Commentary: Intraganglionic reactive oxygen species mediate inflammatory pain and hyperalgesia through TRPA1 in the rat
- Department of Neural and Pain Sciences, University of Maryland School of Dentistry, Baltimore, MD, United States
Reactive oxygen species (ROS) are generated in nociceptive pathways in response to inflammation and injury. ROS are accumulated within the sensory ganglia following peripheral inflammation, but the functional role of intraganlionic ROS in inflammatory pain is not clearly understood. The aims of this study were to investigate whether peripheral inflammation leads to prolonged ROS accumulation within the trigeminal ganglia (TG), whether intraganglionic ROS mediate pain hypersensitivity via activation of TRPA1, and whether TRPA1 expression is upregulated in TG during inflammatory conditions by ROS. We demonstrated that peripheral inflammation causes excess ROS production within TG during the period when inflammatory mechanical hyperalgesia is most prominent. Additionally, scavenging intraganglionic ROS attenuated inflammatory mechanical hyperalgesia and a pharmacological blockade of TRPA1 localized within TG also mitigated inflammatory mechanical hyperalgesia. Interestingly, exogenous administration of ROS into TG elicited mechanical hyperalgesia and spontaneous pain-like responses via TRPA1, and intraganglionic ROS induced TRPA1 upregulation in TG. These results collectively suggest that ROS accumulation in TG during peripheral inflammation contributes to pain and hyperalgesia in a TRPA1 dependent manner, and that ROS further exacerbate pathological pain responses by upregulating TRPA1 expression. Therefore, any conditions that exacerbate ROS accumulation within somatic sensory ganglia can aggravate pain responses and treatments reducing ganglionic ROS may help alleviate inflammatory pain.
1. Introduction
Contributing to a wide range of physiological processes, reactive oxygen species (ROS) are highly reactive derivatives of molecular oxygen produced as a byproduct of normal enzymatic reactions (1). When redox homeostasis is dysregulated, excess ROS accumulate in cells and leads to oxidative stress. A pathological increase in ROS levels can directly damage DNA, protein, and lipids, and initiates cascades of cellular and molecular events that culminate into the development of various pathological conditions, including chronic pain (2, 3).
ROS contribute to a variety of pain conditions at multiple levels of nociceptive pathways, including inflammatory pain, neuropathic pain, cancer pain, and chemotherapy-induced pain, among others. For example, tissue injury or inflammation leads to increased ROS production in local tissues (4, 5, 6, 7) and scavenging of local ROS prevents peripheral sensitization (7, 8, 9). ROS in local tissues also promote peripheral sensitization through their reciprocal interaction with TRPV1 (10), by increasing endogenous agonists for TRPA1 via lipid peroxidation (11, 12, 13) and by modulating the expression of proinflammatory cytokines and chemokines (6, 14, 15).
ROS generated in the spinal cord following nerve injury can lead to central sensitization of spinal cord dorsal horn neurons via phosphorylation of N-methyl-D-aspartate (NMDA) receptors (16), phosphorylation and cell surface localization of α-amino-3-hydroxy-5-methyl-4-isoxazolepropionic acid (AMPA) receptors (17), and through a loss of gamma-aminobutyric acid (GABA) neurons and dysfunction of surviving GABA neurons (18). In addition, ROS enhances excitatory synaptic transmission in rat spinal cord dorsal horn neurons by activating TRPA1 and TRPV1 at the central terminals of primary afferent neurons (19). ROS levels are also elevated within trigeminal ganglia (TG) of rats following peripheral inflammation (20). However, there is limited information on the functional role of intraganglionic ROS under pathological pain conditions.
It is well established that TRPA1 functions as a detector of both environmental irritants and endogenous mediators generated during injury or inflammation (21), and that TRPA1 in both peripheral and central terminals of nociceptive afferents instigates pathological pain conditions (22). Moreover, the expression level of TRPA1 correlates with mechanical hypersensitivity in several inflammatory pain models (23, 24, 25). Interestingly, ROS can directly activate TRPA1, which is also a redox sensitive channel (11). ROS can also induce TRPA1 gene expression via the generation of inflammatory cytokines (26, 27). Therefore, it is possible that ROS generated in the immediate vicinity of primary afferent cell bodies can directly modulate TRPA1 function and expression.
The purpose of this research was to examine whether inflammation in the masseter muscle causes a prolonged accumulation of ROS in TG. Additionally, the study sought to determine if intraganglionic ROS plays a role in pain hypersensitivity by activating TRPA1 and increasing its expression in the TG during inflammation. Understanding these questions is important because intraganglionic ROS can generate and sustain pain responses, even when there is no accumulation of ROS in the peripheral or central sites. Moreover, reducing ROS in various parts along the nociceptive pathway could result in more effective pain relief. Furthermore, it is significant to comprehend the functional role of TRPA1 within sensory ganglia, as TRPA1 activation in these ganglia can independently contribute to pain responses.
2. Materials and methods
2.1. Animals
Adult male Sprague-Dawley rats (3 months old; 150 to 350 g; Harlan, IN, USA) were used. All animals were housed in a temperature-controlled room under a 12:12 light-dark cycle with access to food and water ad libitum. All procedures were conducted in accordance with the National Institutes of Health Guide for the Care and Use of Laboratory Animals (publication no. 80–23) and under a University of Maryland Baltimore approved Institutional Animal Care and Use Committee protocol.
2.2. Masseter inflammation
Inflammation was induced by injecting 50 μl of 50% Complete Freund's Adjuvant (CFA) in isotonic saline (Sigma-Alridch, St. Louis, MO) into the mid-region of the masseter muscle via a 27-gauge needle. Rats were briefly anesthetized with 3% isoflurane for the injection procedure. The characteristics of inflammation following CFA injections in the rat masseter have been described previously (28, 29).
2.3. ROS assay in TG
The methods for the ROS assay were described in our previous study (20). Briefly, ROS levels were quantified using a cell-permeant oxidant-sensing probe 2′,7′-dichlorodihydrofluorescein diacetate (H2DCFDA; Invitrogen, Carlsbad, CA, USA). H2DCFDA is de-esterified within the cytoplasm and turns highly fluorescent upon oxidation. H2DCFDA detects hydrogen peroxide (H2O2), peroxyl radicals (ROO•), and peroxynitrite (ONOO−), but it is possible that other biologically relevant ROS, such as superoxide radicals (O2•−) and hydroxyl radicals (OH•), are also involved. Rats were injected with either CFA or the same volume of vehicle into the left masseter muscle. Naïve rats that did not receive either CFA or vehicle treatment served as a control group. The TG ipsilateral to the injected muscle was removed either 1, 4, 7, 14, or 28 days after the injection. TG was quickly removed and washed with phosphate-buffered saline (PBS). Immediately after extraction and dissection, the tissues were minced finely in PBS and were incubated in 96-well plates in 200 μl PBS for 30 min at 37°C. The background fluorescence for each specimen was determined with a fluorimeter (DTX880 Multimode Detector, Beckman Coulter) at 485 nm for excitation and 535 nm for emission. After the background reading, H2DCFDA was added to each well to a final concentration of 10 μM. The plates were again incubated for 30 min at 37°C, and the fluorescence was re-measured. ROS levels were estimated as the intensity of fluorescence after subtraction of the background fluorescence (Multimode Analysis Software). To minimize experimental variations, samples from naïve, CFA-, and vehicle-treated groups at each time point were analyzed on the same day. The results from CFA- or vehicle-treated group were normalized to the results from naïve rats at each time point. We have previously shown that negative control groups without TG tissues (PBS alone, PBS with H2DCFDA, PBS with H2O2, PBS with H2O2 and H2DCFDA) generated little or no positive signal, which is only approximately 1% or less of signals obtained from TG tissues (20). We have also shown that TG samples with exogenously added H2O2 exhibit robust fluorescence signal in the presence of H2DCFDA (20). These control groups validate that our methods allow reliable detection of ROS in TG tissues by H2DCFDA.
2.4. Microinjections into TG
All microinjections into TG were made through a 26-gauge guide cannula (P1 Technologies, Roanoke, VA, USA) that was surgically implanted over TG (2.5 mm posterior and 1.5 mm lateral to the bregma). Briefly, the scalp was incised 1 cm in length with a sterile scalpel at the midline. A small hole was drilled in the skull using a dental drill with a sterile burr bit (2.35 mm shank, 2.7 × 2.5 mm) to enable implantation of a sterile 26-gauge guide cannula (Roanoke, VA) over TG (2.5 mm posterior and 1.5 mm lateral to the bregma) using stereotaxic coordinates. The cannula was secured to the skull using pharmaceutical grade acrylic cement and two small screws (3 mm) inserted into the parietal bones. The incision edges were cleansed with Betadine and rinsed with 0.9% saline and will be closed with a Wax or Silicone coated braided non-absorbable suture (4-0 or 5-0) material in a simple interrupted pattern. Sutures were removed 7 to 10 days after surgery.
The rats were briefly anesthetized with isoflurane (> 3%–4.5% Induction-chamber) (> 1.5%–3% Maintenance—nosecone) for microinjection procedures. The depth of anesthesia was confirmed by pinching the toe using serrated forceps. All microinjections were made manually using a sterile 30-gauge injection cannula attached to a 1.0 ml syringe (Hamilton, Reno, NV, USA) via PE tubing (0.2 mm ID, 1.75 mm OD). The external end of the cannula was prepped using Betadine solution / scrub and rinsed with alcohol prior to removal of access plug. The substances were infused over a 30 s period and the injection syringe was left in place for an additional 30 s to prevent backflow.
2.5. Behavioral studies
2.5.1. Assessment of inflammatory mechanical hyperalgesia
Persistent mechanical hyperalgesia in the masseter muscle was assessed under CFA-induced inflammatory conditions utilizing a behavioral model specifically developed for testing masseter sensitivity in awake rats (30). The rats were habituated to the environment and the experimenter for five consecutive days prior to the behavioral testing. They were trained to stand on a soft pad and lean against the experimenter's hand wearing a leather work-glove, rather than standing on a meshed metal or grid surface. The habituation required no more than gentle petting, and it was achieved within half an hour. In this model, a series of calibrated von Frey filaments (1–125 gm) were applied to the region over the masseter muscle. The rats were not restrained in any way but remained in position long enough for the experimenter to probe the skin overlying the masseter muscle with von Frey filaments.
An active withdrawal of the head from the filament application was defined as a positive response. Each von Frey filament was applied five times and the response frequencies (i.e., (number of responses/ number of stimuli)×100%) to a range of filament forces were determined. After a non-linear regression analysis, an EF50 value, defined as the filament force (g) necessary to produce a 50% response frequency, was determined. The EF50 value was used as a measure of mechanical threshold. A reduction of EF50 after inflammation suggested the presence of mechanical hypersensitivity. The threshold data were subsequently converted to logarithmic values for statistical analyses. Mechanical sensitivity of the masseter muscle was determined prior to and 1, 4, 7, 14, 21 and 28 days after the CFA injection in the masseter muscle.
To examine the role of intraganglionic ROS in inflammatory mechanical hyperalgesia, a ROS scavenger, phenyl N-tert-butylnitrone (PBN) [0.1 mg in 5 μl, a dose shown to produce analgesic effects (9, 31, 32)] or vehicle control (PBS), was administered directly into TG. For preemptive scavenging of ROS, PBN or vehicle was administered 30 min prior to the CFA treatment. For the post-CFA scavenging of ROS, PBN or vehicle was administered 1, 3 and 7 days after CFA treatment in the same animal. The effect of PBN or vehicle on CFA-indued mechanical sensitivities was assessed one hour later. The contribution of intraganlionic TRPA1 in inflammatory mechanical hyperalgesia was examined similarly to the PBN experiment detailed above. AP18 (20 mM in 5 μl), a TRPA1 antagonist, or the same volume of vehicle (1% DMSO, 10% Tween80 in PBS) was administered directly into TG 1, 3 and 7 days after CFA treatment in the same animal. The post AP18 or vehicle effect on masseter mechanical sensitivity was measured 40 min after the injection. The concentration of AP18 we used was higher than what we used in previous behavior studies, where AP18 was administered intramuscularly (33, 34). We chose to use a higher concentration of AP18 because we were making a focal injection into the trigeminal ganglion with a very small volume (5 μl).
2.5.2. Evaluation of spontaneous muscle pain
We used the Rat Grimace Scale (RGS) assay to examine whether exogenous administration of H2O2 directly into TG can elicit spontaneous pain and whether AP18 treatment can attenuate the H2O2-induced pain. Video Imaging: Rats were acclimated to the testing environment for 2 to 3 days prior to the behavioral assessment. Rats were placed in a transparent, 9 × 5 × 8-inch acrylic container during behavioral experiments. Behavior was monitored via two cameras (Sony Handycam HDR-CX405) placed on the opposing, long sides of the container. For baseline data collected prior to an injection in TG, rats were placed in the container for 5 min of habituation before facial expressions were recorded for 10 min. Following recording the baseline data, H2O2 (20 μM in 5 μl) was co-administered with AP18 (20 mM in 5 μl) or vehicle (1% DMSO, 10% Tween 80 in PBS) directly into TG. Forty minutes after the intraganlionic administration, changes in facial expressions were recorded for an additional 10 min. Scoring System for RGS: To capture face image of rats in an unbiased manner, images were manually extracted from each 10-minute video segment by a blinded observer. When possible, one image was captured at every 60 s interval within the 10-minute recording time. Images were defined as valid and saved for further analysis if the image contained a clear shot of the whiskers, and at least one eye and ear. Images were not captured during sleeping, active sniffing, or grooming. Up to 10 images of each rat were obtained and cropped to enlarge facial features. Video analysis did not always yield 10 valid images, and in these cases the 60 s intervals that did not provide valid images were skipped and no image was captured. Two blinded scorers rated four action units of RGS for each image. Four specific facial action units (AUs) were scored: orbital tightening, nose and cheek flattening, ear position, and whisker change (35, 36). The observer scored 0, 1, or 2 for each AU based on the criteria described previously by Sotocinal et al., (2011) (36). The scores of the four AUs were averaged to yield a RGS score for each individual image. The RGS scores of every image, belonging to one animal, were averaged to yield an overall RGS score for that animal.
2.6. Dissociation of rat TG neurons
The procedures for primary TG cultures are described previously (37, 38, 39). Both TG from each animal were dissected out and dissociated by sequential digestion with 0.1% collagenase D in DMEM-F12 medium (with L-glutamine) at 37°C for 30 min, followed by additional digestion in a medium containing 0.25% trypsin, 50 μg DNase, and 0.02% EDTA at 37°C for 15 min. After trituration, cells were plated on laminin pre-coated 24-well plates and cultured in a 37°C incubator at 5% CO2 for 1 to 3 days.
2.7. Real-time RT-PCR
To examine whether ROS are involved in the transcription of the TRPA1 gene in TG neurons, we took two complimentary approaches. First, we treated TG primary culture with H2O2 (10 μmol, 1 h). Second, we administered a ROS donor, t-BOOH (2 μmol/10 μl, 2 consecutive days), directly into TG of intact animals without inflammation. Total RNA was extracted from either the dissociated TG cells in culture or dissected TG from intact animals, respectively, using an RNeasy kit (Qiagen Sciences, Germantown, MD) followed by DNase treatment to remove genomic DNA. Reverse transcription was carried out using SuperScript II kit (Invitrogen, Waltham, MA) was used to generate cDNA from 500 ng of RNA along with 2.5 ng of random primer per reaction. Real-time PCR analysis of cDNA (equal to 15 ng of RNA) was performed using Maxima SYBR Green/ROX qPCR Master Mix in an Eppendorf Mastercycler Ep Realplex 2.0 (Fermentas, Forest City, CA, USA). In all our RT-PCR experiments, each sample was analyzed in triplicates, and we routinely added a control with no template as a means of checking for any nucleic acid contamination, and a control with no reverse transcriptase to verify that there was no DNA contamination in the RNA preparation. The no template control also serves to identify any potential formation of primer dimers during the SYBR Green assay. The following primer pairs were used to detect Trpa1 mRNA: forward 5′-TCCTATACTG GAAGCAGCGA-3′, reverse 5′-CTCCTGATTGCCATC GACT-3′, and GAPDH, mRNA, used as a control: forward 5′-TCACCACCAT GGAGAAGGC G-3′, reverse 5′-GCTAAGCAGTTGGTG GTGCA-3′. We obtained the ratios between Trpa1 and GAPDH to calculate the relative abundance of mRNA levels in each sample. Relative quantification of the Trpa1 mRNA was calculated by the comparative CT method (2−ΔΔCT method) between control and experimental groups.
2.8. Western blotting
Total proteins were extracted from the TG of naïve and CFA treated rats. The protein samples were dissolved in RIPA buffer containing protease inhibitor cocktail. The protein concentration of lysates was determined using Bio-Rad protein assay kit (Bio-Rad, Hercules, CA, USA). Fifty micrograms of protein for each sample were separated on 4%–12% NuPAGE gel with MOPS SDS running buffer and transferred to a PVDF membrane (Bio-rad, Hercules, CA, USA). After blocking for 1 h in 5% milk PBST at room temperature, membranes were probed with primary antibodies for TRPA1 (1:5,000, Millipore #ABN-1009, Burlington, MA) and an internal control protein GAPDH (1:5,000, Calbiochem, San Diego, CA), diluted in blocking solution. The TRPA1 antibody was raised against the N-terminus of rat TRPA1 and detects a 90–98-kDa protein, which disappears in TG lysates probed with TRPA1 antibody pre-incubated with a commercially available peptide used to generate the antibody. We have validated the specificity of this antibody in our previous study (33). Membranes from TG samples were incubated with primary antibodies overnight at 4°C and washed four times with PBST. HRP-conjugated secondary antibodies (anti-rabbit secondary antibody (Cell Signaling, Danvers, MA) and anti- mouse secondary antibody (Millipore, Burlington, MA) were diluted to 1:5,000 in PBST and incubated with membranes for 1 h at room temperature. Bands were visualized using ECL (Western Lightning, PerkinElmer Inc., Waltham, MA, USA) or ECL plus Western blotting detection reagent (Lumigen PS-3, GE Healthcare, Chicago, IL). Protein level for TRPA1 was normalized to that of GAPDH within the same sample.
2.9. Statistical analyses
The time-dependent changes in mechanical hyperalgesia before and after CFA or vehicle were analyzed with a Two-Way analysis of variance (ANOVA) with repeated measures. Data obtained from RT-PCR and Western blot experiments were analyzed with a one-way ANOVA on means or Kruskal–Wallis one-way ANOVA on ranks depending on the outcome of a normality test. Unless otherwise indicated, statistical comparisons of two independent groups were made with either Student's t- test or Mann–Whitney Rank Sum test. Data are presented as mean ± SE and differences were considered significant at p < 0.05. All multiple group comparisons were followed by Bonferroni post hoc test. Power analyses were conducted to determine the minimum sample size required for the behavioral experiments to detect a significant effect with a power of 0.8. We used G*Power Software (Heinrich-Heine, Universität Düsseldorf) to confirm that the sample sizes we used yielded a power greater than 0.85 even with a moderate effect size of 0.5.
3. Results
3.1. CFA treatment in the masseter muscle results in prolonged mechanical hyperalgesia and ROS upregulation within TG
We previously demonstrated that an injection of CFA in the rat masseter induces a time-dependent and significant decrease in mechanical thresholds that lasts over 3 weeks (33, 38). We confirmed these results, showing the development of mechanical hypersensitivity following CFA treatment in the masseter. Our data show that there were significant treatment (F = 157.7) and time (F = 89.20) effects, as well as significant interactions between treatment and time (F = 96.31), with a peak decrease in mechanical thresholds during the first 3 days (p < 0.0001 for pre-injection vs. post-CFA values, and p < 0.0001 for CFA vs. vehicle groups). A significant decrease in mechanical threshold was observed until day 14 (p < 0.001 for pre-injection vs. post-CFA values and p < 0.0001 for CFA vs. vehicle groups), which gradually returned to a baseline level by 28 days after CFA treatment (Figure 1A). The vehicle treatment did not alter mechanical thresholds for the entire observation period of 28 days (Figure 1A).
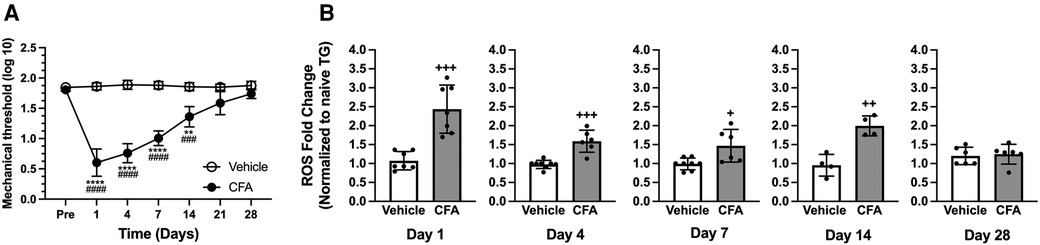
Figure 1. CFA-induced masseter hyperalgesia and ROS upregulation in TG. (A) Line graph shows changes in mechanical hyperalgesia in the masseter muscle following CFA (50 μl of 50% CFA in saline) or vehicle (saline) administration (Intramuscular). Mechanical force (g) that produced the head withdrawal responses in 50% of the trials was log transformed and plotted for pre- and 1, 4, 7, 14, 21, and 28 days post CFA treatment. Two-way ANOVA with repeated measures were used. **p < 0.001 and ****p < 0.0001 for significant time effects compared to the pre-injection values. ###p < 0.001 and ####p < 0.0001 for significant differences between CFA and vehicle groups (n = 8). (B) Changes in ROS within TG following masseter inflammation were assessed by measuring relative intensity of fluorescence using H2DCFDA, an indicator for ROS, of TG obtained from naive, CFA, or vehicle treated rats on days 1, 4, 7, 14 and 28 post CFA treatment. We used 5 to 8 naïve rats for normalization for each time point. Student t-test was used for statistical analysis at each time point. +p < 0.05, ++p < 0.005 and +++p < 0.0005 for significant differences between CFA and vehicle groups. Data are presented as the mean ± SEM.
Our previous study demonstrated ROS levels increase within TG 1 day after masseter inflammation by CFA (20). A more comprehensive temporal profile of intraganglionic ROS under inflammatory conditions needs to be determined to firmly establish the relationship between inflammatory hyperalgesia with intraganglionic ROS. The inflammation-induced production of ROS in TG was assessed by comparing the intensity of fluorescence from ipsilateral TG obtained 1, 4, 7, 14, and 28 days following the injection of CFA or vehicle into the masseter muscle to that of naïve rats. Fluorescent signals from the TG of CFA-injected rats were significantly greater than those from vehicle-treated rats on days 1, 4, 7, and 14 after CFA treatment (Figure 1B, p < 0.0005 for days 1 and 4 post CFA vs. vehicle groups, p < 0.05 for day 7 post CFA vs. vehicle groups, and p < 0.005 for day 14 post CFA vs. vehicle groups). On day 28, intraganglionic levels of ROS were no longer significantly different between the two groups (Figure 1B). We have previously shown that the ROS level in TG contralateral to the CFA-injection site was not significantly different from that of naïve rats (20). These results demonstrate that masseter inflammation upregulates ROS production within TG, especially during the period when inflammatory mechanical hyperalgesia is pronounced. We conducted the present study using only male rats since the CFA condition we employed produces comparable levels and duration of masseter hyperalgesia in both male and female rats (38). We assumed that shared mechanisms account for the similar behavioral responses in both sexes under our CFA condition. However, we acknowledge that there may be potential differences in ROS accumulation between males and females that require further investigation.
3.2. Scavenging of ROS within TG attenuates CFA-induced mechanical hyperalgesia
To examine the functional involvement of intraganglionic ROS in the development of mechanical hyperalgesia, phenyl N-tert-butylnitrone (PBN) was injected directly into TG 30 min prior to CFA treatment in the masseter muscle. There were significant treatment (F = 29.33) and time (F = 390.3) effects, as well as significant interactions between treatment and time (F = 55.98). Preemptive intraganglionic PBN, but not vehicle, treatment significantly attenuated mechanical hyperalgesia one day after CFA treatment (Figure 2A, p < 0.005 for PBN vs. vehicle groups). However, PBN was no longer effective on day 2 (Figure 2A). We then examined whether scavenging of ROS within TG can also attenuate fully developed inflammatory mechanical hyperalgesia. There were significant treatment (F = 21.24) and time (F = 165.0) effects, as well as significant interactions between treatment and time (F = 47.09). PBN administered in TG one day after CFA treatment in the masseter muscle significantly blunted mechanical hyperalgesia (Figure 2B, p < 0.01 for PBN vs. vehicle groups). The PBN effect was transient, lasting only a few hours. Once the effect wears off, hyperalgesia returned as shown by pre-treatment measures at each time point. Interestingly, when the same concentration of PBN was administered in the same animals again on day 3 after CFA treatment, it blocked the CFA-induced mechanical hyperalgesia to a greater extent (Figure 2B, p < 0.0005 for PBN vs. vehicle groups). When PBN was administered a third time on day 7, the mechanical threshold was restored to the pre-CFA baseline sensitivity (Figure 2B). Vehicle administration in TG did not alter mechanical sensitivity at any of the time points (Figure 2B). These data demonstrate that excess production of ROS within TG remote from a peripheral inflammatory site contributes to the development and maintenance of inflammatory hyperalgesia.
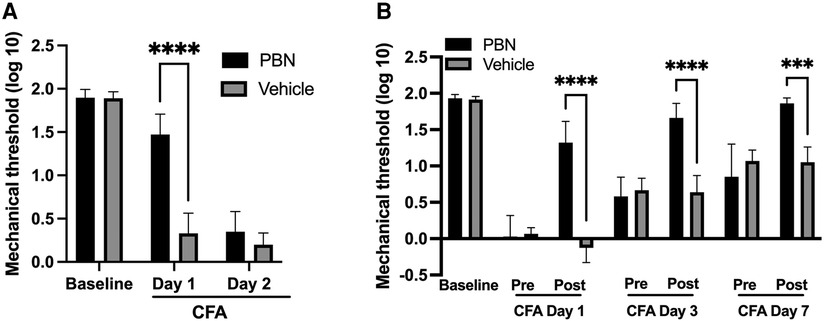
Figure 2. Effects of ROS scavenger (PBN) administration in TG on CFA-induced mechanical hyperalgesia. (A) Phenyl N-tert-butylnitrone (PBN—0.1 mg in 5 μl of PBS) or vehicle was injected directly into TG (intraganlionic) 30 min prior to CFA injection. Mechanical hyperalgesia was attenuated significantly in PBN, but not vehicle (PBS), treated rats when examined 1 day after CFA treatment in the masseter. No PBN effects on day 2 post CFA. (B) PBN or vehicle was administered directly into TG (intraganlionic) 1 day, 3 days and 7 days after CFA injection in the masseter. Each day, mechanical sensitivity was assessed one hour after PBN or saline administration. Two-way ANOVA with repeated measures were used. *p < 0.01, **p < 0.005 and ***p < 0.0005 for significant differences between PBN and vehicle treated groups. Data are presented as the mean ± SEM.
3.3. TRPA1 within TG mediates CFA-induced mechanical hyperalgesia
We have previously shown that CFA-induced mechanical hypersensitivity and spontaneous muscle pain responses were significantly reversed by post-treatment with AP18 in the muscle (33). We have also shown that TRPA1 is amply expressed in the soma of small to medium size TG neurons (34). In order to examine whether TRPA1 expressed within TG could also functionally contribute to inflammatory hyperalgesia, we administered AP18 directly into TG 1, 3 and 7 days after CFA treatment in the masseter muscle. AP18, but not the vehicle, administration in TG one day after CFA treatment in the masseter muscle significantly attenuated the mechanical hyperalgesia (Figure 3, p < 0.0005 for AP18 vs. vehicle groups). The inflamed muscle, however, became hypersensitive again within 24 h of AP18 treatment (Figure 3). The same concentration of AP18 administered in the same animals both 1 day and 3 days after CFA treatment blocked the CFA-induced mechanical hyperalgesia to a similar extent of single AP18 treatment on day 1 (Figure 3, p < 0.0005 for AP18 vs. vehicle groups). The additional administration of AP18 on day 7 continued to be effective (p < 0.005 for AP18 vs. vehicle groups), but it did not further reduce the mechanical hyperalgesia beyond the extent observed on days 1 and 3.
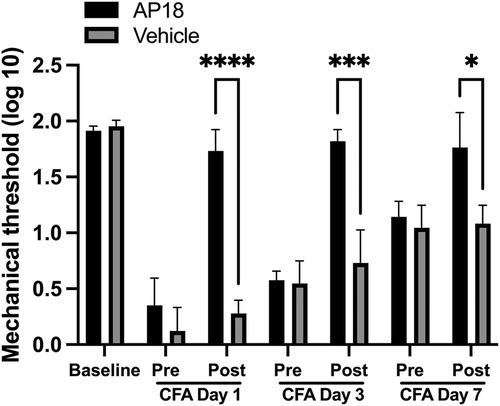
Figure 3. Effects of TRPA1 antagonist (AP18) treatments in TG on CFA-induced mechanical hyperalgesia. AP18 (20 mM in 5 μl) or vehicle (1% DMSO, 10% Tween80 in PBS) was administered directly into TG (intraganlionic) 1 day, 3 days and 7 days after CFA injection in the masseter. Each day, mechanical sensitivity was assessed one hour after AP18 or vehicle administration. Two-way ANOVA with repeated measures were used. *p < 0.005 and **p < 0.0005 for significant differences between PBN and vehicle treated groups. Data are presented as the mean ± SEM.
3.4. Direct injection of ROS into TG induces mechanical hyperalgesia and facial pain in a TRPA1-dependent manner
Since both PBN and AP18 administered directly into TG effectively attenuated inflammatory hyperalgesia, and ROS are known to directly activate TRPA1, we investigated whether exogenous administration of ROS can induce pain-related responses without peripheral inflammation and whether that effect is mediated by TRPA1. Since the effects of H2O2 are expected to be short-lived, we evaluated evoked and spontaneous pain responses within an hour of H2O2 administration. Initially, we directly administered H2O2 into the trigeminal ganglion (TG) with or without AP18 and assessed masseter mechanical hypersensitivity. Direct administration of H2O2 into TG (20 μM in 5 μl) resulted in a significant decrease in the mechanical sensitivity of the masseter muscle, indicating the development of profound hyperalgesia (Figure 4A; p < 0.0001 for before vs. after). However, when the same concentration of H2O2 was co-administered with AP18, it effectively prevented H2O2-induced masseter hyperalgesia (Figure 4B). We also assessed RGS since H2O2 administration in TG could produce a widespread spontaneous pain not limited to selective orofacial regions. Animals administered with either AP18 or vehicle did not show any changes in facial expression prior to H2O2 administration. However, when H2O2 was administered, rats exhibited visible changes in facial expressions, indicated by a high RGS score. The increased RGS score due to H2O2 treatment was significantly attenuated by co-administration with AP18 (Figure 4C, p < 0.005 for AP18 vs. vehicle groups).
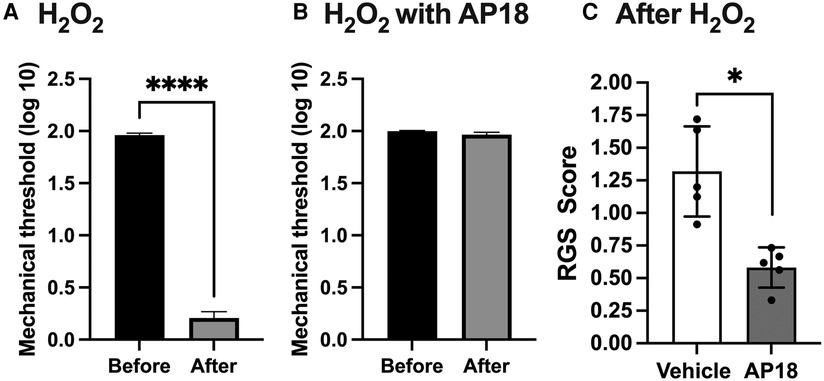
Figure 4. Intraganglionic ROS elicits mechanical hyperalgesia and spontaneous pain via TRPA1. (A) H2O2 (30 μM in 5 μl) was administered directly into TG (intraganlionic) and mechanical sensitivity was assessed 40 min after. Masseter sensitivity was attenuated significantly in rats that received H2O2 (****p < 0.0001). (B). AP18 (20 mM in 5 μl) was administered directly into TG (intraganlionic) and masseter sensitivity was evaluated after H2O2 administration into TG. (C) AP18 (20 mM in 5 μl) or vehicle (1% DMSO, 10% Tween80 in PBS) was co-administered directly into TG (intraganlionic) with H2O2 (20 μM in 5 μl) and the RGS assay was evaluated (There were no noticeable face grimace behaviors prior to H2O2 administration). RGS was attenuated significantly in rats that received H2O2 and AP18 co-administration (*p < 0.005). Data are presented as the mean ± SEM.
3.5. ROS induces TRPA1 upregulation in TG
Since intraganglionic ROS have direct access to transcriptional machineries within the soma, we examined whether ROS are involved in the transcription of the Trpa1 gene in TG. Relative changes in the mRNA levels of the Trpa1 gene was determined from H2O2-treated primary TG culture samples (10 μmol, 1 h). H2O2 treatment induced a significant increase in TRPA1 (Figure 5A, p < 0.05 for H2O2 vs. naive groups). To confirm that ROS build up in TG can induce a transcriptional increase of Trpa1, we administered a ROS donor, t-BOOH, directly into TG of intact animals without inflammation. t-BOOH significantly upregulated the transcript levels of TRPA1 in TG (Figure 5B, p < 0.0001 for t-BOOH vs. naive groups), suggesting that ROS alone is sufficient to induce transcriptional changes of Trpa1 in TG. In our previous study, we showed that CFA-induced masseter inflammation results in a time-dependent increase in the level of Trpa1 mRNA in TG (33). The level of Trpa1 mRNA TG is significantly upregulated on days 1, 3 and 7 compared to the baseline. To examine whether ROS buildup in TG can increase TRPA1 protein expression, we administered PBN or vehicle directly into TG on days 1, 3, and 7 of CFA treatment. Here, we confirmed that TRPA1 protein expression is significantly upregulated on day 7 following CFA treatment in the masseter muscle in vehicle treated rats compared to that of naïve rats (Figure 6). However, TRPA1 expression levels in PBN treated rats were significantly lower compared to vehicle treated rats (Figure 6, p < 0.05 for Saline vs. PBN groups), suggesting that excess intraganglionic ROS under inflammatory conditions induce upregulation of TRPA1 expression in TG.
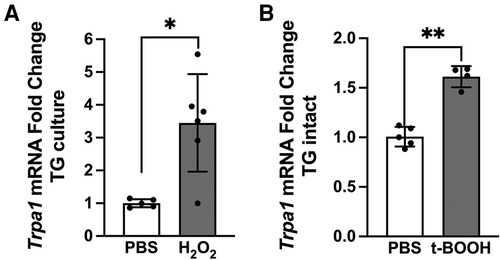
Figure 5. Intraganglionic ROS mediates TRPA1 upregulation. (A) H2O2 (10 μmol, 1 h) treatment in TG culture induces significant increase in Trpa1 mRNA expression (*p < 0.005). (B) Four rats implanted with cannula in left TG received the ROS donor t-BOOH (2 μmol in 10 μl, intragangionic) for two consecutive days. TG were extracted 24 h after the last injection for RT-PCR. Each sample was analyzed in triplicates. t-BOOH treatment in intact TG induces significant increase in Trpa1 mRNA expression (**p < 0.0001). Data are presented as the mean ± SEM.
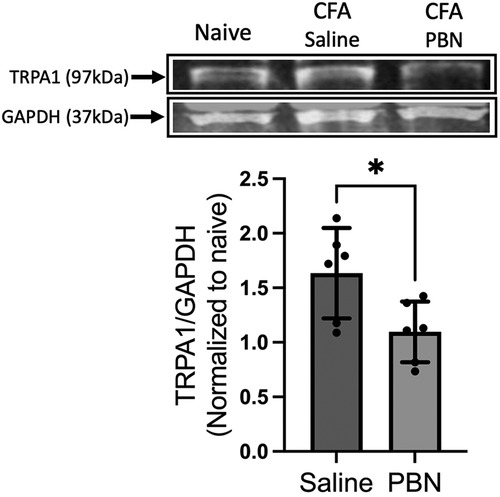
Figure 6. Effects of intraganglionic PBN treatment on CFA-induced upregulation of TRPA1 protein expression. (Top) Representative blots for TRPA1 protein in TG of naïve rats, CFA-inflamed rats (7 days post CFA) with either vehicle or (PBN 0.1 mg in 5 μl) administration. Vehicle or PBN was administered directly into TG (intraganlionic) on days 1, 3, and 7 after the CFA injection in the masseter muscle. (Bottom) Averaged relative optical density (TRPA1/GAPDH). (*p < 0.05). Data are presented as the mean ± SEM.
4. Discussion
We have previously shown that masseter muscle inflammation by CFA results in a significantly elevated level of ROS within the TG of intact animals 24 h after the treatment (20). However, the extent to which ROS expression is altered within TG under pathological pain conditions, as well as how ROS levels in TG contribute to pain and hyperalgesia, is unknown. In the current study, CFA-induced ROS accumulation in TG is maintained at least for 14 days. Such prolonged elevation of ROS in TG could cause a sustained increase in pronociceptive gene expression (40), generation of reactive aldehydes via ROS-dependent lipid peroxidation which could further amplify oxidative stress (2), and activation of TRPA1 by reactive aldehydes that exacerbate neurogenic inflammation (13, 41).
Several findings of the current study provide strong evidence that ROS confined to TG are sufficient to functionally contribute to inflammatory pain responses. First, the time course of ROS elevation paralleled the period of profound mechanical hyperalgesia at the inflamed site and, second, that intraganlionic PBN effectively attenuated CFA-induced mechanical hyperalgesia during this period. It is interesting to note that repetitive treatment with PBN had a cumulative analgesic effect on inflammatory mechanical hyperalgesia. Since PBN has a terminal half-life of 2.01 +/- 0.35 h when administered intravenously in Sprague Dawley rats (42), it is unlikely that the cumulative analgesic effect is due to PBN accumulation in TG. Instead, scavenging ROS could prevent subsequent generation of reactive aldehydes that exert prolonged activity, and therefore act as a second toxic messenger augmenting initial ROS events (28). Thus, ROS-initiated downstream signaling cascades could maintain pain hypersensitivity even after the excess ROS level dissipates to that of the pre-CFA level. Additionally, preemptive treatment of TG with PBN transiently, but significantly, attenuated CFA-induced mechanical hyperalgesia, suggesting that intraganlionic ROS also play a role in the development of inflammatory hyperalgesia. Preemptive administration of ROS scavengers effectively ameliorate inflammatory, neuropathic, and chemotherapy-induced pain responses when given systemically (7, 8, 18, 31, 43). While findings from these studies demonstrated the importance of ROS in local tissue, as well as in the spinal cord dorsal horn, in the development of pathological pain responses, a relative contribution of intraganlionic ROS has not been systematically examined. Lastly, our data showed that direct activation of ROS cascades in TG without peripheral inflammation is sufficient to elicit spontaneous pain. While we did not examine persistent effects of H2O2 on spontaneous pain, our data are consistent with a recent study that showed a rapid and persistent mechanical hypersensitivity following direct administration of H2O2 into the DRG of naïve rats (44). Together, our data suggest that TG is an important source of ROS and that intraganlionic ROS participate in the pathogenesis of inflammatory pain, making TG a viable therapeutic target for orofacial pain management.
There is ample evidence that ROS mediate their cellular effects via multiple types of TRP channels, including TRPA1, TRPV1, and TRPM2. Among those TRP channels, TRPA1 has the highest oxidation sensitivity and is therefore regarded as a redox-sensitive channel (11, 45). ROS directly activate TRPA1 by inducing cysteine oxidation and by promoting disulfide formation between proximal cysteine residues in TRPA1 (46). Direct application of H2O2 to meninges, for example, induces electrical spiking activity of trigeminal nerves in a TRPA1-dependent manner (47). ROS can also enhance the spontaneous release of glutamate from presynaptic terminals onto spinal cord dorsal horn neurons through TRPA1, inducing central sensitization at the central terminals of primary afferent neurons (19). ROS can also indirectly activate TRPA1 by the nonenzymatic generation of endogenous reactive aldehydes (48). There is strong evidence that oxidative aldehydes, such as 4-hydroxy-2E-nonenal (4-HNE), activate TRPA1 through covalent modification of cysteine and lysine residues located within the amino-terminal cytoplasmic domain of the channel (13, 49, 50), and that 4-HNE activation of TRPA1 mediates inflammatory and neuropathic pain (12, 13). Furthermore, TRPA1 activation by H2O2 has been shown to evoke additional H2O2 release in melanoma cell lines that further amplify the oxidative stress (51). Therefore, ROS and TRPA1 interactions in any stage of nociceptive processing can lead to sensitization of neurons, promoting pain hypersensitivity. Here, for the first time, we characterize ROS effect of TRPA1 expression within TG and investigate the functional relationship between intraganlionic ROS and TRPA1.
In the current study, we demonstrate that the blockade of intraganglionic TRPA1 effectively mitigated the CFA-induced mechanical hyperalgesia at various time points during the inflammation. It is possible that excess ROS within TG can directly and indirectly activate TRPA1 expressed in soma of masseter afferents (34), contributing to inflammatory muscular hyperalgesia. Our data demonstrating that spontaneous pain and masseter hypersensitivity elicited by exogenous administration of H2O2 into TG is blocked by a TRPA1 antagonist supports this hypothesis. Our hypothesis is further supported by a recent study showing that TRPA1 activation by ROS and carbonyl species in the soma of TG maintain glyceryl trinitrate-induced periorbital allodynia (41). Collectively, these observations provide strong evidence that interactions between ROS and TRPA1 within sensory ganglia functionally contribute to chronic pain. Previous studies have demonstrated that the soma of primary afferent neurons functionally contribute to chronic pain by intraganlionic communication between a population of DRG neurons, also referred to as cross-excitation (52, 53). More recent studies implicate intraganlionic sensory transmission as a mechanism by which sensory input from one type of tissue can exert sensitizing effects through widespread actions of chemical mediators, such as calcitonin gene-related peptide, on neighboring neurons within the ganglia (54, 55). In this context, cellular mechanisms mediating intraganlionic ROS-TRPA1 interactions, as well as the relative contribution of such interactions in various chronic pain conditions, merit further investigation and could potentially be a therapeutic target.
TRPA1 expression level is highly correlated with inflammatory hyperalgesia (24, 56, 57). We previously reported that CFA-induced masseter hyperalgesia is accompanied by significant up-regulation of Trpa1 mRNA expression in TG (33, 58). While our understanding of the mechanisms regulating Trpa1 transcription in TG under inflammatory conditions is limited, our data suggest that intraganlionic ROS can activate the transcriptional machineries of the Trpa1 gene. ROS could lead to Trpa1 gene upregulation by inflammatory cytokines. TNF-α and IL-1β increase TRPA1 expression in human lung epithelial cells (59), and TRPA1 expression was also significantly upregulated by TNF-α in human odontoblast-like cells (60). Notably, mice with a null mutation of glycoprotein 130, a signal transducing subunit for IL-6, show reduced Trpa1 mRNA expression in DRG (25). It is well known that oxygen-derived free radicals, such as H2O2, stimulate the synthesis of multiple inflammatory cytokines and chemokines in a variety of cells. H2O2 increases the production of IL-6 and IL-8 in airway epithelia (61), IL-1β, IL-6, TNF-α, and TGF-β1 in cardiac fibroblasts (62), TNF-α in DRG cells (10), and IL-6 in TG neurons and satellite glia (20). Interactions of ROS with NFκB is also well known (63). In a previous study, we demonstrated that the accumulation of ROS in the TG upregulated the mRNA and protein levels of IL-6 and chemokine (C-X-C motif) ligand 2 (CXCL2) via the transient receptor potential melastatin 2 (TRPM2) channels, which is expressed in both neurons and satellite glial cells (20). It is known that TRPM2 is directly activated by ROS, including H2O2 (64). Therefore, the accumulation of ROS in TG can activate TRPM2, which in turn generates inflammatory cytokines and chemokines via NFκB pathways, leading to increased expression of the TRPA1 channel.
Oxidative stress is also known to cause a wide range of DNA modifications, such as base modifications, strand breakage, and chromosomal rearrangements. Such modifications have been shown to interfere with the activities of DNA methyl transferases (DNMTs), resulting in global hypomethylation (65). As a prototypical epigenetic factor, DNA methylation plays an important role in regulating gene expression, and alterations in DNA methylation in DRG play a critical role in the underlying mechanisms of many types of somatic chronic pain conditions (66, 67, 68). CFA-induced masseter inflammation leads to both global reductions in DNA methylation and to downregulation of DNMT1 and DNMT3a expression in TG, and the reduction of DNMT3a leads to a decrease in promoter methylation of Trpv1 and Trpa1, which results in their upregulation (37). Thus, intraganglionic ROS could serve as the mechanistic link between peripheral inflammation and the transcription of pro-nociceptive genes, such as Trpa1, by reducing DNA methylation.
Taken together, results from this study demonstrate that excess intraganlionic ROS generated in response to peripheral inflammation functionally contributes to pain and hyperalgesia. Thus, the soma of nociceptors, in addition to peripheral and central terminals, are important sites for the pathogenesis of inflammatory pain. Furthermore, oxidative stress induces transcriptional upregulation of pronociceptive genes, ultimately contributing to peripheral sensitization. Therefore, any conditions that exacerbate ROS accumulation within somatic sensory ganglia can aggravate pain responses. Recent studies have shown that natural antioxidants such as curcumin (69), resveratrol (70), Vitamin E (71), and catechins in green tea (72) reduce pain and inflammation in animal models of pain. These treatments have the potential to reduce ganglionic ROS and help alleviate inflammatory pain.
Data availability statement
The original contributions presented in the study are included in the article, further inquiries can be directed to the corresponding author.
Ethics statement
The animal study was reviewed and approved by University of Maryland Baltimore IACUC.
Author contributions
All authors contributed to the article and approved the submitted version.
Acknowledgements
This study was supported by NIH-NIDCR grant DE016062 (JYR).
Conflict of interest
The authors declare that the research was conducted in the absence of any commercial or financial relationships that could be construed as a potential conflict of interest.
Publisher's note
All claims expressed in this article are solely those of the authors and do not necessarily represent those of their affiliated organizations, or those of the publisher, the editors and the reviewers. Any product that may be evaluated in this article, or claim that may be made by its manufacturer, is not guaranteed or endorsed by the publisher.
References
1. Sies H, Jones DP. Reactive oxygen species (ROS) as pleiotropic physiological signalling agents. Nat Rev Mol Cell Biol. (2020) 21(7):363–83. doi: 10.1038/s41580-020-0230-3
2. Hellenthal KEM, Brabenec L, Gross ER, Wagner NM. TRP Channels as sensors of aldehyde and oxidative stress. Biomolecules. (2021) 11(10):1–22. doi: 10.3390/biom11101401
3. Hendrix J, Nijs J, Ickmans K, Godderis L, Ghosh M, Polli A. The interplay between oxidative stress, exercise, and pain in health and disease: potential role of autonomic regulation and epigenetic mechanisms. Antioxidants (Basel). (2020) 9(11):1–25. doi: 10.3390/antiox9111166
4. Li X, Yin C, Hu Q, Wang J, Nie H, Liu B, et al. Nrf2 activation mediates antiallodynic effect of electroacupuncture on a rat model of complex regional pain syndrome type-I through reducing local oxidative stress and inflammation. Oxid Med Cell Longev. (2022) 2022:8035109. doi: 10.1155/2022/8035109
5. Singh AK, Vinayak M. Resveratrol alleviates inflammatory hyperalgesia by modulation of reactive oxygen species (ROS), antioxidant enzymes and ERK activation. Inflamm Res. (2017) 66(10):911–21. doi: 10.1007/s00011-017-1072-0
6. Westlund KN, Kochukov MY, Lu Y, McNearney TA. Impact of central and peripheral TRPV1 and ROS levels on proinflammatory mediators and nociceptive behavior. Mol Pain. (2010) 6:46. doi: 10.1186/1744-8069-6-46
7. Xue Y, Dai S, Liang J, Ji W. Effect of reactive oxygen species of the psoas major muscle in complete freund’s adjuvant-induced inflammatory pain in rats. Mol Pain. (2020) 16:1744806920929246. doi: 10.1177/1744806920929246
8. Shim HS, Bae C, Wang J, Lee KH, Hankerd KM, Kim HK, et al. Peripheral and central oxidative stress in chemotherapy-induced neuropathic pain. Mol Pain. (2019) 15:1744806919840098. doi: 10.1177/1744806919840098
9. Wang J, Cochran V, Abdi S, Chung JM, Chung K, Kim HK. Phenyl N-t-butylnitrone, a reactive oxygen species scavenger, reduces zymosan-induced visceral pain in rats. Neurosci Lett. (2008) 439(2):216–9. doi: 10.1016/j.neulet.2008.05.018
10. Ma F, Zhang L, Westlund KN. Reactive oxygen species mediate TNFR1 increase after TRPV1 activation in mouse DRG neurons. Mol Pain. (2009) 5:31. doi: 10.1186/1744-8069-5-31
11. Kashio M, Tominaga M. Redox-Sensitive TRP channels: tRPA1 and TRPM2. In: Khalid M, editors. Redox principles and advanced applications. Croatia: IntechOpen (2017). p. 203–23
12. Trevisan G, Benemei S, Materazzi S, De Logu F, De Siena G, Fusi C, et al. TRPA1 Mediates trigeminal neuropathic pain in mice downstream of monocytes/macrophages and oxidative stress. Brain. (2016) 139(Pt 5):1361–77. doi: 10.1093/brain/aww038
13. Trevisani M, Siemens J, Materazzi S, Bautista DM, Nassini R, Campi B, et al. 4-Hydroxynonenal, An endogenous aldehyde, causes pain and neurogenic inflammation through activation of the irritant receptor TRPA1. Proc Natl Acad Sci U S A. (2007) 104(33):13519–24. doi: 10.1073/pnas.0705923104
14. Jaramillo M, Olivier M. Hydrogen peroxide induces murine macrophage chemokine gene transcription via extracellular signal-regulated kinase- and cyclic adenosine 5′-monophosphate (cAMP)-dependent pathways: involvement of NF-kappa B, activator protein 1, and cAMP response element binding protein. J Immunol. (2002) 169(12):7026–38. doi: 10.4049/jimmunol.169.12.7026
15. Lakshminarayanan V, Beno DW, Costa RH, Roebuck KA. Differential regulation of interleukin-8 and intercellular adhesion molecule-1 by H2O2 and tumor necrosis factor-alpha in endothelial and epithelial cells. J Biol Chem. (1997) 272(52):32910–8. doi: 10.1074/jbc.272.52.32910
16. Gao X, Kim HK, Mo Chung J, Chung K. Reactive oxygen species (ROS) are involved in enhancement of NMDA-receptor phosphorylation in animal models of pain. Pain. (2007) 131(3):262–71. doi: 10.1016/j.pain.2007.01.011
17. Lee DZ, Chung JM, Chung K, Kang MG. Reactive oxygen species (ROS) modulate AMPA receptor phosphorylation and cell-surface localization in concert with pain-related behavior. Pain. (2012) 153(9):1905–15. doi: 10.1016/j.pain.2012.06.001
18. Yowtak J, Wang J, Kim HY, Lu Y, Chung K, Chung JM. Effect of antioxidant treatment on spinal GABA neurons in a neuropathic pain model in the mouse. Pain. (2013) 154(11):2469–76. doi: 10.1016/j.pain.2013.07.024
19. Nishio N, Taniguchi W, Sugimura YK, Takiguchi N, Yamanaka M, Kiyoyuki Y, et al. Reactive oxygen species enhance excitatory synaptic transmission in rat spinal dorsal horn neurons by activating TRPA1 and TRPV1 channels. Neuroscience. (2013) 247:201–12. doi: 10.1016/j.neuroscience.2013.05.023
20. Chung MK, Asgar J, Lee J, Shim MS, Dumler C, Ro JY. The role of TRPM2 in hydrogen peroxide-induced expression of inflammatory cytokine and chemokine in rat trigeminal ganglia. Neuroscience. (2015) 297:160–9. doi: 10.1016/j.neuroscience.2015.03.067
21. Bautista DM, Pellegrino M, Tsunozaki M. TRPA1: a gatekeeper for inflammation. Annu Rev Physiol. (2013) 75:181–200. doi: 10.1146/annurev-physiol-030212-183811
22. Koivisto A, Jalava N, Bratty R, Pertovaara A. TRPA1 Antagonists for pain relief. Pharmaceuticals (Basel). (2018) 11(4):1–19. doi: 10.3390/ph11040117
23. Chen J, Winston JH, Sarna SK. Neurological and cellular regulation of visceral hypersensitivity induced by chronic stress and colonic inflammation in rats. Neuroscience. (2013) 248:469–78. doi: 10.1016/j.neuroscience.2013.06.024
24. DeBerry JJ, Schwartz ES, Davis BM. TRPA1 Mediates bladder hyperalgesia in a mouse model of cystitis. Pain. (2014) 155(7):1280–7. doi: 10.1016/j.pain.2014.03.023
25. Malsch P, Andratsch M, Vogl C, Link AS, Alzheimer C, Brierley SM, et al. Deletion of interleukin-6 signal transducer gp130 in small sensory neurons attenuates mechanonociception and down-regulates TRPA1 expression. J Neurosci. (2014) 34(30):9845–56. doi: 10.1523/JNEUROSCI.5161-13.2014
26. Hatano N, Itoh Y, Suzuki H, Muraki Y, Hayashi H, Onozaki K, et al. Hypoxia-inducible factor-1alpha (HIF1alpha) switches on transient receptor potential ankyrin repeat 1 (TRPA1) gene expression via a hypoxia response element-like motif to modulate cytokine release. J Biol Chem. (2012) 287(38):31962–72. doi: 10.1074/jbc.M112.361139
27. Oh MH, Oh SY, Lu J, Lou H, Myers AC, Zhu Z, et al. TRPA1-dependent Pruritus in IL-13-induced chronic atopic dermatitis. J Immunol. (2013) 191(11):5371–82. doi: 10.4049/jimmunol.1300300
28. Ambalavanar R, Moutanni A, Dessem D. Inflammation of craniofacial muscle induces widespread mechanical allodynia. Neurosci Lett. (2006) 399(3):249–54. doi: 10.1016/j.neulet.2006.02.003
29. Imbe H, Dubner R, Ren K. Masseteric inflammation-induced Fos protein expression in the trigeminal interpolaris/caudalis transition zone: contribution of somatosensory-vagal-adrenal integration. Brain Res. (1999) 845(2):165–75. doi: 10.1016/S0006-8993(99)01913-7
30. Ren K. An improved method for assessing mechanical allodynia in the rat. Physiol Behav. (1999) 67(5):711–6. doi: 10.1016/S0031-9384(99)00136-5
31. Kim HK, Park SK, Zhou JL, Taglialatela G, Chung K, Coggeshall RE, et al. Reactive oxygen species (ROS) play an important role in a rat model of neuropathic pain. Pain. (2004) 111(1-2):116–24. doi: 10.1016/j.pain.2004.06.008
32. Lee I, Kim HK, Kim JH, Chung K, Chung JM. The role of reactive oxygen species in capsaicin-induced mechanical hyperalgesia and in the activities of dorsal horn neurons. Pain. (2007) 133(1-3):9–17. doi: 10.1016/j.pain.2007.01.035
33. Asgar J, Zhang Y, Saloman JL, Wang S, Chung MK, Ro JY. The role of TRPA1 in muscle pain and mechanical hypersensitivity under inflammatory conditions in rats. Neuroscience. (2015) 310:206–15. doi: 10.1016/j.neuroscience.2015.09.042
34. Ro JY, Lee JS, Zhang Y. Activation of TRPV1 and TRPA1 leads to muscle nociception and mechanical hyperalgesia. Pain. (2009) 144(3):270–7. doi: 10.1016/j.pain.2009.04.021
35. Langford DJ, Bailey AL, Chanda ML, Clarke SE, Drummond TE, Echols S, et al. Coding of facial expressions of pain in the laboratory mouse. Nat Methods. (2010) 7(6):447–9. doi: 10.1038/nmeth.1455
36. Sotocinal SG, Sorge RE, Zaloum A, Tuttle AH, Martin LJ, Wieskopf JS, et al. The rat grimace scale: a partially automated method for quantifying pain in the laboratory rat via facial expressions. Mol Pain. (2011) 7:55. doi: 10.1186/1744-8069-7-55
37. Bai G, Ross H, Zhang Y, Lee K, Ro JY. The role of DNA methylation in transcriptional regulation of pro-nociceptive genes in rat trigeminal ganglia. Epigenet Insights. (2020) 13:2516865720938677. doi: 10.1177/2516865720938677
38. Niu KY, Zhang Y, Ro JY. Effects of gonadal hormones on the peripheral cannabinoid receptor 1 (CB1R) system under a myositis condition in rats. Pain. (2012) 153(11):2283–91. doi: 10.1016/j.pain.2012.07.037
39. Zhang X, Zhang Y, Asgar J, Niu KY, Lee J, Lee KS, et al. Sex differences in mu-opioid receptor expression in trigeminal ganglia under a myositis condition in rats. Eur J Pain. (2014) 18(2):151–61. doi: 10.1002/j.1532-2149.2013.00352.x
40. Remacle J, Raes M, Toussaint O, Renard P, Rao G. Low levels of reactive oxygen species as modulators of cell function. Mutat Res. (1995) 316(3):103–22. doi: 10.1016/0921-8734(95)90004-7
41. Marone IM, De Logu F, Nassini R, De Carvalho Goncalves M, Benemei S, Ferreira J, et al. TRPA1/NOX In the soma of trigeminal ganglion neurons mediates migraine-related pain of glyceryl trinitrate in mice. Brain. (2018) 141(8):2312–28. doi: 10.1093/brain/awy177
42. Trudeau-Lame ME, Kalgutkar AS, LaFontaine M. Pharmacokinetics and metabolism of the reactive oxygen scavenger alpha-phenyl-N-tert-butylnitrone in the male Sprague-Dawley rat. Drug Metab Dispos. (2003) 31(2):147–52. doi: 10.1124/dmd.31.2.147
43. Khattab MM. TEMPOL, a membrane-permeable radical scavenger, attenuates peroxynitrite- and superoxide anion-enhanced carrageenan-induced paw edema and hyperalgesia: a key role for superoxide anion. Eur J Pharmacol. (2006) 548(1-3):167–73. doi: 10.1016/j.ejphar.2006.08.007
44. Xu J, Wu S, Wang J, Wang J, Yan Y, Zhu M, et al. Oxidative stress induced by NOX2 contributes to neuropathic pain via plasma membrane translocation of PKCepsilon in rat dorsal root ganglion neurons. J Neuroinflammation. (2021) 18(1):106. doi: 10.1186/s12974-021-02155-6
45. Takahashi N, Mori Y. TRP Channels as sensors and signal integrators of redox Status changes. Front Pharmacol. (2011) 2:58. doi: 10.3389/fphar.2011.00058
46. Andersson DA, Gentry C, Moss S, Bevan S. Transient receptor potential A1 is a sensory receptor for multiple products of oxidative stress. J Neurosci. (2008) 28(10):2485–94. doi: 10.1523/JNEUROSCI.5369-07.2008
47. Shatillo A, Koroleva K, Giniatullina R, Naumenko N, Slastnikova AA, Aliev RR, et al. Cortical spreading depression induces oxidative stress in the trigeminal nociceptive system. Neuroscience. (2013) 253:341–9. doi: 10.1016/j.neuroscience.2013.09.002
48. Esterbauer H, Schaur RJ, Zollner H. Chemistry and biochemistry of 4-hydroxynonenal, malonaldehyde and related aldehydes. Free Radic Biol Med. (1991) 11(1):81–128. doi: 10.1016/0891-5849(91)90192-6
49. Hinman A, Chuang HH, Bautista DM, Julius D. TRP Channel activation by reversible covalent modification. Proc Natl Acad Sci U S A. (2006) 103(51):19564–8. doi: 10.1073/pnas.0609598103
50. Macpherson LJ, Dubin AE, Evans MJ, Marr F, Schultz PG, Cravatt BF, et al. Noxious compounds activate TRPA1 ion channels through covalent modification of cysteines. Nature. (2007) 445(7127):541–5. doi: 10.1038/nature05544
51. De Logu F, Souza Monteiro de Araujo D, Ugolini F, Iannone LF, Vannucchi M, Portelli F, et al. The TRPA1 channel amplifies the oxidative stress signal in melanoma. Cells. (2021) 10(11):1–14. doi: 10.3390/cells10113131
52. Devor M, Wall PD. Cross-excitation in dorsal root ganglia of nerve-injured and intact rats. J Neurophysiol. (1990) 64(6):1733–46. doi: 10.1152/jn.1990.64.6.1733
53. Kim YS, Anderson M, Park K, Zheng Q, Agarwal A, Gong C, et al. Coupled activation of primary sensory neurons contributes to chronic pain. Neuron. (2016) 91(5):1085–96. doi: 10.1016/j.neuron.2016.07.044
54. Durham PL. Diverse physiological roles of calcitonin gene-related peptide in migraine pathology: modulation of neuronal-glial-immune cells to promote peripheral and central sensitization. Curr Pain Headache Rep. (2016) 20(8):48. doi: 10.1007/s11916-016-0578-4
55. Messlinger K, Balcziak LK, Russo AF. Cross-talk signaling in the trigeminal ganglion: role of neuropeptides and other mediators. J Neural Transm (Vienna). (2020) 127(4):431–44. doi: 10.1007/s00702-020-02161-7
56. Obata K, Katsura H, Mizushima T, Yamanaka H, Kobayashi K, Dai Y, et al. TRPA1 Induced in sensory neurons contributes to cold hyperalgesia after inflammation and nerve injury. J Clin Invest. (2005) 115(9):2393–401. doi: 10.1172/JCI25437
57. Yang J, Li Y, Zuo X, Zhen Y, Yu Y, Gao L. Transient receptor potential ankyrin-1 participates in visceral hyperalgesia following experimental colitis. Neurosci Lett. (2008) 440(3):237–41. doi: 10.1016/j.neulet.2008.05.093
58. Chung MK, Park J, Asgar J, Ro JY. Transcriptome analysis of trigeminal ganglia following masseter muscle inflammation in rats. Mol Pain. (2016) 12:1–11. doi: 10.1177/1744806916668526
59. Luostarinen S, Hamalainen M, Hatano N, Muraki K, Moilanen E. The inflammatory regulation of TRPA1 expression in human A549 lung epithelial cells. Pulm Pharmacol Ther. (2021) 70:102059. doi: 10.1016/j.pupt.2021.102059
60. Liu J, Que K, Liu Y, Zang C, Wen J. Tumor necrosis factor-alpha regulates the TRPA1 expression in human odontoblast-like cells. J Pain Res. (2020) 13:1655–64. doi: 10.2147/JPR.S255288
61. Chen J, Kinter M, Shank S, Cotton C, Kelley TJ, Ziady AG. Dysfunction of nrf-2 in CF epithelia leads to excess intracellular H2O2 and inflammatory cytokine production. PLoS One. (2008) 3(10):e3367. doi: 10.1371/journal.pone.0003367
62. Li Y, Takemura G, Okada H, Miyata S, Maruyama R, Li L, et al. Reduction of inflammatory cytokine expression and oxidative damage by erythropoietin in chronic heart failure. Cardiovasc Res. (2006) 71(4):684–94. doi: 10.1016/j.cardiores.2006.06.003
63. Morgan MJ, Liu ZG. Crosstalk of reactive oxygen species and NF-kappaB signaling. Cell Res. (2011) 21(1):103–15. doi: 10.1038/cr.2010.178
64. Sumoza-Toledo A, Penner R. TRPM2: a multifunctional ion channel for calcium signalling. J Physiol. (2011) 589(Pt 7):1515–25. doi: 10.1113/jphysiol.2010.201855
65. Donkena KV, Young CY, Tindall DJ. Oxidative stress and DNA methylation in prostate cancer. Obstet Gynecol Int. (2010) 2010:302051. doi: 10.1155/2010/302051
66. Crow M, Denk F, McMahon SB. Genes and epigenetic processes as prospective pain targets. Genome Med. (2013) 5(2):12. doi: 10.1186/gm416
67. Qi F, Zhou Y, Xiao Y, Tao J, Gu J, Jiang X, et al. Promoter demethylation of cystathionine-beta-synthetase gene contributes to inflammatory pain in rats. Pain. (2013) 154(1):34–45. doi: 10.1016/j.pain.2012.07.031
68. Sun L, Zhao JY, Gu X, Liang L, Wu S, Mo K, et al. Nerve injury-induced epigenetic silencing of opioid receptors controlled by DNMT3a in primary afferent neurons. Pain. (2017) 158(6):1153–65. doi: 10.1097/j.pain.0000000000000894
69. Aydin B, Naziroglu M. Involvement of TRPM7 channel on the induction of diabetic neuropathic pain in mice: protective role of selenium and curcumin. Biol Trace Elem Res. (2023) 201(5):2377–95. doi: 10.1007/s12011-022-03518-7
70. Dong ZB, Wang YJ, Wan WJ, Wu J, Wang BJ, Zhu HL, et al. Resveratrol ameliorates oxaliplatin-induced neuropathic pain via anti-inflammatory effects in rats. Exp Ther Med. (2022) 24(3):586. doi: 10.3892/etm.2022.11523
71. Esu KD, Bakare AO, Owoyele BV. Effects of co-administration of vitamin E and lithium chloride on chronic constriction injury-induced neuropathy in male Wistar rats: focus on antioxidant and anti-inflammatory mechanisms. Pain Pract. (2022) 22(2):148–58. doi: 10.1111/papr.13064
Keywords: sensory ganglia, muscle pain, inflammation, oxidative stress, antioxidant, hyperalgesia
Citation: Zhang Y, Asgar J, Shou H, Pak J, Da Silva JT and Ro JY (2023) Intraganglionic reactive oxygen species mediate inflammatory pain and hyperalgesia through TRPA1 in the rat. Front. Pain Res. 4:1204057. doi: 10.3389/fpain.2023.1204057
Received: 11 April 2023; Accepted: 16 May 2023;
Published: 30 May 2023.
Edited by:
Yong Chen, Duke University, United StatesReviewed by:
Jun-Ho La, University of Texas Medical Branch at Galveston, United StatesNicole N Scheff, University of Pittsburgh, United States
© 2023 Zhang, Asgar, Shou, Pak, Da Silva and Ro. This is an open-access article distributed under the terms of the Creative Commons Attribution License (CC BY). The use, distribution or reproduction in other forums is permitted, provided the original author(s) and the copyright owner(s) are credited and that the original publication in this journal is cited, in accordance with accepted academic practice. No use, distribution or reproduction is permitted which does not comply with these terms.
*Correspondence: Jin Y. Ro anJvQHVtYXJ5bGFuZC5lZHU=