- Department of Oral Health and Diagnostic Sciences, University of Connecticut Health Center, Farmington, CT, United States
Lactobacilli are among the most studied bacteria in the microbiome of the orodigestive and genitourinary tracts. As probiotics, lactobacilli may provide various benefits to the host. These benefits include regulating the composition of the resident microbiota, preventing – or even potentially reverting- a dysbiotic state. Candida albicans is an opportunistic pathogen that can influence and be influenced by other members of the mucosal microbiota and, under immune-compromising conditions, can cause disease. Lactobacillus and Candida species can colonize the same mucosal sites; however, certain Lactobacillus species display antifungal activities that can contribute to low Candida burdens and prevent fungal infection. Lactobacilli can produce metabolites with direct anticandidal function or enhance the host defense mechanisms against fungi. Most of the Lactobacillus spp. anticandidal mechanisms of action remain underexplored. This work aims to comprehensively review and provide an update on the current knowledge regarding these anticandidal mechanisms.
Introduction
According to a Human Microbiome Project definition –initially proposed by Lederberg and McCray in 2001 [1], the term microbiota comprises the communities of symbiotic and pathogenic microbes hosted by multicellular eukaryotic organisms –including humans- [2]. An individual may host trillions of these microorganisms –mostly bacteria- with whom they interact physically, chemically, and biologically. The interactions between the host and its associated microbiota as a whole are complex, dynamic, and mostly unknown. Understanding what a “healthy microbiome” is, its composition, and its impact on host health has been a challenge for decades [3]; however, the significant role indigenous microbiota play on the health of the host is well-established [4, 5]. Health-associated microbiota and their metabolic products may play a role in treating or preventing infections. For example, probiotics –live microorganisms-, paraprobiotics –non-viable microbial cells-, and post-biotics –soluble factors produced by bacteria are currently used in food products to promote health.
Around 98% of the microbial constituents of the oral and gastrointestinal (GI) tract of healthy humans fall within four predominant bacterial phyla: Firmicutes, Bacteroidetes, Actinobacteria, and Proteobacteria [3, 6]. The role of bacteria in maintaining oral health has been studied extensively; in contrast, fungal microbiota –comprising members of the mycobiome– remain mostly unexplored [7, 8]. Microbial composition and diversity in the oral cavity and GI tract have also been studied extensively. Environmental filtering and competitive exclusion shape microbial composition, favoring the presence of taxa distantly related to each other, resulting in a greater diversity –at the species level- among healthy hosts. In contrast, closely related taxa are favorably selected within an individual host [9]. Additional factors that shape microbiota diversity are host genetic variation, age, diet, and the ecological local microbial environment, among others [5, 10, 11]. Although microbiota may change over time, any major disruption that significantly alters the microbiota can lead to severe health consequences [12, 13].
Disruption of the health-associated microbiota contributing to infectious and non-communicable systemic diseases is known as dysbiosis. A dysbiotic state may result as a consequence of medical therapies such as antibiotics [14], cancer chemotherapy [15], metabolic disorders such as obesity [16], or infection with pathogenic fungi [17], bacteria [18], or viruses [19]. A dysbiotic state may aggravate inflammatory disorders such as inflammatory bowel disease, asthma, neurodegenerative diseases, and immunopathology [12, 20, 21]. Dysbiosis is also associated with the pathogenesis of metabolic disorders, such as obesity, insulin resistance, and dyslipidemia [22]. Moreover, under a dysbiotic scenario, some pathobionts (commensals that can turn into opportunistic pathogens) may form synergistic interactions with each other, leading to severe infections. Recently, Bertolini et al. showed that oral bacterial pathobionts facilitate fungal infection by increasing the expression of virulence genes in C. albicans or disrupting the mucosal barrier via proteolytic enzymes [23]. Hong et al. suggested oral microbiota disruption is associated with oral mucositis, exacerbating epithelial injury in cancer patients receiving cytotoxic chemotherapy [15].
In this paper, we provide a comprehensive review of the current understanding of the role of lactobacilli in maintaining mucosal homeostasis, with particular emphasis on their interactions with Candida species and mucosal candidiasis. All PubMed available information was analyzed and discussed under their respective thematic sections. We particularly focused on studies that described a mechanism of action, including in vitro, ex vivo, in vivo, and clinical trials.
Candida and Lactobacillus Influence on Host Health and Resident Mucosal Microbiota
Candidiasis and Dysbiosis
Candida albicans is a polymorphic yeast from the Candidaceae family. This yeast is a part of the skin, vaginal, oral, and gastrointestinal microbiota in most healthy humans [24]. Candida albicans has been traditionally defined as an opportunistic pathogen, and more recently as a pathobiont. Candida causes damage to the host under certain conditions, resulting in local (skin, mucosa) or systemic (blood, urinary, nervous system) candidiasis. The ability of C. albicans to transition between different morphological forms is a crucial factor for mucosal virulence [25, 26]. Candida albicans is the most common opportunistic fungal pathogen and the leading cause of death in fungal diseases [27]. Additionally, drug-resistant C. albicans strains and other Candida species such as C. auris, C. glabrata, and C. parapsilosis, are listed by the CDC as a serious threat to human health, as they are challenging to treat. Moreover, many non-albicans species are challenging to detect and identify [28, 29]. Candida species can form biofilms both on tissues and abiotic surfaces, increasing their survival under harsh environments and their resistance to antifungal drugs and disinfectants [30, 31].
Several reports show that mucosal candidiasis occurs at higher rates in immunocompromised patients [32, 33] as a consequence of the interplay between a defective innate or adaptive immunity and fungal virulence factors [18, 34, 35]. Candidiasis may influence and be influenced by members of the mucosal microbiome [36]. For example, a synergistic interaction between C. albicans and mitis group streptococci which was first identified experimentally [13, 37], may have clinical implications on Autosomal-Dominant Hyper IgE Syndrome patients, who are susceptible to this oral infection [21]. An example of Candida influencing members of the bacterial microbiome is the interaction between C. albicans and Staphylococcus aureus, which promotes bacterial colonization and systemic dissemination [38]. Additionally, Candida may alter the reestablishment of resident bacterial communities after broad-spectrum antibiotic treatment. Candida albicans alters the proportion and genus diversity within the main bacterial families (Lachnospiraceae, Ruminococcaceae, Rikenellaceae, and Porphyromonadaceae) in the mouse gut, favoring the overgrowth of Enterococcus and Streptococcus species while preventing the reestablishment of Lactobacillus and Oscillibacter [39, 40]. This disturbance in post-antibiotic communities may contribute to fungal infection since both enterococci and streptococci have been implicated in fungal pathogenic synergy [13, 17]. On the other hand, several bacterial members of the healthy microbiota display direct anticandidal properties or promote host anticandidal responses. Such bacterial species with broad influence on bacterial and fungal members of the microbiome are Lactobacillus spp., which may prevent dysbiosis and associated diseases, as described in the following sections.
Basic Physiology and Current Taxonomy of the Genus Lactobacillus
Lactobacillus spp. belong to the family Lactobacillaceae, a taxon of Gram-positive, rod-shaped bacteria whose main end metabolic product is lactic acid. Lactobacilli are facultative-anaerobic, non-motile, catalase-negative, psychrophilic microorganisms that do not form spores. Since their discovery more than 100 years ago, research regarding their evolution, natural history, and organization of the groups within the genus is under constant change. A decade ago, Singh et al. described the challenges regarding establishing the taxonomy within the genus Lactobacillus due to differences in the 16S rRNA gene, resulting in taxonomic uncertainty even in the four main groups –L. acidophilus, L. casei, L. plantarum, and L. delbrueckii- and their members [41]. Recently, an update by Duar et al. examined the phylogenetic, genomic, and metabolic information available to resolve the natural history of the genus, resulting in a new organization of the groups within the genus Lactobacillus, such as the L. reuteri group and moving the L. acidophilus group within the L. delbrueckii group [42]. Zheng et al. examined the physiology, ecology, core phylogeny, and sequence comparisons of 16S rRNA gene and clade-specific genes. This study resulted in the organization of the genus Lactobacillus into 26 phylogenetic groups, including creating new genera, which remain closely related to the genus Lactobacillus [43]. The classification by Zheng et al. has been adopted in the List of Prokaryotic names with Standing in Nomenclature (LPSN), resulting in significant changes, as follows [43, 44]: Lactobacillus casei group is now the genus Lacticaseibacillus (including L. casei, L. paracasei, and L. rhamnosus), Lactobacillus plantarum is now the genus Lactiplantibacillus (including L. plantarum, L. paraplantarum, and L. pentosus species.), and L. reuteri group is now the genus Limosilactobacillus (including species such as L. reuteri, and L. vaginalis). Lactobacillus delbrueckii group remains within the genus Lactobacillus (including species such as L. acidophilus, L. crispatus, L. gasseri, L. jensenii, L. johnsonii, and the L. delbrueckii subspecies such as L. delbrueckii ssp. bulgaricus and L. delbrueckii ssp. lactis). Due to the difficulty of differentiating between closely related Lactobacillus species (even with molecular techniques) and the proximity of lactobacilli to other genera, the term “Lactobacillus Genus Complex” describe lactobacilli and closely related taxa, such as a newly created genus from former Lactobacillus species, Leuconostococcaceae, and Pediococcus [42, 43, 45]. For this review, the species which were formerly members of the genus Lactobacillus and now belong to closely-related new genera –i.e., Lacticaseibacillus and Lactiplantibacillus- will be considered as members of the genus Lactobacillus. As the taxonomy of the Lactobacillus genus complex becomes more accurate, the identification of common biological traits within species of the same group –i.e., metabolite production, pili-like structures, etc.- is expanded, leading to a better understanding of the interactions with other members of the microbiota and the host.
Role of Lactobacilli in the Protection of Mucosal Barriers
Lactobacilli colonize diverse plants and animals, including humans [42], and have co-evolved with their hosts, becoming a stable member of the resident microbiota [46, 47]. Some lactobacilli display a pilus-like structure [48], which may be associated with probiotic properties. The pilus-associated proteins have only been studied in bacteria from the L. casei group [49–51], mainly in L. rhamnosus GG (LGG) [52]. This pilus-like structure facilitates binding to the mucus associated with mucosal epithelia [53] and plays a role in immunomodulation by reducing the expression of pro-inflammatory molecules (i.e., IL-6, TLR3) [54]. A recent review described the role of L. rhamnosus GG (LGG) in protecting the mucosal barrier, the effect of their metabolites on host cells, and their role on type 1 immune-responsiveness [55]. Like C. albicans, lactobacilli colonize mucosal tissues of the oral, gastrointestinal, and genitourinary tracts. As lactobacilli have been studied for decades, there is mounting evidence showing that they regulate metabolic processes of the host and other microorganisms and influence the host microbiota composition [56–58]. The FDA and the NIH ascertain that lactobacilli are GRAS (Generally Recognized As Safe) microorganisms associated with health and nutritional benefits [59, 60]. Lactobacilli are currently used in various food products and in treating gastrointestinal (GI) and non-GI medical conditions [61]. Among the different clinically relevant properties found in lactobacilli, their broad antimicrobial activity has attracted particular interest.
Effects of Lactobacillus-Based Therapies on Human Disease
Recent multiple clinical studies, including double-blind, randomized placebo-controlled studies, confirm lactobacilli used as probiotics reduce infections in patients, with no serious adverse events [62–64]. Skrzydło-Radomańska et al. reported that adult Irritable Bowel Syndrome (IBS) patients treated with a probiotic combination –from the species Lactobacillus and Bifidobacterium- may show reduced symptoms throughout 8-week treatment [65]. Yoon et al. showed that consumption of fermented milk containing a combination of L. paracasei and the plant Glycyrrhiza glabra reduced Helicobacter pylori numbers, inflammation, and gastrointestinal symptoms –indigestion, diarrhea, and abdominal pain- in infected patients [66]. Acute uncomplicated diverticulitis patients treated with a combination of L. reuteri and an antibiotic therapy showed reduced abdominal pain and inflammatory markers. Treated patients spent fewer hours of hospitalization than the placebo [67]. These studies also conclude that no serious adverse events have been observed in patients treated with lactobacilli.
Moreover, recent meta-analyses and systematic reviews conclude that evidence regarding the effectiveness of lactobacilli and other probiotics in combating infectious diseases in vivo is robust [68–74]. Several meta-analyses have shown that certain Lactobacillus species effectively reduce the burden of bacterial infections in patients, such as those from Helicobacter pylori [62, 75] or Clostridium difficile [63]. Ng et al. concluded that current data demonstrate that lactobacilli effectively combat urinary tract infections in females [64]. A recent meta-analysis by Szajewska et al. concluded that LGG reduces the duration of diarrhea and hospitalization in acute gastroenteritis pediatric patients [68]. Another meta-analysis by Gao et al. suggested that Lactobacillus spp can be a beneficial adjunct to non-surgical treatment of dental or peri-implant-associated inflammation initiated by bacterial biofilms [69]. Similarly, a systematic review suggested that lactobacilli –primarily L. rhamnosus GR-1 and L. reuteri- are beneficial in preventing and even treating recurrent urogenital infections, such as bacterial vaginosis [70]. Overall, despite the fact that some studies report no effect, comprehensive pooled data support the effectiveness of Lactobacillus-based probiotic therapies in bacterial infections.
There has been significant interest in using lactobacilli to combat candida infections. Earlier systematic reviews of older reports suggested that data regarding the antifungal activity of lactobacilli were inconsistent and inconclusive [70, 71], proposing that more research was needed due to the significance of the topic. As more studies have been conducted, there is more evidence supporting the anticandidal properties of lactobacilli. A clinical study showed that consumption of milk supplemented with L. rhamnosus reduced the severity of Candida-associated denture stomatitis in institutionalized elders by decreasing the prevalence of C. albicans [72]. Another clinical study showed that patients with the Familial Mediterranean Fever genetic disease displayed lower C. albicans burdens after treatment with Lactobacillus acidophilus [73]. A clinical study on very-low-birth-weight (VLBW) infants showed that Candida colonization was reduced by a prophylactic L. reuteri supplementation, which was as effective as the antifungal nystatin treatment and safer, as VLBW patients showed lower feeding intolerance and hospitalization time [74]. Women diagnosed with vulvovaginal candidiasis showed reduced vaginal discomfort and healthier vaginal pH after treatment with conventional treatment supplemented with L. plantarum [76].
Thus, there is a wide range of potential clinical applications of Lactobacillus-based therapies, even though currently their incorporation in clinical practice is at best limited. As lactobacilli and their metabolites display broad antimicrobial activity extending to bacteria and fungi, understanding their mechanisms of action is essential. Yet, most of the research thus far focused on antibacterial functions, leaving the antifungal mechanisms understudied. In the following sections, we review current knowledge regarding (1) the anticandidal mode of action of lactobacilli-derived metabolites; and (2) the interactions within the Lactobacillus-Candida-Host framework that prevent Candida infections.
Anticandidal Properties of Lactobacilli
Candida spp. infections are an increasing concern as drug-resistant strains are rising while the effectiveness of conventional antifungal therapies is diminishing. Consequently, innovative alternatives for preventing and treating Candida-related diseases have been explored, such as drug-repurposing [77], development of new molecules [78], nanotechnology [31, 79], and using other microorganisms as probiotics [80]. Lactobacillus species display promising anticandidal activity, yet knowledge on their modes of action is still scarce. The current knowledge regarding these anticandidal mechanisms is comprehensively reviewed and critically appraised in this review. We categorize the lactobacilli-mediated anti-Candida activity into direct and indirect. Direct anticandidal activity is primarily caused by bacterial metabolites that kill or inhibit the growth of yeast cells or prevent attachment, dimorphic transition, and biofilm formation. Indirect activity mechanisms rely on Lactobacillus-host interactions, as they involve modulation of immune processes or epithelial responses that prevent Candida growth on mucosal surfaces and protect the integrity of the mucosal barrier.
Candida and lactobacilli can colonize the same host sites, including the oral, pharyngeal, intestinal, and vaginal mucosae, since they are capable of adapting to a wide variety of environments, likely due to their genomic plasticity [81]. However, mucosal colonization in higher abundance by these organisms may be promoted by distinct nutrient profiles, oxygen tension, temperature, pH, and binding to site-specific mucins or epithelial receptors in each site. For example, lactobacilli are the dominant taxa in the healthy vaginal mucosa, characterized by acidic pH and low oxygen content, since they are aciduric facultative anaerobic organisms. In contrast, C. albicans colonize this site asymptomatically in low numbers, only in about 20% of healthy women [82]. In this site, alkalization may lead to dysbiosis, reducing Lactobacillus abundance and overgrowth of Candida, resulting in candidiasis [83]. In addition to acidic pH and low oxygen tension, a key ecological determinant for the sustained colonization of lactobacilli is retentive areas such as crypts and rugal folds in the stomach and carious lesions in the oral cavity [84]. Although C. albicans has a broad tolerance to pH and can grow well as planktonic yeast cells, their ability to transition to the hyphal phase and adhere to mucosal sites is impaired in acidic pH [85]. Thus, pH acidification by Lactobacillus-produced lactate limits the ability of fungi to cause disease in such mucosal sites. Below we provide a comprehensive analysis of a wide variety of mechanisms employed by lactobacilli which can limit Candida overgrowth on mucosal surfaces.
Direct Antifungal Activity
Lactobacilli produce a variety of active compounds (primary and secondary metabolites) that exhibit broad antimicrobial activity (Figure 1). A number of these metabolites are also effective against C. albicans. The most studied metabolites are bacteriocins [86] and bacteriocin-like peptides [87], cyclic dipeptides [88], proteinaceous compounds [89], enzymes [90], fatty acids [91], biosurfactants [92, 93], and other organic compounds such as reuterin [94], 3-Phenyllactatic acid [88], and LBP102, derived from L. plantarum NTU 102 [95]. Lactobacilli also produce inorganic compounds, such as hydrogen peroxide [96], leading to oxidative stress and genotoxicity in higher amounts. These compounds display broad antimicrobial activity against bacteria and fungi [97–100]. Current knowledge on specific Lactobacillus-produced metabolites and their respective anticandidal mechanisms is summarized in Table 1. In Figure 1, the main anticandidal mechanisms of action exerted by Lactobacillus products are graphically shown. Several species produce diverse metabolites that alter the physiology of the fungus by inducing oxidative stress and ATP depletion leading to cytotoxicity or growth inhibition. Other metabolites compromise the structural integrity of the fungal cell leading to alterations in cell morphology, membrane permeability, and death, while biosurfactants prevent adhesion to mucosal surfaces, as seen in Figure 1.
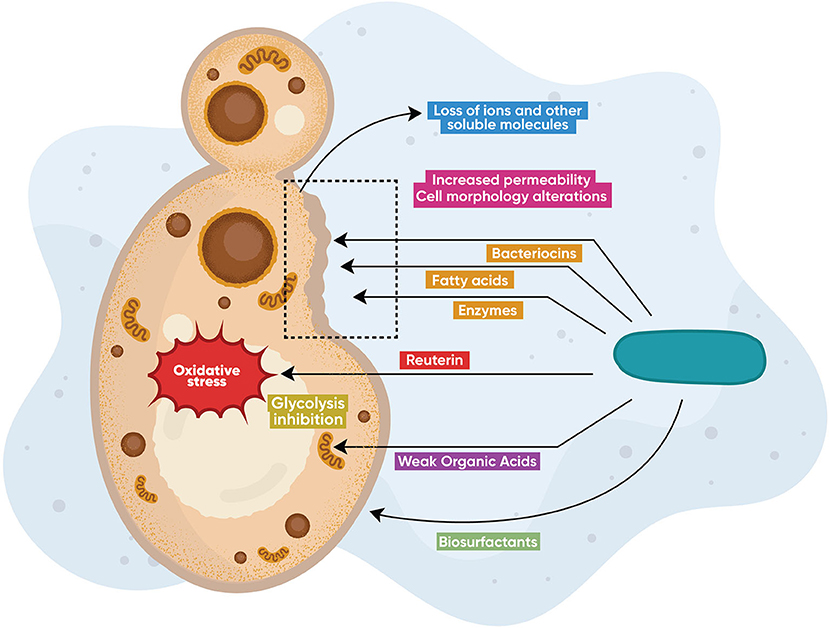
Figure 1. Direct antifungal modes of action. Lactobacillus species produce diverse metabolites including bacteriocins, enzymes (chitinases), hydrogen peroxide, fatty and other organic acids, as well as proteinaceous molecules (reuterin), that exert direct anticandidal activity via different mechanisms of action. Some metabolites alter the physiology of the fungus by inducing oxidative stress or by ATP depletion leading to cytotoxicity or growth inhibition. Other metabolites compromise the structural integrity of the fungal cell leading to alterations in cell morphology, membrane permeability, and death, while biosurfactants prevent adhesion to mucosal surfaces.
Bacteriocins and Bacteriocin-Like Substances
Bacteriocins are antimicrobial peptides, or peptide complexes, categorized into three major classes according to their synthesis and chemical structure [108, 109]. Bacteriocin-like substances (BLS) share traits similar to bacteriocins but do not entirely fit the typical criteria. Several reports suggest that putative lactobacilli-produced bacteriocins and BLS from cell culture supernatants exert anticandidal activity [110, 111]. However, only a handful of reports have studied the susceptibility of Candida to purified and biochemically characterized BLS and bacteriocins [86, 87, 112, 113]. The anticandidal mechanisms of action from lactobacilli-produced bacteriocins are practically unknown. The two-peptide plantaricin (a class IIb bacteriocin from L. plantarum) is the only purified bacteriocin whose effect on C. albicans physiology and morphology has been described. Plantaricin kills C. albicans by disrupting the cell membrane integrity due to depolarization and leakage of essential ions. Plantaricin also increases ROS production, inducing apoptosis [101]. The anticandidal activity of bacteriocins from bacteria other than lactobacilli –i.e., Lactococcus (nisin), Streptococcus (bacteriocin C3603), Enterococcus (bacteriocin Bac-GM17), among others- has also been studied by different groups [114–118].
Weak Organic Acids: Acetic, Lactic, Benzoic, and Sorbic Acids
Benzoic acid has antifungal activity caused by the disruption of glucose fermentation in yeast due to: (1) accumulation of hexose monophosphates; (2) decrease in intermediates beyond the phosphofructokinase cycle; and (3) pH-dependent enzymatic inhibition of phosphofructokinase and hexokinase, leading to subsequent inhibition of glycolysis that causes energy loss (ATP depletion). Other organic acids, such as sorbic acid and acetic acid, also inhibit the anaerobic fermentation on yeast and may share the same mechanism of action observed in benzoic acid [102]. The antifungal activity of benzoic acid is pH-dependent, as the undissociated acid (pKa = 4.2) is active only in acidic pH [119]. Low pH enables free diffusion of benzoic acid across the plasma membrane into the fungal cell [103], leading to intracellular acidification. This acidification causes turgor pressure increase, oxidative stress, and depletion of diverse molecules, including RNA [104]. Cottier et al. reported that acetic and butyric acids (and possibly other WOAs) reduce the C. albicans growth rate and change the expression of specific proteins like the putative glucose transporter Hgt16 protein and the predicted membrane transporter Orf19.7566, which mediate the fungistatic effects [120].
Fatty Acids
These acids disrupt the fungal membrane and the ultrastructural organization of the cell [105], resulting in growth inhibition of C. albicans. Specifically, fatty acids: (1) partition the lipid bilayers of cell membranes, resulting in loss of integrity and an increased fluidity and permeability, leading to an uncontrolled release of intracellular electrolytes and proteins [99]; (2) inhibit the production of ergosterol –an essential component of fungal cell membranes-, affecting the cell membrane integrity [107]; (3) compromise the structure of cell membranes due to their detergent-like properties [106]. Crowley et al. suggested that chain length may play an essential role in antifungal action, with long-chain fatty acids having the highest antifungal activity [99]; however, other studies show no apparent correlation between chain length and antifungal activity [105, 121].
Other Metabolites
Reuterin
Reuterin (3-hydroxypropionaldehyde acid) is a glycerol-derivative that displays fungicidal activity toward different Candida species [122]. The highly-reactive aldehyde group of reuterin may interact with thiol groups of small molecules and proteins, causing oxidative stress that results in growth inhibition [94]. Not surprisingly, reuterin increases the expression of OxyR, a transcriptional regulator that induces upregulation of oxidative stress genes.
Biosurfactants
Biosurfactants are compounds that contain both hydrophilic and hydrophobic moieties that reduce surface tension. Biosurfactants both hinder the ability of C. albicans to adhere to abiotic surfaces and tissues and display direct anticandidal activity. Although the modes of action of biosurfactants are still unknown, adherence reduction may be caused by changes in the cell wall charge, rendering the cell incapable of overcoming its electrostatic repulsion barrier with the substrate [123]. In addition to reducing adhesion, glycolipopeptide biosurfactants from L. pentosus exert direct anticandidal activity [124].
Enzymes
L. johnsonii produces enzymes with a chitinase-like activity, degrading the fungal cell wall of C. glabrata via enzymatic hydrolysis, leading to fungal growth inhibition [90].
Molecules From the Cell Surface
In addition to soluble metabolites, surface molecules from the cell wall have been identified to display anticandidal activity; however, their modes of action are still unknown. LGG cell wall-associated exopolysaccharides (EPS) reduce C. albicans hyphal transition and adhesion to epithelial cells [125].
Indirect Antifungal Activity: Lactobacillus-Host-Candida Interaction-Based Mechanisms
In vivo studies in animal models –particularly in mice- show that lactobacilli can reduce Candida burdens and reverse dysbiosis. Candida-induced stomach lesions, gastric inflammation, and dysbiosis in murine models are attenuated by several species, i.e., L. rhamnosus [126], L. gasseri, L. helveticus [127], and L. pentosus [128], among others. Lactobacilli may also act as prophylactic agents. Immunosuppressed mice that ingested L. rhamnosus 14 days before inoculation with Candida showed a significant reduction in C. albicans numbers and inflammation [129]. Another in vivo study showed that L. crispatus and L. fermentum supernatants reduce Candida burdens in a vulvovaginal candidiasis murine model [130].
Lactobacilli-host-fungal interactions that control Candida infections mainly result from competition in binding to mucus membrane glycoconjugates; strengthening the mucosal barrier through induction of mucins and positive regulation of tight junction proteins; triggering a protective immune response; and biotransformation of host metabolites into active anticandidal compounds, as shown in Figure 2.
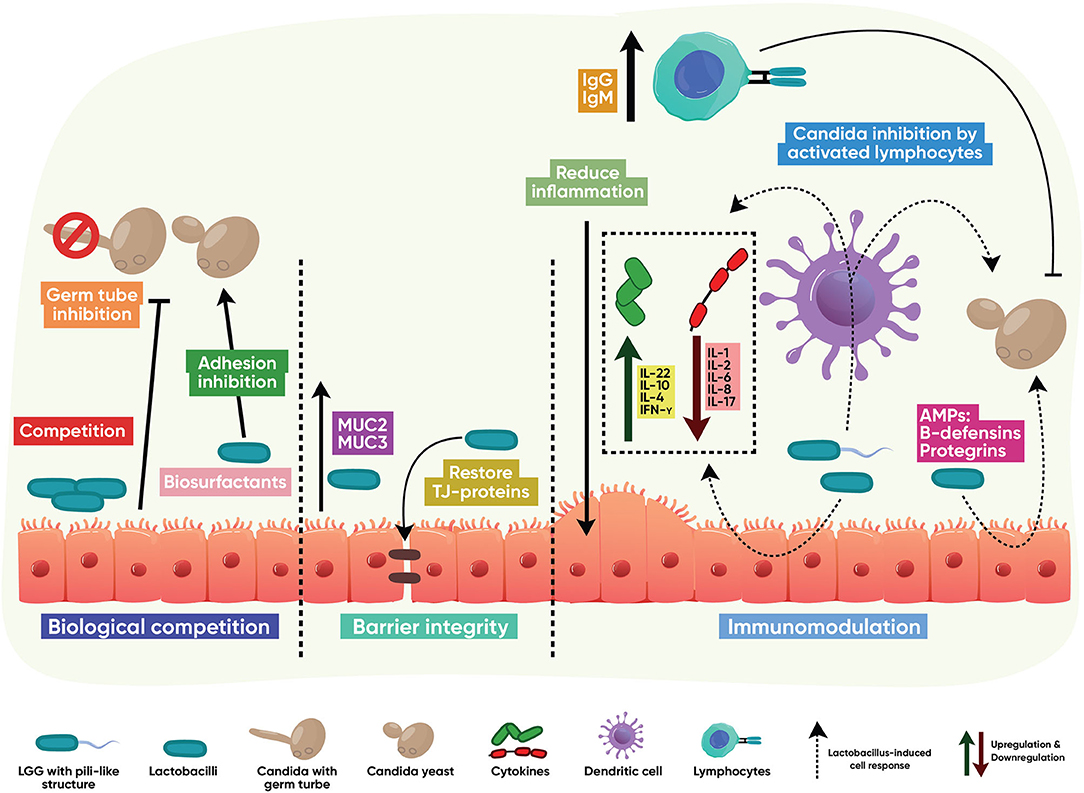
Figure 2. Indirect antifungal modes of action. Lactobacillus interacts with the host to stimulate protective, antifungal functions. These mainly result from competition with Candida in binding to mucus membrane glycoconjugates; strengthening the mucosal barrier through induction of mucins and positive regulation of tight junction proteins; activation of T and B cell immune responses; downregulation of host-destructive inflammation; and induction of innate epithelial antimicrobial peptides.
Biological Competition
Lactobacilli can reduce Candida colonization via competition, exclusion, and displacement mechanisms. Lactobacilli interact with GI tract mucus layer glycoconjugates to adhere avidly to the gut mucosa despite the constant changes in the intestinal environment [131]. Lactobacillus species adhere to epithelium resulting in reduced fungal adhesion and the ability to form mucosal biofilms [128, 132–135]. EPS from L. rhamnosus contribute to its adhesion to the host while reducing the epithelial adhesion of C. albicans [125]. Additionally, supernatants from L. gasseri and L. crispatus reduce the C. albicans ability to adhere to HeLa cells [136].
Immunomodulation
Lactobacilli display immunomodulatory activity by activating the immune response via classic surface-associated Microorganism-Associated Molecular Patterns (MAMPs). Lactobacillus MAMPS include the mannose-binding lectin Msa (L. plantarum), the cell-wall-associated hydrolases p40 and p75 (L. rhamnosus), and the S layer protein A –SlpA- (several Lactobacillus species). MAMPs induce a host response when interacting with host pattern recognition receptors [137]. The MAMP-induced host response can result in the prevention or reduction of Candida colonization, probably by their ability to activate Toll-like (TLRs) and NOD-like receptors (NLRs) [138]. The following epithelial antifungal effector mechanisms are reported to be influenced by lactobacilli:
Induction of Antimicrobial Peptides
Lactobacilli induce the production of several epithelial AMPs with anticandidal activity, such as β-defensins and protegrins [56, 139]. Vylkonova et al. showed that human β-defensins 2 and 3 disintegrate the cell wall of C. albicans, whereas only β-defensin 3 induces permeabilization on the cell membrane. Moreover, both β-defensins showed synergistic anticandidal activity with other AMPs –lysozyme and lactoferrin [140]. Rizzo et al. showed that β-defensin 2 and 3 upregulation is mediated by L. crispatus [139]. Protegrins are reported to disrupt the cell membrane of several microorganisms, including Candida species [141].
Anti-inflammatory Properties
Numerous in vitro reports confirm that different Lactobacillus species downregulate the expression of pro-apoptotic cytokines, i.e., TNFα- and several other pyroptotic or pro-inflammatory cytokines such as IL-1α, IL-1β, IL-2, IL-6, IL-8, IL-17, [56, 58, 89, 90, 139, 142]; whereas other studies showed that the anti-inflammatory cytokine IL-10 is upregulated [143, 144]. The pili-like structure from LGG reduces IL-6 expression while increasing the IL-10 production [54]. Tryptophan catabolism by lactobacilli has been reported to prevent colonization by C. albicans, as the production of indole derivatives from tryptophan degradation induces the production of IL-22 (an IL-10 group cytokine involved in mucosal barrier function and induction of epithelial AMPs) mediated by an aryl-hydrocarbon-receptor (AhR) [145]. Several in vivo studies –in murine models- confirm the modulatory effect of lactobacilli in cytokine production after Candida infection: L. crispatus and L. delbrueckii consumption increase IFN-γ, IL-4, and IgG expression while decreasing IL-17 expression [146], L. rhamnosus consumption reduces IL-6 production [129], and L. reuteri increases the expression IL-22 [147]. Authier et al. showed that combined oral administration of L. helveticus and L. gasseri reduced TNF-α, IL-1β, IL-8, and CRP inflammatory markers while increasing the expression of IL-1ra and IL-10 anti-inflammatory markers in colonic tissues of C. albicans-infected mice. Moreover, mRNA expression of enzymes involved in the synthesis of pro-inflammatory eicosanoids was also decreased [127]. Clinical trials also confirm the immunomodulatory properties of lactobacilli in humans. Macnaughtan et al. showed that L. casei-treated human patients with cirrhosis had lower plasma MIP-1B concentrations in an 8-week course; IL-1B and MPC-1 –in the alcoholic patient subgroup- and IL-17 –in the non-alcoholic cohort- plasma concentrations were also reduced [148]. Another clinical study reported that an L. reuteri-antibiotic combined therapy reduced abdominal pain and inflammatory markers (C-reactive protein levels) in acute uncomplicated diverticulitis patients; while also lowering hospitalization time [67]. The ability to reduce inflammation is critical in balancing immunopathology and protection in mucosal infections. For example, Swidsinski et al. showed that the colonic mucous layer is thinner in inflamed areas, allowing increased microbial adherence and infiltration [149].
Immune Cell Activation
In vitro analyses show that lactobacilli may activate immune cells by signaling viaTLR4 and TLR9 [90, 150] binding to cell wall peptide SlpA [151] or cell wall peptidoglycans (PGN) [152]. Lactobacillus acidophilus promotes the migration of macrophages [89] and regulates T cell responses by interacting with dendritic cells [153]. The LGG pilus-like structure may modulate inflammatory response by signaling via TLR3 and TLR4 in intestinal epithelial cells [54]. Studies in vivo show that lactobacilli may induce a protective IgG and IgM- response [154] and regulate T cell responses [155]. Additionally, purified PGN from L. rhamonosus significantly increases the T-cell titer in malnourished mice challenged with pneumococcal infection [156]. Intravaginal inoculation of L. crispatus biosurfactants reduced the leukocyte influx in mice challenged with C. albicans [133]. A recent clinical study showed that HIV patients treated with L. casei displayed an increase in the CD4+/CD8+ ratio compared to the placebo group; yet, the difference did not reach statistical significance [157].
Mechanisms of Epithelial Barrier Protection
Several pathogens disrupt the mucosal barrier integrity via different mechanisms, such as compromising the production of mucins or the organization of tight junction proteins [158, 159]. In vitro assays show that lactobacilli improve the epithelial barrier function against pathogen invasion, contributing to protection of the GI tract from invasive pathogens [160]. Lactobacilli induce epithelial secretion of mucins MUC2 and MUC3, which improve barrier integrity by preventing microbial adhesion [143, 161, 162]. Zhou et al. suggested that the modulation of the mucosal homeostasis and MUC2 expression may be related to lactobacilli EPS [163]. Additionally, Yu et al. revealed that when the epithelium is challenged with pathogenic bacteria, L. fructosus preserved the intestinal epithelial cell integrity and the transepithelial electrical resistance, an intercellular tight junction integrity marker [164]. In a porcine intestinal epithelial cell line (IPEC-J2) L. plantarum ZLP001 pretreatment inhibits the reduction in tight junction proteins and the increase in gut permeability caused by enteropathogenic E. coli infection. Lactobacilli maintain barrier integrity by increasing the expression of tight junction proteins (claudin-1, occludin, and Zonula occludens-1), thereby protecting the GI tract from invasive pathogens [56]. Also, the ability of lactobacilli to downregulate inflammatory cytokine expression may indirectly preserve the integrity of tight junctions [165]. In organoid models, L. reuteri can stimulate the proliferation of intestinal epithelia by increasing expression of R-spondins, leading to activation of the Wnt/β-catenin pathway [166].
In vivo studies in murine models also confirm the role of lactobacilli in preserving the integrity of the mucosal barrier. Recent studies showed that desmosome-like junctions –in vaginal epithelium- are reduced during Candida infections; however, ultrastructural analysis revealed that L. crispatus and L. delbrueckii increase the number of desmosome-like junctions to almost the numbers in uninfected epithelia [146]. Oral administration of L. reuteri reduced the colonization and intestinal inflammation in mice infected with the mucosal pathogen Citrobacter rodentium [166]. In induced epithelial barrier hyper-permeability mice, L. rhamnosus restored the expression of apical junction proteins Claudin-4, F11r, E-cadherin and occludin, confirming the role of lactobacilli in the modulation of junction proteins [167]. Moreover, L. reuteri ameliorated intestinal mucosa damage in DSS-treated mice by increasing the intestinal stem cell marker Lgr5+ and the antibacterial enzyme lysozyme [147].
Biotransformation of Host Compounds
Lactobacilli may metabolize host-produced macromolecules producing secondary metabolites with antimicrobial activity. This mechanism has been explored for bacteria but remains unexplored for Candida. Recently, McNair et al. reported a new casein-derived Lactobacillus-biotransformed peptide with antifungal activity [168].
Conclusions and Future Directions
Current evidence regarding the anticandidal activities of Lactobacillus supports their use as a promising therapeutic alternative agent for preventing and treating candidiasis; yet, several challenges exist in establishing how lactobacilli inhibit Candida growth. The production and/or activity of antifungal compounds in vitro can be affected by the type of culture media, pH, incubation time, solid vs. liquid culture, and the interaction of lactobacilli with other microorganisms which are part of the same ecosystem [98, 169–172]. Culture conditions influence both the qualitative composition and quantitative aspects of anticandidal metabolites; therefore, diverse metabolite profiles and activity may arise under non-standardized experiments among different laboratories [100]. For example, the composition of culture media influences bacteriocin production in L. pentosus [173]. Another example is the variable anticandidal activity of L. johnsonii in liquid vs. solid media. The anticandidal properties of L. johnsonii are usually reported as “good activity” in broth; in contrast, when the susceptibility assays are performed on agar plates, the inhibition is reported as “weak” or “null” [174–178]. Variable experimental conditions may also influence the detection sensitivity of specific metabolites, leading to contradictory results and potentially erroneous data interpretation. Also, lack of standardization negatively influences assay reproducibility among different laboratories. Many of these compounds are rarely examined under controlled pH conditions [98], which is critical for better understanding their activity. A physiologic range of pH should be maintained during antifungal activity assays to reflect the dynamic changes of the host tissue environment in health and disease. The interaction with other microorganisms also plays a role in lactobacilli metabolism. For example, certain strains of L. plantarum produced bacteriocins only when co-cultured with other microbial species in liquid media in vitro [170].
By regulating the growth of other microorganisms lactobacilli may maintain a healthy microbiome and inhibit dysbiotic disease states. Nevertheless, more research regarding the mutual effect of Lactobacillus spp. with other probiotics and other microorganisms –commensal or pathogenic- is needed. The presence, composition, and metabolic stage of the resident microbiota may affect the efficacy of Lactobacillus species used as probiotics. For example, while lactobacilli modulate the growth and morphologic transition of Candida in vitro, murine studies show that Candida overgrowth may reduce the abundance of oral lactobacilli, favoring an enterococcus-rich dysbiosis, as recently demonstrated by our group [17, 23]. Moreover, Candida may prevent the restoration of the healthy microbiota post-antibiotics, by inhibiting Lactobacillus spp in th murine gut [40].
Beyond the influence of lactobacilli on the host immune system, the indirect anticandidal mechanisms of lactobacilli remain relatively underexplored. Several connections between lactobacilli and host metabolism have been established; however, a comprehensive network of the complex metabolic interactions between probiotic lactobacilli and C. albicans remains to be characterized both in vitro and in vivo.
Lactobacilli-produced metabolites (post-biotics) also remain underexplored. Understanding the mechanism of action behind the anticandidal compounds is critical for assessing implications to non-pathogenic host microbiota, potential adverse effects, and their prospective therapeutically use as auxiliary anticandidal agents. The interactions between lactobacilli-produced antimicrobial post-biotics and current conventional therapies –including antibiotics- remain practically unknown. Would these anticandidal post-biotics display synergistic activity with current antifungal treatments?
In conclusion, lactobacilli exert direct and indirect activity against Candida species via a wide variety of mechanisms. As these organisms are among the most consumed probiotics, understanding their mechanisms of action is clinically relevant. While the pace of new anticandidal drug discovery remains slow and drug-resistance is becoming a serious threat to humans and animals worldwide, Lactobacillus-based antifungals may be used as effective adjunctive therapies in several mucosal fungal diseases.
Author Contributions
All authors contributed in the design and the preparation of the manuscript and have approved the final version of the manuscript.
Funding
This study was funded by NIH/NIDCR Grant RO1 DE013986 and NIH/NIGMS Grant RO1 GM127909.
Conflict of Interest
The authors declare that the research was conducted in the absence of any commercial or financial relationships that could be construed as a potential conflict of interest.
Acknowledgments
The authors would like to thank the graphic designer Salma Carballo for designing the images.
References
1. Lederberg BJ, McCray AT. ‘Ome Sweet' omics– a genealogical treasury of words. Scientist. (2001) 15:8. Available online at: https://www.the-scientist.com/commentary/ome-sweet-omics—a-genealogical-treasury-of-words-54889
2. Peterson J, Garges S, Giovanni M, McInnes P, Wang L, Schloss JA, et al. The NIH human microbiome project. Genome Res. (2009) 19:2317–23. doi: 10.1101/gr.096651.109
3. Lloyd-Price J, Abu-Ali G, Huttenhower C. The healthy human microbiome. Genome Med. (2016) 8:1–11. doi: 10.1186/s13073-016-0307-y
4. Proctor LM. The National Institutes of Health Human Microbiome Project. Semin Fetal Neonatal Med. (2016) 21:368–72. doi: 10.1016/j.siny.2016.05.002
5. Dunlop AL, Mulle JG, Ferranti EP, Edwards S, Dunn AB, Corwin EJ. Maternal microbiome and pregnancy outcomes that impact infant health. Adv Neonatal Care. (2015) 15:377–85. doi: 10.1097/ANC.0000000000000218
6. Huttenhower C, Gevers D, Knight R, Abubucker S, Badger JH, Chinwalla AT, et al. Structure, function and diversity of the healthy human microbiome. Nature. (2012) 486:207–14. doi: 10.1038/nature11234
7. Diaz PI, Strausbaugh LD, Dongari-Bagtzoglou A. Fungal-bacterial interactions and their relevance to oral health: linking the clinic and the bench. Front Cell Infect Microbiol. (2014) 4:101. doi: 10.3389/fcimb.2014.00101
8. Diaz PI, Dongari-Bagtzoglou A. Critically appraising the significance of the oral mycobiome. J Dent Res. (2021) 100:133–40. doi: 10.1177/0022034520956975
9. Walter J, Ley R. The human gut microbiome: ecology and recent evolutionary changes. Ann Rev Microbiol. (2011) 65:411–29. doi: 10.1146/annurev-micro-090110-102830
10. Manor O, Dai CL, Kornilov SA, Smith B, Price ND, Lovejoy JC, et al. Health and disease markers correlate with gut microbiome composition across thousands of people. Nat Commun. (2020) 11:5206. doi: 10.1038/s41467-020-18871-1
11. Blekhman R, Goodrich JK, Huang K, Sun Q, Bukowski R, Bell JT, et al. Host genetic variation impacts microbiome composition across human body sites. Genome Biol. (2015) 16:191. doi: 10.1186/s13059-015-0759-1
12. Das B, Nair GB. Homeostasis and dysbiosis of the gut microbiome in health and disease. J Biosci. (2019) 44:1–8. doi: 10.1007/s12038-019-9926-y
13. Xu H, Sobue T, Thompson A, Xie Z, Poon K, Ricker A, et al. Streptococcal co-infection augments candida pathogenicity by amplifying the mucosal inflammatory response. Cell Microbiol. (2014) 16:214–31. doi: 10.1111/cmi.12216
14. Lamendella R, Wright JR, Hackman J, McLimans C, Toole DR, Bernard Rubio W, et al. Antibiotic treatments for Clostridium difficile infection are associated with distinct bacterial and fungal community structures. mSphere. (2018) 3:572–89. doi: 10.1128/mSphere.00572-17
15. Hong BY, Sobue T, Choquette L, Dupuy AK, Thompson A, Burleson JA, et al. Chemotherapy-induced oral mucositis is associated with detrimental bacterial dysbiosis. Microbiome. (2019) 7:66. doi: 10.1186/s40168-019-0679-5
16. Amabebe E, Robert FO, Agbalalah T, Orubu ESF. Microbial dysbiosis-induced obesity: role of gut microbiota in homoeostasis of energy metabolism. Br J Nutr. (2020) 123:1127–37. doi: 10.1017/S0007114520000380
17. Bertolini M, Ranjan A, Thompson A, Diaz PI, Sobue T, Maas K, et al. Candida albicans induces mucosal bacterial dysbiosis that promotes invasive infection. PLoS Pathog. (2019) 15:e1007717. doi: 10.1371/journal.ppat.1007717
18. Pham TAN, Lawley TD. Emerging insights on intestinal dysbiosis during bacterial infections. Curr Opin Microbiol. (2014) 17:67–74. doi: 10.1016/j.mib.2013.12.002
19. Ashuro AA, Lobie TA, Ye DQ, Leng RX, Li BZ, Pan HF, et al. Review on the alteration of gut microbiota: the role of HIV infection and old age. AIDS Res Hum Retroviruses. (2020) 36:556–65. doi: 10.1089/aid.2019.0282
20. Askarova S, Umbayev B, Masoud AR, Kaiyrlykyzy A, Safarova Y, Tsoy A, et al. The links between the gut microbiome, aging, modern lifestyle and Alzheimer's disease. Front Cell Infect Microbiol. (2020) 10:e00104. doi: 10.3389/fcimb.2020.00104
21. Dutzan N, Kajikawa T, Abusleme L, Greenwell-Wild T, Zuazo CE, Ikeuchi T, et al. A dysbiotic microbiome triggers TH17 cells to mediate oral mucosal immunopathology in mice and humans. Sci Transl Med. (2018) 10:eaat0797. doi: 10.1126/scitranslmed.aat0797
22. Sanz Y, Olivares M, Moya-Pérez Á, Agostoni C. Understanding the role of gut microbiome in metabolic disease risk. Pediatr Res. (2015) 77:236–44. doi: 10.1038/pr.2014.170.
23. Bertolini M, Dongari-Bagtzoglou A. The dysbiosis and inter-kingdom synergy model in oropharyngeal candidiasis, a new perspective in pathogenesis. J Fungi. (2019) 5:87. doi: 10.3390/jof5040087
24. Calderone RA editor. Introduction and historical perspectives. In: Candida and Candidiasis. Washington, DC: ASM Press (2002). p. 3–14. Available online at: https://books.google.com/books?id=V6hpAAAAMAAJ
25. Pappas PG, Lionakis MS, Arendrup MC, Ostrosky-Zeichner L, Kullberg BJ. Invasive candidiasis. Nat Rev Dis Prim. (2018) 4:1–20. doi: 10.1038/nrdp.2018.26
26. Shapiro RS, Robbins N, Cowen LE. Regulatory circuitry governing fungal development, drug resistance, and disease. Microbiol Mol Biol Rev. (2011) 75:213–67. doi: 10.1128/MMBR.00045-10
27. Calderone RA. Taxonomy and biology of candida. In: Calderone RA, Clancy CJ, editors. Candida and Candidiasis. Washington, DC: ASM Press (2012). p. 15–28. Available online at: https://www.asmscience.org/content/book/10.1128/9781555817176 (accessed January 13, 2021).
28. Centers for Disease Control and Prevention. Antibiotic Resistance Threats in the United States. Atlanta, GA: Centers for Disease Control and Prevention (2019). Available online at: www.cdc.gov/DrugResistance/Biggest-Threats.html (accessed January 24, 2020).
29. Arendrup MC, Patterson TF. Multidrug-resistant candida: epidemiology, molecular mechanisms, and treatment. J Infect Dis. (2017) 216(Suppl. 3):S445–51. doi: 10.1093/infdis/jix131
30. Dongari-Bagtzoglou A, Kashleva H, Dwivedi P, Diaz P, Vasilakos J. Characterization of Mucosal Candida albicans biofilms. PLoS ONE. (2009) 4:e7967. doi: 10.1371/journal.pone.0007967
31. Vazquez-Munoz R, Arellano-Jimenez MJ, Lopez-Ribot JL. Bismuth nanoparticles obtained by a facile synthesis method exhibit antimicrobial activity against Staphylococcus aureus and Candida albicans. BMC Biomed Eng. (2020) 2:11. doi: 10.1186/s42490-020-00044-2
32. Pendleton KM, Dickson RP, Newton DW, Hoffman TC, Yanik GA, Huffnagle GB. Respiratory tract colonization by candida species portends worse outcomes in immunocompromised patients. Clin Pulm Med. (2018) 25:197–201. doi: 10.1097/CPM.0000000000000279
33. Shiboski CH, Chen H, Ghannoum MA, Komarow L, Evans S, Mukherjee PK, et al. Role of oral candidiasis in TB and HIV co-infection: AIDS clinical trial group protocol A5253. Int J Tuberc Lung Dis. (2014) 18:682–8. doi: 10.5588/ijtld.13.0729
34. Huffnagle GB, Deepe GS. Innate and adaptive determinants of host susceptibility to medically important fungi. Curr Opin Microbiol. (2003) 6:344–50. doi: 10.1016/S1369-5274(03)00089-4
35. Swidergall M, Solis NV, Millet N, Huang MY, Lin J, Phan QT, et al. Activation of EphA2-EGFR signaling in oral epithelial cells by Candida albicans virulence factors. PLoS Pathog. (2021) 17:e1009221. doi: 10.1371/journal.ppat.1009221
36. Bertolini M, Dongari-Bagtzoglou A. The relationship of Candida albicans with the oral bacterial microbiome in health and disease. Adv Exp Med Biol. (2019) 1197:69–78. doi: 10.1007/978-3-030-28524-1_6
37. Diaz PI, Xie Z, Sobue T, Thompson A, Biyikoglu B, Ricker A, et al. Synergistic interaction between Candida albicans and commensal oral streptococci in a Novel in vitro mucosal model. Infect Immun. (2012) 80:620. doi: 10.1128/IAI.05896-11
38. Schlecht LM, Peters BM, Krom BP, Freiberg JA, Hänsch GM, Filler SG, et al. Systemic Staphylococcus aureus infection mediated by Candida albicans hyphal invasion of mucosal tissue. Microbiol (United Kingdom). (2015) 161:168–81. doi: 10.1099/mic.0.083485-0
39. Downward JRE, Falkowski NR, Mason KL, Muraglia R, Huffnagle GB. Modulation of post-antibiotic bacterial community reassembly and host response by Candida albicans. Sci Rep. (2013) 3:1–11. doi: 10.1038/srep02191
40. Mason KL, Erb Downward JR, Falkowski NR, Young VB, Kao JY, Huffnagle GB. Interplay between the gastric bacterial microbiota and Candida albicans during postantibiotic recolonization and gastritis. Infect Immun. (2012) 80:150–8. doi: 10.1128/IAI.05162-11
41. Singh S, Goswami P, Singh R, Heller KJ. Application of molecular identification tools for Lactobacillus, with a focus on discrimination between closely related species: a review. LWT Food Sci Technol. (2009) 42:448–57. doi: 10.1016/j.lwt.2008.05.019
42. Duar RM, Lin XB, Zheng J, Martino ME, Grenier T, Pérez-Muñoz ME, et al. Lifestyles in transition: evolution and natural history of the genus Lactobacillus. FEMS Microbiol Rev. (2017) 41:S27–48. doi: 10.1093/femsre/fux030
43. Zheng J, Wittouck S, Salvetti E, Franz CMAP, Harris HMB, Mattarelli P, et al. A taxonomic note on the genus Lactobacillus: description of 23 novel genera, emended description of the genus Lactobacillus beijerinck 1901, and union of Lactobacillaceae and Leuconostocaceae. Int J Syst Evol Microbiol. (2020) 70:2782–858. doi: 10.1099/ijsem.0.004107
44. LPSN. Genus: Lactobacillus. List of Prokaryotic Names with Standing in Nomenclature. (2020). Available online at: https://lpsn.dsmz.de/genus/lactobacillus (accessed March 1, 2021).
45. Salvetti E, Harris HMB, Felis GE, O'Toole PW. Comparative genomics of the genus Lactobacillus reveals robust phylogroups that provide the basis for reclassification. Appl Environ Microbiol. (2018) 84:e00993–18. doi: 10.1128/AEM.00993-18
46. Oh PL, Benson AK, Peterson DA, Patil PB, Moriyama EN, Roos S, et al. Diversification of the gut symbiont Lactobacillus reuteri as a result of host-driven evolution. ISME J. (2010) 4:377–87. doi: 10.1038/ismej.2009.123
47. O'Callaghan J, O'Toole PW. Lactobacillus: host-microbe relationships. Curr Top Microbiol Immunol. (2013) 358:119–54. doi: 10.1007/82_2011_187
48. Kankainen M, Paulin L, Tynkkynen S, Von Ossowski I, Reunanen J, Partanen P, et al. Comparative genomic analysis of Lactobacillus rhamnosus GG reveals pili containing a human-mucus binding protein. Proc Natl Acad Sci USA. (2009) 106:17193–8. doi: 10.1073/pnas.0908876106
49. Smokvina T, Wels M, Polka J, Chervaux C, Brisse S, Boekhorst J, et al. Lactobacillus paracasei comparative genomics: towards species pan-genome definition and exploitation of diversity. PLoS ONE. (2013) 8:e68731. doi: 10.1371/journal.pone.0068731
50. Aleksandrzak-Piekarczyk T, Koryszewska-Bagińska A, Grynberg M, Nowak A, Cukrowska B, Kozakova H, et al. Genomic and functional characterization of the unusual pLOCK 0919 plasmid harboring the spaCBA pili cluster in Lactobacillus casei LOCK 0919. Genome Biol Evol. (2016) 8:202–17. doi: 10.1093/gbe/evv247
51. Hill D, Sugrue I, Tobin C, Hill C, Stanton C, Ross RP. The Lactobacillus casei group: history and health related applications. Front Microbiol. (2018) 9:2107. doi: 10.3389/fmicb.2018.02107
52. Tripathi P, Beaussart A, Alsteens D, Dupres V, Claes I, Von Ossowski I, et al. Adhesion and nanomechanics of pili from the probiotic Lactobacillus rhamnosus GG. ACS Nano. (2013) 7:3685–97. doi: 10.1021/nn400705u
53. Douillard FP, Ribbera A, Järvinen HM, Kant R, Pietilä TE, Randazzo C, et al. Comparative genomic and functional analysis of Lactobacillus casei and Lactobacillus rhamnosus strains marketed as probiotics. Appl Environ Microbiol. (2013) 79:1923–33. doi: 10.1128/AEM.03467-12
54. Ganguli K, Collado MC, Rautava J, Lu L, Satokari R, Von Ossowski I, et al. Lactobacillus rhamnosus GG and its SpaC pilus adhesin modulate inflammatory responsiveness and TLR-related gene expression in the fetal human gut. Pediatr Res. (2015) 77:528–35. doi: 10.1038/pr.2015.5
55. Capurso L. Thirty years of Lactobacillus rhamnosus GG. J Clin Gastroenterol. (2019) 53:S1–41. doi: 10.1097/MCG.0000000000001170
56. Wang J, Ji H, Wang S, Liu H, Zhang W, Zhang D, et al. Probiotic Lactobacillus plantarum promotes intestinal barrier function by strengthening the epithelium and modulating gut microbiota. Front Microbiol. (2018) 9:1953. doi: 10.3389/fmicb.2018.01953
57. Teame T, Wang A, Xie M, Zhang Z, Yang Y, Ding Q, et al. Paraprobiotics and postbiotics of probiotic lactobacilli, their positive effects on the host and action mechanisms: a review. Front Nutr. (2020) 7:570344. doi: 10.3389/fnut.2020.570344
58. Zangl I, Pap I-J, Aspöck C, Schüller C. The role of Lactobacillus. species in the control of Candida via biotrophic interactions. Microb Cell. (2020) 7:1–14. doi: 10.15698/mic2020.01.702
59. FDA. Microorganisms & Microbial-Derived Ingredients Used in Food (Partial List) | FDA. (2018). Available online at: https://www.fda.gov/food/generally-recognized-safe-gras/microorganisms-microbial-derived-ingredients-used-food-partial-list (accessed December 10, 2020).
60. Wang Y, Shurtleff D. Probiotics: What You Need To Know | NCCIH (2019). Available online at: https://www.nccih.nih.gov/health/probiotics-what-you-need-to-know (accessed December 10, 2020).
61. Dreher-Lesnick SM, Schreier JE, Stibitz S. Development of phage lysin LysA2 for use in improved purity assays for live biotherapeutic products. Viruses. (2015) 7:6675–88. doi: 10.3390/v7122965
62. Shi X, Zhang J, Mo L, Shi J, Qin M, Huang X. Efficacy and safety of probiotics in eradicating Helicobacter pylori: a network meta-analysis. Medicine (Baltimore). (2019) 98:e15180. doi: 10.1097/MD.0000000000015180
63. McFarland LV, Ship N, Auclair J, Millette M. Primary prevention of Clostridium difficile infections with a specific probiotic combining Lactobacillus acidophilus, L. casei, and L. rhamnosus strains: assessing the evidence. J Hosp Infect. (2018) 99:443–52. doi: 10.1016/j.jhin.2018.04.017
64. Ng QX, Peters C, Venkatanarayanan N, Goh YY, Ho CYX, Yeo W-S. Use of Lactobacillus spp. to prevent recurrent urinary tract infections in females. Med Hypotheses. (2018) 114:49–54. doi: 10.1016/j.mehy.2018.03.001
65. Skrzydło-Radomańska B, Prozorow-Król B, Cichoz-Lach H, Majsiak E, Bierła JB, Kosikowski W, et al. The effectiveness of synbiotic preparation containing Lactobacillus and Bifidobacterium probiotic strains and short chain Fructooligosaccharides in patients with diarrhea predominant irritable bowel syndrome-a randomized double-blind, placebo-controlled study. Nutrients. (2020) 12:1999. doi: 10.3390/nu12071999
66. Yoon JY, Cha JM, Hong SS, Kim HK, Kwak MS, Jeon JW, et al. Fermented milk containing Lactobacillus paracasei and Glycyrrhiza glabra has a beneficial effect in patients with Helicobacter pylori infection. Medicine (Baltimore). (2019) 98:e16601. doi: 10.1097/MD.0000000000016601
67. Petruzziello C, Migneco A, Cardone S, Covino M, Saviano A, Franceschi F, et al. Supplementation with Lactobacillus reuteri ATCC PTA 4659 in patients affected by acute uncomplicated diverticulitis: a randomized double-blind placebo controlled trial. Int J Colorectal Dis. (2019) 34:1087–94. doi: 10.1007/s00384-019-03295-1
68. Szajewska H, Kołodziej M, Gieruszczak-Białek D, Skórka A, Ruszczyński M, Shamir R. Systematic review with meta-analysis: Lactobacillus rhamnosus GG for treating acute gastroenteritis in children - a 2019 update. Aliment Pharmacol Ther. (2019) 49:1376–84. doi: 10.1111/apt.15267
69. Gao J, Yu S, Zhu X, Yan Y, Zhang Y, Pei D. Does probiotic Lactobacillus have an adjunctive effect in the nonsurgical treatment of peri-implant diseases? A systematic review and meta-analysis. J Evid Based Dental Pract. (2020) 20:101398. doi: 10.1016/j.jebdp.2020.101398
70. Abad CL, Safdar N. The role of Lactobacillus probiotics in the treatment or prevention of urogenital infectionsi—a systematic review. J Chemother. (2009) 21:243–52. doi: 10.1179/joc.2009.21.3.243
71. Jeavons HS. Prevention and treatment of vulvovaginal candidiasis using exogenous Lactobacillus. J Obstetr Gynecol Neonatal Nurs. (2003) 32:287–96. doi: 10.1177/0884217503253439
72. Lee X, Vergara C, Lozano C. Severity of Candida-associated denture stomatitis is improved in institutionalized elders who consume Lactobacillus rhamnosus SP1. Aust Dent J. (2019) 64:229–36. doi: 10.1111/adj.12692
73. Pepoyan A, Balayan M, Manvelyan A, Galstyan L, Pepoyan S, Petrosyan S, et al. Probiotic Lactobacillus acidophilus strain INMIA 9602 Er 317/402 administration reduces the numbers of Candida albicans and abundance of Enterobacteria in the gut microbiota of familial Mediterranean fever patients. Front Immunol. (2018) 9:26. doi: 10.3389/fimmu.2018.01426
74. Oncel MY, Arayici S, Sari FN, Simsek GK, Yurttutan S, Erdeve O, et al. Comparison of Lactobacillus reuteri and nystatin prophylaxis on Candida colonization and infection in very low birth weight infants. J Matern Neonatal Med. (2015) 28:1790–4. doi: 10.3109/14767058.2014.968842
75. Fang HR, Zhang GQ, Cheng JY, Li ZY. Efficacy of Lactobacillus-supplemented triple therapy for Helicobacter pylori infection in children: a meta-analysis of randomized controlled trials. Eur J Pediatr. (2019) 178:7–16. doi: 10.1007/s00431-018-3282-z
76. De Seta F, Parazzini F, De Leo R, Banco R, Maso GP, De Santo D, et al. Lactobacillus plantarum P17630 for preventing Candida vaginitis recurrence: a retrospective comparative study. Eur J Obstet Gynecol Reprod Biol. (2014) 182:136–9. doi: 10.1016/j.ejogrb.2014.09.018
77. Wall G, Herrera N, Lopez-Ribot JL. Repositionable compounds with antifungal activity against multidrug resistant candida auris identified in the medicines for malaria venture's pathogen box. J Fungi. (2019) 5:92. doi: 10.3390/jof5040092
78. Jagadesan P, Yu Z, Barboza-Ramos I, Lara HH, Vazquez-Munoz R, López-Ribot JL, et al. Light-activated antifungal properties of imidazolium-functionalized cationic conjugated polymers. Chem Mater. (2020) 32:6186–96. doi: 10.1021/acs.chemmater.0c02076
79. Vazquez-Munoz R, Lopez FD, Lopez-Ribot JL. Silver nanoantibiotics display strong antifungal activity against the emergent multidrug-resistant yeast candida auris under both planktonic and biofilm growing conditions. Front Microbiol. (2020) 11:1673. doi: 10.3389/fmicb.2020.01673
80. Mundula T, Ricci F, Barbetta B, Baccini M, Amedei A. Effect of probiotics on oral candidiasis: a systematic review and meta-analysis. Nutrients. (2019) 11:2449. doi: 10.3390/nu11102449
81. Poulain D. Candida albicans, plasticity and pathogenesis. Criti Rev Microbiol. (2015) 41:208–17. doi: 10.3109/1040841X.2013.813904
82. Sobel JD. Vaginitis, vulvitis, cervicitis and cutaneous vulval lesions. Infect Dis. (2017) 1:483–491.e1. doi: 10.1016/B978-0-7020-6285-8.00053-8
83. van de Wijgert JHHM, Jespers V. The global health impact of vaginal dysbiosis. Res Microbiol. (2017) 168:859–64. doi: 10.1016/j.resmic.2017.02.003
84. Caufield PW, Schön CN, Saraithong P, Li Y, Argimón S. Oral lactobacilli and dental caries: a model for niche adaptation in humans. J Dental Res. (2015) 94:110S−8S. doi: 10.1177/0022034515576052
85. Cauchie M, Desmet S, Lagrou K. Candida and its dual lifestyle as a commensal and a pathogen. Res Microbiol. (2017) 168:802–10. doi: 10.1016/j.resmic.2017.02.005
86. Pascual LM, Daniele MB, Giordano W, Pájaro MC, Barberis IL. Purification and partial characterization of novel bacteriocin L23 produced by Lactobacillus fermentum L23. Curr Microbiol. (2008) 56:397–402. doi: 10.1007/s00284-007-9094-4
87. Okkers DJ, Dicks LMT, Silvester M, Joubert JJ, Odendaal HJ. Characterization of pentocin TV35b, a bacteriocin-like peptide isolated from Lactobacillus pentosus with a fungistatic effect on Candida albicans. J Appl Microbiol. (1999) 87:726–34. doi: 10.1046/j.1365-2672.1999.00918.x
88. Ström K, Sjögren J, Broberg A, Schnürer J. Lactobacillus plantarum MiLAB 393 produces the antifungal cyclic dipeptides cyclo(L-Phe-L-Pro) and cyclo(L-Phe-trans-4-OH-L-Pro) and 3-phenyllactic acid. Appl Environ Microbiol. (2002) 68:4322–7. doi: 10.1128/AEM.68.9.4322-4327.2002
89. Lakhtin M, Alyoshkin V, Lakhtin V, Afanasyev S, Pozhalostina L, Pospelova V. Probiotic Lactobacillus and bifidobacterial lectins against Candida albicans and Staphylococcus aureus clinical strains: new class of the pathogen biofilm destructors. Prob Antimicrob Proteins. (2010) 2:186–96. doi: 10.1007/s12602-010-9046-3
90. Charlet R, Bortolus C, Sendid B, Jawhara S. Bacteroides thetaiotaomicron and Lactobacillus johnsonii modulate intestinal inflammation and eliminate fungi via enzymatic hydrolysis of the fungal cell wall. Sci Rep. (2020) 10:11510. doi: 10.1038/s41598-020-68214-9
91. Lipinska-Zubrycka L, Klewicki R, Sojka M, Bonikowski R, Milczarek A, Klewicka E. Anticandidal activity of Lactobacillus spp. in the presence of galactosyl polyols. Microbiol Res. (2020) 240:126540. doi: 10.1016/j.micres.2020.126540
92. Ceresa C, Tessarolo F, Caola I, Nollo G, Cavallo M, Rinaldi M, et al. Inhibition of Candida albicans adhesion on medical-grade silicone by a Lactobacillus-derived biosurfactant. J Appl Microbiol. (2015) 118:1116–25. doi: 10.1111/jam.12760
93. Zakaria Gomaa E. Antimicrobial and anti-adhesive properties of biosurfactant produced by lactobacilli isolates, biofilm formation and aggregation ability. J Gen Appl Microbiol. (2013) 59:425–36. doi: 10.2323/jgam.59.425
94. Schaefer L, Auchtung TA, Hermans KE, Whitehead D, Borhan B, Britton RA. The antimicrobial compound reuterin (3-hydroxypropionaldehyde) induces oxidative stress via interaction with thiol groups. Microbiology. (2010) 156:1589–99. doi: 10.1099/mic.0.035642-0
95. Lin TH, Pan TM. Characterization of an antimicrobial substance produced by Lactobacillus plantarum NTU 102. J Microbiol Immunol Infect. (2019) 52:409–17. doi: 10.1016/j.jmii.2017.08.003
96. Strus M, Kucharska A, Kukla G, Brzychczy-Włoch M, Maresz K, Heczko PB. The in vitro activity of vaginal Lactobacillus with probiotic properties against Candida. Infect Dis Obstet Gynecol. (2005) 13:69–75. doi: 10.1080/10647440400028136
97. Ribeiro FC, Rossoni RD, Barros PP, Santos JD, Fugisaki LRO, Leão MPV, et al. Action mechanisms of probiotics on Candida spp. and candidiasis prevention: an update. J Appl Microbiol. (2020) 129:175–85. doi: 10.1111/jam.14511
98. Siedler S, Balti R, Neves AR. Bioprotective mechanisms of lactic acid bacteria against fungal spoilage of food. Curr Opin Biotechnol. (2019) 56:138–46. doi: 10.1016/j.copbio.2018.11.015
99. Crowley S, Mahony J, Van Sinderen D. Current perspectives on antifungal lactic acid bacteria as natural bio-preservatives. Trends Food Sci Technol. (2013) 33:93–109. doi: 10.1016/j.tifs.2013.07.004
100. Lipińska L, Klewicki R, Sójka M, Bonikowski R, Zyzelewicz D, Kołodziejczyk K, et al. Antifungal activity of Lactobacillus pentosus ŁOCK 0979 in the presence of polyols and galactosyl-polyols. Probiotics Antimicrob Proteins. (2018) 10:186–200. doi: 10.1007/s12602-017-9344-0
101. Sharma A, Srivastava S. Anti-Candida activity of spent culture filtrate of Lactobacillus plantarum strain LR/14. J Mycol Med. (2014) 24:e25–34. doi: 10.1016/j.mycmed.2013.11.001
102. Krebs HA, Wiggins D, Stubbs M, Sols A, Bedoya F. Studies on the mechanism of the antifungal action of benzoate. Biochem J. (1983) 214:657–63. doi: 10.1042/bj2140657
103. Schwenninger SM, Meile L, Lacroix C. Antifungal lactic acid bacteria and propionibacteria for food biopreservation. Prot Cult Antimicrob Metab Bacteriophages Food Beverage Biopreserv. (2011) 1:27–62. doi: 10.1533/9780857090522.1.27
104. Lourenço A, Pedro NA, Salazar SB, Mira NP. Effect of acetic acid and lactic acid at low pH in growth and azole resistance of Candida albicans and Candida glabrata. Front Microbiol. (2019) 9:3265. doi: 10.3389/fmicb.2018.03265
105. Bergsson G, Arnfinnsson J, Steingrímsson Ó, Thormar H. In vitro killing of Candida albicans by fatty acids and monoglycerides. Antimicrob Agents Chemother. (2001) 45:3209–12. doi: 10.1128/AAC.45.11.3209-3212.2001
106. Sjögren J, Magnusson J, Broberg A, Schnürer J, Kenne L. Antifungal 3-hydroxy fatty acids from Lactobacillus plantarum MiLAB 14. Appl Environ Microbiol. (2003) 69:7554–7. doi: 10.1128/AEM.69.12.7554-7557.2003
107. Lee J, Kim Y, Khadke SK, Lee J. Antibiofilm and antifungal activities of medium-chain fatty acids against Candida albicans via mimicking of the quorum-sensing molecule farnesol. Microb Biotechnol. (2020) 1751–7915.13710. doi: 10.1111/1751-7915.13710
108. Zimina M, Babich O, Prosekov A, Sukhikh S, Ivanova S, Shevchenko M, et al. Overview of global trends in classification, methods of preparation and application of bacteriocins. Antibiotics. (2020) 9:1–21. doi: 10.3390/antibiotics9090553
109. Kumariya R, Garsa AK, Rajput YS, Sood SK, Akhtar N, Patel S. Bacteriocins: classification, synthesis, mechanism of action and resistance development in food spoilage causing bacteria. Microbial Pathogenesis. (2019) 128:171–7. doi: 10.1016/j.micpath.2019.01.002
110. Sabia C, Anacarso I, Bergonzini A, Gargiulo R, Sarti M, Condò C, et al. Detection and partial characterization of a bacteriocin-like substance produced by Lactobacillus fermentum CS57 isolated from human vaginal secretions. Anaerobe. (2014) 26:41–5. doi: 10.1016/j.anaerobe.2014.01.004
111. Stoyancheva G, Marzotto M, Dellaglio F, Torriani S. Bacteriocin production and gene sequencing analysis from vaginal Lactobacillus strains. Arch Microbiol. (2014) 196:645–53. doi: 10.1007/s00203-014-1003-1
112. Smaoui S, Elleuch L, Bejar W, Karray-Rebai I, Ayadi I, Jaouadi B, et al. Inhibition of fungi and Gram-negative bacteria by bacteriocin BacTN635 produced by Lactobacillus plantarum sp. TN635. Appl Biochem Biotechnol. (2010) 162:1132–46. doi: 10.1007/s12010-009-8821-7
113. Wannun P, Piwat S, Teanpaisan R. Purification, characterization, and optimum conditions of fermencin SD11, a bacteriocin produced by human orally Lactobacillus fermentum SD11. Appl Biochem Biotechnol. (2016) 179:572–82. doi: 10.1007/s12010-016-2014-y
114. Akerey B, Le-Lay C, Fliss I, Subirade M, Rouabhia M. In vitro efficacy of nisin Z against Candida albicans adhesion and transition following contact with normal human gingival cells. J Appl Microbiol. (2009) 107:1298–307. doi: 10.1111/j.1365-2672.2009.04312.x
115. Le Lay C, Akerey B, Fliss I, Subirade M, Rouabhia M. Nisin Z inhibits the growth of Candida albicans and its transition from blastospore to hyphal form. J Appl Microbiol. (2008) 105:1630–9. doi: 10.1111/j.1365-2672.2008.03908.x
116. Mouloud G, Daoud H, Bassem J, Laribi Atef I, Hani B. New Bacteriocin from Bacillus clausii StrainGM17: purification, characterization, and biological activity. Appl Biochem Biotechnol. (2013) 171:2186–200. doi: 10.1007/s12010-013-0489-3
117. Ikeda T, Iwanami T, Hirasawa M, Watanabe C, McGhee JR, Shiota T. Purification and certain properties of a bacteriocin from Streptococcus mutans. Infect Immun. (1982) 35:861–8. doi: 10.1128/iai.35.3.861-868.1982
118. Graham CE, Cruz MR, Garsin DA, Lorenz MC. Enterococcus faecalis bacteriocin EntV inhibits hyphal morphogenesis, biofilm formation, and virulence of Candida albicans. Proc Natl Acad Sci. (2017) 114:4507–12. doi: 10.1073/pnas.1620432114
119. Chipley JR. Sodium benzoate and benzoin acid. In: Jairus RDD, Davidson PM, Taylor TM, editors. Antimicrobials in Food. Taylor & Francis Group (2005). p. 41–88. Available online at: https://www.google.com/books/edition/Antimicrobials_in_Food/0ZUBEAAAQBAJ?hl=en&gbpv=1&dq=%22Benzoic+acid%22+AND+Lactobacillus+AND+mechanisms+AND+antifungal&pg=PA41&printsec=frontcover (accessed November 13, 2020).
120. Cottier F, Tan ASM, Yurieva M, Liao W, Lum J, Poidinger M, et al. The transcriptional response of Candida albicans to weak organic acids, carbon source, and MIG1 inactivation unveils a role for HGT16 in mediating the fungistatic effect of acetic acid. G3 Genes Genomes Genet. (2017) 7:3597–604. doi: 10.1534/g3.117.300238
121. Huang CB, Alimova Y, Myers TM, Ebersole JL. Short- and medium-chain fatty acids exhibit antimicrobial activity for oral microorganisms. Arch Oral Biol. (2011) 56:650–4. doi: 10.1016/j.archoralbio.2011.01.011
122. Vimont A, Fernandez B, Ahmed G, Fortin HP, Fliss I. Quantitative antifungal activity of reuterin against food isolates of yeasts and moulds and its potential application in yogurt. Int J Food Microbiol. (2019) 289:182–8. doi: 10.1016/j.ijfoodmicro.2018.09.005
123. Walencka E, Rózalska S, Sadowska B, Rózalska B. The influence of Lactobacillus acidophilus-derived surfactants on staphylococcal adhesion and biofilm formation. Folia Microbiol (Praha). (2008) 53:61–6. doi: 10.1007/s12223-008-0009-y
124. Vecino X, Rodríguez-López L, Ferreira D, Cruz JM, Moldes AB, Rodrigues LR. Bioactivity of glycolipopeptide cell-bound biosurfactants against skin pathogens. Int J Biol Macromol. (2018) 109:971–9. doi: 10.1016/j.ijbiomac.2017.11.088
125. Allonsius CN, Broek MFL, De Boeck I, Kiekens S, Oerlemans EFM, Kiekens F, et al. Interplay between Lactobacillus rhamnosus GG and Candida and the involvement of exopolysaccharides. Microb Biotechnol. (2017) 10:1753–63. doi: 10.1111/1751-7915.12799
126. Panpetch W, Hiengrach P, Nilgate S, Tumwasorn S, Somboonna N, Wilantho A, et al. Additional Candida albicans administration enhances the severity of dextran sulfate solution induced colitis mouse model through leaky gut-enhanced systemic inflammation and gut-dysbiosis but attenuated by Lactobacillus rhamnosus L34. Gut Microbes. (2020) 11:465–80. doi: 10.1080/19490976.2019.1662712
127. Authier H, Salon M, Rahabi M, Bertrand B, Blondeau C, Kuylle S, et al. Oral Administration of Lactobacillus helveticus LA401 and Lactobacillus gasseri LA806 combination attenuates oesophageal and gastrointestinal candidiasis and consequent gut inflammation in mice. J Fungi. (2021) 7:57. doi: 10.3390/jof7010057
128. Maekawa T, Ishijima SA, Ida M, Izumo T, Ono Y, Shibata H, et al. Prophylactic effect of Lactobacillus pentosus strain s-pt84 on Candida infection and gastric inflammation in a Murine gastrointestinal Candidiasis model. Med Mycol J. (2016) 57:E81–92. doi: 10.3314/mmj.16-00012E
129. Pereira Leão MV, Aureliano Tavares TA, Gonçalves e Silva CR, Ferreira dos Santos SS, Campos Junqueira J, Dias de Oliveira L, et al. Lactobacillus rhamnosus intake can prevent the development of Candidiasis. Clin Oral Investig. (2018) 22:2511–8. doi: 10.1007/s00784-018-2347-8
130. Jang SJ, Lee K, Kwon B, You HJ, Ko GP. Vaginal lactobacilli inhibit growth and hyphae formation of Candida albicans. Sci Rep. (2019) 9:1–9. doi: 10.1038/s41598-019-44579-4
131. Nishiyama K, Sugiyama M, Mukai T. Adhesion properties of lactic acid bacteria on intestinal mucin. Microorganisms. (2016) 4:34. doi: 10.3390/microorganisms4030034
132. Parolin C, Marangoni A, Laghi L, Foschi C, Ñahui Palomino RA, Calonghi N, et al. Isolation of vaginal lactobacilli and characterization of anti-candida activity. PLoS ONE. (2015) 10:e0131220. doi: 10.1371/journal.pone.0131220
133. De Gregorio PR, Parolin C, Abruzzo A, Luppi B, Protti M, Mercolini L, et al. Biosurfactant from vaginal Lactobacillus crispatus BC1 as a promising agent to interfere with Candida adhesion. Microb Cell Fact. (2020) 19:133. doi: 10.1186/s12934-020-01390-5
134. Kang CH, Kim YG, Han SH, Kim JS, Paek NS, So JS. In vitro probiotic properties of vaginal Lactobacillus fermentum MG901 and Lactobacillus plantarum MG989 against Candida albicans. Eur J Obstet Gynecol Reprod Biol. (2018) 228:232–7. doi: 10.1016/j.ejogrb.2018.07.005
135. Woo J, Ahn J. Probiotic-mediated competition, exclusion and displacement in biofilm formation by food-borne pathogens. Lett Appl Microbiol. (2013) 56:307–13. doi: 10.1111/lam.12051
136. Matsuda Y, Cho O, Sugita T, Ogishima D, Takeda S. Culture Supernatants of Lactobacillus gasseri and L. crispatus inhibit Candida albicans biofilm formation and adhesion to HeLa Cells. Mycopathologia. (2018) 183:691–700. doi: 10.1007/s11046-018-0259-4
137. Lebeer S, Vanderleyden J, De Keersmaecker SCJ. Host interactions of probiotic bacterial surface molecules: comparison with commensals and pathogens. Nat Rev Microbiol. (2010) 8:171–84. doi: 10.1038/nrmicro2297
138. Willing BP, Russell SL, Finlay BB. Shifting the balance: antibiotic effects on host-microbiota mutualism. Nat Rev Microbiol. (2011) 9:233–43. doi: 10.1038/nrmicro2536
139. Rizzo A, Losacco A, Carratelli CR. Lactobacillus crispatus modulates epithelial cell defense against Candida albicans through Toll-like receptors 2 and 4, interleukin 8 and human β-defensins 2 and 3. Immunol Lett. (2013) 156:102–9. doi: 10.1016/j.imlet.2013.08.013
140. Vylkova S, Nayyar N, Li W, Edgerton M. Human β-defensins kill Candida albicans in an energy-dependent and salt-sensitive manner without causing membrane disruption. Antimicrob Agents Chemother. (2007) 51:154–61. doi: 10.1128/AAC.00478-06
141. Tam JP, Wu C, Yang J-L. Membranolytic selectivity of cystine-stabilized cyclic protegrins. Eur J Biochem. (2000) 267:3289–300. doi: 10.1046/j.1432-1327.2000.01359.x
142. Rodrigues AG, Ping LY, Marcato PD, Alves OL, Silva MCP, Ruiz RC, et al. Biogenic antimicrobial silver nanoparticles produced by fungi. Appl Microbiol Biotechnol. (2013) 97:775–82. doi: 10.1007/s00253-012-4209-7
143. Barroso FAL, de Jesus LCL, de Castro CP, Batista VL, Ferreira Ê, Fernandes RS, et al. Article intake of Lactobacillus delbrueckii (Pexu:Hsp65) prevents the inflammation and the disorganization of the intestinal mucosa in a mouse model of mucositis. Microorganisms. (2021) 9:1–27. doi: 10.3390/microorganisms9010107
144. Villena J, Suzuki R, Fujie H, Chiba E, Takahashi T, Tomosada Y, et al. Immunobiotic Lactobacillus jensenii modulates the toll-like receptor 4-induced inflammatory response via negative regulation in porcine antigen-presenting cells. Clin Vaccine Immunol. (2012) 19:1038–53. doi: 10.1128/CVI.00199-12
145. Zelante T, Iannitti RG, Cunha C, De Luca A, Giovannini G, Pieraccini G, et al. Tryptophan catabolites from microbiota engage aryl hydrocarbon receptor and balance mucosal reactivity via interleukin-22. Immunity. (2013) 39:372–85. doi: 10.1016/j.immuni.2013.08.003
146. Li T, Liu Z, Zhang X, Chen X, Wang S. Local probiotic Lactobacillus crispatus and Lactobacillus delbrueckii exhibit strong antifungal effects against vulvovaginal candidiasis in a rat model. Front Microbiol. (2019) 10:1033. doi: 10.3389/fmicb.2019.01033
147. Hou Q, Ye L, Liu H, Huang L, Yang Q, Turner J, et al. Lactobacillus accelerates ISCs regeneration to protect the integrity of intestinal mucosa through activation of STAT3 signaling pathway induced by LPLs secretion of IL-22. Cell Death Differ. (2018) 25:1657–70. doi: 10.1038/s41418-018-0070-2
148. Macnaughtan J, Figorilli F, García-López E, Lu H, Jones H, Sawhney R, et al. A double-blind, randomized placebo-controlled trial of probiotic Lactobacillus casei shirota in stable cirrhotic patients. Nutrients. (2020) 12:1651. doi: 10.3390/nu12061651
149. Swidsinski A, Loening-Baucke V, Theissig F, Engelhardt H, Bengmark S, Koch S, et al. Comparative study of the intestinal mucus barrier in normal and inflamed colon. Gut. (2007) 56:343–50. doi: 10.1136/gut.2006.098160
150. Tsukida K, Takahashi T, Iida H, Kanmani P, Suda Y, Nochi T, et al. Immunoregulatory effects triggered by immunobiotic Lactobacillus jensenii TL2937 strain involve efficient phagocytosis in porcine antigen presenting cells. BMC Immunol. (2016) 17:21. doi: 10.1186/s12865-016-0160-1
151. Konstantinov SR, Smidt H, De Vos WM, Bruijns SCM, Singh SK, Valence F, et al. S layer protein A of Lactobacillus acidophilus NCFM regulates immature dendritic cell and T cell functions. Proc Natl Acad Sci USA. (2008) 105:19474–9. doi: 10.1073/pnas.0810305105
152. Fernandez EM, Valenti V, Rockel C, Hermann C, Pot B, Boneca IG, et al. Anti-inflammatory capacity of selected lactobacilli in experimental colitis is driven by NOD2-mediated recognition of a specific peptidoglycan-derived muropeptide. Gut. (2011) 60:1050–9. doi: 10.1136/gut.2010.232918
153. Niers LEM, Hoekstra MO, Timmerman HM, Van Uden NO, De Graaf PMA, Smits HH, et al. Selection of probiotic bacteria for prevention of allergic diseases: immunomodulation of neonatal dendritic cells. Clin Exp Immunol. (2007) 149:344–52. doi: 10.1111/j.1365-2249.2007.03421.x
154. Wagner RD, Dohnalek M, Hilty M, Vazquez-Torres A, Balish E. Effects of probiotic bacteria on humoral immunity to Candida albicans in immunodeficient bg/bg-nu/nu and bg/bg-nu/+ mice. Rev Iberoam Micol. (2000) 17:55–9. Available online at: http://www.reviberoammicol.com/2000-17/055059.pdf.
155. Tang C, Kamiya T, Liu Y, Kadoki M, Kakuta S, Oshima K, et al. Inhibition of dectin-1 signaling ameliorates colitis by inducing lactobacillus-mediated regulatory T cell expansion in the intestine. Cell Host Microbe. (2015) 18:183–97. doi: 10.1016/j.chom.2015.07.003
156. Kolling Y, Salva S, Villena J, Alvarez S. Are the immunomodulatory properties of Lactobacillus rhamnosus CRL1505 peptidoglycan common for all Lactobacilli during respiratory infection in malnourished mice? PLoS ONE. (2018) 13:e0194034. doi: 10.1371/journal.pone.0194034
157. Tenore S de B, Avelino-Silva VI, Costa PR, Franco LM, Sabino EC, Kalil J, et al. Immune effects of Lactobacillus casei shirota in treated HIV-infected patients with poor CD4+ T-cell recovery. AIDS. (2020) 34:381–9. doi: 10.1097/QAD.0000000000002420
158. Zou Y, Wang J, Wang Y, Peng B, Liu J, Zhang B, et al. Protection of galacto-oligosaccharide against E. coli O157 colonization through enhancing gut barrier function and modulating gut microbiota. Foods. (2020) 9:1710. doi: 10.3390/foods9111710
159. Mao X, Qiu X, Jiao C, Lu M, Zhao X, Li X, et al. Candida albicans SC5314 inhibits NLRP3/NLRP6 inflammasome expression and dampens human intestinal barrier activity in Caco-2 cell monolayer model. Cytokine. (2020) 126:154882. doi: 10.1016/j.cyto.2019.154882
160. Ohland CL, MacNaughton WK. Probiotic bacteria and intestinal epithelial barrier function. Am J Physiol Gastrointest Liver Physiol. (2010) 298:807–19. doi: 10.1152/ajpgi.00243.2009
161. Xie S, Zhao S, Jiang L, Lu L, Yang Q, Yu Q. Lactobacillus reuteri stimulates intestinal epithelial proliferation and induces differentiation into goblet cells in young chickens. J Agric Food Chem. (2019) 67:13758–66. doi: 10.1021/acs.jafc.9b06256
162. Rodrigues R, Guerra G, Soares J, Santos K, Rolim F, Assis P, et al. Lactobacillus rhamnosus EM1107 in goat milk matrix modulates intestinal inflammation involving NF-κB p65 and SOCs-1 in an acid-induced colitis model. J Funct Foods. (2018) 50:78–92. doi: 10.1016/j.jff.2018.09.013
163. Zhou X, Zhang K, Qi W, Zhou YJ, Hong T, Xiong T, et al. Exopolysaccharides from Lactobacillus plantarum NCU116 enhances colonic mucosal homeostasis by controlling epithelial cell differentiation and c-Jun/Muc2 signaling. J Agric Food Chem. (2019) 67:9831–9. doi: 10.1021/acs.jafc.9b03939
164. Yu Q, Yuan L, Deng J, Yang Q. Lactobacillus protects the integrity of intestinal epithelial barrier damaged by pathogenic bacteria. Front Cell Infect Microbiol. (2015) 5:26. doi: 10.3389/fcimb.2015.00026
165. Capaldo CT, Farkas AE, Hilgarth RS, Krug SM, Wolf MF, Benedik JK, et al. Proinflammatory cytokine-induced tight junction remodeling through dynamic self-assembly of claudins. Mol Biol Cell. (2014) 25:2710–9. doi: 10.1091/mbc.e14-02-0773
166. Wu H, Xie S, Miao J, Li Y, Wang Z, Wang M, et al. Lactobacillus reuteri maintains intestinal epithelial regeneration and repairs damaged intestinal mucosa. Gut Microbes. (2020) 11:997–1014. doi: 10.1080/19490976.2020.1734423
167. Laval L, Martin R, Natividad JN, F Chain FC, Miquel S, Desclée de Maredsous C, et al. Lactobacillus rhamnosus CNCM I-3690 and the commensal bacterium faecalibacterium prausnitzii A2-165 exhibit similar protective effects to induced barrier hyper-permeability in mice. Gut Microbes. (2015) 6:1–9. doi: 10.4161/19490976.2014.990784
168. McNair LKF, Siedler S, Vinther JMO, Hansen AM, Neves AR, Garrigues C, et al. Identification and characterization of a new antifungal peptide in fermented milk product containing bioprotective Lactobacillus cultures. FEMS Yeast Res. (2018) 18:94. doi: 10.1093/femsyr/foy094
169. Juarez Tomas MS, Wiese B, Nader-Macias ME. Effects of culture conditions on the growth and auto-aggregation ability of vaginal Lactobacillus johnsonii CRL 1294. J Appl Microbiol. (2005) 99:1383–91. doi: 10.1111/j.1365-2672.2005.02726.x
170. Maldonado-Barragán A, Caballero-Guerrero B, Lucena-Padrós H, Ruiz-Barba JL. Induction of bacteriocin production by coculture is widespread among plantaricin-producing Lactobacillus plantarum strains with different regulatory operons. Food Microbiol. (2013) 33:40–7. doi: 10.1016/j.fm.2012.08.009
171. Romani L, Zelante T, Palmieri M, Napolioni V, Picciolini M, Velardi A, et al. The cross-talk between opportunistic fungi and the mammalian host via microbiota's metabolism. Sem Immunopathol. (2015) 37:163–71. doi: 10.1007/s00281-014-0464-2
172. Lin XB, Wang T, Stothard P, Corander J, Wang J, Baines JF, et al. The evolution of ecological facilitation within mixed-species biofilms in the mouse gastrointestinal tract. ISME J. (2018) 12:1–15. doi: 10.1038/s41396-018-0211-0.
173. Todorov SD, Dicks LMT. Effect of medium components on bacteriocin production by Lactobacillus pentosus ST151BR, a strain isolated from beer produced by the fermentation of maize, barley and soy flour. World J Microbiol Biotechnol. (2004) 20:643–50. doi: 10.1023/B:WIBI.0000043196.09610.de
174. Assefa S, Ahles K, Bigelow S, Curtis JT, Köhler GA. Lactobacilli with probiotic potential in the prairie vole (Microtus ochrogaster). Gut Pathog. (2015) 7:35. doi: 10.1186/s13099-015-0082-0
175. Köhler GA, Assefa S, Reid G. Probiotic interference of Lactobacillus rhamnosus GR-1 and Lactobacillus reuteri RC-14 with the opportunistic fungal pathogen Candida albicans. Infect Dis Obstet Gynecol. (2012) 2012:1–14. doi: 10.1155/2012/636474
176. Gil NF, Martinez RCR, Gomes BC, Nomizo A, de Martinis ECP. Vaginal lactobacilli as potential probiotics against Candida spp. Brazilian J Microbiol. (2010) 41:6–14. doi: 10.1590/S1517-83822010000100002
177. Osset J, García E, Bartolomé RM, Andreu A. Role of Lactobacillus as protector against vaginal candidiasis. Med Clin (Barc). (2001) 117:285–8. doi: 10.1016/S0025-7753(01)72089-1
178. Tang H, Ren J, Yuan J, Zeng B, Wei H. An in vitro assessment of inhibitory effect of 16 strains of probiotics on the germination of Candida albicans. Afr J Microbiol Res. (2010) 4:1251–6. Available online at: https://academicjournals.org/journal/AJMR/article-full-text-pdf/2FA0F9D13580.
179. Khan R, Petersen FC, Shekhar S. Commensal bacteria: an emerging player in defense against respiratory pathogens. Front Immunol. (2019) 10:1203. doi: 10.3389/fimmu.2019.01203
180. Mathis KA, Bronstein JL. Our current understanding of commensalism. Annu Rev Ecol Evol Syst. (2020) 51:167–89. doi: 10.1146/annurev-ecolsys-011720-040844
181. Dekaboruah E, Suryavanshi MV, Chettri D, Verma AK. Human microbiome: an academic update on human body site specific surveillance and its possible role. Arch Microbiol. (2020) 202:2147–67. doi: 10.1007/s00203-020-01931-x
182. Chow J, Tang H, Mazmanian SK. Pathobionts of the gastrointestinal microbiota and inflammatory disease. Curr Opin Immunol. (2011) 23:473–80. doi: 10.1016/j.coi.2011.07.010
183. Balloux F, van Dorp L. Q&A: what are pathogens, and what have they done to and for us? BMC Biology. (2017) 15:91. doi: 10.1186/s12915-017-0433-z
184. Garcia JR, Gerardo NM. The symbiont side of symbiosis: do microbes really benefit? Front Microbiol. (2014) 5:510. doi: 10.3389/fmicb.2014.00510
185. Shapira M. Gut microbiotas and host evolution: scaling up symbiosis. Trends Ecol Evol. (2016) 31:539–49. doi: 10.1016/j.tree.2016.03.006
Glossary
Commensal: Refers to organisms that benefit from living within the host, while the host experiences no net effect. However, our understanding of microbial commensalism is limited. Also, commensal interactions may change under different host conditions [179–181].
Paraprobiotics: (Also known as “non-viable” or “inactivated” probiotics) refer to non-viable microbial cells that confer health benefits to the host. Paraprobiotics are usually consumed in diverse “healthy” food products or supplements [57].
Pathobionts: Commensal microorganisms that can become opportunistic pathogens associated with chronic inflammatory conditions. Pathobionts are not pathogenic to the host under normal conditions [182].
Pathogen: Is a microorganism that causes disease to its host. They are also called infectious agents, as they may cause infections [183].
Post-biotics: (Metabiotics, biogenics, or metabolites/CFS (cell-free supernatants) are soluble factors (metabolites) secreted by live bacteria or released after bacterial lysis. The bioactivity of these post-biotics offers health benefits to the host [57].
Probiotics: Live microorganisms that confer health benefits to the host. Probiotics are usually consumed in cultured milk and fermented foods.
Symbiosis: In the host-microbiota context, symbiosis encompasses different interactions, from commensalism to mutualistic (win-win) [184, 185].
Keywords: Lactobacillus, Candida, dysbiosis, microbiome, candidiasis, probiotics, microbiota
Citation: Vazquez-Munoz R and Dongari-Bagtzoglou A (2021) Anticandidal Activities by Lactobacillus Species: An Update on Mechanisms of Action. Front. Oral. Health 2:689382. doi: 10.3389/froh.2021.689382
Received: 31 March 2021; Accepted: 21 June 2021;
Published: 16 July 2021.
Edited by:
Gary Moran, Trinity College Dublin, IrelandReviewed by:
Antonio Pedro Ricomini Filho, State University of Campinas, BrazilGeorgios N. Belibasakis, Karolinska Institutet (KI), Sweden
Copyright © 2021 Vazquez-Munoz and Dongari-Bagtzoglou. This is an open-access article distributed under the terms of the Creative Commons Attribution License (CC BY). The use, distribution or reproduction in other forums is permitted, provided the original author(s) and the copyright owner(s) are credited and that the original publication in this journal is cited, in accordance with accepted academic practice. No use, distribution or reproduction is permitted which does not comply with these terms.
*Correspondence: Anna Dongari-Bagtzoglou, YWRvbmdhcmlAdWNoYy5lZHU=; Roberto Vazquez-Munoz, dmF6cXVlem11bm96QHVjaGMuZWR1