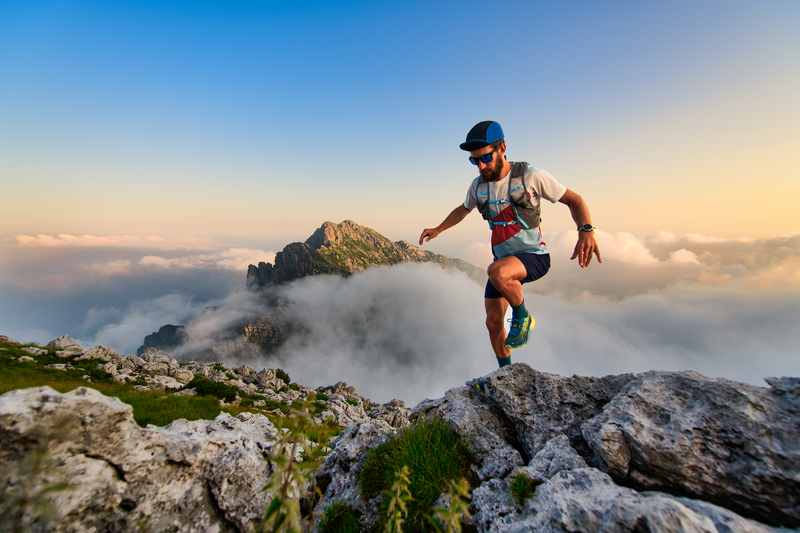
95% of researchers rate our articles as excellent or good
Learn more about the work of our research integrity team to safeguard the quality of each article we publish.
Find out more
REVIEW article
Front. Oral. Health , 14 June 2023
Sec. Oral Infections and Microbes
Volume 4 - 2023 | https://doi.org/10.3389/froh.2023.1210200
This article is part of the Research Topic Salivary Biomarkers for Oral and Systemic Diseases View all 5 articles
The purpose of this narrative review is to highlight the importance of microbial metabolites in the pathogenesis of periodontal diseases. These diseases, involving gingivitis and periodontitis are inflammatory conditions initiated and maintained by the polymicrobial dental plaque/biofilm. Gingivitis is a reversible inflammatory condition while periodontitis involves also irreversible destruction of the periodontal tissues including the alveolar bone. The inflammatory response of the host is a natural reaction to the formation of plaque and the continuous release of metabolic waste products. The microorganisms grow in a nutritious and shielded niche in the periodontal pocket, protected from natural cleaning forces such as saliva. It is a paradox that the consequences of the enhanced inflammatory reaction also enable more slow-growing, fastidious, anaerobic bacteria, with often complex metabolic pathways, to colonize and thrive. Based on complex food chains, nutrient networks and bacterial interactions, a diverse microbial community is formed and established in the gingival pocket. This microbiota is dominated by anaerobic, often motile, Gram-negatives with proteolytic metabolism. Although this alternation in bacterial composition often is considered pathologic, it is a natural development that is promoted by ecological factors and not necessarily a true “dysbiosis”. Normal commensals are adapting to the gingival crevice when tooth cleaning procedures are absent. The proteolytic metabolism is highly complex and involves a number of metabolic pathways with production of a cascade of metabolites in an unspecific manner. The metabolites involve short chain fatty acids (SCFAs; formic, acetic, propionic, butyric, and valeric acid), amines (indole, scatole, cadaverine, putrescine, spermine, spermidine) and gases (NH3, CO, NO, H2S, H2). A homeostatic condition is often present between the colonizers and the host response, where continuous metabolic fluctuations are balanced by the inflammatory response. While it is well established that the effect of the dental biofilm on the host response and tissue repair is mediated by microbial metabolites, the mechanisms behind the tissue destruction (loss of clinical attachment and bone) are still poorly understood. Studies addressing the functions of the microbiota, the metabolites, and how they interplay with host tissues and cells, are therefore warranted.
The microbiota that colonizes various parts of the human body, such as skin, genitals and gastrointestinal tract, lives in a symbiotic relationship with the host (1). Each part of the gastrointestinal tract, e.g., oral cavity, pharynx, esophagus, stomach, small and large intestine, has its own site-specific core microbiome, which is regulated by the physiological and other environmental factors that characterize every ecological unit (habitat). Collectively, these microbial habitats and their genomes constitute the human microbiome, with characteristic compositions for each niche in which the microorganisms live in a symbiotic and homeostatic condition with the host, and together constitute the symbiont. The main benefits of this host-microbiome symbiosis are, among others, resistance to colonization by exogenous pathogens, support in host defense functions and anti-inflammatory properties, antioxidant activity, support in the regulation of the cardiovascular system, additional metabolic potential, and support to maintain a healthy digestive tract (2). The tissue response to the colonizers along the entire gastrointestinal tract is a normal and valuable reaction to the microbial load in each habitat. The degree of mucosal lymphoid tissue manifestation beneath the epithelial barrier of the mucosal membranes is balanced against the metabolic activity and growth of the microorganisms in each habitat (3).
The ecology of the dental biofilm (dental plaque) is developed by an establishment of a highly diversified microbiota, ecologically termed as a climax community (4). This ecology, in the gingival crevice, develops around all teeth, in all humans and even animals (with a similar anatomy and physiology) and the microbiota is typical for this niche. It is true that in populations/individuals that practice dental cleaning the bacterial load and diversity is lower, however, the number of individuals with a gingiva fulfilling the criteria for being “healthy”, with a bleeding on probing < 10%, is a minority (5). Classical experimental gingivitis studies also confirm that the establishment of a climax community within the gingival crevice in adults takes less than 14 days, although there is no time limit for the continuous and life-long maturation process, with further adaptation and reorganization of a dynamic subgingival plaque community (6–9). The response of the host, seen clinically as an inflammatory response (gingivitis), should be regarded as a natural protecting response (a normal condition) rather than pathologic, and is balanced (homeostasis) against the microbial activity and release of metabolic products from the dental biofilm in the gingival crevice.
Numerous studies have been carried out in the past in search for specific species/genera or combinations/communities that are associated with destructive periodontal disease (10–12). Unfortunately, most studies are cross-sectional using advanced molecular methods with a limited number of subjects. Further, the specific details of the sampling strategy and the clinical status of the patients can be elusive to obtain. Several species (putative periodontal pathogens, key stone periodontal pathogens) have been associated with deep (>6 mm) periodontal pockets, however, their roles in the disease process are still far from established. Morphological studies report on bacterial masses in the periodontal pockets, with an adherent part against the root surface and a non-adherent part, including a high number of motile bacteria, densely packed in the most apical part of the pocket (13, 14). Studies on the composition of the periodontal pocket microbiome/microbiota generally show a predominance of Gram-negative strictly anaerobic species using protein fermentation as their main nutritional pathway in a slightly alkaline environment (2, 15). The periodontitis microbiota is associated with an overgrowth of some microorganisms such as Porphyromonas spp. and Treponema spp. (spirochetes), resulting in an increased microbial diversity in periodontitis compared to periodontal health (16). It is possible that these bacteria constitute a higher relative risk for further periodontal breakdown, but they are commonly present in high numbers also in shallow pockets and in gingivitis cases as well, and their impact on the periodontal disease progression is therefore not well established. The term “dysbiosis” is sometimes used to refer to the changes in the composition of the microbiota in particular niches (for example, those found in deep periodontal pockets). It should, however, be borne in mind that such proportional changes, although often considered pathologic, are more likely an adaptation of oral commensals to a modified microenvironment. Dysbiosis is mainly a term that normally refers to microbial imbalance or maladaptation within the microbiome (16, 17).
In recent years the function e.g., metabolic activities of the dental biofilm community rather than its composition have gained an increasing interest in both caries and periodontitis research (18–22). The dental biofilm metabolism is extremely complex and contrasting findings from in vitro and cell culture studies are common. The metabolism of the subgingival dental biofilm and the interaction of bacterial metabolites with the host tissues is still poorly understood (23).
This paper reviews the metabolic processes in the subgingival dental biofilm and its complex interaction with the gingival tissues and inflammatory cells of the host, supporting the view of an alternative perspective on destructive periodontal disease.
The oral cavity and its microbiota should be seen as the portal entry and the functional starting point of the gastrointestinal tract. A major beneficial activity of the gastrointestinal microbiota is the fermentation of food. The processes of degradation of the polymeric components (polysaccharides, proteins, lipids, and nucleic acids) into smaller molecules (oligomers and monomers), together with inorganic ions, provide the nutrients for host cells (Figure 1). They also provide the nutrients and cell materials (polymers) for the bacteria that colonize the entire gastrointestinal tract. This is a major function also for the oral microbiota. Although there are substantial differences between the gastrointestinal microbiota and the microbiota on the teeth and tongue, there are also some similarities, e.g., complex compositions, strong microbial interactions, and the degradation of nutrients (24). Firmicutes (mainly streptococci), which are biochemically the most active species in the oral cavity due to their potent ability to ferment both carbohydrates and peptides, represents the predominant phylum in the oral resident microbiota.
Figure 1. Flow of nutrients through an anaerobic microbial community (e.g., dental biofilm), whereby all the nutrients are converted into methane (CH4) and carbon dioxide (CO2) by the concerted actions of primary and secondary fermenters, sulfate reducers and methanogenic archaea. Detailed information on bacterial species involved in various metabolic processes are found in Table 1. (Adapted from Carlsson J. Growth and nutritions as ecological factors; In Kuramitsu H.K. Ellen R.P (Eds), Oral bacterial ecology. The molecular basis. Horizon Scientific Press, Norfolk, UK. Chapter 2, pages 68-130).
Table 1. Bacterial metabolites produced during the proteolytic, amino acid degradative, and other degradative processes that occur in the periodontal pockets.
The fermentation process in the oral microbiota follows two main pathways, one for carbohydrate (sugars and sugar alcohols) and one for proteins (peptides and amino acids), both described in detail by Takahashi (18). Nutrients from ingested food, especially mono- and di-saccharides, are readily available and efficiently fermented by streptococci, also under anaerobic conditions (19). The oral microbiota is momentarily activated through the carbohydrate-rich diet, especially through intake of sugars (mono and disaccharides). This can easily be followed by measurement of acid formation (mainly lactic acid) and the subsequent pH drop (Stephan curve) (25). For the resting microbiota on mucosal membranes and dental plaque (e.g., at night during sleep), under starvation conditions, the nutrients comprise salivary glycoproteins that are degraded into oligomeric molecules through the fermentation of both carbohydrates and proteins. It is important to emphasize that even during resting and starving conditions the microbiota of various oral niches are still metabolizing and growing and continuously fluctuating in activity (26, 27).
A major metabolic activity that takes place is the oxidation processes that results in the production of hydrogen peroxide (H2O2). This process is mainly occurring in the presence of oxygen in the dental biofilm (Figure 1) (18). The main producers of H2O2 are Streptococcus spp. and it is suggested that that their production of H2O2 has an important regulatory function against anaerobes to become established on exposed surfaces such as the mucosal membranes and teeth surfaces. Thus, H2O2 is regulating the dental plaque since certain bacteria lack protection from peroxides and oxygen radicals. There are, however, many both facultative and anaerobic bacteria, that are protected from oxygen-free radicals by enzymatic antioxidants such as superoxide dismutase, glutathione peroxidase and catalase (28). Since peroxides also have toxic effects on host epithelial and other cells, saliva contains lacto-peroxidase, which convert thiocyanate (SCN−) to hypothiocyanite (OSCN−), which is strongly antibacterial. The source of cyanate is through the diet, but cyanate is present in significant concentrations also in blood. It is therefore likely that this conversion of SCN− to OSCN− also takes place in the gingival pocket by myeloperoxidase from neutrophils. Increasing inflammation may thus efficiently degrade the peroxides and thus work as antioxidants and keep the anaerobiosis in the periodontal pocket. Interestingly, smokers seem to have higher concentration of cyanate in serum and may therefore contribute to more anaerobic conditions, favoring anaerobic bacteria (29). However, little is known about the importance of myeloperoxidase in anaerobic conditions of the periodontal pocket and whether the conversion of SCN− to OSCN− have any role in the periodontal pocket ecology or if this reaction is mainly occurring in supragingival areas.
Interestingly, oral rinsing with urea, momentarily leads to degradation of the urea to ammonia (NH3) and a pH raise followed by a neutralization back to initial status within 30 min (30, 31). The degradation of urea is due to urease which is strongly produced by some Gram-negative bacteria such as Campylobacter ureolyticus, Helicobacter pylori and Haemophilus parainfluenzae and moderately by some Gram-positive bacteria such as Streptococcus spp. and Actinomyces spp (32).
Nitrite (NO−), is another metabolite formed in the oral cavity through the reduction of nitrate (NO3−) by the bacterial enzyme nitrate reductase (33). Numerous oral bacterial species such as Veillonella spp., Rothia spp., Neisseria spp, and Corynebacterium spp. share this ability to reduce nitrate. In acidic environment (stomach acid) nitrite is further reduced to nitric oxide (NO), which is strongly linked to the cardiovascular system and regulation of blood pressure (34–38). Thus, a diet rich in vegetables (that have high nitrate content), in combination with nitrate reducing oral bacteria have indirectly linked the oral nitrate-nitrite-nitric oxide pathway to systemic NO dependent effects and cardiovascular health in previous studies (39–45). Whether the reduction of nitrite to NO takes place also locally in the acidic conditions (at sugar intake) at the surface of teeth is unknown but likely (46, 47). This potential trait of the dental biofilm has been claimed to counteract the caries process (48–50). Significant amounts of nitrite, suggested to have a local antimicrobial effect, is formed after intake of nitrate rich foods. Intragastric NO production has been measured in humans in expelled air but will also contain NO produced locally in the oral cavity (51, 52). It is likely that the formation of nitrite and NO mainly occurs at the tongue, but its potential antibacterial effect here is controversial (34). Also, anti-inflammatory effects of a nitrate-rich diet have been reported for periodontal health (53). It has been reported that periodontitis recall patients presented a decrease in gingival inflammation after regular consumption of juice high in nitrate compared to a control group consuming juice without nitrate (54, 55).
Bacterial fermentation of proteins is complex (Figure 1). In the gingival area, there is absence of sugars but access to proteins/peptides from gingival crevicular fluid (GCF). In particular, under inflammatory conditions, the flow of GCF leaking out through the very thin (4–5 cells) epithelial barrier, is increased (56). The junctional pocket epithelium is non-keratinized, which allows migration of cells such as neutrophils and macrophages by chemotaxis. Thus, GCF, inflammatory cells and desquamated epithelial cells are the main sources of nutrients for the subgingival plaque bacteria. GCF is a serum exudate that contains components found in serum such as proteins, peptides, amino acids, hormones, and vitamins (57). The levels of sugars are negligible in the serum (except in glycemic diabetic patients) and the level of fermentation of carbohydrates/sugars in the subgingival environment is thus low. The fermentation of proteins is, therefore, the predominant metabolic pathway and this favors the proteolytic species in the oral microbiota (secondary fermenters, Figure 1). Nitrogenous compounds can be degraded into short chain fatty acids (SCFAs), NH3, sulfur compounds, and amines by the bacteria of the subgingival biofilm. It should also be noted that urea originates from serum via GCF (58). Therefore, the production of NH3 from urea is mainly occurring in the dental plaque, and the subgingival plaque in particular.
The nutrients present in the oral cavity are further used by secondary fermenters, predominately anaerobic and asaccharolytic bacteria, which collectively produce numerous metabolites, such as SCFAs, amines and gases (Table 1). While most of the metabolites, produced are further used by the microbial community, others are excreted as waste products. This cascade of metabolites increases when the activities and growth rates of the bacteria are increased. The gingival crevice is inaccessible and partly sequestered from the influence of saliva and the conditions prevailing above the gingival margin.
The homofermentative bacteria of the phylum Firmicutes (Streptococcus, Gemella, Granulicatella), are characterized as producing mainly lactic acid from carbohydrates and as being the most abundant bacteria in the human oral cavity. Other primary fermenters, such as lactobacilli, bifidobacteria and clostridia, are prominent fermenters in the proximal part of the intestine but normally constitute only a small fraction of the oral microbiota. Instead, other heterofermentative species with adhesion properties, such as Actinomyces, Corynebacteria, Rothia, Haemophilus, Neisseria, Prevotella and Fusobacterium spp., are better suited to the conditions found in the oral cavity.
Secondary fermenters are predominantly anaerobic bacteria (Figure 1) that require a low redox potential for growth, but these are sensitive to oxidizing substances (oxygen, peroxides, oxygen radicals, isothiocyanate, NO) and can survive and grow only in “hidden areas”, such as the dorsum of the tongue and the interproximal areas between teeth and in and around the gingival crevice. Although many of these anaerobic bacteria can degrade both carbohydrates and proteins, they primarily metabolize monosaccharides and peptides/amino acids and are, therefore, dependent upon the co-operation activities and food chains in a polymicrobial community. There are numerous examples from the oral microbiota of microbial growth only in co-culture with other microorganisms, as well as examples of bacteria providing growth factors to their neighbors (132). Among the secondary fermenters, asaccharolytic bacteria are also found. Veillonella spp. use lactate as a nutrient and have been attributed an important role in producing weaker acids and contributing to neutralizing the pH in dental biofilms (37).
In the subgingival microflora, several proteolytic species (Porphyromonas spp. and Campylobacter spp.), are asaccharolytic, using peptides/amino acids for their energy supply. Most of the amino acids that can be utilized are also essential for growth of the anaerobes. The fermentation process performed by the anaerobes is complex in that it involves extensive degradation of proteins, peptides, and amino acids. The subgingival microbiota constitutes an efficient fermenter (Figure 1), generating plentiful well-known and less-known bioactive metabolites with wide-ranging effects on the gingival tissues (18, 133, 134). These metabolites include an extensive collection of SCFAs, amines, and various gases (Table 1). The impact of these molecules are often crucial for the host, being beneficial at low and detrimental at high concentrations.
SCFAs, which include formic acid, acetic acid, propionic acid, butyric acid and valeric acid, are produced from carbohydrate fermentation. They are also produced during the proteolytic degradation carried out by anaerobic gut bacteria, as well as oral bacteria (62, 135). SCFAs play important roles in both the maintenance of health and the development of inflammatory diseases (18, 136). They exhibit antimicrobial activities against specific bacteria in the microbial community (69). SCFAs (primarily acetate), are extensively produced by F. nucleatum and P. gingivalis (63, 64, 66). Butyric acid is produced during the fermentation of glutamate and has an unpleasant odor (18). A mean concentration of 2.6 mM of butyric acid has been reported in subgingival plaque of periodontitis patients, and 9.5 mM propionic acid, while these SCFAs were below the detection limit at healthy sites (68). In GCF high mean values have been reported, 3.11 mM for butyric and 11.68 mM for propionic acid respectively (67). Furthermore, it has been shown that the concentrations of butyric acid, propionic acid, acetic acid, and isovaleric acid in GCF are significantly reduced two weeks after treatment of periodontitis affected sites (67, 137). Thus, it appears that the concentrations of SCFAs correlate with gingival inflammation (138).
The amines indole and skatole are converted from the amino acid tryptophan during fermentation by several anaerobic oral bacteria, e.g., Fusobacterium, Prevotella, and Porphyromonas, present within the subgingival microbiota (64, 74–76, 78). However, few studies have examined the presence and amounts of indole and skatole in the oral cavity. Other bioactive amines, the polyamines putrescine, spermine and spermidine, have not only been detected in GCF of periodontitis-affected sites but also shown to decrease after treatment (80, 139). Concentrations of 2.76 mM of putrescine have been measured (80). Cadaverine has been investigated in saliva and been shown to increase in the absence of oral hygiene measures (140).
Gases, including carbon monoxide (CO), hydrogen gas (H2), NO, NH3, and hydrogen sulfide (H2S), are critical end-products of the fermentation processes. CO and carbon dioxide (CO2) are main sources for carbon, and even essential for some bacteria, e.g., Capnocytophaga spp (98). H2 is important for many microorganisms (e.g., Campylobacter spp. and Archaea) for their survival and growth. H2S is produced by bacterial fermentation of the sulfur-containing amino acid cysteine by mainly anaerobic species associated with periodontal diseases, including Porphyromonas spp., Fusobacterium spp., Alloprevotella tannerae, Parvimonas micra, T. denticola, V. parvula, and S. anginosus (101, 102, 141, 142). It can also be produced through the reduction of sulfate by the sulfate-reducing bacteria Desulfovibrio spp., Desulfobacter spp., and Desulfomicrobium orale, which may also be present in periodontal pockets (103, 104). Its smell resembles that of rotten eggs, and it is detectable from a concentration of 0.01 ppm in air. H2S, together with methyl mercaptan (CH3SH) and dimethyl disulfide (CH3SSCH3), are important contributors to oral malodor and are usually referred to as volatile sulfur compounds (143, 144). The highest concentrations of H2S reported from diseased periodontal pockets are in the range of 1.5–1.9 mM (109, 110). It has been shown that the subgingival levels of H2S are higher at diseased sites than at healthy sites, as are the capacities of subgingival microbial samples to produce H2S from cysteine (120, 145–147). Thus, the direct measurements of H2S and indirect measurements of the capacity of the microbiota to produce H2S are consistent with each other, in that the more dysbiotic the microflora, the higher is its production of H2S. In addition, accessibility to cysteine is expected to be greater at diseased sites due to the increased secretion of GCF, which contains cysteine. The concentration of cysteine in the serum of healthy blood donors has been reported as 0.26 mM, and it is likely that its level in the GCF is of a similar magnitude (148). Based on these observations, H2S has been suggested to participate in the pathogenesis of periodontal disease (Figure 2A) (110, 120, 121, 147, 149).
Figure 2. (A) A schematic of the production and effects of the bacterial metabolite H2S in the inflamed gingival pocket (reprinted from hydrogen sulfide exposure induces NLRP3 inflammasome-dependent IL-1β and IL-18 secretion in human mononuclear leukocytes in vitro by Basic A, Alizadehgharib S, Dahlén G, Dahlgren U in clin. Exp. Dent. Res. 2017;3:115–120. https://doi.org/10.1002/cre2.69). 1. Serum exudate from blood vessels containing serum proteins, peptides and amino acids, including cysteine. 2. The exudate (gingival crevicular fluid; GCF) passes through the thin pocket epithelium (junctional epithelium) into the subgingival pocket. 3. The subgingival plaque, which contains numerous, mainly Gram-negative, anaerobic bacteria with capacities to degrade proteins, peptides and amino acids, including cysteine. 4. Growing Gram-negative anaerobes release lipopolysaccharides (LPS), which cross the junctional epithelium into the gingival connective tissues. 5. Growing Gram-negative anaerobes (Fusobacterium spp., P. gingivalis, Treponema spp., and others) produce metabolites, e.g., hydrogen sulfide (H2S). 6. The inflammatory lesion attracts monocytes, which migrate into the connective tissue and differentiate into macrophages. 7. The effects of LPS and H2S on macrophages and the subsequent production of the pro-inflammatory cytokines IL-1β and IL-18. This figure has previously been published in an Open Access journal, distributed under the terms of the Creative Commons Attribution ver. 4.0 International License (https://creativecommons.org/licenses/by/4.0/), which permits use, distribution and reproduction in any medium, provided the original work is properly cited. (B) The two signals that lead to the production and secretion of the IL-1β and IL-18 by monocytes/macrophages. For the first signal, the production of pro-IL-1β and pro-IL-18 is stimulated by exposure of the monocytes/macrophages to LPS. The second signal, which can be induced by H2S, results in the release of the two cytokines from the cell after the formation of the NLRP3 inflammasome.
As for bacterial production of H2S, the enzymes involved also catalyze the production of other by-products, such as pyruvate, NH3, and serine (92, 95, 150). NH3 is produced by several bacteria associated with periodontitis, such as Prevotella spp., and Fusobacterium spp. and asserted to contribute to the slightly alkali subgingival environment (65). NH3 can, apart from proteolysis, also be produced from nitrate, as has been shown in saliva and tongue scrapings (139). There are however, to our knowledge no reports to date of measurements of NH3 in periodontal pockets.
Methanogenesis is the process in which methane is produced and usually the final step in the fermentation of organic materials. Methanogenesis takes place only under anaerobic conditions by Archaea. Archaea is regularly missed in culture studies but archaeal DNA can be detected by PCR amplification and by sequencing of subgingival plaque from most adults (151). Especially methane-producing Archaea, e.g., Methanobrevibacter spp., have gained interest in the ecology of the oral microbiota because of their requirement for H2 produced from fermentation by the oral microbiota, e.g., Campylobacter spp. (Figure 1) (152).
The host response to the microbial challenge in periodontal disease is complex and numerous factors are at play (23). It should be noted that the connection between the gingival epithelium and the tooth surface is vulnerable for an exposition of microorganisms of the dental biofilm and their metabolites. The epithelial barrier is extremely thin, non-keratinized and without a mucin layer and is easily penetrable for small molecules such as many of the bacterial metabolites. The host defense in the gingival pocket is supported by the gingival exudate containing serum defense factors such as immunoglobulins and complement factors as well as phagocyting cells such as neutrophils and macrophages. At prolonged exposition of the gingiva for the dental biofilm, an immune-response phase involving lymphocytes and plasma cells, is activated. In contrast to neutrophils and macrophages, which are rapidly and constantly recruited by chemotaxis through the pocket epithelium, lymphocytes and plasma cells are recruited at a later point into the connective tissue beneath the pocket epithelium (inflammatory connective tissue). When activated, these cells produce and release a cascade of cytokines characteristic for a more chronic type of inflammation occurring in long-standing gingivitis. The host response to the dental biofilm is balanced (homeostasis) against the bacterial challenge and the activity in the dental biofilm, characterized by the chronic inflammatory phase, and due to continuous fluctuation of the bacterial activity within the dental biofilm the response includes various degrees of acute phases. In addition, the host is under constant and challenging influence from external and internal host-related factors often referred to as host susceptibility for the tissue breakdown (153). The factors included are systemic diseases (e.g., diabetes), medications (cytostatic drugs), smoking and psychological factors (stress, allostatic load), which results in a highly unpredictable outcome of the inflammatory response at the individual level, after many years of bacterial challenge (23, 154–159).
The many and diverse bacterial waste products in the periodontal pocket are assumed to be abundant due to the lack of salivary cleansing that is seen above the gingival margin. Thus, subgingivally the high concentrations of the waste products of bacterial proteolysis results in a vulnerable oral niche. Apart from potential toxic properties of the waste products at high concentrations, many of them are also present in the human host and may have various functions and contribute to diverse effects on the cells of the host. Several metabolites function as signaling molecules and can trigger or dampen the inflammatory response of the host. It should, however, be highlighted that details and mechanisms known to date are almost exclusively based on in vitro studies and thus, the importance and relevance of these metabolites in vivo are still mostly undetermined. Therefore, more studies are warranted to delineate their relevance in the pathogenesis of periodontal diseases.
The production of the various metabolites is mainly the result of bacterial fermentation for energy recovery, although it also acts to scavenge materials for protein synthesis. This process probably affects the local environment in the biofilm and, thus, may in higher concentrations be harmful to bacterial cells (111, 129). It appears that bacteria can regulate this activity by adjusting the expression of enzymes. This has been shown for H2S where the presence of high concentrations of cysteine in the culture broth during the cultivation of Fusobacterium spp., resulted in downregulation of H2S-producing enzymes (105). Similarly, high concentrations of H2S have been shown to suppress the sulfate uptake and H2S production of sulfate-reducing Desulfovibrio spp (160).
Analyzing from a bacterial point of view, the various metabolites are not only waste products of fermentation but can have protective functions as well. Many of the metabolites, including NH3, spermine, spermidine, H2S, and NO, have been shown to increase bacterial resistance to antibiotics (81, 88, 112, 113, 130). In addition, increased resistance to leukocyte-mediated killing has been described for H2S-producing bacterial strains or strains grown in the presence of H2S, as compared to H2S-deficient strains or strains grown in the absence of H2S (114, 115). There is also evidence that H2S production is advantageous for the bacteria, in that bacterial H2S protects the bacteria from oxidative stress (116). Furthermore, impairment of neutrophil function has been reported for another bacterial metabolite, NH3 (89).
A number of various factors such as bacterial virulence factors (bacterial survival factors such as adhesins, capsule, leukotoxin) participate in the regulation of the biofilm community in addition to the various metabolites formed (161, 162). A beneficial outcome for the bacteria is the reported antimicrobial effect of various metabolites in the competition and cooperation with other species (CO, SCFAs, nitrite, NO) (33, 69, 73, 94, 131). Interestingly, it appears that bacterial producers of various fatty acids are not themselves affected by the acids produced, but the growth of other microorganisms, indicating a competitive advantage for SCFAs-producers in the biofilm (69). Moreover, a synergistic antimicrobial effect in vitro for combinations of fatty acids has been reported (73). Possibly, bacterial metabolites are also involved in the formations of, but also interspecies interactions in, dental biofilms (82, 117, 125).
Plentiful of our knowledge on various gaseous metabolites is based on initial studies of human exposure to very high concentrations, shown to be hazardous to humans (163, 164). H2S, as an example, has previously been in focus as an industrial product in oil refining and mining, resulting in environments with high concentrations of H2S in the air. Also, CO2 is a common industrial gas used in many beverages, and fire extinguishers, among others (99). At high concentrations, both H2S and CO2 have toxic effects with life-threating consequences. A H2S concentration of 100 ppm in air irritates the eyes, and at concentrations of ≥300 ppm there is loss of consciousness, respiratory failure and, potentially, death (118, 119). Concentrations above 10% CO2 in air is comparably hazardous (99). Also, reported exposures to CO and NH3 have similar consequences where inhalation is a common exposure route primarily affecting the respiratory system of the host (90, 96, 97, 163, 165). Both the concentration and the duration of exposure are important factors to consider when evaluating the health effects of these metabolites. Furthermore, other metabolites have been reported toxic when ingested in high amounts, such as amines in fermented foods causing food poisoning, but also nitrate which is converted to nitrite and in high amounts may result in methemoglobinemia in children (85, 166). Whether the metabolites produced in periodontal pockets reach these high local concentrations and exposure times, with potential toxic consequences on host cells, is still undetermined. Theoretically, toxic amounts of the metabolites may potentially result in necrosis of epithelial cells, resulting in bacterial invasions and subsequent infections, clinically manifested as abscesses. Nevertheless, little is known about the concentrations and variations within the periodontal pockets, mainly due to the lack of flexible and reliable methods for estimation of the subgingival metabolites.
The gaseous metabolites, CO, NO, and H2S are all produced by the endogenous cells of humans and animals, and function as signaling molecules, with biological effects evident at low doses (96, 167). In the catabolism of heme, CO is produced, approximately 18 μmol/hour (167). In serum, a concentration of 24 μmol/L has been reported for NO in healthy adults (168). Correspondingly, micromolar concentrations of H2S have been detected in the blood and in brain tissue (119, 169, 170). Among many diverse functions, it has been shown that exposure to CO has neuroprotective effects, that NO regulates blood pressure, and that H2S can vasodilate blood vessels and act as a neurotransmitter in the brain (96, 171–173). The signaling molecules have been implicated in stroke, Dowńs syndrome and Alzheimeŕs disease, among other disorders of the CNS (96, 171). Hence, the hormesis phenomenon, where low doses of a toxic agent are beneficial, can probably be applied on many of the metabolites of the fermentation occurring in periodontal pockets.
So, not only the colonizing bacteria but also the host cells possess CO-producing/NO-producing/H2S -producing enzymes. NO is produced by NO synthase enzymes (NOS) (174). In humans and animals, three H2S-producing enzymes have been identified: cystathionine-β-synthase, cystathionine-γ-lyase, and 3-mercapto-sulfurtransferase (175). These enzymes are present in the majority of human cells, including those in the periodontium (122, 176). Similarly, amines are produced by colonizing bacteria and host cells, but may also be derived from foods (86).
The exact mechanisms by which the bacterial metabolites affect human and bacterial cells are still not fully understood for the majority of them. It is for instance regarded that NH3 can easily penetrate cell membranes and alter intracellular pH (177). It has been reported that the ability of H2S to split disulfide bonds is an important factor for its activity. This cleavage may, by reducing binding to cell surface antigens, contribute to the inhibition of antibody-mediated immune responses reported in the presence of H2S (115). In addition, H2S is a known inhibitor of cytochrome c oxidase (178, 179). Three biochemical pathways for H2S signaling have been described. H2S can react with metal centers, modulate the cysteine residues of proteins by S-sulfhydration, and can react with reactive oxygen and nitrogen species (150, 180, 181). The fact that H2S binds to metals has been exploited for the detection of H2S production with lead-acetate impregnated strips (182). H2S also functions as a reducing agent (92, 183). Furthermore, it has been reported that H2S can pass through cell membranes due to its solubility in lipids (92, 119, 170). HS− ions use the AE-1 channel to pass through the cell membrane in mammals, whereas a hydrosulfide ion channel has been described for bacteria (146, 184).
Of the SCFAs, butyric acid is extensively studied, not only in the oral cavity but also in the intestine (71). It is considered a biologically active signaling component with both anti-inflammatory and inflammation-promoting effects on host cells (70, 71). Butyrate has been shown to have different effects on different cell types, in what is known as the “butyrate paradox”, whereby the presence of butyrate promotes healthy cells in the colon and simultaneously inhibits cancerous cells (185). This phenomenon is still not fully understood. Butyric acid has been reported to be present at increased levels at diseased periodontal sites, and in vitro studies have shown that butyric acid can induce the apoptosis of monocytes and fibroblasts, among other host cells (62, 68, 186–189).
That numerous metabolites are also signaling substances implies that they affect host cells in various ways. These effects are not just connected to the endogenously produced amounts, but also to the quantity of the metabolites produced by the bacteria colonizing the different body compartments. It has been shown that several metabolites, in diverse both pro- and anti-inflammatory manners, affect several host cell types (70, 71, 100, 122, 164, 190, 191). These immunomodulatory consequences have been comprehensively reviewed for H2S elsewhere (122). Briefly, the anti-inflammatory effects of H2S on neutrophils include inhibition of neutrophil adhesion, neutrophil extracellular trap (NET) formation, and myeloperoxidase secretion, as well as the release of other contents of the neutrophil granules (122, 192, 193). Furthermore, monocytes/macrophages have been reported to be affected by H2S exposure in an anti-inflammatory manner by promoting macrophage polarization from the M1 type to the more anti-inflammatory M2 type (194). In addition, H2S can inhibit the secretion of pro-inflammatory cytokines and stimulate the secretion of the anti-inflammatory IL-10 (195). This inhibition and secretion has also been shown for CO (96).
In contrast, exposure to H2S in vitro has been shown to induce: apoptosis in human periodontal ligament cells and human gingival fibroblasts; the secretion of IL-6 and IL-8 from these cells; and IL-8 secretion from epithelial cells (196–198). Moreover, cell death and functional inhibition have been reported for lymphocytes exposed to H2S (199). The in vitro exposure of a monocyte cell line to H2S has been shown to result in the secretion of the pro-inflammatory cytokines IL-1β and IL-18, in a process that requires two signals. The first signal generates the production of pro-IL-1β and pro-IL-18 in the nucleus, while the second signal activates the NLRP3 inflammasome and induces the secretion of the cytokines from the cell (Figure 2B). The NLRP3 inflammasome is a protein complex that, when formed, cleaves the inactive forms of the proteins, i.e., pro-IL-1β and pro-IL-18, to IL-1β and IL-18 (200). The first signal may be activated by LPS, although there is evidence that H2S can also contribute to this first signal (121). The second signal, leading to the formation of the NLRP3 inflammasome, can be induced by various substances, such as peptidoglycans and ATP, but also by H2S (120, 121). The results from a clinical study involving periodontitis patients and healthy controls showed differences between the two groups in terms of the patterns of cytokine expression from monocytes exposed to H2S (120). Various immunomodulatory roles of H2S in the human body are not exclusive for H2S but also seen for other metabolites. SCFAs, for instance, have been shown capable of recruiting neutrophils to the GCF though binding to the neutrophil-specific chemotactic receptor FFA2R (63, 66). NH3 has been reported to affect the functions of neutrophils, such as their affinity of receptors, their phagocytosis, degranulation, and metabolism (89, 201).
Given that bacterial production of H2S in the periodontal pocket promotes the inflammatory response of the host to the microbiota, it can be regarded as both detrimental (increased secretion of pro-inflammatory cytokines) and beneficial (increased GCF and, consequently, higher levels of nutrients in the periodontal pocket) for the bacteria. Additional activities of H2S, e.g., in enhancing bacterial resistance to antibiotics and attenuating leukocyte-mediated killing of bacteria, are beneficial for the bacteria in the host-bacteria interplay (112, 114). In contrast, anti-inflammatory effects of endogenously produced H2S have been reported as favorable for the host (122). Since H2S is recognized by the host as an endogenous molecule, the inflammatory response may be exacerbated, resulting in chronic inflammation (as in periodontitis). Thus, the ultimate impact of H2S in the pathogenesis of periodontal diseases is difficult to determine due to the diverse effects of H2S on the bacteria and host cells. Furthermore, H2S may be oxidized to polysulfides or other sulfur-containing compounds, which also affect host cells, upon entering the host (95, 202, 203). A further complication is that other sulfur-containing metabolites, such as methyl mercaptan from methionine, are produced simultaneously. Moreover, the continuously fluctuating growth rates and activities of the microbes result in variable production levels of the various metabolites in the periodontal pocket. Collectively, these issues illustrate the difficulties associated with studying H2S, but also other metabolites, in the host-bacteria interplay and its roles in periodontal diseases.
Microbial metabolites are the main contributors to evoke an inflammatory response in the gingival tissues. The metabolic pathways in the biofilm are generally shared between different microbial species representing diverse genera. The metabolism of the biofilm is characterized by fluctuations between burst of activities and resting (dormant) microbial cells. The release of metabolites is constantly fluctuating, which normally and in a seemingly homeostatic condition is balanced by the host response inducing and maintaining an inflammatory process seen clinically as gingivitis. This inflammatory response should be regarded as a natural, protective response to a subgingival microbiota that is adapted and thrive in the inflamed and deepened gingival pocket which offers a perfect ecological niche (161). Simply, this is a normal condition occurring in everybody in absence of careful oral hygiene measures. Like the dripping water that cavitates the stone, loss of clinical attachment at long-standing inflammation is a slow process that becomes clinically visible after years of exposition as the host response and colonizing microorganisms increase in complexity as the disease progresses. The transition from gingivitis to destructive periodontitis has been a matter of various hypotheses over the years, involving both biofilm and host factors, and there are advocates for it primarily being a natural aging phenomenon (15, 23, 204, 205). The likelihood of progression or regression can be influenced by various determinants, (e.g., specific bacteria), but these processes will nevertheless occur in the absence of such factors (17). From this it follows that destructive periodontitis can occur in everybody with time, but the probability of disease to occur can be reduced and controlled by measures that reduce the risk, such as for instance daily tooth cleaning procedures that regularly disrupt the dental biofilm formed and decrease the bacterial load and the levels of released metabolic waste products. It is the way the host and the tissues respond, which could be delayed or disproportionate of various reasons, that is responsible in the long-term for the disease progression, based on intrinsic (genetics, age) or acquired factors (smoking, systemic diseases) making the host and tissues susceptible to a variable degree (153, 159, 205, 206). The central role of inflammation in the pathogenesis of destructive periodontitis is generally accepted (204).
In addition to the metabolites, other factors of the bacteria connected to their characteristics, such as endotoxins, capsule formation, fimbriae but also their production of leukotoxin, outer membrane vesicles, bacteriocins and other antimicrobial peptides, are also to be considered in these interactions with the host, as we have reviewed elsewhere (23, 161). These “virulence” factors are of importance for the survival and growth of the bacteria, but their importance in the pathogenesis is undetermined (161).
The net outcome of the very complex interactions between numerous microorganisms in the dental biofilm, where it is impossible to perceive who is doing what, may be estimated by longitudinal and continuous measurement of these waste products, possibly by the use of cheap and simple chair-side methods. But if this is clinically relevant is still mostly unexplored territory.
The role of specific bacterial species may intermittently play a decisive part. So far, predictive studies, using specific risk marker bacteria, have only reached weak sensitivity and/or specificity to prospectively predict future progression on site/individual level, and these markers have not become widely used in current risk evaluation of periodontal diseases (207). However, the Jp2 clone of A. actinomycetemcomitans in populations of African origin is an exception from this by the finding that those individuals with this particular bacterial genotype have a 14 times higher risk for progression of periodontitis within 2 years (208, 209). The reason for the limits to use bacteria as risk markers is probably due to that the prevalence and abundance of bacteria are inadequate indirect measurements for their activity at a given time point (e.g., time of sampling) (138). To evaluate the metabolic activity by measuring the level of metabolites and the capacity of biofilms to produce these in the subgingival niche would thus be a more appropriate approach for identifying risk determinants, such as various metabolites, for the probability of disease progression, but this research area is still in its infancy. GCF has been used as a sampling source for biomarkers of periodontitis using bacterial metabolomics for diagnostics of the microbial and host interaction (210). In a systematic review on four metabolomic studies on biomarkers of GCF, it was shown among 40 significantly discriminant metabolites, predominantly related to amino acid and lipid degradation, that the diagnostic accuracy was low (211). New and more sophisticated molecular methods, such as the use of proteomics and metabolomics, will add knowledge in this area but due to often small sample sizes, also approaches fitting more prospective large-scale studies are needed. Here, initial controlled in vitro investigations of host cells to prolonged exposure to clinically relevant concentrations of various metabolites in an appropriate environment regarding temperature, pH, and oxygen level, may be followed by more advanced clinical studies using sophisticated molecular approaches.
Thus, studies on the major metabolites produced under bacterial activities (burst of activities) are suggested for future research on metabolic biomarkers. Simple and easily performed methods for the measurement of the metabolic load and the capacity to produce various metabolites (at rest and at challenge) experimentally in vivo or ex vivo are needed. Longitudinal follow-up studies using chair-side evaluations at many sampling sites, mapping the metabolic landscape in each individual, would disclose metabolic determinants in identifying individuals with a greater risk for disease progression.
The metabolites are the net outcome of the very complex interplay between numerous and different microorganisms in the dental biofilm. Much research has focused on the composition of the biofilms and the presence of certain periodontopathogens. This review focuses on the functions and dynamics of the biofilm, the metabolites, and their interplay with the host to better understand the impact of the biofilm in destructive periodontal disease. Here, simple chairside methods can complement molecular approaches and be applied longitudinally in clinical studies for determination of major metabolites and their clinical relevance on subjects with various periodontal conditions.
The authors AB and GD contributed to the design and interpretation of the data and drafted and critically revised the work. All authors contributed to the article and approved the submitted version.
Some of the ideas and concepts presented in this manuscript have previously been published in the PhD thesis of the first author (212).
The authors declare that the research was conducted in the absence of any commercial or financial relationships that could be construed as a potential conflict of interest.
All claims expressed in this article are solely those of the authors and do not necessarily represent those of their affiliated organizations, or those of the publisher, the editors and the reviewers. Any product that may be evaluated in this article, or claim that may be made by its manufacturer, is not guaranteed or endorsed by the publisher.
1. Huttenhower C, Gevers D, Knight R, Abubucker S, Badger JH, Chinwalla AT, et al. Structure, function and diversity of the healthy human microbiome. Nature. (2012) 486(7402):207–14. doi: 10.1038/nature11234
2. Kilian M, Chapple ILC, Hannig M, Marsh PD, Meuric V, Pedersen AML, et al. The oral microbiome—an update for oral healthcare professionals. Br Dent J. (2016) 221(10):657–66. doi: 10.1038/sj.bdj.2016.865
3. Cole MFL PM. Oral microbiology and the immune response. In: Lamont RJB R. J., Lantz M. S., LeBlanc D. J., editors. Oral microbiology and immunology. Washington DC, USA: ASM Press (2006). p. 201–29.
5. Trombelli L, Farina R, Silva CO, Tatakis DN. Plaque-induced gingivitis: case definition and diagnostic considerations. J Clin Periodontol. (2018) 45:S44–67. doi: 10.1111/jcpe.12939
6. Loe H, Theilade E, Jensen SB. Experimental gingivitis in man. J Periodontol. (1965) 36:177–87. doi: 10.1902/jop.1965.36.3.177
7. Theilade E, Wright WH, Jensen SB, Löe H. Experimental gingivitis in man. II. A longitudinal clinical and bacteriological investigation. J Periodontal Res. (1966) 1:1–13. doi: 10.1111/j.1600-0765.1966.tb01842.x
8. Loesche WJ, Syed SA. Bacteriology of human experimental gingivitis: effect of plaque and gingivitis score. Infect Immun. (1978) 21(3):830–9. doi: 10.1128/iai.21.3.830-839.1978
9. Moore WEC, Holdeman LV, Smibert RM, Good IJ, Burmeister JA, Palcanis KG, et al. Bacteriology of experimental gingivitis in young adult humans. Infect Immun. (1982) 38(2):651–67. doi: 10.1128/iai.38.2.651-667.1982
10. Belibasakis GN, Belstrøm D, Eick S, Gursoy UK, Johansson A, Könönen E. Periodontal microbiology and microbial etiology of periodontal diseases: historical concepts and contemporary perspectives. Periodontol 2000. (2023). doi: 10.1111/prd.12473. [Epub ahead of print]36661184
11. Perez-Chaparro PJ, Goncalves C, Figueiredo LC, Faveri M, Lobao E, Tamashiro N, et al. Newly identified pathogens associated with periodontitis: a systematic review. J Dent Res. (2014) 93(9):846–58. doi: 10.1177/0022034514542468
12. Socransky SS, Haffajee AD, Cugini MA, Smith C, Kent RL Jr. Microbial complexes in subgingival plaque. J Clin Periodontol. (1998) 25(2):134–44. doi: 10.1111/j.1600-051X.1998.tb02419.x
13. Listgarten MA, Hellden L. Relative distribution of bacteria at clinically healthy and periodontally diseased sites in humans. J Clin Periodontol. (1978) 5(2):115–32. doi: 10.1111/j.1600-051X.1978.tb01913.x
14. Greenstein G, Polson A. Microscopic monitoring of pathogens associated with periodontal diseases. A review. J Periodontol. (1985) 56(12):740–7. doi: 10.1902/jop.1985.56.12.740
15. Lamont RJ, Koo H, Hajishengallis G. The oral microbiota: dynamic communities and host interactions. Nat Rev Microbiol. (2018) 16(12):745–59. doi: 10.1038/s41579-018-0089-x
16. Scannapieco FA, Dongari-Bagtzoglou A. Dysbiosis revisited: understanding the role of the oral microbiome in the pathogenesis of gingivitis and periodontitis: a critical assessment. J Periodontol. (2021) 92(8):1071–8. doi: 10.1002/JPER.21-0120
17. Manji F, Dahlen G, Fejerskov O. Caries and periodontitis: contesting the conventional wisdom on their aetiology. Caries Res. (2018) 52(6):548–64. doi: 10.1159/000488948
18. Takahashi N. Oral microbiome metabolism: from “who are they?” to “what are they doing? ”. J Dental Res. (2015) 94(12):1628–37. doi: 10.1177/0022034515606045
19. Nyvad B, Takahashi N. Integrated hypothesis of dental caries and periodontal diseases. J Oral Microbiol. (2020) 12(1). doi: 10.1080/20002297.2019.1710953
20. Teles R, Teles F, Frias-Lopez J, Paster B, Haffajee A. Lessons learned and unlearned in periodontal microbiology. Periodontol 2000. (2013) 62(1):95–162. doi: 10.1111/prd.12010
21. Wade WG. The oral microbiome in health and disease. Pharmacol Res. (2013) 69(1):137–43. doi: 10.1016/j.phrs.2012.11.006
22. Yost S, Duran-Pinedo AE, Teles R, Krishnan K, Frias-Lopez J. Functional signatures of oral dysbiosis during periodontitis progression revealed by microbial metatranscriptome analysis. Genome Med. (2015) 7(1). doi: 10.1186/s13073-015-0153-3
23. Dahlen G, Fejerskov O, Manji F. Current concepts and an alternative perspective on periodontal disease. BMC Oral Health. (2020) 20(1):235. doi: 10.1186/s12903-020-01221-4
24. Segata N, Haake SK, Mannon P, Lemon KP, Waldron L, Gevers D, et al. Composition of the adult digestive tract bacterial microbiome based on seven mouth surfaces, tonsils, throat and stool samples. Genome Biol. (2012) 13(6). doi: 10.1186/gb-2012-13-6-r42
25. Bowen WH. The stephan curve revisited. Odontology. (2013) 101(1):2–8. doi: 10.1007/s10266-012-0092-z
26. Carlson-Jones JAP, Kontos A, Kennedy D, Martin J, Lushington K, McKerral J, et al. The microbial abundance dynamics of the paediatric oral cavity before and after sleep. J Oral Microbiol. (2020) 12(1):1741254. doi: 10.1080/20002297.2020.1741254
27. Marsh PD, Zaura E. Dental biofilm: ecological interactions in health and disease. J Clin Periodontol. (2017) 44(18):S12–22. doi: 10.1111/jcpe.12679
28. Toczewska J, Konopka T. Activity of enzymatic antioxidants in periodontitis: a systematic overview of the literature. Dent Med Probl. (2019) 56(4):419–26. doi: 10.17219/dmp/112151
29. Madiyal A, Ajila V, Babu SG, Hegde S, Kumari S, Madi M, et al. Status of thiocyanate levels in the serum and saliva of non-smokers, ex-smokers and smokers. Afr Health Sci. (2018) 18(3):727–36. doi: 10.4314/ahs.v18i3.31
30. Piwat S, Hassan H, Kjeang T, Lindehag J, Wedin H, Teanpaisan R, et al. Site-specific dental plaque pH in 13-year-old Thai schoolchildren. Clin Oral Investig. (2015) 19(9):2179–86. doi: 10.1007/s00784-015-1454-z
31. Dahlén G, Hassan H, Blomqvist S, Carlén A. Rapid urease test (RUT) for evaluation of urease activity in oral bacteria in vitro and in supragingival dental plaque ex vivo. BMC Oral Health. (2018) 18(1). doi: 10.1186/s12903-018-0541-3
32. Appelgren L, Dahlen A, Eriksson C, Suksuart N, Dahlen G. Dental plaque pH and ureolytic activity in children and adults of a low caries population. Acta Odontol Scand. (2014) 72(3):194–201. doi: 10.3109/00016357.2013.794952
33. Lundberg JO, Weitzberg E, Gladwin MT. The nitrate-nitrite-nitric oxide pathway in physiology and therapeutics. Nat Rev Drug Discovery. (2008) 7(2):156–67. doi: 10.1038/nrd2466
34. Duncan C, Dougall H, Johnston P, Green S, Brogan R, Leifert C, et al. Chemical generation of nitric oxide in the mouth from the enterosalivary circulation of dietary nitrate. Nat Med. (1995) 1(6):546–51. doi: 10.1038/nm0695-546
35. Lundberg JO, Govoni M. Inorganic nitrate is a possible source for systemic generation of nitric oxide. Free Radical Biol and Med. (2004) 37(3):395–400. doi: 10.1016/j.freeradbiomed.2004.04.027
36. Qu XM, Wu ZF, Pang BX, Jin LY, Qin LZ, Wang SL. From nitrate to nitric oxide: the role of salivary glands and oral Bacteria. J Dent Res. (2016) 95(13):1452–6. doi: 10.1177/0022034516673019
37. Wicaksono DP, Washio J, Abiko Y, Domon H, Takahashia N. Nitrite production from nitrate and its link with lactate metabolism in oral Veillonella spp. Appl Environ Microbiol. (2020) 86(20):1–9. doi: 10.1128/AEM.01255-20
38. Doel JJ, Benjamin N, Hector MP, Rogers M, Allaker RP. Evaluation of bacterial nitrate reduction in the human oral cavity. Eur J Oral Sci. (2005) 113(1):14–9. doi: 10.1111/j.1600-0722.2004.00184.x
39. Lundberg JO, Weitzberg E, Cole JA, Benjamin N. Nitrate. Nitrate, bacteria and human health. Nat Rev Microbiol. (2004) 2(7):593–602. doi: 10.1038/nrmicro929
40. Lundberg JO, Carlström M, Weitzberg E. Metabolic effects of dietary nitrate in health and disease. Cell Metab. (2018) 28(1):9–22. doi: 10.1016/j.cmet.2018.06.007
41. Ma L, Hu L, Feng X, Wang S. Nitrate and nitrite in health and disease. Aging Dis. (2018) 9(5):938–45. doi: 10.14336/AD.2017.1207
42. Govoni M, Jansson EA, Weitzberg E, Lundberg JO. The increase in plasma nitrite after a dietary nitrate load is markedly attenuated by an antibacterial mouthwash. Nitric Oxide—Biol and Chem. (2008) 19(4):333–7. doi: 10.1016/j.niox.2008.08.003
43. Woessner M, Smoliga JM, Tarzia B, Stabler T, Van Bruggen M, Allen JD. A stepwise reduction in plasma and salivary nitrite with increasing strengths of mouthwash following a dietary nitrate load. Nitric Oxide—Biol and Chem. (2016) 54:1–7. doi: 10.1016/j.niox.2016.01.002
44. Sundqvist ML, Lundberg JO, Weitzberg E. Effects of antiseptic mouthwash on resting metabolic rate: a randomized, double-blind, crossover study. Nitric Oxide—Biol and Chem. (2016) 61:38–44. doi: 10.1016/j.niox.2016.10.003
45. Morou-Bermúdez E, Torres-Colón JE, Bermúdez NS, Patel RP, Joshipura KJ. Pathways linking oral Bacteria, nitric oxide metabolism, and health. J Dent Res. (2022) 101(6):623–31. doi: 10.1177/00220345211064571
46. Schreiber F, Stief P, Gieseke A, Heisterkamp IM, Verstraete W, de Beer D, et al. Denitrification in human dental plaque. BMC Biol. (2010) 8. doi: 10.1186/1741-7007-8-24
47. Sato-Suzuki Y, Washio J, Wicaksono DP, Sato T, Fukumoto S, Takahashi N. Nitrite-producing oral microbiome in adults and children. Sci Rep. (2020) 10(1). doi: 10.1038/s41598-020-73479-1
48. Doel JJ, Hector MP, Amirtham CV, Al-Anzan LA, Benjamin N, Allaker RP. Protective effect of salivary nitrate and microbial nitrate reductase activity against caries. Eur J Oral Sci. (2004) 112(5):424–8. doi: 10.1111/j.1600-0722.2004.00153.x
49. Li H, Thompson I, Carter P, Whiteley A, Bailey M, Leifert C, et al. Salivary nitrate—an ecological factor in reducing oral acidity. Oral Microbiol Immunol. (2007) 22(1):67–71. doi: 10.1111/j.1399-302X.2007.00313.x
50. Rosier BT, Palazón C, García-Esteban S, Artacho A, Galiana A, Mira A. A single dose of nitrate increases resilience against acidification derived from sugar fermentation by the oral microbiome. Front Cell Infect Microbiol. (2021) 11. doi: 10.3389/fcimb.2021.692883
51. Lundberg JO, Weitzberg E, Lundberg JM, Alving K. Nitric oxide in exhaled air. Eur Respir J. (1996) 9(12):2671–80. doi: 10.1183/09031936.96.09122671
52. Lundberg JO, Weitzberg E, Lundberg JM, Alving K. Intragastric nitric oxide production in humans: measurements in expelled air. Gut. (1994) 35(11):1543–6. doi: 10.1136/gut.35.11.1543
53. Sánchez GA, Miozza VA, Delgado A, Busch L. Total salivary nitrates and nitrites in oral health and periodontal disease. Nitric Oxide—Biol and Chem. (2014) 36:31–5. doi: 10.1016/j.niox.2013.10.012
54. Jockel-Schneider Y, Schlagenhauf U, Stölzel P, Goßner S, Carle R, Ehmke B, et al. Nitrate-rich diet alters the composition of the oral microbiota in periodontal recall patients. J Periodontol. (2021) 92(11):1536–45. doi: 10.1002/JPER.20-0778
55. Jockel-Schneider Y, Goßner SK, Petersen N, Stölzel P, Hägele F, Schweiggert RM, et al. Stimulation of the nitrate-nitrite-NO-metabolism by repeated lettuce juice consumption decreases gingival inflammation in periodontal recall patients: a randomized, double-blinded, placebo-controlled clinical trial. J Clin Periodontol. (2016) 43(7):603–8. doi: 10.1111/jcpe.12542
56. Lamster IB, Harper DS, Fiorello LA, Oshrain RL, Celenti RS, Gordon JM. Lysosomal and cytoplasmic enzyme activity, crevicular fluid volume, and clinical parameters characterizing gingival sites with shallow to intermediate probing depths. J Periodontol. (1987) 58(9):614–21. doi: 10.1902/jop.1987.58.9.614
57. Curtis MA, Griffiths GS, Price SJ, Coulthurst SK, Johnson NW. The total protein concentration of gingival crevicular fluid: variation with sampling time and gingival inflammation. J Clin Periodontol. (1988) 15(10):628–32. doi: 10.1111/j.1600-051X.1988.tb02263.x
58. Sezer B, Kodaman Dokumacigil N, Kaya R, Guven S, Turkkan ON, Cicek N, et al. Association between serum biomarkers and oral health status in children with chronic kidney disease: a cross-sectional study. Clin Oral Investig. (2023). doi: 10.1007/s00784-023-04989-1. [Epub ahead of print]37014503
59. Zhu L, Kreth J. The role of hydrogen peroxide in environmental adaptation of oral microbial communities. Oxid Med Cell Longev. (2012) 2012:717843. doi: 10.1155/2012/717843
60. Erttmann SF, Gekara NO. Hydrogen peroxide release by bacteria suppresses inflammasome-dependent innate immunity. Nat Commun. (2019) 10(1):3493. doi: 10.1038/s41467-019-11169-x
61. Uematsu H, Sato N, Hossain MZ, Ikeda T, Hoshino E. Degradation of arginine and other amino acids by butyrate-producing asaccharolytic anaerobic gram-positive rods in periodontal pockets. Arch Oral Biol. (2003) 48(6):423–9. doi: 10.1016/S0003-9969(03)00031-1
62. Ochiai K, Kurita-Ochiai T. Effects of butyric acid on the periodontal tissue. Japanese Dental Sci Rev. (2009) 45(2):75–82. doi: 10.1016/j.jdsr.2009.06.002
63. Dahlstrand Rudin A, Khamzeh A, Venkatakrishnan V, Basic A, Christenson K, Bylund J. Short chain fatty acids released by Fusobacterium nucleatum are neutrophil chemoattractants acting via free fatty acid receptor 2 (FFAR2). Cell Microbiol. (2021) 23(8):e13348. doi: 10.1111/cmi.13348
64. Dzink JL, Sheenan MT, Socransky SS. Proposal of three subspecies of Fusobacterium nucleatum knorr 1922: fusobacterium nucleatum subsp. nucleatum subsp., nov., comb. Nov.; Fusobacterium nucleatum subsp. polymorphum subsp. Nov., nom. Rev., comb. Nov.; and Fusobacterium nucleatum subsp. vincentii subsp. Nov., nom. Rev., comb. Nov. Int J Syst Bacteriol. (1990) 40(1):74–8. doi: 10.1099/00207713-40-1-74
65. Takahashi N, Saito K, Schachtele CF, Yamada T. Acid tolerance and acid-neutralizing activity of Porphyromonas gingivalis, Prevotella intermedia and Fusobacterium nucleatum. Oral Microbiol Immunol. (1997) 12(6):323–8. doi: 10.1111/j.1399-302X.1997.tb00733.x
66. Dahlstrand Rudin A, Khamzeh A, Venkatakrishnan V, Persson T, Gabl M, Savolainen O, et al. Porphyromonas gingivalis produce neutrophil specific chemoattractants including short chain fatty acids. Front Cell Infect Microbiol. (2020) 10:620681. doi: 10.3389/fcimb.2020.620681
67. Qiqiang L, Huanxin M, Xuejun G. Longitudinal study of volatile fatty acids in the gingival crevicular fluid of patients with periodontitis before and after nonsurgical therapy. J Periodontal Res. (2012) 47(6):740–9. doi: 10.1111/j.1600-0765.2012.01489.x
68. Niederman R, Buyle-Bodin Y, Lu BY, Robinson P, Naleway C. Short-chain carboxylic acid concentration in human gingival crevicular fluid. J Dent Res. (1997) 76(1):575–9. doi: 10.1177/00220345970760010801
69. Huang CB, Alimova Y, Myers TM, Ebersole JL. Short- and medium-chain fatty acids exhibit antimicrobial activity for oral microorganisms. Arch Oral Biol. (2011) 56(7):650–4. doi: 10.1016/j.archoralbio.2011.01.011
70. Chen J, Vitetta L. The role of butyrate in attenuating pathobiont-induced hyperinflammation. Immune Netw. (2020) 20(2). doi: 10.4110/in.2020.20.e15
71. Guan X, Li W, Meng H. A double-edged sword: role of butyrate in the oral cavity and the gut. Mol Oral Microbiol. (2021) 36(2):121–31. doi: 10.1111/omi.12322
72. Silva YP, Bernardi A, Frozza RL. The role of short-chain fatty acids from gut Microbiota in gut-brain communication. Front Endocrinol (Lausanne). (2020) 11. doi: 10.3389/fendo.2020.00025
73. Peh E, Kittler S, Reich F, Kehrenberg C. Antimicrobial activity of organic acids against Campylobacter spp. And development of combinations-A synergistic effect? PLoS ONE. (2020) 15. doi: 10.1371/journal.pone.0239312
74. Lombard GL, Dowell VR Jr. Comparison of three reagents for detecting indole production by anaerobic bacteria in microtest systems. J Clin Microbiol. (1983) 18(3):609–13. doi: 10.1128/jcm.18.3.609-613.1983
75. Codipilly D, Kleinberg I. Generation of indole/skatole during malodor formation in the salivary sediment model system and initial examination of the oral bacteria involved. J Breath Res. (2008) 2(1). doi: 10.1088/1752-7155/2/1/017017
76. Sasaki-Imamura T, Yano A, Yoshida Y. Production of indole from L-tryptophan and effects of these compounds on biofilm formation by fusobacterium nucleatum ATCC 25586. Appl Environ Microbiol. (2010) 76(13):4260–8. doi: 10.1128/AEM.00166-10
77. Fay GD, Barry AL. Methods for detecting indole production by gram negative nonsporeforming anaerobes. J Appl Microbiol. (1974) 27(3):562–5. doi: 10.1128/am.27.3.562-565.1974
78. Lee JH, Lee J. Indole as an intercellular signal in microbial communities. FEMS Microbiol Rev. (2010) 34(4):426–44. doi: 10.1111/j.1574-6976.2009.00204.x
79. Walters JD, Locaffaro J, Beck FM. Relationship of human gingival crevicular fluid polyamine concentration to the percentage of spirochaetes in subgingival dental plaque. Arch Oral Biol. (1989) 34(5):373–5. doi: 10.1016/0003-9969(89)90111-8
80. Lamster IB, Mandella RD, Zove SM, Harper DS. The polyamines putrescine, spermidine and spermine in human gingival crevicular fluid. Arch Oral Biol. (1987) 32(5):329–33. doi: 10.1016/0003-9969(87)90087-2
81. Kwon DH, Lu CD. Polyamine effects on antibiotic susceptibility in bacteria. Antimicrob Agents Chemother. (2007) 51(6):2070–7. doi: 10.1128/AAC.01472-06
82. Inaba T, Obana N, Habe H, Nomura N. Biofilm formation by streptococcus mutans is enhanced by indole via the quorum sensing pathway. Microbes and Environments. (2020) 35(2). doi: 10.1264/jsme2.ME19164
83. Kumar A, Sperandio V. Indole signaling at the host-microbiota-pathogen interface. MBio. (2019) 10(3). doi: 10.1128/mBio.01031-19
84. Amin M, Tang S, Shalamanova L, Taylor RL, Wylie S, Abdullah BM, et al. Polyamine biomarkers as indicators of human disease. Biomarkers. (2021) 26(2):77–94. doi: 10.1080/1354750X.2021.1875506
85. Wójcik W, Łukasiewicz M, Puppel K. Biogenic amines: formation, action and toxicity—a review. J Sci Food Agric. (2021) 101(7):2634–40. doi: 10.1002/jsfa.10928
86. Ramani D, De Bandt JP, Cynober L. Aliphatic polyamines in physiology and diseases. Clin Nutr. (2014) 33(1):14–22. doi: 10.1016/j.clnu.2013.09.019
87. Ciancio SG, Golub LM, Mosovich L, Katz C, Kleinberg I. Urea levels in the gingival crevices of diabetic and normal adolescents. J Dent Res. (1977) 56(10):1144. doi: 10.1177/00220345770560100201
88. Bernier SP, Létoffé S, Delepierre M, Ghigo JM. Biogenic ammonia modifies antibiotic resistance at a distance in physically separated bacteria. Mol Microbiol. (2011) 81(3):705–16. doi: 10.1111/j.1365-2958.2011.07724.x
89. Niederman R, Brunkhorst B, Smith S, Weinreb RN, Ryder MI. Ammonia as a potential mediator of adult human periodontal infection: inhibition of neutrophil function. Arch Oral Biol. (1990) 35:S205–9. doi: 10.1016/0003-9969(90)90159-8
90. Smith DD. Acute inhalation injury: how to assess, how to treat. J Resp Dis. (1999) 20(6):405–14.
91. Shawcross DL, Wright GAK, Stadlbauer V, Hodges SJ, Davies NA, Wheeler-Jones C, et al. Ammonia impairs neutrophil phagocytic function in liver disease. Hepatology. (2008) 48(4):1202–12. doi: 10.1002/hep.22474
92. Wang R. Two's company, three's A crowd: can H2S be the third endogenous gaseous transmitter? FASEB J. (2002) 16(13):1792–8. doi: 10.1096/fj.02-0211hyp
93. Engel RR, Matsen JM, Chapman SS, Schwartz S. Carbon monoxide production from heme compounds by bacteria. J Bacteriol. (1972) 112(3):1310–5. doi: 10.1128/jb.112.3.1310-1315.1972
94. Nguyen D, Nguyen TK, Rice SA, Boyer C. CO-releasing polymers exert antimicrobial activity. Biomacromolecules. (2015) 16(9):2776–86. doi: 10.1021/acs.biomac.5b00716
95. Li L, Rose P, Moore PK. Hydrogen sulfide and cell signaling. Annu Rev Pharmacol Toxicol. (2011) 51:169–87. doi: 10.1146/annurev-pharmtox-010510-100505
96. Siracusa R, Voltarelli VA, Salinaro AT, Modafferi S, Cuzzocrea S, Calabrese EJ, et al. NO, CO and H(2)S: a trinacrium of bioactive gases in the brain. Biochem Pharmacol. (2022) 202:115122. doi: 10.1016/j.bcp.2022.115122
97. Jaffe FA. Pathogenicity of carbon monoxide. Am J Forensic Med Pathol. (1997) 18(4):406–10. doi: 10.1097/00000433-199712000-00017
98. Wood HG. Life with CO or CO2 and H2 as a source of carbon and energy. FASEB J. (1991) 5(2):156–63. doi: 10.1096/fasebj.5.2.1900793
99. Langford NJ. Carbon dioxide poisoning. Toxicol Rev. (2005) 24(4):229–35. doi: 10.2165/00139709-200524040-00003
100. Sano M, Tamura T. Hydrogen gas therapy: from preclinical studies to clinical trials. Curr Pharm Des. (2021) 27(5):650–8. doi: 10.2174/1381612826666201221150857
101. Persson S, Edlund MB, Claesson R, Carlsson J. The formation of hydrogen sulfide and methyl mercaptan by oral bacteria. Oral Microbiol Immunol. (1990) 5(4):195–201. doi: 10.1111/j.1399-302X.1990.tb00645.x
102. Basic A, Blomqvist S, Carlén A, Dahlén G. Estimation of bacterial hydrogen sulfide production in vitro. J Oral Microbiol. (2015) 7(1). doi: 10.3402/jom.v7.28166
103. Van Der Hoeven JS, Van Den Kieboom CW, Schaeken MJ. Sulfate-reducing bacteria in the periodontal pocket. Oral Microbiol Immunol. (1995) 10(5):288–90. doi: 10.1111/j.1399-302X.1995.tb00156.x
104. Langendijk PS, Kulik EM, Sandmeier H, Meyer J, van der Hoeven JS. Isolation of Desulfomicrobium orale sp. Nov. And Desulfovibrio strain NY682, oral sulfate-reducing bacteria involved in human periodontal disease. Int J Syst Evol Microbiol. (2001) 51(3):1035–44. doi: 10.1099/00207713-51-3-1035
105. Basic A, Blomqvist M, Dahlén G, Svensäter G. The proteins of Fusobacterium spp. Involved in hydrogen sulfide production from L-cysteine. BMC Microbiol. (2017) 17(1). doi: 10.1186/s12866-017-0967-9
106. Claesson R, Edlund MB, Persson S, Carlsson J. Production of volatile sulfur compounds by various Fusobacterium species. Oral Microbiol Immunol. (1990) 5(3):137–42. doi: 10.1111/j.1399-302X.1990.tb00411.x
107. Yano T, Fukamachi H, Yamamoto M, Igarashi T. Characterization of L-cysteine desulfhydrase from Prevotella intermedia. Oral Microbiol Immunol. (2009) 24(6):485–92. doi: 10.1111/j.1399-302X.2009.00546.x
108. Morita M, Wang HL. Relationship of sulcular sulfide level to severity of periodontal disease and BANA test. J Periodontol. (2001) 72(1):74–8. doi: 10.1902/jop.2001.72.1.74
109. Morhart RE, Mata LJ, Sinskey AJ, Harris RS. A microbiological and biochemical study of gingival crevice debris obtained from Guatemalan mayan Indians. J Periodontol. (1970) 41(11):644–9. doi: 10.1902/jop.1970.41.11.644
110. Persson S. Hydrogen sulfide and methyl mercaptan in periodontal pockets. Oral Microbiol Immunol. (1992) 7(6):378–9. doi: 10.1111/j.1399-302X.1992.tb00641.x
111. Icgen B, Harrison S. Exposure to sulfide causes populations shifts in sulfate-reducing consortia. Res Microbiol. (2006) 157(8):784–91. doi: 10.1016/j.resmic.2006.04.004
112. Shatalin K, Shatalina E, Mironov A, Nudler E. H2s: a universal defense against antibiotics in bacteria. Science. (2011) 334(6058):986–90. doi: 10.1126/science.1209855
113. Darland G, Davis BR. Biochemical and serological characterization of hydrogen sulfide positive variants of Escherichia coli. Appl Microbiol. (1974) 27(1):54–8. doi: 10.1128/am.27.1.54-58.1974
114. Toliver-Kinsky T, Cui W, Törö G, Lee SJ, Shatalin K, Nudler E, et al. H2s, a bacterial defense mechanism against the host immune response. Infect Immun. (2019) 87(1). doi: 10.1128/IAI.00272-18
115. Zhang Z, Fang X, Yang X, Mitsui T, Huang Y, Mao Z, et al. Hydrogen sulfide donor NaHS alters antibody structure and function via sulfhydration. Int Immunopharmacol. (2019) 73:491–501. doi: 10.1016/j.intimp.2019.05.052
116. Mironov A, Seregina T, Nagornykh M, Luhachack LG, Korolkova N, Lopes LE, et al. Mechanism of H2S-mediated protection against oxidative stress in Escherichia coli. Proc Natl Acad Sci U S A. (2017) 114(23):6022–7. doi: 10.1073/pnas.1703576114
117. Audrain B, Farag MA, Ryu CM, Ghigo JM. Role of bacterial volatile compounds in bacterial biology. FEMS Microbiol Rev. (2015) 39(2):222–33. doi: 10.1093/femsre/fuu013
118. Dorman DC, Moulin FJM, McManus BE, Mahle KC, James RA, Struve MF. Cytochrome oxidase inhibition induced by acute hydrogen sulfide inhalation: correlation with tissue sulfide concentrations in the rat brain, liver, lung, and nasal epithelium. Toxicol Sci. (2002) 65(1):18–25. doi: 10.1093/toxsci/65.1.18
119. Hughes MN, Centelles MN, Moore KP. Making and working with hydrogen sulfide. The chemistry and generation of hydrogen sulfide in vitro and its measurement in vivo: a review. Free Radic Biol Med. (2009) 47(10):1346–53. doi: 10.1016/j.freeradbiomed.2009.09.018
120. Basic A, Serino G, Leonhardt Å, Dahlén G. H2s mediates increased interleukin (IL)-1β and IL-18 production in leukocytes from patients with periodontitis. J Oral Microbiol. (2019) 11(1). doi: 10.1080/20002297.2019.1617015
121. Basic A, Alizadehgharib S, Dahlén G, Dahlgren U. Hydrogen sulfide exposure induces NLRP3 inflammasome-dependent IL-1β and IL-18 secretion in human mononuclear leukocytes in vitro. Clin and Exp Dental Res. (2017) 3(3):115–20. doi: 10.1002/cre2.69
122. Dilek N, Papapetropoulos A, Toliver-Kinsky T, Szabo C. Hydrogen sulfide: an endogenous regulator of the immune system. Pharmacol Res. (2020) 161. doi: 10.1016/j.phrs.2020.105119
123. Sogodogo E, Drancourt M, Grine G. Methanogens as emerging pathogens in anaerobic abscesses. Eur J Clin Microbiol and Inf Dis. (2019) 38(5):811–8. doi: 10.1007/s10096-019-03510-5
124. Chaudhary PP, Conway PL, Schlundt J. Methanogens in humans: potentially beneficial or harmful for health. Appl Microbiol Biotechnol. (2018) 102(7):3095–104. doi: 10.1007/s00253-018-8871-2
125. Stephen AS, Millhouse E, Sherry L, Aduse-Opoku J, Culshaw S, Ramage G, et al. In vitro effect of porphyromonas gingivalis methionine gamma lyase on biofilm composition and oral inflammatory response. PLoS ONE. (2016) 11(12). doi: 10.1371/journal.pone.0169157
126. Johnson P, Yaegakl K, Tonzetich J. Effect of methyl mercaptan on synthesis and degradation of collagen. J Periodontal Res. (1996) 31(5):323–9. doi: 10.1111/j.1600-0765.1996.tb00499.x
127. Ratkay LG, Waterfield JD, Tonzetich J. Stimulation of enzyme and cytokine production by methyl mercaptan in human gingival fibroblast and monocyte cell cultures. Arch Oral Biol. (1995) 40(4):337–44. doi: 10.1016/0003-9969(94)00165-8
128. Gokmenoglu C, Ozmeric N, Sungur C, Sahin Bildik R, Erguder I, Elgun S. Nitric oxide and arginase levels in peri-implant tissues after delayed loading. Arch Oral Biol. (2018) 85:207–11. doi: 10.1016/j.archoralbio.2017.10.019
129. Backlund CJ, Worley BV, Sergesketter AR, Schoenfisch MH. Kinetic-dependent killing of oral pathogens with nitric oxide. J Dent Res. (2015) 94(8):1092–8. doi: 10.1177/0022034515589314
130. Gusarov I, Shatalin K, Starodubtseva M, Nudler E. Endogenous nitric oxide protects bacteria against a wide spectrum of antibiotics. Science. (2009) 325(5946):1380–4. doi: 10.1126/science.1175439
131. Allaker RP, Silva Mendez LS, Hardie JM, Benjamin N. Antimicrobial effect of acidified nitrite on periodontal bacteria. Oral Microbiol Immunol. (2001) 16(4):253–6. doi: 10.1034/j.1399-302X.2001.160410.x
132. Marsh P, Lewis M, Rogers H, Williams D, Wilson M. Marsh and Martin's Oral microbiology. 6th ed.: Edinburgh: Churchill Livingstone (2016). 272.
133. Kuboniwa M, Sakanaka A, Hashino E, Bamba T, Fukusaki E, Amano A. Prediction of periodontal inflammation via metabolic profiling of saliva. J Dent Res. (2016) 95(12):1381–6. doi: 10.1177/0022034516661142
134. Sakanaka A, Kuboniwa M, Hashino E, Bamba T, Fukusaki E, Amano A. Distinct signatures of dental plaque metabolic byproducts dictated by periodontal inflammatory status. Sci Rep. (2017) 7. doi: 10.1038/srep42818
135. Singer RE, Buckner BA. Butyrate and propionate: important components of toxic dental plaque extracts. Infect Immun. (1981) 32(2):458–63. doi: 10.1128/iai.32.2.458-463.1981
136. Tan J, McKenzie C, Potamitis M, Thorburn AN, Mackay CR, Macia L. The role of short-chain fatty acids in health and disease. Adv Immunol. (2014) 121:91–119. doi: 10.1016/B978-0-12-800100-4.00003-9
137. Lu R, Meng H, Gao X, Xu L, Feng X. Effect of non-surgical periodontal treatment on short chain fatty acid levels in gingival crevicular fluid of patients with generalized aggressive periodontitis. J Periodontal Res. (2014) 49(5):574–83. doi: 10.1111/jre.12137
138. Niederman R, Buyle-Bodin Y, Lu BY, Naleway C, Robinson P, Kent R. The relationship of gingival crevicular fluid short chain carboxylic acid concentration to gingival inflammation. J Clin Periodontol. (1996) 23(8):743–9. doi: 10.1111/j.1600-051X.1996.tb00604.x
139. Ozeki M, Nozaki T, Aoki J, Bamba T, Jensen KR, Murakami S, et al. Metabolomic analysis of gingival crevicular fluid using gas chromatography/mass spectrometry. Mass Spectrom (Tokyo). (2016) 5(1):A0047. doi: 10.5702/massspectrometry.A0047
140. Tábi T, Lohinai Z, Pálfi M, Levine M, Szöko E. CE-LIF determination of salivary cadaverine and lysine concentration ratio as an indicator of lysine decarboxylase enzyme activity. Anal Bioanal Chem. (2008) 391(2):647–51. doi: 10.1007/s00216-008-2026-8
141. Persson S, Claesson R, Carlsson J. The capacity of subgingival microbiotas to produce volatile sulfur compounds in human serum. Oral Microbiol Immunol. (1989) 4(3):169–72. doi: 10.1111/j.1399-302X.1989.tb00246.x
142. Torresyap G, Haffajee AD, Uzel NG, Socransky SS. Relationship between periodontal pocket sulfide levels and subgingival species. J Clin Periodontol. (2003) 30(11):1003–10. doi: 10.1034/j.1600-051X.2003.00377.x
143. Tonzetich J. Direct gas chromatographic analysis of sulphur compounds in mouth air in man. Arch Oral Biol. (1971) 16(6):587–97. doi: 10.1016/0003-9969(71)90062-8
144. Kleinberg I, Westbay G. Oral malodor. Crit Rev Oral Biol Med. (1990) 1(4):247–59. doi: 10.1177/10454411900010040401
145. Horowitz A, Folke LE. Hydrogen sulfide and periodontal disease. Periodontal Abstr. (1972) 20(2):59–62.4262421
146. Solis-Gaffar MC, Rustogi KN, Gaffar A. Hydrogen sulfide production from gingival crevicular fluid. J Periodontol. (1980) 51(10):603–6. doi: 10.1902/jop.1980.51.10.603
147. Basic A, Dahlén G. Hydrogen sulfide production from subgingival plaque samples. Anaerobe. (2015) 35:21–7. doi: 10.1016/j.anaerobe.2014.09.017
148. Stabler SP, Marcell PD, Podell ER, Allen RH. Quantitation of total homocysteine, total cysteine, and methionine in normal serum and urine using capillary gas chromatography-mass spectrometry. Anal Biochem. (1987) 162(1):185–96. doi: 10.1016/0003-2697(87)90026-1
149. Greabu M, Totan A, Miricescu D, Radulescu R, Virlan J, Calenic B. Hydrogen sulfide, oxidative stress and periodontal diseases: a concise review. Antioxidants. (2016) 5(1). doi: 10.3390/antiox5010003
150. Walsh BJC, Giedroc DP. H2s and reactive sulfur signaling at the host-bacterial pathogen interface. J Biol Chem. (2020) 295(38):13150–68. doi: 10.1074/jbc.REV120.011304
151. Nguyen-Hieu T, Khelaifia S, Aboudharam G, Drancourt M Methanogenic archaea in subgingival sites: a review. APMIS. (2013) 121(6):467–77. doi: 10.1111/apm.12015
152. Kulik EM, Sandmeier H, Hinni K, Meyer J. Identification of archaeal rDNA from subgingival dental plaque by PCR amplification and sequence analysis. FEMS Microbiol Lett. (2001) 196(2):129–33. doi: 10.1111/j.1574-6968.2001.tb10553.x
153. Cekici A, Kantarci A, Hasturk H, Van Dyke TE. Inflammatory and immune pathways in the pathogenesis of periodontal disease. Periodontol 2000. (2014) 64(1):57–80. doi: 10.1111/prd.12002
154. Albandar JM. Global risk factors and risk indicators for periodontal diseases. Periodontol 2000. (2002) 29(1):177–206. doi: 10.1034/j.1600-0757.2002.290109.x
155. Borrell LN, Papapanou PN. Analytical epidemiology of periodontitis. J Clin Periodontol. (2005) 32(S6):132–58. doi: 10.1111/j.1600-051X.2005.00799.x
156. Bouchard P, Carra MC, Boillot A, Mora F, Rangé H. Risk factors in periodontology: a conceptual framework. J Clin Periodontol. (2017) 44(2):125–31. doi: 10.1111/jcpe.12650
157. Sanz M, Ceriello A, Buysschaert M, Chapple I, Demmer RT, Graziani F, et al. Scientific evidence on the links between periodontal diseases and diabetes: consensus report and guidelines of the joint workshop on periodontal diseases and diabetes by the international diabetes federation and the European federation of periodontology. J Clin Periodontol. (2018) 45(2):138–49. doi: 10.1111/jcpe.12808
158. Jepsen S, Caton JG, Albandar JM, Bissada NF, Bouchard P, Cortellini P, et al. Periodontal manifestations of systemic diseases and developmental and acquired conditions: consensus report of workgroup 3 of the 2017 world workshop on the classification of periodontal and peri-implant diseases and conditions. J Clin Periodontol. (2018) 45:S219–29. doi: 10.1111/jcpe.12951
159. Leite FRM, Nascimento GG, Scheutz F, López R. Effect of smoking on periodontitis: a systematic review and meta-regression. Am J Prev Med. (2018) 54(6):831–41. doi: 10.1016/j.amepre.2018.02.014
160. Reis MAM, Lemos PC, Almeida JS, Carrondo MJT. Evidence for the intrinsic toxicity of H2S to sulphate-reducing bacteria. Appl Microbiol Biotechnol. (1991) 36(1):145–7. doi: 10.1007/BF00164716
161. Dahlen G, Basic A, Bylund J. Importance of virulence factors for the persistence of oral Bacteria in the inflamed gingival crevice and in the pathogenesis of periodontal disease. J Clin Med. (2019) 8(9). doi: 10.3390/jcm8091339
162. Hojo K, Nagaoka S, Ohshima T, Maeda N. Bacterial interactions in dental biofilm development. J Dent Res. (2009) 88(11):982–90. doi: 10.1177/0022034509346811
163. Tustin AW, Jones A, Lopez GP, Ketcham GR, Hodgson MJ. Occupational chemical exposures: a collaboration between the Georgia poison center and the occupational safety and health administration. Clin Toxicol (Phila). (2018) 56(1):55–62. doi: 10.1080/15563650.2017.1338718
164. Szabo C. A timeline of hydrogen sulfide (H2S) research: from environmental toxin to biological mediator. Biochem Pharmacol. (2018) 149:5–19. doi: 10.1016/j.bcp.2017.09.010
165. Meulenbelt J. Irritant gases. Medicine (Baltimore). (2016) 44(3):175–8. doi: 10.1016/j.mpmed.2015.12.004
166. Mielech A, Puścion-Jakubik A, Socha K. Assessment of the risk of contamination of food for infants and toddlers. Nutrients. (2021) 13(7). doi: 10.3390/nu13072358
167. Coburn RF, Blakemore WS, Forster RE. Endogenous carbon monoxide production in man. J Clin Invest. (1963) 42(7):1172–8. doi: 10.1172/JCI104802
168. Ghasemi A, Zahedi Asl S, Mehrabi Y, Saadat N, Azizi F. Serum nitric oxide metabolite levels in a general healthy population: relation to sex and age. Life Sci. (2008) 83(9–10):326–31. doi: 10.1016/j.lfs.2008.06.010
169. Mancardi D, Penna C, Merlino A, Del Soldato P, Wink DA, Pagliaro P. Physiological and pharmacological features of the novel gasotransmitter: hydrogen sulfide. Biochim Biophys Acta. (2009) 1787(7):864–72. doi: 10.1016/j.bbabio.2009.03.005
170. Kimura H. Hydrogen sulfide: from brain to gut. Antioxid Redox Signal. (2010) 12(9):1111–23. doi: 10.1089/ars.2009.2919
171. Kimura H. Hydrogen sulfide signalling in the CNS—comparison with NO. Br J Pharmacol. (2020) 177(22):5031–45. doi: 10.1111/bph.15246
172. Hosoki R, Matsuki N, Kimura H. The possible role of hydrogen sulfide as an endogenous smooth muscle relaxant in synergy with nitric oxide. Biochem Biophys Res Commun. (1997) 237(3):527–31. doi: 10.1006/bbrc.1997.6878
173. Koenitzer JR, Isbell TS, Patel HD, Benavides GA, Dickinson DA, Patel RP, et al. Hydrogen sulfide mediates vasoactivity in an O2-dependent manner. American Journal of Physiology—Heart and Circulatory Physiology. (2007) 292(4):H1953–60. doi: 10.1152/ajpheart.01193.2006
174. de Farias JO, de Freitas Lima SM, Rezende TMB. Physiopathology of nitric oxide in the oral environment and its biotechnological potential for new oral treatments: a literature review. Clin Oral Investig. (2020) 24(12):4197–212. doi: 10.1007/s00784-020-03629-2
175. Shen X, Pattillo CB, Pardue S, Bir SC, Wang R, Kevil CG. Measurement of plasma hydrogen sulfide in vivo and in vitro. Free Radic Biol Med. (2011) 50(9):1021–31. doi: 10.1016/j.freeradbiomed.2011.01.025
176. Chun-Mei J, Wu C, Guo-Liang M, Yue G, Ning C, Ji Y. Production of endogenous hydrogen sulfide in human gingival tissue. Arch Oral Biol. (2017) 74:108–13. doi: 10.1016/j.archoralbio.2016.11.016
177. Busa WB. Mechanisms and consequences of pH-mediated cell regulation. Annu Rev Physiol. (1986) 48:389–402. doi: 10.1146/annurev.ph.48.030186.002133
178. Nicholls P, Kim JK. Sulphide as an inhibitor and electron donor for the cytochrome c oxidase system. Can J Biochem. (1982) 60(6):613–23. doi: 10.1139/o82-076
179. Nicholls P, Marshall DC, Cooper CE, Wilson MT. Sulfide inhibition of and metabolism by cytochrome c oxidase. Biochem Soc Trans. (2013) 41(5):1312–6. doi: 10.1042/BST20130070
180. Beauchamp RO Jr, Bus JS, Popp JA. A critical review of the literature on hydrogen sulfide toxicity. Crit Rev Toxicol. (1984) 13(1):25–97. doi: 10.3109/10408448409029321
181. Filipovic MR. Persulfidation (S-sulfhydration) and H2S. In: Moore PK, Whiteman W, editors. Handbook of experimental pharmacology. Switzerland: Springer International Publishing (2015). p. 29–59.
182. Lennette E, Balows A, Hausler W, Shadomy H. Manual of clinical microbiology. 4th ed. Washington DC: America Society for Microbiology (1985). 385.
183. Sen N. Functional and molecular insights of hydrogen sulfide signaling and protein sulfhydration. J Mol Biol. (2017) 429(4):543–61. doi: 10.1016/j.jmb.2016.12.015
184. Kimura H. Hydrogen polysulfide (H2Sn) signaling along with hydrogen sulfide (H2S) and nitric oxide (NO). J Neural Transm. (2016) 123(11):1235–45. doi: 10.1007/s00702-016-1600-z
185. Cueno ME, Ochiai K. Re-discovering periodontal butyric acid: new insights on an old metabolite. Microb Pathog. (2015) 94:48–53. doi: 10.1016/j.micpath.2015.10.006
186. Botta GA, Radin L, Costa A, Schito G, Blasi G. Gas-liquid chromatography of the gingival fluid as an aid in periodontal diagnosis. J Periodontal Res. (1985) 20(5):450–7. doi: 10.1111/j.1600-0765.1985.tb00827.x
187. Kurita-Ochiai T, Fukushima K, Ochiai K. Lipopolysaccharide stimulates butyric acid-induced apoptosis in human peripheral blood mononuclear cells. Infect Immun. (1999) 67(1):22–9. doi: 10.1128/IAI.67.1.22-29.1999
188. Kurita-Ochiai T, Seto S, Suzuki N, Yamamoto M, Otsuka K, Abe K, et al. Butyric acid induces apoptosis in inflamed fibroblasts. J Dent Res. (2008) 87(1):51–5. doi: 10.1177/154405910808700108
189. Shirasugi M, Nakagawa M, Nishioka K, Yamamoto T, Nakaya T, Kanamura N. Relationship between periodontal disease and butyric acid produced by periodontopathic bacteria. Inflamm Regen. (2018) 38:23. doi: 10.1186/s41232-018-0081-x
190. Zhang H, Bhatia M. Hydrogen sulfide: a novel mediator of leukocyte activation. Immunopharmacol Immunotoxicol. (2008) 30(4):631–45. doi: 10.1080/08923970802278045
191. Whiteman M, Winyard PG. Hydrogen sulfide and inflammation: the good, the bad, the ugly and the promising. Expert Rev Clin Pharmacol. (2011) 4(1):13–32. doi: 10.1586/ecp.10.134
192. Mariggiò MA, Pettini F, Fumarulo R. Sulfide influence on polymorphonuclear functions: a possible role for Ca2 + involvement. Immunopharmacol Immunotoxicol. (1997) 19(3):393–404. doi: 10.3109/08923979709046984
193. Pálinkás Z, Furtmüller PG, Nagy A, Jakopitsch C, Pirker KF, Magierowski M, et al. Interactions of hydrogen sulfide with myeloperoxidase. Br J Pharmacol. (2015) 172(6):1516–32. doi: 10.1111/bph.12769
194. Miao L, Shen X, Whiteman M, Xin H, Shen Y, Xin X, et al. Hydrogen sulfide mitigates myocardial infarction via promotion of mitochondrial biogenesis-dependent M2 polarization of macrophages. Antioxidants and Redox Signaling. (2016) 25(5):268–81. doi: 10.1089/ars.2015.6577
195. Whiteman M, Li L, Rose P, Tan CH, Parkinson DB, Moore PK. The effect of hydrogen sulfide donors on lipopolysaccharide-induced formation of inflammatory mediators in macrophages. Antioxidants and Redox Signaling. (2010) 12(10):1147–54. doi: 10.1089/ars.2009.2899
196. Chen W, Kajiya M, Giro G, Ouhara K, Mackler HE, Mawardi H, et al. Bacteria-derived hydrogen sulfide promotes IL-8 production from epithelial cells. Biochem Biophys Res Commun. (2010) 391(1):645–50. doi: 10.1016/j.bbrc.2009.11.113
197. Zhang JH, Dong Z, Chu L. Hydrogen sulfide induces apoptosis in human periodontium cells. J Periodontal Res. (2010) 45(1):71–8. doi: 10.1111/j.1600-0765.2009.01202.x
198. Chi XP, Ouyang XY, Wang YX. Hydrogen sulfide synergistically upregulates Porphyromonas gingivalis lipopolysaccharide-induced expression of IL-6 and IL-8 via NF-κB signalling in periodontal fibroblasts. Arch Oral Biol. (2014) 59(9):954–61. doi: 10.1016/j.archoralbio.2014.05.022
199. Mirandola P, Gobbi G, Sponzilli I, Pambianco M, Malinverno C, Cacchioli A, et al. Exogenous hydrogen sulfide induces functional inhibition and cell death of cytotoxic lymphocytes subsets. J Cell Physiol. (2007) 213(3):826–33. doi: 10.1002/jcp.21151
200. Pétrilli V, Papin S, Dostert C, Mayor A, Martinon F, Tschopp J. Activation of the NALP3 inflammasome is triggered by low intracellular potassium concentration. Cell Death Differ. (2007) 14(9):1583–9. doi: 10.1038/sj.cdd.4402195
201. Coppi M, Niederman R. Effects of ammonia on human neutrophil N-formyl chemotactic peptide receptor-ligand interaction and cytoskeletal association. Biochem Biophys Res Commun. (1989) 165(1):377–83. doi: 10.1016/0006-291X(89)91081-4
202. Chen KY, Morris JC. Kinetics of oxidation of aqueous sulfide by O2. Environ Sci Technol. (1972) 6(6):529–37. doi: 10.1021/es60065a008
203. Kimura H. Signaling molecules: hydrogen sulfide and polysulfide. Antioxidants and Redox Signaling. (2015) 22(5):362–76. doi: 10.1089/ars.2014.5869
204. Van Dyke TE, Bartold PM, Reynolds EC. The nexus between periodontal inflammation and dysbiosis. Front Immunol. (2020) 11. doi: 10.3389/fimmu.2020.00511
205. Ebersole JL, Dawson DA 3rd, Emecen Huja P, Pandruvada S, Basu A, Nguyen L, et al. Age and periodontal health—immunological view. Curr Oral Health Rep. (2018) 5(4):229–41. doi: 10.1007/s40496-018-0202-2
206. Loos BG, Van Dyke TE. The role of inflammation and genetics in periodontal disease. Periodontol 2000. (2020) 83(1):26–39. doi: 10.1111/prd.12297
207. Charalampakis G, Dahlen G, Carlen A, Leonhardt A. Bacterial markers vs. Clinical markers to predict progression of chronic periodontitis: a 2-yr prospective observational study. Eur J Oral Sci. (2013) 121(5):394–402. doi: 10.1111/eos.12080
208. Hoglund Aberg C, Kwamin F, Claesson R, Dahlen G, Johansson A, Haubek D. Progression of attachment loss is strongly associated with presence of the JP2 genotype of Aggregatibacter actinomycetemcomitans: a prospective cohort study of a young adolescent population. J Clin Periodontol. (2014) 41(3):232–41. doi: 10.1111/jcpe.12209
209. Haubek D, Ennibi OK, Poulsen K, Vaeth M, Poulsen S, Kilian M. Risk of aggressive periodontitis in adolescent carriers of the JP2 clone of Aggregatibacter (Actinobacillus) actinomycetemcomitans in Morocco: a prospective longitudinal cohort study. Lancet. (2008) 371(9608):237–42. doi: 10.1016/S0140-6736(08)60135-X
210. Barros SP, Williams R, Offenbacher S, Morelli T. Gingival crevicular fluid as a source of biomarkers for periodontitis. Periodontol 2000. (2016) 70(1):53–64. doi: 10.1111/prd.12107
Keywords: host-microbe interplay, periodontal diseases, inflammation, bacterial metabolites, proteolytic activity, hydrogen sulfide, ammonia, short chain fatty acids
Citation: Basic A and Dahlén G (2023) Microbial metabolites in the pathogenesis of periodontal diseases: a narrative review. Front. Oral. Health 4:1210200. doi: 10.3389/froh.2023.1210200
Received: 21 April 2023; Accepted: 31 May 2023;
Published: 14 June 2023.
Edited by:
Georgios N. Belibasakis, Karolinska Institutet (KI), SwedenReviewed by:
Roger Junges, University of Oslo, Norway© 2023 Basic and Dahlén. This is an open-access article distributed under the terms of the Creative Commons Attribution License (CC BY). The use, distribution or reproduction in other forums is permitted, provided the original author(s) and the copyright owner(s) are credited and that the original publication in this journal is cited, in accordance with accepted academic practice. No use, distribution or reproduction is permitted which does not comply with these terms.
*Correspondence: Amina Basic YW1pbmEuYmFzaWNAZ3Uuc2U=
Disclaimer: All claims expressed in this article are solely those of the authors and do not necessarily represent those of their affiliated organizations, or those of the publisher, the editors and the reviewers. Any product that may be evaluated in this article or claim that may be made by its manufacturer is not guaranteed or endorsed by the publisher.
Research integrity at Frontiers
Learn more about the work of our research integrity team to safeguard the quality of each article we publish.