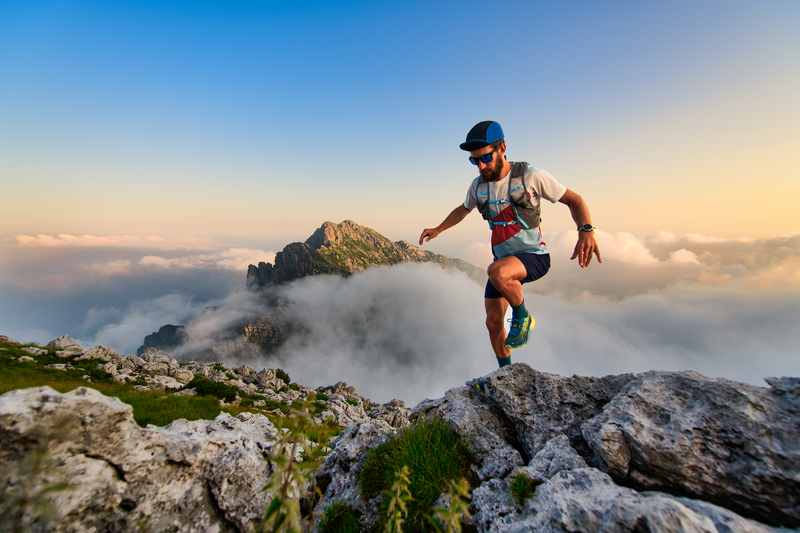
95% of researchers rate our articles as excellent or good
Learn more about the work of our research integrity team to safeguard the quality of each article we publish.
Find out more
REVIEW article
Front. Oncol. , 04 March 2025
Sec. Breast Cancer
Volume 15 - 2025 | https://doi.org/10.3389/fonc.2025.1551561
This article is part of the Research Topic Exploring the Breast Tumor Microenvironment: Association to Metastasis, Novel Risk Factors and Novel Treatments and Immunotherapies: Volume II. View all articles
Chemotherapy remains a central component of breast cancer treatment, significantly improving patient survival rates. However, its toxic side effects, along with cancer-related paraneoplastic syndromes, can lead to the loss of skeletal muscle mass and function, impairing physical abilities and increasing the risk of complications during treatment. Chemotherapeutic agents directly impact skeletal muscle cells by promoting protein degradation, inhibiting protein synthesis, and triggering systemic inflammation, all of which contribute to muscle atrophy. Additionally, these drugs can interfere with the proliferation and differentiation of stem cells, such as satellite cells, disrupting muscle regeneration and repair while inducing abnormal differentiation of intermuscular tissue, thereby worsening muscle wasting. These effects not only reduce the effectiveness of chemotherapy but also negatively affect patients’ quality of life and disease prognosis. Recent studies have emphasized the role of exercise as an effective non-pharmacological strategy for preventing muscle loss and preserving muscle mass in cancer patients. This review examines the clinical manifestations of muscle dysfunction following breast cancer chemotherapy, the potential mechanisms underlying these changes, and the evidence supporting exercise as a therapeutic approach for improving muscle function.
Chemotherapy is a key component of systemic therapy for breast cancer, particularly in metastatic cases (1). Administered either orally or intravenously, chemotherapy drugs circulate throughout the body, targeting and eliminating cancer cells at multiple sites. These drugs operate through various mechanisms, including the inhibition of DNA synthesis, induction of direct DNA damage, disruption of nucleic acid synthesis, interference with topoisomerase functions, and suppression of mitosis (2). These mechanisms enable chemotherapy to eliminate a broad range of cancer cells, highlighting its wide-ranging anti-cancer efficacy. However, chemotherapy drugs have limited selectivity between normal and malignant cells. While cancer cells proliferate more rapidly and are therefore more susceptible to these drugs (3), normal cells with high turnover rates—such as those in hair follicles, bone marrow, and the gastrointestinal tract—are also affected, leading to significant side effects (4). Commonly used chemotherapeutic agents in breast cancer treatment, including anthracyclines, taxanes, and platinum-based drugs, can cause cardiotoxicity, nephrotoxicity, fatigue, cachexia, muscle atrophy, leukopenia, neutropenia, anorexia, and other gastrointestinal complications (5). These adverse effects may, in part, stem from chemotherapy-induced inflammation. Inflammatory mediators play a critical role in carcinogenesis, as chronic inflammation promotes cellular mutations and proliferation, creating an environment conducive to cancer progression (6). While chemotherapy aims to eliminate cancer cells, it can also inadvertently activate signaling pathways such as nuclear factor kappa B (NF-κB) and mitogen-activated protein kinase (MAPK), leading to an increase in pro-inflammatory mediators (7). This response triggers systemic inflammation and causes collateral damage to normal tissues, exacerbating chemotherapy-related side effects. In some instances, the doses of chemotherapy drugs used in clinical settings may be insufficient to fully suppress tumor cell growth, potentially facilitating cancer progression or recurrence (1).
Skeletal muscle dysfunction is a common side effect of chemotherapy in breast cancer patients, significantly reducing quality of life and potentially contributing to cancer-related fatigue (8). Studies have demonstrated an inverse correlation between lean body mass and chemotherapy toxicity (9), suggesting that muscle atrophy may reduce patients’ tolerance to treatment. If left unchecked, this condition can progress to sarcopenia, characterized by the gradual loss of skeletal muscle mass, strength, and function. Some researchers have suggested that sarcopenia is exclusively age-related rather than a consequence of factors such as cancer (10). However, this perspective is not entirely accurate, as multiple studies have reported a high prevalence of sarcopenia among cancer patients (11, 12), which is associated with poor prognosis (13, 14). While sarcopenia shares features with aging and cachexia (15), all forms of the condition elevate the risk of falls, fractures, physical disability, and mortality (16), reduce survival (17), and negatively influence prognosis (18). Among breast cancer patients, sarcopenia prevalence ranges from 13.9% to 32.5% (19, 20), posing a serious threat to survivors’ long-term well-being. Despite its significant impact, muscle health in post-chemotherapy breast cancer management has received limited attention, with most research focusing on cardiotoxicity. Consequently, there is a lack of routine monitoring and intervention regarding body composition, including skeletal muscle mass and lean body mass.
Growing clinical evidence supports the safety and efficacy of physical exercise as an adjunctive therapy for cancer patients (7). Exercise has been shown to regulate inflammatory factors such as interleukin-6 (IL-6) and tumor necrosis factor-alpha (TNF-α), reducing cancer-related side effects and helping restore muscle mass homeostasis (21). Even in patients with established sarcopenia, exercise can partially counteract muscle loss (22), improve resistance to chemotherapy-related complications, and improve overall physical fitness and quality of life.
This review aims to examine the effects of chemotherapy on muscle health in breast cancer patients, with a focus on muscle mass, strength, and functional capacity. It will introduce assessment methods and biochemical markers for the early detection of skeletal muscle decline, facilitating timely clinical intervention. Furthermore, the cellular mechanisms underlying muscle dysfunction in breast cancer patients will be explored to identify physiological pathways and potential therapeutic targets for preventing muscle atrophy. Finally, the impact of exercise interventions on muscle function will be discussed, along with strategies for designing structured and personalized exercise programs to counteract chemotherapy-induced muscle loss.
Chemotherapy drugs have off-target effects, with 13.9% to 32.5% of breast cancer patients developing sarcopenia following treatment (19, 20). This condition is characterized by declines in both muscle mass and function. In clinical practice, various methods are used to assess muscle condition, depending on the specific needs of each case (Table 1). A thorough evaluation of these indicators provides insights into muscle volume, texture, and related factors, aiding in the diagnosis and monitoring of muscle deterioration.
As presented in Table 1 (Muscle Mass), multiple studies have reported significant reductions in pectoral muscle volume in breast cancer patients before and after chemotherapy (37, 38). However, muscle loss following chemotherapy does not necessarily occur uniformly across the body. Muscle biopsies from cancer patients have revealed that Type II muscle fibers are particularly susceptible to cancer-induced atrophy (66). A decline in the cross-sectional area of the vastus lateralis in breast cancer patients has been observed, paralleling the reduction in the cross-sectional area of Type II fibers (67). Interestingly, Sara Mijwel et al. noted a decrease in the proportion of Type I muscle fibers in breast cancer patients who were physically inactive during chemotherapy (68), suggesting that muscle loss may also be influenced by a reduction in overall physical activity during treatment (69, 70). Despite these findings, preclinical research on the specific types of muscle fiber atrophy induced by chemotherapy in breast cancer remains limited. Additionally, the loss of skeletal muscle mass is closely associated with poor clinical outcomes and reduced chemotherapy efficacy (71, 72), highlighting the need for further investigation into the mechanisms and patterns of muscle deterioration in these patients.
Traditional assessments of muscle loss, particularly in the context of cachexia, present several limitations due to the confounding effects of inflammation and edema. Cachexia is frequently associated with systemic inflammation and tissue edema, leading to fluctuations in body weight and reductions in muscle mass (73). However, some of these reductions may stem from fluid retention rather than actual muscle degradation. To accurately evaluate muscle quality, it is crucial to incorporate advanced imaging techniques such as MRI, CT, or ultrasound (74–76).
One key factor influencing muscle quality is the accumulation of intermuscular adipose tissue (IMAT), a mesenchymal tissue found between muscle fibers, which has been strongly linked to muscle dysfunction (77). IMAT consists of adipocytes interspersed between muscle fibers and fascicles (78), and excessive deposition of this fat can impair muscle strength and endurance (79, 80). Notably, a preclinical study demonstrated that IMAT accumulation, even in the presence of muscle atrophy, independently compromises muscle contraction (81). Two cross-sectional studies comparing breast cancer patients with healthy controls have reported increased IMAT content in the thighs, as assessed by MRI. Furthermore, recent research has identified IMAT as a significant prognostic factor for survival outcomes in breast cancer patients, emphasizing its clinical relevance (82).
Chemotherapy also affects bone health (83). While the direct impact of chemotherapy-induced bone loss on skeletal muscle function remains unclear, substantial evidence suggests a relationship between osteoporosis and muscle mass reduction (84). This interaction may be influenced by changes in mechanical loading, as decreased muscle mass leads to reduced stress on bones, contributing to bone loss and, eventually, osteoporosis (85). However, no studies have yet examined skeletal muscle changes at different tumor progression stages in preclinical models.
Table 1 (Motor Function) outlines various performance tests that serve as cost-effective alternatives to advanced imaging modalities for assessing skeletal muscle composition in cancer patients. Given the heterogeneity of clinical populations, establishing a universal threshold for sarcopenia remains challenging. Therefore, evaluating muscle function through performance-based tests holds substantial clinical value.
The impact of chemotherapy on muscle strength in breast cancer patients varies considerably. Some studies have reported no significant changes in the strength of large muscle groups, such as those in the upper and lower limbs (86), while others have documented notable reductions in grip strength and knee extensor strength (87). In addition to muscle strength, chemotherapy significantly affects exercise endurance in breast cancer patients (88). The 6-minute walk test (6MWT) is frequently used to assess endurance capacity (50), whereas maximal oxygen uptake (VO2 max), the gold standard for measuring exercise capacity, also shows a declining trend following chemotherapy (89). These findings indicate that chemotherapy may impair physical performance and endurance.
Adequate blood circulation is essential for skeletal muscle function, as it delivers oxygen and nutrients, removes metabolic waste, regulates temperature, and facilitates muscle repair and growth. Cardiopulmonary exercise testing is a valuable method for evaluating both cardiovascular endurance and muscle blood flow. Due to the cardiotoxic effects of chemotherapy drugs (90), chemotherapy-induced cardiopulmonary dysfunction appears to exacerbate cancer-related fatigue, particularly physical fatigue. This dysfunction may contribute to reduced exercise tolerance (91), further impairing overall motor function. While proper blood circulation is generally associated with stronger muscle performance, some studies have suggested that blood flow restriction (BFR) training can temporarily improve muscle strength and endurance (92, 93). However, the long-term effects of BFR interventions on overall patient health remain uncertain (94), necessitating further research to determine the safety and efficacy of such training over extended periods.
Over the years, biochemical markers for muscle mass assessment have been developed, offering valuable tools for the early detection and management of sarcopenia. Given the complexity of muscle wasting, relying on a single biomarker is often insufficient for an accurate diagnosis. Therefore, a combination of biochemical markers and clinical evaluations is typically necessary for a comprehensive assessment.
The serum creatinine-to-cystatin C ratio (CCR) and the Sarcopenia Index (SI), both derived from SCr and cystatin C (CysC), provide simple and objective measures for evaluating muscle mass. Abdominal CT scans, which quantify the paraspinal muscle area at the L4 level, are considered one of the gold standards for muscle mass assessment (95, 96). CCR correlates closely with paraspinal muscle mass, making it a reliable marker for estimating muscle volume (96). The SI, an extension of CCR, has been identified as a valuable biomarker for sarcopenia in cancer patients and is linked to postoperative complications and long-term survival outcomes (97, 98). Both CCR and SI exhibit a strong positive correlation with the appendicular skeletal muscle index (ASMI) and have demonstrated independent predictive value for sarcopenia in advanced cancer patients, helping to identify individuals who may benefit from targeted interventions (99). These biochemical markers offer a rapid and accessible approach for diagnosing sarcopenia, particularly in cases where kidney function remains stable.
The development of skeletal muscle dysfunction is closely associated with chemotherapy (45, 100). These conditions present a wide range of clinical manifestations (Figure 1) and significantly impact patients’ quality of life, making them a key risk factor for poor cancer prognosis (18). Despite progress in muscle mass assessment, accurately identifying muscle atrophy remains a challenge (101). Beyond the commonly observed clinical symptoms, further research is needed to develop more sensitive and specific clinical markers for detecting skeletal muscle changes and other body composition alterations during chemotherapy. Identifying high-risk patients at an early stage would allow timely intervention to slow or potentially reverse muscle loss, thereby improving both quality of life and overall prognosis.
Figure 1. Clinical manifestations of skeletal muscle dysregulation in breast cancer patients post-chemotherapy.
Muscle wasting in cancer patients can arise from the systemic cytotoxic effects of chemotherapy (67) or from tumor-secreted pro-inflammatory factors that disrupt skeletal muscle homeostasis by increasing proteolysis and suppressing protein synthesis (102). In the early stages of tumor development, inflammatory cells release pro-inflammatory cytokines that promote tumor growth, angiogenesis, and invasion. However, as the tumor advances, the composition of infiltrating immune cells may shift, with an increased presence of infiltrating lymphocytes (103). These lymphocytes play a crucial role in recognizing and eliminating tumor cells, and their presence is strongly associated with patient prognosis and response to immunotherapy. Despite these insights, the relationship between skeletal muscle inflammation, cancer progression, and chemotherapy remains poorly understood. A deeper understanding of these cellular alterations could help identify new therapeutic targets, potentially improving cancer treatment strategies.
Research on muscle dysfunction in breast cancer patients has highlighted mitochondrial alterations as a key concern, particularly in response to chemotherapy. RNA sequencing (RNA-seq) studies have identified significant dysregulation of genes involved in mitochondrial function and oxidative phosphorylation in breast cancer patients (104, 105). Mitochondria, which serve as the primary energy-generating organelles, typically make up 2% to 7% of muscle cell volume (106). Chemotherapy has been shown to reduce mitochondrial numbers, as indicated by decreased citrate synthase activity (68) and direct observations from muscle biopsies, likely due to the mitotic toxicity of anticancer drugs (67). Additionally, muscle biopsies from chemotherapy-treated patients have revealed lower levels of PGC-1α protein, suggesting a decline in mitochondrial biogenesis (107).
Preclinical studies have demonstrated that doxorubicin (DOX) inhibits mitochondrial respiration (108, 109), leading to mitochondrial dysfunction. Fragmented and damaged mitochondria accumulate in skeletal muscle, reducing bioenergetic efficiency (107) and contributing to muscle weakness. Furthermore, excessive production of reactive oxygen species (ROS) (108, 110) damages healthy mitochondria (111), triggering oxidative stress at the cellular level. This oxidative stress activates protein degradation pathways, including caspase-3 and the ubiquitin-proteasome system, accelerating muscle protein breakdown (110). Additionally, increased expression of the apoptosis-related protein Bax suggests that chemotherapy-induced mitochondrial damage may lead to apoptosis, further exacerbating muscle wasting (107).
Mitochondrial network dynamics—including biogenesis, fusion, fission, and fragmentation—play a critical role in regulating muscle mass through their influence on key signaling pathways (112). Disruptions in mitochondrial fission alone are sufficient to impair organelle function and activate AMPK, ultimately leading to muscle wasting in adult animals (113). A study examining the effects of doxorubicin (DOX) on myocardial mitochondria in rats found that DOX treatment reduced the expression of fusion-related proteins (Mfn1, Mfn2, OPA1) while increasing the expression of the fission-related protein DRP1 (114). Similar findings have been reported in chemotherapy-treated breast cancer patients, where reductions in mitochondrial membrane fusion markers OPA1 (115) and Mfn2 (107) suggest a decline in mitochondrial fusion capacity. The imbalance between reduced fusion and increased fission promotes mitochondrial fragmentation (107).
Under normal physiological conditions, damaged mitochondria undergo fission and are subsequently cleared through mitophagy. However, chemotherapy appears to impair this process, as evidenced by decreased levels of PINK1 protein (115) and Parkin ubiquitin ligase (108), which may lead to the accumulation of damaged and fragmented mitochondria. This accumulation results in the excessive release of ROS and pro-apoptotic factors, further damaging muscle fibers and contributing to muscle loss (112). Additionally, mitochondrial fragmentation can activate the AMPK-FoxO3 axis, driving the expression of atrophy-related genes, accelerating protein degradation, and worsening muscle wasting (113).
The regulation of myofibers is a dynamic process in which the balance between protein synthesis and degradation plays a crucial role. Skeletal muscle protein synthesis is primarily driven by the PI3K-Akt-mTOR pathway (116). Both clinical and preclinical studies have shown a decline in muscle protein synthesis in cancer patients, particularly in rodent models treated with DOX, where a reduction in PI3K-Akt-mTOR signaling has been observed (117). Protein degradation in skeletal muscle occurs through two major pathways: the ubiquitin-proteasome system (UPS) and the autophagy-lysosome pathway (ALP). Both pathways are regulated by FoxO transcription factors, whose excessive activation leads to significant muscle wasting (118), a key process in DOX-induced myotoxic proteolysis (119–121).
The UPS is a central protein degradation system in eukaryotic cells and plays a fundamental role in muscle atrophy (122). Increased UPS activity has been reported in cancer patients (123). In mice treated with DOX, elevated expression of FoxO1 and FoxO3 mRNA has been noted, along with increased transcription of FoxO target genes, including MAFbx/Atrogin-1 and MuRF-1 (121). The E3 ubiquitin ligases MAFbx/Atrogin-1 and MuRF-1, predominantly expressed in skeletal muscle, mediate the polyubiquitination of proteins, marking them for degradation by the 26S proteasome (124). Elevated levels of MAFbx/Atrogin-1 and MuRF-1 have been associated with muscle-wasting conditions, including cancer (125). These ligases and the subsequent proteolysis are typically upregulated when the IGF1-AKT growth-promoting pathway is inhibited (126, 127). FoxO transcription factors are key downstream targets of AKT and play a central role in regulating the expression of Atrogin-1 while driving atrophy-related pathways (126). Activation of Akt/PKB suppresses FoxO3 activity (128). In models of muscle atrophy, reduced Akt pathway activity leads to nuclear accumulation of FoxO proteins, promoting the expression of Atrogin-1/MAFbx and MuRF1, thereby accelerating protein degradation (126, 127, 129). Beyond its role in protein degradation, FoxO also influences protein synthesis. When Akt signaling is suppressed, FoxO activation downregulates mTOR, further inhibiting protein synthesis (130).
Autophagy in muscle cells contributes to increased proteolysis in cancer patients, functioning as a proteolytic mechanism activated by oxidative stress. While autophagy is essential for maintaining muscle mass by degrading damaged or aggregated proteins and facilitating baseline protein turnover (131), a more precise understanding of changes in autophagic flux is needed. Disruptions in autophagy have been linked to myofiber degeneration and atrophy, characterized by the accumulation of dysfunctional mitochondria and inclusion bodies, as seen in muscle diseases (118). The expression of autophagy-related genes (ATG), including LC3, GABARAP, and BNIP3, is regulated by FoxO3 (128). In C2C12 myotubes, FoxO3-driven proteolysis is predominantly lysosome-dependent (132). Studies have shown that DOX administration increases the expression of autophagy genes in skeletal muscle, whereas endurance exercise may provide a protective effect against DOX-induced autophagic activation (119).
Beyond these pathways, protein degradation is also influenced by fluctuations in intracellular calcium ion concentrations and the activity of inflammatory cytokines such as TNF-α. As previously noted, DOX can increase mitochondrial ROS production, which damages calcium-regulating proteins and leads to elevated intracellular calcium levels. This rise in calcium activates autophagy through calcium/calmodulin-dependent protein kinase kinase (CAMKK) and AMP-activated protein kinase (AMPK) (133, 134). In rats treated with DOX, increased oxidative stress and increased activation of proteases such as calpains and caspase-3 have been observed in skeletal muscle. However, exercise has been shown to prevent DOX-induced oxidative damage and protease activation in trained muscles (120).
Inflammatory factors also play a significant role in the proteolytic process. Activation of NF-κB has been shown to upregulate MAFbx/Atrogin-1 and MuRF1 expression in muscle atrophy models (135). Tumor-related inflammatory cytokines, such as TNF-α and IL-6, contribute to skeletal muscle atrophy by promoting protein degradation through activation of the NF-κB and UPS pathways (136, 137). In rodent models, DOX treatment has been found to elevate TNF-α levels, leading to muscle contractile dysfunction through the TNF receptor subtype TNFR1 (138).
The regenerative capacity of skeletal muscle is a critical factor in maintaining its function, particularly after injury (139). Successful muscle regeneration is a complex and highly coordinated process involving multiple cell types, with satellite cells (SCs) playing a central role (141). SCs are located at the myotendinous junction and beneath the basal lamina of muscle fibers (140). Studies have shown that impaired regeneration in mouse skeletal muscle is linked to insufficient SC activation and proliferation, as well as a progressive depletion of the SC pool with aging (142). In cases of muscle atrophy, muscle fibers become increasingly fragile and undergo continuous cycles of degeneration, inflammation, and impaired regeneration (143). These signs indicate persistent skeletal muscle damage, potentially leading to prolonged degeneration-regeneration cycles. The high metabolic demands of tumor proliferation can create energy shortages in non-cancerous tissues, which may alter protein turnover rates (144, 145). Unlike traditional muscle atrophy, which results primarily from protein turnover imbalances, cancer-associated muscle wasting may stem from reduced muscle repair and regeneration. Specifically, while SCs may proliferate in the cancerous environment, they often fail to differentiate properly (146), resulting in impaired muscle regeneration. Regarding the impact of chemotherapy, studies have reported that long-term DOX treatment reduces SC content and capillary density in rat skeletal muscle (147), suggesting that chemotherapy may suppress myogenic differentiation. The combined effects of cancer and chemotherapy may lead to SC dysfunction and loss in breast cancer patients, further impairing muscle regeneration. Additional research is needed to clarify how SC evolution is affected in these patients.
Fibrosis within skeletal muscle (148) and the abnormal deposition of IMAT are strong indicators of impaired muscle regeneration and pathological hallmarks of sarcopenia, contributing to muscle dysfunction (81). An increase in IMAT has been reported in breast cancer patients (115). While muscle wasting and elevated IMAT levels have been observed in chemotherapy-treated cancer models (149), research on the cellular mechanisms underlying IMAT formation and the effects of commonly used chemotherapy drugs remains limited. Fibro-adipogenic progenitor cells (FAPs) play a crucial role in skeletal muscle regeneration by maintaining tissue homeostasis and assisting SCs in responding to minor injuries (150, 151). In healthy muscle, SCs are activated at the site of damage, while FAPs proliferate to support muscle repair. These FAPs contribute to SC expansion by providing transient differentiation signals, creating an environment that enhances myogenic differentiation and facilitates muscle regeneration (152). However, under conditions of muscle disuse or disease, FAPs can differentiate into adipose or fibrotic tissue, a process widely recognized as a key contributor to IMAT accumulation (153, 154). In chemotherapy-treated breast cancer patients, studies have observed that FAPs preferentially differentiate into adipose rather than fibrotic tissue (155). In the context of skeletal muscle atrophy, research suggests that FAPs drive muscle wasting through IL-6/STAT3 signaling, and inhibiting this pathway has been shown to effectively counteract muscle atrophy and fibrosis (156). Given the dual role of FAPs in IMAT formation and muscle wasting, further studies should investigate their behavior in preclinical and clinical models of cancer-related skeletal muscle deterioration to determine their potential as therapeutic targets.
The endoplasmic reticulum (ER) is responsible for protein synthesis, folding, and modification. In the abnormal tumor microenvironment, sustained ER stress can occur in tumor cells, leading to an accumulation of misfolded or unfolded proteins (157). This accumulation activates the unfolded protein response (UPR) (158), which is thought to contribute to paraneoplastic syndromes (159). Under moderate stress conditions, the UPR acts as a protective mechanism, facilitating protein clearance through three primary pathways: the PERK-eIF2α-ATF4, IRE1α-XBP1, and ATF6-ATF6N signaling axes. These pathways upregulate genes that promote cell survival and restore cellular homeostasis. However, when ER stress is prolonged, the UPR shifts from a pro-survival response to an apoptotic signal (160).
In skeletal muscle, the UPR contributes to muscle atrophy by reducing protein synthesis, increasing protein degradation, and promoting apoptosis. Persistent UPR activation has been observed in atrophied skeletal muscle (161). This activation is regulated by E3 ubiquitin ligases such as MuRF1 and MAFbx/Atrogin-1, which facilitate protein degradation in muscle tissue (162). Among the UPR signaling pathways, PERK plays a critical role in muscle atrophy. In immobilization-induced muscle wasting models, increased expression of atrogin-1, p-PERK, and Parkin proteins, along with reduced COXIV protein levels, have been observed. Partial improvements in these markers have been reported following electrical stimulation therapy (163). Preclinical studies indicate that PERK is essential for maintaining skeletal muscle mass and function in adult mice. Deletion of PERK in tumor-bearing mice has been shown to worsen muscle atrophy, with increased activation of ubiquitin-proteasome and autophagy pathways in skeletal muscle (164).
Myokines are cytokines secreted by active muscles (165), exerting autocrine, paracrine, or endocrine effects (166) that contribute to muscle function and homeostasis. Various forms of exercise, particularly resistance training, have been shown to stimulate the release of myokines, which play crucial roles in anti-inflammatory, metabolic, and immune regulation (167). In cancer patients, selective atrophy of Type II muscle fibers has been observed, often accompanied by a shift from fast-twitch to slow-twitch fibers (66). DOX has also been reported to alter Type II muscle fiber composition, though endurance exercise appears to counteract these effects and provide skeletal muscle protection in mice (168). During physical activity, the secretion of myokines in response to muscle contractions is essential for preserving skeletal muscle mass (169).
Interleukin-6 (IL-6) is one of the most studied myokines, with its levels rising significantly in skeletal muscle following exercise (170). This multifunctional cytokine activates or regulates several key signaling pathways, including JAK/STAT, p38/MAPK, and NF-κB (171). IL-6 promotes SC proliferation, differentiation, and fusion (172), playing a role in muscle regeneration and contributing to skeletal muscle protein synthesis and hypertrophy. In addition to its direct effects on SCs, IL-6 promotes cell proliferation via paracrine signaling (173). However, while low IL-6 levels facilitate SC activation and muscle fiber regeneration, persistently high IL-6 levels contribute to skeletal muscle atrophy (172), which may partly explain the muscle wasting seen in cancer. As a potent pro-inflammatory cytokine, IL-6 is overexpressed in various cancers, including breast cancer (174), where abnormal activation of the IL-6/JAK/STAT3 pathway has been documented (175). Chemotherapy drugs can further activate NF-κB signaling, inducing IL-6 expression in both tumor and stromal cells (176). Studies have shown that prolonged treatment with IL-6 receptor (IL-6R) antibodies can block IL-6 signaling and reduce muscle atrophy in tumor-bearing mice, suggesting that anti-IL-6 receptor antibodies could have therapeutic potential in addressing cancer-associated muscle wasting (177). Additionally, IL-6 has been implicated in disrupting the growth hormone/insulin-like growth factor-1 (GH/IGF-1) axis, which is critical for muscle growth (178). Research has demonstrated that short-term IL-6 administration in wild-type mice (179) and humans (180) reduces circulating IGF-1 levels, thereby downregulating IGF-1/PI3K/AKT signaling, a key pathway for skeletal muscle hypertrophy.
Insulin-like growth factor 1 (IGF-1) plays a crucial role in muscle hypertrophy by activating the IGF-1/Akt/mTOR signaling pathway, which is essential for muscle growth and repair (181). However, in various forms of muscle atrophy, IGF-1 expression is downregulated, often occurring before the onset of cachexia (182). Chronic inflammation suppresses the hypothalamic GH-IGF-1 axis, leading to reduced circulating IGF-1 levels, increased protein breakdown, and the development of skeletal muscle atrophy and cachexia (183). Beyond its role in muscle maintenance, IGF-1 is vital for myogenesis, as it promotes myoblast proliferation and differentiation (184). It regulates the cell cycle of SCs and extends their replicative lifespan in vitro. Studies conducted in vivo further highlight the importance of IGF-1 signaling in SC function. Transgenic mice with skeletal muscle-specific IGF-1 overexpression exhibit increased muscle mass and strength, which correlate with enhanced SC activation and regeneration (185). In contrast, IGF-1 receptor knockout mice show impaired satellite cell function and reduced muscle regeneration (186). Exercise has been shown to activate the IGF-1/IGF-1R-PI3K/Akt signaling pathway, increasing the expression of muscle regulatory factors and promoting protein synthesis while suppressing protein degradation and apoptosis. This mechanism plays a protective role against skeletal muscle atrophy (187). In breast cancer patients undergoing chemotherapy, resistance training has been found to elevate serum IGF-1 levels, improve lean body mass, and improve muscle strength in both the upper and lower limbs. These findings suggest that targeting the IGF-1 signaling pathway may offer a promising approach for counteracting muscle atrophy and promoting muscle hypertrophy.
Myostatin, also known as growth differentiation factor 8 (GDF-8), is a member of the TGF-β family primarily expressed and secreted by skeletal muscle. It plays a critical role in regulating muscle development and adaptation in adulthood (188). As a negative regulator of muscle growth (189), myostatin inhibits protein synthesis through the myostatin-Smad2/3 signaling pathway (116). It binds to the ActRIIB receptor on the cell membrane (190), initiating the phosphorylation of Smad proteins and activating downstream intracellular signaling cascades (191, 192). By suppressing AKT phosphorylation—a key driver of skeletal muscle hypertrophy—myostatin counteracts the IGF-1/PI3K/AKT hypertrophic pathway, leading to an increase in active FoxO1 levels. This promotes the expression of atrophy-related genes such as atrogin-1, MuRF-1, and E214k (193). Additionally, Smad3 has been shown to enhance FoxO-induced expression of muscle-specific ubiquitin ligases, Atrogin-1 and MuRF-1, further accelerating muscle degradation (194). Myostatin is expressed in various tumor cell lines in both mice and humans (195), and chemotherapy has been reported to upregulate its expression, promoting catabolism and muscle atrophy (196). Research has demonstrated that systemic overexpression of myostatin in adult mice induces severe muscle and fat loss, resembling the cachexia observed in human cancer patients (197). In contrast, pharmacological inhibition of myostatin has been shown to prevent muscle loss and prolong survival (190). Clinical trials using anti-myostatin antibodies have reported increases in muscle mass and lean body mass in cancer patients with sarcopenia (198), along with improvements in functional performance (199). Additionally, alternative myostatin-targeting approaches, such as the myostatin-blocking peptide PINTA 745, have demonstrated similar benefits, increasing muscle mass and improving function in models of stroke and chronic kidney disease (200, 201).
The cellular mechanisms underlying skeletal muscle dysfunction involve multiple interconnected pathophysiological processes (Figure 2). Chemotherapy disrupts mitochondrial homeostasis in skeletal muscle, leading to oxidative stress and impaired energy metabolism. It stimulates the secretion of inflammatory cytokines, triggering systemic inflammation, and interferes with key signaling pathways, including IGF-PI3K-AKT-mTOR, IL-6-JAK-STAT3, NF-κB, and MAPK. These disruptions affect the balance between muscle protein synthesis and degradation, as well as cellular autophagy. Consequently, atrophy-related genes such as atrogin-1 and MuRF-1 are upregulated, promoting protein degradation and apoptosis, ultimately compromising skeletal muscle function. In addition to its impact on protein homeostasis, chemotherapy also affects skeletal muscle cell evolution by impairing the proliferation and differentiation of endogenous stem cells. This results in abnormal muscle regeneration, defective repair, and altered intermuscular tissue differentiation, further exacerbating muscle loss. The equilibrium between protein synthesis and degradation is a fundamental mechanism in maintaining muscle mass and is regulated by complex molecular and cellular interactions. While some studies have investigated the effects of doxorubicin (DOX) on muscle, particularly cardiac muscle, further research is required to better understand skeletal muscle protein degradation in breast cancer models. This knowledge could contribute to the development of targeted interventions aimed at preserving muscle health in cancer patients.
Figure 2. Potential cellular mechanisms of skeletal muscle dysregulation in breast cancer patients post-chemotherapy.
Chemotherapy for breast cancer frequently leads to anorexia and reduced physical activity in patients (202), while also directly accelerating muscle protein degradation. Approximately 13.9%–32.5% of patients develop sarcopenia, which negatively impacts both quality of life and tolerance to treatment (19, 20). Given these consequences, effective prevention and intervention strategies are essential. Current treatments for skeletal muscle atrophy include physical exercise, nutritional supplementation, and pharmacological therapies, although no specific drug has yet been approved for clinical use (203). Preclinical studies suggest that exercise can partially counteract cisplatin-induced muscle atrophy and restore normal food intake in mice, potentially through the regulation of appetite-related hormones such as ghrelin (204). Clinical research further supports that increasing physical activity during and after breast cancer chemotherapy improves dietary intake and improves overall quality of life (205). While nutritional therapy alone may not be sufficient to significantly increase muscle mass (206, 207) and typically produces slower effects (208), its combination with exercise has been shown to enhance exercise capacity, facilitate muscle adaptation to training, and reduce muscle atrophy (209, 210).
We reviewed research on the mechanisms by which exercise helps counteract muscle dysregulation associated with breast cancer (Table 2). The findings suggest that exercise plays a vital role in maintaining muscle homeostasis by improving mitochondrial function, reducing abnormal inflammatory responses, normalizing dysregulated myokine levels, and modulating the HPA axis. These processes work together to promote protein synthesis and protect skeletal muscle.
Table 2. Research on the mechanisms of exercise in counteracting breast cancer-related muscle dysregulation.
Exercise has been shown to combat systemic chronic inflammation, enhance mitochondrial function, promote satellite cell-mediated repair of damaged skeletal muscle, and stimulate protein synthesis. These effects collectively help reduce skeletal muscle atrophy in breast cancer patients after chemotherapy. Physical activity, especially resistance training, has been proven to significantly increase lean body mass (224, 225). While an increase in lean mass can counteract some of the muscle loss, cancer patients generally still have lower muscle mass compared to healthy individuals. Therefore, the goal of exercise should focus on maintaining muscle mass rather than significantly increasing it, tailored to the patient’s overall health status (226). Studies also suggest that high-intensity resistance training can enhance natural killer cell activity (NKCA) and improve immune cell function (212), implying that exercise may strengthen the immune system, which could help the patient better cope with the adverse effects of cancer and its treatments.
In the early stages of treatment, exercise training was often discouraged due to concerns about the physical toll of chemotherapy. However, with growing evidence supporting the benefits of exercise therapy, this perspective has shifted. Increasing research suggests that exercise not only reduces treatment-related toxicity but may also enhance the effectiveness of conventional cancer therapies (227), reduce the risk of tumor recurrence and metastasis (7), and improve overall quality of life (228). As a result, exercise has become an integral component of supportive care for cancer patients, showing promise in preserving skeletal muscle mass (229, 230). Exercise programs typically include a combination of aerobic training, resistance exercises, and balance training, tailored to the patient’s specific condition and treatment stage. A review of multiple studies supports these findings (Table 3).
Exercise interventions have demonstrated significant benefits for breast cancer patients. Early studies suggest that engaging in physical activity can positively influence long-term disease-free survival and quality of life (QoL) outcomes (231). Compared to patients receiving standard care, those who incorporate exercise into their routine exhibit improved cardiopulmonary function, grip strength, and muscle strength in the chest, legs, and other muscle groups. Additionally, exercise has been linked to favorable changes in body composition, metabolic parameters, and muscle strength, along with higher patient-reported QoL scores. Regarding chemotherapy-related side effects, patients in exercise groups report less severe gastrointestinal disturbances and lower rates of myelosuppression compared to control groups. A recent study highlighted the added benefits of combining exercise with kinesiology taping (KT), showing greater improvements in muscle strength and QoL than exercise alone. These findings suggest that KT may serve as a non-invasive adjunct to breast cancer treatment, aiding recovery (240). Resistance training (RT) has also been investigated for its impact on pain management in breast cancer survivors. One study found that RT significantly improved one-repetition maximum strength (1RM) and pain pressure threshold (PPT) in patients experiencing persistent pain post-treatment. Notably, while strength levels remained stable after the cessation of training, PPT did not, suggesting that the analgesic effects of RT were linked to the training process itself rather than strength gains alone (241).
Exercise has been shown to improve muscle mass and function in cancer survivors, counteracting chemotherapy-induced toxicities and cancer-related muscle wasting. It may even reverse sarcopenia, significantly enhancing physical capacity, endurance, and QoL. Additionally, exercise has been associated with better chemotherapy tolerance, improved disease prognosis, and potentially extended survival (Table 4).
Regular exercise has been demonstrated to enhance muscle strength and physical function in breast cancer patients undergoing chemotherapy, helping to slow or prevent further muscle loss and sarcopenia. Furthermore, even when introduced later in treatment, exercise interventions provide measurable health benefits and improve clinical outcomes. A study comparing different supervised exercise programs in obese breast cancer patients with sarcopenia undergoing adjuvant chemotherapy found that, after 17 weeks, resistance exercise (R.E.) yielded greater improvements in muscle mass and strength compared to usual care or aerobic exercise. Notably, 26.2% of participants experienced a reversal of sarcopenia, along with improvements in self-reported QoL, fatigue, and anemia (22). In addition, a combined aerobic and resistance training program performed three times per week for four months in obese breast cancer survivors with sarcopenia resulted in significant increases in the appendicular skeletal muscle mass index (ASMI). It also led to reductions in obesity-related parameters, including BMI, body weight, waist circumference, and body fat percentage, effectively improving the muscle-wasting phenotype associated with obesity (242).
Most cancer patients exhibit good tolerance to supervised exercise programs, highlighting their feasibility even in cases of sarcopenia onset. Long-term unsupervised aerobic exercise has also been shown to positively influence muscle mass, as seen in prehabilitation programs for colorectal cancer patients (244). However, supervised exercise appears to be more effective for cancer patients with sarcopenia, ensuring optimal health outcomes (245). For maximal benefit, patients should be referred to supervised exercise programs. Resistance training, in particular, has been found to be more effective in reversing sarcopenia than other exercise modalities (246–249). Among various training regimens, moderate- to high-intensity combined aerobic and resistance training has consistently shown the greatest efficacy in improving muscle mass and overall physical function (250).
Current guidelines from the American College of Sports Medicine (ACSM) (251) and the American Society of Clinical Oncology (ASCO) (252) recommend exercise both during and after cancer treatment. As previously discussed, exercise during chemotherapy provides substantial short-term benefits for patients. However, long-term follow-up studies have not demonstrated sustained improvements in fatigue reduction or the maintenance of physical function, suggesting that the benefits of exercise during treatment may decrease over time (253, 254). Despite the advantages of exercise, not all patients are suitable candidates for structured physical activity. Bedridden or severely weakened individuals may not tolerate exercise therapy, and those with advanced muscle atrophy or nerve damage may derive only minimal benefits (255, 256). Nevertheless, cancer patients are generally encouraged to increase their physical activity levels throughout and beyond treatment. Engaging in various forms of movement, even at low intensity, is preferable to complete inactivity.
Chemotherapy remains a cornerstone of breast cancer treatment, significantly improving survival rates. However, its side effects, particularly muscle atrophy, present major challenges. Chemotherapy drugs disrupt mitochondrial homeostasis, leading to oxidative stress and impairing the balance between muscle protein synthesis and degradation. These effects are mediated through key signaling pathways, including IGF-PI3K-AKT-mTOR, IL-6-JAK-STAT3, and TNF-α-MAPK, as well as disruptions in autophagy regulation. Among commonly used chemotherapeutic agents, the myotoxicity of anthracyclines such as DOX has been extensively studied. DOX has been shown to increase ROS production in muscle fibers, induce oxidative damage, and activate proteolytic pathways, including calpains and caspase-3 (119). These mechanisms, coupled with alterations in inflammatory factors, impact satellite cells, skeletal muscle fibers, and intermuscular tissues, leading to fatigue, reduced physical function, and muscle atrophy. Despite substantial research on muscle dysfunction mechanisms, the processes underlying chemotherapy-induced muscle deterioration in breast cancer patients remain poorly understood.
The loss of skeletal muscle during chemotherapy is associated with poor prognosis in breast cancer patients (71, 72). Various assessment tools, including dynamometers, anthropometric measurements, and body composition analysis methods such as DEXA, BIA, and MRI, provide valuable diagnostic insights. However, unlike the well-defined criteria for age-related sarcopenia (16), there is no standardized diagnostic framework for chemotherapy-induced sarcopenia, particularly in breast cancer patients. Given the clinical significance of muscle loss in different contexts, a precise assessment of muscle wasting following chemotherapy is essential rather than relying on broad estimations (257). Improved diagnostic accuracy will allow for more targeted treatment strategies and better prognostic evaluations.
Exercise, as a key non-pharmacological intervention, plays an essential role in modern medical practice. It has been shown to enhance the effectiveness of chemotherapy while reducing the risk of recurrence and cancer-related mortality (7). Regarding muscle health, numerous studies suggest that exercise lowers systemic inflammation, promotes protein synthesis, and improves muscle quality and function in cancer survivors. It helps counteract chemotherapy-induced muscle atrophy and cancer cachexia and may even reverse sarcopenia. Although exercise is widely regarded as the most effective strategy for addressing skeletal muscle atrophy, it is not suitable for all patients (203). Exercise prescriptions must be tailored to individual needs, considering factors such as disease progression, treatment stage, and overall physical condition. Therefore, it is crucial to investigate how exercise modulates inflammatory responses and oxidative stress to protect muscle cells, as well as how it promotes protein synthesis and enhances mitochondrial function to improve muscle fiber strength and endurance. Integrating exercise therapy with molecular research can provide deeper insights into these mechanisms, paving the way for more personalized and scientifically based treatment strategies for breast cancer patients. In conclusion, exercise stands out as a highly effective non-pharmacological approach for addressing muscle dysfunction in breast cancer patients. Further research and development in this area are essential.
PZ: Conceptualization, Funding acquisition, Investigation, Project administration, Writing – original draft, Writing – review & editing. XL: Investigation, Visualization, Writing – original draft, Writing – review & editing. JL: Project administration, Supervision, Writing – review & editing.
The author(s) declare that no financial support was received for the research, authorship, and/or publication of this article.
The authors declare that the research was conducted in the absence of any commercial or financial relationships that could be construed as a potential conflict of interest.
The author(s) declare that no Generative AI was used in the creation of this manuscript.
All claims expressed in this article are solely those of the authors and do not necessarily represent those of their affiliated organizations, or those of the publisher, the editors and the reviewers. Any product that may be evaluated in this article, or claim that may be made by its manufacturer, is not guaranteed or endorsed by the publisher.
NF-κB, nuclear factor kappa B; MAPK, mitogen-activated protein kinase; IL-6, Interleukin-6; TNF-α, tumor necrosis factor-alpha; DEXA, dual-energy X-ray absorptiometry; BIA, bioelectrical impedance analysis; TUG test, Timed Up and Go; CST, Chair Stand test; STS test, Sit-to-Stand; MRI, magnetic resonance imaging; CT, computed tomography; pQCT, Peripheral quantitative computed tomography; SCr, serum creatinine; IMAT, intermuscular adipose tissue; K, Potassium; TBK, total body potassium; D3-Cr, deuterium-labeled creatine; CCR, serum creatinine-to-cystatin C ratio; SI, Sarcopenia Index; CysC, Cystatin C; GFR, glomerular filtration rate; ASM, appendicular skeletal muscle mass; ASMI, appendicular skeletal muscle index; P3NP/PIIINP, Type III procollagen N-terminal peptide; ELISA, Enzyme-Linked Immunosorbent Assay; RIA, Radioimmunoassay; ECLIA, Electrochemiluminescence Immunoassay; SMI, skeletal muscle index; 6MWT, The 6-minute walk test; VO2 max, maximal oxygen uptake; BFR, blood flow restriction; RNA-seq, RNA sequencing; DOX, doxorubicin; ROS, reactive oxygen species; UPS, ubiquitin-proteasome system; ALP, autophagy/lysosome pathway; ATG, autophagy-related genes; CAMKK, calcium/calmodulin-dependent protein kinase kinase; AMPK, AMP-activated protein kinase; SCs, satellite cells; FAPs, Fibro-adipogenic progenitor cell; ER, endoplasmic reticulum; UPR, unfolded protein response; GH, growth hormone; IGF-1, insulin-like growth factor-1; GDF-8, growth differentiation factor 8.
1. Behranvand N, Nasri F, Zolfaghari Emameh R, Khani P, Hosseini A, Garssen J, et al. Chemotherapy: A double-edged sword in cancer treatment. Cancer immunology immunotherapy: CII. (2022) 71:507–26. doi: 10.1007/s00262-021-03013-3
2. Amjad MT, Chidharla A, Kasi A. Cancer chemotherapy. Treasure Island (FL: Statpearls (2024). ineligible companies. Disclosure: Anusha Chidharla declares no relevant financial relationships with ineligible companies. Disclosure: Anup Kasi declares no relevant financial relationships with ineligible companies.: StatPearls Publishing Copyright © 2024, StatPearls Publishing LLC.
3. Anand U, Dey A, Chandel AKS, Sanyal R, Mishra A, Pandey DK, et al. Cancer chemotherapy and beyond: current status, drug candidates, associated risks and progress in targeted therapeutics. Genes Dis. (2023) 10:1367–401. doi: 10.1016/j.gendis.2022.02.007
4. Pérez-Herrero E, Fernández-Medarde A. Advanced targeted therapies in cancer: drug nanocarriers, the future of chemotherapy. Eur J Pharm Biopharm. (2015) 93:52–79. doi: 10.1016/j.ejpb.2015.03.018
5. Di Nardo P, Lisanti C, Garutti M, Buriolla S, Alberti M, Mazzeo R, et al. Chemotherapy in patients with early breast cancer: clinical overview and management of long-term side effects. Expert Opin Drug Saf. (2022) 21:1341–55. doi: 10.1080/14740338.2022.2151584
6. Singh N, Baby D, Rajguru JP, Patil PB, Thakkannavar SS, Pujari VB. Inflammation and cancer. Ann Afr Med. (2019) 18:121–6. doi: 10.4103/aam.aam_56_18
7. Ashcraft KA, Warner AB, Jones LW, Dewhirst MW. Exercise as adjunct therapy in cancer. Semin Radiat Oncol. (2019) 29:16–24. doi: 10.1016/j.semradonc.2018.10.001
8. Iop A, Manfredi AM, Bonura S. Fatigue in cancer patients receiving chemotherapy: an analysis of published studies. Ann Oncol. (2004) 15:712–20. doi: 10.1093/annonc/mdh102
9. Wopat H, Harrod T, Brem RF, Kaltman R, Anderson K, Robien K. Body composition and chemotherapy toxicity among women treated for breast cancer: A systematic review. J Cancer Surviv. (2024) 18:1356–69. doi: 10.1007/s11764-023-01380-7
10. Cruz-Jentoft AJ, Sayer AA. Sarcopenia. Lancet. (2019) 393:2636–46. doi: 10.1016/s0140-6736(19)31138-9
11. Zhang FM, Wu HF, Shi HP, Yu Z, Zhuang CL. Sarcopenia and Malignancies: epidemiology, clinical classification and implications. Ageing Res Rev. (2023) 91:102057. doi: 10.1016/j.arr.2023.102057
12. Zhang G, Li X, Sui C, Zhao H, Zhao J, Hou Y, et al. Incidence and risk factor analysis for sarcopenia in patients with cancer. Oncol Lett. (2016) 11:1230–4. doi: 10.3892/ol.2015.4019
13. He J, Luo W, Huang Y, Song L, Mei Y. Sarcopenia as a prognostic indicator in colorectal cancer: an updated meta-analysis. Front Oncol. (2023) 13:1247341. doi: 10.3389/fonc.2023.1247341
14. Choi MH, Yoon SB. Sarcopenia in pancreatic cancer: effect on patient outcomes. World J Gastrointest Oncol. (2022) 14:2302–12. doi: 10.4251/wjgo.v14.i12.2302
15. Fearon K, Strasser F, Anker SD, Bosaeus I, Bruera E, Fainsinger RL, et al. Definition and classification of cancer cachexia: an international consensus. Lancet Oncol. (2011) 12:489–95. doi: 10.1016/s1470-2045(10)70218-7
16. Cruz-Jentoft AJ, Bahat G, Bauer J, Boirie Y, Bruyère O, Cederholm T, et al. Sarcopenia: revised european consensus on definition and diagnosis. Age Ageing. (2019) 48:16–31. doi: 10.1093/ageing/afy169
17. Aleixo GFP, Williams GR, Nyrop KA, Muss HB, Shachar SS. Muscle composition and outcomes in patients with breast cancer: meta-analysis and systematic review. Breast Cancer Res Treat. (2019) 177:569–79. doi: 10.1007/s10549-019-05352-3
18. Baracos VE, Martin L, Korc M, Guttridge DC, Fearon KCH. Cancer-associated cachexia. Nat Rev Dis Primers. (2018) 4:17105. doi: 10.1038/nrdp.2017.105
19. Morlino D, Marra M, Cioffi I, Santarpia L, De Placido P, Giuliano M, et al. Prevalence of sarcopenia in women with breast cancer. Nutrients. (2022) 14(9):1839. doi: 10.3390/nu14091839
20. Jang MK, Park S, Raszewski R, Park CG, Doorenbos AZ, Kim S. Prevalence and clinical implications of sarcopenia in breast cancer: A systematic review and meta-analysis. Supportive Care cancer: Off J Multinational Assoc Supportive Care Cancer. (2024) 32:328. doi: 10.1007/s00520-024-08532-0
21. Aires I, Duarte JA, Vitorino R, Moreira-Gonçalves D, Oliveira P, Ferreira R. Restoring Skeletal Muscle Health through Exercise in Breast Cancer Patients and after Receiving Chemotherapy. Int J Mol Sci. (2024) 25(14):7533. doi: 10.3390/ijms25147533
22. Adams SC, Segal RJ, McKenzie DC, Vallerand JR, Morielli AR, Mackey JR, et al. Impact of resistance and aerobic exercise on sarcopenia and dynapenia in breast cancer patients receiving adjuvant chemotherapy: A multicenter randomized controlled trial. Breast Cancer Res Treat. (2016) 158:497–507. doi: 10.1007/s10549-016-3900-2
23. Lee ST, Lim JP, Tan CN, Yeo A, Chew J, Lim WS. Sarc-F and modified versions using arm and calf circumference: diagnostic performance for sarcopenia screening and the impact of obesity. Geriatr Gerontol Int. (2024) 24 Suppl 1:182–8. doi: 10.1111/ggi.14758
24. Tosato M, Marzetti E, Cesari M, Savera G, Miller RR, Bernabei R, et al. Measurement of muscle mass in sarcopenia: from imaging to biochemical markers. Aging Clin Exp Res. (2017) 29:19–27. doi: 10.1007/s40520-016-0717-0
25. Thet D, Lappichetpaiboon S, Trakultritrung C, Sotangkur N, Phonphithak S, Lwin HMS, et al. The risk of malnutrition and sarcopenia in elderly people living with hiv during the covid-19 pandemic. Nutrients. (2024) 16(15):2540. doi: 10.3390/nu16152540
26. Lian R, Jiang G, Liu Q, Shi Q, Luo S, Lu J, et al. Validated tools for screening sarcopenia: A scoping review. J Am Med Dir Assoc. (2023) 24:1645–54. doi: 10.1016/j.jamda.2023.06.036
27. Chen LK, Woo J, Assantachai P, Auyeung TW, Chou MY, Iijima K, et al. Asian working group for sarcopenia: 2019 consensus update on sarcopenia diagnosis and treatment. J Am Med Dir Assoc. (2020) 21:300–7.e2. doi: 10.1016/j.jamda.2019.12.012
28. Gonzalez MC, Mehrnezhad A, Razaviarab N, Barbosa-Silva TG, Heymsfield SB. Calf circumference: cutoff values from the nhanes 1999-2006. Am J Clin Nutr. (2021) 113:1679–87. doi: 10.1093/ajcn/nqab029
29. Rolland Y, Lauwers-Cances V, Cournot M, Nourhashémi F, Reynish W, Rivière D, et al. Sarcopenia, calf circumference, and physical function of elderly women: A cross-sectional study. J Am Geriatr Soc. (2003) 51:1120–4. doi: 10.1046/j.1532-5415.2003.51362.x
30. Albano D, Messina C, Vitale J, Sconfienza LM. Imaging of sarcopenia: old evidence and new insights. Eur Radiol. (2020) 30:2199–208. doi: 10.1007/s00330-019-06573-2
31. Guglielmi G, Ponti F, Agostini M, Amadori M, Battista G, Bazzocchi A. The role of dxa in sarcopenia. Aging Clin Exp Res. (2016) 28:1047–60. doi: 10.1007/s40520-016-0589-3
32. Giannini M, Charles AL, Evrard C, Blaess J, Bouchard-Marmen M, Debrut L, et al. Sarcopenia assessed by dxa and hand-grip dynamometer: A potential marker of damage, disability and myokines imbalance in inflammatory myopathies. Rheumatol (Oxford). (2024) 63:2503–14. doi: 10.1093/rheumatology/keae207
33. Cruz-Jentoft AJ, Baeyens JP, Bauer JM, Boirie Y, Cederholm T, Landi F, et al. Sarcopenia: european consensus on definition and diagnosis: report of the european working group on sarcopenia in older people. Age Ageing. (2010) 39:412–23. doi: 10.1093/ageing/afq034
34. Bioelectrical impedance analysis in body composition measurement: national institutes of health technology assessment conference statement. Am J Clin Nutr. (1996) 64:524s–32s. doi: 10.1093/ajcn/64.3.524S
35. Aleixo GFP, Shachar SS, Nyrop KA, Muss HB, Battaglini CL, Williams GR. Bioelectrical impedance analysis for the assessment of sarcopenia in patients with cancer: A systematic review. Oncologist. (2020) 25:170–82. doi: 10.1634/theoncologist.2019-0600
36. Mitsiopoulos N, Baumgartner RN, Heymsfield SB, Lyons W, Gallagher D, Ross R. Cadaver validation of skeletal muscle measurement by magnetic resonance imaging and computerized tomography. J Appl Physiol (Bethesda Md: 1985). (1998) 85:115–22. doi: 10.1152/jappl.1998.85.1.115
37. Rossi F, Lambertini M, Brunetti N, De Giorgis S, Razeti MG, Calabrese M, et al. Muscle mass loss in breast cancer patients of reproductive age (≤ 45 years) undergoing neoadjuvant chemotherapy. La Radiologia Med. (2023) 128:49–57. doi: 10.1007/s11547-022-01574-6
38. Rossi F, Torri L, Lambertini M, De Giorgis S, Calabrese M, Tagliafico AS. Muscle mass loss after neoadjuvant chemotherapy in breast cancer: estimation on breast magnetic resonance imaging using pectoralis muscle area. Eur Radiol. (2020) 30:4234–41. doi: 10.1007/s00330-020-06799-5
39. Fu H, Wang L, Zhang W, Lu J, Yang M. Diagnostic test accuracy of ultrasound for sarcopenia diagnosis: A systematic review and meta-analysis. J cachexia sarcopenia Muscle. (2023) 14:57–70. doi: 10.1002/jcsm.13149
40. Perkisas S, Bastijns S, Baudry S, Bauer J, Beaudart C, Beckwée D, et al. Application of ultrasound for muscle assessment in sarcopenia: 2020 sarcus update. Eur Geriatr Med. (2021) 12:45–59. doi: 10.1007/s41999-020-00433-9
41. Kızılarslanoğlu MC, Eryılmaz MA, Yortanlı B, Ünal İR, Ünal BC, Baran N, et al. Pectoralis muscle index might be a factor associated with frailty in older women with breast cancer. Turk J Med Sci. (2023) 53:824–34. doi: 10.55730/1300-0144.5645
42. Frank-Wilson AW, Johnston JD, Olszynski WP, Kontulainen SA. Measurement of muscle and fat in postmenopausal women: precision of previously reported pqct imaging methods. Bone. (2015) 75:49–54. doi: 10.1016/j.bone.2015.01.016
43. Thapa N, Yang JG, Bae S, Kim GM, Park HJ, Park H. Effect of electrical muscle stimulation and resistance exercise intervention on physical and brain function in middle-aged and older women. Int J Environ Res Public Health. (2023) 20(1):101. doi: 10.3390/ijerph20010101
44. Li YH, Wang XH, Ya S. Prevalence and diagnostic agreement of sarcopenia based on handgrip strength and 5-time chair-stand test among chinese community-dwelling older adults. Int J Older People Nurs. (2024) 19:e12635. doi: 10.1111/opn.12635
45. Klassen O, Schmidt ME, Ulrich CM, Schneeweiss A, Potthoff K, Steindorf K, et al. Muscle strength in breast cancer patients receiving different treatment regimes. J cachexia sarcopenia Muscle. (2017) 8:305–16. doi: 10.1002/jcsm.12165
46. Pinheiro PA, Carneiro JA, Coqueiro RS, Pereira R, Fernandes MH. Chair stand test" as simple tool for sarcopenia screening in elderly women. J Nutr Health Aging. (2016) 20:56–9. doi: 10.1007/s12603-016-0676-3
47. Perez-Sousa MA, Venegas-Sanabria LC, Chavarro-Carvajal DA, Cano-Gutierrez CA, Izquierdo M, Correa-Bautista JE, et al. Gait speed as a mediator of the effect of sarcopenia on dependency in activities of daily living. J cachexia sarcopenia Muscle. (2019) 10:1009–15. doi: 10.1002/jcsm.12444
48. Scharf G, Heineke J. Finding good biomarkers for sarcopenia. J cachexia sarcopenia Muscle. (2012) 3:145–8. doi: 10.1007/s13539-012-0081-7
49. Kim M, Won CW. Sarcopenia is associated with cognitive impairment mainly due to slow gait speed: results from the korean frailty and aging cohort study (Kfacs). Int J Environ Res Public Health. (2019) 16(9):1491. doi: 10.3390/ijerph16091491
50. Agarwala P, Salzman SH. Six-minute walk test: clinical role, technique, coding, and reimbursement. Chest. (2020) 157:603–11. doi: 10.1016/j.chest.2019.10.014
51. Enright PL, McBurnie MA, Bittner V, Tracy RP, McNamara R, Arnold A, et al. The 6-min walk test: A quick measure of functional status in elderly adults. Chest. (2003) 123:387–98. doi: 10.1378/chest.123.2.387
52. Schmidt K, Vogt L, Thiel C, Jäger E, Banzer W. Validity of the six-minute walk test in cancer patients. Int J Sports Med. (2013) 34:631–6. doi: 10.1055/s-0032-1323746
53. Martinez BP, Gomes IB, Oliveira CS, Ramos IR, Rocha MD, Forgiarini Júnior LA, et al. Accuracy of the timed up and go test for predicting sarcopenia in elderly hospitalized patients. Clinics (Sao Paulo). (2015) 70:369–72. doi: 10.6061/clinics/2015(05)11
54. Heymsfield SB, Gallagher D, Visser M, Nuñez C, Wang ZM. Measurement of skeletal muscle: laboratory and epidemiological methods. journals gerontology Ser A Biol Sci Med Sci. (1995) 50:23–9. doi: 10.1093/gerona/50a.special_issue.23
55. Kehayias JJ, Fiatarone MA, Zhuang H, Roubenoff R. Total body potassium and body fat: relevance to aging. Am J Clin Nutr. (1997) 66:904–10. doi: 10.1093/ajcn/66.4.904
56. Wielopolski L, Ramirez LM, Spungen AM, Swaby S, Asselin P, Bauman WA. Measuring partial body potassium in the legs of patients with spinal cord injury: A new approach. J Appl Physiol (Bethesda Md: 1985). (2009) 106:268–73. doi: 10.1152/japplphysiol.90435.2008
57. Keshaviah PR, Nolph KD, Moore HL, Prowant B, Emerson PF, Meyer M, et al. Lean body mass estimation by creatinine kinetics. J Am Soc Nephrology: JASN. (1994) 4:1475–85. doi: 10.1681/asn.V471475
58. Patel SS, Molnar MZ, Tayek JA, Ix JH, Noori N, Benner D, et al. Serum creatinine as a marker of muscle mass in chronic kidney disease: results of a cross-sectional study and review of literature. J cachexia sarcopenia Muscle. (2013) 4:19–29. doi: 10.1007/s13539-012-0079-1
59. Evans WJ, Hellerstein M, Orwoll E, Cummings S, Cawthon PM. D(3) -creatine dilution and the importance of accuracy in the assessment of skeletal muscle mass. J cachexia sarcopenia Muscle. (2019) 10:14–21. doi: 10.1002/jcsm.12390
60. Stimpson SA, Leonard MS, Clifton LG, Poole JC, Turner SM, Shearer TW, et al. Longitudinal changes in total body creatine pool size and skeletal muscle mass using the D(3)-creatine dilution method. J cachexia sarcopenia Muscle. (2013) 4:217–23. doi: 10.1007/s13539-013-0110-1
61. Clark RV, Walker AC, O'Connor-Semmes RL, Leonard MS, Miller RR, Stimpson SA, et al. Total body skeletal muscle mass: estimation by creatine (Methyl-D3) dilution in humans. J Appl Physiol (Bethesda Md: 1985). (2014) 116:1605–13. doi: 10.1152/japplphysiol.00045.2014
62. Curcio F, Ferro G, Basile C, Liguori I, Parrella P, Pirozzi F, et al. Biomarkers in sarcopenia: A multifactorial approach. Exp gerontology. (2016) 85:1–8. doi: 10.1016/j.exger.2016.09.007
63. Pellegrino R, Paganelli R, Di Iorio A, Bandinelli S, Moretti A, Iolascon G, et al. Muscle quality, physical performance, and comorbidity are predicted by circulating procollagen type iii N-terminal peptide (P3np): the inchianti follow-up study. GeroScience. (2024) 46:1259–69. doi: 10.1007/s11357-023-00894-3
64. Chen YY, Chiu YL, Kao TW, Peng TC, Yang HF, Chen WL. Cross-sectional associations among P3np, htra, hsp70, apelin and sarcopenia in Taiwanese population. BMC geriatrics. (2021) 21:192. doi: 10.1186/s12877-021-02146-5
65. Shin HE, Kim M, Won CW. Association between plasma procollagen type iii N-terminal peptide (P3np) levels and physical performance in elderly men: the korean frailty and aging cohort study (Kfacs). Exp gerontology. (2021) 154:111523. doi: 10.1016/j.exger.2021.111523
66. Ciciliot S, Rossi AC, Dyar KA, Blaauw B, Schiaffino S. Muscle type and fiber type specificity in muscle wasting. Int J Biochem Cell Biol. (2013) 45:2191–9. doi: 10.1016/j.biocel.2013.05.016
67. Guigni BA, Callahan DM, Tourville TW, Miller MS, Fiske B, Voigt T, et al. Skeletal muscle atrophy and dysfunction in breast cancer patients: role for chemotherapy-derived oxidant stress. Am J Physiol Cell Physiol. (2018) 315:C744–c56. doi: 10.1152/ajpcell.00002.2018
68. Mijwel S, Cardinale DA, Norrbom J, Chapman M, Ivarsson N, Wengström Y, et al. Exercise training during chemotherapy preserves skeletal muscle fiber area, capillarization, and mitochondrial content in patients with breast cancer. FASEB journal: Off Publ Fed Am Societies Exp Biol. (2018) 32:5495–505. doi: 10.1096/fj.201700968R
69. De Groef A, Geraerts I, Demeyer H, van der Gucht E, Dams L, de Kinkelder C, et al. Physical activity levels after treatment for breast cancer: two-year follow-up. Breast (Edinburgh Scotland). (2018) 40:23–8. doi: 10.1016/j.breast.2018.04.009
70. Yildiz Kabak V, Gursen C, Aytar A, Akbayrak T, Duger T. Physical activity level, exercise behavior, barriers, and preferences of patients with breast cancer-related lymphedema. Supportive Care cancer: Off J Multinational Assoc Supportive Care Cancer. (2021) 29:3593–602. doi: 10.1007/s00520-020-05858-3
71. Lee BM, Cho Y, Kim JW, Ahn SG, Kim JH, Jeung HC, et al. Association between skeletal muscle loss and the response to neoadjuvant chemotherapy for breast cancer. Cancers. (2021) 13(8):1806. doi: 10.3390/cancers13081806
72. Amitani M, Oba T, Kiyosawa N, Morikawa H, Chino T, Soma A, et al. Skeletal muscle loss during neoadjuvant chemotherapy predicts poor prognosis in patients with breast cancer. BMC Cancer. (2022) 22:327. doi: 10.1186/s12885-022-09443-1
73. Roland PE, Skinhøj E. Extrastriate cortical areas activated during visual discrimination in man. Brain Res. (1981) 222:166–71. doi: 10.1016/0006-8993(81)90953-7
74. Hooijmans MT, Schlaffke L, Bolsterlee B, Schlaeger S, Marty B, Mazzoli V. Compositional and functional mri of skeletal muscle: A review. J magnetic resonance imaging: JMRI. (2024) 60:860–77. doi: 10.1002/jmri.29091
75. Amini B, Boyle SP, Boutin RD, Lenchik L. Approaches to assessment of muscle mass and myosteatosis on computed tomography: A systematic review. journals gerontology Ser A Biol Sci Med Sci. (2019) 74:1671–8. doi: 10.1093/gerona/glz034
76. Fazzini B, Märkl T, Costas C, Blobner M, Schaller SJ, Prowle J, et al. The rate and assessment of muscle wasting during critical illness: A systematic review and meta-analysis. Crit Care (London England). (2023) 27:2. doi: 10.1186/s13054-022-04253-0
77. Addison O, Marcus RL, Lastayo PC, Ryan AS. Intermuscular fat: A review of the consequences and causes. Int J Endocrinol. (2014) 2014:309570. doi: 10.1155/2014/309570
78. Brioche T, Pagano AF, Py G, Chopard A. Muscle wasting and aging: experimental models, fatty infiltrations, and prevention. Mol aspects Med. (2016) 50:56–87. doi: 10.1016/j.mam.2016.04.006
79. Pagano AF, Brioche T, Arc-Chagnaud C, Demangel R, Chopard A, Py G. Short-term disuse promotes fatty acid infiltration into skeletal muscle. J cachexia sarcopenia Muscle. (2018) 9:335–47. doi: 10.1002/jcsm.12259
80. Kuzel BR, Grindel S, Papandrea R, Ziegler D. Fatty infiltration and rotator cuff atrophy. J Am Acad Orthopaedic Surgeons. (2013) 21:613–23. doi: 10.5435/jaaos-21-10-613
81. Biltz NK, Collins KH, Shen KC, Schwartz K, Harris CA, Meyer GA. Infiltration of intramuscular adipose tissue impairs skeletal muscle contraction. J Physiol. (2020) 598:2669–83. doi: 10.1113/jp279595
82. Jeon YW, Park HS, Ko Y, Sung YS, Shim BY, Suh YJ, et al. Intermuscular fat density as a novel prognostic factor in breast cancer patients treated with adjuvant chemotherapy. Breast Cancer Res Treat. (2021) 189:759–68. doi: 10.1007/s10549-021-06322-4
83. Barreto R, Kitase Y, Matsumoto T, Pin F, Colston KC, Couch KE, et al. Acvr2b/fc counteracts chemotherapy-induced loss of muscle and bone mass. Sci Rep. (2017) 7:14470. doi: 10.1038/s41598-017-15040-1
84. Taniguchi Y, Makizako H, Kiyama R, Tomioka K, Nakai Y, Kubozono T, et al. The association between osteoporosis and grip strength and skeletal muscle mass in community-dwelling older women. Int J Environ Res Public Health. (2019) 16(7):1228. doi: 10.3390/ijerph16071228
85. Lu TW, Taylor SJ, O'Connor JJ, Walker PS. Influence of muscle activity on the forces in the femur: an in vivo study. J biomechanics. (1997) 30:1101–6. doi: 10.1016/s0021-9290(97)00090-0
86. Ramos da Silva B, Mialich MS, Cruz LP, Rufato S, Gozzo T, Jordao AA. Performance of functionality measures and phase angle in women exposed to chemotherapy for early breast cancer. Clin Nutr ESPEN. (2021) 42:105–16. doi: 10.1016/j.clnesp.2021.02.007
87. Toth MJ, Voigt TB, Tourville TW, Prior SM, Guigni BA, Schlosberg AV, et al. Effect of neuromuscular electrical stimulation on skeletal muscle size and function in patients with breast cancer receiving chemotherapy. J Appl Physiol (Bethesda Md: 1985). (2020) 128:1654–65. doi: 10.1152/japplphysiol.00203.2020
88. But-Hadzic J, Dervisevic M, Karpljuk D, Videmsek M, Dervisevic E, Paravlic A, et al. Six-minute walk distance in breast cancer survivors-a systematic review with meta-analysis. Int J Environ Res Public Health. (2021) 18(5):2591. doi: 10.3390/ijerph18052591
89. Schumacher AN, Shackelford DYK, Brown JM, Hayward R. Validation of the 6-min walk test for predicting peak V˙O2 in cancer survivors. Med Sci sports Exercise. (2019) 51:271–7. doi: 10.1249/mss.0000000000001790
90. Bird BR, Swain SM. Cardiac toxicity in breast cancer survivors: review of potential cardiac problems. Clin Cancer research: an Off J Am Assoc Cancer Res. (2008) 14:14–24. doi: 10.1158/1078-0432.Ccr-07-1033
91. Neil SE, Klika RJ, Garland SJ, McKenzie DC, Campbell KL. Cardiorespiratory and neuromuscular deconditioning in fatigued and non-fatigued breast cancer survivors. Supportive Care cancer: Off J Multinational Assoc Supportive Care Cancer. (2013) 21:873–81. doi: 10.1007/s00520-012-1600-y
92. Perera E, Zhu XM, Horner NS, Bedi A, Ayeni OR, Khan M. Effects of blood flow restriction therapy for muscular strength, hypertrophy, and endurance in healthy and special populations: A systematic review and meta-analysis. Clin J Sport Med. (2022) 32:531–45. doi: 10.1097/jsm.0000000000000991
93. Miller BC, Tirko AW, Shipe JM, Sumeriski OR, Moran K. The systemic effects of blood flow restriction training: A systematic review. Int J Sports Phys Ther. (2021) 16:978–90. doi: 10.26603/001c.25791
94. Reina-Ruiz ÁJ, Martínez-Cal J, Molina-Torres G, Romero-Galisteo RP, Galán-Mercant A, Carrasco-Vega E, et al. Effectiveness of blood flow restriction on functionality, quality of life and pain in patients with neuromusculoskeletal pathologies: A systematic review. Int J Environ Res Public Health. (2023) 20(2):1401. doi: 10.3390/ijerph20021401
95. Mijnarends DM, Meijers JM, Halfens RJ, ter Borg S, Luiking YC, Verlaan S, et al. Validity and reliability of tools to measure muscle mass, strength, and physical performance in community-dwelling older people: A systematic review. J Am Med Dir Assoc. (2013) 14:170–8. doi: 10.1016/j.jamda.2012.10.009
96. Kashani KB, Frazee EN, Kukrálová L, Sarvottam K, Herasevich V, Young PM, et al. Evaluating muscle mass by using markers of kidney function: development of the sarcopenia index. Crit Care Med. (2017) 45:e23–e9. doi: 10.1097/ccm.0000000000002013
97. Tang T, Xie L, Hu S, Tan L, Lei X, Luo X, et al. Serum creatinine and cystatin C-based diagnostic indices for sarcopenia in advanced non-small cell lung cancer. J cachexia sarcopenia Muscle. (2022) 13:1800–10. doi: 10.1002/jcsm.12977
98. Zheng C, Wang E, Li JS, Xie K, Luo C, Ge QY, et al. Serum creatinine/cystatin C ratio as a screening tool for sarcopenia and prognostic indicator for patients with esophageal cancer. BMC geriatrics. (2022) 22:207. doi: 10.1186/s12877-022-02925-8
99. Ding L, Wang X, Mao T, Li J. Diagnostic value of serum creatinine and cystatin-C-based indices and ishii score in cancer-related sarcopenia. Diagnostics (Basel Switzerland). (2023) 13(13):2179. doi: 10.3390/diagnostics13132179
100. Park SE, Choi JH, Park JY, Kim BJ, Kim JG, Kim JW, et al. Loss of skeletal muscle mass during palliative chemotherapy is a poor prognostic factor in patients with advanced gastric cancer. Sci Rep. (2020) 10:17683. doi: 10.1038/s41598-020-74765-8
101. Stout NL, Santa Mina D, Lyons KD, Robb K, Silver JK. A systematic review of rehabilitation and exercise recommendations in oncology guidelines. CA: Cancer J Clin. (2021) 71:149–75. doi: 10.3322/caac.21639
102. Ryan AM, Power DG, Daly L, Cushen SJ, Ní Bhuachalla Ē, Prado CM. Cancer-associated malnutrition, cachexia and sarcopenia: the skeleton in the hospital closet 40 years later. Proc Nutr Soc. (2016) 75:199–211. doi: 10.1017/s002966511500419x
103. Stanton SE, Disis ML. Clinical significance of tumor-infiltrating lymphocytes in breast cancer. J Immunother Cancer. (2016) 4:59. doi: 10.1186/s40425-016-0165-6
104. Bohlen J, McLaughlin SL, Hazard-Jenkins H, Infante AM, Montgomery C, Davis M, et al. Dysregulation of metabolic-associated pathways in muscle of breast cancer patients: preclinical evaluation of interleukin-15 targeting fatigue. J cachexia sarcopenia Muscle. (2018) 9:701–14. doi: 10.1002/jcsm.12294
105. Wilson HE, Stanton DA, Rellick S, Geldenhuys W, Pistilli EE. Breast cancer-associated skeletal muscle mitochondrial dysfunction and lipid accumulation is reversed by pparg. Am J Physiol Cell Physiol. (2021) 320(4):C577–90. doi: 10.1152/ajpcell.00264.2020
106. Marzetti E, Calvani R, Coelho-Júnior HJ, Landi F, Picca A. Mitochondrial quantity and quality in age-related sarcopenia. Int J Mol Sci. (2024) 25(4):2052. doi: 10.3390/ijms25042052
107. Mallard J, Hucteau E, Charles AL, Bender L, Baeza C, Pélissie M, et al. Chemotherapy impairs skeletal muscle mitochondrial homeostasis in early breast cancer patients. J cachexia sarcopenia Muscle. (2022) 13:1896–907. doi: 10.1002/jcsm.12991
108. Gouspillou G, Scheede-Bergdahl C, Spendiff S, Vuda M, Meehan B, Mlynarski H, et al. Anthracycline-containing chemotherapy causes long-term impairment of mitochondrial respiration and increased reactive oxygen species release in skeletal muscle. Sci Rep. (2015) 5:8717. doi: 10.1038/srep08717
109. Gilliam LAA, Fisher-Wellman KH, Lin CT, Maples JM, Cathey BL, Neufer PD. The anticancer agent doxorubicin disrupts mitochondrial energy metabolism and redox balance in skeletal muscle. Free Radic Biol Med. (2013) 65:988–96. doi: 10.1016/j.freeradbiomed.2013.08.191
110. Gilliam LA, Moylan JS, Patterson EW, Smith JD, Wilson AS, Rabbani Z, et al. Doxorubicin acts via mitochondrial ros to stimulate catabolism in C2c12 myotubes. Am J Physiol Cell Physiol. (2012) 302:C195–202. doi: 10.1152/ajpcell.00217.2011
111. Brand MD. Mitochondrial generation of superoxide and hydrogen peroxide as the source of mitochondrial redox signaling. Free Radic Biol Med. (2016) 100:14–31. doi: 10.1016/j.freeradbiomed.2016.04.001
112. Romanello V, Sandri M. Mitochondrial biogenesis and fragmentation as regulators of muscle protein degradation. Curr hypertension Rep. (2010) 12:433–9. doi: 10.1007/s11906-010-0157-8
113. Romanello V, Guadagnin E, Gomes L, Roder I, Sandri C, Petersen Y, et al. Mitochondrial fission and remodelling contributes to muscle atrophy. EMBO J. (2010) 29:1774–85. doi: 10.1038/emboj.2010.60
114. Marques-Aleixo I, Santos-Alves E, Torrella JR, Oliveira PJ, Magalhães J, Ascensão A. Exercise and doxorubicin treatment modulate cardiac mitochondrial quality control signaling. Cardiovasc Toxicol. (2018) 18:43–55. doi: 10.1007/s12012-017-9412-4
115. Mallard J, Hucteau E, Bender L, Moinard-Butot F, Rochelle E, Boutonnet L, et al. A single chemotherapy administration induces muscle atrophy, mitochondrial alterations and apoptosis in breast cancer patients. J cachexia sarcopenia Muscle. (2024) 15:292–305. doi: 10.1002/jcsm.13414
116. Schiaffino S, Dyar KA, Ciciliot S, Blaauw B, Sandri M. Mechanisms regulating skeletal muscle growth and atrophy. FEBS J. (2013) 280:4294–314. doi: 10.1111/febs.12253
117. Nie L, Liu M, Chen J, Wu Q, Li Y, Yi J, et al. Hydrogen sulfide ameliorates doxorubicin−Induced myocardial fibrosis in rats via the pi3k/akt/mtor pathway. Mol Med Rep. (2021) 23(4):299. doi: 10.3892/mmr.2021.11938
118. Masiero E, Agatea L, Mammucari C, Blaauw B, Loro E, Komatsu M, et al. Autophagy is required to maintain muscle mass. Cell Metab. (2009) 10:507–15. doi: 10.1016/j.cmet.2009.10.008
119. Smuder AJ, Kavazis AN, Min K, Powers SK. Exercise protects against doxorubicin-induced markers of autophagy signaling in skeletal muscle. J Appl Physiol (Bethesda Md: 1985). (2011) 111:1190–8. doi: 10.1152/japplphysiol.00429.2011
120. Smuder AJ, Kavazis AN, Min K, Powers SK. Exercise protects against doxorubicin-induced oxidative stress and proteolysis in skeletal muscle. J Appl Physiol (Bethesda Md: 1985). (2011) 110:935–42. doi: 10.1152/japplphysiol.00677.2010
121. Kavazis AN, Smuder AJ, Powers SK. Effects of short-term endurance exercise training on acute doxorubicin-induced foxo transcription in cardiac and skeletal muscle. J Appl Physiol (Bethesda Md: 1985). (2014) 117:223–30. doi: 10.1152/japplphysiol.00210.2014
122. Bilodeau PA, Coyne ES, Wing SS. The ubiquitin proteasome system in atrophying skeletal muscle: roles and regulation. Am J Physiol Cell Physiol. (2016) 311(3):C392–403. doi: 10.1152/ajpcell.00125.2016
123. Frankland-Searby S, Bhaumik SR. The 26s proteasome complex: an attractive target for cancer therapy. Biochim Biophys Acta. (2012) 1825:64–76. doi: 10.1016/j.bbcan.2011.10.003
124. Bodine SC, Baehr LM. Skeletal muscle atrophy and the E3 ubiquitin ligases murf1 and mafbx/atrogin-1. Am J Physiol Endocrinol Metab. (2014) 307:E469–84. doi: 10.1152/ajpendo.00204.2014
125. Yoshida T, Delafontaine P. Mechanisms of cachexia in chronic disease states. Am J Med Sci. (2015) 350:250–6. doi: 10.1097/maj.0000000000000511
126. Sandri M, Sandri C, Gilbert A, Skurk C, Calabria E, Picard A, et al. Foxo transcription factors induce the atrophy-related ubiquitin ligase atrogin-1 and cause skeletal muscle atrophy. Cell. (2004) 117:399–412. doi: 10.1016/s0092-8674(04)00400-3
127. Stitt TN, Drujan D, Clarke BA, Panaro F, Timofeyva Y, Kline WO, et al. The igf-1/pi3k/akt pathway prevents expression of muscle atrophy-induced ubiquitin ligases by inhibiting foxo transcription factors. Mol Cell. (2004) 14:395–403. doi: 10.1016/s1097-2765(04)00211-4
128. Mammucari C, Milan G, Romanello V, Masiero E, Rudolf R, Del Piccolo P, et al. Foxo3 controls autophagy in skeletal muscle in vivo. Cell Metab. (2007) 6:458–71. doi: 10.1016/j.cmet.2007.11.001
129. Lee SW, Dai G, Hu Z, Wang X, Du J, Mitch WE. Regulation of muscle protein degradation: coordinated control of apoptotic and ubiquitin-proteasome systems by phosphatidylinositol 3 kinase. J Am Soc Nephrology: JASN. (2004) 15:1537–45. doi: 10.1097/01.asn.0000127211.86206.e1
130. Southgate RJ, Neill B, Prelovsek O, El-Osta A, Kamei Y, Miura S, et al. Foxo1 regulates the expression of 4e-bp1 and inhibits mtor signaling in mammalian skeletal muscle. J Biol Chem. (2007) 282:21176–86. doi: 10.1074/jbc.M702039200
131. Xia Q, Huang X, Huang J, Zheng Y, March ME, Li J, et al. The role of autophagy in skeletal muscle diseases. Front Physiol. (2021) 12:638983. doi: 10.3389/fphys.2021.638983
132. Zhao J, Brault JJ, Schild A, Cao P, Sandri M, Schiaffino S, et al. Foxo3 coordinately activates protein degradation by the autophagic/lysosomal and proteasomal pathways in atrophying muscle cells. Cell Metab. (2007) 6:472–83. doi: 10.1016/j.cmet.2007.11.004
133. Høyer-Hansen M, Bastholm L, Szyniarowski P, Campanella M, Szabadkai G, Farkas T, et al. Control of macroautophagy by calcium, calmodulin-dependent kinase kinase-beta, and bcl-2. Mol Cell. (2007) 25:193–205. doi: 10.1016/j.molcel.2006.12.009
134. Zhang YW, Shi J, Li YJ, Wei L. Cardiomyocyte death in doxorubicin-induced cardiotoxicity. Archivum immunologiae therapiae experimentalis. (2009) 57:435–45. doi: 10.1007/s00005-009-0051-8
135. Kelleher AR, Fairchild TJ, Keslacy S. Stz-induced skeletal muscle atrophy is associated with increased P65 content and downregulation of insulin pathway without nf-κb canonical cascade activation. Acta diabetologica. (2010) 47:315–23. doi: 10.1007/s00592-010-0209-1
136. Nicolini A, Ferrari P, Masoni MC, Fini M, Pagani S, Giampietro O, et al. Malnutrition, anorexia and cachexia in cancer patients: A mini-review on pathogenesis and treatment. Biomedicine pharmacotherapy = Biomedecine pharmacotherapie. (2013) 67:807–17. doi: 10.1016/j.biopha.2013.08.005
137. Zhang G, Liu Z, Ding H, Miao H, Garcia JM, Li YP. Toll-like receptor 4 mediates lewis lung carcinoma-induced muscle wasting via coordinate activation of protein degradation pathways. Sci Rep. (2017) 7:2273. doi: 10.1038/s41598-017-02347-2
138. Gilliam LA, Ferreira LF, Bruton JD, Moylan JS, Westerblad H, St Clair DK, et al. Doxorubicin acts through tumor necrosis factor receptor subtype 1 to cause dysfunction of murine skeletal muscle. J Appl Physiol (Bethesda Md: 1985). (2009) 107:1935–42. doi: 10.1152/japplphysiol.00776.2009
139. Günther S, Kim J, Kostin S, Lepper C, Fan CM, Braun T. Myf5-positive satellite cells contribute to pax7-dependent long-term maintenance of adult muscle stem cells. Cell Stem Cell. (2013) 13:590–601. doi: 10.1016/j.stem.2013.07.016
140. Mauro A. Satellite cell of skeletal muscle fibers. J Biophys Biochem cytology. (1961) 9:493–5. doi: 10.1083/jcb.9.2.493
141. Eliazer S, Muncie JM, Christensen J, Sun X, D'Urso RS, Weaver VM, et al. Wnt4 from the niche controls the mechano-properties and quiescent state of muscle stem cells. Cell Stem Cell. (2019) 25:654–65.e4. doi: 10.1016/j.stem.2019.08.007
142. Milanesi A, Lee JW, Yang A, Liu YY, Sedrakyan S, Cheng SY, et al. Thyroid hormone receptor alpha is essential to maintain the satellite cell niche during skeletal muscle injury and sarcopenia of aging. Thyroid: Off J Am Thyroid Assoc. (2017) 27:1316–22. doi: 10.1089/thy.2017.0021
143. Rahimov F, Kunkel LM. The cell biology of disease: cellular and molecular mechanisms underlying muscular dystrophy. J Cell Biol. (2013) 201:499–510. doi: 10.1083/jcb.201212142
144. Brown JL, Lawrence MM, Borowik A, Oliver L, Peelor FF 3rd, Van Remmen H, et al. Tumor burden negatively impacts protein turnover as a proteostatic process in noncancerous liver, heart, and muscle, but not brain. J Appl Physiol (Bethesda Md: 1985). (2021) 131:72–82. doi: 10.1152/japplphysiol.01026.2020
145. Tayek JA, Blackburn GL, Bistrian BR. Alterations in whole body, muscle, liver, and tumor tissue protein synthesis and degradation in novikoff hepatoma and yoshida sarcoma tumor growth studied in vivo. Cancer Res. (1988) 48:1554–8.
146. Marchildon F, Lamarche É, Lala-Tabbert N, St-Louis C, Wiper-Bergeron N. Expression of ccaat/enhancer binding protein beta in muscle satellite cells inhibits myogenesis in cancer cachexia. PloS One. (2015) 10:e0145583. doi: 10.1371/journal.pone.0145583
147. D'Lugos AC, Fry CS, Ormsby JC, Sweeney KR, Brightwell CR, Hale TM, et al. Chronic doxorubicin administration impacts satellite cell and capillary abundance in a muscle-specific manner. Physiol Rep. (2019) 7:e14052. doi: 10.14814/phy2.14052
148. Mahdy MAA. Skeletal muscle fibrosis: an overview. Cell Tissue Res. (2019) 375:575–88. doi: 10.1007/s00441-018-2955-2
149. Albano D, Dondi F, Treglia G, Tucci A, Ravanelli M, Farina D, et al. Longitudinal body composition changes detected by [(18)F]Fdg pet/ct during and after chemotherapy and their prognostic role in elderly hodgkin lymphoma. Cancers. (2022) 14(20):5147. doi: 10.3390/cancers14205147
150. Chen W, You W, Valencak TG, Shan T. Bidirectional roles of skeletal muscle fibro-adipogenic progenitors in homeostasis and disease. Ageing Res Rev. (2022) 80:101682. doi: 10.1016/j.arr.2022.101682
151. Giuliani G, Rosina M, Reggio A. Signaling pathways regulating the fate of fibro/adipogenic progenitors (Faps) in skeletal muscle regeneration and disease. FEBS J. (2022) 289:6484–517. doi: 10.1111/febs.16080
152. Joe AW, Yi L, Natarajan A, Le Grand F, So L, Wang J, et al. Muscle injury activates resident fibro/adipogenic progenitors that facilitate myogenesis. Nat Cell Biol. (2010) 12:153–63. doi: 10.1038/ncb2015
153. Uezumi A, Fukada S, Yamamoto N, Ikemoto-Uezumi M, Nakatani M, Morita M, et al. Identification and characterization of pdgfrα+ Mesenchymal progenitors in human skeletal muscle. Cell Death Dis. (2014) 5:e1186. doi: 10.1038/cddis.2014.161
154. Uezumi A, Ito T, Morikawa D, Shimizu N, Yoneda T, Segawa M, et al. Fibrosis and adipogenesis originate from a common mesenchymal progenitor in skeletal muscle. J Cell Sci. (2011) 124:3654–64. doi: 10.1242/jcs.086629
155. Mallard J, Hucteau E, Bender L, Charlot A, Debrut L, Pflumio C, et al. Development of skeletal muscle atrophy and intermuscular adipose tissue in patients with early breast cancer treated with chemotherapy. Am J Physiol Cell Physiol. (2022) 323:C1325–c32. doi: 10.1152/ajpcell.00373.2022
156. Madaro L, Passafaro M, Sala D, Etxaniz U, Lugarini F, Proietti D, et al. Denervation-activated stat3-il-6 signalling in fibro-adipogenic progenitors promotes myofibres atrophy and fibrosis. Nat Cell Biol. (2018) 20:917–27. doi: 10.1038/s41556-018-0151-y
157. Chen X, Cubillos-Ruiz JR. Endoplasmic reticulum stress signals in the tumour and its microenvironment. Nat Rev Cancer. (2021) 21:71–88. doi: 10.1038/s41568-020-00312-2
158. Wang M, Kaufman RJ. Protein misfolding in the endoplasmic reticulum as a conduit to human disease. Nature. (2016) 529:326–35. doi: 10.1038/nature17041
159. Sisinni L, Pietrafesa M, Lepore S, Maddalena F, Condelli V, Esposito F, et al. Endoplasmic reticulum stress and unfolded protein response in breast cancer: the balance between apoptosis and autophagy and its role in drug resistance. Int J Mol Sci. (2019) 20(4):857. doi: 10.3390/ijms20040857
160. Walter P, Ron D. The unfolded protein response: from stress pathway to homeostatic regulation. Sci (New York NY). (2011) 334:1081–6. doi: 10.1126/science.1209038
161. Cohen S, Brault JJ, Gygi SP, Glass DJ, Valenzuela DM, Gartner C, et al. During muscle atrophy, thick, but not thin, filament components are degraded by murf1-dependent ubiquitylation. J Cell Biol. (2009) 185:1083–95. doi: 10.1083/jcb.200901052
162. Afroze D, Kumar A. Er stress in skeletal muscle remodeling and myopathies. FEBS J. (2019) 286:379–98. doi: 10.1111/febs.14358
163. Zhang QB, Liu AY, Fang QZ, Wang F, Wang H, Zhou Y. Effect of electrical stimulation on disuse muscular atrophy induced by immobilization: correlation with upregulation of perk signal and parkin-mediated mitophagy. Am J Phys Med Rehabil. (2023) 102:692–700. doi: 10.1097/phm.0000000000002182
164. Gallot YS, Bohnert KR, Straughn AR, Xiong G, Hindi SM, Kumar A. Perk regulates skeletal muscle mass and contractile function in adult mice. FASEB journal: Off Publ Fed Am Societies Exp Biol. (2019) 33:1946–62. doi: 10.1096/fj.201800683RR
165. Whitham M, Febbraio MA. The ever-expanding myokinome: discovery challenges and therapeutic implications. Nat Rev Drug Discovery. (2016) 15:719–29. doi: 10.1038/nrd.2016.153
166. Mancinelli R, Checcaglini F, Coscia F, Gigliotti P, Fulle S, Fanò-Illic G. Biological aspects of selected myokines in skeletal muscle: focus on aging. Int J Mol Sci. (2021) 22(16):8520. doi: 10.3390/ijms22168520
167. Zunner BEM, Wachsmuth NB, Eckstein ML, Scherl L, Schierbauer JR, Haupt S, et al. Myokines and resistance training: A narrative review. Int J Mol Sci. (2022) 23(7):3501. doi: 10.3390/ijms23073501
168. Kwon I. Protective effects of endurance exercise on skeletal muscle remodeling against doxorubicin-induced myotoxicity in mice. Phys activity Nutr. (2020) 24:11–21. doi: 10.20463/pan.2020.0010
169. Petersen AM, Pedersen BK. The anti-inflammatory effect of exercise. J Appl Physiol (Bethesda Md: 1985). (2005) 98:1154–62. doi: 10.1152/japplphysiol.00164.2004
170. Nash D, Hughes MG, Butcher L, Aicheler R, Smith P, Cullen T, et al. Il-6 signaling in acute exercise and chronic training: potential consequences for health and athletic performance. Scandinavian J Med Sci sports. (2023) 33:4–19. doi: 10.1111/sms.14241
171. Guo Y, Xu F, Lu T, Duan Z, Zhang Z. Interleukin-6 signaling pathway in targeted therapy for cancer. Cancer Treat Rev. (2012) 38:904–10. doi: 10.1016/j.ctrv.2012.04.007
172. Belizário JE, Fontes-Oliveira CC, Borges JP, Kashiabara JA, Vannier E. Skeletal muscle wasting and renewal: A pivotal role of myokine il-6. SpringerPlus. (2016) 5:619. doi: 10.1186/s40064-016-2197-2
173. Serrano AL, Baeza-Raja B, Perdiguero E, Jardí M, Muñoz-Cánoves P. Interleukin-6 is an essential regulator of satellite cell-mediated skeletal muscle hypertrophy. Cell Metab. (2008) 7:33–44. doi: 10.1016/j.cmet.2007.11.011
174. Dethlefsen C, Højfeldt G, Hojman P. The role of intratumoral and systemic il-6 in breast cancer. Breast Cancer Res Treat. (2013) 138:657–64. doi: 10.1007/s10549-013-2488-z
175. Johnson DE, O'Keefe RA, Grandis JR. Targeting the il-6/jak/stat3 signalling axis in cancer. Nat Rev Clin Oncol. (2018) 15:234–48. doi: 10.1038/nrclinonc.2018.8
176. Chen MF, Chen PT, Lu MS, Lin PY, Chen WC, Lee KD. Il-6 expression predicts treatment response and outcome in squamous cell carcinoma of the esophagus. Mol Cancer. (2013) 12:26. doi: 10.1186/1476-4598-12-26
177. Fujita J, Tsujinaka T, Yano M, Ebisui C, Saito H, Katsume A, et al. Anti-interleukin-6 receptor antibody prevents muscle atrophy in colon-26 adenocarcinoma-bearing mice with modulation of lysosomal and atp-ubiquitin-dependent proteolytic pathways. Int J Cancer. (1996) 68:637–43. doi: 10.1002/(sici)1097-0215(19961127)68:5<637::Aid-ijc14>3.0.Co;2-z
178. Muñoz-Cánoves P, Scheele C, Pedersen BK, Serrano AL. Interleukin-6 myokine signaling in skeletal muscle: A double-edged sword? FEBS J. (2013) 280:4131–48. doi: 10.1111/febs.12338
179. De Benedetti F, Alonzi T, Moretta A, Lazzaro D, Costa P, Poli V, et al. Interleukin 6 causes growth impairment in transgenic mice through a decrease in insulin-like growth factor-I. A model for stunted growth in children with chronic inflammation. J Clin Invest. (1997) 99:643–50. doi: 10.1172/jci119207
180. Nemet D, Eliakim A, Zaldivar F, Cooper DM. Effect of rhil-6 infusion on gh–>Igf-I axis mediators in humans. Am J Physiol Regulatory Integr Comp Physiol. (2006) 291:R1663–8. doi: 10.1152/ajpregu.00053.2006
181. Glass DJ. Molecular mechanisms modulating muscle mass. Trends Mol Med. (2003) 9:344–50. doi: 10.1016/s1471-4914(03)00138-2
182. Yoshida T, Delafontaine P. Mechanisms of igf-1-mediated regulation of skeletal muscle hypertrophy and atrophy. Cells. (2020) 9(9):1970. doi: 10.3390/cells9091970
183. Martín AI, Priego T, Moreno-Ruperez Á, González-Hedström D, Granado M, López-Calderón A. Igf-1 and igfbp-3 in inflammatory cachexia. Int J Mol Sci. (2021) 22(17):9469. doi: 10.3390/ijms22179469
184. Engert JC, Berglund EB, Rosenthal N. Proliferation precedes differentiation in igf-I-stimulated myogenesis. J Cell Biol. (1996) 135:431–40. doi: 10.1083/jcb.135.2.431
185. Chakravarthy MV, Abraha TW, Schwartz RJ, Fiorotto ML, Booth FW. Insulin-like growth factor-I extends in vitro replicative life span of skeletal muscle satellite cells by enhancing G1/S cell cycle progression via the activation of phosphatidylinositol 3'-kinase/akt signaling pathway. J Biol Chem. (2000) 275:35942–52. doi: 10.1074/jbc.M005832200
186. Zhang L, Wang XH, Wang H, Du J, Mitch WE. Satellite cell dysfunction and impaired igf-1 signaling cause ckd-induced muscle atrophy. J Am Soc Nephrology: JASN. (2010) 21:419–27. doi: 10.1681/asn.2009060571
187. Feng L, Li B, Xi Y, Cai M, Tian Z. Aerobic exercise and resistance exercise alleviate skeletal muscle atrophy through igf-1/igf-1r-pi3k/akt pathway in mice with myocardial infarction. Am J Physiol Cell Physiol. (2022) 322(2):C164–76. doi: 10.1152/ajpcell.00344.2021
188. Chen JL, Colgan TD, Walton KL, Gregorevic P, Harrison CA. The tgf-β Signalling network in muscle development, adaptation and disease. Adv Exp Med Biol. (2016) 900:97–131. doi: 10.1007/978-3-319-27511-6_5
189. Bonaldo P, Sandri M. Cellular and molecular mechanisms of muscle atrophy. Dis Model Mech. (2013) 6:25–39. doi: 10.1242/dmm.010389
190. Hoogaars WMH, Jaspers RT. Past, present, and future perspective of targeting myostatin and related signaling pathways to counteract muscle atrophy. Adv Exp Med Biol. (2018) 1088:153–206. doi: 10.1007/978-981-13-1435-3_8
191. Rebbapragada A, Benchabane H, Wrana JL, Celeste AJ, Attisano L. Myostatin signals through a transforming growth factor beta-like signaling pathway to block adipogenesis. Mol Cell Biol. (2003) 23:7230–42. doi: 10.1128/mcb.23.20.7230-7242.2003
192. Zhu X, Topouzis S, Liang LF, Stotish RL. Myostatin signaling through smad2, smad3 and smad4 is regulated by the inhibitory smad7 by a negative feedback mechanism. Cytokine. (2004) 26:262–72. doi: 10.1016/j.cyto.2004.03.007
193. McFarlane C, Plummer E, Thomas M, Hennebry A, Ashby M, Ling N, et al. Myostatin induces cachexia by activating the ubiquitin proteolytic system through an nf-kappab-independent, foxo1-dependent mechanism. J Cell Physiol. (2006) 209:501–14. doi: 10.1002/jcp.20757
194. Bollinger LM, Witczak CA, Houmard JA, Brault JJ. Smad3 augments foxo3-induced murf-1 promoter activity in a DNA-binding-dependent manner. Am J Physiol Cell Physiol. (2014) 307(3):C278–87. doi: 10.1152/ajpcell.00391.2013
195. Smith RC, Lin BK. Myostatin inhibitors as therapies for muscle wasting associated with cancer and other disorders. Curr Opin supportive palliative Care. (2013) 7:352–60. doi: 10.1097/spc.0000000000000013
196. Chen JA, Splenser A, Guillory B, Luo J, Mendiratta M, Belinova B, et al. Ghrelin prevents tumour- and cisplatin-induced muscle wasting: characterization of multiple mechanisms involved. J cachexia sarcopenia Muscle. (2015) 6:132–43. doi: 10.1002/jcsm.12023
197. Zimmers TA, Davies MV, Koniaris LG, Haynes P, Esquela AF, Tomkinson KN, et al. Induction of cachexia in mice by systemically administered myostatin. Sci (New York NY). (2002) 296:1486–8. doi: 10.1126/science.1069525
198. Bhattacharya I, Manukyan Z, Chan P, Heatherington A, Harnisch L. Application of quantitative pharmacology approaches in bridging pharmacokinetics and pharmacodynamics of domagrozumab from adult healthy subjects to pediatric patients with duchenne muscular disease. J Clin Pharmacol. (2018) 58:314–26. doi: 10.1002/jcph.1015
199. Becker C, Lord SR, Studenski SA, Warden SJ, Fielding RA, Recknor CP, et al. Myostatin antibody (Ly2495655) in older weak fallers: A proof-of-concept, randomised, phase 2 trial. Lancet Diabetes Endocrinol. (2015) 3:948–57. doi: 10.1016/s2213-8587(15)00298-3
200. Desgeorges MM, Devillard X, Toutain J, Castells J, Divoux D, Arnould DF, et al. Pharmacological inhibition of myostatin improves skeletal muscle mass and function in a mouse model of stroke. Sci Rep. (2017) 7:14000. doi: 10.1038/s41598-017-13912-0
201. Zhang L, Rajan V, Lin E, Hu Z, Han HQ, Zhou X, et al. Pharmacological inhibition of myostatin suppresses systemic inflammation and muscle atrophy in mice with chronic kidney disease. FASEB journal: Off Publ Fed Am Societies Exp Biol. (2011) 25:1653–63. doi: 10.1096/fj.10-176917
202. Hojman P, Gehl J, Christensen JF, Pedersen BK. Molecular mechanisms linking exercise to cancer prevention and treatment. Cell Metab. (2018) 27:10–21. doi: 10.1016/j.cmet.2017.09.015
203. Yin L, Li N, Jia W, Wang N, Liang M, Yang X, et al. Skeletal muscle atrophy: from mechanisms to treatments. Pharmacol Res. (2021) 172:105807. doi: 10.1016/j.phrs.2021.105807
204. Hojman P, Fjelbye J, Zerahn B, Christensen JF, Dethlefsen C, Lonkvist CK, et al. Voluntary exercise prevents cisplatin-induced muscle wasting during chemotherapy in mice. PloS One. (2014) 9:e109030. doi: 10.1371/journal.pone.0109030
205. Puklin LS, Ferrucci LM, Harrigan M, McGowan C, Zupa M, Cartmel B, et al. Improving lifestyle behaviors during chemotherapy for breast cancer: the lifestyle, exercise, and nutrition early after diagnosis (Leaner) trial. Cancer. (2024) 130:2440–52. doi: 10.1002/cncr.35280
206. Anton SD, Hida A, Mankowski R, Layne A, Solberg LM, Mainous AG, et al. Nutrition and exercise in sarcopenia. Curr Protein Pept Sci. (2018) 19:649–67. doi: 10.2174/1389203717666161227144349
207. Papadopoulou SK, Papadimitriou K, Voulgaridou G, Georgaki E, Tsotidou E, Zantidou O, et al. Exercise and nutrition impact on osteoporosis and sarcopenia-the incidence of osteosarcopenia: A narrative review. Nutrients. (2021) 13(12):4499. doi: 10.3390/nu13124499
208. Parsowith EJ, Stock MS, Kocuba O, Schumpp A, Jackson K, Brooks AM, et al. Impact of short-term creatine supplementation on muscular performance among breast cancer survivors. Nutrients. (2024) 16(7):979. doi: 10.3390/nu16070979
209. Dolan E, Artioli GG, Pereira RMR, Gualano B. Muscular atrophy and sarcopenia in the elderly: is there a role for creatine supplementation? Biomolecules. (2019) 9(11):642. doi: 10.3390/biom9110642
210. Martinez-Aguirre-Betolaza A, Cacicedo J, Castañeda-Babarro A. Creatine supplementation and resistance training in patients with breast cancer (Cartic study): protocol for a randomized controlled trial. Am J Clin Oncol. (2024) 47:161–8. doi: 10.1097/coc.0000000000001070
211. Brown JC, Spielmann G, Yang S, Compton SLE, Jones LW, Irwin ML, et al. Effects of exercise or metformin on myokine concentrations in patients with breast and colorectal cancer: A phase ii multi-centre factorial randomized trial. J cachexia sarcopenia Muscle. (2024) 15:1520–7. doi: 10.1002/jcsm.13509
212. Lee KJ, An KO. Impact of high-intensity circuit resistance exercise on physical fitness, inflammation, and immune cells in female breast cancer survivors: A randomized control trial. Int J Environ Res Public Health. (2022) 19(9):5463. doi: 10.3390/ijerph19095463
213. Hiraoui M, Gmada N, Al-Hadabi B, Mezlini A, Al Busafi M, Doutrellot PL, et al. Effects of multimodal training program on muscle deoxygenation in women with breast cancer: A randomized controlled trial. Physiol Int. (2022) 109:246–60. doi: 10.1556/2060.2022.00148
214. Mader T, Chaillou T, Alves ES, Jude B, Cheng AJ, Kenne E, et al. Exercise reduces intramuscular stress and counteracts muscle weakness in mice with breast cancer. J cachexia sarcopenia Muscle. (2022) 13:1151–63. doi: 10.1002/jcsm.12944
215. Khosravi N, Hanson ED, Farajivafa V, Evans WS, Lee JT, Danson E, et al. Exercise-induced modulation of monocytes in breast cancer survivors. Brain Behav Immun Health. (2021) 14:100216. doi: 10.1016/j.bbih.2021.100216
216. Hiensch AE, Mijwel S, Bargiela D, Wengström Y, May AM, Rundqvist H. Inflammation mediates exercise effects on fatigue in patients with breast cancer. Med Sci sports Exercise. (2021) 53:496–504. doi: 10.1249/mss.0000000000002490
217. Schauer T, Mazzoni AS, Henriksson A, Demmelmaier I, Berntsen S, Raastad T, et al. Exercise intensity and markers of inflammation during and after (Neo-) adjuvant cancer treatment. Endocr Relat Cancer. (2021) 28:191–201. doi: 10.1530/erc-20-0507
218. Alizadeh AM, Isanejad A, Sadighi S, Mardani M, Kalaghchi B, Hassan ZM. High-intensity interval training can modulate the systemic inflammation and hsp70 in the breast cancer: A randomized control trial. J Cancer Res Clin Oncol. (2019) 145:2583–93. doi: 10.1007/s00432-019-02996-y
219. Schmidt ME, Meynköhn A, Habermann N, Wiskemann J, Oelmann J, Hof H, et al. Resistance exercise and inflammation in breast cancer patients undergoing adjuvant radiation therapy: mediation analysis from a randomized, controlled intervention trial. Int J Radiat Oncol Biol Phys. (2016) 94:329–37. doi: 10.1016/j.ijrobp.2015.10.058
220. Dieli-Conwright CM, Sami N, Norris MK, Wan J, Kumagai H, Kim SJ, et al. Effect of aerobic and resistance exercise on the mitochondrial peptide mots-C in hispanic and non-hispanic white breast cancer survivors. Sci Rep. (2021) 11:16916. doi: 10.1038/s41598-021-96419-z
221. Møller AB, Lønbro S, Farup J, Voss TS, Rittig N, Wang J, et al. Molecular and cellular adaptations to exercise training in skeletal muscle from cancer patients treated with chemotherapy. J Cancer Res Clin Oncol. (2019) 145:1449–60. doi: 10.1007/s00432-019-02911-5
222. Evans ES, Little J, McNeill KT, Poole C, Bailey SP. Hypothalamic-pituitary-adrenal axis responses to an acute bout of moderate intensity aerobic exercise in breast cancer survivors. FASEB J. (2019) 33:534. doi: 10.1096/fasebj.2019.33.1_supplement.534.3
223. Toohey K, Pumpa K, McKune A, Cooke J, Welvaert M, Northey J, et al. The impact of high-intensity interval training exercise on breast cancer survivors: A pilot study to explore fitness, cardiac regulation and biomarkers of the stress systems. BMC Cancer. (2020) 20:787. doi: 10.1186/s12885-020-07295-1
224. Courneya KS, Segal RJ, Mackey JR, Gelmon K, Reid RD, Friedenreich CM, et al. Effects of aerobic and resistance exercise in breast cancer patients receiving adjuvant chemotherapy: A multicenter randomized controlled trial. J Clin oncology: Off J Am Soc Clin Oncol. (2007) 25:4396–404. doi: 10.1200/jco.2006.08.2024
225. Schmidt T, Weisser B, Dürkop J, Jonat W, Van Mackelenbergh M, Röcken C, et al. Comparing endurance and resistance training with standard care during chemotherapy for patients with primary breast cancer. Anticancer Res. (2015) 35:5623–9.
226. Campbell KL, Lane K, Martin AD, Gelmon KA, McKenzie DC. Resting energy expenditure and body mass changes in women during adjuvant chemotherapy for breast cancer. Cancer Nurs. (2007) 30:95–100. doi: 10.1097/01.NCC.0000265004.64440.5f
227. Schmitz KH, Courneya KS, Matthews C, Demark-Wahnefried W, Galvão DA, Pinto BM, et al. American college of sports medicine roundtable on exercise guidelines for cancer survivors. Med Sci sports Exercise. (2010) 42:1409–26. doi: 10.1249/MSS.0b013e3181e0c112
228. Hayes SC, Newton RU, Spence RR, Galvão DA. The exercise and sports science Australia position statement: exercise medicine in cancer management. J Sci Med Sport. (2019) 22:1175–99. doi: 10.1016/j.jsams.2019.05.003
229. Lynch GS, Schertzer JD, Ryall JG. Therapeutic approaches for muscle wasting disorders. Pharmacol Ther. (2007) 113:461–87. doi: 10.1016/j.pharmthera.2006.11.004
230. McNeely ML, Campbell KL, Rowe BH, Klassen TP, Mackey JR, Courneya KS. Effects of exercise on breast cancer patients and survivors: A systematic review and meta-analysis. CMAJ: Can Med Assoc J = J l'Association medicale Can. (2006) 175:34–41. doi: 10.1503/cmaj.051073
231. Courneya KS, Segal RJ, McKenzie DC, Dong H, Gelmon K, Friedenreich CM, et al. Effects of exercise during adjuvant chemotherapy on breast cancer outcomes. Med Sci sports Exercise. (2014) 46:1744–51. doi: 10.1249/mss.0000000000000297
232. Li H, Sang D, Gong L, Wang B, Wang Y, Jia X, et al. Improving physical and mental health in women with breast cancer undergoing anthracycline-based chemotherapy through wearable device-based aerobic exercise: A randomized controlled trial. Front Public Health. (2024) 12:1451101. doi: 10.3389/fpubh.2024.1451101
233. Antunes P, Joaquim A, Sampaio F, Nunes C, Ascensão A, Vilela E, et al. Exercise training benefits health-related quality of life and functional capacity during breast cancer chemotherapy: A randomized controlled trial. Med Sci sports Exercise. (2024) 56:600–11. doi: 10.1249/mss.0000000000003341
234. Casanovas-Álvarez A, Estanyol B, Ciendones M, Padròs J, Cuartero J, Barnadas A, et al. Effectiveness of an exercise and educational-based prehabilitation program in patients with breast cancer receiving neoadjuvant chemotherapy (Preoptimize) on functional outcomes: A randomized controlled trial. Phys Ther. (2024) 104(12). doi: 10.1093/ptj/pzae151
235. Vikmoen O, Strandberg E, Svindland KV, Henriksson A, Mazzoni AS, Johansson B, et al. Effects of heavy-load strength training during (Neo-)Adjuvant chemotherapy on muscle strength, muscle fiber size, myonuclei, and satellite cells in women with breast cancer. FASEB journal: Off Publ Fed Am Societies Exp Biol. (2024) 38:e23784. doi: 10.1096/fj.202400634R
236. Artigas-Arias M, Alegría-Molina A, Vidal-Seguel N, Muñoz-Cofre R, Carranza-Leiva J, Sepúlveda-Lara A, et al. Skeletal muscle mass, strength, and physical performance gains are similar between healthy postmenopausal women and postmenopausal breast cancer survivors after 12 weeks of resistance exercise training. Supportive Care cancer: Off J Multinational Assoc Supportive Care Cancer. (2024) 32:818. doi: 10.1007/s00520-024-08973-7
237. Pagola I, Morales JS, Alejo LB, Barcelo O, Montil M, Oliván J, et al. Concurrent exercise interventions in breast cancer survivors with cancer-related fatigue. Int J Sports Med. (2020) 41:790–7. doi: 10.1055/a-1147-1513
238. Carpenter DJ, Peluso C, Hilton C, Velasquez F, Annichine A, Matsko K, et al. Exert-bc: A pilot study of an exercise regimen designed to improve functional mobility, body composition, and strength after the treatment for breast cancer. Cancer Med. (2024) 13:e7001. doi: 10.1002/cam4.7001
239. Santagnello SB, Martins FM, de Oliveira Junior GN, de Freitas Rodrigues de Sousa J, Nomelini RS, Murta EFC, et al. Improvements in muscle strength, power, and size and self-reported fatigue as mediators of the effect of resistance exercise on physical performance breast cancer survivor women: A randomized controlled trial. Supportive Care cancer: Off J Multinational Assoc Supportive Care Cancer. (2020) 28:6075–84. doi: 10.1007/s00520-020-05429-6
240. Ramadan AM, ElDeeb AM, Ramadan AA, Aleshmawy DM. Effect of combined kinesiotaping and resistive exercise on muscle strength and quality of life in breast cancer survivors: A randomized clinical trial. J Egypt Natl Canc Inst. (2024) 36:1. doi: 10.1186/s43046-023-00205-z
241. Rasmussen GHF, Kristiansen M, Arroyo-Morales M, Voigt M, Madeleine P. The analgesic effect of resistance training after breast cancer (Antrac): A randomized controlled trial. Med Sci sports Exercise. (2023) 55:167–76. doi: 10.1249/mss.0000000000003034
242. Dieli-Conwright CM, Courneya KS, Demark-Wahnefried W, Sami N, Lee K, Buchanan TA, et al. Effects of aerobic and resistance exercise on metabolic syndrome, sarcopenic obesity, and circulating biomarkers in overweight or obese survivors of breast cancer: A randomized controlled trial. J Clin oncology: Off J Am Soc Clin Oncol. (2018) 36:875–83. doi: 10.1200/jco.2017.75.7526
243. Delrieu L, Martin A, Touillaud M, Pérol O, Morelle M, Febvey-Combes O, et al. Sarcopenia and serum biomarkers of oxidative stress after a 6-month physical activity intervention in women with metastatic breast cancer: results from the able feasibility trial. Breast Cancer Res Treat. (2021) 188:601–13. doi: 10.1007/s10549-021-06238-z
244. Sacomori C, Lorca LA, Martinez-Mardones M, Salas-Ocaranza RI, Reyes-Reyes GP, Pizarro-Hinojosa MN, et al. A randomized clinical trial to assess the effectiveness of pre- and post-surgical pelvic floor physiotherapy for bowel symptoms, pelvic floor function, and quality of life of patients with rectal cancer: carret protocol. Trials. (2021) 22:448. doi: 10.1186/s13063-021-05396-1
245. Winters-Stone KM, Torgrimson-Ojerio B, Dieckmann NF, Stoyles S, Mitri Z, Luoh SW. A randomized-controlled trial comparing supervised aerobic training to resistance training followed by unsupervised exercise on physical functioning in older breast cancer survivors. J geriatric Oncol. (2022) 13:152–60. doi: 10.1016/j.jgo.2021.08.003
246. Binder EF, Yarasheski KE, Steger-May K, Sinacore DR, Brown M, Schechtman KB, et al. Effects of progressive resistance training on body composition in frail older adults: results of a randomized, controlled trial. journals gerontology Ser A Biol Sci Med Sci. (2005) 60:1425–31. doi: 10.1093/gerona/60.11.1425
247. Bonnefoy M, Cornu C, Normand S, Boutitie F, Bugnard F, Rahmani A, et al. The effects of exercise and protein-energy supplements on body composition and muscle function in frail elderly individuals: A long-term controlled randomised study. Br J Nutr. (2003) 89:731–9. doi: 10.1079/bjn2003836
248. Cruz-Jentoft AJ, Landi F, Schneider SM, Zúñiga C, Arai H, Boirie Y, et al. Prevalence of and interventions for sarcopenia in ageing adults: A systematic review. Report of the international sarcopenia initiative (Ewgsop and iwgs). Age Ageing. (2014) 43:748–59. doi: 10.1093/ageing/afu115
249. Dent E, Morley JE, Cruz-Jentoft AJ, Arai H, Kritchevsky SB, Guralnik J, et al. International clinical practice guidelines for sarcopenia (Icfsr): screening, diagnosis and management. J nutrition Health Aging. (2018) 22:1148–61. doi: 10.1007/s12603-018-1139-9
250. van Waart H, Stuiver MM, van Harten WH, Geleijn E, Kieffer JM, Buffart LM, et al. Effect of low-intensity physical activity and moderate- to high-intensity physical exercise during adjuvant chemotherapy on physical fitness, fatigue, and chemotherapy completion rates: results of the paces randomized clinical trial. J Clin oncology: Off J Am Soc Clin Oncol. (2015) 33:1918–27. doi: 10.1200/jco.2014.59.1081
251. Campbell KL, Winters-Stone KM, Wiskemann J, May AM, Schwartz AL, Courneya KS, et al. Exercise guidelines for cancer survivors: consensus statement from international multidisciplinary roundtable. Med Sci sports Exercise. (2019) 51:2375–90. doi: 10.1249/mss.0000000000002116
252. Ligibel JA, Bohlke K, May AM, Clinton SK, Demark-Wahnefried W, Gilchrist SC, et al. Exercise, diet, and weight management during cancer treatment: asco guideline. J Clin oncology: Off J Am Soc Clin Oncol. (2022) 40:2491–507. doi: 10.1200/jco.22.00687
253. Binyam D, Naaktgeboren WR, Groen WG, Aaronson NK, Hiensch AE, van Harten WH, et al. Eight-year follow-up of patient-reported outcomes in patients with breast cancer participating in exercise studies during chemotherapy. J Cancer Surviv. (2024). doi: 10.1007/s11764-024-01640-0
254. Matsuura H, Ehara T. Modulation of the muscarinic K+ Channel by P2-purinoceptors in Guinea-pig atrial myocytes. J Physiol. (1996) 497 :379–93. doi: 10.1113/jphysiol.1996.sp021775
255. Saeman MR, DeSpain K, Liu MM, Carlson BA, Song J, Baer LA, et al. Effects of exercise on soleus in severe burn and muscle disuse atrophy. J Surg Res. (2015) 198:19–26. doi: 10.1016/j.jss.2015.05.038
256. Roesner K, Scheffler B, Kaehler M, Schmidt-Maciejewski B, Boettger T, Saal S. Effects of physical therapy modalities for motor function, functional recovery, and post-stroke complications in patients with severe stroke: A systematic review update. Syst Rev. (2024) 13:270. doi: 10.1186/s13643-024-02676-0
Keywords: breast cancer post-chemotherapy, skeletal muscle dysfunction, inflammatory factors, sarcopenia, exercise intervention, muscle atrophy
Citation: Zhong P, Li X and Li J (2025) Mechanisms, assessment, and exercise interventions for skeletal muscle dysfunction post-chemotherapy in breast cancer: from inflammation factors to clinical practice. Front. Oncol. 15:1551561. doi: 10.3389/fonc.2025.1551561
Received: 25 December 2024; Accepted: 13 February 2025;
Published: 04 March 2025.
Edited by:
Jorge Morales-Montor, National Autonomous University of Mexico, MexicoReviewed by:
Rebekah Wilson, Dana–Farber Cancer Institute, United StatesCopyright © 2025 Zhong, Li and Li. This is an open-access article distributed under the terms of the Creative Commons Attribution License (CC BY). The use, distribution or reproduction in other forums is permitted, provided the original author(s) and the copyright owner(s) are credited and that the original publication in this journal is cited, in accordance with accepted academic practice. No use, distribution or reproduction is permitted which does not comply with these terms.
*Correspondence: Jiehua Li, bGlqaWVodWEwMUBzaW5hLmNvbQ==
†These authors have contributed equally to this work and share first authorship
Disclaimer: All claims expressed in this article are solely those of the authors and do not necessarily represent those of their affiliated organizations, or those of the publisher, the editors and the reviewers. Any product that may be evaluated in this article or claim that may be made by its manufacturer is not guaranteed or endorsed by the publisher.
Research integrity at Frontiers
Learn more about the work of our research integrity team to safeguard the quality of each article we publish.