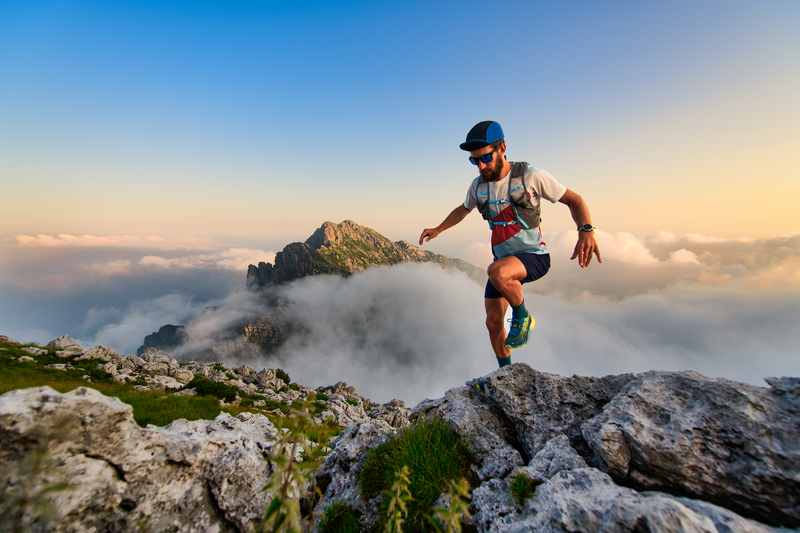
95% of researchers rate our articles as excellent or good
Learn more about the work of our research integrity team to safeguard the quality of each article we publish.
Find out more
REVIEW article
Front. Pain Res. , 17 October 2022
Sec. Pain Mechanisms
Volume 3 - 2022 | https://doi.org/10.3389/fpain.2022.1013577
This article is part of the Research Topic Insights in Pain Mechanisms: 2022 View all 6 articles
Rheumatic diseases, such as osteoarthritis and rheumatoid arthritis, affect over 750 million people worldwide and contribute to approximately 40% of chronic pain cases. Inflammation and tissue damage contribute to pain in rheumatic diseases, but pain often persists even when inflammation/damage is resolved. Mechanisms that cause this persistent pain are still unclear. Mitochondria are essential for a myriad of cellular processes and regulate neuronal functions. Mitochondrial dysfunction has been implicated in multiple neurological disorders, but its role in sensory processing and pain in rheumatic diseases is relatively unexplored. This review provides a comprehensive understanding of how mitochondrial dysfunction connects inflammation and damage-associated pathways to neuronal sensitization and persistent pain. To provide an overall framework on how mitochondria control pain, we explored recent evidence in inflammatory and neuropathic pain conditions. Mitochondria have intrinsic quality control mechanisms to prevent functional deficits and cellular damage. We will discuss the link between neuronal activity, mitochondrial dysfunction and chronic pain. Lastly, pharmacological strategies aimed at reestablishing mitochondrial functions or boosting mitochondrial dynamics as therapeutic interventions for chronic pain are discussed. The evidence presented in this review shows that mitochondria dysfunction may play a role in rheumatic pain. The dysfunction is not restricted to neuronal cells in the peripheral and central nervous system, but also includes blood cells and cells at the joint level that may affect pain pathways indirectly. Pre-clinical and clinical data suggest that modulation of mitochondrial functions can be used to attenuate or eliminate pain, which could be beneficial for multiple rheumatic diseases.
Rheumatic diseases are often grouped under the term “arthritis”, which is used to describe over 100 diseases that include rheumatoid arthritis (RA), osteoarthritis (OA), fibromyalgia, systemic lupus erythematosus (SLE), ankylosing spondylitis (AS), psoriatic arthritis (PsA), and juvenile idiopathic arthritis (JIA). These rheumatic diseases are characterized by inflammation and tissue damage (1). In several of these rheumatic pathologies, e.g., RA and SLE, an autoimmune component is present (2). In most rheumatic diseases, joints, cartilage, tendons, ligaments, bones, and muscles are the main and most commonly affected tissues. Although specific mechanisms differ between diseases, the release of pro-inflammatory molecules or damage-associated molecules by cells, contributing to tissue inflammation and destruction, is a common feature among these rheumatic conditions. For example, in RA, fibroblast-like synoviocytes (FLSs) release pro-inflammatory molecules, leading to tissue damage. In OA, chondrocyte cell death triggers the release of inflammatory cytokines in surrounding tissues (3). Another shared element among rheumatic patients is that they often report pain as their most debilitating symptom (4–7). Fibromyalgia has an unknown pathophysiology, but is characterized by chronic widespread pain, which is also present in 65%–80% of SLE patients (8).
Although inflammatory components are present in rheumatic diseases, it is still not completely understood what drives pain in these diseases. The magnitude of inflammation in RA or SLE, or the severity of damage assessed by radiographic knee damage in OA, do not correlate with pain intensity (8–13). Moreover, 12%–70% of RA patients have persistent pain after remission or under minimal disease activity (13–15). In OA, 10%–40% of the patients still have pain even 5 years after total knee replacement surgery (11). In summary, pain is not directly associated with the magnitude of damage/inflammation and often persists even when the inflammation or damage is minimal or resolved.
Chronic pain affects at least 20% of the world population (∼1.4 billion people), with rheumatic diseases, such as OA and RA, contributing to approximately 40% of these chronic pain cases (1, 16–19). Currently available pain treatments include analgesics [e.g., paracetamol, non-steroidal anti-inflammatory drugs (NSAIDs), opioids, and steroids], physiotherapy or surgery (20). The available treatments are often not very effective to treat chronic pain (21, 22). NSAIDs and steroids are the most common treatments for rheumatic diseases, due to their anti-inflammatory and analgesic properties. Nonetheless, renal, hepatic, cardiovascular and gastrointestinal adverse effects are commonly reported (23, 24). Most importantly, despite their anti-inflammatory properties, the highest pain reduction reported was lower than 10% in average, further supporting a lack of correlation between inflammation and pain intensity in rheumatic disease (24). Additionally, preclinical evidence supports clinical observations, as the anti-inflammatory corticosteroid (dexamethasone) prevents acute inflammatory pain, but no longer has an effect when pain becomes chronic (25). Finally, opioids, the last resource in terms of analgesics, reduce pain intensity 20%–30% in OA patients and only 10% in musculoskeletal pain in general (22). The limited therapeutic outcome of opioids comes with potential side effects, including risk for addiction, which significantly contributes to the current opioid crisis (26, 27).
Most studies on pain in rheumatic disease focus on cellular and molecular alterations in joint tissues induced by inflammation. It is well known that inflammatory mediators can directly activate or sensitize sensory neurons (28–30). Yet, given that pain often persists with minimal or no joint inflammation/damage, it is likely that changes in the peripheral and central nervous system also drive pain in rheumatic disease. Indeed, inflammation and tissue damage may determine long lasting neuronal plasticity (28–30). Joint inflammation alters protein expression in sensory neurons that innervate the joints and have their cell bodies in the dorsal root ganglia (DRG) (31). In patients with mild and severe OA, sensory innervation is increased in the subchondral bone (32), potentially enhancing pain signaling pathways. Primary sensory neurons synapse in the dorsal horn in the spinal cord. In rheumatic diseases, exacerbated sensory neuron activity or neuronal damage may promote the activation of astrocytes and microglia in these spinal regions (31). Increased activation of glia may explain why the levels of pro-inflammatory cytokine interleukine-1β (IL-1β) are increased in the cerebrospinal fluid of RA patients (33). Overall, the magnitude of rheumatic pain likely depends on the interplay between joint damage and pain signaling pathways at various levels including DRG, spinal cord and brain.
Mitochondria are essential for a myriad of cellular processes and play a key role in regulating inflammatory responses (34), but also neuronal functions. Neurons have a higher energetic demand in comparison to other cell types (35). Mitochondria are a main source of adenosine triphosphate (ATP) in neurons. This ATP is essential to maintain the membrane potential and restore it after an action potential (36). Mitochondria are Ca2+ reservoirs, regulating intracellular Ca2+ concentration (37, 38). Moreover, they are a source of reactive oxygen species (ROS). Mitochondria control the release of neurotransmitters, neuronal excitability, signaling and plasticity (39). The role of mitochondria in neuronal activity has been extensively explored in the context of neurodegenerative diseases. For example, changes in mitochondrial axonal transport or the removal of damaged mitochondria in neurons contribute to Alzheimer's disease (40) and Parkinson's disease, respectively (41). Furthermore, intercellular transfer of mitochondria from astrocytes or macrophages to neurons promotes neuronal survival after stroke, or resolves inflammatory pain, respectively (25, 42). However, the role of mitochondria in the regulation of pain is only begun to be understood. Importantly, modulating mitochondrial functions in sensory neurons reduces hyperalgesia in pre-clinical models of neuropathic and inflammatory pain (43–48). Genetic disruption of complex IV of the mitochondrial electron transport chain in primary sensory neurons causes pain hypersensitivity (49). Moreover, 70% of humans with inherited mitochondrial deficits develop chronic pain (50). Thus these data suggest an association between mitochondrial dysfunction, neuronal activity, and chronic pain.
The role of mitochondria in rheumatic diseases in general has been discussed in other reviews (51–55). However, their role in the development of rheumatic pain has not been well covered. In this review, we will focus on the contribution of mitochondria to pain in rheumatic diseases. In the following sections, we will discuss how mitochondria affect sensory processing and pain development, using inflammatory and neuropathic pain models. Furthermore, we discuss to what extent modulating mitochondrial functions may be promising to treat chronic rheumatic pain.
Inflammatory pain is caused by tissue damage and inflammatory responses, while neuropathic pain is usually described as a consequence of neuronal damage in the peripheral or central nervous system (16, 56, 57). Pain in rheumatic diseases is often considered as inflammatory, but nerve damage may also contribute to pain. Vice versa, neuropathic pain shares features of inflammatory pain. Inflammation can trigger neuronal damage and consequently neuropathic pain. Therefore, the discrimination between these two types of pain can be sometimes difficult (16, 56). As example, inflammatory mediators sensitize afferent nociceptive nerve fibers and trigger damage of sympathetic nerve fibers in the joints of rodents in experimental models of inflammatory arthritic pain (58). In addition, several OA patients show signs of neuropathic pain (9). Therefore, we will discuss findings in common inflammatory pain models [e.g., induced by carrageenan or Complete Freund's Adjuvant (CFA)], but also discuss the role of mitochondria in neuropathic pain (e.g., induced by nerve ligation or chemotherapeutic drugs), as it may inform us on what happens in rheumatic diseases when nerves are damaged. These models are very relevant and their significance in the pain field is discussed elsewhere (59, 60).
Mitochondria are presumably developed from engulfed prokaryotes that were once independent organisms (61). Mitochondria are complex organelles that have their own mitochondrial deoxyribonucleic acid (mtDNA) and a very characteristic morphology. Each mitochondrion is formed by a double membrane. The outer membrane has a composition similar to the plasma membrane of an eukaryotic cell. The inner membrane is organized in several cristae to maximize efficient ATP production during oxidative phosphorylation (OxPhos) (53, 62). The main mitochondrial functions and associated pathways are displayed in Figure 1. Each of these functions will be discussed in the context of rheumatic pain. A detailed overview of all specific alterations in mitochondrial functions reported in pre-clinical studies and in humans with rheumatic diseases can be found in Tables 1, 2, respectively.
Figure 1. Overview of mitochondrial functions. Mitochondria are pleiotropic organelles with multiple functions. The most relevant ones are depicted in the figure. Mitochondria produce ATP via oxidative phosphorylation (OxPhos), which involves the interaction between the mitochondrial electron transport chain (mETC) and enzymes from the tricarboxylic acid (TCA) cycle. The mETC is the main source of mtROS, which are eliminated by mitochondrial antioxidant enzymes like superoxide dismutase (SOD), peroxidase or glutathione. Ca2+ enters the mitochondria through the mitochondrial Ca2+ uniporter (MCU) and is released through the Na+/Ca2+ exchanger (NCLX), or in case of Ca2+ overload in the mitochondrial matrix through assembly of the mitochondrial permeability transition pore (mPTP). Increased mtROS production or mitochondrial Ca2+ concentration can trigger nucleotide-binding oligomerization domain-like receptor pyrin domain containing 3 (NLRP3) inflammasome activation and/or binding of Parkin and PTEN-induced kinase 1 (PINK1) to mitochondria, which leads to the elimination of damaged mitochondria via mitophagy. Quality control mechanisms, such as mitophagy, fusion, fission and biogenesis ensure a healthy and functional mitochondria pool. Optic atrophy 1 (OPA1) and mitofusins1 and 2 (MFN1 or MFN2) allow mitochondria to merge (fusion), while dynamin-related protein (DRP1) permits mitochondria to segment (fission). New mitochondria are produced through biogenesis, which is promoted by peroxisome proliferator-activated receptor gamma coactivator 1-alpha (PGC-1α). Decreased energy production detected by energy sensors sirtuin1 (SIRT1) and adenosine monophosphate (AMP)-activated protein kinase (AMPK) will activate PGC-1α. Quality control mechanisms are highly dynamic and adapt to the cells' needs. Mitochondria are transported to the area where they are required. In neurons, mitochondria travel from the soma to the axons and damaged mitochondria back to the soma. Anterograde transport of mitochondria is mediated by mitochondrial Rho GTPases (MIROs) and kinesins, whilst retrograde transport is mediated by MIROs and dynein-dynactin complexes. Syntaphilin is an anchor protein that stabilizes mitochondria in a fixed spot. Histone deacetylase 6 (HDAC6) indirectly reduces mitochondrial transport by deacetylation of the cytoskeletal α-tubulin, a protein that facilitates dynein-dependent mitochondrial transport when acetylated. Figure created with BioRender.com.
Table 1. Detailed overview of pre-clinical findings showing mitochondrial dysfunction in inflammatory and neuropathic pain models.
The five mitochondrial respiratory chain complexes (complex I–V), known as the mitochondrial electron transport chain (mETC), are located in the inner membrane. These series of complexes transfer electrons from nicotinamide adenine dinucleotide (NADH) or flavin adenine dinucleotide (FADH), formed in the tricarboxylic acid cycle (TCA) in the mitochondrial matrix, to oxygen. This electron transfer is needed to create a transmembrane electrochemical gradient by pumping protons across the membrane. The flow of protons back into the matrix through complex V allows ATP production (45, 122, 123). OxPhos is responsible for 90% of the ATP consumed by neurons. Its importance is highlighted by deficits in OxPhos, which reduces dendritic synaptic plasticity in neurons, leading to neuronal injury or even cell death (39, 124). Exposure of neurons to inflammatory cytokines, such as TNF and/or IFNγ, depolarizes the mitochondrial membrane potential, impairs OxPhos and ATP production (125, 126). Conversely, exposure of sensory neurons to IL-17, IL-1α or IL-1β increased mitochondrial respiration to support neurite outgrowth (127, 128). Thus, inflammation affects mitochondrial respiration and neuronal function directly. Importantly, interfering with mitochondrial respiration in neurons affects sensory processing and pain. For example, intrathecal administration of complex I or III inhibitors induced mechanical allodynia in naïve mice (129). Furthermore, complex I deficiency in humans is associated with muscle pain (111). In addition, a deficit in mitochondrial respiration may also indirectly contribute to pain development. Genetic disruption of mETC complex IV activity in mouse DRG neurons increased the adenosine diphosphate (ADP)/ATP ratio due to impaired ATP production. The relative increase in ADP induced mechanical and thermal hypersensitivity through activation of purinergic receptor P2Y1 expressed on sensory neurons afferents (49).
The oxygen consumption rate (OCR), a measure of mitochondrial respiration, is reduced in lumbar DRG neurons at the peak of transient carrageenan-induced inflammatory pain, but is increased again when pain had resolved (25). In CFA-induced persistent inflammatory pain, mass spectrometry analysis identified that several proteins involved in mETC are reduced, suggesting that mitochondrial respiration in the DRG is affected in persistent inflammatory pain (66). In the lumbar spinal cord, OxPhos is reduced in rats with CFA-induced pain, or in mice after chronic constriction induced nerve injury (65), indicating that deficits in mitochondrial respiration may contribute to persistent inflammatory pain and neuropathic pain, respectively. Dichloroacetate (DCA), which activates pyruvate dehydrogenase through inhibiting pyruvate dehydrogenase kinase, boosts the TCA cycle and OxPhos (130). The reversal in mitochondrial respiration deficit induced by DCA in the spinal cord reduced pain-associated behaviors in rats and mice after CFA-induced inflammatory pain and chronic construction induced nerve injury (65). In contrast, when rotenone, a mETC complex I inhibitor that decreases OxPhos, is injected at the site of inflammation, it decreased CFA-induced mechanical hypersensitivity (66). Even though these findings seem contradictory, they may be explained by differential effects of modulating mitochondrial respiration at peripheral nerves and at the spinal cord/DRG. Indeed, preliminary data shows that intrathecal administration of complex III inhibitor myxothiazol into the spinal cord/DRG inhibited hyperalgesic priming, a form of latent nociceptor plasticity, but not when myxothiazol was injected locally in the inflamed paw (64). Moreover, intrathecal injection of rotenone (complex I inhibitor) or antimycin (complex III inhibitor) in naive mice generated persistent pain (129), whilst rotenone decreased CFA-pain when injected into the inflamed hind paw (66). To what extent these differences are merely a difference between non-inflammatory and inflammatory conditions remains to be determined. Moreover these injections do not target only neurons, but also various other cells such as skin cells, immune cells, glia, and others. Thus, differences may depend on which cells are targeted.
There is also evidence that a single inflammatory agent changes mitochondrial activity in sensory neurons. As example, the prototypic inflammatory prostaglandin E2 (PGE2) increased the TCA cycle and OxPhos through an EPAC2 dependent pathway, contributing to acute inflammatory pain (63). Another indication that mitochondrial respiration may contribute to inflammatory pain is that expression of ATPSc-KMT (also known as FAM173B), a mitochondrial methyltransferase that promotes OxPhos and mtROS production in neurons (131), is increased in DRG neurons of mice with chronic inflammatory pain. Knockdown of ATPSc-KMT in the lumbar DRG during the established chronic inflammatory pain attenuated hyperalgesia. Conversely, expression of ATPSc-KMT in sensory neurons of mice with transient inflammatory pain caused persistent pain (46). These findings may be relevant to rheumatic pain patients because a genetic polymorphism downstream of the ATPSc-KMT gene is linked to joint-specific chronic widespread pain (132). Moreover, ATPSc-KMT expression is increased in the subchondral bone of OA patients and is negatively correlated with pressure pain sensitivity (104). The cells in which ATPs-KMT expression is increased remain to be determined. Nerve cells may be possible candidates, because innervation is increased in the subchondral bone of OA patients (32), but nerve cells are still much less abundant than joint cells in this case.
Hyperalgesic priming triggered by peripheral inflammation affects the plasticity of sensory neurons, by augmenting mRNA translation and causing a switch from cyclic adenosine monophosphate (cAMP) to protein kinase C ε (PKCε-dependent signaling, following a subsequent inflammatory stimulus that acts on G-protein-coupled receptors (GPCRs) (30, 133). Hyperalgesic priming requires the activity of mETC complexes and various mitochondrial proteins are targets of PKCε-dependent signaling (134, 135), pointing to a role of mitochondria in hyperalgesic priming. Activated PKCε promotes the opening of mitochondrial ATP-dependent K+ channels (mitoKATP) and increases OxPhos (136, 137). Moreover, preliminary data suggest that hyperalgesic priming increased OxPhos in DRG sensory neurons (64).
Overall, inflammatory mediators alter mitochondrial respiration in neurons. Based on current evidence, some inflammatory agents promote mitochondrial respiration, whilst others reduce it. Possibly, the effect differs depending on the time point after the administration of the inflammatory agent (25). Nevertheless, current data show that both increased and decreased OxPhos result in pain. The question remains why both reduced and increased OxPhos may contribute to pain? Possibly, impaired OxPhos reduces ATP production, which could affect the stability of the membrane potential and disturb neuronal excitability (45, 138). In contrast, increased OxPhos may come at the costs of more production of mtROS as a byproduct of mETC, leading to hyperexcitability, as will be discussed in the next section. Further research will need to contemplate how exactly mitochondrial respiration is linked to sensory neuron function.
Mitochondria generate approximately 90% of cellular ROS (139). MtROS production damages mitochondria in a range of pathologies, including neuropathies (45), and is important in redox signaling (140). Superoxide (O2−) is the proximal mtROS, mainly released at the mETC during OxPhos. Complex I and Complex III are the major sources of mtROS, and complex II to a lesser extent. Although the mETC is the main source of ROS, several other matrix proteins and complexes, like TCA cycle enzymes (e.g., pyruvate dehydrogenase), or some inner mitochondrial membrane proteins whose activity is partially dependent on mitochondrial membrane potential, also produce ROS.
Approximately 0.2%–2.0% of the oxygen consumed by mitochondria is reduced to O2−, which is subsequently converted to other ROS, such as hydrogen peroxide (H2O2) and hydroxyl ions (OH−) (140, 141). An imbalance between mtROS production and its removal, either due to ROS overproduction and/or decreased antioxidants defense, causes oxidative stress (139, 142). Neurons, like all other mammalian cells, have antioxidant enzyme systems, such as superoxide dismutase, peroxidases or catalases, which scavenge mtROS when they are generated. Despite contributing to pathology, mtROS also act as signaling molecules, to ensure quality control and maintenance of functional cells, and regulate a variety of physiological processes, such neuronal differentiation, synaptic pruning, and neurotransmission (143, 144). A disturbed mETC is one of the major drivers of mtROS (139), but also decreased mitochondrial membrane potential, disrupted mitochondrial Ca2+ buffering, altered mitochondrial morphology, or cellular stress in general augment mtROS production, which disrupts the redox balance and causes oxidative stress that negatively affects neuron function (143, 145).
Neurons are more likely to suffer from mtROS-induced oxidative stress compared to other cell types, because of their large energy consumption, mainly supported through OxPhos. Moreover, neurons have a high content of unsaturated fatty acids and proteins that are vulnerable to oxidation (146). A disrupted redox balance has detrimental consequences for neuronal functioning (139, 141, 147). As an example, mutations in superoxide dismutase (Cu-Zn), also known as superoxide dismutase 1 (SOD1), cause motor neuron degeneration in amyotrophic lateral sclerosis patients (125, 148), of which 60% develop pain (149). Antimycin A (mETC complex III inhibitor) induces mtROS, which activates TRPA1 receptor and increases the excitability of sensory neurons (150–152). Similarly, treatment of spinal cord neurons with ROS donors augmented their excitability and intrathecal administration of these ROS donors induced mechanical hypersensitivity in rats (153). Increased ROS production in the spinal cord also promoted pain through reducing firing of spinal inhibitory neurons. In contrast, others have found that ROS increase the activity of a different subset of inhibitory neurons (154, 155). Although some studies have shown decreased activity, in general, ROS appears to increase neuronal excitability and promote pain.
Both inflammatory and neuropathic pain are associated with increased ROS production in the peripheral and central nervous system. In mice, intraplantar capsaicin administration induced mtROS in spinal cord neurons. Overexpression of the anti-oxidant mitochondrial manganese dependent superoxide dismutase (MnSOD), also called SOD2, prevented capsaicin-induced hyperalgesia, indicating requirement of mtROS for hyperalgesia development (69). Importantly, rare variants present in mETC genes that are major sites of mtROS formation, are associated with the severity of erosive RA (156). Accordingly, increased mtDNA mutations, often a consequence of mtROS, are found in RA and PsA patients (157). These mtDNA mutations affect mitochondrial function and further promote mtROS production (144, 158). Even though these mutations were identified in synoviocytes, similar mutations may occur in sensory neurons innervating chronically inflamed tissues. Indeed, inflammation triggers mtROS production causing mutations in mtDNA in neurons (146). A role for mtROS in pain is further substantiated by findings that overexpression of mitochondrial ATPSc-KMT induced mtROS formation in DRG neurons and prolonged inflammatory pain. The antioxidant phenyl-N-t-butylnitrone (PBN) or the mitochondria-targeted antioxidant mitoTEMPOL reversed the persistent pain induced by ATPSc-KMT overexpression (46, 64). Various other studies showed that antioxidants (e.g., resveratrol) reduced pain in rodent models of OA and neuropathic pain (159–162). Overall, these data indicate that ROS, likely mitochondrial derived, contributes to inflammatory pain.
There is even more evidence for a role of mtROS in neuropathic pain. In spinal dorsal horn neurons, mtROS is increased in rats 1 week after spinal nerve ligation (70). SOD2-like antioxidants reduced mechanical and heat hyperalgesia induced by spinal nerve ligation or chronic constriction injury in rats (163, 164). Chemotherapeutic agents, such as cisplatin and oxaliplatin, cause nerve damage and pain through mitochondrial dysfunction, due to increased mtROS production and decreased antioxidant protection (58, 71, 144, 158, 165, 166). Intraperitoneal administration of mitochondria specific (SS-31, TEMPOL, or a SOD2 mimetic) or unspecific antioxidants (PBN, pioglitazone) reduced ROS production and attenuated pain in chemotherapy-induced neuropathy models (45, 47, 48, 71), showing that mtROS production is a driver of neuronal dysfunction and pain.
Although direct evidence for a contribution of mtROS in the nervous system for pain in rheumatic disease is still lacking, findings in neuropathic pain and inflammatory pain models suggest that oxidative stress induced by mitochondrial dysfunction may contribute to pain by increasing neuronal excitability.
The endoplasmic reticulum and mitochondria are the two major intracellular Ca2+ storages (167). In neurons, mitochondria take up Ca2+ into the mitochondrial matrix through the mitochondrial Ca2+ uniporter (MCU) complex (55, 167–169). The MCU is Ca2+-sensitive and opens by elevated cytosolic Ca2+, allowing Ca2+ to flow into the matrix. Mitochondria release Ca2+ primarily by the Na+/Ca2+ exchanger (NCLX) (170). The maximal rate of release is much lower than the maximal uptake rate. Therefore, under conditions of continuous high cytosolic Ca2+, e.g., due to continuous firing of neurons, mitochondrial Ca2+ accumulates. Mitochondria have an enormous capacity to accumulate and store Ca2+. In resting neurons, total mitochondrial Ca2+ is approximately 100 μM and free mitochondrial Ca2+ only 0.1 μM (171). These concentrations steeply increase when neurons are active and mitochondria start accumulating Ca2+ (172). In extreme conditions, mitochondrial Ca2+ reaches a concentration of up to 1,500 μM (173). In case of mitochondrial Ca2+ overload, the mitochondrial permeability transition pore (mPTP) is formed, which releases Ca2+ and other molecules, such as Cytochrome C, into the cytosol, inducing apoptosis. This pore has been mainly studied under pathological conditions, and several core components like ATP synthase, cyclophilin D, and the adenine nucleotide translocators are thought to be involved (123, 167, 174).
Only a relatively small fraction of Ca2+ is handled by mitochondria during physiological cytosolic Ca2+ signals. Nevertheless, mitochondrial Ca2+ influx and efflux play a role in the spatiotemporal organization of the cytosolic Ca2+ signals (175), which regulates activity-dependent signaling and neuronal excitability in nociceptors, thus is important to prevent aberrant signaling and pain (176). A rise in mitochondrial matrix Ca2+ stimulates OxPhos-mediated ATP and mtROS production, regulates organelle dynamics and trafficking, and modulates neurotransmitter release, synaptic transmission, and excitability. Mitochondria are a major regulator of Ca2+ signaling at the first sensory synapse (39, 76, 174, 177, 178). Moreover, mitochondrial Ca2+ mediates signaling to the nucleus and the release of death signals, in case of very high Ca2+ (39, 75, 76, 174, 177, 179–181).
Inflammatory stimuli can lead to a rise in cytosolic Ca2+ in sensory neurons, which facilitates the release of neurotransmitters, excitability, and pain (39, 76, 177, 181). Studies showed that inflammatory cytokines increase spontaneous Ca2+ oscillations in organotypic spinal cord slices (182). Intraplantar carrageenan or CFA injection in rats increased intracellular Ca2+ in brain neurons or increased evoked Ca2+ transients in DRG neurons, respectively (72–74). These studies show that cytosolic Ca2+ is regulated by inflammation, yet direct evidence that mitochondrial Ca2+ is affected during inflammatory pain is lacking. Because mitochondrial and cytosolic Ca2+ are interdependent, the data may suggest a potential involvement of mitochondrial Ca2+ in inflammatory pain.
Nerve damage causes disturbed mitochondrial Ca2+ buffering (180). For example, spinal nerve ligation in rats reduced mitochondrial Ca2+ storage capacity in lumbar DRG neurons (75). Rats with chemotherapy-induced neuropathic pain have a decreased duration of depolarization-evoked Ca2+ transient, which is partially mediated by an augmented mitochondrial Ca2+ uptake and increased mitochondrial volume (78). In vitro studies showed that the chemotherapeutic drug paclitaxel induces the formation of mPTP and promotes the release of mitochondrial Ca2+, contributing to sensory neuron hyperexcitability and cell death (76). The chemotherapeutic drugs cisplatin and oxaliplatin increased cytosolic Ca2+ concentration and depolarization-evoked Ca2+ transients in cultured sensory neurons (71, 165). Taken together, different insults disturb distinctive aspects of mitochondrial Ca2+ buffering.
Does modulating mitochondrial Ca2+ buffering affect neuronal excitability and pain? The noxious heat-activated receptor TRPV1 conducts Ca2+ and Na+, producing a depolarizing receptor potential that activates nociceptors. Knockdown of NCLX in DRG neurons decreased mitochondrial Ca2+ release, reduced capsaicin-induced TRPV1 activation and neuronal firing (183), indicating that mitochondrial Ca2+ buffering is important for neurons' ability to respond to pungent reagents. Blocking MCU, with an intrathecal injection of a MCU inhibitor, prevents mitochondrial Ca2+ uptake in spinal cord neurons and diminished capsaicin-induced neuron hyperexcitability and pain in mice (184). Mechanistically, by regulating cytosolic and mitochondrial ionic transients, NLCX and MCU modulate Ca2+-dependent desensitization of TRPV1 channels, thereby controlling nociceptive signaling (183). Oxaliplatin increases cytosolic Ca2+ by increasing the expression of non-selective cation channel TRPV1 in DRG neurons, thus increasing sensory neurons' excitability. Silencing or blocking TRPV1 reduced both oxaliplatin and paclitaxel induced neuropathic pain (185–188), which is indirect evidence that mitochondrial Ca2+ buffering is also involved in chemotherapy-induced neuropathic pain. A role for MCU in mechanical allodynia has been found in mice with painful diabetic neuropathy. Selective knockdown of the MCU in Nav1.8-positive DRG neurons resolved mechanical allodynia in diabetic mice (79). Likewise, blocking mPTP through intraperitoneal injection of cyclosporine A reversed spared nerve injury-induced allodynia in rats, by reducing Cytochrome C release and loss in activity of spinal cord GABAergic inhibitory neurons (76, 77).
Mitochondrial Ca2+ buffering also plays a role in spinal synaptic plasticity (184). Inhibition of spinal mitochondrial Ca2+ uptake in mice, using different pharmacological strategies, blocked N-methyl D-aspartate (NMDA)-induced activation of downstream protein kinases that mediate spinal synaptic plasticity, and reduced induction of long term potentiation, a process that increases synaptic strength in the dorsal horn of the spinal cord and contributes to chronic pain. Importantly, inhibition of mitochondrial Ca2+ uptake in the spinal cord prevented animals from developing mechanical hyperalgesia in response to intrathecal NMDA or intradermal capsaicin injection (184).
Inflammasomes are intracellular multiproteic complexes, composed of a sensor protein that oligomerizes, in order to recruit caspase-1. MtROS production is a main trigger of nucleotide-binding oligomerization domain-like receptor pyrin domain containing 3 (NLRP3) inflammasome activation (189, 190). MtROS causes translocation of the inner mitochondrial membrane protein cardiolipin to the outer mitochondrial membrane, where cardiolipin serves as a docking place for caspase-1 and NLRP3. Subsequently, the adaptor apoptosis-associated speck-like protein containing a CARD (ASC) binds both NLRP3 and caspase-1, activating the inflammasome to initiate caspase-1-mediated cleavage of pro-IL-1β and pro-IL-18 into their active mature form (189). Mitochondria can also sustain NLRP3 inflammasome activation via generation of ATP (191) and disturbed Ca2+ signaling (189, 190). While NLRP3 inflammasome activation is classically thought to occur in immune cells, such as macrophages, it has also been detected in neurons (97, 192). Moreover, NLRP3 expression is increased in the DRG neurons of patients with neuropathic pain in comparison with controls with no pain (121). Neuronal NLRP3 inflammasome activation likely contributes to neuro-inflammation and neurodegeneration, as it has been detected in brain neurons in Parkinson's and Huntington's disease (193–195). It is not known whether NLRP3 inflammasome activation interferes directly with neuronal functioning.
In the context of rheumatic diseases, mitochondrial dysfunction (e.g., increased mtROS, disturbed OxPhos or Ca2+ uptake) may trigger NLRP3 inflammasome activation in the nervous system, affecting sensory processing. For example, in the CFA-induced chronic inflammatory pain, the compound muscone, which diminishes ROS production, prevented the loss of mitochondrial membrane potential and Ca2+ influx. Moreover, it blocked the CFA-induced increase in spinal cord NLRP3 and IL-1β expression and hyperalgesia (87, 196). Importantly, the downstream product of inflammasome activation, IL-1β, induces firing of nociceptors and mediates CFA-induced pain (189, 197, 198), suggesting a putative role for NLRP3 inflammasome activation in inflammatory pain.
Although studies about the role of NLRP3 inflammasome in the nervous system in inflammatory pain models are still scarce, various findings suggest the involvement of this pathway in other types of pain. Intraperitoneal or intrathecal administration of MCC950, a specific NLRP3 inflammasome inhibitor, alleviated mechanical allodynia, and decreased IL-1β and IL-18 release in the lumbar dorsal spinal cord in cancer-induced bone pain and oxaliplatin-induced neuropathy, respectively (97, 98). In the cancer-induced bone pain model, IL-1β and IL-18 were detected predominantly in neurons (97). Suppressing NLRP3 inflammasome activation with different microRNAs, reduced NLRP3 inflammasome activation in the sciatic nerve, spinal astrocytes and microglia, while concurrently attenuating mechanical allodynia after chronic constriction injury in mice and rats (92–96). The chemotherapeutic drug bortezomib, which disrupts mitochondrial Ca2+ buffering and promotes mtROS production in neurons, increased NLRP3 expression in the DRG (138). Silencing NLRP3 in the DRG prevented the development of bortezomib-induced neuropathic pain in mice and rats (99). Treatment with a ROS scavenger decreased paclitaxel-induced mechanical allodynia and reduced NLRP3 expression in DRG macrophages and caspase 1/IL-1β activation in lumbar DRG and sciatic nerve (100). These studies indicate that nerve damage and inflammation may lead to inflammasome activation in the nervous system, although the majority of these studies did not identify in which cells the inflammasome was activated. Given that nerve damage and inflammation promote mtROS production in neurons, it is possible that mtROS triggers inflammasome activation in neurons and contributes to pain.
Mitochondria are highly dynamic organelles and have several quality control pathways in order to maintain their integrity. A controlled balance between mitophagy and mitochondrial biogenesis guarantees optimal mitochondrial turnover. Mitochondrial dynamics involve continuous fission and fusion forming a dynamic network to maintain their content, morphology and quality (145, 199).
Damaged mitochondria are selectively degraded by auto-phagosomes engulfment, followed by lysosomal degradation, a controlled process called mitophagy. Mitophagy involves PTEN-induced kinase 1 (PINK1), which binds the surface of depolarized and damaged mitochondria and to recruit Parkin and trigger mitochondrial aggregation, engulfment, and digestion by lysosomes (44). The production of new functional mitochondria, mitochondrial biogenesis, is mainly driven by peroxisome proliferator-activated receptor-gamma coactivator-1α (PGC-1α). Increased AMP/ATP and ADP/ATP ratios activate the energy sensor AMPK, which activates PGC-1α by phosphorylation. An increase in the NAD+/NADH ratio triggers PGC-1α activation via sirtuin1 (SIRT1)-mediated deacetylation. Thus, mitochondrial biogenesis is highly influenced by the cellular energy state and redox balance. PGC-1α promotes mtDNA replication and the expression of mitochondrial genes, such as transcription nuclear factors (NRF-1 and NRF-2), which prompt the expression of mitochondrial transcription factor A (TFAM) and mETC complexes subunits (200). Dysfunctional mitophagy or defective mitochondrial biogenesis results in an overall less efficient mitochondrial pool with impaired ATP production and increased mtROS production, which ultimately may affect sensory processing (201).
Some studies suggest a link between dysfunctional mitophagy and pain. Following spinal nerve ligation, PINK1 expression is increased in inhibitory GABAergic interneurons, suggesting increased mitophagy in these spinal cord neurons (80). Pink1-KO mice have normal responses to mechanical stimuli, but develop less mechanical allodynia after monosodium iodoacetate (MIA)-induced OA or after spinal nerve ligation (44, 80). Morover, Pink1-KO mice developed less spontaneous pain in the second inflammatory phase of formalin-induced pain (202). These data suggest that PINK1/Parkin-mediated mitophagy is required for development of inflammatory, osteoarthritis and neuropathic pain. But why does reducing mitophagy alleviate pain? One could expect that diminished mitophagy would increase the pool of damaged mitochondria, increase mtROS production and enhance neuronal excitability (145). However, in chronic pain states, if the rate of mitophagy is increased whilst not being in balance with the rate of biogenesis, there is an overall depletion of mitochondria. In Pink1-KO mice this balance may be restored due to diminished mitophagy (201). Indeed, in conditions such as ischemic stroke, diabetes or autosomal dominant optic atrophy, insufficient biogenesis and excessive mitophagy diminished the number of mitochondria in neurons leading to cell death (203–205). Similarly, decreased mitochondrial biogenesis reduced the total pool of mitochondria in brain neurons in Parkinson's and Alzheimer's disease leading to neuro-inflammation and -degeneration (55, 206, 207).
Some indirect evidence suggests mitochondrial biogenesis is impaired in several pain conditions, because AMPK activation, an essential driver for mitochondrial biogenesis, has an analgesic effect. Local or systemic AMPK activation with various pharmacological compounds reduced formalin, zymosan (208), and CFA-induced inflammatory pain in mice (198, 208). Intrathecal AMPK activation reduced oxaliplatin-induced neuropathy (209), cancer-induced bone pain (210), and painful diabetic neuropathy (211). In addition, systemic AMPK activation using metformin or resveratrol attenuated spinal nerve ligation induced pain (212), post-surgical pain (213, 214), paclitaxel-induced neuropathy (215, 216), and plantar incision-induced hyperalgesic priming (214–216). Notably, AMPK activation reduced the excitability of sensory neurons (209, 212, 217). Unfortunately, effects on mitochondria were not assessed in these studies. Thus, further research is required to confirm that mitochondrial biogenesis is at the root of the analgesic properties of AMPK activation.
Adjacent mitochondria can merge, a process called fusion, permitting mixture of mitochondrial content. Conversely, fission is when mitochondria constrict and segment. The GTPases mitofusins1 and 2 (MFN1, MFN2) and optic atrophy 1 (OPA1) induce intermembrane fusion, whilst dynamin-related protein (DRP1) drives mitochondrial fission (84, 218). The fission/fusion ratio controls mitochondria's morphology, size and number (219). Damaged mitochondria can undergo fusion, to create a more interconnected and complementary mitochondrial network to reduce cellular stress. Fission is required for mitochondrial biogenesis and promotes mitophagy in case of cellular stress (220). These dynamics are essential for local mitochondrial quality control, which is particularly important in neurons due to their long axons. Excessive fission results in fragmented mitochondria, impaired energy production, and disrupted mitochondrial homeostasis. Fusion, on the other hand, promotes a more efficient and interconnected mitochondrial network (219, 220). Most evidence for a role of these dynamics in neuronal functioning comes from studies in brain neurons. Increased fission diminishes dendritic and axonal branching and contributes to neurodegeneration in an ischemic stroke model (124, 221). Accordingly, inhibition of DRP1 prevents mitochondrial dysfunction, improves neuronal survival and axonal integrity (55, 145, 221, 222). Finally, depletion of DRP1 reduces neurotoxic Aβ oligomers-induced fission and improves mitochondrial health and synaptic activity in cortical neurons, in a mouse model of Alzheimer disease (39, 223). Altogether, excessive fission appears detrimental for neuronal functioning, whilst reducing fission is neuroprotective.
To what extent the balance between fission and fusion controls sensory neuron function has not been extensively explored. Silencing DRP1 or pharmacologically blocking its activity in the spinal cord and in the DRG decreased HIV/AIDS- and chemotherapy-induced neuropathic pain (224). Likewise, intrathecal administration of 2-bromopalmitate, an inhibitor of protein palmitoylation, decreased DRP1 and increased OPA1 expression in spinal cord astrocytes, inhibiting fission and promoting fusion, respectively. Restoring the balance in fission/fusion reduced CFA-induced inflammatory pain and cancer-induced bone pain (83, 84, 225, 226). Intrathecal administration of SRT1720, a SIRT1 agonist, increases SIRT1-mediated mitochondrial biogenesis and decreased spinal cord DRP1 expression, which was associated with a reduction in cancer-induced bone pain and chronic construction injury-induced neuropathic pain (218, 227). The reduction in mitochondrial Ca2+ influx in diabetic mice, with a nociceptor-specific deletion of MCU, prevented mitochondrial fragmentation due to excessive fission in DRG neurons, nerve degeneration, and pain (79). Additionally, intrathecal injection of resveratrol to reduce spinal cord oxidative stress, decreased spinal DRP1 activity and reversed cancer-induced bone pain in rats (159). These data clearly show that mitochondrial dynamics are linked to various other mitochondrial functions and to sensory processing. Inflammation disturbs OxPhos, promotes oxidative stress and Ca2+ influx into the mitochondria via MCU. All these processes can induce mitochondrial fission (127, 128, 182, 228). As such, inflammation is more likely to induce mitochondrial fission rather than fusion (229), but future research is needed to fully understand how fission and fusion contribute to pain in rheumatic disease.
Mitochondrial biogenesis occurs close to the nucleus, since the majority of mitochondrial proteins are encoded by the nuclear genome. In neurons, mitochondria are mainly made in the soma, therefore mitochondria have to be transported to the dendrites and axonal terminals (230). Some studies identified that mitochondrial biogenesis also occurs to some extent in the axons, but the mechanisms are not completely clear (200). Motor proteins from the kinesin family mediate anterograde transport of new mitochondria from the soma to the axons, whilst motor proteins that form a dynein-dynactin complex mediate retrograde transport towards the soma (199, 230). Motor proteins interact with the microtubule network and mitochondrial Rho GTPases (MIROs) to regulate mitochondrial motility for both anterograde and retrograde transport (231).
Neuron-specific knockdown of Miro1 in mice depletes mitochondria in dendrites and diminishes neuron survival, highlighting the importance of mitochondrial motility for neuronal homeostasis (232). Impairing mitochondrial transport by decreasing the activity of motor proteins in cervical ganglion neurons diminished the number of axonal and dendritic mitochondria, which was associated with synaptic dysfunction that limited neurotransmission and neuroplasticity (231). Restoring the diminished local ATP pool that was affected by the impaired transport, reestablished these parameters, indicating that mitochondrial transport is essential to maintain sufficient ATP levels throughout neurons (233). Enhancing mitochondrial transport in adult murine DRG or cortical neurons by knockdown of syntaphilin, an anchoring protein that keeps mitochondria static, improved axonal regrowth and normalized cellular ATP/ADP ratio after axotomy (233, 234). Inflammation in the peripheral nervous system, induced by experimental autoimmune neuritis, reduced retro- and anterograde transport of mitochondria in nerves (126), indicating that inflammation reduces mitochondrial transport. Similarly, exposure of murine brain slices to lipopolysacharide (LPS) reduced retrograde transport (235). Thus, efficient mitochondrial transport is a requirement for effective energy production and synaptic transmission in neurons. Inflammation can reduce this mitochondrial transport through axons, thereby affecting the local ATP pool.
Histone deacetylase 6 (HDAC6) deacetylates the cytoskeletal protein α-tubulin, limiting mitochondrial transport and distribution in neurons. Systemic administration of HDAC6 inhibitors decreased inflammation-induced mechanical hypersensitivity in CFA- and collagen-induced arthritis models (236, 237), and neuropathic pain models (236, 238). Pharmacological inhibition of HDAC6 also decreased LPS-induced neuronal loss and pro-inflammatory cytokines production in the brain, demonstrating a neuroprotective effect of improving mitochondrial transport (239, 240). Cognitive impairment and neuro-inflammation (microgliosis, production of TNF and IL-1β) in the spinal cord/brain in neuropathic pain models are reversed by HDAC6 inhibition (236, 238). In line with the previous results, oral administration of HDAC6 inhibitors, Hdac6 knockout, or cell-specific deletion of Hdac6 in advillin-positive sensory neurons, promoted mitochondrial transport in sensory neurons, attenuated mechanical hyperalgesia, and spontaneous pain in chemotherapy-induced neuropathy (85, 86). HDAC6 inhibition reestablished OCR in lumbar DRG neurons and the tibial nerve of cisplatin-treated mice to the values of control animals (85, 86), indicating mitochondrial transport in neurons is essential to ensure sufficient OxPhos when damaged by neurotoxic agents. Currently, oral administration of ACY-1215 (Ricolinostat, HDAC6 inhibitor) is being assessed in patients suffering from painful diabetic peripheral neuropathy (clinical trial NCT03176472). A selective HDAC6 inhibitor reduced the inflammatory phenotype of immune cells from RA patients in vitro and pre-clinical studies show an analgesic effect of this approach in RA models (236, 237, 241). Thus facilitating mitochondrial transport through HDAC6 inhibitors may be a promising approach to relieve rheumatic pain.
Mitochondrial defects in non-neuronal cells may also contribute to changes in sensory processing. Neuro-inflammation contributes to inflammatory and neuropathic pain (65, 92, 95, 210, 238, 242, 243). Mitochondrial dysfunction is a driver of neuro-inflammation and neuronal damage in neurodegenerative diseases (34, 229). Impaired mitochondrial function, such as disturbed Ca2+ buffering or increased oxidative stress, in neurons may result in the intracellular release of mitochondrial components, such as mitochondrial DNA, mtROS or Cytochrome C. When extracellular, these components act as damage-associated molecular patterns (DAMPs) that can trigger an inflammatory response and engage spinal microglia or DRG macrophages (34). On the other hand, inflammatory mediators released by the immune cells can contribute to mitochondrial dysfunction, further promoting neuro-inflammation (34, 229). Tissue damage and inflammatory processes in rheumatic joints induce mitochondrial alterations in cells of the joint, such as chondrocytes and synoviocytes. These mitochondrial defects may promote the release of inflammatory mediators that regulate neuronal activity and pain.
In several models of rheumatic diseases and in rheumatic patients, mitochondrial respiration is reduced in cells of the joint. As an example, chondrocytes of rabbits and guinea pigs with experimental OA, have an impaired mitochondrial respiration, reduced intracellular ATP levels, and a rise in the lactate/pyruvate ratio (67, 68). These differences worsened during the progression of cartilage damage (68). Similarly, chondrocytes collected from OA patients have decreased activity of mETC complexes and reduced ATP levels compared to control patients, which correlated with an increase in the number of apoptotic chondrocytes (101–103). Apoptotic chondrocytes release substances that can activate receptors on sensory neurons and elicit pain (244). Increased formation of oxidative metabolites (e.g., H2O2) and decreased expression of endogenous antioxidant molecules (e.g., SOD2, peroxidase) are found in the knee (synovium and cartilage) in pre-clinical rheumatic disease models (112, 245), and in humans with OA (112–116), RA (51, 115, 117) and PsA (118). In RA, an imbalance between oxidants and antioxidants leads to mitochondrial oxidative stress in synoviocytes, which triggers activation of transcription factor NF-κB and subsequent release of inflammatory mediators, such as IL-8 or PGE2 (246), that can activate receptors on sensory neurons to induce pain (247, 248). MtROS is important in this process, because mitoTEMPOL, a mitochondrial specific ROS scavenger, reduced the release of inflammatory mediators by human synoviocytes (246).
Oxidative stress can also induce apoptosis of chondrocytes via reduction in the expression of FOXO3, a transcription factor upstream of various antioxidant genes, such as SOD2 (248, 249). Intra-articular or oral treatment with antioxidants, such as amobarbital, N-acetylcysteine (NAC), resveratrol or methylene blue have analgesic effects in different species (mice, rat, porcine) with OA (250, 251) and CFA-induced joint inflammation (160). Similarly, a specific mitochondrial targeted-antioxidant, plastoquinonyl-decyl-triphenylphosphonium bromide (SkQ1), reduced bone destruction and cartilage damage in OA mice (252). Chondrocytes damage may lead to the release of neurotrophin NGF by immune cells, which activates its high-affinity receptor TrkA on neurons and induces expression of a variety of ion channels (e.g., TRPV1, voltage gated sodium channels) and pain (253, 254).
Increased ROS production is a feature of senescence cells, i.e., cells that have entered a non-proliferative state. Cellular senescence is accompanied by a distinct secretory phenotype, senescence-associated secretory phenotype (SASP), which includes a variety of secreted proteins, cytokines and chemokines that can drive chronic inflammation (255, 256). Recently, cellular senescence has been identified in nervous tissue in mice with long-lasting (>4 months) neuropathic pain. Intriguingly, p53-mediated senescence drove neuropathic pain specifically in male, but not female mice. Moreover, a mutation in the P53 gene is associated with chronic pain in men (256). In mice with experimental OA, the number of senescent cells in the cartilage is increased. Selective elimination of these cells, either genetically or pharmacologically by intra-articular administration of a senolytic, reduced pain-associated behavior and enhanced cartilage reconstruction (257). Similarly, blocking p53-mediated senescence or removing senescent cells reversed neuropathic pain, by reducing the expression of SASP effectors (e.g., p53, IL-1β, IL-6) (256, 258). These results indicate a potential role of mitochondria-driven cellular senescence in pain.
NLRP3 inflammasome activation has been linked to amplified inflammatory cytokine signaling in senescent cells (259, 260). The expression of NLRP3 inflammasome related molecules (NLRP3, ASC, caspase-1, IL-1β, IL-18) is increased in the synovial tissue of collagen-induced arthritis (CIA) mice (88, 192, 261) and MIA-induced OA rats (89, 90). Intraperitoneal administration of the specific NLRP3 inhibitor MCC950, or blocking IL-18, diminished synovial inflammation and cartilage damage in RA. Moreover, pharmacological inhibition of the inflammasome products IL-1β or IL-18 attenuates RA or neuropathic pain (262, 263). Additionally, administration of NLRP3 inflammasome inhibitors reversed NLRP3 activation in FLSs and relieved OA-induced pain (89, 90). Moreover, indirect regulation of the inflammasome, by downregulating microRNA miR-30b-5p in the joint, attenuated the increase in NLRP3, ASC, and cleaved caspase-1 in joints, and decreased cartilage damage and pain in OA rats (91). In the joint tissue of OA patients, miR-30b-5p is elevated compared to healthy controls and its expression correlates with disease severity and levels of IL-1β in the synovial fluid (91), suggesting a putative role of inflammasome activation in OA patients. However, it remains unclear if inflammasome activation also mediates pain in this rheumatic disease in humans, as pain-associated outcomes were not investigated in this study.
Interestingly, the expression of translocator protein (TSPO), found in the outer mitochondrial membrane, is increased in the synovial membrane of OA patients and its expression levels correlated with lower pain intensity (104). Although TSPO has been commonly used as a target for PET imaging to assess neuro-inflammation and microglia/macrophage activation (264), it is a mitochondrial protein that has also been linked to mitochondrial bioenergetics. Knockout of TSPO in human microglia cell lines reduced OxPhos and ATP production. In addition, TSPO ligands in in vivo models prevented mitochondrial membrane depolarization, mPTP formation and NLRP3 inflammasome activation (265, 266), suggesting TSPO activity may indirectly affect some mitochondrial functions. Thus, the TSPO correlation with lower pain intensity may potentially link to overall better mitochondrial performance.
Mitochondrial quality control mechanisms are essential to maintain cellular homeostasis and prevent the production of inflammatory cytokines. Intra-articular MIA administration, to induce experimental OA in rats, increased the expression of PINK1 and Parkin in knee cartilage. Likewise, treatment of human chondrocytes with MIA increases PINK1 expression in these cells. In OA patients, PINK1 expression in chondrocytes in damaged parts of joint cartilage is higher compared to healthy cartilage (44). These data point to exacerbated PINK-1-mediated mitophagy in chondrocytes, which may result in cartilage damage and pain. Several studies also described possible disturbances in mitochondrial biogenesis in chondrocytes of rodents or OA patients, since AMPK activity, SIRT-1, PGC-1α, TFAM, and NRF-2 expression are reduced compared to controls (81, 82). In CIA-induced arthritis, intraperitoneal administration of mitochondrial fission inhibitor mdivi-1 reduced arthritis scores and paw thickness in mice (120). Moreover, DRP1, a protein that promotes fission, is increased in synovial tissues and FLSs from RA patients and in primary human chondrocytes following MIA treatment (120). In RA, DRP1 expression correlates with disease severity (44). Finally, neurotrophins, such as NGF or BDNF, are released in the synovial fluid of RA, OA and AS patients and can induce mitochondrial fission in neurons (267–269). These data suggest that mitochondrial fission is enhanced in various rheumatic diseases and that inhibiting fission reduces arthritis. Whether changes in fission also contribute to pain remains to be explored.
In conclusion, several mitochondrial functions are clearly affected in the local tissue in rheumatic diseases. It is not fully understood how these mitochondrial alterations contribute to rheumatic pain. We propose that changes in mitochondrial functions in the site of injury may promote the release of inflammatory mediators that sensitize or activate the nervous system, contributing to pain.
Collection of human joint tissue is relatively common in rheumatic conditions, because it can be obtained during total knee or hip replacement surgeries. On the other hand, DRG, nerves and spinal cord are not easily accessible tissues, which explains the lack of human data regarding mitochondrial functions in the nervous system in rheumatic disease. In contrast, blood is very easily accessible and there is a correlation between mitochondrial dysfunction in blood cells and several other tissues (270, 271). Moreover, studies suggest that mitochondrial alterations in blood cells could be used as a peripheral marker for diseases affecting the nervous system (272, 273). For these reasons, there are several studies that have explored changes in mitochondrial functions in blood components and cells from patients with rheumatic diseases, which could shed some light into how mitochondrial alterations may be associated with rheumatic conditions. An overview of the findings in human patients is depicted in Table 2.
Compared to healthy controls, peripheral blood mononuclear cells (PBMCs) from RA and fibromyalgia patients express less mETC complexes at the protein level, have reduced cellular ATP levels, and have a diminished mitochondrial membrane potential (105–107). Similarly, genes associated with OxPhos are downregulated in peripheral leukocytes of JIA patients in comparison with healthy children. In SLE patients, ATP levels are reduced in T lymphocytes (109, 110). PBMCs and skin fibroblasts from patients suffering from SLE (119) and fibromyalgia (108), respectively, have elevated mtROS levels, similar to what was observed in blood monocytes from PsA patients (118). Given that all these rheumatic diseases have an inflammatory nature, and that inflammatory mediators affect mitochondrial functions and cellular metabolism in immune cells (274, 275), it is highly possible that these changes are caused by inflammation. Conceivably, similar changes are induced in sensory neurons and affect their function.
In fibromyalgia patients with pain, complex III activity, the expression of multiple mitochondrial biogenesis related genes (PGC-1α, TFAM, NRF1), and the expression of coenzyme Q10 are decreased in PBMCs and skin fibroblasts. In contrast, mtROS production is increased compared to controls without pain (105). These data suggest that mitochondrial dysfunction in PBMCs is linked to pain in fibromyalgia. Moreover, inflammasome related molecules (e.g., IL-1β, IL-18) were significantly increased in serum of fibromyalgia patients and correlated with pain VAS scores (108). In RA, mutations in mitochondrial related genes are detected in MT-ND1, which encodes a complex I subunit, as well as in NLRP3 and CARD8, which recruits caspase to form NLRP3 complex (276). Additionally, a mutation in the genomic region encoding ATPSc-KMT, a mitochondrial localized protein, is linked to chronic widespread pain and increased pressure pain sensitivity in OA (104, 132).
In conclusion, these data suggest that markers of mitochondrial dysfunction and mitochondrial related genetic modifications are present in rheumatic patients. In some cases, these mitochondrial alterations are associated with the magnitude of pain. Future research has to show whether these cause pain, or are rather a consequence of the pathology that is causing pain.
Over the recent years, the possibility to target mitochondria for the treatment of several pathologies, like primary mitochondrial diseases, neurodegenerative diseases, heart failure, chronic kidney disease, and stroke-like episodes has gained attention (277, 278). Even though most evidence for efficacy comes from pre-clinical work, some compounds that target mitochondria are already being assessed for safety in humans. Improving mitochondrial bioenergetics by targeting deficits in mitochondria is still at an early development stage. We will discuss various compounds that are being developed and could be of interest for treating painful (rheumatic) diseases.
Given that inflammation reduces mitochondrial respiration in sensory neurons and diminished OxPhos is associated with prolonged pain, boosting mitochondrial respiration may resolve pain. DCA boosts the TCA cycle and OxPhos. DCA reduced pain-associated behaviors in pre-clinical inflammatory pain models and showed a safe profile when tested in humans (65, 130, 279). DCA reduced lactate levels in endometriosis cells, and is currently being tested as treatment for endometriosis-associated pain (clinical trial NCT04046081) (279).
Altered mitochondrial respiration can lead to unfavorable cellular redox balance, e.g., NAD/NADH ratio. NAD+ is an essential cofactor in the regulation of mitochondrial health. A decline in NAD+ in various tissues and cells, including neurons, is associated with pathologies, such as neurodegenerative diseases, diabetic-induced and chemotherapy-induced neuropathic pain (280–283). Recently, preliminary data showed that peripheral inflammation reduces NAD+ levels in DRG of mice. Systemic or intrathecal administration of nicotinamide riboside, a precursor of NAD+, reversed CFA-induced inflammatory pain (64). Importantly, NAD+ supplementation with NAD+ precursors, such as nicotinamide riboside, mitigates oxidative stress and improves mitochondrial functions. More than 70 years ago, studies already showed that NAD+ supplementation reduces pain in RA patients (284, 285). More recently, oral NAD+ supplementation reversed diabetic and chemotherapy-induced neuropathic pain in pre-clinical models (281, 283). Other recent studies also showed that NAD+ supplementation has favorable outcomes on RA and SLE, but pain was not assessed in these studies (286). NAD+ supplementation also improved the global impact of OA, possibly through the increase of NAD+ in the synovial fluid and cartilage matrix, ensuring proper energy levels for cartilage repair. However, in this study NAD+ supplementation did not affect pain (287). Recent clinical trials in larger cohorts and with more extensive assessment exams have confirmed that oral supplementation with NAD+ precursors (including nicotinamide riboside) are safe and well-tolerated (288, 289). Future larger studies will have to show whether NAD+ supplementation may hold promise for treatment of inflammation and pain in patients with rheumatic disease.
Antioxidants are promising in reversing oxidative stress in diseases of the nervous system (290, 291). The antioxidant resveratrol is effective in managing rheumatoid arthritis by decreasing plasma inflammatory markers and joint swelling, when given in combination with common anti-rheumatic drugs (117). Similarly, resveratrol administered as adjuvant with the anti-rheumatic drug meloxicam decreased serum levels of inflammatory mediators (TNF, IL-1β, IL-6) and reduced pain by ∼70%, compared to OA patients that received meloxicam together with placebo (292, 293). Oral resveratrol also attenuated chronic musculoskeletal pain by 20% in postmenopausal women (294), indicating a potential analgesic effect of resveratrol in several pain conditions. Currently studies are further exploring resveratrol's analgesic effect in knee OA patients as combined (anti-inflammatory drugs and/or analgesics) and as mono-therapy (clinical trial NCT02905799) (295).
Pre-clinical studies show that targeting inflammasome-related proteins has analgesic effects (92–98). In a clinical trial, oral treatment with Dapansutrile, a specific NLRP3 inflammasome inhibitor, reduced joint pain 56%–68% and reduced joint and systemic inflammation in gout flare patients (296). Other drugs that target the inflammasome pathway, such as the caspase-1 inhibitor Pralnacasan, the anti-IL18 monoclonal antibody GSK1070806, and compounds targeting IL-1β or its receptor (e.g., anakinra and canakinumab), are being clinically evaluated or already approved to treat inflammatory diseases, including rheumatic disease. Anakinra and canakinumab reduce pain in RA and JIA, but they still need to be assessed in other rheumatic conditions. The ability of GSK1070806 to reduce pain has not been studied (clinical trial NCT03681067) (297–300).
Mitochondria are transferred between cells via extracellular vesicles, tunneling nanotubes, gap junctions or free mitochondrial ejection (42, 301–308). Intercellular transfer of healthy mitochondria can improve the energy production status of recipient cells and restore their viability. The release of damaged mitochondria might function as a help request to surrounding cells, triggering signaling pathways to restore homeostasis in the cell releasing damaged mitochondria (42, 301–304). Mitochondrial transfer has been observed in a growing list of pathologies that include brain injury (42, 309), neurodegenerative diseases (306, 310), cancer (303, 311, 312), and cardiomyopathy (304). Mitochondria can be transferred from human synovial mesenchymal stem cells (sMSCs) to Th17 cells, which reduces the production of the pro-inflammatory cytokine IL-17. Mitochondrial transfer between sMSCs and Th17 is impaired in RA patients (313). Likewise, sMSCs donate mitochondria to stressed articular chondrocytes and failure of this transfer has been hypothesized to impair healing of orthopedic tissues (314). Importantly, transfer of mitochondria from macrophages to DRG neurons is required to resolve inflammatory pain (25). These findings highlight that intercellular mitochondrial transfer may be an approach to reduce inflammatory pain.
Some research groups have explored the delivery of entire functional mitochondria into damaged cells, a technique called mitochondrial transplantation (315, 316). Pre-clinical studies show mitochondrial transplantation is neuroprotective (317, 318). Additionally, a small clinical trial with 5 patients showed that autologous mitochondrial transplantation (i.e., mitochondria from non-ischemic skeletal muscle of each patient were injected in the myocardium) improved myocardial function in patients with ischemia-reperfusion injury, without inducing short-term complications (319). In all these studies, “naked”/free mitochondria were directly injected in the tissue of interest. Classically, some free mitochondria components are thought to act as DAMPs, thus triggering an inflammatory response (317). However, “naked” mitochondria are found in the human blood, suggesting that healthy free mitochondria are not necessarily inflammatory (320). Nevertheless, to prevent any adverse effect of free mitochondria, packaging mitochondria into vesicles would prevent them from acting as DAMPs, whilst it would allow specific targeting strategies by adjusting lipid composition. Synaptosomes are lipidic membranous particles released at nerve terminals. Synaptosomes can be obtained by homogenization and gradient centrifugation of nervous tissue (synaptic terminals), and contain several synaptic vesicles and mitochondria. Synaptosomes are specifically taken up by neuronal cells, making them an ideal delivery system to target neurons (316).
In summary, targeting mitochondria shows some beneficial effects in multiple pathologies and could be a novel approach to treat chronic pain. The challenge is to develop a strategy to deliver a drug to mitochondria in specific cell types. Possibly, encapsulation of drugs/mitochondria into nanolipidic carriers may overcome these challenges (321–324) and allow the use of mitochondria/mitochondria targeting drugs to treat pain. As an example, in a pre-clinical study, extracellular vesicle containing mitochondria resolved persistent inflammatory pain, albeit transiently (25).
The contribution of mitochondrial dysfunction for chemotherapy, diabetes and HIV-induced neuropathic pain is clear and has been extensively reviewed elsewhere (45). Currently available data point to changes in mitochondria in various cells/tissues in rheumatic diseases (Figure 2; Tables 1, 2). In rheumatic diseases, peripheral inflammation at the joint level may affect mitochondrial function, and mitochondrial dysfunction can further promote inflammation. These inflammatory mediators may affect mitochondria function in sensory neurons, impacting their excitability. Pain development has been associated with altered OxPhos, disturbed Ca2+ buffering, increased mtROS production, NLRP3 inflammasome activation and defective mitochondrial quality control mechanisms in the nervous system, but also surrounding cells, such as astrocytes, microglia or other immune cells. Various mitochondrial functions in sensory neurons have been linked to changes in sensory processing, but it is important to note that mitochondrial functions are tightly connected. Therefore, it remains difficult to know if a specific function controls pain/sensory processing. Finally, given the intertwined role of damage and inflammation in mitochondrial function and vice versa, it remains difficult to conclude if mitochondrial dysfunction is a cause or a consequence of rheumatic diseases.
Figure 2. Mitochondrial dysfunction in rheumatic pain conditions. (A) Oxidative stress, NLRP3 inflammasome activation and mitophagy are increased in the joint in various rheumatic conditions. Mitochondrial dysfunction is not limited to the damaged joint tissue; it is also present in the nervous system. Decreased mitochondrial respiration and increased inflammasome activation have been detected in RA animal models. Indirect evidence points to decreased mitochondrial biogenesis and excessive mitophagy in the nervous system, but it is not clear in which cells. (B) Evidence from clinical and pre-clinical studies points to impaired mitochondrial functions in chondrocytes and fibroblast-like synoviocytes (FLSs), which could contribute to the inflammatory milieu observed in the joint and may activate resident immune cells like macrophages. Mutations in mtDNA may contribute to impaired mitochondrial function. Peripheral inflammation and damage activate and induce long-lasting changes in sensory neurons, and increase their excitability. (C) Sensory neuron activation increases mitochondrial Ca2+ levels that promote OxPhos, but also mtROS production. Continuous mtROS production may impair mitochondrial respiration or cause mtDNA mutations. Inflammation and oxidative stress reduce mitochondrial transport and impair quality control mechanisms (mitophagy, mitochondrial biogenesis, fusion and fission). Disturbed mitochondrial Ca2+ buffering and mtROS trigger NLRP3 inflammasome activation in neurons. (D) Prolonged activation of nociceptors in the periphery affects the spinal cord/brain axis of pain processing and can induce permanent central changes in neuroplasticity and mitochondrial dysfunction in neurons and supporting cells like astrocytes. (E) Changes in mitochondrial functions in blood components and cells appear to be present in patients with various rheumatic diseases. Even if these alterations were not directly linked to pain, they could shed some light on how mitochondria are affected in rheumatic disease. A question mark is used when there is indirect evidence or there are contradictory findings. Dashed arrows were used to represent hypothetical connections or consequences that have not been directly linked to rheumatic pain. DRG, dorsal root ganglia; PBMC, peripheral blood cells; RBC, red blood cell. Figure created with BioRender.com.
A striking observation is that many of the pre-clinical studies investigating mitochondria in pain used only male animals. Given that RA, OA, SLE or fibromyalgia are more prevalent in females than males (325–328) and various studies show that pain mechanisms are sex-dependent (256, 329–332), sex differences should be taken into account in the contribution of mitochondria to sensory processing. Especially since mitochondrial biogenesis and mitochondrial-mediated cell death signaling after oxygen and glucose withdrawal are sex dependent (333, 334).
Various alterations in mitochondrial functions, such as respiration, oxidative stress, biogenesis and NLRP3 inflammasome activation appear to occur both in human and mice in the context of rheumatic disease. Other studies have also shown similarities between rodents and humans, e.g., they have a similar distribution of synaptic and non-synaptic mitochondria in the brain (335). Moreover, mitochondrial response to aging is conserved between different species (humans, monkey, mice, rats) in terms of changes in mETC, OxPhos, oxidative stress and mitophagy at the pathway level (336, 337). Nevertheless, there are examples of species differences. For example human and mouse astrocytes have different OxPhos rates in vitro, with human astrocytes being more susceptible to oxidative stress (338). Regardless, to gain an accurate understanding of the putative role of mitochondria in pain, more research should include humans material, as findings in rodents, the most commonly used animals in pain research, are not always translatable to humans (339). A limitation of the rodent models is that behavioral assays used often do not assess actual pain, but rather pain-associated behavior such as hyperalgesia. As such, most existing data only shows a relation with the development of hypersensivity to noxious stimuli and not per se spontaneous pain (340). At the molecular level, species difference may also exist. Even though the overall signature of DRG-enriched genes is conserved between mice and human (341), various ion channels or cholinergic receptors are differentially expressed in neuronal subpopulations between mouse versus human DRG (342). Finally. aging is a risk factor for rheumatic diseases and patients experience pain during years (340, 343). However, for ethical and financial reasons, pain and putative underlying mechanisms are rarely followed for more than a few months in animal models. More studies should be performed in aged mice and at later time points after the model is established (256, 340), because potentially relevant aspects are missed. As example, targeting senescence, which is closely linked to mitochondrial function, in the spinal cord decreased neuropathic pain when it was established for 9 months, but not when it was only established for 2 weeks after spared nerve injury (199, 256). These rodent models are still valuable, because some compounds that had an analgesic effect in pre-clinical studies (e.g., NAD+ supplementation, resveratrol, NLRP3 inflammasome inhibitors) also relieved pain in clinical trials (64, 97, 98, 160, 161, 281, 283, 285, 292, 293, 298, 299).
Until now, human data on mitochondria function in the nervous system is scarce. Skin biopsies and, occasionally, DRG can be collected from live human donors, but central nervous tissues are usually obtained only post mortem (339). Interestingly, recent imaging techniques may allow to obtain this information to a certain extent. Imaging techniques (e.g., magnetic resonance spectroscopy, infrared spectroscopy or positron emission tomography) can be used to measure mitochondrial oxygen consumption, mitochondrial membrane potential, ATP and metabolite levels in the brain or spinal cord (278, 344). For example, TSPO-PET, which has been commonly used to measure immune cell activation in the nervous system, may also be useful to assess mitochondrial respiration and function, according to some recent pre-clinical studies (265, 266, 345). Therefore, these non-invasive techniques could potentially be applied to collect information about mitochondrial dysfunction not only in the brain, but also DRG, spinal cord and nerves in rheumatic pain patients.
In conclusion, impaired mitochondrial health at the peripheral and central level may contribute to rheumatic pain. Considering the central role of mitochondria in cellular function after inflammation and their emerging role in sensory processing, modulation of mitochondrial functions may be a promising approach to attenuate or eliminate pain in rheumatic diseases.
PSSR: conceptualization, writing, review and editing, visualization. HLDMW: writing, reviewing funding acquisition and editing. NE: conceptualization, review and editing, supervision, funding acquisition. All authors contributed to the article and approved the submitted version.
This work has received funding from the European Union’s Horizon 2020 research and innovation program under the Marie Skłodowska-Curie grant agreement No 814244. HLDMW is supported by the Netherlands Organization for Scientific Research (NWO) (016.VENI.192.053)
The authors would like to thank the members of the Eijkelkamp group, Judith Prado Sanchez, Leire Almandoz Gil and Hui Ying Yeoh for proof-reading the manuscript. Figures were created with BioRender.
The authors declare that the research was conducted in the absence of any commercial or financial relationships that could be construed as a potential conflict of interest.
All claims expressed in this article are solely those of the authors and do not necessarily represent those of their affiliated organizations, or those of the publisher, the editors and the reviewers. Any product that may be evaluated in this article, or claim that may be made by its manufacturer, is not guaranteed or endorsed by the publisher.
1. Lampa J. Pain without inflammation in rheumatic diseases. Best Pract Res Clin Rheumatol. (2019) 33(3):101439. doi: 10.1016/j.berh.2019.10143
2. Rafael-Vidal C, Pérez N, Altabás I, Garcia S, Pego-Reigosa JM. Blocking IL-17: a promising strategy in the treatment of systemic rheumatic diseases. Int J Mol Sci. (2020) 21(19):7100. doi: 10.3390/ijms21197100
3. Szekanecz Z, McInnes IB, Schett G, Szamosi S, Benkő S, Szűcs G. Autoinflammation and autoimmunity across rheumatic and musculoskeletal diseases. Nat Rev Rheumatol. (2021) 17(10):585–95. doi: 10.1038/s41584-021-00652-9
4. Seifert O, Baerwald C. Interaction of pain and chronic inflammation. Z Rheumatol. (2021) 80(3):205–13. doi: 10.1007/s00393-020-00951-8
5. Maiuolo J, Muscoli C, Gliozzi M, Musolino V, Carresi C, Paone S, et al. Endothelial dysfunction and extra-articular neurological manifestations in rheumatoid arthritis. Biomolecules. (2021) 11(1):81. doi: 10.3390/biom11010081
6. Pisetsky DS, Eudy AM, Clowse MEB, Rogers JL. The categorization of pain in systemic lupus erythematosus. Rheum Dis Clin North Am. (2021) 47(2):215–28. doi: 10.1016/j.rdc.2020.12.004
7. Martini A, Lovell DJ, Albani S, Brunner HI, Hyrich KL, Thompson SD, et al. Juvenile idiopathic arthritis. Nat Rev Dis Prim. (2022) 8(1):5. doi: 10.1038/s41572-021-00332-8
8. Di Franco M, Guzzo MP, Spinelli FR, Atzeni F, Sarzi-Puttini P, Conti F, et al. Pain and systemic lupus erythematosus. Reumatismo. (2014) 66(1):33–8. doi: 10.4081/reumatismo.2014.762
9. van Helvoort EM, Welsing PMJ, Jansen MP, Gielis WP, Loef M, Kloppenburg M, et al. Neuropathic pain in the IMI-APPROACH knee osteoarthritis cohort: prevalence and phenotyping. RMD Open. (2021) 7(3):e002025. doi: 10.1136/rmdopen-2021-002025
10. Momoli A, Giarretta S, Modena M, Micheloni GM. The painful knee after total knee arthroplasty: evaluation and management. Acta Biomed. (2017) 88(2S):60–7. doi: 10.23750/abm.v88i2-S.6515
11. Wylde V, Beswick A, Bruce J, Blom A, Howells N, Gooberman-Hill R. Chronic pain after total knee arthroplasty. EFORT open Rev. (2018) 3(8):461–70. doi: 10.1302/2058-5241.3.180004
12. Wylde V, Hewlett S, Learmonth ID, Dieppe P. Persistent pain after joint replacement: prevalence, sensory qualities, and postoperative determinants. Pain. (2011) 152(3):566–72. doi: 10.1016/j.pain.2010.11.023
13. Taylor P, Manger B, Alvaro-Gracia J, Johnstone R, Gomez-Reino J, Eberhardt E, et al. Patient perceptions concerning pain management in the treatment of rheumatoid arthritis. J Int Med Res. (2010) 38(4):1213–24. doi: 10.1177/147323001003800402
14. Lee YC, Cui J, Lu B, Frits ML, Iannaccone CK, Shadick NA, et al. Pain persists in DAS28 rheumatoid arthritis remission but not in ACR/EULAR remission: a longitudinal observational study. Arthritis Res Ther. (2011) 13(3):R83. doi: 10.1186/ar3353
15. Lee YC, Frits ML, Iannaccone CK, Weinblatt ME, Shadick NA, Williams DA, et al. Subgrouping of patients with rheumatoid arthritis based on pain, fatigue, inflammation, and psychosocial factors. Arthritis Rheumatol. (2014) 66(8):2006–14. doi: 10.1002/art.38682
16. Minhas D, Clauw DJ. Pain mechanisms in patients with rheumatic diseases. Rheum Dis Clin North Am. (2021) 47(2):133–48. doi: 10.1016/j.rdc.2021.01.001
17. Gabriel SE, Michaud K. Epidemiological studies in incidence, prevalence, mortality, and comorbidity of the rheumatic diseases. Arthritis Res Ther. (2009) 11(3):229. doi: 10.1186/ar2669
18. Jackson TP, Stabile VS, McQueen KAK. The Global burden of chronic pain. ASA Newsl. (2014) 78(6):24–7.
19. Überall MA. A review of scientific evidence for THC:CBD oromucosal spray (nabiximols) in the management of chronic pain. J Pain Res. (2020) 13:399–410. doi: 10.2147/JPR.S240011
20. Hylands-White N, Duarte RV, Raphael JH. An overview of treatment approaches for chronic pain management. Rheumatol Int. (2017) 37(1):29–42. doi: 10.1007/s00296-016-3481-8
21. Nalamachu S. An overview of pain management: the clinical efficacy and value of treatment. Am J Manag Care. (2013) 19(14 Suppl):S261–6.
22. Urits I, Gress K, Charipova K, Habib K, Lee D, Lee C, et al. Use of cannabidiol (CBD) for the treatment of chronic pain. Best Pract Res Clin Anaesthesiol. (2020) 34(3):463–77. doi: 10.1016/j.bpa.2020.06.004
23. Crofford LJ. Use of NSAIDs in treating patients with arthritis. Arthritis Res Ther. (2013) 15(Suppl 3):S2. doi: 10.1186/ar4174
24. Paglia MDG, Silva MT, Lopes LC, Barberato-Filho S, Mazzei LG, Abe FC, et al. Use of corticoids and non-steroidal anti-inflammatories in the treatment of rheumatoid arthritis: systematic review and network meta-analysis. PLoS One. (2021) 16(4):e0248866. doi: 10.1371/journal.pone.0248866
25. van der Vlist M, Raoof R, Willemen HLDM, Prado J, Versteeg S, Martin Gil C, et al. Macrophages transfer mitochondria to sensory neurons to resolve inflammatory pain. Neuron. (2022) 110(4):613–626.e9. doi: 10.1016/j.neuron.2021.11.020
26. Coussens NP, Sittampalam GS, Jonson SG, Hall MD, Gorby HE, Tamiz AP, et al. The opioid crisis and the future of addiction and pain therapeutics. J Pharmacol Exp Ther. (2019) 371(2):396–408. doi: 10.1124/jpet.119.259408
27. Volkow ND, Blanco C. The changing opioid crisis: development, challenges and opportunities. Mol Psychiatry. (2021) 26(1):218–33. doi: 10.1038/s41380-020-0661-4
28. Rahn EJ, Guzman-Karlsson MC, David Sweatt J. Cellular, molecular, and epigenetic mechanisms in non-associative conditioning: implications for pain and memory. Neurobiol Learn Mem. (2013) 105:133–50. doi: 10.1016/j.nlm.2013.06.008
29. Price TJ, Inyang KE. Commonalities between pain and memory mechanisms and their meaning for understanding chronic pain. Prog Mol Biol Transl Sci. (2015) 131:409–34. doi: 10.1016/bs.pmbts.2014.11.010
30. Ferrari LF, Bogen O, Chu C, Levine JD. Peripheral administration of translation inhibitors reverses increased hyperalgesia in a model of chronic pain in the rat. J Pain. (2013) 14(7):731–8. doi: 10.1016/j.jpain.2013.01.779
31. Cao Y, Fan D, Yin Y. Pain mechanism in rheumatoid arthritis: from cytokines to central sensitization. Mediators Inflamm. (2020) 2020:2076328. doi: 10.1155/2020/2076328
32. Grässel S, Muschter D. Peripheral nerve fibers and their neurotransmitters in osteoarthritis pathology. Int J Mol Sci. (2017) 18(5):931. doi: 10.3390/ijms18050931
33. Süß P, Rothe T, Hoffmann A, Schlachetzki JCM, Winkler J. The joint-brain axis: insights from rheumatoid arthritis on the crosstalk between chronic peripheral inflammation and the brain. Front Immunol. (2020) 11:612104. doi: 10.3389/fimmu.2020.612104
34. Marchi S, Guilbaud E, Tait SWG, Yamazaki T, Galluzzi L. Mitochondrial control of inflammation. Nat Rev Immunol. (2022):1–15. doi: 10.1038/s41577-022-00760-x
35. Vergara RC, Jaramillo-Riveri S, Luarte A, Moënne-Loccoz C, Fuentes R, Couve A, et al. The energy homeostasis principle: neuronal energy regulation drives local network dynamics generating behavior. Front Comput Neurosci. (2019) 13:49. doi: 10.3389/fncom.2019.00049
36. Pekkurnaz G, Wang X. Mitochondrial heterogeneity and homeostasis through the lens of a neuron. Nat Metab. (2022) 4(7):802–12. doi: 10.1038/s42255-022-00594-w
37. Romero-Garcia S, Prado-Garcia H. Mitochondrial calcium: transport and modulation of cellular processes in homeostasis and cancer (review). Int J Oncol. (2019) 54(4):1155–67. doi: 10.3892/ijo.2019.4696
38. Rueda CB, Llorente-Folch I, Amigo I, Contreras L, González-Sánchez P, Martínez-Valero P, et al. Ca2+ regulation of mitochondrial function in neurons. Biochim Biophys Acta. (2014) 1837(10):1617–24. doi: 10.1016/j.bbabio.2014.04.010
39. Devine MJ, Kittler JT. Mitochondria at the neuronal presynapse in health and disease. Nat Rev Neurosci. (2018) 19(2):63–80. doi: 10.1038/nrn.2017.170
40. Wang X, Perry G, Smith MA, Zhu X. Amyloid-beta-derived diffusible ligands cause impaired axonal transport of mitochondria in neurons. Neurodegener Dis. (2010) 7(1–3):56–9. doi: 10.1159/000283484
41. Cole NB, Dieuliis D, Leo P, Mitchell DC, Nussbaum RL. Mitochondrial translocation of alpha-synuclein is promoted by intracellular acidification. Exp Cell Res. (2008) 314(10):2076–89. doi: 10.1016/j.yexcr.2008.03.012
42. Hayakawa K, Esposito E, Wang X, Terasaki Y, Liu Y, Xing C, et al. Transfer of mitochondria from astrocytes to neurons after stroke. Nature. (2016) 535(7613):551–55. doi: 10.1038/nature18928
43. Zheng Y, Chen Y, Lu X, Weng Q, Dai G, Yu Y, et al. Inhibition of histone deacetylase 6 by tubastatin A attenuates the progress of osteoarthritis via improving mitochondrial function. Am J Pathol. (2020) 190(12):2376–86. doi: 10.1016/j.ajpath.2020.08.013
44. Shin HJ, Park H, Shin N, Kwon HH, Yin Y, Hwang J-A, et al. Pink1-mediated chondrocytic mitophagy contributes to cartilage degeneration in osteoarthritis. J Clin Med. (2019) 8(11):1849. doi: 10.3390/jcm8111849
45. Flatters SJL. The contribution of mitochondria to sensory processing and pain. Prog Mol Biol Transl Sci. (2015) 131:119–46. doi: 10.1016/bs.pmbts.2014.12.004
46. Willemen HLDM, Kavelaars A, Prado J, Maas M, Versteeg S, Nellissen LJJ, et al. Identification of FAM173B as a protein methyltransferase promoting chronic pain. PLoS Biol. (2018) 16(2):e2003452. doi: 10.1371/journal.pbio.2003452
47. Toyama S, Shimoyama N, Ishida Y, Koyasu T, Szeto HH, Shimoyama M. Characterization of acute and chronic neuropathies induced by oxaliplatin in mice and differential effects of a novel mitochondria-targeted antioxidant on the neuropathies. Anesthesiology. (2014) 120(2):459–73. doi: 10.1097/01.anes.0000435634.34709.65
48. Kim HK, Zhang YP, Gwak YS, Abdi S. Phenyl N-tert-butylnitrone, a free radical scavenger, reduces mechanical allodynia in chemotherapy-induced neuropathic pain in rats. Anesthesiology. (2010) 112(2):432–9. doi: 10.1097/ALN.0b013e3181ca31bd
49. Mitchell R, Campbell G, Mikolajczak M, McGill K, Mahad D, Fleetwood-Walker SM. A targeted mutation disrupting mitochondrial complex IV function in primary afferent neurons leads to pain hypersensitivity through P2Y1 receptor activation. Mol Neurobiol. (2019) 56(8):5917–33. doi: 10.1007/s12035-018-1455-4
50. van den Ameele J, Fuge J, Pitceathly RDS, Berry S, McIntyre Z, Hanna MG, et al. Chronic pain is common in mitochondrial disease. Neuromuscul Disord. (2020) 30(5):413–9. doi: 10.1016/j.nmd.2020.02.017
51. Fearon U, Canavan M, Biniecka M, Veale DJ. Hypoxia, mitochondrial dysfunction and synovial invasiveness in rheumatoid arthritis. Nat Rev Rheumatol. (2016) 12(7):385–97. doi: 10.1038/nrrheum.2016.69
52. Panga V, Kallor AA, Nair A, Harshan S, Raghunathan S. Mitochondrial dysfunction in rheumatoid arthritis: a comprehensive analysis by integrating gene expression, protein-protein interactions and gene ontology data. PLoS One. (2019) 14(11):1–28. doi: 10.1371/journal.pone.0224632
53. Blanco FJ, Rego I, Ruiz-Romero C. The role of mitochondria in osteoarthritis. Nat Rev Rheumatol. (2011) 7(3):161–9. doi: 10.1038/nrrheum.2010.213
54. Kan S, Duan M, Liu Y, Wang C, Xie J. Role of mitochondria in physiology of chondrocytes and diseases of osteoarthritis and rheumatoid arthritis. Cartilage. (2021) 13(2_suppl):1102S–21S. doi: 10.1177/19476035211063858
55. Mao X, Fu P, Wang L, Xiang C. Mitochondria: potential targets for osteoarthritis. Front Med. (2020) 7:581402. doi: 10.3389/fmed.2020.581402
56. Mantyh PW. The neurobiology of skeletal pain. Eur J Neurosci. (2015) 39(3):508–19. doi: 10.1111/ejn.12462
57. Falk S, Dickenson AH. Pain and nociception: mechanisms of cancer-induced bone pain. J Clin Oncol. (2014) 32(16):1647–54. doi: 10.1200/JCO.2013.51.7219
58. Pongratz G, Straub RH. The sympathetic nervous response in inflammation. Arthritis Res Ther. (2014) 16(6):504. doi: 10.1186/s13075-014-0504-2
59. Burma NE, Leduc-Pessah H, Fan CY, Trang T. Animal models of chronic pain: advances and challenges for clinical translation. J Neurosci Res. (2017) 95(6):1242–56. doi: 10.1002/jnr.23768
60. Krock E, Jurczak A, Svensson CI. Pain pathogenesis in rheumatoid arthritis-what have we learned from animal models? Pain. (2018) 159(Suppl 1):S98–109. doi: 10.1097/j.pain.0000000000001333
61. Roger AJ, Muñoz-Gómez SA, Kamikawa R. The origin and diversification of mitochondria. Curr Biol. (2017) 27(21):R1177–92. doi: 10.1016/j.cub.2017.09.015
62. McCarron JG, Wilson C, Sandison ME, Olson ML, Girkin JM, Saunter C, et al. From structure to function: mitochondrial morphology, motion and shaping in vascular smooth muscle. J Vasc Res. (2013) 50(5):357–71. doi: 10.1159/000353883
63. Goode DJ, Molliver DC. Regulation of mitochondrial function by Epac2 contributes to acute inflammatory hyperalgesia. J Neurosci. (2021) 41(13):2883–98. doi: 10.1523/JNEUROSCI.2368-20.2021
64. Willemen H, Ribeiro P, Broeks M, Meijer N, Versteeg S, Malecki J, et al. Inflammation-induced mitochondrial and metabolic disturbances in sensory neurons control the switch from acute to chronic pain. bioRxiv [Preprint]. (2022). doi: 10.1101/2022.08.29.505682
65. Lagos-Rodríguez V, Martínez-Palma L, Marton S, Miquel E, Escobar-Pintos R, Cassina A, et al. Mitochondrial bioenergetics, glial reactivity, and pain-related behavior can be restored by dichloroacetate treatment in rodent pain models. Pain. (2020) 161(12):2786–7. doi: 10.1097/j.pain.0000000000001992
66. Rouwette T, Sondermann J, Avenali L, Gomez-Varela D, Schmidt M. Standardized profiling of the membrane-enriched proteome of mouse dorsal root ganglia (DRG) provides novel insights into chronic pain. Mol Cell Proteomics. (2016) 15(6):2152–68. doi: 10.1074/mcp.M116.058966
67. Goetz JE, Coleman MC, Fredericks DC, Petersen E, Martin JA, McKinley TO, et al. Time-dependent loss of mitochondrial function precedes progressive histologic cartilage degeneration in a rabbit meniscal destabilization model. J Orthop Res Off Publ Orthop Res Soc. (2017) 35(3):590–9. doi: 10.1002/jor.23327
68. Johnson K, Svensson CI, Etten DV, Ghosh SS, Murphy AN, Powell HC, et al. Mediation of spontaneous knee osteoarthritis by progressive chondrocyte ATP depletion in hartley Guinea pigs. Arthritis Rheum. (2004) 50(4):1216–25. doi: 10.1002/art.20149
69. Schwartz ES, Kim HY, Wang J, Lee I, Klann E, Chung JM, et al. Persistent pain is dependent on spinal mitochondrial antioxidant levels. J Neurosci Off J Soc Neurosci. (2009) 29(1):159–68. doi: 10.1523/JNEUROSCI.3792-08.2009
70. Park E-S, Gao X, Chung JM, Chung K. Levels of mitochondrial reactive oxygen species increase in rat neuropathic spinal dorsal horn neurons. Neurosci Lett. (2006) 391(3):108–11. doi: 10.1016/j.neulet.2005.08.055
71. Khasabova IA, Khasabov SG, Olson JK, Uhelski ML, Kim AH, Albino-Ramírez AM, et al. Pioglitazone, a PPARγ agonist, reduces cisplatin-evoked neuropathic pain by protecting against oxidative stress. Pain. (2019) 160(3):688–701. doi: 10.1097/j.pain.0000000000001448
72. Voitenko N, Gerber G, Youn D, Randic M. Peripheral inflammation-induced increase of AMPA-mediated currents and Ca2+ transients in the presence of cyclothiazide in the rat substantia gelatinosa neurons. Cell Calcium. (2004) 35(5):461–9. doi: 10.1016/j.ceca.2003.11.002
73. Lu S-G, Gold MS. Inflammation-induced increase in evoked calcium transients in subpopulations of rat dorsal root ganglion neurons. Neuroscience. (2008) 153(1):279–88. doi: 10.1016/j.neuroscience.2008.02.006
74. Scheff NN, Lu S-G, Gold MS. Contribution of endoplasmic reticulum Ca2+ regulatory mechanisms to the inflammation-induced increase in the evoked Ca2+ transient in rat cutaneous dorsal root ganglion neurons. Cell Calcium. (2013) 54(1):46–56. doi: 10.1016/j.ceca.2013.04.002
75. Hogan QH, Sprick C, Guo Y, Mueller S, Bienengraeber M, Pan B, et al. Divergent effects of painful nerve injury on mitochondrial ca(2+) buffering in axotomized and adjacent sensory neurons. Brain Res. (2014) 1589:112–25. doi: 10.1016/j.brainres.2014.09.040
76. Yousuf MS, Maguire AD, Simmen T, Kerr BJ. Endoplasmic reticulum-mitochondria interplay in chronic pain: the calcium connection. Mol Pain. (2020) 16:1744806920946889. doi: 10.1177/1744806920946889
77. Kim WS, Park JY, Kim TK, Baik SW. The changes of mitochondrial cytochrome c and GABAergic neuron in neuropathic pain model. Korean J Anesthesiol. (2012) 62(4):365–70. doi: 10.4097/kjae.2012.62.4.365
78. Yilmaz E, Watkins SC, Gold MS. Paclitaxel-induced increase in mitochondrial volume mediates dysregulation of intracellular ca(2+) in putative nociceptive glabrous skin neurons from the rat. Cell Calcium. (2017) 62:16–28. doi: 10.1016/j.ceca.2017.01.005
79. George DS, Hackelberg S, Jayaraj ND, Ren D, Edassery SL, Rathwell C, et al. Mitochondrial calcium uniporter deletion prevents painful diabetic neuropathy by restoring mitochondrial morphology and dynamics. Pain. (2021) 163(3):560–78. doi: 10.1097/j.pain.0000000000002391
80. Yi M-H, Shin J, Shin N, Yin Y, Lee SY, Kim C-S, et al. PINK1 mediates spinal cord mitophagy in neuropathic pain. J Pain Res. (2019) 12:1685–99. doi: 10.2147/JPR.S198730
81. Zhao X, Petursson F, Viollet B, Lotz M, Terkeltaub R, Liu-Bryan R. Peroxisome proliferator–activated receptor γ coactivator 1α and FoxO3A mediate chondroprotection by AMP-activated protein kinase. Arthritis Rheumatol. (2014) 66(11):3073–82. doi: 10.1002/art.38791
82. Wang Y, Zhao X, Lotz M, Terkeltaub R, Liu-Bryan R. Mitochondrial biogenesis is impaired in osteoarthritis chondrocytes but reversible via peroxisome proliferator–activated receptor γ coactivator 1α. Arthritis Rheumatol. (2015) 67(8):2141–53. doi: 10.1002/art.39182
83. Xie M, Cheng M, Wang B, Jiao M, Yu L, Zhu H. 2-Bromopalmitate Attenuates inflammatory pain by maintaining mitochondrial fission/fusion balance and function. Acta Biochim Biophys Sin. (2021) 53(1):72–84. doi: 10.1093/abbs/gmaa150
84. Meng W, Hao M-M, Yu N, Li M-Y, Ding J-Q, Wang B-H, et al. 2-Bromopalmitate attenuates bone cancer pain via reversing mitochondrial fusion and fission imbalance in spinal astrocytes. Mol Pain. (2019) 15:1744806919871813. doi: 10.1177/1744806919871813
85. Krukowski K, Ma J, Golonzhka O, Laumet GO, Gutti T, van Duzer JH, et al. HDAC6 inhibition effectively reverses chemotherapy-induced peripheral neuropathy. Pain. (2017) 158(6):1126–37. doi: 10.1097/j.pain.0000000000000893
86. Ma J, Trinh RT, Mahant ID, Peng B, Matthias P, Heijnen CJ, et al. Cell-specific role of histone deacetylase 6 in chemotherapy-induced mechanical allodynia and loss of intraepidermal nerve fibers. Pain. (2019) 160(12):2877–90. doi: 10.1097/j.pain.0000000000001667
87. Yu S, Zhao G, Han F, Liang W, Jiao Y, Li Z, et al. Muscone relieves inflammatory pain by inhibiting microglial activation-mediated inflammatory response via abrogation of the NOX4/JAK2-STAT3 pathway and NLRP3 inflammasome. Int Immunopharmacol. (2020) 82:106355. doi: 10.1016/j.intimp.2020.106355
88. Zhang Y, Zheng Y, Li H. NLRP3 inflammasome plays an important role in the pathogenesis of collagen-induced arthritis. Mediators Inflamm. (2016) 2016:9656270. doi: 10.1155/2016/9656270
89. Liao T, Ding L, Wu P, Zhang L, Li X, Xu B, et al. Chrysin attenuates the NLRP3 inflammasome cascade to reduce synovitis and pain in KOA rats. Drug Des Devel Ther. (2020) 14:3015–27. doi: 10.2147/DDDT.S261216
90. Ma Z, Huang Z, Zhang L, Li X, Xu B, Xiao Y, et al. Vanillic acid reduces pain-related behavior in knee osteoarthritis rats through the inhibition of NLRP3 inflammasome-related synovitis. Front Pharmacol. (2021) 11:599022. doi: 10.3389/fphar.2020.599022
91. Xu H, Zhang J, Shi X, Li X, Zheng C. NF-κB inducible miR-30b-5p aggravates joint pain and loss of articular cartilage via targeting SIRT1-FoxO3a-mediated NLRP3 inflammasome. Aging. (2021) 13(16):20774–92. doi: 10.18632/aging.203466
92. Xu L, Wang Q, Jiang W, Yu S, Zhang S. MiR-34c ameliorates neuropathic pain by targeting NLRP3 in a mouse model of chronic constriction injury. Neuroscience. (2019) 399:125–34. doi: 10.1016/j.neuroscience.2018.12.030
93. Huang A, Ji L, Huang Y, Yu Q, Li Y. miR-185-5p alleviates CCI-induced neuropathic pain by repressing NLRP3 inflammasome through dual targeting MyD88 and CXCR4. Int Immunopharmacol. (2022) 104:108508. doi: 10.1016/j.intimp.2021.108508
94. Derangula K, Javalgekar M, Kumar Arruri V, Gundu C, Kumar Kalvala A, Kumar A. Probucol attenuates NF-κB/NLRP3 signalling and augments nrf-2 mediated antioxidant defence in nerve injury induced neuropathic pain. Int Immunopharmacol. (2022) 102:108397. doi: 10.1016/j.intimp.2021.108397
95. Tonkin RS, Bowles C, Perera CJ, Keating BA, Makker PGS, Duffy SS, et al. Attenuation of mechanical pain hypersensitivity by treatment with Peptide5, a connexin-43 mimetic peptide, involves inhibition of NLRP3 inflammasome in nerve-injured mice. Exp Neurol. (2018) 300:1–12. doi: 10.1016/j.expneurol.2017.10.016
96. Pan Z, Shan Q, Gu P, Wang XM, Tai LW, Sun M, et al. miRNA-23a/CXCR4 regulates neuropathic pain via directly targeting TXNIP/NLRP3 inflammasome axis. J Neuroinflammation. (2018) 15(1):29. doi: 10.1186/s12974-018-1073-0
97. Chen S-P, Zhou Y-Q, Wang X-M, Sun J, Cao F, HaiSam S, et al. Pharmacological inhibition of the NLRP3 inflammasome as a potential target for cancer-induced bone pain. Pharmacol Res. (2019) 147:104339. doi: 10.1016/j.phrs.2019.104339
98. Wahlman C, Doyle TM, Little JW, Luongo L, Janes K, Chen Z, et al. Chemotherapy-induced pain is promoted by enhanced spinal adenosine kinase levels through astrocyte-dependent mechanisms. Pain. (2018) 159(6):1025–34. doi: 10.1097/j.pain.0000000000001177
99. Liu C-C, Huang Z-X, Li X, Shen K-F, Liu M, Ouyang H-D, et al. Upregulation of NLRP3 via STAT3-dependent histone acetylation contributes to painful neuropathy induced by bortezomib. Exp Neurol. (2018) 302:104–11. doi: 10.1016/j.expneurol.2018.01.011
100. Jia M, Wu C, Gao F, Xiang H, Sun N, Peng P, et al. Activation of NLRP3 inflammasome in peripheral nerve contributes to paclitaxel-induced neuropathic pain. Mol Pain. (2017) 13:1744806917719804. doi: 10.1177/1744806917719804
101. Liu H, Li Z, Cao Y, Cui Y, Yang X, Meng Z, et al. Effect of chondrocyte mitochondrial dysfunction on cartilage degeneration: a possible pathway for osteoarthritis pathology at the subcellular level. Mol Med Rep. (2019) 20(4):3308–16. doi: 10.3892/mmr.2019.10559
102. Maneiro E, Martín MA, de Andres MC, López-Armada MJ, Fernández-Sueiro JL, del Hoyo P, et al. Mitochondrial respiratory activity is altered in osteoarthritic human articular chondrocytes. Arthritis Rheum. (2003) 48(3):700–8. doi: 10.1002/art.10837
103. Liu JT, Guo X, Ma WJ, Zhang YG, Xu P, Yao JF, et al. Mitochondrial function is altered in articular chondrocytes of an endemic osteoarthritis, kashin-beck disease. Osteoarthr Cartil. (2010) 18(9):1218–26. doi: 10.1016/j.joca.2010.07.003
104. Palada V, Siddiqah Ahmed A, Hugo A, Radojčić MR, Svensson CI, Kosek E. Expression of mitochondrial TSPO and FAM173B is associated with inflammation and symptoms in patients with painful knee osteoarthritis. Rheumatology. (2021) 60(4):1724–33. doi: 10.1093/rheumatology/keaa565
105. Cordero MD, Alcocer-Gómez E, Culic O, Carrión AM, de Miguel M, Díaz-Parrado E, et al. NLRP3 inflammasome is activated in fibromyalgia: the effect of coenzyme Q10. Antioxid Redox Signal. (2014) 20(8):1169–80. doi: 10.1089/ars.2013.5198
106. Jaiswal KS, Khanna S, Ghosh A, Padhan P, Raghav SK, Gupta B. Differential mitochondrial genome in patients with rheumatoid arthritis. Autoimmunity. (2021) 54(1):1–12. doi: 10.1080/08916934.2020.1846182
107. Khanna S, Padhan P, Jaiswal KS, Jain AP, Ghosh A, Tripathy A, et al. Altered mitochondrial proteome and functional dynamics in patients with rheumatoid arthritis. Mitochondrion. (2020) 54:8–14. doi: 10.1016/j.mito.2020.06.005
108. Cordero MD, Alcocer-Gómez E, Marín-Aguilar F, Rybkina T, Cotán D, Pérez-Pulido A, et al. Mutation in cytochrome b gene of mitochondrial DNA in a family with fibromyalgia is associated with NLRP3-inflammasome activation. J Med Genet. (2016) 53(2):113–22. doi: 10.1136/jmedgenet-2015-103392
109. Ishikawa S, Mima T, Aoki C, Yoshio-Hoshino N, Adachi Y, Imagawa T, et al. Abnormal expression of the genes involved in cytokine networks and mitochondrial function in systemic juvenile idiopathic arthritis identified by DNA microarray analysis. Ann Rheum Dis. (2009) 68(2):264–72. doi: 10.1136/ard.2007.079533
110. Perl A, Nagy G, Gergely P, Puskas F, Qian Y, Banki K. Apoptosis and mitochondrial dysfunction in lymphocytes of patients with systemic lupus erythematosus. Methods Mol Med. (2004) 102:87–114. doi: 10.1385/1-59259-805-6:087
111. Sánchez-Caballero L, Ruzzenente B, Bianchi L, Assouline Z, Barcia G, Metodiev MD, et al. Mutations in complex I assembly factor TMEM126B result in muscle weakness and isolated complex I deficiency. Am J Hum Genet. (2016) 99(1):208–16. doi: 10.1016/j.ajhg.2016.05.022
112. Fu Y, Kinter M, Hudson J, Humphries KM, Lane RS, White JR, et al. Aging promotes sirtuin 3-dependent cartilage superoxide dismutase 2 acetylation and osteoarthritis. Arthritis Rheumatol. (2016) 68(8):1887–98. doi: 10.1002/art.39618
113. Lepetsos P, Papavassiliou AG. ROS/oxidative stress signaling in osteoarthritis. Biochim Biophys Acta. (2016) 1862(4):576–91. doi: 10.1016/j.bbadis.2016.01.003
114. Regan E, Flannelly J, Bowler R, Tran K, Nicks M, Carbone BD, et al. Extracellular superoxide dismutase and oxidant damage in osteoarthritis. Arthritis Rheum. (2005) 52(11):3479–91. doi: 10.1002/art.21387
115. Ersoy Y, Özerol E, Baysal O, Temel I, MacWalter RS, Meral U, et al. Serum nitrate and nitrite levels in patients with rheumatoid arthritis, ankylosing spondylitis, and osteoarthritis. Ann Rheum Dis. (2002) 61(1):76–8. doi: 10.1136/ard.61.1.76
116. Karan A, Karan MA, Vural P, Erten N, Taşçıoğlu C, Aksoy C, et al. Synovial fluid nitric oxide levels in patients with knee osteoarthritis. Clin Rheumatol. (2003) 22(6):397–9. doi: 10.1007/s10067-003-0761-y
117. Khojah HM, Ahmed S, Abdel-Rahman MS, Elhakeim EH. Resveratrol as an effective adjuvant therapy in the management of rheumatoid arthritis: a clinical study. Clin Rheumatol. (2018) 37(8):2035–42. doi: 10.1007/s10067-018-4080-8
118. Chimenti MS, Sunzini F, Fiorucci L, Botti E, Fonti GL, Conigliaro P, et al. Potential role of cytochrome c and tryptase in psoriasis and psoriatic arthritis pathogenesis: focus on resistance to apoptosis and oxidative stress. Front Immunol. (2018) 9:2363. doi: 10.3389/fimmu.2018.02363
119. Leishangthem BD, Sharma A, Bhatnagar A. Role of altered mitochondria functions in the pathogenesis of systemic lupus erythematosus. Lupus. (2015) 25(3):272–81. doi: 10.1177/0961203315605370
120. Wang X, Chen Z, Fan X, Li W, Qu J, Dong C, et al. Inhibition of DNM1l and mitochondrial fission attenuates inflammatory response in fibroblast-like synoviocytes of rheumatoid arthritis. J Cell Mol Med. (2020) 24(2):1516–28. doi: 10.1111/jcmm.14837
121. North RY, Li Y, Ray P, Rhines LD, Tatsui CE, Rao G, et al. Electrophysiological and transcriptomic correlates of neuropathic pain in human dorsal root ganglion neurons. Brain. (2019) 142(5):1215–26. doi: 10.1093/brain/awz063
122. Blanco FJ, Rego-Pérez I. Mitochondria and mitophagy: biosensors for cartilage degradation and osteoarthritis. Osteoarthritis Cartilage. (2018) 26:989–91. doi: 10.1016/j.joca.2018.05.018
123. Sui B, Xu T, Liu J, Wei W, Zheng C, Guo B, et al. Understanding the role of mitochondria in the pathogenesis of chronic pain. Postgrad Med J. (2013) 89(1058):709–14. doi: 10.1136/postgradmedj-2012-131068
124. Rangaraju V, Lewis TL, Hirabayashi Y, Bergami M, Motori E, Cartoni R, et al. Pleiotropic mitochondria: the influence of mitochondria on neuronal development and disease. J Neurosci. (2019) 39(42):8200–8. doi: 10.1523/JNEUROSCI.1157-19.2019
125. Ferri A, Nencini M, Cozzolino M, Carrara P, Moreno S, Carrì MT. Inflammatory cytokines increase mitochondrial damage in motoneuronal cells expressing mutant SOD1. Neurobiol Dis. (2008) 32(3):454–60. doi: 10.1016/j.nbd.2008.08.004
126. Sajic M, Ida KK, Canning R, Gregson NA, Duchen MR, Smith KJ. Mitochondrial damage and “plugging” of transport selectively in myelinated, small-diameter axons are major early events in peripheral neuroinflammation. J Neuroinflammation. (2018) 15(1):61. doi: 10.1186/s12974-018-1094-8
127. Habash T, Saleh A, Roy Chowdhury SK, Smith DR, Fernyhough P. The proinflammatory cytokine, interleukin-17A, augments mitochondrial function and neurite outgrowth of cultured adult sensory neurons derived from normal and diabetic rats. Exp Neurol. (2015) 273:177–89. doi: 10.1016/j.expneurol.2015.08.016
128. Saleh A, Roy Chowdhury SK, Smith DR, Balakrishnan S, Tessler L, Schartner E, et al. Diabetes impairs an interleukin-1[beta]-dependent pathway that enhances neurite outgrowth through JAK/STAT3 modulation of mitochondrial bioenergetics in adult sensory neurons. Mol Brain. (2013) 6:45. doi: 10.1186/1756-6606-6-45
129. Kim HY, Chung JM, Chung K. Increased production of mitochondrial superoxide in the spinal cord induces pain behaviors in mice: the effect of mitochondrial electron transport complex inhibitors. Neurosci Lett. (2008) 447(1):87–91. doi: 10.1016/j.neulet.2008.09.041
130. Subramani K, Lu S, Warren M, Chu X, Toque HA, Caldwell RW, et al. Mitochondrial targeting by dichloroacetate improves outcome following hemorrhagic shock. Sci Rep. (2017) 7(1):2671. doi: 10.1038/s41598-017-02495-5
131. Małecki JM, Willemen HLDM, Pinto R, Ho AYY, Moen A, Kjønstad IF, et al. Lysine methylation by the mitochondrial methyltransferase FAM173B optimizes the function of mitochondrial ATP synthase. J Biol Chem. (2019) 294(4):1128–41. doi: 10.1074/jbc.RA118.005473
132. Peters MJ, Broer L, Willemen HLDM, Eiriksdottir G, Hocking LJ, Holliday KL, et al. Genome-wide association study meta-analysis of chronic widespread pain: evidence for involvement of the 5p15.2 region. Ann Rheum Dis. (2013) 72(3):427–36. doi: 10.1136/annrheumdis-2012-201742
133. Reichling DB, Levine JD. Critical role of nociceptor plasticity in chronic pain. Trends Neurosci. (2009) 32(12):611–8. doi: 10.1016/j.tins.2009.07.007
134. Yonekawa H, Akita Y. Protein kinase cε: the mitochondria-mediated signaling pathway. FEBS J. (2008) 275(16):4005–13. doi: 10.1111/j.1742-4658.2008.06558.x
135. Joseph EK, Levine JD. Multiple PKCε-dependent mechanisms mediating mechanical hyperalgesia. Pain. (2010) 150(1):17–21. doi: 10.1016/j.pain.2010.02.011
136. Sato T, O’Rourke B, Marbán E. Modulation of mitochondrial ATP-dependent K+ channels by protein kinase C. Circ Res. (1998) 83(1):110–4. doi: 10.1161/01.RES.83.1.110
137. Szewczyk A, Jarmuszkiewicz W, Kunz WS. Mitochondrial potassium channels. IUBMB Life. (2009) 61(2):134–43. doi: 10.1002/iub.155
138. Canta A, Pozzi E, Carozzi VA. Mitochondrial dysfunction in chemotherapy-induced peripheral neuropathy (CIPN). Toxics. (2015) 3(2):198–223. doi: 10.3390/toxics3020198
139. Kausar S, Wang F, Cui H. The role of mitochondria in reactive oxygen species generation and its implications for neurodegenerative diseases. Cells. (2018) 7(12):274. doi: 10.3390/cells7120274
140. Balaban RS, Nemoto S, Finkel T. Mitochondria, oxidants, and aging. Cell. (2005) 120(4):483–95. doi: 10.1016/j.cell.2005.02.001
141. Duchen MR. Mitochondria in health and disease: perspectives on a new mitochondrial biology. Mol Aspects Med. (2004) 25(4):365–451. doi: 10.1016/j.mam.2004.03.001
142. Dan Dunn J, Alvarez LA, Zhang X, Soldati T. Reactive oxygen species and mitochondria: a nexus of cellular homeostasis. Redox Biol. (2015) 6:472–85. doi: 10.1016/j.redox.2015.09.005
143. Biswas K, Alexander K, Francis MM. Reactive oxygen species: angels and demons in the life of a neuron. NeuroSci. (2022) 3(1):130–45. doi: 10.3390/neurosci3010011
144. Zorov DB, Juhaszova M, Sollott SJ. Mitochondrial reactive oxygen species (ROS) and ROS-induced ROS release. Physiol Rev. (2014) 94(3):909–50. doi: 10.1152/physrev.00026.2013
145. de Oliveira LG, Angelo YS, Iglesias AH, Peron JPS. Unraveling the link between mitochondrial dynamics and neuroinflammation. Front Immunol. (2021) 12:624919. doi: 10.3389/fimmu.2021.624919
146. Zhao Y, Liu B, Xu L, Yu S, Fu J, Wang J, et al. ROS-induced mtDNA release: the emerging messenger for communication between neurons and innate immune cells during neurodegenerative disorder progression. Antioxidants. (2021) 10(12):1917. doi: 10.3390/antiox10121917
147. Tirichen H, Yaigoub H, Xu W, Wu C, Li R, Li Y. Mitochondrial reactive oxygen species and their contribution in chronic kidney disease progression through oxidative stress. Front Physiol. (2021) 12(12):627837. doi: 10.3389/fphys.2021.627837
148. Rosen DR, Siddique T, Patterson D, Figlewicz DA, Sapp P, Hentati A, et al. Mutations in Cu/Zn superoxide dismutase gene are associated with familial amyotrophic lateral sclerosis. Nature. (1993) 362(6415):59–62. doi: 10.1038/362059a0
149. Hurwitz N, Radakovic R, Boyce E, Peryer G. Prevalence of pain in amyotrophic lateral sclerosis: a systematic review and meta-analysis. Amyotroph Lateral Scler Frontotemporal Degener. (2021) 22(7–8):449–58. doi: 10.1080/21678421.2021.1892765
150. Stanford KR, Hadley SH, Barannikov I, Ajmo JM, Bahia PK, Taylor-Clark TE. Antimycin A-induced mitochondrial dysfunction activates vagal sensory neurons via ROS-dependent activation of TRPA1 and ROS-independent activation of TRPV1. Brain Res. (2019) 1715:94–105. doi: 10.1016/j.brainres.2019.03.029
151. Nesuashvili L, Hadley SH, Bahia PK, Taylor-Clark TE. Sensory nerve terminal mitochondrial dysfunction activates airway sensory nerves via transient receptor potential (TRP) channels. Mol Pharmacol. (2013) 83(5):1007–19. doi: 10.1124/mol.112.084319
152. Hadley SH, Bahia PK, Taylor-Clark TE. Sensory nerve terminal mitochondrial dysfunction induces hyperexcitability in airway nociceptors via protein kinase C. Mol Pharmacol. (2014) 85(6):839–48. doi: 10.1124/mol.113.091272
153. Kim HY, Lee I, Chun SW, Kim HK. Reactive oxygen Species donors increase the responsiveness of dorsal horn neurons and induce mechanical hyperalgesia in rats. Neural Plast. (2015) 2015:293423. doi: 10.1155/2015/293423
154. Yowtak J, Lee KY, Kim HY, Wang J, Kim HK, Chung K, et al. Reactive oxygen species contribute to neuropathic pain by reducing spinal GABA release. Pain. (2011) 152(4):844–52. doi: 10.1016/j.pain.2010.12.034
155. Zhou H-Y, Zhang H-M, Chen S-R, Pan H-L. Increased C-fiber nociceptive input potentiates inhibitory glycinergic transmission in the spinal dorsal horn. J Pharmacol Exp Ther. (2008) 324(3):1000–10. doi: 10.1124/jpet.107.133470
156. Mitsunaga S, Hosomichi K, Okudaira Y, Nakaoka H, Suzuki Y, Kuwana M, et al. Aggregation of rare/low-frequency variants of the mitochondria respiratory chain-related proteins in rheumatoid arthritis patients. J Hum Genet. (2015) 60(8):449–54. doi: 10.1038/jhg.2015.50
157. Harty LC, Biniecka M, Fox E, O'Sullivan J, Veale DJ, Fearon U. The mutation of mitochondrial DNA is central to the pathogenesis of rheumatoid and psoriatic arthritis. Ann Rheum Dis. (2011) 70(Suppl 2):A20. doi: 10.1136/ard.2010.148965.18
158. Hroudová J, Singh N, Fišar Z. Mitochondrial dysfunctions in neurodegenerative diseases: relevance to Alzheimer’s disease. Biomed Res Int. (2014) 2014:175062. doi: 10.1155/2014/175062
159. Hao M, Tang Q, Wang B, Li Y, Ding J, Li M, et al. Resveratrol suppresses bone cancer pain in rats by attenuating inflammatory responses through the AMPK/Drp1 signaling. Acta Biochim Biophys Sin. (2020) 52(3):231–40. doi: 10.1093/abbs/gmz162
160. Ma Y, Liu S, Shu H, Crawford J, Xing Y, Tao F. Resveratrol alleviates temporomandibular joint inflammatory pain by recovering disturbed gut microbiota. Brain Behav Immun. (2020) 87:455–64. doi: 10.1016/j.bbi.2020.01.016
161. Tao L, Ding Q, Gao C, Sun X. Resveratrol attenuates neuropathic pain through balancing pro-inflammatory and anti-inflammatory cytokines release in mice. Int Immunopharmacol. (2016) 34:165–72. doi: 10.1016/j.intimp.2016.02.033
162. Tiwari V, Kuhad A, Chopra K. Suppression of neuro-inflammatory signaling cascade by tocotrienol can prevent chronic alcohol-induced cognitive dysfunction in rats. Behav Brain Res. (2009) 203(2):296–303. doi: 10.1016/j.bbr.2009.05.016
163. Tanabe M, Nagatani Y, Saitoh K, Takasu K, Ono H. Pharmacological assessments of nitric oxide synthase isoforms and downstream diversity of NO signaling in the maintenance of thermal and mechanical hypersensitivity after peripheral nerve injury in mice. Neuropharmacology. (2009) 56(3):702–8. doi: 10.1016/j.neuropharm.2008.12.003
164. Tal M. A novel antioxidant alleviates heat hyperalgesia in rats with an experimental painful peripheral neuropathy. Neuroreport. (1996) 7(8):1382–4. doi: 10.1097/00001756-199605310-00010
165. Leo M, Schmitt L-I, Küsterarent P, Kutritz A, Rassaf T, Kleinschnitz C, et al. Platinum-based drugs cause mitochondrial dysfunction in cultured dorsal root ganglion neurons. Int J Mol Sci. (2020) 21(22):8636. doi: 10.3390/ijms21228636
166. Burgess J, Ferdousi M, Gosal D, Boon C, Matsumoto K, Marshall A, et al. Chemotherapy-induced peripheral neuropathy: epidemiology, pathomechanisms and treatment. Oncol Ther. (2021) 9(2):385–450. doi: 10.1007/s40487-021-00168-y
167. Huser CAM, Davies ME. Calcium signaling leads to mitochondrial depolarization in impact-induced chondrocyte death in equine articular cartilage explants. Arthritis Rheum. (2007) 56(7):2322–34. doi: 10.1002/art.22717
168. Gunter TE, Buntinas L, Sparagna G, Eliseev R, Gunter K. Mitochondrial calcium transport: mechanisms and functions. Cell Calcium. (2000) 28(5–6):285–96. doi: 10.1054/ceca.2000.0168
169. Xu Z, Zhang D, He X, Huang Y, Shao H. Transport of calcium ions into mitochondria. Curr Genomics. (2016) 17(3):215–9. doi: 10.2174/1389202917666160202215748
170. Crompton M, Moser R, Lüdi H, Carafoli E. The interrelations between the transport of sodium and calcium in mitochondria of various mammalian tissues. Eur J Biochem. (1978) 82(1):25–31. doi: 10.1111/j.1432-1033.1978.tb11993.x
171. Pivovarova NB, Andrews SB. Calcium-dependent mitochondrial function and dysfunction in neurons. FEBS J. (2010) 277(18):3622–36. doi: 10.1111/j.1742-4658.2010.07754.x
172. Pivovarova NB, Hongpaisan J, Andrews SB, Friel DD. Depolarization-induced mitochondrial ca accumulation in sympathetic neurons: spatial and temporal characteristics. J Neurosci Off J Soc Neurosci. (1999) 19(15):6372–84. doi: 10.1523/JNEUROSCI.19-15-06372.1999
173. Montero M, Alonso MT, Carnicero E, Cuchillo-Ibáñez I, Albillos A, García AG, et al. Chromaffin-cell stimulation triggers fast millimolar mitochondrial Ca2+ transients that modulate secretion. Nat Cell Biol. (2000) 2(2):57–61. doi: 10.1038/35000001
174. Brookes PS, Yoon Y, Robotham JL, Anders MW, Sheu S-S. Calcium, ATP, and ROS: a mitochondrial love-hate triangle. Am J Physiol Physiol. (2004) 287(4):C817–33. doi: 10.1152/ajpcell.00139.2004
175. Rizzuto R, De Stefani D, Raffaello A, Mammucari C. Mitochondria as sensors and regulators of calcium signalling. Nat Rev Mol Cell Biol. (2012) 13(9):566–78. doi: 10.1038/nrm3412
176. Gleichmann M, Mattson MP. Neuronal calcium homeostasis and dysregulation. Antioxid Redox Signal. (2011) 14(7):1261–73. doi: 10.1089/ars.2010.3386
177. Baev AY, Vinokurov AY, Novikova IN, Dremin VV, Potapova EV, Abramov AY. Interaction of mitochondrial calcium and ROS in neurodegeneration. Cells. (2022) 11(4):706. doi: 10.3390/cells11040706
178. Shutov LP, Kim M-S, Houlihan PR, Medvedeva YV, Usachev YM. Mitochondria and plasma membrane Ca2+-ATPase control presynaptic Ca2+ clearance in capsaicin-sensitive rat sensory neurons. J Physiol. (2013) 591(10):2443–62. doi: 10.1113/jphysiol.2012.249219
179. Jackson JG, Thayer SA. Mitochondrial modulation of Ca2+-induced Ca2+-release in rat sensory neurons. J Neurophysiol. (2006) 96(3):1093–104. doi: 10.1152/jn.00283.2006
180. Voitenko NV. Role of calcium signalling in the development of pain syndromes. Neurophysiology. (2005) 37(2):166–71. doi: 10.1007/s11062-005-0061-z
181. Hagenston AM, Simonetti M. Neuronal calcium signaling in chronic pain. Cell Tissue Res. (2014) 357(2):407–26. doi: 10.1007/s00441-014-1942-5
182. Panattoni G, Amoriello R, Memo C, Thalhammer A, Ballerini C, Ballerini L. Diverse inflammatory threats modulate astrocytes Ca2+ signaling via connexin43 hemichannels in organotypic spinal slices. Mol Brain. (2021) 14(1):159. doi: 10.1186/s13041-021-00868-6
183. Nita II, Caspi Y, Gudes S, Fishman D, Lev S, Hersfinkel M, et al. Privileged crosstalk between TRPV1 channels and mitochondrial calcium shuttling machinery controls nociception. Biochim Biophys Acta. (2016) 1863(12):2868–80. doi: 10.1016/j.bbamcr.2016.09.009
184. Kim HY, Lee KY, Lu Y, Wang J, Cui L, Kim SJ, et al. Mitochondrial Ca(2+) uptake is essential for synaptic plasticity in pain. J Neurosci Off J Soc Neurosci. (2011) 31(36):12982–91. doi: 10.1523/JNEUROSCI.3093-11.2011
185. Akhilesh , Uniyal A, Gadepalli A, Tiwari V, Allani M, Chouhan D, et al. Unlocking the potential of TRPV1 based siRNA therapeutics for the treatment of chemotherapy-induced neuropathic pain. Life Sci. (2022) 288:120187. doi: 10.1016/j.lfs.2021.120187
186. Christoph T, Grünweller A, Mika J, Schäfer MK-H, Wade EJ, Weihe E, et al. Silencing of vanilloid receptor TRPV1 by RNAi reduces neuropathic and visceral pain in vivo. Biochem Biophys Res Commun. (2006) 350(1):238–43. doi: 10.1016/j.bbrc.2006.09.037
187. Lee JH, Ji H, Ko S-G, Kim W. JI017 Attenuates oxaliplatin-induced cold allodynia via spinal TRPV1 and astrocytes inhibition in mice. Int J Mol Sci. (2021) 22(16):8811. doi: 10.3390/ijms22168811
188. Wang Z, Ling D, Wu C, Han J, Zhao Y. Baicalin prevents the up-regulation of TRPV1 in dorsal root ganglion and attenuates chronic neuropathic pain. Vet Med Sci. (2020) 6(4):1034–40. doi: 10.1002/vms3.318
189. Kelley N, Jeltema D, Duan Y, He Y. The NLRP3 inflammasome: an overview of mechanisms of activation and regulation. Int J Mol Sci. (2019) 20(13):3328. doi: 10.3390/ijms20133328
190. Liu Q, Zhang D, Hu D, Zhou X, Zhou Y. The role of mitochondria in NLRP3 inflammasome activation. Mol Immunol. (2018) 103:115–24. doi: 10.1016/j.molimm.2018.09.010
191. Billingham LK, Stoolman JS, Vasan K, Rodriguez AE, Poor TA, Szibor M, et al. Mitochondrial electron transport chain is necessary for NLRP3 inflammasome activation. Nat Immunol. (2022) 23(5):692–704. doi: 10.1038/s41590-022-01185-3
192. Chen R, Yin C, Fang J, Liu B. The NLRP3 inflammasome: an emerging therapeutic target for chronic pain. J Neuroinflammation. (2021) 18(1):84. doi: 10.1186/s12974-021-02131-0
193. Panicker N, Kam T-I, Neifert S, Hinkle J, Mao X, Karuppagounder S, et al. NLRP3 Inflammasome activation in dopamine neurons contributes to neurodegeneration in Parkinson’s disease. FASEB J. (2020) 34(S1):1. doi: 10.1096/fasebj.2020.34.s1.01881
194. Chen K-P, Hua K-F, Tsai F-T, Lin T-Y, Cheng C-Y, Yang D-I, et al. A selective inhibitor of the NLRP3 inflammasome as a potential therapeutic approach for neuroprotection in a transgenic mouse model of huntington’s disease. J Neuroinflammation. (2022) 19(1):56. doi: 10.1186/s12974-022-02419-9
195. von Herrmann KM, Salas LA, Martinez EM, Young AL, Howard JM, Feldman MS, et al. NLRP3 expression in mesencephalic neurons and characterization of a rare NLRP3 polymorphism associated with decreased risk of Parkinson’s disease. NPJ Park Dis. (2018) 4(1):24. doi: 10.1038/s41531-018-0061-5
196. Ducza L, Szücs P, Hegedűs K, Bakk E, Gajtkó A, Wéber I, et al. NLRP2 is overexpressed in spinal astrocytes at the peak of mechanical pain sensitivity during complete freund adjuvant-induced persistent pain. Int J Mol Sci. (2021) 22(21):11408. doi: 10.3390/ijms222111408
197. Starobova H, Nadar EI, Vetter I. The NLRP3 inflammasome: role and therapeutic potential in pain treatment. Front Physiol. (2020) 11:1016. doi: 10.3389/fphys.2020.01016
198. Xiang H-C, Lin L-X, Hu X-F, Zhu H, Li H-P, Zhang R-Y, et al. AMPK activation attenuates inflammatory pain through inhibiting NF-κB activation and IL-1β expression. J Neuroinflammation. (2019) 16(1):34. doi: 10.1186/s12974-019-1411-x
199. Vasileiou PVS, Evangelou K, Vlasis K, Fildisis G, Panayiotidis MI, Chronopoulos E, et al. Mitochondrial homeostasis and cellular senescence. Cells. (2019) 8(7):686. doi: 10.3390/cells8070686
200. Cardanho-Ramos C, Morais VA. Mitochondrial biogenesis in neurons: how and where. Int J Mol Sci. (2021) 22(23):13059. doi: 10.3390/ijms222313059
201. Martinez-Vicente M. Neuronal mitophagy in neurodegenerative diseases. Front Mol Neurosci. (2017) 10:64. doi: 10.3389/fnmol.2017.00064
202. Lopes DM, Cater HL, Thakur M, Wells S, McMahon SB. A refinement to the formalin test in mice. F1000Res. (2019) 8:891. doi: 10.12688/f1000research.18338.2
203. Doxaki C, Palikaras K. Neuronal mitophagy: friend or foe? Front Cell Dev Biol. (2021) 8:611938. doi: 10.3389/fcell.2020.611938
204. Zaninello M, Palikaras K, Naon D, Iwata K, Herkenne S, Quintana-Cabrera R, et al. Inhibition of autophagy curtails visual loss in a model of autosomal dominant optic atrophy. Nat Commun. (2020) 11(1):4029. doi: 10.1038/s41467-020-17821-1
205. Park H, Chung KM, An H-K, Gim J-E, Hong J, Woo H, et al. Parkin promotes mitophagic cell death in adult hippocampal neural stem cells following insulin withdrawal. Front Mol Neurosci. (2019) 12:46. doi: 10.3389/fnmol.2019.00046
206. Gureev AP, Shaforostova EA, Popov VN. Regulation of mitochondrial biogenesis as a way for active longevity: interaction between the Nrf2 and PGC-1α signaling pathways. Front Genet. (2019) 10:435. doi: 10.3389/fgene.2019.00435
207. Chen C, Turnbull DM, Reeve AK. Mitochondrial dysfunction in Parkinson’s disease-cause or consequence? Biology. (2019) 8(2):38. doi: 10.3390/biology8020038
208. Russe OQ, Möser CV, Kynast KL, King TS, Stephan H, Geisslinger G, et al. Activation of the AMP-activated protein kinase reduces inflammatory nociception. J Pain. (2013) 14(11):1330–40. doi: 10.1016/j.jpain.2013.05.012
209. Ling Y-Z, Li Z-Y, Ou-Yang H-D, Ma C, Wu S-L, Wei J-Y, et al. The inhibition of spinal synaptic plasticity mediated by activation of AMP-activated protein kinase signaling alleviates the acute pain induced by oxaliplatin. Exp Neurol. (2017) 288:85–93. doi: 10.1016/j.expneurol.2016.11.009
210. Song H, Han Y, Pan C, Deng X, Dai W, Hu L, et al. Activation of adenosine monophosphate-activated protein kinase suppresses neuroinflammation and ameliorates bone cancer pain: involvement of inhibition on mitogen-activated protein kinase. Anesthesiology. (2015) 123(5):1170–85. doi: 10.1097/ALN.0000000000000856
211. Cao X-J, Wu R, Qian H-Y, Chen X, Zhu H-Y, Xu G-Y, et al. Metformin attenuates diabetic neuropathic pain via AMPK/NF-κB signaling pathway in dorsal root ganglion of diabetic rats. Brain Res. (2021) 1772:147663. doi: 10.1016/j.brainres.2021.147663
212. Melemedjian OK, Asiedu MN, Tillu DV, Sanoja R, Yan J, Lark A, et al. Targeting adenosine monophosphate-activated protein kinase (AMPK) in preclinical models reveals a potential mechanism for the treatment of neuropathic pain. Mol Pain. (2011) 7:70. doi: 10.1186/1744-8069-7-70
213. Mejia GL, Asiedu MN, Hitoshi Y, Dussor G, Price TJ. The potent, indirect adenosine monophosphate- activated protein kinase activator R419 attenuates mitogen-activated protein kinase signaling, inhibits nociceptor excitability, and reduces pain hypersensitivity in mice. Pain Reports. (2016) 1(1):e562. doi: 10.1097/PR9.0000000000000562
214. Burton MD, Tillu DV, Mazhar K, Mejia GL, Asiedu MN, Inyang K, et al. Pharmacological activation of AMPK inhibits incision-evoked mechanical hypersensitivity and the development of hyperalgesic priming in mice. Neuroscience. (2017) 359:119–29. doi: 10.1016/j.neuroscience.2017.07.020
215. Inyang KE, McDougal TA, Ramirez ED, Williams M, Laumet G, Kavelaars A, et al. Alleviation of paclitaxel-induced mechanical hypersensitivity and hyperalgesic priming with AMPK activators in male and female mice. Neurobiol Pain. (2019) 6:100037. doi: 10.1016/j.ynpai.2019.100037
216. Mao-Ying Q-L, Kavelaars A, Krukowski K, Huo X-J, Zhou W, Price TJ, et al. The anti-diabetic drug metformin protects against chemotherapy-induced peripheral neuropathy in a mouse model. PLoS One. (2014) 9(6):e100701. doi: 10.1371/journal.pone.0100701
217. Price TJ, Das V, Dussor G. Adenosine monophosphate-activated protein kinase (AMPK) activators for the prevention, treatment and potential reversal of pathological pain. Curr Drug Targets. (2016) 17(8):908–20. doi: 10.2174/1389450116666151102095046
218. Li M-Y, Ding J-Q, Tang Q, Hao M-M, Wang B-H, Wu J, et al. SIRT1 activation by SRT1720 attenuates bone cancer pain via preventing Drp1-mediated mitochondrial fission. Biochim Biophys Acta. (2019) 1865(3):587–98. doi: 10.1016/j.bbadis.2018.12.017
219. Chan DC. Fusion and fission: interlinked processes critical for mitochondrial health. Annu Rev Genet. (2012) 46(1):265–87. doi: 10.1146/annurev-genet-110410-132529
220. Youle RJ, van der Bliek AM. Mitochondrial fission, fusion, and stress. Science. (2012) 337(6098):1062–5. doi: 10.1126/science.1219855
221. Barsoum MJ, Yuan H, Gerencser AA, Liot G, Kushnareva Y, Gräber S, et al. Nitric oxide-induced mitochondrial fission is regulated by dynamin-related GTPases in neurons. EMBO J. (2006) 25(16):3900–11. doi: 10.1038/sj.emboj.7601253
222. Chiang H, Ohno N, Hsieh Y-L, Mahad DJ, Kikuchi S, Komuro H, et al. Mitochondrial fission augments capsaicin-induced axonal degeneration. Acta Neuropathol. (2015) 129(1):81–96. doi: 10.1007/s00401-014-1354-3
223. Cho D-H, Nakamura T, Fang J, Cieplak P, Godzik A, Gu Z, et al. S-Nitrosylation of Drp1 mediates beta-amyloid-related mitochondrial fission and neuronal injury. Science. (2009) 324(5923):102–5. doi: 10.1126/science.1171091
224. Ferrari LF, Chum A, Bogen O, Reichling DB, Levine JD. Role of Drp1, a key mitochondrial fission protein, in neuropathic pain. J Neurosci. (2011) 31(31):11404–10. doi: 10.1523/JNEUROSCI.2223-11.2011
225. Henry SC, Schmidt EA, Fessler MB, Taylor GA. Palmitoylation of the immunity related GTPase, Irgm1: impact on membrane localization and ability to promote mitochondrial fission. PLoS One. (2014) 9(4):e95021. doi: 10.1371/journal.pone.0095021
226. Kathayat RS, Cao Y, Elvira PD, Sandoz PA, Zaballa M-E, Springer MZ, et al. Active and dynamic mitochondrial S-depalmitoylation revealed by targeted fluorescent probes. Nat Commun. (2018) 9(1):334. doi: 10.1038/s41467-017-02655-1
227. Lv C, Hu H-Y, Zhao L, Zheng H, Luo X-Z, Zhang J. Intrathecal SRT1720, a SIRT1 agonist, exerts anti-hyperalgesic and anti-inflammatory effects on chronic constriction injury-induced neuropathic pain in rats. Int J Clin Exp Med. (2015) 8(5):7152–9. PMCID: PMC4509198, PMID: 26221253
228. Wendt MMN, de Sá-Nakanishi AB, de Castro Ghizoni CV, Bersani Amado CA, Peralta RM, Bracht A, et al. Oxidative state and oxidative metabolism in the brain of rats with adjuvant-induced arthritis. Exp Mol Pathol. (2015) 98(3):549–57. doi: 10.1016/j.yexmp.2015.04.002
229. Lin M, Liu N, Qin Z, Wang Y. Mitochondrial-derived damage-associated molecular patterns amplify neuroinflammation in neurodegenerative diseases. Acta Pharmacol Sin. (2022) 43:2439–47. doi: 10.1038/s41401-022-00879-6
230. Zheng Y-R, Zhang X-N, Chen Z. Mitochondrial transport serves as a mitochondrial quality control strategy in axons: implications for central nervous system disorders. CNS Neurosci Ther. (2019) 25(7):876–86. doi: 10.1111/cns.13122
231. Ma H, Cai Q, Lu W, Sheng Z-H, Mochida S. KIF5B motor adaptor syntabulin maintains synaptic transmission in sympathetic neurons. J Neurosci. (2009) 29(41):13019–29. doi: 10.1523/JNEUROSCI.2517-09.2009
232. López-Doménech G, Higgs NF, Vaccaro V, Roš H, Arancibia-Cárcamo IL, MacAskill AF, et al. Loss of dendritic complexity precedes neurodegeneration in a mouse model with disrupted mitochondrial distribution in mature dendrites. Cell Rep. (2016) 17(2):317–27. doi: 10.1016/j.celrep.2016.09.004
233. Zhou B, Yu P, Lin M-Y, Sun T, Chen Y, Sheng Z-H. Facilitation of axon regeneration by enhancing mitochondrial transport and rescuing energy deficits. J Cell Biol. (2016) 214(1):103–19. doi: 10.1083/jcb.201605101
234. Chen Y, Sheng Z-H. Kinesin-1-syntaphilin coupling mediates activity-dependent regulation of axonal mitochondrial transport. J Cell Biol. (2013) 202(2):351–64. doi: 10.1083/jcb.201302040
235. Errea O, Moreno B, Gonzalez-Franquesa A, Garcia-Roves PM, Villoslada P. The disruption of mitochondrial axonal transport is an early event in neuroinflammation. J Neuroinflammation. (2015) 12(1):152. doi: 10.1186/s12974-015-0375-8
236. Sakloth F, Manouras L, Avrampou K, Mitsi V, Serafini RA, Pryce KD, et al. HDAC6-selective Inhibitors decrease nerve-injury and inflammation-associated mechanical hypersensitivity in mice. Psychopharmacology. (2020) 237(7):2139–49. doi: 10.1007/s00213-020-05525-9
237. Vishwakarma S, Iyer LR, Muley M, Singh PK, Shastry A, Saxena A, et al. Tubastatin, a selective histone deacetylase 6 inhibitor shows anti-inflammatory and anti-rheumatic effects. Int Immunopharmacol. (2013) 16(1):72–8. doi: 10.1016/j.intimp.2013.03.016
238. Chen C, Liu A, Lu Q, Luo L, Li J, Ke J, et al. HDAC6 inhibitor ACY-1215 improves neuropathic pain and its comorbidities in rats of peripheral nerve injury by regulating neuroinflammation. Chem Biol Interact. (2022) 353:109803. doi: 10.1016/j.cbi.2022.109803
239. Song Y, Qin L, Yang R, Yang F, Kenechukwu NA, Zhao X, et al. Inhibition of HDAC6 alleviating lipopolysaccharide-induced p38MAPK phosphorylation and neuroinflammation in mice. Pharm Biol. (2019) 57(1):263–8. doi: 10.1080/13880209.2018.1563620
240. Ran J, Zhou J. Targeted inhibition of histone deacetylase 6 in inflammatory diseases. Thorac Cancer. (2019) 10(3):405–12. doi: 10.1111/1759-7714.12974
241. Oh BR, Suh D-H, Bae D, Ha N, Il CY, Yoo HJ, et al. Therapeutic effect of a novel histone deacetylase 6 inhibitor, CKD-L, on collagen-induced arthritis in vivo and regulatory T cells in rheumatoid arthritis in vitro. Arthritis Res Ther. (2017) 19(1):154. doi: 10.1186/s13075-017-1357-2
242. Miller RE, Malfait A-M. Osteoarthritis pain: what are we learning from animal models? Best Pract Res Clin Rheumatol. (2017) 31(5):676–87. doi: 10.1016/j.berh.2018.03.003
243. Wang Y, Shi Y, Huang Y, Liu W, Cai G, Huang S, et al. Resveratrol mediates mechanical allodynia through modulating inflammatory response via the TREM2-autophagy axis in SNI rat model. J Neuroinflammation. (2020) 17(1):311. doi: 10.1186/s12974-020-01991-2
244. Chen O, Donnelly CR, Ji R-R. Regulation of pain by neuro-immune interactions between macrophages and nociceptor sensory neurons. Curr Opin Neurobiol. (2020) 62:17–25. doi: 10.1016/j.conb.2019.11.006
245. Wolff KJ, Ramakrishnan PS, Brouillette MJ, Journot BJ, McKinley TO, Buckwalter JA, et al. Mechanical stress and ATP synthesis are coupled by mitochondrial oxidants in articular cartilage. J Orthop Res. (2013) 31(2):191–6. doi: 10.1002/jor.22223
246. Valcárcel-Ares MN, Riveiro-Naveira RR, Vaamonde-García C, Loureiro J, Hermida-Carballo L, Blanco FJ, et al. Mitochondrial dysfunction promotes and aggravates the inflammatory response in normal human synoviocytes. Rheumatology. (2014) 53(7):1332–43. doi: 10.1093/rheumatology/keu016
247. St-Jacques B, Ma W. Prostaglandin E2/EP4 signalling facilitates EP4 receptor externalization in primary sensory neurons in vitro and in vivo. Pain. (2013) 154(2):313–23. doi: 10.1016/j.pain.2012.11.005
248. Shen C, Cai G-Q, Peng J-P, Chen X-D. Autophagy protects chondrocytes from glucocorticoids-induced apoptosis via ROS/akt/FOXO3 signaling. Osteoarthr Cartil. (2015) 23(12):2279–87. doi: 10.1016/j.joca.2015.06.020
249. Pemmari A, Leppänen T, Hämäläinen M, Moilanen T, Vuolteenaho K, Moilanen E. Widespread regulation of gene expression by glucocorticoids in chondrocytes from patients with osteoarthritis as determined by RNA-seq. Arthritis Res Ther. (2020) 22(1):271. doi: 10.1186/s13075-020-02289-7
250. Coleman MC, Goetz JE, Brouillette MJ, Seol D, Willey MC, Petersen EB, et al. Targeting mitochondrial responses to intra-articular fracture to prevent posttraumatic osteoarthritis. Sci Transl Med. (2018) 10(427):eaan5372. doi: 10.1126/scitranslmed.aan5372
251. Wang Z-M, Chen Y-C, Wang D-P. Resveratrol, a natural antioxidant, protects monosodium iodoacetate-induced osteoarthritic pain in rats. Biomed Pharmacother. (2016) 83:763–70. doi: 10.1016/j.biopha.2016.06.050
252. Andreev-Andrievskiy AA, Kolosova NG, Stefanova NA, Lovat MV, Egorov MV, Manskikh VN, et al. Efficacy of mitochondrial antioxidant plastoquinonyl-decyl-triphenylphosphonium bromide (SkQ1) in the rat model of autoimmune arthritis. Oxid Med Cell Longev. (2016) 2016:8703645. doi: 10.1155/2016/8703645
253. Pecchi E, Priam S, Gosset M, Pigenet A, Sudre L, Laiguillon M-C, et al. Induction of nerve growth factor expression and release by mechanical and inflammatory stimuli in chondrocytes: possible involvement in osteoarthritis pain. Arthritis Res Ther. (2014) 16(1):R16. doi: 10.1186/ar4443
254. Malfait A-M, Miller RE, Miller RJ. Basic mechanisms of pain in osteoarthritis: experimental observations and new perspectives. Rheum Dis Clin North Am. (2021) 47(2):165–80. doi: 10.1016/j.rdc.2020.12.002
255. Gorgoulis VG, Pefani D-E, Pateras IS, Trougakos IP. Integrating the DNA damage and protein stress responses during cancer development and treatment. J Pathol. (2018) 246(1):12–40. doi: 10.1002/path.5097
256. Muralidharan A, Sotocinal SG, Yousefpour N, Akkurt N, Lima LV, Tansley S, et al. Long-term male-specific chronic pain via telomere- and p53 mediated spinal cord cellular senescence. J Clin Invest. (2022) 132(8):e151817. doi: 10.1172/JCI151817
257. Jeon OH, David N, Campisi J, Elisseeff JH. Senescent cells and osteoarthritis: a painful connection. J Clin Invest. (2018) 128(4):1229–37. doi: 10.1172/JCI95147
258. Acklin S, Zhang M, Du W, Zhao X, Plotkin M, Chang J, et al. Depletion of senescent-like neuronal cells alleviates cisplatin-induced peripheral neuropathy in mice. Sci Rep. (2020) 10(1):14170. doi: 10.1038/s41598-020-71042-6
259. Tomimatsu K, Bihary D, Olan I, Parry AJ, Schoenfelder S, Chan ASL, et al. Locus-specific induction of gene expression from heterochromatin loci during cellular senescence. Nat Aging. (2022) 2(1):31–45. doi: 10.1038/s43587-021-00147-y
260. Acosta JC, Banito A, Wuestefeld T, Georgilis A, Janich P, Morton JP, et al. A complex secretory program orchestrated by the inflammasome controls paracrine senescence. Nat Cell Biol. (2013) 15(8):978–90. doi: 10.1038/ncb2784
261. Plater-Zyberk C, Joosten LA, Helsen MM, Sattonnet-Roche P, Siegfried C, Alouani S, et al. Therapeutic effect of neutralizing endogenous IL-18 activity in the collagen-induced model of arthritis. J Clin Invest. (2001) 108(12):1825–32. doi: 10.1172/JCI200112097
262. Miyoshi K, Obata K, Kondo T, Okamura H, Noguchi K. Interleukin-18-mediated microglia/astrocyte interaction in the spinal cord enhances neuropathic pain processing after nerve injury. J Neurosci Off J Soc Neurosci. (2008) 28(48):12775–87. doi: 10.1523/JNEUROSCI.3512-08.2008
263. Ebbinghaus M, Uhlig B, Richter F, von Banchet GS, Gajda M, Bräuer R, et al. The role of interleukin-1β in arthritic pain: main involvement in thermal, but not mechanical, hyperalgesia in rat antigen-induced arthritis. Arthritis Rheum. (2012) 64(12):3897–907. doi: 10.1002/art.34675
264. Lee Y, Park Y, Nam H, Lee J-W, Yu S-W. Translocator protein (TSPO): the new story of the old protein in neuroinflammation. BMB Rep. (2020) 53(1):20–7. doi: 10.5483/BMBRep.2020.53.1.273
265. Betlazar C, Middleton RJ, Banati R, Liu G-J. The translocator protein (TSPO) in mitochondrial bioenergetics and immune processes. Cells. (2020) 9(2):512. doi: 10.3390/cells9020512
266. Rupprecht R, Papadopoulos V, Rammes G, Baghai TC, Fan J, Akula N, et al. Translocator protein (18 kDa) (TSPO) as a therapeutic target for neurological and psychiatric disorders. Nat Rev Drug Discov. (2010) 9(12):971–88. doi: 10.1038/nrd3295
267. Grimsholm O, Guo Y, Ny T, Forsgren S. Expression patterns of neurotrophins and neurotrophin receptors in articular chondrocytes and inflammatory infiltrates in knee joint arthritis. Cells Tissues Orgas. (2008) 188(3):299–309. doi: 10.1159/000121432
268. Barthel C, Yeremenko N, Jacobs R, Schmidt RE, Bernateck M, Zeidler H, et al. Nerve growth factor and receptor expression in rheumatoid arthritis and spondyloarthritis. Arthritis Res Ther. (2009) 11(3):R82. doi: 10.1186/ar2716
269. Armijo-Weingart L, Ketschek A, Sainath R, Pacheco A, Smith GM, Gallo G. Neurotrophins induce fission of mitochondria along embryonic sensory axons. Elife. (2019) 8:e49494. doi: 10.7554/eLife.49494
270. Karamercan MA, Weiss SL, Villarroel JPP, Guan Y, Werlin E, Figueredo R, et al. Can peripheral blood mononuclear cells be used as a proxy for mitochondrial dysfunction in vital organs during hemorrhagic shock and resuscitation? Shock. (2013) 40(6):476–84. doi: 10.1097/SHK.0000000000000026
271. Villarroel JPP, Guan Y, Werlin E, Selak MA, Becker LB, Sims CA. Hemorrhagic shock and resuscitation are associated with peripheral blood mononuclear cell mitochondrial dysfunction and immunosuppression. J Trauma Acute Care Surg. (2013) 75(1):24–31. doi: 10.1097/TA.0b013e3182988b1f
272. Leuner K, Pantel J, Frey C, Schindowski K, Schulz K, Wegat T, et al. Enhanced apoptosis, oxidative stress and mitochondrial dysfunction in lymphocytes as potential biomarkers for Alzheimer’s disease. J Neural Transm Suppl. (2007) 72:207–15.
273. Leuner K, Schulz K, Schütt T, Pantel J, Prvulovic D, Rhein V, et al. Peripheral mitochondrial dysfunction in Alzheimer’s disease: focus on lymphocytes. Mol Neurobiol. (2012) 46(1):194–204. doi: 10.1007/s12035-012-8300-y
274. West AP. Mitochondrial dysfunction as a trigger of innate immune responses and inflammation. Toxicology. (2017) 391:54–63. doi: 10.1016/j.tox.2017.07.016
275. Tall AR, Yvan-Charvet L. Cholesterol, inflammation and innate immunity. Nat Rev Immunol. (2015) 15(2):104–16. doi: 10.1038/nri3793
276. Jenko B, Praprotnik S, Tomšić M, Dolžan V. NLRP3 And CARD8 polymorphisms influence higher disease activity in rheumatoid arthritis. J Med Biochem. (2016) 35(3):319–23. doi: 10.1515/jomb-2016-0008
277. Tinker RJ, Lim AZ, Stefanetti RJ, McFarland R. Current and emerging clinical treatment in mitochondrial disease. Mol Diagn Ther. (2021) 25(2):181–206. doi: 10.1007/s40291-020-00510-6
278. Murphy MP, Hartley RC. Mitochondria as a therapeutic target for common pathologies. Nat Rev Drug Discov. (2018) 17(12):865–86. doi: 10.1038/nrd.2018.174
279. Leow HW, Koscielniak M, Williams L, Saunders PTK, Daniels J, Doust AM, et al. Dichloroacetate as a possible treatment for endometriosis-associated pain: a single-arm open-label exploratory clinical trial (EPiC). Pilot Feasibility Stud. (2021) 7(1):67. doi: 10.1186/s40814-021-00797-0
280. Katsyuba E, Romani M, Hofer D, Auwerx J. NAD(+) homeostasis in health and disease. Nat Metab. (2020) 2(1):9–31. doi: 10.1038/s42255-019-0161-5
281. Trammell SAJ, Weidemann BJ, Chadda A, Yorek MS, Holmes A, Coppey LJ, et al. Nicotinamide riboside opposes type 2 diabetes and neuropathy in mice. Sci Rep. (2016) 6(1):26933. doi: 10.1038/srep26933
282. Cantó C, Menzies KJ, Auwerx J. NAD(+) metabolism and the control of energy homeostasis: a balancing act between mitochondria and the nucleus. Cell Metab. (2015) 22(1):31–53. doi: 10.1016/j.cmet.2015.05.023
283. Hamity MV, White SR, Blum C, Gibson-Corley KN, Hammond DL. Nicotinamide riboside relieves paclitaxel-induced peripheral neuropathy and enhances suppression of tumor growth in tumor-bearing rats. Pain. (2020) 161(10):2364–75. doi: 10.1097/j.pain.0000000000001924
284. The common form of niacin amide deficiency disease: aniacinamidosis. J Am Med Assoc. (1943) 123(11):732. doi: 10.1001/jama.1943.02840460066035
285. Hoffer A. Treatment of arthritis by nicotinic acid and nicotinamide. Can Med Assoc J. (1959) 81(4):235–8.
286. Peclat TR, Shi B, Varga J, Chini EN. The NADase enzyme CD38: an emerging pharmacological target for systemic sclerosis, systemic lupus erythematosus and rheumatoid arthritis. Curr Opin Rheumatol. (2020) 32(6):488–96. doi: 10.1097/BOR.0000000000000737
287. Jonas WB, Rapoza CP, Blair WF. The effect of niacinamide on osteoarthritis: a pilot study. Inflamm Res Off J Eur Histamine Res Soc. (1996) 45(7):330–4.
288. Martens CR, Denman BA, Mazzo MR, Armstrong ML, Reisdorph N, McQueen MB, et al. Chronic nicotinamide riboside supplementation is well-tolerated and elevates NAD(+) in healthy middle-aged and older adults. Nat Commun. (2018) 9(1):1286. doi: 10.1038/s41467-018-03421-7
289. Mehmel M, Jovanović N, Spitz U. Nicotinamide riboside-the current state of research and therapeutic uses. Nutrients. (2020) 12(6):1616. doi: 10.3390/nu12061616
290. Moneim AEA. Oxidant/antioxidant imbalance and the risk of Alzheimer’s disease. Curr Alzheimer Res. (2015) 12(4):335–49. doi: 10.2174/1567205012666150325182702
291. Cui X, Lin Q, Liang Y. Plant-derived antioxidants protect the nervous system from aging by inhibiting oxidative stress. Front Aging Neurosci. (2020) 12:209. doi: 10.3389/fnagi.2020.00209
292. Marouf BH, Hussain SA, Ali ZS, Ahmmad RS. Resveratrol supplementation reduces pain and inflammation in knee osteoarthritis patients treated with meloxicam: a randomized placebo-controlled study. J Med Food. (2018) 21(21):1253–59. doi: 10.1089/jmf.2017.4176
293. Hussain SA, Marouf BH, Ali ZS, Ahmmad RS. Efficacy and safety of co-administration of resveratrol with meloxicam in patients with knee osteoarthritis: a pilot interventional study. Clin Interv Aging. (2018) 13:1621–30. doi: 10.2147/CIA.S172758
294. Thaung Zaw JJ, Howe PRC, Wong RHX. Long-term resveratrol supplementation improves pain perception, menopausal symptoms, and overall well-being in postmenopausal women: findings from a 24-month randomized, controlled, crossover trial. Menopause. (2021) 28(1):40–9. doi: 10.1097/GME.0000000000001643
295. Nguyen C, Boutron I, Baron G, Coudeyre E, Berenbaum F, Poiraudeau S, et al. Evolution of pain at 3 months by oral resveratrol in knee osteoarthritis (ARTHROL): protocol for a multicentre randomised double-blind placebo-controlled trial. BMJ Open. (2017) 7(9):e017652. doi: 10.1136/bmjopen-2017-017652
296. Klück V, Jansen TLTA, Janssen M, Comarniceanu A, Efdé M, Tengesdal IW, et al. Dapansutrile, an oral selective NLRP3 inflammasome inhibitor, for treatment of gout flares: an open-label, dose-adaptive, proof-of-concept, phase 2a trial. Lancet Rheumatol. (2020) 2(5):e270–80. doi: 10.1016/S2665-9913(20)30065-5
297. Fenini G, Contassot E, French LE. Potential of IL-1, IL-18 and inflammasome inhibition for the treatment of inflammatory skin diseases. Front Pharmacol. (2017) 8:278. doi: 10.3389/fphar.2017.00278
298. Atemnkeng Ntam V, Klein A, Horneff G. Safety and efficacy of anakinra as first-line or second-line therapy for systemic onset juvenile idiopathic arthritis - data from the German BIKER registry. Expert Opin Drug Saf. (2021) 20(1):93–100. doi: 10.1080/14740338.2021.1843631
299. Shin JI, Lee KH, Joo YH, Lee JM, Jeon J, Jung HJ, et al. Inflammasomes and autoimmune and rheumatic diseases: a comprehensive review. J Autoimmun. (2019) 103:102299. doi: 10.1016/j.jaut.2019.06.010
300. Braddock M, Quinn A. Targeting IL-1 in inflammatory disease: new opportunities for therapeutic intervention. Nat Rev Drug Discov. (2004) 3(4):330–40. doi: 10.1038/nrd1342
301. Li X, Li Y, Zhang Z, Bian Q, Gao Z, Zhang S. Mild hypothermia facilitates mitochondrial transfer from astrocytes to injured neurons during oxygen-glucose deprivation/reoxygenation. Neurosci Lett. (2021) 756:135940. doi: 10.1016/j.neulet.2021.135940
302. Vallabhaneni KC, Haller H, Dumler I. Vascular smooth muscle cells initiate proliferation of mesenchymal stem cells by mitochondrial transfer via tunneling nanotubes. Stem Cells Dev. (2012) 21(17):3104–13. doi: 10.1089/scd.2011.0691
303. Marlein CR, Piddock RE, Mistry JJ, Zaitseva L, Hellmich C, Horton RH, et al. CD38-driven mitochondrial trafficking promotes bioenergetic plasticity in multiple myeloma. Cancer Res. (2019) 79(9):2285–97. doi: 10.1158/0008-5472.CAN-18-0773
304. Zhang Y, Yu Z, Jiang D, Liang X, Liao S, Zhang Z, et al. iPSC-MSCs with high intrinsic MIRO1 and sensitivity to TNF-α yield efficacious mitochondrial transfer to rescue anthracycline-induced cardiomyopathy. Stem Cell Rep. (2016) 7(4):749–63. doi: 10.1016/j.stemcr.2016.08.009
305. He K, Shi X, Zhang X, Dang S, Ma X, Liu F, et al. Long-distance intercellular connectivity between cardiomyocytes and cardiofibroblasts mediated by membrane nanotubes. Cardiovasc Res. (2011) 92(1):39–47. doi: 10.1093/cvr/cvr189
306. Peruzzotti-Jametti L, Bernstock JD, Willis CM, Manferrari G, Rogall R, Fernandez-Vizarra E, et al. Neural stem cells traffic functional mitochondria via extracellular vesicles. PLoS Biol. (2021) 19(4):e3001166. doi: 10.1371/journal.pbio.3001166
307. Li H, Wang C, He T, Zhao T, Chen Y-Y, Shen Y-L, et al. Mitochondrial transfer from bone marrow mesenchymal stem cells to motor neurons in spinal cord injury rats via gap junction. Theranostics. (2019) 9(7):2017–35. doi: 10.7150/thno.29400
308. Boudreau LH, Duchez A-C, Cloutier N, Soulet D, Martin N, Bollinger J, et al. Platelets release mitochondria serving as substrate for bactericidal group IIA-secreted phospholipase A2 to promote inflammation. Blood. (2014) 124(14):2173–83. doi: 10.1182/blood-2014-05-573543
309. Lippert T, Borlongan CV. Prophylactic treatment of hyperbaric oxygen treatment mitigates inflammatory response via mitochondria transfer. CNS Neurosci Ther. (2019) 25(8):815–23. doi: 10.1111/cns.13124
310. Lampinen R, Belaya I, Saveleva L, Liddell JR, Rait D, Huuskonen MT, et al. Neuron-astrocyte transmitophagy is altered in Alzheimer’s disease. Neurobiol Dis. (2022) 170:105753. doi: 10.1016/j.nbd.2022.105753
311. Moschoi R, Imbert V, Nebout M, Chiche J, Mary D, Prebet T, et al. Protective mitochondrial transfer from bone marrow stromal cells to acute myeloid leukemic cells during chemotherapy. Blood. (2016) 128(2):253–64. doi: 10.1182/blood-2015-07-655860
312. Wang J, Liu X, Qiu Y, Shi Y, Cai J, Wang B, et al. Cell adhesion-mediated mitochondria transfer contributes to mesenchymal stem cell-induced chemoresistance on T cell acute lymphoblastic leukemia cells. J Hematol Oncol. (2018) 11(1):11. doi: 10.1186/s13045-018-0554-z
313. Luz-Crawford P, Hernandez J, Djouad F, Luque-Campos N, Caicedo A, Carrère-Kremer S, et al. Mesenchymal stem cell repression of Th17 cells is triggered by mitochondrial transfer. Stem Cell Res Ther. (2019) 10(1):232. doi: 10.1186/s13287-019-1307-9
314. Thomas MA, Fahey MJ, Pugliese BR, Irwin RM, Antonyak MA, Delco ML. Human mesenchymal stromal cells release functional mitochondria in extracellular vesicles. Front Bioeng Biotechnol. (2022) 10:870193. doi: 10.3389/fbioe.2022.870193
315. Huang T, Zhang T, Gao J. Targeted mitochondrial delivery: a therapeutic new era for disease treatment. J Control Release Off J Control Release Soc. (2022) 343:89–106. doi: 10.1016/j.jconrel.2022.01.025
316. Picone P, Porcelli G, Bavisotto CC, Nuzzo D, Galizzi G, Biagio PLS, et al. Synaptosomes: new vesicles for neuronal mitochondrial transplantation. J Nanobiotechnology. (2021) 19(1):6. doi: 10.1186/s12951-020-00748-6
317. Gollihue JL, Patel SP, Eldahan KC, Cox DH, Donahue RR, Taylor BK, et al. Effects of mitochondrial transplantation on bioenergetics, cellular incorporation, and functional recovery after spinal cord injury. J Neurotrauma. (2018) 35(15):1800–18. doi: 10.1089/neu.2017.5605
318. Yan C, Ma Z, Ma H, Li Q, Zhai Q, Jiang T, et al. Mitochondrial transplantation attenuates brain dysfunction in sepsis by driving microglial M2 polarization. Mol Neurobiol. (2020) 57(9):3875–90. doi: 10.1007/s12035-020-01994-3
319. Emani SM, Piekarski BL, Harrild D, Del Nido PJ, McCully JD. Autologous mitochondrial transplantation for dysfunction after ischemia-reperfusion injury. J Thorac Cardiovasc Surg. (2017) 154(1):286–9. doi: 10.1016/j.jtcvs.2017.02.018
320. Al Amir Dache Z, Otandault A, Tanos R, Pastor B, Meddeb R, Sanchez C, et al. Blood contains circulating cell-free respiratory competent mitochondria. FASEB J. (2020) 34(3):3616–30. doi: 10.1096/fj.201901917RR
321. Heller A, Brockhoff G, Goepferich A. Targeting drugs to mitochondria. Eur J Pharm Biopharm. (2012) 82(1):1–18. doi: 10.1016/j.ejpb.2012.05.014
322. Xu J, Du W, Zhao Y, Lim K, Lu L, Zhang C, et al. Mitochondria targeting drugs for neurodegenerative diseases-design, mechanism and application. Acta Pharm Sin B. (2022) 12(6):2778–89. doi: 10.1016/j.apsb.2022.03.001
323. Zinovkin RA, Zamyatnin AA. Mitochondria-targeted drugs. Curr Mol Pharmacol. (2019) 12(3):202–14. doi: 10.2174/1874467212666181127151059
324. Chen J, Zhong J, Wang L-L, Chen Y-Y. Mitochondrial transfer in cardiovascular disease: from mechanisms to therapeutic implications. Front Cardiovasc Med. (2021) 8:771298. doi: 10.3389/fcvm.2021.771298
325. Kvien TK, Uhlig T, Ødegård S, Heiberg MS. Epidemiological aspects of rheumatoid arthritis: the sex ratio. Ann N Y Acad Sci. (2006) 1069:212–22. doi: 10.1196/annals.1351.019
326. Pereira D, Peleteiro B, Araújo J, Branco J, Santos RA, Ramos E. The effect of osteoarthritis definition on prevalence and incidence estimates: a systematic review. Osteoarthr Cartil. (2011) 19(11):1270–85. doi: 10.1016/j.joca.2011.08.009
327. Siracusa R, Paola RD, Cuzzocrea S, Impellizzeri D. Fibromyalgia: pathogenesis, mechanisms, diagnosis and treatment options update. Int J Mol Sci. (2021) 22(8):3891. doi: 10.3390/ijms22083891
328. Kamitaki N, Sekar A, Handsaker RE, de Rivera H, Tooley K, Morris DL, et al. Complement genes contribute sex-biased vulnerability in diverse disorders. Nature. (2020) 582(7813):577–81. doi: 10.1038/s41586-020-2277-x
329. Falk S, Uldall M, Appel C, Ding M, Heegaard A-M. Influence of sex differences on the progression of cancer-induced bone pain. Anticancer Res. (2013) 33(5):1963–9.
330. Mapplebeck JCS, Dalgarno R, Tu Y, Moriarty O, Beggs S, Kwok CHT, et al. Microglial P2X4R-evoked pain hypersensitivity is sexually dimorphic in rats. Pain. (2018) 159(9):1752–63. doi: 10.1097/j.pain.0000000000001265
331. Rosen S, Ham B, Mogil JS. Sex differences in neuroimmunity and pain. J Neurosci Res. (2017) 95(1–2):500–8. doi: 10.1002/jnr.23831
332. Mogil JS. Sex differences in pain and pain inhibition: multiple explanations of a controversial phenomenon. Nat Rev Neurosci. (2012) 13(12):859–66. doi: 10.1038/nrn3360
333. Sharma J, Nelluru G, Wilson MA, Johnston MV, Hossain MA. Sex-specific activation of cell death signalling pathways in cerebellar granule neurons exposed to oxygen glucose deprivation followed by reoxygenation. ASN Neuro. (2011) 3(2):e00056. doi: 10.1042/AN20100032
334. Sharma J, Johnston MV, Hossain MA. Sex differences in mitochondrial biogenesis determine neuronal death and survival in response to oxygen glucose deprivation and reoxygenation. BMC Neurosci. (2014) 15:9. doi: 10.1186/1471-2202-15-9
335. Graham LC, Eaton SL, Brunton PJ, Atrih A, Smith C, Lamont DJ, et al. Proteomic profiling of neuronal mitochondria reveals modulators of synaptic architecture. Mol Neurodegener. (2017) 12(1):77. doi: 10.1186/s13024-017-0221-9
336. Börsch A, Ham DJ, Mittal N, Tintignac LA, Migliavacca E, Feige JN, et al. Molecular and phenotypic analysis of rodent models reveals conserved and species-specific modulators of human sarcopenia. Commun Biol. (2021) 4(1):194. doi: 10.1038/s42003-021-01723-z
337. Mercken EM, Capri M, Carboneau BA, Conte M, Heidler J, Santoro A, et al. Conserved and species-specific molecular denominators in mammalian skeletal muscle aging. NPJ Aging Mech Dis. (2017) 3(1):8. doi: 10.1038/s41514-017-0009-8
338. Li J, Pan L, Pembroke WG, Rexach JE, Godoy MI, Condro MC, et al. Conservation and divergence of vulnerability and responses to stressors between human and mouse astrocytes. Nat Commun. (2021) 12(1):3958. doi: 10.1038/s41467-021-24232-3
339. Mogil JS. The translatability of pain across species. Philos Trans R Soc London Ser B, Biol Sci. (2019) 374(1785):20190286. doi: 10.1098/rstb.2019.0286
340. Fischer BD, Adeyemo A, O’Leary ME, Bottaro A. Animal models of rheumatoid pain: experimental systems and insights. Arthritis Res Ther. (2017) 19(1):146. doi: 10.1186/s13075-017-1361-6
341. Ray P, Torck A, Quigley L, Wangzhou A, Neiman M, Rao C, et al. Comparative transcriptome profiling of the human and mouse dorsal root ganglia: an RNA-seq-based resource for pain and sensory neuroscience research. Pain. (2018) 159(7):1325–45. doi: 10.1097/j.pain.0000000000001217
342. Shiers S, Klein RM, Price TJ. Quantitative differences in neuronal subpopulations between mouse and human dorsal root ganglia demonstrated with RNAscope in situ hybridization. Pain. (2020) 161(10):2410–24. doi: 10.1097/j.pain.0000000000001973
343. O’Brien M, Philpott HT, McDougall JJ. Understanding osteoarthritis pain through animal models. Clin Exp Rheumatol. (2017) 35(5):47–52.
344. Henning A, Koning W, Fuchs A, Raaijmakers A, Bluemink JJ, van den Berg CAT, et al. 1H MRS in the human spinal cord at 7T using a dielectric waveguide transmitter, RF shimming and a high density receive array. NMR Biomed. (2016) 29(9):1231–9. doi: 10.1002/nbm.3541
345. Cropper HC, Johnson EM, Haight ES, Cordonnier SA, Chaney AM, Forman TE, et al. Longitudinal translocator protein-18 kDa-positron emission tomography imaging of peripheral and central myeloid cells in a mouse model of complex regional pain syndrome. Pain. (2019) 160(9):2136–48. doi: 10.1097/j.pain.0000000000001607
Keywords: mitochondria, sensory neurons, inflammation, neuro-inflammation, chronic pain, rheumatic disease, neuropathy
Citation: Silva Santos Ribeiro P, Willemen HLDM and Eijkelkamp N (2022) Mitochondria and sensory processing in inflammatory and neuropathic pain. Front. Pain Res. 3:1013577. doi: 10.3389/fpain.2022.1013577
Received: 7 August 2022; Accepted: 26 September 2022;
Published: 17 October 2022.
Edited by:
Marzia Malcangio, King's College London, United KingdomReviewed by:
Vinod Tiwari, Indian Institute of Technology (BHU), India© 2022 Silva Santos Ribeiro, Willemen and Eijkelkamp. This is an open-access article distributed under the terms of the Creative Commons Attribution License (CC BY). The use, distribution or reproduction in other forums is permitted, provided the original author(s) and the copyright owner(s) are credited and that the original publication in this journal is cited, in accordance with accepted academic practice. No use, distribution or reproduction is permitted which does not comply with these terms.
*Correspondence: Niels Eijkelkamp bi5laWprZWxrYW1wQHVtY3V0cmVjaHQubmw=
Specialty Section: This article was submitted to Pain Mechanisms, a section of the journal Frontiers in Pain Research
Disclaimer: All claims expressed in this article are solely those of the authors and do not necessarily represent those of their affiliated organizations, or those of the publisher, the editors and the reviewers. Any product that may be evaluated in this article or claim that may be made by its manufacturer is not guaranteed or endorsed by the publisher.
Research integrity at Frontiers
Learn more about the work of our research integrity team to safeguard the quality of each article we publish.