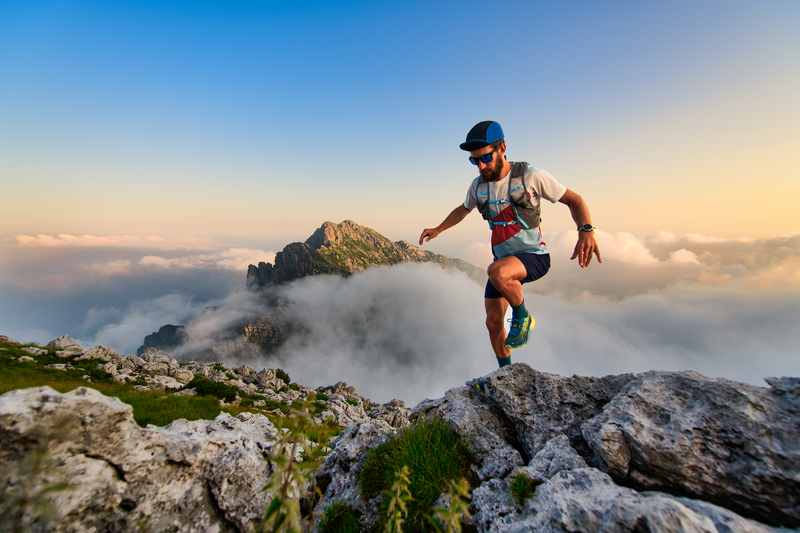
95% of researchers rate our articles as excellent or good
Learn more about the work of our research integrity team to safeguard the quality of each article we publish.
Find out more
REVIEW article
Front. Oncol. , 14 March 2025
Sec. Cancer Immunity and Immunotherapy
Volume 15 - 2025 | https://doi.org/10.3389/fonc.2025.1519355
This article is part of the Research Topic Immuno-Metabolic Interactions and Cancer Progression in the Tumor Microenvironment View all articles
The research and development of new anti-cancer drugs face challenges such as high costs, lengthy development cycles, and limited data on side effects. In contrast, the clinical safety and side effects of traditional drugs have been well established through long-term use. The development or repurposing of traditional drugs with potential applications in cancer treatment offers an economical, feasible, and promising strategy for new drug development. This article reviews the novel applications of traditional drugs in tumor immunotherapy, discussing how they can enhance tumor treatment efficacy through functional repositioning, while also reducing development time and costs. Recent advancements in cancer immunotherapy have revolutionized treatment options, but resistance to ICIs remains a significant challenge. Drug repurposing has emerged as a promising strategy to identify novel agents that can enhance the efficacy of immunotherapies by overcoming ICI resistance. A study suggests that drug repositioning has the potential to modulate immune cell activity or alter the tumor microenvironment, thereby circumventing the resistance mechanisms associated with immune checkpoint blockade. This approach provides a rapid and cost-effective pathway for identifying therapeutic candidates that can be quickly transitioned into clinical trials. To improve the effectiveness of tumor immunotherapy, it is crucial to explore systematic methods for identifying repurposed drug candidates. Methods such as high-throughput screening, computational drug repositioning, and bioinformatic analysis have been employed to efficiently identify potential candidates for cancer treatment. Furthermore, leveraging databases related to immunotherapy and drug repurposing can provide valuable resources for drug discovery and facilitate the identification of promising compounds. It focuses on the latest advancements in the use of antidiabetic drugs, antihypertensive agents, weight-loss medications, antifungal agents, and antiviral drugs in tumor immunotherapy, examining their mechanisms of action, clinical application prospects, and associated challenges. In this context, our aim is to explore these strategies and highlight their potential for expanding the therapeutic options available for cancer immunotherapy, providing valuable references for cancer research and treatment.
As tumor treatment methodologies continue to evolve, conventional approaches prove insufficient in addressing the complexities and variability of tumors. As a groundbreaking therapeutic strategy, tumor immunotherapy aims to disrupt the immune evasion mechanisms employed by tumor cells, reinitiate and sustain the tumor immune cycle, and restore the body’s normal anti-tumor immune response. Through various modalities—including monoclonal antibodies, immune checkpoint inhibitors, therapeutic antibodies, cancer vaccines, cell therapy, and small molecule inhibitors—immunotherapy can precisely target tumors and has the potential for personalized treatment, paving a new avenue for tumor management. In this context, the concept of repurposing old drugs has emerged. Scientists are able to efficiently identify drug candidates with repurposing potential by utilizing phenotypic screening, CRISPR/Cas9 functional screening, and virtual screening combined with machine learning. These methods rely on high-throughput experimental techniques and computational models, specifically focusing on discovering novel drugs that can reverse T-cell exhaustion, regulate tumor immune function, or ameliorate the immunosuppressive tumor microenvironment (1). In addition to their unique mechanisms of action, many traditional drugs have demonstrated significant efficacy in regulating the immune system, providing fresh perspectives for tumor treatment. For instance, recent studies have shown that metformin inhibits tumor cell growth and metastasis, with its mechanism linked to immune regulation. Similarly, antihypertensive agents have been found to enhance immune cell activity and promote anti-tumor immune responses, underscoring the potential of established drugs in tumor immunotherapy and offering valuable insights for further exploration of novel applications for these agents. This review aims to systematically summarize the new advancements of old drugs in tumor immunotherapy, analyze their potential mechanisms, and anticipate their broad applicability (Figure 1; Table 1). By uncovering the new value of old drugs, this research seeks to inject renewed vitality into tumor treatment and bring greater benefits to patients.
Figure 1. Schematic representation of repurposed conventional drugs in cancer immunotherapy. This figure illustrates seven categories of conventional medications—hypoglycemic agents, antihypertensives, weight-loss drugs, antifungal drugs, antiviral drugs, mood stabilizers, and alcohol deterrents—arranged around a central tumor cell. Each sector highlights representative agents, their principal mechanisms (e.g., metabolic reprogramming, induction of immunogenic cell death), and their capacity to enhance tumor immunity (such as augmenting checkpoint blockade). By modulating the tumor microenvironment and immune cell function, these “old drugs with new uses” show promise in strengthening antitumor immune responses and improving clinical treatment outcomes.
Table 1. The immunosensitizing effect of old drugs on solid tumors has been observed in recent studies.
Traditional antidiabetic drugs, such as Metformin (Met), have shown remarkable potential in cancer treatment, exhibiting effects such as metabolic regulation, anti-cancer properties, and immune modulation. Metformin, a small molecule drug commonly used for treating type 2 diabetes, has demonstrated metabolic regulatory effects (2), tumor-suppressive properties, and immunomodulatory capabilities.
Met exerts a direct anti-tumor effect by inhibiting cell proliferation and inducing apoptosis. It significantly inhibits proliferation and induces apoptosis in various tumor cell types through modulation of both AMPK-dependent and AMPK-independent pathways (3, 4). Furthermore, it reduces tumor incidence and enhances chemosensitivity (5). Enhanced sensitivity to radiotherapy and chemotherapy: Metformin increases the sensitivity of tumor cells to both radiotherapy and chemotherapy by inhibiting DNA damage repair, regulating the cell cycle, and enhancing cellular metabolism. Additionally, Metformin has multifaceted effects on both the innate and adaptive immune systems (6, 7). It can activate dendritic cells (DCs), promote the proliferation of CD4+ T cells (8), and sustain the activity of cytotoxic T lymphocytes (CTLs). Furthermore, it plays a crucial role in the anti-tumor immune response by regulating the differentiation and activity of peripheral regulatory T cells (Tregs) (9). By increasing the secretion of tumor necrosis factor (TNF-α) (10) and reducing the number of immunosuppressive cells such as myeloid-derived suppressor cells (MDSCs) (11), Metformin can reshape the tumor immune micro-environment and promote tumor cell death. Moreover, it effectively blocks the PD-1/PD-L1 axis (12) by activating AMPK to phosphorylate PD-L1 at serine 195, inducing abnormal glycosylation and degradation of PD-L1. Additionally, it enhances the efficacy of CTLA-4 inhibitors without increasing toxicity, regulates the immune system, and strengthens anti-tumor immune responses. Recent studies have demonstrated that Metformin can inhibit the secretion of pro-inflammatory cytokines by senescent T cells and reduce the expression of the senescence-associated secretory phenotype (SASP), thereby exerting an anti-aging effect and further enhancing the immune response (13) (Figure 2).
Figure 2. Diagram of the mechanisms by which metformin enhances tumor immunity. This figure delineates the action pathways of metformin in enhancing the tumor immune response through three primary mechanisms: activating AMP-activated protein kinase AMPK, inhibiting the IL-6/JAK2/STAT3 pathway, and regulating metabolic pathways.
Through an in-depth exploration of the anti-tumor effects of Metformin and its immunomodulatory mechanisms, researchers have achieved significant findings. These studies confirm Metformin’s ability to inhibit tumor cell proliferation and induce apoptosis by modulating AMPK-dependent and AMPK-independent pathways, while elucidating its unique role in remodeling the tumor immune micro-environment, enhancing sensitivity to radiotherapy and chemotherapy, and regulating the immune system.
Research on Metformin in colorectal cancer (CRC) has shown that it can affect the metabolic pathways of tumor cells and reduce tryptophan uptake, thereby altering the composition of the tumor immune micro-environment (TME). Through single-cell transcriptome analysis, researchers observed an increased proportion of CD8+ T cells and a decreased proportion of CD4+ T cells in the colon tissues of APCmin/+ mice treated with Metformin, alongside an elevated presence of macrophages, plasmacytoid dendritic cells (pDCs), classical dendritic cells 1 (cDC1s), and mast cells. Conversely, the percentage of neutrophils was reduced (14). These alterations contribute to overcoming the immunosuppressive state associated with CRC and offer a novel strategy for immunotherapy.
In studies of esophageal squamous cell carcinoma (ESCC) and other tumor types, Metformin has significantly inhibited disease progression by improving the immunosuppressive tumor micro-environment and immune evasion mechanisms (15). Through various signaling pathways, such as the AMPK/DACH1/CXCL1 axis (16) and the IL-6/JAK2/STAT3 pathway (17), Metformin influences immune cell homeostasis. Combination therapy with PD-1 inhibitors can synergistically enhance anti-tumor immune responses while inhibiting PD-L1 expression. It reduces the migration of immunosuppressive cells, such as MDSCs, and increases the activity of anti-tumor immune cells, including CD8+ T cells and natural killer (NK) cells (18). These findings provide new targets and strategies for tumor immunotherapy.
Metformin has also demonstrated a significant effect in enhancing sensitivity to chemoradiotherapy. By inhibiting DNA damage repair, regulating the cell cycle, and improving cellular metabolism, Metformin can increase the sensitivity of tumor cells to both radiotherapy and chemotherapy, thereby enhancing therapeutic efficacy. In a phase I trial involving head and neck squamous cell carcinoma (HNSCC), patients received 500 mg of metformin daily for 3 days, followed by 1000 mg daily until the day of surgery, for a minimum of 9 days. This regimen of metformin combined with chemoradiotherapy significantly prolonged overall survival and enhanced the activity of NK cells, CD4+ T cells, and CD8+ T cells (19). Additionally, significant progress has been made in the research of Metformin in malignant melanoma (MM) and pancreatic ductal adenocarcinoma (PDAC). In MM, the combination of Metformin and PD-1 antibodies has shown notable antitumor synergistic effects, enhancing the antitumor activity of PD-1 blockade (20) and improving overall survival, although the sample size was relatively small (55 patients) (21). Furthermore, large-scale clinical trials have confirmed that Metformin does not diminish the efficacy of the PD-1 antibody pembrolizumab nor worsen the prognosis for BRAF-mutated MM (22). In PDAC, metformin enhanced the immune status of the TME by inhibiting the infiltration of M2-type tumor-associated macrophages (TAMs) and increasing the infiltration and functionality of immune-activated DCs. In this study, patients were further stratified based on their metformin usage. Those who had been taking metformin for at least 6 months at the time of randomization and adhered to the standard metformin dosage recommended by their treating physicians (ranging from 500 to 2000 mg daily) were classified as “metformin users.” Patients with no history of metformin use were defined as “non-metformin users” (23). Although Metformin did not significantly improve survival in patients with PDAC when used alone in certain clinical trials (24), its potential benefits for patients with early-stage pancreatic tumors warrant further investigation.
In addition to traditional delivery methods, Metformin has been utilized as a novel immune adjuvant to significantly reverse immunosuppression (25) and enhance anticancer immunity in conjunction with photodynamic therapy or chemotherapy. Innovative drug delivery systems, such as novel biomimetic “Gemini nanoimmunomodulators,” have been developed to target tumor cells, PD-L1 checkpoints, and TAMs in a spatiotemporal manner. The precise and safe delivery of Metformin to tumor sites enhances the efficacy of photoimmunotherapy (26). Furthermore, studies have indicated that new glucose-lowering agents, such as glucagon-like peptide-1 (GLP-1) analogues, can offer new strategies and methodologies for tumor immunotherapy by improving NK cell function among other mechanisms (27).
In summary, the research findings regarding Metformin’s anti-tumor effects and its immunomodulatory mechanisms are substantial, providing not only new strategies and methodologies for tumor treatment but also revitalizing the development of immunotherapy. The achievement of these results is a testament to the relentless efforts and exploratory spirit of researchers, offering valuable insights and guidance for future investigations in tumor immunotherapy.
Traditional antihypertensive agents include angiotensin-converting enzyme inhibitors (ACEIs), angiotensin II receptor blockers (ARBs), β-receptor antagonists, calcium channel blockers (CCBs), diuretics, and α2 receptor agonists (28). Numerous studies in recent years have demonstrated that these agents may exert indirect or direct effects on the tumor immune micro-environment by altering tumor angiogenesis and blood supply, thereby influencing tumor growth and immune responses (29).
β-receptor antagonists, exemplified by propranolol, have shown the capacity to inhibit tumor growth and metastasis across various tumor models (30, 31). Preclinical and clinical studies indicate that propranolol can modulate the functional activity of immune cells, restore the vitality of cytotoxic T cells, and reduce the population of MDSCs. Furthermore, propranolol has been shown to enhance the anti-tumor efficacy of ICIs, offering a novel strategy for tumor immunotherapy.
In the tumor micro-environment, both tumor cells and immune cells frequently express ADRB (32). Activation of ADRB can lead to an increase in MDSCs and enhance immunosuppressive functions in a mouse model of breast cancer; however, propranolol can inhibit ADRB signal transduction and reduce the population of MDSCs in the spleen and tumors of tumor-bearing mice (33). In a clinical trial, patients were randomly assigned to one of four groups: a control group, a propranolol group, a parecoxib group, and a combination group receiving both propranolol and parecoxib. Patients in the propranolol group were administered oral propranolol (20 mg, three times daily) from the day of surgery to postoperative day 3. In the parecoxib group, patients received intravenous parecoxib (40 mg once daily) from the day of surgery to postoperative day 2. In the combination group, patients received both propranolol (orally) and parecoxib (intravenously) according to the same schedules. Peripheral blood samples (10 mL) were collected from each patient at five time points: on the morning of surgery (preoperatively, Pre-OP), at the end of surgery (End-OP), and on postoperative days 1 (POD1), 3 (POD3), and 7 (POD7). Additionally, propranolol mitigated the beneficial effects on regulatory T cell (Treg) responses, diminishing the elevation of Tregs induced by surgical stress in breast cancer patients undergoing radical mastectomy (34). Propranolol can delay tumor progression in the MCA205 fibrosarcoma model and the MC38 colon cancer model, increase T cell infiltration, reduce MDSC infiltration, improve the survival rate of tumor-bearing mice, and TAMs to upregulate PD-L1 while altering their chemokine expression profile. This combination may also enhance the antitumor efficacy of ICIs (35, 36). In a mouse model of pancreatic cancer, ICIs alone, atenolol combined with ICIs, or highly selective β2 receptor blockers (ICI118551) combined with ICIs were ineffective in shrinking tumors; however, propranolol—a non-selective β-receptor antagonist—was able to sensitize tumors that were otherwise resistant to immunotherapy. Additionally, markers of T-cell exhaustion, such as PD-1 and TIM-3, exhibited increased expression in these tumors (36).
α2 receptor agonists can directly activate macrophages and promote the recruitment and activation of CD4+ and CD8+ T lymphocytes within the tumor micro-environment. When administered alone, α2 agonists have demonstrated potent antitumor effects across various immunocompetent tumor models, primarily by targeting host cells rather than tumor cells (37).
The AT1 receptor antagonist losartan has been shown to reverse the tumor micro-environment in pancreatic cancer and improve overall survival rates among patients. Losartan can downregulate the expression of immunosuppressive and invasion-related genes in PDAC. Results from phase II clinical trials indicated that, when combined with chemoradiotherapy, losartan significantly reduced the number of immunosuppressive regulatory T cells and FOXP3+ tumor cells within PDAC lesions, increased the infiltration of anti-tumor CD8+ T cells, and readjusted the immunosuppressive micro-environment (38).
However, not all anti-hypertensive agents demonstrate positive antitumor effects; for instance, spironolactone and furosemide attenuate the tumor-suppressive efficacy of PD-1 antibodies, while losartan and hydrochlorothiazide promote tumor growth and diminish the effectiveness of immunotherapy (39). Conversely, verapamil inhibits tumor growth by modulating NFAT2 expression and enhancing the immune response to PD-1 antibodies, making it a viable treatment option for cervical cancer, particularly in patients with hypertension (40). In summary, antihypertensive drugs present new application prospects in regulating the tumor immune micro-environment and augmenting the effects of immunotherapy. Nevertheless, further clinical studies are required to validate the safety and efficacy of these findings to determine optimal medication strategies that maximize their potential in tumor immunotherapy.
In recent years, research on weight-loss medications in the medical field has expanded beyond traditional approaches focused on calorie control and weight management, increasingly highlighting their potential value in tumor immunotherapy. These weight-loss agents primarily facilitate weight reduction by influencing fat absorption, suppressing appetite, enhancing satiety, and improving energy expenditure (41). Although there are currently no specific weight-loss drugs available in the domestic market, existing agents such as orlistat (a fatty acid synthase (FASN) inhibitor) and GLP-1 inhibitors have demonstrated significant weight loss effects.
More importantly, studies have shown that weight-loss medications possess considerable application prospects in tumor immunotherapy. They primarily function through two mechanisms: first, by improving the TME, which enhances the activity and functionality of immune cells by reducing the accumulation of inflammatory factors and cells; second, by augmenting the anti-tumor immune response and activating the immune system to release cytokines such as IFN-γ and IL-2, thereby inhibiting tumor growth and metastasis.
Fatty acid binding protein 1 (FABP1) is overexpressed in various tumors and is closely associated with tumor progression. FASN inhibitors, such as orlistat, can significantly inhibit FABP1 activity. The combined use of these inhibitors with PD-1 inhibitors has demonstrated a synergistic anti-tumor effect in cases of hepatocellular carcinoma (HCC) (42). Additionally, FASN inhibitors promote the infiltration and activation of CD8+ T cells by upregulating the expression of MHC-I, thereby enhancing the efficacy of tumor immunotherapy. It has also been shown that the combination of two distinct FASN inhibitors, orlistat and TVB-2640, with anti-PD-L1 antibodies significantly inhibits tumor growth in vivo (43). Notably, FASN inhibitors markedly enhance the therapeutic effect of PD-1 monoclonal antibodies in patients with intrahepatic cholangiocarcinoma (ICC) associated with liver fluke infection (44), presenting a promising new treatment strategy for liver fluke-related ICC. Furthermore, GLP-1 inhibitors—widely utilized in recent years for their glucose-lowering and weight-loss effects—can restore the tumor-killing capacity NK cells (27).
In conclusion, weight-loss pharmacotherapies are increasingly recognized as promising adjuncts to cancer immunotherapy due to their ability to enhance the TME and bolster anti-tumor immune responses. Research on FASN inhibitors and GLP-1 analogues presents novel strategies for cancer immunotherapy that warrant further attention. Nevertheless, additional investigations and clinical trials are essential to assess potential adverse effects, evaluate efficacy across various tumor types, and determine the safety and effectiveness of combination therapies.
The role of antifungal agents in tumor immunotherapy represents a burgeoning area of research. These agents undergo metabolic processes within the body, inhibiting the activity of the CYP3A4 enzyme in the liver, which serves as both a common metabolic pathway for triazole antifungals and a crucial enzyme for the metabolism of immunosuppressive drugs. Antifungal agents have been shown to suppress tumor growth and proliferation, induce apoptosis in tumor cells, inhibit angiogenesis and various signaling pathways (45, 46), participate in modulating immune responses against tumors, and enhance DNA repair mechanisms along with overall anti-tumor efficacy (47). Investigating combinations of these agents with drugs targeting distinct pathways or cancer stem cells, as well as their potential roles in immune modulation, merits further exploration.
Recent in vitro studies have demonstrated that itraconazole can repolarize tumor-promoting M2 tumor-associated macrophages into an anti-tumor M1-like phenotype (48), which subsequently inhibits the proliferation of cervical cancer cells (49). While research has indicated the potential role of antifungal agents in tumor immunotherapy, further investigation is required to elucidate their specific mechanisms of action, efficacy, and safety. As research advances, antifungal drugs may emerge as significant adjuvant therapies for tumor immunotherapy.
In the realm of antiviral therapy, IFN-α serves as an endogenous regulator that has demonstrated significant antiviral activity at multiple critical stages of the viral replication cycle. Notably, its role in tumor immunotherapy is equally compelling. In this context, IFN-α not only directly activates innate and adaptive immune cells, including CD4+ T cells, CD8+ T cells, and NK cells, but also markedly enhances CTL-mediated cytotoxicity by promoting the expression of class I MHC molecules, thereby playing a crucial role in tumor immune editing processes. Subsequent studies have revealed that the combination of IFN-α with PD-1 blockade exhibits pronounced synergistic effects across various tumor models, including HCC.
In vitro studies have demonstrated that IFN-α increases tumor PD-L1 expression, while PD-1 blockade enhances IFN-α production, resulting in synergistic anti-tumor effects in a B16 melanoma mouse model (50). Clinical phase Ib/II trials have also validated the safety and efficacy of pembrolizumab in combination with PEG-IFN for patients with advanced melanoma (51). Furthermore, the combination of PEG-IFNα and PD-1 blockade significantly promotes T cell infiltration—particularly cytotoxic CD8+ T cells—into the tumor micro-environment, thereby improving the efficacy of PD-1 antibodies and prolonging survival in mice. In HCC, PEG-IFNα attracts CD8+ T cells by inducing CCL4 chemokine expression and upregulates PD-1 levels on these cells; subsequent PD-1 blockade restores T cell-mediated cytotoxicity, achieving a synergistic therapeutic effect against HCC (52).
It is noteworthy that the efficacy of IFN-α in postoperative adjuvant therapy for HCC has been clinically acknowledged and is recommended as one of the preferred treatment options by the latest clinical practice guidelines (53). Domestic studies have also elucidated a novel mechanism through which IFN-α can reshape the tumor immune micro-environment by modulating glucose metabolism in HCC cells, thereby enhancing immunotherapeutic effects. This discovery further substantiates the strategy of combining IFN-α with ICIs, such as PD-1 antibodies, for treating advanced HCC, demonstrating significant efficacy in tumor reduction, metastasis suppression, and survival prolongation (54).
In summary, IFN-α demonstrates significant potential across the domains of antiviral therapy, immune regulation, and tumor immunotherapy. Particularly in the treatment of HCC, the combinatorial strategy involving IFN-α offers a novel approach to immunotherapy, holds promise for addressing ICI treatment resistance, and represents an important clinical application for repurposing established drugs.
Lithium carbonate (LC), recognized as a mood stabilizer, is extensively employed in clinical practice to treat psychiatric disorders such as mania, bipolar disorder, and depression by modulating the release and reuptake of neurotransmitters while promoting the synthesis of specific neurotransmitters like serotonin (55). However, in recent years, the potential role of lithium carbonate in tumor immunotherapy has garnered increasing attention.
Research has demonstrated that the accumulation of lactate within the TME is intricately linked to tumor progression and prognosis. Elevated levels of lactate can impair the functionality of TILs, rendering them immunosuppressive (56), which subsequently fosters tumor growth (57). LC inhibits lysosomal acidification by disrupting proton pump activity and activates specific signaling pathways that facilitate the transport of lactate from the cytoplasm into mitochondria, serving as an energy substrate for CD8+ T cells. This mechanism not only mitigates lactate-induced immunosuppression but also stimulates tumor-reactive CD8+ T cells, indicating potential anti-tumor efficacy. In preclinical studies, lithium carbonate administered either alone or in conjunction with PD-1 monoclonal antibodies has been shown to effectively inhibit tumor growth and extend the survival of mice. Furthermore, treatment with lithium carbonate enhances the activity of tumor-infiltrating CD8+ T cells in colon and breast cancer patients exhibiting elevated lactate levels, indicating the potential of targeting lactate metabolism as a novel strategy for anti-tumor therapy. Subsequent investigations revealed that lithium carbonate augmented the functional capacity of macrophages when delivered in nanoparticle form, enhancing their ability to produce nitric oxide, which may play a role in modulating immune responses within the TME. By significantly altering lysosomal pH and rescuing the diacylglycerol-protein kinase C (PKC) signaling pathway, lithium carbonate facilitates the localization of monocarboxylic acid transporter 1 (MCT1) (58) to the mitochondrial membrane and converts lactate into energy for CD8+ T cells, thereby counteracting the immunosuppressive effects induced by lactate. Additionally, alternative strategies have been proposed, including targeting MCT1 to diminish lactate uptake and inhibiting lactate dehydrogenase A (LDHA) to prevent lactate production, aimed at directly reducing lactate levels and activating T-cell-mediated anti-tumor immunity. In specific experiments, the administration of nano-lithium carbonate (nano-LC) particles into a male mouse model of liver cancer significantly enhanced macrophage functional activity, as evidenced by increased nitric oxide levels, further substantiating the beneficial role of lithium carbonate in modulating the tumor immune micro-environment (59). The assessment of macrophage functional activity following LC nanoparticle injection after liver cancer onset in male mice was conducted through measurements of nitric oxide production, arginase activity, and phagocytosis of zymosan particles. It was observed that nitric oxide levels produced by macrophages progressively increased, indicating that LC nanoparticles positively influence macrophage functionality during liver cancer progression (60).
In conclusion, lithium carbonate has great potential as a mood stabilizer in the field of tumor immunotherapy. As a strategy to target lactate metabolism, LC provides a new avenue for tumor immunotherapy. In the future, more clinical trials and research are needed to verify its efficacy and safety. We cannot ignore the potential side effects of LC; thus, continued study of its mechanism and safety is essential to better apply it in tumor immunotherapy.
Disulfiram (DSF), originally developed as an anti-alcohol addiction medication, is emerging as a potential non-chemotherapeutic adjuvant anticancer agent (61). Molecular encapsulation with cyclodextrin enhances the solubility and stability of DSF. The metal complexes of DSF exhibit tumor cell growth inhibition through various mechanisms. Further investigations have demonstrated that DSF can induce apoptosis in tumor cells by arresting the cell cycle, inhibiting mitochondrial function, and enhancing antitumor immunity (62). Beyond its direct cytotoxic effects, DSF effectively induces immunogenic cell death (ICD) (63) in cancer cells and modulates the immunosuppressive tumor micro-environment, thereby augmenting ICI therapy and triggering systemic immune responses (64–66).
DSF has demonstrated multiple mechanisms of action in anti-tumor immunotherapy, significantly enhancing its antitumor efficacy. Both animal and in vitro studies have indicated that DSF induces apoptosis in CRC cells while upregulating the expression of key signaling molecules associated with ICD, such as calreticulin and heat shock protein 70 (66), thereby activating antitumor immune responses. Furthermore, DSF enhances T cell functionality, interleukin-2 production, T cell proliferation, and metabolic reprogramming by directly binding to and activating lymphocyte-specific protein tyrosine kinase (LCK). Notably, the activation of CD8+ T cells exhibits potent antitumor immune effects against melanoma and colon cancer, which are further amplified when combined with PD-1 inhibitors (67).
Additionally, DSF inhibits tumor growth and metastasis by modulating the function of the chemokine signaling regulator FROUNT, thereby affecting the activity of tumor-associated macrophages (TAMs), reducing intratumoral macrophage accumulation, and inhibiting their functionality while simultaneously increasing the population of cytotoxic CD8+ T cells (68). DSF binds to copper (Cu²+) ions, inhibits glycogen synthase kinase 3 beta (GSK3β) activity through PARP1 signaling inhibition, upregulates programmed death-ligand 1 (PD-L1) expression, and synergistically enhances the efficacy of anti-PD-1 antibodies to effectively slow HCC progression (69). Furthermore, DSF/Cu complexes augment the effectiveness of CD47 blockade, promote dendritic cell maturation, and further enhance CD8+ T cell cytotoxicity via ICD-mediated immune activation (70).
The DSF/Cu complex also promotes type I interferon release and DC maturation, demonstrating enhanced efficacy in the treatment of metastatic breast cancer when combined with cytotoxic agents such as docetaxel (DTX), compared to monotherapy, thereby providing a novel therapeutic strategy for patients with metastasis (71). In non-small cell lung cancer (NSCLC), the combination of DSF with anti-PD-L1 effectively overcomes drug resistance and enhances antitumor effects by modulating hypoxia-inducible factor 1 (HIF-1) signaling (72). Additionally, DSF activates the STING signaling pathway through PARP1 inhibition and upregulates the expression of IFNα and IFNβ, which, in conjunction with chemoimmunotherapy, significantly inhibits PDAC tumor growth in murine models (73). Finally, DSF exhibits superior antitumor efficacy compared to monotherapy in triple-negative breast cancer (TNBC) by enhancing PD-L1 expression via DNMT1-mediated hypomethylation of IRF7 (74).
Taken together, DSF enhances anti-tumor immune responses through multiple mechanisms, providing new hope and directions for the treatment of a variety of cancers.
The repurposing of established drugs in tumor immunotherapy holds significant importance, primarily reflected in three key aspects: accelerating the research and development process, shortening timelines, and reducing costs. Since these drugs have already undergone clinical validation, they can swiftly progress to trial phases with well-established pharmacological profiles and safety data, thereby mitigating research and development risks. Additionally, the identification of new indications allows for the exploration of novel therapeutic targets or pathways that can substantially enhance immunotherapeutic efficacy. For instance, hypoglycemic agents, antihypertensives, lipid-lowering medications, psychotropic drugs, and antibiotics have demonstrated potential anti-tumor effects. By broadening their applications, repurposed drugs can rapidly address clinical needs while expanding the therapeutic scope of marketed products and providing timely and effective treatment options for patients.
The combination of established drugs with immunotherapeutic agents has emerged as a promising strategy to significantly enhance tumor therapeutic efficacy. This approach primarily works through several mechanisms: improving the tumor micro-environment by reducing inflammation and immunosuppression, enhancing immune cell function, increasing the sensitivity of tumor cells to immune recognition, and regulating other immune system components that affect the recruitment and activation of immune cells. This comprehensive approach effectively reduces immune evasion and inhibits tumor cells from escaping immune attack (Figure 3).
Figure 3. Changes in TME before and after drug treatment. This figure illustrates the alterations in the tumor microenvironment before and after drug intervention, emphasizing contrasts in cell composition, signaling pathways, vascular properties, and immune cell infiltration. The left panel depicts the tumor microenvironment prior to treatment, characterized by a substantial presence of myeloid-derived suppressor cells MDSCs, M2 macrophages (M2), and regulatory Tregs, all contributing to an immunosuppressive state. The PD-L1/PD-1 pathway is highly active, facilitating tumor cell evasion of immune surveillance. Vascular structures exhibit increased permeability due to elevated levels of angiogenic factor vascular endothelial growth factor (VEGF), which supports tumor proliferation and metastasis. Concurrently, leukocyte infiltration is limited at this stage; thus, the capacity for immune cells to penetrate the tumor microenvironment is compromised, further exacerbating immunosuppression while high concentrations of immunosuppressive cytokines such as IL-10 and TGF-β prevail. The right panel presents the tumor microenvironment following drug treatment, where there is a marked increase in activation of CTLs and natural NK cells, accompanied by a significant reduction in Treg and MDSC populations. Inhibition of the PD-L1/PD-1 pathway renders the immune system more active while effectively diminishing tumor cell immune evasion capabilities. At this juncture, features indicative of apoptosis are observed within tumor cells—signifying a pronounced effect from enhanced immune responses on these malignant entities. Vascular permeability decreases due to reduced VEGF levels, thereby limiting nutrient access for tumors and impairing their metastatic potential; additionally, white blood cell infiltration increases significantly allowing more immune cells to effectively enter the tumor microenvironment for targeted attack against cancerous tissues. Levels of pro-inflammatory cytokines such as TNF-α and IFN-γ rise substantially further amplifying anti-tumor immunity. The contents depicted in this figure underscore the dynamic influence of drug therapy on the TME, highlighting its transition from an immunosuppressive state to one characterized by immune activation—a critical aspect for effective cancer immunotherapy.
In exploring the indications for established drugs in immunotherapy, it is crucial to consider not only their direct antitumor effects but also their potential interactions with various immune pathways. One such important axis is the PD-1/PD-L1 pathway, which has demonstrated significant efficacy in various tumor immunotherapies. However, it is important to recognize that the PD-1/PD-L1 axis is not the sole mechanism by which tumors evade immune surveillance and clearance. For instance, during T-cell activation, there is a complex interplay between the CTLA-4 and PD-1 pathways. Anti-CTLA-4 treatment can increase PD-1 expression, while anti-PD-1 treatment can reverse CTLA-4-mediated T-cell dysfunction. Similarly, the IDO and TGF-β pathways also exhibit complex interactions with the PD-1/PD-L1 axis, jointly regulating tumor immune escape. These interactions underscore the need for a more holistic approach to tumor immunotherapy that targets multiple pathways simultaneously. Furthermore, in combination tumor therapies, older drugs can also play a pivotal role in enhancing tumor immune responses. By activating AMPK, inhibiting the IL-6/JAK2/STAT3 pathway, and regulating metabolic pathways, these drugs can further bolster the antitumor efficacy of immunotherapies. Given the intertwined nature of these immunosuppressive mechanisms within the tumor microenvironment, the combined use of older drugs with immunotherapies targeting these pathways may produce synergistic effects, leading to even better clinical outcomes. illustrates the multifaceted mechanisms by which established drugs and immunotherapies can work together to enhance tumor therapeutic efficacy.
The analgesic and anesthetic agent ketamine has been shown to participate in immunomodulation by inhibiting mRNA synthesis in lipopolysaccharide (LPS)-activated macrophages, thereby reducing the production of TNF-α, interleukins, and nitric oxide, which subsequently affects macrophage-mediated immunity. The role of ketamine in antitumor therapy remains unexplored (75). Pitavastatin effectively inhibits the expression of IL-33 and prevents the progression from chronic inflammation to tumors by blocking activation of the TBK1-IRF3 signaling pathway (76), which triggers Th2 cell activation and type 2 innate lymphocyte (ILC2) responses that drive type 2 immune reactions. Hydroxychloroquine is primarily utilized for rheumatic diseases, cardiovascular conditions, and chronic kidney disorders; it also exhibits immunomodulatory effects and anti-inflammatory properties. However, there is currently no relevant research within the realm of tumor immunity (77, 78), which opens up new potential for hydroxychloroquine as a multi-system anticancer agent with high efficacy and low toxicity (79), warranting further investigation in the field of tumor immunotherapy. In recent years, numerous in-depth studies have demonstrated that Traditional Chinese Medicine (TCM) exhibits significant potential in regulating the TME, not only reversing the resistance to ICI but also enhancing clinical treatment outcomes and effectively mitigating the severity of immune-related adverse events (80). Specifically, in various tumor-bearing mouse models, artemisinin has been found to inhibit the functional activity of MDSCs in the TME by inducing the transition of macrophages from the M2 to M1 phenotype (81). Furthermore, in lung cancer models, the active ingredient cryptotanshinone from Salviae Miltiorrhizae Radix has been shown to increase the infiltration levels of CD8+ T cells and CD4+ T cells in the TME (82). Similarly, in breast cancer animal models, another extract from Salviae Miltiorrhizae Radix, Salviaric acid B, has demonstrated the ability to enhance the infiltration of CD8+ T cells in the TME (83), thereby amplifying the antitumor effect of PD-1 blockade therapy. Additionally, in experiments targeting the mouse melanoma B16 model, intranasal administration of astragalus polysaccharides activated DC in mesenteric lymph nodes and promoted the activity of NK and T cells, significantly enhancing the antitumor action of anti-PD-L1 monoclonal antibody (mAb) (84).
The clinical efficacy of many repurposed drugs in cancer treatment has thus far been demonstrated only in a subset of patients (85), highlighting the urgent need for systematic workflows and simplified strategies to identify potentially repurposed drugs for enhancing ICI therapy in the era of personalized medicine. To address this challenge, several systematic approaches have been developed for identifying drug repurposing candidates in tumor immunotherapy. These include high-throughput screening (HTS) of existing drug libraries against tumor-immune interaction models to identify immunomodulatory agents (86, 87), computational repositioning using machine learning algorithms to analyze drug-target interactions and predict potential candidates (88), bioinformatic integration of multi-omics data to pinpoint drugs that reverse immune suppression or enhance immune activation (89), CRISPR/Cas9 functional screening to map genome-wide knockout targets to approved drugs (90, 91), and network pharmacology to prioritize candidates with polypharmacological effects on immune checkpoints or tumor microenvironment modulation (92).
To further support drug repositioning studies in immunotherapy, several publicly accessible databases can also be leveraged. These include DrugBank (https://go.drugbank.com), a comprehensive repository of FDA-approved drugs with detailed annotations on targets, pathways, and clinical trial data; ImmPort (https://www.immport.org), which shares datasets from immunotherapy studies, including immune cell profiles and cytokine responses; RepoDB (http://apps.chiragjpgroup.org/repoDB), curating successes and failures of drug repositioning across diseases; ClinicalTrials.gov (https://clinicaltrials.gov), tracking ongoing trials of repurposed drugs in cancer immunotherapy; and PharmGKB (https://www.pharmgkb.org), linking drug-gene interactions to immune-related pathways to aid in mechanistic hypothesis generation (93). Collectively, these databases provide valuable resources for identifying and validating drug repurposing candidates in the context of tumor immunotherapy.
The exploration of established drugs in tumor immunotherapy encompasses several directions: modifying drug delivery methods to enhance efficacy and safety, investigating new therapeutic applications, optimizing dosage and administration, and integrating with novel therapies. Drug function retargeting holds significant promise in anti-tumor immunotherapy. It is essential to conduct comprehensive mechanistic studies, integrate treatment approaches, evaluate safety profiles, and foster interdisciplinary collaboration to provide more effective treatment options for cancer patients.
JD: Writing – original draft, Writing – review & editing. TS: Writing – original draft. JW: Writing – original draft. YX: Writing – original draft. MS: Conceptualization, Data curation, Writing – review & editing. CH: Conceptualization, Writing – review & editing. LS: Funding acquisition, Writing – review & editing. YY: Writing – review & editing. SC: Funding acquisition, Resources, Supervision, Validation, Writing – review & editing.
The author(s) declare that financial support was received for the research and/or publication of this article. This research was generously funded by the General Program of the National Natural Science Foundation of China (Grant Number: 32271225), the Youth Fund Project of the National Natural Science Foundation of China (Grant Number: 82203874), and the Special Project of the International Science and Technology Cooperation Program of the Guangdong Provincial Science and Technology Plan (Grant Number: 20190510). These funding sources facilitated the conduct of the study and the analysis of the collected data. The funders had no involvement in the study design, data collection and analysis, decision to publish, or preparation of the manuscript. We deeply appreciate their support and dedication, which have significantly contributed to the advancement of medical research.
We sincerely thank all members of the research team who contributed to the writing of this review. Their willingness to advance medical knowledge and improve patient outcomes is greatly appreciated. Special thanks are also extended to the funding agencies and institutions that made this research possible. Their financial support and encouragement were crucial in enabling us to conduct this important study. Furthermore, we express our heartfelt gratitude to the external reviewers and editorial board members for their guidance and feedback, which significantly enhanced the quality and clarity of our manuscript.
The authors declare that the research was conducted in the absence of any commercial or financial relationships that could be construed as a potential conflict of interest.
The author(s) declare that no Generative AI was used in the creation of this manuscript.
All claims expressed in this article are solely those of the authors and do not necessarily represent those of their affiliated organizations, or those of the publisher, the editors and the reviewers. Any product that may be evaluated in this article, or claim that may be made by its manufacturer, is not guaranteed or endorsed by the publisher.
1. To KKW, Cho WC. Drug repurposing to circumvent immune checkpoint inhibitor resistance in cancer immunotherapy. Pharmaceutics. (2023) 15:2166. doi: 10.3390/pharmaceutics15082166
2. Bailey CJ. Metformin: Therapeutic profile in the treatment of type 2 diabetes. Diabetes Obes Metab. (2024) 26:3–19. doi: 10.1111/dom.v26.S3
3. Provinciali N, Lazzeroni M, Cazzaniga M, Gorlero F, Dunn BK, DeCensi A. Metformin: risk-benefit profile with a focus on cancer. Expert Opin Drug Safety. (2015) 14:1573–85. doi: 10.1517/14740338.2015.1084289
4. Gonçalves J, Pinto S, Carmo F, Silva C, Andrade N, Martel F. Additive cytotoxic and colony-formation inhibitory effects of aspirin and metformin on PI3KCA-mutant colorectal cancer cells. Int J Mol Sci. (2024) 25:5381. doi: 10.3390/ijms25105381
5. Amengual-Cladera E, Morla-Barcelo PM, Morán-Costoya A, Sastre-Serra J, Pons DG, Valle A, et al. Metformin: from diabetes to cancer-unveiling molecular mechanisms and therapeutic strategies. Biol (Basel). (2024) 13:302. doi: 10.3390/biology13050302
6. Krastinaite I, Charkavliuk S, Navakauskiene R, Borutinskaite VV. Metformin as an enhancer for the treatment of chemoresistant CD34+ Acute myeloid leukemia cells. Genes (Basel). (2024) 15:648. doi: 10.3390/genes15050648
7. O’Connor L, Bailey-Whyte M, Bhattacharya M, Butera G, Hardell KNL, Seidenberg AB, et al. Association of metformin use and cancer incidence: a systematic review and meta-analysis. J Natl Cancer Inst. (2024) 116:518–29. doi: 10.1093/jnci/djae021
8. Turpin R, Liu R, Munne PM, Peura A, Rannikko JH, Philips G, et al. Respiratory complex I regulates dendritic cell maturation in explant model of human tumor immune microenvironment. J Immunother Cancer. (2024) 12:e008053. doi: 10.1136/jitc-2023-008053
9. Eikawa S, Nishida M, Mizukami S, Yamazaki C, Nakayama E, Udono H. Immune-mediated antitumor effect by type 2 diabetes drug, metformin. Proc Natl Acad Sci U S A. (2015) 112:1809–14. doi: 10.1073/pnas.1417636112
10. Nishida M, Yamashita N, Ogawa T, Koseki K, Warabi E, Ohue T, et al. Mitochondrial reactive oxygen species trigger metformin-dependent antitumor immunity via activation of Nrf2/mTORC1/p62 axis in tumor-infiltrating CD8T lymphocytes. J Immunother Cancer. (2021) 9:e002954. doi: 10.1136/jitc-2021-002954
11. Veeramachaneni R, Yu W, Newton JM, Kemnade JO, Skinner HD, Sikora AG, et al. Metformin generates profound alterations in systemic and tumor immunity with associated antitumor effects. J Immunother Cancer. (2021) 9:e002773. doi: 10.1136/jitc-2021-002773
12. Cha JH, Yang WH, Xia W, Wei Y, Chan LC, Lim SO, et al. Metformin promotes antitumor immunity via endoplasmic-reticulum-associated degradation of PD-L1. Mol Cell. (2018) 71:606–20.e7. doi: 10.1016/j.molcel.2018.07.030
13. Yang J, Liu HC, Zhang JQ, Zou JY, Zhang X, Chen WM, et al. The effect of metformin on senescence of T lymphocytes. Immun Ageing. (2023) 20:73. doi: 10.1186/s12979-023-00394-0
14. Huang X, Sun T, Wang J, Hong X, Chen H, Yan T, et al. Metformin reprograms tryptophan metabolism to stimulate CD8+ T-cell function in colorectal cancer. Cancer Res. (2023) 83:2358–71. doi: 10.1158/0008-5472.CAN-22-3042
15. Takei R, Miyashita T, Takada S, Tajima H, Ninomiya I, Takamura H, et al. Dynamic switch of immunity and antitumor effects of metformin in rat spontaneous esophageal carcinogenesis. Cancer Immunol Immunotherap: CII. (2022) 71:777–89. doi: 10.1007/s00262-021-03027-x
16. Qin G, Lian J, Huang L, Zhao Q, Liu S, Zhang Z, et al. Metformin blocks myeloid-derived suppressor cell accumulation through AMPK-DACH1-CXCL1 axis. Oncoimmunology. (2018) 7:e1442167. doi: 10.1080/2162402X.2018.1442167
17. Lu Y, Xin D, Guan L, Xu M, Yang Y, Chen Y, et al. Metformin downregulates PD-L1 expression in esophageal squamous cell catrcinoma by inhibiting IL-6 signaling pathway. Front Oncol. (2021) 11:762523. doi: 10.3389/fonc.2021.762523
18. Wang S, Lin Y, Xiong X, Wang L, Guo Y, Chen Y, et al. Low-dose metformin reprograms the tumor immune microenvironment in human esophageal cancer: results of a phase II clinical trial. Clin Cancer Res: an Off J Am Assoc Cancer Res. (2020) 26:4921–32. doi: 10.1158/1078-0432.CCR-20-0113
19. Crist M, Yaniv B, Palackdharry S, Lehn MA, Medvedovic M, Stone T, et al. Metformin increases natural killer cell functions in head and neck squamous cell carcinoma through CXCL1 inhibition. J Immunother Cancer. (2022) 10:e005632. doi: 10.1136/jitc-2022-005632
20. Kim SH, Li M, Trousil S, Zhang Y, Pasca di Magliano M, Swanson KD, et al. Phenformin inhibits myeloid-derived suppressor cells and enhances the anti-tumor activity of PD-1 blockade in melanoma. J Invest Dermatol. (2017) 137:1740–8. doi: 10.1016/j.jid.2017.03.033
21. Afzal MZ, Mercado RR, Shirai K. Efficacy of metformin in combination with immune checkpoint inhibitors (anti-PD-1/anti-CTLA-4) in metastatic Malignant melanoma. J Immunotherap Cancer. (2018) 6:64. doi: 10.1186/s40425-018-0375-1
22. Kennedy OJ, Kicinski M, Valpione S, Gandini S, Suciu S, Blank CU, et al. Prognostic and predictive value of metformin in the European Organisation for Research and Treatment of Cancer 1325/KEYNOTE-054 phase III trial of pembrolizumab versus placebo in resected high-risk stage III melanoma. Eur J Cancer. (2023) 189:112900. doi: 10.1016/j.ejca.2023.04.016
23. van Eijck CWF, Vadgama D, van Eijck CHJ, Wilmink JW. Metformin boosts anti-tumor immunity and improves prognosis in upfront resected pancreatic cancer: an observational study. J Natl Cancer Inst. (2024) 116:1374–83. doi: 10.1093/jnci/djae070
24. Kordes S, Pollak MN, Zwinderman AH, Mathôt RA, Weterman MJ, Beeker A, et al. Metformin in patients with advanced pancreatic cancer: a double-blind, randomised, placebo-controlled phase 2 trial. Lancet Oncol. (2015) 16:839–47. doi: 10.1016/S1470-2045(15)00027-3
25. Xiong W, Qi L, Jiang N, Zhao Q, Chen L, Jiang X, et al. Metformin liposome-mediated PD-L1 downregulation for amplifying the photodynamic immunotherapy efficacy. ACS Appl Mater Interf. (2021) 13:8026–41. doi: 10.1021/acsami.0c21743
26. Huang H, Li N, Wei X, Li Q, Guo J, Yang G, et al. Biomimetic “Gemini nanoimmunoregulators” orchestrated for boosted photoimmunotherapy by spatiotemporally modulating PD-L1 and tumor-associated macrophages. Acta Pharm Sin B. (2024) 14:1345–61. doi: 10.1016/j.apsb.2023.11.005
27. De Barra C, Khalil M, Mat A, O’Donnell C, Shaamile F, Brennan K, et al. Glucagon-like peptide-1 therapy in people with obesity restores natural killer cell metabolism and effector function. Obes (Silver Spring). (2023) 31:1787–97. doi: 10.1002/oby.23772
28. Chrysant SG, Chrysant GS. New and emerging cardiovascular and antihypertensive drugs. Expert Opin Drug Saf. (2020) 19:1315–27. doi: 10.1080/14740338.2020.1810232
29. Bryniarski P, Nazimek K, Marcinkiewicz J. Immunomodulatory properties of antihypertensive drugs and digitalis glycosides. Expert Rev Cardiovasc Ther. (2022) 20:111–21. doi: 10.1080/14779072.2022.2039627
30. Chang A, Botteri E, Gillis RD, Löfling L, Le CP, Ziegler AI, et al. Beta-blockade enhances anthracycline control of metastasis in triple-negative breast cancer. Sci Transl Med. (2023) 15:eadf1147. doi: 10.1126/scitranslmed.adf1147
31. Wang J, Lu S, Meng Y, Fu W, Zhou X. Beta adrenergic blockade and clinical outcomes in patients with colorectal cancer: A systematic review and meta-analysis. Eur J Pharmacol. (2022) 929:175135. doi: 10.1016/j.ejphar.2022.175135
32. Eng JW-L, Kokolus KM, Reed CB, Hylander BL, Ma WW, Repasky EA. A nervous tumor microenvironment: the impact of adrenergic stress on cancer cells, immunosuppression, and immunotherapeutic response. Cancer Immunol Immunother (2014) 63:1115–28. doi: 10.1007/s00262-014-1617-9
33. Mohammadpour H, MacDonald CR, Qiao G, Chen M, Dong B, Hylander BL, et al. [amp]]beta;2 adrenergic receptor-mediated signaling regulates the immunosuppressive potential of myeloid-derived suppressor cells. J Clin Invest. (2019) 129:5537–52. doi: 10.1172/JCI129502
34. Zhou L, Li Y, Li X, Chen G, Liang H, Wu Y, et al. Propranolol attenuates surgical stress-induced elevation of the regulatory T cell response in patients undergoing radical mastectomy. J Immunol. (2016) 196:3460–9. doi: 10.4049/jimmunol.1501677
35. Fjæstad KY, Rømer AMA, Goitea V, Johansen AZ, Thorseth ML, Carretta M, et al. Blockade of beta-adrenergic receptors reduces cancer growth and enhances the response to anti-CTLA4 therapy by modulating the tumor microenvironment. Oncogene. (2022) 41:1364–75. doi: 10.1038/s41388-021-02170-0
36. Globig AM, Zhao S, Roginsky J, Maltez VI, Guiza J, Avina-Ochoa N, et al. The β(1)-adrenergic receptor links sympathetic nerves to T cell exhaustion. Nature. (2023) 622:383–92. doi: 10.1038/s41586-023-06568-6
37. Zhu J, Naulaerts S, Boudhan L, Martin M, Gatto L, Van den Eynde BJ. Tumour immune rejection triggered by activation of α2-adrenergic receptors. Nature. (2023) 618:607–15. doi: 10.1038/s41586-023-06110-8
38. Boucher Y, Posada JM, Subudhi S, Kumar AS, Rosario SR, Gu L, et al. Addition of losartan to FOLFIRINOX and chemoradiation reduces immunosuppression-associated genes, Tregs, and FOXP3+ Cancer cells in locally advanced pancreatic cancer. Clin Cancer Res: an Off J Am Assoc Cancer Res. (2023) 29:1605–19. doi: 10.1158/1078-0432.CCR-22-1630
39. Sun J, Zhang C, Su X, Zhou H, Zhou S, Jiang M, et al. Several first-line anti-hypertensives act on fibrosarcoma progression and PD1ab blockade therapy. J Orthop Surg Res. (2024) 19:147. doi: 10.1186/s13018-024-04627-w
40. Liao YQ, Fang BB, Wu QX, Dong WY, Deng GM. Verapamil modulates NFAT2 to inhibit tumor growth and potentiates PD1ab immune checkpoint inhibitor therapy in cervical cancer treatment. J Recept Signal Transduct Res. (2023) 43:93–101. doi: 10.1080/10799893.2023.2291562
41. Finer N. Future directions in obesity pharmacotherapy. Eur J Intern Med. (2021) 93:13–20. doi: 10.1016/j.ejim.2021.04.024
42. Tang W, Sun G, Ji GW, Feng T, Zhang Q, Cao H, et al. Single-cell RNA-sequencing atlas reveals an FABP1-dependent immunosuppressive environment in hepatocellular carcinoma. J Immunother Cancer. (2023) 11:e007030. doi: 10.1136/jitc-2023-007030
43. Huang J, Tsang WY, Fang XN, Zhang Y, Luo J, Gong LQ, et al. FASN inhibition decreases MHC-I degradation and synergizes with PD-L1 checkpoint blockade in hepatocellular carcinoma. Cancer Res. (2024) 84:855–71. doi: 10.1158/0008-5472.CAN-23-0966
44. Xu L, Zhang Y, Lin Z, Deng X, Ren X, Huang M, et al. FASN-mediated fatty acid biosynthesis remodels immune environment in Clonorchis sinensis infection-related intrahepatic cholangiocarcinoma. J Hepatol. (2024) 81:265–77. doi: 10.1016/j.jhep.2024.03.016
45. Kanagavel M, Sparjan Samuvel RM, Ramalingam V, Nechipadappu SK. Repurposing of antifungal drug flucytosine/flucytosine cocrystals for anticancer activity against prostate cancer targeting apoptosis and inflammatory signaling pathways. Mol Pharm. (2024) 21:2577–89. doi: 10.1021/acs.molpharmaceut.4c00156
46. Moreno I, Hernández T, Calvo E, Fudio S, Kahatt C, Martínez S, et al. Pharmacokinetics and safety of lurbinectedin administrated with itraconazole in cancer patients: A drug-drug interaction study. Mar Drugs. (2024) 22:178. doi: 10.3390/md22040178
47. Fukumoto T, Harada T, Ito T, Fukushima S, Ono R, Furue M, et al. DNA repair ability in a patient with voriconazole-related squamous cell carcinoma that required differential diagnosis from xeroderma pigmentosum. J Dermatol Sci. (2024) 114:83–5. doi: 10.1016/j.jdermsci.2024.02.005
48. Takimoto Y, Tsubamoto H, Isono-Taniguchi R, Ueda T, Sakata K, Nakagawa K, et al. Itraconazole modulates phospholipid levels in tumor-associated macrophages. Anticancer Res. (2023) 43:1981–4. doi: 10.21873/anticanres.16358
49. Takimoto Y, Tsubamoto H, Taniguchi R, Sakata K, Takada Y, Adachi J, et al. Itraconazole repolarizes tumor-associated macrophages and suppresses cervical cancer cell growth. Anticancer Res. (2023) 43:569–80. doi: 10.21873/anticanres.16193
50. Iwai Y, Ishida M, Tanaka Y, Okazaki T, Honjo T, Minato N. Involvement of PD-L1 on tumor cells in the escape from host immune system and tumor immunotherapy by PD-L1 blockade. Proc Natl Acad Sci U S A. (2002) 99:12293–7. doi: 10.1073/pnas.192461099
51. Davar D, Wang H, Chauvin JM, Pagliano O, Fourcade JJ, Ka M, et al. Phase Ib/II study of pembrolizumab and pegylated-interferon Alfa-2b in advanced melanoma. J Clin Oncol: Off J Am Soc Clin Oncol. (2018) 36:Jco1800632. doi: 10.1200/JCO.18.00632
52. Zhu Y, Chen M, Xu D, Li TE, Zhang Z, Li JH, et al. The combination of PD-1 blockade with interferon-α has a synergistic effect on hepatocellular carcinoma. Cell Mol Immunol. (2022) 19:726–37. doi: 10.1038/s41423-022-00848-3
53. Sun HC, Tang ZY, Wang L, Qin LX, Ma ZC, Ye QH, et al. Postoperative interferon alpha treatment postponed recurrence and improved overall survival in patients after curative resection of HBV-related hepatocellular carcinoma: a randomized clinical trial. J Cancer Res Clin Oncol. (2006) 132:458–65. doi: 10.1007/s00432-006-0091-y
54. Hu B, Yu M, Ma X, Sun J, Liu C, Wang C, et al. IFNα Potentiates anti-PD-1 efficacy by remodeling glucose metabolism in the hepatocellular carcinoma microenvironment. Cancer Discovery. (2022) 12:1718–41. doi: 10.1158/2159-8290.CD-21-1022
55. Saunders KE, Cipriani A, Rendell J, Attenburrow MJ, Nelissen N, Bilderbeck AC, et al. Oxford Lithium Trial (OxLith) of the early affective, cognitive, neural and biochemical effects of lithium carbonate in bipolar disorder: study protocol for a randomised controlled trial. Trials. (2016) 17:116. doi: 10.1186/s13063-016-1230-7
56. Brand A, Singer K, Koehl GE, Kolitzus M, Schoenhammer G, Thiel A, et al. LDHA-associated lactic acid production blunts tumor immunosurveillance by T and NK cells. Cell Metab. (2016) 24:657–71. doi: 10.1016/j.cmet.2016.08.011
57. Zong Z, Xie F, Wang S, Wu X, Zhang Z, Yang B, et al. Alanyl-tRNA synthetase, AARS1, is a lactate sensor and lactyltransferase that lactylates p53 and contributes to tumorigenesis. Cell. (2024) 187:2375–2392.e33. doi: 10.1016/j.cell.2024.04.002
58. Xu Y, Ma K, Zhang L, Li G. Supercharging cancer-fighting T cells with lithium carbonate. Cell Metab. (2024) 36:463–5. doi: 10.1016/j.cmet.2024.02.006
59. Ma J, Tang L, Tan Y, Xiao J, Wei K, Zhang X, et al. Lithium carbonate revitalizes tumor-reactive CD8(+) T cells by shunting lactic acid into mitochondria. Nat Immunol. (2024) 25:552–61. doi: 10.1038/s41590-023-01738-0
60. Konenkov VI, Borodin YI, Makarova OP, Bgatova NP, Rachkovskaya LN. Effects of nanosized lithium carbonate particles on the functional activity of macrophages during development of hepatocarcinoma 29. Bull Exp Biol Med. (2015) 159:490–3. doi: 10.1007/s10517-015-3000-z
61. Benkő BM, Tóth G, Moldvai D, Kádár S, Szabó E, Szabó ZI, et al. Cyclodextrin encapsulation enabling the anticancer repositioning of disulfiram: Preparation, analytical and in vitro biological characterization of the inclusion complexes. Int J Pharm. (2024) 657:124187. doi: 10.1016/j.ijpharm.2024.124187
62. Sun T, Yang W, Toprani SM, Guo W, He L, DeLeo AB, et al. Induction of immunogenic cell death in radiation-resistant breast cancer stem cells by repurposing anti-alcoholism drug disulfiram. Cell Commun Signal. (2020) 18:36. doi: 10.1186/s12964-019-0507-3
63. Zhao Y, Zhao B, Zhu S. Disulfiram/copper activates ER stress to promote immunogenic cell death of oral squamous cell carcinoma. Cell Biochem Biophys. (2024) 82:1291–8. doi: 10.1007/s12013-024-01283-z
64. Li Q, Chao Y, Liu B, Xiao Z, Yang Z, Wu Y, et al. Disulfiram loaded calcium phosphate nanoparticles for enhanced cancer immunotherapy. Biomaterials. (2022) 291:121880. doi: 10.1016/j.biomaterials.2022.121880
65. Zhang S, Zong Y, Chen L, Li Q, Li Z, Meng R. The immunomodulatory function and antitumor effect of disulfiram: paving the way for novel cancer therapeutics. Discovery Oncol. (2023) 14:103. doi: 10.1007/s12672-023-00729-9
66. You SY, Rui W, Chen ST, Chen HC, Liu XW, Huang J, et al. Process of immunogenic cell death caused by disulfiram as the anti-colorectal cancer candidate. Biochem Biophys Res Commun. (2019) 513:891–7. doi: 10.1016/j.bbrc.2019.03.192
67. Wang Q, Zhu T, Miao N, Qu Y, Wang Z, Chao Y, et al. Disulfiram bolsters T-cell anti-tumor immunity through direct activation of LCK-mediated TCR signaling. EMBO J. (2022) 41:e110636. doi: 10.15252/embj.2022110636
68. Terashima Y, Toda E, Itakura M, Otsuji M, Yoshinaga S, Okumura K, et al. Targeting FROUNT with disulfiram suppresses macrophage accumulation and its tumor-promoting properties. Nat Commun. (2020) 11:609. doi: 10.1038/s41467-020-14338-5
69. Zhou B, Guo L, Zhang B, Liu S, Zhang K, Yan J, et al. Disulfiram combined with copper induces immunosuppression via PD-L1 stabilization in hepatocellular carcinoma. Am J Cancer Res. (2019) 9:2442–55.
70. Gao X, Huang H, Pan C, Mei Z, Yin S, Zhou L, et al. Disulfiram/copper induces immunogenic cell death and enhances CD47 blockade in hepatocellular carcinoma. Cancers. (2022) 14:4715. doi: 10.3390/cancers14194715
71. Guo J, Ma Y, Tang T, Bian Z, Li Q, Tang L, et al. Modulation of immune-responses by DSF/Cu enhances the anti-tumor effects of DTX for metastasis breast cancer. J Cancer. (2024) 15:1523–35. doi: 10.7150/jca.89120
72. Li P, Sun Q, Bai S, Wang H, Zhao L. Combination of the cuproptosis inducer disulfiram and anti−PD−L1 abolishes NSCLC resistance by ATP7B to regulate the HIF−1 signaling pathway. Int J Mol Med. (2024) 53:19. doi: 10.3892/ijmm.2024.5446
73. Huang S, Xie P, Huang X, Chen Z, Yang J, Wang J, et al. Disulfiram combined with chemoimmunotherapy potentiates pancreatic cancer treatment efficacy through the activation of cGAS-STING signaling pathway via suppressing PARP1 expression. Am J Cancer Res. (2023) 13:2055–65.
74. Zheng X, Liu Z, Mi M, Wen Q, Wu G, Zhang L. Disulfiram improves the anti-PD-1 therapy efficacy by regulating PD-L1 expression via epigenetically reactivation of IRF7 in triple negative breast cancer. Front Oncol. (2021) 11:734853. doi: 10.3389/fonc.2021.734853
75. Dale O, Somogyi AA, Li Y, Sullivan T, Shavit Y. Does intraoperative ketamine attenuate inflammatory reactivity following surgery? A systematic review and meta-analysis. Anesth Analg. (2012) 115:934–43. doi: 10.1213/ANE.0b013e3182662e30
76. Park JH, Mortaja M, Son HG, Zhao X, Sloat LM, Azin M, et al. Statin prevents cancer development in chronic inflammation by blocking interleukin 33 expression. Nat Commun. (2024) 15:4099. doi: 10.1038/s41467-024-48441-8
77. Lee SW, Kim HK, Lee NH, Yi HY, Kim HS, Hong SH, et al. The synergistic effect of combination temozolomide and chloroquine treatment is dependent on autophagy formation and p53 status in glioma cells. Cancer Lett. (2015) 360:195–204. doi: 10.1016/j.canlet.2015.02.012
78. Weyerhäuser P, Kantelhardt SR, Kim EL. Re-purposing chloroquine for glioblastoma: potential merits and confounding variables. Front Oncol. (2018) 8:335. doi: 10.3389/fonc.2018.00335
79. Yu M, Tong X, Qi B, Qu H, Dong S, Yu B, et al. Berberine enhances chemosensitivity to irinotecan in colon cancer via inhibition of NF−κB. Mol Med Rep. (2014) 9:249–54. doi: 10.3892/mmr.2013.1762
80. Yu YX, Wang S, Liu ZN, Zhang X, Hu ZX, Dong HJ, et al. Traditional Chinese medicine in the era of immune checkpoint inhibitor: theory, development, and future directions. Chin Med. (2023) 18:59. doi: 10.1186/s13020-023-00751-7
81. Zhang M, Wang L, Liu W, Wang T, De Sanctis F, Zhu L, et al. Targeting Inhibition of Accumulation and Function of Myeloid-Derived Suppressor Cells by Artemisinin via PI3K/AKT, mTOR, and MAPK Pathways Enhances Anti-PD-L1 Immunotherapy in Melanoma and Liver Tumors. J Immunol Res. (2022) 2022:2253436. doi: 10.1155/2022/2253436
82. Liu S, Han Z, Trivett AL, Lin H, Hannifin S, Yang D, et al. Cryptotanshinone has curative dual anti-proliferative and immunotherapeutic effects on mouse Lewis lung carcinoma. Cancer Immunol Immunotherap: CII. (2019) 68:1059–71. doi: 10.1007/s00262-019-02326-8
83. Chen Q, Hong Y, Weng S, Guo P, Li B, Zhang Y, et al. Traditional Chinese medicine Pien-Tze-Huang inhibits colorectal cancer growth and immune evasion by reducing β-catenin transcriptional activity and PD-L1 expression. Front Pharmacol. (2022) 13:828440. doi: 10.3389/fphar.2022.828440
84. Hwang J, Zhang W, Dhananjay Y, An EK, Kwak M, You S, et al. Astragalus membranaceus polysaccharides potentiate the growth-inhibitory activity of immune checkpoint inhibitors against pulmonary metastatic melanoma in mice. Int J Biol Macromol. (2021) 182:1292–300. doi: 10.1016/j.ijbiomac.2021.05.073
85. Sharma P, Hu-Lieskovan S, Wargo JA, Ribas A. Primary, adaptive, and acquired resistance to cancer immunotherapy. Cell. (2017) 168:707–23. doi: 10.1016/j.cell.2017.01.017
86. Tian S, Xu M, Geng X, Fang J, Xu H, Xue X, et al. Network medicine-based strategy identifies maprotiline as a repurposable drug by inhibiting PD-L1 expression via targeting SPOP in cancer. Adv Sci (Weinheim Baden-Wurttemberg Germany). (2025) 12:e2410285. doi: 10.1002/advs.202410285
87. Xia Y, Li X, Bie N, Pan W, Miao YR, Yang M, et al. A method for predicting drugs that can boost the efficacy of immune checkpoint blockade. Nat Immunol. (2024) 25:659–70. doi: 10.1038/s41590-024-01789-x
88. Nowak-Sliwinska P, Scapozza L, Ruiz i Altaba A. Drug repurposing in oncology: Compounds, pathways, phenotypes and computational approaches for colorectal cancer. Biochim Biophys Acta Rev Cancer. (2019) 1871:434–54. doi: 10.1016/j.bbcan.2019.04.005
89. Schneider L, Kehl T, Thedinga K, Grammes NL, Backes C, Mohr C, et al. ClinOmicsTrailbc: a visual analytics tool for breast cancer treatment stratification. Bioinf (Oxford England). (2019) 35:5171–81. doi: 10.1093/bioinformatics/btz302
90. Dubrot J, Lane-Reticker SK, Kessler EA, Ayer A, Mishra G, Wolfe CH, et al. In vivo screens using a selective CRISPR antigen removal lentiviral vector system reveal immune dependencies in renal cell carcinoma. Immunity. (2021) 54:571–85.e6. doi: 10.1016/j.immuni.2021.01.001
91. Miles LA, Garippa RJ, Poirier JT. Design, execution, and analysis of pooled in vitro CRISPR/Cas9 screens. FEBS J. (2016) 283:3170–80. doi: 10.1111/febs.2016.283.issue-17
92. Wan X, Cui L, Xiao Q. Metabolomics and network pharmacology-based identification of phenolic acids in Polygonatum kingianum var. grandifolium rhizomes as anti-cancer/Tumor active ingredients. PloS One. (2024) 19:e0315857. doi: 10.1371/journal.pone.0315857
Keywords: old medicine new use, drug functional repositioning, tumor immunotherapy, immune sensitization, cancer
Citation: Dong J, Su T, Wu J, Xiang Y, Song M, He C, Shao L, Yang Y and Chen S (2025) Drug functional remapping: a new promise for tumor immunotherapy. Front. Oncol. 15:1519355. doi: 10.3389/fonc.2025.1519355
Received: 29 October 2024; Accepted: 26 February 2025;
Published: 14 March 2025.
Edited by:
Vincenzo Flati, University of L’Aquila, ItalyReviewed by:
Yinyin Wang, China Pharmaceutical University, ChinaCopyright © 2025 Dong, Su, Wu, Xiang, Song, He, Shao, Yang and Chen. This is an open-access article distributed under the terms of the Creative Commons Attribution License (CC BY). The use, distribution or reproduction in other forums is permitted, provided the original author(s) and the copyright owner(s) are credited and that the original publication in this journal is cited, in accordance with accepted academic practice. No use, distribution or reproduction is permitted which does not comply with these terms.
*Correspondence: Yubin Yang, eWFuZ3l1YmluXzIwMjNAc2N1LmVkdS5jbg==; Size Chen, Y2hlbnNpemVAZ2RwdS5lZHUuY24=; Lijuan Shao, c2hhb3NoYW8xMjA3MUAxNjMuY29t
Disclaimer: All claims expressed in this article are solely those of the authors and do not necessarily represent those of their affiliated organizations, or those of the publisher, the editors and the reviewers. Any product that may be evaluated in this article or claim that may be made by its manufacturer is not guaranteed or endorsed by the publisher.
Research integrity at Frontiers
Learn more about the work of our research integrity team to safeguard the quality of each article we publish.