- 1Department of Anatomy and Cell Biology, University of Kansas Medical Center, Kansas City, KS, United States
- 2University of Kansas Medical Center, Hoglund Biomedical Imaging Center, Kansas City, KS, United States
- 3Department of Molecular and Integrative Physiology, University of Kansas Medical Center, Kansas City, KS, United States
- 4Department of Radiology, University of Kansas Medical Center, Kansas City, KS, United States
- 5Department of Neurology, University of Kansas Medical Center, Kansas City, KS, United States
- 6Department of Anesthesiology, Pain, and Perioperative Medicine, University of Kansas Medical Center, Kansas City, KS, United States
Early life stress exposure significantly increases the risk of developing chronic pain syndromes and comorbid mood and metabolic disorders later in life. Structural and functional changes within the hippocampus have been shown to contribute to many early life stress-related outcomes. We have previously reported that adult mice that underwent neonatal maternal separation (NMS) exhibit urogenital hypersensitivity, altered anxiety- and depression-like behaviors, increased adiposity, and decreased gene expression and neurogenesis in the hippocampus. Here, we are using magnetic resonance imaging and spectroscopy (MRI and MRS) to further investigate both NMS- and acute stress-induced changes in the hippocampus of female mice. Volumetric analysis of the whole brain revealed that the left hippocampus of NMS mice was 0.038 mm3 smaller compared to naïve mice. MRS was performed only on the right hippocampus and both total choline (tCho) and total N-acetylaspartate (tNAA) levels were significantly decreased due to NMS, particularly after WAS. Phosphoethanolamine (PE) levels were decreased in naïve mice after WAS, but not in NMS mice, and WAS increased ascorbate levels in both groups. The NMS mice showed a trend toward increased body weight and body fat percentage compared to naïve mice. A significant negative correlation was observed between body weight and phosphocreatine levels post-WAS in NMS mice, as well as a positive correlation between body weight and glutamine for NMS mice and a negative correlation for naïve mice. Together, these data suggest that NMS in mice reduces left hippocampal volume and may result in mitochondrial dysfunction and reduced neuronal integrity of the right hippocampus in adulthood. Hippocampal changes also appear to be related to whole body metabolic outcomes.
Introduction
According to the National Survey of Children's Health, nearly 40% of children in the United States experienced at least one or more serious psychological traumas in 2018 (1). Exposure to early life stress (ELS) has a significant and long-lasting impact on health outcomes later in life, including chronic pain, mood, and metabolic disorders (2) and a higher rate of comorbidity among these outcomes (3–8). Experiencing multiple adverse childhood events (ACEs) can permanently alter higher level affective processing (6) and is associated with psychiatric disorders including depression and anxiety (9). A growing base of research looking into the effects of ELS on pain processing has shown a heightened sensitization to painful stimuli, an increased likelihood of developing chronic pain syndromes, and a decreased likelihood of symptom improvement over time (7, 10, 11).
Many of the long-term effects of ELS are believed to be mediated via impaired development of the hippocampus. Excess exposure to glucocorticoids during early development, either through pharmacological or physiological sources, significantly impairs the structure, function, and gene expression of the hippocampus (12). Considering that the hippocampus is a major negative regulator of the hypothalamic-pituitary-adrenal (HPA) axis, disruption of hippocampal integrity can result in unregulated activation of the stress response (13, 14). Clinical studies have correlated ELS with elevated resting and responsive cortisol levels in adulthood (11). High ACE exposure rates are associated with smaller hippocampal volumes in adults (15, 16), and the reduction in hippocampal volume appears to mediate the relationship between anxiety and ELS (17). In rodents, ELS disrupts synaptic potentiation within the CA3 layer of the hippocampus (18) and decreases neurogenesis in the dentate gyrus (19).
Recent studies in patients with Urologic Chronic Pelvic Pain Syndrome (UCPPS), which encompasses interstitial cystitis/painful bladder syndrome (IC/PBS) and chronic prostatitis/chronic pelvic pain syndrome (CP/CPPS), have uncovered symptomatic and central functional/connectivity features related to ELS exposure. Adult UCPPS patients exposed to ELS tend to report more widespread pain, more severe functional symptoms (painful urination), poorer self-perceived well-being, and were less likely to have symptom relief after a year follow up (7). UCPPS patients exposed to ELS also have altered regional cortical centrality patterns compared to both healthy controls and UCPPS patients without ELS exposure (20). These findings suggest that ELS results in distinct changes in brain functionality that may predispose patients to a worsened pain phenotype. Our laboratory has developed a clinically-relevant mouse model of ELS-induced chronic urogenital pain using neonatal maternal separation (NMS). Adult male and female NMS mice demonstrate urogenital hypersensitivity and dysfunction, widespread hypersensitivity, altered anxiety- and depression-like behaviors, increased adiposity, and reduced regulatory gene expression and neurogenesis in the hippocampus (21–27). Exposure to water avoidance stress (WAS) exacerbates many of the NMS-induced features, including gene expression within the hippocampus (21, 22, 28). Here we are using our NMS model in female mice to determine if ELS impacts hippocampal gray matter volume and its neurochemical responsiveness to WAS exposure using magnetic resonance imaging/spectroscopy (MRI/MRS) and voxel-based morphometry (VBM) analysis.
Methods
Animals
Experiments were performed on female C57Bl/6 mice (Charles River, Wilmington, MA) born and housed in the Research Support Facility at the University of Kansas Medical Center. Mice were housed at 22°C on a 12-h light cycle from 600 to 1800 h and received water and food ad libitum. All research performed conformed to the National Institute of Health Guide for the Care and Use of Laboratory Animals and was approved by the University of Kansas Medical Center Institutional Animal Care and Use Committee. To avoid exposing the mice to additional confounding stressors, no attempts were made to control or track the estrus cycle of the mice. In addition, our previous studies have shown no impact of cycle stage on other outcomes related to NMS exposure (22, 24, 29).
Neonatal Maternal Separation
All mice used in this study were born in house from pregnant dams (Charles River, Wilmington, MA) delivered to the animal facility during the last week of gestation. The separation procedure was performed as previously described (23). Day of birth was designated as postnatal day (P) 0 and from P1 until P21 individual litters were removed daily and placed en masse into clean glass beakers containing a small amount of home cage bedding to maintain scent. Pups were held at 34°C and 50% humidity from 1100 to 1400 h. Fresh gloves were rubbed with home cage bedding before handling each litter to avoid rejection by the dam. Corresponding naïve mice were born, housed, and weaned during the same time frame to avoid potential complications arising from variations in prenatal shipping conditions, housing environment, and normal husbandry procedures. All mice were weaned on P22 and group housed with same-sex littermates. All litters also contained male pups, which were similarly handled, but not investigated in this study.
Study Design and Timeline
Mice from the same cohort (naïve, n = 5; NMS, n = 9) were used for the reported results. The baseline and post-WAS MRI/MRS scans were performed 1 month apart at 8–9 months of age. Bladder sensitivity was evaluated in a subset of mice at 16 months of age. All mice remained on standard chow diet (8604; Harlan Teklad, Madison WI) and in group housing conditions with standard corncob bedding throughout the experiment.
Body Composition Analysis
Prior to the baseline scan, mice were weighed and placed in an EchoMRI 2015 (EchoMRI LLC, Houston, TX) to quantify lean mass and fat mass.
Water Avoidance Stress
Water avoidance stress (WAS) was performed for 1 h, within the first 6 h of the light cycle. Mice were placed individually on a round platform (5 cm diameter) centrally affixed to the bottom of a container (36 cm length × 31 cm width × 27 cm height) filled with room temperature tap water up to 1 cm below the top of the platform, as previously described (21, 22, 28).
Magnetic Resonance Imaging (MRI) and Spectroscopy (MRS)
All Magnetic Resonance (MR) experiments were performed within the first 6 h of the light cycle on a 9.4 T MR system (Agilent Technologies, Santa Clara, CA) equipped with a 12 cm gradient coil (40 G/cm) (Magnex Scientific, Abingdon, UK). A custom-made quadrature surface radiofrequency (RF) coil consisting of two geometrically decoupled 18 mm loops and adjusted to transmit and receive at 400 MHz was used for acquisition of all MR data.
In vivo high-resolution T2-weighted MR images were acquired for brain volumetric analyses using a fast spin echo multi slice (FSEMS) sequence (TR = 4,000 ms, echo spacing = 0.012 ms, echo train length = 5, FOV = 2.56 × 2.56 cm2, matrix = 256 × 256, slice thickness = 0.2 mm, number of averages = 2, and total acquisition time = 6.25 m). Unbiased volumetric analysis of the whole brain was performed using Voxel Base Morphometry (VBM) implemented in SPM 8 and SPMMouse toolboxes within MATLAB (Mathworks Inc., Natick, MA) following published methodologies (30–32).
In vivo Magnetic Resonance Spectroscopy (MRS) was performed using a single-voxel SPECIAL sequence (33) (Volume of interest (VOI) = 2 × 1.2 × 1.8 mm3 positioned over the right hippocampus; TR = 4,000 ms; TE = 3 ms; Number of averages = 480; Total acquisition time = 32 m). The right hippocampus was chosen a priori due to clinical evidence illustrating unilateral functional defects in the right hippocampus in patients with anxious-depressive disorder related to changes in glucocorticoid receptor methylation patterns (34). Water suppression was performed with VAPOR (35) and linear and second order shims were automatically adjusted with FASTMAP (36) to achieve a water linewidth of no more than 18 Hz in a volume containing the VOI region. MR spectra was analyzed with LCModel software, using the unsuppressed water signal for each scan to calculate absolute metabolite concentrations (37, 38) and a metabolite basis set including alanine (Ala), ascorbate (Asc), aspartate (Asp), creatine (Cr), phosphocreatine (PCr), phosphocholine (PCho), glycerophosphocholine (GPC), gamma aminobutyric acid (GABA), glutamate (Glu), glutamine (Gln), glutathione (GSH), myo-inositol (mI), taurine (tau), lactate (Lac), N-acetylaspartate (NAA), N-acetylaspartylglutamate (NAAG), and phosphoethanolamine (PE) (full list found in Table 1). Total levels of choline (tCho) and NAA (tNAA) were also calculated and reported, as well as Glu+Gln (Glx).
Urinary Bladder Distention
Mice were placed under inhaled isoflurane anesthesia (4% induction, 2% maintenance) and the bare ends of two Teflon-coated stainless steel electrode wires (0.003' diameter; Grass Technologies, West Warwick, RI) were inserted into the left and right abdominal musculature using a 26-guage needle. A 24-guage angiocatheter was inserted into the urethra and anchored to the tail using surgical tape. Anesthesia was lowered (~1%) until hindlimb reflexes, but not escape behaviors, were present. The bladder was distended with air from a compressed nitrogen tank equipped with a dual-stage low delivery pressure regulator (Matheson-Linweld, Kansas City, MO) controlled by a custom-made distention control device (The University of Iowa Medical Instruments, Iowa City, IA). A separate pressure monitor (World Precision Instruments, Sarasota, FL) was used to regulate the pressure within the bladder. Three 60 mmHg distentions were performed to establish stable responses and then each pressure (15, 30, 45, 60 mmHg) was applied in triplicate for 20 s with a 2-min rest period in between. Electromyographic (EMG) activity from the abdominal musculature was amplified, filtered, and recorded using Spike 2 software (Cambridge Electronic Design, Cambridge, UK) on a personal computer and analyzed off-line. The visceromotor response (VMR) was quantified by measuring the area under the curve of the distention period, divided by the duration of the distention, and expressed as a percent the baseline EMG activity immediately prior to the distention.
Statistical Analysis
Calculations were made in Excel (Microsoft, Redmond, WA) and statistical analysis was performed using GraphPad Prism 9 (GraphPad, La Jolla, CA) or IBM SPSS Statistics 27 (IBM Corporation, Armonk, NY). Differences in volume between groups were determined by statistical parametric mapping in SPM 8 and SPM mouse. MRS and VMR results were analyzed via two-way ANOVA, with or without repeated measures, followed by Bonferroni posttest. Pearson correlation was used to correlate body weight and hippocampal spectral contents. All data are expressed as mean ± standard error of the mean (SEM). Statistical significance was set at p < 0.05.
Results
NMS Mice Have Smaller Left Hippocampal Volumes
We have previously published evidence of decreased hippocampal gene expression (21, 22, 24, 29) and neurogenesis (25) in NMS mice. Here, we are determining whether hippocampal volume is also impacted by NMS. Brain wide volume was analyzed using Voxel Based Morphometry (VBM) in female naïve and NMS mice prior to WAS exposure. The left hippocampal volume in the composite NMS brain was 0.038 mm3 smaller compared to naïve composite (p < 0.005) (Figure 1). We did not observe significant volume changes in the right hippocampus or in other identifiable structures in the brain (p > 0.05).
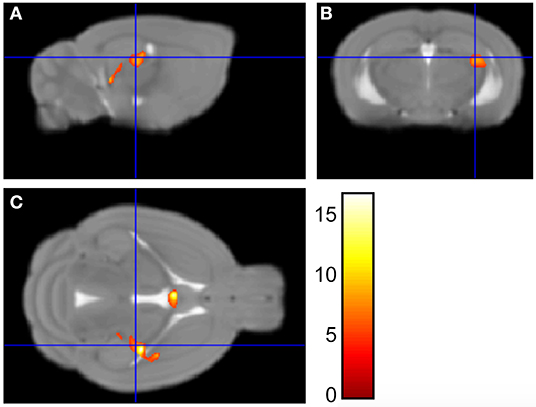
Figure 1. Voxel Based Morphometry analysis revealed regions that were significantly different in size between NMS (n = 9) and naïve (n = 5) female mice. Maximum intensity projections in the sagittal (A), coronal (B), and horizontal (C) plane are represented on the Mortimer Space Atlas, where the blue lines represent bregma corresponding to the left hippocampus.
NMS and WAS Significantly Impacted Hippocampal Neurochemical Concentrations
Single voxel MRS was used to measure the neurochemical content of only the right hippocampus prior to (baseline) and 24 h after a single exposure to WAS (Figure 2, Table 1). A significant reduction due to NMS was observed for total choline (tCho, p = 0.007) and tNAA levels (p = 0.044, Figure 2, Table 1). WAS exposure significantly decreased PE levels (p = 0.006) and significantly increased ascorbate levels (p = 0.026, Figure 2, Table 1). An interaction effect of NMS and WAS was observed for tCho (p = 0.029), tNAA (p = 0.035), and PE (p = 0.028, Figure 2, Table 1). Post-WAS, NMS mice had significantly decreased levels of tCho and tNAA compared to naïve mice and compared to baseline levels, only for tNAA (all p < 0.05, Figure 2, Table 1). PE levels were significantly lower post-WAS in naïve mice compared to NMS mice and baseline measurements (p < 0.05, Figure 2, Table 1). No significant differences were observed for the other measured chemicals (Table 1).
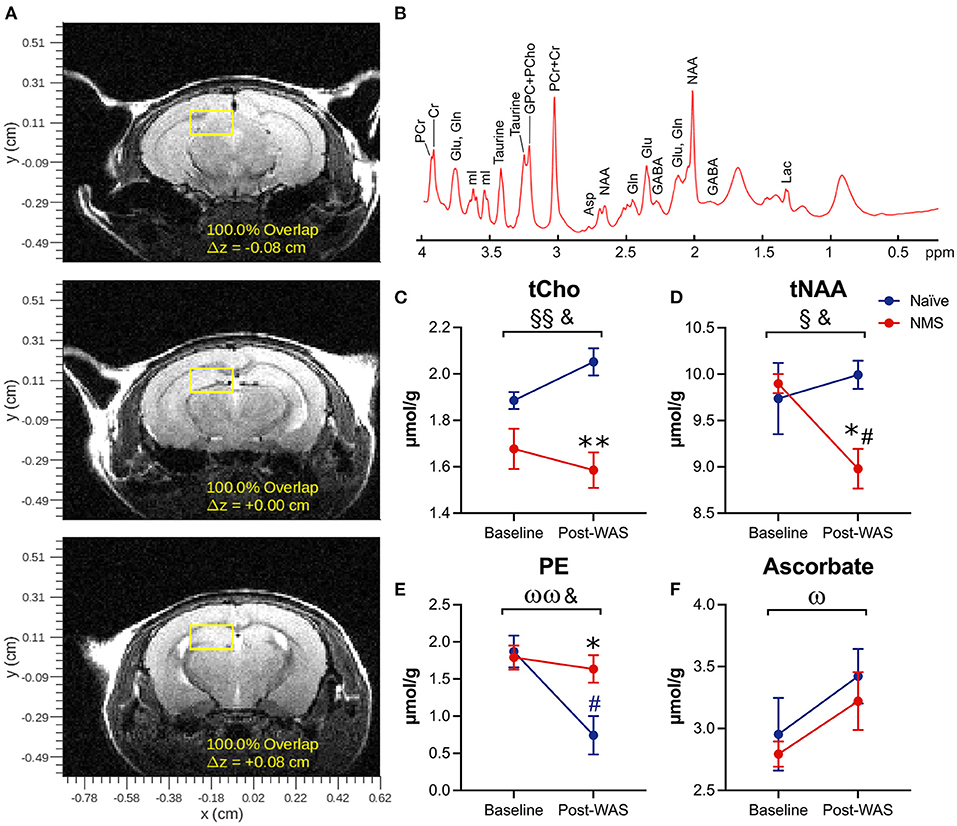
Figure 2. Magnetic Resonance Spectroscopy was performed on the right hippocampus prior to (baseline) and following water avoidance stress (Post-WAS) in naïve (n = 5) and NMS (n = 9) female mice. (A) Examples of the region of interest containing the right hippocampus for the collection of our MRS spectral data. From the resulting spectra (B) we measured absolute chemical concentrations. (C) A significant effect of NMS and an NMS/WAS interaction was observed on the level of total Choline (tCho). The post-WAS levels of tCho in NMS mice were significantly lower than in naïve mice. (D) A significant effect of NMS and an NMS/WAS interaction was observed on the combined level of N-acetylaspartate and N-acetylaspartylglutamate (tNAA). The level of tNAA in post-WAS NMS mice was significantly lower compared to both that in naïve mice and to their baseline measurements. (E) A significant effect of WAS and an NMS/WAS interaction was observed on phosphoethanolamine (PE). The level of PE in naïve mice, post-WAS, was significantly lower than in NMS mice and compared to their baseline measurements. (F) A significant effect of WAS was observed on the level of ascorbate. Brackets indicate a significant impact of NMS (§, §§p < 0.05, 0.01), WAS (ω, ωωp < 0.05, 0.01), or a NMS/WAS interaction effect (&p < 0.05) two-way RM ANOVA; *p < 0.05 vs. naïve, #p < 0.05 vs. baseline, Bonferroni posttest.
Body Weight Was Correlated With Post-WAS Neurochemical Concentrations
We have previously published evidence of increased body weight and fat mass in adult NMS mice (26, 27), however, these changes have not been investigated in conjunction with alterations in the hippocampus. Prior to the baseline scans, the body weight and body fat percentage (Figure 3) of NMS mice were slightly higher than naïve mice. When baseline body weight was correlated to post-WAS neurochemical concentrations in the hippocampus, there was a significant negative correlation for PCr in NMS mice (p = 0.0051, Figure 3) and a significant negative correlation for Glutamine in naïve mice (p = 0.0467), while NMS mice showed a positive correlation for Glutamine (p = 0.007, Figure 3). A significant negative correlation was also observed for body fat and PCr in NMS mice (p = 0.001, data not shown). No significant correlations were observed for the other measured chemicals.
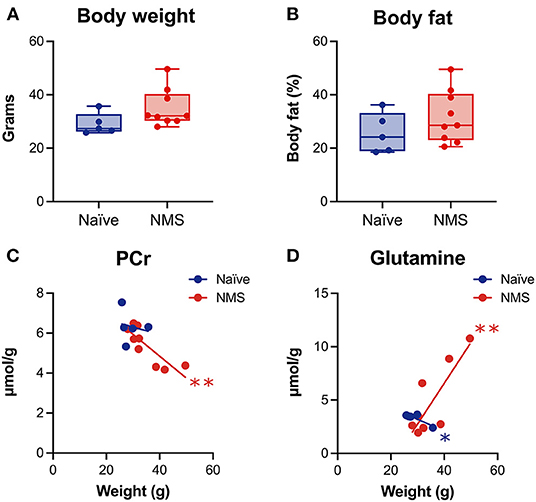
Figure 3. Body weight and percent body fat were measured prior to imaging and correlated with spectral contents after water avoidance stress exposure in naïve (n = 5) and NMS (n = 9) female mice. A non-significant increase in body weight (A) and percent body fat (B) was observed in female NMS mice compared to naïve mice. (C) A significant negative correlation was observed between hippocampal phosphocreatine (PCr) levels and body weight in NMS mice. (D) Significant negative and positive correlations were observed in naïve and NMS mice, respectively, between hippocampal glutamine levels and body weight. *, **p < 0.05, 0.01, Pearson correlation.
Bladder Sensitivity Was Significantly Increased in NMS Mice
To verify that NMS increased urogenital hypersensitivity, a subset of mice were assessed for their visceromotor response (VMR) during urinary bladder distention (UBD). As previously reported (22, 29), we observed a significant increase in VMR during UBD in the female NMS mice compared to naïve mice (p = 0.0094, Figure 4).
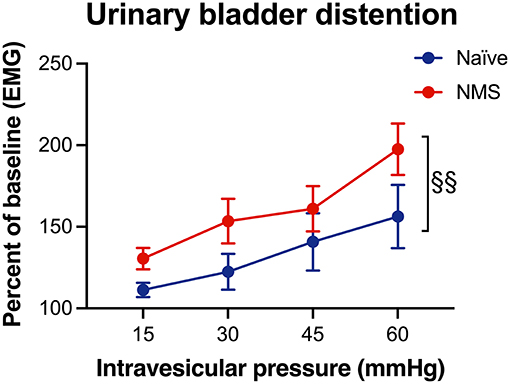
Figure 4. Visceromotor response (VMR) during urinary bladder distention (UBD) was measured seven months after water avoidance stress exposure and MR imaging in naïve and NMS female mice. An increase in VMR during UBD was observed in NMS mice (p = 0.0094) compared to naïve mice. Two-way ANOVA with Bonferroni posttest. Naïve, n = 5, NMS, n = 7.
Discussion
This study examined the effects of ELS, in the form of NMS, on hippocampal volume as well as hippocampal neurochemical concentrations before and after an acute stressor in adult female mice. We also determined the potential impact of body weight on post-WAS hippocampal neurochemical content, as we have previously observed that NMS increases body weight and fat content. We demonstrated that NMS mice had reduced volume in the left hippocampus. NMS significantly reduced the levels of several neurochemicals in the hippocampus, particularly after WAS exposure, which supports our previous work showing decreased hippocampal gene expression (21, 22, 24, 25, 29) and neurogenesis (25) in NMS mice.
Voxel based morphometry (VBM), while a very popular tool in human research, is less frequently used in rodent research. With the development of rodent compatible software and the use of higher-powered MRI, VBM is capable of whole brain gray and white matter volume comparisons in rodents (31, 32). Importantly, VBM avoids the human error of atlas-based manual measurements, which are prone to subjective user variability. Furthermore, unlike region of interest-based MRI volume analysis, VBM is not limited to studying a single region. As such, VBM allows for a brain-wide analysis of gray or white matter volume that can detect subtle differences in structural volume (30, 39, 40). Implemented here, VBM allowed for comparisons of whole brain gray matter volume to identify any significant changes between NMS and naive mice. We observed significantly reduced volume in the left hippocampus of NMS mice, compared to naïve mice. The hippocampal finding is clinically important as patient-reported ELS is associated with decreased hippocampal gray matter volume (15, 41–45), which is also a common feature of patients with anxious-depressive disorders (34, 45–47). Interestingly, clinical findings reported gray matter losses specifically in the left hippocampus, whereas changes in functional connectivity appear to affect the right hippocampus (34). The developing hippocampus is particularly sensitive to excess glucocorticoids and overexposure can permanently affect hippocampal structure, function, and gene expression (12). Rodent models of ELS have shown delayed maturation of the dentate gyrus (DG), resulting in fewer neural stem cells in the adult DG (48). We have previously shown reduced hippocampal neurogenesis in male NMS mice, compared to naïve mice (25), and our results here suggest that similar reductions may be occurring in the female mice, as well.
We have previously shown that exposure to WAS significantly increased urogenital sensitivity and impacted bladder output in both male and female NMS mice, along with concordant gene expression changes in the hypothalamus, amygdala, and hippocampus (21, 22). Although in the previous studies we observed hippocampal gene expression changes up to 8 days post-WAS, here we used MRS, a non-invasive imaging technique, to determine neurochemical abnormalities in the hippocampus 1 day after WAS exposure to examine more immediate changes due to acute stress exposure. Although relatively few MRS studies have looked at the impact of ELS, studies of chronic and acute adult stress exposure have shown neurochemical changes within the hippocampus and other related structures. NAA is a marker of neuronal integrity and viability, and is an indicator of neuronal mitochondria function (49). Bi-lateral decreased hippocampal NAA was observed in adult males with an early history of socioeconomic deprivation (50). Lower hippocampal NAA concentrations have also been repeatedly demonstrated in patients diagnosed with PTSD (51) and major depressive disorder (52), with or without a concordant loss of gray matter volume. Here we observed a significant decrease in tNAA due to NMS, as well as an NMS/WAS interaction. NMS mice had a significantly lower level of tNAA after WAS exposure, compared to their baseline measurements and to WAS-exposed naïve mice. In addition, PE is an ethanolamine derivative involved in phospholipid formation and has been implicated in mitochondrial dysfunction contributing to depressive disorders (53). Previous studies have reported increased levels of PE in the brains of mice that were exposed to chronic unpredictable stress to model depression (54). We observed a significant impact of WAS on PE expression in naïve mice, although it remained unchanged in NMS. Together these observations suggest that there may be mitochondrial dysfunction in the hippocampus of NMS mice, which could lead to reduced resilience and inappropriate response to an acute stressor.
The level of tCho, which consists of glycerophosphocholine (GPC) and phosphocholine (PCho), was also significantly decreased in the right hippocampus of NMS mice. PCho is a phosphomonoester and its concentration has been positively associated with dendritic sprouting, such as that occurring during development, in response to cortical lesion, and in early Alzheimer's disease progression (55). It is also a precursor for phosphatidylcholine, which is highly enriched in the hippocampus and its homeostatic regulation is vital for maintaining membrane trafficking and axonal integrity (56). The diminished level of tCho in the hippocampus of NMS mice was further exacerbated following WAS exposure, again suggesting that NMS may have diminished the neuronal integrity of the hippocampus.
Ascorbate is an endogenous antioxidant that is highly expressed in the brain, particularly in the amygdala, hypothalamus, and hippocampus (57), which are the main initiators and regulators of the stress response system (3). Ascorbate is neuroprotective and prevents injury from ischemia and excitotoxicity (57). We interpret the increase in ascorbate levels to be a neuroprotective mechanism in response to WAS exposure. The equivalent response of both groups suggests that general oxidative stress in not contributing to the NMS-related outcomes.
Adults who report high adverse childhood events (ACE) scores are more likely to present with obesity and/or metabolic syndrome (58). The brain is susceptible to obesity-related pathology, including endothelial damage and more pronounced age-related volume reductions (59, 60). Patients with bipolar disorder have a 60% higher obesity rate, which is also related to decreased hippocampal volume and lower neurochemical levels, including that of PCr and PCho (61). PCr is a high-energy phosphate that serves as an intracellular energy buffer supporting mitochondrial respiration, and also exhibits neuroprotective effects through reduction of reactive oxygen species (62). Several clinical MRS studies have found altered brain PCr concentrations in schizophrenia, depression, panic disorder, and substance abuse patients (63–65). Although, we only observed a trend toward increased body weight and fat in the NMS mice, there was a significant negative correlation between body weight and body fat with PCr, suggesting that hippocampal expression of this important metabolic compound may be linked to adiposity. A positive relationship between body mass index (BMI) and hippocampal Glx (glutamate/glutamine) was also reported for patients with bipolar disorder (66), however the opposite was observed in rats fed a high fat diet (67). While we did not observe a significant relationship between body weight and Glx in our study, we did observe a negative correlation between body weight and glutamine for naïve mice and a positive correlation for NMS mice. Glutamate is the most abundant excitatory neurotransmitter in the brain and is taken up by astrocytes and converted to glutamine following synaptic release (66). Leptin receptors on astrocytes in the hippocampus have been shown to regulate glutamate homeostasis and neurotransmission (68). High fat diet has also been shown to impact both the morphology of and increase the expression of glutamate transporters on astrocytes (69). Together, these correlative outcomes suggest that whole body metabolism may be influencing neuronal-glial communications and local synaptic activity within the hippocampus, particularly in response to acute stress exposure.
We acknowledge a number of limitations within our study that may affect the impact of our results. First, we only investigated female mice for the current study. Based on our previous observations of decreased hippocampal gene expression and neurogenesis following NMS (21, 25), we predict that similar changes would be observed in male mice and studies are currently underway to determine any sex differences in these outcomes. Second, we were only able to perform single voxel MRS and a priori chose the right hippocampus due to clinical evidence of diminished unilateral hippocampal connectivity following ELS (34) and prior to completed VBM analysis of the MRI results. We do not report these results as evidence of unilateral neurochemical changes in the hippocampus and our ongoing studies will incorporate bi-lateral MRS analysis of the hippocampus to determine if NMS and WAS effects are observed in both hippocampi. Finally, our groups were not of even number and the naïve group may have been underpowered at n = 5.
Our results reported here support our previous findings of impaired hippocampal integrity following NMS exposure in mice. The left hippocampus of NMS mice was significantly smaller than naïve mice and single voxel MRS of only the right hippocampus revealed that it was less metabolically sensitive to acute stress exposure. In addition, correlations between body weight and neurochemical content within the hippocampus suggest that whole body metabolic state may influence neuronal-glial communication and synaptic function within the hippocampus, or perhaps vice versa. Understanding the effects, and directionality, of ELS on metabolic and structural changes in the brain and accompanying urogenital and metabolic outcomes, will provide important mechanistic insight toward developing preventative measures and/or treatment options for patients with chronic pain, mood, and metabolic disorders and a history of ELS.
Data Availability Statement
The raw data supporting the conclusions of this article will be made available by the authors, without undue reservation.
Ethics Statement
The animal study was reviewed and approved by the University of Kansas Medical Center Institutional Animal Care and Use Committee.
Author Contributions
I-YC, JH, and JC designed the research study. AB, XY, C-YL, PL, PK, and OE performed the experiments. AB, XY, C-YL, PL, PK, I-YC, JH, and JC analyzed the data. AB and JC wrote the manuscript. All authors contributed to the article and approved the submitted version.
Funding
This work was supported by NIH grants R01 DK099611 (JC), R01 DK103872 (JC), the KUMC Research Institute, Lied Basic Science Grant Program (JC), Center of Biomedical Research Excellence (COBRE) grant P20 GM104936 (JC), T32 HD057850 (AB and OE), start-up funds and core support from the Kansas Institutional Development Award (IDeA) P20 GM103418, and core support from the Kansas IDDRC P30 HD00228.
Conflict of Interest
The authors declare that the research was conducted in the absence of any commercial or financial relationships that could be construed as a potential conflict of interest.
Publisher's Note
All claims expressed in this article are solely those of the authors and do not necessarily represent those of their affiliated organizations, or those of the publisher, the editors and the reviewers. Any product that may be evaluated in this article, or claim that may be made by its manufacturer, is not guaranteed or endorsed by the publisher.
Acknowledgments
We would like to thank Dr. John Thyfault for use of the EchoMRI and Jenna Frick, Brittni Jones, and Ruipeng Wang for technical assistance.
References
1. Child Adolescent Health Measurement Initiative. U.S. Department of Health and Human Services, Health Resources and Services Administration (HRSA), Maternal and Child Health Bureau (MCHB) (2018).
2. Hughes K, Bellis MA, Hardcastle KA, Sethi D, Butchart A, Mikton C, et al. The effect of multiple adverse childhood experiences on health: a systematic review and meta-analysis. Lancet Public Health. (2017) 2:e356–66. doi: 10.1016/S2468-2667(17)30118-4
3. Fuentes IM, Christianson JA. The influence of early life experience on visceral pain. Front Syst Neurosci. (2018) 12:2. doi: 10.3389/fnsys.2018.00002
4. Jones GT, Power C, Macfarlane GJ. Adverse events in childhood and chronic widespread pain in adult life: results from the 1958 British Birth Cohort Study. Pain. (2009) 143:92–6. doi: 10.1016/j.pain.2009.02.003
5. Nicol AL, Sieberg CB, Clauw DJ, Hassett AL, Moser SE, Brummett CM. The association between a history of lifetime traumatic events and pain severity, physical function, and affective distress in patients with chronic pain. J Pain. (2016) 17:1334–48. doi: 10.1016/j.jpain.2016.09.003
6. Pechtel P, Pizzagalli DA. Effects of early life stress on cognitive and affective function: an integrated review of human literature. Psychopharmacology. (2011) 214:55–70. doi: 10.1007/s00213-010-2009-2
7. Schrepf A, Naliboff B, Williams DA, Stephens-Shields AJ, Landis JR, Gupta A, et al. Adverse childhood experiences and symptoms of urologic chronic pelvic pain syndrome: a multidisciplinary approach to the study of chronic pelvic pain research network study. Ann Behav Med. (2018) 52:865–77. doi: 10.1093/abm/kax060
8. Varinen A, Kosunen E, Mattila K, Koskela T, Sumanen M. The relationship between childhood adversities and fibromyalgia in the general population. J Psychosom Res. (2017) 99:137–42. doi: 10.1016/j.jpsychores.2017.06.011
9. Agid O, Shapira B, Zislin J, Ritsner M, Hanin B, Murad H, et al. Environment and vulnerability to major psychiatric illness: a case control study of early parental loss in major depression, bipolar disorder and schizophrenia. Mol Psychiatry. (1999) 4:163–72. doi: 10.1038/sj.mp.4000473
10. Harper DE, Ichesco E, Schrepf A, Halvorson M, Puiu T, Clauw DJ, et al. Relationships between brain metabolite levels, functional connectivity, and negative mood in urologic chronic pelvic pain syndrome patients compared to controls: a MAPP research network study. Neuroimage Clin. (2018) 17:570–8. doi: 10.1016/j.nicl.2017.11.014
11. Burke NN, Finn DP, McGuire BE, Roche M. Psychological stress in early life as a predisposing factor for the development of chronic pain: Clinical and preclinical evidence and neurobiological mechanisms. J Neurosci Res. (2017) 95:1257–70. doi: 10.1002/jnr.23802
12. Uno H, Eisele S, Sakai A, Shelton S, Baker E, DeJesus O, et al. Neurotoxicity of glucocorticoids in the primate brain. Horm Behav. (1994) 28:336–48. doi: 10.1006/hbeh.1994.1030
13. Raber J. Detrimental effects of chronic hypothalamic-pituitary-adrenal axis activation. From obesity to memory deficits. Mol Neurobiol. (1998) 18:1–22. doi: 10.1007/BF02741457
14. Conrad CD. Chronic stress-induced hippocampal vulnerability: the glucocorticoid vulnerability hypothesis. Rev Neurosc. (2008) 19:395–411. doi: 10.1515/REVNEURO.2008.19.6.395
15. Hanson JL, Nacewicz BM, Sutterer MJ, Cayo AA, Schaefer SM, Rudolph KD, et al. Behavioral problems after early life stress: contributions of the hippocampus and amygdala. Biol Psychiatry. (2015) 77:314–23. doi: 10.1016/j.biopsych.2014.04.020
16. Hodel AS, Hunt RH, Cowell RA, Van Den Heuvel SE, Gunnar MR, Thomas KM. Duration of early adversity and structural brain development in post-institutionalized adolescents. Neuroimage. (2015) 105:112–9. doi: 10.1016/j.neuroimage.2014.10.020
17. Gorka AX, Hanson JL, Radtke SR, Hariri AR. Reduced hippocampal and medial prefrontal gray matter mediate the association between reported childhood maltreatment and trait anxiety in adulthood and predict sensitivity to future life stress. Biol Mood Anxiety Disord. (2014) 4:12. doi: 10.1186/2045-5380-4-12
18. Wang XD, Rammes G, Kraev I, Wolf M, Liebl C, Scharf SH, et al. Forebrain CRF(1) modulates early-life stress-programmed cognitive deficits. J Neurosci. (2011) 31:13625–34. doi: 10.1523/JNEUROSCI.2259-11.2011
19. Mirescu C, Peters JD, Gould E. Early life experience alters response of adult neurogenesis to stress. Nat Neurosci. (2004) 7:841–6. doi: 10.1038/nn1290
20. Gupta A, Bhatt RR, Naliboff BD, Kutch JJ, Labus JS, Vora PP, et al. Impact of early adverse life events and sex on functional brain networks in patients with urological chronic pelvic pain syndrome (UCPPS): a MAPP Research Network study. PloS ONE. (2019) 14:e0217610. doi: 10.1371/journal.pone.0217610
21. Fuentes IM, Pierce AN, Di Silvestro ER, Maloney MO, Christianson JA. Differential influence of early life and adult stress on urogenital sensitivity and function in male mice. Front Syst Neurosci. (2017) 11:97. doi: 10.3389/fnsys.2017.00097
22. Pierce AN, Di Silvestro ER, Eller OC, Wang R, Ryals JM, Christianson JA. Urinary bladder hypersensitivity and dysfunction in female mice following early life and adult stress. Brain Res. (2016) 1639:58–73. doi: 10.1016/j.brainres.2016.02.039
23. Fuentes IM, Pierce AN, O'Neil PT, Christianson JA. Assessment of perigenital sensitivity and prostatic mast cell activation in a mouse model of neonatal maternal separation. J Vis Exp. (2015) 102:e53181. doi: 10.3791/53181
24. Pierce AN, Ryals JM, Wang R, Christianson JA. Vaginal hypersensitivity and hypothalamic-pituitary-adrenal axis dysfunction as a result of neonatal maternal separation in female mice. Neuroscience. (2014) 263:216–30. doi: 10.1016/j.neuroscience.2014.01.022
25. Fuentes IM, Jones BM, Brake AD, Pierce AN, Eller OC, Supple RM, et al. Voluntary wheel running improves outcomes in an early life stress-induced model of urologic chronic pelvic pain syndrome in male mice. Pain. (2021) 162:1681–91. doi: 10.1097/j.pain.0000000000002178
26. Eller OC, Morris EM, Thyfault JP, Christianson JA. Early life stress reduces voluntary exercise and its prevention of diet-induced obesity and metabolic dysfunction in mice. Physiol Behav. (2020) 223:113000. doi: 10.1016/j.physbeh.2020.113000
27. Eller OC, Foright RM, Brake AD, Winter MK, Bantis LE, Morris EM, et al. An omega-3-rich anti-inflammatory diet improved widespread allodynia and worsened metabolic outcomes in adult mice exposed to neonatal maternal separation. Neuroscience. (2021) 468:53–67. doi: 10.1016/j.neuroscience.2021.06.001
28. Fuentes IM, Walker NK, Pierce AN, Holt BR, Di Silvestro ER, Christianson JA. Neonatal maternal separation increases susceptibility to experimental colitis and acute stress exposure in male mice. IBRO Rep. (2016) 1:10–8. doi: 10.1016/j.ibror.2016.07.001
29. Pierce AN, Eller-Smith OC, Christianson JA. Voluntary wheel running attenuates urinary bladder hypersensitivity and dysfunction following neonatal maternal separation in female mice. Neurourol Urodyn. (2018) 37:1623–32. doi: 10.1002/nau.23530
30. Meyer CE, Kurth F, Lepore S, Gao JL, Johnsonbaugh H, Oberoi MR, et al. In vivo magnetic resonance images reveal neuroanatomical sex differences through the application of voxel-based morphometry in C57BL/6 mice. Neuroimage. (2017) 163:197–205. doi: 10.1016/j.neuroimage.2017.09.027
31. Sawiak SJ, Wood NI, Williams GB, Morton AJ, Carpenter TA. Voxel-based morphometry in the R6/2 transgenic mouse reveals differences between genotypes not seen with manual 2D morphometry. Neurobiol Dis. (2009) 33:20–7. doi: 10.1016/j.nbd.2008.09.016
32. Sawiak SJ, Wood NI, Williams GB, Morton AJ, Carpenter TA. Use of magnetic resonance imaging for anatomical phenotyping of the R6/2 mouse model of Huntington's disease. Neurobiol Dis. (2009) 33:12–9. doi: 10.1016/j.nbd.2008.09.017
33. Mlynárik V, Gambarota G, Frenkel H, Gruetter R. Localized short-echo-time proton MR spectroscopy with full signal-intensity acquisition. Magn Reson Med. (2006) 56:965–70. doi: 10.1002/mrm.21043
34. Palma-Gudiel H, Cordova-Palomera A, Tornador C, Falcon C, Bargallo N, Deco G, et al. Increased methylation at an unexplored glucocorticoid responsive element within exon 1D of NR3C1 gene is related to anxious-depressive disorders and decreased hippocampal connectivity. Eur Neuropsychopharmacol. (2018) 28:579–88. doi: 10.1016/j.euroneuro.2018.03.015
35. Tkac I, Starcuk Z, Choi IY, Gruetter R. In vivo 1H NMR spectroscopy of rat brain at 1 ms echo time. Magn Reson Med. (1999) 41:649–56.
36. Gruetter R. Automatic, localized in vivo adjustment of all first- and second-order shim coils. Magn Reson Med. (1993) 29:804–11. doi: 10.1002/mrm.1910290613
37. Pfeuffer J, Tkac I, Provencher SW, Gruetter R. Toward an in vivo neurochemical profile: quantification of 18 metabolites in short-echo-time (1)H NMR spectra of the rat brain. J Magn Reson. (1999) 141:104–20. doi: 10.1006/jmre.1999.1895
38. Provencher SW. Automatic quantitation of localized in vivo 1H spectra with LCModel. NMR Biomed. (2001) 14:260–4. doi: 10.1002/nbm.698
39. Ashburner J, Friston KJ. Voxel-based morphometry–the methods. Neuroimage. (2000) 11(Pt. 1):805–21. doi: 10.1006/nimg.2000.0582
40. Ashburner J, Friston KJ. Why voxel-based morphometry should be used. Neuroimage. (2001) 14:1238–43. doi: 10.1006/nimg.2001.0961
41. Bootsman F, Kemner SM, Hillegers MH, Brouwer RM, Vonk R, van der Schot AC, et al. The association between hippocampal volume and life events in healthy twins. Hippocampus. (2016) 26:1088–95. doi: 10.1002/hipo.22589
42. Dahmen B, Puetz VB, Scharke W, von Polier GG, Herpertz-Dahlmann B, Konrad K. Effects of early-life adversity on hippocampal structures and associated HPA axis functions. Dev Neurosci. (2018) 40:13–22. doi: 10.1159/000484238
43. Vythilingam M, Heim C, Newport J, Miller AH, Anderson E, Bronen R, et al. Childhood trauma associated with smaller hippocampal volume in women with major depression. Am J Psychiatry. (2002) 159:2072–80. doi: 10.1176/appi.ajp.159.12.2072
44. Dannlowski U, Stuhrmann A, Beutelmann V, Zwanzger P, Lenzen T, Grotegerd D, et al. Limbic scars: long-term consequences of childhood maltreatment revealed by functional and structural magnetic resonance imaging. Biol Psychiatry. (2012) 71:286–93. doi: 10.1016/j.biopsych.2011.10.021
45. Saleh A, Potter GG, McQuoid DR, Boyd B, Turner R, MacFall JR, et al. Effects of early life stress on depression, cognitive performance and brain morphology. Psychol Med. (2017) 47:171–81. doi: 10.1017/S0033291716002403
46. Heim C, Newport DJ, Mletzko T, Miller AH, Nemeroff CB. The link between childhood trauma and depression: insights from HPA axis studies in humans. Psychoneuroendocrinology. (2008) 33:693–710. doi: 10.1016/j.psyneuen.2008.03.008
47. Campbell S, Marriott M, Nahmias C, MacQueen GM. Lower hippocampal volume in patients suffering from depression: a meta-analysis. Am J Psychiatry. (2004) 161:598–607. doi: 10.1176/appi.ajp.161.4.598
48. Youssef M, Atsak P, Cardenas J, Kosmidis S, Leonardo ED, Dranovsky A. Early life stress delays hippocampal development and diminishes the adult stem cell pool in mice. Sci Rep. (2019) 9:4120. doi: 10.1038/s41598-019-40868-0
49. Moffett JR, Ross B, Arun P, Madhavarao CN, Namboodiri AM. N-Acetylaspartate in the CNS: from neurodiagnostics to neurobiology. Prog Neurobiol. (2007) 81:89–131. doi: 10.1016/j.pneurobio.2006.12.003
50. McLean J, Krishnadas R, Batty GD, Burns H, Deans KA, Ford I, et al. Early life socioeconomic status, chronic physiological stress and hippocampal N-acetyl aspartate concentrations. Behav Brain Res. (2012) 235:225–30. doi: 10.1016/j.bbr.2012.08.013
51. Quadrelli S, Mountford C, Ramadan S. Systematic review of in-vivo neuro magnetic resonance spectroscopy for the assessment of posttraumatic stress disorder. Psychiatry Res Neuroimaging. (2018) 282:110–25. doi: 10.1016/j.pscychresns.2018.07.001
52. Lefebvre D, Langevin LM, Jaworska N, Harris AD, Lebel RM, Jasaui Y, et al. A pilot study of hippocampal N-acetyl-aspartate in youth with treatment resistant major depression. J Affect Disord. (2017) 207:110–3. doi: 10.1016/j.jad.2016.05.077
53. Prabhu VV, Nguyen TB, Cui Y, Oh YE, Piao YH, Baek HM, et al. Metabolite signature associated with stress susceptibility in socially defeated mice. Brain Res. (2019) 1708:171–80. doi: 10.1016/j.brainres.2018.12.020
54. Faria R, Santana MM, Aveleira CA, Simoes C, Maciel E, Melo T, et al. Alterations in phospholipidomic profile in the brain of mouse model of depression induced by chronic unpredictable stress. Neuroscience. (2014) 273:1–11. doi: 10.1016/j.neuroscience.2014.04.042
55. Geddes JW, Panchalingam K, Keller JN, Pettegrew JW. Elevated phosphocholine and phosphatidylcholine following rat entorhinal cortex lesions. Neurobiol Aging. (1997) 18:305–8. doi: 10.1016/S0197-4580(97)80312-0
56. Glynn P. Neuronal phospholipid deacylation is essential for axonal and synaptic integrity. Biochim Biophys Acta. (2013) 1831:633–41. doi: 10.1016/j.bbalip.2012.07.023
57. Kim EJ, Won R, Sohn JH, Chung MA, Nam TS, Lee HJ, et al. Anti-oxidant effect of ascorbic and dehydroascorbic acids in hippocampal slice culture. Biochem Biophys Res Commun. (2008) 366:8–14. doi: 10.1016/j.bbrc.2007.11.050
58. Gilbert LK, Breiding MJ, Merrick MT, Thompson WW, Ford DC, Dhingra SS, et al. Childhood adversity and adult chronic disease: an update from ten states and the District of Columbia, 2010. Am J Prev Med. (2015) 48:345–9. doi: 10.1016/j.amepre.2014.09.006
59. Toda N, Ayajiki K, Okamura T. Obesity-induced cerebral hypoperfusion derived from endothelial dysfunction: one of the risk factors for Alzheimer's disease. Curr Alzheimer Res. (2014) 11:733–44. doi: 10.2174/156720501108140910120456
60. Enzinger C, Fazekas F, Matthews PM, Ropele S, Schmidt H, Smith S, et al. Risk factors for progression of brain atrophy in aging: six-year follow-up of normal subjects. Neurology. (2005) 64:1704–11. doi: 10.1212/01.WNL.0000161871.83614.BB
61. Bond DJ, Silveira LE, MacMillan EL, Torres IJ, Lang DJ, Su W, et al. Diagnosis and body mass index effects on hippocampal volumes and neurochemistry in bipolar disorder. Transl Psychiatry. (2017) 7:e1071. doi: 10.1038/tp.2017.42
62. Li H, Tang Z, Chu P, Song Y, Yang Y, Sun B, et al. Neuroprotective effect of phosphocreatine on oxidative stress and mitochondrial dysfunction induced apoptosis in vitro and in vivo: involvement of dual PI3K/Akt and Nrf2/HO-1 pathways. Free Radic Biol Med. (2018) 120:228–38. doi: 10.1016/j.freeradbiomed.2018.03.014
63. Christensen JD, Kaufman MJ, Levin JM, Mendelson JH, Holman BL, Cohen BM, et al. Abnormal cerebral metabolism in polydrug abusers during early withdrawal: a 31P MR spectroscopy study. Magn Reson Med. (1996) 35:658–63. doi: 10.1002/mrm.1910350506
64. Deicken RF, Calabrese G, Merrin EL, Vinogradov S, Fein G, Weiner MW. Asymmetry of temporal lobe phosphorous metabolism in schizophrenia: a 31phosphorous magnetic resonance spectroscopic imaging study. Biol Psychiatry. (1995) 38:279–86. doi: 10.1016/0006-3223(94)00372-A
65. Pazini FL, Cunha MP, Rodrigues ALS. The possible beneficial effects of creatine for the management of depression. Prog Neuro-psychopharmacol Biol Psychiatry. (2019) 89:193–206. doi: 10.1016/j.pnpbp.2018.08.029
66. Bond DJ, da Silveira LE, MacMillan EL, Torres IJ, Lang DJ, Su W, et al. Relationship between body mass index and hippocampal glutamate/glutamine in bipolar disorder. Br J Psychiatry. (2016) 208:146–52. doi: 10.1192/bjp.bp.115.163360
67. Raider K, Ma D, Harris JL, Fuentes I, Rogers RS, Wheatley JL, et al. A high fat diet alters metabolic and bioenergetic function in the brain: a magnetic resonance spectroscopy study. Neurochem Int. (2016) 97:172–80. doi: 10.1016/j.neuint.2016.04.008
68. Naranjo V, Contreras A, Merino B, Plaza A, Lorenzo MP, García-Cáceres C, et al. Specific deletion of the astrocyte leptin receptor induces changes in hippocampus glutamate metabolism, synaptic transmission and plasticity. Neuroscience. (2020) 447:182–90. doi: 10.1016/j.neuroscience.2019.10.005
Keywords: magnetic resonance imaging, magnetic resonance spectroscopy, early life stress, pain, obesity
Citation: Brake AD, Yang X, Lee C-Y, Lee P, Keselman P, Eller OC, Choi I-Y, Harris JL and Christianson JA (2022) Reduced Hippocampal Volume and Neurochemical Response to Adult Stress Exposure in a Female Mouse Model of Urogenital Hypersensitivity. Front. Pain Res. 3:809944. doi: 10.3389/fpain.2022.809944
Received: 05 November 2021; Accepted: 06 January 2022;
Published: 27 January 2022.
Edited by:
Nick Spencer, Flinders University, AustraliaReviewed by:
Andelain Erickson, University Hospital RWTH Aachen, GermanyAnthony Hannan, The University of Melbourne, Australia
Copyright © 2022 Brake, Yang, Lee, Lee, Keselman, Eller, Choi, Harris and Christianson. This is an open-access article distributed under the terms of the Creative Commons Attribution License (CC BY). The use, distribution or reproduction in other forums is permitted, provided the original author(s) and the copyright owner(s) are credited and that the original publication in this journal is cited, in accordance with accepted academic practice. No use, distribution or reproduction is permitted which does not comply with these terms.
*Correspondence: Julie A. Christianson, amNocmlzdGlhbnNvbkBrdW1jLmVkdQ==