Many studies have suggested the role of plant-based therapy against blood-borne parasites, which are under development and further require in vivo studies (Kaur et al., 2021; Qureshi, 2021; Kamaraj et al., 2022; Benlarbi et al., 2023; Wulandari et al., 2023). Apart from drug treatment strategies, various preventive measures are required to control the spread of these zoonotic infections worldwide (Molyneux, 2006; Ghodsi et al., 2019; Luan et al., 2023).
Iron is one of the most important nutrient elements that help humans and other organisms grow. It is also the most important element responsible for host–pathogen interaction as it involves various cellular and metabolic processes. Previous studies have found an association between parasitic infections and low iron anemia in children (Osazuwa et al., 2011; Arroyo et al., 2015; Gujo and Kare, 2021; Kaur and Juneja, 2022). Various parasites sequester the iron present at the cellular level through proteins such as transferrin (Tf), lactoferrin (Lf), hemoglobin (Hb), and other iron pools (Collins, 2003). These proteins are the main source of Fe for intracellular pathogens in macrophages and red blood cells (RBCs), and for extracellular pathogens, epithelial cells act as an iron source. Upon pathogenic attack, Fe is released in the form of degraded cell components or degradation of RBCs, and parasites acquire this iron for their growth and multiplication. Availability of this iron may be diminished if it can be sequestered and if it is not available for pathogens for their multiplication, pathogenesis, or growth (Skaar, 2010).
Lf is a multifunctional glycoprotein, belonging to the family of Tf, which helps in the binding and transferring of iron (Fe3+) molecules and acts as an iron sequester. It was first isolated in 1939 and identified as a red protein from bovine milk (Sorensen, 1939). The three-dimensional structure of Lf was identified in 1987, the first from its family to be identified (Anderson et al., 1987). These proteins are found in mammals and are involved in providing defense against various microbial infections and also act as an essential growth factor (Naot et al., 2005; Siqueiros-Cendón et al., 2014). Lf is predominantly present in mammary glands, in secretions of exocrine glands, and in specific granules of neutrophils, reaching a concentration as high as 8 mg/mL. The major source of Lf present in the blood is derived from neutrophil degranulation (Lepanto et al., 2019). All of these proteins possess a common structural pattern, consisting of a single polypeptide chain of approximately 700 amino acid residues with two homologs, N- and C-terminal lobes of a similar length (Baker and Baker, 2009). These two lobes and four domain structures provide the basic functional understanding of the protein. Each lobe binds reversibly to two iron (Fe3+) and together with carbonate ions (CO23−) (Legrand et al., 2008).
There are three different structural forms of Lf according to its iron saturation: apo Lf (no iron), mono Lf (one iron), and holo Lf (two iron), which provide its three different dimensional structures (Baker and Baker, 2009). Besides Fe3+, Lf protein is also capable of binding to lipopolysaccharides (LPS), heparin, DNA, and metal ions such as Al3+, Mn3+, Co3+, Cu2+, and Zn2+, which express its antimicrobial ability with diverse modes of action (Honarparvar et al., 2019; Rogowska et al., 2023). Holo Lf gives it the most packed and stable structure, with two Fe3+ that synergistically bind two CO23− ions (Baker and Baker, 2009). This form of Lf allows unrestricted access for binding with the neighboring iron molecule and various other metal ions. The apo Lf is much less stable and less compact and is therefore prone to heat denaturation and proteolysis (Baker and Baker, 2004). The release of iron from holo Lf results in its destabilization, which could be triggered by lowering the pH to 4. Therefore, depending on the presence of one or two iron molecules or its absence, Lf protein can donate/deprive or sequester the iron molecule and hence can perform antitumor, antiparasitic, antibacterial, antifungal, or antiviral activities, along with immunomodulation (Hao et al., 2019; Ahmed et al., 2021). There are many review articles that have discussed the antimicrobial activity of Lf protein, but there are a handful of publications that have suggested Lf protein as an antiparasitic agent in its different formulation. This review article highlights the importance of this novel drug molecule that can be used in its various derivations, like its peptide form, and can be incorporated in nanoformulations to target parasitic infections.
2 Effect of Lf protein on intestinal parasites
This section discusses important studies of human intestinal parasites such as Entamoeba, Giardia, and Cryptosporidium, which are known to be vulnerable to the treatment of Lf protein and its peptides. Different forms of Lf studied against intestinal parasites with various sources of origin, target stages, and concentrations are summarized in Table 1.
2.1 Endocytosis of holo Lf protecting Entamoeba histolytica trophozoite and apo Lf depleting its nutrients
Entamoeba histolytica (E. histolytica) is a protozoan parasite and the causative agent of amoebiasis in humans causing 500 million cases and approximately 100,000 deaths annually. The disease is transmitted through parasitic cysts that are ingested through contaminated food and water, thus increasing the incidence of this disease in areas with poor sanitation (Nasrallah et al., 2022). Amoeba requires iron for its growth and obtain it by engulfing bacteria and RBCs that help increase its iron source through its endocytic pathway. A previous study has suggested the possible interaction between the amoebic parasite with bovine Lf (bLf) and human Lf (hLf). It was theorized that parasites takes up Lf iron through membrane internalization and tubular invaginations (de JO Batista et al., 2000; Leon-Sicairos et al., 2005; López-Soto et al., 2009). Further potential evidence suggested the amoebicidal activity of the apo form of Lf present in human and bovine milk and tested at the trophozite stage. Effect was seen through iron sequestration via receptor-mediated binding and depriving its essential nutrient source (Leon-Sicairos et al., 2006a). This anti-amoebic activity of Lf was further supported by an in vivo study where infected C3H/HeJ mice were orally administered with bLf and post-treatment showed a significantly increased Th1 type of immune response and reduced swelling in the cecum with no histopathological damage to the intestinal tissue (León-Sicairos et al., 2012). These findings were further confirmed using the hamster model of amoebic liver abscess, and upon treatment, liver abscess was found to heal completely and showed a high blood cell count and normal liver function (Ordaz-Pichardo et al., 2012).
2.2 Anti-amoebic and immunomodulatory effects of Lf peptides
Different antimicrobial peptides (AMPs) derived from hLf and bLf have been used against intestinal parasites. Lactoferricin B (Lfcin B) obtained from calf rennet hydrolysate Lf (4–14) and Lfcin (17–30) obtained after cleavage with pepsin showed inhibition towards the trophozoite stage of E. histolytica. Lfcin showed a synergistic effect with metronidazole against the amoebic parasite when observed in a time- and dose-dependent manner (Leon-Sicairos et al., 2006b) (Table 1). Other potent peptides, namely, Lfampin (265–284) and Lfchimera, which is composed of Lfcin and Lfamphin with a lysin link, were also found to be amoebicidal in nature and showed the highest parasiticidal activity among the tested compounds (López-Soto et al., 2010). A previous study has demonstrated the significant effect of Lfamphin peptide on E. histolytica and has demonstrated its receptor-mediated internalization in the trophozoite, through the P13K signaling pathway (Díaz-Godínez et al., 2019). The internalization of peptide induced changes in the actin cytoskeleton and its remodeling resulted in necrosis the parasite. The anti-amoebic effect of this peptide was later revealed in C3H/HeJ mice, which showed a complete absence of parasite infection in cecum tissue. The authors postulate the immunomodulatory effect of the Lfamphin peptide on mice, which helped their prolonged survival (Díaz-Godínez et al., 2019). These studies suggested the use of a small peptide sequence of Lf protein to act specifically in binding and its functional aspects over the native form of Lf.
2.3 Antigiardial activity of apo Lf protein against Giardia
Giardia is an intestinal parasite known to cause acute and chronic diarrhea, especially in children, and causes symptomatic infection in almost 280 million people annually, out of which 200 million are from Asia, Africa, and Latin America (Feng and Xiao, 2011). The most effective drugs against giardiasis are metronidazole, ornidazole, and tinidazole, showing a recovery rate of up to 90% (Escobedo and Cimerman, 2007; Vivancos et al., 2018). Giardia infection is more prone in iron-deficient children and induces iron deficiency anemia (IDA) and malabsorption (Gardner and Hill, 2001; Hussein et al., 2016). Because of the immunomodulatory activity of human breast milk and the presence of Lf protein, its efficacy was tested against Giardia trophozoites, which showed its potent antigiardial property and mitigating effect in children. These findings for the first time highlighted the potential role of human milk and presence of apo Lf causing the iron chelating effect on the parasite trophozoite (Breakey et al., 2015).
2.4 Antigiardial activity of Lf peptides on the trophozoite stage
Peptides derived from the N terminal of hLf (18 to 40) and bLf (17 to 41) peptides are the most potent AMPs that have demonstrated antimicrobial effects (Bellamy et al., 1992; Tomita et al., 1994). The N-terminal peptide derived from bLf has previously shown antigiardial activity when used at significantly low concentrations as compared with native form (Table 1). This peptide showed direct interaction with the trophozoite of the parasite, resulting in its killing effect (Turchany et al., 1995). Previous studies described the Lfchimera peptide as superior to the standard metronidazole drug when used in a dose- and time-dependent manner (Aguilar-Diaz et al., 2017). Treatment with Lfchimera led to morphological changes in the trophozoite such as flagellar disruption and distorted peripheral vacuoles, leading to apoptosis or programmed cell death (Aguilar-Diaz et al., 2017). Detailed ultra-structural examination on the Giardia trophozoite illustrated the direct interaction of native Lf and its peptide on the surface of the trophozoites, leading to deformation via flagellar swelling, disruption in plasma membrane, and ultimately, lysis of the cell (Turchany et al., 1997). Another study further illustrated the detailed mechanism of action of bLfcin against G. lamblia. Giardia trophozoites showed internalization of low-density lipoprotein (LDL) and chylomicrons through receptor-mediated engulfment (RME), and a similar pathway has been suggested by the authors for the engulfment of bLf and bLfcin. The authors suggested that this mechanism is essential for morphometric changes and lysis of the parasite, which was not known before (Frontera et al., 2018).
2.5 Antiparasitic effect of Lf and its peptides against Cryptosporidium
Cryptosporidium parvum (Ferrer et al., 2015; Thipubon et al., 2015) is responsible for the disease cryptosporidiosis, which causes intestinal infection in humans and animals, with an incidence rate of 1%–3% in Europe and North America and can also infect extra-intestinal organs in the case of an immunocompromised host (Hunter and Nichols, 2002; Semenza and Nichols, 2007; Ghallab et al., 2022), whereas C. hominis is only known to infect humans, but due to the lack of laboratory models to propagate C. hominis culture, most of the in vitro and in vivo studies have been performed with C. parvum (Gerace et al., 2019). Antibiotics such as nitazoxanide are used as the first line of treatment against C. parvum, but the results are not satisfactory and the mode of action of the drug is also not fully understood (Rossignol et al., 2006). Lf protein and its peptides, namely, Lfcin B and Lf hydrolysate (LfH), have previously demonstrated antiparasitic activity against C. parvum sporozoites with the highest inhibition rate achieved by LfH. This peptide also showed antiparasitic activity when used in combination with Cryptosporidium monoclonal antibody (3E2 MAb). The 3E2 MAb has been raised against the apical complex and surface glycoprotein ligand (CSL) of the parasite, which helps the parasite attach to the host cell. The combination of Mab and the Lf peptide helped inhibit sporozoite viability and infectivity (Carryn et al., 2012). Previous studies also examined the role of hLf on the excystation efficacy of Cryptosporidium. The authors showed possible inhibition of sporozoite viability and infectivity, but no effect was found on the excystation stage of the oocyst wall. These studies suggested that Lf protein or its peptides are impermeable to the thick wall of the oocyst but can inhibit the viability of free forms of this parasite through internalization via the endocytic pathway (Paredes et al., 2017).
3 Effect of Lf protein on intracellular blood parasites
Intracellular blood parasites such as Leishmania, Plasmodium, Toxoplasma, Trypanosoma, and Babesia, which are responsible for blood-borne infections, have been reported to be susceptible to the treatment of Lf protein. The apo/mono form of the Lf has been documented to show significant antiparasitic activity against these parasites by chelating the iron molecule with the help of the Lf receptor present on the parasite membrane (Figure 1), whereas holo Lf has shown support for their multiplication through internalization via endocytosis and further multiplication. Also holo Lf may lead to increased oxidative stress and kill the parasite. Various peptides and nanoformulations of Lf, along with its native form, have been used in studies and are summarized in Table 2.
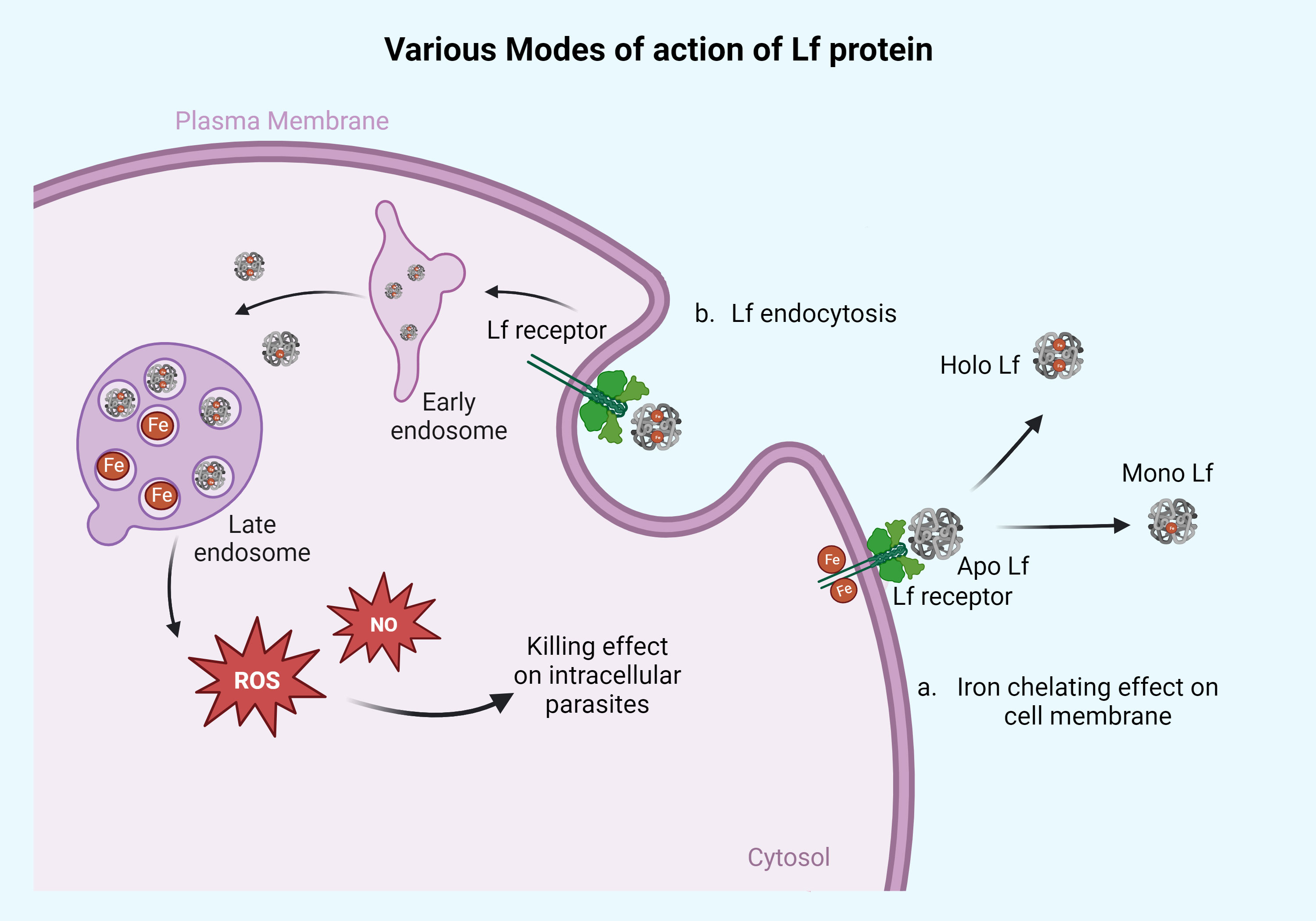
Figure 1 Action of Lf protein on the surface membrane of the parasites and intracellularly. Lf protein mainly acts on the parasites in two different ways. (a) Iron-chelating effect on cell membrane: the apo form of Lf chelates the Fe molecule present on the parasite surface membrane and converts it into the mono or holo Lf form. This way, apo Lf deprives the parasite of its iron source. This mechanism of action can be seen in parasites that reside/adhere extracellularly on the cell membrane of the host like Giardia, Trichomonas, and Entamoeba. The other part of the mechanism is based on Lf engulfment or through endocytosis, as seen in panel (b). The Lf gets endocytosed and diffused with early and late endosomes, releasing the iron molecule into the cytoplasm, which causes free radical ion production in the form of ROS and NO leading to cell death of parasites. This high ROS or NO killing effect can be seen in parasites like Leishmania, Plasmodium, Toxoplasma, Babesia, and Trypanosoma. Retrieved from https://app.biorender.com/biorender-templates, December 2023.
3.1 Role of Lf against Leishmania
Leishmaniasis is a disease that is caused by the protozoan parasite Leishmania and it affects approximately 13 million people annually (Kevric et al., 2015). The parasite alters between promastigote (insect host) and amastigote stages (mammalian host). There are three different forms of leishmaniasis: visceral, cutaneous, and mucocutaneous. Visceral leishmaniasis is caused by L. donovani, L. chagasi, and L. infantum. Cutaneous leishmaniasis is caused by L. brazilensis and L. (L.) amazonensis, and mucocutaneous leishmaniasis is caused by L. guyanensis and L. panamensis (Palumbo, 2010; Reimão et al., 2020). The treatment for the disease is pentavalent antimonial compounds, but due to the emerging resistance, these drugs are not effective (Sundar et al., 2000; Maertens et al., 2022). However, the most recent and effective therapy against leishmaniasis is amphotericin B and its liposomal nanoformulations, but due to the high cost of the drug, and toxicity, its usage is limited (Saravolatz et al., 2006; Maertens et al., 2022). The antileishmanial activity of Lf has been rarely studied; however, some reports have manifested the acquisition of radiolabeled iron by the promastigote form of L. chagasi through in vitro studies describing the role of a common receptor or convergent pathway. The promastigote form of the parasite has been known to acquire iron from various sources, which include Lf, Tf, and hemin. This study describes the uptake of the reduced form of iron (Fe2+) instead of the oxidized form (Fe3+) derived from Lf protein by the promastigotes (Wilson et al., 1994). These results suggested that the promastigote form of the parasite may have a different pathway of internalization, and based on that, the parasite may show different aspects of pathogenicity and survival.
3.2 Potent activity of Lf peptides against Leishmania
Potent Lf AMPs, namely, Lfchimera, Lfcin, and Lfamphin, were found to be leishmanicidal in nature when evaluated through an in vitro study (Table 2) (Silva et al., 2012). These peptides showed internalization and accumulation inside the cell, leading to impaired ATP production, and loss of ionic gradient, resulting in parasite death. The activity of Lfchimera has largely surpassed the other two derivatives as it appears to attach the membrane of the parasite leading to its destruction. The role of either the Lf native protein form or its various derivatives has suggested the destruction of the parasite through imbalance in the gradient ion channel, which might have changed the electrolyte balance and osmoregulation (Silva et al., 2012).
3.3 Lactoferrin nanotherapy used against Leishmania
There are several natural biological molecules that are used as a loading drug in nanotherapy. Lf nanoformulation has been studied against a wide range of diseases such as cancer and parasitic infection (Kondapi, 2020; Varma et al., 2020). Previously, the antileishmanial activity of Lf-appended amphotericin B bearing nano reservoirs has been thoroughly addressed against this parasite using in vitro and in vivo studies, showing reduced parasite burden (Asthana et al., 2015). The nanoparticles were taken up more efficiently by the host macrophages and suggested their targeted delivery. This nanoformulation induced a high Th1 immune response in mice that contributed to reduced parasite burden in the visceral organs with low toxicity (Asthana et al., 2015). Another study showed the antileishmanial activity of the modified polylactic-co-glycolic acid (PLGA) nanoparticle (NP) incorporated with Lf protein. These nanoparticles were loaded with betulinic acid (BA), which is a naturally occurring triterpenoid and has shown promising antitumor and anti-inflammatory properties (Halder et al., 2018). The mechanism of action of BA has been found to be through the destruction of mitochondrial membrane potential and ROS production (Cichewicz and Kouzi, 2004). However, BA has poor solubility, but its loading with PLGA nanoformulation has been found to enhance its targeted delivery. Moreover, author found the incorporation of Lf protein helped in the receptor-mediated binding of these NPs and improved its further engulfment inside the cells. With these modifications, Lf-embedded BANP showed a significant reduction in intracellular amastigotes through high production of NO and enhanced Th1 response through elevated levels of IL-12 and reduced expression of IL-10 in host macrophages (Halder et al., 2018).
3.4 Antiparasitic activity of apo Lf against Plasmodium
Plasmodium is an intracellular protozoan parasite known to cause malaria, which leads to the mortality of 600,000 people annually (WHO, 2022). The most deadly form of the disease is called falciparum malaria, which is caused by, P. falciparum, responsible for almost 90% of malaria cases alone in Africa (Snow et al., 2005). The Plasmodium parasite completes its life cycle in two different hosts, an invertebrate host (mosquito) and a vertebrate host (human). The sporozoite stage of the parasite is transmitted through the mosquito bite to the human host and multiplies inside the liver cells. This sporozoite stage leads to the formation of merozoite, which transmits into the bloodstream and multiplies inside the RBCs. To avoid this transmission cycle, various transmission-blocking vaccines have also been under development (Buchholz et al., 2011; Nikolaeva et al., 2015). The current treatment strategy for malaria includes artemisinin therapy. Artemisinin and its derivates in combination with other drugs have also shown promising results against malaria (Abumsimir and Al-Qaisi, 2023). The parasite resides in the RBCs and feeds on the iron-containing Hb as it develops from the ring to the schizont stage and utilizes all the Hb iron as its nutrient (Egan et al., 2002). Therefore, iron chelators have been studied against P. falciparum as antimalarial agents for several decades (Thuma et al., 1998). Earliest reports have shown the effect of iron chelators like desferriferrithiocin (DFT), desferricrocin (DFC), and hLf on the in vitro growth of P. falciparum. The apo form of hLf showed growth arrest at the ring form of the parasite and inhibited its further conversion to the trophozoite stage, whereas no effect was observed after treatment with iron-saturated hLf (Fritsch et al., 1987). Pretreatment of Lf with the circumsporozoite (CS) protein of malarial sporozoites has shown interference in its attachment and invasion to liver cells, disrupting attachment with heparin sulfate (HS) and LDL receptor-related protein (LRP) (Shakibaei and Frevert, 1996; Sinnis et al., 1996). Lf has also shown anti-adherent properties. Lf-treated infected RBCs showed hindrance in their attachment to various receptors present on placenta and endothelial cells, namely, CD36 (Eda et al., 1999), intercellular cell adhesion molecule (ICAM-1), thrombospondin (TSP) (Roberts et al., 1985; Berendt et al., 1989), chondroitin sulfate A (CSA) (Robert et al., 1995; Fried and Duffy, 1996), and vascular cell adhesion molecule-1 (VCAM-1) (Ockenhouse et al., 1992).
Previous clinical studies have suggested the role of Lf protein as a major component of human milk in providing protection to the fetus against malaria. Breast milk has been found to be high in IgM and IgA, and the role of Lf in protecting the mother and the developing fetus through vertical transmission has been suggested (Kassim et al., 2000). Another study postulated that BCG immunization in mice led to increased concentration of Lf protein in the plasma and helped reduce P. yoelli infection in mice along with an increased number of CD4 and CD8 cells (Parra et al., 2013). These results suggest the immunomodulatory action of Lf protein. Previous studies have explored the role of Lf protein in reducing the invasion capacity of parasitized erythrocytes inside the new RBCs. Reduced invasion of RBCs leads to a significant reduction in parasite levels, by 71% and 95% at day 14 and 17 post-infection (poi) compared to the untreated group. This reduction in parasite levels was attributed to the presence of Lf as a major factor and was found to be independent of antibody production (Parra et al., 2013). All these studies have pointed out the role of Lf in inhibiting malarial parasites, but a detailed mode of action was not fully demonstrated.
The studies performed using Lf as an antimalarial agent did not fully illustrate its effect on the RBCs; therefore, it is of utmost importance to understand its cytotoxicity and mode of action against malarial parasites. A previous extensive study conducted by our group elaborated on the interaction of RBCs with various iron-saturated forms of bLf and buffalo Lf (buLf) (Lfs), such as the apo, mono, and holo form (Anand et al., 2015a). Different concentrations of these two forms of Lf and different iron saturation were examined against the human RBCs and effect on its shape and size. All different forms of Lfs were found to be nontoxic in nature and did not show any alteration in the size and shape of RBCs when given in a dose- and time-dependent manner. RBCs treated with holo Lfs showed high production of ROS as compared to apo/mono forms of the Lfs (Anand et al., 2015a). After analyzing the nontoxic nature of various Lfs toward RBCs, we intended to study its effect on intracellular parasites, and we chose to work with buLf protein in mouse models, which showed better effect over bLf.
3.5 Antimalarial activity of Lf in its native and nanoformulation form
Our group has studied the role of buLf protein in its native form and nanoformulation against P. berghei-infected mice. Previous studies from our group have suggested that Lf protein works best when it is being administered orally (Kanwar et al., 2012). However, Lf is a cationic protein and can degrade through the gastric pH and enzymatic activity when incorporated via the oral route of administration. Therefore, our group has coupled this protein with biodegradable polymers like alginate and chitosan to make a nontoxic bioactive drug and studied its activity against colon cancer and further against the P. berghei mouse model (Kanwar et al., 2012; Anand et al., 2016). A significant reduction in parasite load was observed when infected BALB/c mice were treated with the buLf mono form. However, enhanced antiparasitic activity was seen after giving its nanoformulation using alginate and chitosan nanocarriers (buLf NC) through oral administration (Anand et al., 2016). Mice treated with either native buLf or buLf NC did not show any pathophysiological alteration and had significantly less peripheral parasitemia and high ROS production in spleen and liver cells when compared to the untreated group. Biodistribution of buLf in the liver and spleen showed its entrapment in liver Kupffer cells and the red pulp of the spleen. Moreover, the treatment with either native buLf or buLF NC resulted in maintaining the iron levels and iron metabolism in mice when compared with control mice, which were found to be anemic. The expression for miRNAs of iron metabolism, such as miR-Let7d, miR122, miR-196 miR-200b, miR-210, miR-214, miR320, miR-485 and miR-584, was found to be elevated in mice which showed balanced iron metabolism (Anand et al., 2016). Therefore, our study suggests the chelating effect of Lf protein and the targeted delivery of Lf protein inside the macrophages, which help prevent parasite multiplication and help maintain iron metabolism.
3.6 The promising antiparasitic activity of apo/mono Lf and its peptide against toxoplasmosis
Toxoplasma gondii is an obligate parasite known to cause toxoplasmosis in humans and immunocompromised patients. It is generally assumed that approximately 25% to 30% of the world’s human population is infected by Toxoplasma and approximately 500 million humans have antibodies to this parasite (Ajioka and Morrissette, 2009). T. gondii mice model has been well studied before and known to cause enchephalitis. Various reports have studied the chronic infection of T. gondii in mice previously which causes the formation of brain cyst. These mice mimic the ideal pathological conditions as with humans and shows cytokine response that can be picturalizsed in human in order to study the pathophysiology of the diseases (Lutshumba et al., 2020; Anand et al., 2022). Conventional drugs used for the treatment of toxoplasmosis are sulfadiazine and pyrimethamine, but resistance against sulfadiazine has been emerging for the last few years (Doliwa et al., 2013). The efficacy of Lf protein studied against Toxoplasma dates back to 1995, when bLf and its peptides, namely, the Lfcin B and C-terminal peptide, were used to assess their antitoxoplasmal activity. The parasite was incubated with the bLf and its various peptides and studied for its invasive tendency. Interestingly, the Lfcin B peptide significantly hindered the invasive capacity of tachyzoites to infect the cells when compared to untreated control parasites and the C-terminal peptide. The authors suggested that although the tachyzoites were alive after the treatment with Lfcin B, they possibly lost the capability to invade the cells (Tanaka et al., 1995). This effect was further studied in a mouse model, wherein all mice treated with Lfcin B survived until day 30 post-injection as compared to untreated mice, which died by day 9 post-infection (Tanaka et al., 1995). These results suggest that the Lf peptide diminishes the invasion capacity of tachyzoites in in vitro and in vivo models. The authors further investigated a number of studies to identify the mode of action of Lf protein. The authors intended to study the pretreatment effect of Lf on murine somatic host cells and revealed a reduction in the number of intracellular tachyzoites invading when seen in comparison to the untreated control group (Tanaka et al., 1996). Furthermore, the authors tried to subject intracellular tachyzoites to different iron-saturated forms of Lf, and it was observed that only apo/mono bLf forms showed the best inhibitory activity with increased free radical ion production (Tanaka et al., 1997). Similar results were obtained when the antiparasitic activity of bLf was observed in mice that were orally administered with the protein after infection with T. gondii. Treatment results suggest reduced parasite load through the production of intracellular ROS and NO (Tanaka et al., 1997). These studies suggested iron chelation and ROS production as potential mechanisms of action of bLf. However, the authors discovered the possible role of tyrosine kinase phosphorylation inside the cells to be the mode of action of Lf effect, along with ROS production (Tanaka et al., 1998).
The effect of the holo form of hLf was observed against intracellular tachyzoites and monitored; it did show inhibition when used in a dose- and time-dependent manner, but no remarkable effect was found on the extracellular stages of the parasite (Dzitko et al., 2007). This study correlates with the finding that the iron-saturated form of Lf protein can induce ROS production and kill the parasite, whereas the apo/mono form can inhibit the extracellular stages. Another study has documented the role of parasite rhoptry proteins, namely, ROP2 and ROP4, as important genes responsible for the invasion and pathogenesis of the parasite (Carruthers, 1999). However, these proteins have been reported to acquire iron molecules from hLf, helping the attachment of tachyzoites with cell surface-promoting parasite multiplication and enhanced pathogenicity (Dzitko et al., 2007).
To detail the interaction between the parasite and the macrophage host cells, our group has performed a multiparametric study to examine the same (Anand et al., 2015a). Our study demonstrated the effect of various iron saturations of bLf and buLf on morphology, cytotoxicity, the production of free radical ions, and the phagocytic property of macrophages using the THP1 cell line. After the treatment of the holo form of Lf, the macrophages showed increased ROS and phagocytic properties with no cytotoxicity and morphometric alterations (Anand et al., 2015a). We studied a similar effect on macrophages that were infected with tachyzoites of the parasite. Infected macrophages were incubated with different concentrations of apo/mono Lf and the treatment showed reduced number of infected macrophages when compared with holo Lf. However, when the intracellular parasites were counted per macrophage, again, the apo/mono form of the bLf showed both a significantly smaller number of infected macrophages and a smaller number of intracellular tachyzoites per macrophage (Anand et al., 2015b).
3.7 Lactoferrin nanotherapy used against Toxoplasma
In our previous study we investigated the role of bLf on acute stage of the mice. The in vivo activity of mono bLf and its nanoformulation using alginate chitosan bLf NC were analyzed by our group (Anand et al., 2015b). The bLf NC showed better antiparasitic activity in comparison to mono bLf protein in reducing the parasite load and provided a high Th1 immune response, resulting in the prolonged survival of mice. We also found cyst development inside the liver tissue of the mouse which were treated with bLf and bLf NC. These results showed the treatment effect of Lf protein on the parasite which lead it to the cyst formation. Our study proposed that bLf NC treatment induces increased ROS production in the liver and spleen, followed by high NO production. With the help of immunohistochemistry, we observed immunoreactivity of Lf in the spleen and liver, suggesting the targeted delivery of Lf in respective visceral organs with very low toxicity. Also the biodistribution of Lf protein was found in the liver of the mice. The proposed mechanism of action from our study suggested that the increased production of ROS and NO caused intracellular parasite killing. Additionally, Lf caused potential disruption of iron availability for parasite survival and maintained iron metabolism, leading to improved protection against parasitic infections in mouse models (Anand et al., 2015b).
3.8 Antiparasitic activity of Lf against Trypanosoma
Trypanosoma is a blood-borne parasite that causes sleeping sickness, also known as African trypanosomiasis or Chagas disease in humans. The incidence rate of the disease may be as high as 90% in endemic areas, especially in Africa (Büscher et al., 2017). Conventional drugs such as suramin, pentamidine, and melarsoprol are the treatment of choice (Garcia-Salcedo et al., 2016). The parasite multiplies in the reticuloendothelial system (RES) of humans as well as in muscle cells. It relies on the source of iron for its multiplication and for its conversion from one form to another (Stijlemans et al., 2015, 2018). Previous studies have suggested the correlation of iron content in a patient’s serum with the severity of trypanosomiasis (Murray et al., 1978). Many studies have investigated the potential of Lf against Trypanosoma parasite due to its structural similarity with Leishmania parasite. An earlier study examined the effect of Lf protein on intracellular amastigotes’ growth using different host cells, namely, mouse peritoneal macrophages (MPMs) and human blood monocytes (HBMs) (Lima and Kierszenbaum, 1987). The inhibitory effect of Lf was found to be more pronounced in MPM cells than in HBM cells. The rationale for the difference in inhibitory activity was correlated with the higher number of Lf receptors present on the surface of MPM cells, which may facilitate the entry of Lf inside the cell and show an inhibition effect (Lima and Kierszenbaum, 1987). Another study showed that Lf can serve as a cell surface marker on the amastigote form of T. cruzi, resulting in opsonization, to inhibit the parasite load in host cells such as monocytes and macrophages (Lima et al., 1988). A study conducted by Tanaka et al. suggested that bLf binds to a particular surface protein of 40 kDa, identified as Glyceraldehyde 3 phosphate (G3Ph) through receptor ligand binding and suggested a possible correlation with parasite inhibition (Tanaka et al., 2004).
3.9 Antiparasitic effect of native apo Lf protein against Babesia cabelii
Babesiosis is a disease caused by the protozoon blood-borne parasite Babesia. It has similar morphology and pathogenicity to that of the Plasmodium genus; however, the two parasites differ in their mortality rate (Allred and Al-Khedery, 2004; Pritt, 2015). Plasmodium is responsible for almost 600,000 deaths annually, whereas Babesia has a low incidence rate, which has been reported only in North America, Japan, Korea, Taiwan, and India (Vannier and Krause, 2012; Onyiche et al., 2021). In North America alone, a total of 50,856 cases were found in 2019 (Swanson et al., 2023). Like Plasmodium, Babesia also feeds on Hb of the RBCs and hunts for iron; few studies have investigated the role of Lf against this parasite. A previous study suggested the effect of different iron-saturated forms of bLf such as apo, holo, and mono Lf, and the peptide LfH against two species of Babesia such as B. cabelli and B. equi, but only the apo form had shown inhibition toward B. cabelli and not against B. equi (Ikadai et al., 2005). The mode of action of Lf against Babesia was found to be similar to that of Plasmodium, i.e., through iron chelation, but still, in vivo studies are required to justify the antiparasitic nature with a detailed mode of action.
The mechanism of action summarized from the above-mentioned studies on these parasites demonstrated the iron-chelating effect of apo Lf from the parasite through receptor-mediated binding, whereas the holo form of Lf has provided growth and helped enhance the pathogenesis of the parasite through surface internalization. However, in some intracellular parasites such as Plasmodium, Toxoplasma, and Leishmania, holo Lf may act through the production of free radical ions, reactive oxygen species (ROS), and nitric oxide (NO), which results in the intracellular killing of the parasite (Figure 1).
4 Effect of Lf protein on extracellular parasites
Lf protein isolated from bovine or human milk has also shown inhibition against different extracellular parasites, and the mechanism of action is fairly similar to that of intracellular parasites.
4.1 Role of Lf against Acanthamoeba
Acanthamoeba is a free-living parasite that can cause opportunistic infections in humans and has an estimated incidence rate of 1.2 per million adults. The parasite is known to invade host cells and acquire iron for its pathogenesis, causing keratitis and corneal infections (Page and Mathers, 2013; Niederkorn, 2021). Previous research has shown that A. castellanii proteases play a role in acquiring iron from holo hLf and holo Tf, which helps in its multiplication and pathogenesis (Ramírez-Rico et al., 2015), whereas the apo bLf has been reported to show amoebicidal effect against the trophozoite form of A. keratitis (Tomita et al., 2016). After the apo Lf treatment, the trophozoite shape was found to be globulus as compared to the normal non-globulus form of the trophozoites. However, the study did not report any notable effect on the cystic stage of the parasite, but the treatment with apo Lf prevented the conversion of trophozoites to cysts. This could result from the inability of Lf to penetrate the cell wall of the parasite (Alsam et al., 2008). A recent study by Ramírez-Rico et al., (2023) conducted similar experiments using apo-bLf protein on A. castellanii, which causes granulomatous amoebic encephalitis and keratitis in humans. The authors did not see any amoebicidal effect on the parasite trophozoite form and showed resistance in a dose- and time-dependent manner. The cytopathic effect was observed on A. castellanii when preincubated with apo-bLf. The cytopathic effect was found to be diminished after the apo-bLf treatment, whereas its absence caused damage to the parasite. Authors suggested the role of cysteine and serine proteases in the pathogenesis of the amoeba, which showed a cytopathic effect (Ramírez-Rico et al., 2023).
4.2 Role of Lf against Trichomonas
Trichomonas vaginalis is a parasite that causes sexually transmitted disease (STD) in men and women, affecting 275 million cases annually, but most of them remain asymptomatic (Johnston and Mabey, 2008; Kissinger et al., 2022). Parasites reside in flagellated trophozoites inside the female genital tract and replicate by binary fission (Yadav, 2023). Metronidazole and tinidazole are the drugs of choice for the treatment of T. vaginalis infections (Rigo et al., 2022). Several studies have identified iron as an essential micronutrient for the virulence and pathogenicity of Trichomonas (Song, 2016; Rivera-Rivas et al., 2020). The first interactive study of Trichomonas with Lf described how the parasite takes up the iron from the iron pool of the plasma membrane of the cells. The parasite sequesters the iron from hLf through receptor-mediated binding and its accumulation inside the trophozoite resulted in its increased pathogenesis. The enzymatic activity of pyruvate/ferredoxin oxidoreductase was suggested to be responsible for the binding of hLf to the parasite as it helps with iron acquisition (Peterson and Alderete, 1984).
Another previous study has suggested that the synergistic effect of complement C3 with Lf protein causes cell lysis of T. vaginalis. This study demonstrated that the presence of iron in the parasite cultivation media sourced from either Lf or Tf showed an inhibitory effect. However, in the absence of an iron source, the antiparasitic effect predominantly came from complement-mediated lysis (Alderete et al., 1995). Trichomonas is known to secrete a number of cysteine proteases, collectively called cysteine proteases 30 (CP30), which have shown an apoptotic effect on the epithelial cells of the host (Kummer et al., 2008). The authors reported that the presence of iron sourced from holo Lf in cell culture media leads to the reduced activity of CP30 and the survival of the parasite. However, in the absence of an iron source, the CP30 enzyme performed the proteolytic function and damages the epithelial cells (Kummer et al., 2008). Thus, these studies signify the role of Lf in promoting the growth of Trichomonas by stimulating different signaling pathways, resulting in its pathogenesis.
5 Discussion
Lf research has progressed over several decades, expanding its utility in various health-related areas. It is mentioned as a growth supplement in infant formulas and for its role in iron metabolism. Lf protein has shown its efficacy not only by chelating iron but also through its binding efficacy to the DNA molecule. Lf protein has a DNA binding site, which makes this protein more crucial in targeting or activating different transcription factors responsible for various cellular or biochemical activities (Fleet, 1995). It has the ability to provide adaptive and innate immunity to fight various diseases. Lf is extracted from bovine, sheep, or goat milk. Over decades, there have been improvements in the production of Lf. This protein is able to express in many microbes such as Escherichia coli and some fungi such as Aspergillus oryzae and Pichia pastoris, and its production has been improved through recombinant technology. Studies have also used CHO cell lines for the expression of this protein, and other cell-free protein methods have also been successful in its production. There has been a significant improvement in the yield of Lf after producing a transgenic fetal calf that can express this protein and enhance the yield.
Overall, this review suggests that the Lf protein exerts its effect on human parasites through iron chelation and free radical ion production where other possible mechanisms of action may also play a role. Parasites gain their nutrition and enhance their virulence by withdrawing the iron molecule from host cells or its surrounding. Treatment with the low-iron form of the Lf protein, such as the apo or mono form, can chelate the surrounding iron and prevent the iron from being available to the parasite. More specific binding of Lf protein with the target protein site of the parasite can be achieved by Lf peptide formulation, which can show a more specific and targeted killing effect with minimum toxicity. Nanoformulation of Lf protein is another strategy for delivering this drug. Nanoformulation of the Lf drug has been suggested because it is easy to incorporate with a variety of particles and targeted drug delivery. This review not only covers the antiparasitic nature of Lf but also highlights the immunomodulatory effect of this drug. Lf research has advanced over several decades and has expanded this protein’s value and importance in various health-related areas as a growth booster and for iron metabolism. Many countries are considering using Lf protein in clinical trials as a growth and immune supplement. Therefore, this review emphasizes the need for ongoing research to better understand the role of Lf and its mechanism of action, especially in the context of emerging infectious diseases. In-depth studies are thus required to provide possible insights into how Lf can be optimally utilized for parasitic infections.
Author contributions
NA: Conceptualization, Writing – original draft, Writing – review & editing.
Funding
The author(s) declare that no financial support was received for the research, authorship, and/or publication of this article.
Conflict of interest
The author declares that the research was conducted in the absence of any commercial or financial relationships that could be construed as a potential conflict of interest.
Publisher’s note
All claims expressed in this article are solely those of the authors and do not necessarily represent those of their affiliated organizations, or those of the publisher, the editors and the reviewers. Any product that may be evaluated in this article, or claim that may be made by its manufacturer, is not guaranteed or endorsed by the publisher.
References
Abumsimir B., Al-Qaisi T. S. (2023). The next generation of malaria treatments: the great expectations. Future Science. 9, FSO834. doi: 10.2144/fsoa-2023-0018
Aguilar-Diaz H., Canizalez-Roman A., Tomas N.-M., Francisco G.-V., Yolanda H.-O., Kamran N., et al. (2017). Parasiticidal effect of synthetic bovine Lactoferrin peptides on the enteric parasite Giardia intestinalis. Biochem. Cell Biol. 95, 82–90. doi: 10.1139/bcb-2016-0079
Ahmed K. A., Saikat A. S. M., Moni A., Kakon S. A. M., Islam M. R., Uddin M. J. (2021). Lactoferrin: potential functions, pharmacological insights, and therapeutic promises. J. Adv. Biotechnol. Exp. Ther. 4, 223. doi: 10.5455/jabet.2021.d123
Ajioka J. W., Morrissette N. S. (2009). A century of Toxoplasma research. Int. J. Parasitol. 39, 859–860. doi: 10.1016/j.ijpara.2009.02.006
Alderete J. F., Provenzano D., Lehker M. W. (1995). Iron mediates Trichomonas vaginalis resistance to complement lysis. Microb. Pathog. 19, 93–103. doi: 10.1006/mpat.1995.0049
Allred D. R., Al-Khedery B. (2004). Antigenic variation and cytoadhesion in Babesia bovis and Plasmodium falciparum: different logics achieve the same goal. Mol. Biochem. Parasitol. 134, 27–35. doi: 10.1016/j.molbiopara.2003.09.012
Alsam S., Jeong S. R., Dudley R., Khan N. A. (2008). Role of human tear fluid in Acanthamoeba interactions with the human corneal epithelial cells. Int. J. Med. Microbiol. 298, 329–336. doi: 10.1016/j.ijmm.2007.05.010
Anand N., Sehgal R., Kanwar K. R., Dubey M. L., Vashishta R. K., Kanwar J. K. (2015a). Effect of Lactoferrin protein on RBCs and Macrophages: mechanism of parasite host interaction. Drug Design Dev. Ther. 9, 3821–3835.
Anand N., Sehgal R., Kanwar R. K., Dubey M. L., Vasishta R. K., Kanwar J. R. (2015b). Oral administration of encapsulated bovine lactoferrin protein nanocapsules against intracellular parasite Toxoplasma gondii. Int. J. nanomedicine 10, 6355. doi: doi..10.2147/IJN.S85286
Anand N., Kanwar R. K., Sehgal R., Kanwar J. R. (2016). Antiparasitic and immunomodulatory potential of oral nanocapsules encapsulated lactoferrin protein against Plasmodium berghei. Nanomedicine 11, 47–62. doi: 10.2217/nnm.15.181
Anand N., Lutshumba J., Whitlow M., Abdelaziz M. H., Mani R., Suzuki Y. (2022). Deficiency in indoleamine-2, 3-dioxygenase induces upregulation of guanylate binding protein 1 and inducible nitric oxide synthase expression in the brain during cerebral infection with Toxoplasma gondii in genetically resistant BALB/c mice but not in genetically susceptible C57BL/6 mice. Microbes Infect. 24 (3), 104908.
Anderson B. F., Baker H. M., Dodson E. J., Norris G. E., Rumball S. V., Waters J. M., et al. (1987). Structure of human lactoferrin at 3.2-A resolution. Proc. Natl. Acad. Sci. U.S.A. 84, 1769–1773. doi: 10.1073/pnas.84.7.1769
Arroyo R., Ochoa T., Tai J.-H., de la Garza M. (2015). Iron and parasites (Hindawi). Biomed. Res. Int. 2015, 291672. doi: 10.1155/2015/291672
Asthana S., Gupta P. K., Jaiswal A. K., Dube A., Chourasia M. K. (2015). Targeted chemotherapy of visceral leishmaniasis by lactoferrin-appended amphotericin B-loaded nanoreservoir: in vitro and in vivo studies. Nanomedicine 10, 1093–1109. doi: 10.2217/nnm.14.182
Auld S. K., Tinsley M. (2015). The evolutionary ecology of complex lifecycle parasites: linking phenomena with mechanisms. Heredity 114, 125–132. doi: 10.1038/hdy.2014.84
Baker H. M., Baker E. N. (2004). Lactoferrin and iron: structural and dynamic aspects of binding and release. Biometals 17, 209–216. doi: 10.1023/B:BIOM.0000027694.40260.70
Baker E. N., Baker H. M. (2009). A structural framework for understanding the multifunctional character of lactoferrin. Biochimie 91, 3–10. doi: 10.1016/j.biochi.2008.05.006
Bellamy W., Takase M., Wakabayashi H., Kawase K., Tomita M. (1992). Antibacterial spectrum of lactoferricin B, a potent bactericidal peptide derived from the N-terminal region of bovine lactoferrin. J. Appl. Bacteriology 73, 472–479. doi: 10.1111/j.1365-2672.1992.tb05007.x
Benlarbi F., Mimoune N., Chaachouay N., Souttou K., Saidi R., Mokhtar M. R., et al. (2023). Ethnobotanical survey of the traditional antiparasitic use of medicinal plants in humans and animals in Laghouat (Southern Algeria). Veterinary World 16, 357. doi: 10.14202/vetworld.2023.357-368
Berendt A., Simmons D., Tansey J., Newbold C. I., Marsh K. (1989). Intercellular adhesion molecule-1 is an endothelial cell adhesion receptor for Plasmodium falciparum. Nature 341, 57. doi: 10.1038/341057a0
Breakey A. A., Hinde K., Valeggia C. R., Sinofsky A., Ellison P. T. (2015). Medicine, public health illness in breastfeeding infants relates to concentration of lactoferrin and secretory immunoglobulin a in mother’s milk issue 1-21-23. J. Evol. doi: 10.1093/emph/eov002
Buchholz K., Burke T. A., Williamson K. C., Wiegand R. C., Wirth D. F., Marti M. (2011). A high-throughput screen targeting malaria transmission stages opens new avenues for drug development. J. Infect. Dis. 203, 1445–1453. doi: 10.1093/infdis/jir037
Büscher P., Cecchi G., Jamonneau V., Priotto G. (2017). Human african trypanosomiasis. Lancet 390, 2397–2409. doi: 10.1016/S0140-6736(17)31510-6
Carruthers V. B. (1999). Armed and dangerous: Toxoplasma gondii uses an arsenal of secretory proteins to infect host cells. Parasitol. Int. 48, 1–10. doi: 10.1016/s1383-5769(98)00042-7
Carryn S., Schaefer D. A., Imboden M., Homan E. J., Bremel R. D., Riggs M. W. (2012). Phospholipases and cationic peptides inhibit Cryptosporidium parvum sporozoite infectivity by parasiticidal and non-parasiticidal mechanisms. J. Parasitol. 98 (1), 199–204. doi: 10.1645/GE-2822.1
Chen X., Saeed N. M., Ding J., Dong H., Kulyar M.F.-e., Bhutta Z. A., et al. (2022). Molecular Epidemiological Investigation of Cryptosporidium sp., Giardia duodenalis, Enterocytozoon bieneusi and Blastocystis sp. Infection in Free-ranged Yaks and Tibetan Pigs on the Plateau. Pakistan Veterinary J. 42. doi: 10.29261/pakvetj/2022.060
Cichewicz R. H., Kouzi S. A. (2004). Chemistry, biological activity, and chemotherapeutic potential of betulinic acid for the prevention and treatment of cancer and HIV infection. Medicinal Res. Rev. 24, 90–114. doi: 10.1002/med.10053
Collins H. L. (2003). The role of iron in infections with intracellular bacteria. Immunol. Lett. 85, 193–195. doi: 10.1016/S0165-2478(02)00229-8
de JO Batista E., de Menezes Feitosa L. F., de Souza W. (2000). The endocytic pathway in Entamoeba histolytica. Parasitol. Res. 86, 881–890. doi: 10.1007/s004360000269
Díaz-Godínez C., González-Galindo X., Meza-Menchaca T., Bobes R. J., de la Garza M., León-Sicairos N., et al. (2019). Synthetic bovine lactoferrin peptide Lfampin kills Entamoeba histolytica trophozoites by necrosis and resolves amoebic intracecal infection in mice. Bioscience Rep. 39, BSR20180850. doi: 10.1042/BSR20180850
Doliwa C., Escotte-Binet S., Aubert D., Sauvage V., Velard F., Schmid A., et al. (2013). Sulfadiazine resistance in Toxoplasma gondii: no involvement of overexpression or polymorphisms in genes of therapeutic targets and ABC transporters. Parasite 20, 19. doi: 10.1051/parasite/2013020
Drame P. M., Montavon C., Pion S. D., Kubofcik J., Fay M. P., Nutman T. B. (2016). Molecular epidemiology of blood-borne human parasites in a Loa loa-, Mansonella perstans-, and Plasmodium falciparum-endemic region of Cameroon. Am. J. Trop. Med. hygiene 94, 1301. doi: 10.4269/ajtmh.15-0746
Dzitko K., Dziadek B., Dziadek J., Dlugonska H. (2007). Toxoplasma gondii: inhibition of the intracellular growth by human lactoferrin. Pol. J. Microbiol. 56, 25–32.
Eda S., Eda K., Prudhomme J. G., Sherman I. W. (1999). Inhibitory activity of human lactoferrin and its peptide on chondroitin sulfate A-, CD36-, and thrombospondin-mediated cytoadherence of plasmodium falciparum-infected erythrocytes. Blood 94, 326–332. doi: 10.1182/blood.V94.1.326.413a32_326_332
Egan T. J., Combrinck J. M., Egan J., Hearne G. R., Marques H. M., Ntenteni S., et al. (2002). Fate of haem iron in the malaria parasite Plasmodium falciparum. Biochem. J. 365, 343. doi: 10.1042/bj20020793
Escobedo A. A., Cimerman S. (2007). Giardiasis: a pharmacotherapy review. Expert Opin. pharmacotherapy 8, 1885–1902. doi: 10.1517/14656566.8.12.1885
Feng Y., Xiao L. (2011). Zoonotic potential and molecular epidemiology of Giardia species and giardiasis. Clin. Microbiol. Rev. 24, 110–140. doi: 10.1128/CMR.00033-10
Ferrer P., Vega-Rodriguez J., Tripathi A. K., Jacobs-Lorena M., Sullivan D. J. Jr (2015). Antimalarial iron chelator FBS0701 blocks transmission by Plasmodium falciparum gametocyte activation inhibition. Antimicrobial Agents chemotherapy 59, 1418–1426. doi: 10.1128/AAC.04642-14
Fleet J. C. (1995). A new role for lactoferrin: DNA binding and transcription activation. J. Nutr. Rev. 53 (8), 226–227.
Fried M., Duffy P. E. (1996). Adherence of Plasmodium falciparum to chondroitin sulfate A in the human placenta. Science 272, 1502–1504. doi: 10.1126/science.272.5267.1502
Fritsch G., Sawatzki G., Treumer J., Jung A., Spira D. T. (1987). Plasmodium falciparum: inhibition in vitro with lactoferrin, desferriferrithiocin, and desferricrocin. Exp. Parasitol. 63, 1–9. doi: 10.1016/0014-4894(87)90072-5
Frontera L. S., Moyano S., Quassollo G., Lanfredi-Rangel A., Rópolo A. S., Touz M. C. (2018). Lactoferrin and lactoferricin endocytosis halt Giardia cell growth and prevent infective cyst production. Sci. Rep. 8, 1–15. doi: 10.1038/s41598-018-36563-1
Garcia-Salcedo J. A., Unciti-Broceta J. D., Valverde-Pozo J., Soriano M. (2016). New approaches to overcome transport related drug resistance in trypanosomatid parasites. Front. Pharmacol. 7, 351. doi: 10.3389/fphar.2016.00351
Gardner T. B., Hill D. R. (2001). Treatment of giardiasis. Clin. Microbiol. Rev. 14, 114–128. doi: 10.1128/CMR.14.1.114-128.2001
Gerace E., Presti V. D. M. L., Biondo C. (2019). Cryptosporidium infection: epidemiology, pathogenesis, and differential diagnosis. Eur. J. Microbiol. Immunol. 9, 119–123. doi: 10.1556/1886.2019.00019
Ghallab M. M., Ismail M. A., Haydara T., Morsy S. M. (2022). Cryptosporidiosis: molecular analysis, risk factors and seasonal abundance in immunocompetent and immunocompromised patients, kafrelsheikh university hospitals. J. Egyptian Soc. Parasitol. 52, 117–122. doi: 10.21608/jesp.2022.235822
Ghodsi M., Maheri M., Joveini H., Rakhshani M. H., Mehri A. (2019). Designing and evaluating educational intervention to improve preventive behavior against cutaneous leishmaniasis in endemic areas in Iran. Osong Public Health Res. Perspect. 10, 253. doi: 10.24171/j.phrp.2019.10.4.09
Gujo A. B., Kare A. P. (2021). Prevalence of intestinal parasite infection and its association with Anemia among children aged 6 to 59 months in sidama national regional state, Southern Ethiopia. Clin. Med. Insights: Pediatr. 15, 11795565211029259. doi: 10.1177/11795565211029259
Hajare S. T., Gobena R. K., Chauhan N. M., Eriso F. (2021). Prevalence of intestinal parasite infections and their associated factors among food handlers working in selected catering establishments from Bule Hora, Ethiopia. BioMed. Res. Int. 2021, 6669742. doi: 10.1155/2021/6669742
Halder A., Shukla D., Das S., Roy P., Mukherjee A., Saha B. (2018). Lactoferrin-modified Betulinic Acid-loaded PLGA nanoparticles are strong anti-leishmanials. Cytokine 110, 412–415. doi: 10.1016/j.cyto.2018.05.010
Hao L., Shan Q., Wei J., Ma F., Sun P. (2019). Lactoferrin: major physiological functions and applications. Curr. Protein Pept. Sci. 20, 139–144. doi: 10.2174/1389203719666180514150921
Honarparvar B., Kanchi S., Bisetty K. (2019). Theoretical insights into the competitive metal bioaffinity of lactoferrin as a metal ion carrier: A DFT study. New J. Chem. 43, 16374–16384. doi: 10.1039/C9NJ03786A
Hunter P. R., Nichols G. (2002). Epidemiology and clinical features of Cryptosporidium infection in immunocompromised patients. Clin. Microbiol. Rev. 15, 145–154. doi: 10.1128/CMR.15.1.145-154.2002
Hussein E. M., Zaki W. M., Ahmed S. A., Almatary A. M., Nemr N. I., Hussein A. M. (2016). Predominance of Giardia lamblia assemblage A among iron deficiency anaemic pre-school Egyptian children. Parasitol. Res. 115, 1537–1545. doi: 10.1007/s00436-015-4888-y
Ikadai H., Tanaka T., Shibahara N., Tanaka H., Matsuu A., Kudo N., et al. (2005). Inhibitory effect of lactoferrin on in vitro growth of Babesia caballi. Am. J. Trop. Med. Hyg 73, 710–712. doi: 10.4269/ajtmh.2005.73.710
Johnston V. J., Mabey D. C. (2008). Global epidemiology and control of Trichomonas vaginalis. Curr. Opin. Infect. Dis. 21, 56–64. doi: 10.1097/QCO.0b013e3282f3d999
Kagoro F. M., Barnes K. I., Marsh K., Ekapirat N., Mercado C. E. G., Sinha I., et al. (2022). Mapping genetic markers of artemisinin resistance in Plasmodium falciparum malaria in Asia: a systematic review and spatiotemporal analysis. Lancet Microbe 3 (3), E184–E192. doi: 10.1016/S2666-5247(21)00249-4
Kamaraj C., Ragavendran C., Kumar R. C. S., Ali A., Khan S. U., Luna-Arias J. P., et al. (2022). Antiparasitic potential of asteraceae plants: A comprehensive review on therapeutic and mechanistic aspects for biocompatible drug discovery. Phytomedicine Plus 2, 100377. doi: 10.1016/j.phyplu.2022.100377
Kamau P., Aloo-Obudho P., Kabiru E., Ombacho K., Langat B., Mucheru O., et al. (2012). Prevalence of intestinal parasitic infections in certified food-handlers working in food establishments in the City of Nairobi, Kenya. J. Biomed. Res. 26, 84–89. doi: 10.1016/S1674-8301(12)60016-5
Kanwar J. R., Mahidhara G., Kanwar R. K. (2012). Novel alginate-enclosed chitosan–calcium phosphate-loaded iron-saturated bovine lactoferrin nanocarriers for oral delivery in colon cancer therapy. Nanomedicine 7, 1521–1550. doi: 10.2217/nnm.12.29
Kassim O. O., Ako-Anai K. A., Torimiro S. E., Hollowell G. P., Okoye V. C., Martin S. K. (2000). Inhibitory factors in breastmilk, maternal and infant sera against in vitro growth of Plasmodium falciparum malaria parasite. J. Trop. Pediatr. 46, 92–96. doi: 10.1093/tropej/46.2.92
Kaur G., Chauhan K., Anand N., Kaur S. (2021). Evaluation of in vitro and in vivo protective efficacy of Bauhinia variegata against Leishmania donovani in Murine Model. Acta Parasitologica 66, 812–826. doi: 10.1007/s11686-020-00326-8
Kaur M., Juneja R. (2022). An analysis of relation between malaria and anaemia. Int. J. Innovative Res. Eng. Manage. 9, 303–306. doi: 10.55524/ijirem.2022.9.1.60
Kevric I., Cappel M. A., Keeling J. H. (2015). New world and old world Leishmania infections: a practical review. Dermatologic Clinics 33, 579–593. doi: 10.1016/j.det.2015.03.018
Kissinger P. J., Gaydos C. A., Seña A. C., Scott McClelland R., Soper D., Secor W. E., et al. (2022). Diagnosis and management of Trichomonas vaginalis: summary of evidence reviewed for the 2021 Centers for Disease Control and Prevention sexually transmitted infections treatment guidelines. Clin. Infect. Dis. 74, S152–S161. doi: 10.1093/cid/ciac030
Kondapi A. K. (2020). Targeting cancer with lactoferrin nanoparticles: recent advances. Nanomedicine 15, 2071–2083. doi: 10.2217/nnm-2020-0090
Kummer S., Hayes G. R., Gilbert R. O., Beach D. H., Lucas J. J., Singh B. N. (2008). Induction of human host cell apoptosis by Trichomonas vaginalis cysteine proteases is modulated by parasite exposure to iron. Microbial Pathogenesis 44, 197–203. doi: 10.1016/j.micpath.2007.09.004
Laniado-Laborín R., Cabrales-Vargas M. N. (2009). Amphotericin B: side effects and toxicity. Rev. iberoamericana micología 26, 223–227. doi: 10.1016/j.riam.2009.06.003
Legrand D., Pierce A., Elass E., Carpentier M., Mariller C., Mazurier J. (2008). Lactoferrin structure and functions bioactive components milk. Adv. Exp. Med. Biol. 606, 163–194. doi: 10.1007/978-0-387-74087-4_6
Leon-Sicairos N., Lopez-Soto F., Reyes-Lopez M., Godinez-Vargas D., Ordaz-Pichardo C., de la Garza M. (2006a). Amoebicidal activity of milk, apo-lactoferrin, sIgA and lysozyme. Clin. Med. Res. 4, 106–113. doi: 10.3121/cmr.4.2.106
León-Sicairos N., Martínez-Pardo L., Sánchez-Hernández B., de la Garza M., Carreroc C. J. (2012). Oral lactoferrin treatment resolves amoebic intracecal infection in C3H/HeJ mice. Biochem. Cell Biol. 90, 435–441. doi: 10.1139/o2012-008
Leon-Sicairos N., Reyes-Lopez M., Canizalez-Roman A., Bermudez-Cruz R. M., Serrano-Luna J., Arroyo R., et al. (2005). Human hololactoferrin: endocytosis and use as an iron source by the parasite Entamoeba histolytica. Microbiology 151, 3859–3871. doi: 10.1099/mic.0.28121-0
Leon-Sicairos N., Reyes-Lopez M., Ordaz-Pichardo C., de la Garza M. (2006b). Microbicidal action of lactoferrin and lactoferricin and their synergistic effect with metronidazole in Entamoeba histolytica. Biochem. Cell Biol. 84, 327–336. doi: 10.1139/o06-060
Lepanto M. S., Rosa L., Paesano R., Valenti P., Cutone A. (2019). Lactoferrin in aseptic and septic inflammation. Molecules 24, 1323. doi: 10.3390/molecules24071323
Lima M. F., Beltz L. A., Kierszenbaum F. (1988). Trypanosoma cruzi: a specific surface marker for the amastigote form. J. Protozool 35, 108–110. doi: 10.1111/j.1550-7408.1988.tb04086.x
Lima M. F., Kierszenbaum F. (1987). Lactoferrin effects on the interaction of blood forms of Trypanosoma cruzi with mononuclear phagocytes. Int. J. Parasitol. 17, 1205–1208. doi: 10.1016/0020-7519(87)90173-1
López-Soto F., González-Robles A., Salazar-Villatoro L., León-Sicairos N., Piña-Vázquez C., Salazar E. P., et al. (2009). Entamoeba histolytica uses ferritin as an iron source and internalises this protein by means of clathrin-coated vesicles. Int. J. Parasitol. 39, 417–426. doi: 10.1016/j.ijpara.2008.08.010
López-Soto F., León-Sicairos N., Nazmi K., Bolscher J. G., de la Garza M. (2010). Microbicidal effect of the lactoferrin peptides lactoferricin17–30, lactoferrampin265–284, and lactoferrin chimera on the parasite Entamoeba histolytica. Biometals 23, 563–568. doi: 10.1007/s10534-010-9295-3
Luan K., McCord M. G., West A. J., Cave G., Travanty N. V., Apperson C. S., et al. (2023). Mosquito blood feeding prevention using an extra-low DC voltage charged cloth. Insects 14, 405. doi: 10.3390/insects14050405
Lutshumba J., Ochiai E., Sa Q., Anand N., Suzuki Y. (2020). Selective upregulation of transcripts for six molecules related to T cell costimulation and phagocyte recruitment and activation among 734 immunity-related genes in the brain during perforin-dependent, CD8 + T Cell-Mediated elimination of toxoplasma gondii cysts. mSystems 5 (2), e00189–20. doi: 10.1128/mSystems.00189-20
Maertens J., Pagano L., Azoulay E., Warris A. (2022). Liposomal amphotericin B—the present. J. Antimicrobial Chemotherapy 77, ii11–ii20. doi: 10.1093/jac/dkac352
Maltezou H. C. (2010). Drug resistance in visceral leishmaniasis. J. Biomed. Biotechnol. 2010, 617521. doi:10.1155/2010/617521
Molyneux D. H. (2006). Control of human parasitic diseases: Context and overview. Adv. Parasitol. 61, 1–45. doi: 10.1016/S0065-308X(05)61001-9
Murray M., Murray A. B., Murray M. B., Murray C. (1978). The adverse effect of iron repletion on the course of certain infections. Br. Med. J. 2, 1113–1115. doi: 10.1136/bmj.2.6145.1113
Naot D., Grey A., Reid I. R., Cornish J. (2005). Lactoferrin–a novel bone growth factor. Clin. Med. Res. 3, 93–101. doi: 10.3121/cmr.3.2.93
Nasrallah J., Akhoundi M., Haouchine D., Marteau A., Mantelet S., Wind P., et al. (2022). Updates on the worldwide burden of amoebiasis: A case series and literature review. J. Infection Public Health 15, 1134–1141. doi: 10.1016/j.jiph.2022.08.013
Nath T. C., Eom K. S., Choe S., Islam S., Sabuj S. S., Saha E., et al. (2022). Insights to helminth infections in food and companion animals in Bangladesh: Occurrence and risk profiling. Parasite Epidemiol. Control 17, e00245. doi: 10.1016/j.parepi.2022.e00245
Niederkorn J. Y. (2021). The biology of Acanthamoeba keratitis. Exp. eye Res. 202, 108365. doi: 10.1016/j.exer.2020.108365
Nikolaeva D., Draper S. J., Biswas S. (2015). Toward the development of effective transmission-blocking vaccines for malaria. Expert Rev. Vaccines 14, 653–680. doi: 10.1586/14760584.2015.993383
Ockenhouse C. F., Tegoshi T., Maeno Y., Benjamin C., Ho M., Kan K. E., et al. (1992). Human vascular endothelial cell adhesion receptors for Plasmodium falciparum-infected erythrocytes: roles for endothelial leukocyte adhesion molecule 1 and vascular cell adhesion molecule 1. J. Exp. Med. 176, 1183–1189. doi: 10.1084/jem.176.4.1183
Onyiche T. E., Răileanu C., Fischer S., Silaghi C. (2021). Global distribution of Babesia species in questing ticks: A systematic review and meta-analysis based on published literature. Pathogens 10, 230. doi: 10.3390/pathogens10020230
Ordaz-Pichardo C., León-Sicairos N., Hernández-Ramírez V. I., Talamás-Rohana P., de la Garza M. (2012). Effect of bovine lactoferrin in a therapeutic hamster model of hepatic amoebiasis. Biochem. Cell Biol. 90, 425–434. doi: 10.1139/o11-084
Osazuwa F., Ayo O. M., Imade P. (2011). A significant association between intestinal helminth infection and anaemia burden in children in rural communities of Edo state, Nigeria. North Am. J. Med. Sci. 3, 30. doi: 10.4297/najms.2011.330
Page M. A., Mathers W. D. (2013). Acanthamoeba keratitis: a 12-year experience covering a wide spectrum of presentations, diagnoses, and outcomes. J. Ophthalmol. 2013 (670242), 1–6. doi: 10.1155/2013/670242
Palumbo E. (2010). Treatment strategies for mucocutaneous leishmaniasis. J. Global Infect. Dis. 2, 147. doi: 10.4103/0974-777X.62879
Paredes J. L., Sparks H., White A. C. Jr., Martinez-Traverso G., Ochoa T., Castellanos-González A. (2017). Killing of cryptosporidium sporozoites by lactoferrin. Am. J. Trop. Med. hygiene 97, 774–776. doi: 10.4269/ajtmh.16-0804
Parra M., Liu X., Derrick S. C., Yang A., Tian J., Kolibab K., et al. (2013). Molecular analysis of non-specific protection against murine malaria induced by BCG vaccination. PloS One 8, e66115. doi: 10.1371/journal.pone.0066115
Peterson K. M., Alderete J. F. (1984). Iron uptake and increased intracellular enzyme activity follow host lactoferrin binding by Trichomonas vaginalis receptors. J. Exp. Med. 160, 398–410. doi: 10.1084/jem.160.2.398
Pritt B. S. (2015). “Plasmodium and babesia,” in Manual of Clinical Microbiology, 11th Edition, Chapter 136.
Qureshi N. A. (2021). In vitro anticoccidial, antioxidant activities and biochemical screening of methanolic and aqueous leaves extracts of selected plants. Pak Vet J. 41 (1), 57–63. doi: 10.29261/pakvetj/2020.071
Ramírez-Rico G., Martinez-Castillo M., Cárdenas-Zúñiga R., Coronado-Velázquez D., Silva-Olivares A., de la Garza M., et al. (2023). Acanthamoeba castellanii genotype T4: inhibition of proteases activity and cytopathic effect by bovine apo-lactoferrin. Microorganisms 11, 708. doi: 10.3390/microorganisms11030708
Ramírez-Rico G., Martínez-Castillo M., Garza M., Shibayama M., Serrano-Luna J. (2015). Acanthamoeba castellanii proteases are capable of degrading iron-binding proteins as a possible mechanism of pathogenicity. J. Eukaryotic Microbiol. 62, 614–622. doi: 10.1111/jeu.12215
Reimão J. Q., Coser E. M., Lee M. R., Coelho A. C. (2020). Laboratory diagnosis of cutaneous and visceral leishmaniasis: current and future methods. Microorganisms 8, 1632. doi: 10.3390/microorganisms8111632
Rigo G. V., Frank L. A., Galego G. B., d. Santos A. L. S., Tasca T. (2022). Novel treatment approaches to combat trichomoniasis, a neglected and sexually transmitted infection caused by Trichomonas vaginalis: Translational perspectives. Venereology 1, 47–80. doi: 10.3390/venereology1010005
Rivera-Rivas L. A., Lorenzo-Benito S., Sánchez-Rodríguez D. B., Miranda-Ozuna J. F., Euceda-Padilla E. A., Ortega-López J., et al. (2020). The effect of iron on Trichomonas vaginalis TvCP2: A cysteine proteinase found in vaginal secretions of trichomoniasis patients. Parasitology 147, 760–774. doi: 10.1017/S0031182020000438
Robert C., Pouvelle B., Meyer P., Muanza K., Fujioka H., Aikawa M., et al. (1995). Chondroitin-4-sulphate (proteoglycan) a receptor for Plasmodium falciparum-infected erythrocyte adherence on brain microvascular endothelial cells. Res. Immunol. 146, 383–393. doi: 10.1016/0923-2494(96)81042-X
Roberts D. D., Sherwood J. A., Spitalnik S. L., Panton L. J., Howard R. J., Dixit V. M., et al. (1985). Thrombospondin binds falciparum malaria parasitized erythrocytes and may mediate cytoadherence. Nature 318, 64. doi: 10.1038/318064a0
Rogowska A., Pryshchepa O., Som N. N., Śpiewak P., Gołębiowski A., Rafińska K., et al. (2023). Study on the zinc ions binding to human lactoferrin. J. Mol. Structure 1282, 135149. doi: 10.1016/j.molstruc.2023.135149
Rossignol J. F., Kabil S. M., El–Gohary Y., Younis A. M. (2006). Effect of nitazoxanide in diarrhea and enteritis caused by Cryptosporidium species. Clin. Gastroenterol. Hepatol. 4, 320–324. doi: 10.1016/j.cgh.2005.12.020
Saravolatz L. D., Bern C., Adler-Moore J., Berenguer J., Boelaert M., den Boer M., et al. (2006). Liposomal amphotericin B for the treatment of visceral leishmaniasis. Clin. Infect. Dis. 43, 917–924. doi: 10.1086/507530
Semenza J., Nichols G. (2007). Cryptosporidiosis surveillance and water-borne outbreaks in Europe. Eurosurveillance 12, 13–14. doi: 10.2807/esm.12.05.00711-en
Shakibaei M., Frevert U. (1996). Dual interaction of the malaria circumsporozoite protein with the low density lipoprotein receptor-related protein (LRP) and heparan sulfate proteoglycans. J. Exp. Med. 184, 1699–1711. doi: 10.1084/jem.184.5.1699
Shibeshi M. A., Kifle Z. D., Atnafie S. A. (2020). Antimalarial drug resistance and novel targets for antimalarial drug discovery. Infect. Drug Resist. 13, 4047–4060. doi: 10.2147/IDR.S279433
Silva T., Abengózar M. C., Fernández-Reyes M., Andreu D., Nazmi K., Bolscher J. G., et al. (2012). Enhanced leishmanicidal activity of cryptopeptide chimeras from the active N1 domain of bovine lactoferrin. Amino Acids 43, 2265–2277. doi: 10.1007/s00726-012-1304-0
Sinnis P., Willnow T. E., Briones M. R., Herz J., Nussenzweig V. (1996). Remnant lipoproteins inhibit malaria sporozoite invasion of hepatocytes. J. Exp. Med. 184, 945–954. doi: 10.1084/jem.184.3.945
Siqueiros-Cendón T., Arévalo-Gallegos S., Iglesias-Figueroa B. F., García-Montoya I. A., Salazar-Martínez J., Rascón-Cruz Q. (2014). Immunomodulatory effects of lactoferrin. Acta Pharmacologica Sin. 35, 557. doi: 10.1038/aps.2013.200
Skaar E. P. (2010). The battle for iron between bacterial pathogens and their vertebrate hosts. PloS Pathog. 6, e1000949. doi: 10.1371/journal.ppat.1000949
Snow R. W., Guerra C. A., Noor A. M., Myint H. Y., Hay S. I. (2005). The global distribution of clinical episodes of Plasmodium falciparum malaria. Nature 434, 214. doi: 10.1038/nature03342
Song H.-O. (2016). Influence of 120 kDa pyruvate: ferredoxin oxidoreductase on pathogenicity of Trichomonas vaginalis. Korean J. Parasitol. 54, 71. doi: 10.3347/kjp.2016.54.1.71
Sorensen M. (1939). The Proteins in Whey Volume 23. In Volume 27 of Comptes Rendus des travaux du Laboratoire Carlsberg. Hagerup in Komm, 44.
Stijlemans B., Beschin A., Magez S., Van Ginderachter J. A., De Baetselier P. (2015). Iron homeostasis and Trypanosoma brucei associated immunopathogenicity development: a battle/quest for iron. BioMed. Res. Int. 2015 (819389), 1–15. doi: 10.1155/2015/819389
Stijlemans B., De Baetselier P., Magez S., Van Ginderachter J. A., De Trez C. (2018). African trypanosomiasis-associated anemia: the contribution of the interplay between parasites and the mononuclear phagocyte system. Front. Immunol. 9, 218. doi: 10.3389/fimmu.2018.00218
Sundar S., More D. K., Singh M. K., Singh V. P., Sharma S., Makharia A., et al. (2000). Failure of pentavalent antimony in visceral leishmaniasis in India: report from the center of the Indian epidemic. Clin. Infect. Dis. 31, 1104–1107. doi: 10.1086/318121
Suntaravitun P., Dokmaikaw A. (2018). Prevalence of intestinal parasites and associated risk factors for infection among rural communities of Chachoengsao Province, Thailand. Korean J. Parasitol. 56, 33. doi: 10.3347/kjp.2018.56.1.33
Swanson M., Pickrel A., Williamson J., Montgomery S. (2023). Trends in reported babesiosis cases—United States 2011–2019. Am. J. Transplant. 23, 582–584. doi: 10.1016/j.ajt.2023.03.013
Tanaka T., Abe Y., Inoue N., Kim W. S., Kumura H., Nagasawa H., et al. (2004). The detection of bovine lactoferrin binding protein on Trypanosoma brucei. J. Vet. Med. Sci. 66, 619–625. doi: 10.1292/jvms.66.619
Tanaka T., Omata Y., Isamida T., Saito A., Shimazaki K., Yamauchi K., et al. (1998). Growth inhibitory effect of bovine lactoferrin to Toxoplasma gondii tachyzoites in murine macrophages: tyrosine phosphorylation in murine macrophages induced by bovine lactoferrin. J. Vet. Med. Sci. 60, 369–371. doi: 10.1292/jvms.60.369
Tanaka T., Omata Y., Narisawa M., Saito A., Shimazaki K., Igarashi I., et al. (1997). Growth inhibitory effect of bovine lactoferrin on Toxoplasma gondii tachyzoites in murine macrophages: role of radical oxygen and inorganic nitrogen oxide in Toxoplasma growth-inhibitory activity. Vet. Parasitol. 68, 27–33. doi: 10.1016/S0304-4017(96)01069-2
Tanaka T., Omata Y., Saito A., Shimazaki K., Suzuki N. (1996). Growth inhibitory effect of bovine lactoferrin on toxoplasma gondii parasites in mouse somatic cells. J. Vet. Med. Sci. 58, 61–65. doi: 10.1292/jvms.58.61
Tanaka T., Omata Y., Saito A., Shimazaki K., Yamauchi K., Takase M., et al. (1995). Toxoplasma gondii: parasiticidal effects of bovine lactoferricin against parasites. Exp. Parasitol. 81, 614–617. doi: 10.1006/expr.1995.1157
Theel E. S., Pritt B. S. (2016). Parasites. Microbiol. Spectr. 4 (4). doi: 10.1128/microbiolspec.dmih1122-0013-2015
Thipubon P., Uthaipibull C., Kamchonwongpaisan S., Tipsuwan W., Srichairatanakool S. (2015). Inhibitory effect of novel iron chelator, 1-(N-acetyl-6-aminohexyl)-3-hydroxy-2-methylpyridin-4-one (CM1) and green tea extract on growth of Plasmodium falciparum. Malaria J. 14, 1–9. doi: 10.1186/s12936-015-0910-1
Thuma P. E., Olivieri N. F., Mabeza G. F., Biemba G., Parry D., Zulu S., et al. (1998). Assessment of the effect of the oral iron chelator deferiprone on asymptomatic Plasmodium falciparum parasitemia in humans. Am. J. Trop. Med. hygiene 58, 358–364. doi: 10.4269/ajtmh.1998.58.358
Tomita S., Suzuki C., Wada H., Nomachi M., Imayasu M., Araki-Sasaki K. (2016). Effects of lactoferrin on the viability and the encystment of Acanthamoeba trophozoites. Biochem. Cell Biol. 95, 48–52. doi: 10.1139/bcb-2016-0054
Tomita M., Takase M., Bellamy W., Shimamura S. (1994). A review: the active peptide of lactoferrin. Acta Paediatr. Jpn 36, 585–591. doi: 10.1111/j.1442-200X.1994.tb03250.x
Turchany J. M., Aley S. B., Gillin F. D. (1995). Giardicidal activity of lactoferrin and N-terminal peptides. Infect. Immun. 63, 4550–4552. doi: 10.1128/iai.63.11.4550-4552.1995
Turchany J. M., Mccaffery J. M., Aley S. B., Gillin F. D. (1997). Ultrastructural effects of lactoferrin binding on Giardia lamblia trophozoites. J. Eukaryotic Microbiol. 44, 68–72. doi: 10.1111/j.1550-7408.1997.tb05694.x
Vannier E., Krause P. J. (2012). Human babesiosis. New Engl. J. Med. 366, 2397–2407. doi: 10.1056/NEJMra1202018
Varma D. M., Redding E. A., Bachelder E. M., Ainslie K. M. (2020). Nano-and microformulations to advance therapies for visceral leishmaniasis. ACS Biomaterials Sci. Eng. 7, 1725–1741. doi: 10.1021/acsbiomaterials.0c01132
Velázquez-Antunez J., Olivares-Perez J., Olmedo-Juárez A., Rojas-Hernandez S., Villa-Mancera A., RomeroRosales T. (2023). Biological Activity of the Secondary Compounds of Guazuma ulmifolia Leaves to Inhibit the Hatching of Eggs of Haemonchus contortus. Pak Vet. J. 43 (1), 55–60. doi: 10.29261/pakvetj/2022.075
Vivancos V., González-Alvarez I., Bermejo M., Gonzalez-Alvarez M. (2018). Giardiasis: characteristics, pathogenesis and new insights about treatment. Curr. Topics Medicinal Chem. 18, 1287–1303. doi: 10.2174/1568026618666181002095314
Wilson M. E., Vorhies R. W., Andersen K. A., Britigan B. E. (1994). Acquisition of iron from transferrin and lactoferrin by the protozoan Leishmania chagasi. Infect. Immun. 62, 3262–3269. doi: 10.1128/iai.62.8.3262-3269.1994
Wulandari A. R., Nurlaelasari A., Nugroho H. A., Cahyadi M., Kurniawan W., Hamid P. H. (2023). Ethanolic extract of Etlingera elatior flower exhibits anthelmintic properties to Fasciola gigantica in vitro. Open Veterinary J. 13, 576. doi: 10.5455/OVJ.2023.v13.i5.10
Keywords: parasites, lactoferrin (Lf), bovine lactoferrin (bLf), human lactoferrin (hLf), iron chelation, Lf peptide, Lf nanoformulation
Citation: Anand N (2024) Antiparasitic activity of the iron-containing milk protein lactoferrin and its potential derivatives against human intestinal and blood parasites. Front. Parasitol. 2:1330398. doi: 10.3389/fpara.2023.1330398
Received: 30 October 2023; Accepted: 30 December 2023;
Published: 28 February 2024.
Edited by:
Zia Ud Din Sindhu, University of Agriculture, Faisalabad, PakistanReviewed by:
Muhammad Imran, University of Agriculture, Faisalabad, PakistanAlejandro Castellanos-Gonzalez, University of Texas Medical Branch at Galveston, United States
Copyright © 2024 Anand. This is an open-access article distributed under the terms of the Creative Commons Attribution License (CC BY). The use, distribution or reproduction in other forums is permitted, provided the original author(s) and the copyright owner(s) are credited and that the original publication in this journal is cited, in accordance with accepted academic practice. No use, distribution or reproduction is permitted which does not comply with these terms.
*Correspondence: Namrata Anand, bmFtcmF0YS5hbmFuZEB1a3kuZWR1