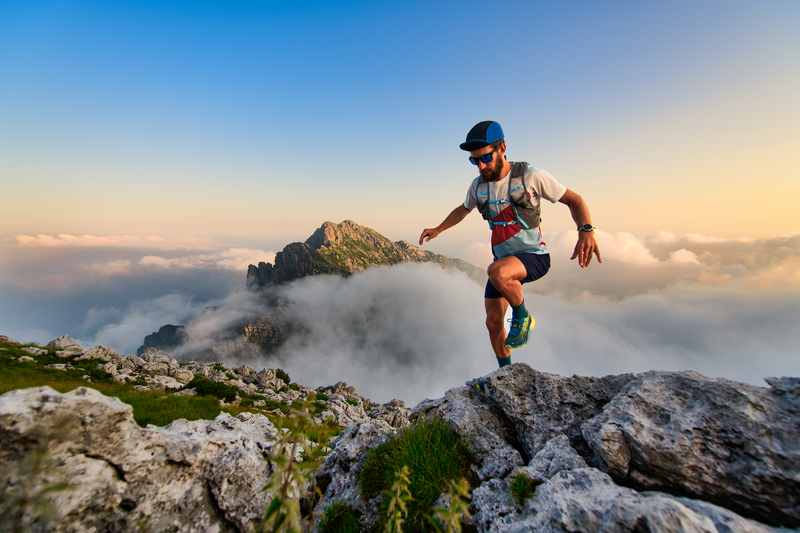
95% of researchers rate our articles as excellent or good
Learn more about the work of our research integrity team to safeguard the quality of each article we publish.
Find out more
MINI REVIEW article
Front. Pain Res. , 11 January 2023
Sec. Neuromodulatory Interventions
Volume 3 - 2022 | https://doi.org/10.3389/fpain.2022.1084701
This article is part of the Research Topic Advancements in Deep Brain Stimulation for Chronic Pain Control View all 7 articles
The use of deep brain stimulation (DBS) for the treatment of chronic pain was one of the first applications of this technique in functional neurosurgery. Established brain targets in the clinic include the periaqueductal (PAG)/periventricular gray matter (PVG) and sensory thalamic nuclei. More recently, the anterior cingulum (ACC) and the ventral striatum/anterior limb of the internal capsule (VS/ALIC) have been investigated for the treatment of emotional components of pain. In the clinic, most studies showed a response in 20%–70% of patients. In various applications of DBS, animal models either provided the rationale for the development of clinical trials or were utilized as a tool to study potential mechanisms of stimulation responses. Despite the complex nature of pain and the fact that animal models cannot reliably reflect the subjective nature of this condition, multiple preparations have emerged over the years. Overall, DBS was shown to produce an antinociceptive effect in rodents when delivered to targets known to induce analgesic effects in humans, suggesting a good predictive validity. Compared to the relatively high number of clinical trials in the field, however, the number of animal studies has been somewhat limited. Additional investigation using modern neuroscience techniques could unravel the mechanisms and neurocircuitry involved in the analgesic effects of DBS and help to optimize this therapy.
Chronic pain is a major health problem associated with individual suffering and an important social and economic burden. The prevalence of this condition in the general population ranges from 8% to 50% (1–3). It is estimated that approximately 30% of chronic pain patients have neuropathic pain (4, 5). This may be defined as pain due to lesions and/or dysfunction of the nervous system. Though pharmacotherapy, physiotherapy and nerve blocks are often effective, the treatment of neuropathic pain may be quite challenging. This is in contrast to nociceptive pain, which is associated with physical damage to the body.
Deep brain stimulation (DBS) involves the delivery of electrical current to the brain parenchyma through implanted electrodes (6, 7). Its use for the treatment of pain was one of the first applications of this technique in Functional Neurosurgery (8–10). Despite being proposed approximately 70 years ago, it was only in the 1970s and 1980s that DBS became more widely studied for the treatment of pain, with stimulation being more frequently delivered to the periaqueductal (PAG)/periventricular gray matter (PVG), sensory thalamic nuclei, or the internal capsule (IC) (11, 12). More recently, with a better appreciation of the emotional components of pain, stimulation of the anterior cingulate cortex (ACC) (13–16) and the ventral striatum/anterior limb of the internal capsule (VS/ALIC) (17) has also been proposed.
In various applications of DBS, animal models have either provided the rational for clinical use or were utilized to study potential mechanisms of therapeutic responses (7, 18). Despite being conducted for over 50 years, the number of reports studying the effects of DBS in preclinical models may be considered limited compared to that of human publications.
In this review, we summarize the effects of DBS in preclinical models and in patients with chronic pain.
Prior to discussing preclinical work, it is worth differentiating nociception from pain. The former refers to the neural encoding of impending or actual tissue damage, whereas the later denotes the subjective experience of actual or impending harm. In general, preclinical models are largely suited to measure nociception. That said, animals are likely to have a subjective experience associated with nociception. Unfortunately, measuring this component is not straightforward. To decrease the gap with the human condition, proposed approaches include measures of spontaneous and evoked pain, pain memory, avoidance, anxiety- or depression-like behaviors, and the social modulation of pain (empathy).
Structures targeted in preclinical DBS studies are either part of the pain matrix, limbic system, descending analgesic systems, or those potentially capable of modulating nociceptive signals (Figure 1). Itemized results according to target are presented below.
Figure 1. Deep brain stimulation (DBS) targets. Schematic representation of DBS targets studied in recent clinical trials (A) and preclinical models (B). ACC, anterior cingulum; PAG, periaqueductal gray matter; PVG, periventricular gray matter; PVN, paraventricular nucleus; VS/ALIC, ventral striatum/anterior limb of the internal capsule.
The PAG is a highly conserved midbrain region that surrounds the cerebral aqueduct (19). It is a critical site for encoding aversive prediction errors and the processing of nociceptive information (19). The PAG receives strong nociceptive input from the spinal cord and provides top-down brainstem–spinal cord modulation to flexibly shape the experience of pain in different contexts, including the transition from acute to chronic states (19). Two separate nociceptive modulatory systems operate in the caudal PAG: a dorsal system, which encompasses its dorsomedial, dorsolateral and lateral subdivisions, and a ventral system that includes the ventrolateral PAG and dorsal raphe (20).
In an initial report, Reynolds (21) showed that PAG electrical stimulation produced analgesia in rats, opening a new research venue for the treatment of pain. Following that work, PAG electrical stimulation was considered a pain suppression method against various nocifensive behaviors (22, 23). In rats, electrical stimulation of the PAG inhibited spinal nociceptive transmission from the brainstem by activating descending inhibition mediated by opioids and glutamate (24). Analgesia-producing PAG stimulation alters the spontaneous activity of neurons in the medullary reticular formation (MRF) and inhibits the noxious-evoked excitation of MRF neurons. The microinjection of morphine into the PAG mitigated the reduced spontaneous activity of MRF units and inhibited noxious-evoked neuronal excitation. These effects were particularly observed in analgesia produced by PAG manipulations and were partially reversed by naloxone (25). PAG stimulation attenuated formalin-induced pain and increased the nociceptive threshold of complete Freund's adjuvant (CFA)-induced inflammatory pain (20, 26, 27). In addition, PAG stimulation has also been shown to inhibit visceral nociceptive responses of spinal dorsal horn neurons (28). Overall, the results described above suggest that electrical stimulation of the PAG works more effectively on nociceptive pain, including that related to acute and chronic inflammation.
Initial evidence for targeting the sensory thalamus in pain derived from the ablative neurosurgical literature (29–31). Sensory thalamic nuclei receive somatotopically oriented input from lemniscal fibers involved in sensory processing. Afferents from the face and body innervate the ventral posteromedial (VPM) and ventral posterolateral (VPL) nuclei, respectively. In rats with neuropathic pain, VPL-DBS reduced mechanical allodynia (27, 32–34), cold allodynia (35), and thermal hyperalgesia (36). Similar to humans, VPL-induced analgesia in rats with peripheral neuropathy was only documented when electrodes were implanted in a somatotopic manner (33). In inflammatory pain models, VPL stimulation was found to produce analgesia in chronic, but not acute preparations (27). Sensory thalamic stimulation also failed to reduce experimental pain in healthy animals (37, 38). These observations are well aligned with clinical findings suggesting that VPL-induced analgesia is more effective for the treatment of neuropathic pain (39–41).
The mechanisms through which thalamic DBS induces analgesia are still unclear. VPM-DBS inhibited the abnormal hyperactivity of nociceptive neurons in the trigeminal medullary dorsal horn of deafferented cats (42). In primates, sensory thalamic stimulation decreased the activation of spinothalamic tract neurons (43), an effect that seems to be partly mediated by corticofugal and descending inhibitory pathways (44). Corroborating these findings, VPL-DBS has been suggested to induce serotonin release in the spinal cord of primates via the modulation of raphe-spinal tract fibers (45). VPL stimulation has also been shown to inhibit local neurons and induce neuronal reorganization, modifying spontaneous pathological activity, and inhibiting nociceptive impulse transmission to cortical areas (46, 47).
In cats (48) and rats (49), electrical stimulation of IC fibers reduced thalamic nociceptive activity, having no effect on activity of dorsal horn neurons in response to noxious heat (50). IC stimulation inhibited neuronal hyperactivation in the spinal trigeminal nucleus in a cat model of trigeminal nerve deafferentation (51). In rats, IC stimulation induced analgesia that was partially associated to aversive responses (52). Moreover, IC stimulation inhibited nociceptive neurons in the rat medullary dorsal horn, suggesting that its inhibitory nociceptive effect may occur via second-order neurons, following the activation of corticofugal fibers (53).
The insula is a key integration center of nociceptive stimuli, representing an interface between sensory and limbic systems (54). Neuronal tracing studies in primates has identified the posterior insula as an important relay in the spinothalamic pathway via connections with the VPM (54–57). Furthermore, the posterior insula is considered an important cortical center for the integration of pain and interoception (54, 58–63).
In addition to its role as a relay in ascending pain pathways, the posterior insula is densely connected with the anterior insula, which projects to (i) brainstem nuclei involved in the descending pain modulation system (raphe, locus coeruleus, ventral tegmental area, and PAG), and (ii) limbic structures involved in the emotional valence to painful stimuli, such as the ACC and the amygdala (61, 62, 64). Intracranial recordings during painful stimuli have demonstrated the existence of nociceptive neurons in the posterior insula (54, 65). In addition, most chronic pain syndromes are associated with abnormal functional connectivity of the posterior insula. Finally, electrical stimulation of the posterior insula in neuropathic-pain rats induced antinociception by functionally modulating the opioid, cannabinoid and GABAergic systems in the PAG (66, 67). Along these lines, electrical stimulation of the insula has been proposed as a potential therapeutic alternative to restore maladaptive connectivity and activity in pathways mediating sensory and affective hypersensitivity in patients with chronic pain (54).
The limbic system includes a wide range of interconnected brain regions that process and regulate cognitive function, sensory perception, memory, emotions, and affective motivation (68). Various limbic regions participate in pain processing circuits, including the hypothalamus (68–70), amygdala, and ACC (68, 71–73).
The hypothalamus, a central structure in the hypothalamic-pituitary-adrenal (HPA) axis, is involved in mechanisms of psychiatric disorders, including depression, anxiety, and anorexia. The ventromedial hypothalamus (VMH), in particular, contributes to the processing of the affective dimension of pain (74), whereas the paraventricular nucleus (PVN) is part of the pain inhibitory system (75–78). Pain induced by electrical stimulation or chemical methods increases immediate early gene expression in the PVN (79–81).
In rodents, electrical stimulation of the PVN induced analgesia (78), decreased thermal pain sensitivity, knee inflammation and synovial neutrophilic infiltration (82). PVN electric stimulation enhanced c-Fos expression in the dorsal horn of the spinal cord, nucleus raphe magnus, locus coeruleus, and the PAG (75). Some of the analgesic effects of PVN stimulation seem to be mediated via the release of oxytocin from descending fibers to the dorsal horn (75–77).
As described above, the ACC is involved in affective-emotional aspects of pain processing (83–89). Long-term potentiation of excitatory synaptic transmission in the ACC sustains chronic neuropathic pain conditions and pain-related anxiety (90). In rodents, ACC stimulation inhibits activity of dorsal horn neurons induced by noxious mechanical stimuli (91, 92), reduces mechanical allodynia in neuropathic pain models (93), and the aversive response to noxious tactile stimulation (94). Some of these effects were associated with the activation of ACC inhibitory neurons, as optogenetic stimulation of these cells inhibited thalamic activity and reduced nociceptive behavior in experimental models of acute and chronic pain (95, 96). Similar to other brain structures, the ACC opioid system is critical to selectively modulate the aversive quality of noxious mechanical stimulation in neuropathic pain models (97). In rodents, ACC high-frequency stimulation reduced aversive pain responses induced by mechanical nociceptive stimuli, while low-frequency stimulation increased pain aversive behaviors (98). Optogenetic or chemogenetic stimulation of the ACC reduced abdominal hyperalgesia and pain-related anxiety in a rat model of chronic pancreatitis (99).
Structures commonly targeted in patients with chronic pain are the PAG/PVG and sensory thalamic nuclei (11, 12) (Figure 1). Targets investigated to reduce the emotional components of pain include the ACC (13–16) and the VS/ALIC (17). DBS targets proposed in the past that are not routinely used to date include the IC (100, 101), septal region (102, 103), and medial thalamus (104–106).
The choice of surgical target in humans depends on whether patients present predominant neuropathic or nociceptive pain and the clinical condition associated with its development. As in animal models, it seems that stimulation of the sensory thalamus is more effective for neuropathic pain, whereas PAG/PVG has also been offered in the past to patients with nociceptive pain (39, 107–110).
The atlas most frequently used by functional neurosurgeons is the one by Schaltenbrand and Wahren (111, 112). Because the nomenclature of thalamic subdivisions in that atlas is the one proposed by Hassler (113), his system has been commonly used. According to Hassler, sensory modalities from lemniscal fibers innervate the ventralis caudalis nucleus (Vc) (113), which corresponds to the ventral posterior nuclei descried in animal studies (114). In most centers, target selection in the sensory thalamus is based on coordinates from the anterior-posterior (AC-PC) commissural plane and midcommissural point (12). As in preclinical models, Vc stimulation has to be delivered somatotopically, with face, arm, and leg regions represented from medial to lateral (114). PAG/PVG is largely targeted based on direct visualization, as this structure lies near the boundaries of the III ventricle and cerebral aqueduct. In addition to neuroimaging strategies, several centers use electrophysiology to establish the ideal target. In the sensory thalamus (115–118), stimulation induces paresthesias in projected fields, which may be used to define the somatotopic coverage of the region of interest. In addition, cells in the sensory thalamus tend to fire in specific patterns and respond to tactile and sensory stimuli (114). In contrast, PAG/PVG stimulation occasionally induces a sensation of warmth that may be characterized as pleasurable (100, 119, 120). Stimulation delivered to the PAG may sometimes induce a sense of anxiety and fear (100, 119, 120).
As described above, thalamic stimulation has been commonly used to treat neuropathic pain associated with stroke, spinal cord injury, multiple sclerosis, phantom limb pain, among others, whereas PAG/PVG was also used in the past to treat nociceptive pain (110). With the development of effective pharmacological and non-invasive treatment modalities for nociceptive pain, the use of DBS for these indications has rapidly declined.
The long-term outcome of DBS for the treatment of chronic neuropathic pain is quite variable, with most studies showing a response in 20%–70% of the patients (39, 100, 107–109, 118–136). In fact, the variability observed across studies in a recent systematic review did not allow the authors to conduct a meta-analysis (137). The inconsistency in reported outcomes has been attributed to multiple factors, including the treatment of different clinical conditions, technical aspects, and the selection of different brain targets. As described above, the overall impression in the field is that patients with neuropathic pain tend to do worse with the procedure. Since these patients predominate over those with nociceptive pain in recent studies, outcome in some recent trials tends to be worse than that of older studies. Another aspect is that patients may lose clinical benefit over time. Reasons to explain this phenomenon are unclear but may involve neuroplasticity and tolerance (100, 110, 138). The largest open-labeled multicenter trials in the field were conducted by one of the manufacturers of DBS systems (Medtronic) (139). Both studies were negative and showed a relatively poor outcome with a higher than 50% improvement being reported in 16%–47% and 13%–18% at 12 and 24 months, respectively (139).
Additional DBS targets investigated in recent clinical trials are the ACC and VS/ALIC. Following ACC stimulation, pain scores were improved by 60% and 43% at 6 and 12 months, respectively (13). Of note, ACC DBS not only induced an analgesic effect, but also improved affective components of pain (13, 14). As for the VS/ALIC, a recent randomized clinical trial in patients with poststroke pain found no difference between active or sham stimulation on the Pain Disability Index (primary outcome variable), but revealed a significant improvement in outcome measures related to the affective sphere of pain (17).
As DBS is a surgical procedure, it is important to describe potential adverse effects. There is a 2%–3% risk of intracranial hemorrhages, mostly asymptomatic. The surgery involves the implantation of hardware (electrodes, extension cables and pulse generators) (6, 39, 108, 109, 130, 140) and lead problems occur in 4%–5% of the patients. Infections may occur in 3%–5% of the patients. In approximately 50% of these subjects, parts or the entire system may need to be removed (141). Although not particularly considered a side effect, some patients implanted with Vc electrodes do not tolerate stimulation-induced paresthesias. As described above, PAG DBS has been associated with the development of stimulation-induced anxiety (110, 120, 130). A recently reported complication of ACC DBS involves the development of afterdischarges and seizures, which can be controlled by changing stimulation settings (13, 142).
As described above, optimal DBS targets for the treatment of chronic pain are still under investigation. Possible explanations for the variable results of different preclinical and clinical studies include the complex nature of pain, the study of different conditions leading to the development of pain, and the potential involvement of multiple networks. In recent years, the concept of a traditional pain matrix has been complemented by that of a pain connectome (143). This is because pain has various salient qualities, being influenced by attention, mood and cognitive aspects (143). For example, attention-demanding tasks and stimuli can alter the quality and salience of pain and the processing of nociceptive input (144–146). In addition, connectomes can take into account the intrinsically dynamic and fluctuating relations of multiple brain structures that play a role in different aspects of pain and cognitive processes (147). Recent studies suggest that human networks constantly change over time, presenting a dynamic repertoire of brain states, including those relevant to pain and attention (148–150). Three brain systems are critical components of the pain connectome: The first is the salience network, comprised of the anterior insula, mid-cingulate cortex, temporoparietal junction, and dorsolateral prefrontal cortex (151, 152). The second system is the default mode network (DMN), which is active when subjects are instructed not to think about something specific (153). The DMN is comprised of the posterior cingulate cortex, precuneus, medial prefrontal cortex (PFC), lateral parietal lobe, and areas within the medial temporal lobe. The third system is the descending pain modulatory system and includes the PAG (143, 145, 154, 155).
The thalamus and PAG are part of the canonical analgesic pathway. The somatotopically organized sensory thalamus receives input from wide-dynamic range and nociceptive-specific neurons from the dorsal horn of the spinal cord and projects to somatosensory cortical regions (156). The disinhibition of the PAG leads to a release of norepinephrine and serotonin from the locus coeruleus and raphe into the dorsal horn, dampening pain transmission (157). Considering that high frequency DBS inhibits neuronal activity (158–160), one of the potential analgesic mechanisms of this therapy could be related to a decrease in glutamatergic transmission from thalamic cells projecting to the primary sensory cortex or a reduction in GABAergic PAG firing, followed by the disinhibition of analgesic descending pathways. This would be in line with a stimulation-induced modulation of somatosensory pain processes. In addition, chronic pain is associated with important affective-emotional components that result from the activation of the dynamic pain matrix, which involves several brain areas. The anterior limb of the internal capsule is considered a pivotal structure that interconnects limbic regions, thalamic nuclei, the ACC and PFC (161). In addition to the inhibition of neuronal cell bodies, DBS has been shown to activate neuronal appendages (i.e., axonal projections and dendrites) (7, 158, 160). Because the ALIC is predominantly composed by prefrontal/orbitofrontal-subcortical projections (161–163), stimulation of some of its limbic connections may regulate affective and emotional components of pain. Of note, ALIC DBS has been approved for the treatment of obsessive compulsive disorders and is under investigation for major depressive disorder (164–166). Another important structure in the pain neurocircuitry and connectome is the ACC, as it plays a role in depressive- and anxiety-like behaviors (167). The ACC is interconnected with the PFC, thalamus and amygdala, a set of structures rich in opiod receptors that are associated with emotional-affective deficits in individuals with chronic pain (168–170). Therefore, it is conceivable that the analgesic effects of ACC-DBS may be, at least in part, attributed to the modulation of ACC-amygdala opioid pathway. Amygdaloid projection to the PVN (171) have been shown to mediate pain transmission via the release of oxytocin into the dorsal horn (76, 77, 172) or following the activation of the locus coeruleus and raphe (173, 174). These data suggest that the pain matrix and connectome may be modulated through the stimulation of different structures, which may not only be involved in somatosensory, but also in cognitive and emotional aspects of pain.
In the clinic, not many DBS targets have been systematically investigated in patients with chronic pain. It is possible that some of the additional structures proposed in preclinical studies may yield more pronounced analgesic effects. A question that remains unanswered is whether any specific target is better than the other or if potential synergistic effects may occur. It is possible that a combination of targets affecting different aspects or hubs in the pain connectome may induce summative effects and more robust responses (e.g., habenula, PFC, thalamus, PAG/PVG, nucleus accumbens). This question can be potentially addressed in preclinical models using a battery of tests to investigate multiple domains. Also to be examined in preclinical models is the contribution of additional DBS mechanisms for the analgesic effects of this therapy, which go beyond the activation and inhibition of neuronal cell bodies and fibers (175). These include multiple forms of neuroplasticity, metabolic changes, neurotransmitter release, structural receptor changes, among other (7, 175, 176).
The outcome of DBS for the treatment of chronic pain is quite variable. At present, reasons for this variability have been speculated, but not clearly established. In some recently published series and in two multicenter trials, outcome was worse than the one reported in older studies (119, 135, 177). Despite the relatively small number of patients who benefit from the procedure, it seems that responders derive substantial benefit from DBS (119). With that in mind, an aspect that needs to be addressed is the development of potential biomarkers of treatment response.
As some of the effects of PAG/PVG DBS in preclinical models are mediated by endorphins, the clinical use of the morphine-naloxone test has been advocated (100, 120, 126, 178). During this test, patients are given morphine, followed by naloxone. Individuals who experience a substantial recurrence of symptoms are considered to have a prominent nociceptive component and amenable to PAG/PVG DBS. That said, the validity of this test is still unclear (179). As described above, older studies have shown a good postoperative DBS response, particularly when stimulation was delivered to the PAG/PVG, in patients with nociceptive pain. In general, nociceptive pain has a better response to opioids than neuropathic pain (180). In rodents, PAG/PVG stimulation modulates endogenous opioid transmission, suggesting a potential opioid-mediated mechanism for the antinociceptive effect of stimulation in this target. Though pain involves brain processes, the effects of DBS delivered to different regions on multiple neural circuits and neurotransmitter systems may help to explain the variable response of this therapy in patients with neuropathic and nociceptive pain.
One factor that seems to be related to a good postoperative outcome is the clinical condition leading to the development of pain. Patients with brachial plexus avulsion, complex regional pain syndrome, and peripheral neuropathy seem to have a better response to DBS than those with postherpetic neuralgia or thalamic pain (39, 108, 109, 130, 135). Psychological or litigation problems forecast a poor prognosis (39, 110, 130). Preclinical work could help to address some of these aspects, as animals, in theory, do not have a strong psychosocial overlay. That said, the effects of cognitive, stress and depression-like behaviors in animal models of nociception have been poorly explored. This field of research could certainly be expanded, since DBS delivered to different targets has been shown to induce antidepressant-, antianhedonic-, and anxiolytic-like effects in rodents (7, 159, 181). To more closely mimic the multiple components of pain in humans and increase its translational value of preclinical studies, models could include not only nociceptive assessments, but a battery of paradigms to evaluate cognitive and psychiatric-like behaviors as well.
Another factor suggested to forecast a positive response to invasive neuromodulation procedures is the so-called insertional effect, characterized by the amelioration of pain immediately after electrode implantation in the absence of stimulation (119, 182). This may also explain the better results observed in preclinical models compared to humans, as the ratio between the electrode diameter and target volume is far more pronounced in rodents.
In a recent series of studies in patients implanted with both Vc and PVG electrodes, field potentials were recorded in the thalamus during stimulation of the latter (183, 184). A decrease in low frequency thalamic potentials after PVG stimulation was found to predict a good therapeutic response to DBS (183, 184).
Despite the complex nature of pain and the fact that animal models do not reflect the subjective nature of this condition, multiple preparations have emerged over the years. These are rooted in dimensions of face, construct and predictive validity (7, 18, 159). The former refers to similarities between the model and clinical symptoms. Construct validity reflects neurobiological similarities between the model and the human condition. Predictive validity reflects commonalities in treatment response between patients and the preclinical scenario. Our study confirms the predictive validity of animal models, as DBS delivered to clinically relevant targets in animals reduces nociception. Compared to the vast clinical literature, a limited number of studies have been published in animal models. With the translational potential described above and the well-described mechanisms of nociception, work in preclinical models is certainly underutilized in the field of DBS for pain. Additional studies using modern neuroscience techniques could unravel the mechanisms and neurocircuitry involved in the analgesic effects of DBS and help to optimize this therapy. These could include the use of batteries of tests to measure the effects of DBS in different behavioral domains, the use of connectivity analyses, the stimulation of multiple brain targets at the same time, or the co-treatment of animals with DBS and different medications to assess whether certain classes of drugs may potentiate the effects of stimulation (7, 185, 186). In addition, chemogenetics, optogenetics and other molecular techniques can be used to deconstruct and dissect the neural circuits and cells involved in the mechanisms of DBS.
RP, CD and CH wrote the manuscript. AP reviewed the manuscript for intellectual content and contributed with the figure. All authors contributed to the article and approved the submitted version.
CD received support from the São Paulo Research Foundation, Brazil (FAPESP; Fundação de Amparo a Pesquisa do Estado de São Paulo).
The authors declare that the research was conducted in the absence of any commercial or financial relationships that could be construed as a potential conflict of interest.
All claims expressed in this article are solely those of the authors and do not necessarily represent those of their affiliated organizations, or those of the publisher, the editors and the reviewers. Any product that may be evaluated in this article, or claim that may be made by its manufacturer, is not guaranteed or endorsed by the publisher.
1. Gerdle B, Björk J, Henriksson C, Bengtsson A. Prevalence of current and chronic pain and their influences upon work and healthcare-seeking: a population study. J Rheumatol. (2004) 31:1399–406. doi: 10.1186/1471-2474-9-102
4. Davies H, Crombie I, Macrae W, Rogers K. Pain clinic patients in northern britain. Pain Clin. (1992) 5:129–35.
5. Torrance N, Smith BH, Bennett MI, Lee AJ. The epidemiology of chronic pain of predominantly neuropathic origin. Results from a general population survey. J Pain. (2006) 7:281–9. doi: 10.1016/j.jpain.2005.11.008
6. Awan NR, Lozano A, Hamani C. Deep brain stimulation: current and future perspectives. Neurosurg Focus. (2009) 27:E2. doi: 10.3171/2009.4.FOCUS0982
7. Hamani C, Temel Y. Deep brain stimulation for psychiatric disease: contributions and validity of animal models. Sci Transl Med. (2012) 4:142rv8. doi: 10.1126/scitranslmed.3003722
8. Heath R. Studies in schizophrenia: A multidisciplinary approach to mind-brain relationships. Cambridge, Massachusetts: Harvard University Press (1954).
9. Heath R, Mickle WA. Evaluation of seven years’ experience with depth electrode studies in human patients. Electrical studies on the unanesthetized human brain. New York: Paul B. Hoeber, Inc. (1960). 214–47.
10. Pool JL, Clark WK, Hudson P, Lombardo M. Steroid hormonal response to stimulation of electrodes implanted in the subfrontal parts of the brain).
11. Mazars G, Merienne L, Cioloca C. Treatment of certain types of pain with implantable thalamic stimulators. Neurochirurgie. (1974) 20:117–24.4418054
12. Hamani C, Fontaine D, Lozano A. DBS for persistent non-cancer pain. In: Lozano AM, Gildenberg PL, Tasker TT, editors. Textbook of stereotactic and functional neurosurgery. Berlin: Springer (2009). p. 2227–38.
13. Boccard SGJ, Prangnell SJ, Pycroft L, Cheeran B, Moir L, Pereira EAC, et al. Long-term results of deep brain stimulation of the anterior cingulate cortex for neuropathic pain. World Neurosurg. (2017) 106:625–37. doi: 10.1016/j.wneu.2017.06.173
14. Boccard SGJ, Fitzgerald JJ, Pereira EAC, Moir L, Van Hartevelt TJ, Kringelbach ML, et al. Targeting the affective component of chronic pain: a case series of deep brain stimulation of the anterior cingulate cortex. Neurosurgery. (2014) 74:628–35; discussion 635–37. doi: 10.1227/NEU.0000000000000321
15. Boccard SGJ, Pereira EAC, Moir L, Van Hartevelt TJ, Kringelbach ML, FitzGerald JJ, et al. Deep brain stimulation of the anterior cingulate cortex: targeting the affective component of chronic pain. Neuroreport. (2014) 25:83–8. doi: 10.1097/WNR.0000000000000039
16. Spooner J, Yu H, Kao C, Sillay K, Konrad P. Neuromodulation of the cingulum for neuropathic pain after spinal cord injury. Case report. J Neurosurg. (2007) 107:169–72. doi: 10.3171/JNS-07/07/0169
17. Lempka SF, Malone DA, Hu B, Baker KB, Wyant A, Ozinga JG, et al. Randomized clinical trial of deep brain stimulation for poststroke pain. Ann Neurol. (2017) 81:653–63. doi: 10.1002/ana.24927
18. Hamani C, Nobrega JN, Lozano AM. Deep brain stimulation in clinical practice and in animal models. Clin Pharmacol Ther. (2010) 88:559–62. doi: 10.1038/clpt.2010.133
19. Eippert F, Tracey I. Pain and the PAG: learning from painful mistakes. Nat Neurosci. (2014) 17:1438–9. doi: 10.1038/nn.3844
20. Morgan MM, Gold MS, Liebeskind JC, Stein C. Periaqueductal gray stimulation produces a spinally mediated, opioid antinociception for the inflamed hindpaw of the rat. Brain Res. (1991) 545:17–23. doi: 10.1016/0006-8993(91)91264-2
21. Reynolds DV. Surgery in the rat during electrical analgesia induced by focal brain stimulation. Science. (1969) 164:444–5. doi: 10.1126/science.164.3878.444
22. Levine R, Morgan MM, Cannon JT, Liebeskind JC. Stimulation of the periaqueductal gray matter of the rat produces a preferential ipsilateral antinociception. Brain Res. (1991) 567:140–4. doi: 10.1016/0006-8993(91)91446-8
23. Lee BH, Park SH, Won R, Park YG, Sohn JH. Antiallodynic effects produced by stimulation of the periaqueductal gray matter in a rat model of neuropathic pain. Neurosci Lett. (2000) 291:29–32. doi: 10.1016/s0304-3940(00)01375-6
24. Jones SL, Gebhart GF. Inhibition of spinal nociceptive transmission from the midbrain, pons and medulla in the rat: activation of descending inhibition by morphine, glutamate and electrical stimulation. Brain Res. (1988) 460:281–96. doi: 10.1016/0006-8993(88)90373-3
25. Mohrland JS, Gebhart GF. Effects of focal electrical stimulation and morphine microinjection in the periaqueductal gray of the rat mesencephalon on neuronal activity in the medullary reticular formation. Brain Res. (1980) 201:23–37. doi: 10.1016/0006-8993(80)90772-6
26. Morgan MM, Sohn JH, Liebeskind JC. Stimulation of the periaqueductal gray matter inhibits nociception at the supraspinal as well as spinal level. Brain Res. (1989) 502:61–6. doi: 10.1016/0006-8993(89)90461-7
27. Wang N, Zhang T, Su Y-L, Wang J-Y, Luo F. Differential modulation of electrical stimulation of periaqueductal gray and thalamus on nociceptive behaviors of rats. Sheng Li Xue Bao. (2016) 68:115–25.27108897
28. Okada K, Murase K, Kawakita K. Effects of electrical stimulation of thalamic nucleus submedius and periaqueductal gray on the visceral nociceptive responses of spinal dorsal horn neurons in the rat. Brain Res. (1999) 834:112–21. doi: 10.1016/s0006-8993(99)01593-0
29. Mark VH, Ervin FR, Hackett TP. Clinical aspects of stereotactic thalamotomy in the human. Part I. The treatment of chronic severe pain. Arch Neurol. (1960) 3:351–67. doi: 10.1001/archneur.1960.00450040001001
30. Mark VH, Ervin FR. Role of thalamotomy in treatment of chronic severe pain. Postgrad Med. (1965) 37:563–71. doi: 10.1080/00325481.1965.11695514
31. Ervin FR, Brown CE, Mark VH. Striatal influence on facial pain. Confin Neurol. (1966) 27:75–90. doi: 10.1159/000103936
32. Kupers RC, Gybels JM. Electrical stimulation of the ventroposterolateral thalamic nucleus (VPL) reduces mechanical allodynia in a rat model of neuropathic pain. Neurosci Lett. (1993) 150:95–8. doi: 10.1016/0304-3940(93)90116-3
33. Kim J, Kim J, Min KS, Lee SE, Kim SJ, Chang JW. VPL-DBS on neuropathic pain rat model is effective in mechanical allodynia than cold allodynia. Neurol Sci. (2012) 33:1265–70. doi: 10.1007/s10072-012-1097-7
34. Kim J, Lee SE, Shin J, Jung HH, Kim SJ, Chang JW. The neuromodulation of neuropathic pain by measuring pain response rate and pain response duration in animal. J Korean Neurosurg Soc. (2015) 57:6–11. doi: 10.3340/jkns.2015.57.1.6
35. Kim J, Eun Lee S, Sik Min K, Jung HH, Lee JE, Kim SJ, et al. Ventral posterolateral deep brain stimulation treatment for neuropathic pain shortens pain response after cold stimuli. J Neurosci Res. (2013) 91:997–1004. doi: 10.1002/jnr.23222
36. Iwata M, LeBlanc BW, Kadasi LM, Zerah ML, Cosgrove RG, Saab CY. High-frequency stimulation in the ventral posterolateral thalamus reverses electrophysiologic changes and hyperalgesia in a rat model of peripheral neuropathic pain. Pain. (2011) 152:2505–13. doi: 10.1016/j.pain.2011.07.011
37. Schmidek HH, Fohanno D, Ervin FR, Sweet WH. Pain threshold alterations by brain stimulation in the monkey. J Neurosurg. (1971) 35:715–22. doi: 10.3171/jns.1971.35.6.0715
38. Mayer DJ, Liebeskind JC. Pain reduction by focal electrical stimulation of the brain: an anatomical and behavioral analysis. Brain Res. (1974) 68:73–93. doi: 10.1016/0006-8993(74)90534-4
39. Levy RM, Lamb S, Adams JE. Treatment of chronic pain by deep brain stimulation: long term follow-up and review of the literature. Neurosurgery. (1987) 21:885–93. doi: 10.1227/00006123-198712000-00017
40. Falowski SM. Deep brain stimulation for chronic pain. Curr Pain Headache Rep. (2015) 19:27. doi: 10.1007/s11916-015-0504-1
41. Nguyen J-P, Nizard J, Keravel Y, Lefaucheur J-P. Invasive brain stimulation for the treatment of neuropathic pain. Nat Rev Neurol. (2011) 7:699–709. doi: 10.1038/nrneurol.2011.138
42. Tsubokawa T, Katayama Y, Yamamoto T, Hirayama T. Deafferentation pain and stimulation of the thalamic sensory relay nucleus: clinical and experimental study. Appl Neurophysiol. (1985) 48:166–71. doi: 10.1159/000101122
43. Gerhart KD, Yezierski RP, Wilcox TK, Grossman AE, Willis WD. Inhibition of primate spinothalamic tract neurons by stimulation in ipsilateral or contralateral ventral posterior lateral (VPLc) thalamic nucleus. Brain Res. (1981) 229:514–9. doi: 10.1016/0006-8993(81)91014-3
44. Gerhart KD, Yezierski RP, Fang ZR, Willis WD. Inhibition of primate spinothalamic tract neurons by stimulation in ventral posterior lateral (VPLc) thalamic nucleus: possible mechanisms. J Neurophysiol. (1983) 49:406–23. doi: 10.1152/jn.1983.49.2.406
45. Sorkin LS, McAdoo DJ, Willis WD. Stimulation in the ventral posterior lateral nucleus of the primate thalamus leads to release of serotonin in the lumbar spinal cord. Brain Res. (1992) 581:307–10. doi: 10.1016/0006-8993(92)90722-l
46. Benabid AL, Benazzous A, Pollak P. Mechanisms of deep brain stimulation. Mov Disord. (2002) 17(Suppl 3):S73–74. doi: 10.1002/mds.10145
47. Hammond C, Ammari R, Bioulac B, Garcia L. Latest view on the mechanism of action of deep brain stimulation. Mov Disord. (2008) 23:2111–21. doi: 10.1002/mds.22120
48. Nishimoto A, Namba S, Nakao Y, Matsumoto Y, Ohmoto T. Inhibition of nociceptive neurons by internal capsule stimulation. Appl Neurophysiol. (1984) 47:117–27. doi: 10.1159/000101212
49. Okada N, Matsumoto N, Kitada Y. Responses of diencephalic nociceptive neurones to orofacial stimuli and effects of internal capsule stimulation in the rat. Arch Oral Biol. (2002) 47:815–29. doi: 10.1016/s0003-9969(02)00118-8
50. Siegel J, Morton CR, Sandkühler J, Xiao HM, Zimmermann M. Spinal neuronal inhibition and EEG synchrony by electrical stimulation in subcortical forebrain regions of the cat. Exp Brain Res. (1986) 62:363–72. doi: 10.1007/BF00238856
51. Namba S, Nishimoto A. Stimulation of internal capsule, thalamic sensory nucleus (VPM) and cerebral cortex inhibited deafferentation hyperactivity provoked after gasserian ganglionectomy in cat. Acta Neurochir Suppl. (1988) 42:243–7. doi: 10.1007/978-3-7091-8975-7_47
52. Morgan MJ, Franklin KBJ. Stimulation-produced analgesia (SPA) from brain-stem and diencephalic sites in the rat: relationships between analgesia, aversion, seizures and catalepsy. Pain. (1988) 33:109–21. doi: 10.1016/0304-3959(88)90210-2
53. Matsumoto N, Fukuda D, Murata J, Yamada H, Miura H, Kitada Y. Effect of electrical stimulation of the internal capsule on nociceptive neurons responding to orofacial stimuli in the medullary dorsal horn of the rat. Arch Oral Biol. (2006) 51:930–9. doi: 10.1016/j.archoralbio.2006.04.005
54. Bergeron D, Obaid S, Fournier-Gosselin M-P, Bouthillier A, Nguyen DK. Deep brain stimulation of the posterior insula in chronic pain: a theoretical framework. Brain Sci. (2021) 11:639. doi: 10.3390/brainsci11050639
55. Craig AD, Zhang ET, Blomqvist A. Association of spinothalamic lamina I neurons and their ascending axons with calbindin-immunoreactivity in monkey and human. Pain. (2002) 97:105–15. doi: 10.1016/s0304-3959(02)00009-x
56. Craig ADB. Pain mechanisms: labeled lines versus convergence in central processing. Annu Rev Neurosci. (2003) 26:1–30. doi: 10.1146/annurev.neuro.26.041002.131022
57. Craig ADB. Topographically organized projection to posterior insular cortex from the posterior portion of the ventral medial nucleus in the long-tailed macaque monkey. J Comp Neurol. (2014) 522:36–63. doi: 10.1002/cne.23425
58. Craig AD. Interoception: the sense of the physiological condition of the body. Curr Opin Neurobiol. (2003) 13:500–5. doi: 10.1016/s0959-4388(03)00090-4
59. Craig AD, Bushnell MC, Zhang ET, Blomqvist A. A thalamic nucleus specific for pain and temperature sensation. Nature. (1994) 372:770–3. doi: 10.1038/372770a0
60. Craig AD, Blomqvist A. Is there a specific lamina I spinothalamocortical pathway for pain and temperature sensations in primates? J Pain. (2002) 3:95–101; discussion 113–14. doi: 10.1054/jpai.2002.122953
61. Evrard HC, Logothetis NK, Craig ADB. Modular architectonic organization of the insula in the macaque monkey. J Comp Neurol. (2014) 522:64–97. doi: 10.1002/cne.23436
62. Evrard HC. The organization of the primate insular cortex. Front Neuroanat. (2019) 13:43. doi: 10.3389/fnana.2019.00043
63. Baumgärtner U, Tiede W, Treede R-D, Craig ADB. Laser-evoked potentials are graded and somatotopically organized anteroposteriorly in the operculoinsular cortex of anesthetized monkeys. J Neurophysiol. (2006) 96:2802–8. doi: 10.1152/jn.00512.2006
64. Strigo IA, Craig ADB. Interoception, homeostatic emotions and sympathovagal balance. Philos Trans R Soc Lond B Biol Sci. (2016) 371:20160010. doi: 10.1098/rstb.2016.0010
65. Schneider RJ, Friedman DP, Mishkin M. A modality-specific somatosensory area within the insula of the rhesus monkey. Brain Res. (1993) 621:116–20. doi: 10.1016/0006-8993(93)90305-7
66. Dimov LF, Toniolo EF, Alonso-Matielo H, de Andrade DC, Garcia-Larrea L, Ballester G, et al. Electrical stimulation of the insular cortex as a novel target for the relief of refractory pain: an experimental approach in rodents. Behav Brain Res. (2018) 346:86–95. doi: 10.1016/j.bbr.2017.11.036
67. Alonso-Matielo H, Gonçalves ES, Campos M, Oliveira VRS, Toniolo EF, Alves AS, et al. Electrical stimulation of the posterior insula induces mechanical analgesia in a rodent model of neuropathic pain by modulating GABAergic signaling and activity in the pain circuitry. Brain Res. (2021) 1754:147237. doi: 10.1016/j.brainres.2020.147237
68. Xu Z, Fang J, Xiang X, Sun H, Wang S, Fang J, et al. Electroacupuncture alleviates pain-related emotion by upregulating the expression of NPS and its receptor NPSR in the anterior cingulate cortex and hypothalamus. Evid Based Complement Alternat Med. (2020) 2020:8630368. doi: 10.1155/2020/8630368
69. Ulrich-Lai YM, Xie W, Meij JTA, Dolgas CM, Yu L, Herman JP. Limbic and HPA axis function in an animal model of chronic neuropathic pain. Physiol Behav. (2006) 88:67–76. doi: 10.1016/j.physbeh.2006.03.012
70. Palkovits M. Stress-induced expression of co-localized neuropeptides in hypothalamic and amygdaloid neurons. Eur J Pharmacol. (2000) 405:161–6. doi: 10.1016/s0014-2999(00)00549-5
71. Villemure C, Bushnell MC. Mood influences supraspinal pain processing separately from attention. J Neurosci. (2009) 29:705–15. doi: 10.1523/JNEUROSCI.3822-08.2009
72. Stevens FL, Hurley RA, Taber KH. Anterior cingulate cortex: unique role in cognition and emotion. J Neuropsychiatry Clin Neurosci. (2011) 23:121–5. doi: 10.1176/jnp.23.2.jnp121
73. Ohmatsu S, Nakano H, Tominaga T, Terakawa Y, Murata T, Morioka S. Activation of the serotonergic system by pedaling exercise changes anterior cingulate cortex activity and improves negative emotion. Behav Brain Res. (2014) 270:112–7. doi: 10.1016/j.bbr.2014.04.017
74. Borszcz GS. Contribution of the ventromedial hypothalamus to generation of the affective dimension of pain. Pain. (2006) 123:155–68. doi: 10.1016/j.pain.2006.02.026
75. Condés-Lara M, Martínez-Lorenzana G, Rubio-Beltrán E, Rodríguez-Jiménez J, Rojas-Piloni G, González-Hernández A. Hypothalamic paraventricular nucleus stimulation enhances c-fos expression in spinal and supraspinal structures related to pain modulation. Neurosci Res. (2015) 98:59–63. doi: 10.1016/j.neures.2015.04.004
76. Miranda-Cardenas Y, Rojas-Piloni G, Martínez-Lorenzana G, Rodríguez-Jiménez J, López-Hidalgo M, Freund-Mercier MJ, et al. Oxytocin and electrical stimulation of the paraventricular hypothalamic nucleus produce antinociceptive effects that are reversed by an oxytocin antagonist. Pain. (2006) 122:182–9. doi: 10.1016/j.pain.2006.01.029
77. Rojas-Piloni G, López-Hidalgo M, Martínez-Lorenzana G, Rodríguez-Jiménez J, Condés-Lara M. GABA-mediated oxytocinergic inhibition in dorsal horn neurons by hypothalamic paraventricular nucleus stimulation. Brain Res. (2007) 1137:69–77. doi: 10.1016/j.brainres.2006.12.045
78. Shiraishi T, Onoe M, Kojima T, Sameshima Y, Kageyama T. Effects of hypothalamic paraventricular nucleus: electrical stimulation produce marked analgesia in rats. Neurobiology. (1995) 3:393–403.8696307
79. Besedovsky HO, del Rey A. Immune-neuro-endocrine interactions: facts and hypotheses. Endocr Rev. (1996) 17:64–102. doi: 10.1210/edrv-17-1-64
80. Elmquist JK, Saper CB. Activation of neurons projecting to the paraventricular hypothalamic nucleus by intravenous lipopolysaccharide. J Comp Neurol. (1996) 374:315–31. doi: 10.1002/(SICI)1096-9861(19961021)374:3-/lt;315::AID-CNE1%3E3.0.CO;2-4
81. Gavrilov YV, Perekrest SV, Novikova NS. Intracellular expression of c-fos protein in various structures of the hypothalamus in electrical pain stimulation and administration of antigens. Neurosci Behav Physiol. (2008) 38:87–92. doi: 10.1007/s11055-008-0012-4
82. Bassi GS, Kanashiro A, Rodrigues GJ, Cunha FQ, Coimbra NC, Ulloa L. Brain stimulation differentially modulates nociception and inflammation in aversive and non-aversive behavioral conditions. Neuroscience. (2018) 383:191–204. doi: 10.1016/j.neuroscience.2018.05.008
83. Treede RD, Kenshalo DR, Gracely RH, Jones AK. The cortical representation of pain. Pain. (1999) 79:105–11. doi: 10.1016/s0304-3959(98)00184-5
84. Seifert F, Maihöfner C. Functional and structural imaging of pain-induced neuroplasticity. Curr Opin Anaesthesiol. (2011) 24:515–23. doi: 10.1097/ACO.0b013e32834a1079
85. DosSantos MF, Ferreira N, Toback RL, Carvalho AC, DaSilva AF. Potential mechanisms supporting the value of motor cortex stimulation to treat chronic pain syndromes. Front Neurosci. (2016) 10:18. doi: 10.3389/fnins.2016.00018
86. Zhuo M. Molecular mechanisms of pain in the anterior cingulate cortex. J Neurosci Res. (2006) 84:927–33. doi: 10.1002/jnr.21003
87. Fuchs PN, Peng YB, Boyette-Davis JA, Uhelski ML. The anterior cingulate cortex and pain processing. Front Integr Neurosci. (2014) 8:35. doi: 10.3389/fnint.2014.00035
88. King T, Vera-Portocarrero L, Gutierrez T, Vanderah TW, Dussor G, Lai J, et al. Unmasking the tonic-aversive state in neuropathic pain. Nat Neurosci. (2009) 12:1364–6. doi: 10.1038/nn.2407
89. Johansen JP, Fields HL, Manning BH. The affective component of pain in rodents: direct evidence for a contribution of the anterior cingulate cortex. Proc Natl Acad Sci U S A. (2001) 98:8077–82. doi: 10.1073/pnas.141218998
90. Bliss TVP, Collingridge GL, Kaang B-K, Zhuo M. Synaptic plasticity in the anterior cingulate cortex in acute and chronic pain. Nat Rev Neurosci. (2016) 17:485–96. doi: 10.1038/nrn.2016.68
91. Senapati AK, Huntington PJ, Peng YB. Spinal dorsal horn neuron response to mechanical stimuli is decreased by electrical stimulation of the primary motor cortex. Brain Res. (2005) 1036:173–9. doi: 10.1016/j.brainres.2004.12.043
92. Ma J-H, Xiao T-H, Chang C-W, Gao L, Wang X-L, Gao G-D, et al. Activation of anterior cingulate cortex produces inhibitory effects on noxious mechanical and electrical stimuli-evoked responses in rat spinal WDR neurons. Eur J Pain. (2011) 15:895–9. doi: 10.1016/j.ejpain.2011.04.003
93. Park SI, Oh JH, Hwang YS, Kim SJ, Chang JW. Electrical stimulation of the anterior cingulate cortex in a rat neuropathic pain model. Acta Neurochir Suppl. (2006) 99:65–71. doi: 10.1007/978-3-211-35205-2_13
94. LaBuda CJ, Fuchs PN. Attenuation of negative pain affect produced by unilateral spinal nerve injury in the rat following anterior cingulate cortex activation. Neuroscience. (2005) 136:311–22. doi: 10.1016/j.neuroscience.2005.07.010
95. Gu L, Uhelski ML, Anand S, Romero-Ortega M, Kim Y, Fuchs PN, et al. Pain inhibition by optogenetic activation of specific anterior cingulate cortical neurons. PLoS One. (2015) 10:e0117746. doi: 10.1371/journal.pone.0117746
96. Elina KC, Moon HC, Islam J, Kim HK, Park YS. The effect of optogenetic inhibition of the anterior cingulate cortex in neuropathic pain following sciatic nerve injury. J Mol Neurosci. (2021) 71:638–50. doi: 10.1007/s12031-020-01685-7
97. LaGraize SC, Borzan J, Peng YB, Fuchs PN. Selective regulation of pain affect following activation of the opioid anterior cingulate cortex system. Exp Neurol. (2006) 197:22–30. doi: 10.1016/j.expneurol.2005.05.008
98. Liu Y, Xu H, Sun G, Vemulapalli B, Jee HJ, Zhang Q, et al. Frequency dependent electrical stimulation of PFC and ACC for acute pain treatment in rats. Front Pain Res. (2021) 2:728045. doi: 10.3389/fpain.2021.728045
99. Ren D, Li J-N, Qiu X-T, Wan F-P, Wu Z-Y, Fan B-Y, et al. Anterior cingulate cortex mediates hyperalgesia and anxiety induced by chronic pancreatitis in rats. Neurosci Bull. (2022) 38:342–58. doi: 10.1007/s12264-021-00800-x
100. Kumar K, Wyant GM, Nath R. Deep brain stimulation for control of intractable pain in humans, present and future: a ten-year follow-up. Neurosurgery. (1990) 26:774–81; discussion 781–82. doi: 10.1097/00006123-199005000-00007
101. Adams JE, Hosobuchi Y, Fields HL. Stimulation of internal capsule for relief of chronic pain. J Neurosurg. (1974) 41:740–4. doi: 10.3171/jns.1974.41.6.0740
102. Schvarcz JR. Chronic stimulation of the septal area for the relief of intractable pain. Appl Neurophysiol. (1985) 48:191–4. doi: 10.1159/000101126
103. Schvarcz JR. Long-term results of stimulation of the septal area for relief of neurogenic pain. Acta Neurochir Suppl. (1993) 58:154–5. doi: 10.1007/978-3-7091-9297-9_35
104. Andy OJ. Parafascicular-center median nuclei stimulation for intractable pain and dyskinesia (painful-dyskinesia). Appl Neurophysiol. (1980) 43:133–44. doi: 10.1159/000102247
105. Schvarcz JR. Chronic self-stimulation of the medial posterior inferior thalamus for the alleviation of deafferentation pain. Acta Neurochir Suppl. (1980) 30:295–301. doi: 10.1007/978-3-7091-8592-6_36
106. Thoden U, Doerr M, Dieckmann G, Krainick JU. Medial thalamic permanent electrodes for pain control in man: an electrophysiological and clinical study. Electroencephalogr Clin Neurophysiol. (1979) 47:582–91. doi: 10.1016/0013-4694(79)90259-1
107. Bittar RG, Burn SC, Bain PG, Owen SL, Joint C, Shlugman D, et al. Deep brain stimulation for movement disorders and pain. J Clin Neurosci. (2005) 12:457–63. doi: 10.1016/j.jocn.2004.09.001
108. Rezai A, Lozano A. Deep brain stimulation for chronic pain. In: Burchiel KJ, editors. Surgical management of pain. New York: Thieme Medical Piblishers, Inc. (2002). p. 565–74.
109. Wallace BA, Ashkan K, Benabid A-L. Deep brain stimulation for the treatment of chronic, intractable pain. Neurosurg Clin N Am. (2004) 15:343–57. doi: 10.1016/j.nec.2004.03.004
110. Hamani C. Deep brain stimulation for pain: indications and technique. In: Freitas T, Assumpcao de Monaco B, Golovac S, editors. Neuromodulation techniques for pain treatment. Cham: Springer (2022). p. 73–80.
112. Schaltenbrand G, Bailey P. Introduction to stereotaxis with an atlas of the human brain. Stuttgart: Thieme (1959).
113. Hassler R. Architectonic organization of the thalamic nuclei. In: Walker AE, editor. Stereotaxy of the human brain. Anatomical, physiological and clinical application. Georg Thieme Verlag (1982). p. 140–80.
114. Hamani C, Dostrovsky JO, Lozano AM. The motor thalamus in neurosurgery. Neurosurgery. (2006) 58:146–58; discussion 146–58. doi: 10.1227/01.neu.0000192166.62017.c1
115. Davis KD, Kiss ZH, Tasker RR, Dostrovsky JO. Thalamic stimulation-evoked sensations in chronic pain patients and in nonpain (movement disorder) patients. J Neurophysiol. (1996) 75:1026–37. doi: 10.1152/jn.1996.75.3.1026
116. Lenz FA, Dostrovsky JO, Tasker RR, Yamashiro K, Kwan HC, Murphy JT. Single-unit analysis of the human ventral thalamic nuclear group: somatosensory responses. J Neurophysiol. (1988) 59:299–316. doi: 10.1152/jn.1988.59.2.299
117. Lenz FA, Kwan HC, Martin R, Tasker R, Richardson RT, Dostrovsky JO. Characteristics of somatotopic organization and spontaneous neuronal activity in the region of the thalamic principal sensory nucleus in patients with spinal cord transection. J Neurophysiol. (1994) 72:1570–87. doi: 10.1152/jn.1994.72.4.1570
118. Tasker RR. Microelectrode findings in the thalamus in chronic pain and other conditions. Stereotact Funct Neurosurg. (2001) 77:166–8. doi: 10.1159/000064605
119. Hamani C, Schwalb JM, Rezai AR, Dostrovsky JO, Davis KD, Lozano AM. Deep brain stimulation for chronic neuropathic pain: long-term outcome and the incidence of insertional effect. Pain. (2006) 125:188–96. doi: 10.1016/j.pain.2006.05.019
120. Kumar K, Toth C, Nath RK. Deep brain stimulation for intractable pain: a 15-year experience. Neurosurgery. (1997) 40:736–46; discussion 746–47. doi: 10.1097/00006123-199704000-00015
121. Mazars GJ. Intermittent stimulation of nucleus ventralis posterolateralis for intractable pain. Surg Neurol. (1975) 4:93–5.1080908
122. Hosobuchi Y, Adams JE, Rutkin B. Chronic thalamic stimulation for the control of facial anesthesia dolorosa. Arch Neurol. (1973) 29:158–61. doi: 10.1001/archneur.1973.00490270040005
123. Richardson DE, Akil H. Long term results of periventricular gray self-stimulation. Neurosurgery. (1977) 1:199–202. doi: 10.1097/00006123-197709000-00018
124. Dieckmann G, Witzmann A. Initial and long-term results of deep brain stimulation for chronic intractable pain. Appl Neurophysiol. (1982) 45:167–72. doi: 10.1159/000101593
125. Gybels J, Kupers R. Central and peripheral electrical stimulation of the nervous system in the treatment of chronic pain. Acta Neurochir Suppl. (1987) 38:64–75. doi: 10.1007/978-3-7091-6975-9_10
126. Hosobuchi Y. Subcortical electrical stimulation for control of intractable pain in humans. Report of 122 cases (1970-1984). J Neurosurg. (1986) 64:543–53. doi: 10.3171/jns.1986.64.4.0543
127. Tasker RR, Vilela Filho O. Deep brain stimulation for neuropathic pain. Stereotact Funct Neurosurg. (1995) 65:122–4. doi: 10.1159/000098682
128. Turnbull IM, Shulman R, Woodhurst WB. Thalamic stimulation for neuropathic pain. J Neurosurg. (1980) 52:486–93. doi: 10.3171/jns.1980.52.4.0486
129. Bittar RG, Kar-Purkayastha I, Owen SL, Bear RE, Green A, Wang S, et al. Deep brain stimulation for pain relief: a meta-analysis. J Clin Neurosci. (2005) 12:515–9. doi: 10.1016/j.jocn.2004.10.005
130. Levy RM. Deep brain stimulation for the treatment of intractable pain. Neurosurg Clin N Am. (2003) 14:389–99. doi: 10.1016/s1042-3680(03)00036-6
131. Nandi D, Aziz TZ. Deep brain stimulation in the management of neuropathic pain and multiple sclerosis tremor. J Clin Neurophysiol. (2004) 21:31–9. doi: 10.1097/00004691-200401000-00005
132. Owen SLF, Green AL, Nandi DD, Bittar RG, Wang S, Aziz TZ. Deep brain stimulation for neuropathic pain. Acta Neurochir Suppl. (2007) 97:111–6. doi: 10.1007/978-3-211-33081-4_13
133. Siegfried J. Monopolar electrical stimulation of nucleus ventroposteromedialis thalami for postherpetic facial pain. Appl Neurophysiol. (1982) 45:179–84. doi: 10.1159/000101595
134. Owen SLF, Green AL, Stein JF, Aziz TZ. Deep brain stimulation for the alleviation of post-stroke neuropathic pain. Pain. (2006) 120:202–6. doi: 10.1016/j.pain.2005.09.035
135. Rasche D, Rinaldi PC, Young RF, Tronnier VM. Deep brain stimulation for the treatment of various chronic pain syndromes. Neurosurg Focus. (2006) 21:E8. doi: 10.3171/foc.2006.21.6.10
136. Nandi D, Smith H, Owen S, Joint C, Stein J, Aziz T. Peri-ventricular grey stimulation versus motor cortex stimulation for post stroke neuropathic pain. J Clin Neurosci. (2002) 9:557–61. doi: 10.1054/jocn.2001.1042
137. Frizon LA, Yamamoto EA, Nagel SJ, Simonson MT, Hogue O, Machado AG. Deep brain stimulation for pain in the modern era: a systematic review. Neurosurgery. (2020) 86:191–202. doi: 10.1093/neuros/nyy552
138. Tsubokawa T, Yamamoto T, Katayama Y, Hirayama T, Sibuya H. Thalamic relay nucleus stimulation for relief of intractable pain. Clinical results and beta-endorphin immunoreactivity in the cerebrospinal fluid. Pain. (1984) 18:115–26. doi: 10.1016/0304-3959(84)90879-0
139. Coffey RJ. Deep brain stimulation for chronic pain: results of two multicenter trials and a structured review. Pain Med. (2001) 2:183–92. doi: 10.1046/j.1526-4637.2001.01029.x
140. Hamani C, Ewerton FIS, Bonilha SM, Ballester G, Mello LEAM, Lozano AM. Bilateral anterior thalamic nucleus lesions and high-frequency stimulation are protective against pilocarpine-induced seizures and status epilepticus. Neurosurgery. (2004) 54:191–5; discussion 195–97. doi: 10.1227/01.neu.0000097552.31763.ae
141. Hamani C, Lozano AM. Hardware-related complications of deep brain stimulation: a review of the published literature. Stereotact Funct Neurosurg. (2006) 84:248–51. doi: 10.1159/000096499
142. Huang Y, Cheeran B, Green AL, Denison TJ, Aziz TZ. Applying a sensing-enabled system for ensuring safe anterior cingulate deep brain stimulation for pain. Brain Sci. (2019) 9:E150. doi: 10.3390/brainsci9070150
143. Kucyi A, Davis KD. The dynamic pain connectome. Trends Neurosci. (2015) 38:86–95. doi: 10.1016/j.tins.2014.11.006
144. Eccleston C, Crombez G. Pain demands attention: a cognitive-affective model of the interruptive function of pain. Psychol Bull. (1999) 125:356–66. doi: 10.1037/0033-2909.125.3.356
145. Tracey I. Neuroimaging mechanisms in pain: from discovery to translation. Pain. (2017) 158(Suppl 1):S115–22. doi: 10.1097/j.pain.0000000000000863
146. Wiech K, Ploner M, Tracey I. Neurocognitive aspects of pain perception. Trends Cogn Sci. (2008) 12:306–13. doi: 10.1016/j.tics.2008.05.005
147. Chang C, Glover GH. Time-frequency dynamics of resting-state brain connectivity measured with fMRI. Neuroimage. (2010) 50:81–98. doi: 10.1016/j.neuroimage.2009.12.011
148. Allen EA, Damaraju E, Plis SM, Erhardt EB, Eichele T, Calhoun VD. Tracking whole-brain connectivity dynamics in the resting state. Cereb Cortex. (2014) 24:663–76. doi: 10.1093/cercor/bhs352
149. Deco G, Jirsa VK, McIntosh AR. Resting brains never rest: computational insights into potential cognitive architectures: (trends in neurosciences 36, 268-274, 2013). Trends Neurosci. (2018) 41:161. doi: 10.1016/j.tins.2017.12.007
150. Handwerker DA, Roopchansingh V, Gonzalez-Castillo J, Bandettini PA. Periodic changes in fMRI connectivity. Neuroimage. (2012) 63:1712–9. doi: 10.1016/j.neuroimage.2012.06.078
151. Kucyi A, Hodaie M, Davis KD. Lateralization in intrinsic functional connectivity of the temporoparietal junction with salience- and attention-related brain networks. J Neurophysiol. (2012) 108:3382–92. doi: 10.1152/jn.00674.2012
152. Seeley WW, Allman JM, Carlin DA, Crawford RK, Macedo MN, Greicius MD, et al. Divergent social functioning in behavioral variant frontotemporal dementia and Alzheimer disease: reciprocal networks and neuronal evolution. Alzheimer Dis Assoc Disord. (2007) 21:S50–57. doi: 10.1097/WAD.0b013e31815c0f14
153. Andrews-Hanna JR, Smallwood J, Spreng RN. The default network and self-generated thought: component processes, dynamic control, and clinical relevance. Ann N Y Acad Sci. (2014) 1316:29–52. doi: 10.1111/nyas.12360
154. Petrovic P, Petersson KM, Ghatan PH, Stone-Elander S, Ingvar M. Pain-related cerebral activation is altered by a distracting cognitive task. Pain. (2000) 85:19–30. doi: 10.1016/s0304-3959(99)00232-8
155. Valet M, Sprenger T, Boecker H, Willoch F, Rummeny E, Conrad B, et al. Distraction modulates connectivity of the cingulo-frontal cortex and the midbrain during pain–an fMRI analysis. Pain. (2004) 109:399–408. doi: 10.1016/j.pain.2004.02.033
156. Dostrovsky JO. Role of thalamus in pain. Prog Brain Res. (2000) 129:245–57. doi: 10.1016/S0079-6123(00)29018-3
157. Basbaum AI, Fields HL. Endogenous pain control systems: brainstem spinal pathways and endorphin circuitry. Annu Rev Neurosci. (1984) 7:309–38. doi: 10.1146/annurev.ne.07.030184.001521
158. McIntyre CC, Grill WM, Sherman DL, Thakor NV. Cellular effects of deep brain stimulation: model-based analysis of activation and inhibition. J Neurophysiol. (2004) 91:1457–69. doi: 10.1152/jn.00989.2003
159. Hamani C, Nobrega JN. Preclinical studies modeling deep brain stimulation for depression. Biol Psychiatry. (2012) 72:916–23. doi: 10.1016/j.biopsych.2012.05.024
160. Reznikov R, Binko M, Nobrega JN, Hamani C. Deep brain stimulation in animal models of fear, anxiety, and posttraumatic stress disorder. Neuropsychopharmacology. (2016) 41:2810–7. doi: 10.1038/npp.2016.34
161. Mithani K, Davison B, Meng Y, Lipsman N. The anterior limb of the internal capsule: anatomy, function, and dysfunction. Behav Brain Res. (2020) 387:112588. doi: 10.1016/j.bbr.2020.112588
162. Chowdhury F, Haque M, Sarkar M, Ara S, Islam M. White fiber dissection of brain; the internal capsule: a cadaveric study. Turk Neurosurg. (2010) 20:314–22. doi: 10.5137/1019-5149.JTN.3052-10.2
163. Axer H, Lippitz BE, von Keyserlingk DG. Morphological asymmetry in anterior limb of human internal capsule revealed by confocal laser and polarized light microscopy. Psychiatry Res. (1999) 91:141–54. doi: 10.1016/s0925-4927(99)00029-3
164. Bergfeld IO, Mantione M, Hoogendoorn MLC, Ruhé HG, Notten P, van Laarhoven J, et al. Deep brain stimulation of the ventral anterior limb of the internal capsule for treatment-resistant depression: a randomized clinical trial. JAMA Psychiatry. (2016) 73:456–64. doi: 10.1001/jamapsychiatry.2016.0152
165. Dougherty DD, Rezai AR, Carpenter LL, Howland RH, Bhati MT, O’Reardon JP, et al. A randomized sham-controlled trial of deep brain stimulation of the ventral capsule/ventral Striatum for chronic treatment-resistant depression. Biol Psychiatry. (2015) 78:240–8. doi: 10.1016/j.biopsych.2014.11.023
166. Nuttin B, Cosyns P, Demeulemeester H, Gybels J, Meyerson B. Electrical stimulation in anterior limbs of internal capsules in patients with obsessive-compulsive disorder. Lancet. (1999) 354:1526. doi: 10.1016/S0140-6736(99)02376-4
167. Barthas F, Sellmeijer J, Hugel S, Waltisperger E, Barrot M, Yalcin I. The anterior cingulate cortex is a critical hub for pain-induced depression. Biol Psychiatry. (2015) 77:236–45. doi: 10.1016/j.biopsych.2014.08.004
168. Corder G, Castro DC, Bruchas MR, Scherrer G. Endogenous and exogenous opioids in pain. Annu Rev Neurosci. (2018) 41:453–73. doi: 10.1146/annurev-neuro-080317-061522
169. Kimmey BA, McCall NM, Wooldridge LM, Satterthwaite TD, Corder G. Engaging endogenous opioid circuits in pain affective processes. J Neurosci Res. (2022) 100:66–98. doi: 10.1002/jnr.24762
170. Gao Y-J, Ren W-H, Zhang Y-Q, Zhao Z-Q. Contributions of the anterior cingulate cortex and amygdala to pain- and fear-conditioned place avoidance in rats. Pain. (2004) 110:343–53. doi: 10.1016/j.pain.2004.04.030
171. Silverman AJ, Hoffman DL, Zimmerman EA. The descending afferent connections of the paraventricular nucleus of the hypothalamus (PVN). Brain Res Bull. (1981) 6:47–61. doi: 10.1016/s0361-9230(81)80068-8
172. Condés-Lara M, Rojas-Piloni G, Martínez-Lorenzana G, Rodríguez-Jiménez J, López Hidalgo M, Freund-Mercier MJ. Paraventricular hypothalamic influences on spinal nociceptive processing. Brain Res. (2006) 1081:126–37. doi: 10.1016/j.brainres.2006.01.050
173. Rojas-Piloni G, Rodríguez-Jiménez J, Martínez-Lorenzana G, Condés-Lara M. Dorsal horn antinociception mediated by the paraventricular hypothalamic nucleus and locus coeruleous: a comparative study. Brain Res. (2012) 1461:41–50. doi: 10.1016/j.brainres.2012.04.042
174. Condés-Lara M, Rojas-Piloni G, Martínez-Lorenzana G, Diez-Martínez DC, Rodríguez-Jiménez J. Functional interactions between the paraventricular hypothalamic nucleus and raphe magnus. A comparative study of an integrated homeostatic analgesic mechanism. Neuroscience. (2012) 209:196–207. doi: 10.1016/j.neuroscience.2012.02.032
175. Florence G, Sameshima K, Fonoff ET, Hamani C. Deep brain stimulation: more complex than the inhibition of cells and excitation of fibers. Neuroscientist. (2016) 22:332–45. doi: 10.1177/1073858415591964
176. Bambico FR, Bregman T, Diwan M, Li J, Darvish-Ghane S, Li Z, et al. Neuroplasticity-dependent and -independent mechanisms of chronic deep brain stimulation in stressed rats. Transl Psychiatry. (2015) 5:e674. doi: 10.1038/tp.2015.166
177. Coffey RJ, Lozano AM. Neurostimulation for chronic noncancer pain: an evaluation of the clinical evidence and recommendations for future trial designs. J Neurosurg. (2006) 105:175–89. doi: 10.3171/jns.2006.105.2.175
178. Hosobuchi Y, Adams JE, Linchitz R. Pain relief by electrical stimulation of the central gray matter in humans and its reversal by naloxone. Science. (1977) 197:183–6. doi: 10.1126/science.301658
179. Young RF, Chambi VI. Pain relief by electrical stimulation of the periaqueductal and periventricular gray matter. Evidence for a non-opioid mechanism. J Neurosurg. (1987) 66:364–71. doi: 10.3171/jns.1987.66.3.0364
180. Dellemijn P. Are opioids effective in relieving neuropathic pain? Pain. (1999) 80:453–62. doi: 10.1016/S0304-3959(98)00256-5
181. Hamani C, Nóbrega JN. Deep brain stimulation in clinical trials and animal models of depression. Eur J Neurosci. (2010) 32:1109–17. doi: 10.1111/j.1460-9568.2010.07414.x
182. Hamani C, Fonoff ET, Parravano DC, Silva VA, Galhardoni R, Monaco BA, et al. Motor cortex stimulation for chronic neuropathic pain: results of a double-blind randomized study. Brain. (2021) 144:2994–3004. doi: 10.1093/brain/awab189
183. Nandi D, Aziz T, Carter H, Stein J. Thalamic field potentials in chronic central pain treated by periventricular gray stimulation – a series of eight cases. Pain. (2003) 101:97–107. doi: 10.1016/s0304-3959(02)00277-4
184. Nandi D, Liu X, Joint C, Stein J, Aziz T. Thalamic field potentials during deep brain stimulation of periventricular gray in chronic pain. Pain. (2002) 97:47–51. doi: 10.1016/s0304-3959(01)00486-9
185. Hamani C, Giacobbe P, Diwan M, Balbino ES, Tong J, Bridgman A, et al. Monoamine oxidase inhibitors potentiate the effects of deep brain stimulation. Am J Psychiatry. (2012) 169:1320–1. doi: 10.1176/appi.ajp.2012.12060754
Keywords: deep brain stimulation, pain, animal models, clinical trials, thalamus, periaqueductal grey matter
Citation: Pagano RL, Dale CS, Campos ACP and Hamani C (2023) Translational aspects of deep brain stimulation for chronic pain. Front. Pain Res. 3:1084701. doi: 10.3389/fpain.2022.1084701
Received: 30 October 2022; Accepted: 22 December 2022;
Published: 11 January 2023.
Edited by:
Nasser Khaled Yaghi, Oregon Health and Science University, United StatesReviewed by:
Prasad Shirvalkar, University of California San Francisco, United States© 2023 Pagano, Dale, Campos and Hamani. This is an open-access article distributed under the terms of the Creative Commons Attribution License (CC BY). The use, distribution or reproduction in other forums is permitted, provided the original author(s) and the copyright owner(s) are credited and that the original publication in this journal is cited, in accordance with accepted academic practice. No use, distribution or reproduction is permitted which does not comply with these terms.
*Correspondence: Clement Hamani Y2xlbWVudC5oYW1hbmlAc3Vubnlicm9vay5jYQ==
†These authors have contributed equally to this work
Specialty Section: This article was submitted to Neuromodulatory Interventions, a section of the journal Frontiers in Pain Research
Disclaimer: All claims expressed in this article are solely those of the authors and do not necessarily represent those of their affiliated organizations, or those of the publisher, the editors and the reviewers. Any product that may be evaluated in this article or claim that may be made by its manufacturer is not guaranteed or endorsed by the publisher.
Research integrity at Frontiers
Learn more about the work of our research integrity team to safeguard the quality of each article we publish.