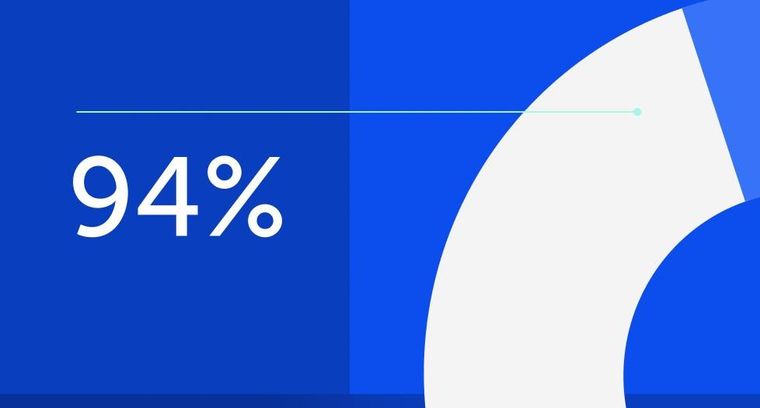
94% of researchers rate our articles as excellent or good
Learn more about the work of our research integrity team to safeguard the quality of each article we publish.
Find out more
MINI REVIEW article
Front. Oral. Health, 31 October 2022
Sec. Oral Infections and Microbes
Volume 3 - 2022 | https://doi.org/10.3389/froh.2022.1001790
Coronaviruses, including SARS-CoV-2, have caused pandemics in the past two decades. The most prevalent SARS-CoV-2 variants of concern can re-infect individuals who have been previously infected with other variants or had protection from vaccines targeting the original SARS-CoV-2 variant. Given the high risk of transmission of coronavirus via aerosols produced during dental procedures, it is important to understand the future risk of coronavirus infection for oral health professionals and to diagnose quickly early stages of outbreaks. Testing of saliva for coronavirus may be the least invasive and most convenient method for following the outbreak at the individual and community level. This review will describe strategies for diagnosis of coronavirus in saliva.
The Coronavirus disease 19 (COVID-19) pandemic, caused by infection with the Severe Acute Respiratory Syndrome Coronavirus 2 (SARS-CoV-2), has killed more than 6 million individuals globally as of July 2022 (1). The symptoms of infection range from asymptomatic; to coughs, fever, and fatigue in moderate disease; to severe pulmonary pathology requiring hospitalization and ventilators. Persons with underlying co-morbidities are at a higher risk for severe disease. Though the patients with mild to moderate disease recover quickly, some report post-COVID-19 symptoms months to years after infection, even in individuals who experienced mild symptoms. For those who suffered a severe infection, it is possible for lung and cardiac function to be impaired permanently leading, to an increased risk for other complications in the future (2).
The first modern coronavirus pandemic occurred in 2002 in China and the disease was named the Severe Acute Respiratory Syndrome (SARS). In 2012, a pandemic caused by another coronavirus emerged in the middle east and was named the Middle East Respiratory Syndrome (MERS-CoV).
SARS-CoV-2 is the fifth recent coronavirus to infect humans on a wide scale, and as the mutations continue to evolve rapidly, it is crucial to understand the mechanics of prevention, genomics, and pathogenesis of the new mutations (3). The virus responsible for this pandemic has a higher rate of transmissibility and infectivity compared to the previous pandemic coronaviruses, SARS-CoV and MERS-CoV (4).
SARS-CoV-2 is a single-strand positive-sense RNA virus. The SARS-CoV-2 spike (S) protein, the essential antigenic determinant of the virus (5), binds to its receptor angiotensin-converting enzyme 2 (ACE2), initiating viral entry to the host cell (6–8). The S protein consists of 1,273 amino acids and contains a signal peptide (amino acids 1–13), the S1 subunit (14–685) and the S2 subunit (686–1,273). The receptor-binding domain (RBD) in the S1 subunit recognizes ACE2, while S2 subunits play a role in membrane fusion (9). ACE2 is expressed by a variety of tissues, including pulmonary and extrapulmonary tissues (10). This accounts at least partially for extrapulmonary manifestations associated with COVID-19 (11). It has been proposed that the nasal cavity (12, 13) and oral cavity (14–17) are potential initial targets among the upper respiratory system.
SARS-CoV-2 utilizes a dual entry mechanism, cell surface and endosomal pathways, for its internalization (18–20). In the cell surface pathway, S protein can be proteolytically cleaved by host cell-derived transmembrane serine proteases (TMPRSSs). This results in the exposure of the fusion domain in the S2 subunit, which subsequently mediates viral—host cell membrane fusion (18). The clathrin-mediated endosomal pathway is carried out when the availability of TMPRSSs is limited (19). In this pathway, cathepsins play a major role in cleaving the S protein (20).
COVID-19 is primarily a disease of pulmonary tissues. However, many patients with COVID-19 also develop gastrointestinal (GI) symptoms. Given the high expression of ACE2 and the visualization of viral nucleocapsid in the GI tract (21), SARS-CoV-2 potentially enters GI cells via ACE2 and directly causes damage to the GI tract. In such a case, the oral cavity, in particular saliva (22), may be a potential reservoir of SARS-CoV-2 since the oral cavity and GI system are linked by constant flow of saliva. An inflammatory cytokine storm (23) and alteration of the GI microflora in response to viral infection (24, 25) have been considered as indirect factors that damage the GI tract.
SARS-CoV-2, like other respiratory viruses, can be transmitted via direct (physical) contact with infected individuals, indirect contact with fomites, and droplets and aerosols produced by coughing (26). In addition, the fecal-oral route has been suggested as a potential transmission route. This is based on the observation in the nonhuman primate model that intranasal or intragastric inoculation with SARS-CoV-2 resulted in both pulmonary and GI infections (27).
SARS-CoV-2 continuously evolves by genetic mutations in the S gene. A number of SARS-CoV-2 lineages have been identified, such as Alpha (B.1.1.7), Beta (B.1.351), Delta (B.1.617.2), and Omicron (B.1.1.529) (27–30). The most prevalent SARS-CoV-2 variants of concern are currently the BA.4 and BA.5 subvariants of the Omicron variant, which can re-infect individuals who have been previously infected with other variants or had protection from vaccines targeting the original SARS-CoV-2 variant (31, 32).
Host immunity against SARS-CoV-2 is activated once the virus gains access into the host cells. Type I interferons (IFNs) play a pivotal role in both innate (33) and adaptive (34) antiviral immunity. SARS-CoV-2 can evade host immune responses by inhibition of type I IFNs, and interfering with downstream signaling molecules of Toll-like receptors (TLRs) and the JAK-STAT pathway (35–39). It has been reported that the risk of death from COVID-19 is much greater in patients with type I IFN autoantibodies (40–42).
The S protein associated with the B.1.1.7 lineage reveals several amino acid mutations. However, among them, N501Y in the RBD region accounts for at least part of the enhanced binding affinity for ACE2, enhanced virulence, and increased transmissibility (30). The B.1.351 variant expresses amino acid mutations K417N, E484K, and N501Y in RBD. This variant is reported to enter host cells more easily due to its enhanced binding affinity for ACE2 (28). It is also known to reduce the efficacy of neutralizing antibodies and the original mRNA vaccines.
The B.1.617.2 variant, first identified in India, is one of three sublineages of B.1617 and became the most predominant variant globally in late 2020 by outcompeting pre-existing lineages, such as B.1.1.7 and B.1.617.1 (43). This lineage is characterized by higher transmissibility than the wild-type Wuhan-1 D614G-bearing lineages. It is also associated with a sixfold decrease in sensitivity to convalescent antibodies and eightfold decrease in sensitivity to vaccine-elicited antibodies than the ancestral strain (44). This is partially due to the mutations L452R and T478K in RBD that play a role in increased receptor-binding affinity, infectivity, and reduced sensitivity to vaccine-elicited antibodies. The E484Q mutation in RBD is reported to augment the receptor binding affinity and reduce sensitivity to antibody (45). In addition, the P681R mutation between the S1/S2 cleavage site contributes to enhanced transmissibility (29).
The B.1.1.529 lineage (Omicron) was originally detected in South Africa in November 2021 (46). This lineage is reported to express several mutations observed in other lineages. They include N501Y, E484K, T478K, and P681R, suggesting that this lineage is potentially highly infectious and transmissible and has the ability to evade detection by the host immune system. At the same time, while being highly transmissible, the B.1.1.529 lineage has a decreased ability to infiltrate the lungs, thus the observed reduced pathogenicity (47). Accumulating evidence has elucidated that the enhanced binding of the B.1.1.529 variant to host ACE2 accounts for the enhanced transmissibility of this lineage compared with other lineages. Nonetheless, B.1.1.529 entry into lung cells is compromised since the S protein of this lineage is not cleaved efficiently by TMPRSS2. This implies that the B.1.1.529 virus relies heavily on the endosomal pathway for internalization into the host cell. Collectively, B.1.1.529 has a decreased capacity to enter the host lung cells (i.e., it is less infectious), resulting in lower replication potential than other lineages.
The Centers for Disease Control (CDC) in the US has classified the variants into 3 main categories: Variant of Concern (VOC), Variant of Interest (VOI), and Variant Being Monitored (VBM). Thus, in November 2021, the Omicron variant was classified as a VOC (48, 49).
The variants have piqued the interest of researchers because of different molecular features that they exhibit in different infected populations, which in turn, has led to further studies on factors influencing the mutations of the virus. The findings might be valuable with respect to predicting vaccine efficacy against infection with future variants (50–54). One study retrospectively analyzed the emergence of the Omicron variant and determined that the epidemiology of Omicron infection was an accurate predictor of mutations in the virus, and the global trajectory was a better predictor than epidemiology at a country level. This type of understanding could help us to prepare for future pandemics (55).
Research done during earlier stages of this pandemic showed the virus's predilection for certain sites in the body, including the oral cavity, due to the presence of the ACE2 receptors in the epithelium of oral tissues. In fact, the oral cavity might be the initial site of entry for the coronavirus. The tongue, floor of the mouth, gingival sulcus, and gingival epithelium of the buccal and lingual surfaces of teeth all express ACE2 receptors (56) (Figure 1). Oral symptoms like loss of taste often precede other symptoms of the infection. Dry mouth, inflammation of gingival tissues and ulcerations have also been reported. Since a variety of viruses and other pathogens are usually present in the gingival sulcus, this site might act as a reservoir for SARS-CoV-2. The oral viral load has also been correlated with the severity of SARS-CoV-2 infection. Therefore, by reducing the viral load in the oral cavity, it may be possible to reduce the risk of transmission (14, 57, 58).
Figure 1. Reservoirs of SARS-CoV-2 in the head and neck regions, especially parts of the oral cavity: tongue, gingival sulci, hard palate, parotid glands, submandibular glands, sublingual glands, nasal cavity; nasopharynx, oropharynx, hypopharynx, esophagus, and trachea. Specimens for diagnostics could be derived from nasopharyngeal swabs or saliva.
IgA appears to be the first antibody detected in response to SARS-CoV-2 infection (59) and IgA dominates SARS-CoV-2-specific antibody responses over IgG and IgM during the early stages of infection (60). Virus-specific salivary IgA and IgG have been detected in saliva up to 73 days and 9 months, respectively (60, 61). Specific serum IgA decreases 1 month after the disease onset while serum IgG is detectable up to 9 months (60, 61). Taken together, the observations suggest that SARS-CoV-2 specific salivary IgA may be a potential biomarker during the initial stage of infection. Salivary IgG response may be useful to monitor the systemic immunity to SARS-CoV-2.
An accurate and early diagnosis of the oral manifestations of the infection (62) may also contribute to minimizing progression of infection to severe disease and prevent transmission to other individuals. By analyzing risk factors for SARS-CoV-2 infection, researchers have attempted to understand and predict the progression of this infection in patients with underlying medical conditions such as diabetes or cardiovascular disease.
Saliva has proven to be a very convenient, non-invasive source of biomarkers for many cellular and systemic reactions occurring in the body during various disease states (63–67), including COVID-19. Since SARS-CoV-2 infection is also initiated in the oral and nasal cavity, detection of the coronavirus in saliva is likely to reflect early stages of infection.
Saliva can be collected using swabs, by coughing, or by collecting it directly from the salivary glands (68, 69). One study showed that the salivary glands are a reservoir for the coronavirus (70) (Figure 1). By directly measuring the viral load in the secretions of the glands, acute infection could be assessed, and appropriate measures taken to inhibit and/or prevent transmission.
Previous studies on COVID-19 have already shown that the coronavirus could be observed in the saliva before it could be isolated from the lungs. This reinforces the view that salivary diagnostics could be used to detect and therefore to prevent transmission, especially in asymptomatic individuals (71).
While some studies discovered that impairment of the gustatory senses was an early symptom of asymptomatic patients and patients with a mild degree of infection, they also concluded that ageusia is an important and unique feature of SARS-CoV-2 infection (72, 73). The various oral manifestations of SARS-CoV-2 infection could also shed light on the interplay between ACE2 receptors, angiotensin enzyme, hyposalivation, zinc deficiency, cellular inflammation in the taste buds, and possible central nervous system degradation by the virus (74).
Once saliva was deemed to be a good diagnostic tool to diagnose early infection, researchers found that self-collected specimens of saliva from the oral cavity and fluid from the nasal swabs provided the best sensitivity and specificity compared with specimens collected through invasive methods, such as collections from the trachea and bronchiolar lavage (75, 76). In one study, saliva was shown to be positive even before nasopharyngeal swabs tested positive for the infection (77). A recent study argued that studies did not indicate clearly how their samples of saliva were obtained and questioned the conclusions (78). Another study suggested future research should consider cellular proteases to understand why the virus was able to overcome natural immune responses to the infection (79). The authors of the study postulated that a modified device to collect secretions from salivary glands directly would bypass the concern that viruses were transported from elsewhere into the saliva. The biggest challenge with salivary specimens was the quality of the salivary specimens from hospitalized patients that were thick and stringy, requiring additives before processing (80).
Oral healthcare professionals can help to identify individuals at risk by using salivary diagnostics. Though it has shown that the viral load is high in nasopharyngeal swabs and the saliva during the first week of infection, a higher viral load and increase in the age of the patients correlated with severity of disease; the accuracy of the detection of the virus in the salivary samples thus requires more investigation (81). Despite these limitations, Point of Care (POC) salivary diagnostics is still promising with respect to early detection by oral healthcare professionals. Since patients may have viral presence in their saliva and be asymptomatic, oral healthcare professionals need to take adequate precautions to prevent transmission to themselves and others by using POC diagnostics (82). This would be the best strategy to slow down spread of the infection and prevent emergence of further mutations.
The current diagnostic tests for SARS-CoV-2 infection are based on molecular detection of viral RNA via (polymerase chain reaction) PCR analysis, and rapid-antigen tests that detect viral protein. In general, PCR tests are more accurate, but the technique is slower than detection of viral antigen, and PCR can detect nucleic acids when the individuals are no longer infectious (83–89). However, newer molecular tests based on CRISPR nucleases can measure viral nucleic acids faster than traditional PCR tests (90).
In conclusion, regardless of the method used to detect the coronavirus, future research should be directed to predicting the trajectory of mutations in coronaviruses, the mechanisms of pathogenesis due to infection with new variants, and development of POC systems that are high in sensitivity and specificity. The hope is that early diagnosis will help to prevent or dampen future waves of SARS-CoV-2 infections.
PI, TC and DMO wrote text for the article; PI prepared the figure. All authors contributed to the article and approved the submitted version.
This work was funded by intramural funds from the University of the Pacific.
The authors declare that the research was conducted in the absence of any commercial or financial relationships that could be construed as a potential conflict of interest.
All claims expressed in this article are solely those of the authors and do not necessarily represent those of their affiliated organizations, or those of the publisher, the editors and the reviewers. Any product that may be evaluated in this article, or claim that may be made by its manufacturer, is not guaranteed or endorsed by the publisher.
1. Chan FHM, Ataide R, Richards JS, Narh CA. Contrasting epidemiology and population genetics of COVID-19 infections defined by multilocus genotypes in SARS-CoV-2 genomes sampled globally. Viruses. (2022) 14:1434. doi: 10.3390/v14071434
2. Pinney SP, Giustino G, Halperin JL, Mechanick JI, Neibart E, Olin JW, et al. Coronavirus historical perspective, disease mechanisms, and clinical outcomes: JACC focus seminar. J Am Coll Cardiol. (2020) 76:1999–2010. doi: 10.1016/j.jacc.2020.08.058
3. Wang M, Li M, Ren R, Li L, Chen EQ, Li W, et al. International expansion of a novel SARS-CoV-2 mutant. J Virol. (2020) 94:e00567-20. doi: 10.1128/JVI.00567-20
4. Liu YC, Kuo RL, Shih SR. COVID-19: the first documented coronavirus pandemic in history. Biomed J. (2020) 43:328–33. doi: 10.1016/j.bj.2020.04.007
5. Nechipurenko YD, Anashkina AA, Matveeva OV. Change of antigenic determinants of SARS-CoV-2 virus S-protein as a possible cause of antibody-dependent enhancement of virus infection and cytokine storm. Biophysics (Oxf). (2020) 65:703–9. doi: 10.1134/S0006350920040119
6. Marsh M, Helenius A. Virus entry: open sesame. Cell. (2006) 124:729–40. doi: 10.1016/j.cell.2006.02.007
7. Sobhy H. A comparative review of viral entry and attachment during large and giant dsDNA virus infections. Arch Virol. (2017) 162:3567–85. doi: 10.1007/s00705-017-3497-8
8. Shang J, Wan Y, Luo C, Ye G, Geng Q, Auerbach A, et al. Cell entry mechanisms of SARS-CoV-2. Proc Natl Acad Sci USA. (2020) 117:11727–34. doi: 10.1073/pnas.2003138117
9. Huang Y, Yang C, Xu XF, Xu W, Liu SW. Structural and functional properties of SARS-CoV-2 spike protein: potential antivirus drug development for COVID-19. Acta Pharmacol Sin. (2020) 41:1141–9. doi: 10.1038/s41401-020-0485-4
10. Chen L, Li X, Chen M, Feng Y, Xiong C. The ACE2 expression in human heart indicates new potential mechanism of heart injury among patients infected with SARS-CoV-2. Cardiovasc Res. (2020) 116:1097–100. doi: 10.1093/cvr/cvaa078
11. Elrobaa IH, New KJ. COVID-19: pulmonary and extra pulmonary manifestations. Front Public Health. (2021) 9:711616. doi: 10.3389/fpubh.2021.711616
12. Ahn JH, Kim J, Hong SP, Choi SY, Yang MJ, Ju YS, et al. Nasal ciliated cells are primary targets for SARS-CoV-2 replication in the early stage of COVID-19. J Clin Invest. (2021) 131:e148517. doi: 10.1172/JCI148517
13. Sungnak W, Huang N, Becavin C, Berg M, Queen R, Litvinukova M, et al. SARS-CoV-2 entry factors are highly expressed in nasal epithelial cells together with innate immune genes. Nat Med. (2020) 26:681–7. doi: 10.1038/s41591-020-0868-6
14. Huang N, Perez P, Kato T, Mikami Y, Okuda K, Gilmore RC, et al. SARS-CoV-2 infection of the oral cavity and saliva. Nat Med. (2021) 27:892–903. doi: 10.1038/s41591-021-01296-8
15. Okui T, Matsuda Y, Karino M, Hideshima K, Kanno T. Oral mucosa could be an infectious target of SARS-CoV-2. Healthcare (Basel). (2021) 9:1068. doi: 10.3390/healthcare9081068
16. Sakaguchi W, Kubota N, Shimizu T, Saruta J, Fuchida S, Kawata A, et al. Existence of SARS-CoV-2 entry molecules in the oral cavity. Int J Mol Sci. (2020) 21:6000. doi: 10.3390/ijms21176000
17. Xu H, Zhong L, Deng J, Peng J, Dan H, Zeng X, et al. High expression of ACE2 receptor of 2019-nCoV on the epithelial cells of oral mucosa. Int J Oral Sci. (2020) 12:8. doi: 10.1038/s41368-020-0074-x
18. Lai AL, Millet JK, Daniel S, Freed JH, Whittaker GR. The SARS-CoV fusion peptide forms an extended bipartite fusion platform that perturbs membrane order in a calcium-dependent manner. J Mol Biol. (2017) 429:3875–92. doi: 10.1016/j.jmb.2017.10.017
19. Bayati A, Kumar R, Francis V, Mcpherson PS. SARS-CoV-2 infects cells after viral entry via clathrin-mediated endocytosis. J Biol Chem. (2021) 296:100306. doi: 10.1016/j.jbc.2021.100306
20. Tang T, Jaimes JA, Bidon MK, Straus MR, Daniel S, Whittaker GR. Proteolytic activation of SARS-CoV-2 spike at the S1/S2 boundary: potential role of proteases beyond furin. ACS Infect Dis. (2021) 7:264–72. doi: 10.1021/acsinfecdis.0c00701
21. Sweed D, Abdelsameea E, Khalifa EA, Abdallah H, Moaz H, Moaz I, et al. SARS-CoV-2-associated gastrointestinal and liver diseases: what is known and what is needed to explore. Egypt Liver J. (2021) 11:64. doi: 10.1186/s43066-021-00123-6
22. Alenquer M, Milheiro Silva T, Akpogheneta O, Ferreira F, Vale-Costa S, Medina-Lopes M, et al. Saliva molecular testing bypassing RNA extraction is suitable for monitoring and diagnosing SARS-CoV-2 infection in children. PLoS One. (2022) 17:e0268388. doi: 10.1371/journal.pone.0268388
23. Ye Q, Wang B, Mao J. The pathogenesis and treatment of the ‘cytokine storm’ in COVID-19. J Infect. (2020) 80:607–13. doi: 10.1016/j.jinf.2020.03.037
24. Mak JWY, Chan FKL, Ng SC. Probiotics and COVID-19: one size does not fit all. Lancet Gastroenterol Hepatol. (2020) 5:644–5. doi: 10.1016/S2468-1253(20)30122-9
25. Mak JWY, Chan FKL, Ng SC. Probiotics and COVID-19—authors’ reply. Lancet Gastroenterol Hepatol. (2020) 5:722–3. doi: 10.1016/S2468-1253(20)30197-7
26. Leung NHL. Transmissibility and transmission of respiratory viruses. Nat Rev Microbiol. (2021) 19:528–45. doi: 10.1038/s41579-021-00535-6
27. Jiao L, Li H, Xu J, Yang M, Ma C, Li J, et al. The gastrointestinal tract is an alternative route for SARS-CoV-2 infection in a nonhuman primate model. Gastroenterology. (2021) 160:1647–61. doi: 10.1053/j.gastro.2020.12.001
28. Khan A, Zia T, Suleman M, Khan T, Ali SS, Abbasi AA, et al. Higher infectivity of the SARS-CoV-2 new variants is associated with K417N/T, E484K, and N501Y mutants: an insight from structural data. J Cell Physiol. (2021) 236:7045–57. doi: 10.1002/jcp.30367
29. Lopez Bernal J, Gower C, Andrews N, Public Health England Delta Variant Vaccine Effectiveness Study, G. Effectiveness of COVID-19 vaccines against the B.1.617.2 (Delta) variant. Reply. N Engl J Med. (2021) 385:e92. doi: 10.1056/NEJMoa2108891
30. Zhu X, Mannar D, Srivastava SS, Berezuk AM, Demers JP, Saville JW, et al. Cryo-electron microscopy structures of the N501Y SARS-CoV-2 spike protein in complex with ACE2 and 2 potent neutralizing antibodies. PLoS Biol. (2021) 19:e3001237. doi: 10.1371/journal.pbio.3001237
31. Prillaman M. Prior Omicron infection protects against BA.4 and BA.5 variants. Nature. (2022) doi: 10.1038/d41586-022-01950-2. [Epub ahead of print]
32. Kliker L, Zuckerman N, Atari N, Barda N, Gilboa M, Nemet I, et al. COVID-19 vaccination and BA.1 breakthrough infection induce neutralising antibodies which are less efficient against BA.4 and BA.5 Omicron variants, Israel, March to June 2022. Euro Surveill. (2022) 27:2200559. doi: 10.2807/1560-7917.ES.2022.27.30.2200559
33. Gallucci S, Lolkema M, Matzinger P. Natural adjuvants: endogenous activators of dendritic cells. Nat Med. (1999) 5:1249–55. doi: 10.1038/15200
34. Jego G, Palucka AK, Blanck JP, Chalouni C, Pascual V, Banchereau J. Plasmacytoid dendritic cells induce plasma cell differentiation through type I interferon and interleukin 6. Immunity. (2003) 19:225–34. doi: 10.1016/S1074-7613(03)00208-5
35. Zhang Y, Gargan S, Roche FM, Frieman M, Stevenson NJ. Inhibition of the IFN-alpha JAK/STAT pathway by MERS-CoV and SARS-CoV-1 proteins in human epithelial cells. Viruses. (2022) 14:667. doi: 10.3390/v14040667
36. Low ZY, Zabidi NZ, Yip AJW, Puniyamurti A, Chow VTK, Lal SK. SARS-CoV-2 non-structural proteins and their roles in host immune evasion. Viruses. (2022) 14:1991. doi: 10.3390/v14091991
37. Khaledi M, Sameni F, Yahyazade S, Radandish M, Owlia P, Bagheri N, et al. COVID-19 and the potential of Janus family kinase (JAK) pathway inhibition: a novel treatment strategy. Front Med (Lausanne). (2022) 9:961027. doi: 10.3389/fmed.2022.961027
38. Kandhaya-Pillai R, Yang X, Tchkonia T, Martin GM, Kirkland JL, Oshima J. TNF-alpha/IFN-gamma synergy amplifies senescence-associated inflammation and SARS-CoV-2 receptor expression via hyper-activated JAK/STAT1. Aging Cell. (2022) 21:e13646. doi: 10.1111/acel.13646
39. Chen DY, Khan N, Close BJ, Goel RK, Blum B, Tavares AH, et al. SARS-CoV-2 disrupts proximal elements in the JAK-STAT pathway. J Virol. (2021) 95:e0086221. doi: 10.1128/JVI.00862-21
40. Van Der Wijst MGP, Vazquez SE, Hartoularos GC, Bastard P, Grant T, Bueno R, et al. Type I interferon autoantibodies are associated with systemic immune alterations in patients with COVID-19. Sci Transl Med. (2021) 13:eabh2624. doi: 10.1126/scitranslmed.abh2624
41. Manry J, Bastard P, Gervais A, Le Voyer T, Rosain J, Philippot Q, et al. The risk of COVID-19 death is much greater and age dependent with type I IFN autoantibodies. Proc Natl Acad Sci USA. (2022) 119:e2200413119. doi: 10.1073/pnas.2200413119
42. Bastard P, Rosen LB, Zhang Q, Michailidis E, Hoffmann HH, Zhang Y, et al. Autoantibodies against type I IFNs in patients with life-threatening COVID-19. Science. (2020) 370:eabd4585. doi: 10.1126/science.abd4585
43. Ferreira I, Kemp SA, Datir R, Saito A, Meng B, Rakshit P, et al. SARS-CoV-2 B.1.617 mutations L452R and E484Q are not synergistic for antibody evasion. J Infect Dis. (2021) 224:989–94. doi: 10.1093/infdis/jiab368
44. Mlcochova P, Kemp SA, Dhar MS, Papa G, Meng B, Ferreira I, et al. SARS-CoV-2 B.1.617.2 Delta variant replication and immune evasion. Nature. (2021) 599:114–9. doi: 10.1038/s41586-021-03944-y
45. Kirola L. Genetic emergence of B.1.617.2 in COVID-19. New Microbes New Infect. (2021) 43:100929. doi: 10.1016/j.nmni.2021.100929
46. Saxena SK, Kumar S, Ansari S, Paweska JT, Maurya VK, Tripathi AK, et al. Characterization of the novel SARS-CoV-2 Omicron (B.1.1.529) variant of concern and its global perspective. J Med Virol. (2022) 94:1738–44. doi: 10.1002/jmv.27524
47. Meng B, Abdullahi A, Ferreira I, Goonawardane N, Saito A, Kimura I, et al. Altered TMPRSS2 usage by SARS-CoV-2 Omicron impacts infectivity and fusogenicity. Nature. (2022) 603:706–14. doi: 10.1038/s41586-022-04474-x
48. Liu L, Iketani S, Guo Y, Chan JF, Wang M, Liu L, et al. Striking antibody evasion manifested by the Omicron variant of SARS-CoV-2. Nature. (2022) 602:676–81. doi: 10.1038/s41586-021-04388-0
49. Dhawan M, Priyanka , Choudhary OP. Omicron SARS-CoV-2 variant: reasons of emergence and lessons learnt. Int J Surg. (2022) 97:106198. doi: 10.1016/j.ijsu.2021.106198
50. Van Doremalen N, Schulz JE, Adney DR, Saturday TA, Fischer RJ, Yinda CK, et al. Efficacy of ChAdOx1 vaccines against SARS-CoV-2 variants of concern beta, delta and Omicron in the syrian hamster model. Res Sq. (2022):rs.3.rs-1343927. doi: 10.21203/rs.3.rs-1343927/v1. [Epub ahead of print]35194602
51. Lauring AS, Tenforde MW, Chappell JD, Gaglani M, Ginde AA, Mcneal T, et al. Clinical severity of, and effectiveness of mRNA vaccines against, COVID-19 from Omicron, delta, and alpha SARS-CoV-2 variants in the United States: prospective observational study. Br Med J. (2022) 376:e069761. doi: 10.1136/bmj-2021-069761
52. Kodera S, Rashed EA, Hirata A. Estimation of real-world vaccination effectiveness of mRNA COVID-19 vaccines against delta and Omicron variants in Japan. Vaccines (Basel). (2022) 10:430. doi: 10.3390/vaccines10030430
53. El-Shesheny R, El Taweel A, Gomaa MR, Roshdy WH, Kandeil A, Webby RJ, et al. Induced humoral immunity of different types of vaccines against most common variants of SARS-CoV-2 in Egypt prior to Omicron outbreak. Vaccine. (2022) 40:4303–6. doi: 10.1016/j.vaccine.2022.05.086
54. Ai J, Zhang H, Zhang Y, Lin K, Zhang Y, Wu J, et al. Omicron variant showed lower neutralizing sensitivity than other SARS-CoV-2 variants to immune sera elicited by vaccines after boost. Emerg Microbes Infect. (2022) 11:337–43. doi: 10.1080/22221751.2021.2022440
55. Maher MC, Bartha I, Weaver S, Di Iulio J, Ferri E, Soriaga L, et al. Predicting the mutational drivers of future SARS-CoV-2 variants of concern. Sci Transl Med. (2022) 14:eabk3445. doi: 10.1126/scitranslmed.abk3445
56. Kusiak A, Cichonska D, Tubaja M, Skorek A, Jereczek-Fossa BA, Corrao G, et al. COVID-19 manifestation in the oral cavity—a narrative literature review. Acta Otorhinolaryngol Ital. (2021) 41:395–400. doi: 10.14639/0392-100X-N1584
57. Herrera D, Serrano J, Roldan S, Sanz M. Is the oral cavity relevant in SARS-CoV-2 pandemic? Clin Oral Investig. (2020) 24:2925–30. doi: 10.1007/s00784-020-03413-2
58. Troeltzsch M, Berndt R, Troeltzsch M. Is the oral cavity a reservoir for prolonged SARS-CoV-2 shedding? Med Hypotheses. (2021) 146:110419. doi: 10.1016/j.mehy.2020.110419
59. Yu HQ, Sun BQ, Fang ZF, Zhao JC, Liu XY, Li YM, et al. Distinct features of SARS-CoV-2-specific IgA response in COVID-19 patients. Eur Respir J. (2020) 56:2001526. doi: 10.1183/13993003.01526-2020
60. Sterlin D, Mathian A, Miyara M, Mohr A, Anna F, Claer L, et al. Iga dominates the early neutralizing antibody response to SARS-CoV-2. Sci Transl Med. (2021) 13:eabd2223. doi: 10.1126/scitranslmed.abd2223
61. Alkharaan H, Bayati S, Hellstrom C, Aleman S, Olsson A, Lindahl K, et al. Persisting salivary IgG against SARS-CoV-2 at 9 months after mild COVID-19: a complementary approach to population surveys. J Infect Dis. (2021) 224:407–14. doi: 10.1093/infdis/jiab256
62. La Rosa GRM, Libra M, De Pasquale R, Ferlito S, Pedulla E. Association of viral infections with oral cavity lesions: role of SARS-CoV-2 infection. Front Med (Lausanne). (2020) 7:571214. doi: 10.3389/fmed.2020.571214
63. Xiang Z, Koo H, Chen Q, Zhou X, Liu Y, Simon-Soro A. Potential implications of SARS-CoV-2 oral infection in the host microbiota. J Oral Microbiol. (2020) 13:1853451. doi: 10.1080/20002297.2020.1853451
64. Zhang X, Zhang Y, Gui X, Zhang Y, Zhang Z, Chen W, et al. Salivary Fusobacterium nucleatum serves as a potential biomarker for colorectal cancer. iScience. (2022) 25:104203. doi: 10.1016/j.isci.2022.104203
65. Robinson JL, German GJ. Salivary antibodies are detected with a commercial anti-SARS-CoV-2 assay only after two doses of vaccine using serum thresholds. Clin Biochem. (2022) 104:66–9. doi: 10.1016/j.clinbiochem.2022.02.002
66. Nijakowski K, Gruszczynski D, Kopala D, Surdacka A. Salivary metabolomics for oral squamous cell carcinoma diagnosis: a systematic review. Metabolites. (2022) 12:294. doi: 10.3390/metabo12040294
67. Nguyen-Kim H, Beckmann C, Redondo M, Ziliox J, Vallet V, Berger-Sturm K, et al. COVID Salivary diagnostics: a comparative technical study. J Med Virol. (2022) 94:4277–86. doi: 10.1002/jmv.27883
68. Wyllie AL, Fournier J, Casanovas-Massana A, Campbell M, Tokuyama M, Vijayakumar P, et al. Saliva or nasopharyngeal swab specimens for detection of SARS-CoV-2. N Engl J Med. (2020) 383:1283–6. doi: 10.1056/NEJMc2016359
69. Borghi E, Massa V, Carmagnola D, Dellavia C, Parodi C, Ottaviano E, et al. Saliva sampling for chasing SARS-CoV-2: a game-changing strategy. Pharmacol Res. (2021) 165:105380. doi: 10.1016/j.phrs.2020.105380
70. Naqvi AR, Schwartz J, Brandini DA, Schaller S, Hussein H, Valverde A, et al. COVID-19 and oral diseases: assessing manifestations of a new pathogen in oral infections. Int Rev Immunol. (2022) 41:423–37. doi: 10.1080/08830185.2021.1967949
71. Xu J, Li Y, Gan F, Du Y, Yao Y. Salivary glands: potential reservoirs for COVID-19 asymptomatic infection. J Dent Res. (2020) 99:989. doi: 10.1177/0022034520918518
72. Qiu C, Cui C, Hautefort C, Haehner A, Zhao J, Yao Q, et al. Olfactory and gustatory dysfunction as an early identifier of COVID-19 in adults and children: an international multicenter study. Otolaryngol Head Neck Surg. (2020) 163:714–21. doi: 10.1177/0194599820934376
73. Lee Y, Min P, Lee S, Kim SW. Prevalence and duration of acute loss of smell or taste in COVID-19 patients. J Korean Med Sci. (2020) 35:e174. doi: 10.3346/jkms.2020.35.e174
74. Tsuchiya H. Oral symptoms associated with COVID-19 and their pathogenic mechanisms: a literature review. Dent J (Basel). (2021) 9:32. doi: 10.3390/dj9030032
75. Sapkota D, Soland TM, Galtung HK, Sand LP, Giannecchini S, To KKW, et al. COVID-19 salivary signature: diagnostic and research opportunities. J Clin Pathol. (2021) 74:344–9. doi: 10.1136/jclinpath-2020-206834
76. Kojima N, Turner F, Slepnev V, Bacelar A, Deming L, Kodeboyina S, et al. Self-collected oral fluid and nasal swabs demonstrate comparable sensitivity to clinician collected nasopharyngeal swabs for coronavirus disease 2019 detection. Clin Infect Dis. (2021) 73:e3106–9. doi: 10.1093/cid/ciaa1589
77. Azzi L, Maurino V, Baj A, Dani M, D’aiuto A, Fasano M, et al. Diagnostic salivary tests for SARS-CoV-2. J Dent Res. (2021) 100:115–23. doi: 10.1177/0022034520969670
78. Michailidou E, Poulopoulos A, Tzimagiorgis G. Salivary diagnostics of the novel coronavirus SARS-CoV-2 (COVID-19). Oral Dis. (2022) 28(Suppl 1):867–77. doi: 10.1111/odi.13729
79. Vinayachandran D, Balasubramanian S. Salivary diagnostics in COVID-19: future research implications. J Dent Sci. (2020) 15:364–6. doi: 10.1016/j.jds.2020.04.006
80. Landry ML, Criscuolo J, Peaper DR. Challenges in use of saliva for detection of SARS CoV-2 RNA in symptomatic outpatients. J Clin Virol. (2020) 130:104567. doi: 10.1016/j.jcv.2020.104567
81. Kapoor P, Chowdhry A, Kharbanda OP, Bablani Popli D, Gautam K, Saini V. Exploring salivary diagnostics in COVID-19: a scoping review and research suggestions. BDJ Open. (2021) 7:8. doi: 10.1038/s41405-021-00064-7
82. Sabino-Silva R, Jardim ACG, Siqueira WL. Coronavirus COVID-19 impacts to dentistry and potential salivary diagnosis. Clin Oral Investig. (2020) 24:1619–21. doi: 10.1007/s00784-020-03248-x
83. Tapari A, Braliou GG, Papaefthimiou M, Mavriki H, Kontou PI, Nikolopoulos GK, et al. Performance of antigen detection tests for SARS-CoV-2: a systematic review and meta-analysis. Diagnostics (Basel). (2022) 12:1388. doi: 10.3390/diagnostics12061388
84. Robinson ML, Mirza A, Gallagher N, Boudreau A, Garcia Jacinto L, Yu T, et al. Limitations of molecular and antigen test performance for SARS-CoV-2 in symptomatic and asymptomatic COVID-19 contacts. J Clin Microbiol. (2022) 60:e0018722. doi: 10.1128/jcm.00187-22
85. Mostafa M, Barhoum A, Sehit E, Gewaid H, Mostafa E, Omran MM, et al. Current trends in COVID-19 diagnosis and its new variants in physiological fluids: surface antigens, antibodies, nucleic acids, and RNA sequencing. Trends Analyt Chem. (2022) 157:116750. doi: 10.1016/j.trac.2022.116750
86. Kliegr T, Jarkovsky J, Jirincova H, Kuchar J, Karel T, Tachezy R. Role of population and test characteristics in antigen-based SARS-CoV-2 diagnosis, Czechia, August to November 2021. Euro Surveill. (2022) 27:2200070. doi: 10.2807/1560-7917.ES.2022.27.33.2200070
87. Kivrane A, Igumnova V, Liepina EE, Skrastina D, Leonciks A, Rudevica Z, et al. Development of rapid antigen test prototype for detection of SARS-CoV-2 in saliva samples. Ups J Med Sci. (2022) 127:1434. doi: 10.48101/ujms.v127.8207
88. Kastner A, Lucker P, Sombetzki M, Ehmke M, Koslowski N, Mittmann S, et al. SARS-CoV-2 surveillance by RT-qPCR-based pool testing of saliva swabs (lollipop method) at primary and special schools-A pilot study on feasibility and acceptability. PLoS One. (2022) 17:e0274545. doi: 10.1371/journal.pone.0274545
89. Jegerlehner S, Suter-Riniker F, Jent P, Bittel P, Nagler M. Diagnostic accuracy of SARS-CoV-2 saliva antigen testing in a real-life clinical setting. Int J Infect Dis. (2022) 119:38–40. doi: 10.1016/j.ijid.2022.03.037
Keywords: COVID-19, coronavirus, SARS-CoV-2, diagnostic, saliva
Citation: Iyer P, Chino T and Ojcius DM (2022) Infection of the oral cavity with SARS-CoV-2 variants: Scope of salivary diagnostics. Front. Oral. Health 3:1001790. doi: 10.3389/froh.2022.1001790
Received: 24 July 2022; Accepted: 5 October 2022;
Published: 31 October 2022.
Edited by:
Gena D Tribble, University of Texas Health Science Center at Houston, United StatesReviewed by:
Chiara Parodi, Columbia University, United States© 2022 Iyer, Chino and Ojcius. This is an open-access article distributed under the terms of the Creative Commons Attribution License (CC BY). The use, distribution or reproduction in other forums is permitted, provided the original author(s) and the copyright owner(s) are credited and that the original publication in this journal is cited, in accordance with accepted academic practice. No use, distribution or reproduction is permitted which does not comply with these terms.
*Correspondence: David Ojcius ZG9qY2l1c0BwYWNpZmljLmVkdQ==
Specialty Section: This article was submitted to Oral Infections and Microbes, a section of the journal Frontiers in Oral Health
Disclaimer: All claims expressed in this article are solely those of the authors and do not necessarily represent those of their affiliated organizations, or those of the publisher, the editors and the reviewers. Any product that may be evaluated in this article or claim that may be made by its manufacturer is not guaranteed or endorsed by the publisher.
Research integrity at Frontiers
Learn more about the work of our research integrity team to safeguard the quality of each article we publish.