- 1Department of Life Sciences, Imperial College London, London, United Kingdom
- 2MRC London Institute of Medical Sciences, Imperial College London, London, United Kingdom
- 3Department of Biochemistry, University of Oxford, Oxford, United Kingdom
Despite recent advances, animal-parasitic nematodes have thus far been largely refractory to genetic manipulation. We describe here a new approach providing proof of principle that CRISPR/Cas9-mediated gene editing of parasitic nematodes is achievable using vesicular stomatitis virus glycoprotein-pseudotyped extracellular vesicles for the delivery of Cas9-single guide ribonucleoprotein complexes. We demonstrate that extracellular vesicle-delivered ribonucleoproteins can be used to disrupt a secreted deoxyribonuclease in Nippostrogylus brasiliensis. Introduction of a repair template encoding multiple stop codons led to measurable reduction in expression of the targeted gene. Altered transcripts corresponding to the edited locus were detected by RT-PCR, demonstrating that vesicles can access cells of tissues actively expressing the gene of interest. These data provide evidence that this technique can be employed for targeted gene editing in N. brasiliensis, making this species genetically tractable for the first time, although further refinement will be necessary for routine and robust interrogation of gene function.
Introduction
Over a quarter of the world’s population are estimated to be infected with soil-transmitted helminths, representing a severe burden of disease and disability (Jourdan et al., 2018). Additionally, gastrointestinal nematodes are responsible for major economic losses to the livestock industry, with rising multi-drug resistance to the major classes of anthelmintics (Kaplan, 2020). A major bottleneck to identifying molecules that might serve as new drug targets or vaccine candidates is the genetic intractability of most parasitic nematodes, which limits screening proteins for biological properties and essential functions.
The delivery of foreign DNA or RNA has been identified as a limiting factor for gene silencing and transgenesis in parasitic nematodes (Selkirk et al., 2012; Castelletto et al., 2020). Recently, we showed that Nippostrongylus brasiliensis could be transduced with vesicular stomatitis virus glycoprotein (VSV-G)-pseudotyped lentiviral particles, with entry most likely mediated by binding of VSV-G to low-density lipoprotein receptor-related (LRP) proteins (Finkelshtein et al., 2013; Hagen et al., 2021). However, the delivered expression cassette was subjected to gene silencing during worm development, such that further optimisation of the system is required in order to achieve robust and reliable transgene expression (Hagen et al., 2021).
Clustered Regularly Interspaced Short Palindromic Repeat (CRISPR)/Cas9 mediated gene editing is a powerful tool which facilitates permanent modifications in genomic DNA, and this has been successfully applied to a range of helminth species for the generation of gene knockout and knockin mutants (Gang et al., 2017; Quinzo et al., 2022). Delivery of expression cassettes or Cas9/single guide (sg)RNA ribonucleoprotein (RNP) complexes to parasitic nematodes has generally proven problematic, with two exceptions, in which a unique free-living phase (Strongyloides) or development of a culture system which promoted parasite development (Brugia malayi) facilitated genetic manipulation of parasites and selection of mutants (Gang et al., 2017; Liu et al., 2018; Liu et al., 2020).
Recently, a new approach was established for transient delivery of RNP complexes into mammalian cells using VSV-G-pseudotyped extracellular vesicles, named NanoMEDIC (nanomembrane-derived extracellular vesicles for the delivery of macromolecular cargo) (Gee et al., 2020). Proof of principle was demonstrated in several cell types which had proven difficult to transfect such as induced pluripotent stem cells, neurons and myoblasts (Gee et al., 2020). We thought that this could be a useful tool to apply to helminths, as lengthy optimisation of the viral delivery system for individual parasite species would be circumvented by production and assembly of RNP complexes in mammalian cells for which optimised expression cassettes and transfection protocols are readily available. Following the success of VSV-G-mediated uptake of lentiviral particles, we investigated whether NanoMEDIC could be utilised in N. brasiliensis as a model gastrointestinal nematode.
Materials and methods
Expression of recombinant DNase II in yeast
The coding sequence of dnase2_1590 was amplified from N. brasiliensis cDNA omitting the signal peptide and stop codon and cloned into into pPICZalpha-A downstream of the coding sequence for the Saccharomyces cerevisiae a-mating secretion factor and in frame with an N-terminal myc and 6xHis Tag. PCR was carried out using Q5 polymerase (New England Biolabs) according to manufacturer’s recommendations with 500 nM of the following primers (lower case indicating nucleotides added for cloning purposes, restriction site underlined):
F-5´-aagctGAATTCGGTCTGAGTTGCAAGAACATGGAGG-3´
R-5-ttttgtTCTAGAGCGGTTTTGTTTGTCTTCTTGCTCG-3´
Following transformation of Pichia pastoris X-33, protein expression was optimised for single colonies and scaled up following the EasySelect Pichia expression protocol (Invitrogen). His-tagged proteins were purified from yeast supernatants by nickel chelating chromatography and protein concentration determined by Bradford assay.
Antibody production, protein preparation and western blotting
A polyclonal antiserum to N. brasiliensis recombinant DNase II was raised by subcutaneous immunisation of a rat with 100 µg protein emulsified in alum, followed by 3 boosts of 50 µg protein via the same route at weeks 4, 6 and 8, and the animal bled at week 9. Parasite proteins were prepared from larval and adult parasites by homogenising in phosphate buffered saline, 0.25 mM n-dodecyl-β-D-maltoside and protease inhibitors for somatic extracts, whereas secreted proteins were collected by in vitro culture in serum-free RPMI-1640 plus antibiotics and concentrated in vivaspin concentrators with a 3 kDa cutoff, as previously described (Huang et al., 2010). Western blotting was performed via standard procedures following resolution of 5 µg parasite proteins by SDS-12% polyacrylamide gel electrophoresis and blotting to polyvinylidene difluoride membrane. Rat anti-DNase II was used at 1:500 dilution, rabbit anti-rat IgG-horseradish peroxidase (Sigma) used as secondary antibody and the blot visualised using enhanced chemiluminescence western blotting detection reagents (Amersham Bioscience).
Vector construction
For facilitated cloning of synthesised sgRNA oligos, a NheI restriction site was introduced into the RGR expression vector (pL-5LTR-RGR(DMD#1)-AmCyan-A, Addgene plasmid #138482). To do this, the entire sequence between two SpeI restriction sites flanking the RGR region was amplified by PCR adding an NheI and AvrII restriction site to the 3´ end and used to replace the original sequence via SpeI and AvrII to allow for cloning of gRNA coding regions via KpnI and NheI. PCR was carried out in 50 μl reactions using Q5 polymerase (New England Biolabs) according to manufacturer’s recommendations with 1 ng of the RGR plasmid as template and 500 nM of the following primers (lower case indicating nucleotides added for cloning purposes and restriction site underlined): F-5´-GCTTGCATGCCGACATGGATTATTGACTAGTCCC-3´; R-5´-attgaCCTAGGGCTAGCTCTAGAGCGGCCGTCCCATTCGCCATGC-3.
The CRISPR/Cas-derived RNA-guided endonucleases (RGEN) algorithm was used to predict Streptococcus pyogenes (Sp) Cas9 gRNA targets with a 5´-NGG-3´ Protospacer Adjacent Motif (PAM) in exon 3 of the dnase-2 gene and subsequent off-target screening against the N. brasiliensis genome. Oligonucleotides encoding gRNAs for subsequent integration into the RGR plasmid via KpnI and NheI were synthesized (GeneArt, Thermo Fisher Scientific) with the following structure: 5´-KpnI-inverted first 6 nt of the guide RNA-HH ribozyme-guide RNA-gRNA scaffold-HDV ribozyme-NheI-3´ (complete sequence Supplementary Figure 3). Plasmids were maintained in NEB stable Escherichia coli (New England Biolabs). Positive transformants were selected on LB agar plates containing 50 μg ml-1 ampicillin. Constructs were verified for error-free integration of transgenes by routine Sanger sequencing (Eurofins Genomics).
Extracellular vesicle production
NanoMEDIC were produced in HEK293T cells maintained in Dulbecco’s Modified Eagle’s Medium (DMEM) at 37°C, 10% foetal calf serum (FCS), 2 mM L-glutamine, 100 units ml-1 penicillin and 100 μg ml-1 streptomycin, as described previously (Gee et al., 2020) with some modifications. In brief, per well of a 6-well plate, 3 x 106 cells were transfected with 1.25 μg of gRNA-encoding plasmid, 1.25 μg of pHLS-EF1a-FRB-SpCas9-A (Addgene plasmid #138477), 1.25 μg of pHLS-EF1a-FKBP12-GagHIV (Addgene plasmid #138476), 250 ng of pcDNA1- TatHIV (Addgene plasmid #138478) and 500 ng of pMD2.G (VSV-G) (Addgene plasmid #12259), using Lipofectamine 2000 at a ratio of 1:2.5 (Life Technologies). After 16 hours, the transfection medium was replaced with 2 ml of reduced serum culture medium (5% FCS) supplemented with 300 μM A/C heterodimerization agent (formerly AP21967, Takara Bio), 20 mM HEPES and 10 μM cholesterol (balanced with methyl-β-cyclodextrin, Sigma) (Chen et al., 2009). VSV-G-EVs were produced in HEK293T cells following transfection of 3 x 106 cells with 1.25 μg of pMD2.G per well of a 6-well plate with Lipofectamine 2000 at a ratio of 1:2.5. The cell culture supernatant was changed after 18 hours as for NanoMEDIC, omitting the heterodimerisation agent. After an additional incubation of 48 hours at 37°C and 10% CO2, the EV-containing cell supernatant was harvested, centrifuged at 2,000 x g for 20 min at 4°C and passed through a 0.45 μm Acrodisc syringe filter. NanoMEDIC and VSV-G-EVs were generally concentrated using 10 kDa vivaspin columns and washed twice with serum-free growth medium (NanoMEDIC) or trehalose buffer (10% trehalose in PBS) (VSV-G-EVs). Following centrifugation at 4,000 x g for 15 min at 4°C, the flow-through was discarded and EVs gently resuspended in 2 ml of the respective buffer before aliquoting into cryovials and storage at -80°C. For enrichment by polymer-based precipitation, 4 ml of Lenti-X concentrator (Takara Bio) was added to 12 ml of cell supernatant and the mixture incubated at 4°C with gentle agitation for 18 hours. Precipitated EVs were then pelleted by centrifugation at 3000 x g for 45 min and 4C and resuspended in 2 ml of trehalose buffer.
Analysis of extracellular vesicles
The composition of EVs was analysed by Western blot and Nano-flow cytometry. Western blotting was performed via standard procedures following resolution of 12 µl concentrated EV preparations by SDS-12% polyacrylamide gel electrophoresis under reducing (Cas9, VSV-G) or non-reducing (CD63, CD81) conditions. Primary antibodies were used at 1:1000 dilution: mouse anti-human CD63 (clone H5C6, Biolegend); mouse anti-human CD81 (TAPA-1) (clone 5A6, Biolegend); rabbit anti-VSV-G (Covance); mouse anti-CRISPR (Cas9) (clone 7A9, Biolegend).
Concentrated NanoMEDIC preparations were analysed for their nanoparticle content by nano-flow cytometry using a NanoAnalyzer calibrated against trehalose buffer. Before acquisition, samples were diluted 1:100 and 1:200 in trehalose buffer. The concentration of particles with diameters larger than 100 nm was determined following gating using the NanoFCM™ Silica Nanospheres Cocktail (S16M-Exo, diameter:68~155 nm) as a standard.
Loading of extracellular vesicles with homology directed repair templates
Oligonucleotides encoding the HDR templates (Supplementary Figure 3) were synthesized (Invitrogen) and reconstituted in nuclease-free water at a concentration of 1 µg µl-1. VSV-G-EVs were then loaded with ssDNA as described previously (Lamichhane et al., 2015). In brief, 5 µg of ssDNA were added to 95 µl of VSV-G-EVs in trehalose buffer and EVs then transferred to a 1 mm electroporation cuvette (BioRad) placed on ice. EVs were electroporated by exponential decay with two pulses at 400 V and 125 µF using a GenePulser Xcell electroporator (Bio-Rad). Cuvettes were placed on ice immediately after electroporation and incubated on ice for 20 min. EVs were then transferred to fresh microfuge tubes and the cuvette washed with one volume (100 µl) of RPMI and added to the tube. To alleviate aggregation, EDTA was added to a final concentration of 1 mM and EVs incubated at room temperature for 15 min, gently resuspended several times during incubation (Lamichhane et al., 2015).
Parasite infection, recovery and exposure to extracellular vesicles
N. brasiliensis were maintained in male SD rats, and infective larvae isolated from faecal cultures using a Baermann apparatus. Larvae were activated to feed as previously described (Huang et al., 2010) for 48 to 72 hrs in RPMI1640, 0.65% glucose, 2 mM L-glutamine, 100 U ml-1 penicillin, 100 μg ml-1 streptomycin, 100 μg ml-1 gentamicin, 20 mM HEPES, 2% rat serum (worm culture medium), then washed twice in serum-free medium prior to exposure to EVs. Per well of a 12-well plate, approximately 3,000 - 4,000 activated L3 were exposed to 200 µl NanoMEDIC and/or 200 µl of VSV-G-EVs, volumes adjusted to 1 ml with serum-free medium and 10 μg ml-1 polybrene (Sigma) and 200 µg ml-1 gentamicin added. EV preparations in control worms were substituted with HEK293T cell supernatant from untransfected cells. Following incubation for 18 to 24 hrs, worms were transferred to a 15 ml tube and washed twice in 10 ml of warm serum-free worm medium containing 1 mM EDTA with centrifugation at 150 x g for 1 min between washes. Worms were then resuspended in 2 ml complete worm medium (or serum-free medium when testing for ES products) and incubated for another 24 to 48 hours at 37°C, 5% CO2.
Detection of homology directed repair in genomic DNA
Homology directed repair (HDR) was effected utilising a single stranded oligodeoxynucleotide with ~50 nt homology arms, which introduced a series of 6 stop codons following a single (sgRNA91) or double (sgRNA46 and 91) stranded DNA break. Detection of HDR was performed by PCR analysis of genomic DNA following exposure of activated L3 to NanoMEDIC with or without the addition of HDR-containing EVs. Worms were washed twice in 10 ml of PBS at 37°C, 72 hrs post exposure to EVs, and genomic DNA isolated using the DNeasy Blood and Tissue DNA extraction kit (Qiagen). PCR was performed using Q5 polymerase with an annealing/extension temperature of 72°C. Primer pairs were designed to amplify fragments from the adjacent exon (2 or 4) and the HDR region (6xstop). The following primers were used with resultant fragment sizes indicative of editing. Exon 2 forward primer (5´-GATTCGGCTATTGGTGCAACTGTTAAGC-3´) and 6xstop reverse primer (5´-ATCCCCGTGCTACTCAGTTACCTAGTCACTTA-3´) gave rise to a 240 bp fragment with a single stranded DNA break (sgRNA91) and a 200 bp fragment with a double stranded DNA break (sgRNA46 and 91). The 6xstop forward primer (TAAGTGACTAGGTAACTGAGTAGC-3´) and exon 4 reverse primer (5´-TCGAGCCTGATTCGGGGTAGTCG-3´) gave rise to a 165 bp fragment in both cases. Exon 3 forward primer (5´-ACCTCAAAATTGCCTACAACGAC-3´) and 6xstop reverse primer gave rise to a 125 bp fragment with a single stranded DNA break (sgRNA91) and an 85 bp fragment with a double stranded DNA break (sgRNA46 and 91). PCR was also performed with primers specific to exon 3 flanking the HDR integration site: exon 3 forward primer as indicated above and exon 3 reverse primer (5´-GGAATCTTGGCACACTGTGTACCAGC-3´). Amplification of eif-3c (eukaryotic translation initiation factor 3 subunit C) was performed to control for genomic DNA integrity (forward primer 5´-GAACACGTTGTAGCTGCGTCA-3´ and reverse primer 5´-AATAGGTTCTCAGCGATTCCGTT-3´).
Preparation of DNA libraries and deep sequencing
PCR was performed using Q5 polymerase with an annealing/extension temperature of 72°C using exon 3 forward (5´-ACCTCAAAATTGCCTACAACGAC-3´) and reverse (5´-GGAATCTTGGCACACTGTGTACCAGC-3´) primer to amplify the WT allele and the edited allele, and the product was purified using gel extraction. DNA libraries for deep sequencing were made using the NEBNext Ultra II DNA library preparation kit (New England Biolabs) according to the manufacturer’s instructions. Sequencing was performed by the London Institute of Medical Sciences Genomics facility. Reads were mapped to the predicted PCR products from wild type and edited alleles using Bowtie2 (Langmead and Salzberg, 2012). SNPs and indels were mapped using samtools mPileup (Li et al., 2009). We then used Varscan2 (Koboldt et al., 2012) using the commands pileup2snp and pileup2indel to identify putative SNPs and indels respectively, requiring a minimum average quality of 20 and setting a minimum frequency of 1 in 10000 to enable low frequency alterations to be detected. Data were read into R using the read.table function and comparison of the different files enabled SNPs that were present in the untransfected control to be identified and removed as likely sequencing errors or PCR artefacts. Line plots indicating the frequency of SNPs at each position divided by total reads aligned to that position were constructed to illustrate the distribution of mutations along the template.
Reverse transcription PCR and Real-Time quantitative PCR
Total RNA was extracted using TRIreagent (Sigma) and converted to cDNA using an iScript cDNA kit (Biorad) following removal of contaminating genomic DNA by DNAse I. Reverse Transcription PCR (RT-PCR) was carried out using Q5 DNA polymerase (New England Biolabs) according to the manufacturer’s recommendations. An edited dnase2 transcript was confirmed with an exon 2-specific forward primer (5´-GATTCGGCTATTGGTGCAACTGTTAAGC-3´) and a reverse primer binding the 6xSTOP region in the HDR template (5´-ATCCCCGTGCTACTCAGTTACCTAGTCACTTA-3´), resulting in a product of approximately 140 bp. RT-PCR amplification of eif-3c was performed to control for RNA integrity (forward primer 5´-GAACACGTTGTAGCTGCGTCA-3´ and reverse primer 5´-AATAGGTTCTCAGCGATTCCGTT-3´).
Real-Time quantitative PCR (RT-qPCR) was carried out with a Step-One PLUS Fast Real-time PCR cycler (Applied Biosystems) under standard fast cycling conditions using PowerUP SYBR Green PCR Master Mix (Applied Biosystems) and 250 nM target gene specific forward and reverse primers. PCR amplification efficiencies were established for each primer pair (Pfaffl, 2001) and ranged between 1.9 and 2.1. Cycle threshold (Ct) values of target genes were normalised to the geometric mean of eif-3C (NBR_0001150401; eukaryotic translation initiation factor 3 subunit C) and idhg-1 (NBR_0000658601; isocitrate dehydrogenase gamma) (Hagen et al., 2021) and calibrated to the mean untreated control (wild type) samples for relative quantification by the comparative Ct method (Livak and Schmittgen, 2001). The primers were (forward (F), reverse (R)): Nb-nuc-1 F: 5´- TGACGAACCATACAACGGCA-3´, R: 5´- TGGAACACTGTGGATCAGCC-3´; eif-3C F: 5´-GAACACGTTGTAGCTGCGTCA-3´, R: 5´-AATAGGTTCTCAGCGATTCCGTT-3´; idhg-1 F: 5´-CAGAAATTGGGAGACGGCCT-3´, R: 5´-CCGAGAAACCAGCTGCATAGA-3´; dnase2_1590_E6F: 5´-TGGAAACTTGGAGAAACGGTGCTG-3´; dnase2_1590_E7R: 5´-ACATCTCCGATACAAACTAGGGGCTCC-3´.
Deoxyribonuclease activity assay
Supernatant collected from worms cultured in serum-free medium was thawed and all reactions prepared on ice. Per test sample, 500 ng of a plasmid DNA substrate in 10 µl nuclease free water was placed in PCR tubes and a 10 µl droplet of worm supernatant transferred to the side of the tube. Reactions were initiated by centrifugation and samples placed immediately into a PCR cycler pre-warmed to 37°C and incubated for 2 to 10 min. After heat inactivation at 75°C for 10 min, 4 µl of agarose loading dye was added and 10 µl of the sample separated on a 1% agarose gel.
To assess the dynamics of DNase secretion by L3, worms were extensively washed in serum-free medium, counted and resuspended at 2500 L3 ml-1. Per time point tested, 80 µl of worm suspension (200 L3) was added to 20 µl of plasmid DNA solution (2 µg in 20 µl RPMI). Following incubation for 15, 30, 60 or 120 min at 37°C, 5% CO2, 80 µl of supernatant was carefully aspirated and transferred to a PCR tube. Samples were immediately frozen at -20°C. For analysis, tubes were placed directly from the freezer into a PCR cycler prewarmed to 75°C and heat inactivated for 10 min. Loading dye was added and the samples separated on a 1% agarose gel.
Statistics
Treatment groups were analysed for significant differences with the Kruskal-Wallis test and Dunn’s post-hoc test in relation to the control group. Values are expressed as the median with range or the mean ± SEM, and significant differences were determined using GraphPad Prism. P values of <0.05 were considered significant, *p<0.05.
Results
Characterisation of dnase2 expression in different life cycle stages
To assess susceptibility of CRISPR-mediated gene editing in N. brasiliensis, we chose secreted DNase II as a target gene (Bouchery et al., 2020), as endonuclease activity in secreted products provides a means for functional analysis of gene expression. While the DNase II has been described to be secreted from infective larvae (Sotillo et al., 2014), a profile of expression in different stages has not been determined. Because a shift in temperature to 37°C acts as a cue for exsheathment and initiation of feeding in infective larvae (L3) (Huang et al., 2010), we first analysed levels of DNase II transcripts and secretion of active enzyme in L3 prior to and during activation by culture at 37°C in the presence of rat serum. Incubation at the elevated temperature led to a sharp increase in DNase II transcripts relative to those of unactivated L3, i.e. isolated directly from faecal cultures in a state of diapause, peaking at an approximate 1000-fold increase after 2-3 days (Figure 1A). Transcript levels were drastically reduced in adult worms compared with activated L3, but remained approximately 5-fold above those in non-activated L3 (Figure 1A). Western blot analysis of parasite secreted products confirmed that the DNase II was released predominantly by activated L3 (Figure 1B). Given the detection of low levels of DNase II transcripts in non-activated L3, there was a possibility that the protein might be pre-synthesised and stored in secretory vesicles allowing for immediate release upon entering the host. To assess this, we exposed non-activated and activated L3 to plasmid DNA during culture at 37°C. However, non-activated L3 did not secrete sufficient amounts of DNase II for effective degradation of DNA within the first 2 hours of incubation at 37°C, although complete degradation was achieved following overnight incubation (Figure 1C). In contrast, degradation of DNA in the culture supernatant was observed immediately following exposure to previously activated L3.
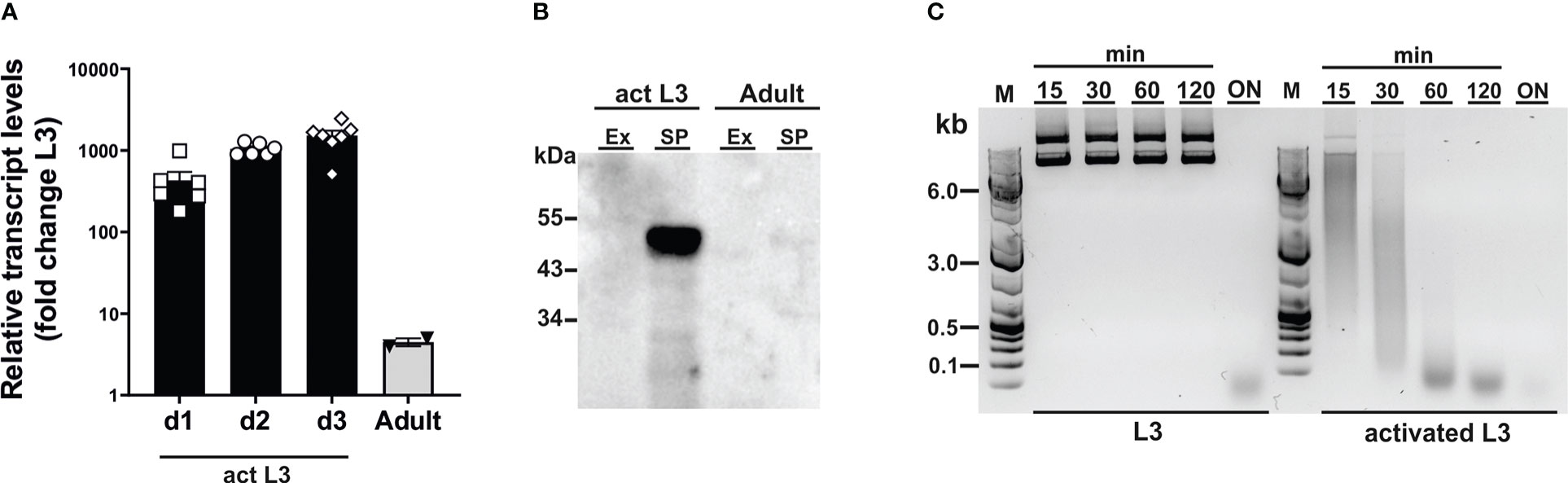
Figure 1 Dnase2 expression in Nippostrongylus brasiliensis. (A) Dnase2 transcripts are upregulated in L3 following activation at 37°C. Freshly isolated L3 were washed extensively, incubated at 37°C, and collected after 1 to 3 days of in vitro culture. Adult worms were recovered from the intestine of rats at day 8 post-infection. Activated L3 and adult worms were analysed by RT-qPCR for dnase2 transcript levels relative to those of unactivated L3. (B) DNase II is predominantly secreted by activated L3. Adult worms or activated L3 were incubated for 7 days or 14 days respectively in serum-free medium, culture supernatants collected and concentrated. Subsequently, 5 ug of secreted products (SP) or worm extract (Ex) were analysed for the presence of DNase II by western blot. (C) Non-activated L3 do not readily secrete DNase II. Freshly isolated or activated L3 (200 worms) were incubated in 100 µl of serum-free medium containing 2 µg of plasmid DNA for 15 min to 18 hours (overnight, ON) at 37°C. Supernatants were collected at various time points and assessed for plasmid DNA degradation by resolution of DNA fragments on 1% agarose gels.
Generation of extracellular vesicles containing DNase II-ribonucleoprotein complexes or a single stranded oligodeoxynucleotide
The genomic DNA sequence of DNase II was identified in NBR_scaffold_0001590 following alignment of the published cDNA sequence (GenBank: M938457) (Bouchery et al., 2020) to the genomic N. brasilensis database PRJEB511 available on WormBase ParaSite. Nine exons were defined. Exon 3 and exon 7 both encode HxK motifs; this is characteristic of DNase II as the two motifs are required to form the active site (Schafer et al., 2007) (Figure 2A). Notably, while the corresponding amino acid sequence derived from NBR_scaffold_0001590 and M938457 was mainly conserved, some differences were detected in the cDNA sequences which could affect the prediction of effective sgRNAs (Supplementary Figures 1A, B). Expression of NB_Dnase2_1590 was confirmed in our laboratory strain of N. brasiliensis following cloning of the cDNA sequence into a yeast expression vector and subsequent sequencing such that sgRNA design was based on NB_Dnase2_1590.
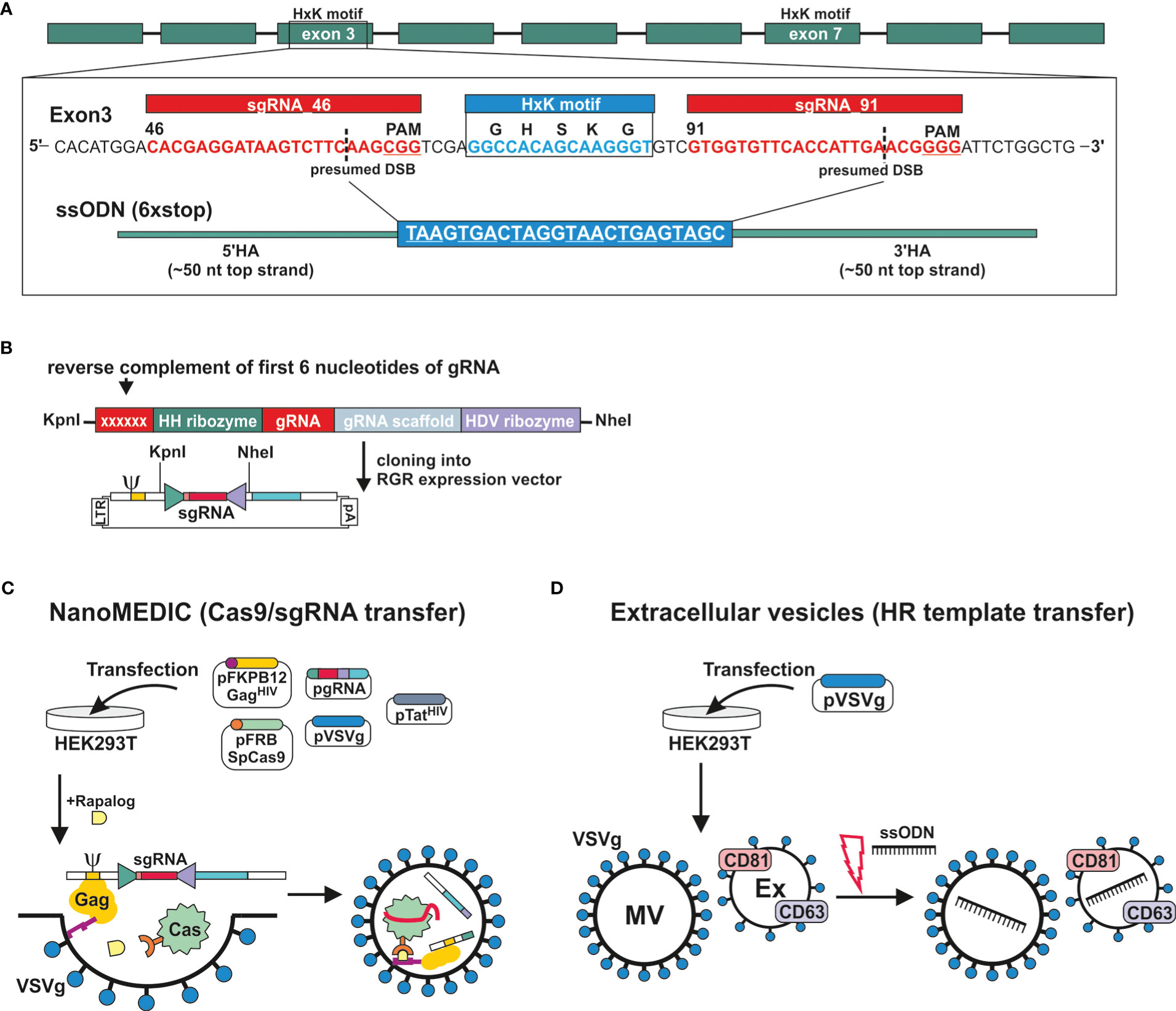
Figure 2 Generation of extracellular vesicles carrying ribonucleoprotein complexes or a homology directed repair template. (A) Gene model of dnase2 (NBR_00001590) showing the position of its nine exons, eight introns and the location of the two HxK motifs. Inset of exon three: nucleotide sequence of the (+) strand indicating location and sequence of gRNA target sites (red), protospacer adjacent motifs (PAM, underlined), the presumed double-stranded breaks (DSB) (dashed line, and the 119-nucleotide sequence of the single-stranded DNA donor template provided for DSB repair by homologous recombination. Homology arms (HA) of 45 or 50 nt flank the central 24 nt of a six-stop-codon transgene. (B) Small guide (sg)RNA sequences were cloned between the self-cleaving Hammerhead (HH) and hepatitis delta virus (HDV) ribozymes of the transfer plasmid (ribozyme-guide-ribozyme, RGR) encoding a virus-like mRNA with a packaging signal sequence (Ψ). The reverse complement of the first 6 nucleotides of the sgRNA are required to form the distinct secondary structure required for the catalytic activity of the hammerhead ribozyme, and determines its cleavage site. (C) VSV-G-pseudotyped EVs containing Cas9/gRNA complexes (NanoMEDIC) were produced in HEK293T cells following transfection. (Sp)Cas9 is selectively packaged into extracellular vesicles by chemical-induced dimerisation. This is mediated by the FKB12 and FRB (rapamycin-binding domain) protein dimerisation system. Cas9 is modified with FRB, whereas FKB12 is fused to HIV Gag protein, and addition of the rapalog ligand A/C mediates heterodimerisation of FRB-Cas9 with FKB12-Gag and packaging into budding extracellular vesicles. The sgRNA encoded in virus-like mRNA is liberated in NanoMEDIC following cleavage by two self-cleaving ribozymes and incorporated into Cas9. (D) For the HDR template transfer, EVs were produced by VSV-G-expressing HEK293T cells. EVs consisting of microvesicles (MV) and exosomes (Ex) were then loaded with HDR templates by electroporation.
For transient delivery of Cas9/gRNA complexes, we adapted a recently developed NanoMEDIC approach established in mammalian cells using VSV-G-pseudotyped extracellular vesicles (Gee et al., 2020). To predict sgRNA target sequences, the Cas-Designer (RGEN) algorithm was used, which allows for off-target screening against the N. brasiliensis genome (Bae et al., 2014a; Park et al., 2015). We chose exon 3 as a target region as it encodes one of the two HxK motifs of functional DNases (Schafer et al., 2007) (Figure 2A). While some gRNAs were predicted that covered the HxK region, these did not achieve a frameshift prediction score over 66, which is recommended in order to minimise the possibility of unwanted in-frame deletions (Bae et al., 2014b). The two highest scoring gRNAs, guide 46 and 91, flanking the HxK motif in exon 3, were cloned between the self-cleaving Hammerhead (HH) and hepatitis delta virus (HDV) ribozymes of the transfer plasmid (ribozyme-guide-ribozyme, RGR) (Figure 2B). If the deletion was insufficient or frameshift not achievable using either guide, then the EV approach would allow for multiplexing to include both guide RNAs deleting the entire HxK motif coding sequence. Corresponding VSV-G-pseudotyped EVs containing RNP complexes (NanoMEDIC) were produced in HEK293T cells following transfection with the RGR and four packaging plasmids encoding Cas9, Gag, VSV-G and Tat (Figure 2C) (Gee et al., 2020).
The NanoMEDIC delivery system utilises two distinct homing mechanisms to recruit Cas9 and a sgRNA into extracellular vesicles in HEK293 producer cells. Streptococcus pyogenes (Sp)Cas9 is selectively packaged into extracellular vesicles by chemical-induced dimerisation. This is mediated by the FKB12 and FRB (rapamycin-binding domain) protein dimerisation system (Putyrski and Schultz, 2012). Cas9 is modified with FRB, whereas FKB12 is fused to HIV Gag protein, and addition of the rapalog (rapamycin analogue) ligand A/C mediates heterodimerisation of FRB-Cas9 with FKB12-Gag and packaging into budding extracellular vesicles. Long terminal repeat (LTR)-driven transcription of the RGR transfer plasmid produces a virus-like mRNA encoding the sgRNA and containing a packaging signal sequence (Ψ, Figure 2B) which directs RNA incorporation into budding vesicles. The sgRNA encoded in virus-like mRNA is liberated in NanoMEDIC by self-cleavage of the flanking ribozymes. Dissociation of the complexes occurs after dilution of the A/C heterodimerizer once the vesicles fuse with recipient cell membranes, in this case those of N. brasiliensis.
To test whether homology directed repair (HDR) could be achieved following induction of double strand breaks, we generated a single-strand oligonucleotide (ssODN) template encoding a series of 6 stop codons interspaced by single nucleotides to allow for all possible open reading frames and ~50 nt homology arms (Figure 2A), as previously described (Ittiprasert et al., 2019). The introduction of premature stop codons allows for degradation of modified transcripts by nonsense-mediated decay and/or premature termination of translation (Hug et al., 2016). Overexpression of VSV-G in HEK293T cells leads to an increased production of VSV-G-expressing EVs, termed ‘gesicles’, that can be utilised for transfer of membrane, cytoplasmic and nuclear proteins (Mangeot et al., 2011). We therefore loaded VSV-G-pseudotyped EVs with the ssODN template and tested whether they could be transferred to N. brasiliensis L3 (Figure 2D) (Lamichhane et al., 2015; Meyer et al., 2017).
NanoMEDIC in conjunction with an extracellular vesicle-delivered homology directed repair template induces site directed mutagenesis in Nippostrongylus infective larvae
In our first series of experiments, activated L3 were exposed to NanoMEDIC containing sgRNAs binding at nucleotide 46 and/or 91 of exon 3 in the presence or absence of EVs containing the STOP_ssODN for HDR. Parasite genomic DNA was then assessed by PCR for modifications introduced by HDR (Figures 3A, B). PCR with primers binding the stop codon region and adjacent exon 2 or 4 led to amplification of expected PCR products (Figure 3C), indicating that NanoMEDIC in conjunction with EV-delivered ssODN templates could be used for site directed mutagenesis in N. brasiliensis. Furthermore, the presence of modified dnase2 transcripts was confirmed by RT-PCR with an exon 2-specific forward primer and a reverse primer binding the 6xSTOP region, resulting in a product of approximately 140 bp (Figure 3D). PCR from genomic DNA with the same primer pair produced an amplicon of approximately 200 bp (Figure 3C). Importantly, the presence of modified cDNA provided evidence that NanoMEDIC and EV-delivered ssODN can reach tissues actively expressing DNase II following ingestion.
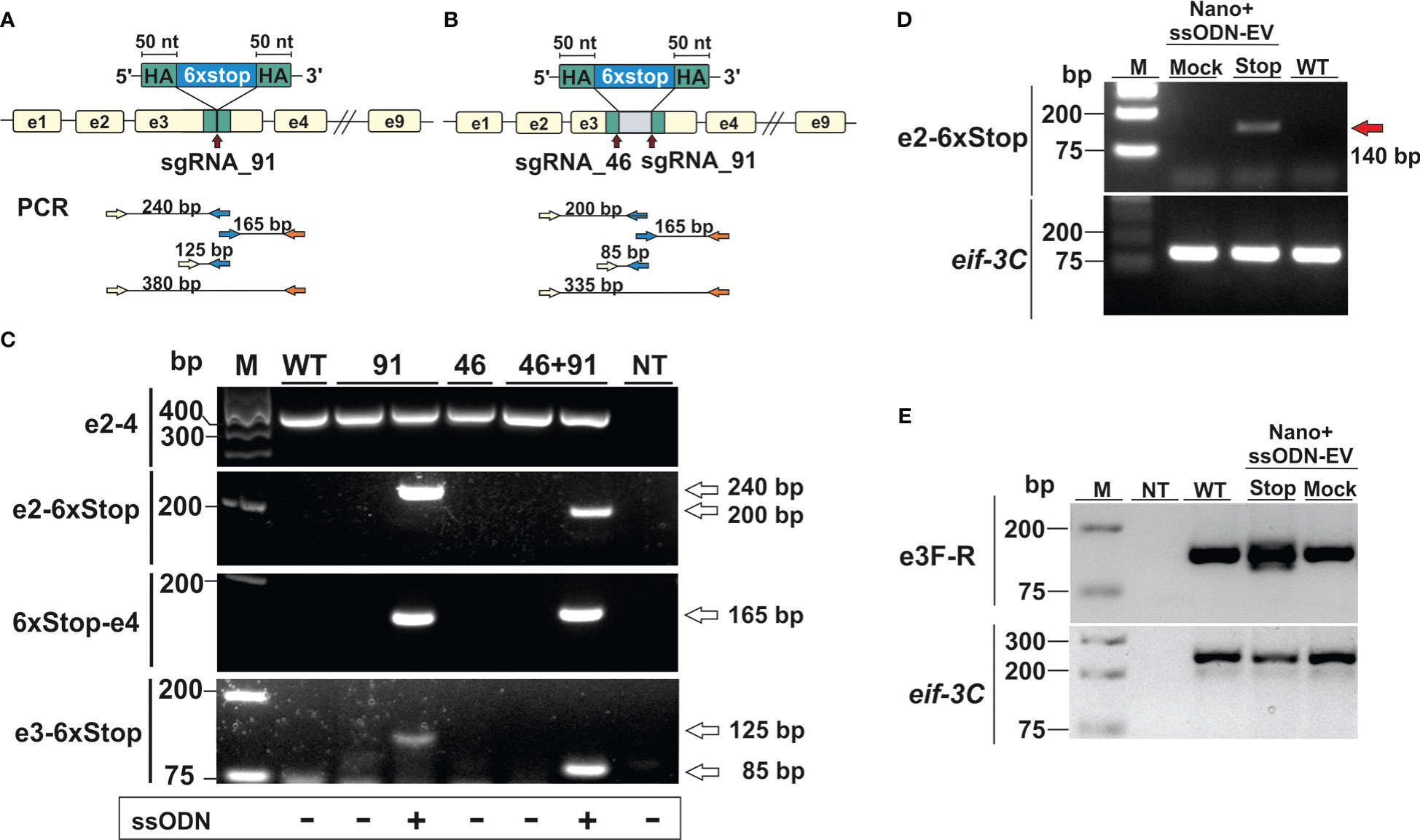
Figure 3 CRISPR/Cas9-mediated gene editing in N. brasiliensis infective larvae following delivery of Cas9/sgRNA complexes and homology directed repair templates via extracellular vesicles. (A, B) Knock-in of a homology directed repair (HDR) template expression cassette following a single (A, sgRNA91) or double (B, sgRNA46 and 91) stranded DNA break. (C) Detection of HDR sequences by PCR analysis of genomic DNA from wild type and edited parasites. Primer pairs were designed to amplify fragments from the adjacent exon (2 or 4) and the HDR region (6xstop), with respective amplicon sizes indicated (A and B). WT, wild type; NT, no template control. (D) Detection of modified dnase2 transcripts by RT-PCR with an exon2-specific forward and HDR-specific reverse primer, resulting in a ~140 bp product. (E) PCR of exon 3 with primers flanking the HDR integration site. Mock EVs were electroporated with a single-strand oligonucleotide encoding an irrelevant sequence of the same length as the HDR template. Eif-3C was amplified to control for genomic DNA and RNA integrity. WT, wild type; NT, no template control.
Non-homologous end joining does not lead to effective mutations in infective larvae
In previous studies with Strongyloides stercoralis, non-homologous end joining (NHEJ) of CRISPR/Cas9-induced double-strand breaks following microinjection of RNP complexes into the gonad resulted in large deletions, while smaller insertions or deletions (indels) appeared to be absent. Similarly, PCR of genomic DNA carried out with an exon 2-binding forward and an exon 4-binding reverse primer resulted in a single fragment of ~350 bp in all samples tested, which could not resolve possible indels (Figure 3C). To compensate for the possibility that deletions were insufficient, or frameshift not achievable using either guide, further experiments were carried out using a combination of both guide RNAs, with or without the addition of a ssODN allowing for deletion of the entire motif. To facilitate detection of smaller changes, a further PCR was carried out with primers flanking the double stranded break sites of exon 3 (Figure 3E). While multiple PCR fragments were generated in the NanoMEDIC + 6xSTOP_ssODN sample indicating integration of the HDR template, PCR with exon 3-binding forward and reverse primers still failed to resolve possible indels in worms exposed to NanoMEDIC (Figure 3E).
To examine potential low frequency errors induced by CRISPR/Cas9-mediated editing, we performed deep sequencing of PCR products derived from worms transfected with CRISPR/Cas9 either with or without the repair template. In worms transfected with CRISPR/Cas9 alone, after removing potential sequencing errors and PCR artefacts (see methods) single nucleotide polymorphisms (SNPs) at low frequency were detected mapping to the wild type allele of the DNase II gene, indicating that the enzyme could introduce breaks triggering error prone repair (Figure 4A). Interestingly, addition of the repair template led to an altered pattern of mutations in the wild type allele, without changing the frequency (Figure 4B). Similar patterns were seen for small indels mapping to the wild type allele (Figures 4C, D). However, the total frequency of SNPs and indels mapping to the edited allele was extremely low and similar to the frequency of mutations mapping to the wild type allele (Figures 4E, F), confirming that the homology directed repair was mostly accurate.
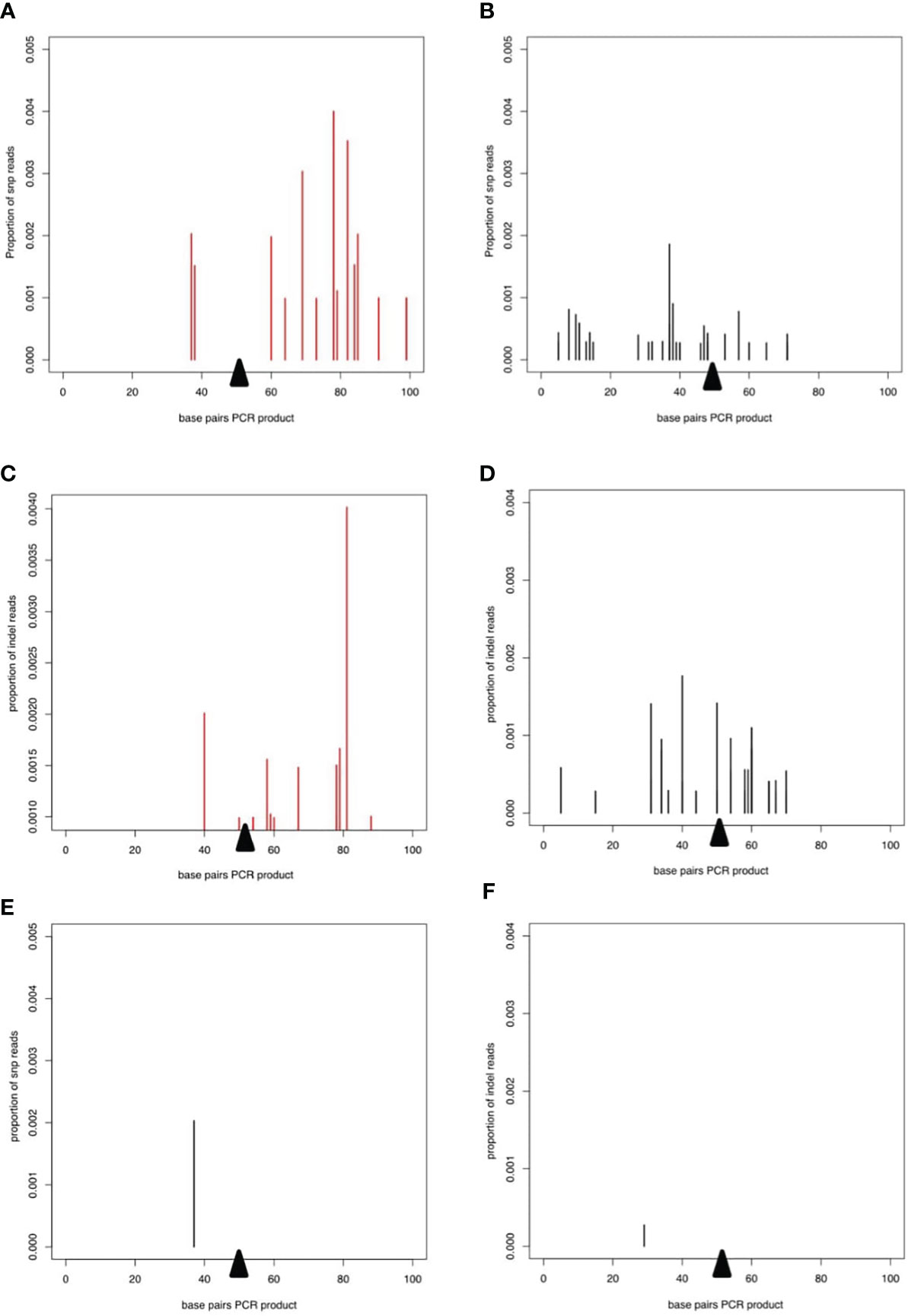
Figure 4 Deep sequencing of PCR product from worms transfected with CRISPR/Cas9 guide RNA complexes. In all plots, the proportion of reads corresponding to SNPs or indels is shown on the y axis and the presumed breakpoint is indicated by a black triangle. (A, B) SNPs on the WT allele after transfection with the CRISPR/Cas9 guide RNA complex without (A) or with (B) the repair template. (C) and (D) Indels on the WT allele for CRISPR/Cas9 guide RNA complex without (C, D) the repair template. (E) SNPs and (F) indels mapping to the repair template after transfection with the CRISPR/Cas9 guide RNA complex and the repair template.
Diminished DNase activity in CRISPR/Cas9 mutated larvae
We next assessed whether modifications introduced into the DNase II gene resulted in measurable reduction of secreted enzyme. Reduced DNase activity was observed in supernatants collected for 3 days following exposure of activated L3 to NanoMEDIC and ssODN (Figure 5A). While complete degradation of donor DNA was observed 5 min after exposure to supernatant from control worms, this required longer (10 min) incubation with supernatant of worms exposed to NanoMEDIC and the STOP_ssODN. Delayed DNA degradation was only observed following delivery of the STOP_ssODN via VSV-G-EVs (ssODN-EV), while direct electroporation of NanoMEDIC with the STOP_ssODN (Nano+ssODN) was unsuccessful. Reduced nuclease activity was also not observed in supernatants from worms exposed to NanoMEDIC and Mock-EVs electroporated with a ssODN encoding an irrelevant sequence of the same length as the HDR template.
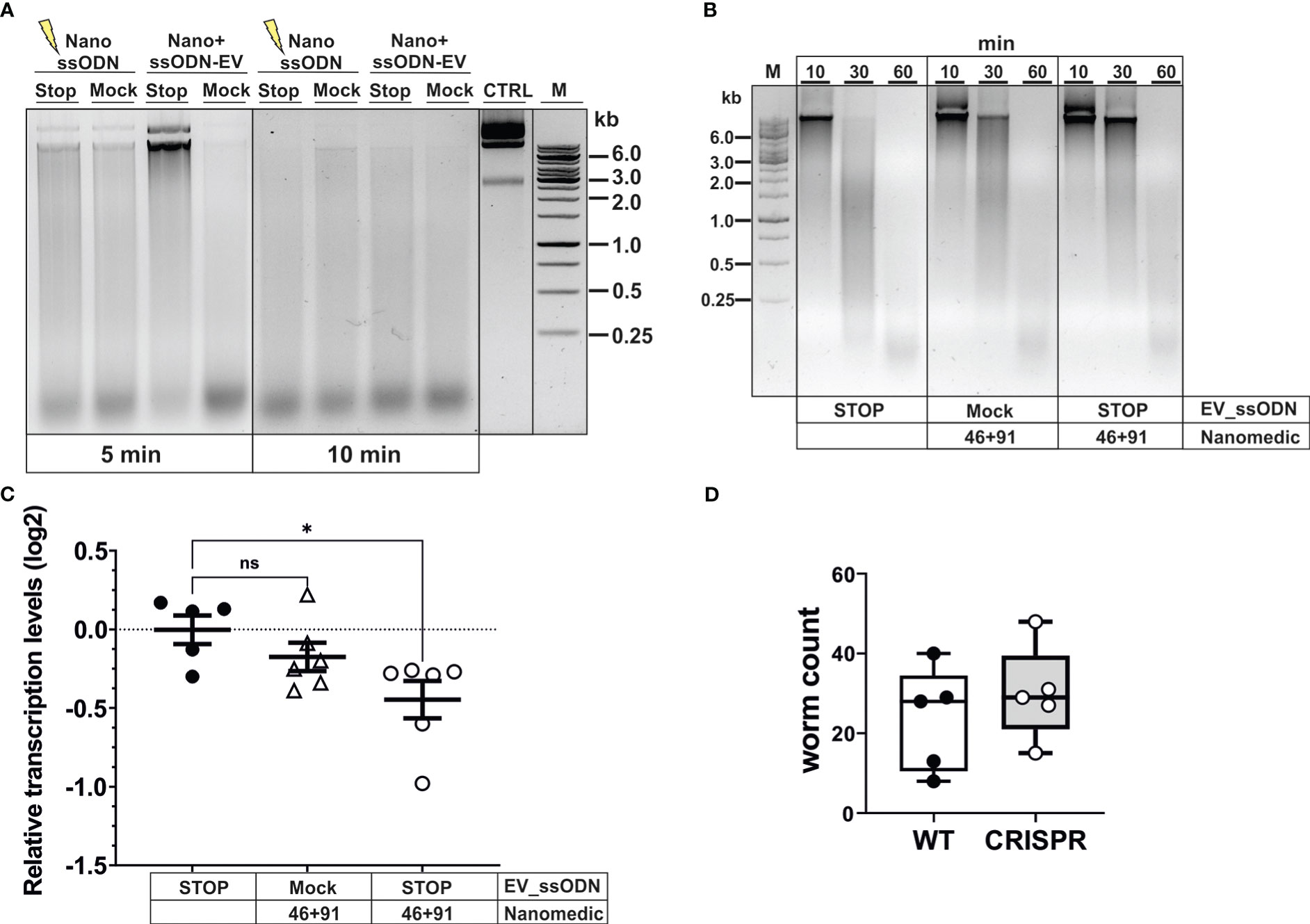
Figure 5 Editing of dnase2 results in reduced enzyme activity in larval secreted products. (A) Reduced DNase activity in larval secreted products. Activated L3 were exposed to EVs for 18 hours, washed and incubated for a further 48 hours. The HDR template was either electroporated into NanoMEDIC (NanossODN) or VSV-G-pseudotyped EVs (ssODN-EV). Mock EVs were electroporated with a ssODN encoding an irrelevant sequence of the same length as the HDR template. Larval secreted products were collected and assessed for DNase activity as described in Materials and methods. Undigested plasmid DNA (CTRL) was resolved on gels with test samples. (B) Time course of delayed DNA degradation by secreted products from modified worms. Activated L3 were exposed to EVs for 18 hours then incubated with plasmid DNA for 10, 30 or 60 min and supernatants analysed for DNA degradation by gel electrophoresis. (C) Downregulation of secreted dnase2 transcripts in activated L3s after exposure to EVs. Transcript levels were assessed by RT-qPCR three days after transduction relative to wild type control larvae and normalised against the geometric mean of Ct values of reference genes eif-3C and idhg-1. Scatter plot with the mean ± sem of data from 2 independent experiments with 3 biological replicates each consisting of ~2,000 larvae. Treatment groups were analysed for significant differences with the Kruskal-Wallis test and Dunns post-hoc test in relation to the wild type. *Statistical significance: p<0.05. ns, not significant. (D) Reduction of DNase II secretion did not lead to lower parasite recovery in mice. Activated L3 were exposed to EVs for 18 hrs, washed and used to infect BALB/c mice. Adult worms were recovered from the intestines at day 5 post-infection and counted.
To assess the timeframe of delayed DNA degradation by modified worms, secreted products were assessed for their nuclease activity by adding a substrate plasmid DNA to the culture medium alongside the worms and following degradation over time (Figure 5B). No intact DNA was detected after 30 min, and complete degradation recorded 60 min after culture with worms exposed to ssODN_EVs only. In contrast, co-culture of NanoMEDIC-exposed worms revealed the presence of some intact plasmid DNA after 30 min. This was more pronounced when worms were exposed to NanoMEDIC + ssODN_EVs, with some larger DNA fragments still present 60 min after co-culture (Figure 2B). Furthermore, RT-qPCR revealed a reduction in dnase2 transcript levels by ~25% (mean log2 ± SEM = -0.45 ± 0.12) in NanoMEDIC + ssODN_EV samples, compared to the ssODN_EV only group (-0.003 ± 0.09) (p=0.048, Kruskal-Wallis test with Dunn’s post-hoc; Figure 5C), indicating nonsense-mediated decay of modified transcripts. No significant decrease of dnase2 transcripts (-0.17 ± 0.09) was recorded in the NanoMEDIC-only group (p=0.74). These data, together with PCR analysis of genomic DNA, indicate that the majority of NanoMEDIC-induced gene disruption can be repaired by non-homologous end joining, and that editing is enhanced by homology-directed repair.
Because N. brasiliensis DNase II has been demonstrated to degrade neutrophil extracellular traps, it has been suggested to facilitate migration of L3 through the skin and lung tissues of their mammalian host (Bouchery et al., 2020). The moderate silencing of the dnase2 gene achieved in this study did not lead to a reduction in worm numbers in the intestines of infected mice (Figure 5D). Nevertheless, these data provide proof of principle that CRISPR/Cas9-induced gene editing can be achieved in infective larvae of N. brasiliensis by harnessing extracellular vesicle-mediated delivery of RNPs and HDR templates, providing a new route for genetic manipulation of parasitic nematodes.
Expression of a NUC-1 orthologue by Nippostrongylus brasiliensis
As reduction of secreted DNase II activity did not result in altered recovery of adult worms from infected mice, we hypothesised that additional DNase II activities may be encoded in the parasite genome providing some degree of redundancy. A search of WormBase ParaSite revealed that NBR_0000088201 encoded an orthologue of lysosomal DNase II from Caenorhabditis elegans termed NUC-1 (Hedgecock et al., 1983; Wu et al., 2000). The predicted amino acid sequence is shown in Figure 6A, aligned with that of the N. brasiliensis secreted DNase II (NBR_00001590) (Bouchery et al., 2020) and C. elegans NUC-1, revealing 65% identity between the mature N. brasiliensis and C. elegans NUC-1 proteins. Examination of transcript levels by real-time RT-PCR (qPCR) revealed that Nb_nuc-1 is expressed at fairly constant levels through to adult worms (Figure 6B). Transcript levels for the secreted DNase II were 10-fold higher than Nb_nuc-1 in activated L3, and at least 10-fold lower than Nb_nuc-1 in resting L3 and adult worms (Figure 6C). This suggests that Nb_nuc-1 has more of a housekeeping role, consistent with a lysosomal function, and it is notable that no nuclease activity was detected in culture supernatants of L3 within the first 2 hours following activation (Figure 1C) despite appreciable levels of Nb_nuc-1 transcripts. In contrast, expression of the secreted DNase II was almost exclusively associated with larval activation, suggesting that this is the major or sole enzyme released into the mammalian host and primarily responsible for degradation of extracellular/environmental DNA in the early stages of infection.
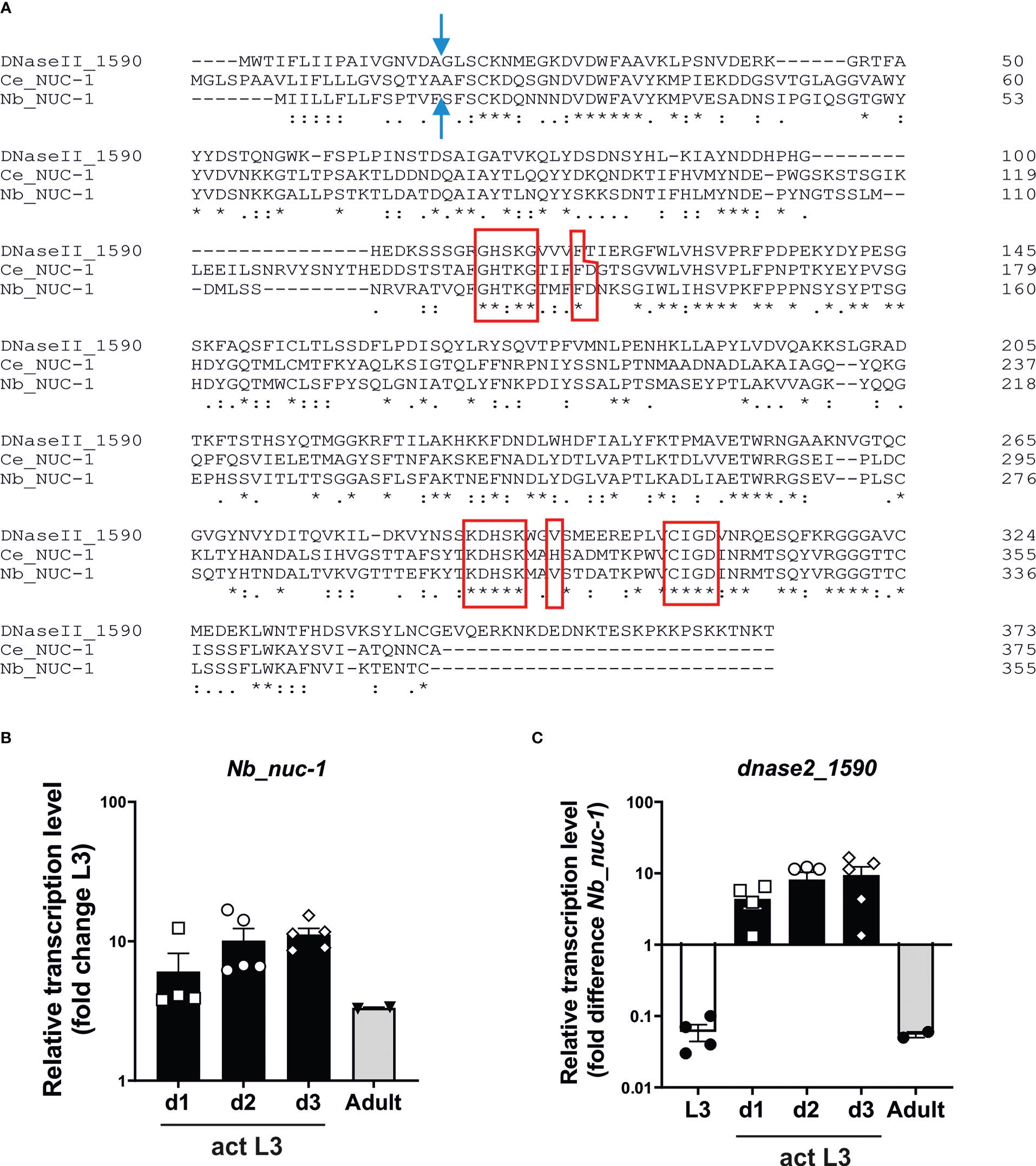
Figure 6 Detection of a nuc-1 orthologue in Nippostrongylus brasiliensis. (A) Amino acid sequence of Nb_NUC-1 (NBR_0000088201) inferred by orthology to C. elegans NUC-1. Signal peptide cleavage site (blue arrow) and HKD motifs are indicated (red border). (B) Nb_nuc-1 is upregulated in L3 following activation at 37°C. Transcript levels are shown relative to unactivated L3. (C) Dnase2_1590 transcript levels relative to Nb_nuc-1 in different life cycle stages and culture of L3 at 37°C up to 3 days. Interleaved scatter plot with bars and mean ± sem of 2 independent experiments with 2-3 biological replicates. * indicates identity of amino acids in alignment.
Discussion
Nanoparticles, including EVs, have become an important tool for delivery of cargo including drugs, proteins, and nucleic acids to mammalian cells both in vitro and in vivo. EVs released by cells include microvesicles, exosomes, and apoptotic bodies, which differ in their biogenesis and size. However, while EVs have been described in secreted products of many helminths and suggested to be involved in signalling to the host (Drurey and Maizels, 2021), utilisation as vehicles to deliver functional cargo to the parasites remains unexplored.
Here, we demonstrate that CRISPR/Cas9-mediated gene editing of N. brasiliensis is achievable using VSV-G-pseudotyped EVs (NanoMEDIC) for the delivery of RNP complexes. NanoMEDIC induced DNA double strand breaks, and homology directed repair was achieved through simultaneous delivery of a ssODN template via VSV-G-pseudotyped EVs (Figure 1B). Edited transcripts were detected by RT-PCR, demonstrating that EVs can access cells of tissues actively expressing the gene of interest. Furthermore, gene disruption and the introduction of a repair template encoding 6 stop codons led to measurable reduction of target gene expression (dnase2), demonstrating that the technique can be employed for the identification and characterisation of molecules in parasites involved in disease processes. Deep sequencing of PCR products from populations of edited worms and alignment to the predicted edited locus (which would have identical sequence to the recombination cassette) or to the wild type locus indicated that almost all the reads which mapped to the edited allele did so perfectly (Figure 4). This means that the process of insertion that occurs during CRISPR targeting must have a very high accuracy, i.e. it almost always inserts an exact copy of the repair template rather than one with a mutation in it.
As in previous studies with Strongyloides stercoralis (Gang et al., 2017), in the absence of a HDR template we could not detect smaller insertions or deletions following NHEJ. However, large genetic deletions of up to 500 bp in length following NHEJ have been described in S. stercoralis (Gang et al., 2017) and Schistosoma mansoni (Sankaranarayanan et al., 2020). Due to the PCR design in our study, with primers spanning exon 2 to exon 4 of the DNase II gene, the presence of larger deletions cannot be excluded. However, detection of large deletions by whole genome sequencing may be challenging, as targeting tissues with direct access to EVs will result in mosaic genomes. The high abundance of wild type alleles masking edited genes has also been an issue in S. stercoralis (Gang et al., 2017). While not reaching significance in our readouts so far, some reduction in transcript and DNase activity was observed in the absence of HDR, such that optimisation of NanoMEDIC production and purity may lead to improved gene disruption by NHEJ. However, consistent with previous studies in other species (Gang et al., 2017; Ittiprasert et al., 2019), gene disruption is more effective when HDR is employed, and it facilitates detection of edited genes by providing unique primer binding sites. Interestingly, while a ssODN template was sufficient to achieve HDR in N. brasiliensis L3 in this study, a double stranded DNA template was necessary for repair in S. stercoralis following injection of the gonad (Gang et al., 2017). This may be indicative of different repair mechanisms in somatic and germline cells, as previously described in C. elegans (van Schendel et al., 2015).
Direct injection into the gonad has the advantage of potentially generating homozygous offspring, whereas NanoMEDIC targets tissues with direct access to EVs. The EVs used in this study are similar in size to lentiviral particles (~150-200 nm) and possess a cell membrane-derived envelope expressing VSV-G. Lentiviral particles can access cells in the intestine of N. brasiliensis L3, and interference with expression of secreted acetylcholinesterases suggests that they can also access subventral glands (Hagen et al., 2021). This is important, as it suggests that NanoMEDIC may be utilised for targeting tissues expressing secreted proteins, as shown here for the DNAse II. The same study showed that lentiviral particles can gain access to the germline, as integrated viral genomes were evident in a small proportion of the F1 generation (Hagen et al., 2021). The route to the germline is unclear, and further studies are required to determine whether this is similarly possible with NanoMEDIC. If so, then this introduces the possibility of generating stable homozygous gene-edited parasite lines, which may be necessary for robust interrogation of gene function.
Although we have shown that N. brasiliensis could be transduced with lentivirus, the transgene expression cassettes were subject to epigenetic silencing, and RNAi could not be maintained following development to adult stages (Hagen et al., 2021). CRISPR/Cas9-mediated gene editing offers a means to circumvent these problems, as more stable expression should be possible by site-directed integration of transgenes into regions less prone to epigenetic silencing. Lentiviral delivery of a Cas9 expression cassette has been superseded in S. mansoni by lipofection of in vitro assembled RNP complexes and simultaneous delivery of an HDR template by electroporation (Ittiprasert et al., 2019). Furthermore, RNP complexes outperformed plasmid DNA-encoded Cas9 expression cassettes in Strongyloides (Gang et al., 2017). Unlike Strongyloides, most parasitic nematodes do not have a free-living phase to facilitate microinjection. Using EVs for delivery of pre-assembled RNP complexes (Montagna et al., 2018; Campbell et al., 2019; Mangeot et al., 2019; Gee et al., 2020) and HDR templates (Lamichhane et al., 2015) thus offers an alternative to techniques employed thus far, and pseudotyping EVs with VSV-G allows for receptor-mediated uptake similar to viral transduction (Montagna et al., 2018; Campbell et al., 2019; Mangeot et al., 2019; Gee et al., 2020).
Due to their short half-life, RNPs are rapidly degraded, resulting in precise site-directed editing with low off-target frequencies (Vakulskas and Behlke, 2019). Another major advantage is that RNPs are produced and assembled in mammalian cell lines for which optimised expression systems are readily available, avoiding lengthy optimisation of Cas9 expression cassettes for expression in the respective parasite. Use of mammalian cell lines facilitates cost effective, large scale production of EVs. Furthermore, in contrast to other vesicular delivery approaches relying on stochastic uptake of RNPs (Mangeot et al., 2019) or fusion of SpCas9 to Gag (van Schendel et al., 2015), NanoMEDIC utilise the Gag and FRB-FKB12 homing system to actively incorporate RNPs into budding EVs, and contain an average of 7 Cas9 molecules per vesicle (Gee et al., 2020). Moreover, VSV-G and Gag actively mediate the release of EVs from cells resulting in average titres of approximately 1 x 1010 particles per ml in our studies (Supplementary Figure S2).
Further optimisation of preparation and delivery of EVs will be necessary to improve editing efficiencies. Nanoflow analysis showed that NanoMEDIC preparations contained up to 90% exosomes (Supplementary Figure S2). Concentration in spin columns resulted in slightly less exosome (vesicles <100 nm) content than polymer-based precipitation (Supplementary Figure S2). NanoMEDIC have an average size of ~150 nm, and DNA is predominantly taken up by microvesicles (~150 nm) on electroporation (Lamichhane et al., 2015). The high content of exosomes is likely to saturate available receptors and impair effective uptake by NanoMEDIC. Polymer-based precipitation results in large quantities of lipids which may compete with available LDL receptors. Improved purity may thus require affinity chromatography (Gee et al., 2020) or a combination of filter columns (Brennan et al., 2020).
In addition to loss of gene function by gene disruption, CRISPR/Cas9 provides a means to integrate foreign genes and expression cassettes via HDR (Chu et al., 2015). Introduction of a traceable reporter or tag would allow sorting or enrichment of mutant larvae, which would aid in generating stable edited parasite lines. It would also help to define the limitations of the EV delivery system in terms of which tissues can be accessed and manipulated, and facilitate investigation of gene expression patterns. For such studies, targeting a constitutively expressed, common gene such as tubulin, might be more effective. Introduction of a reporter would require delivery of a dsDNA donor. Utilisation of a dsDNA template might allow editing in a wider range of tissues and improve HDR efficiencies as dsDNA templates usually have longer homology arms. Delivery of dsDNA via EVs is limited by their loading capacity, with an optimal length of DNA up to 750 bp but not exceeding 1000 bp (Lamichhane et al., 2015), although loading capacities might be improved through optimisation of electroporation conditions (Lamichhane et al., 2015; Lennaard et al., 2021).
In summary, we have demonstrated that EVs can be utilised as a vehicle to deliver functional cargo to a parasitic nematode and achieve CRIPSR/Cas9-mediated gene editing. Although the methodology clearly needs further development and optimisation in order to rigorously interrogate gene function, it provides a new route for genetic manipulation of this important group of pathogens and should be applicable to a wide range of species.
Data availability statement
The data presented in the study are deposited in the NCBI SRA (Sequence Read Archive) repository, accession number PRJNA907367.
Ethics statement
The animal study was reviewed and approved by Animal Welfare Ethical Review Board at Imperial College London.
Author contributions
Conceived and designed the experiments: JH, MS, PS. Performed the experiments: JH, SG, MS, PS. Analyzed the data: JH, PS, MS. Wrote the paper: JH, MS, PS. All authors contributed to the article and approved the submitted version.
Funding
This study was funded by a BBSRC grant to MES, PS and JH (BB/S001085/1).
Conflict of interest
The authors declare that the research was conducted in the absence of any commercial or financial relationships that could be construed as a potential conflict of interest.
Publisher’s note
All claims expressed in this article are solely those of the authors and do not necessarily represent those of their affiliated organizations, or those of the publisher, the editors and the reviewers. Any product that may be evaluated in this article, or claim that may be made by its manufacturer, is not guaranteed or endorsed by the publisher.
Supplementary material
The Supplementary Material for this article can be found online at: https://www.frontiersin.org/articles/10.3389/fpara.2023.1071738/full#supplementary-material
Supplementary Figure 1 | Alignment of cDNA and derived amino acid sequences for MN938457.1 (GenBank) and NBR_00001590 (Wormbase ParaSite). (A) cDNA sequence alignment. (B) Derived amino acid sequence alignment
Supplementary Figure 2 | Analysis of extracellular vesicle preparations. (A) Analysis of extracellular vesicle production by western blot. Extracellular vesicles (EV) or NanoMEDIC (Nano)-containing cell supernatants were concentrated using vivaspin columns (VIVA) or precipitation with Lenti-X concentrator (LX) as described in Materials and methods. Western blotting was performed following SDS-PAGE under reducing conditions to determine the presence of Cas9 and VSV-G, or under non-reducing conditions for the presence of CD63 and CD81. (B) Analysis by Nano-flow cytometry. NanoMEDIC preparations concentrated by ultrafiltration (VIVAspin) or precipitation (Lenti-X) were analysed for their nanoparticle content by nano-flow cytometry as described in Materials and methods. The table shows the concentration and proportion of particles with diameters larger than 100 nm.
Supplementary Figure 3 | Guide RNA-encoding region synthesised for cloning into RGR plasmid and ssODN sequences for homology directed repair. Key: Red: guide RNA target sequence; Yellow: reverse complement of first 6 nucleotides of target; Blue: Hammerhead ribozyme; Green: Hepatitis Delta virus ribozyme; Lower case: restriction endonuclease sites.
References
Bae S., Kweon J., Kim H. S., Kim J. S. (2014b). Microhomology-based choice of Cas9 nuclease target sites. Nat. Methods 11 (7), 705–706. doi: 10.1038/nmeth.3015
Bae S., Park J., Kim J. S. (2014a). Cas-OFFinder: a fast and versatile algorithm that searches for potential off-target sites of Cas9 RNA-guided endonucleases. Bioinformatics 30 (10), 1473–1475. doi: 10.1093/bioinformatics/btu048
Bouchery T., Moyat M., Sotillo J., Silverstein S., Volpe B., Coakley G., et al. (2020). Hookworms evade host immunity by secreting a deoxyribonuclease to degrade neutrophil extracellular traps. Cell Host Microbe 27 (2), 277–289 e276. doi: 10.1016/j.chom.2020.01.011
Brennan K., Martin K., FitzGerald S. P., O'Sullivan J., Wu Y., Blanco A., et al. (2020). A comparison of methods for the isolation and separation of extracellular vesicles from protein and lipid particles in human serum. Sci. Rep. 10 (1), 1039. doi: 10.1038/s41598-020-57497-7
Campbell L. A., Coke L. M., Richie C. T., Fortuno L. V., Park A. Y., Harvey B. K. (2019). Gesicle-mediated delivery of CRISPR/Cas9 ribonucleoprotein complex for inactivating the HIV provirus. Mol. Ther. 27 (1), 151–163. doi: 10.1016/j.ymthe.2018.10.002
Castelletto M. L., Gang S. S., Hallem E. A. (2020). Recent advances in functional genomics for parasitic nematodes of mammals. J. Exp. Biol. 223 (Pt Suppl 1), 1–11. doi: 10.1242/jeb.206482
Chen Y., Ott C. J., Townsend K., Subbaiah P., Aiyar A., Miller W. M. (2009). Cholesterol supplementation during production increases the infectivity of retroviral and lentiviral vectors pseudotyped with the vesicular stomatitis virus glycoprotein (VSV-G). Biochem. Eng. J. 44 (2-3), 199–207. doi: 10.1016/j.bej.2008.12.004
Chu V. T., Weber T., Wefers B., Wurst W., Sander S., Rajewsky K., et al. (2015). Increasing the efficiency of homology-directed repair for CRISPR-Cas9-induced precise gene editing in mammalian cells. Nat. Biotechnol. 33 (5), 543–548. doi: 10.1038/nbt.3198
Drurey C., Maizels R. M. (2021). Helminth extracellular vesicles: Interactions with the host immune system. Mol. Immunol. 137, 124–133. doi: 10.1016/j.molimm.2021.06.017
Finkelshtein D., Werman A., Novick D., Barak S., Rubinstein M. (2013). LDL receptor and its family members serve as the cellular receptors for vesicular stomatitis virus. Proc. Natl. Acad. Sci. U.S.A. 110 (18), 7306–7311. doi: 10.1073/pnas.1214441110
Gang S. S., Castelletto M. L., Bryant A. S., Yang E., Mancuso N., Lopez J. B., et al. (2017). Targeted mutagenesis in a human-parasitic nematode. PloS Pathog. 13 (10), e1006675. doi: 10.1371/journal.ppat.1006675
Gee P., Lung M. S. Y., Okuzaki Y., Sasakawa N., Iguchi T., Makita Y., et al. (2020). Extracellular nanovesicles for packaging of CRISPR-Cas9 protein and sgRNA to induce therapeutic exon skipping. Nat. Commun. 11 (1), 1334. doi: 10.1038/s41467-020-14957-y
Hagen J., Sarkies P., Selkirk M. E. (2021). Lentiviral transduction facilitates RNA interference in the nematode parasite Nippostrongylus brasiliensis. PloS Pathog. 17 (1), e1009286. doi: 10.1371/journal.ppat.1009286
Hedgecock E. M., Sulston J. E., Thomson J. N. (1983). Mutations affecting programmed cell deaths in the nematode Caenorhabditis elegans. Science 220 (4603), 1277–1279. doi: 10.1126/science.6857247
Huang S. C., Chan D. T., Smyth D. J., Ball G., Gounaris K., Selkirk M. E. (2010). Activation of Nippostrongylus brasiliensis infective larvae is regulated by a pathway distinct from the hookworm Ancylostoma caninum. Int. J. Parasitol. 40 (14), 1619–1628. doi: 10.1016/j.ijpara.2010.06.004
Hug N., Longman D., Caceres J. F. (2016). Mechanism and regulation of the nonsense-mediated decay pathway. Nucleic Acids Res. 44 (4), 1483–1495. doi: 10.1093/nar/gkw010
Ittiprasert W., Mann V. H., Karinshak S. E., Coghlan A., Rinaldi G., Sankaranarayanan G., et al. (2019). Programmed genome editing of the omega-1 ribonuclease of the blood fluke, Schistosoma mansoni. Elife 8, e41337. doi: 10.7554/eLife.41337
Jourdan P. M., Lamberton P. H. L., Fenwick A., Addiss D. G. (2018). Soil-transmitted helminth infections. Lancet 391 (10117), 252–265. doi: 10.1016/S0140-6736(17)31930-X
Kaplan R. M. (2020). Biology, epidemiology, diagnosis, and management of anthelmintic resistance in gastrointestinal nematodes of livestock. Vet. Clin. North Am. Food Anim. Pract. 36 (1), 17–30. doi: 10.1016/j.cvfa.2019.12.001
Koboldt D. C., Zhang Q., Larson D. E., Shen D., McLellan M. D., Lin L., et al. (2012). VarScan 2: somatic mutation and copy number alteration discovery in cancer by exome sequencing. Genome Res. 22 (3), 568–576. doi: 10.1101/gr.129684.111
Lamichhane T. N., Raiker R. S., Jay S. M. (2015). Exogenous DNA loading into extracellular vesicles via electroporation is size-dependent and enables limited gene delivery. Mol. Pharm. 12 (10), 3650–3657. doi: 10.1021/acs.molpharmaceut.5b00364
Langmead B., Salzberg S. L. (2012). Fast gapped-read alignment with bowtie 2. Nat. Methods 9 (4), 357–359. doi: 10.1038/nmeth.1923
Lennaard A. J., Mamand D. R., Wiklander R. J., El Andaloussi S., Wiklander O. P. B. (2021). Optimised electroporation for loading of extracellular vesicles with doxorubicin. Pharmaceutics 14 (1), 38. doi: 10.3390/pharmaceutics14010038
Li H., Handsaker B., Wysoker A., Fennell T., Ruan J., Homer N., et al. (2009). The sequence alignment/map format and SAMtools. Bioinformatics 25 (16), 2078–2079. doi: 10.1093/bioinformatics/btp352
Liu C., Grote A., Ghedin E., Unnasch T. R. (2020). CRISPR-mediated transfection of Brugia malayi. PloS Negl. Trop. Dis. 14 (8), e0008627. doi: 10.1371/journal.pntd.0008627
Liu C., Mhashilkar A. S., Chabanon J., Xu S., Lustigman S., Adams J. H., et al. (2018). Development of a toolkit for piggyBac-mediated integrative transfection of the human filarial parasite Brugia malayi. PloS Negl. Trop. Dis. 12 (5), e0006509. doi: 10.1371/journal.pntd.0006509
Livak K. J., Schmittgen T. D. (2001). Analysis of relative gene expression data using real-time quantitative PCR and the 2(-delta delta C(T)) method. Methods 25 (4), 402–408. doi: 10.1006/meth.2001.1262
Mangeot P. E., Dollet S., Girard M., Ciancia C., Joly S., Peschanski M., et al. (2011). Protein transfer into human cells by VSV-g-induced nanovesicles. Mol. Ther. 19 (9), 1656–1666. doi: 10.1038/mt.2011.138
Mangeot P. E., Risson V., Fusil F., Marnef A., Laurent E., Blin J., et al. (2019). Genome editing in primary cells and in vivo using viral-derived nanoblades loaded with Cas9-sgRNA ribonucleoproteins. Nat. Commun. 10 (1), 45. doi: 10.1038/s41467-018-07845-z
Meyer C., Losacco J., Stickney Z., Li L., Marriott G., Lu B. (2017). Pseudotyping exosomes for enhanced protein delivery in mammalian cells. Int. J. Nanomed. 12, 3153–3170. doi: 10.2147/IJN.S133430
Montagna C., Petris G., Casini A., Maule G., Franceschini G. M., Zanella I., et al. (2018). VSV-g-enveloped vesicles for traceless delivery of CRISPR-Cas9. Mol. Ther. Nucleic Acids 12, 453–462. doi: 10.1016/j.omtn.2018.05.010
Park J., Bae S., Kim J. S. (2015). Cas-designer: a web-based tool for choice of CRISPR-Cas9 target sites. Bioinformatics 31 (24), 4014–4016. doi: 10.1093/bioinformatics/btv537
Pfaffl M. W. (2001). A new mathematical model for relative quantification in real-time RT-PCR. Nucleic Acids Res. 29 (9), e45. doi: 10.1093/nar/29.9.e45
Putyrski M., Schultz C. (2012). Protein translocation as a tool: The current rapamycin story. FEBS Lett. 586 (15), 2097–2105. doi: 10.1016/j.febslet.2012.04.061
Quinzo M. J., Perteguer M. J., Brindley P. J., Loukas A., Sotillo J. (2022). Transgenesis in parasitic helminths: a brief history and prospects for the future. Parasit. Vectors 15 (1), 110. doi: 10.1186/s13071-022-05211-z
Sankaranarayanan G., Coghlan A., Driguez P., Lotkowska M. E., Sanders M., Holroyd N., et al. (2020). Large CRISPR-cas-induced deletions in the oxamniquine resistance locus of the human parasite Schistosoma mansoni. Wellcome Open Res. 5, 178. doi: 10.12688/wellcomeopenres.16031.1
Schafer P., Cymerman I. A., Bujnicki J. M., Meiss G. (2007). Human lysosomal DNase IIalpha contains two requisite PLD-signature (HxK) motifs: evidence for a pseudodimeric structure of the active enzyme species. Protein Sci. 16 (1), 82–91. doi: 10.1110/ps.062535307
Selkirk M. E., Huang S. C., Knox D. P., Britton C. (2012). The development of RNA interference (RNAi) in gastrointestinal nematodes. Parasitology 139 (5), 605–612. doi: 10.1017/S0031182011002332
Sotillo J., Sanchez-Flores A., Cantacessi C., Harcus Y., Pickering D., Bouchery T., et al. (2014). Secreted proteomes of different developmental stages of the gastrointestinal nematode Nippostrongylus brasiliensis. Mol. Cell Proteomics 13 (10), 2736–2751. doi: 10.1074/mcp.M114.038950
Vakulskas C. A., Behlke M. A. (2019). Evaluation and reduction of CRISPR off-target cleavage events. Nucleic Acid Ther. 29 (4), 167–174. doi: 10.1089/nat.2019.0790
van Schendel R., Roerink S. F., Portegijs V., van den Heuvel S., Tijsterman M. (2015). Polymerase theta is a key driver of genome evolution and of CRISPR/Cas9-mediated mutagenesis. Nat. Commun. 6, 7394. doi: 10.1038/ncomms8394
Keywords: gene editing, CRISPR/Cas9, nippostrongylus, nematode, extracellular vesicles
Citation: Hagen J, Ghosh S, Sarkies P and Selkirk ME (2023) Gene editing in the nematode parasite Nippostrongylus brasiliensis using extracellular vesicles to deliver active Cas9/guide RNA complexes. Front. Parasitol. 2:1071738. doi: 10.3389/fpara.2023.1071738
Received: 16 October 2022; Accepted: 10 January 2023;
Published: 26 January 2023.
Edited by:
Mohamed A. Dkhil, Helwan University, EgyptReviewed by:
Lamjed Mansour, Carthage University, TunisiaRohit K. Jangra, LSU Health Sciences Center-Shreveport, United States
Copyright © 2023 Hagen, Ghosh, Sarkies and Selkirk. This is an open-access article distributed under the terms of the Creative Commons Attribution License (CC BY). The use, distribution or reproduction in other forums is permitted, provided the original author(s) and the copyright owner(s) are credited and that the original publication in this journal is cited, in accordance with accepted academic practice. No use, distribution or reproduction is permitted which does not comply with these terms.
*Correspondence: Murray E. Selkirk, bS5zZWxraXJrQGltcGVyaWFsLmFjLnVr