- 1Division of Orthodontics, College of Dentistry, The Ohio State University, Columbus, OH, United States
- 2Department of Neural and Pain Sciences, School of Dentistry, University of Maryland Baltimore, Baltimore, MD, United States
- 3Center to Advance Chronic Pain Research, University of Maryland Baltimore, Baltimore, MD, United States
Orthodontic forces are strongly associated with pain, the primary complaint among patients wearing orthodontic braces. Compared to other side effects of orthodontic treatment, orthodontic pain is often overlooked, with limited clinical management. Orthodontic forces lead to inflammatory responses in the periodontium, which triggers bone remodeling and eventually induces tooth movement. Mechanical forces and subsequent inflammation in the periodontium activate and sensitize periodontal nociceptors and produce orthodontic pain. Nociceptive afferents expressing transient receptor potential vanilloid subtype 1 (TRPV1) play central roles in transducing nociceptive signals, leading to transcriptional changes in the trigeminal ganglia. Nociceptive molecules, such as TRPV1, transient receptor potential ankyrin subtype 1, acid-sensing ion channel 3, and the P2X3 receptor, are believed to mediate orthodontic pain. Neuropeptides such as calcitonin gene-related peptides and substance P can also regulate orthodontic pain. While periodontal nociceptors transmit nociceptive signals to the brain, they are also known to modulate alveolar bone remodeling in periodontitis. Therefore, periodontal nociceptors and nociceptive molecules may contribute to the modulation of orthodontic tooth movement, which currently remains undetermined. Future studies are needed to better understand the fundamental mechanisms underlying neuroskeletal interactions in orthodontics to improve orthodontic treatment by developing novel methods to reduce pain and accelerate orthodontic tooth movement—thereby achieving “big gains with no pain” in clinical orthodontics.
1 Introduction
The primary objective of orthodontic treatment is to correct malocclusion by moving teeth within the alveolar bone. Orthodontic tooth movement due to the application of force is often accompanied by side effects such as root resorption, periodontal disease, pulp reaction, and orthodontic pain. Among these, pain is the leading complaint, with 90% of patients affected. Orthodontic pain is also one of the most common reasons for reduced patient compliance and treatment discontinuation (2–4), with approximately 30% of patients having considered stopping treatment due to pain (5). Moreover, orthodontic pain decreases patient health-related quality of life by impairing daily-life activities such as eating and talking (6, 7). Unfortunately, the clinical management of orthodontic pain is unsatisfactory. Orthodontists often recommend treatment with nonsteroidal anti-inflammatory drugs (NSAIDs) and most of the randomized controlled trials studies orthodontic pain compared other interventions with NSAIDs (8), which adversely affect the efficiency of tooth movement (9). Therefore, it is essential to improve our understanding of the mechanisms underlying orthodontic pain at the molecular and cell biology levels to develop new therapies for orthodontic pain that do not interfere with tooth movement.
Orthodontic pain is primarily due to acute inflammation. The self-limiting pain usually occurs approximately four hours after placing the initial archwire, peaks around day one, gradually decreases after three to seven days, and returns to the baseline level in a month (1, 10, 11). During the first week, the patient's quality of life is affected in terms of difficult eating, impaired talking, and oral ulcers. Once orthodontic forces are applied to the teeth, compression and tension zones occur in the periodontal ligament around the affected teeth. On the compression side, inflammatory mediators are released from resident immune cells, which further recruit circulating immune cells (12–18). This aseptic inflammation is an essential element for inducing osteoclastogenesis, leading to orthodontic tooth movement on the compression side (19). Primary afferent terminals within the periodontal ligament [a subpopulation of trigeminal ganglia (TG) neurons] and pain pathways in the brain have been determined. Nociceptors at the periodontal ligament transduce mechanical stimulation from orthodontic forces and chemical stimuli via inflammation, and nociceptive signals during orthodontic treatment are transmitted through the TG (Figure 1A). In this review, we highlight recent progress in understanding primary afferent mechanisms leading to orthodontic pain. As psychological factors such as anxiety, stress, and environmental factors can also affect orthodontic pain perception (20, 21), understanding central pathways involved in orthodontic pain is also crucial–but has been reviewed elsewhere (22). Orthodontic forces induce bone remodeling, which involves the contributions of immune cells and their regulations of bone cells in periodontium. This process is modulated by sensory nerves and, therefore, nociceptive nerves at the site of orthodontic force application should contribute to orthodontic bone remodeling as well as orthodontic pain (Figures 1A,B).
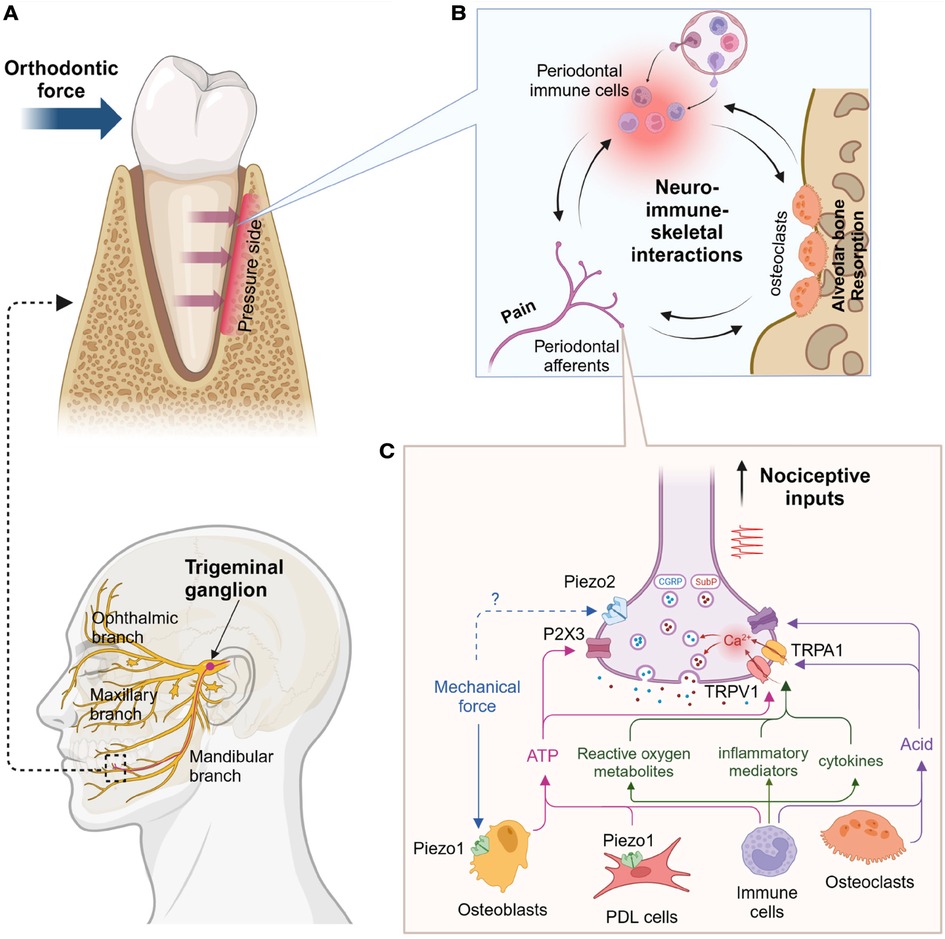
Figure 1. Proposed primary afferent mechanisms of orthodontic pain. (A) Anatomy of trigeminal afferents (bottom) and mandibular premolar under orthodontic pressure (top). Alveolar bone in the pressure side undergoes resorption. (B) Hypothetical neural-immune-skeletal interactions at the site of orthodontic pressure. Primary afferent terminals and immune cells within periodontal ligaments can regulate alveolar bone through the modulation of osteoclasts, which can also affect both neural and immune system. (C) Proinflammatory cytokines (e.g., interleukin 1β, tumor necrosis factor), inflammatory mediators (e.g., prostaglandin E2, bradykinin), reactive oxygen metabolites (e.g., hydrogen peroxide, 8-hydroxy-2'-deoxyguanosine) can activate and sensitize transient receptor potential vanilloid subtype 1 (TRPV1) and transient receptor potential ankyrin subtype 1 (TRPA1). Their activation induces calcium influx into nerve terminals and triggers exocytosis of the vesicles containing calcitonin gene-related peptide (CGRP) and substance P (SubP). Mechanical forces can activate Piezo1 in periodontal ligament (PDL) cells and osteoblasts, which induces ATP release likely activating P2X3 receptor. Immune cells or osteoclasts can increase acids to activate acid-sensing ion channels 3 (ASIC3). Concerted activation of these cationic ion channels in the terminal can generate action potential, which is transmitted into brain and leads to nociception. Created using Biorender.
2 Clinical factors influencing orthodontic pain and its management
Various patient-related factors, including the patient's age (1, 23, 24), gender (1, 2, 10, 23–27), race (23, 28), and baseline pain threshold (1, 23), affect orthodontic pain perception. However, another systematic review found no effects of age and sex on orthodontic pain (29).
The types of orthodontic appliances, such as separator placements, archwire placements, fixed and removable appliances, and growth modification appliances (including expanders and headgear) also cause varying levels of pain and discomfort (4). Currently, with the increasing use of self-ligation brackets and clear aligners, clinicians have found that self-ligation brackets produce less pain during extraction space closure compared to conventional brackets (30). Clear aligners produces lower level of pain and anxiety than fixed appliances during the first few days of treatment (and for up to 3 months) (31).
Pain and discomfort occur once the initial archwire is placed and last until bracket debonding. At the debonding appointment, the removal force is greater for metal brackets than other types, and pain tends to be greater in the anterior segments than posterior ones in the upper and lower dental arch (32).
Currently, the most effective method to decrease orthodontic pain is via analgesics. NSAIDs are often used to relieve orthodontic pain by blocking the formation of prostaglandins (23, 28). Pharmacological treatments are beneficial because of their rapid and reasonably effective pain relief along with easy over-the-counter access. However, pharmacological treatments may potentially slow down the rate of tooth movement, cause various adverse side effects, and offer only transient pain alleviation. While pharmacological treatment is a mainstay for orthodontic pain management in clinical orthodontics, there is growing interest in exploring non-pharmacological interventions, such as low-level laser therapy (LLLT), vibratory devices, chewing adjuncts, brainwave music, cognitive behavioral therapy, and post-treatment communication in the form of text messages (33–35). The non-pharmacological approaches can be advantageous due to their low risk of adverse side effects. However, the analgesic efficacy of these non-pharmacological approaches is inconclusive due to low evidence quality—although LLLT was shown to reduce pain up to seven days after initial archwire placement in a study (34, 36). A transcutaneous electrical nerve stimulation-based device also shows analgesic efficacy, which need more validation (37).
3 Primary afferent contributions to orthodontic pain
3.1 Peripheral nerve innervations in the periodontium
The periodontium is well-innervated by primary afferent neurons. Early ultrastructural studies identified myelinated and unmyelinated mechanosensitive nerve terminals within the periodontal ligaments of humans and experimental animals (38, 39). The periodontium is projected by multiple mechanosensory afferents whose cell bodies are located in the TG and mesencephalic trigeminal nucleus (40). Morphologically, several kinds of nerve endings have been identified in the periodontal ligaments of rat molars: nerve fibers with large Ruffini-like endings, bundles of free nerve endings of unmyelinated axons, and free myelinated axons (41). Single fiber recordings have shown nerve terminals innervating the periodontal ligament that include rapidly and slowly adapting Aβ-fibers, medium-diameter Aδ-fibers, and small-diameter C-fibers (42–45). Retrograde labeling of periodontal afferents has shown that periodontal trigeminal afferents comprise small- to medium-diameter neurons. Neurochemically, approximately 25% of periodontal afferents contain calcitonin gene-related peptide (CGRP) and transient receptor potential vanilloid subtype 1 (TRPV1). Periodontal afferents express various chemical receptor molecules, which transmit noxious chemical stimuli to the brain as a pain sensation. They also express mechanosensitive ion channels, which transduce sensations of pressure and stretching to the brain. These receptors and molecules, including TRPV1 (46–51), transient receptor potential ankyrin 1 (TRPA1) (47, 48, 52), Piezo1 (48, 53–57), Piezo2 (48, 53), acid-sensing ion channel 3 (ASIC3) (58–61), purinergic receptor (e.g., P2X3) (62, 63), are discussed below.
Sympathetic nerves, the component of the autonomic nervous system, originate from the superior cervical ganglion, are closely associated with the vasculature within the periodontium and regulate blood flow. Sympathetic nervous system contributes to wound healing in periodontal tissues (64, 65). While sympathetic nervous system regulates orthodontic tooth movement (66), evidence supporting the roles of sympathetic nerves in orthodontic pain is lacking and this review will focus on nociceptive primary afferents.
3.2 Periodontal nociceptors
Orthodontic pain is initiated by orthodontic force applied to the teeth. This force mechanically irritates periodontal tissues, which subsequently induces a cascade of vascular and chemical reactions, possibly affecting pain and bone remodeling (reviewed in Long, et al. and Tang, et al. (7, 67). The International Association for the Study of Pain (IASP) defines nociceptor as “a high-threshold sensory receptor of the peripheral somatosensory nervous system that is capable of transducing and encoding noxious stimuli.” The term refers to the nerve endings that initiate the transduction of noxious stimuli. Periodontal nociceptors are nerve endings that transduce noxious stimuli in the periodontium. Single-fiber recordings in rats have shown that periodontal nociceptors in the lower incisors are composed of thinly myelinated Aδ or unmyelinated c-fibers. Unlike nociceptors in the oral mucosa or tooth pulp (68), periodontal nociceptors are mostly Aδ (approximately 90%) rather than c nociceptors (69). In rat molars, most periodontal ligament units rapidly adapt, and two-thirds are Aδ fibers (44). The Aδ nociceptors may mediate immediate, sharp pain upon the application of orthodontic forces. This initial pain is followed by delayed pain, a major component of patient discomfort. Pain intensity gradually increases four hours following the application of orthodontic forces, peaking after 24 h, and lasts for days (1, 10, 23, 27, 70). Orthodontic force loading in rat molars reduces the mechanical threshold in behavioral assays and action potential conduction velocity in single-fiber recordings after 3–14 days (71). Such delayed pain may be derived from peripheral sensitization of the periodontal nociceptor terminals, as orthodontic forces induce inflammation in the periodontium to produce an array of inflammatory mediators and cytokines (7).
3.3 TRPV1-expressing nociceptors in orthodontic pain
Retrograde labeling by injecting a tracer into mouse gingiva has demonstrated that periodontal afferents are primarily small- and medium-diameter neurons (46, 72). Among these, 23% are CGRP-positive and 28% are TRPV1-positive. Non-peptidergic nociceptors binding to isolectin B4 are rare among periodontal afferents. Although TRPV1-expressing afferents mediate heat pain in the skin, they also mediate mechanical hyperalgesia from deep tissues such as muscles and joints (73–76). TRPV1-expressing afferents also play a critical role in orthodontic pain. Chemical ablation of TRPV1-expressing nociceptors from the TG of mice by injecting resiniferatoxin (RTX) into the TG substantially reduces orthodontic pain-like behaviors. Orthodontic forces increase mouse grimace scale scores and reduce bite force after one and three days, which is partially prevented by the ablation of TRPV1-expressing TG neurons (46).
TRPV1-expressing nociceptors can also contribute to the development of orthodontic pain. As TRPV1 and neuropeptides are highly colocalized in periodontal afferents (46, 72), and nerve terminals containing neuropeptides such as CGRP and substance P (SP) are projected into periodontal ligaments (77, 78), the activation of TRPV1-expressing nerve terminals by orthodontic forces can induce the release of neuropeptides such as CGRP or SP into the periodontal tissues. These neuropeptides play crucial roles in vasodilation and the recruitment of immune cells to damaged tissue (79). This neurogenic inflammation should then initiate sterile inflammation, leading to orthodontic tooth movement and peripheral sensitization. Therefore, interactions of periodontal nociceptors, immune cells, and bone cells should be critical for orthodontic tooth movement and pain (Figure 1B). The roles of neuropeptides in orthodontic pain are discussed in the sections below.
The results of a recent study indicate that TRPV1-expressing afferents contribute to the plastic changes in gene expression within the TG after applying an orthodontic force in mice (50). Orthodontic forces changed the expression of >1,200 genes in the TG after two days. These genes include those implicated in pain processing, such as neuropeptides (Adcyap1 and Gal), neurotrophins (Bdnf), neurotrophin receptors (Gfra1), cytokines (Csf1 and Cx3cl1), cytokine receptors (Cxcr4 and Tnfrsf1a), transcription factors (Atf3 and Sox11), and ion channels (Trpa1 and Trpv2). The contribution of these gene changes to orthodontic pain needs to be determined in future studies. Gene ontology analyses have shown increased cholesterol biosynthesis processes and decreased organization of connective tissue and extracellular matrix (50). Genes associated with synaptic organization and overall ion channel activities, especially voltage-gated sodium and potassium channels, are downregulated. The biological pathways and differentially expressed genes in the TG after orthodontic tooth movement resemble early changes following peripheral nerve injury rather than craniofacial inflammation. The implication of this finding is unclear, but orthodontic forces may induce injury to the afferent terminals in the periodontal ligament, which leads to transcriptomic changes in the TG similar to those following nerve injury. Interestingly, in mice with RTX injections into the TG to ablate TRPV1-expressing sensory neurons, the transcriptomic changes in the TG by orthodontic forces are eliminated (50). However, this does not imply that orthodontic force-induced transcriptomic changes are exclusively confined to TRPV1-expressing nociceptors. Instead, neuronal inputs through TRPV1-expressing nerves drive TG-wide transcriptomic changes after an orthodontic force. Therefore, TRPV1-expressing neurons are critical for transducing nociceptive inputs and inducing neural plasticity in the TG, which should contribute to orthodontic pain development.
4 Role of ion channels in periodontal nociceptors in orthodontic pain
4.1 Transient receptor potential ion channels
TRPV1 is enriched in peptidergic afferents, most of which are polymodal afferents (73). Its activation mediates the influx of cations into the nerve terminals, followed by the firing of action potentials, which leads to burning pain. Activation of TRPV1 also mediates the Ca2+ influx, which produces the release of neuropeptides from afferent terminals. Inflammatory mediators enhance TRPV1 function. Activation of receptors of multiple inflammatory mediators such as prostaglandins, bradykinin, and ATP invokes the activation of protein kinases. This, in turn, phosphorylates TRPV1 to enhance the function of TRPV1, which could increase pathological pain (73, 80, 81). Therefore, local inflammation in the periodontium following orthodontic tooth movement likely enhances the activation of TRPV1, which increases the release of neuropeptides. TRPV1 expressed in afferent terminals within the periodontal ligaments may also be phosphorylated by hypoxia-inducible factor-1α, activated by local hypoxia within the periodontal ligament (82, 83). Tissue inflammation has upregulated the expression of TRPV1 in nociceptive afferents in multiple preclinical models. Indeed, TRPV1 is upregulated in the TG following experimental tooth movement in rats, where the upregulation of TRPV1 peaked after one day and returned close to baseline after a week, which correlated with tooth movement-induced nocifensive behaviors such as face-grooming (84). Local administration of TRPV1 antagonist in the periodontium reduces tooth movement-induced nocifensive behaviors (85). Genetic knockout of TRPV1 attenuates spontaneous and bite-evoked pain after applying orthodontic forces (46). Knockdown of TRPV1 in the TG also reduces pain-like behaviors upon the application of orthodontic forces (86). Thus, TRPV1 in periodontal nociceptors mediates burning pain from orthodontic forces, and the inhibition of TRPV1 may attenuate pain related to orthodontic tooth movement.
TRPA1, which is activated by mustard oil and endogenous electrophiles such as hydrogen peroxide (H2O2) (73, 87, 88), also contributes to neuropeptide release and neurogenic inflammation. Thirty percent of TRPV1-expressing neurons express TRPA1, while up to 97% of TRPA1-expressing sensory neurons express TRPV1 (89), and the calcium influx TRPV1 causes can lead to the activation of TRPA1 (90). TRPA1 and TRPV1 play an integral role in neurogenic inflammation and pain after noxious stimuli due to their high co-expression, and the calcium influx caused by one can lead to the activation of the other (91–93). TRPA1 contributes to mechanical paresthesia caused by trigeminal neuropathic pain in mice downstream of oxidative stress (94). Oxidative stress may induce pain via the activation/sensitization of TRPA1 in the periodontium in a rat tooth-movement model (52). The tooth movement induced nociceptive behaviors, such as facial wiping, which matched the level of 8-hydroxy-2'-deoxyguanosine, an oxidative stress marker in the periodontal ligament and dental pulp. This change gradually diminished to the original level, which was related to decreases in oxidative stress, probably due to the remodeling of the periodontal ligament and alveolar bone (52). Orthodontic force-induced local hypoxia and reduced fluid flow occur in both periodontal tissue and dental pulp (95). Ischemia increases the intracellular Ca2+ concentration by increasing the concentration of protons and promoting TRPA1 activation (96, 97). Ca2+ influx through TRPA1 in sensory neuronal soma and nerve terminals can induce the release of neuropeptides, promoting the inflammatory periodontal tissue response. The expression of TRPV1 increases in the TG within a day (as an early response), while the upregulation of TRPA1 gradually increases from day one to day three as a late response during experimental tooth movement in rats (47). Therefore, the combined inhibition of TRPV1 and TRPA1 can additively attenuate orthodontic pain.
4.2 Mechanosensitive ion channels
Piezo1 and Piezo2 have emerged as important mediators in various aspects of mechanotransduction (98). They convert applied force into electrochemical signals critical for proprioception, touch, and mechanical pain. Piezo1 is expressed in non-neuronal tissues, such as the vasculature, bone, and heart, and has been shown to sense different mechanical stresses, such as compression and stretch. Piezo2 is exclusively expressed in sensory neurons and is related to touch sensation and mechanical pain.
Research concerning Piezo1 and Piezo2 in orthodontic tooth movements has rapidly developed from in vitro to in vivo studies. After exposure to mechanical loading in primary human periodontal ligament cells, the expression of Piezo1 and markers for osteoclastogenesis, such as receptor activator of nuclear kB ligand and cyclooxygenase-2, were significantly increased (99). Furthermore, grammostola mechanotoxin 4 (GsMTx4), a Piezo1 inhibitor, blocked osteoclastogenesis (99), suggesting Piezo1 contributes to mechanical stress-induced osteoclastogenesis. In murine cementoblastic cells, the expression of Piezo1 was decreased under a static compression force (100). Compression force also decreases cementoblastic genes such as osteoprotegerin, osteopontin, osteocalcin, and protein tyrosine phosphatase-like member A (100), suggesting that Piezo1 may contribute to the remodeling of cementum during tooth movement. When hydrostatic pressure is applied to mesenchymal stem cells (MSCs), Piezo1 is activated and plays a role in the cell fate determination of MSCs (either osteoblast or adipocyte differentiation) by regulating bone morphogenetic protein 2 expression (101). However, activation of Piezo1 by its agonist, Yoda1, induces a Ca2+ response and activates cationic currents in osteoblastic cells, followed by reduced proliferation that is reversed by the knockdown of Piezo1 (102). Furthermore, the C-terminus of Piezo1, which contains the R-Ras binding domain, plays an essential role in Ca2+ influx and activation of the ERK1/2 signaling pathway, suggesting that this domain is crucial for the mechanotransduction of osteoblastic differentiation in MSCs (103). Piezo1 also plays a role in macrophage infiltrates in the periodontal ligament via the Piezo1-AKT/GSK3b signaling-Cyclin D1 axis during tooth movement (104) and mediating both osteogenesis and osteoclastic activities on the tension side during orthodontic tooth movement (54, 57, 105). However, in vivo tooth movement models, the function of Piezo1 is controversial. In rats, orthodontic tooth movement was modestly reduced by the local injection of GsMTx4 into the alveolar bone (57). In contrast, tooth movement was not altered in Piezo1 conditional knockouts in mineralized tissue cells by Dmp1-cre (106).
The roles of Piezo1 and Piezo2 in orthodontic pain are undetermined. Piezo1 is functionally expressed in the TG and dorsal root ganglion neurons in rodents, a subset of which co-express TRPV1 (107, 108). Yoda1, a Piezo1 agonist, induces the release of CGRP from TG neurons (107). Therefore, Piezo1 in peptidergic afferents may transduce mechanical pain during orthodontic tooth movement. However, evidence that Piezo1 in sensory neurons mediates mechanical pain is lacking. Instead, Piezo1 expressed in non-neuronal cells may indirectly contribute to pain. For example, Piezo1 expressed in keratinocytes regulates mechanotransduction (109) and, when expressed in odontoblasts, it may mediate nociceptive signaling through the release of ATP by the activation of P2X3 in sensory neurons (110). The activation of Piezo1 in human periodontal ligament cells by Yoda1 increases intracellular Ca2+ and extracellular ATP (55). Mechanically stimulated ATP released from human periodontal ligament cells is inhibited by GsMTx4 or the knockdown of Piezo1 (55), suggesting that Piezo1 in periodontal ligament cells may contribute to purinergic signaling during orthodontic tooth movement, leading to pain.
Piezo2 is expressed more abundantly in sensory neurons, including low-threshold mechanosensitive neurons, as well as Aδ and C nociceptors (111, 112). Piezo2 mediates innocuous touch sensation and mechanical hyperalgesia following inflammation and nerve injury (111, 112). Piezo2 is also expressed in TRPV1-expressing nociceptors and mediates corneal mechanical pain, visceral mechanical hypersensitivity, and mechanical hyperalgesia in knee joint osteoarthritis (113–115). Therefore, Piezo2 in TRPV1-expressing TG neurons may mediate orthodontic pain; however, this needs to be determined in future studies.
4.3 Other nociceptive ion channels
Acid-sensing ion channels sense extracellular acidification. Among this family, ASIC3 is predominantly expressed in the peripheral nervous system and contributes to nociception. In rats, 26% of ASIC3-expressing TG neurons co-express CGRP (116). Orthodontic force could induce localized tissue acidosis at the site of compression. Tissue inflammation can lead to the accumulation of lactic acids, which causes prolonged acidosis (117). Orthodontic forces induce the occlusion of the blood vessels in the periodontal ligament on the compression side, which leads to hypoxia (118). Hypoxia can then induce tissue acidosis and regulate bone metabolism (119). The suppression of orthodontic pain-like behaviors by a pharmacological inhibitor or genetic knockdown against ASIC3 has been reported in an orthodontic tooth movement model in rats (58, 60). Orthodontic force-induced upregulation of nerve growth factor in the periodontium, which is retrogradely transported to the TG, induces increased expression of ASIC3 (61). In another study, inserting an elastic between the molars of rats acidified gingival crevicular fluid (from pH 7.4 to 7) after one day (59). Buffering periodontal acidification by repeated injections of phosphate-buffered saline or a periodontal injection of the inhibitor of ASIC3 decreased mechanical hyperalgesia in the facial skin after elastic insertion. However, in that study, elastic insertion did not change the expression of ASIC3 in the TG but increased the phosphorylation of ASIC3 in the periodontal tissues.
Extracellular ATP is an important signaling coordinator of cellular responses to mechanical stimulation in bone (120). It activates a group of purinergic receptors, of which P2X3 has been implicated in orthodontic pain. Although the P2X3 receptor is more often expressed in non-peptidergic TG neurons, a subset of P2X3-expressing neurons also co-express CGRP and SP (121). Orthodontic tooth movement upregulates the P2X3 receptor in the TG, while an inhibitor of P2X3 reduces orthodontic pain-like behaviors in rats (62). Orthodontic tooth movement in rats upregulates the nociceptin/orphanin FQ-opioid receptor-like receptor pathway in the TG, which exacerbates pain-like behaviors and likely mediates P2X3 upregulation in TG neurons (122, 123). Interestingly, the exposure of mice to static magnetic fields decreases orthodontic pain-like behaviors, accompanied by a decrease in the expression of P2X3 (124), supporting the roles of P2X3 in orthodontic pain.
5 Role of neuropeptides in orthodontic pain
SP and CGRP are the primary neuropeptides studied in orthodontic pain. The increased expression levels in the periodontal ligaments and dental pulp during tooth movement are positively correlated with orthodontic pain, clarifying the role of neurogenic inflammation in early injury response (125). Increased expression levels of CGRP and SP occur during tooth movement and are evident for a considerable time after movement has ended (78). Furthermore, neuropeptides stimulate human pulp fibroblasts to produce large amounts of interleukin-1β (IL-1β), interleukin-6, and tumor necrosis factor-α (TNF-α) during orthodontic tooth movement (126).
CGRP is expressed in the TG, and the central terminals of primary afferents are terminated in the trigeminal subnucleus caudalis (127). Inflammation induced by tooth movement sensitizes TRPV1 and TRPA1 in the primary afferent terminals (52). Activation of TRPV1, as an early response, and TRPA1, as a late response, results in CGRP release at the end of neuronal axons in vesicles through exocytosis (47). CGRP released from periodontal afferent terminals into the periodontal tissue plays the roles of vasodilator and transmitter for nociceptive information. Local injection of a CGRP receptor antagonist into the periodontium reduces pain-like behaviors by rats in experimental tooth movement (128). CGRP also mediates neuron-glia crosstalk by upregulating the expression of nitric oxide in the p38 signaling pathway in glial cells, accelerating the release of signal molecules that stimulate neurons and promote orofacial pain (129).
SP is produced in peptidergic sensory neurons in the TG and is secreted from their axons after Ca2+ influx (130). SP plays an essential role in bone remodeling and orthodontic pain during tooth movement. Orthodontic tooth movement activates nociceptors in periodontal tissues and leads to the release of SP from primary afferents. Moreover, tooth movement increases the Ca2+ influx after the activation of cation channels, causing SP release. SP promotes local inflammation by increasing the permeability of blood vessels (131, 132). It also promotes the secretion of inflammatory cytokines, including IL-1β and TNF-α through the neurokinin-1 receptor, enhances the phosphorylation of the mitogen-activated protein kinase signaling pathway to activate crosstalk between neurons and glial cells, and speeds the inflammatory process on the compression side of tooth movement (94, 133–135). Inflammation further promotes bone remodeling and tooth movement. Exogenous SP can promote alveolar bone remodeling and accelerate orthodontic tooth movement (136). However, it also increases inflammatory mediators released from immune cells, stimulating TRPV1 nociceptors conducting pain signals to the brain (137, 138). Ibuprofen decreases SP levels in gingival fluid and scores of visual analog scale one day following initial archwire placement (139).
Proposed primary afferent mechanisms of orthodontic pain is summarized in Figure 1C.
6 Bone remodeling and the interactions between bone and nociceptors
6.1 Bone remodeling in tooth movement
Bone remodeling is an important biological metabolic function that maintains healthy bone density and homeostasis and serves as a mechanism to regulate orthodontic tooth movement. Among the skeletal system, alveolar bone is under the most active bone remodeling (140–143). According to the pressure-tension theory, alveolar-bone-coupled remodeling events are orchestrated by the activities of osteoclasts, osteoblasts, and osteocytes in the periodontal ligament space and alveolar bone after a force is applied to teeth by archwires.
Osteoclasts are specialized cells originating from hematopoietic progenitors, which can resorb host bone tissue (144, 145). Osteoblasts are mononucleated, specialized cells originating from MSCs and are primarily responsible for alveolar bone apposition (146). Osteocytes are derived from functional osteoblasts and are the dominant cells embedded in mineralized bone during the apposition process (146).
On the tension side, the periodontium (including the periodontal ligaments, alveolar bone, and cementum) undergoes bone deposition. Osteoblasts originating from MSCs form the osteoid or type I collagen matrix following mineralization (147). Meanwhile, alveolar bone resorption takes place on the compression side due to osteoclastic activity, resulting in irregular cavities in the bone, which are filled in by new bone from osteoblast activity (148). Orthodontic forces lead to increased blood vascular permeability and disarrangement of tissues in the periodontal ligament space. Subsequently, blood flow and periodontal tissue must adapt to the compression force. If they fail to adapt, tissue necrosis and hyalinization will occur (149). Force magnitude is considered to be related to the origination of osteoclastogenesis and the type of bone resorption. Applying light force leads to front resorption, the recruitment of hematopoietic progenitors from blood vessels in the periodontal ligament space, and cell and tissue preservation. Application of a heavy force causes hyalinization, cell death, tissue necrosis, and cell-free periodontal ligaments and adjacent alveolar bone zones, leading to delayed bone resorption. In human and animal studies, a heavy force causes more discomfort and pain than a light force (150–152).
6.2 The role of bone cells in nociceptor activation
During tooth movement, alveolar bone remodeling involves three major bone cells: osteoclasts, osteoblasts, and osteocytes. Compared to osteoblasts and osteocytes, osteoclasts have drawn more attention due to their association with nociceptors and pain, especially in skeletal diseases with increased bone resorption. In bone remodeling during tooth movement, osteoclasts may interact with nociceptive nerve terminals and contribute to pain-like behaviors differently. First, osteoclasts secrete large amounts of acid through vacuolar H+-ATPase, leading to bone matrix degradation. Nociceptors that innervate bone respond to acid through ASIC3 and TRPV1, and inhibition of these receptors attenuates experimental bone pain-related behaviors (153, 154). Second, osteoclasts produce pronociceptive factors, such as neurotrophic factors (155). Additionally, orthodontic pain is often related to periodontal ligament inflammation, where several pronociceptive mechanisms act synchronously (7). Unlike osteoclasts, the direct effect of osteoblasts and osteocytes on nociceptors and orthodontic pain is not well understood, even though they have been reported to participate in pathological bone pain, such as in osteoarthritis (156).
6.3 The role of nociceptors in regulating alveolar bone remodeling
Bone is richly innervated, and sensory and autonomic nerve fibers have been identified in both the periodontal ligament space and bone, with sensory fibers being associated with nociception and mechanoreception.
Recent studies have highlighted the regulatory role of the sensory nervous system in alveolar bone remodeling. While TRPV1-expressing nociceptive nerves contribute to pain during orthodontic forces, their roles in orthodontic tooth movement are not well-defined. More focus has been placed on studying the role of periodontal nociceptors in regulating alveolar bone remodeling in the context of periodontitis in mice. However, past studies investigating the control of bone remodeling and periodontal bone loss by nociceptive sensory nerves have produced contradictory results. The systemic injection of vanilloid compounds, such as capsaicin or RTX, either increases (157–159) or decreases (160–162) bone resorption. These discrepancies could be attributed to caveats in the neural manipulation adopted in past studies.
First, capsaicin and RTX are specific agonists of TRPV1. TRPV1 activation mediates Ca2+ influx, and an excessive Ca2+ influx often leads to the ablation of TRPV1 + nerve terminals and the soma (163, 164). The extent of activation and subsequent ablation by vanilloids depends on the dose, timing, location, frequency, route of administration, etc. Therefore, without thorough biological validations, it is difficult to conclude whether the effects of capsaicin are mediated by the activation or ablation of TRPV1 + afferent nerve terminals, leading to conflicting outcomes and complicating the interpretation of results. For example, the oral administration of capsaicin suppressed bone loss in a rodent periodontitis model, which was interpreted as having a protective role for TRPV1 + afferents (157). However, it is unclear whether this protective role was mediated by the activation or ablation of TRPV1 + nerve terminals. In humans, topical capsaicin on the gingiva increases neurogenic inflammation and the level of matrix metalloproteinase-8 (a periodontitis-associated protease) in crevicular fluid (165, 166), which is harmful rather than protective.
Second, intraperitoneal injection of RTX or capsaicin accelerates alveolar bone destruction in experimental periodontitis and reduces long bone density (157, 159). However, this treatment needs careful interpretation as it produces a deficiency of TRPV1 + neurons throughout the body and likely involves strong compensatory processes in the nervous system. Systemic treatment in neonatal animals is more problematic due to potential alterations in nervous system development (157, 158). Many studies have shown that systemic capsaicin injections in neonates increase sympathetic activity (167–171). Given the well-established role of sympathetic nerves in enhancing bone resorption (172–174), it is difficult to interpret the bone changes following systemic treatment of vanilloids as a pure effect of selective manipulation of sensory neurons. Indeed, systemic genetic ablation of tropomyosin receptor kinase A expressing neurons (largely overlapping with TRPV1 + neurons) increases serum norepinephrine and bone resorption, which can be reversed by propranolol (a β-adrenergic antagonist). This suggests the involvement of enhanced sympathetic activity (175). Likewise, nerve transection-induced aggravation of periodontitis (176) is difficult to interpret because transection of the inferior alveolar nerve produces neuropathic pain (177, 178), increasing sympathetic activity (179, 180).
Third, systemically injected vanilloids may directly affect bone cells, as TRPV1 is also known to be expressed in these cells (181–183). Conventional knockouts of TRPV1 have the same limitation, potentially affecting both neural and bone cells.
A recent study ablated TRPV1 + afferents by microinjecting RTX directly into the maxillary/ophthalmic region of a unilateral TG in adult mice to determine their role in periodontal bone remodeling (49). The resulting localized ablation of TRPV1 + afferents decreased bone loss in a mouse model of periodontitis (49), which is in contrast to the results obtained by the systemic injection of vanilloids (157, 158). This finding was further validated by localized chemogenetic silencing of TRPV1-lineage neurons using inhibitory designer receptors exclusively activated by designer drugs. Continuous functional silencing of TRPV1-expressing neurons after the induction of experimental periodontitis prevented the progression of bone loss in a mouse model of periodontitis (49). In these approaches, ablation or silencing was confined to the ipsilateral TG without altering TRPV1 afferents in the dorsal root ganglia or vagal ganglia, minimizing uncontrollable effects on nervous control. Circulating norepinephrine was not altered by the localized ablation of TRPV1-expressing TG neurons. Experimental periodontitis-induced increases in immune cell infiltration and pro-inflammatory cytokines were prevented in the mice using nociceptor ablation, which was accompanied by the reduced activation of osteoclasts in the alveolar bone. Interestingly, the ablation of TRPV1-expressing trigeminal nociceptors did not alter the periodontal microbiome within the ligature, suggesting TRPV1-expressing afferents enhance bone destruction in periodontitis by promoting hyperactive host responses in the periodontium (49). These findings support the theory that nociceptors magnify the host response and regulate bone loss in the periodontium without affecting host defenses. Although TRPV1-expressing afferents magnify alveolar bone remodeling in a periodontitis model, it is important to note that the contribution of nociceptive afferents to alveolar bone remodeling is context-dependent. Nociceptive nerves are protective in apical periodontitis following tooth pulp infection (184). Therefore, it is essential to determine the contribution of nociceptors on orthodontic tooth movement.
The activation of peptidergic nociceptors leads to Ca2+-dependent exocytosis of neuropeptides. SP and CGRP are the most abundant neuropeptides in sensory afferents, and both directly regulate bone cells in vitro. CGRP increases differentiation of osteoblasts and decreases osteoclastogenesis, thereby producing osteogenic effects (157, 185). In contrast, SP enhances osteogenesis and bone resorption in vitro (186–188). It increases the differentiation of osteoblasts and osteogenic activity at a physiologically relevant concentration (186). Consistently, SP knockouts exhibit reduced bone volume and trabecular number/thickness in the femur (189). At higher concentrations that are likely to be detected at the injury site, SP also enhances differentiation and the resorptive activity of osteoclasts in vitro (186, 190, 191). Thus, the consequences of SP signaling on pathological bone regulation in vivo is complicated to predict. Pharmacological or genetic inhibition of SP has shown both resorptive and osteogenic effects in osteoarthritis progression and fracture healing (189, 190, 192–194). Both CGRP and SP are detectable in human gingival crevicular fluid. The level of CGRP in crevicular fluid is lower at the site of periodontitis than at healthy sites (195–197). In contrast, the level of SP in the crevicular fluid is higher at sites of periodontitis than at healthy sites, and SP levels decrease after treatment (198, 199). However, due to the ambivalent role of SP, the consequences of increased SP for alveolar bone loss are uncertain. Interestingly, the deletion of tachykinin precursor 1, a gene that encodes SP, or the treatment of gingiva with an SP antagonist, significantly reduces bone loss in ligature-induced periodontitis. Whereas the deletion of calcitonin-related polypeptide alpha, a gene that encodes CGRP, has a marginal role in bone loss (72). Furthermore, exogenous SP, but not neurokinin A, induces a vigorous inflammatory response and osteoclast activation in alveolar bone and facilitates bone loss in ligature-induced periodontitis (72). SP knockouts show decreased immune cell infiltration and pro-inflammatory cytokines at the site of ligature, which recapitulates the findings from mice with nociceptor ablations (72). These findings suggest that SP plays significant roles in regulating host responses and bone resorption in ligature-induced periodontitis and that the resorptive effect of SP is sufficiently dominant to overcome the osteogenic activity of CGRP or SP.
Given the modest, or absence of, pain in periodontitis, the tooth movement model is better than the periodontitis model for determining the role of nociceptors in pain and the neural regulation of bone remodeling. Although the nociceptive regulation of orthodontic tooth movement has long been hypothesized (200), there is no strong evidence supporting the underlying mechanisms. The mechanistic contribution of neuropeptides in orthodontic tooth movement is not well known. Only one study has shown that the systemic administration of exogenous SP accelerates orthodontic tooth movement and promotes alveolar bone remodeling (136). The detailed regulatory mechanisms of orthodontic tooth movement by the nervous system, periodontal nociceptors, and nociceptive molecules should be determined in future studies.
6.4 The role of sympathetic nervous system in regulating alveolar bone remodeling
The critical role of the nervous system in regulating bone remodeling has been uncovered in recent decades (201). Besides sensory systems as discussed above, multiple other pathways, including adrenergic, dopaminergic, and serotonergic systems, are known to modulate bone remodeling. The roles of sympathetic nerves in enhancing bone resorption is well established (172–174). The autonomic fibers are mainly associated with supplying blood vessels in the periodontal ligament space and bone marrow and are concentrated in areas with high osteogenic activity (202). In the sympathetic nervous system, the β2-adrenergic receptor and dopamine receptors (203), expressed by osteoblasts and osteoclasts, are recognized as mediating the action of sympathetic nerves on bone remodeling. Sympathetic nerve terminals and local norepinephrine level in alveolar bone increase in periodontium after experimental tooth movement in mice (66, 204). Sympathectomy or β-adrenergic receptor antagonist propranolol decreased, whereas β-agonist isoproterenol increased experimental tooth movements in mice, suggesting that signaling from sympathetic nerve terminals within periodontium could regulate orthodontic tooth movements (66). These effects are mediated through Sympathetic signaling β2-adrenergic receptor expressed in osteoclasts and periodontal ligament cells (66, 205). Orthodontic tooth movement is also regulated through central modulation of the sympathetic nervous system, particularly through ventromedial hypothalamic nucleus, a region modulating sympathetic nervous system activity in periphery. Orthodontic tooth separations in patients increase the activation of hypothalamus (206). Experimental tooth movement in rodents increase tyrosine hydroxylase in ventromedial hypothalamic nucleus (204) and lesioning of the area suppresses tooth movement (207). Interestingly, the ablation of peripheral nociceptors by systemic injection of capsaicin reduces orthodontic tooth movements in mice, which is accompanied by decreased sympathetic nerve terminals in periodontium (207), suggesting potential interactions between nociceptive and sympathetic system for alveolar bone remodeling. Despite its roles in tooth movement, it is not known if sympathetic nervous system contributes to modulation of orthodontic pain.
On the other hand, the overall effect of parasympathetic activity on bone is likely to be anabolic, although various nicotinic or muscarinic acetylcholine receptors of the parasympathetic nerves have also been found in osteoclasts and osteoblasts (208). However, the role of parasympathetic activity on orthodontic tooth movement or pain is not known yet.
6.5 Implications of nociceptor-bone remodeling interactions in clinical orthodontics
Given the roles of nociceptors in alveolar bone remodeling, the activities of periodontal nociceptors and molecules enriched in periodontal nociceptors likely modulate orthodontic tooth movement. Therefore, the extent of tooth movement by orthodontic force can be interfered with when the activity of periodontal nociceptors is suppressed for treating pain. Although NSAIDs reduce orthodontic pain (8), they also reduce orthodontic tooth movement in humans and rats (209, 210). In contrast, acetaminophen does not inhibit tooth movement (211, 212), although the analgesic effects of acetaminophen and NSAIDs are comparable (8). Therefore, acetaminophen may be better for patients who suffer from orthodontic pain. To prevent tooth movement interference, the development of novel therapies for orthodontic pain should consider their effects on alveolar bone remodeling. For example, targeting TRPV1, ASIC3, or CGRP for orthodontic pain needs validation given that their inhibition does not interfere with tooth movement. Conversely, it would be ideal to develop methods to enhance tooth movement without inducing or increasing pain. Non-surgical interventions, such as light vibration, do not cause pain but fail to accelerate tooth movement (213, 214). The only method with evidence of accelerating tooth movement is corticotomy, which causes pain, discomfort, and functional impairments (215). Thus, better understanding and considering nociceptor-bone remodeling interactions is important for improving clinical orthodontics.
7 Conclusion
Despite decades of research, details on the mechanisms of orthodontic pain and tooth movement remain unclear. In particular, ongoing knowledge gaps and challenges remain substantial in terms of understanding the mechanistic interactions between the nervous and skeletal systems during tooth movement. A better understanding of the fundamental mechanisms of neuroskeletal interactions may improve orthodontic treatment by developing new methods to reduce pain and accelerate orthodontic tooth movement. Future studies in this area should meet clinical needs by developing new therapies for “big gains with no pain” in clinical orthodontics.
Author contributions
SW: Conceptualization, Writing – original draft, Writing – review & editing. CK: Conceptualization, Writing – original draft, Writing – review & editing. MC: Conceptualization, Writing – original draft, Writing – review & editing.
Funding
The author(s) declare financial support was received for the research, authorship, and/or publication of this article.
This work was supported by National Institute of Health grant R35 DE030045 to MKC and Research Aid Award by the American Association of Orthodontists Foundation to SW.
Conflict of interest
The authors declare that the research was conducted in the absence of any commercial or financial relationships that could be construed as a potential conflict of interest.
The author(s) declared that they were an editorial board member of Frontiers, at the time of submission. This had no impact on the peer review process and the final decision.
Publisher's note
All claims expressed in this article are solely those of the authors and do not necessarily represent those of their affiliated organizations, or those of the publisher, the editors and the reviewers. Any product that may be evaluated in this article, or claim that may be made by its manufacturer, is not guaranteed or endorsed by the publisher.
References
1. Ngan P, Kess B, Wilson S. Perception of discomfort by patients undergoing orthodontic treatment. Am J Orthod Dentofacial Orthop. (1989) 96(1):47–53. doi: 10.1016/0889-5406(89)90228-X
2. Bergius M, Berggren U, Kiliaridis S. Experience of pain during an orthodontic procedure. Eur J Oral Sci. (2002) 110(2):92–8. doi: 10.1034/j.1600-0722.2002.11193.x
3. Bergius M, Kiliaridis S, Berggren U. Pain in orthodontics. A review and discussion of the literature. J Orofac Orthop. (2000) 61(2):125–37. doi: 10.1007/BF01300354
4. Krishnan V. Orthodontic pain: from causes to management–a review. Eur J Orthod. (2007) 29(2):170–9. doi: 10.1093/ejo/cjl081
5. Krukemeyer AM, Arruda AO, Inglehart MR. Pain and orthodontic treatment. Angle Orthod. (2009) 79(6):1175–81. doi: 10.2319/121308-632R.1
6. Johal A, Fleming PS, Al Jawad FA. A prospective longitudinal controlled assessment of pain experience and oral health-related quality of life in adolescents undergoing fixed appliance treatment. Orthod Craniofac Res. (2014) 17(3):178–86. doi: 10.1111/ocr.12044
7. Long H, Wang Y, Jian F, Liao LN, Yang X, Lai WL. Current advances in orthodontic pain. Int J Oral Sci. (2016) 8(2):67–75. doi: 10.1038/ijos.2016.24
8. Monk AB, Harrison JE, Worthington HV, Teague A. Pharmacological interventions for pain relief during orthodontic treatment. Cochrane Database Syst Rev. (2017) 11(11):CD003976. doi: 10.1002/14651858.CD003976.pub2
9. Walker JB, Buring SM. NSAID impairment of orthodontic tooth movement. Ann Pharmacother. (2001) 35(1):113–5. doi: 10.1345/aph.10185
10. Polat O, Karaman AI. Pain control during fixed orthodontic appliance therapy. Angle Orthod. (2005) 75(2):214–9. doi: 10.1043/0003-3219(2005)075%3C0210:PCDFOA%3E2.0.CO;2
11. Rakhshan H, Rakhshan V. Pain and discomfort perceived during the initial stage of active fixed orthodontic treatment. Saudi Dent J. (2015) 27(2):81–7. doi: 10.1016/j.sdentj.2014.11.002
12. De Filippo K, Dudeck A, Hasenberg M, Nye E, van Rooijen N, Hartmann K, et al. Mast cell and macrophage chemokines CXCL1/CXCL2 control the early stage of neutrophil recruitment during tissue inflammation. Blood. (2013) 121(24):4930–7. doi: 10.1182/blood-2013-02-486217
13. Zeng M, Kou X, Yang R, Liu D, Wang X, Song Y, et al. Orthodontic force induces systemic inflammatory monocyte responses. J Dent Res. (2015) 94(9):1295–302. doi: 10.1177/0022034515592868
14. He D, Kou X, Yang R, Liu D, Wang X, Luo Q, et al. M1-like macrophage polarization promotes orthodontic tooth movement. J Dent Res. (2015) 94(9):1286–94. doi: 10.1177/0022034515589714
15. Scott DA, Krauss J. Neutrophils in periodontal inflammation. Front Oral Biol. (2012) 15:56–83. doi: 10.1159/000329672
16. Yan Y, Liu F, Kou X, Liu D, Yang R, Wang X, et al. T cells are required for orthodontic tooth movement. J Dent Res. (2015) 94(10):1463–70. doi: 10.1177/0022034515595003
17. Yamasaki K, Shibasaki Y, Fukuhara T. Behavior of mast cells in periodontal ligament associated with experimental tooth movement in rats. J Dent Res. (1982) 61(12):1447–50. doi: 10.1177/00220345820610121501
18. Gameiro GH, Schultz C, Trein MP, Mundstock KS, Weidlich P, Goularte JF. Association among pain, masticatory performance, and proinflammatory cytokines in crevicular fluid during orthodontic treatment. Am J Orthod Dentofacial Orthop. (2015) 148(6):967–73. doi: 10.1016/j.ajodo.2015.05.029
19. Teixeira CC, Khoo E, Tran J, Chartres I, Liu Y, Thant LM, et al. Cytokine expression and accelerated tooth movement. J Dent Res. (2010) 89(10):1135–41. doi: 10.1177/0022034510373764
20. Bergius M, Broberg AG, Hakeberg M, Berggren U. Prediction of prolonged pain experiences during orthodontic treatment. Am J Orthod Dentofacial Orthop. (2008) 133(3):339.e1–8. doi: 10.1016/j.ajodo.2007.09.013
21. Quartana PJ, Campbell CM, Edwards RR. Pain catastrophizing: a critical review. Expert Rev Neurother. (2009) 9(5):745–58. doi: 10.1586/ern.09.34
22. Tian X, Li YH, Deng LZ, Han WZ, Pu D, Han XL, et al. Anxiety and depression mediate the relationship between digestive tract conditions and oral health-related quality of life in orthodontic patients. Front Psychol. (2022) 13:873983. doi: 10.3389/fpsyg.2022.873983
23. Scheurer PA, Firestone AR, Burgin WB. Perception of pain as a result of orthodontic treatment with fixed appliances. Eur J Orthod. (1996) 18(4):349–57. doi: 10.1093/ejo/18.4.349
24. Jones ML. An investigation into the initial discomfort caused by placement of an archwire. Eur J Orthod. (1984) 6(1):48–54. doi: 10.1093/ejo/6.1.48
25. Asiry MA, Albarakati SF, Al-Marwan MS, Al-Shammari RR. Perception of pain and discomfort from elastomeric separators in Saudi adolescents. Saudi Med J. (2014) 35(5):504–7.24825814
26. Abu Alhaija ES, Aldaikki A, Al-Omairi MK, Al-Khateeb SN. The relationship between personality traits, pain perception and attitude toward orthodontic treatment. Angle Orthod. (2010) 80(6):1141–9. doi: 10.2319/012710-59.1
27. Cozzani M, Ragazzini G, Delucchi A, Barreca C, Rinchuse DJ, Servetto R, et al. Self-reported pain after orthodontic treatments: a randomized controlled study on the effects of two follow-up procedures. Eur J Orthod. (2016) 38(3):266–71. doi: 10.1093/ejo/cjv032
28. Firestone AR, Scheurer PA, Burgin WB. Patients’ anticipation of pain and pain-related side effects, and their perception of pain as a result of orthodontic treatment with fixed appliances. Eur J Orthod. (1999) 21(4):387–96. doi: 10.1093/ejo/21.4.387
29. Inauen DS, Papadopoulou AK, Eliades T, Papageorgiou SN. Pain profile during orthodontic levelling and alignment with fixed appliances reported in randomized trials: a systematic review with meta-analyses. Clin Oral Investig. (2023) 27(5):1851–68. doi: 10.1007/s00784-023-04931-5
30. Gonzalez-Saez A, Antonio-Zancajo L, Montero J, Albaladejo A, Melo M, Garcovich D, et al. The influence of friction on design of the type of bracket and its relation to OHRQoL in patients who use multi-bracket appliances: a randomized clinical trial. Medicina (Kaunas). (2021) 57(2):171. doi: 10.3390/medicina57020171
31. Cardoso PC, Espinosa DG, Mecenas P, Flores-Mir C, Normando D. Pain level between clear aligners and fixed appliances: a systematic review. Prog Orthod. (2020) 21(1):3. doi: 10.1186/s40510-019-0303-z
32. Nakada N, Uchida Y, Inaba M, Kaetsu R, Shimizu N, Namura Y, et al. Pain and removal force associated with bracket debonding: a clinical study. J Appl Oral Sci. (2021) 29:e20200879. doi: 10.1590/1678-7757-2020-0879
33. Fleming PS, Strydom H, Katsaros C, MacDonald L, Curatolo M, Fudalej P, et al. Non-pharmacological interventions for alleviating pain during orthodontic treatment. Cochrane Database Syst Rev. (2016) 12(12):CD010263. doi: 10.1002/14651858.CD010263.pub2
34. Aljudaibi S, Duane B. Non-pharmacological pain relief during orthodontic treatment. Evid Based Dent. (2018) 19(2):48–9. doi: 10.1038/sj.ebd.6401305
35. Wang J, Jian F, Chen J, Ye NS, Huang YH, Wang S, et al. Cognitive behavioral therapy for orthodontic pain control: a randomized trial. J Dent Res. (2012) 91(6):580–5. doi: 10.1177/0022034512444446
36. Genc G, Kocadereli I, Tasar F, Kilinc K, El S, Sarkarati B. Effect of low-level laser therapy (LLLT) on orthodontic tooth movement. Lasers Med Sci. (2013) 28(1):41–7. doi: 10.1007/s10103-012-1059-6
37. Haralambidis C. Pain-free orthodontic treatment with the dental pain eraser. J Clin Orthod. (2019) 53(4):234–42.31390610
38. Harris R. Innervation of the human periodontium. Monogr Oral Sci. (1975) 4:27–44. doi: 10.1159/000397865
39. Linden RW. An update on the innervation of the periodontal ligament. Eur J Orthod. (1990) 12(1):91–100. doi: 10.1093/ejo/12.1.91
40. Linden RW, Scott BJ. Distribution of mesencephalic nucleus and trigeminal ganglion mechanoreceptors in the periodontal ligament of the cat. J Physiol. (1989) 410:35–44. doi: 10.1113/jphysiol.1989.sp017519
41. Byers MR. Sensory innervation of periodontal ligament of rat molars consists of unencapsulated ruffini-like mechanoreceptors and free nerve endings. J Comp Neurol. (1985) 231(4):500–18. doi: 10.1002/cne.902310408
42. Mengel MK, Jyvasjarvi E, Kniffki KD. Identification and characterization of afferent periodontal A delta fibres in the cat. J Physiol. (1993) 464:393–405. doi: 10.1113/jphysiol.1993.sp019641
43. Mengel MKC, Jyvasjarvi E, Kniffki KD. Identification and characterization of afferent periodontal C fibres in the cat. Pain. (1992) 48(3):413–20. doi: 10.1016/0304-3959(92)90094-R
44. Ishii N, Soma K, Toda K. Response properties of periodontal mechanoreceptors in rats, in vitro. Brain Res Bull. (2002) 58(4):357–61. doi: 10.1016/S0361-9230(02)00771-2
45. Linden RW, Millar BJ. The effect of rate of force application on the threshold of periodontal ligament mechanoreceptors in the cat canine tooth. Arch Oral Biol. (1988) 33(10):715–9. doi: 10.1016/0003-9969(88)90004-0
46. Wang S, Kim M, Ali Z, Ong K, Pae EK, Chung MK. TRPV1 and TRPV1-expressing nociceptors mediate orofacial pain behaviors in a mouse model of orthodontic tooth movement. Front Physiol. (2019) 10:1207. doi: 10.3389/fphys.2019.01207
47. Thammanichanon P, Kaewpitak A, Binlateh T, Pavasant P, Leethanakul C. Varied temporal expression patterns of trigeminal TRPA1 and TRPV1 and the neuropeptide CGRP during orthodontic force-induced pain. Arch Oral Biol. (2021) 128:105170. doi: 10.1016/j.archoralbio.2021.105170
48. Lukacs L, Rennekampff I, Tenenhaus M, Rennekampff HO. The periodontal ligament, temperature-sensitive ion channels TRPV1-4, and the mechanosensitive ion channels Piezo1 and 2: a nobel connection. J Periodontal Res. (2023) 58(4):687–96. doi: 10.1111/jre.13137
49. Wang S, Nie X, Siddiqui Y, Wang X, Arora V, Fan X, et al. Nociceptor neurons magnify host responses to aggravate periodontitis. J Dent Res. (2022) 101(7):812–20. doi: 10.1177/00220345211069956
50. Wang S, Chung MK. Orthodontic force induces nerve injury-like transcriptomic changes driven by TRPV1-expressing afferents in mouse trigeminal ganglia. Mol Pain. (2020) 16:1744806920973141. doi: 10.1177/1744806920973141
51. Sooampon S, Manokawinchoke J, Pavasant P. Transient receptor potential vanilloid-1 regulates osteoprotegerin/RANKL homeostasis in human periodontal ligament cells. J Periodontal Res. (2013) 48(1):22–9. doi: 10.1111/j.1600-0765.2012.01493.x
52. Morii A, Miyamura Y, Sago MI, Mizuhara M, Shikayama T, Naniwa M, et al. Orthodontic force-induced oxidative stress in the periodontal tissue and dental pulp elicits nociception via activation/sensitization of TRPA1 on nociceptive fibers. Free Radic Biol Med. (2020) 147:175–86. doi: 10.1016/j.freeradbiomed.2019.12.016
53. Gaite JJ, Sole-Magdalena A, Garcia-Mesa Y, Cuendias P, Martin-Cruces J, Garcia-Suarez O, et al. Immunolocalization of the mechanogated ion channels PIEZO1 and PIEZO2 in human and mouse dental pulp and periodontal ligament. Anat Rec (Hoboken). (2023). doi: 10.1002/ar.25351
54. Schroder A, Neher K, Krenmayr B, Paddenberg E, Spanier G, Proff P, et al. Impact of PIEZO1-channel on inflammation and osteoclastogenesis mediated via periodontal ligament fibroblasts during mechanical loading. Eur J Oral Sci. (2023) 131(1):e12913. doi: 10.1111/eos.12913
55. Horie S, Nakatomi C, Ito-Sago M, Morii A, Orimoto A, Ikeda H, et al. PIEZO1 promotes ATP release from periodontal ligament cells following compression force. Eur J Orthod. (2023) 45(5):565–74. doi: 10.1093/ejo/cjad052
56. Zhang D, Lin W, Jiang S, Deng P, Liu L, Wang Q, et al. Lepr-expressing PDLSCs contribute to periodontal homeostasis and respond to mechanical force by Piezo1. Adv Sci (Weinh. (2023) 10(29):e2303291. doi: 10.1002/advs.202303291
57. Du Y, Yang K. Role of mechanosensitive ion channel Piezo1 in tension-side orthodontic alveolar bone remodeling in rats. Arch Oral Biol. (2023) 155:105798. doi: 10.1016/j.archoralbio.2023.105798
58. Gao M, Long H, Ma W, Liao L, Yang X, Zhou Y, et al. The role of periodontal ASIC3 in orofacial pain induced by experimental tooth movement in rats. Eur J Orthod. (2016) 38(6):577–83. doi: 10.1093/ejo/cjv082
59. Osada A, Hitomi S, Nakajima A, Hayashi Y, Shibuta I, Tsuboi Y, et al. Periodontal acidification contributes to tooth pain hypersensitivity during orthodontic tooth movement. Neurosci Res. (2022) 177:103–10. doi: 10.1016/j.neures.2021.11.007
60. Yang H, Shan D, Jin Y, Liang H, Liu L, Guan Y, et al. The role of acid-sensing Ion channel 3 in the modulation of tooth mechanical hyperalgesia induced by orthodontic tooth movement. Neuroscience. (2020) 442:274–85. doi: 10.1016/j.neuroscience.2020.06.023
61. Gao M, Yan X, Lu Y, Ren L, Zhang S, Zhang X, et al. Retrograde nerve growth factor signaling modulates tooth mechanical hyperalgesia induced by orthodontic tooth movement via acid-sensing ion channel 3. Int J Oral Sci. (2021) 13(1):18. doi: 10.1038/s41368-021-00124-6
62. Yang Z, Cao Y, Wang Y, Luo W, Hua X, Lu Y, et al. Behavioural responses and expression of P2X3 receptor in trigeminal ganglion after experimental tooth movement in rats. Arch Oral Biol. (2009) 54(1):63–70. doi: 10.1016/j.archoralbio.2008.09.003
63. Lu Y, Yang Z, Hua XC, Chang X, Gui L, Wang S, et al. Expression of ligand-gated cation channels P2X3 receptor in rat pulp during experimental tooth movement. Hua Xi Kou Qiang Yi Xue Za Zhi. (2011) 29(2):183–6.21598495
64. Karita K, Izumi H, Tabata T, Kuriwada S, Sasano T, Sanjo D. The blood flow in the periodontal ligament regulated by the sympathetic and sensory nerves in the cat. Proc Finn Dent Soc. (1989) 85(4-5):289–94.2561430
65. Wucherpfennig AL, Chiego DJ Jr., Avery JK. Tritiated thymidine autoradiographic study on the influence of sensory and sympathetic innervation on periodontal wound healing in the rat. Arch Oral Biol. (1990) 35(6): 443–8. doi: 10.1016/0003-9969(90)90207-Q
66. Kondo M, Kondo H, Miyazawa K, Goto S, Togari A. Experimental tooth movement-induced osteoclast activation is regulated by sympathetic signaling. Bone. (2013) 52(1):39–47. doi: 10.1016/j.bone.2012.09.007
67. Tang Z, Zhou J, Long H, Gao Y, Wang Q, Li X, et al. Molecular mechanism in trigeminal nerve and treatment methods related to orthodontic pain. J Oral Rehabil. (2022) 49(2):125–37. doi: 10.1111/joor.13263
68. Toda K, Ishii N, Nakamura Y. Characteristics of mucosal nociceptors in the rat oral cavity: an in vitro study. Neurosci Lett. (1997) 228(2):95–8. doi: 10.1016/S0304-3940(97)00372-8
69. Toda K, Zeredo JL, Fujiyama R, Okada Y, Oi K, Hayashi Y, et al. Characteristics of nociceptors in the periodontium–an in vitro study in rats. Brain Res Bull. (2004) 62(4):345–9. doi: 10.1016/j.brainresbull.2003.10.005
70. Tortamano A, Lenzi DC, Haddad AC, Bottino MC, Dominguez GC, Vigorito JW. Low-level laser therapy for pain caused by placement of the first orthodontic archwire: a randomized clinical trial. Am J Orthod Dentofacial Orthop. (2009) 136(5):662–7. doi: 10.1016/j.ajodo.2008.06.028
71. Nakanishi H, Seki Y, Kohno T, Muramoto T, Toda K, Soma K. Changes in response properties of periodontal mechanoreceptors after experimental orthodontic tooth movement in rats. Angle Orthod. (2004) 74(1):93–9. doi: 10.1043/0003-3219(2004)074%3C0093:CIRPOP%3E2.0.CO;2
72. Siddiqui YD, Nie X, Wang S, Abbasi Y, Park L, Fan X, et al. Substance P aggravates ligature-induced periodontitis in mice. Front Immunol. (2023) 14:1099017. doi: 10.3389/fimmu.2023.1099017
73. Chung MK, Jung SJ, Oh SB. Role of TRP channels in pain sensation. Adv Exp Med Biol. (2011) 704:615–36. doi: 10.1007/978-94-007-0265-3_33
74. Chung MK, Wang S, Yang J, Alshanqiti I, Wei F, Ro JY. Neural pathways of craniofacial muscle pain: implications for novel treatments. J Dent Res. (2020) 99(9):1004–12. doi: 10.1177/0022034520919384
75. Chung MK, Ro JY. Peripheral glutamate receptor and transient receptor potential channel mechanisms of craniofacial muscle pain. Mol Pain. (2020) 16:1744806920914204. doi: 10.1177/1744806920914204
76. Wang S, Lim J, Joseph J, Wang S, Wei F, Ro JY, et al. Spontaneous and bite-evoked muscle pain are mediated by a common nociceptive pathway with differential contribution by TRPV1. J Pain. (2017) 18(11):1333–45. doi: 10.1016/j.jpain.2017.06.005
77. Kvinnsland I, Kvinnsland S. Changes in CGRP-immunoreactive nerve fibres during experimental tooth movement in rats. Eur J Orthod. (1990) 12(3):320–9. doi: 10.1093/ejo/12.3.320
78. Norevall LI, Forsgren S, Matsson L. Expression of neuropeptides (CGRP, substance P) during and after orthodontic tooth movement in the rat. Eur J Orthod. (1995) 17(4):311–25. doi: 10.1093/ejo/17.4.311
79. Holzer P. Neurogenic vasodilatation and plasma leakage in the skin. Gen Pharmacol. (1998) 30(1):5–11. doi: 10.1016/S0306-3623(97)00078-5
80. Cho A, Hall BE, Limaye AS, Wang S, Chung MK, Kulkarni AB. Nociceptive signaling through transient receptor potential vanilloid 1 is regulated by cyclin dependent kinase 5-mediated phosphorylation of T407 in vivo. Mol Pain. (2022) 18:17448069221111473. doi: 10.1177/17448069221111473
81. Joseph J, Qu L, Wang S, Kim M, Bennett D, Ro J, et al. Phosphorylation of TRPV1 S801 contributes to modality-specific hyperalgesia in mice. J Neurosci. (2019) 39(50):9954–66. doi: 10.1523/JNEUROSCI.1064-19.2019
82. Park HJ, Baek KH, Lee HL, Kwon A, Hwang HR, Qadir AS, et al. Hypoxia inducible factor-1alpha directly induces the expression of receptor activator of nuclear factor-kappaB ligand in periodontal ligament fibroblasts. Mol Cells. (2011) 31(6):573–8. doi: 10.1007/s10059-011-1055-x
83. Ristoiu V, Shibasaki K, Uchida K, Zhou Y, Ton BH, Flonta ML, et al. Hypoxia-induced sensitization of transient receptor potential vanilloid 1 involves activation of hypoxia-inducible factor-1 alpha and PKC. Pain. (2011) 152(4):936–45. doi: 10.1016/j.pain.2011.02.024
84. Qiao H, Gao Y, Zhang C, Zhou H. Increased expression of TRPV1 in the trigeminal ganglion is involved in orofacial pain during experimental tooth movement in rats. Eur J Oral Sci. (2015) 123(1):17–23. doi: 10.1111/eos.12158
85. Gao Y, Liu Y, Zhu K, Zhang Z, Qiao H, Lu Z, et al. Blocking of TRPV-1 in the parodontium relieves orthodontic pain by inhibiting the expression of TRPV-1 in the trigeminal ganglion during experimental tooth movement in rats. Neurosci Lett. (2016) 628:67–72. doi: 10.1016/j.neulet.2016.06.007
86. Guo R, Zhou Y, Long H, Shan D, Wen J, Hu H, et al. Transient receptor potential vanilloid 1-based gene therapy alleviates orthodontic pain in rats. Int J Oral Sci. (2019) 11(1):11. doi: 10.1038/s41368-019-0044-3
87. Trevisan G, Hoffmeister C, Rossato MF, Oliveira SM, Silva MA, Silva CR, et al. TRPA1 receptor stimulation by hydrogen peroxide is critical to trigger hyperalgesia and inflammation in a model of acute gout. Free Radic Biol Med. (2014) 72:200–9. doi: 10.1016/j.freeradbiomed.2014.04.021
88. Sugiyama D, Kang S, Brennan TJ. Muscle reactive oxygen species (ROS) contribute to post-incisional guarding via the TRPA1 receptor. PLoS One. (2017) 12(1):e0170410. doi: 10.1371/journal.pone.0170410
89. Story GM, Peier AM, Reeve AJ, Eid SR, Mosbacher J, Hricik TR, et al. ANKTM1, A TRP-like channel expressed in nociceptive neurons, is activated by cold temperatures. Cell. (2003) 112(6):819–29. doi: 10.1016/S0092-8674(03)00158-2
90. Spahn V, Stein C, Zollner C. Modulation of transient receptor vanilloid 1 activity by transient receptor potential ankyrin 1. Mol Pharmacol. (2014) 85(2):335–44. doi: 10.1124/mol.113.088997
91. Geppetti P, Nassini R, Materazzi S, Benemei S. The concept of neurogenic inflammation. BJU Int. (2008) 101(Suppl 3):2–6. doi: 10.1111/j.1464-410X.2008.07493.x
92. Bevan S, Andersson DA. TRP channel antagonists for pain–opportunities beyond TRPV1. Curr Opin Investig Drugs. (2009) 10(7):655–63.19579171
93. Fernandes ES, Russell FA, Spina D, McDougall JJ, Graepel R, Gentry C, et al. A distinct role for transient receptor potential ankyrin 1, in addition to transient receptor potential vanilloid 1, in tumor necrosis factor alpha-induced inflammatory hyperalgesia and freund’s complete adjuvant-induced monarthritis. Arthritis Rheum. (2011) 63(3):819–29. doi: 10.1002/art.30150
94. Trevisan G, Benemei S, Materazzi S, De Logu F, De Siena G, Fusi C, et al. TRPA1 Mediates trigeminal neuropathic pain in mice downstream of monocytes/macrophages and oxidative stress. Brain. (2016) 139(Pt 5):1361–77. doi: 10.1093/brain/aww038
95. Li Y, Jacox LA, Little SH, Ko CC. Orthodontic tooth movement: the biology and clinical implications. Kaohsiung J Med Sci. (2018) 34(4):207–14. doi: 10.1016/j.kjms.2018.01.007
96. Hamilton NB, Kolodziejczyk K, Kougioumtzidou E, Attwell D. Proton-gated ca(2+)-permeable TRP channels damage myelin in conditions mimicking ischaemia. Nature. (2016) 529(7587):523–7. doi: 10.1038/nature16519
97. Son GY, Hong JH, Chang I, Shin DM. Induction of IL-6 and IL-8 by activation of thermosensitive TRP channels in human PDL cells. Arch Oral Biol. (2015) 60(4):526–32. doi: 10.1016/j.archoralbio.2014.12.014
98. Nie X, Chung MK. Piezo channels for skeletal development and homeostasis: insights from mouse genetic models. Differentiation. (2022) 126:10–5. doi: 10.1016/j.diff.2022.06.001
99. Jin Y, Li J, Wang Y, Ye R, Feng X, Jing Z, et al. Functional role of mechanosensitive ion channel Piezo1 in human periodontal ligament cells. Angle Orthod. (2015) 85(1):87–94. doi: 10.2319/123113-955.1
100. Zhang YY, Huang YP, Zhao HX, Zhang T, Chen F, Liu Y. Cementogenesis is inhibited under a mechanical static compressive force via Piezo1. Angle Orthod. (2017) 87(4):618–24. doi: 10.2319/110616-799.1
101. Sugimoto A, Miyazaki A, Kawarabayashi K, Shono M, Akazawa Y, Hasegawa T, et al. Piezo type mechanosensitive ion channel component 1 functions as a regulator of the cell fate determination of mesenchymal stem cells. Sci Rep. (2017) 7(1):17696. doi: 10.1038/s41598-017-18089-0
102. Yoneda M, Suzuki H, Hatano N, Nakano S, Muraki Y, Miyazawa K, et al. PIEZO1 and TRPV4, which are distinct mechano-sensors in the osteoblastic MC3T3-E1 cells, modify cell-proliferation. Int J Mol Sci. (2019) 20(19):4960. doi: 10.3390/ijms20194960
103. Sugimoto A, Iwata K, Kurogoushi R, Tanaka M, Nakashima Y, Yamakawa Y, et al. C-terminus of PIEZO1 governs ca(2+) influx and intracellular ERK1/2 signaling pathway in mechanotransduction. Biochem Biophys Res Commun. (2023) 682:39–45. doi: 10.1016/j.bbrc.2023.09.080
104. Xu H, Guan J, Jin Z, Yin C, Wu S, Sun W, et al. Mechanical force modulates macrophage proliferation via Piezo1-AKT-cyclin D1 axis. FASEB J. (2022) 36(8):e22423. doi: 10.1096/fj.202200314R
105. Jiang Y, Guan Y, Lan Y, Chen S, Li T, Zou S, et al. Mechanosensitive Piezo1 in periodontal ligament cells promotes alveolar bone remodeling during orthodontic tooth movement. Front Physiol. (2021) 12:767136. doi: 10.3389/fphys.2021.767136
106. Nottmeier C, Lavicky J, Gonzalez Lopez M, Knauth S, Kahl-Nieke B, Amling M, et al. Mechanical-induced bone remodeling does not depend on Piezo1 in dentoalveolar hard tissue. Sci Rep. (2023) 13(1):9563. doi: 10.1038/s41598-023-36699-9
107. Mikhailov N, Leskinen J, Fagerlund I, Poguzhelskaya E, Giniatullina R, Gafurov O, et al. Mechanosensitive meningeal nociception via piezo channels: implications for pulsatile pain in migraine? Neuropharmacology. (2019) 149:113–23. doi: 10.1016/j.neuropharm.2019.02.015
108. Roh J, Hwang SM, Lee SH, Lee K, Kim YH, Park CK. Functional expression of Piezo1 in dorsal root ganglion (DRG) neurons. Int J Mol Sci. (2020) 21(11):3834. doi: 10.3390/ijms21113834
109. Mikesell AR, Isaeva O, Moehring F, Sadler KE, Menzel AD, Stucky CL. Keratinocyte PIEZO1 modulates cutaneous mechanosensation. Elife. (2022) 11:e65987. doi: 10.7554/eLife.65987
110. Ohyama S, Ouchi T, Kimura M, Kurashima R, Yasumatsu K, Nishida D, et al. Piezo1-pannexin-1-P2X(3) axis in odontoblasts and neurons mediates sensory transduction in dentinal sensitivity. Front Physiol. (2022) 13:891759. doi: 10.3389/fphys.2022.891759
111. Murthy SE, Loud MC, Daou I, Marshall KL, Schwaller F, Kuhnemund J, et al. The mechanosensitive ion channel Piezo2 mediates sensitivity to mechanical pain in mice. Sci Transl Med. (2018) 10(462):eaat9897. doi: 10.1126/scitranslmed.aat9897
112. Ranade SS, Woo SH, Dubin AE, Moshourab RA, Wetzel C, Petrus M, et al. Piezo2 is the major transducer of mechanical forces for touch sensation in mice. Nature. (2014) 516(7529):121–5. doi: 10.1038/nature13980
113. Xie Z, Feng J, Hibberd TJ, Chen BN, Zhao Y, Zang K, et al. Piezo2 channels expressed by colon-innervating TRPV1-lineage neurons mediate visceral mechanical hypersensitivity. Neuron. (2023) 111(4):526–538.e4. doi: 10.1016/j.neuron.2022.11.015
114. Obeidat AM, Wood MJ, Adamczyk NS, Ishihara S, Li J, Wang L, et al. Piezo2 expressing nociceptors mediate mechanical sensitization in experimental osteoarthritis. Nat Commun. (2023) 14(1):2479. doi: 10.1038/s41467-023-38241-x
115. Fernandez-Trillo J, Florez-Paz D, Inigo-Portugues A, Gonzalez-Gonzalez O, Del Campo AG, Gonzalez A, et al. Piezo2 mediates low-threshold mechanically evoked pain in the Cornea. J Neurosci. (2020) 40(47):8976–93. doi: 10.1523/JNEUROSCI.0247-20.2020
116. Ichikawa H, Sugimoto T. The co-expression of ASIC3 with calcitonin gene-related peptide and parvalbumin in the rat trigeminal ganglion. Brain Res. (2002) 943(2):287–91. doi: 10.1016/S0006-8993(02)02831-7
117. Reeh PW, Steen KH. Tissue acidosis in nociception and pain. Prog Brain Res. (1996) 113:143–51. doi: 10.1016/S0079-6123(08)61085-7
118. Niklas A, Proff P, Gosau M, Romer P. The role of hypoxia in orthodontic tooth movement. Int J Dent. (2013) 2013: 841840. doi: 10.1155/2013/841840
119. Arnett TR. Acidosis, hypoxia and bone. Arch Biochem Biophys. (2010) 503(1):103–9. doi: 10.1016/j.abb.2010.07.021
120. Dsouza C, Moussa MS, Mikolajewicz N, Komarova SV. Extracellular ATP and its derivatives provide spatiotemporal guidance for bone adaptation to wide spectrum of physical forces. Bone Rep. (2022) 17:101608. doi: 10.1016/j.bonr.2022.101608
121. Ambalavanar R, Moritani M, Dessem D. Trigeminal P2X3 receptor expression differs from dorsal root ganglion and is modulated by deep tissue inflammation. Pain. (2005) 117(3):280–91. doi: 10.1016/j.pain.2005.06.029
122. Yan X, Han H, Zhang S, Lu Y, Ren L, Tang Y, et al. N/OFQ modulates orofacial pain induced by tooth movement through CGRP-dependent pathways. BMC Neurosci. (2021) 22(1):25. doi: 10.1186/s12868-021-00632-5
123. Wang Y, Long H, Jian F, Li X, Yang X, Zhao Z, et al. Nociceptin/orphanin FQ up-regulates P2X(3) receptors in primary cultures of neonatal rat trigeminal ganglion neurons. Eur J Oral Sci. (2015) 123(6):409–15. doi: 10.1111/eos.12228
124. Zhu Y, Wang S, Long H, Zhu J, Jian F, Ye N, et al. Effect of static magnetic field on pain level and expression of P2X3 receptors in the trigeminal ganglion in mice following experimental tooth movement. Bioelectromagnetics. (2017) 38(1):22–30. doi: 10.1002/bem.22009
125. Chavarria-Bolanos D, Martinez-Zumaran A, Lombana N, Flores-Reyes H, Pozos-Guillen A. Expression of substance P, calcitonin gene-related peptide, beta-endorphin and methionine-enkephalin in human dental pulp tissue after orthodontic intrusion: a pilot study. Angle Orthod. (2014) 84(3):521–6. doi: 10.2319/060313-423.1
126. Yamaguchi M, Kojima T, Kanekawa M, Aihara N, Nogimura A, Kasai K. Neuropeptides stimulate production of interleukin-1 beta, interleukin-6, and tumor necrosis factor-alpha in human dental pulp cells. Inflamm Res. (2004) 53(5):199–204. doi: 10.1007/s00011-003-1243-z
127. Zhou Y, Long H, Ye N, Liao L, Yang X, Jian F, et al. The effect of capsaicin on expression patterns of CGRP in trigeminal ganglion and trigeminal nucleus caudalis following experimental tooth movement in rats. J Appl Oral Sci. (2016) 24(6):597–606. doi: 10.1590/1678-775720160150
128. Long H, Liao L, Gao M, Ma W, Zhou Y, Jian F, et al. Periodontal CGRP contributes to orofacial pain following experimental tooth movement in rats. Neuropeptides. (2015) 52:31–7. doi: 10.1016/j.npep.2015.06.006
129. Liang H, Hu H, Shan D, Lyu J, Yan X, Wang Y, et al. CGRP modulates orofacial pain through mediating neuron-glia crosstalk. J Dent Res. (2021) 100(1):98–105. doi: 10.1177/0022034520950296
130. Sacerdote P, Levrini L. Peripheral mechanisms of dental pain: the role of substance P. Mediators Inflamm. (2012) 2012:951920. doi: 10.1155/2012/951920
131. Doganay Yildiz E, Arslan H, Koseoglu S, Arabaci T, Yildiz DA, Savran L. The effect of photobiomodulation on total amount of substance P in gingival crevicular fluid: placebo-controlled randomized clinical trial. Lasers Med Sci. (2019) 34(3):517–23. doi: 10.1007/s10103-018-2625-3
132. Park CK, Bae JH, Kim HY, Jo HJ, Kim YH, Jung SJ, et al. Substance P sensitizes P2X3 in nociceptive trigeminal neurons. J Dent Res. (2010) 89(10):1154–9. doi: 10.1177/0022034510377094
133. Goltzman D, Hendy GN. The calcium-sensing receptor in bone–mechanistic and therapeutic insights. Nat Rev Endocrinol. (2015) 11(5):298–307. doi: 10.1038/nrendo.2015.30
134. Correa AS, Almeida VL, Lopes BMV, Franco A, Matos FR, Quintans-Junior LJ, et al. The influence of non-steroidal anti-inflammatory drugs and paracetamol used for pain control of orthodontic tooth movement: a systematic review. An Acad Bras Cienc. (2017) 89(4):2851–63. doi: 10.1590/0001-3765201720160865
135. Bowen EJ, Schmidt TW, Firm CS, Russo AF, Durham PL. Tumor necrosis factor-alpha stimulation of calcitonin gene-related peptide expression and secretion from rat trigeminal ganglion neurons. J Neurochem. (2006) 96(1):65–77. doi: 10.1111/j.1471-4159.2005.03524.x
136. An S, Zhang Y, Chen Q, Xiong B, Hao J, Zheng Y, et al. Effect of systemic delivery of substance P on experimental tooth movement in rats. Am J Orthod Dentofacial Orthop. (2019) 155(5):642–9. doi: 10.1016/j.ajodo.2018.05.026
137. Mistrova E, Kruzliak P, Chottova Dvorakova M. Role of substance P in the cardiovascular system. Neuropeptides. (2016) 58:41–51. doi: 10.1016/j.npep.2015.12.005
138. Rozas P, Lazcano P, Pina R, Cho A, Terse A, Pertusa M, et al. Targeted overexpression of tumor necrosis factor-alpha increases cyclin-dependent kinase 5 activity and TRPV1-dependent Ca2+influx in trigeminal neurons. Pain. (2016) 157(6):1346–62. doi: 10.1097/j.pain.0000000000000527
139. Kaya Y, Alkan O, Komuroglu AU, Keskin S. Effects of ibuprofen and low-level laser therapy on orthodontic pain by means of the analysis of interleukin 1-beta and substance P levels in the gingival crevicular fluid. J Orofac Orthop. (2021) 82(3):143–52. doi: 10.1007/s00056-020-00254-2
140. Gruber R. Osteoimmunology: inflammatory osteolysis and regeneration of the alveolar bone. J Clin Periodontol. (2019) 46(Suppl 21):52–69. doi: 10.1111/jcpe.13056
141. Lerner UH, Kindstedt E, Lundberg P. The critical interplay between bone resorbing and bone forming cells. J Clin Periodontol. (2019) 46(Suppl 21):33–51. doi: 10.1111/jcpe.13051
142. Connizzo BK, Sun L, Lacin N, Gendelman A, Solomonov I, Sagi I, et al. Nonuniformity in periodontal ligament: mechanics and matrix composition. J Dent Res. (2021) 100(2):179–86. doi: 10.1177/0022034520962455
143. Huja SS, Fernandez SA, Hill KJ, Li Y. Remodeling dynamics in the alveolar process in skeletally mature dogs. Anat Rec A Discov Mol Cell Evol Biol. (2006) 288(12):1243–9. doi: 10.1002/ar.a.20396
144. Nijweide PJ, Burger EH, Feyen JH. Cells of bone: proliferation, differentiation, and hormonal regulation. Physiol Rev. (1986) 66(4):855–86. doi: 10.1152/physrev.1986.66.4.855
145. Holtrop ME, King GJ. The ultrastructure of the osteoclast and its functional implications. Clin Orthop Relat Res. (1977) 123:177–96.856515
146. Jiang N, Guo W, Chen M, Zheng Y, Zhou J, Kim SG, et al. Periodontal ligament and alveolar bone in health and adaptation: tooth movement. Front Oral Biol. (2016) 18:1–8. doi: 10.1159/000351894
147. Sprogar S, Vaupotic T, Cor A, Drevensek M, Drevensek G. The endothelin system mediates bone modeling in the late stage of orthodontic tooth movement in rats. Bone. (2008) 43(4):740–7. doi: 10.1016/j.bone.2008.06.012
148. Omi M, Mishina Y. Roles of osteoclasts in alveolar bone remodeling. Genesis. (2022) 60(8-9):e23490. doi: 10.1002/dvg.23490
149. Kitase Y, Yokozeki M, Fujihara S, Izawa T, Kuroda S, Tanimoto K, et al. Analysis of gene expression profiles in human periodontal ligament cells under hypoxia: the protective effect of CC chemokine ligand 2 to oxygen shortage. Arch Oral Biol. (2009) 54(7):618–24. doi: 10.1016/j.archoralbio.2009.03.010
150. Luppanapornlarp S, Kajii TS, Surarit R, Iida J. Interleukin-1beta levels, pain intensity, and tooth movement using two different magnitudes of continuous orthodontic force. Eur J Orthod. (2010) 32(5):596–601. doi: 10.1093/ejo/cjp158
151. Limsiriwong S, Khemaleelakul W, Sirabanchongkran S, Pothacharoen P, Kongtawelert P, Ongchai S, et al. Biochemical and clinical comparisons of segmental maxillary posterior tooth distal movement between two different force magnitudes. Eur J Orthod. (2018) 40(5):496–503. doi: 10.1093/ejo/cjx092
152. Liao L, Long H, Zhang L, Chen H, Zhou Y, Ye N, et al. Evaluation of pain in rats through facial expression following experimental tooth movement. Eur J Oral Sci. (2014) 122(2):121–4. doi: 10.1111/eos.12110
153. Nagae M, Hiraga T, Wakabayashi H, Wang L, Iwata K, Yoneda T. Osteoclasts play a part in pain due to the inflammation adjacent to bone. Bone. (2006) 39(5):1107–15. doi: 10.1016/j.bone.2006.04.033
154. Kanaya K, Iba K, Abe Y, Dohke T, Okazaki S, Matsumura T, et al. Acid-sensing ion channel 3 or P2X2/3 is involved in the pain-like behavior under a high bone turnover state in ovariectomized mice. J Orthop Res. (2016) 34(4):566–73. doi: 10.1002/jor.23047
155. Andriessen AS, Donnelly CR, Ji RR. Reciprocal interactions between osteoclasts and nociceptive sensory neurons in bone cancer pain. Pain Rep. (2021) 6(1):e867. doi: 10.1097/PR9.0000000000000867
156. Kwan Tat S, Pelletier JP, Lajeunesse D, Fahmi H, Lavigne M, Martel-Pelletier J. The differential expression of osteoprotegerin (OPG) and receptor activator of nuclear factor kappaB ligand (RANKL) in human osteoarthritic subchondral bone osteoblasts is an indicator of the metabolic state of these disease cells. Clin Exp Rheumatol. (2008) 26(2):295–304.18565252
157. Takahashi N, Matsuda Y, Sato K, de Jong PR, Bertin S, Tabeta K, et al. Neuronal TRPV1 activation regulates alveolar bone resorption by suppressing osteoclastogenesis via CGRP. Sci Rep. (2016) 6:29294. doi: 10.1038/srep29294
158. Austah ON, Ruparel NB, Henry MA, Fajardo RJ, Schmitz JE, Diogenes A. Capsaicin-sensitive innervation modulates the development of apical periodontitis. J Endod. (2016) 42(10):1496–502. doi: 10.1016/j.joen.2016.06.009
159. Offley SC, Guo TZ, Wei T, Clark JD, Vogel H, Lindsey DP, et al. Capsaicin-sensitive sensory neurons contribute to the maintenance of trabecular bone integrity. J Bone Miner Res. (2005) 20(2):257–67. doi: 10.1359/JBMR.041108
160. Adam C, Llorens A, Baroukh B, Cherruau M, Saffar JL. Effects of capsaicin-induced sensory denervation on osteoclastic resorption in adult rats. Exp Physiol. (2000) 85(1):62–6. doi: 10.1017/s0958067000019308
161. Hill EL, Turner R, Elde R. Effects of neonatal sympathectomy and capsaicin treatment on bone remodeling in rats. Neuroscience. (1991) 44(3):747–55. doi: 10.1016/0306-4522(91)90094-5
162. Breivik T, Gundersen Y, Gjermo P, Fristad I, Opstad PK. Systemic chemical desensitization of peptidergic sensory neurons with resiniferatoxin inhibits experimental periodontitis. Open Dent J. (2011) 5:1–6. doi: 10.2174/1874210601105010001
163. Chung MK, Campbell JN. Use of capsaicin to treat pain: mechanistic and therapeutic considerations. Pharmaceuticals (Basel). (2016) 9(4):E66. doi: 10.3390/ph9040066
164. Wang S, Asgar J, Joseph J, Ro JY, Wei F, Campbell JN, et al. Ca2+ and calpain mediate capsaicin-induced ablation of axonal terminals expressing transient receptor potential vanilloid 1. J Biol Chem. (2017) 292:8291–303. doi: 10.1074/jbc.M117.778290
165. Avellan NL, Kemppainen P, Tervahartiala T, Vilppola P, Forster C, Sorsa T. Capsaicin-induced local elevations in collagenase-2 (matrix metalloproteinase-8) levels in human gingival crevice fluid. J Periodontal Res. (2006) 41(1):33–8. doi: 10.1111/j.1600-0765.2005.00836.x
166. Scardina GA, Carini F, Messina P. Vasodilatation of human gingiva and neurogenic inflammation. Clin Hemorheol Microcirc. (2005) 32(4):279–85.15894826
167. Ralevic V, Karoon P, Burnstock G. Long-term sensory denervation by neonatal capsaicin treatment augments sympathetic neurotransmission in rat mesenteric arteries by increasing levels of norepinephrine and selectively enhancing postjunctional actions. J Pharmacol Exp Ther. (1995) 274(1):64–71.7616449
168. Schicho R, Kanai Y, Ishikawa T, Skofitsch G, Donnerer J. Involvement of NGF in the induction of increased noradrenergic innervation of the ureter in neonatally capsaicin-treated rats. J Auton Nerv Syst. (1998) 73(1):46–53. doi: 10.1016/S0165-1838(98)00125-8
169. Sann H, Jancso G, Ambrus A, Pierau FK. Capsaicin treatment induces selective sensory degeneration and increased sympathetic innervation in the rat ureter. Neuroscience. (1995) 67(4):953–66. doi: 10.1016/0306-4522(95)00102-O
170. Luthman J, Stromberg I, Brodin E, Jonsson G. Capsaicin treatment to developing rats induces increase of noradrenaline levels in the iris without affecting the adrenergic terminal density. Int J Dev Neurosci. (1989) 7(6):613–22. doi: 10.1016/0736-5748(89)90020-8
171. Wang DH, Wu W, Lookingland KJ. Degeneration of capsaicin-sensitive sensory nerves leads to increased salt sensitivity through enhancement of sympathoexcitatory response. Hypertension. (2001) 37(2 Pt 2):440–3. doi: 10.1161/01.HYP.37.2.440
172. Takeda S, Elefteriou F, Levasseur R, Liu X, Zhao L, Parker KL, et al. Leptin regulates bone formation via the sympathetic nervous system. Cell. (2002) 111(3):305–17. doi: 10.1016/S0092-8674(02)01049-8
173. Khosla S, Drake MT, Volkman TL, Thicke BS, Achenbach SJ, Atkinson EJ, et al. Sympathetic beta1-adrenergic signaling contributes to regulation of human bone metabolism. J Clin Invest. (2018) 128(11):4832–42. doi: 10.1172/JCI122151
174. Maryanovich M, Takeishi S, Frenette PS. Neural regulation of bone and bone marrow. Cold Spring Harb Perspect Med. (2018) 8(9):a031344. doi: 10.1101/cshperspect.a031344
175. Chen H, Hu B, Lv X, Zhu S, Zhen G, Wan M, et al. Prostaglandin E2 mediates sensory nerve regulation of bone homeostasis. Nat Commun. (2019) 10(1):181. doi: 10.1038/s41467-018-08097-7
176. Yu X, Gong Z, Lin Q, Wang W, Liu S, Li S. Denervation effectively aggravates rat experimental periodontitis. J Periodontal Res. (2017) 52(6):1011–20. doi: 10.1111/jre.12472
177. Batbold D, Shinoda M, Honda K, Furukawa A, Koizumi M, Akasaka R, et al. Macrophages in trigeminal ganglion contribute to ectopic mechanical hypersensitivity following inferior alveolar nerve injury in rats. J Neuroinflammation. (2017) 14(1):249. doi: 10.1186/s12974-017-1022-3
178. Politis C, Lambrichts I, Agbaje JO. Neuropathic pain after orthognathic surgery. Oral Surg Oral Med Oral Pathol Oral Radiol. (2014) 117(2):e102–7. doi: 10.1016/j.oooo.2013.08.001
179. Leonard G, Chalaye P, Goffaux P, Mathieu D, Gaumond I, Marchand S. Altered autonomic nervous system reactivity to pain in trigeminal neuralgia. Can J Neurol Sci. (2015) 42(2):125–31. doi: 10.1017/cjn.2015.10
180. Strittmatter M, Grauer MT, Fischer C, Hamann G, Hoffmann KH, Blaes F, et al. Autonomic nervous system and neuroendocrine changes in patients with idiopathic trigeminal neuralgia. Cephalalgia. (1996) 16(7):476–80. doi: 10.1046/j.1468-2982.1996.1607476.x
181. Rossi F, Bellini G, Torella M, Tortora C, Manzo I, Giordano C, et al. The genetic ablation or pharmacological inhibition of TRPV1 signalling is beneficial for the restoration of quiescent osteoclast activity in ovariectomized mice. Br J Pharmacol. (2014) 171(10):2621–30. doi: 10.1111/bph.12542
182. He LH, Liu M, He Y, Xiao E, Zhao L, Zhang T, et al. TRPV1 Deletion impaired fracture healing and inhibited osteoclast and osteoblast differentiation. Sci Rep. (2017) 7:42385. doi: 10.1038/srep42385
183. Idris AI, Landao-Bassonga E, Ralston SH. The TRPV1 ion channel antagonist capsazepine inhibits osteoclast and osteoblast differentiation in vitro and ovariectomy induced bone loss in vivo. Bone. (2010) 46(4):1089–99. doi: 10.1016/j.bone.2010.01.368
184. Austah ON, Lillis KV, Akopian AN, Harris SE, Grinceviciute R, Diogenes A. Trigeminal neurons control immune-bone cell interaction and metabolism in apical periodontitis. Cell Mol Life Sci. (2022) 79(6):330. doi: 10.1007/s00018-022-04335-w
185. Wang L, Shi X, Zhao R, Halloran BP, Clark DJ, Jacobs CR, et al. Calcitonin-gene-related peptide stimulates stromal cell osteogenic differentiation and inhibits RANKL induced NF-kappaB activation, osteoclastogenesis and bone resorption. Bone. (2010) 46(5):1369–79. doi: 10.1016/j.bone.2009.11.029
186. Wang L, Zhao R, Shi X, Wei T, Halloran BP, Clark DJ, et al. Substance P stimulates bone marrow stromal cell osteogenic activity, osteoclast differentiation, and resorption activity in vitro. Bone. (2009) 45(2):309–20. doi: 10.1016/j.bone.2009.04.203
187. Shih C, Bernard GW. Neurogenic substance P stimulates osteogenesis in vitro. Peptides. (1997) 18(2):323–6. doi: 10.1016/S0196-9781(96)00280-X
188. Sohn SJ. Substance P upregulates osteoclastogenesis by activating nuclear factor kappa B in osteoclast precursors. Acta Otolaryngol. (2005) 125(2):130–3. doi: 10.1080/00016480410017710
189. Niedermair T, Kuhn V, Doranehgard F, Stange R, Wieskotter B, Beckmann J, et al. Absence of substance P and the sympathetic nervous system impact on bone structure and chondrocyte differentiation in an adult model of endochondral ossification. Matrix Biol. (2014) 38:22–35. doi: 10.1016/j.matbio.2014.06.007
190. Liu HJ, Yan H, Yan J, Li H, Chen L, Han LR, et al. Substance P promotes the proliferation, but inhibits differentiation and mineralization of osteoblasts from rats with spinal cord injury via RANKL/OPG system. PLoS One. (2016) 11(10):e0165063. doi: 10.1371/journal.pone.0165063
191. Yan K, Lin Q, Tang K, Liu S, Du Y, Yu X, et al. Substance P participates in periodontitis by upregulating HIF-1alpha and RANKL/OPG ratio. BMC Oral Health. (2020) 20(1):27. doi: 10.1186/s12903-020-1017-9
192. Muschter D, Fleischhauer L, Taheri S, Schilling AF, Clausen-Schaumann H, Grassel S. Sensory neuropeptides are required for bone and cartilage homeostasis in a murine destabilization-induced osteoarthritis model. Bone. (2020) 133:115181. doi: 10.1016/j.bone.2019.115181
193. Hofman M, Rabenschlag F, Andruszkow H, Andruszkow J, Mockel D, Lammers T, et al. Effect of neurokinin-1-receptor blockage on fracture healing in rats. Sci Rep. (2019) 9(1):9744. doi: 10.1038/s41598-019-46278-6
194. Niedermair T, Straub RH, Brochhausen C, Grassel S. Impact of the sensory and sympathetic nervous system on fracture healing in ovariectomized mice. Int J Mol Sci. (2020) 21(2):405. doi: 10.3390/ijms21020405
195. Lundy FT, Shaw C, McKinnell J, Lamey PJ, Linden GJ. Calcitonin gene-related peptide in gingival crevicular fluid in periodontal health and disease. J Clin Periodontol. (1999) 26(4):212–6. doi: 10.1034/j.1600-051X.1999.260403.x
196. Sert S, Sakallioglu U, Lutfioglu M, Aydogdu A, Acarel E, Gunaydin M. Neurogenic inflammation in periimplant and periodontal disease: a case-control split-mouth study. Clin Oral Implants Res. (2019) 30(8):800–7. doi: 10.1111/clr.13486
197. Sakallioglu EE, Lutfioglu M, Sakallioglu U, Diraman E, Pamuk F, Odyakmaz S. Local peptidergic innervation of gingiva in smoking and non-smoking periodontitis patients. J Periodontol. (2008) 79(8):1451–6. doi: 10.1902/jop.2008.070667
198. Pradeep AR, Raj S, Aruna G, Chowdhry S. Gingival crevicular fluid and plasma levels of neuropeptide substance-P in periodontal health, disease and after nonsurgical therapy. J Periodontal Res. (2009) 44(2):232–7. doi: 10.1111/j.1600-0765.2008.01138.x
199. Lundy FT, Mullally BH, Burden DJ, Lamey PJ, Shaw C, Linden GJ. Changes in substance P and neurokinin A in gingival crevicular fluid in response to periodontal treatment. J Clin Periodontol. (2000) 27(7):526–30. doi: 10.1034/j.1600-051x.2000.027007526.x
200. Kyrkanides S, Huang H, Faber RD. Neurologic regulation and orthodontic tooth movement. Front Oral Biol. (2016) 18:64–74. doi: 10.1159/000351900
201. Elefteriou F. Regulation of bone remodeling by the central and peripheral nervous system. Arch Biochem Biophys. (2008) 473(2):231–6. doi: 10.1016/j.abb.2008.03.016
202. Duan Y, Liang Y, Yang F, Ma Y. Neural regulations in tooth development and tooth-periodontium complex homeostasis: a literature review. Int J Mol Sci. (2022) 23(22):14150. doi: 10.3390/ijms232214150
203. Lee DJ, Tseng HC, Wong SW, Wang Z, Deng M, Ko CC. Dopaminergic effects on in vitro osteogenesis. Bone Res. (2015) 3:15020. doi: 10.1038/boneres.2015.20
204. Cao H, Fang B, Wang X, Zhou Y. Sympathetic nervous system contributes to orthodontic tooth movement by central neural regulation from hypothalamus. Histol Histopathol. (2020) 35(12):1493–502. doi: 10.14670/HH-18-280
205. Cao H, Kou X, Yang R, Liu D, Wang X, Song Y, et al. Force-induced Adrb2 in periodontal ligament cells promotes tooth movement. J Dent Res. (2014) 93(11):1163–9. doi: 10.1177/0022034514551769
206. Ariji Y, Kondo H, Miyazawa K, Tabuchi M, Koyama S, Kise Y, et al. Orthodontic tooth separation activates the hypothalamic area in the human brain. Int J Oral Sci. (2018) 10(2):8. doi: 10.1038/s41368-017-0001-y
207. Kondo H, Kondo M, Hayashi K, Kusafuka S, Hamamura K, Tanaka K, et al. Orthodontic tooth movement-activated sensory neurons contribute to enhancing osteoclast activity and tooth movement through sympathetic nervous signalling. Eur J Orthod. (2022) 44(4):404–11. doi: 10.1093/ejo/cjab072
208. Bajayo A, Bar A, Denes A, Bachar M, Kram V, Attar-Namdar M, et al. Skeletal parasympathetic innervation communicates central IL-1 signals regulating bone mass accrual. Proc Natl Acad Sci U S A. (2012) 109(38):15455–60. doi: 10.1073/pnas.1206061109
209. Hammad SM, El-Hawary YM, El-Hawary AK. The use of different analgesics in orthodontic tooth movements. Angle Orthod. (2012) 82(5):820–6. doi: 10.2319/110911-691.1
210. Fang J, Li Y, Zhang K, Zhao Z, Mei L. Escaping the adverse impacts of NSAIDs on tooth movement during orthodontics: current evidence based on a meta-analysis. Medicine (Baltimore). (2016) 95(16):e3256. doi: 10.1097/MD.0000000000003256
211. Roche JJ, Cisneros GJ, Acs G. The effect of acetaminophen on tooth movement in rabbits. Angle Orthod. (1997) 67(3):231–6. doi: 10.1043/0003-3219(1997)067%3C0231:TEOAOT%3E2.3.CO;2
212. Arias OR, Marquez-Orozco MC. Aspirin, acetaminophen, and ibuprofen: their effects on orthodontic tooth movement. Am J Orthod Dentofacial Orthop. (2006) 130(3):364–70. doi: 10.1016/j.ajodo.2004.12.027
213. El-Angbawi A, McIntyre GT, Fleming PS, Bearn DR. Non-surgical adjunctive interventions for accelerating tooth movement in patients undergoing fixed orthodontic treatment. Cochrane Database Syst Rev. (2015) 2015(11):CD010887. doi: 10.1002/14651858.CD010887.pub2
214. El-Angbawi A, McIntyre G, Fleming PS, Bearn D. Non-surgical adjunctive interventions for accelerating tooth movement in patients undergoing orthodontic treatment. Cochrane Database Syst Rev. (2023) 6(6):CD010887. doi: 10.1002/14651858.CD010887.pub3
Keywords: orthodontic pain, nociceptors, transient receptor potential (TRP) channel, alveolar bone remodeling, neuropeptides
Citation: Wang S, Ko C-C and Chung M-K (2024) Nociceptor mechanisms underlying pain and bone remodeling via orthodontic forces: toward no pain, big gain. Front. Pain Res. 5:1365194. doi: 10.3389/fpain.2024.1365194
Received: 3 January 2024; Accepted: 12 February 2024;
Published: 22 February 2024.
Edited by:
Armen N. Akopian, The University of Texas Health Science Center at San Antonio, United StatesReviewed by:
Yi Ye, New York University, United StatesJie Lei, Peking University Hospital of Stomatology, China
© 2024 Wang, Ko and Chung. This is an open-access article distributed under the terms of the Creative Commons Attribution License (CC BY). The use, distribution or reproduction in other forums is permitted, provided the original author(s) and the copyright owner(s) are credited and that the original publication in this journal is cited, in accordance with accepted academic practice. No use, distribution or reproduction is permitted which does not comply with these terms.
*Correspondence: Man-Kyo Chung bWNodW5nQHVtYXJ5bGFuZC5lZHU=