- 1Department of Oral and Maxillofacial Surgery, Hadassah Medical Center, Faculty of Dental Medicine, The Hebrew University of Jerusalem, Jerusalem, Israel
- 2The Institute of Biomedical and Oral Research, The Hebrew University of Jerusalem, Jerusalem, Israel
- 3Department of Oral and Maxillofacial Surgery, Boston University and Boston Medical Center, Boston, MA, United States
- 4Earle A. Chiles Research Institute, Robert W. Franz Cancer Center, Providence Portland Medical Center, Portland, OR, United States
Although treatment modalities for head and neck cancer have evolved considerably over the past decades, survival rates have plateaued. The treatment options remained limited to definitive surgery, surgery followed by fractionated radiotherapy with optional chemotherapy, and a definitive combination of fractionated radiotherapy and chemotherapy. Lately, immunotherapy has been introduced as the fourth modality of treatment, mainly administered as a single checkpoint inhibitor for recurrent or metastatic disease. While other regimens and combinations of immunotherapy and targeted therapy are being tested in clinical trials, adapting the appropriate regimens to patients and predicting their outcomes have yet to reach the clinical setting. Radiotherapy is mainly regarded as a means to target cancer cells while minimizing the unwanted peripheral effect. Radiotherapy regimens and fractionation are designed to serve this purpose, while the systemic effect of radiation on the immune response is rarely considered a factor while designing treatment. To bridge this gap, this review will highlight the effect of radiotherapy on the tumor microenvironment locally, and the immune response systemically. We will review the methodology to identify potential targets for therapy in the tumor microenvironment and the scientific basis for combining targeted therapy and radiotherapy. We will describe a current experience in preclinical models to test these combinations and propose how challenges in this realm may be faced. We will review new players in targeted therapy and their utilization to drive immunogenic response against head and neck cancer. We will outline the factors contributing to head and neck cancer heterogeneity and their effect on the response to radiotherapy. We will review in-silico methods to decipher intertumoral and intratumoral heterogeneity and how these algorithms can predict treatment outcomes. We propose that (a) the sequence of surgery, radiotherapy, chemotherapy, and targeted therapy should be designed not only to annul cancer directly, but to prime the immune response. (b) Fractionation of radiotherapy and the extent of the irradiated field should facilitate systemic immunity to develop. (c) New players in targeted therapy should be evaluated in translational studies toward clinical trials. (d) Head and neck cancer treatment should be personalized according to patients and tumor-specific factors.
1. Introduction
1.1. Head and neck cancer
Head and neck squamous cell carcinoma (HNSCC) accounted for approximately 878,000 newly diagnosed cases worldwide in 2020 (1), and approximately 68% of patients with oral cavity and pharyngeal cancer are expected to survive five years (2). The two conventional approaches to treating HNSCC are primary surgery followed by risk-adapted chemoradiotherapy or upfront definitive chemoradiotherapy. Chemotherapy for HNSCC is mainly based on high-dose cisplatin and fractionated radiation therapy (RT) delivered to a total of 66–70 Gy. For patients with advanced comorbidities or poor performance status, these approaches often lead to unacceptable treatment-associated morbidity and mortality. Recurrent or metastatic (R/M) HNSCC poses an even greater challenge as only one-third of patients respond to treatment, primarily chemoradiotherapy, and the median survival period is 6–8 months (3).
1.2. Radiotherapy
RT has evolved over the years, and more than ever, it targets cancer cells. Its design serves this purpose by utilizing the principles of radiation physics and fractioning into smaller doses. The conventional RT that most patients undergo is fractionated, during which small doses of radiation (around 2 Gy per fraction) are delivered daily. This method presumably allows for normal tissue to undergo repair better than tumor tissue (4), thus targeting the destructive radiation effect on cancer cells more than on their surrounding healthy counterparts.
An alternative fractionation method is based on delivering high-dose radiation in either a single dose or a limited number of doses. Defined as hypofractionation, or stereotactic body radiotherapy (SBRT), this method enables a high radiation dose to be focused on a specific location while maintaining a steep dose gradient beyond (5). For patients who are unable to withstand the prolonged fractionated RT regimen or surgery, primary SBRT has yielded impressive local control and overall survival (OS) rates while maintaining relatively low radiation-related adverse features (6–8).
1.3. The immunogenic radiation and SBRT
SBRT can be seen, like surgery, as an opportunity to focally treat a cancer site. For many years, the effect of RT on the immune system was generally perceived as immunosuppressive. It was backed by data showing lymphopenia, leukocyte cytotoxicity, and impaired leukocyte function in response to RT (9–12).
However, a growing amount of evidence supports an additional, synergistic effect of RT. The synergistic effect, under certain conditions, functions as an in-situ vaccine that primes the immune response both locally and systemically (13, 14), and drives the immune response to control distant disease (15). Preclinical models and clinical reports have linked the induction of the immune response by RT to the abscopal effect. This effect is evident when locally irradiating a primary tumor and consequently witnessing the regression of distant metastases outside the irradiated field (16, 17). This process clearly stems from a systemic response to radiation, pointing to the immune system as a potential key factor in this process.
A different synergistic effect is demonstrated by the radiation-induced changes in the tumor environment and its surviving cancer cells to drive an immune-mediated local clearance of residual disease. Radiation-induced cell damage triggers the tumor to release antigens, which have the potential to generate new T-cells to attack the tumor with antigen specificities that were not formerly involved—termed epitope spreading (10, 18, 19) (Figure 1). However, antigen release alone is insufficient, as innate adjuvants are essential for effective immunity (20). In the sterile immunity of radiation-induced cell death, these innate adjuvants are endogenous adjuvants released by dying cancer cells (20, 21). Understanding the pattern of innate adjuvants released by dying cancer cells is critical, since some forms of cell death are differentially immunogenic (21–23). Similarly, the cell types that respond to these adjuvants and their differentiation dramatically impact the immune consequences of cancer cell death and adjuvant release (24–29). Thus, if the response to radiation is optimal, cancer cell death will release antigen and adjuvant to promote dendritic cells (DC) maturation to boost existing T-cell responses and generate new T-cell responses. It will also generate a pro-inflammatory environment in the tumor to help attract effector T-cells and guide myeloid differentiation into anti-tumor patterns. A suboptimal response will fail to mature DC (30, 31), and generate suppressive cytokine release from cells, such as M2-differentiated macrophages in the tumor environment (25).
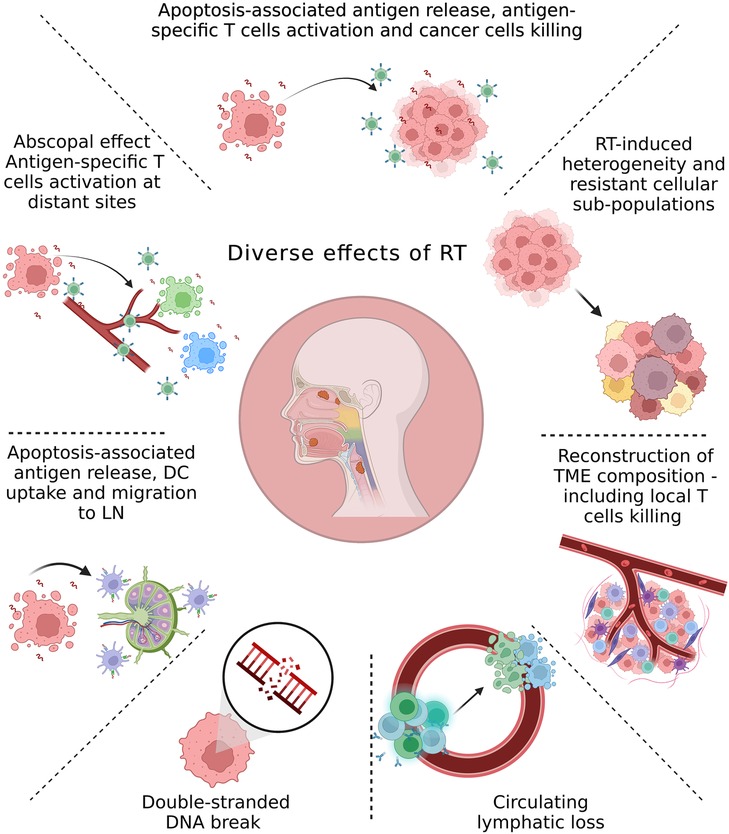
Figure 1. Overview of the main radiation-induced changes in the tumor environment and cancer cells. The local and systemic effects of irradiation can be linked to the radiation dose and fractionation. Although the initial effect of radiation therapy (RT) is cell death due to DNA damage, accumulating evidence from recent studies demonstrates multiple local and systemic molecular alterations induced by RT. These include antigen release and antigen-specific T-cells activation; molecular changes in the tumor microenvironment (TME) due to direct effects of RT or interactions between dying cells and the TME; RT-induced development of resistant subpopulations within the tumor; lymphocyte loss; abscopal effect; trafficking of dendritic cells (DC) from tumors to the tumor-draining lymph node (LN).
The local and systemic effects can be linked to the radiation dose and fractionation, and no discussion of RT is complete without their appreciation. Basic radiobiology demonstrates that splitting doses into multiple fractions has non-linear effects on radiation-mediated cell death (32). This concept was demonstrated in the clinical setting after the introduction of SBRT into the field of intracranial tumors (33), and was followed by its application to extracranial sites using ablative doses of radiation (8–30 Gy per fraction) (4).
Since then, it was shown that SBRT could be both effective and well-tolerated in various types of cancer, including non-small-cell lung cancer (NSCLC) (34), with local control rates of above 90% at three years (35–37); and prostate cancer with progression-free survival (PFS) rates of 97% at five years (38). In contrast to lymphopenia that may be triggered by conventional RT, administering neoadjuvant SBRT concurrently with durvalumab (anti-PD1) in HNSCC led to increased white blood cell counts (39). We will review the immunological basis of dose and fractionation-dependent effects, and describe the related data from preclinical models and clinical trials in head and neck cancer.
1.4. Immunotherapy
Immunotherapy has been developing rapidly over the last decade. It has the potential to activate an immune response to target cancer cells by utilizing the function of the immune system to survey the body for abnormal cells and eliminate them continually. Cancer cells that were not eliminated can exist in equilibrium with the immune response until their eventual evasion from it, defined as immune escape (40). The balance may be tilted in favor of the immune response with targeted therapy.
Programmed cell death protein 1 (PD-1) is a receptor expressed on immune cells that drives the downregulation of the immune response (41) and is blocked by immune checkpoint inhibitors. Nivolumab and pembrolizumab, both PD-1 checkpoint inhibitors, were approved by the Food and Drug Administration (FDA) in 2016 as second-line treatment modalities for R/M HNSCC, following clinical trials demonstrating a favorable response in platinum-refractory R/M HNSCC (42, 43). Although the absolute number of months added to OS in these trials was limited, one must remember they were conducted in the R/M setting (42, 44–46).
In 2019, pembrolizumab was approved as first-line monotherapy for R/M HNSCC or in combination with chemotherapy. Due to the low response rates to monotherapy checkpoint inhibitors in R/M HNSCC, more clinical trials focus on combining immunotherapeutic agents, concurrent immunotherapy and chemotherapy, and concurrent immunotherapy and RT. However, the results of trials adding immunotherapy to the standard treatment for locally-advanced HNSCC have not been as promising as expected. The JAVELIN Head and Neck 100 trial tested the addition of a programmed cell death ligand 1 (PD-L1) inhibitor to chemoradiotherapy and was halted when the primary objective of prolonging PFS was not reached (47). The GORTEC 2015-01 PembroRad trial replaced high-dose cisplatin with pembrolizumab in combination with RT, and showed similar disease control rates (48). The outcomes of these trials underscore the complexity in assigning the most suitable treatment to a particular cancer patient and predicting its success (49).
2. The immunogenic radiation
2.1. Introduction to dose/fractionation-dependent effects
Technological developments in physics and computing have permitted highly conformal targeting of tumors while avoiding normal tissues. It allowed higher doses of radiation to be delivered in fewer fractions and optimization of RT-fractionation to permit tumor-selective death. Currently, most patients are treated with standard fractionation with individual doses at or below 2 Gy, and treatments are delivered over several weeks. In HNSCC, these doses add up to 66–70 Gy over 6–7 weeks and may be delivered concurrently with chemotherapy.
In preclinical models, however, a daily regimen of 2 Gy fractionation over multiple weeks is rarely tested (50). First, it stems from a practical limitation in the growth rate and timeline of murine models. Second, fractionated radiation negatively affects the proliferating T-cells, and studies are generally designed to avoid this effect. For example, in a model where high-dose RT (30 Gy) resulted in effective CD8+ T-cells anti-tumor response, adding fractionated RT (3 Gy ×10) decreased tumor control (51). These data demonstrate that extending the timeline of radiation treatment can kill T-cells which are critical for tumor control (52).
As for the radiation dose, this area of research has not reached a firm conclusion. In some cases, preclinical models show that higher radiation doses lead to optimal synergism with immune combinations. For example, 5–8 Gy per fraction has been successfully employed (53–55), which is consistent with the optimal dose which led to the release of immunological adjuvants from cancer cells following RT (56, 57).
Morisada et al. compared the effect of administering 2 and 8 Gy RT to mouse oral cancer cells in-vitro and in-vivo. 8 Gy induced greater tumor-cells susceptibility to T-cell cytotoxicity than 2 Gy, and dose-dependency was demonstrated in terms of antigen release, antigen-specific T-cells activation, and cytotoxic targeting of cells (58). The same group later showed enhanced anti-tumor immunity when administering hypofractionated RT (8 Gy ×2), compared to hyperfractionated RT (2 Gy ×10). When RT was combined with PD-1 blockade, better control of primary and distant tumors was achieved (59).
In contrast, other preclinical studies have shown that synergy with immunotherapy was achieved at low doses of RT (60–63).
Clinical studies are no different. While some use higher doses of radiation in combination with immunotherapy (64–66), others use conventional fractionation (67). Certain immunotherapies likely require specific radiation dose as well as an optimal timeline of delivery (68), each deserves preclinical modeling prior to its clinical translation (50).
2.2. Direct effects of radiation on immune cells in the field
T-cells are a dynamic population that recirculates in and out of tissues via the draining lymphatics and back into the peripheral blood (69). Hence, understanding the effect of RT on T-cells should encompass data from all sites: The peripheral blood, the tissues, and the draining lymphatics.
The clearest data relating to the impact of radiation on the immune profile of tumors is the direct radiation-mediated killing of T-cells in the treatment field [reviewed in (52)]. Systemic lymphocyte loss is another immune-related outcome observed in patients treated with conventionally fractionated radiation (Figure 1) (11, 12, 70–75), though alterations in dose and fractionation can limit this effect (76, 77).
Following total body radiation, T-cells in the tumor are relatively radio-resistant compared to circulating T-cells (78) but are still killed by focal radiation therapy (78, 79). This is critical since the tumor is enriched for tumor antigen-specific T-cells, and tumors with a more significant proportion of tumor-specific T-cells are associated with improved prognosis (80). If radiation of the tumor eliminated all tumor-specific T-cells, then it would likely be a poor partner for T-cell-targeted immunotherapies (52). However, since T-cells recirculate in and out of tissues via lymphatics and back into the peripheral blood (69), a transient local loss of T-cells following treatment can be recovered by infiltration from the circulation, and local proliferation (81). Notably, irradiation of T-cells in the tumor-draining lymph node can impair reconstitution and impair tumor control by radiation and some immunotherapy combinations (82). These data suggest that reconstitution from some non-tumor sources is critical to the success of some radiation and immunotherapy combinations.
In-vitro studies exposing circulating blood cells to low doses of RT ex-vivo have demonstrated that a significant increase in T-cell death was detectable at 0.125 Gy, and approximately half of the T-cells underwent apoptosis at 2 Gy (83). By contrast, myeloid populations were relatively radio-resistant at these doses (83, 84). Among myeloid populations, DC and Langerhans cells have been shown to be more radio-resistant than T-cell populations (83, 85–87). When monocytes are differentiated into DC ex-vivo, the cells become less radiosensitive (83), in part due to the terminally differentiated and non-proliferative status of DC (85, 86, 88). However, DC can be directly impacted by radiation. Human DC given high-dose radiation (30 Gy) demonstrated a suppressed co-stimulatory phenotype and MHC Class II expression (89). Irradiation of murine bone marrow-derived DC has been shown to affect antigen-presentation pathways and their ability to generate T-cell responses following injection into mice (85). Irradiation (30 Gy) of human monocyte-derived DC resulted in inhibited IL-12 secretion and reduced ability to stimulate T-cells, but phagocytosis and migration were not impaired (88). Similarly, radiation doses above 6 Gy resulted in decreased IL-23 secretion and decreased ex-vivo Th17 priming ability (90). These data suggest that while DC are radio-resistant, they are susceptible to radiation-mediated direct effects.
It is important to note that these studies of DC use higher doses than are typically used for in-vitro studies, and in ex-vivo studies, the positive impact of radiation-induced adjuvant release is not well modeled. Using a fluorescence model to label infiltrating cells in murine tumors, we observed the trafficking of dendritic cells from tumors to the tumor-draining lymph node (30). Treatment of the tumors with 12 Gy resulted in migration and maturation of DC in radio-immunogenic tumor models but not in poorly radio-immunogenic models (30). The difference between these models is that the poorly radio-immunogenic model had minimal T-cell involvement in tumor control following radiation (24, 31), suggesting that DC migration is a potential reason for the discrepancy. However, since each model was treated with the same 12 Gy radiation dose, it demonstrates that DC can be fully functional when exposed to high single doses of RT in-vivo and can be a critical cell type to propagate immune responses following RT.
2.3. The immune effect of treating lymph nodes of the neck
Elective neck irradiation is frequently employed to irradicate microscopic disease in the draining lymph nodes of the neck. However, as lymph nodes are the site in which tumor-specific T-cells reside, antigen-presenting cells are primed, and central memory is established (91–95), irradiation of the draining lymph nodes may hinder the immune response and the effect of immunotherapy.
Using an in-vivo model of HNSCC, Darragh et al. administered 8 Gy ×3 to the primary oral tumor, with or without elective nodal irradiation. The resulting data showed that sparing the neck led to better local control, better distant control, induction of epitope spreading, increased activation of CD8+ T-cells, and no lung metastases. However, while sparing the neck led to better local and distant control, regional recurrence was observed only in this group. Elective neck dissection performed five days after tumor cells implantation demonstrated similar results to elective nodal irradiation (91). These data suggest that while maintaining the integrity of the draining lymph nodes may induce immune response propagating to local and distant control, their persistence may eventually lead to regional recurrence. Notably, removing the sentinel lymph nodes was sufficient to prevent regional recurrence (91). Thus, the timing of surgery relative to immunotherapy and RT is critical. While upfront surgery followed by an immunotherapy-RT combination led to worse local control and immune response, a neoadjuvant immunotherapy-RT combination followed by surgery resulted in better local control and systemic immunity. This benefit was maintained even if the neck lymph nodes were removed, either entirely or by removing sentinel lymph nodes alone, as long as they were removed after administering immunotherapy and SBRT to the primary tumor (91).
Similar results were observed when checkpoint inhibitors were preceded by neck dissection or high-dose neck RT in murine orthotopic tongue tumors, both significantly reducing OS (96). The tumor-draining lymph nodes were found to be the critical component for immune checkpoint inhibitors response after ipsilateral, and not contralateral, neck dissection led to a compromised response to immune checkpoint inhibitors in a lateralized orthotopic model (96). A significant increase in CD45-negative cells and a decrease in the amount of CD8+ and CD4+ T-cells within the tumor was observed in the neck dissection group (96). Complete response of murine orthotopic tongue tumors was achieved following anti-CTLA-4 (cytotoxic T-lymphocyte–associated antigen 4) or anti-PD-1, and this effect was unchanged following a subsequent late (+6 days) neck dissection. However, an early neck dissection (+1 day) hindered the complete response (96). These data suggest that administering immunotherapy should commence in the neoadjuvant setting, and that neck irradiation or dissection should be timed late enough to allow systemic immunity to develop.
2.4. Cancer cell death as a source of antigen and adjuvant
Many other changes that occur in the tumor immune environment following radiation are secondary to the effects of radiation on cancer cells (Figure 1). The primary focus of RT is cancer cell death, which necessitates phagocytic clearance, a defining feature of the immune response in the vicinity of dying cells (22, 97, 98). The interaction of dying cancer cells with phagocytic myeloid cells in the tumor environment can drive their differentiation into suppressive states that limit the immune control of tumors following radiation (99, 100). However, DC uptake and cross-presentation of tumor-associated antigen is critical for subsequent T-cell responses in the tumor-draining lymph nodes (101).
This cross-presentation of cell-associated antigen to T-cells provides Signal 1 to T-cells via cognate interaction with the T-cell receptor. Signal 2 is an essential second step in T-cell activation provided by the antigen-presenting cell in response to adjuvant signals in their environment. Signal 2 is delivered by costimulatory molecules such as CD80 and CD86 that are induced on antigen-presenting cells following their exposure to innate adjuvants, as well as following antigen presentation to CD4+ T-cells (29, 102–105). In the case of infectious disease, these immunological adjuvants are bacterial or viral components directly recognized by Toll-Like Receptor or similar pathways in the antigen-presenting cell. T-cells receive signal 2 through CD28, which synergizes with TCR ligation to activate critical activation pathways in the T-cell (106–110). Signal 1 without signal 2 can tolerize T-cells or result in their deletion (111, 112), so without immunological adjuvant release, cancer cell death is potentially able to delete tumor antigen-specific T-cells.
Importantly, as we will discuss later, a range of immunological adjuvants being released following radiation-mediated cancer cell death in-vitro and in-vivo have been described (20, 21, 113, 114). Together, cancer cell death following RT has the potential to provide signal 1 to T-cells following antigen release from dying cells via antigen delivered to cross-presenting DC, and signal 2 in the form of endogenous adjuvants. This can cause DC maturation, migration to the draining lymph nodes, and upregulation of CD80 and CD86. In this way, DC are the immunological mechanism that links cancer cell death to signal 1 and signal 2 in T-cells. Notably, the degree of DC maturation varies between preclinical tumor models exposed to identical RT (31), which in turn affects DC migration to the tumor-draining lymph node and, therefore, T-cell involvement in the control of residual disease following radiation (24, 30). Factors contributing to this heterogeneity in radiation response and methods to analyze heterogeneity will be discussed later.
2.5. The abscopal effect
Other than the direct effects of radiation on immune cell types discussed above, a large portion of the early work in the immune aspect of RT resulted from studies exploring the mechanisms of post-radiation fibrosis, and the elusive abscopal effect. The abscopal effect is evident when locally irradiating a primary tumor and consequently witnessing the regression of distant metastases outside the irradiated field (16, 17). In fibrosis, immune cells, cytokines, and growth factors underlie the transition from cell death following radiation to abnormal repair in field (115, 116). In studying the abscopal effects, it was necessary to find mechanisms that could support radiation-mediated cell killing in the treated tumor and act at a distance, and a range of angiogenic and cytokine mediators were initially proposed (117–121). Thanks to pioneering studies, it is now appreciated that abscopal effects can be mediated by T-cells (14), which through recirculation, can move between distant sites, including distant tumors (69).
2.6. The oligometastatic status
In 1995, Hellman & Weichselbaum defined the oligometastatic status as an intermediate condition on a spectrum extending from localized disease to a rapidly advancing systemic disease. On the one hand, the limited spread of metastases to the lymph nodes may be perceived as an aggressive disease since the involved nodes harbor cancer cells and are, thereby, a source for seeding cancer (122). On the other hand, albeit an advanced disease, the oligometastatic status has not yet progressed into a systemic state, so it can be potentially cured.
To allow for clinical decision-making, the European Society for Radiotherapy and Oncology (ESTRO) and the European Organisation for Research and Treatment of Cancer (EORTC) proposed a consensus for classifying and characterizing the oligometastatic disease. It was based on the first cohort of the ESTRO and EORTC OligoCare registry project, focusing on differentiating between oligometastatic states and subclassifying the oligometastatic disease into oligorecurrence, oligoprogression, and oligopersistence (123). Although this system requires further evaluation, it underlines the importance of perceiving the oligometastatic disease as a heterogeneous state that needs to be addressed as such.
Currently, HNSCC patients with distant metastasis are classified as M1 and treated systemically. However, surgical removal of a metastasis (metastasectomy) or targeting it with stereotactic ablative radiotherapy (SABR) is employed in certain types of cancer. Metastasectomy was beneficial for lung metastases, prolonging life, and potentially curative in a selected group of patients (124).
By introducing the definition of oligometastatic disease into the diagnostic process, metastases originating from the lung, adrenal, liver, and spine may be targeted by SABR/SBRT (125). In a study that concentrated on delivering SABR to lung oligometastases originating from HNSCC, Bates et al. showed that although the 2-year disease-free survival was only 14%, the 2-year OS was more encouraging, reaching 43% (126). These data suggest that targeting oligometastases with SBRT should be considered to improve OS in HNSCC. Whether adding targeted therapy to prime the immune response to irradiation of oligometastases will improve OS and disease-free survival remains to be seen in future trials.
2.7. Combining radiation and immune checkpoint inhibitors in preclinical models
The combination of RT and immunotherapy is gaining interest as an avenue for cancer treatment, as recently reviewed (49, 127–129). Although limited initially, data on the combination of RT and immunotherapy in preclinical models is now accumulating. Using preclinical models allows for the testing of combinations regimens and mechanistic interventions that help understand how treatments impact tumors, both not practically feasible in large-scale clinical trials (50). Selecting the optimum immunotherapy to combine with radiation may depend on the immune status of the patient's tumor. For example, immunotherapies that target exhausted T-cells, such as checkpoint inhibitors, will likely be most successful where the patient has an extensive immune infiltrate limited by expression of these checkpoint molecules. By contrast, where a patient lacks extensive pre-existing immunity, the optimum immunotherapy may be better targeted to initiate anti-tumor immune responses in the tumor-draining lymph nodes, focusing on DC-related innate adjuvants (29–31), or costimulatory molecules such as ICOS and OX40 that are induced following antigen exposure (130, 131). A range of immune interventions in combination with radiation are discussed below.
Given the T-cell mechanism of action, it becomes logical to deliver therapies that act on T-cells to improve local and distant tumor control following radiation. Currently, almost all candidate T-cell targeted immunotherapies have shown synergy with radiation in some preclinical settings. The dominant players have been anti-CTLA-4 and anti-PD1.
Inhibition of CTLA-4 in combination with irradiating mouse primary mammary tumor led to an anti-tumoral immune response which inhibited the formation of lung metastases (132). To test dose fractionation that induces an abscopal effect, breast carcinoma tumors were implanted in-vivo in two separate sites and treated with different combinations of systemic CTLA-4 blockade and RT targeted to a single tumor site in a range of doses and fractions. By following the irradiated and unirradiated tumors, the experiments allowed an assessment of local control and abscopal effects, respectively. Both the fractionated and single-dose regimens caused a delay in the growth of the irradiated tumor, and the addition of anti-CTLA-4 further enhanced the effect. However, the non-irradiated tumor exhibited growth delay only in mice treated with the combination of fractionated radiation and CTLA-4 blockade (55). These data suggest that fractionated RT is a better partner for CTLA-4 blockade than single-dose RT to generate an abscopal effect. Combining RT and CTLA-4 blockade in a similar model also demonstrated a significant survival benefit (132).
Using the murine pancreatic ductal adenocarcinoma model, it was shown that the addition of anti-PD-L1 to high-dose RT improved tumor response and further prevented the development of liver metastases. This effect was evident following hypofractionated high doses of radiation but not after using low-dose radiation (133). Combining a single dose (10 Gy) of SBRT and PD-1 blockade gave rise to a significant long-term survival advantage in the orthotropic mouse glioma model; mice treated with PD-1 blockade or SBRT as monotherapy did not exhibit a significant advantage over the untreated group (134). A combination of PD-1 blockade and SBRT induced near-complete regression of irradiated mouse melanoma and partially reduced the size of the non-irradiated tumor. This effect was less prominent in the sole blockade of PD-1 or the RT-only groups (135).
The importance of timing and fractionation regimen was studied using the murine colon carcinoma and breast cancer models; blocking PD-1 or PD-L1 enhanced the efficacy of RT, while fractionated RT upregulated PD-L1 expression. Importantly, the highest efficacy was noted in the concurrent RT/PD-L1 blockade arm but diminished after delaying PD-L1 blockade for five days and became virtually non-beneficial following PD-L1 blockade initiated seven days after completion of RT (60). A single 10 Gy dose to an orthotopic model of HNSCC led to the upregulation of PD-L1 on tumor cells and increased T-cell infiltration, thereby improving local control and OS (136). Integration of single-dose RT with immunotherapy has also upregulated the expression of murine PD-L1 in the tumor microenvironment (TME), and blockade of PD-L1/PD-1 in combination with radiation showed a cumulative positive effect (137).
Notably, the combination of CTLA-4 blockade and PD-1/PD-L1 blockade provides distinct synergy with radiation such that the combination is more effective than any alone (138). Other T-cell targets that have shown preclinical efficacy include a range of TNFRSF members, including LIGHT (54), OX40 (131), 41BB (139), GITR (140), and ICOS (130), as well as alternative targets showing efficacy in combination such as Tim3, TIGIT, and Lag3 (141, 142).
These data establish the basis for the RT-immune checkpoint inhibitors combination and the importance of choosing a radiation dose, fractionation, timing, and sequencing with immune checkpoint inhibitors.
3. Radiotherapy and immunotherapy in clinical trials
3.1. From conventional radiotherapy to SBRT
Previously, SBRT in HNSCC was mainly used in patients unable to tolerate the prolonged course of RT due to comorbidities, advanced age, poor social and financial support, or inability to travel daily (143, 144). Thus, studies mainly concentrated on utilizing SBRT for second primary tumors, reirradiation (145), or in recurrent or metastatic settings (146–150), and rarely as an upfront modality for newly diagnosed patients (151).
SBRT was also applied to boost conventional RT, mainly in nasopharyngeal and oropharyngeal cancers (152–154). Lee et al. described high 1-year and 2-year locoregional recurrence-free rates of 91.4% and 86.3%, respectively (n = 26). However, there was also a high frequency of acute complications (27%) and severe late complications (34.6%), which was more frequent among individuals who received concurrent chemo-RT two weeks prior (153). These data demonstrate the importance of fractionation dose and timing to the development of treatment-related toxicities.
Earlier studies reported the use of SBRT in patients unfit to undergo standard-of-care. Amini et al. described three patients aged 72–88 treated with 5.0–7.2 Gy ×5 and witnessed either a clinical or radiographic complete response, with no grade 3 toxicities or greater, at 4–8 months of follow-up (155). Khan et al. described 24 sites in 21 patients aged 25–103 (median 87), most of them diagnosed with SCC, who were treated with 4–6 fractions of 7–8 Gy and exhibited 25% complete response and 67% partial response at eight months of follow up (6). It was uncertain, however, whether SBRT could be used in place of fractionated RT and lead to comparable outcomes.
To test the potential of SBRT for reirradiation of recurrent or second primary head and neck cancer (squamous cell carcinoma in most patients), Vargo et al. retrospectively compared SBRT (n = 197) to intensity-modulated radiation therapy IMRT (n = 217). The two groups had different characteristics at baseline, with patients in the SBRT group being older and more heavily treated, more likely to be treated for recurrence than a second primary, and having more lifetime doses of RT. Although the unadjusted 2-year overall survival (OS) and median survival were higher among the IMRT group, after controlling for baseline differences, there was no difference between the groups in OS (HR 0.877; 95% CI: 0.702–1.097; p = 0.251) or cumulative incidence of locoregional failure (HR 1.154; 95% CI: 0.886–1.505; p = 0.289). A subset analysis, however, revealed that the OS of the two groups was similar as long as the tumor volume was small and the SBRT dose was ≥35 Gy. Otherwise, IMRT led to better OS. Patients in the IMRT group had a higher rate of acute grade ≥4 toxicity than in the SBRT group (5.1% vs. 0.5%, p < 0.01) (156). Thus, SBRT may be considered for patients with small tumors and should be administered to a total dose of no less than 35 Gy. These benefits are highlighted when compared to previous clinical trials investigating salvage reirradiation. Under the same settings, salvage reirradiation with conventional RT resulted in a median OS of 8.5 months in RTOG 9610 (157) and 12.1 months in RTOG 9911 (158). These trials showed grade ≥3 acute toxicity in 63%–78% and late toxicity in 22%–37% (157, 158). In clinically negative neck (N0) cases, locally recurrent and previously irradiated head and neck cancer patients pose a significant therapeutic challenge (159). Due to the limited data available on the potential benefits of surgical treatment for the N0 neck, it is important to investigate whether neoadjuvant targeted therapy can provide advantages in managing this challenging group of patients.
Considering its lower toxicity rate and comparable outcome to IMRT under certain circumstances, studies focused on identifying the factors contributing to better outcomes following SBRT. Comparing previously-irradiated with never-irradiated patients was among the main factors examined. To evaluate the effect of previous irradiation on treatment outcomes following SBRT, a retrospective review of unresectable head and neck cancer in medically unfit patients was carried out (n = 114, squamous cell carcinoma n = 81, skin primary n = 41, non-skin primary n = 59). Patients received a total dose of 35–50 Gy SBRT divided into 4–6 fractions and stratified according to their baseline disease status. There was a statistically significant difference in median progression-free survival (PFS) between the groups: 23.7 months (untreated primaries), 14.8 months (recurrent unirradiated primaries), 10.5 months (metastatic non-head and neck cancer primaries), and 7.8 months (recurrent irradiated head and neck cancer primaries) (p = 0.04). Although the local control in the recurrent irradiated primaries at 12 months (78.9%) did not significantly differ from other groups, both the PFS (7.8 months) and the locoregional recurrence rate (38.4%) were the worst among the recurrent irradiated primaries compared to the other groups. Indeed, multivariate analysis showed that the only significant variable was previously irradiated lesions, which were more likely to have shorter PFS than previously unirradiated lesions (HR 4.09, p = 0.03) (160).
These data align with a previous publication by Kodani et al. (n = 34), who observed a superior OS rate in SBRT-treated patients who have not undergone a prior RT within the previous two years or in cases of reduced target volume. In the same group of patients, 17.6% experienced severe late complications, all having a history of prior RT (161).
In a more recent publication focusing on previously unirradiated head and neck cancer patients unfit to standard-of-care (n = 66, SCC n = 44), 7–8 Gy ×5 SBRT was delivered biweekly. Thirty-four patients also received adjuvant therapy. Median time to local failure was 28.3 months, and 1-year local control and OS rates were 73% and 64%, respectively. The toxicity rate was low, with 3% grade 3 and no grade 4 or above toxicities (8). Compared to smaller studies, this larger study demonstrated similar OS and local control and reiterated the role of SBRT in patients unfit to undergo surgery and standard-of-care chemoradiation.
3.2. Combination therapies of SBRT, other than immunotherapy
Given the benefit of combining RT with chemotherapy and the systemic therapy administered in the recurrent or metastatic setting, the next step was to study the effect of combining SBRT with other modalities. Recurrent head and neck cancer patients (total n = 137, squamous cell carcinoma n = 98) were treated in a single institution with 4–5 fractions of SBRT to a total mean dose of 45 Gy (range 36–47.5 Gy) on an every-other-day schedule (median follow up 19.3 months) (145). This regimen resulted in OS of 78% (1-year) and 62% (2-year), and local, regional, and distant control of 78%, 66%, and 83%, respectively. Importantly, among patients who had disease progression, OS was significantly improved in those who received salvage therapy (surgery, RT, or systemic), compared to patients who did not (median OS 44.3 months vs. 15.3 months, p = 0.03). Concurrent systemic therapy was associated with increased regional control (73% vs. 53%, 1-year, p = 0.004) (145). Taken together, these data demonstrate the advantage of SBRT over conventional RT in treating recurrent head and neck cancer, and the benefit of combining SBRT with concurrent systemic therapy in these patients.
The combination of SBRT with cetuximab was the focus of many studies, including clinical trials. In a phase II clinical trial conducted in inoperable locoregional confined recurrent HNSCC (n = 50, median follow-up 18 months), the combination of SBRT (8–8.8 Gy ×5) and cetuximab was tested. The median OS was ten months (95% CI: 7–16), the median PFS was seven months (95% CI: 5–12), and the 1-year OS was 40% (95% CI: 26%–54%). Although the primary efficacy in this study was not met, the PFS was similar to conventional fractionated RT combination with cetuximab but with lower toxicity rates (149). Similar results were obtained in a multi-institutional phase II clinical trial testing the combination of SBRT (6 Gy ×6) and cetuximab in recurrent HNSCC (n = 60, median follow-up 11.4 months). The median PFS was 7.1 months (95% CI: 5.5–8.9), and the 1-year OS was 47.5% (95% CI: 30.8–62.4) (150). Although SBRT showed no benefit in OS in these populations over fractionated RT, it required a shorter overall treatment time and led to lower toxicity rates.
3.3. SBRT to metastases
Conventional RT in metastatic HNSCC was previously seen as a palliative measure, with hypofractionated RT also utilized for symptom relief (162). However, SBRT may generate impressive local control of metastatic disease. In a multi-institutional retrospective registry analysis of SBRT for the management of HNSCC, lung, non-regional lymph nodes, and spine metastases, 1-year and 2-year OS rates were 66.4% (95% CI: 53.4%–76.4%) and 43.1% (95% CI: 30.3%–55.2%), respectively, and local control rate was 93.3% (95% CI: 75.4%–99.3%) at 1-year and 2-year, and 76.4% (95% CI: 44.7%–91.4%) at three years (163). Due to the retrospective nature of this registry analysis, much data was missing, including the extent of disease, HPV status, the intent of RT (palliative vs. local control), and concurrent systemic treatment. However, it showed that using SBRT may induce local control of metastasis. Although there was a high variability of RT regimens and fractionation doses (6–22 Gy per fraction over 1–5 doses), and no correlation was identified between local control and either prescription dose or fractionation schedule, local control was notably higher in smaller metastatic lesions and lack of spinal osseous metastatic disease (163).
3.4. Summary—SBRT without immunotherapy
This body of data supports using SBRT as an alternative to fractionated RT under certain circumstances. First, it remains advantageous for medically unfit patients who cannot withstand standard-of-care treatment. Second, it should be considered in cases where the gross tumor volume (GTV) is small. GTV < 15 cm3 was associated with better OS in (161); recurrent GTV < 25 cm3 was associated with improved 1-year locoregional PFS (53% vs. 22%, p = 0.029) and 1-year OS (70% vs. 22%, p < 0.001) in (149); and ≤50 ml was associated with better median OS and PFS (21.9 and 19.1 months for ≤50 ml, 12.6 and 12.1 months for 50–100 ml, 8.6 and 8.6 months for >100 ml, respectively) in (164). Thirdly, in re-irradiated patients, an interval greater than one year since the previous irradiation correlated with better survival (157) and favorable treatment response (164), and greater than two years in (161, 165). Fourth, analysis of a national cancer database on SBRT for HNSCC showed that combining SBRT with surgery or chemotherapy yielded better OS than administering SBRT as a monotherapy (166). Lastly, better OS was associated with a fractionation regimen of 7 Gy ×5 or greater (166).
Ongoing SBRT clinical trials are summarized in Table 1.
3.5. Combination of conventional radiation therapy and immunotherapy
The first phase III clinical trial to test the addition of a checkpoint inhibitor to chemoradiotherapy was the JAVELIN Head and Neck 100 trial. It was a placebo-controlled double-blind phase III study (n = 697) of locally advanced HNSCC patients treated with definitive fractionated RT and chemotherapy combination, and randomized to receive the anti-PD-L1 inhibitor avelumab (n = 350) or a placebo (n = 347). The trial was discontinued after the primary objective of prolonging PFS with avelumab was not reached (median PFS: 95% CI: 16.9 months-not estimable for avelumab, 23.0 months-not estimable for placebo), and an HR favoring the placebo group (HR 1.21; 95% CI: 0.93–1.75, p = 0.92) (47).
Given it was the first phase III trial to test the addition of immunotherapy to chemoradiotherapy in the upfront setting for locally advanced HNSCC, comparing it to other studies is challenging. However, based on other trials showing more favorable results following the addition of immunotherapy to chemotherapy without RT (46, 167), it is worth considering a possible negative effect of concurrent fractionated RT on the immune response. Moreover, the irradiated field in this trial included the neck draining lymph nodes, which might have impeded the priming of T-cells and hindered the effect of immune checkpoint inhibitors (91, 82, 96, 168).
Another trial focused on RT-immunotherapy combination is the phase II multicenter GORTEC 2015-01 PembroRad trial, which enrolled patients with locally-advanced HNSCC. This trial, however, recruited patients unfit to high-dose cisplatin, so it was not administered. Patients were randomized into pembrolizumab-RT (n = 67) vs. standard-of-care cetuximab-RT (n = 66) combinations, with a median follow-up of 25 months. Most patients had the oropharynx as the primary site (62% and 59%, cetuximab-RT and pembrolizumab-RT, respectively), and the minority had the oral cavity as the primary site (8% and 6%). Fractionated RT was administered in 33 daily fractions to a total dose of 69.96 Gy or 52.8 Gy. Three concurrent doses of pembrolizumab were administered at 3-week intervals. Both regimens achieved similar 15-month locoregional control (60% vs. 59%, pembrolizumab-RT vs. cetuximab-RT, respectively), and there was no significant difference in PFS (HR 0.85, 95% CI: 0.55–1.32; p = 0.47) or OS (HR 0.83. 95% CI: 0.49–1.40; p = 0.49). Although there was no statistically significant difference in PFS or OS, both trended in favor of the pembrolizumab-RT combination. Notably, the pembrolizumab-RT combination led to a statistically significant lower toxicity rate, with 74% vs. 92% of patients with adverse events ≥ grade 3 (p = 0.006) (48). These data suggest that pembrolizumab-RT may be a less toxic alternative to a high-dose cisplatin-RT combination, while still achieving similar OS and PFS. However, the neck was included in the irradiated field, and considering the data pointing to the possible role of an intact neck when commencing immunotherapy, a study designed to deliver immunotherapy-RT combination while limiting neck RT is the natural next step. The phase II REWRITe clinical trial in HNSCC (NCT03726775) will evaluate the combination of durvalumab and RT, restricted to the primary tumor and the adjacent neck levels.
These trials focused on administering immunotherapy and fractionated RT at the definitive setting. Given the potential of SBRT to induce an immune response, clinical trials are testing the combination of SBRT and immunotherapy in the metastatic setting.
3.6. SBRT-immunotherapy combination in the metastatic setting
An ample amount of data in solid tumors other than HNSCC emerges from combining SBRT and CTLA-4 blockade. Prescribing ipilimumab (anti-CTLA-4) and a single fraction 8 Gy RT in metastatic castration-resistant prostate cancer had not produced significant superiority over single-arm treatment (169). However, more encouraging results came from a study of solid metastatic tumors refractory to standard therapy. Five cohorts of patients were administered concurrent or sequential ipilimumab with 12.5 Gy ×4 or 6 Gy ×10 RT. Results indicated a possible correlation between an early increase in peripheral CD8+ T-cells, expression of 4–1BB and PD-1 on CD8+ T-cells, and a possible clinical benefit (170).
Concurrent delivery of ipilimumab and radiosurgery (median 21 Gy in 2 fractions) to melanoma brain metastases produced favorable regional control and amount of time to brain metastases progression, as opposed to the RT alone group (171, 172). However, in a different study, no superiority of immunotherapy in combination with RT was apparent over RT alone (173).
On the one hand, these data demonstrate a limited benefit at best; on the other hand, there is still no considerable amount of published data regarding this combination, and the already published data represents primarily studies conducted in advanced cancer populations.
In a single-center phase II trial (n = 62, median follow-up 20.2 months), a possible synergy between SBRT (9 Gy ×3) and anti-PD-1 immunotherapy was assessed in the metastatic HNSCC setting. Patients were randomized into nivolumab alone (n = 30) or nivolumab-SBRT combination (n = 32). At 12 months, there was no statistically significant difference in PFS (32.2% nivolumab; 95% CI, 19%–54.9%; 16.8% nivolumab-SBRT; 95% CI: 7.2%–39.3%), nor in median OS (14.2 nivolumab, 13.9 nivolumab-SBRT), overall response rate (34.5% nivolumab, 29.0% nivolumab-SBRT) or grade 3–5 toxicities rates (13.3% nivolumab, 9.7% nivolumab-SBRT; p = 0.7) (174). This trial showed no benefit to SBRT when added to nivolumab in the metastatic setting. To test whether concurrent targeting of the PD-1 and CTLA-4 pathways in addition to SBRT will lead to a benefit, the phase I/II clinical trial (NCT03283605) will administer both CTLA-4 (tremelimumab) and PD-1 (durvalumab) inhibitors concurrently with SBRT to metastatic head and neck carcinoma (n = 35, 2–10 extracranial metastases) (175). Another possibility is that this combination of SBRT and immunotherapy is insufficient to counteract the immune escape inherent to the metastatic state.
3.7. Neoadjuvant immunotherapy
The combination trials described thus far focused on administering immunotherapy and SBRT at the definitive setting, either as a concurrent treatment to standard-of-care or replacing chemotherapy. However, a growing body of data points to a potential benefit of administering immunotherapy in the neoadjuvant setting.
In a phase II randomized clinical trial (n = 29), neoadjuvant immunotherapy prior to surgical resection of oral cavity SCC resulted in a major to complete pathologic response in 8% (n = 1) of patients treated with neoadjuvant nivolumab, and in 20% (n = 3) of patients treated with neoadjuvant nivolumab + ipilimumab. Pathologic response greater than 50% was observed in 15% of patients receiving neoadjuvant nivolumab and 33% receiving nivolumab + ipilimumab. Pretreatment CD4+ T-cells were associated with pathologic response in the nivoloumab + ipilimumab combination but not in nivolumab alone (176). In a similar HNSCC non-randomized phase Ib/IIa clinical trial (n = 32), major pathologic response (MPR) was observed in 17% (n = 1) following nivolumab monotherapy and 35% (n = 8) following nivolumab + ipilimumab combination therapy. There was a trend of higher baseline intratumoral CD8+ T-cells density among major-pathological responders, albeit not statistically significant (p = 0.31). Interestingly, in both major-pathological responders and non-responders, there was an increase in intratumoral CD8+ T-cells density after neoadjuvant immunotherapy (177). Neoadjuvant pembrolizumab (n = 36, phase II trial, HPV-unrelated HNSCC), however, did not result in a complete response, and a major pathologic response was only evident in two patients (178).
Some studies showed a positive correlation between high-expressing PD-L1 populations and clinical outcomes following treatment with PD-1 inhibitors (42, 46, 47, 167, 179). However, while T-cells expressing PD-1 may decrease in responders post-treatment, baseline cell-specific expression of PD-L1 or combined positive score (CPS) do not necessarily differ between responders and non-responders to SBRT and anti-PD1 combination (39), or fractionated RT and anti-PD-1 combination (48). Since PD-1 is expressed by both PD-1+CD8+ T-cells and PD-1+ T-regulatory (Treg) cells, PD-1 blockade reactivates the effector effect of CD8+ T-cells and immunosuppressive Treg cells. Thus, it may be the ratio between the two, rather than the absolute levels of PD-1+CD8, which predicts response to PD-1 immune checkpoint inhibition (180).
While these data show a somewhat limited potential for certain immunotherapy regimens to induce a major pathologic response in HNSCC, the response was achieved shortly after administering it as monotherapy. Given the baseline differences in T-cells populations between patients and after treatment, it is possible that other regimens or combinations of immunotherapy will augment the immune response.
3.8. Neoadjuvant immunotherapy-SBRT combination
Although insufficient to signify a reversal of cancerous processes, the data reviewed so far points to possible positive trends in tilting the immune-cancer balance: Potential induction of immune response by SBRT, a possible benefit in combination therapies, a potential benefit, albeit slight, in starting immunotherapy in the neoadjuvant setting, and the supporting preclinical data. These logically lead to design studies that combine SBRT and immunotherapy in the neoadjuvant setting.
The combination of SBRT with durvalumab (anti-PD1) was assessed in the neoadjuvant setting of HPV-negative HNSCC in a phase I/Ib clinical trial (n = 21). The most common features were the oral cavity as the primary subsite (n = 18, 85.7%), T3 or T4 disease (n = 19, 90.5%), and node-positive disease (n = 14, 67%). The patients received one neoadjuvant dose of durvalumab, and the study was designed with an escalating radiation dose, starting 6 Gy ×2 to a maximum of 8 Gy ×3. For the 6 Gy ×3 or 8 Gy ×3 groups (n = 18), OS at 16 months was 80.1% (CI 95%: 62.0%–100%), and PFS and locoregional control were both 75% (CI 95%: 57%–99.8%). There was a positive association between 8 Gy ×3 dose and a better response (p = 0.07). In contrast, none of the recurred patients had a major pathologic or complete response (39).
Importantly, tissue samples obtained pretreatment (baseline) and at surgery (after neoadjuvant SBRT and durvalumab), demonstrated an increase in the CD103+CD39+CD8+ T-cells at baseline and at surgery among responders, similar to (80) and (181). Responders also had increased IFN-gamma within cytokine-producing T-cells and an increase in activated T-cells (PD1, CD69, Ki-67, and DNAM-1); responders had an increase in CD45RO+ memory T-cells, while non-responders had a less consistent pattern (39).
Gene expression analysis among responders revealed increased expression patterns associated with immune activation. In contrast, neither PD-L1 expression nor CPS scores correlated with response to durvalumab and SBRT (39). Although Treg cells in the TME were linked to decreased response to immunotherapy (182), a decrease in Treg cells was not a differentiator between responders and non-responders (39). Instead, the ratio between the amount of CD8+ T-cells to Tregs was correlated with response-to-treatment, with a decrease in total T-cells in non-responders, leading to a significant difference in the CD8+ T-cells to Treg ratio (39). The CD8+ T-cells to Treg ratio increased in patients receiving 8 Gy ×3 compared to lower doses of SBRT. Only by administering neoadjuvant 8 Gy ×3 were consistent MPR and CR observed in HPV-negative HNSCC concurrently treated with neoadjuvant durvalumab (39). This study is pivotal to the field as it systematically uncovers processes and trends underlying the resulting outcome. In addition to the optimal 8 Gy ×3 dose, it points to the CD8+ T-cells to Treg-cells ratio, baseline CD103+CD39+CD8+ T-cells, and T-cell activation markers as differentiators, and perhaps possible predictors of response.
In another phase Ib clinical trial, previously untreated locally-advanced HNSCC patients (n = 21) were treated with neoadjuvant SBRT (to GTV only) over one week. The doses studied were 8 Gy ×3 (24 Gy total dose) or 8 Gy ×5 (40 Gy total dose), with or without neoadjuvant nivolumab. Three cohorts (n = 16) were HPV positive, while the fourth was HPV negative (n = 5). All patients underwent standard-of-care surgery five weeks after SBRT, followed by adjuvant nivolumab for three months. The overall MPR was 86%, the CR was 67%, and 90% of patients were downstaged. The extent of resection was reduced in most patients, and no treatment-related surgical delays occurred. 20 of the 21 patients did not require adjuvant radiotherapy postoperatively. Delayed treatment-related adverse events were more common in the 40 Gy cohort. Of note, although major pathologic response was achieved in 86% of patients, partial radiologic response prior to surgery was evident in 10 patients, while 10 patients had stable radiographic disease. There was no correlation between the pathologic and radiographic response (64).
3.9. Summary—SBRT with immunotherapy
Currently, data on the combination of SBRT and immunotherapy in HNSCC is limited. So far, combining SBRT and immunotherapy in the metastatic setting has resulted in limited pathologic response. However, the published clinical trials indicate a more favorable outcome following neoadjuvant SBRT and immunotherapy. Of these two trials, the total dose of 24 Gy seems optimal, as a lower dosage led to a less favorable outcome (39), and a higher dose led to a similar outcome but a higher toxicity rate (64). The total RT dose, fractionation regimen, and the timeframe after completion of SBRT and before surgery may play a significant role in improving the pathologic outcome. Sparing the neck from the irradiation field may also play a significant role in allowing an immune response to develop after irradiating the primary site and administering immunotherapy.
Ongoing clinical trials of SBRT-immunotherapy combinations are summarized in Table 2.
4. New players in immunotherapy and radiotherapy
4.1. Cytokines
Checkpoint inhibitors such as anti-CTLA-4 and anti-PD-1 are clearly the major players in cancer immunotherapy, but they are the second phase of FDA-approved immunotherapies for cancer. The first phase was recombinant cytokine therapies; some are still in use. The anti-tumor activity of recombinant IL-2 led to FDA approval for patients with metastatic kidney cancer in 1992 and metastatic melanoma in 1998, and high-dose recombinant IL-2 is still in clinical practice (183). The toxicity of high-dose IL-2 and relatively low response rates limit its clinical use to specialized centers and some community hospital programs (183). However, it can generate clinically meaningful and durable responses and remains part of published treatment guidelines for both melanoma and renal cancer (184, 185). An initial phase I study demonstrated that SBRT combined with high-dose IL-2 resulted in an objective response of 71% in previously untreated patients with metastatic melanoma and 60% in renal cell carcinoma (66). There was no increase in the toxicities associated with high-dose IL-2 and no dose-limiting toxicities associated with radiation. A subsequent phase II randomized study showed an improved disease control rate in patients receiving SBRT combined with high-dose IL-2 compared to high-dose IL-2 alone (65). While the combination therapy was similar to the phase I results, an unexpectedly strong response in the IL-2 alone group limited the ability to detect an improvement with SBRT. This may have been influenced by dramatic improvements in treatment options for these patients in the past decade, including BRAF-targeted therapies and prior anti-PD-1 and anti-CTLA-4 treatments. Retrospective analyses suggest a higher-than-anticipated response to anti-PD-1 following IL-2 (186). Preclinical studies suggest novel IL-2 formulations can enhance immune activity with limited toxicity, and that these agents synergize with radiation therapy in preclinical models (187). The response rate could be increased through combination with innate adjuvants and correlated with antigen-presenting cells maturation (188), suggesting that improving antigen cross-presentation in combination with antigen release and T-cell support could provide multifaceted support of anti-tumor immunity.
Similarly, various cytokines within the Type I interferon (IFN) family have been studied as adjuvant therapy for cancer in the past 30 years. Treatment of patients with type I IFN resulted in improved recurrence-free survival (189, 190) but not OS (191). Preclinical studies in pancreatic cancer have shown improved outcomes with type I IFN and chemotherapy in pancreatic cancer (192, 193). However, clinical studies suggested that while patients receiving type I IFN and adjuvant chemoradiation showed improved outcomes (194), the use was limited by high-grade toxicity in 85%–90% of patients (195, 196). To control for systemic toxicity while sustaining tumor effects, type I IFN can be injected into the local tumor environment to generate tumor control (197) and can be engineered to accumulate in the vicinity of cancer cells using immune conjugates (198, 199). However, a range of alternative therapies has been developed to induce type I IFN in the tumor environment through local administration, improving the in-vivo efficacy and toxicity profile [reviewed in (20)]. Examples of these will be discussed below.
4.2. Innate adjuvants
The use of innate adjuvants to support the immune response to RT has been widely reviewed (20, 21, 113, 114). This is based in part on a wide range of studies using exogenous adjuvants injected into tumors to improve radiation-mediated control of tumors. For example, an important series of studies demonstrated that single fraction and fractionated RT regimens resulted in improved local control when combined with CpG in a mouse fibrosarcoma model (200, 201). These studies demonstrated that the 50% tumor cure dose for fractionated radiotherapy is reduced from 83.1 to 23 Gy when combined with CpG. As discussed above, more recent studies have identified that endogenous innate adjuvants that stimulate the STING pathway are generated by RT and are a critical component of the immune effects of radiation (56, 57, 202). Exogenous administration of STING ligands also synergizes with radiation to control tumors in preclinical models (203), and an array of methods to deliver STING ligands and similar innate therapies have been developed (204–206). However, these discoveries have proven difficult to translate, given the limited efficacy of STING ligands in clinical trials (207, 208). Considering the high potency of STING ligands in preclinical models and the lack of potency in patients, these data suggest either a problem in how these agents are translated to clinical use, or a fundamental limitation in the murine preclinical models used to develop these agents (50). Extensive further study is ongoing in this area.
4.3. Myeloid-targeted agents
As discussed above, while exogenous adjuvants can synergize with RT to control tumors, cancer cells killed by RT can provide endogenous adjuvants such as STING ligands (56), heat shock proteins (209–211), HMGB1 (212, 213), and calreticulin (214). However, macrophages in tumors are generally polarized such that they respond to TLR ligands with an M2 pattern of response by secreting cytokines such as VEGF, IL-10, and TGFβ (215–217). In addition, exposure of unpolarized macrophages to irradiated cancer cells can drive macrophages to become M2 polarized (97, 215, 218, 219), regardless of any adjuvants released. Since myeloid cells can be an obstacle to RT, there is a range of strategies focused on eliminating these cells or preventing M2 polarization [reviewed in (99)]. One such approach has been to target CSF1R, which drives macrophage differentiation and supports macrophages in peripheral tissues. CSF1 or CSF1R inhibition with blocking antibodies or small molecules has synergized with both chemotherapy and RT to control tumors (220, 221). Despite improved responses, this approach has a marginal effect and has not been shown to result in tumor cures.
As an alternative to macrophage depletion, targeting the pathways that drive M2 differentiation following macrophage interaction with dying cells has provided stronger impacts. Blocking phosphatidylserine (PS) (222), milk fat globulin E8 (MFGE8) (223), and Mertk (224, 225), have all altered macrophage differentiation following exposure to dying cells, and resulted in improved control of tumors. Mertk is a particularly relevant target since it is the signaling component downstream of PS ligation by Gas6 (226) and MFGE8 ligation by integrins (227), as well as complement-mediated opsonization of dying cells via C1q (228). Importantly, Mertk blockade combined with radiation can also be improved in resistant tumors by additional therapies such as TGFb inhibition or checkpoint regulators, which can permit control of distant tumors (99, 224, 225, 229), suggesting it is a good target to overcome macrophage suppression following radiation (99).
Recent studies have highlighted CD47 as a novel phagocytosis-related target in cancer therapy. CD47 binds SIRPa, where SIRPa is predominantly expressed on macrophages and some myeloid subpopulations, while CD47 is expressed on most cells, and particularly on hematopoietic cells and red blood cells (230). CD47 expression prevents phagocytosis of red blood cells (231). CD47 expression varies on immune cells through their activation, and high-level expression of CD47 on acute myeloid leukemia (AML) cells was associated with a worse prognosis (232). Antibodies blocking human CD47 on AML cells transplanted into immunodeficient mice resulted in limited engraftment of the human cells due to increased phagocytosis by host cells (232). In patients, where anti-CD47 can bind normal cells as well as cancer cells, most patients exhibit hematological toxicities with 100% receptor occupancy on red blood cells observed at doses above 1 mg/kg (233). Novel CD47 antibodies are in development that can potentially limit toxicity, but an alternative is to target the SIRPa molecule on myeloid cells. In immunocompetent preclinical models, the addition of anti-SIRPa to radiation resulted in improved control of tumors compared to either agent alone, and compared to anti-CD47 combined with RT (234). Further improvements in local and distant responses could be made by adding anti-PD1 (234), indicating again that myeloid targeting works well in combination with T-cell targeted therapies to improve radiation outcomes.
To provide a degree of certainty in selecting CD8+ T-cells to become long-lived memory cells, the immune system uses multi-factor authentication that depends on the presence of innate adjuvants as well as distinct CD4+ and CD8+ T-cell antigenic epitopes. These signals are integrated via dendritic cells, which traffic to lymph nodes in the presence of adjuvant and where CD4+ T-cells license dendritic cells via CD40-CD40l interactions to optimally activate CD8+ T-cells to cross-presented antigen (102–104). In the absence of CD4+ T-cells, effector CD8+ T-cell responses to infectious agents can still be generated, but memory responses are generally decreased (235, 236). CD40-CD40l signals are necessary to develop the T-cell immune environment of tumors, and this is, in turn, necessary for tumor control by radiation and immune checkpoint inhibitors (79). Therapeutically, CD8+ T-cell memory can be generated in the absence of CD4+ help by providing polyIC and anti-CD40 antibodies (237), thus, exogenously providing the DC adjuvant and the critical aspect of CD4+ help (102). Anti-CD40 treatment has been shown to improve responses to RT in a range of preclinical models (238–240). Anti-CD40 treatment has resulted in some on-target toxicity in patients (241), so novel approaches are in development to target this agonist. Locally administered anti-CD40, designed to slowly release into the tumor-draining lymph node, has shown an equivalent single agent response as systemic delivery and decreased toxicity (242), suggesting that targeted CD40 therapies have the potential to improve the use of this agent. A distinct sustained release system, providing both anti-CD40 and anti-PD1, has shown synergy with radiation in preclinical models (243), and antibody alone directly injected into tumors has shown synergy with radiation in preclinical models (244). Alternatively, a dual fibroblast and CD40 targeting antibody has been developed, which shows synergy with RT in preclinical models (245). These data are interesting since they suggest that DC help is relevant in the tumor environment rather than following trafficking to the lymph node, as has been shown for endogenous T-cell responses following radiation (30, 31). Importantly, as with other myeloid-targeted therapies, anti-CD40 therapy has been shown to be a strong partner for T-cell targeted immunotherapies (246), suggesting that these treatments can be layered to optimally treat tumors that have limited pre-existing immunity.
4.4. Metabolic targets in the tumor environment
The unique environment of a growing tumor can engender a range of unusual metabolic conditions that are a target for therapy. In general, the active proliferation of cancer cells can lead to the depletion of metabolites along with hypoxic conditions of high growth outstripping vascular supply (247). Many of these metabolic conditions are immunoregulatory, and critical pathways can impair immune responses in the tumor environment. For example, prostaglandin E2 (PGE2) is a long-defined feature of the tumor environment that suppresses the immunostimulatory activity of DAMPs on DC and macrophages (248–250). Radiation increases PGE2 production by irradiated tumor cells and tumor stroma cells, and this impacts cancer cell repopulation and results in poor therapeutic outcomes (251).
Even positive features of cancer treatment can have negative metabolic consequences that impact outcomes. For example, signaling through type I IFN signaling following treatment with radiation and exogenous adjuvants increases the expression of indoleamine 2,3-dioxygenase 1 (IDO1) (252), which can negatively regulate immune activation. IDO1 expression in tumors can promote tumor growth (253, 254), and patients with higher IDO1 expression have been shown to have worse outcomes (255). IDO1 impacts a range of immune cells in the tumor immune environment, including CD8+ T-cells and myeloid cells [reviewed in (254)]. Similarly, arginase induction in myeloid cells following RT of tumors can result in metabolic suppression of T-cells and limited T-cell control of irradiated tumors (256), and macrophages infiltrating tumors following immunotherapy can suppress T-cell control of tumors via arginase expression (100). These data suggest that targeting this specific metabolic feature can enhance the radiation control of tumors.
Recently, several studies have pointed to purinergic signaling as an important target in cancer [reviewed in (257)] and following RT. Extracellular ATP concentrations are regulated by the ectonucleotidases CD39 and CD73, which are themselves regulated on immune cells in tumors (80, 258, 259). ATP is hydrolyzed to ADP and AMP by CD39, and AMP is further hydrolyzed to adenosine by CD73. Adenosine can, in turn, generate anti-inflammatory and immunosuppressive effects in the tumor environment, including promoting a tolerogenic phenotype in DC (260), and directly inducing T-cell anergy and Treg differentiation (260–262). While CD39 is enriched on tumor-specific T-cells in tumors (80, 263) as well as Treg cells, increased expression of CD73 is associated with a poor prognosis in a range of tumors (264–266). Importantly, hypoxia and inflammation in the tumor can upregulate the expression of CD39 and CD73, resulting in radio-resistance (267, 268). Thus, blocking CD73 can improve the response to radiation in preclinical models (269). Similarly, a range of studies have demonstrated that targeting adenosine metabolism and purinergic signaling has improved immune control of tumors (261, 270), the response to radiation in preclinical models (271, 272), and a range of related approaches are in clinical development (273). Again, given that these factors limit T-cell control of tumors, these can be layered with T-cell-targeted immunotherapy to improve tumor control.
5. The effect of heterogeneity on response to radiotherapy
5.1. Signaling mechanisms and intratumor heterogeneity
Recently, advances in molecular biology and a better understanding of the molecular mechanisms underlying HNSCC have resulted in the development of targeted therapy to boost radio-sensitization. A few agents are being studied, including anti-epidermal growth factor receptor (EGFR). EGFR is overexpressed in over 90% of head and neck tumors and is linked to poor prognosis and increased tumor growth and metastasis. Moreover, EGFR was found as one of the critical components of resistance to RT (274, 275) through activation of downstream pro-survival mechanisms, such as pAkt/ MAPK or DNA repair pathways, when it internalizes to nuclei and activates DNA-PK (274) (Figure 2, right panel).
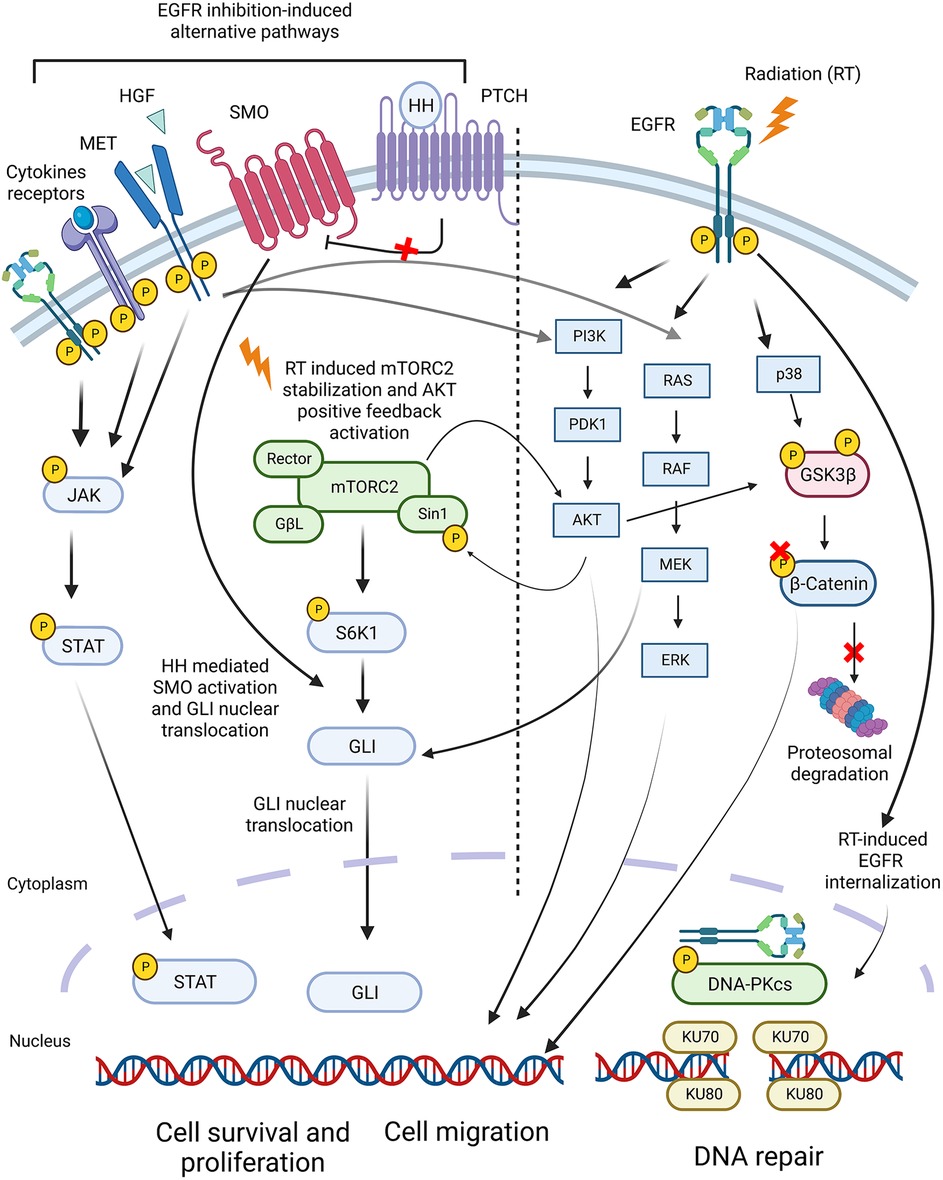
Figure 2. Signaling pathways in HNSCC involved in the development of resistance to radiotherapy. The Epidermal Growth Factor Receptor (EGFR) was found as one of the central components of resistance to RT due to activation of pro-survival mechanisms, such as pAkt/MAPK or DNA repair pathways. However, combined inhibition of EGFR with RT did not improve HNSCC response to RT. This is due to alternative pathways activated in response to EGFR inhibition, such as HGF/MET, JAK-STAT, and Hedgehog pathways. Inhibition of these pathways, along with EGFR, improved the response of HNSCC to RT in preclinical studies.
However, the combined therapy of anti-EGFR with RT did not significantly improve treatment response due to alternative pathways activated in response to EGFR inhibition, such as HGF/MET, JAK-STAT, and Hedgehog pathways. Indeed, inhibition of these pathways along with EGFR improved HNSCC response to RT in preclinical studies (274, 276, 277) (Figure 2, left panel).
Recent data suggest that the activation of alternative pathways is often patient-specific (278). For example, certain HNSCC malignancies can activate cMet pathways along with EGFR+ processes, whereas others may harbor EGFR+ and Src+ distinct subnetworks. Hence, in this example, two different drug combinations should be selected to treat these HNSCC malignancies. Analyzing proteomic and phospho-proteomic alterations, 61 distinct tumor subtypes were found in a cohort of 203 HNSCC patients (278), suggesting a high level of intertumor heterogeneity, and thus an urgent need for personalized therapies.
Complex, often spatial-dependent (279–281) interactions between cancer cells, the immune system, microbiome, and additional individualized elements in the TME, contribute to the intratumor cellular diversity, thereby complicating the intra- and intertumor heterogeneity of HNSCC. Intratumor processes and communication with the TME generate constant selective pressure, which promotes continuous diversification of malignant and nonmalignant compartments of TME, thereby increasing a degree of intratumoral heterogeneity, aggressive disease progression, and resistance to treatments (282). An example of spatial heterogeneity in HNSCC was provided by Forum et al., who demonstrated that the invasive leading edge of primary tumors was occupied by cancer cells expressing epithelial-mesenchymal transition (EMT) signature genes, while cancer cells located within the core of the tumor did not show EMT transcription factors (283). In addition to being a factor involved in intratumor heterogeneity, EMT is also associated with, and is widely considered, a potential cause of drug resistance, invasion, and metastasis (284).
Non-homogeneous distribution of immune cells within solid tumors is another example of non-homogeneous intratumor evolution, which may contribute to immunotherapy-RT resistance. For example, it was shown that hypoxia might drive the localization of tumor-associated macrophages. M1 macrophages, a subtype displaying an anti-tumor phenotype, were found mainly in normoxic areas approximate to blood vessels, while M2 macrophages, the protumor subtype, were more dominant in hypoxic areas in lung cancer (285). The intratumor diversity of TME, including hypoxia and cancer-associated fibroblasts (CAF), was found to have an essential role in developing the M2 macrophage subpopulation in head and neck cancers (286) and development of the HNSCC resistance to RT and immunotherapy.
Thus, an in-depth understanding of intratumoral heterogeneity, along with the changes occurring in response to RT/immunotherapy, can be crucial to the design of individualized therapy for head and neck cancer patients.
Studies that capture and target intratumor evolution are underway in other cancer fields. For example, Alkhatib et al. have shown that RT resistance may occur due to the evolution of the intratumor cellular populations in response to RT, towards a more radio-resistant molecular composition. The study characterized evolving processes in triple-negative breast tumors in response to RT and found two different HER2 and cMET-positive cellular subpopulations, which have been expanded in the resistant tumors. Simultaneous inhibition of HER2 and cMET receptors sensitized the tumor responses to RT (287). This research suggests that a similar approach, providing an accurate molecular characterization of tumors undergoing RT, may be beneficial for HNSCC as well.
5.2. Computational tools to resolve heterogeneous cancer responses
Growing evidence for the extensive intertumor and intratumor heterogeneities in HNSCC, and their potential influence on different types of treatments, led to the development of quantitative approaches addressing the challenge of accurately classifying cancer patients (or cells within a tumor) into distinct subgroups.
For example, in a phase I trial of neoadjuvant SBRT and anti-PD1 prior to surgery, responders exhibited higher levels of proinflammatory cytokines IFN gamma and TNF alpha, and an increase in circulating memory T-cells, while non-responders had more prominent TGF beta, IL-17A, and DNAM-1 expressing myeloid populations (39). While each attribute may constitute a potential biomarker for patient response, accumulating knowledge in precision oncology suggests that quantitative strategies should classify cancer patients based on specific features of their altered protein-protein networks rather than on overexpression or mutation of a specific biomarker. Once differences and similarities in these networks are identified and found to differentiate between individuals, protein hubs from each subgroup-specific network can be transformed into practical clinical solutions. Namely, an accurate stratification will significantly increase response to existing therapies or lead to the development of new therapeutic strategies.
Machine deep-learning algorithms play an essential role in these efforts. These techniques attempt to derive a general rule from the input proteomics (or genomics) data, and then to “allocate” each patient into a specific subgroup according to this rule. This classification can generate a prediction of drug sensitivity for a given patient.
Examples of machine-learning methods include clustering algorithms, such as the K-nearest neighbor algorithm, K-Means algorithm, and support vector machine (SVM). These calculate from the experimental (such as protein expression data) how similar the samples are in terms of protein expression alterations, and then calculate the distance between every two samples. These machine-learning algorithms, and others that will be described next, were frequently used for early diagnosis and prevention of head and neck cancer (288). Recently, they have been applied to differentiate responders from non-responders to anticancer drugs in oral (289) and additional cancer types (290), as well as predictors for cancer subtypes (291) and survival of cancer patients (289, 292).
Additional examples include Bayesian Networks classifiers, which use conditional probabilities to calculate the probability of sample B to belong to a particular cluster, given a set of samples features, e.g., a particular protein expression signature (293), or Decision Tree algorithms, which sequentially use data variables to compute the sequence of branch choices. These types of machine-learning algorithms were used to predict clinical outcomes (289, 294) through the identification of essential gene lists or clinical features associated with survival (Bayesian Networks classifiers) (295, 296), or to predict aggressive behaviors of tumors via DNA methylation differences (Decision Tree algorithms) (289, 297).
Neural network-based models are an example of the most popular deep learning algorithms employed today to study cancer systems. Similar to the connections of neurons and synapses found in the brain, the algorithm processes several “layers” of data, each one using increasingly complex analysis than the previous one. In oral cancer, this approach was applied, for example, to transcriptomic data to identify different immune subtypes (298). Additional discussion on machine-learning and deep-learning approaches can be found in (293, 299).
5.3. Physics-based models
While predictions based on probabilities identify abundant patterns in a patient population, and then assume that a patient possessing this pattern should respond to a drug in a certain way, physical approaches base their predictions on the stabilities of systems. They are derived, for example, from free energy quantifications for each state of the system (300–304). According to the basic physicochemical laws, spontaneous transitions from a higher to lower free energy state occurs in a system, and thus would define a direction of spontaneous change in the course of any process, including pathological process. Therefore, identifying stable and unstable states in tissues, including cancer tissues, should allow us to predict different phenotypes and recommend how those phenotypes can be manipulated.
This notion inspired us and others to implement thermodynamic-based approaches in cancer (302, 305, 306) and extend it further to the field of personalized medicine (301, 307). A series of works demonstrated that the thermodynamic-based strategies could provide detailed information not only on the central oncomarkers (oncological biomarkers) or common patterns characterizing a particular subgroup of patients, but also on the role of each oncomarker in the patient-specific ongoing processes (301, 307, 308). Once a patient-specific set of unbalanced processes is resolved, then a prediction on how a tumor-specific imbalance should be targeted is readily provided (287, 307).
Using unbalanced processes resolved in each HNSCC malignancy, we have recently demonstrated how they can be used to design patient-specific drug combinations. Jubran et al. have shown that combining the anti-EGFR inhibitor with additional, patient-specific targeted drugs, results in higher efficacy than the anti-EGFR monotherapy. Using in-vivo studies, Jubran et al. demonstrated that the resistance to anti-EGFR therapies could be inhibited when the unbalanced processes, occurring in addition to the EGFR+ processes, are resolved and targeted simultaneously in a patient-specific manner (278). Moreover, the study provided evidence that patient-specific drug therapies can also increase the potential of T-cell activation. These results suggest that resolving the individualized unbalanced processes, basal or induced to a specific type of therapy, provides an essential step towards accurately designing patient-specific drug combinations (278, 287, 307, 309).
In summary, quantitative, statistical, or physicochemical approaches provide great promise for accurate patient stratification to individualize diagnostics and treatments. Future methods will likely focus on integrating omics, clinical data, and image data, using multimodal learning (310, 311) to reveal novel molecular aberrations that differentiate certain groups of tumors from others. One of the central clinical challenges would be to find and implement a strategy that addresses each tumor individually, and provides a complete molecular characterization to any new patient. In particular, to those who do not necessarily possess the features learned from the previous patient populations.
6. Conclusions
The introduction of immunotherapy, targeted therapy, and hypofractionated RT into the field of head and neck cancer has opened new possibilities for treatment. Recent developments in diagnostic and computational methods allow better characterization of the patients and tumors and assess treatment outcomes. However, adapting the appropriate regimens to patients, and predicting their outcomes have not matured into practical tools.
RT, once regarded only as a means to kill cancer cells directly, is transforming into a multilayered tool to impact the immune response. Preclinical studies and clinical trials have uncovered the impact of dose adjustment, hypofractionation, and lymph node sparing to allow and even augment systemic immunity against cancer cells. A better understanding of immune cells trafficking, response-to-treatment, and implementation of in-silico methods, will facilitate treatment design and prediction of response.
FDA-approved immunotherapy and targeted therapy in head and neck cancer are still limited to a narrow set of agents, with most published clinical trials resulting in less-than-optimal results. However, the natural propagation of clinical trials from the recurrent and metastatic setting into the upfront and even neoadjuvant settings have resulted in encouraging data upon which ongoing and future clinical trials will follow. Current data show that RT fractionation and the sequence of surgery, RT, chemotherapy, immunotherapy, and targeted therapy should be designed to allow systemic immunity to develop. In particular, while upfront surgery followed by an immunotherapy-RT combination led to worse local control and immune response, neoadjuvant immunotherapy-RT combination followed by surgery resulted in better local control and systemic immunity. The optimal RT dose and its combination with immunotherapy and targeted therapy are still under investigation.
In conclusion, in recent years, there have been major advancements in the field of head and neck cancer. Although mostly confined to clinical trial settings, the evolution of an immune-directed approach to treat head and neck cancer seems well underway. Basic research studies and clinical trials are key to advancing toward the next milestone. And then to the next.
Author contributions
Conceptualization: SS, MG, NK; Investigation: SS, MG, NK; Data analysis and interpretation: SS, MG, NK, ND; Writing: SS, MG, NK, ND, DZ; Visualization: ND, NK, SS. All authors contributed to the article and approved the submitted version.
Acknowledgments
Figures 1 and 2 were created with BioRender.com.
Conflict of interest
The authors declare that the research was conducted in the absence of any commercial or financial relationships that could be construed as a potential conflict of interest.
Publisher's note
All claims expressed in this article are solely those of the authors and do not necessarily represent those of their affiliated organizations, or those of the publisher, the editors and the reviewers. Any product that may be evaluated in this article, or claim that may be made by its manufacturer, is not guaranteed or endorsed by the publisher.
References
1. Sung H, Ferlay J, Siegel RL, Laversanne M, Soerjomataram I, Jemal A, et al. Global cancer statistics 2020: GLOBOCAN estimates of incidence and mortality worldwide for 36 cancers in 185 countries. CA Cancer J Clin. (2021) 71(3):209–49. doi: 10.3322/caac.21660
3. León X, Hitt R, Constenla M, Rocca A, Stupp R, Kovács AF, et al. A retrospective analysis of the outcome of patients with recurrent and/or metastatic squamous cell carcinoma of the head and neck refractory to a platinum-based chemotherapy. Clin Oncol (R Coll Radiol). (2005) 17(6):418–24. doi: 10.1016/j.clon.2005.02.014
4. Park C, Papiez L, Zhang S, Story M, Timmerman RD. Universal survival curve and single fraction equivalent dose: useful tools in understanding potency of ablative radiotherapy. Int J Radiat Oncol Biol Phys. (2008) 70(3):847–52. doi: 10.1016/j.ijrobp.2007.10.059
5. Potters L, Kavanagh B, Galvin JM, Hevezi JM, Janjan NA, Larson DA, et al. American society for therapeutic radiology and oncology (ASTRO) and American college of radiology (ACR) practice guideline for the performance of stereotactic body radiation therapy. Int J Radiat Oncol Biol Phys. (2010) 76(2):326–32. doi: 10.1016/j.ijrobp.2009.09.042
6. Khan L, Tjong M, Raziee H, Lee J, Erler D, Chin L, et al. Role of stereotactic body radiotherapy for symptom control in head and neck cancer patients. Support Care Cancer. (2015) 23(4):1099–103. doi: 10.1007/s00520-014-2421-y
7. Siddiqui F, Patel M, Khan M, McLean S, Dragovic J, Jin JY, et al. Stereotactic body radiation therapy for primary, recurrent, and metastatic tumors in the head-and-neck region. Int J Radiat Oncol Biol Phys. (2009) 74(4):1047–53. doi: 10.1016/j.ijrobp.2008.09.022
8. Gogineni E, Rana Z, Vempati P, Karten J, Sharma A, Taylor P, et al. Stereotactic body radiotherapy as primary treatment for elderly and medically inoperable patients with head and neck cancer. Head Neck. (2020) 42(10):2880–6. doi: 10.1002/hed.26342
9. Wara WM. Immunosuppression associated with radiation therapy. Int J Radiat Oncol Biol Phys. (1977) 2:593–6. doi: 10.1016/0360-3016(77)90174-2
10. Walle T, Martinez Monge R, Cerwenka A, Ajona D, Melero I, Lecanda F. Radiation effects on antitumor immune responses: current perspectives and challenges. Ther Adv Med Oncol. (2018) 10:1758834017742575. doi: 10.1177/1758834017742575
11. Grossman SA, Ellsworth S, Campian J, Wild AT, Herman JM, Laheru D, et al. Survival in patients with severe lymphopenia following treatment with radiation and chemotherapy for newly diagnosed solid tumors. J Natl Compr Canc Netw. (2015) 13(10):1225–31. doi: 10.6004/jnccn.2015.0151
12. Damen PJJ, Kroese TE, van Hillegersberg R, Schuit E, Peters M, Verhoeff JJC, et al. The influence of severe radiation-induced lymphopenia on overall survival in solid tumors: a systematic review and meta-analysis. Int J Radiat Oncol Biol Phys. (2021) 111(4):936–48. doi: 10.1016/j.ijrobp.2021.07.1695
13. Formenti SC, Demaria S. Radiation therapy to convert the tumor into an in situ vaccine. Int J Radiat Oncol Biol Phys. (2012) 84(4):879–80. doi: 10.1016/j.ijrobp.2012.06.020
14. Demaria S, Ng B, Devitt ML, Babb JS, Kawashima N, Liebes L, et al. Ionizing radiation inhibition of distant untreated tumors (abscopal effect) is immune mediated. Int J Radiat Oncol Biol Phys. (2004) 58(3):862–70. doi: 10.1016/j.ijrobp.2003.09.012
15. Sharabi AB, Lim M, DeWeese TL, Drake CG. Radiation and checkpoint blockade immunotherapy: radiosensitisation and potential mechanisms of synergy. Lancet Oncol. (2015) 16(13):e498–e509. doi: 10.1016/S1470-2045(15)00007-8
16. Postow MA, Callahan MK, Barker CA, Yamada Y, Yuan J, Kitano S, et al. Immunologic correlates of the abscopal effect in a patient with melanoma. N Engl J Med. (2012) 366(10):925–31. doi: 10.1056/NEJMoa1112824
17. Ferris RL. Immunology and immunotherapy of head and neck cancer. J Clin Oncol. (2015) 33(29):3293–304. doi: 10.1200/JCO.2015.61.1509
18. Takeshima T, Chamoto K, Wakita D, Ohkuri T, Togashi Y, Shirato H, et al. Local radiation therapy inhibits tumor growth through the generation of tumor-specific CTL: its potentiation by combination with Th1 cell therapy. Cancer Res. (2010) 70(7):2697–706. doi: 10.1158/0008-5472.CAN-09-2982
19. Gulley JL, Arlen PM, Bastian A, Morin S, Marte J, Beetham P, et al. Combining a recombinant cancer vaccine with standard definitive radiotherapy in patients with localized prostate cancer. Clin Cancer Res. (2005) 11(9):3353–62. doi: 10.1158/1078-0432.CCR-04-2062
20. Baird JR, Monjazeb AM, Shah O, McGee H, Murphy WJ, Crittenden MR, et al. Stimulating innate immunity to enhance radiation therapy-induced tumor control. Int J Radiat Oncol Biol Phys. (2017) 99(2):362–73. doi: 10.1016/j.ijrobp.2017.04.014
21. Medler T, Patel JM, Alice A, Baird JR, Hu HM, Gough MJ. Activating the nucleic acid-sensing machinery for anticancer immunity. Int Rev Cell Mol Biol. (2019) 344:173–214. doi: 10.1016/bs.ircmb.2018.08.006
22. Melcher A, Gough M, Todryk S, Vile R. Apoptosis or necrosis for tumor immunotherapy: what’s in a name? J Mol Med (Berl). (1999) 77(12):824–33. doi: 10.1007/s001099900066
23. Galluzzi L, Vitale I, Aaronson SA, Abrams JM, Adam D, Agostinis P, et al. Molecular mechanisms of cell death: recommendations of the Nomenclature committee on cell death 2018. Cell Death Differ. (2018) 25:486–541. doi: 10.1038/s41418-017-0012-4
24. Medler TR, Blair TC, Crittenden MR, Gough MJ. Defining immunogenic and radioimmunogenic tumors. Front Oncol. (2021) 11:667075. doi: 10.3389/fonc.2021.667075
25. Medler TR, Blair TC, Alice AF, Dowdell AK, Piening BD, Crittenden MR, et al. Myeloid MyD88 restricts CD8+ T cell response to radiation therapy in pancreatic cancer. Sci Rep. (2023) 13(1):8634. doi: 10.1038/S41598-023-35834-W
26. Gough MJ, Young K, Crittenden M. The impact of the myeloid response to radiation therapy. Clin Dev Immunol. (2013) 2013:281958. doi: 10.1155/2013/281958
27. Melcher A, Bateman A, Harrington K, Ahmed A, Gough M, Vile R. Dendritic cells for the immunotherapy of cancer. Clin Oncol (R Coll Radiol). (2002) 14(3):185–92. doi: 10.1053/clon.2001.0038
28. Gough MJ, Melcher AA, Crittenden MR, Riddle DS, Linardakis E, Ruchatz AN, et al. Macrophages orchestrate the immune response to tumor cell death. Cancer Res. (2001) 61(19):7240–7.11585761
29. Preet Kaur A, Alice A, Crittenden MR, Gough MJ. The role of dendritic cells in radiation-induced immune responses. Int Rev Cell Mol Biol. (2023). doi: 10.1016/BS.IRCMB.2023.02.002
30. Blair TC, Bambina S, Kramer GF, Dowdell AK, Alice AF, Baird JR, et al. Fluorescent tracking identifies key migratory dendritic cells in the lymph node after radiotherapy. Life Sci Alliance. (2022) 5(9):e202101337. doi: 10.26508/lsa.202101337
31. Blair TC, Bambina S, Alice AF, Kramer GF, Medler TR, Baird JR, et al. Dendritic cell maturation defines immunological responsiveness of tumors to radiation therapy. J Immunol. (2020) 204(12):3416–24. doi: 10.4049/jimmunol.2000194
32. Gough MJ, Crittenden MR, Young KH. Comparing equals when evaluating immunotherapy with different doses and fractions of radiation therapy. Immunotherapy. (2015) 7(8):847–9. doi: 10.2217/IMT.15.58
33. Grandhi R, Kondziolka D, Panczykowski D, Monaco EA, Kano H, Niranjan A, et al. Stereotactic radiosurgery using the Leksell Gamma Knife Perfexion unit in the management of patients with 10 or more brain metastases. J Neurosurg. (2012) 117(2):237–45. doi: 10.3171/2012.4.JNS11870
34. Murray P, Franks K, Hanna GG. A systematic review of outcomes following stereotactic ablative radiotherapy in the treatment of early-stage primary lung cancer. Br J Radiol. (2017) 90(1071):20160732. doi: 10.1259/bjr.20160732
35. Haasbeek CJA, Lagerwaard FJ, Antonisse ME, Slotman BJ, Senan S. Stage I nonsmall cell lung cancer in patients aged ≥75 years. Cancer. (2010) 116(2):406–14. doi: 10.1002/cncr.24759
36. Bongers EM, Haasbeek CJA, Lagerwaard FJ, Slotman BJ, Senan S. Incidence and risk factors for chest wall toxicity after risk-adapted stereotactic radiotherapy for early-stage lung cancer. J Thorac Oncol. (2011) 6(12):2052–7. doi: 10.1097/JTO.0b013e3182307e74
37. Shaverdian N, Veruttipong D, Wang J, Schaue D, Kupelian P, Lee P. Pretreatment immune parameters predict for overall survival and toxicity in early-stage non–small-cell lung cancer patients treated with stereotactic body radiation therapy. Clin Lung Cancer. (2016) 17(1):39–46. doi: 10.1016/j.cllc.2015.07.007
38. Meier RM, Bloch DA, Cotrutz C, Beckman AC, Henning GT, Woodhouse SA, et al. Multicenter trial of stereotactic body radiation therapy for low- and intermediate-risk prostate cancer: survival and toxicity endpoints. Int J Radiat Oncol Biol Phys. (2018) 102(2):296–303. doi: 10.1016/j.ijrobp.2018.05.040
39. Darragh LB, Knitz MM, Hu J, Clambey ET, Backus J, Dumit A, et al. A phase I/Ib trial and biological correlate analysis of neoadjuvant SBRT with single-dose durvalumab in HPV-unrelated locally advanced HNSCC. Nat Cancer. (2022) 3(11):1300–17. doi: 10.1038/s43018-022-00450-6
40. Dunn GP, Bruce AT, Ikeda H, Old LJ, Schreiber RD. Cancer immunoediting: from immunosurveillance to tumor escape. Nat Immunol. (2002) 3(11):991–8. doi: 10.1038/ni1102-991
41. Freeman GJ, Long AJ, Iwai Y, Bourque K, Chernova T, Nishimura H, et al. Engagement of the PD-1 immunoinhibitory receptor by a novel B7 family member leads to negative regulation of lymphocyte activation. J Exp Med. (2000) 192(7):1027–34. doi: 10.1084/jem.192.7.1027
42. Ferris RL, Blumenschein G, Fayette J, Guigay J, Colevas AD, Licitra L, et al. Nivolumab for recurrent squamous-cell carcinoma of the head and neck. N Engl J Med. (2016) 375(19):1856–67. doi: 10.1056/NEJMoa1602252
43. Chow LQM, Haddad R, Gupta S, Mahipal A, Mehra R, Tahara M, et al. Antitumor activity of pembrolizumab in biomarker-unselected patients with recurrent and/or metastatic head and neck squamous cell carcinoma: results from the phase Ib KEYNOTE-012 expansion cohort. J Clin Oncol. (2016) 34(32):3838–45. doi: 10.1200/JCO.2016.68.1478
44. Ferris RL, Blumenschein G, Fayette J, Guigay J, Colevas AD, Licitra L, et al. Nivolumab vs investigator’s choice in recurrent or metastatic squamous cell carcinoma of the head and neck: 2-year long-term survival update of CheckMate 141 with analyses by tumor PD-L1 expression. Oral Oncol. (2018) 81:45–51. doi: 10.1016/j.oraloncology.2018.04.008
45. Even C, Daste A, Saada-Bouzid E, Lefebvre G, Fayette J, Zanetta S, et al. A safety study of nivolumab in patients with recurrent and/or metastatic platinum-refractory squamous cell carcinoma of the head and neck (R/M SCCHN): interim analysis on 199 patients—the TOPNIVO study on behalf of the GORTEC and the unicancer head & neck group. J Clin Oncol. (2019) 37(15_suppl):6032. doi: 10.1200/JCO.2019.37.15_suppl.6032
46. Burtness B, Harrington KJ, Greil R, Soulières D, Tahara M, de Castro G, et al. Pembrolizumab alone or with chemotherapy versus cetuximab with chemotherapy for recurrent or metastatic squamous cell carcinoma of the head and neck (KEYNOTE-048): a randomised, open-label, phase 3 study. Lancet. (2019) 394(10212):1915–28. doi: 10.1016/S0140-6736(19)32591-7
47. Lee NY, Ferris RL, Psyrri A, Haddad RI, Tahara M, Bourhis J, et al. Avelumab plus standard-of-care chemoradiotherapy versus chemoradiotherapy alone in patients with locally advanced squamous cell carcinoma of the head and neck: a randomised, double-blind, placebo-controlled, multicentre, phase 3 trial. Lancet Oncol. (2021) 22(4):450–62. doi: 10.1016/S1470-2045(20)30737-3
48. Tao Y, Biau J, Sun XS, Sire C, Martin L, Alfonsi M, et al. Pembrolizumab versus cetuximab concurrent with radiotherapy in patients with locally advanced squamous cell carcinoma of head and neck unfit for cisplatin (GORTEC 2015-01 PembroRad): a multicenter, randomized, phase II trial. Ann Oncol. (2023) 34(1):101–10. doi: 10.1016/j.annonc.2022.10.006
49. Sharon S, Bryan Bell R. Immunotherapy in head and neck squamous cell carcinoma: a narrative review. Front Oral Maxillofac Med. (2022) 4:28. doi: 10.21037/fomm-21-48
50. Gough MJ, Sharon S, Crittenden MR, Young KH. Using preclinical data to design combination clinical trials of radiation therapy and immunotherapy. Semin Radiat Oncol. (2020) 30(2):158–72. doi: 10.1016/j.semradonc.2019.12.002
51. Filatenkov A, Baker J, Mueller AM, Kenkel J, Ahn GO, Dutt S, et al. Ablative tumor radiation can change the tumor immune cell microenvironment to induce durable complete remissions. Clin Cancer Res. (2015) 21(16):3727–39. doi: 10.1158/1078-0432.CCR-14-2824
52. Gough MJ, Crittenden MR. The paradox of radiation and T cells in tumors. Neoplasia. (2022) 31:100808. doi: 10.1016/j.neo.2022.100808
53. Lugade AA, Moran JP, Gerber SA, Rose RC, Frelinger JG, Lord EM. Local radiation therapy of B16 melanoma tumors increases the generation of tumor antigen-specific effector cells that traffic to the tumor. J Immunol. (2005) 174(12):7516–23. doi: 10.4049/jimmunol.174.12.7516
54. Lee Y, Auh SL, Wang Y, Burnette B, Wang Y, Meng Y, et al. Therapeutic effects of ablative radiation on local tumor require CD8+ T cells: changing strategies for cancer treatment. Blood. (2009) 114(3):589–95. doi: 10.1182/blood-2009-02-206870
55. Dewan MZ, Galloway AE, Kawashima N, Dewyngaert JK, Babb JS, Formenti SC, et al. Fractionated but not single-dose radiotherapy induces an immune-mediated abscopal effect when combined with anti-CTLA-4 antibody. Clin Cancer Res. (2009) 15(17):5379–88. doi: 10.1158/1078-0432.CCR-09-0265
56. Harding SM, Benci JL, Irianto J, Discher DE, Minn AJ, Greenberg RA. Mitotic progression following DNA damage enables pattern recognition within micronuclei. Nature. (2017) 548(7668):466–70. doi: 10.1038/nature23470
57. Vanpouille-Box C, Alard A, Aryankalayil MJ, Sarfraz Y, Diamond JM, Schneider RJ, et al. DNA Exonuclease Trex1 regulates radiotherapy-induced tumour immunogenicity. Nat Commun. (2017) 8:15618. doi: 10.1038/ncomms15618
58. Morisada M, Moore EC, Hodge R, Friedman J, Cash HA, Hodge JW, et al. Dose-dependent enhancement of T-lymphocyte priming and CTL lysis following ionizing radiation in an engineered model of oral cancer. Oral Oncol. (2017) 71:87–94. doi: 10.1016/j.oraloncology.2017.06.005
59. Morisada M, Clavijo PE, Moore E, Sun L, Chamberlin M, Van Waes C, et al. PD-1 blockade reverses adaptive immune resistance induced by high-dose hypofractionated but not low-dose daily fractionated radiation. Oncoimmunology. (2018) 7(3):e1395996. doi: 10.1080/2162402X.2017.1395996
60. Dovedi SJ, Adlard AL, Lipowska-Bhalla G, McKenna C, Jones S, Cheadle EJ, et al. Acquired resistance to fractionated radiotherapy can be overcome by concurrent PD-L1 blockade. Cancer Res. (2014) 74(19):5458–68. doi: 10.1158/0008-5472.CAN-14-1258
61. Dovedi SJ, Cheadle EJ, Popple AL, Poon E, Morrow M, Stewart R, et al. Fractionated radiation therapy stimulates antitumor immunity mediated by both resident and infiltrating polyclonal T-cell populations when combined with PD-1 blockade. Clin Cancer Res. (2017) 23(18):5514–26. doi: 10.1158/1078-0432.CCR-16-1673
62. Klug F, Prakash H, Huber PE, Seibel T, Bender N, Halama N, et al. Low-dose irradiation programs macrophage differentiation to an iNOS(+)/M1 phenotype that orchestrates effective T cell immunotherapy. Cancer Cell. (2013) 24(5):589–602. doi: 10.1016/j.ccr.2013.09.014
63. Gunderson AJ, Yamazaki T, McCarty K, Fox N, Phillips M, Alice A, et al. TGFbeta suppresses CD8(+) T cell expression of CXCR3 and tumor trafficking. Nat Commun. (2020) 11(1):1749. doi: 10.1038/s41467-020-15404-8
64. Leidner R, Crittenden M, Young K, Xiao H, Wu Y, Couey MA, et al. Neoadjuvant immunoradiotherapy results in high rate of complete pathological response and clinical to pathological downstaging in locally advanced head and neck squamous cell carcinoma. J Immunother Cancer. (2021) 9(5):e002485. doi: 10.1136/jitc-2021-002485
65. Curti B, Crittenden M, Seung SK, Fountain CB, Payne R, Chang SC, et al. Randomized phase II study of stereotactic body radiotherapy and interleukin-2 versus interleukin-2 in patients with metastatic melanoma. J Immunother Cancer. (2020) 8(1):e000773. doi: 10.1136/jitc-2020-000773
66. Seung SK, Curti BD, Crittenden M, Walker E, Coffey T, Siebert JC, et al. Phase 1 study of stereotactic body radiotherapy and interleukin-2--tumor and immunological responses. Sci Transl Med. (2012) 4(137):137ra74. doi: 10.1126/scitranslmed.3003649
67. Yamazaki T, Gunderson AJ, Gilchrist M, Whiteford M, Kiely MX, Hayman A, et al. Galunisertib plus neoadjuvant chemoradiotherapy in patients with locally advanced rectal cancer: a single-arm, phase 2 trial. Lancet Oncol. (2022) 23(9):1189–200. doi: 10.1016/S1470-2045(22)00446-6
68. Young KH, Baird JR, Savage T, Cottam B, Friedman D, Bambina S, et al. Optimizing timing of immunotherapy improves control of tumors by hypofractionated radiation therapy. PLoS One. (2016) 11(6):e0157164. doi: 10.1371/journal.pone.0157164
69. Blair TC, Alice AF, Zebertavage L, Crittenden MR, Gough MJ. The dynamic entropy of tumor immune infiltrates: the impact of recirculation, antigen-specific interactions, and retention on T cells in tumors. Front Oncol. (2021) 11:653625. doi: 10.3389/fonc.2021.653625
70. Fuks Z, Strober S, Bobrove AM, Sasazuki T, McMichael A, Kaplan HS. Long term effects of radiation of T and B lymphocytes in peripheral blood of patients with Hodgkin’s disease. J Clin Invest. (1976) 58(4):803–14. doi: 10.1172/JCI108532
71. Stewart CC, Perez CA. Effect of irradiation on immune responses. Radiology. (1976) 118(1):201–10. doi: 10.1148/118.1.201
72. Wild AT, Ye X, Ellsworth SG, Smith JA, Narang AK, Garg T, et al. The association between chemoradiation-related lymphopenia and clinical outcomes in patients with locally advanced pancreatic adenocarcinoma. Am J Clin Oncol. (2015) 38(3):259–65. doi: 10.1097/COC.0b013e3182940ff9
73. Savage AM, Pritchard JA, Deeley TJ, Davies BH. Immunological state of patients with carcinoma of the bronchus before and after radiotherapy. Thorax. (1980) 35(7):500–5. doi: 10.1136/thx.35.7.500
74. Yovino S, Kleinberg L, Grossman SA, Narayanan M, Ford E. The etiology of treatment-related lymphopenia in patients with malignant gliomas: modeling radiation dose to circulating lymphocytes explains clinical observations and suggests methods of modifying the impact of radiation on immune cells. Cancer Invest. (2013) 31(2):140–4. doi: 10.3109/07357907.2012.762780
75. Ellsworth SG. Field size effects on the risk and severity of treatment-induced lymphopenia in patients undergoing radiation therapy for solid tumors. Adv Radiat Oncol. (2018) 3(4):512–9. doi: 10.1016/j.adro.2018.08.014
76. Crocenzi T, Cottam B, Newell P, Wolf RF, Hansen PD, Hammill C, et al. A hypofractionated radiation regimen avoids the lymphopenia associated with neoadjuvant chemoradiation therapy of borderline resectable and locally advanced pancreatic adenocarcinoma. J Immunother Cancer. (2016) 4:45. doi: 10.1186/s40425-016-0149-6
77. Wild AT, Herman JM, Dholakia AS, Moningi S, Lu Y, Rosati LM, et al. Lymphocyte-sparing effect of stereotactic body radiation therapy in patients with unresectable pancreatic cancer. Int J Radiat Oncol Biol Phys. (2016) 94(3):571–9. doi: 10.1016/j.ijrobp.2015.11.026
78. Arina A, Beckett M, Fernandez C, Zheng W, Pitroda S, Chmura SJ, et al. Tumor-reprogrammed resident T cells resist radiation to control tumors. Nat Commun. (2019) 10(1):3959. doi: 10.1038/s41467-019-11906-2
79. Crittenden MR, Zebertavage L, Kramer G, Bambina S, Friedman D, Troesch V, et al. Tumor cure by radiation therapy and checkpoint inhibitors depends on pre-existing immunity. Sci Rep. (2018) 8(1):7012. doi: 10.1038/s41598-018-25482-w
80. Duhen T, Duhen R, Montler R, Moses J, Moudgil T, de Miranda NF, et al. Co-expression of CD39 and CD103 identifies tumor-reactive CD8 T cells in human solid tumors. Nat Commun. (2018) 9(1):2724. doi: 10.1038/s41467-018-05072-0
81. Muroyama Y, Nirschl TR, Kochel CM, Lopez-Bujanda Z, Theodros D, Mao W, et al. Stereotactic radiotherapy increases functionally suppressive regulatory T cells in the tumor microenvironment. Cancer Immunol Res. (2017) 5(11):992–1004. doi: 10.1158/2326-6066.CIR-17-0040
82. Marciscano AE, Ghasemzadeh A, Nirschl TR, Theodros D, Kochel CM, Francica BJ, et al. Elective nodal irradiation attenuates the combinatorial efficacy of stereotactic radiation therapy and immunotherapy. Clin Cancer Res. (2018) 24(20):5058–71. doi: 10.1158/1078-0432.CCR-17-3427
83. Heylmann D, Ponath V, Kindler T, Kaina B. Comparison of DNA repair and radiosensitivity of different blood cell populations. Sci Rep. (2021) 11(1):2478. doi: 10.1038/s41598-021-81058-1
84. Falcke SE, Ruhle PF, Deloch L, Fietkau R, Frey B, Gaipl US. Clinically relevant radiation exposure differentially impacts forms of cell death in human cells of the innate and adaptive immune system. Int J Mol Sci. (2018) 19(11):3574. doi: 10.3390/ijms19113574
85. Liao YP, Wang CC, Butterfield LH, Economou JS, Ribas A, Meng WS, et al. Ionizing radiation affects human MART-1 melanoma antigen processing and presentation by dendritic cells. J Immunol. (2004) 173(4):2462–9. doi: 10.4049/jimmunol.173.4.2462
86. Persa E, Szatmari T, Safrany G, Lumniczky K. In vivo irradiation of mice induces activation of dendritic cells. Int J Mol Sci. (2018) 19(8). doi: 10.3390/ijms19082391
87. Merad M, Manz MG, Karsunky H, Wagers A, Peters W, Charo I, et al. Langerhans cells renew in the skin throughout life under steady-state conditions. Nat Immunol. (2002) 3(12):1135–41. doi: 10.1038/ni852
88. Merrick A, Errington F, Milward K, O’Donnell D, Harrington K, Bateman A, et al. Immunosuppressive effects of radiation on human dendritic cells: reduced IL-12 production on activation and impairment of naive T-cell priming. Br J Cancer. (2005) 92(8):1450–8. doi: 10.1038/sj.bjc.6602518
89. Cao MD, Chen ZD, Xing Y. Gamma irradiation of human dendritic cells influences proliferation and cytokine profile of T cells in autologous mixed lymphocyte reaction. Cell Biol Int. (2004) 28(3):223–8. doi: 10.1016/j.cellbi.2003.12.006
90. Malecka A, Wang Q, Shah S, Sutavani RV, Spendlove I, Ramage JM, et al. Stromal fibroblasts support dendritic cells to maintain IL-23/Th17 responses after exposure to ionizing radiation. J Leukoc Biol. (2016) 100(2):381–9. doi: 10.1189/jlb.3A1015-474R
91. Darragh LB, Gadwa J, Pham TT, Van Court B, Neupert B, Olimpo NA, et al. Elective nodal irradiation mitigates local and systemic immunity generated by combination radiation and immunotherapy in head and neck tumors. Nat Commun. (2022) 13(1):7015. doi: 10.1038/s41467-022-34676-w
92. Matsuda T, Miyauchi E, Hsu YW, Nagayama S, Kiyotani K, Zewde M, et al. TCR Sequencing analysis of cancer tissues and tumor draining lymph nodes in colorectal cancer patients. Oncoimmunology. (2019) 8(6):e1588085. doi: 10.1080/2162402X.2019.1588085
93. Munn DH, Mellor AL. The tumor-draining lymph node as an immune-privileged site. Immunol Rev. (2006) 213(1):146–58. doi: 10.1111/j.1600-065X.2006.00444.x
94. Swartz MA, Lund AW. Lymphatic and interstitial flow in the tumour microenvironment: linking mechanobiology with immunity. Nat Rev Cancer. (2012) 12(3):210–9. doi: 10.1038/nrc3186
95. Cochran AJ, Huang RR, Lee J, Itakura E, Leong SPL, Essner R. Tumour-induced immune modulation of sentinel lymph nodes. Nat Rev Immunol. (2006) 6(9):659–70. doi: 10.1038/nri1919
96. Saddawi-Konefka R, O’Farrell A, Faraji F, Clubb L, Allevato MM, Jensen SM, et al. Lymphatic-preserving treatment sequencing with immune checkpoint inhibition unleashes cDC1-dependent antitumor immunity in HNSCC. Nat Commun. (2022) 13(1):4298. doi: 10.1038/s41467-022-31941-w
97. Golpon HA, Fadok VA, Taraseviciene-Stewart L, Scerbavicius R, Sauer C, Welte T, et al. Life after corpse engulfment: phagocytosis of apoptotic cells leads to VEGF secretion and cell growth. FASEB J. (2004) 18(14):1716–8. doi: 10.1096/fj.04-1853fje
98. Savill J, Fadok V. Corpse clearance defines the meaning of cell death. Nature. (2000) 407(6805):784–8. doi: 10.1038/35037722
99. Tormoen GW, Crittenden MR, Gough MJ. The TAM family as a therapeutic target in combination with radiation therapy. Emerg Top Life Sci. (2017) 1(5):493–500. doi: 10.1042/ETLS20170066
100. Gough MJ, Killeen N, Weinberg AD. Targeting macrophages in the tumour environment to enhance the efficacy of alphaOX40 therapy. Immunology. (2012) 136(4):437–47. doi: 10.1111/j.1365-2567.2012.03600.x
101. Garg AD, Vara Perez M, Schaaf M, Agostinis P, Zitvogel L, Kroemer G, et al. Trial watch: dendritic cell-based anticancer immunotherapy. Oncoimmunology. (2017) 6(7):1328341. doi: 10.1080/2162402X.2017.1328341
102. Schoenberger SP, Toes RE, van der Voort EI, Offringa R, Melief CJ. T-cell help for cytotoxic T lymphocytes is mediated by CD40-CD40l interactions. Nature. (1998) 393(6684):480–3. doi: 10.1038/31002
103. Ridge JP, Di Rosa F, Matzinger P. A conditioned dendritic cell can be a temporal bridge between a CD4+ T-helper and a T-killer cell. Nature. (1998) 393(6684):474–8. doi: 10.1038/30989
104. Bennett SR, Carbone FR, Karamalis F, Flavell RA, Miller JF, Heath WR. Help for cytotoxic-T-cell responses is mediated by CD40 signalling. Nature. (1998) 393(6684):478–80. doi: 10.1038/30996
105. Cella M, Engering A, Pinet V, Pieters J, Lanzavecchia A. Inflammatory stimuli induce accumulation of MHC class II complexes on dendritic cells. Nature. (1997) 388(6644):782–7. doi: 10.1038/42030
106. Hui E, Cheung J, Zhu J, Su X, Taylor MJ, Wallweber HA, et al. T cell costimulatory receptor CD28 is a primary target for PD-1-mediated inhibition. Science. (2017) 355(6332):1428–33. doi: 10.1126/science.aaf1292
107. Caux C, Vanbervliet B, Massacrier C, Azuma M, Okumura K, Lanier LL, et al. B70/B7-2 is identical to CD86 and is the major functional ligand for CD28 expressed on human dendritic cells. J Exp Med. (1994) 180(5):1841–7. doi: 10.1084/jem.180.5.1841
108. Azuma M, Ito D, Yagita H, Okumura K, Phillips JH, Lanier LL, et al. B70 antigen is a second ligand for CTLA-4 and CD28. Nature. (1993) 366(6450):76–9. doi: 10.1038/366076a0
109. Harding FA, McArthur JG, Gross JA, Raulet DH, Allison JP. CD28-mediated Signalling co-stimulates murine T cells and prevents induction of anergy in T-cell clones. Nature. (1992) 356(6370):607–9. doi: 10.1038/356607a0
110. Jenkins MK, Taylor PS, Norton SD, Urdahl KB. CD28 Delivers a costimulatory signal involved in antigen-specific IL-2 production by human T cells. J Immunol. (1991) 147(8):2461–6. doi: 10.4049/jimmunol.147.8.2461
111. Li Y, Li XC, Zheng XX, Wells AD, Turka LA, Strom TB. Blocking both signal 1 and signal 2 of T-cell activation prevents apoptosis of alloreactive T cells and induction of peripheral allograft tolerance. Nat Med. (1999) 5(11):1298–302. doi: 10.1038/15256
112. Matzinger P. Tolerance, danger, and the extended family. Annu Rev Immunol. (1994) 12:991–1045. doi: 10.1146/annurev.iy.12.040194.005015
113. Burdette DL, Vance RE. STING And the innate immune response to nucleic acids in the cytosol. Nat Immunol. (2013) 14(1):19–26. doi: 10.1038/ni.2491
114. Chen Q, Sun L, Chen ZJ. Regulation and function of the cGAS-STING pathway of cytosolic DNA sensing. Nat Immunol. (2016) 17(10):1142–9. doi: 10.1038/ni.3558
115. Quarmby S, Kumar P, Kumar S. Radiation-induced normal tissue injury: role of adhesion molecules in leukocyte–endothelial cell interactions. Int J Cancer. (1999) 82(3):385–95. doi: 10.1002/(SICI)1097-0215(19990730)82:3%3C385::AID-IJC12%3E3.0.CO;2-5
116. Rubin P, Johnston CJ, Williams JP, McDonald S, Finkelstein JN. A perpetual cascade of cytokines postirradiation leads to pulmonary fibrosis. Int J Radiat Oncol Biol Phys. (1995) 33(1):99–109. doi: 10.1016/0360-3016(95)00095-G
117. Hallahan DE, Haimovitz-Friedman A, Kufe DW, Fuks Z, Weichselbaum RR. The role of cytokines in radiation oncology. Important Adv Oncol. (1993):71–80.8505057
118. Hallahan DE, Virudachalam S, Sherman ML, Huberman E, Kufe DW, Weichselbaum RR. Tumor necrosis factor gene expression is mediated by protein kinase C following activation by ionizing radiation. Cancer Res. (1991) 51(17):4565–9.1873801
119. Weichselbaum RR, Kufe DW, Hellman S, Rasmussen HS, King CR, Fischer PH, et al. Radiation-induced tumour necrosis factor-alpha expression: clinical application of transcriptional and physical targeting of gene therapy. Lancet Oncol. (2002) 3(11):665–71. doi: 10.1016/S1470-2045(02)00900-2
120. Camphausen K, Moses MA, Beecken WD, Khan MK, Folkman J, O’Reilly MS. Radiation therapy to a primary tumor accelerates metastatic growth in mice. Cancer Res. (2001) 61(5):2207–11.11280788
121. Seymour CB, Mothersill C. Radiation-induced bystander effects — implications for cancer. Nat Rev Cancer. (2004) 4(2):158–64. doi: 10.1038/nrc1277
122. Hellman S, Weichselbaum RR. Oligometastases. J Clin Oncol. (1995) 13(1):8–10. doi: 10.1200/JCO.1995.13.1.8
123. Guckenberger M, Lievens Y, Bouma AB, Collette L, Dekker A, deSouza NM, et al. Characterisation and classification of oligometastatic disease: a European society for radiotherapy and oncology and European organisation for research and treatment of cancer consensus recommendation. Lancet Oncol. (2020) 21(1):e18–e28. doi: 10.1016/S1470-2045(19)30718-1
124. Pastorino U, Buyse M, Friedel G, Ginsberg RJ, Girard P, Goldstraw P, et al. Long-term results of lung metastasectomy: prognostic analyses based on 5206 cases. J Thorac Cardiovasc Surg. (1997) 113(1):37–49. doi: 10.1016/S0022-5223(97)70397-0
125. Haridass A. Developments in stereotactic body radiotherapy. Cancers (Basel). (2018) 10(12):497. doi: 10.3390/cancers10120497
126. Bates JE, de Leo AN, Morris CG, Amdur RJ, Dagan R. Oligometastatic squamous cell carcinoma of the head and neck treated with stereotactic body ablative radiotherapy: single-institution outcomes. Head Neck. (2019) 41(7):2309–14. doi: 10.1002/hed.25695
127. Zhang Z, Liu X, Chen D, Yu J. Radiotherapy combined with immunotherapy: the dawn of cancer treatment. Signal Transduct Target Ther. (2022) 7(1):258. doi: 10.1038/s41392-022-01102-y
128. Jagodinsky JC, Harari PM, Morris ZS. The promise of combining radiation therapy with immunotherapy. Int J Radiat Oncol Biol Phys. (2020) 108(1):6–16. doi: 10.1016/j.ijrobp.2020.04.023
129. Van Limbergen EJ, De Ruysscher DK, Olivo Pimentel V, Marcus D, Berbee M, Hoeben A, et al. Combining radiotherapy with immunotherapy: the past, the present and the future. Br J Radiol. (2017) 90(1076):20170157. doi: 10.1259/bjr.20170157
130. Blair T, Baird J, Bambina S, Kramer G, Gostissa M, Harvey CJ, et al. ICOS Is upregulated on T cells following radiation and agonism combined with radiation results in enhanced tumor control. Sci Rep. (2022) 12(1):14954. doi: 10.1038/s41598-022-19256-8
131. Gough MJ, Crittenden MR, Sarff M, Pang P, Seung SK, Vetto JT, et al. Adjuvant therapy with agonistic antibodies to CD134 (OX40) increases local control after surgical or radiation therapy of cancer in mice. J Immunother. (2010) 33(8):798–809. doi: 10.1097/CJI.0b013e3181ee7095
132. Demaria S, Kawashima N, Yang AM, Devitt ML, Babb JS, Allison JP, et al. Immune-mediated inhibition of metastases after treatment with local radiation and CTLA-4 blockade in a mouse model of breast cancer. Clin Cancer Res. (2005) 11(2 Pt 1):728–34. doi: 10.1158/1078-0432.728.11.2
133. Azad A, Yin Lim S, D’Costa Z, Jones K, Diana A, Sansom OJ, et al. PD-L1 blockade enhances response of pancreatic ductal adenocarcinoma to radiotherapy. EMBO Mol Med. (2017) 9(2):167–80. doi: 10.15252/emmm.201606674
134. Zeng J, See AP, Phallen J, Jackson CM, Belcaid Z, Ruzevick J, et al. Anti-PD-1 blockade and stereotactic radiation produce long-term survival in mice with intracranial gliomas. Int J Radiat Oncol Biol Phys. (2013) 86(2):343–9. doi: 10.1016/j.ijrobp.2012.12.025
135. Park SS, Dong H, Liu X, Harrington SM, Krco CJ, Grams MP, et al. PD-1 restrains radiotherapy-induced abscopal effect. Cancer Immunol Res. (2015) 3(6):610–9. doi: 10.1158/2326-6066.CIR-14-0138
136. Oweida A, Lennon S, Calame D, Korpela S, Bhatia S, Sharma J, et al. Ionizing radiation sensitizes tumors to PD-L1 immune checkpoint blockade in orthotopic murine head and neck squamous cell carcinoma. Oncoimmunology. (2017) 6(10):e1356153. doi: 10.1080/2162402X.2017.1356153
137. Deng L, Liang H, Burnette B, Beckett M, Darga T, Weichselbaum RR, et al. Irradiation and anti–PD-L1 treatment synergistically promote antitumor immunity in mice. J Clin Invest. (2014) 124(2):687–95. doi: 10.1172/JCI67313
138. Twyman-Saint Victor C, Rech AJ, Maity A, Rengan R, Pauken KE, Stelekati E, et al. Radiation and dual checkpoint blockade activate non-redundant immune mechanisms in cancer. Nature. (2015) 520(7547):373–7. doi: 10.1038/nature14292
139. Newcomb EW, Lukyanov Y, Kawashima N, Alonso-Basanta M, Wang SC, Liu M, et al. Radiotherapy enhances antitumor effect of anti-CD137 therapy in a mouse Glioma model. Radiat Res. (2010) 173(4):426–32. doi: 10.1667/RR1904.1
140. Patel MA, Kim JE, Theodros D, Tam A, Velarde E, Kochel CM, et al. Agonist anti-GITR monoclonal antibody and stereotactic radiation induce immune-mediated survival advantage in murine intracranial glioma. J Immunother Cancer. (2016) 4:28. doi: 10.1186/s40425-016-0132-2
141. Kim JE, Patel MA, Mangraviti A, Kim ES, Theodros D, Velarde E, et al. Combination therapy with anti-PD-1, anti-TIM-3, and focal radiation results in regression of murine gliomas. Clin Cancer Res. (2017) 23(1):124–36. doi: 10.1158/1078-0432.CCR-15-1535
142. Hu Y, Paris S, Bertolet G, Barsoumian HB, He K, Sezen D, et al. Combining a nanoparticle-mediated immunoradiotherapy with dual blockade of LAG3 and TIGIT improves the treatment efficacy in anti-PD1 resistant lung cancer. J Nanobiotechnology. (2022) 20(1):417. doi: 10.1186/s12951-022-01621-4
143. Machtay M, Moughan J, Trotti A, Garden AS, Weber RS, Cooper JS, et al. Factors associated with severe late toxicity after concurrent chemoradiation for locally advanced head and neck cancer: an RTOG analysis. J Clin Oncol. (2008) 26(21):3582–9. doi: 10.1200/JCO.2007.14.8841
144. Daly ME, Lau DH, Farwell DG, Luu Q, Donald PJ, Chen AM. Feasibility and toxicity of concurrent chemoradiation for elderly patients with head and neck cancer. Am J Otolaryngol. (2013) 34(6):631–5. doi: 10.1016/j.amjoto.2013.07.010
145. Diao K, Nguyen TP, Moreno AC, Reddy JP, Garden AS, Wang CH, et al. Stereotactic body ablative radiotherapy for reirradiation of small volume head and neck cancers is associated with prolonged survival: large, single-institution, modern cohort study. Head Neck. (2021) 43(11):3331–44. doi: 10.1002/hed.26820
146. Yamazaki H, Ogita M, Himei K, Nakamura S, Suzuki G, Yoshida K, et al. Reirradiation using robotic image-guided stereotactic radiotherapy of recurrent head and neck cancer. J Radiat Res. (2016) 57(3):288–93. doi: 10.1093/jrr/rrw004
147. Shikama N, Kumazaki Y, Tsukamoto N, Ebara T, Makino S, Abe T, et al. Validation of nomogram-based prediction of survival probability after salvage re-irradiation of head and neck cancer. Jpn J Clin Oncol. (2013) 43(2):154–60. doi: 10.1093/jjco/hys210
148. Rwigema JCM, Heron DE, Ferris RL, Andrade RS, Gibson MK, Yang Y, et al. The impact of tumor volume and radiotherapy dose on outcome in previously irradiated recurrent squamous cell carcinoma of the head and neck treated with stereotactic body radiation therapy. Am J Clin Oncol. (2011) 34(4):372–9. doi: 10.1097/COC.0b013e3181e84dc0
149. Vargo JA, Ferris RL, Ohr J, Clump DA, Davis KS, Duvvuri U, et al. A prospective phase 2 trial of reirradiation with stereotactic body radiation therapy plus cetuximab in patients with previously irradiated recurrent squamous cell carcinoma of the head and neck. Int J Radiat Oncol Biol Phys. (2015) 91(3):480–8. doi: 10.1016/j.ijrobp.2014.11.023
150. Lartigau EF, Tresch E, Thariat J, Graff P, Coche-Dequeant B, Benezery K, et al. Multi institutional phase II study of concomitant stereotactic reirradiation and cetuximab for recurrent head and neck cancer. Radiother Oncol. (2013) 109(2):281–5. doi: 10.1016/j.radonc.2013.08.012
151. Karam I, Yao M, Heron DE, Poon I, Koyfman SA, Yom SS, et al. Survey of current practices from the international stereotactic body radiotherapy consortium (ISBRTC) for head and neck cancers. Future Oncol. (2017) 13(7):603–13. doi: 10.2217/fon-2016-0403
152. Yamazaki H, Ogita M, Himei K, Nakamura S, Yoshida K, Kotsuma T, et al. Hypofractionated stereotactic radiotherapy using CyberKnife as a boost treatment for head and neck cancer, a multi-institutional survey: impact of planning target volume. Anticancer Res. (2014) 34(10):5755–9.25275085
153. Lee DS, Kim YS, Cheon JS, Song JH, Son SH, Jang JS, et al. Long-term outcome and toxicity of hypofractionated stereotactic body radiotherapy as a boost treatment for head and neck cancer: the importance of boost volume assessment. Radiat Oncol. (2012) 7:85. doi: 10.1186/1748-717x-7-85
154. Uno T, Isobe K, Ueno N, Fukuda A, Sudo S, Shirotori H, et al. Fractionated stereotactic radiotherapy as a boost treatment for tumors in the head and neck region. J Radiat Res. (2010) 51(4):449–54. doi: 10.1269/jrr.10040
155. Amini A, McDermott JD, Gan G, Bhatia S, Sumner W, Fisher CM, et al. Stereotactic body radiotherapy as primary therapy for head and neck cancer in the elderly or patients with poor performance. Front Oncol. (2014) 4:274. doi: 10.3389/fonc.2014.00274
156. Vargo JA, Ward MC, Caudell JJ, Riaz N, Dunlap NE, Isrow D, et al. A multi-institutional comparison of SBRT and IMRT for definitive reirradiation of recurrent or second primary head and neck cancer. Int J Radiat Oncol Biol Phys. (2018) 100(3):595–605. doi: 10.1016/j.ijrobp.2017.04.017
157. Spencer SA, Harris J, Wheeler RH, Machtay M, Schultz C, Spanos W, et al. Final report of RTOG 9610, a multi-institutional trial of reirradiation and chemotherapy for unresectable recurrent squamous cell carcinoma of the head and neck. Head Neck. (2008) 30(3):281–8. doi: 10.1002/hed.20697
158. Langer CJ, Harris J, Horwitz EM, Nicolaou N, Kies M, Curran W, et al. Phase II study of low-dose paclitaxel and cisplatin in combination with split-course concomitant twice-daily reirradiation in recurrent squamous cell carcinoma of the head and neck: results of radiation therapy oncology group protocol 9911. J Clin Oncol. (2007) 25(30):4800–5. doi: 10.1200/JCO.2006.07.9194
159. Finegersh A, Moss WJ, Saddawi-Konefka R, Faraji F, Coffey CS, Califano JA, et al. Meta-analysis of risk of occult lymph node metastasis in the irradiated, clinically N0 neck. Head Neck. (2020) 42(9):2355–63. doi: 10.1002/hed.26248
160. Al-Assaf H, Erler D, Karam I, Lee JW, Higgins K, Enepekides D, et al. Stereotactic body radiotherapy for medically unfit patients with cancers to the head and neck. Head Neck. (2020) 42(8):2050–7. doi: 10.1002/hed.26138
161. Kodani N, Yamazaki H, Tsubokura T, Shiomi H, Kobayashi K, Nishimura T, et al. Stereotactic body radiation therapy for head and neck tumor: disease control and morbidity outcomes. J Radiat Res. (2011) 52(1):24–31. doi: 10.1269/jrr.10086
162. Adlakha P, Maheshwari G, Dhanawat A, Sinwer R, Singhal M, Jakhar S, et al. Comparison of two schedules of hypo-fractionated radiotherapy in locally advanced head-and-neck cancers. J Cancer Res Ther. (2022) 18(Suppl.):S151–6. doi: 10.4103/JCRT.JCRT_1793_20
163. Singh R, Jenkins J, Davis J, Song S, Sharma S, Vargo JA. A multi-institutional analysis of outcomes following stereotactic body radiation therapy for management of metastases from squamous cell carcinomas of the head and neck. J Radiosurg SBRT. (2022) 8(1):11.35387401
164. Huang TL, Chuang HC, Tsai MH, Chien CY, Su YY, Lin YT, et al. Stereotactic body radiotherapy plus cetuximab for previously irradiated un-resectable head and neck cancer. Biomed J. (2022) 45(5):838. doi: 10.1016/j.bj.2021.10.013
165. Kress MAS, Sen N, Unger KR, Lominska CE, Deeken JF, Davidson BJ, et al. Safety and efficacy of hypofractionated stereotactic body reirradiation in head and neck cancer: long-term follow-up of a large series. Head Neck. (2015) 37(10):1403–9. doi: 10.1002/hed.23763
166. Park JJ, Qureshi MM, Lam CM, Faden DL, Truong MT. A national cancer database analysis on stereotactic body radiation therapy of head and neck cancers. Am J Otolaryngol. (2021) 42(3):102913. doi: 10.1016/J.AMJOTO.2021.102913
167. Cohen EEW, Soulières D, Le Tourneau C, Dinis J, Licitra L, Ahn MJ, et al. Pembrolizumab versus methotrexate, docetaxel, or cetuximab for recurrent or metastatic head-and-neck squamous cell carcinoma (KEYNOTE-040): a randomised, open-label, phase 3 study. Lancet. (2019) 393(10167):156–67. doi: 10.1016/S0140-6736(18)31999-8
168. Fransen MF, Schoonderwoerd M, Knopf P, Camps MG, Hawinkels LJ, Kneilling M, et al. Tumor-draining lymph nodes are pivotal in PD-1/PD-L1 checkpoint therapy. JCI Insight. (2018) 3(23):e124507. doi: 10.1172/jci.insight.124507
169. Kwon ED, Drake CG, Scher HI, Fizazi K, Bossi A, van den Eertwegh AJM, et al. Ipilimumab versus placebo after radiotherapy in patients with metastatic castration-resistant prostate cancer that had progressed after docetaxel chemotherapy (CA184-043): a multicentre, randomised, double-blind, phase 3 trial. Lancet Oncol. (2014) 15(7):700–12. doi: 10.1016/S1470-2045(14)70189-5
170. Tang C, Welsh JW, de Groot P, Massarelli E, Chang JY, Hess KR, et al. Ipilimumab with stereotactic ablative radiation therapy: phase I results and immunologic correlates from peripheral T cells. Clin Cancer Res. (2017) 23(6):1388–96. doi: 10.1158/1078-0432.CCR-16-1432
171. Skrepnik T, Sundararajan S, Cui H, Stea B. Improved time to disease progression in the brain in patients with melanoma brain metastases treated with concurrent delivery of radiosurgery and ipilimumab. Oncoimmunology. (2017) 6(3):e1283461. doi: 10.1080/2162402X.2017.1283461
172. Kiess AP, Wolchok JD, Barker CA, Postow MA, Tabar V, Huse JT, et al. Stereotactic radiosurgery for melanoma brain metastases in patients receiving ipilimumab: safety profile and efficacy of combined treatment. Int J Radiat Oncol Biol Phys. (2015) 92(2):368–75. doi: 10.1016/j.ijrobp.2015.01.004
173. Mathew M, Tam M, Ott PA, Pavlick AC, Rush SC, Donahue BR, et al. Ipilimumab in melanoma with limited brain metastases treated with stereotactic radiosurgery. Melanoma Res. (2013) 23(3):191–5. doi: 10.1097/CMR.0b013e32835f3d90
174. McBride S, Sherman E, Jillian Tsai C, Baxi S, Aghalar J, Eng J, et al. Randomized phase II trial of nivolumab with stereotactic body radiotherapy versus nivolumab alone in metastatic head and neck squamous cell carcinoma. J Clin Oncol. (2021) 39(1):30–7. doi: 10.1200/JCO.20.00290
175. Bahig H, Aubin F, Stagg J, Gologan O, Ballivy O, Bissada E, et al. Phase I/II trial of Durvalumab plus Tremelimumab and stereotactic body radiotherapy for metastatic head and neck carcinoma. BMC Cancer. (2019) 19(1):68. doi: 10.1186/s12885-019-5266-4
176. Schoenfeld JD, Hanna GJ, Jo VY, Rawal B, Chen YH, Catalano PS, et al. Neoadjuvant nivolumab or nivolumab plus ipilimumab in untreated oral cavity squamous cell carcinoma: a phase 2 open-label randomized clinical trial. JAMA Oncol. (2020) 6(10):1563–70. doi: 10.1001/jamaoncol.2020.2955
177. Vos JL, Elbers JBW, Krijgsman O, Traets JJH, Qiao X, van der Leun AM, et al. Neoadjuvant immunotherapy with nivolumab and ipilimumab induces major pathological responses in patients with head and neck squamous cell carcinoma. Nat Commun. (2021) 12:7348. doi: 10.1038/S41467-021-26472-9
178. Uppaluri R, Campbell KM, Egloff AM, Zolkind P, Skidmore ZL, Nussenbaum B, et al. Neoadjuvant and adjuvant pembrolizumab in resectable locally advanced, human papillomavirus–unrelated head and neck cancer: a multicenter, phase II trial. Clin Cancer Res. (2020) 26(19):5140–52. doi: 10.1158/1078-0432.CCR-20-1695
179. Seiwert TY, Burtness B, Mehra R, Weiss J, Berger R, Eder JP, et al. Safety and clinical activity of pembrolizumab for treatment of recurrent or metastatic squamous cell carcinoma of the head and neck (KEYNOTE-012): an open-label, multicentre, phase 1b trial. Lancet Oncol. (2016) 17(7):956–65. doi: 10.1016/S1470-2045(16)30066-3
180. Kumagai S, Togashi Y, Kamada T, Sugiyama E, Nishinakamura H, Takeuchi Y, et al. The PD-1 expression balance between effector and regulatory T cells predicts the clinical efficacy of PD-1 blockade therapies. Nat Immunol. (2020) 21(11):1346–58. doi: 10.1038/s41590-020-0769-3
181. Duhen R, Ballesteros-Merino C, Frye AK, Tran E, Rajamanickam V, Chang SC, et al. Neoadjuvant anti-OX40 (MEDI6469) therapy in patients with head and neck squamous cell carcinoma activates and expands antigen-specific tumor-infiltrating T cells. Nat Commun. (2021) 12:1047. doi: 10.1038/s41467-021-21383-1
182. Oweida AJ, Darragh L, Phan A, Binder D, Bhatia S, Mueller A, et al. STAT3 modulation of regulatory T cells in response to radiation therapy in head and neck cancer. J Natl Cancer Inst. (2019) 111(12):1339. doi: 10.1093/jnci/djz036
183. Payne R, Glenn L, Hoen H, Richards B, Smith JW 2nd, Lufkin R, et al. Durable responses and reversible toxicity of high-dose interleukin-2 treatment of melanoma and renal cancer in a community hospital biotherapy program. J Immunother Cancer. (2014) 2:13. doi: 10.1186/2051-1426-2-13
184. Kaufman HL, Kirkwood JM, Hodi FS, Agarwala S, Amatruda T, Bines SD, et al. The society for immunotherapy of cancer consensus statement on tumour immunotherapy for the treatment of cutaneous melanoma. Nat Rev Clin Oncol. (2013) 10(10):588–98. doi: 10.1038/nrclinonc.2013.153
185. Motzer RJ, Agarwal N, Beard C, Bolger GB, Boston B, Carducci MA, et al. NCCN Clinical practice guidelines in oncology: kidney cancer. J Natl Compr Canc Netw. (2009) 7(6):618–30. doi: 10.6004/jnccn.2009.0043
186. Alva A, Daniels GA, Wong MK, Kaufman HL, Morse MA, McDermott DF, et al. Contemporary experience with high-dose interleukin-2 therapy and impact on survival in patients with metastatic melanoma and metastatic renal cell carcinoma. Cancer Immunol Immunother. (2016) 65(12):1533–44. doi: 10.1007/s00262-016-1910-x
187. Walker JM, Rolig AS, Charych DH, Hoch U, Kasiewicz MJ, Rose DC, et al. NKTR-214 immunotherapy synergizes with radiotherapy to stimulate systemic CD8(+) T cell responses capable of curing multi-focal cancer. J Immunother Cancer. (2020) 8(1):e000464. doi: 10.1136/jitc-2019-000464
188. Rolig AS, Rose DC, McGee GH, Rubas W, Kivimae S, Redmond WL. Combining bempegaldesleukin (CD122-preferential IL-2 pathway agonist) and NKTR-262 (TLR7/8 agonist) improves systemic antitumor CD8(+) T cell cytotoxicity over BEMPEG+RT. J Immunother Cancer. (2022) 10:e004218. doi: 10.1136/jitc-2021-004218
189. Grob JJ, Dreno B, de la Salmoniere P, Delaunay M, Cupissol D, Guillot B, et al. Randomised trial of interferon alpha-2a as adjuvant therapy in resected primary melanoma thicker than 1.5 mm without clinically detectable node metastases. French cooperative group on melanoma. Lancet. (1998) 351(9120):1905–10. doi: 10.1016/S0140-6736(97)12445-X
190. Eggermont AM, Suciu S, MacKie R, Ruka W, Testori A, Kruit W, et al. Post-surgery adjuvant therapy with intermediate doses of interferon alfa 2b versus observation in patients with stage IIb/III melanoma (EORTC 18952): randomised controlled trial. Lancet. (2005) 366(9492):1189–96. doi: 10.1016/S0140-6736(05)67482-X
191. Wheatley K, Ives N, Hancock B, Gore M, Eggermont A, Suciu S. Does adjuvant interferon-alpha for high-risk melanoma provide a worthwhile benefit? A meta-analysis of the randomised trials. Cancer Treat Rev. (2003) 29(4):241–52. doi: 10.1016/S0305-7372(03)00074-4
192. Zhu Y, Tibensky I, Schmidt J, Ryschich E, Marten A. Interferon-alpha enhances antitumor effect of chemotherapy in an orthotopic mouse model for pancreatic adenocarcinoma. J Immunother. (2008) 31(7):599–606. doi: 10.1097/CJI.0b013e3181818769
193. Zhu Y, Tibensky I, Schmidt J, Hackert T, Ryschich E, Jager D, et al. Interferon-alpha in combination with chemotherapy has potent antiangiogenic properties in an orthotopic mouse model for pancreatic adenocarcinoma. J Immunother. (2008) 31(1):28–33. doi: 10.1097/CJI.0b013e318157c682
194. Linehan DC, Tan MC, Strasberg SM, Drebin JA, Hawkins WG, Picus J, et al. Adjuvant interferon-based chemoradiation followed by gemcitabine for resected pancreatic adenocarcinoma: a single-institution phase II study. Ann Surg. (2008) 248(2):145–51. doi: 10.1097/SLA.0b013e318181e4e9
195. Picozzi VJ, Abrams RA, Decker PA, Traverso W, O’Reilly EM, Greeno E, et al. Multicenter phase II trial of adjuvant therapy for resected pancreatic cancer using cisplatin, 5-fluorouracil, and interferon-alfa-2b-based chemoradiation: ACOSOG trial Z05031. Ann Oncol. (2011) 22(2):348–54. doi: 10.1093/annonc/mdq384
196. Schmidt J, Abel U, Debus J, Harig S, Hoffmann K, Herrmann T, et al. Open-label, multicenter, randomized phase III trial of adjuvant chemoradiation plus interferon Alfa-2b versus fluorouracil and folinic acid for patients with resected pancreatic adenocarcinoma. J Clin Oncol. (2012) 30(33):4077–83. doi: 10.1200/JCO.2011.38.2960
197. Spaapen RM, Leung MY, Fuertes MB, Kline JP, Zhang L, Zheng Y, et al. Therapeutic activity of high-dose intratumoral IFN-beta requires direct effect on the tumor vasculature. J Immunol. (2014) 193(8):4254–60. doi: 10.4049/jimmunol.1401109
198. Xuan C, Steward KK, Timmerman JM, Morrison SL. Targeted delivery of interferon-alpha via fusion to anti-CD20 results in potent antitumor activity against B-cell lymphoma. Blood. (2010) 115(14):2864–71. doi: 10.1182/blood-2009-10-250555
199. Yang X, Zhang X, Fu ML, Weichselbaum RR, Gajewski TF, Guo Y, et al. Targeting the tumor microenvironment with interferon-β bridges innate and adaptive immune responses. Cancer Cell. (2014) 25(1):37–48. doi: 10.1016/j.ccr.2013.12.004
200. Mason KA, Ariga H, Neal R, Valdecanas D, Hunter N, Krieg AM, et al. Targeting toll-like receptor 9 with CpG oligodeoxynucleotides enhances tumor response to fractionated radiotherapy. Clin Cancer Res. (2005) 11(1):361–9. doi: 10.1158/1078-0432.361.11.1
201. Mason KA, Neal R, Hunter N, Ariga H, Ang K, Milas L. CpG oligodeoxynucleotides are potent enhancers of radio- and chemoresponses of murine tumors. Radiother Oncol. (2006) 80(2):192–8. doi: 10.1016/j.radonc.2006.07.024
202. Deng L, Liang H, Xu M, Yang X, Burnette B, Arina A, et al. STING-dependent cytosolic DNA sensing promotes radiation-induced type I interferon-dependent antitumor immunity in immunogenic tumors. Immunity. (2014) 41(5):843–52. doi: 10.1016/j.immuni.2014.10.019
203. Baird JR, Friedman D, Cottam B, Dubensky TW, Kanne DB, Bambina S, et al. Radiotherapy combined with novel STING-targeting oligonucleotides results in regression of established tumors. Cancer Res. (2016) 76(1):50–61. doi: 10.1158/0008-5472.CAN-14-3619
204. Gough MJ, Baird JR, Bell RB. Implantable biomaterials to provide local immunotherapy following surgical resection. Oncotarget. (2018) 9(102):37612–3. doi: 10.18632/oncotarget.26487
205. Baird JR, Bell RB, Troesch V, Friedman D, Bambina S, Kramer G, et al. Evaluation of explant responses to STING ligands: personalized immunosurgical therapy for head and neck squamous cell carcinoma. Cancer Res. (2018) 78(21):6308–19. doi: 10.1158/0008-5472.CAN-18-1652
206. Garland KM, Sheehy TL, Wilson JT. Chemical and biomolecular strategies for STING pathway activation in cancer immunotherapy. Chem Rev. (2022) 122(6):5977–6039. doi: 10.1021/acs.chemrev.1c00750
207. Meric-Bernstam F, Sweis RF, Hodi FS, Messersmith WA, Andtbacka RHI, Ingham M, et al. Phase I dose-escalation trial of MIW815 (ADU-S100), an intratumoral STING agonist, in patients with advanced/metastatic solid tumors or lymphomas. Clin Cancer Res. (2022) 28(4):677–88. doi: 10.1158/1078-0432.CCR-21-1963
208. Meric-Bernstam F, Sweis RF, Kasper S, Hamid O, Bhatia S, Dummer R, et al. Combination of the STING agonist MIW815 (ADU-S100) and PD-1 inhibitor spartalizumab in advanced/metastatic solid tumors or lymphomas: an open-label, multicenter, phase ib study. Clin Cancer Res. (2023) 29(1):110–21. doi: 10.1158/1078-0432.CCR-22-2235
209. Gough MJ, Melcher AA, Crittenden MR, Sanchez-Perez L, Voellmy R, Vile RG. Induction of cell stress through gene transfer of an engineered heat shock transcription factor enhances tumor immunogenicity. Gene Ther. (2004) 11(13):1099–104. doi: 10.1038/sj.gt.3302274
210. Daniels GA, Sanchez-Perez L, Diaz RM, Kottke T, Thompson J, Lai M, et al. A simple method to cure established tumors by inflammatory killing of normal cells. Nat Biotechnol. (2004) 22(9):1125–32. doi: 10.1038/nbt1007
211. Todryk S, Melcher AA, Hardwick N, Linardakis E, Bateman A, Colombo MP, et al. Heat shock protein 70 induced during tumor cell killing induces Th1 cytokines and targets immature dendritic cell precursors to enhance antigen uptake. J Immunol. (1999) 163(3):1398–408. doi: 10.4049/jimmunol.163.3.1398
212. Apetoh L, Ghiringhelli F, Tesniere A, Criollo A, Ortiz C, Lidereau R, et al. The interaction between HMGB1 and TLR4 dictates the outcome of anticancer chemotherapy and radiotherapy. Immunol Rev. (2007) 220:47–59. doi: 10.1111/j.1600-065X.2007.00573.x
213. Telusma G, Datta S, Mihajlov I, Ma W, Li J, Yang H, et al. Dendritic cell activating peptides induce distinct cytokine profiles. Int Immunol. (2006) 18(11):1563–73. doi: 10.1093/intimm/dxl089
214. Obeid M, Panaretakis T, Tesniere A, Joza N, Tufi R, Apetoh L, et al. Leveraging the immune system during chemotherapy: moving calreticulin to the cell surface converts apoptotic death from “silent” to immunogenic. Cancer Res. (2007) 67(17):7941–4. doi: 10.1158/0008-5472.CAN-07-1622
215. Crittenden MR, Cottam B, Savage T, Nguyen C, Newell P, Gough MJ. Expression of NF-kappaB p50 in tumor stroma limits the control of tumors by radiation therapy. PLoS One. (2012) 7(6):e39295. doi: 10.1371/journal.pone.0039295
216. Mantovani A, Sozzani S, Locati M, Allavena P, Sica A. Macrophage polarization: tumor-associated macrophages as a paradigm for polarized M2 mononuclear phagocytes. Trends Immunol. (2002) 23:549–55. doi: 10.1016/S1471-4906(02)02302-5
217. Saccani A, Schioppa T, Porta C, Biswas SK, Nebuloni M, Vago L, et al. P50 nuclear factor-kappaB overexpression in tumor-associated macrophages inhibits M1 inflammatory responses and antitumor resistance. Cancer Res. (2006) 66(23):11432–40. doi: 10.1158/0008-5472.CAN-06-1867
218. Huynh ML, Fadok VA, Henson PM. Phosphatidylserine-dependent ingestion of apoptotic cells promotes TGF-beta1 secretion and the resolution of inflammation. J Clin Invest. (2002) 109(1):41–50. doi: 10.1172/JCI0211638
219. Fadok VA, Bratton DL, Konowal A, Freed PW, Westcott JY, Henson PM. Macrophages that have ingested apoptotic cells in vitro inhibit proinflammatory cytokine production through autocrine/paracrine mechanisms involving TGF-beta, PGE2, and PAF. J Clin Invest. (1998) 101(4):890–8. doi: 10.1172/JCI1112
220. Xu J, Escamilla J, Mok S, David J, Priceman S, West B, et al. CSF1R Signaling blockade stanches tumor-infiltrating myeloid cells and improves the efficacy of radiotherapy in prostate cancer. Cancer Res. (2013) 73(9):2782–94. doi: 10.1158/0008-5472.CAN-12-3981
221. Shiao SL, Ruffell B, DeNardo DG, Faddegon BA, Park CC, Coussens LM. TH2-polarized CD4(+) T cells and macrophages limit efficacy of radiotherapy. Cancer Immunol Res. (2015) 3(5):518–25. doi: 10.1158/2326-6066.CIR-14-0232
222. He J, Yin Y, Luster TA, Watkins L, Thorpe PE. Antiphosphatidylserine antibody combined with irradiation damages tumor blood vessels and induces tumor immunity in a rat model of glioblastoma. Clin Cancer Res. (2009) 15(22):6871–80. doi: 10.1158/1078-0432.CCR-09-1499
223. Jinushi M, Sato M, Kanamoto A, Itoh A, Nagai S, Koyasu S, et al. Milk fat globule epidermal growth factor-8 blockade triggers tumor destruction through coordinated cell-autonomous and immune-mediated mechanisms. J Exp Med. (2009) 206(6):1317–26. doi: 10.1084/jem.20082614
224. Tormoen GW, Blair TC, Bambina S, Kramer G, Baird J, Rahmani R, et al. Targeting MerTK enhances adaptive immune responses after radiation therapy. Int J Radiat Oncol Biol Phys. (2020) 108(1):93–103. doi: 10.1016/j.ijrobp.2020.04.013
225. Crittenden MR, Baird J, Friedman D, Savage T, Uhde L, Alice A, et al. Mertk on tumor macrophages is a therapeutic target to prevent tumor recurrence following radiation therapy. Oncotarget. (2016) 7(48):78653–66. doi: 10.18632/oncotarget.11823
226. Hafizi S, Dahlback B. Gas6 and protein S. Vitamin K-dependent ligands for the Axl receptor tyrosine kinase subfamily. FEBS J. (2006) 273(23):5231–44. doi: 10.1111/j.1742-4658.2006.05529.x
227. Wu Y, Singh S, Georgescu MM, Birge RB. A role for Mer tyrosine kinase in alphavbeta5 integrin-mediated phagocytosis of apoptotic cells. J Cell Sci. (2005) 118(Pt 3):539–53. doi: 10.1242/jcs.01632
228. Galvan MD, Foreman DB, Zeng E, Tan JC, Bohlson SS. Complement component C1q regulates macrophage expression of Mer tyrosine kinase to promote clearance of apoptotic cells. J Immunol. (2012) 188(8):3716–23. doi: 10.4049/jimmunol.1102920
229. Caetano MS, Younes AI, Barsoumian HB, Quigley M, Menon H, Gao C, et al. Triple therapy with MerTK and PD1 inhibition plus radiotherapy promotes abscopal antitumor immune responses. Clin Cancer Res. (2019) 25(24):7576–84. doi: 10.1158/1078-0432.CCR-19-0795
230. Barclay AN, Van den Berg TK. The interaction between signal regulatory protein alpha (SIRPalpha) and CD47: structure, function, and therapeutic target. Annu Rev Immunol. (2014) 32:25–50. doi: 10.1146/annurev-immunol-032713-120142
231. Oldenborg PA, Zheleznyak A, Fang YF, Lagenaur CF, Gresham HD, Lindberg FP. Role of CD47 as a marker of self on red blood cells. Science (1979). (2000) 288(5473):2051–4. doi: 10.1126/science.288.5473.2051
232. Majeti R, Chao MP, Alizadeh AA, Pang WW, Jaiswal S, Gibbs KD Jr, et al. CD47 Is an adverse prognostic factor and therapeutic antibody target on human acute myeloid leukemia stem cells. Cell. (2009) 138(2):286–99. doi: 10.1016/j.cell.2009.05.045
233. Sikic BI, Lakhani N, Patnaik A, Shah SA, Chandana SR, Rasco D, et al. First-in-human, first-in-class phase I trial of the anti-CD47 antibody Hu5F9-G4 in patients with advanced cancers. J Clin Oncol. (2019) 37(12):946–53. doi: 10.1200/JCO.18.02018
234. Hsieh RC, Krishnan S, Wu RC, Boda AR, Liu A, Winkler M, et al. ATR-mediated CD47 and PD-L1 up-regulation restricts radiotherapy-induced immune priming and abscopal responses in colorectal cancer. Sci Immunol. (2022) 7(72):eabl9330. doi: 10.1126/sciimmunol.abl9330
235. Marzo AL, Vezys V, Klonowski KD, Lee SJ, Muralimohan G, Moore M, et al. Fully functional memory CD8 T cells in the absence of CD4 T cells. J Immunol. (2004) 173(2):969–75. doi: 10.4049/jimmunol.173.2.969
236. Bennett SR, Carbone FR, Karamalis F, Miller JF, Heath WR. Induction of a CD8+ cytotoxic T lymphocyte response by cross-priming requires cognate CD4+ T cell help. J Exp Med. (1997) 186(1):65–70. doi: 10.1084/jem.186.1.65
237. Edwards LE, Haluszczak C, Kedl RM. Phenotype and function of protective, CD4-independent CD8 T cell memory. Immunol Res. (2013) 55(1–3):135–45. doi: 10.1007/s12026-012-8356-9
238. Aguilera TA, Elghonaimy EA, Shehade H, Rafat M, Castellini L, Jiang D, et al. Induced tumor heterogeneity reveals factors informing radiation and immunotherapy combinations. Clin Cancer Res. (2020) 26(12):2972–85. doi: 10.1158/1078-0432.CCR-19-4220
239. Rech AJ, Dada H, Kotzin JJ, Henao-Mejia J, Minn AJ, Twyman-Saint Victor C, et al. Radiotherapy and CD40 activation separately augment immunity to checkpoint blockade in cancer. Cancer Res. (2018) 78(15):4282–91. doi: 10.1158/0008-5472.CAN-17-3821
240. Dovedi SJ, Lipowska-Bhalla G, Beers SA, Cheadle EJ, Mu L, Glennie MJ, et al. Antitumor efficacy of radiation plus immunotherapy depends upon dendritic cell activation of effector CD8+ T cells. Cancer Immunol Res. (2016) 4(7):621–30. doi: 10.1158/2326-6066.CIR-15-0253
241. Vonderheide RH, Flaherty KT, Khalil M, Stumacher MS, Bajor DL, Hutnick NA, et al. Clinical activity and immune modulation in cancer patients treated with CP-870,893, a novel CD40 agonist monoclonal antibody. J Clin Oncol. (2007) 25(7):876–83. doi: 10.1200/JCO.2006.08.3311
242. Fransen MF, Sluijter M, Morreau H, Arens R, Melief CJ. Local activation of CD8 T cells and systemic tumor eradication without toxicity via slow release and local delivery of agonistic CD40 antibody. Clin Cancer Res. (2011) 17(8):2270–80. doi: 10.1158/1078-0432.CCR-10-2888
243. Liu HC, Viswanath DI, Pesaresi F, Xu Y, Zhang L, Di Trani N, et al. Potentiating antitumor efficacy through radiation and sustained intratumoral delivery of anti-CD40 and anti-PDL1. Int J Radiat Oncol Biol Phys. (2021) 110(2):492–506. doi: 10.1016/j.ijrobp.2020.07.2326
244. Yasmin-Karim S, Bruck PT, Moreau M, Kunjachan S, Chen GZ, Kumar R, et al. Radiation and local anti-CD40 generate an effective in situ vaccine in preclinical models of pancreatic cancer. Front Immunol. (2018) 9:2030. doi: 10.3389/fimmu.2018.02030
245. Labiano S, Roh V, Godfroid C, Hiou-Feige A, Romero J, Sum E, et al. CD40 agonist targeted to fibroblast activation protein alpha synergizes with radiotherapy in murine HPV-positive head and neck tumors. Clin Cancer Res. (2021) 27(14):4054–65. doi: 10.1158/1078-0432.CCR-20-4717
246. Morrison AH, Diamond MS, Hay CA, Byrne KT, Vonderheide RH. Sufficiency of CD40 activation and immune checkpoint blockade for T cell priming and tumor immunity. Proc Natl Acad Sci U S A. (2020) 117(14):8022–31. doi: 10.1073/pnas.1918971117
247. Hanahan D, Weinberg RA. Hallmarks of cancer: the next generation. Cell. (2011) 144(5):646–74. doi: 10.1016/j.cell.2011.02.013
248. Hayashi K, Nikolos F, Lee YC, Jain A, Tsouko E, Gao H, et al. Tipping the immunostimulatory and inhibitory DAMP balance to harness immunogenic cell death. Nat Commun. (2020) 11(1):6299. doi: 10.1038/s41467-020-19970-9
249. Hangai S, Ao T, Kimura Y, Matsuki K, Kawamura T, Negishi H, et al. PGE2 Induced in and released by dying cells functions as an inhibitory DAMP. Proc Natl Acad Sci U S A. (2016) 113(14):3844–9. doi: 10.1073/pnas.1602023113
250. Bottcher JP, Bonavita E, Chakravarty P, Blees H, Cabeza-Cabrerizo M, Sammicheli S, et al. NK cells stimulate recruitment of cDC1 into the tumor microenvironment promoting cancer immune control. Cell. (2018) 172(5):1022–37.e14. doi: 10.1016/j.cell.2018.01.004
251. Huang Q, Li F, Liu X, Li W, Shi W, Liu FF, et al. Caspase 3-mediated stimulation of tumor cell repopulation during cancer radiotherapy. Nat Med. (2011) 17(7):860–6. doi: 10.1038/nm.2385
252. Monjazeb AM, Kent MS, Grossenbacher SK, Mall C, Zamora AE, Mirsoian A, et al. Blocking indolamine-2,3-dioxygenase rebound immune suppression boosts antitumor effects of radio-immunotherapy in murine models and spontaneous canine malignancies. Clin Cancer Res. (2016) 22(17):4328–40. doi: 10.1158/1078-0432.CCR-15-3026
253. Lemos H, Mohamed E, Huang L, Ou R, Pacholczyk G, Arbab AS, et al. STING promotes the growth of tumors characterized by low antigenicity via IDO activation. Cancer Res. (2016) 76(8):2076–81. doi: 10.1158/0008-5472.CAN-15-1456
254. Munn DH, Mellor AL. IDO in the tumor microenvironment: inflammation, counter-regulation, and tolerance. Trends Immunol. (2016) 37(3):193–207. doi: 10.1016/j.it.2016.01.002
255. Mitra D, Horick NK, Brackett DG, Mouw KW, Hornick JL, Ferrone S, et al. High IDO1 expression is associated with poor outcome in patients with anal cancer treated with definitive chemoradiotherapy. Oncologist. (2019) 24(6):e275–83. doi: 10.1634/theoncologist.2018-0794
256. Crittenden MR, Savage T, Cottam B, Baird J, Rodriguez PC, Newell P, et al. Expression of arginase I in myeloid cells limits control of residual disease after radiation therapy of tumors in mice. Radiat Res. (2014) 182(2):182–90. doi: 10.1667/RR13493.1
257. Vigano S, Alatzoglou D, Irving M, Ménétrier-Caux C, Caux C, Romero P, et al. Targeting adenosine in cancer immunotherapy to enhance T-cell function. Front Immunol. (2019) 10:925. doi: 10.3389/fimmu.2019.00925
258. Zhao H, Bo C, Kang Y, Li H. What else can CD39 tell US? Front Immunol. (2017) 8:727. doi: 10.3389/fimmu.2017.00727
259. Da M, Chen L, Enk A, Ring S, Mahnke K. The multifaceted actions of CD73 during development and suppressive actions of regulatory T cells. Front Immunol. (2022) 13:914799. doi: 10.3389/fimmu.2022.914799
260. Novitskiy SV, Ryzhov S, Zaynagetdinov R, Goldstein AE, Huang Y, Tikhomirov OY, et al. Adenosine receptors in regulation of dendritic cell differentiation and function. Blood. (2008) 112(5):1822–31. doi: 10.1182/blood-2008-02-136325
261. Ohta A, Gorelik E, Prasad SJ, Ronchese F, Lukashev D, Wong MK, et al. A2A adenosine receptor protects tumors from antitumor T cells. Proc Natl Acad Sci U S A. (2006) 103(35):13132–7. doi: 10.1073/pnas.0605251103
262. Zarek PE, Huang CT, Lutz ER, Kowalski J, Horton MR, Linden J, et al. A2A receptor signaling promotes peripheral tolerance by inducing T-cell anergy and the generation of adaptive regulatory T cells. Blood. (2008) 111(1):251–9. doi: 10.1182/blood-2007-03-081646
263. Canale FP, Ramello MC, Nunez N, Araujo Furlan CL, Bossio SN, Gorosito Serran M, et al. CD39 expression defines cell exhaustion in tumor-infiltrating CD8(+) T cells. Cancer Res. (2018) 78(1):115–28. doi: 10.1158/0008-5472.CAN-16-2684
264. Loi S, Pommey S, Haibe-Kains B, Beavis PA, Darcy PK, Smyth MJ, et al. CD73 promotes anthracycline resistance and poor prognosis in triple negative breast cancer. Proc Natl Acad Sci U S A. (2013) 110(27):11091–6. doi: 10.1073/pnas.1222251110
265. Jiang T, Xu X, Qiao M, Li X, Zhao C, Zhou F, et al. Comprehensive evaluation of NT5E/CD73 expression and its prognostic significance in distinct types of cancers. BMC Cancer. (2018) 18(1):267. doi: 10.1186/s12885-018-4073-7
266. Buisseret L, Pommey S, Allard B, Garaud S, Bergeron M, Cousineau I, et al. Clinical significance of CD73 in triple-negative breast cancer: multiplex analysis of a phase III clinical trial. Ann Oncol. (2018) 29(4):1056–62. doi: 10.1093/annonc/mdx730
267. Antonioli L, Pacher P, Vizi ES, Hasko G. CD39 and CD73 in immunity and inflammation. Trends Mol Med. (2013) 19(6):355–67. doi: 10.1016/j.molmed.2013.03.005
268. de Leve S, Wirsdorfer F, Jendrossek V. Targeting the immunomodulatory CD73/adenosine system to improve the therapeutic gain of radiotherapy. Front Immunol. (2019) 10(698):698. doi: 10.3389/fimmu.2019.00698
269. Wennerberg E, Spada S, Rudqvist NP, Lhuillier C, Gruber S, Chen Q, et al. CD73 blockade promotes dendritic cell infiltration of irradiated tumors and tumor rejection. Cancer Immunol Res. (2020) 8(4):465–78. doi: 10.1158/2326-6066.CIR-19-0449
270. Waickman AT, Alme A, Senaldi L, Zarek PE, Horton M, Powell JD. Enhancement of tumor immunotherapy by deletion of the A2A adenosine receptor. Cancer Immunol Immunother. (2012) 61(6):917–26. doi: 10.1007/s00262-011-1155-7
271. Huang J, Zhang D, Bai Y, Yang P, Xing L, Yu J. A(2A)R antagonism with DZD2269 augments antitumor efficacy of irradiation in murine model. J Cancer. (2020) 11(12):3685–92. doi: 10.7150/jca.43966
272. Vaupel P, Multhoff G. Adenosine can thwart antitumor immune responses elicited by radiotherapy: therapeutic strategies alleviating protumor ADO activities. Strahlenther Onkol. (2016) 192(5):279–87. doi: 10.1007/s00066-016-0948-1
273. Augustin RC, Leone RD, Naing A, Fong L, Bao R, Luke JJ. Next steps for clinical translation of adenosine pathway inhibition in cancer immunotherapy. J Immunother Cancer. (2022) 10(2):e004089. doi: 10.1136/jitc-2021-004089
274. Bossi P, Platini F. Radiotherapy plus EGFR inhibitors: synergistic modalities. Cancers of the Head & Neck. (2017) 2(1):1–9. doi: 10.1186/s41199-016-0020-y
275. Ang K, Berkey B, Tu X, Zhang H, Katz R, Hammond E, et al. Impact of epidermal growth factor receptor expression on survival and pattern of relapse in patients with advanced head and neck carcinoma. Cancer Res. (2002) 62(24):7350–6.12499279
276. Bonner JA, Trummell HQ, Bonner AB, Willey CD, Bredel M, Yang ES. Enhancement of cetuximab-induced radiosensitization by JAK-1 inhibition. BMC Cancer. (2015) 15(1):1–12. doi: 10.1186/s12885-015-1679-x
277. Bowles DW, Keysar SB, Eagles JR, Wang G, Glogowska MJ, McDermott JD, et al. A pilot study of cetuximab and the hedgehog inhibitor IPI-926 in recurrent/metastatic head and neck squamous cell carcinoma. Oral Oncol. (2016) 53:74–9. doi: 10.1016/j.oraloncology.2015.11.014
278. Jubran MR, Vilenski D, Flashner-Abramson E, Shnaider E, Vasudevan S, Rubinstein AM, et al. Overcoming resistance to EGFR monotherapy in HNSCC by identification and inhibition of individualized cancer processes. Theranostics. (2022) 12(3):1204–19. doi: 10.7150/thno.64347
279. Strobl MAR, Gallaher J, West J, Robertson-Tessi M, Maini PK, Anderson ARA. Spatial structure impacts adaptive therapy by shaping intra-tumoral competition. Commun Med. (2022) 2(1):1–18. doi: 10.1038/s43856-021-00067-3
280. Bai Z, Su G, Fan R. Single-cell analysis technologies for immuno-oncology research: from mechanistic delineation to biomarker discovery. Genomics Proteomics Bioinformatics. (2021) 19(2):191–207. doi: 10.1016/j.gpb.2021.02.004
281. Gallaher JA, Enriquez-Navas PM, Luddy KA, Gatenby RA, Anderson ARA. Spatial heterogeneity and evolutionary dynamics modulate time to recurrence in continuous and adaptive cancer therapies. Cancer Res. (2018) 78(8):2127–39. doi: 10.1158/0008-5472.CAN-17-2649
282. Vitale I, Shema E, Loi S, Galluzzi L. Intratumoral heterogeneity in cancer progression and response to immunotherapy. Nat Med. (2021) 27(2):212–24. doi: 10.1038/s41591-021-01233-9
283. Puram SV, Tirosh I, Parikh AS, Patel AP, Yizhak K, Gillespie S, et al. Single-cell transcriptomic analysis of primary and metastatic tumor ecosystems in head and neck cancer. Cell. (2017) 171(7):1611–1624.e24. doi: 10.1016/j.cell.2017.10.044
284. De Las Rivas J, Brozovic A, Izraely S, Casas-Pais A, Witz IP, Figueroa A. Cancer drug resistance induced by EMT: novel therapeutic strategies. Arch Toxicol. (2021) 95(7):2279–97. doi: 10.1007/s00204-021-03063-7
285. Laoui D, Van Overmeire E, Conza G, Aldeni C, Keirsse J, Morias Y, et al. Tumor hypoxia does not drive differentiation of tumor-associated macrophages but rather fine-tunes the M2-like macrophage population. Cancer Res. (2014) 74(1):24–30. doi: 10.1158/0008-5472.CAN-13-1196
286. Van den bossche V, Zaryouh H, Vara-Messler M, Vignau J, Machiels JP, Wouters A, et al. Microenvironment-driven intratumoral heterogeneity in head and neck cancers: clinical challenges and opportunities for precision medicine. Drug Resist Updates. (2022) 60:100806. doi: 10.1016/j.drup.2022.100806
287. Alkhatib H, Rubinstein AM, Vasudevan S, Flashner-Abramson E, Stefansky S, Chowdhury SR, et al. Computational quantification and characterization of independently evolving cellular subpopulations within tumors is critical to inhibit anti-cancer therapy resistance. Genome Med. (2022) 14(1):1–17. doi: 10.1186/s13073-022-01121-y
288. López-Cortés XA, Matamala F, Venegas B, Rivera C. Machine-learning applications in oral cancer: a systematic review. Appl Scie. (2022) 12(11):5715. doi: 10.3390/app12115715
289. Adeoye J, Tan JY, Choi SW, Thomson P. Prediction models applying machine learning to oral cavity cancer outcomes: a systematic review. Int J Med Inform. (2021) 154:104557. doi: 10.1016/j.ijmedinf.2021.104557
290. Dong Z, Zhang N, Li C, Wang H, Fang Y, Wang J, et al. Anticancer drug sensitivity prediction in cell lines from baseline gene expression through recursive feature selection. BMC Cancer. (2015) 15(1):1–12. doi: 10.1186/1471-2407-15-1
291. Nidheesh N, Abdul Nazeer KA, Ameer PM. An enhanced deterministic K-means clustering algorithm for cancer subtype prediction from gene expression data. Comput Biol Med. (2017) 91:213–21. doi: 10.1016/j.compbiomed.2017.10.014
292. Siddalingappa R, Kanagaraj S. K-nearest-neighbor algorithm to predict the survival time and classification of various stages of oral cancer: a machine learning approach. F1000Res. (2022) 11:70. doi: 10.12688/f1000research.75469.1
293. Cohen S. The basics of machine learning: strategies and techniques. In: Cohen S, editor. Artificial intelligence and deep learning in pathology. Amsterdam, Netherlands: Elsevier (2021). p. 13–40.
294. Maity AK, Bhattacharya A, Mallick BK, Baladandayuthapani V. Bayesian data integration and variable selection for pan-cancer survival prediction using protein expression data. Biometrics. (2020) 76(1):316. doi: 10.1111/biom.13132
295. Jiang Y, Huang Y, Du Y, Zhao Y, Ren J, Ma S, et al. Identification of prognostic genes and pathways in lung adenocarcinoma using a Bayesian approach. Cancer Inform. (2020) 16:1176935116684825. doi: 10.1177/1176935116684825
296. Wang W, Baladandayuthapani V, Morris JS, Broom BM, Manyam G, Do KA. iBAG: integrative Bayesian analysis of high-dimensional multiplatform genomics data. Bioinformatics. (2013) 29(2):149–59. doi: 10.1093/bioinformatics/bts655
297. Toth R, Schiffmann H, Hube-Magg C, Büscheck F, Höflmayer D, Weidemann S, et al. Random forest-based modelling to detect biomarkers for prostate cancer progression. Clin Epigenetics. (2019) 11(1):1–15. doi: 10.1186/s13148-019-0736-8
298. Yang D, Wu Y, Wan Z, Xu Z, Li W, Yuan P, et al. HISMD: a novel immune subtyping system for HNSCC. J Dent Res. (2023) 102(3):270–9. doi: 10.1177/00220345221134605
299. Adam G, Rampášek L, Safikhani Z, Smirnov P, Haibe-Kains B, Goldenberg A. Machine learning approaches to drug response prediction: challenges and recent progress. npj Precis Oncol. (2020) 4(1):1–10. doi: 10.1038/s41698-020-0122-1
300. Rockne RC, Branciamore S, Qi J, Frankhouser DE, O’Meally D, Hua WK, et al. State-transition analysis of time-sequential gene expression identifies critical points that predict development of acute myeloid leukemia. Cancer Res. (2020) 80(15):3157–69. doi: 10.1158/0008-5472.CAN-20-0354
301. Vasudevan S, Flashner-Abramson E, Remacle F, Levine RD, Kravchenko-Balasha N. Personalized disease signatures through information-theoretic compaction of big cancer data. Proc Natl Acad Sci U S A. (2018) 115(30):7694–9. doi: 10.1073/pnas.1804214115
302. Shin YS, Remacle F, Fan R, Hwang K, Wei W, Ahmad H, et al. Protein signaling networks from single cell fluctuations and information theory profiling. Biophys J. (2011) 100(10):2378–86. doi: 10.1016/j.bpj.2011.04.025
303. Frankhouser DE, O’meally D, Branciamore S, Uechi L, Zhang L, Chen YC, et al. Dynamic patterns of microRNA expression during acute myeloid leukemia state-transition. Sci Adv. (2022) 8:1664. doi: 10.1126/sciadv.abj1664
304. Kravchenko-Balasha N, Shin YS, Sutherland A, Levine RD, Heath JR. Intercellular signaling through secreted proteins induces free-energy gradient-directed cell movement. Proc Natl Acad Sci USA. (2016) 113(20):5520–5. doi: 10.1073/pnas.1602171113
305. Remacle F, Kravchenko-Balasha N, Levitzki A, Levine RD. Information-theoretic analysis of phenotype changes in early stages of carcinogenesis. Proc Natl Acad Sci U S A. (2010) 107(22):10324–9. doi: 10.1073/pnas.1005283107
306. Zadran S, Remacle F, Levine RD. miRNA and mRNA cancer signatures determined by analysis of expression levels in large cohorts of patients. Proc Natl Acad Sci U S A. (2013) 110(47):19160–5. doi: 10.1073/pnas.1316991110
307. Vasudevan S, Flashner-Abramson E, Alkhatib H, Roy Chowdhury S, Adejumobi IA, Vilenski D, et al. Overcoming resistance to BRAFV600E inhibition in melanoma by deciphering and targeting personalized protein network alterations. NPJ Precis Oncol. (2021) 5:50. doi: 10.1038/s41698-021-00190-3
308. Sharon S, Duhen T, Bambina S, Baird J, Leidner R, Bell B, et al. Explant modeling of the immune environment of head and neck cancer. Front Oncol. (2021) 11:611365. doi: 10.3389/fonc.2021.611365
309. Flashner-Abramson E, Vasudevan S, Adejumobi IA, Sonnenblick A, Kravchenko-Balasha N. Decoding cancer heterogeneity: studying patient-specific signaling signatures towards personalized cancer therapy. Theranostics. (2019) 9(18):5149–65. doi: 10.7150/thno.31657
310. Tran KA, Kondrashova O, Bradley A, Williams ED, Pearson JV, Waddell N. Deep learning in cancer diagnosis, prognosis and treatment selection. Genome Med. (2021) 13(1):1–17. doi: 10.1186/s13073-020-00808-4
Keywords: head and neck cancer, immunotherapy, radiotherapy, SBRT, hypofractionated, targeted therapy, squamous cell carcinoma, HNSCC
Citation: Sharon S, Daher-Ghanem N, Zaid D, Gough MJ and Kravchenko-Balasha N (2023) The immunogenic radiation and new players in immunotherapy and targeted therapy for head and neck cancer. Front. Oral. Health 4:1180869. doi: 10.3389/froh.2023.1180869
Received: 6 March 2023; Accepted: 27 June 2023;
Published: 11 July 2023.
Edited by:
Liangliang Wang, The University of Chicago, United StatesReviewed by:
Fermín E. González, University of Chile, ChileRobert Saddawi-Konefka, University of California, San Diego, United States
© 2023 Sharon, Daher-Ghanem, Zaid, Gough and Kravchenko-Balasha. This is an open-access article distributed under the terms of the Creative Commons Attribution License (CC BY). The use, distribution or reproduction in other forums is permitted, provided the original author(s) and the copyright owner(s) are credited and that the original publication in this journal is cited, in accordance with accepted academic practice. No use, distribution or reproduction is permitted which does not comply with these terms.
*Correspondence: Shay Sharon c2hhcm9uc2hheUBnbWFpbC5jb20=
†These authors have contributed equally to this work and share last authorship