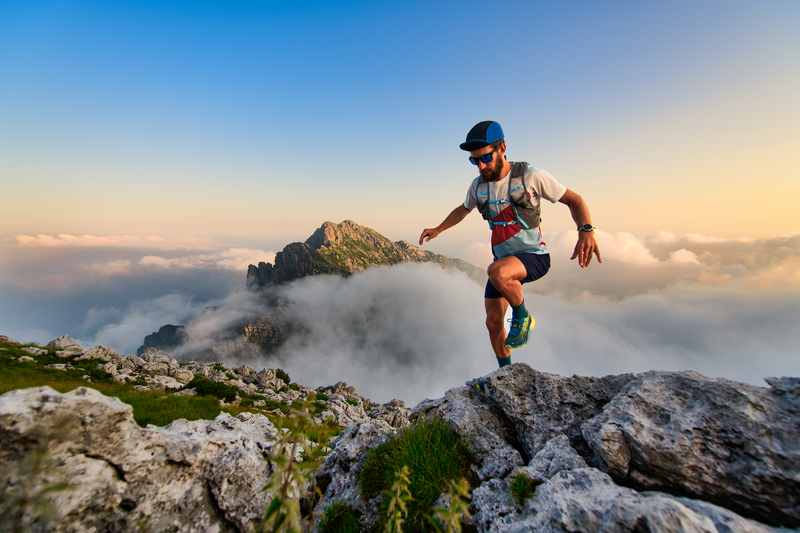
95% of researchers rate our articles as excellent or good
Learn more about the work of our research integrity team to safeguard the quality of each article we publish.
Find out more
REVIEW article
Front. Oncol. , 05 February 2025
Sec. Genitourinary Oncology
Volume 15 - 2025 | https://doi.org/10.3389/fonc.2025.1454392
This article is part of the Research Topic Innovative Strategies and Advancements in Phototherapy for Enhanced Cancer Treatment View all articles
Over the past 20 years, early diagnosis of prostate cancer has become increasingly prevalent due to the promotion of prostate-specific antigens, and its treatment has become a focal point. However, there are some drawbacks associated with therapies for early prostate cancer, such as active surveillance and radical prostatectomy, which may include urinary incontinence, erectile dysfunction, and urinary tract infection. In contrast, photodynamic therapy (PDT) is introduced into the treatment of prostate cancer because of its advantages, such as high precision to tumor cells, low toxicity, and no radiation. Compared to radical prostatectomy, the PDT has low risk and minimal trauma. Although PDT is in the early stages of clinical development, it holds promise for the effective treatment of localized prostate cancer. Herein, we reviewed studies on the mechanisms of PDT and photosensitizers for prostate cancer. Given the rapid development of nanotechnology, photosensitizers wrapped by nanomaterials have emerged as new option with significant advantages, particularly of in achieving high tumor selectivity using functional nanomaterials. Numerous PDT clinical trials on prostate cancer have been conducted worldwide. We also reviewed the results of a few photosensitizers in these clinical trials. However, a few limitations and challenges regarding PDT for prostate cancer still exist. In addition, future development and potential clinical application strategies of future PDT are predicted.
Prostate cancer is the second common malignant tumor in males (1). Over the past 20 years, there has been significant development in therapies for localized prostate cancer. In the past, traditional approaches for the treatment of localized prostate cancer mainly included active surveillance, radical prostatectomy (RP), and radiation therapy. However, RP can lead to some side effects, such as erectile dysfunction, urinary incontinence, and urinary tract infections (2). Active surveillance is another option. It may reduce overtreatment in some patients with early-stage prostate cancer, but the potential disadvantage of this approach is the psychological burden arising from delaying RP (3). Consequently, focal treatment for localized prostate cancer has gained researchers’ attention. This approach causes minimal damage to the surrounding tissues and preserves quality of life (4), because it destroys only the tumor or the area containing it (2). The current treatments for localized prostate cancer mainly include high-intensity focused ultrasound (HIFU) (4), cryotherapy (5), irreversible electroporation (6), and photodynamic therapy (PDT).
PDT originated in the 1960s when Lipson et al. reported a porphyrin mixture (7). Schwartz then prepared and named it a hematoporphyrin derivative (HPD). In the 1970s, early preclinical and clinical studies expanded rapidly to explore the therapeutic potential of HPD (8–10). Since the approval of certain light applicators and photosensitizers (PSs), PDT has gained increasing attention. It mainly involves three components: light, tissue oxygen, and PSs (11). Compared with other focal therapies, PDT has lower toxicity and limited side effects, and does not utilize ionizing radiation (12). Furthermore, PDT equipment is cheaper and requires less room. PDT is a clinically approved and minimally invasive treatment for early-stage cancer (13). The photodynamic therapy procedures for prostate cancer is shown in Figure 1 (14).
Figure 1. The use of PDT for prostate cancer. (A) The photosensitizers are administered either orally or intravenously. After a suitable drug–light interval, the drug is activated by a low-power laser light. (B) The light is most commonly delivered using a brachytherapy-style perineal template with transrectal ultrasound guidance. Hollow plastic needles are inserted into the prostate, and cylindrically diffusing fibers are positioned within the hollow needles. This part of the procedure is carried out under general anesthetic, with the patient in the lithotomy position. (C) The cylindrical diffuser fibers deliver light to the prostate at the appropriate drug–light interval (14).
This article is an overall review of studies on PSs and PDT in localized prostate cancer. Moreover, it addresses current limitations and future perspectives.
The mechanisms of PDT are depicted in Figure 2 (15). Light, oxygen, and photosensitizers are the three primary elements of PDT for tumor elimination (16). Photodynamic responses involve both photochemical and photophysical reactions. When PSs are exposed to light of a particular wavelength, they reach a higher-energy state called the singlet state. In this state, activated PSs release energy by emitting light, heat, or transforming into a triplet state (intermediate energy state) before returning to the ground state (11). Triplet-state PS can produce reactive oxygen species (ROS) via two mechanisms. In one of the mechanisms, PSs interact directly with substrates, transferring protons or electrons to produce organic radicals. Further reactions between cellular oxygen and these free radicals result in the generation of ROS, such as superoxide anions, peroxides, and hydroxyl radicals. The other mechanism involves the formation of singlet oxygen. When singlet oxygen interacts with biomolecules, its primary products undergo chain reactions related to free-radical peroxidation (17, 18). Ultimately, these processes lead to vascular damage, affecting the immune system and causing cell death (19).
Figure 2. The mechanism of PDT. Light, oxygen, and photosensitizers are three primary elements of PDT required to eliminate tumors. The activated photosensitizers release energy by emitting light and heat or transforming into a triplet state. Then the triplet state PS produces reactive oxygen species (ROS) by two types of mechanisms. Ultimately, these processes lead to vascular damage, affecting the immune system and causing cell death (15).
There are three mechanisms that can explain the cytotoxicity of ROS in tumors. Firstly, ROS can lead to vascular disorders and thrombosis associated with tumors by destroying the vascular endothelial cells of the tumors. This means a reduction in the supply of oxygen and nutrients to the tumor. Secondly, ROS can cause an oxidative stress reaction, leading to the activation of the protein kinase pathway or the expression of cytokines and transcription factors. These reactions can lead to cell necrosis and apoptosis. Thirdly, ROS can induce necrosis and apoptosis of tumor cells, stimulating the activation of T cells and releasing numerous pro-inflammatory factors. Moreover, they induce acute inflammatory and anticancer immune responses (20, 21).
Light, oxygen, and PSs are the three primary elements of PDT for tumor elimination. Among them, the selection and application of PSs are the key factors affecting the efficacy of PDT. Since the 1950s, different kinds of photosensitizers have been discovered and tried for the treatment of cancer (15). They are divided into three generations according to the time of discovery. The characteristics of different PSs are listed in Table 1.
In the 1950s, hematoporphyrin was found to accumulate in tumor tissues and induce cell death after exposure to light (33). The observed phenomenon indicated that the photodynamic effect of hematoporphyrin resulted in cell death, involving the production of toxic byproducts and ROS (25). In the 1970s, an HPD was used as a PS in PTD to treat bladder cancer (34). By 1978, the successful application of first-generation PSs in treating different tumors was reported by Dougherty et al (10). In 1985, the photoinduced toxicity of an HPD used on rat prostate cancer cells was studied (25). The occurrence of prostate tissue necrosis during PDT depends on the PS content in the prostate. Although the distribution of porphyrin in the prostate and other urological organs has been investigated in mice, there are significant discrepancies between the mouse model and humans. Physiological and morphological similarities exist only between the prostates of humans and other primates. Therefore, primates are preferred for assessing porphyrin distribution in the prostate and other organs. In addition, the primate prostate contains a high concentration of zinc (35), making it a suitable choice to study zinc-containing metalloporphyrins in prostate cancer treatment (36). Although zinc accumulation is high in prostatic tissues, preferential localization to the two lobes of the primate prostate has not been achieved. Interpreting this result is challenging because of limited data on the effects of zinc on prostatic physiology. There are three possible explanations for this finding: (1) Zinc may be preferentially taken up by prostatic epithelial cells, especially when the zinc atoms are placed inside the porphyrin molecule rather than at the periphery. (2) The zinc characteristics of the metalloporphyrin complexes may have disappeared. (3) Zinc accumulation is under the androgenic control of the prostate (37). Even if zinc-HPD is preferentially taken up by prostatic tissues, it is clear from the spectrum that the most useful absorption peak at 630 nm is lost. There exist a few other first-generation photosensitizers, such as verteporfin (24) and talaporfin (22).
However, numerous shortcomings limit the application of HPD. For example, the low absorption rate of light, owing to the short excitation wavelength, makes it difficult for light to penetrate deeper tissues and effectively execute PDT. In addition, it is difficult to synthesize and purify HPDs. Lastly, HPDs can easily induce phototoxicity due to their long half-lives.
Second-generation PSs include phthalocyanines, phenothiazines, polycyclic quinones (32), and porphyrin derivatives. Compared with first-generation PSs, second-generation PSs have many improvements. First, they can be activated by near-infrared (NIR) light due to their longer absorption wavelengths. The ROS rate is related to the effect depth of these PSs. In addition, second-generation PSs can be rapidly metabolized, thereby reducing their side effects (38, 39).
Porphyrin-derived PSs primarily contain endogenous and exogenous porphyrins. Among endogenous porphyrins, 5-aminolevulinic acid (5-ALA) is widely used as a precursor for heme synthesis (26). It is an endogenous biochemical substance that is subjected to ALA anhydrase and a series of enzymatic actions to produce protoporphyrin IX with strong photosensitizing effect (40). In 1990, Kennedy et al. first used this technique for tumor ablation (41). Later, Nakayama et al. used ALA-PDT to treat prostate cancer cells and found that it led to rapid ROS-induced cell death and the suppression of cell proliferation through several pathways (42). However, 5-ALA is easy to decompose if it is in direct sunlight and its penetration is low. 5-ALA is not the optimal for refractory and thick tumors. Methotrexate was combined with ALA because it could cause an increase in protoporphyrin IX, which overcame disadvantages of 5-ALA (30). Exogenous porphyrins, such as chlorin e6 (Ce6) and photochlor (HPPH), are comprehensively used in PDT (43). HPPH, with its long excitation wavelength, is used for solid tumors. Furthermore, its low phototoxicity eliminates the need to avoid light exposure after injection. Ce6 (44), synthesized from pyropheophorbide-a, is suitable for PDT because it can efficiently produce singlet oxygen. However, Ce6 has a hydrophobic nature, making its application difficult. Using HPPH for treatment presents a significant limitation: the non-specific uptake and extensive diffusion of HPPH can weaken its effectiveness and result in off-target effects. Researchers try to revise the physical structure of HPPH, which can load it into new drug delivery systems. This will be mentioned in discussing the application of third-generation photosensitizers.
Polycyclic quinone, a natural PS, is widely found in plants. It can generate free radicals, singlet oxygen, and superoxide anions when used in PDT, which suggests that it can trigger two types of photosensitive reactions (45). Representative polycyclic compounds include hypericin, hypocrellin, and curcumin. Hypericin extracted from Hypericum perforatum L. has excellent photosensitivity to light (28). It can rapidly induce the release of Ca2+ from the endoplasmic reticulum, a process possibly correlated with cell apoptosis. In addition, the light-independent effects of hypericin, including the ultrastructure of organelles, protein synthesis, and ROS generation, have been reported by Huntosova and Stroffekova (46). Hypocrellin occurs as two types: HA and HB; both exhibit high ROS generation ability and rapid clearance. Therefore, hypocrellin is a promising anticancer PS for PDT. Curcumin is well known for its photosensitivity and anticancer properties. These properties exert synergistic effects when used in PDT.
Phenothiazine is a widely used PS. Methylene blue (MB), new methylene blue, dimethyl methylene blue (DMMB), and toluidine blue O are commonly used in this research. A previous study revealed that DMMB may act through the cellular stress axis to induce cell death. MB triggers cellular responses such as necrosis, apoptosis, and autophagy, relying on the nonspecific generation of many oxidant species (47).
Phthalocyanine is a porphyrin analog that can modify cores and substituents, incorporating zinc, silicon, or aluminum to optimize its properties (48). Consequently, they can be activated by light in the wavelength range of 750–900 nm. In addition, phthalocyanine PSs have ideal characteristics, such as low phototoxicity, quick clearance, and high fluorescence signal generation (29). Therefore, they can be used in photodiagnosis and photodynamic treatments.
However, second-generation PSs still have some disadvantages, such as poor tumor-targeting capacity and low permeability for local delivery.
The third-generation PSs are based on second-generation PSs and combined with substances with biological properties to improve the targeting of photodynamic therapy and they can attain high tumor selectivity by using functional nanomaterials (49) or conjugating target molecules. DR2 is a bimolecular conjugate composed of an anti-androgen molecule that can release NO under the influence of light and a photosensitizer (pheophorbide). DR2 can modulate the NF-KB/YY1/RKIP loop, which, in turn, controls the growth and apoptosis of prostate cancer cells (50). The basic method of research on PSs for prostate cancer is depicted in Figure 3 (31). Prostate-specific membrane antigen (PSMA) is a glycoprotein commonly found on the surface of prostate cancer cells and has attracted considerable attention as a target for delivery. Pyropheophorbide-a is a PS that lacks specificity for cancer cells (52). However, inhibitors known as phosphoramidate peptidomimetic PSMA are used for both intracellular delivery and cell-surface labeling of prostate cancer cells (53). Thus, a conjugation of the PSMA inhibitor to pyropheophorbide-a has been analyzed (54, 55). Deks et al. effectively improved the accuracy and efficacy of surgical treatment of prostate cancer by combining fluorescence-guided surgery for PSMA with tumor-targeted PDT (56).
Figure 3. Basic research on photosensitizers in prostate cancer therapy. (A) PDT was administered to the mice. (B) After rejection, the initial electrophoresis variations and those after 30 min. (C) The different absorbances of AuNPs SPR and encapsulated Pc4 at different wavelengths. (D) Imaging and fluorescent labeling after rejection (51).
Various functional nanomaterials have emerged owing to the rapid development of nanotechnology (57). These materials are carriers for delivering PSs with low water solubility (58). In addition, certain nanomaterials can be used directly as PSs (59). Examples include gold nanoparticles (60), silica nanoparticles (61), magnetic nanoparticles (62), polymeric nanoparticles (63), liposomes (64), and quantum dots (65).
In recent years, gold nanoparticles have been shown to have unique chemical and physical properties, including fluorescence enhancement and surface plasmon resonance, making them suitable for drug delivery and targeting (66). PSMA-targeted nanoparticles complex is a new drug delivery system. By combining nanoparticles with PSMA-targeted ligands, it is possible to improve the targeting and bioavailability of drugs in prostate tissue. PSMA-targeted gold nanoparticles have been synthesized and can effectively deliver drugs to targeted cells. The animals treated with these nanoparticles showed tumor remission after 14 days, indicating their potential for therapeutic intervention (31). Luo et al. bound PSMA-targeting ligands and gadolinium (Gd III) complexes to the gold nanoparticles surface. They are used in magnetic resonance (MR)-guided prostate cancer-targeted therapy. The results showed that the binding of gold to Gd (III) inhibited prostate cancer more effectively after radiotherapy (67). Titanium dioxide (TiO2) has been found to be photoactive upon irradiation. It also has high stability, appropriate biocompatibility, and low toxicity and is used in anticancer PDT (68). However, only UV light can activate pure TiO2, and its tissue penetration is low, limiting its application in PDT (69). Therefore, researchers have attempted to use targeted TiO2 nanoparticles in PDT. The results showed that TiO2 decreased the viability of cancer cells (61). Silica has a tetrahedral structure. According to pore diameter, the silica nanoparticles are classified as macropores (>50 nm), mesopores (2–50 nm), and micropores (<2 nm) (70). Mesoporous silica nanoparticles (MSNs) are considered suitable PS carriers because of their excellent biocompatibility and large surface area. As biocompatible materials, MSNs can be decomposed into water-soluble and non-toxic orthosilicic acid (Si (OH)4) in a physiological environment and then extracted in urine (70, 71). Magnetic nanoparticles (MNPs) have unique biocompatibility, stability, and physical properties (72). It is known that a permanent magnet can generate an external magnetic field with controlled interactions with MNPs. These MNPs can be used to study the specific location of a tumor within an organism and release drugs, thus creating a targeted drug delivery system. PSMA-targeted MNPs were developed by Ngen et al. After intravenous administration of 50 mg/kg of MNPs in a mouse model, evaluation using T2-weighted MRI determined the concentration required for therapy. Compared to PSMA (-) tumors, the nanoparticles initially aggregated at the tumor periphery, and contrast enhancement was observed in PSMA (+) tumors after administration (73). Although the U.S. Food and Drug Administration (FDA) has approved some MNPs, many of them cause side effects, such as damage to the brain, liver, skin, and nervous system. Therefore, their use should be controlled using organic or inorganic compounds (74). In summary, the researches of targeting PS in prostate cancer are listed in Table 2.
In addition, new nanoparticles have been used in PDT for prostate cancer treatment (75). Polymeric nanoparticles are prepared from synthetic or natural polymers with diameters less than 1 µm. These polymeric nanoparticles are further classified as nanocapsules (NCs) or nanospheres (NSs) (76, 77). NCs consist of a solid/liquid nucleus with a shell, with the drug dissolved in the core, although some can be on the surface of the NCS. In contrast, NSs consist of a solid matrix without a polymer shell. The drug can then be absorbed into the matrix (78). Chen et al. developed polymeric NCs that encapsulated a combination of quercetin and doxorubicin for active targeting of prostate cancer. Polymeric NCs with well-defined covalently stabilized structures have been generated as potentially safe and universal therapeutic nanocarriers (79). Since liposomes were first described by Bangham, active research has been conducted in this field (80). Liposome self-assembly and multifunctional carrier material containing lipid bilayers with cholesterol or phospholipids and hydrophobic drugs can bind with the lipid bilayers, as hydrophilic drugs are encapsulated in the internal water chambers (81). Lipid carriers are easier to prepare than other nanocarriers and are non-toxic (82, 83). The co-delivery of resveratrol (Res) and docetaxel (Doc) via liposomes was introduced by Zhang et al. Animal experiments showed that the drugs could be delivered to prostate PC-3 cells. Mice treated with Res/Doc-containing liposomes exhibited prolonged survival compared to the controls exposed to Res/Doc without liposomes (84). Quantum dots with diameters of 2−10 nm are composed of semiconductor elements with unique electronic and photoluminescent properties (85). The core of semiconductor materials, such as lead selenide or cadmium selenide, determines their optical properties (86–88). In castration-resistant prostate cancer (CRPC), a nanosystem with graphene oxide for intravenous therapy was developed by Jiang et al. The graphene quantum dots were first cross-linked by disulfide bonds and then formed by approximately 200 nm derivatives that could load enzalutamide. In in vitro and in vivo studies, this carrier showed an ability to target prostate cancer, which could be internalized by CRPC cells, inhibit the proliferation of cancer cells, and reduce the side effects of drugs in vivo (23). Taken together, nanomaterials are gaining attention in PDT for prostate cancer due to their numerous advantages (75, 89, 90). Besides, there are also some other manners of using nanomaterials to the cancer therapy. Researchers reported that they designed a new carrier loaded glucose oxidase and disulfiram prodrug, and the carrier used copper (II)-based metal–organic framework, which could be combined with Cu2+ (27) was used to enhance tumor-specific therapy (91).
As we all know, generating cytotoxic singlet oxygen for prostate cancer treatment depends on the ability that PS transfers energy from lasers to dissolved oxygen (92, 93). However, in tumors the oxygen supply is not adequate, which will impair the effectiveness of PDT. Hypoxia is common because of the reduced oxygen supply by deteriorated diffusion and disturbed microcirculation in prostate cancer, which are also the unique characteristics and antitumor mechanisms of prostate cancer. In addition, PDT worsens hypoxia through vascular shutdown effects and oxygen consumption. Low oxygen can reduce photodynamic efficacy about PS, preventing PDT from achieving full potential of cancer therapeutic. To ensure PDT efficacy, some traditional experiments have tried to optimize tumor oxygenation. For example, extending irradiation with low fluence rate and dividing irradiation into dark-light circles have both been investigated for better tumor reoxygenation. To actively increase the oxygen of tumor, hyperbaric oxygen inhalation has been used. However, there exist potential toxic effects about excessive oxygen, an impediment to its clinical use. Therefore, it is important for photodynamic therapy to optimize the efficacy with limited oxygen. To overcome this challenge, researchers load PS into perfluorocarbon nanodroplets, developing novel oxygen self-enriched photodynamic therapy. At the given oxygen partial pressure, perfluorocarbon can maintain a high oxygen content because of its high oxygen capacity. In their study, they demonstrated a platform called Oxy-PDT to achieve enhance efficacy (94). The PDT efficacy is determined by 1O2 generation rate because it is 1O2 for PDT to kill the cancer cells. In the Oxy-PDT agent, both oxygen and PS are enriched in the nanodroplet. Oxy-PDT can realize higher efficacy in hypoxic conditions and is considered the first PDT design. We anticipate its wide clinical application in the future.
We can discover that some of the third generation PSs are enhanced by some materials in therapy of prostate cancer compared to the first generation PSs. For example, Mesquita et al. demonstrated significant therapeutic potential by synthesizing fluorinated porphyrin derivatives and optimizing their application using nanotechnology (95).
A great number of PDT clinical trials to treat prostate cancer have been performed worldwide. The first clinical study on PDT to treat prostate cancer was reported in The Lancet in 1990 (96).
As mentioned earlier, 5-ALA produces PpIX, which has strong photosensitivity and is activated at 420−460 nm. Clinical research in Germany has reported the efficacy of PpIX in treating prostate cancer. Adam et al. reported the use of ALA to identify positive surgical margins in endoscopic extraperitoneal and open retropubic RP, which could improve RP outcomes. Thirty-nine patients with prostate cancer received oral ALA and underwent RP (15 underwent open retroperitoneal RP and 24 underwent endoscopic extraperitoneal RP). The results showed that the sensitivity of the open RP group was significantly lower than that of the endoscopic RP group. As ALA-PDD/PTT (photodynamic diagnosis and photothermal therapy) can reduce the interval between drug administration and irradiation to 4 hours, the authors considered ALA-PDD during RP to be an effective method (97).
Many physicians have explored the treatment of prostate cancer using temoporfin activated at 652 nm (98). In 2002, Nathan et al. reported their phase I study involving 14 patients with prostate cancer experiencing local recurrence after radiotherapy. These patients were administered temoporfin-PDT, and the interval from intravenous injection to irradiation was 72 hours. After PDT, the prostate specific antigen (PSA) levels of nine patients decreased, and no tumors were found in the biopsies of five patients. Unfortunately, all patients underwent androgen deprivation therapy (ADT) as their PSA levels eventually increased. The researchers attributed this to partial gland coverage during irradiation (99). In 2006 (98), doctors in London reported another study involving temoporfin-PDT, wherein six patients with prostate cancer underwent focal PDT, resulting in a 48.3% decrease in PSA levels. Biopsy results revealed that all patients who underwent PDT had residual cancer. Among them, two patients underwent cryotherapy and brachytherapy, while three patients underwent external beam radiotherapy. Only one patient remained cancer-free 6 years after PDT. It is important to note that these trials were limited to areas detected by biopsy (100). The efficacy of temoporfin can be enhanced with improvements in light dosimetry and the accuracy of needle placement.
Motexafin lutetium is a potent PS, activated at 730−770 nm (101). It has been approved by the FDA for the treatment of malignant melanomas and breast cancer (102). In 2006, a phase I study of 16 patients with recurrent prostate cancer was reported by Du et al. (103). This study was the first to assess the PSA value, ranging from day one to several weeks after PDT. The results indicated that PSA levels significantly increased shortly after PDT, suggesting that cellular damage induced by PDT may result in a transient increase in PSA levels. Consistent with the results of other trials, the PSA value dropped below baseline within 2 months after PDT and then increased again (101).
At 763 nm NIR light can active vascular-targeted PSs called Tookad®, destroying tumor blood vessels and effectively killing cancer cells. This approach is called vascular-targeted photodynamic therapy (VTP). VTP is administered using a photosensitizer that is delivered intravenously and locally activated by laser fibers that emit a specific wavelength of light. Once activated, photosensitizers produce free radicals that cause severe forms of uniform local vascular damage within tissues, leading to controlled, non-thermal forms of coagulated tissue necrosis. Padeliporfin (WST11, Tookad®) is hydrophilic, whereas Padoporfin (WST09, Tookad®) is hydrophobic. WST11 is a new negatively charged, water-soluble palladium-bacterial chlorophyll derivative that has been used in vascular-targeted photodynamic therapy (VTP). In vitro results suggest that WST11 cell uptake, clearance, and phototoxicity are mediated by serum albumin trafficking. The first phase I/II trial of VTP in prostate cancer patients after external beam radiotherapy was conducted by Trachtenberg et al (104). Only two fibers were placed in the prostate to demonstrate safety in the first trial (105). Subsequently, a phase II trial was conducted. In this trial, 28 patients received WST09 (2 mg/kg). The results indicated a notable therapeutic effect with an appropriate light intensity and a safe drug concentration (106). In France, a phase II trial reported that 56 patients with prostate cancer (Gleason score ≤3 + 3) underwent VTP. After treatment for 6 months, the mean PSA value was 3.7 ng/mL, and there was no residual tumor in the targeted area (107). To determine the WST11 concentration, 40 patients with prostate cancer (Gleason score ≤3 + 3) received 2, 4, or 6 mg/kg of WST11. The results showed that 4 mg/kg of WST11 was the optimal dose (108). A Latin American trial (PCM304) evaluated the efficacy of PDT in men with prostate cancer. Twelve months after VTP, 60 (74%) patients had negative biopsy results (109). In 2019, a phase II trial assessed tumor control in 68 patients after VTP. The results were similar to those of earlier phase II studies; a quarter of the patients had positive biopsy results after 3 years. Without removing or destroying the prostate, it only causes short-term urinary incontinence and erectile problems and can be recovered within three months (110). Although VTP has many advantages, such as easy metabolism, lack of obvious side effects, and no drug-light interval, it must be used carefully in low-risk patients (111). The initial experience of patients who underwent VTP for unilateral low-risk prostate cancer was reported in a real-world study conducted in Germany. The researchers compared the short-term oncological outcomes in patients undergoing continuous RP. The biopsy results indicated that at 12 and 24 months after PDT, 27% of patients had low-and intermediate-risk prostate cancer, respectively. This study suggests that the complication rate of VTP is lower than that of RP. However, after VTP, recurrence and progression are common; therefore, a rigorous surveillance strategy is required (112). In addition, salvage RP after VTP seems feasible because it does not involve thermal ablation. Above all, the clinical researches on PDT for prostate cancer are listed in Table 3.
There are several limitations to use PDT for prostate cancer. These limitations differ between focal and whole-gland therapies.
The challenges with using focal therapy include the initial and future aspects. The former involves accurately predicting the behavior of prostate cancer, identifying cancer within the prostate, and treating the identified target volume. The latter involves ascertaining whether the intended treatment is administered at the planned treatment volume and ensuring the untreated part of the prostate is handled appropriately. In the PDT of prostate cancer using a PS linked to a monoclonal antibody, there is heterogeneity in antigen expression within a tumor or among different regions in the same prostate cancer. Multiple targeting devices can resolve this problem; however, their efficacy should be analyzed in preclinical studies.
There are some limitations to PDT of the whole gland. An adequate dose of the drug, oxygen, and light should be delivered to the entire gland to reliably achieve the whole-gland effect of PDT. When the effect does not extend beyond the gland, excess light or drugs do not matter. Future studies should explore the correlation between drug, oxygen, and light measurements, and PDT outcomes. The lack of an appropriate follow-up protocol for PDT of the entire gland remains a challenge. Although MRI is useful for identifying avascular lesions, the correlation between these lesions and long-term clinical outcomes and biopsy materials is not available (113). Whole-gland PDT has demonstrated significant morbidity after radiotherapy compared to that with other salvage treatments. For example, in a study assessing temoporfin, a rectourethral fistula was observed after a PDT rectal biopsy (99). Another study assessing padoporfin levels after radiotherapy reported two rectourethral fistulas (106). Whole-gland treatment has mainly been studied in patients who received radiotherapy before whole-gland PDT. Some patients may experience urinary dysfunction after whole-gland PDT. On the contrary, PDT for focal prostate cancer can identify accurately the tumor within the gland.
Several studies in canine and human prostates have demonstrated the ability of different PSs to induce necrosis in prostate cancer cells or prostate tissue. However, some PSs, such as porfimer sodium (Photofrin), induce toxicities, such as skin photosensitization (96). After using such PSs, it is necessary to avoid bright light for several days, thereby reducing the quality of life and introducing additional problems. For example, patients should stay in a room with reduced lighting for 3 days after using meso-tetra-hydroxyphenyl-chlorin to prevent skin photosensitivity (99).
The future holds the promise of detecting more prostate cancers at an early stage, thanks to the development of early detection techniques such as liquid biopsy. This implies that minimally invasive treatments, such as PDT, will benefit an increasing number of patients with prostate cancer. Additionally, there are treatment options available for locally recurrent prostate cancer and CRPC. This vast potential opens avenues for patients with prostate cancer, paving the way for more effective therapies. Studying the optical characteristics of different types of prostate cancer can help design a clinical reference strategy. The development of molecular targeting techniques has made PDT highly selective for tumors, with lower toxicity to surrounding tissues. While PDT is in its early stages compared to other ablative modalities, such as cryotherapy and HIFU, it has the suitable characteristics required for focal treatment in prostate cancer. In the future, PDT warrants further exploration in clinical trials. As mentioned above, the use of advanced nanoparticles in the third generation can overcome the known limitation of low penetration in PDT. Future objectives should focus on addressing biodegradability as the primary concern, enhancing the utilization of PDT for prostate cancer. Additionally, ongoing developments, especially in photosensitizer delivery and real-time feedback systems, make PDT an important addition to the range of treatment options under investigation for organ-confined prostate cancer (114). Development of new therapeutic diagnostics based on the combination of PDT and different imaging techniques to achieve treatment and diagnosis. This combination can help address poor biodistribution and selectivity through regional imaging, while therapeutics enable effective personalized treatment. Biomarkers can identify patients who are sensitive to PDT, allowing for personalized treatment. Besides, biomarkers such as photosensitizer-specific expression levels, tumor hypoxia, and immune status can predict PDT response.
Although PDT has these limitations, we can use it with other therapies. For example, PDT can combine the photothermal therapy (PTT) to enhance selectivity of cancer therapy (115).
The traditional treatments mainly include RP and active surveillance. However, RP can lead to some side effects, such as erectile dysfunction, urinary incontinence, and urinary tract infections. And the potential disadvantage of active surveillance is the psychological burden arising from delaying RP. Herein, we reviewed PDT for localized prostate cancer and noted that it is a minimally invasive treatment for localized prostate cancer and can preserve the functions of the surrounding organs, such as rectum, bladder, and neurovascular bundle. We also reviewed different PSs, spanning from the first to the third generations. The third generation is important because it is extensively used in PDT for prostate cancer, where an increasing number of new nanoparticles is being combined with PSs. This integration is expected to enhance the accuracy and safety of PDT for localized prostate cancer. Moreover, the promotion of prostate-specific antigens can facilitate early diagnosis of prostate cancer. It is noteworthy that we gathered not only studies on PSs but also those on PDT. An important point is that PDT can be repeated without cumulative toxicity. Therefore, if recurrent or residual diseases are identified, PDT can be used to ablate the remaining prostate tissue after focal therapy. Although there are limitations to PDT for localized prostate cancer, we anticipate that in the future, PDT will overcome these limitations and advance to the next level. We believe that PDT will undergo comprehensive development leading to precise application for the treatment of localized prostate cancer.
YX: Writing – original draft. QT: Writing – original draft. CS: Writing – review & editing. YJ: Writing – review & editing. SL: Conceptualization, Supervision, Writing – review & editing. XY: Supervision, Writing – review & editing.
The author(s) declare financial support was received for the research, authorship, and/or publication of this article. This study received financial support from Youth Research Fund of Affiliated Hospital of Qingdao University (QDFYQN2023117).
The authors gratefully acknowledge the figures sourced from other articles and the research of other doctors.
The authors declare that the research was conducted in the absence of any commercial or financial relationships that could be construed as a potential conflict of interest.
All claims expressed in this article are solely those of the authors and do not necessarily represent those of their affiliated organizations, or those of the publisher, the editors and the reviewers. Any product that may be evaluated in this article, or claim that may be made by its manufacturer, is not guaranteed or endorsed by the publisher.
1. Cronin KA, Scott S, Firth AU, Sung H, Henley SJ, Sherman RL, et al. Annual report to the nation on the status of cancer, part 1: National cancer statistics. Cancer. (2022) 128:4251–84. doi: 10.1002/cncr.344792
2. Kasivisvanathan V, Emberton M, Ahmed HU. Focal therapy for prostate cancer: rationale and treatment opportunities. Clin Oncol (R Coll Radiol). (2013) 25:461–73. doi: 10.1016/j.clon.2013.05.002
3. Alvisi MF, Dordoni P, Rancati T, Avuzzi B, Nicolai N, Badenchini F, et al. Supporting patients with untreated prostate cancer on active surveillance: what causes an increase in anxiety during the first 10 months? Front Psychol. (2020) 11:576459. doi: 10.3389/fpsyg.2020.576459
4. Barqawi AB, Crawford ED. The current use and future trends of focal surgical therapy in the management of localized prostate cancer. Cancer J. (2007) 13:313–7. doi: 10.1097/PPO.0b013e318156eb99
5. Chin YF, Lynn N. Systematic review of focal and salvage cryotherapy for prostate cancer. Cureus. (2022) 14:e26400. doi: 10.7759/cureus.26400
6. Ong S, Leonardo M, Chengodu T, Bagguley D, Lawrentschuk N. Irreversible electroporation for prostate cancer. Life (Basel). (2021) 11:490. doi: 10.3390/life11060490
7. Lipson RL, Baldes EJ, Olsen AM. Hematoporphyrin derivative: a new aid for endoscopic detection of Malignant disease. J Thorac Cardiovasc Surg. (1961) 42:623–9. doi: 10.1016/S0022-5223(19)32560-7
8. Dougherty TJ, Lawrence G, Kaufman JH, Boyle D, Weishaupt KR, Goldfarb A. Photoradiation in the treatment of recurrent breast carcinoma. J Natl Cancer Inst. (1979) 62:231–7. doi: 10.1093/jnci/62.2.231
9. Dougherty TJ. Photoradiation therapy for the treatment of Malignant tumors. Cancer Res. (1978) 38:2628–35.
10. Dougherty TJ, Grindey GB, Fiel R, Weishaupt KR, Boyle DG. Photoradiation therapy. II. Cure of animal tumors with hematoporphyrin and light. J Natl Cancer Inst. (1975) 55:115–21. doi: 10.1093/jnci/55.1.115
11. Arumainayagam N, Moore CM, Ahmed HU, Emberton M. Photodynamic therapy for focal ablation of the prostate. World J Urol. (2010) 28:571–6. doi: 10.1007/s00345-010-0554-2
12. Gunaydin G, Gedik ME, Ayan S. Photodynamic therapy for the treatment and diagnosis of cancer-a review of the current clinical status. Front Chem. (2021) 9:686303. doi: 10.3389/fchem.2021.686303
13. Felsher DW. Cancer revoked: oncogenes as therapeutic targets. Nat Rev Cancer. (2003) 3:375–80. doi: 10.1038/nrc1070
14. Moore CM, Pendse D, Emberton M. Photodynamic therapy for prostate cancer–a review of current status and future promise. Nat Clin Pract Urol. (2009) 6:18–30. doi: 10.1038/ncpuro1274
15. Xue Q, Zhang J, Jiao J, Qin W, Yang X. Photodynamic therapy for prostate cancer: recent advances, challenges and opportunities. Front Oncol. (2022) 12:980239. doi: 10.3389/fonc.2022.980239
16. Luby BM, Walsh CD, Zheng G. Advanced photosensitizer activation strategies for smarter photodynamic therapy beacons. Angew Chem Int Ed Engl. (2019) 58:2558–69. doi: 10.1002/anie.201805246
17. Alzeibak R, Mishchenko TA, Shilyagina NY, Balalaeva IV, Vedunova MV, Krysko DV. Targeting immunogenic cancer cell death by photodynamic therapy: past, present and future. J Immunother Cancer. (2021) 91:e001926. doi: 10.1136/jitc-2020-001926
18. Deng K, Li C, Huang S, Xing B, Jin D, Zeng Q, et al. Recent progress in near infrared light triggered photodynamic therapy. Small. (2017) 13. doi: 10.1002/smll.201702299
19. Donohoe C, Senge MO, Arnaut LG, Gomes-da-Silva LC. Cell death in photodynamic therapy: from oxidative stress to anti-tumor immunity. Biochim Biophys Acta Rev Cancer. (2019) 1872:188308. doi: 10.1016/j.bbcan.2019.07.003
20. Li W, Yang J, Luo L, Jiang M, Qin B, Yin H, et al. Targeting photodynamic and photothermal therapy to the endoplasmic reticulum enhances immunogenic cancer cell death. Nat Commun. (2019) 10:3349. doi: 10.1038/s41467-019-11269-8
21. Mo J, Mai Le NP, Priefer R. Evaluating the mechanisms of action and subcellular localization of ruthenium(II)-based photosensitizers. Eur J Med Chem. (2021) 225:113770. doi: 10.1016/j.ejmech.2021.113770
22. Akter S, Saito S, Inai M, Honda N, Hazama H, Nishikawa T, et al. Efficient photodynamic therapy against drug-resistant prostate cancer using replication-deficient virus particles and talaporfin sodium. Lasers Med Sci. (2021) 36:743–50. doi: 10.1007/s10103-020-03076-1
23. Jiang W, Chen J, Gong C, Wang Y, Gao Y, Yuan Y. Intravenous delivery of enzalutamide based on high drug loading multifunctional graphene oxide nanoparticles for castration-resistant prostate cancer therapy. J Nanobiotechnology. (2020) 18:50. doi: 10.1186/s12951-020-00607-4
24. Chen B, Crane C, He C, Gondek D, Agharkar P, Savellano MD, et al. Disparity between prostate tumor interior versus peripheral vasculature in response to verteporfin-mediated vascular-targeting therapy. Int J Cancer. (2008) 123:695–701. doi: 10.1002/ijc.23538
25. Camps JL Jr., Powers SK, Beckman WC Jr, Brown JT, Weissman RM. Photodynamic therapy of prostate cancer: an in vitro study. J Urol. (1985) 134:1222–6. doi: 10.1016/S0022-5347(17)47699-3
26. Panetta JV, Cvetkovic D, Chen X, Chen L, Ma CC. Radiodynamic therapy using 15-MV radiation combined with 5-aminolevulinic acid and carbamide peroxide for prostate cancer in vivo. Phys Med Biol. (2020) 65:165008. doi: 10.1088/1361-6560/ab9776
27. Zhao L, Wang X, Jiang M, Wu X, Zhang M, Guan X, et al. A nanosystem of copper(II)-disulfiram for cancer treatment with high efficacy and few side effects. Front Materials Sci. (2021) 15:553–66. doi: 10.1007/s11706-021-0576-2
28. Colasanti A, Kisslinger A, Liuzzi R, Quarto M, Riccio P, Roberti G, et al. Hypericin photosensitization of tumor and metastatic cell lines of human prostate. J Photochem Photobiol B. (2000) 54:103–7. doi: 10.1016/S1011-1344(99)00149-9
29. Chang SC, Buonaccorsi GA, MacRobert AJ, Brown SG. Interstitial photodynamic therapy in the canine prostate with disulfonated aluminum phthalocyanine and 5-aminolevulinic acid-induced protoporphyrin IX. Prostate. (1997) 32:89–98. doi: 10.1002/(SICI)1097-0045(19970701)32:2<89::AID-PROS3>3.0.CO;2-A
30. Sinha AK, Anand S, Ortel BJ, Chang Y, Mai Z, Hasan T, et al. Methotrexate used in combination with aminolaevulinic acid for photodynamic killing of prostate cancer cells. Br J Cancer. (2006) 95:485–95. doi: 10.1038/sj.bjc.6603273
31. Mangadlao JD, Wang X, McCleese C, Escamilla M, Ramamurthy G, Wang Z, et al. Prostate-specific membrane antigen targeted gold nanoparticles for theranostics of prostate cancer. ACS Nano. (2018) 12:3714–25. doi: 10.1021/acsnano.8b00940
32. Cavalieri E, Rogan E. The molecular etiology and prevention of estrogen-initiated cancers: Ockham's Razor: Pluralitas non est ponenda sine necessitate. Plurality should not be posited without necessity. Mol Aspects Med. (2014) 36:1–55. doi: 10.1016/j.mam.2013.08.002
33. Figge FH, Weiland GS, Manganiello LO. Cancer detection and therapy; affinity of neoplastic, embryonic, and traumatized tissues for porphyrins and metalloporphyrins. Proc Soc Exp Biol Med. (1948) 68:640. doi: 10.3181/00379727-68-16580
34. Kelly JF, Snell ME, Berenbaum MC. Photodynamic destruction of human bladder carcinoma. Br J Cancer. (1975) 31:237–44. doi: 10.1038/bjc.1975.30
35. Schoonees R, de Klerk JN, Murphy GP. Correlation of prostatic blood flow with 65-zinc activity in intact, castrated and testosterone-treated baboons. Invest Urol. (1969) 6:476–84.
36. Pantelides ML, Moore JV, Forbes E, Truscott TG, Blacklock NJ. The uptake of porphyrin and zinc-metalloporphyrin by the primate prostate. Photochem Photobiol. (1993) 57:838–41. doi: 10.1111/j.1751-1097.1993.tb09220.x
37. Wallace AM, Grant JK. Effect of zinc on androgen metabolism in the human hyperplastic prostate. Biochem Soc Trans. (1975) 3:540–2. doi: 10.1042/bst0030540
38. Suzuki T, Tanaka M, Sasaki M, Ichikawa H, Nishie H, Kataoka H. Vascular shutdown by photodynamic therapy using talaporfin sodium. Cancers (Basel). (2020) 12:2369. doi: 10.3390/cancers12092369
39. Santos KLM, Barros RM, da Silva Lima DP, Nunes AMA, Sato MR, Faccio R, et al. Prospective application of phthalocyanines in the photodynamic therapy against microorganisms and tumor cells: A mini-review. Photodiagnosis Photodyn Ther. (2020) 32:102032. doi: 10.1016/j.pdpdt.2020.102032
40. Owari T, Tanaka N, Nakai Y, Miyake M, Anai S, Kishi S, et al. 5-Aminolevulinic acid overcomes hypoxia-induced radiation resistance by enhancing mitochondrial reactive oxygen species production in prostate cancer cells. Br J Cancer. (2022) 127:350–63. doi: 10.1038/s41416-022-01789-4
41. Kennedy JC, Pottier RH, Pross DC. Photodynamic therapy with endogenous protoporphyrin IX: basic principles and present clinical experience. J Photochem Photobiol B. (1990) 6:143–8. doi: 10.1016/1011-1344(90)85083-9
42. Nakayama T, Kobayashi T, Shimpei O, Fukuhara H, Namikawa T, Inoue K, et al. Photoirradiation after aminolevulinic acid treatment suppresses cancer cell proliferation through the HO-1/p21 pathway. Photodiagnosis Photodyn Ther. (2019) 28:10–7. doi: 10.1016/j.pdpdt.2019.07.021
43. Zhang X, Han G, Guo C, Ma Z, Lin M, Wang Y, et al. Design, synthesis and biological evaluation of novel 3(1)-hexyloxy chlorin e(6)-based 15(2)- or 13(1)-amino acid derivatives as potent photosensitizers for photodynamic therapy. Eur J Med Chem. (2020) 207:112715. doi: 10.1016/j.ejmech.2020.112715
44. Song C, Xu W, Wei Z, Ou C, Wu J, Tong J, et al. Anti-LDLR modified TPZ@Ce6-PEG complexes for tumor hypoxia-targeting chemo-/radio-/photodynamic/photothermal therapy. J Mater Chem B. (2020) 8:648–54. doi: 10.1039/C9TB02248A
45. Loewen GM, Pandey R, Bellnier D, Henderson B, Dougherty T. Endobronchial photodynamic therapy for lung cancer. Lasers Surg Med. (2006) 38:364–70. doi: 10.1002/lsm.20354
46. Huntosova V, Stroffekova K. Hypericin in the dark: foe or ally in photodynamic therapy? Cancers (Basel). (2016) 8:93. doi: 10.3390/cancers8100093
47. Moorthy B, Chu C, Carlin DJ. Polycyclic aromatic hydrocarbons: from metabolism to lung cancer. Toxicol Sci. (2015) 145:5–15. doi: 10.1093/toxsci/kfv040
48. Naidoo C, Kruger CA, Abrahamse H. Simultaneous photodiagnosis and photodynamic treatment of metastatic melanoma. Molecules. (2019) 24:3153. doi: 10.3390/molecules24173153
49. Patra JK, Das G, Fraceto LF, Campos EVR, Rodriguez-Torres MP, Acosta-Torres LS, et al. Nano based drug delivery systems: recent developments and future prospects. J Nanobiotechnology. (2018) 16:71. doi: 10.1186/s12951-018-0392-8
50. Rapozzi V, Varchi G, Pietra ED, Ferroni C, Xodo LE. A photodynamic bifunctional conjugate for prostate cancer: an in vitro mechanistic study. Invest New Drugs. (2017) 35:115–23. doi: 10.1007/s10637-016-0396-x
51. Mangadlao JD, Wang X, McCleese C, Escamilla M, Ramamurthy G, Wang Z, et al. Prostate-specific membrane antigen targeted gold nanoparticles for theranostics of prostate cancer. ACS Nano. (2018) 12:3714–25. doi: 10.1021/acsnano.8b00940
52. Liu T, Wu LY, Choi JK, Berkman CE. In vitro targeted photodynamic therapy with a pyropheophorbide–a conjugated inhibitor of prostate-specific membrane antigen. Prostate. (2009) 69:585–94. doi: 10.1002/pros.20909
53. Liu T, Wu LY, Kazak M, Berkman CE. Cell-Surface labeling and internalization by a fluorescent inhibitor of prostate-specific membrane antigen. Prostate. (2008) 68:955–64. doi: 10.1002/pros.20753
54. Stefflova K, Li H, Chen J, Zheng G. Peptide-based pharmacomodulation of a cancer-targeted optical imaging and photodynamic therapy agent. Bioconjug Chem. (2007) 18:379–88. doi: 10.1021/bc0602578
55. Savellano MD, Pogue BW, Hoopes PJ, Vitetta ES, Paulsen KD. Multiepitope HER2 targeting enhances photoimmunotherapy of HER2-overexpressing cancer cells with pyropheophorbide-a immunoconjugates. Cancer Res. (2005) 65:6371–9. doi: 10.1158/0008-5472.CAN-05-0426
56. Derks YHW, Schilham MG, Rijpkema M, Smeets EM, Amatdjais-Groenen HI, Kip A, et al. Imaging and photodynamic therapy of prostate cancer using a theranostic PSMA-targeting ligand. Eur J Nucl Med Mol Imaging. (2023) 50:2872–84. doi: 10.1007/s00259-023-06224-1
57. Zhu D, Liu Z, Li Y, Huang Q, Li X, Li K, et al. Delivery of manganese carbonyl to the tumor microenvironment using Tumor-Derived exosomes for cancer gas therapy and low dose radiotherapy. Biomaterials. (2021) 274:120894. doi: 10.1016/j.biomaterials.2021.120894
58. Bechet D, Couleaud P, Frochot C, Viriot M, Guillemin F, Barberi-Heyob M. Nanoparticles as vehicles for delivery of photodynamic therapy agents. Trends Biotechnol. (2008) 26:612–21. doi: 10.1016/j.tibtech.2008.07.007
59. Gbadamosi A, Ajayi A. Recent advances in molecular biology and biochemistry relationships among gene expression profiles and stress tolerance indices of morphological traits of accessions of cowpea screened under differential drought stress. Recent Advances in Molecular Biology and Biochemistry. (2023) 1:1–24.
60. Alnaimi A, Al-Hamry A, Makableh Y, Adiraju A, Kanoun O. Gold nanoparticles-MWCNT based aptasensor for early diagnosis of prostate cancer. Biosensors (Basel). (2022) 12:1130. doi: 10.3390/bios12121130
61. Aksel M, Kesmez O, Yavas A, Bilgin MD. Titaniumdioxide mediated sonophotodynamic therapy against prostate cancer. J Photochem Photobiol B. (2021) 225:112333. doi: 10.1016/j.jphotobiol.2021.112333
62. Chowdhury P, Roberts AM, Khan S, Hafeez BB, Chauhan SC, Jaggi M, et al. Magnetic nanoformulations for prostate cancer. Drug Discovery Today. (2017) 22:1233–41. doi: 10.1016/j.drudis.2017.04.018
63. de Oliveira CR, Droppa-Almeida D, Padilha FF, de Souza RR, de Albuquerque-Junior RLC. Polymeric nanoparticles for the treatment of prostate cancer- technological prospecting and critical analysis. Recent Pat Nanotechnol. (2023) 17:8–14. doi: 10.2174/1872210516666220131092642
64. Silva VL, Ruiz A, Ali A, Pereira S, Seitsonen J, Ruokolainen J, et al. Hypoxia-targeted cupric-tirapazamine liposomes potentiate radiotherapy in prostate cancer spheroids. Int J Pharm. (2021) 607:121018. doi: 10.1016/j.ijpharm.2021.121018
65. Ncapayi V, Ninan N, Lebepe TC, Parani S, Girija AR, Bright R, et al. Diagnosis of prostate cancer and prostatitis using near infra-red fluorescent agInSe/znS quantum dots. Int J Mol Sci. (2021) 22:12514. doi: 10.3390/ijms222212514
66. Cole AJ, Yang VC, David AE. Cancer theranostics: the rise of targeted magnetic nanoparticles. Trends Biotechnol. (2011) 29:323–32. doi: 10.1016/j.tibtech.2011.03.001
67. Luo D, Johnson A, Wang X, Li H, Erokwu BO, Springer S, et al. Targeted radiosensitizers for MR-guided radiation therapy of prostate cancer. Nano Lett. (2020) 20:7159–67. doi: 10.1021/acs.nanolett.0c02487
68. Lagopati N, Evangelou K, Falaras P, Tsilibary E, Vasileiou P, Havaki S, et al. Nanomedicine: Photo-activated nanostructured titanium dioxide, as a promising anticancer agent. Pharmacol Ther. (2021) 222:107795. doi: 10.1016/j.pharmthera.2020.107795
69. Youssef Z, Vanderesse R, Colombeau L, Baros F, Roques-Carmes T, Frochot C, et al. The application of titanium dioxide, zinc oxide, fullerene, and graphene nanoparticles in photodynamic therapy. Cancer Nanotechnol. (2017) 8):6. doi: 10.1186/s12645-017-0032-2
70. Zou Y, Huang B, Cao L, Deng Y, Su J. Tailored mesoporous inorganic biomaterials: assembly, functionalization, and drug delivery engineering. Adv Mater. (2021) 33:e2005215. doi: 10.1002/adma.202005215
71. Wang X, Xiong T, Cui M, Li N, Li Q, Zhu L, et al. A novel targeted co-delivery nanosystem for enhanced ovarian cancer treatment via multidrug resistance reversion and mTOR-mediated signaling pathway. J Nanobiotechnoly. (2021) 19:444. doi: 10.1186/s12951-021-01139-1
72. Lima-Tenório MK, Pineda EAG, Ahmad NM, Fessi H, Elaissari A. Magnetic nanoparticles: In vivo cancer diagnosis and therapy. Int J Pharm. (2015) 493:313–27. doi: 10.1016/j.ijpharm.2015.07.059
73. Ngen EJ, Azad BB, Boinapally S, Lisok A, Brummet M, Jacob D, et al. MRI assessment of prostate-specific membrane antigen (PSMA) targeting by a PSMA-targeted magnetic nanoparticle: potential for image-guided therapy. Mol Pharm. (2019) 16):2060–8. doi: 10.1021/acs.molpharmaceut.9b00036
74. Manescu Paltanea V, Paltanea G, Antoniac L, Vasilescu M. Magnetic nanoparticles used in oncology. Materials (Basel). (2021) 14:5948. doi: 10.3390/ma14205948
75. Ning S, Zhang T, Lyu M, Lam JWY, Zhu D, Huang Q, et al. A type I AIE photosensitiser-loaded biomimetic nanosystem allowing precise depletion of cancer stem cells and prevention of cancer recurrence after radiotherapy. Biomaterials. (2023) 295:122034. doi: 10.1016/j.biomaterials.2023.122034
76. Karlsson J, Vaughan HJ, Green JJ. Biodegradable polymeric nanoparticles for therapeutic cancer treatments. Annu Rev Chem Biomol Eng. (2018) 9:105–27. doi: 10.1146/annurev-chembioeng-060817-084055
77. Łukasiewicz S, Szczepanowicz K, Podgórna K, Błasiak E, Majeed N, Ogren SOÖ, et al. Encapsulation of clozapine in polymeric nanocapsules and its biological effects. Colloids Surf B Biointerfaces. (2016) 140:342–52. doi: 10.1016/j.colsurfb.2015.12.044
78. Deng S, Gigliobianco MS, Censi R, Di Martino P. Polymeric nanocapsules as nanotechnological alternative for drug delivery system: current status, challenges and opportunities. Nanomaterials (Basel). (2020) 10:847. doi: 10.3390/nano10050847
79. Chen CK, Law WC, Aalinkeel R, Yu Y, Nair B, Wu J, et al. Biodegradable cationic polymeric nanocapsules for overcoming multidrug resistance and enabling drug-gene co-delivery to cancer cells. Nanoscale. (2014) 6:1567–72. doi: 10.1039/c3nr04804g
80. Bangham AD, Standish MM, Watkins JC. Diffusion of univalent ions across the lamellae of swollen phospholipids. J Mol Biol. (1965) 13:238–52. doi: 10.1016/s0022-2836(65)80093-6
81. Lou H, Chu L, Zhou W, Dou J, Teng X, Tan W, et al. A diselenium-bridged covalent organic framework with pH/GSH/photo-triple-responsiveness for highly controlled drug release toward joint chemo/photothermal/chemodynamic cancer therapy. J Mater Chem B. (2022) 10:7955–66. doi: 10.1039/D2TB01015A
82. Apolinário AC, Hauschke L, Nunes JR, Lopes LB. Lipid nanovesicles for biomedical applications: 'What is in a name'? Prog Lipid Res. (2021) 82:101096. doi: 10.1016/j.plipres.2021.101096
83. Moosavian SA, Bianconi V, Pirro M, Sahebkar A. Challenges and pitfalls in the development of liposomal delivery systems for cancer therapy. Semin Cancer Biol. (2021) 69:337–48. doi: 10.1016/j.semcancer.2019.09.025
84. Zhang L, Lin Z, Chen Y, Gao D, Wang P, Lin Y, et al. Co-delivery of Docetaxel and Resveratrol by liposomes synergistically boosts antitumor efficiency against prostate cancer. Eur J Pharm Sci. (2022) 174:106199. doi: 10.1016/j.ejps.2022.106199
85. He Y, de Araujo Junior RF, Cruz LJ, Eich C. Functionalized nanoparticles targeting tumor-associated macrophages as cancer therapy. Pharmaceutics. (2021) 13:1670. doi: 10.3390/pharmaceutics1310167
86. Bruchez M, Moronne M, Gin P, Weiss S, Alivisatos AP. Semiconductor nanocrystals as fluorescent biological labels. Science. (1998) 281:2013–6. doi: 10.1126/science.281.5385.2013
87. Chan WC, Nie S. Quantum dot bioconjugates for ultrasensitive nonisotopic detection. Science. (1998) 281:2016–8. doi: 10.1126/science.281.5385.2016
88. Kargozar S, Hoseini SJ, Milan PB, Hooshmand S, Kim HW, Mozafari M, et al. Quantum dots: A review from concept to clinic. Biotechnol J. (2020) 15:e2000117. doi: 10.1002/biot.202000117
89. Song C, Ran J, Wei Z, Wang Y, Chen S, Lin L, et al. Organic near-infrared-II nanophotosensitizer for safe cancer phototheranostics and improving immune microenvironment against metastatic tumor. ACS Appl Mater Interfaces. (2021) 13:3547–58. doi: 10.1021/acsami.0c18841
90. Ning S, Lyu M, Zhu D, Lam JW, Huang Q, Zhang T, et al. Type-I AIE photosensitizer loaded biomimetic system boosting cuproptosis to inhibit breast cancer metastasis and rechallenge. ACS Nano. (2023) 17:10206–17. doi: 10.1021/acsnano.3c00326
91. Zhao L, Wang X, Lou H, Jiang M, Wu X, Qin J, et al. Buffet-style Cu(II) for enhance disulfiram-based cancer therapy. J Colloid Interface Sci. (2022) 624:734–46. doi: 10.1016/j.jcis.2022.06.009
92. Celli JP, Spring BQ, Rizvi I, Evans CL, Samkoe KS, Verma S, et al. Imaging and photodynamic therapy: mechanisms, monitoring, and optimization. Chem Rev. (2010) 110:2795–838. doi: 10.1021/cr900300p
93. Agostinis P, Berg K, Cengel KA, Foster TH, Girotti AW, Gollnick SO, et al. Photodynamic therapy of cancer: an update. CA Cancer J Clin. (2011) 61:250–81. doi: 10.3322/caac.20114
94. Castro CI, Briceno JC. Perfluorocarbon-based oxygen carriers: review of products and trials. Artif Organs. (2010) 34:622–34. doi: 10.1111/j.1525-1594.2009.00944.x
95. Mesquita MQ, Ferreira AR, Maria da Graça PMS, Ribeiro D, Fardilha M, Faustino MA, et al. Photodynamic therapy of prostate cancer using porphyrinic formulations. J Photochem Photobiol B. (2021) 223:112301. doi: 10.1016/j.jphotobiol.2021.112301
96. Windahl T, Andersson SO, Lofgren L. Photodynamic therapy of localised prostatic cancer. Lancet. (1990) 336:1139. doi: 10.1016/0140-6736(90)92626-s
97. Adam C, Salomon G, Walther S, Zaak D, Khoder W, Becker A, et al. Photodynamic diagnosis using 5-aminolevulinic acid for the detection of positive surgical margins during radical prostatectomy in patients with carcinoma of the prostate: a multicentre, prospective, phase 2 trial of a diagnostic procedure. Eur Urol. (2009) 55:1281–8. doi: 10.1016/j.eururo.2009.02.027
98. Swartling J, Höglund OV, Hansson K, Södersten F, Axelsson J, Lagerstedt AS, et al. Online dosimetry for temoporfin-mediated interstitial photodynamic therapy using the canine prostate as model. J BioMed Opt. (2016) 21:28002. doi: 10.1117/1.JBO.21.2.028002
99. Nathan TR, Whitelaw DE, Chang SC, Lees WR, Ripley PM, Payne H, et al. Photodynamic therapy for prostate cancer recurrence after radiotherapy: a phase I study. J Urol. (2002) 168:1427–32. doi: 10.1016/S0022-5347(05)64466-7
100. Moore CM, Nathan TR, Lees WR, Moose CA, Freeman A, Emberton M, et al. Photodynamic therapy using meso tetra hydroxy phenyl chlorin (mTHPC) in early prostate cancer. Lasers Surg Med. (2006) 38:356–63. doi: 10.1002/lsm.20275
101. Patel H, Mick R, Finlay J, Zhu TC, Rickter E, Cengel KA, et al. Motexafin lutetium-photodynamic therapy of prostate cancer: short- and long-term effects on prostate-specific antigen. Clin Cancer Res. (2008) 14:4869–76. doi: 10.1158/1078-0432.CCR-08-0317
102. Sessler JL, Miller RA. Texaphyrins: new drugs with diverse clinical applications in radiation and photodynamic therapy. Biochem Pharmacol. (2000) 59:733–9. doi: 10.1016/s0006-2952(99)00314-7
103. Du KL, Mick R, Busch TM, Zhu TC, Finlay JC, Yu G, et al. Preliminary results of interstitial motexafin lutetium-mediated PDT for prostate cancer. Lasers Surg Med. (2006) 38:427–34. doi: 10.1002/lsm.20341
104. Weersink RA, Forbes J, Bisland S, Trachtenberg J, Elhilali M, Brun PH, et al. Assessment of cutaneous photosensitivity of TOOKAD (WST09) in preclinical animal models and in patients. Photochem Photobiol. (2005) 81:106–13. doi: 10.1562/2004-05-31-RA-182
105. Weersink RA, Boggards A, Gertner M, Davidson SRH, Zhang K, Netchev G, et al. Techniques for delivery and monitoring of TOOKAD (WST09)-mediated photodynamic therapy of the prostate: clinical experience and practicalities. J Photochem Photobiol B. (2005) 79:211–22. doi: 10.1016/j.jphotobiol.2005.01.008
106. Trachtenberg J, Weersink RA, Davidson SRH, Haider MA, Bogaards A, Gertner MR, et al. Vascular-targeted photodynamic therapy (padoporfin, WST09) for recurrent prostate cancer after failure of external beam radiotherapy: a study of escalating light doses. BJU Int. (2008) 102:556–62. doi: 10.1111/j.1464-410X.2008.07753.x
107. Eymerit-Morin C, Zidane M, Lebdai S, Triau S, Azzouzi AR, Rousselet MC. Histopathology of prostate tissue after vascular-targeted photodynamic therapy for localized prostate cancer. Virchows Arch. (2013) 463:547–52. doi: 10.1007/s00428-013-1454-9
108. Moore CM, Azzouzi A, Barret E, Villers A, Muir GH, Barber NJ, et al. Determination of optimal drug dose and light dose index to achieve minimally invasive focal ablation of localised prostate cancer using WST11-vascular-targeted photodynamic (VTP) therapy. BJU Int. (2015) 116:888–96. doi: 10.1111/bju.12816
109. Rodriguez-Rivera JA, Rodriguez-Lay R, Zegarra-Montes L, Benzaghou F, Gaillac B, Azzouzi AR, et al. Expanding indication of padeliporfin (WST11) vascular-targeted photodynamic therapy: results of prostate cancer Latin-American multicenter study. Actas Urol Esp (Engl Ed). (2018) 42:632–8. doi: 10.1016/j.acuro.2018.02.009
110. Noweski A, Roosen A, Lebdai S, Barret E, Emberton M, Benzaghou F, et al. Medium-term follow-up of vascular-targeted photodynamic therapy of localized prostate cancer using TOOKAD soluble WST-11 (Phase II trials). Eur Urol Focus. (2019) 5:1022–8. doi: 10.1016/j.euf.2018.04.003
111. Flegar L, Baunacke M, Buerk BT, Proschmann R, Zacharis A, Propping S, et al. Decision regret and quality of life after focal therapy with vascular-targeted photodynamic therapy (TOOKAD®) for localized prostate cancer. Urol Int. (2022) 106:903–8. doi: 10.1159/000520084
112. Flegar L, Buerk B, Proschmann R, Propping S, Groeben C, Baunacke M, et al. Vascular-targeted photodynamic therapy in unilateral low-risk prostate cancer in Germany: 2-yr single-centre experience in a real-world setting compared with radical prostatectomy. Eur Urol Focus. (2022) 8:121–7. doi: 10.1016/j.euf.2021.01.018
113. Haider MA, Davidson SRH, Kale AV, Weersink RA, Evans AJ, Toi A, et al. Prostate gland: MR imaging appearance after vascular targeted photodynamic therapy with palladium-bacteriopheophorbide. Radiology. (2007) 244:196–204. doi: 10.1148/radiol.2441060398
114. Yang F, Li S, Jiao M, Wu D, Wang L, Cui Z, et al. Advances of light/ultrasound/magnetic-responsive nanoprobes for visualized theranostics of urinary tumors. ACS Appl Bio Mater. (2022) 5:438–50. doi: 10.1021/acsabm.1c01284
Keywords: photodynamic therapy, localized prostate cancer, photosensitizer, precise treatment, combined therapy
Citation: Xu Y, Tan Q, Sun C, Jia Y, Li S and Yang X (2025) Photodynamic therapy for the precise treatment of localized prostate cancer. Front. Oncol. 15:1454392. doi: 10.3389/fonc.2025.1454392
Received: 25 June 2024; Accepted: 10 January 2025;
Published: 05 February 2025.
Edited by:
Sanjeev Soni, CSIR-Central Scientific Instruments Organisation, IndiaReviewed by:
Daoming Zhu, Southern Medical University, ChinaCopyright © 2025 Xu, Tan, Sun, Jia, Li and Yang. This is an open-access article distributed under the terms of the Creative Commons Attribution License (CC BY). The use, distribution or reproduction in other forums is permitted, provided the original author(s) and the copyright owner(s) are credited and that the original publication in this journal is cited, in accordance with accepted academic practice. No use, distribution or reproduction is permitted which does not comply with these terms.
*Correspondence: Shengxian Li, bGlzaGVuZ3hpYW5AcWR1LmVkdS5jbg==; Xuecheng Yang, bTE4NjYxODA1MDYyQDE2My5jb20=
Disclaimer: All claims expressed in this article are solely those of the authors and do not necessarily represent those of their affiliated organizations, or those of the publisher, the editors and the reviewers. Any product that may be evaluated in this article or claim that may be made by its manufacturer is not guaranteed or endorsed by the publisher.
Research integrity at Frontiers
Learn more about the work of our research integrity team to safeguard the quality of each article we publish.