- Department of Pharmacy, Shaoxing People’s Hospital, Shaoxing, China
The development of tumors and their metastasis relies heavily on the process of angiogenesis. When the volume of a tumor expands, the resulting internal hypoxic conditions trigger the body to enhance the production of various angiogenic factors. These include vascular endothelial growth factor (VEGF), fibroblast growth factor (FGF), platelet-derived growth factor (PDGF), and transforming growth factor-α (TGF-α), all of which work together to stimulate the activation of endothelial cells and catalyze angiogenesis. Antiangiogenic therapy (AAT) aims to normalize tumor blood vessels by inhibiting these angiogenic signals. In this review, we will explore the molecular mechanisms of angiogenesis within the tumor microenvironment, discuss traditional antiangiogenic drugs along with their limitations, examine new antiangiogenic drugs and the advantages of combination therapy, and consider future research directions in the field of antiangiogenic drugs. This comprehensive overview aims to provide insights that may aid in the development of more effective anti-tumor treatments.
1 Introduction
Tumor angiogenesis is the process through which tumor tissue acquires the nutrients and oxygen necessary for its growth and metastasis by inducing the formation of new blood vessels. In 1971, Folkman was the first to propose a connection between tumor growth and angiogenesis (1, 2). The growth of tumors relies on angiogenesis; in situations of internal hypoxia, tumors have the ability to enhance the expression of several pro-angiogenic factors, thus facilitating the activation of endothelial cells and the process of angiogenesis. This process influences the tumor microenvironment, facilitating tumor growth, invasion, and metastasis (3). Key factors involved in this process include the activation and expression rates of vascular endothelial growth factor (VEGF), hypoxia-inducible factor (HIF), Notch signaling, metabolic reprogramming, and non-coding RNAs (4). Antiangiogenic therapy (AAT) aims to normalize tumor blood vessels by inhibiting these angiogenic signals. Given that these signaling pathways play a critical role in tumor angiogenesis, they have become targets for antiangiogenic therapy (5). At present, AAT has become an important therapeutic approach for a range of illnesses, such as cancer. This paper examines the processes that drive tumor angiogenesis and discusses the progress made in antiangiogenic treatments, with the goal of offering fresh perspectives for addressing tumors and other associated disorders.
2 Molecular mechanisms of angiogenesis in the tumor microenvironment
2.1 The pro-angiogenic factors
Tumor cells secrete numerous proangiogenic factors that interact with receptors on endothelial cells in blood vessels, promoting their growth, movement, and the development of new vascular structures. Key factors implicated in this process consist of vascular endothelial growth factor (VEGF), fibroblast growth factor (FGF), platelet-derived growth factor (PDGF), and transforming growth factor-α (TGF-α), among others.
2.1.1 Vascular endothelial growth factor (VEGF) family
The VEGF signaling pathway consists of various VEGF ligands and receptors, including VEGF-A, B, C, D, E, F, and placental growth factor, along with receptors such as VEGFR1, 2, and 3. VEGF-A acts as the primary agent that triggers the activation of endothelial cells (ECs) for angiogenesis (6). It attaches to VEGFR2 located on the ECs’ surface, resulting in the phosphorylation of its intracellular domain and the ensuing activation of downstream signaling cascades, including PI3K/AKT and MAPK/ERK. These mechanisms promote the proliferation and migration of ECs, thereby aiding in neovascularization. As a result, VEGF-A has become a significant focus for anti-angiogenic treatments. Additionally, VEGF is an essential factor in angiogenesis that drives the creation of disorganized and primitive blood vessels in various tumor tissues. It initiates various biological processes, many of which are linked to cancer (7); therefore, VEGF has turned into a critical target for anti-angiogenic therapies.
2.1.2 Fibroblast growth factor (FGF) family
The FGFR family encompasses FGFR1 to FGFR5, characterized by a sequence that is notably conserved and homologous. Comprising an extracellular domain, a hydrophobic transmembrane domain, and an intracellular domain responsible for tyrosine kinase activity, FGFR1 to FGFR4 exhibit considerable conservation across these receptors. Conversely, FGFR5 does not possess the intracellular tyrosine kinase domain, leading to its overactivity in the regulatory fibroblast growth factor (FGF) - FGFR signaling pathway (8, 9). The fibroblast growth factor receptor (FGFR) is classified as a receptor tyrosine kinase (RPTK). The activation of the FGFR signaling axis primarily relies on the binding of the ligand FGF to the FGFR dimer, followed by dimerization with fibroblast growth factor receptor substrate 2 (FRS2) and subsequent autophosphorylation. The activation of downstream signaling pathways, including PI3K/AKT and RAS-MAPK, is initiated by this cascade, both of which are crucial for regulating cell proliferation and apoptosis (10, 11).
2.1.3 Platelet-derived growth factor (PDGF)
Platelet-derived growth factor (PDGF) represents an important category of growth factors, predominantly secreted by platelets, and is essential in the mechanisms of cell proliferation, migration, and differentiation (12). This factor typically exists as a homodimer, primarily consisting of PDGF-AA, PDGF-AB, PDGF-BB, PDGF-CC, and PDGF-DD. It exerts its effects through specific receptors, namely PDGFR-α and PDGFR-β. The binding of PDGF to these receptors activates intracellular signaling pathways, including the PI3K/Akt and MAPK pathways, which promote cell proliferation, migration, lumen formation, and the development of new blood vessels (13, 14). PDGF exhibits a dual role in tumor development; it not only supports tumor growth by enhancing the proliferation and migration of tumor-associated fibroblasts but also promotes tumor angiogenesis, thereby increasing the nutrient supply to tumors (15).
2.1.4 Transformation growth factor- α (TGF-α)
Transforming growth factor alpha (TGF-α) is a small, varied factor belonging to the epidermal growth factor (EGF) family. It plays an essential role in controlling cell growth, differentiation, migration, and survival (16). The effects of TGF-α are mediated through its interaction with the epidermal growth factor receptor (EGFR).This binding activates EGFR, resulting in dimerization and autophosphorylation of the receptor, which subsequently triggers downstream signaling pathways (17). Specifically, it activates the Ras/MAPK signaling pathway to promote cell proliferation and survival, as well as the PI3P/Akt pathway to enhance cell survival and inhibit apoptosis (18). Additionally, TGF-α can alter the tumor microenvironment, promoting vasculogenesis and metastasis (19).
2.2 Extracellular matrix and angiogenesis
Matrix metalloproteinases (MMPs) represent a group of enzymes that depend on zinc and are responsible for breaking down the extracellular matrix, playing an essential role in the angiogenesis process (20). These enzymes notably aid in tumor progression and metastasis by degrading the matrix that encases the tumor, leading to the release of pro-angiogenic factors such as VEGF, which facilitate the formation of new blood vessels that supply oxygen and nutrients to the tumor (21, 22). Moreover, MMPs promote tumor infiltration by modifying the tumor microenvironment, allowing cancer cells to invade adjacent tissues and access the bloodstream, consequently facilitating tumor invasion and metastasis (23).
2.3 Effects of endothelial cells and pericytes
Endothelial cells are specialized cells primarily responsible for forming the inner lining of blood vessels and covering the inner epidermis of both blood and lymphatic vessels. These cells play an essential role in the process of neovascularization. The activation of tumor endothelial cells relies on various signaling pathways. The PI3K/Akt pathway fosters the growth and survival of endothelial cells by regulating their proliferation, survival, and metabolic functions. Additionally, the MAPK/ERK pathway participates in cell proliferation, differentiation, and metastasis, significantly influencing the activation and proliferation of endothelial cells (24). Moreover, the Notch pathway is critical for cell-cell interactions, aiding in the maturation and stability of endothelial cells. Endothelial cells react to angiogenic factors, such as VEGF, which triggers their activation, proliferation, and migration from the surrounding matrix. This sequence of events results in the establishment of new vascular channels through the processes of aggregation, arrangement, and interaction, ultimately leading to the formation of new blood vessels that facilitate tumor growth and dissemination. This process is crucial for tumor progression (25, 26).
Pericytes are specialized cells situated on the lateral aspect of endothelial cells found in the walls of blood vessels, where they play essential roles in vascular development, stability, functionality, and the tumor microenvironment. The relationship between tumor endothelial cells and pericytes is crucial for angiogenesis, tumor progression, and metastasis (27, 28). Growth factors secreted by endothelial cells, such as Vascular Endothelial Growth Factor (VEGF), can stimulate pericyte proliferation and differentiation. Pericytes facilitate endothelial cell differentiation through tight contact with vascular endothelial cells, enabling the formation of a mature vascular lumen. Simultaneously, pericytes contribute to the stabilization and improvement of the structural integrity of blood vessels through the secretion of extracellular matrix elements, which include fibronectin and collagen, as well as multiple growth factors such as Transforming Growth Factor-beta (TGF-β) and Platelet-Derived Growth Factor (PDGF). Activated endothelial cells can increase blood vessel permeability, resulting in fluid and cellular component accumulation within the tumor microenvironment. Nevertheless, pericytes have the ability to control the condition of endothelial cells by means of factors they secrete, aiding in the preservation of standard vascular permeability and thwarting excessive permeability (29). Moreover, the relationship between pericytes and endothelial cells is crucial in the metastatic process of tumor cells. The activation of endothelial cells promotes tumor cell adhesion and enables their traversal across the blood vessel wall by expressing specific cell adhesion molecules. Additionally, pericytes may secrete factors that support tumor cell infiltration during this process. Notably, the deregulation or abnormal activation of pericyte function can lead to alterations in the tumor microenvironment, facilitating tumor cell invasion and metastasis (30).
2.4 Signaling pathway
Tumor angiogenesis is a complex biological process affected by various signaling pathways, such as hypoxia induction factor pathway (HIF) (31), vascular endothelial growth factor pathway (VEGF) (32), fibroblast growth factor pathway (FGF) (33), PI3K/Akt pathway (34), Ras/Raf/MEK/ERK pathway (35), Notch signaling pathway (36), transforming growth factor- β pathway (TGF- β) (37), Wnt/β -catenin pathway (38), these pathways are interwoven and synergistic, determine the dynamic balance of tumor angiogenesis. By effectively targeting these key pathways, it may significantly inhibit tumor growth and metastasis, providing new hope for cancer therapy.
2.5 Impact on the tumor immune microenvironment
The influence of tumor angiogenesis on the tumor immune microenvironment is bidirectional. On one hand, newly formed blood vessels facilitate the infiltration of immune cells; on the other hand, alterations in the tumor microenvironment and abnormal vascular function can impair the functionality of immune cells, thereby promoting tumor growth and metastasis (39) Figure 1.
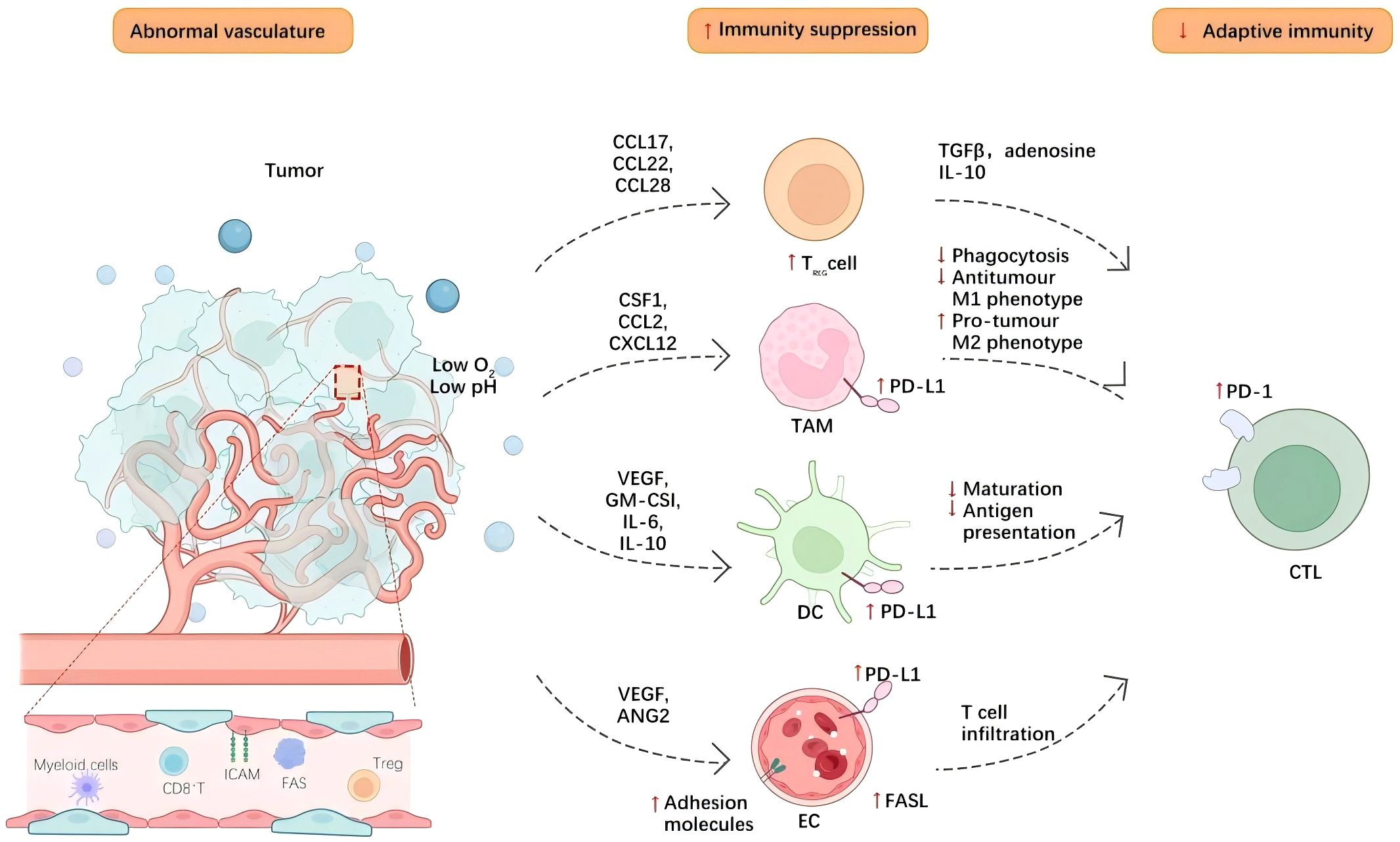
Figure 1. Tumor angiogenesis contributes to an immunosuppressive tumor microenvironment through various mechanisms. Blood vessels within tumors aid in the infiltration of immune cells into the tissue of the tumor, while neovascularization enables lymphocytes, macrophages, and other immune cells to infiltrate the tumor microenvironment more effectively. Cell adhesion molecules, such as selectins and integrins, expressed by tumor endothelial cells, promote interactions between endothelial cells and immune cells, thereby enhancing the adhesion and migration of immune cells. Additionally, neovascularization can induce hypoxia within the tumor microenvironment, leading to functional changes in immune cells. The hypoxic environment can inhibit T-cell function and promote the activation of immunosuppressive cells, including regulatory T cells and tumor-associated macrophages. Concurrently, endothelial cells and tumor cells secrete various cytokines, such as TGF-β-and- IL-10, which can suppress effective antitumor immune responses and further facilitate immune evasion.Hypoxia also elevates the expression of CTLA-4 and TIM-3 on regulatory T cells (Tregs), as well as PD-L1 on myeloid-derived suppressor cells (MDSCs), tumor-associated macrophages (TAMs), and cancerous cells, consequently diminishing the effectiveness of immune-supportive cells. Moreover, endothelial cells within the tumor vasculature, which display diminished amounts of cell adhesion molecules, may trigger endothelial anergy, limiting the ingress of effector T cells into the tumor microenvironment.
Above, we discuss the molecular mechanisms of angiogenesis in the tumor microenvironment, and we know that they not only provide essential nutrients and oxygen for tumor growth and metastasis, also provide help for tumor cell adaptability and tolerance. To combat this tumor process, the researchers have developed a series of therapeutic strategies for angiogenesis. Next, we will explore the mechanism of action of these antiangiogenic drugs, their clinical application and their potential impact in cancer treatment from the traditional antiangiogenic drugs and the new antiangiogenic drugs.
3 Classification and mechanism of action of traditional anti-angiogenic drugs
Traditional anti-angiogenic drugs mainly include macromolecular monoclonal antibodies (mAbs), small molecule tyrosine kinase inhibitors (TKIs), and other categories. Macromolecular monoclonal antibodies are a class of anti-tumor agents that specifically target tumor cells to inhibit their growth or induce cell death. Due to their high specificity and reduced side effects, these antibodies have become a significant class of drugs in cancer treatment. Small-molecule tyrosine kinase inhibitors (TKIs) are targeted therapies employed in the treatment of cancer and other diseases; they block cell proliferation, migration, and survival signals by inhibiting the activity of tyrosine kinases. Additionally, other anti-angiogenic agents include recombinant human vascular endostatin, endothelial cell migration inhibitors, and certain natural traditional Chinese medicines. For further details, please refer to the Table 1 below.
4 New progress in the research of anti-angiogenic drugs
In the treatment of solid tumors, conventional anti-angiogenic therapies that target growth factors, primarily anti-VEGF therapies, have not met expectations regarding efficacy and patient survival. This shortfall can be attributed to both intrinsic and acquired treatment resistance, as well as complications arising from adverse drug reactions. Consequently, current research on anti-angiogenic drugs has focused on strategies to mitigate or delay drug resistance while simultaneously enhancing drug efficacy and reducing or eliminating toxic side effects.
4.1 Drug development based on new targets
Drug development based on new targets is one of the important directions of cancer therapy research in recent years. Researchers continue to explore new angiogenesis-related targets and molecular mechanisms.
4.1.1 gC1qR
The gC1qR protein, referred to as P32/C1qBP/HABP1, is a versatile protein that is found at elevated levels in various types of cancer and possesses prognostic significance. It governs multiple related signaling pathways, such as the Wnt/β-catenin, PKC-NF-κB, and Akt/PKB pathways, which may contribute to the promotion of apoptosis in tumor cells and possess antiangiogenic properties (81). In studies conducted by Peerschke E (82), both in vitro and in vivo experiments demonstrated that the specific monoclonal antibody gC1qR mAb 60.11 could inhibit tumor growth in mouse models of mesothelioma, consequently reducing the proliferation and spread of cancer cells, thus positioning it as a novel target for treating mesothelioma. Currently, several preclinical studies exploring gC1qR-targeted interventions, which include approaches like monoclonal antibodies, chimeric antigen receptor T cell (CAR-T) therapy, and tumor vaccination, are being systematically pursued, yielding promising preclinical therapeutic outcomes (83). The investigation into the targeting of gC1qR marks an emerging area in cancer immunotherapy, offering both prospects and challenges for the progression of clinical treatments.
4.1.2 PDGF
Platelet-derived growth factor (PDGF) represents a class of inhibitors that target PDGF and its receptors (PDGFR). PDGF binds to its receptor (PDGFR), initiating the cell signal transduction pathway and promoting angiogenesis and tumor cell proliferation (84). For instance, Imatinib was initially utilized as a therapeutic agent for acute myeloid leukemia and chronic myeloid leukemia, with its mechanism of action involving the inhibition of PDGFR, thereby demonstrating antitumor activity against certain solid tumors. Moreover, multitargeted tyrosine kinase inhibitors like Sunitinib and Sorafenib not only inhibit the vascular endothelial growth factor receptor (VEGFR) but also target PDGFR, demonstrating their efficacy in treating renal cell carcinoma (85) and hepatocellular carcinoma (86). Novel small-molecule targeted drugs have also entered preclinical or clinical trials, aiming to more precisely inhibit the PDGF/PDGFR signaling pathway without affecting other critical growth factor signaling pathways, thus attracting the attention of developers. Despite some advancements in the development of antitumor drugs targeting PDGF, challenges persist. For example, certain tumors have developed resistance to PDGF intervention (87, 88). Moreover, the specific roles and mechanisms of PDGF in various cancers, beyond lung and kidney cancers, require further elucidation.
4.1.3 MMPs
Metalloproteinases (MMPs) are essential in the processes of angiogenesis and the spread of tumors. In the past few years, there have been notable improvements in the study of inhibitors for metalloproteases (89). Early MMP inhibitors, such as Marimastat, demonstrated some efficacy in preclinical and certain clinical trials; however, their widespread use has been limited due to side effects and efficacy concerns (90). The new generation of small-molecule MMP inhibitors aims to optimize selectivity and pharmacokinetic properties, with examples including Siramesin, BMS-981016, and BMS-845145. These novel compounds exhibit enhanced drug resistance and anti-tumor activity across various cancer types (91). Additionally, inhibitors targeting specific MMPs are under development, such as selective inhibitors against MMP-9, which may offer greater effectiveness and reduced side effects (92). While the prospects for new MMP targets are promising, challenges such as drug resistance and side effects persist. Consequently, enhancing the selectivity and relevance of these inhibitors remains a primary focus of current research and development.
4.1.4 miRNA
Certain microRNAs (miRNAs) can regulate the expression of angiogenesis-related genes by binding to target mRNA, and current drugs targeting miRNAs are under development. miRNA replacement therapy involves introducing a tumor-suppressor miRNA that has been deleted or impaired, such as targeting the deleted miRNAs like miR-34a and miR-29. This area of research is actively progressing (93). MRX 34 is a nanoparticle medication derived from human miR-34a, specifically formulated for the treatment of liver cancer and is presently undergoing assessment for its safety and effectiveness in clinical trials (94). TargomiRs represent an endogenous miRNA repair therapy that utilizes liposome delivery systems encapsulating miRNA combined with targeting mechanisms (such as antibodies) to specifically target tumor cells and enhance the expression of tumor-suppressor miRNAs (95). AntagomiRs, developed in the form of antisense RNA, function by inhibiting specific tumor-promoting miRNAs (e.g., miR-21) (96). Ongoing studies on miR-155 inhibitors are also anticipated to facilitate treatment for lymphoma and some sarcomas (97). Novel tumor drugs based on miRNAs offer new hope for cancer treatment (93, 98, 99). However, despite their considerable potential, miRNA drugs face several challenges. For instance, the effective delivery of miRNAs or their inhibitors is a critical issue due to the biocompatibility and tissue specificity of the drugs themselves. Additionally, the specificity and durability of these drugs require attention to ensure the development of selective miRNA therapies that avoid potential adverse effects on normal cells, while also ensuring the stability and longevity of the drug within the body to prevent early degradation (100).
4.2 Multi-target inhibitors
Tumors are complex diseases that often involve the metabolic processes of multiple genes and signaling pathways. Traditional single-target drugs exhibit limited efficacy regarding tumor specificity and the development of drug resistance. In contrast, this new class of multi-target tumor inhibitors can simultaneously target multiple signaling pathways or molecules, thereby enhancing drug efficacy and mitigating or delaying the onset of drug resistance.
Multi-target Tyrosine Kinase Inhibitors (MTKIs) are a type of medication that can inhibit several tyrosine kinases at once. These compounds affect the growth, proliferation, angiogenesis, and metastasis of tumor cells, thus having a broad impact on the tumor microenvironment and playing a vital part in cancer therapy. For instance, Cabozantinib can inhibit the kinase activity of VEGFR, MET, and other receptors, and is utilized in the treatment of thyroid adenocarcinoma (101) and renal cell carcinoma (102). Similarly, Lapatinib targets EGFR and HER2, primarily serving patients with HER2-positive breast cancer (103, 104). Sorafenib inhibits RAF kinase, VEGFR, and PDGFR concurrently, and is predominantly used for hepatocellular carcinoma and renal cell carcinoma (105, 106). Lenvatinib, which targets VEGFR, FGFR, and PDGFR, is indicated for thyroid cancer and hepatocellular carcinoma (60, 107). Apatinib focuses on the inhibition of VEGFR-2 and other related kinases, primarily treating advanced gastric cancer and liver cancer (108, 109). Ponatinib, which targets BCR-ABL and various other kinases, demonstrates significant efficacy in certain leukemia treatments (110). Entrectinib serves as an oral inhibitor targeting the tyrosine kinases TRKA/B/C, ROS1, and ALK, and is utilized in the treatment of solid tumors that harbor NTRK gene fusions as well as non-small cell lung cancer featuring ROS1 mutations (111). European Organisation for Research and Trearch and Treatment of Cancer phase II trial 1317 The ‘CaboGIST’ clinical trial showed that (112), cabbotinib (a multitarget TKI inhibitor) achieved an 82% disease control rate in the treatment of GISTs, which confirmed preclinical findings. The clinical application of anlotinib (a novel multi-target TKI inhibitor) by SunY (113) in patients with advanced solid tumors showed that Anlotinib shows controlled toxicity, long circulation and broad-spectrum antitumor potential.
4.3 Novel antibody drugs
In recent years, with the rapid development of biomedical technology, some new therapeutic strategies have gradually emerged, including Antibody-drug conjugates (ADCs), Peptide-drug conjugates (PDCs), Bispecific antibodies (BsAb), etc., which provide new hope for the treatment of cancer and other diseases.
4.3.1 Antibody-drug conjugate
Antibody-drug conjugates (ADCs) combine antibodies with cytotoxic drugs to deliver toxins directly to the tumor microenvironment through targeted mechanisms, thereby reducing side effects (114, 115). The formation of new carrier proteins and the targeting of drugs to the tumor microenvironment can effectively enhance tumor distribution or activation, thus improving the anti-cancer activity against difficult-to-treat tumors (116). ADC molecules combine the accuracy of antibody-targeted tumor antigen recognition with powerful cytotoxic components, leading to targeted delivery systems for cancers. A key benefit of ADCs is their capacity to enhance the therapeutic index by precisely directing the cytotoxic payload to tumor tissues, which in turn lowers the minimum effective dose (MED) and notably reduces the incidence of adverse events (117). See Table 2 for details.
4.3.2 Peptide-drug conjugates
Peptide-drug conjugates (PDCs) represent an emerging class of targeted therapies composed of a carrier peptide, a toxic payload, and an adaptor that links the payload to the peptide. These conjugates demonstrate enhanced tumor permeability and increased specificity for targeted cancers (132). The first PDC approved by the FDA is Lu-177 DOTA-TATE (Lutatera), which is a conjugate of a radionuclide and the peptide octreotide. Singh S (133) and other studies have shown that the first-line treatment of 177Lu-Dotatate in combination with octreotide LAR significantly prolongs the median progression-free survival of patients with grade 2 or grade 3 advanced gastroenteropancreatic neuroendocrine tumors.For instance, Melphalan flufenamide(a first-in-class alkylating peptide-drug conjugate), In an open-label, phase III LIGHTHOUSE studyp (134)Shows, it has a good efficacy and a high safety profile in the treatment of triple-class refractory relapsed/refractory multiple myeloma (RRMM).ANG1005 is a brain-penetrating peptide-drug conjugate that consists of three paclitaxel molecules covalently linked to Angiopep-2. Research by Kumthekar P (135) and others indicates that ANG1005 exhibits high activity in breast cancer patients with leptomeningeal carcinoma and recurrent brain metastases.
4.3.3 Bispecific antibody
Bispecific antibodies (BISpecific Antibodies) are a class of antibodies capable of simultaneously binding to two distinct target antigens. These antibodies can recognize and attach to two different molecules, typically targeting tumor surface antigens and the surfaces of immune cells. See Table 3 for details.
4.3.4 Other novel targeted antibodies
Other novel targeting antibodies, such as T-cell engager antibodies (TEGEV), are designed to direct T cells to tumors, thereby enhancing the immune system’s attack on cancer. Bispecific T-cell adapter (BiTE) therapy has emerged as one of the most promising treatments (147). For instance, Blinatumomab, an exemplary medication in BiTE therapy, has shown considerable effectiveness in treating advanced acute lymphoblastic leukemia (148). Furthermore, Tarlatamab (AMG 757), a molecule that conjugates dual-specific T cells and interacts with DLL3 and CD3, enhances tumor lysis mediated by T cells and has demonstrated promising outcomes in treating small cell lung cancer (149). Despite the advancements from traditional monoclonal antibodies to the next generation of antibody drugs, several challenges remain. First, drug resistance poses a significant obstacle; thus, developing therapies that address resistance mechanisms is a critical direction for future research. Second, the side effects of antibody drugs, particularly immune-related adverse reactions, require careful attention. The development of more selective antibodies aimed at minimizing these adverse reactions is also a key area of ongoing research.
Emerging anti-tumor treatment strategies such as Antibody-drug conjugates (ADCs), Peptide-drug conjugates (PDCs) and Bispecific antibodies (BsAb) not only have their own characteristics in mechanism, also show good therapeutic effects in clinical application, and gradually show their unique advantages and broad application prospects. However, it is worth noting that monotherapy is often difficult to meet the treatment needs of complex tumor microenvironment and specific tumors, and therefore, the combined application of drugs has gradually become an important research direction. Next, we will explore the strategies for combining antiangiogenic agents with other drugs and their potential advantages in cancer treatment.
4.4 Combination with antiangiogenic agents
4.4.1 Combination of anti-VEGF antibodies with other antitumor agents
Studies have demonstrated that the combination of Bevacizumab with chemotherapy agents, such as cisplatin and paclitaxel, can enhance therapeutic efficacy, particularly in non-small cell lung cancer, colorectal cancer, breast cancer, and other malignancies (150–154). The anti-VEGF antibody has been shown to increase tumor sensitivity to radiotherapy, and its combination with radiotherapy has yielded improved outcomes in liver cancer and brain tumors. Wang et al. (155) tracked 100 patients with esophageal cancer and found that the combination of Bevacizumab with gemcitabine and cisplatin resulted in significant clinical efficacy, effectively improving patient survival rates. Wilke et al. (156) conducted a randomized, placebo-controlled, double-blind phase 3 trial across North America, South America, and 170 countries in Europe, Asia, and Australia. After two years of clinical follow-up, they observed that the combination of paclitaxel and Bevacizumab for advanced gastric cancer outperformed the placebo and paclitaxel group. Additionally, Pfisterer et al. (157) explored the use of Bevacizumab in conjunction with platinum-based agents for recurrent ovarian cancer and established that the combination of carboplatin-PEGylated liposomal doxorubicin and Bevacizumab signifies a novel standard treatment choice for patients with platinum-eligible recurrent ovarian cancer. Eskander and colleagues (158) showed in a randomized phase 3 trial that was both double-blind and placebo-controlled that incorporating Pabolizumab into the standard chemotherapy regimen notably extended progression-free survival among patients suffering from advanced or recurrent endometrial cancer, in comparison to chemotherapy without the addition of Pabolizumab. Furthermore, after five years of clinical observation, de Castro Jr. et al. (159) discovered that patients with locally advanced or metastatic non-small cell lung cancer experienced enhanced treatment outcomes when treated with a combination of Pabolizumab and chemotherapy.
4.4.2 The combination of small-molecule tyrosine kinase inhibitors (TKIs)
Tyrosine kinase inhibitors (TKIs), such as Sorafenib and Sunitinib, when combined with vascular endothelial growth factor (VEGF) inhibitors, can target multiple receptors and inhibit the proliferation and angiogenesis of tumor cells, resulting in a synergistic effect. In a single-arm phase II study, McNamara et al. (160) revealed that Axitinib showed promising and tolerable clinical efficacy in individuals with hepatocellular carcinoma (HCC) who had previously received VEGF inhibitors during the advanced stages of their illness. In the phase 3 COSMIC-312 study, Cabozantinib combined with Atezolizumab significantly improved progression-free survival compared to Sorafenib, showing enhanced disease control and reduced primary progression (161). Motzer and colleagues (162) discovered that the combination of Avelumab (a PD-L1 inhibitor) with Axitinib significantly extended progression-free survival and resulted in an objective response as the first-line treatment for advanced renal cell carcinoma. In an open-label phase 3 trial (KEYNOTE-426), Pembrolizumab combined with Axitinib significantly extended overall survival and progression-free survival, while also increasing objective response rates in previously untreated patients with advanced renal cell carcinoma (163). Long-term follow-up data revealed that the combination of Navolimab and Cabozantinib significantly enhanced overall survival in individuals suffering from advanced renal cell carcinoma, showcasing notable targeting and antitumor effects, with a median overall survival of 32.9 months (164). Xia et al. (165) concluded from a phase II clinical trial that perioperative Carilizumab combined with Apatinib showed promising efficacy and manageable toxicity in patients with resectable liver cancer. The phase 3 trial (CARES-310) conducted by Qin et al. (166) across 95 centers in 13 countries confirmed that Carilizumab provided statistically and clinically significant benefits in progression-free survival and overall survival, establishing it as a new effective first-line treatment option for this patient population. A phase Ib/IIa study showed that (167), allotinib (a multi-target TKI enzyme inhibitor) combined with natuluzumab (immune checkpoint inhibitor) showed a controlled safety and promising efficacy signal in the clinical treatment of non-small cell lung cancer (NSCLC). A phase Ib study (168) showed that vorolanib (a multi-target TKI inhibitor) and pembrolizumab or nivolumab (immune checkpoint inhibitor) showed lower toxicity and promising efficacy in the clinical treatment of advanced solid tumors.
4.4.3 Combination of multiple-target inhibitors
Multi-target anti-angiogenic drugs, such as Cabozantinib, target VEGFR, MET, and other pathways, and their combination with immunotherapy, such as PD-1 antibodies, can significantly enhance anti-tumor efficacy. The preliminary interim evaluation of the randomized phase 3 KEYNOTE-811 study indicated that pabolizumab was evaluated in conjunction with trastuzumab, along with fluoropyrimidine and platinum-based chemotherapy, for individuals with HER2-positive metastatic gastric and gastroesophageal junction cancer, as opposed to a placebo. This interim analysis further demonstrated that the first-line combination of pabolizumab with trastuzumab and chemotherapy was superior to placebo in terms of progression-free survival (169). Margetuximab, an innovative Fc-engineered monoclonal antibody targeting HER2, boosts antibody-dependent cytotoxicity and may promote interaction between innate and adaptive immune systems. This effect is further intensified through checkpoint inhibition, leading to enhanced antitumor responses. Catenacci et al. first reported the combination of anti-HER2 and anti-PD-1 therapies in treating gastroesophageal adenocarcinoma, confirming the safety and efficacy of this approach through phase 3 of KEYNOTE-811 and phase 2 studies of INTEGA (170). Additionally, Shah et al. (171) utilized penpulimab and anlotinib in combination with albumin-bound paclitaxel/gemcitabine (PAAG) in patients with first-line metastatic pancreatic cancer, demonstrating the promising clinical efficacy of anti-angiogenic therapy combined with chemotherapy in this patient population. Conforti et al. (172) discussed the effectiveness and safety of using avelumab, an anti-PD-L1 inhibitor, in conjunction with the anti-angiogenic drug acacitinib for individuals suffering from advanced B3 thymoma and thymic carcinoma.
4.4.4 Combination of nanotech drug delivery systems
The combination of anti-angiogenic drugs and chemotherapy agents utilizing nanotechnology (such as liposomes and polymer nanoparticles) can enhance drug bioavailability and targeting, reduce side effects, and improve anti-tumor efficacy (173). See Table 4 for details.
4.4.5 Antiangiogenic drugs were used in combination with cell therapy
Cell therapy, a form of immunotherapy, utilizes living cells as therapeutic agents to repair, replace, or enhance cellular function, thereby exerting a therapeutic effect. This category includes CAR-T cell therapy, T-cell immunotherapy, and cancer vaccines (181). CAR-T cell therapy involves the modification of a patient’s own T cells through genetic engineering to specifically identify and attack tumor cells. FDA-approved products such as Kymriah and Yescarta have demonstrated significant efficacy in treating acute lymphoblastic leukemia and large B-cell lymphoma, respectively (182). T-cell immunotherapy (183) enhances T cells by introducing them in vitro to the patient, employing technologies such as CRISPR/Cas9 (184) for optimization. Cancer vaccines are formulated using tumor cells or tumor-associated antigens, aimed at activating the body’s immune system to combat tumors. The combination of traditional anti-angiogenic drugs with emerging anti-tumor therapies can produce a synergistic effect, thereby enhancing overall efficacy. Combining anti-angiogenic drugs and cell therapy can improve the therapeutic effect on the one hand. The first use of anti-angiogenic drugs can improve the infiltration of immune cells (such as T cells, NK cells, etc.) in the tumor, and improve the effect of cell therapy. At the same time, antiangiogenic drugs can change the tumor microenvironment, reduce the immune escape ability of tumor cells, and promote the activity of T cells in cell therapy. On the other hand, by reducing drug resistance. The combination of these two treatments can inhibit adaptive changes in tumor cells, slow down drug resistance, and improve efficacy. Finally, the combination may allow lowering the dose required for cell therapy but maintain efficacy (185). At present, several clinical trials are evaluating the effect of combining antiangiogenic drugs with cell therapy, mainly in solid tumors such as mamomas, lung cancer, as well as some hematologic malignancies (such as leukemia, lymphoma), and have achieved good results. Zhang J and colleagues (186) engineered gene-specific targeted CAR-T cells using non-viral approaches via CRISPR-Cas9 technology. Their research indicated that employing non-viral anti-CD19 CAR-T cells integrated with PD1 resulted in an improved safety profile for patients suffering from relapsed or refractory invasive B-cell non-Hodgkin lymphoma (r/rB-NHL), characterized by a reduced rate of mild cytokine release syndrome (CRS) and the absence of neurotoxic effects. Elpamotide functions as an epitope peptide associated with the vascular endothelial growth factor receptor 2 (VEGFR-2), stimulating cytotoxic T lymphocytes (CTL) to target and eliminate VEGFR2-expressing endothelial cells. A single-arm phase II trial showed that gemcitabine with an antiangiogenic vaccine had good resistance and moderate antitumor effects in patients with advanced or recurrent biliary tract cancer (187).Wang and colleagues developed a ferritin nanoparticle platform modified with SpyCatcher that facilitates straightforward and stable covalent attachment to tumor-specific antigens containing SpyTag. They validated through experiments that ferritin nanoparticles loaded with either the HPV16 oncogene E7 peptide antigen or neoantigens derived from MC38 tumor mutations provoke a more robust antigen-specific cytotoxic T lymphocyte (CTL) response and significantly reduce the growth of tumors associated with E7 or MC38. Additionally, the therapeutic impact was further amplified when paired with a PD-1 checkpoint inhibitor (188) Figure 2.
Antiangiogenic agents have been utilized in conjunction with oncolytic cells to enhance the anti-tumor effect (189, 190). These agents reduce tumor blood flow, thereby improving the infection efficiency of the oncolytic virus (191). A lower blood flow rate allows the oncolytic virus to remain within the tumor for a longer duration, facilitating its function. Additionally, antiangiogenic agents may decrease the immunosuppressive environment within the tumor, promoting more efficient replication of the oncolytic virus and its spread to adjacent tumor cells (192). Furthermore, these agents can inhibit tumor recurrence. To some extent, antiangiogenic drugs reduce immunosuppressive factors in the tumor microenvironment, thereby supporting the immune response triggered by oncolytic viruses and aiding in the prevention of tumor recurrence and metastasis (193). Concurrently, the combination of antiangiogenic drugs with oncolytic cells can enhance the immune response (194). The application of oncolytic cells induces the release and presentation of tumor antigens. In this context, antiangiogenic drugs can further opsonize the tumor microenvironment, thereby promoting the immune system’s response and anticancer activity (195). In recent years, several clinical trials have assessed the combination of anti-angiogenic drugs and oncolytic viruses, demonstrating promising efficacy in various refractory solid tumors, including pancreatic and liver cancers (196, 197). Endostatin, an angiogenesis inhibitor, plays a crucial role in tumor growth, tissue invasion, and metastasis. Some researchers have employed adenoviruses containing the human endostatin gene for intratumoral injection without any reported safety concerns. During treatment, serum levels of endostatin increased, and patients exhibited good tolerance (198). Oncolytic virus (OV) therapy represents a novel approach to cancer treatment. Chesney et al. conducted the first randomized trial evaluating the combination of T-VEC and ipilimumab for melanoma, which assessed the addition of oncolytic viruses to checkpoint inhibitors. The results indicated that the combination of Talimogene Laherparepvec and ipilimumab exhibited significantly higher efficacy compared to ipilimumab alone. This combination therapy demonstrated enhanced antitumor activity without introducing any additional safety concerns (199). Meng Y (200) constructed a novel dual-gene recombinant oncolytic adenovirus called RCAd-LTH-shPD-L1 based on the RCAd virus platform, emphasizing the potential of combining local virotherapy and antiangiogenic therapy with ICIs as an effective TME therapy for the treatment of poorly infiltrating tumors. Additionally, the MASTERKEY-265 trial, a Phase Ib/III investigation, assessed the effectiveness of T-VEC in combination with pembrolizumab for individuals with unresectable stage IIIB-IVM1c melanoma. The study demonstrated enhanced antitumor activity and verified that there were no unidentified safety concerns (201).
4.5 The development of individualized treatment strategies
The personalized approach to tumor treatment, often known as precision medicine, entails developing a customized therapeutic plan for every patient, grounded in their distinctive biological traits, genomic data, tumor features, and the associated microenvironment. This method seeks to improve the effectiveness of treatment while reducing avoidable side effects by more precisely pinpointing the particular tumor type and its possible weaknesses.
4.5.1 Antiangiogenic drugs selected according to the molecular characteristics of the tumor
The initial strategy emphasizes vascular endothelial growth factor (VEGF) along with its receptors. One can evaluate the expression levels of VEGF or its receptors through immunohistochemistry (IHC) (202, 203) or molecular biology assays (204). In tumors, heightened levels of VEGF or its receptors often indicate a likelihood of responding to anti-VEGF therapies (205). The second approach examines the characteristics of the tumor microenvironment. Elevated levels of inflammatory markers, such as T cells, in certain tumors may suggest a greater responsiveness to anti-angiogenic drugs (206). The third approach is based on molecular markers. Mutations in specific oncogenes (e.g., HRAS, KRAS) (207) may be associated with sensitivity to anti-angiogenic therapies, necessitating the monitoring of these genetic statuses to guide drug selection. Additionally, some cancer cells harbor mutations in their signaling pathways (such as PI3K/AKT/mTOR) (208), indicating the potential need for combination therapies involving anti-angiogenic drugs and agents targeting these pathways. Lastly, the development of targeted therapies focuses on tumor-specific molecular features, such as particular gene mutations (209). These targeted agents can selectively affect tumor cells while minimizing impact on normal cells, exemplified by drugs like EGFR inhibitors and ALK inhibitors.
4.5.2 Development of treatment plan based on patient genetic background
Initially, tumor genome sequencing (210) can be conducted. Through comprehensive genomic sequencing of patient tumor tissue, we can identify mutations, copy number variants, gene rearrangements, and other genomic alterations (211). These data are instrumental in defining the molecular characteristics of tumors, identifying driver mutations, and pinpointing potential drug targets (212, 213). By pairing a driver mutation with an appropriate drug, we can tailor treatments for molecularly complex and heterogeneous cancers using a combination of customized therapies (214). Song et al. (215) demonstrated for the first time that treatment plans for bispecific antibodies can be customized for advanced non-small cell lung cancer based on the genetic information of the stromal region, with the potential to predict treatment efficacy.
Additionally, we can perform a liquid biopsy, which provides non-invasive options for tumor detection and personalized therapy by detecting circulating tumor DNA (ctDNA) and other biomarkers in cells. Some studies have indicated that urine DNA methylation detection can facilitate early detection and recurrence monitoring of bladder cancer (216).
Moreover, we can develop individualized vaccines based on tumor-specific antigens to activate and enhance the patient’s immune response. The high tumor specificity and immunogenicity of these vaccines are considered the ultimate goal of tumor immunotherapy. New antigen-based dendritic cell (DC) vaccines represent a promising therapeutic modality. One investigator initiated a single-arm pilot study (ChiCTR-O NC-16009100, NCT02956551) using a personalized neoantigen peptide-pulsed autologous DC vaccine for lung cancer.
Furthermore, screening for biomarkers is crucial. Patients most likely to benefit from treatment can be selected based on their tumor biomarkers, such as PD-L1 expression, DNA repair defects (e.g., BRCA mutations), and fusion gene status. Lastly, a risk assessment can be performed by evaluating genetic susceptibility, family history, and other factors to customize surveillance and prevention strategies (217, 218).
5 Conclusion and outlook
Anti-angiogenic drugs have demonstrated remarkable efficacy in the clinical treatment of cancer; however, they continue to encounter numerous limitations and challenges, including response rates, drug resistance, toxicity, side effects, and individual patient variability. A primary concern is the low response rate associated with these therapies. While the efficacy of certain anti-angiogenic agents, such as bevacizumab and sorafenib, is notable in specific cancer types, the overall response rate remains relatively low, resulting in many patients failing to derive significant benefits. Anti-angiogenic agents work by inhibiting tumor growth and dissemination through the disruption of blood supply. Nonetheless, prolonged use can lead to adverse long-term outcomes, including an increased risk of hypoxia-induced local tumor invasion, distant metastasis, revascularization, and tumor recurrence after discontinuation of therapy (219). Additionally, immune evasion by tumor cells significantly contributes to the reduced effectiveness of anti-angiogenic drugs. For instance, Zhang et al. (220) found that inhibition of ALDH2 resulted in decreased PD-L1 protein levels in colorectal cancer (CRC) cells, thereby promoting the infiltration of tumor-infiltrating lymphocytes (TILs) and facilitating the escape of colorectal tumors from immune surveillance. Furthermore, the mechanisms underlying drug resistance are multifaceted. Resistance to anti-angiogenic therapies can be either innate, linked to the host, or acquired by tumor cells. Following anti-angiogenic treatment, tumors may develop resistance through various mechanisms, ultimately leading to treatment failure (221, 222). Examples include the activation of alternative angiogenic pathways, alterations in tumor cell surface antigens, and enhancement of the tumor microenvironment (223–225). Moreover, elements like genetic alterations, vascular mimicry, co-selection of vascular components, and intussusception angiogenesis could also play a role in the emergence of drug resistance (219). Finally, the side effects. Antiangiogenic drugs in the inhibition of tumor angiogenesis, may affect the normal physiological blood vessels, hypertension (226), skin adverse reactions (such as alopecia, hairy, papules pus rash, etc.) (227), cardiotoxicity (228), bleeding, wound healing (229), and jaw bone necrosis (ONJ) (230). Rare adverse reactions such as gastrointestinal tract perforation (231). In addition, the different physical conditions, complications and tumor characteristics of different patients cause great changes in the efficacy and tolerance of drugs (232).
Future research on antiangiogenic drugs can be categorized into several key areas. First, there is a need for the development of new drugs and the identification of novel targets. This includes the creation of more selective and specific anti-angiogenic agents, cancer vaccines, and the exploration of drugs that can simultaneously act on multiple signaling pathways. Second, combination therapy should be emphasized. Antiangiogenic drugs can be effectively combined with modalities such as cell therapy or chemotherapy to enhance overall efficacy. Individualized treatment approaches are also being pursued. With the advancement of biomarkers, genomic analysis, and liquid biopsy methods, as well as molecular monitoring technologies, it becomes possible to track alterations in tumor genomics and evaluate treatment impacts and necessary modifications in a dynamic manner. Ultimately, innovation in technology is essential for future progress. This encompasses the creation of sophisticated targeted drug delivery systems to guarantee that antiangiogenic medications effectively arrive at tumor locations, thereby reducing systemic adverse effects. At the same time, it is important to develop biological imaging technologies that can monitor the distribution and effectiveness of drugs within the body in real-time, facilitating the assessment of treatment responses and the fine-tuning of treatment strategies.
Considerable opportunities exist for the future progression of antiangiogenic medications. Continuous improvements in technology, new drugs, and creative therapeutic approaches are expected to boost the effectiveness and safety of antiangiogenic treatments. With extensive biological studies and thorough clinical trials, it is anticipated that patients will receive more accurate, convenient, and efficient treatment alternatives.
Author contributions
JX: Writing – original draft, Writing – review & editing. ZT: Writing – original draft, Writing – review & editing.
Funding
The author(s) declare that no financial support was received for the research, authorship, and/or publication of this article.
Conflict of interest
The authors declare that the research was conducted in the absence of any commercial or financial relationships that could be construed as a potential conflict of interest.
Publisher’s note
All claims expressed in this article are solely those of the authors and do not necessarily represent those of their affiliated organizations, or those of the publisher, the editors and the reviewers. Any product that may be evaluated in this article, or claim that may be made by its manufacturer, is not guaranteed or endorsed by the publisher.
References
1. Folkman J, Merler E, Abernathy C, Williams G. Isolation of a tumor factor responsible for angiogenesis. J Exp Med. (1971) 133:275–88. doi: 10.1084/jem.133.2.275
2. Folkman J. Tumor angiogenesis: therapeutic implications. N Engl J Med. (1971) 285:1182–6. doi: 10.1056/NEJM197111182852108
3. Jiang X, Wang J, Deng X, Xiong F, Zhang S, Gong Z, et al. The role of microenvironment in tumor angiogenesis. J Exp Clin Cancer Res. (2020) 39:204. doi: 10.1186/s13046-020-01709-5
4. Sobierajska K, Ciszewski WM, Sacewicz-Hofman I, Niewiarowska J. Endothelial cells in the tumor microenvironment. Adv Exp Med Biol. (2020) 1234:71–86. doi: 10.1007/978-3-030-37184-5_6
5. Gacche RN. Changing landscape of anti-angiogenic therapy: Novel approaches and clinical perspectives. Biochim Biophys Acta Rev Cancer. (2023) 1878:189020. doi: 10.1016/j.bbcan.2023.189020
6. Apte RS, Chen DS, Ferrara N. VEGF in signaling and disease: beyond discovery and development. Cell. (2019) 176:1248–64. doi: 10.1016/j.cell.2019.01.021
7. Dai J, Rabie AB. VEGF: an essential mediator of both angiogenesis and endochondral ossification. J Dent Res. (2007) 86:937–50. doi: 10.1177/154405910708601006
8. Xie Y, Su N, Yang J, Tan Q, Huang S, Jin M, et al. FGF/FGFR signaling in health and disease. Signal Transduct Target Ther. (2020) 5:181. doi: 10.1038/s41392-020-00222-7
9. Zhou Y, Wu C, Lu G, Hu Z, Chen Q, Du X. FGF/FGFR signaling pathway involved resistance in various cancer types. J Cancer. (2020) 11:2000–7. doi: 10.7150/jca.40531
10. Giacomini A, Grillo E, Rezzola S, Ribatti D, Rusnati M, Ronca R, et al. The FGF/FGFR system in the physiopathology of the prostate gland. Physiol Rev. (2021) 101:569–610. doi: 10.1152/physrev.00005.2020
11. Yang L, Zhou F, Zheng D, Wang D, Li X, Zhao C, et al. FGF/FGFR signaling: From lung development to respiratory diseases. Cytokine Growth Factor Rev. (2021) 62:94–104. doi: 10.1016/j.cytogfr.2021.09.002
12. Zou X, Tang XY, Qu ZY, Sun ZW, Ji CF, Li YJ, et al. Targeting the PDGF/PDGFR signaling pathway for cancer therapy: A review. Int J Biol Macromol. (2022) 202:539–57. doi: 10.1016/j.ijbiomac.2022.01.113
13. Heldin CH. Targeting the PDGF signaling pathway in tumor treatment. Cell Commun Signal. (2013) 11:97. doi: 10.1186/1478-811X-11-97
14. Fredriksson L, Li H, Eriksson U. The PDGF family: four gene products form five dimeric isoforms. Cytokine Growth Factor Rev. (2004) 15:197–204. doi: 10.1016/j.cytogfr.2004.03.007
15. Ostman A. PDGF receptors-mediators of autocrine tumor growth and regulators of tumor vasculature and stroma. Cytokine Growth Factor Rev. (2004) 15:275–86. doi: 10.1016/j.cytogfr.2004.03.002
17. Kimura M, Moteki H, Ogihara M. Role of hepatocyte growth regulators in liver regeneration. Cells. (2023) 12:208. doi: 10.3390/cells12020208
18. Kamentseva RS, Kharchenko MV, Gabdrahmanova GV, Kotov MA, Kosheverova VV, Kornilova ES. EGF, TGF-α and amphiregulin differently regulate endometrium-derived mesenchymal stromal/stem cells. Int J Mol Sci. (2023) 24:13408. doi: 10.3390/ijms241713408
19. Wang Y, Zhang Y, Chen R, Tian X. Autocrine EGF and TGF-α promote primary and acquired resistance to ALK/c-Met kinase inhibitors in non-small-cell lung cancer. Pharmacol Res Perspect. (2023) 11:e01047. doi: 10.1002/prp2.1047
20. Bassiouni W, Ali MAM, Schulz R. Multifunctional intracellular matrix metalloproteinases: implications in disease. FEBS J. (2021) 288:7162–82. doi: 10.1111/febs.15701
21. Moracho N, Learte AIR, Muñoz-Sáez E, Marchena MA, Cid MA, Arroyo AG, et al. Emerging roles of MT-MMPs in embryonic development. Dev Dyn. (2022) 251:240–75. doi: 10.1002/dvdy.398
22. Asgari R, Vaisi-Raygani A, Aleagha MSE, Mohammadi P, Bakhtiari M, Arghiani N. CD147 and MMPs as key factors in physiological and pathological processes. BioMed Pharmacother. (2023) 157:113983. doi: 10.1016/j.biopha.2022.113983
23. Zitka O, Kukacka J, Krizkova S, Huska D, Adam V, Masarik M, et al. Matrix metalloproteinases. Curr Med Chem. (2010) 17:3751–68. doi: 10.2174/092986710793213724
24. Trimm E, Red-Horse K. Vascular endothelial cell development and diversity. Nat Rev Cardiol. (2023) 20:197–210. doi: 10.1038/s41569-022-00770-1
25. Li Y, Lui KO, Zhou B. Reassessing endothelial-to-mesenchymal transition in cardiovascular diseases. Nat Rev Cardiol. (2018) 15:445–56. doi: 10.1038/s41569-018-0023-y
26. Uldry E, Faes S, Demartines N, Dormond O. Fine-tuning tumor endothelial cells to selectively kill cancer. Int J Mol Sci. (2017) 18:1401. doi: 10.3390/ijms18071401
27. Martinez-Morga M, Garrigos D, Rodriguez-Montero E, Pombero A, Garcia-Lopez R, Martinez S. Pericytes are immunoregulatory cells in glioma genesis and progression. Int J Mol Sci. (2024) 25:5072. doi: 10.3390/ijms25105072
28. Alarcon-Martinez L, Yemisci M, Dalkara T. Pericyte morphology and function. Histol Histopathol. (2021) 36:633–43. doi: 10.14670/HH-18-314
29. Giannoni P, Badaut J, Dargazanli C, Fayd’Herbe De Maudave A, Klement W, Costalat V, et al. The pericyte-glia interface at the blood-brain barrier. Clin Sci (Lond). (2018) 132:361–74. doi: 10.1042/CS20171634
30. Attwell D, Mishra A, Hall CN, O’Farrell FM, Dalkara T. What is a pericyte? J Cereb Blood Flow Metab. (2016) 36:451–5. doi: 10.1177/0271678X15610340
31. Lee JW, Ko J, Ju C, Eltzschig HK. Hypoxia signaling in human diseases and therapeutic targets. Exp Mol Med. (2019) 51:1–13. doi: 10.1038/s12276-019-0235-1
32. Ahmad A, Nawaz MI. Molecular mechanism of VEGF and its role in pathological angiogenesis. J Cell Biochem. (2022) 123:1938–65. doi: 10.1002/jcb.30344
33. Lieu C, Heymach J, Overman M, Tran H, Kopetz S. Beyond VEGF: inhibition of the fibroblast growth factor pathway and antiangiogenesis. Clin Cancer Res. (2011) 17:6130–9. doi: 10.1158/1078-0432.CCR-11-0659
34. Maharati A, Moghbeli M. PI3K/AKT signaling pathway as a critical regulator of epithelial-mesenchymal transition in colorectal tumor cells. Cell Commun Signal. (2023) 21:201. doi: 10.1186/s12964-023-01225-x
35. Huang Y, Zhen Y, Chen Y, Sui S, Zhang L. Unraveling the interplay between RAS/RAF/MEK/ERK signaling pathway and autophagy in cancer: From molecular mechanisms to targeted therapy. Biochem Pharmacol. (2023) 217:115842. doi: 10.1016/j.bcp.2023.115842
36. Kopan R, Ilagan MX. The canonical Notch signaling pathway: unfolding the activation mechanism. Cell. (2009) 137:216–33. doi: 10.1016/j.cell.2009.03.045
37. Peng D, Fu M, Wang M, Wei Y, Wei X. Targeting TGF-β signal transduction for fibrosis and cancer therapy. Mol Cancer. (2022) 21:104. doi: 10.1186/s12943-022-01569-x
38. Clevers H, Nusse R. Wnt/β-catenin signaling and disease. Cell. (2012) 149:1192–205. doi: 10.1016/j.cell.2012.05.012
39. De Palma M, Biziato D, Petrova TV. Microenvironmental regulation of tumour angiogenesis. Nat Rev Cancer. (2017) 17:457–74. doi: 10.1038/nrc.2017.51
40. Spicer J, Baird R, Suder A, Cresti N, Corbacho JG, Hogarth L, et al. Phase 1 dose-escalation study of S-222611, an oral reversible dual tyrosine kinase inhibitor of EGFR and HER2, in patients with solid tumours. Eur J Cancer. (2015) 51:137–45. doi: 10.1016/j.ejca.2014.11.003
41. Meric-Bernstam F, Makker V, Oaknin A, Oh DY, Banerjee S, González-Martín A, et al. Efficacy and safety of trastuzumab deruxtecan in patients with HER2-expressing solid tumors: primary results from the DESTINY-panTumor02 phase II trial. J Clin Oncol. (2024) 42:47–58. doi: 10.1200/JCO.23.02005
42. Van Cutsem E, Köhne CH, Hitre E, Zaluski J, Chang Chien CR, Makhson A, et al. Cetuximab and chemotherapy as initial treatment for metastatic colorectal cancer. N Engl J Med. (2009) 360:1408–17. doi: 10.1056/NEJMoa0805019
43. Burtness B, Harrington KJ, Greil R, Soulières D, Tahara M, de Castro G Jr, et al. Pembrolizumab alone or with chemotherapy versus cetuximab with chemotherapy for recurrent or metastatic squamous cell carcinoma of the head and neck (KEYNOTE-048): a randomised, open-label, phase 3 study. Lancet. (2019) 394:1915–28. doi: 10.1016/S0140-6736(19)32591-7. Erratum in: Lancet. 2020 Jan 25;395(10220):272. doi: 10.1016/S0140-6736(20)30116-1. Erratum in: Lancet. 2020 Feb 22;395(10224):564. doi: 10.1016/S0140-6736(20)30254-3. Erratum in: Lancet. 2021 Jun 12;397(10291):2252. doi: 10.1016/S0140-6736(21)01119-3.
44. Park S, Kim TM, Han JY, Lee GW, Shim BY, Lee YG, et al. Randomized study of atezolizumab plus bevacizumab and chemotherapy in patients with EGFR- or ALK-mutated non-small-cell lung cancer (ATTLAS, KCSG-LU19-04). J Clin Oncol. (2024) 42:1241–51. doi: 10.1200/JCO.23.01891. Erratum in: J Clin Oncol. 2024 Jun 17:JCO2401092. doi: 10.1200/JCO. 24. 01092.
45. Zsiros E, Lynam S, Attwood KM, Wang C, Chilakapati S, Gomez EC, et al. Efficacy and safety of pembrolizumab in combination with bevacizumab and oral metronomic cyclophosphamide in the treatment of recurrent ovarian cancer: A phase 2 nonrandomized clinical trial. JAMA Oncol. (2021) 7:78–85. doi: 10.1001/jamaoncol.2020.5945
46. Finn RS, Qin S, Ikeda M, Galle PR, Ducreux M, Kim TY, et al. IMbrave150 investigators. Atezolizumab plus bevacizumab in unresectable hepatocellular carcinoma. N Engl J Med. (2020) 382:1894–905. doi: 10.1056/NEJMoa1915745
47. Gandhi L, Rodríguez-Abreu D, Gadgeel S, Esteban E, Felip E, De Angelis F, et al. KEYNOTE-189 investigators. Pembrolizumab plus chemotherapy in metastatic non-small-cell lung cancer. N Engl J Med. (2018) 378:2078–92. doi: 10.1056/NEJMoa1801005
48. Kelley RK, Ueno M, Yoo C, Finn RS, Furuse J, Ren Z, et al. Pembrolizumab in combination with gemcitabine and cisplatin compared with gemcitabine and cisplatin alone for patients with advanced biliary tract cancer (KEYNOTE-966): a randomised, double-blind, placebo-controlled, phase 3 trial. Lancet. (2023) 401:1853–65. doi: 10.1016/S0140-6736(23)00727-4. Erratum in: Lancet. 2023 Sep 16;402(10406):964. doi: 10.1016/S0140-6736(23)01904-9. Erratum in: Lancet. 2024 Mar 23;403(10432):1140. doi: 10.1016/S0140-6736(24)00545-2.
49. Bockorny B, Macarulla T, Semenisty V, Borazanci E, Feliu J, Ponz-Sarvise M, et al. Motixafortide and pembrolizumab combined to nanoliposomal irinotecan, fluorouracil, and folinic acid in metastatic pancreatic cancer: the COMBAT/KEYNOTE-202 trial. Clin Cancer Res. (2021) 27:5020–7. doi: 10.1158/1078-0432.CCR-21-0929
50. Leonard JP, Trneny M, Izutsu K, Fowler NH, Hong X, Zhu J, et al. AUGMENT: A phase III study of lenalidomide plus rituximab versus placebo plus rituximab in relapsed or refractory indolent lymphoma. J Clin Oncol. (2019) 37:1188–99. doi: 10.1200/JCO.19.00010
51. Younes A, Sehn LH, Johnson P, Zinzani PL, Hong X, Zhu J, et al. Randomized phase III trial of ibrutinib and rituximab plus cyclophosphamide, doxorubicin, vincristine, and prednisone in non-germinal center B-cell diffuse large B-cell lymphoma. J Clin Oncol. (2019) 37:1285–95. doi: 10.1200/JCO.18.02403
52. Camidge DR, Barlesi F, Goldman JW, Morgensztern D, Heist R, Vokes E, et al. Phase ib study of telisotuzumab vedotin in combination with erlotinib in patients with c-met protein-expressing non-small-cell lung cancer. J Clin Oncol. (2023) 41:1105–15. doi: 10.1200/JCO.22.00739
53. Tan L, Tran B, Tie J, Markman B, Ananda S, Tebbutt NC, et al. A phase ib/II trial of combined BRAF and EGFR inhibition in BRAF V600E positive metastatic colorectal cancer and other cancers: the EVICT (Erlotinib and vemurafenib in combination trial) study. Clin Cancer Res. (2023) 29:1017–30. doi: 10.1158/1078-0432.CCR-22-3094
54. Chan KK, Glenny AM, Weldon JC, Furness S, Worthington HV, Wakeford H. Interventions for the treatment of oral and oropharyngeal cancers: targeted therapy and immunotherapy. Cochrane Database Syst Rev. (2015) 2015:CD010341. doi: 10.1002/14651858.CD010341.pub2
55. Mok TS, Wu YL, Thongprasert S, Yang CH, Chu DT, Saijo N, et al. Gefitinib or carboplatin-paclitaxel in pulmonary adenocarcinoma. N Engl J Med. (2009) 361:947–57. doi: 10.1056/NEJMoa0810699
56. Lu S, Dong X, Jian H, Chen J, Chen G, Sun Y, et al. AENEAS: A randomized phase III trial of aumolertinib versus gefitinib as first-line therapy for locally advanced or metastaticNon-small-cell lung cancer with EGFR exon 19 deletion or L858R mutations. J Clin Oncol. (2022) 40:3162–71. doi: 10.1200/JCO.21.02641
57. Soria JC, Ohe Y, Vansteenkiste J, Reungwetwattana T, Chewaskulyong B, Lee KH, et al. FLAURA investigators. Osimertinib in untreated EGFR-mutated advanced non-small-cell lung cancer. N Engl J Med. (2018) 378:113–25. doi: 10.1056/NEJMoa1713137
58. Wu YL, Tsuboi M, He J, John T, Grohe C, Majem M, et al. ADAURA investigators. Osimertinib in resected EGFR-mutated non-small-cell lung cancer. N Engl J Med. (2020) 383:1711–23. doi: 10.1056/NEJMoa2027071
59. Burchert A, Bug G, Fritz LV, Finke J, Stelljes M, Röllig C, et al. Sorafenib maintenance after allogeneic hematopoietic stem cell transplantation for acute myeloid leukemia with FLT3-internal tandem duplication mutation (SORMAIN). J Clin Oncol. (2020) 38:2993–3002. doi: 10.1200/JCO.19.03345
60. Kudo M, Finn RS, Qin S, Han KH, Ikeda K, Piscaglia F, et al. Lenvatinib versus sorafenib in first-line treatment of patients with unresectable hepatocellular carcinoma: a randomised phase 3 non-inferiority trial. Lancet. (2018) 391:1163–73. doi: 10.1016/S0140-6736(18)30207-1
61. Motzer RJ, Tannir NM, McDermott DF, Arén Frontera O, Melichar B, Choueiri TK, et al. CheckMate 214 Investigators. Nivolumab plus Ipilimumab versus Sunitinib in Advanced Renal-Cell Carcinoma. N Engl J Med. (2018) 378:1277–90. doi: 10.1056/NEJMoa1712126
62. Jabbour E, Kantarjian HM, Aldoss I, Montesinos P, Leonard JT, Gómez-Almaguer D, et al. Ponatinib vs imatinib in frontline philadelphia chromosome-positive acute lymphoblastic leukemia: A randomized clinical trial. JAMA. (2024) 331:1814–23. doi: 10.1001/jama.2024.4783
63. Bauer S, Jones RL, Blay JY, Gelderblom H, George S, Schöffski P, et al. Ripretinib versus sunitinib in patients with advanced gastrointestinal stromal tumor after treatment with imatinib (INTRIGUE): A randomized, open-label, phase III trial. J Clin Oncol. (2022) 40:3918–28. doi: 10.1200/JCO.22.00294
64. Foà R, Bassan R, Vitale A, Elia L, Piciocchi A, Puzzolo MC, et al. GIMEMA investigators. Dasatinib-blinatumomab for ph-positive acute lymphoblastic leukemia in adults. N Engl J Med. (2020) 383:1613–23. doi: 10.1056/NEJMoa2016272
65. Herold CI, Chadaram V, Peterson BL, Marcom PK, Hopkins J, Kimmick GG, et al. Phase II trial of dasatinib in patients with metastatic breast cancer using real-time pharmacodynamic tissue biomarkers of Src inhibition to escalate dosing. Clin Cancer Res. (2011) 17:6061–70. doi: 10.1158/1078-0432.CCR-11-1071
66. Wildes TM, Procknow E, Gao F, Dipersio JF, Vij R. Dasatinib in relapsed or plateau-phase multiple myeloma. Leuk Lymphoma. (2009) 50:137–40. doi: 10.1080/10428190802563363
67. Hochhaus A, Saglio G, Hughes TP, Larson RA, Kim DW, Issaragrisil S, et al. Long-term benefits and risks of frontline nilotinib vs imatinib for chronic myeloid leukemia in chronic phase: 5-year update of the randomized ENESTnd trial. Leukemia. (2016) 30:1044–54. doi: 10.1038/leu.2016.5
68. Kantarjian HM, Hughes TP, Larson RA, Kim DW, Issaragrisil S, le Coutre P, et al. Long-term outcomes with frontline nilotinib versus imatinib in newly diagnosed chronic myeloid leukemia in chronic phase: ENESTnd 10-year analysis. Leukemia. (2021) 35:440–53. doi: 10.1038/s41375-020-01111-2. Erratum in: Leukemia. 2021 Jul;35(7):2142-2143. doi: 10.1038/s41375-021-01306-1.
69. Li J, Qin S, Xu R, Yau TC, Ma B, Pan H, et al. CONCUR Investigators. Regorafenib plus best supportive care versus placebo plus best supportive care in Asian patients with previously treated metastatic colorectal cancer (CONCUR): a randomised, double-blind, placebo-controlled, phase 3 trial. Lancet Oncol. (2015) 16:619–29. doi: 10.1016/S1470-2045(15)70156-7
70. Zhai Y, Ma H, Hui Z, Zhao L, Li D, Liang J, et al. HELPER study: A phase II trial of continuous infusion of endostar combined with concurrent etoposide plus cisplatin and radiotherapy for treatment of unresectable stage III non-small-cell lung cancer. Radiother Oncol. (2019) 131:27–34. doi: 10.1016/j.radonc.2018.10.032
71. Hao YZ, Li ML, Ning FL, Wang XW. APJ is associated with treatment response in gastric cancer patients receiving concurrent chemoradiotherapy and endostar therapy. Cancer Biother Radiopharm. (2017) 32:133–8. doi: 10.1089/cbr.2016.2138
72. Spunt SL, Grupp SA, Vik TA, Santana VM, Greenblatt DJ, Clancy J, et al. Phase I study of temsirolimus in pediatric patients with recurrent/refractory solid tumors. J Clin Oncol. (2011) 29:2933–40. doi: 10.1200/JCO.2010.33.4649
73. James ND, Hussain SA, Hall E, Jenkins P, Tremlett J, Rawlings C, et al. BC2001 Investigators. Radiotherapy with or without chemotherapy in muscle-invasive bladder cancer. N Engl J Med. (2012) 366:1477–88. doi: 10.1056/NEJMoa1106106
74. Hirst LW. Randomized controlled trial of topical mitomycin C for ocular surface squamous neoplasia: early resolution. Ophthalmology. (2007) 114:976–82. doi: 10.1016/j.ophtha.2006.09.026
75. Shitara K, Bang YJ, Iwasa S, Sugimoto N, Ryu MH, Sakai D, et al. DESTINY-gastric01 investigators. Trastuzumab deruxtecan in previously treated HER2-positive gastric cancer. N Engl J Med. (2020) 382:2419–30. doi: 10.1056/NEJMoa2004413
76. Wainberg ZA, Melisi D, Macarulla T, Pazo Cid R, Chandana SR, de la Fouchardière C, et al. NALIRIFOX versus nab-paclitaxel and gemcitabine in treatment-naive patients with metastatic pancreatic ductal adenocarcinoma (NAPOLI 3): a randomised, open-label, phase 3 trial. Lancet. (2023) 402:1272–81. doi: 10.1016/S0140-6736(23)01366-1
77. van Hagen P, Hulshof MC, van Lanschot JJ, Steyerberg EW, van Berge Henegouwen MI, Wijnhoven BP, et al. Preoperative chemoradiotherapy for esophageal or junctional cancer. N Engl J Med. (2012) 366:2074–84. doi: 10.1056/NEJMoa1112088
78. Umar SM, Patra S, Kashyap A, Dev JRA, Kumar L, Prasad CP. Quercetin impairs huR-driven progression and migration of triple negative breast cancer (TNBC) cells. Nutr Cancer. (2022) 74:1497–510. doi: 10.1080/01635581.2021.1952628
79. Mokbel K, Mokbel K. Chemoprevention of breast cancer with vitamins and micronutrients: A concise review. In Vivo. (2019) 33:983–97. doi: 10.21873/invivo.11568
80. Chan CY, Lien CH, Lee MF, Huang CY. Quercetin suppresses cellular migration and invasion in human head and neck squamous cell carcinoma (HNSCC). Biomed (Taipei). (2016) 6:15. doi: 10.7603/s40681-016-0015-3
81. Ghebrehiwet B, Geisbrecht BV, Xu X, Savitt AG, Peerschke EIB. The C1q Receptors: Focus on gC1qR/p33 (C1qBP, p32, HABP-1)1. Semin Immunol. (2019) 45:101338. doi: 10.1016/j.smim.2019.101338
82. Peerschke E, Stier K, Li X, Kandov E, de StanChina E, Chang Q, et al. gC1qR/HABP1/p32 is a potential new therapeutic target against mesothelioma. Front Oncol. (2020) 10:1413. doi: 10.3389/fonc.2020.01413
83. Lei Y, Li X, Qin D, Zhang Y, Wang Y. gC1qR: A new target for cancer immunotherapy. Front Immunol. (2023) 14:1095943. doi: 10.3389/fimmu.2023.1095943
84. Antoniades HN. PDGF: a multifunctional growth factor. Baillieres Clin Endocrinol Metab. (1991) 5:595–613. doi: 10.1016/s0950-351x(10)80005-9
85. Bex A, Mulders P, Jewett M, Wagstaff J, van Thienen JV, Blank CU, et al. Comparison of immediate vs deferred cytoreductive nephrectomy in patients with synchronous metastatic renal cell carcinoma receiving sunitinib: the SURTIME randomized clinical trial. JAMA Oncol. (2019) 5:164–70. doi: 10.1001/jamaoncol.2018.5543. Erratum in: JAMA Oncol. 2019 Feb 1;5(2):271. doi: 10.1001/jamaoncol. 2019. 0117.
86. Gounder MM, Mahoney MR, Van Tine BA, Ravi V, Attia S, Deshpande HA, et al. Sorafenib for advanced and refractory desmoid tumors. N Engl J Med. (2018) 379:2417–28. doi: 10.1056/NEJMoa1805052
87. Di Vito A, Ravegnini G, Gorini F, Aasen T, Serrano C, Benuzzi E, et al. The multifaceted landscape behind imatinib resistance in gastrointestinal stromal tumors (GISTs): A lesson from ripretinib. Pharmacol Ther. (2023) 248:108475. doi: 10.1016/j.pharmthera.2023.108475
88. Jin J, Xie Y, Zhang JS, Wang JQ, Dai SJ, He WF, et al. Sunitinib resistance in renal cell carcinoma: From molecular mechanisms to predictive biomarkers. Drug Resist Updat. (2023) 67:100929. doi: 10.1016/j.drup.2023.100929
89. Cui N, Hu M, Khalil RA. Biochemical and biological attributes of matrix metalloproteinases. Prog Mol Biol Transl Sci. (2017) 147:1–73. doi: 10.1016/bs.pmbts.2017.02.005
90. Steward WP, Thomas AL. Marimastat: the clinical development of a matrix metalloproteinase inhibitor. Expert Opin Investig Drugs. (2000) 9:2913–22. doi: 10.1517/13543784.9.12.2913
91. Allemailem KS, Almatroudi A, Alrumaihi F, Almatroodi SA, Alkurbi MO, Basfar GT, et al. Novel approaches of dysregulating lysosome functions in cancer cells by specific drugs and its nanoformulations: A smart approach of modern therapeutics. Int J Nanomed. (2021) 16:5065–98. doi: 10.2147/IJN.S321343
92. Mondal S, Adhikari N, Banerjee S, Amin SA, Jha T. Matrix metalloproteinase-9 (MMP-9) and its inhibitors in cancer: A minireview. Eur J Med Chem. (2020) 194:112260. doi: 10.1016/j.ejmech.2020.112260. Erratum in: Eur J Med Chem. 2020 Nov 1;205:112642. doi: 10.1016/j.ejmech.2020.112642.
93. Diener C, Keller A, Meese E. Emerging concepts of miRNA therapeutics: from cells to clinic. Trends Genet. (2022) 38:613–26. doi: 10.1016/j.tig.2022.02.006
94. Hong DS, Kang YK, Borad M, Sachdev J, Ejadi S, Lim HY, et al. Phase 1 study of MRX34, a liposomal miR-34a mimic, in patients with advanced solid tumours. Br J Cancer. (2020) 122:1630–7. doi: 10.1038/s41416-020-0802-1
95. van Zandwijk N, Pavlakis N, Kao SC, Linton A, Boyer MJ, Clarke S, et al. Safety and activity of microRNA-loaded minicells in patients with recurrent Malignant pleural mesothelioma: a first-in-man, phase 1, open-label, dose-escalation study. Lancet Oncol. (2017) 18:1386–96. doi: 10.1016/S1470-2045(17)30621-6
96. Kara G, Calin GA, Ozpolat B. RNAi-based therapeutics and tumor targeted delivery in cancer. Adv Drug Delivery Rev. (2022) 182:114113. doi: 10.1016/j.addr.2022.114113
97. Kalkusova K, Taborska P, Stakheev D, Smrz D. The role of miR-155 in antitumor immunity. Cancers (Basel). (2022) 14:5414. doi: 10.3390/cancers14215414
98. Mishra S, Yadav T, Rani V. Exploring miRNA based approaches in cancer diagnostics and therapeutics. Crit Rev Oncol Hematol. (2016) 98:12–23. doi: 10.1016/j.critrevonc.2015.10.003
99. Parayath NN, Gandham SK, Amiji MM. Tumor-targeted miRNA nanomedicine for overcoming challenges in immunity and therapeutic resistance. Nanomed (Lond). (2022) 17:1355–73. doi: 10.2217/nnm-2022-0130
100. Hill M, Tran N. miRNA interplay: mechanisms and consequences in cancer. Dis Model Mech. (2021) 14:dmm047662. doi: 10.1242/dmm.047662
101. Elisei R, Schlumberger MJ, Müller SP, Schöffski P, Brose MS, Shah MH, et al. Cabozantinib in progressive medullary thyroid cancer. J Clin Oncol. (2013) 31:3639–46. doi: 10.1200/JCO.2012.48.4659. Erratum in: J Clin Oncol. 2014 Jun 10;32(17):1864.
102. Choueiri TK, Powles T, Burotto M, Escudier B, Bourlon MT, Zurawski B, et al. Nivolumab plus Cabozantinib versus Sunitinib for Advanced Renal-Cell Carcinoma. N Engl J Med. (2021) 384:829–41. doi: 10.1056/NEJMoa2026982
103. Saura C, Oliveira M, Feng YH, Dai MS, Chen SW, Hurvitz SA, et al. Neratinib plus capecitabine versus lapatinib plus capecitabine in HER2-positive metastatic breast cancer previously treated with ≥ 2 HER2-directed regimens: phase III NALA trial. J Clin Oncol. (2020) 38:3138–49. doi: 10.1200/JCO.20.00147
104. Xu B, Yan M, Ma F, Hu X, Feng J, Ouyang Q, et al. Pyrotinib plus capecitabine versus lapatinib plus capecitabine for the treatment of HER2-positive metastatic breast cancer (PHOEBE): a multicentre, open-label, randomised, controlled, phase 3 trial. Lancet Oncol. (2021) 22:351–60. doi: 10.1016/S1470-2045(20)30702-6
105. Ren Z, Xu J, Bai Y, Xu A, Cang S, Du C, et al. ORIENT-32 study group. Sintilimab plus a bevacizumab biosimilar (IBI305) versus sorafenib in unresectable hepatocellular carcinoma (ORIENT-32): a randomised, open-label, phase 2-3 study. Lancet Oncol. (2021) 22:977–90. doi: 10.1016/S1470-2045(21)00252-7. Erratum in: Lancet Oncol. 2021 Aug;22(8):e347. doi: 10.1016/S1470-2045(21)00386-7.
106. Rini BI, Pal SK, Escudier BJ, Atkins MB, Hutson TE, Porta C, et al. Tivozanib versus sorafenib in patients with advanced renal cell carcinoma (TIVO-3): a phase 3, multicentre, randomised, controlled, open-label study. Lancet Oncol. (2020) 21:95–104. doi: 10.1016/S1470-2045(19)30735-1
107. Schlumberger M, Tahara M, Wirth LJ, Robinson B, Brose MS, Elisei R, et al. Lenvatinib versus placebo in radioiodine-refractory thyroid cancer. N Engl J Med. (2015) 372:621–30. doi: 10.1056/NEJMoa1406470
108. Geng R, Li J. Apatinib for the treatment of gastric cancer. Expert Opin Pharmacother. (2015) 16:117–22. doi: 10.1517/14656566.2015.981526
109. Qin S, Li Q, Gu S, Chen X, Lin L, Wang Z, et al. Apatinib as second-line or later therapy in patients with advanced hepatocellular carcinoma (AHELP): a multicentre, double-blind, randomised, placebo-controlled, phase 3 trial. Lancet Gastroenterol Hepatol. (2021) 6:559–68. doi: 10.1016/S2468-1253(21)00109-6
110. Jabbour E, Short NJ, Jain N, Huang X, Montalban-Bravo G, Banerjee P, et al. Ponatinib and blinatumomab for Philadelphia chromosome-positive acute lymphoblastic leukaemia: a US, single-centre, single-arm, phase 2 trial. Lancet Haematol. (2023) 10:e24–34. doi: 10.1016/S2352-3026(22)00319-2
111. Jiang Q, Li M, Li H, Chen L. Entrectinib, a new multi-target inhibitor for cancer therapy. BioMed Pharmacother. (2022) 150:112974. doi: 10.1016/j.biopha.2022.112974
112. Schöffski P, Mir O, Kasper B, Papai Z, Blay JY, Italiano A, et al. Activity and safety of the multi-target tyrosine kinase inhibitor cabozantinib in patients with metastatic gastrointestinal stromal tumour after treatment with imatinib and sunitinib: European Organisation for Research and Treatment of Cancer phase II trial 1317 ‘CaboGIST’. Eur J Cancer. (2020) 134:62–74. doi: 10.1016/j.ejca.2020.04.021
113. Sun Y, Niu W, Du F, Du C, Li S, Wang J, et al. Safety, pharmacokinetics, and antitumor properties of anlotinib, an oral multi-target tyrosine kinase inhibitor, in patients with advanced refractory solid tumors. J Hematol Oncol. (2016) 9:105. doi: 10.1186/s13045-016-0332-8
114. von Arx C, De Placido P, Caltavituro A, Di Rienzo R, Buonaiuto R, De Laurentiis M, et al. The evolving therapeutic landscape of trastuzumab-drug conjugates: Future perspectives beyond HER2-positive breast cancer. Cancer Treat Rev. (2023) 113:102500. doi: 10.1016/j.ctrv.2022.102500
115. Fu Z, Li S, Han S, Shi C, Zhang Y. Antibody drug conjugate: the “biological missile” for targeted cancer therapy. Signal Transduct Target Ther. (2022) 7:93. doi: 10.1038/s41392-022-00947-7
116. Dumontet C, Reichert JM, Senter PD, Lambert JM, Beck A. Antibody-drug conjugates come of age in oncology. Nat Rev Drug Discovery. (2023) 22:641–61. doi: 10.1038/s41573-023-00709-2
117. Maecker H, Jonnalagadda V, Bhakta S, Jammalamadaka V, Junutula JR. Exploration of the antibody-drug conjugate clinical landscape. MAbs. (2023) 15:2229101. doi: 10.1080/19420862.2023.2229101
118. Bardia A, Messersmith WA, Kio EA, Berlin JD, Vahdat L, Masters GA, et al. Sacituzumab govitecan, a Trop-2-directed antibody-drug conjugate, for patients with epithelial cancer: final safety and efficacy results from the phase I/II IMMU-132-01 basket trial. Ann Oncol. (2021) 32:746–56. doi: 10.1016/j.annonc.2021.03.005
119. Doi T, Shitara K, Naito Y, Shimomura A, Fujiwara Y, Yonemori K, et al. Safety, pharmacokinetics, and antitumour activity of trastuzumab deruxtecan (DS-8201), a HER2-targeting antibody-drug conjugate, in patients with advanced breast and gastric or gastro-oesophageal tumours: a phase 1 dose-escalation study. Lancet Oncol. (2017) 18:1512–22. doi: 10.1016/S1470-2045(17)30604-6
120. Shimizu T, Sands J, Yoh K, Spira A, Garon EB, Kitazono S, et al. First-in-human, phase I dose-escalation and dose-expansion study of trophoblast cell-surface antigen 2-directed antibody-drug conjugate datopotamab deruxtecan in non-small-cell lung cancer: TROPION-panTumor01. J Clin Oncol. (2023) 41:4678–87. doi: 10.1200/JCO.23.00059
121. McDermott MSJ, O’Brien NA, Hoffstrom B, Gong K, Lu M, Zhang J, et al. Preclinical efficacy of the antibody-drug conjugate CLDN6-23-ADC for the treatment of CLDN6-positive solid tumors. Clin Cancer Res. (2023) 29:2131–43. doi: 10.1158/1078-0432.CCR-22-2981
122. Ma Y, Huang Y, Zhao Y, Zhao S, Xue J, Yang Y, et al. BL-B01D1, a first-in-class EGFR-HER3 bispecific antibody-drug conjugate, in patients with locally advanced or metastatic solid tumours: a first-in-human, open-label, multicentre, phase 1 study. Lancet Oncol. (2024) 25:901–11. doi: 10.1016/S1470-2045(24)00159-1
123. Qiu MZ, Zhang Y, Guo Y, Guo W, Nian W, Liao W, et al. Evaluation of safety of treatment with anti-epidermal growth factor receptor antibody drug conjugate MRG003 in patients with advanced solid tumors: A phase 1 nonrandomized clinical trial. JAMA Oncol. (2022) 8:1042–6. doi: 10.1001/jamaoncol.2022.0503
124. Gazzah A, Bedard PL, Hierro C, Kang YK, Abdul Razak A, Ryu MH, et al. Safety, pharmacokinetics, and antitumor activity of the anti-CEACAM5-DM4 antibody-drug conjugate tusamitamab ravtansine (SAR408701) in patients with advanced solid tumors: first-in-human dose-escalation study. Ann Oncol. (2022) 33:416–25. doi: 10.1016/j.annonc.2021.12.012
125. Krop IE, Masuda N, Mukohara T, Takahashi S, Nakayama T, Inoue K, et al. Patritumab deruxtecan (HER3-DXd), a human epidermal growth factor receptor 3-directed antibody-drug conjugate, in patients with previously treated human epidermal growth factor receptor 3-expressing metastatic breast cancer: A multicenter, phase I/II trial. J Clin Oncol. (2023) 41:5550–60. doi: 10.1200/JCO.23.00882
126. Banerjee S, Oza AM, Birrer MJ, Hamilton EP, Hasan J, Leary A, et al. Anti-NaPi2b antibody-drug conjugate lifastuzumab vedotin (DNIB0600A) compared with pegylated liposomal doxorubicin in patients with platinum-resistant ovarian cancer in a randomized, open-label, phase II study. Ann Oncol. (2018) 29:917–23. doi: 10.1093/annonc/mdy023
127. Bardia A, Sun S, Thimmiah N, Coates JT, Wu B, Abelman RO, et al. Antibody-drug conjugate sacituzumab govitecan enables a sequential TOP1/PARP inhibitor therapy strategy in patients with breast cancer. Clin Cancer Res. (2024) 30:2917–24. doi: 10.1158/1078-0432.CCR-24-0428
128. Bardia A, Hurvitz SA, Tolaney SM, Loirat D, Punie K, Oliveira M, et al. Sacituzumab govitecan in metastatic triple-negative breast cancer. N Engl J Med. (2021) 384:1529–41. doi: 10.1056/NEJMoa2028485
129. Rottey S, Clarke J, Aung K, Machiels JP, Markman B, Heinhuis KM, et al. Phase I/IIa trial of BMS-986148, an anti-mesothelin antibody-drug conjugate, alone or in combination with nivolumab in patients with advanced solid tumors. Clin Cancer Res. (2022) 28:95–105. doi: 10.1158/1078-0432.CCR-21-1181
130. Shimizu T, Fujiwara Y, Yonemori K, Koyama T, Sato J, Tamura K, et al. First-in-human phase 1 study of MORAb-202, an antibody-drug conjugate comprising farletuzumab linked to eribulin mesylate, in patients with folate receptor-α-positive advanced solid tumors. Clin Cancer Res. (2021) 27:3905–15. doi: 10.1158/1078-0432.CCR-20-4740
131. Sheng X, Yan X, Wang L, Shi Y, Yao X, Luo H, et al. Open-label, multicenter, phase II study of RC48-ADC, a HER2-targeting antibody-drug conjugate, in patients with locally advanced or metastatic urothelial carcinoma. Clin Cancer Res. (2021) 27:43–51. doi: 10.1158/1078-0432
132. Heh E, Allen J, Ramirez F, Lovasz D, Fernandez L, Hogg T, et al. Peptide drug conjugates and their role in cancer therapy. Int J Mol Sci. (2023) 24:829. doi: 10.3390/ijms24010829
133. Singh S, Halperin D, Myrehaug S, Herrmann K, Pavel M, Kunz PL, et al. 177Lu. Lu-DOTA-TATE plus long-acting octreotide versus high-dose long-acting octreotide for the treatment of newly diagnosed, advanced grade 2-3, well-differentiated, gastroenteropancreatic neuroendocrine tumours (NETTER-2): an open-label, randomised, phase 3 study. Lancet. (2024) 403:2807–17. doi: 10.1016/S0140-6736(24)00701-3
134. Pour L, Szarejko M, Bila J, Schjesvold FH, Spicka I, Maisnar V, et al. Efficacy and safety of melflufen plus daratumumab and dexamethasone in relapsed/refractory multiple myeloma: results from the randomized, open-label, phase III LIGHTHOUSE study. Haematologica. (2024) 109:895–905. doi: 10.3324/haematol.2023.283509
135. Kumthekar P, Tang SC, Brenner AJ, Kesari S, Piccioni DE, Anders C, et al. ANG1005, a brain-penetrating peptide-drug conjugate, shows activity in patients with breast cancer with leptomeningeal carcinomatosis and recurrent brain metastases. Clin Cancer Res. (2020) 26:2789–99. doi: 10.1158/1078-0432.CCR-19-3258
136. Thieblemont C, Phillips T, Ghesquieres H, Cheah CY, Clausen MR, Cunningham D, et al. Epcoritamab, a novel, subcutaneous CD3xCD20 bispecific T-cell-engaging antibody, in relapsed or refractory large B-cell lymphoma: dose expansion in a phase I/II trial. J Clin Oncol. (2023) 41:2238–47. doi: 10.1200/JCO.22.01725
137. Budde LE, Sehn LH, Matasar M, Schuster SJ, Assouline S, Giri P, et al. Safety and efficacy of mosunetuzumab, a bispecific antibody, in patients with relapsed or refractory follicular lymphoma: a single-arm, multicentre, phase 2 study. Lancet Oncol. (2022) 23:1055–65. doi: 10.1016/S1470-2045(22)00335-7
138. Chari A, Minnema MC, Berdeja JG, Oriol A, van de Donk NWCJ, Rodríguez-Otero P, et al. Talquetamab, a T-cell-redirecting GPRC5D bispecific antibody for multiple myeloma. N Engl J Med. (2022) 387:2232–44. doi: 10.1056/NEJMoa2204591
139. Hutchings M, Morschhauser F, Iacoboni G, Carlo-Stella C, Offner FC, Sureda A, et al. Glofitamab, a novel, bivalent CD20-targeting T-cell-engaging bispecific antibody, induces durable complete remissions in relapsed or refractory B-cell lymphoma: A phase I trial. J Clin Oncol. (2021) 39:1959–70. doi: 10.1200/JCO.20.03175
140. Usmani SZ, Garfall AL, van de Donk NWCJ, Nahi H, San-Miguel JF, Oriol A, et al. Teclistamab, a B-cell maturation antigen × CD3 bispecific antibody, in patients with relapsed or refractory multiple myeloma (MajesTEC-1): a multicentre, open-label, single-arm, phase 1 study. Lancet. (2021) 398:665–74. doi: 10.1016/S0140-6736(21)01338-6
141. Pillarisetti K, Edavettal S, Mendonça M, Li Y, Tornetta M, Babich A, et al. A T-cell-redirecting bispecific G-protein-coupled receptor class 5 member D x CD3 antibody to treat multiple myeloma. Blood. (2020) 135:1232–43. doi: 10.1182/blood.2019003342
142. Muik A, Garralda E, Altintas I, Gieseke F, Geva R, Ben-Ami E, et al. Preclinical characterization and phase I trial results of a bispecific antibody targeting PD-L1 and 4-1BB (GEN1046) in patients with advanced refractory solid tumors. Cancer Discovery. (2022) 12:1248–65. doi: 10.1158/2159-8290.CD-21-1345
143. Bannerji R, Arnason JE, Advani RH, Brown JR, Allan JN, Ansell SM, et al. Odronextamab, a human CD20×CD3 bispecific antibody in patients with CD20-positive B-cell Malignancies (ELM-1): results from the relapsed or refractory non-Hodgkin lymphoma cohort in a single-arm, multicentre, phase 1 trial. Lancet Haematol. (2022) 9:e327–39. doi: 10.1016/S2352-3026(22)00072-2
144. Gao X, Xu N, Li Z, Shen L, Ji K, Zheng Z, et al. Safety and antitumour activity of cadonilimab, an anti-PD-1/CTLA-4 bispecific antibody, for patients with advanced solid tumours (COMPASSION-03): a multicentre, open-label, phase 1b/2 trial. Lancet Oncol. (2023) 24:1134–46. doi: 10.1016/S1470-2045(23)00411-4
145. Meric-Bernstam F, Beeram M, Hamilton E, Oh DY, Hanna DL, Kang YK, et al. Zanidatamab, a novel bispecific antibody, for the treatment of locally advanced or metastatic HER2-expressing or HER2-amplified cancers: a phase 1, dose-escalation and expansion study. Lancet Oncol. (2022) 23:1558–70. doi: 10.1016/S1470-2045(22)00621-0
146. Wang L, Luo Y, Ren S, Zhang Z, Xiong A, Su C, et al. A phase 1b study of ivonescimab, a programmed cell death protein-1 and vascular endothelial growth factor bispecific antibody, as first- or second-line therapy for advanced or metastatic immunotherapy-naive NSCLC. J Thorac Oncol. (2024) 19:465–75. doi: 10.1016/j.jtho.2023.10.014
147. Zhou S, Liu M, Ren F, Meng X, Yu J. The landscape of bispecific T cell engager in cancer treatment. biomark Res. (2021) 9:38. doi: 10.1186/s40364-021-00294-9
148. Kantarjian H, Stein A, Gökbuget N, Fielding AK, Schuh AC, Ribera JM, et al. Blinatumomab versus chemotherapy for advanced acute lymphoblastic leukemia. N Engl J Med. (2017) 376:836–47. doi: 10.1056/NEJMoa1609783
149. Paz-Ares L, Champiat S, Lai WV, Izumi H, Govindan R, Boyer M, et al. Tarlatamab, a first-in-class DLL3-targeted bispecific T-cell engager, in recurrent small-cell lung cancer: an open-label, phase I study. J Clin Oncol. (2023) 41:2893–903. doi: 10.1200/JCO.22.02823
150. Nyflot MJ, Kruser TJ, Traynor AM, Khuntia D, Yang DT, Hartig GK, et al. Phase 1 trial of bevacizumab with concurrent chemoradiation therapy for squamous cell carcinoma of the head and neck with exploratory functional imaging of tumor hypoxia, proliferation, and perfusion. Int J Radiat Oncol Biol Phys. (2015) 91:942–51. doi: 10.1016/j.ijrobp.2014.11.029
151. Pujade-Lauraine E, Hilpert F, Weber B, Reuss A, Poveda A, Kristensen G, et al. Bevacizumab combined with chemotherapy for platinum-resistant recurrent ovarian cancer: The AURELIA open-label randomized phase III trial. J Clin Oncol. (2014) 32:1302–8. doi: 10.1200/JCO.2013.51.4489. Erratum in: J Clin Oncol. 2014 Dec 10;32(35):4025.
152. Socinski MA, Jotte RM, Cappuzzo F, Orlandi F, Stroyakovskiy D, Nogami N, et al. IMpower150 study group. Atezolizumab for first-line treatment of metastatic nonsquamous NSCLC. N Engl J Med. (2018) 378:2288–301. doi: 10.1056/NEJMoa1716948
153. Nogami N, Barlesi F, Socinski MA, Reck M, Thomas CA, Cappuzzo F, et al. IMpower150 final exploratory analyses for atezolizumab plus bevacizumab and chemotherapy in key NSCLC patient subgroups with EGFR mutations or metastases in the liver or brain. J Thorac Oncol. (2022) 17:309–23. doi: 10.1016/j.jtho.2021.09.014
154. Tewari KS, Burger RA, Enserro D, Norquist BM, Swisher EM, Brady MF, et al. Final overall survival of a randomized trial of bevacizumab for primary treatment of ovarian cancer. J Clin Oncol. (2019) 37:2317–28. doi: 10.1200/JCO.19.01009
155. Wang J, Zhao Q, Cai L, Li J, Chen S. Efficacy of bevacizumab and gemcitabine in combination with cisplatin in the treatment of esophageal cancer and the effect on the incidence of adverse reactions. BioMed Res Int. (2022) 2022:2317181. doi: 10.1155/2022/2317181
156. Wilke H, Muro K, Van Cutsem E, Oh SC, Bodoky G, Shimada Y, et al. Ramucirumab plus paclitaxel versus placebo plus paclitaxel in patients with previously treated advanced gastric or gastro-oesophageal junction adenocarcinoma (RAINBOW): a double-blind, randomised phase 3 trial. Lancet Oncol. (2014) 15:1224–35. doi: 10.1016/S1470-2045(14)70420-6
157. Pfisterer J, Shannon CM, Baumann K, Rau J, Harter P, Joly F, et al. Bevacizumab and platinum-based combinations for recurrent ovarian cancer: a randomised, open-label, phase 3 trial. Lancet Oncol. (2020) 21:699–709. doi: 10.1016/S1470-2045(20)30142-X. Erratum in: Lancet Oncol. 2022 Jun;23(6):e248. doi: 10.1016/S1470-2045(22)00303-5.
158. Eskander RN, Sill MW, Beffa L, Moore RG, Hope JM, Musa FB, et al. Pembrolizumab plus chemotherapy in advanced endometrial cancer. N Engl J Med. (2023) 388:2159–70. doi: 10.1056/NEJMoa2302312
159. De Castro G Jr, Kudaba I, Wu YL, Lopes G, Kowalski DM, Turna HZ, et al. Five-year outcomes with pembrolizumab versus chemotherapy as first-line therapy in patients with non-small-cell lung cancer and programmed death ligand-1 tumor proportion score ≥ 1% in the KEYNOTE-042 study. J Clin Oncol. (2023) 41:1986–91. doi: 10.1200/JCO.21.02885
160. McNamara MG, Le LW, Horgan AM, Aspinall A, Burak KW, Dhani N, et al. A phase II trial of second-line axitinib following prior antiangiogenic therapy in advanced hepatocellular carcinoma. Cancer. (2015) 121:1620–7. doi: 10.1002/cncr.29227
161. Kelley RK, Oliver J W, Hazra S, Benzaghou F, Yau T, Cheng AL, et al. Cabozantinib in combination with atezolizumab versus sorafenib in treatment-naive advanced hepatocellular carcinoma: COSMIC-312 Phase III study design. Future Oncol. (2020) 16:1525–36. doi: 10.2217/fon-2020-0283
162. Motzer RJ, Penkov K, Haanen J, Rini B, Albiges L, Campbell MT, et al. Avelumab plus Axitinib versus Sunitinib for Advanced Renal-Cell Carcinoma. N Engl J Med. (2019) 380:1103–15. doi: 10.1056/NEJMoa1816047
163. Rini BI, Plimack ER, Stus V, Gafanov R, Hawkins R, Nosov D, et al. Pembrolizumab plus Axitinib versus Sunitinib for Advanced Renal-Cell Carcinoma. N Engl J Med. (2019) 380:1116–27. doi: 10.1056/NEJMoa1816714
164. Motzer RJ, Powles T, Burotto M, Escudier B, Bourlon MT, Shah AY, et al. Nivolumab plus cabozantinib versus sunitinib in first-line treatment for advanced renal cell carcinoma (CheckMate 9ER): long-term follow-up results from an open-label, randomised, phase 3 trial. Lancet Oncol. (2022) 23:888–98. doi: 10.1016/S1470-2045(22)00290-X. Erratum in: Lancet Oncol. 2022 Jul;23(7):e319. doi: 10.1016/S1470-2045(22)00346-1. Erratum in: Lancet Oncol. 2022 Sep;23(9):e404. doi: 10.1016/S1470-2045(22)00511-3.
165. Xia Y, Tang W, Qian X, Li X, Cheng F, Wang K, et al. Efficacy and safety of camrelizumab plus apatinib during the perioperative period in resectable hepatocellular carcinoma: a single-arm, open label, phase II clinical trial. J Immunother Cancer. (2022) 10:e004656. doi: 10.1136/jitc-2022-004656
166. Qin S, Chan SL, Gu S, Bai Y, Ren Z, Lin X, et al. CARES-310 Study Group. Camrelizumab plus rivoceranib versus sorafenib as first-line therapy for unresectable hepatocellular carcinoma (CARES-310): a randomised, open-label, international phase 3 study. Lancet. (2023) 402:1133–46. doi: 10.1016/S0140-6736(23)00961-3
167. Zhang B, Liu H, Shi C, Gao Z, Zhong R, Gu A, et al. Safety and efficacy of multi-target TKI combined with nivolumab in check-point inhibitor-refractory patients with advanced NSCLC: a prospective, single-arm, two-stage study. BMC Cancer. (2024) 24:715. doi: 10.1186/s12885-024-12479-0
168. Bagegni NA, Park H, Kraft K, O-Toole M, Gao F, Waqar SN, et al. Phase 1b trial of anti-VEGF/PDGFR vorolanib combined with immune checkpoint inhibitors in patients with advanced solid tumors. Cancer Chemother Pharmacol. (2022) 89:487–97. doi: 10.1007/s00280-022-04406-6
169. Janjigian YY, Kawazoe A, Bai Y, Xu J, Lonardi S, Metges JP, et al. Pembrolizumab plus trastuzumab and chemotherapy for HER2-positive gastric or gastro-oesophageal junction adenocarcinoma: interim analyses from the phase 3 KEYNOTE-811 randomised placebo-controlled trial. Lancet. (2023) 402:2197–208. doi: 10.1016/S0140-6736(23)02033-0
170. Catenacci DVT, Kang YK, Park H, Uronis HE, Lee KW, Ng MCH, et al. Margetuximab plus pembrolizumab in patients with previously treated, HER2-positive gastro-oesophageal adenocarcinoma (CP-MGAH22-05): a single-arm, phase 1b-2 trial. Lancet Oncol. (2020) 21:1066–76. doi: 10.1016/S1470-2045(20)30326-0
171. Sha H, Tong F, Ni J, Sun Y, Zhu Y, Qi L, et al. First-line penpulimab (an anti-PD1 antibody) and anlotinib (an angiogenesis inhibitor) with nab-paclitaxel/gemcitabine (PAAG) in metastatic pancreatic cancer: a prospective, multicentre, biomolecular exploratory, phase II trial. Signal Transduct Target Ther. (2024) 9:143. doi: 10.1038/s41392-024-01857-6
172. Conforti F, Zucali PA, Pala L, Catania C, Bagnardi V, Sala I, et al. Avelumab plus axitinib in unresectable or metastatic type B3 thymomas and thymic carcinomas (CAVEATT): a single-arm, multicentre, phase 2 trial. Lancet Oncol. (2022) 23:1287–96. doi: 10.1016/S1470-2045(22)00542-3
173. Hu Q, Li H, Wang L, Gu H, Fan C. DNA nanotechnology-enabled drug delivery systems. Chem Rev. (2019) 119:6459–506. doi: 10.1021/acs.chemrev.7b00663
174. Tian F, Dahmani FZ, Qiao J, Ni J, Xiong H, Liu T, et al. A targeted nanoplatform co-delivering chemotherapeutic and antiangiogenic drugs as a tool to reverse multidrug resistance in breast cancer. Acta Biomater. (2018) 75:398–412. doi: 10.1016/j.actbio.2018.05.050
175. Chen Y, Li N, Xu B, Wu M, Yan X, Zhong L, et al. Polymer-based nanoparticles for chemo/gene-therapy: Evaluation its therapeutic efficacy and toxicity against colorectal carcinoma. BioMed Pharmacother. (2019) 118:109257. doi: 10.1016/j.biopha.2019.109257
176. Wang Z, Duan X, Lv Y, Zhao Y. Low density lipoprotein receptor (LDLR)-targeted lipid nanoparticles for the delivery of sorafenib and Dihydroartemisinin in liver cancers. Life Sci. (2019) 239:117013. doi: 10.1016/j.lfs.2019.117013
177. Chan MH, Huang WT, Wang J, Liu RS, Hsiao M. Next-generation cancer-specific hybrid theranostic nanomaterials: MAGE-A3 NIR persistent luminescence nanoparticles conjugated to afatinib for in situ suppression of lung adenocarcinoma growth and metastasis. Adv Sci (Weinh). (2020) 7:1903741. doi: 10.1002/advs.201903741
178. Chen W, Yu D, Sun SY, Li F. Nanoparticles for co-delivery of osimertinib and selumetinib to overcome osimertinib-acquired resistance in non-small cell lung cancer. Acta Biomater. (2021) 129:258–68. doi: 10.1016/j.actbio.2021.05.018
179. Zhang Y, Chen H, Feng N, Xin X, Xu Y, Huo P, et al. Construction and antitumor effects of antitumor micelles with cyclic RGD-modified anlotinib. Nanomedicine. (2020) 28:102224. doi: 10.1016/j.nano.2020.102224
180. Nie L, Zheng Z, Chen R, Liang S, Fu P, Wu S, et al. Novel erythrocyte-shaped electrosprayed nanoparticles for co-delivery of paclitaxel and osimertinib: Preparation, characterization, and evaluation. Eur J Pharm Biopharm. (2024) 200:114315. doi: 10.1016/j.ejpb.2024.114315
181. Hu Z, Ott PA, Wu CJ. Towards personalized, tumour-specific, therapeutic vaccines for cancer. Nat Rev Immunol. (2018) 18:168–82. doi: 10.1038/nri.2017.131
182. Maude SL, Laetsch TW, Buechner J, Rives S, Boyer M, Bittencourt H, et al. Tisagenlecleucel in children and young adults with B-cell lymphoblastic leukemia. N Engl J Med. (2018) 378:439–48. doi: 10.1056/NEJMoa1709866
183. Waldman AD, Fritz JM, Lenardo MJ. A guide to cancer immunotherapy: from T cell basic science to clinical practice. Nat Rev Immunol. (2020) 20:651–68. doi: 10.1038/s41577-020-0306-5
184. Chen M, Mao A, Xu M, Weng Q, Mao J, Ji J. CRISPR-Cas9 for cancer therapy: Opportunities and challenges. Cancer Lett. (2019) 447:48–55. doi: 10.1016/j.canlet.2019.01.017
185. Major A, Kamdar M. Selection of bispecific antibody therapies or CAR-T cell therapy in relapsed lymphomas. Hematol Am Soc Hematol Educ Program. (2023) 2023:370–81. doi: 10.1182/hematology.2023000438
186. Zhang J, Hu Y, Yang J, Li W, Zhang M, Wang Q, et al. Non-viral, specifically targeted CAR-T cells achieve high safety and efficacy in B-NHL. Nature. (2022) 609:369–74. doi: 10.1038/s41586-022-05140-y
187. Matsuyama M, Ishii H, Furuse J, Ohkawa S, Maguchi H, Mizuno N, et al. Phase II trial of combination therapy of gemcitabine plus anti-angiogenic vaccination of elpamotide in patients with advanced or recurrent biliary tract cancer. Invest New Drugs. (2015) 33:490–5. doi: 10.1007/s10637-014-0197-z
188. Wang W, Liu Z, Zhou X, Guo Z, Zhang J, Zhu P, et al. Ferritin nanoparticle-based SpyTag/SpyCatcher-enabled click vaccine for tumor immunotherapy. Nanomedicine. (2019) 16:69–78. doi: 10.1016/j.nano.2018.11.009
189. Chakraborty E, Sarkar D. Emerging therapies for hepatocellular carcinoma (HCC). Cancers (Basel). (2022) 14:2798. doi: 10.3390/cancers14112798
190. Wirsching HG, Weller M. Immunotherapy for meningiomas. Adv Exp Med Biol. (2023) 1416:225–34. doi: 10.1007/978-3-031-29750-2_17
191. Li Y, Su Z, Zhao W, Zhang X, Momin N, Zhang C, et al. Multifunctional oncolytic nanoparticles deliver self-replicating IL-12 RNA to eliminate established tumors and prime systemic immunity. Nat Cancer. (2020) 1:882–93. doi: 10.1038/s43018-020-0095-6
192. Kasakovski D, Skrygan M, Gambichler T, Susok L. Advances in targeting cutaneous melanoma. Cancers (Basel). (2021) 13:2090. doi: 10.3390/cancers13092090
193. Du YN, Wei Q, Zhao LJ, Fan CQ, Guo LR, Ye JF, et al. Hydrogel-based co-delivery of CIK cells and oncolytic adenovirus armed with IL12 and IL15 for cancer immunotherapy. BioMed Pharmacother. (2022) 151:113110. doi: 10.1016/j.biopha.2022.113110
194. Ryapolova A, Minskaia E, Gasanov N, Moroz V, Krapivin B, Egorov AD, et al. Development of recombinant oncolytic rVSV-mIL12-mGMCSF for cancer immunotherapy. Int J Mol Sci. (2023) 25:211. doi: 10.3390/ijms25010211
195. Thoidingjam S, Bhatnagar AR, Sriramulu S, Siddiqui F, Nyati S. Optimizing pancreatic cancer therapy: the promise of immune stimulatory oncolytic viruses. Int J Mol Sci. (2024) 25:9912. doi: 10.3390/ijms25189912
196. Wold WS, Toth K. Adenovirus vectors for gene therapy, vaccination and cancer gene therapy. Curr Gene Ther. (2013) 13:421–33. doi: 10.2174/1566523213666131125095046
197. Kumar M, Thangavel C, Becker RC, Sadayappan S. Monoclonal antibody-based immunotherapy and its role in the development of cardiac toxicity. Cancers (Basel). (2020) 13:86. doi: 10.3390/cancers13010086
198. Li HL, Li S, Shao JY, Lin XB, Cao Y, Jiang WQ, et al. Pharmacokinetic and pharmacodynamic study of intratumoral injection of an adenovirus encoding endostatin in patients with advanced tumors. Gene Ther. (2008) 15:247–56. doi: 10.1038/sj.gt.3303038
199. Chesney J, Puzanov I, Collichio F, Singh P, Milhem MM, Glaspy J, et al. Randomized, open-label phase II study evaluating the efficacy and safety of talimogene laherparepvec in combination with ipilimumab versus ipilimumab alone in patients with advanced, unresectable melanoma. J Clin Oncol. (2018) 36:1658–67. doi: 10.1200/JCO.2017.73.7379
200. Meng Y, Liu H, Zhu H, Zhang W, Sun D, Han X, et al. RCAd-LTH-shPD-L1, a double-gene recombinant oncolytic adenovirus with enhanced antitumor immunity, increases lymphocyte infiltration and reshapes the tumor microenvironment. J Immunother Cancer. (2024) 12:e007171. doi: 10.1136/jitc-2023-007171. Erratum in: J Immunother Cancer. 2024 Jan 30;12(1):e007171corr1. doi: 10.1136/jitc-2023-007171corr1. Erratum in: J Immunother Cancer. 2024 Feb 15;12(2):e007171corr2. doi: 10.1136/jitc-2023-007171corr2.
201. LaRocca CJ, Warner SG. Oncolytic viruses and checkpoint inhibitors: combination therapy in clinical trials. Clin Transl Med. (2018) 7:35. doi: 10.1186/s40169-018-0214-5
202. Tan WCC, Nerurkar SN, Cai HY, Ng HHM, Wu D, Wee YTF, et al. Overview of multiplex immunohistochemistry/immunofluorescence techniques in the era of cancer immunotherapy. Cancer Commun (Lond). (2020) 40:135–53. doi: 10.1002/cac2.12023
203. Sukswai N, Khoury JD. Immunohistochemistry innovations for diagnosis and tissue-based biomarker detection. Curr Hematol Malig Rep. (2019) 14:368–75. doi: 10.1007/s11899-019-00533-9
204. Nowak-Sliwinska P, Alitalo K, Allen E, Anisimov A, Aplin AC, Auerbach R, et al. Consensus guidelines for the use and interpretation of angiogenesis assays. Angiogenesis. (2018) 21:425–532. doi: 10.1007/s10456-018-9613-x
205. Neagu AN, Whitham D, Bruno P, Morrissiey H, Darie CA, Darie CC. Omics-based investigations of breast cancer. Molecules. (2023) 28:4768. doi: 10.3390/molecules28124768
206. Giese MA, Hind LE, Huttenlocher A. Neutrophil plasticity in the tumor microenvironment. Blood. (2019) 133:2159–67. doi: 10.1182/blood-2018-11-844548
207. Timar J, Kashofer K. Molecular epidemiology and diagnostics of KRAS mutations in human cancer. Cancer Metastasis Rev. (2020) 39:1029–38. doi: 10.1007/s10555-020-09915-5
208. Tabibzadeh A, Tameshkel FS, Moradi Y, Soltani S, Moradi-Lakeh M, Ashrafi GH, et al. Signal transduction pathway mutations in gastrointestinal (GI) cancers: a systematic review and meta-analysis. Sci Rep. (2020) 10:18713. doi: 10.1038/s41598-020-73770-1
209. Fu C, Yu L, Miao Y, Liu X, Yu Z, Wei M. Peptide-drug conjugates (PDCs): a novel trend of research and development on targeted therapy, hype or hope? Acta Pharm Sin B. (2023) 13:498–516. doi: 10.1016/j.apsb.2022.07.020
210. Nakamura Y, Taniguchi H, Ikeda M, Bando H, Kato K, Morizane C, et al. Clinical utility of circulating tumor DNA sequencing in advanced gastrointestinal cancer: SCRUM-Japan GI-SCREEN and GOZILA studies. Nat Med. (2020) 26:1859–64. doi: 10.1038/s41591-020-1063-5
211. Razavi P, Li BT, Brown DN, Jung B, Hubbell E, Shen R, et al. High-intensity sequencing reveals the sources of plasma circulating cell-free DNA variants. Nat Med. (2019) 25:1928–37. doi: 10.1038/s41591-019-0652-7
212. Xu J, Liao K, Yang X, Wu C, Wu W. Using single-cell sequencing technology to detect circulating tumor cells in solid tumors. Mol Cancer. (2021) 20:104. doi: 10.1186/s12943-021-01392-w. Erratum in: Mol Cancer. 2022 Apr 18;21(1):100. doi: 10.1186/s12943-022-01564-2.
213. Zhang L, Li Z, Skrzypczynska KM, Fang Q, Zhang W, O’Brien SA, et al. Single-cell analyses inform mechanisms of myeloid-targeted therapies in colon cancer. Cell. (2020) 181:442–459. e29. doi: 10.1016/j.cell.2020.03.048
214. Sicklick JK, Kato S, Okamura R, Schwaederle M, Hahn ME, Williams CB, et al. Molecular profiling of cancer patients enables personalized combination therapy: the I-PREDICT study. Nat Med. (2019) 25:744–50. doi: 10.1038/s41591-019-0407-5
215. Song X, Xiong A, Wu F, Li X, Wang J, Jiang T, et al. Spatial multi-omics revealed the impact of tumor ecosystem heterogeneity on immunotherapy efficacy in patients with advanced non-small cell lung cancer treated with bispecific antibody. J Immunother Cancer. (2023) 11:e006234. doi: 10.1136/jitc-2022-006234. Erratum in: J Immunother Cancer. 2023 Jul;11(7):e006234corr1. doi: 10.1136/jitc-2022-006234corr1.
216. Chen X, Zhang J, Ruan W, Huang M, Wang C, Wang H, et al. Urine DNA methylation assay enables early detection and recurrence monitoring for bladder cancer. J Clin Invest. (2020) 130:6278–89. doi: 10.1172/JCI139597
217. Ding Z, Li Q, Zhang R, Xie L, Shu Y, Gao S, et al. Personalized neoantigen pulsed dendritic cell vaccine for advanced lung cancer. Signal Transduct Target Ther. (2021) 6:26. doi: 10.1038/s41392-020-00448-5
218. Jackson SE, Chester JD. Personalised cancer medicine. Int J Cancer. (2015) 137:262–6. doi: 10.1002/ijc.28940
219. Liu ZL, Chen HH, Zheng LL, Sun LP, Shi L. Angiogenic signaling pathways and anti-angiogenic therapy for cancer. Signal Transduct Target Ther. (2023) 8:198. doi: 10.1038/s41392-023-01460-1
220. Zhang H, Xia Y, Wang F, Luo M, Yang K, Liang S, et al. Aldehyde dehydrogenase 2 mediates alcohol-induced colorectal cancer immune escape through stabilizing PD-L1 expression. Adv Sci (Weinh). (2021) 8:2003404. doi: 10.1002/advs.202003404
221. Wang X, Zhang H, Chen X. Drug resistance and combating drug resistance in cancer. Cancer Drug Resist. (2019) 2:141–60. doi: 10.20517/cdr.2019.10
222. Filippelli A, Ciccone V, Donnini S, Ziche M, Morbidelli L. Molecular mechanisms of resistance to anti-angiogenic drugs. Crit Rev Oncog. (2021) 26:39–66. doi: 10.1615/CritRevOncog.2020035422
223. Greenberg JI, Shields DJ, Barillas SG, Acevedo LM, Murphy E, Huang J, et al. A role for VEGF as a negative regulator of pericyte function and vessel maturation. Nature. (2008) 456:809–13. doi: 10.1038/nature07424. Erratum in: Nature. 2009 Feb 26;457(7233):1168.
224. Paolillo M, Schinelli S. Extracellular matrix alterations in metastatic processes. Int J Mol Sci. (2019) 20:4947. doi: 10.3390/ijms20194947
225. Piersma B, Hayward MK, Weaver VM. Fibrosis and cancer: A strained relationship. Biochim Biophys Acta Rev Cancer. (2020) 1873:188356. doi: 10.1016/j.bbcan.2020.188356
226. Cohen JB, Brown NJ, Brown SA, Dent S, van Dorst DCH, Herrmann SM, et al. American heart association council on hypertension; council on arteriosclerosis, thrombosis and vascular biology; and council on the kidney in cardiovascular disease. Cancer Therapy-Related Hypertension: A Sci Statement From Am Heart Assoc Hypertension. (2023) 80:e46–57. doi: 10.1161/HYP.0000000000000224
227. Macdonald JB, Macdonald B, Golitz LE, LoRusso P, Sekulic A. Cutaneous adverse effects of targeted therapies: Part I: Inhibitors of the cellular membrane. J Am Acad Dermatol. (2015) 72:203–18; quiz 219-20. doi: 10.1016/j.jaad.2014.07.032
228. Alameddine RS, Yakan AS, Skouri H, Mukherji D, Temraz S, Shamseddine A. Cardiac and vascular toxicities of angiogenesis inhibitors: The other side of the coin. Crit Rev Oncol Hematol. (2015) 96:195–205. doi: 10.1016/j.critrevonc.2015.05.004
229. Touyz RM, Herrmann SMS, Herrmann J. Vascular toxicities with VEGF inhibitor therapies-focus on hypertension and arterial thrombotic events. J Am Soc Hypertens. (2018) 12:409–25. doi: 10.1016/j.jash.2018.03.008
230. Antonuzzo L, Lunghi A, Petreni P, Brugia M, Laffi A, Giommoni E, et al. Osteonecrosis of the Jaw and Angiogenesis inhibitors: A Revival of a Rare but Serous Side Effect. Curr Med Chem. (2017) 24:3068–76. doi: 10.2174/0929867324666170511113811
231. Wichelmann TA, Abdulmujeeb S, Ehrenpreis ED. Bevacizumab and gastrointestinal perforations: a review from the FDA Adverse Event Reporting System (FAERS) database. Aliment Pharmacol Ther. (2021) 54:1290–7. doi: 10.1111/apt.16601
Keywords: tumor, angiogenic, antiangiogenic agents, anti-angiogenic drugs, antiangiogenic therapy
Citation: Xu J and Tang Z (2024) Progress on angiogenic and antiangiogenic agents in the tumor microenvironment. Front. Oncol. 14:1491099. doi: 10.3389/fonc.2024.1491099
Received: 04 September 2024; Accepted: 31 October 2024;
Published: 19 November 2024.
Edited by:
Sina Naserian, Hôpital Paul Brousse, FranceReviewed by:
Irfan Naseem Bandey, University of Texas MD Anderson Cancer Center, United StatesMohamed Essameldin Abdelgawad, Wake Forest University, United States
Copyright © 2024 Xu and Tang. This is an open-access article distributed under the terms of the Creative Commons Attribution License (CC BY). The use, distribution or reproduction in other forums is permitted, provided the original author(s) and the copyright owner(s) are credited and that the original publication in this journal is cited, in accordance with accepted academic practice. No use, distribution or reproduction is permitted which does not comply with these terms.
*Correspondence: Zhihua Tang, c3h0emhAMTYzLmNvbQ==