- 1Edinburgh Medical School, The University of Edinburgh, Edinburgh, United Kingdom
- 2University/British Heart Foundation Centre for Cardiovascular Science, The University of Edinburgh, Queens Medical Research Institute, Edinburgh, United Kingdom
- 3Edinburgh Imaging, The University of Edinburgh, Queens Medical Research Institute, Edinburgh, United Kingdom
Recent discoveries demonstrated the skeleton’s role as an endocrine organ regulating whole-body glucose homeostasis. Glucose metabolism is critical for rapid cell proliferation and tumour growth through increasing glucose uptake and fermentation of glucose to lactate despite being in an aerobic environment. This hypothesis paper discusses emerging evidence on how bones can regulate whole-body glucose homeostasis with potential to impact on tumour growth and proliferation. Moreover, it proposes a clinical link between bone glucose metabolism and prognosis of cancer based on recent clinical trial data. Targeting metabolic pathways related with classic glucose metabolism and also bone metabolism, novel methods of cancer therapy and treatment could be developed. This paper objective is to highlight the need for future research on this altered metabolism with potential to change future management of cancer patients.
Introduction
Historically it was believed that human diseases were compartmentalised to different organs. However, the human body is a multi-dimensional network of interconnected organs and diseases that materialise at many levels of complexity frequently including multiple systems (1, 2). This review aims to propose and discuss a potential link between the skeletal system and tumour growth and proliferation through glucose affecting both cancer and whole-body metabolism.
Bones have classically been considered as rigid structures, providing support and protection to organs (3). The skeleton undergoes continuous lifelong remodelling to sustain its changing mechanical needs and repair micro-damages (4). This is achieved by specialised cells, osteoblasts, osteoclasts and osteocytes which perform specific roles. Recent discoveries in bone biology have identified the skeleton not only as a structural supportive network but also as an endocrine organ itself. These advances reveal that bones act as key regulators of whole-body glucose metabolism and elucidate the many novel pathways and mechanisms by which bones achieve this (5). Given these recent results showing that bone-derived hormones are involved in regulation of whole-body glucose homeostasis, it could be hypothesised that bone health could impact systemic glucose homeostasis potentially accelerating development and progression of systemic disease such as cancer.
Cancer is one of the leading causes of death worldwide (6). In the US alone, 1,958, 310 cases of cancer were diagnosed in 2023 (7) The American Cancer society estimates that 400,000 new cases of malignant bone metastasis are diagnosed in the United States each year (8). Therefore, its gravity cannot be overlooked. Cancer is a process that starts locally but can develop to have systemic effects and it is common for bones to be sites of metastases. The cause for cancer related mortality to be high is due to metastases which occur due to dissemination of tumour cells from the primary site via circulation or lymphatic system (9). It is known that only a small fraction of disseminating tumour cells cause cancer metastases however if the primary tumour spreads to bone, osteolytic or osteoblastic metastases can occur resulting in a vicious cycle of bone destruction and tumour growth (10, 11). Osteolytic bone metastases are more common in breast, lung, renal, thyroid cancers and in multiple myeloma. Osteoblastic bone metastases are most prevalent in prostate cancers but also exist in breast, colon and cervical cancers (12). Bone is the most common site of secondary metastases, following lung and liver. Metastatic cells from primary tumours travel through the lymphatic system to bone, where they continue to grow the tumour. Bone metastases can occur in almost all tumours, but breast and prostate cancer are the most common to develop in skeletal metastases (13). The relative incidence of bone metastasis in patients with advanced metastatic disease is as follows; 65-75% in breast cancer; 65-75% in prostate; 60% in thyroid; 30-40% in lung; 40% in bladder; 20-25% in renal cell carcinoma (14).
Bone homeostasis is controlled by a tightly regulated balance between bone deposition and bone resorption. However cancers that metastasise to bone or cancer treatments such as chemoradiation can severely disrupt bone homeostasis (15). In bone metastasis, cancer cells stimulate osteoclasts, which are responsible for bone resorption, leading to excessive bone degradation (Figure 1). At the same time, osteoblast activity, which is essential for depositing new bone, is suppressed or insufficient, resulting in a net loss of bone mass and structural integrity (16). This imbalance between bone resorption and formation can lead to skeletal complications. When bones are metastasised by cancer cells such those from breast cancer, osteoblasts increase their production of inflammatory cytokines including interleukin-6 (IL-6), monocyte chemoattractant protein-1 (MCP-1), regulated on activation normal T cell expressed and secreted (RANTES), and macrophage inflammatory protein-1 alpha (MIP-1 alpha); factors that stimulate osteoclastogenesis including granulocyte-colony stimulating factor (G-CSF), RANK-L, and granulocyte macrophage-colony stimulating factor (GM-CSF); and cytokines that recruit both innate and adaptive immune cells including interleukin-8 (IL-8), interleukin-12 (IL-12), and interferon gamma-induced protein 10 (IP-10) (17, 18).
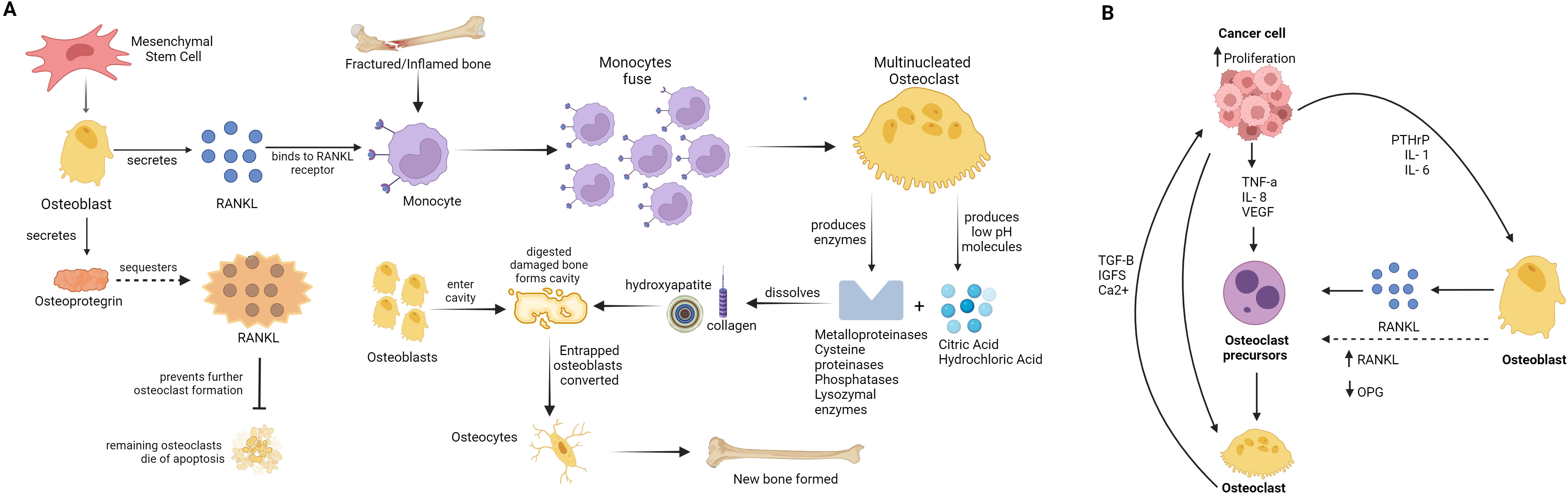
Figure 1. Scheme of bone remodelling and cancer proliferation and metastisation. (A) Bone remodelling cycle: The diagram shows the normal process of bone remodelling, where osteoclasts and osteoblasts coordinate to maintain bone health. Osteoblasts originate from mesenchymal stem cell (MSC) producing RANKL, RANKL binds to RANKL-receptor on monocytes in fractured or injured/inflamed bone. Monocytes fuse to form multi-nucleated osteoclast, osteoclast produces enzymes like metalloproteinases (MMPs), cysteine proteinases (including cathepsin K), phosphatases, lysozymal enzymes, and low pH molecules including citric acid and hydrochloric acid, that digest damaged bone area (dissolving collagen and hydroxyapatite) forming cavity. Osteoblast produced osteoprotegrin, which sequesters RANKL and prevents further osteoclast formation, while previously formed osteoclast die. Osteoblast enter cavity formed by osteoclast activity and form new bone. Entraped osteoblast in new bone-mass, converts to osteocyte. (B) The development of osteoclastic and osteoblastic bone metastases: The formation of osteoclastic and osteoblastic bone metastases involves interactions between tumour cells and bone cells in the bone microenvironment. Tumour cells release factors that enhance the activity of osteoclasts (osteoclastogenesis) and osteoblasts (osteoblastogenesis). As osteoclasts mature, they release factors such as Insulin-like growth factor 1 (IGF-1) and transforming growth factor beta (TGF-β), which help tumour cells survive and multiply, further promoting the cycle of bone resorption and formation. This interaction fosters a conducive environment for tumour growth within the bone.
Unfortunately, bone metastases can often cause limb dysfunction, pathological fractures, spinal cord compression and severe pain, and is associated with significant morbidity, reduction in quality of life and poor prognoses (19).
Glucose and cancer initiation, progression and treatment
The metabolism of glucose allows for energy to be utilised in the form of ATP through oxidation of its carbon bonds. This process is essential for life. The end products of this reaction in cases of full oxidation of glucose through respiration in the mitochondria are CO2, or lactate in hypoxic conditions (20). In 1924, Warburg described a phenomenon in which cancer cells opt for glycolysis as their primary energy source even in aerobic conditions (21). Evidence confirms that tumour and proliferative cells metabolise up to ten-fold more lactate when compared to normal cells under aerobic conditions. This process is known as the Warburg effect (22).
Sugar cannot diffuse across the phospholipid bilayer, hence glucose can be considered as the rate-limiting step for tumour progression (23) (Figure 2). It is important to study these metabolic pathways since cell signalling pathways are important in cancer cell growth and proliferation. Regulation of these pathways allows the amalgamation of nutrients essential for tumour cells into biomass (24). Moreover, it has been identified that activation of certain oncogenes including c-myc, ras and src, as well as transcription factors such as hypoxia inducible factor-1a can result in overexpression and activity of glycolytic enzymes and GLUTs (25). Moreover, these oncogenes are known to regulate metabolic phenotypes of tumours and play a significant role in how the tricarboxylic acid cycle (TCA cycle) is utilised in these cancer cells (26).
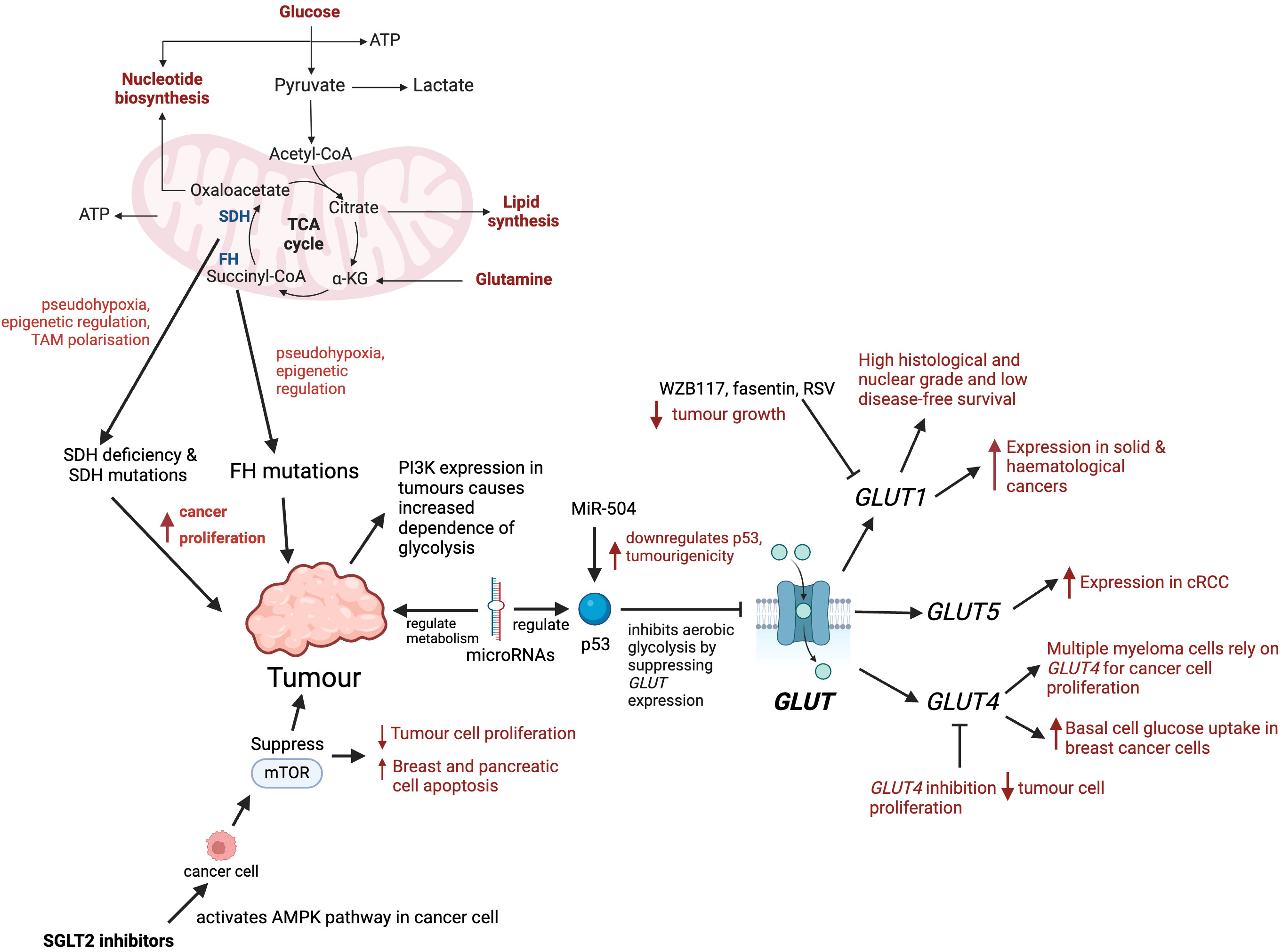
Figure 2. Role of glucose metabolism and transporters in tumour progression. Mutations in key enzymes such as SDH and FH disrupt the TCA cycle causing pseudohypoxia, epigenetic changes, and tumour-associated macrophage (TAM) polarisation, which promote tumour proliferation. PI3K expression in tumours increases their dependence on glycolysis. microRNAs regulate glucose metabolism by targeting enzymes, transcription factors, oncogenes or tumour suppressors. microRNA-504 downregulates p53, enhancing tumour growth. microRNAs regulate p53, and p53 inhibits aerobic glycolysis by supressing GLUT expression. GLUT1 is expressed in solid and haematological cancers, and its overexpression is associated with high histological grade and poor survival. Inhibitors of GLUT1, such as fasentin, WZB117 and RSV have been shown to reduce tumour progression. Multiple myeloma cells rely on GLUT4 for glucose consumption allowing greater cancer cell proliferation. GLUT4 has been identified to have an important role in basal glucose uptake in breast cancer cells. GLUT4 inhibition reduces tumour cell proliferation and affects cell survival under hypoxic conditions. cRCC’s have high glycolytic rates and shown to express GLUT5. SGLT2 inhibitors initiate AMPK pathway in cancer cells which results in the inhibition of mTOR, and in turn causing apoptosis of cancer cells.
The TCA cycle is a fundamental metabolic pathway that allows cells to utilise glucose, amino and fatty acids (27). Despite the evidence that cancer cells do not take part in the TCA cycle, upcoming research highlights that various cancer cells, in particular those with deregulated oncogene and tumour gene suppression actually heavily rely on TCA as an energy source (28). Moreover, in many types of cancer there are mutations that affect the integrity of this cycle, in turn the dysregulation in the production of the TCA cycle metabolites (29). The biochemical reactions in the TCA cycle are catalysed by multiple enzymes and recent research demonstrates these enzymes can undergo mutations or can be dysregulated in a spectrum of cancer types. Succinate dehydrogenase (SDH) has roles in the TCA cycle and mutations in this enzyme have been identified in gastrointestinal stromal tumours, renal tumours, thyroid tumours and many more implicating the significance of SDH in a variety of cancers (30). Furthermore, SDH deficiency has been shown to promote cancer proliferation and metastasis by inducing pseudohypoxia, epigenetic regulation, and tumour assisted macrophages (TAM) polarisation (31). Similarly, heterogeneous mutations of fumarate hydratase (FH) in the TCA cycle, predispose individuals to multiple cutaneous and uterine leiomyomas, hereditary leiomyomatosis and renal cell cancer (32). Furthermore, mutations in FH have been identified in bladder, breast and testicular cancer (33). A study has shown that FH promotes cancer proliferation and metastasis by inducing pseudohypoxia, epigenetic regulation of gene expression (34). These enzymes are an integral part of the TCA cycle and it could be hypothesised that they have the potential to affect cancer progression. A further understanding of TCA metabolites roles and molecular mechanisms in oncogenesis is required which could prompt novel metabolite-based cancer therapy.
The cellular transport of sugars requires transport proteins to mediate uptake or release from cells. Currently there are three families of glucose transporters that have been identified, including sodium-driven glucose symporters (SGLTs), glucose transporters (GLUTs) and more recently SWEETs, which have not yet been explored in mammals, but in plants they are mainly responsible for efflux and intracellular trafficking of sugars (35). These glucose transporter families have distinct mechanisms and physiological functions. SGLT carriers are important for glucose absorption in the small intestine and glucose reabsorption in the renal cortex. They facilitate the translocation of glucose against its own concentration gradient using the energy released from the downhill flow of Na+ (36). With regards to the GLUT family there are 14 members that have been identified in humans which can be subdivided into three classes. GLUTs catalyse the diffusion of glucose along its own concentration gradient (37).
GLUT1 is expressed in erythrocytes, placenta and endothelial cells and regulates entry across the blood brain barrier (38). In comparison with other GLUTs, GLUT1 has a high selectivity for glucose thus playing an imperative role in those tissues that rely on glucose for their energy source (39). GLUT1 expression is an important hallmark for many cancers and studies demonstrate that GLUT1 has been overexpressed in both solid and haematological malignancies (Figure 2). Some of these cancer types where GLUT1 has been found to be overexpressed include breast cancer, non-small cell lung cancer, large B-cell lymphoma, head and neck cancers and glioblastomas (40). Moreover, another study reinforced this by comparing the length time of disease-free survival in patients with GLUT1 expression in breast cancer cells and those without, and it was much shorter in patients with GLUT1 expression compared to those without. GLUT1 expression was associated with pathological poor prognostic factors such as high histological and nuclear grade and low disease-free survival. This suggests that GLUT1 could be used as a prognostic marker in breast cancer patients and could also potentially be used as a target in personalised treatment approaches (41).
Additionally, in breast cancer, non-small cell lung cancer, large B-cell lymphoma, head and neck cancers and glioblastomas cancer types there is data that supports the concept that GLUT1 overexpression correlates with the grade and stage of tumours and the patients clinical outcome (42). Studies have shown that clear renal cell carcinomas (cRCC) have high glycolytic rates and express GLUT transporters (43). The Lidgren et al. study had 80 samples of cRCCs and through creating an isoform of GLUT5, many cancer pathological parameters such as grade of differentiation, pelvis invasion and breaking capsule were shown to have a relationship with GLUT5. The expression of GLUT5 correlated more with cRCC in comparison to renal cell carcinoma (44). This data further suggests that the isoform of GLUT5 in fructose uptake in cRCC could potentially have a therapeutic benefit to hinder the progression to malignant renal cell carcinoma (45) (Figure 2).
GLUT 4 is primarily found in adipose tissues and striated muscle (skeletal and cardiac) (46). Studies have shown that multiple myeloma cells rely on GLUT4 for glucose consumption allowing greater cancer cell proliferation, viability and survival (47). Moreover, GLUT4 has been identified to have an important role in basal glucose uptake in MCF7 and MDA-MB-231 breast cancer cells (48). When GLUT4 is downregulated, there is a diminished glucose uptake and forced metabolic reprogramming into oxidative phosphorylation. This reallocation causes an increase in activity of aerobic respiration, reducing the production of lactate. As a result GLUT4 inhibition reduces tumour cell proliferation and affects cell survival under hypoxic conditions (49). Additionally, the majority of gastrointestinal stromal tumours have a gain of function mutation called c-KIT. It has been shown that gastrointestinal stromal tumour cells after treatment with imatinib, have reduced the expression of GLUT4 in the plasma membrane (50). This is because imatinib, which reduces glucose uptake via decreased levels of plasma membrane-bound GLUT4, induces apoptosis or growth arrest by inhibiting c-KIT activity (50).
Interestingly, several inhibitors of glucose transporters, such as fasentin, phloretin, STF-31, and WZB117 have already been discovered, and experiments with preclinical models demonstrated their impact in reducing tumour progression (51–53). WZB117 is a small molecule which inhibits GLUT1 and it acts on cancer cells in vitro and in vivo (54). Inhibition of GLUT1 by WZB117 decreases the levels of intracellular ATP and glycolytic enzymes. Animal studies performed on mice revealed that WZB117 inhibits cancer growth. After daily intraperitoneal injection of this inhibitor, the sizes of the compound-treated tumours were on average more than 70% smaller in comparison with control animals (55). Moreover, fasentin, an inhibitor of GLUT1, which binds directly to GLUT1 and inhibits glucose uptake, increases apoptosis in prostate cancer, multiple myeloma cells, and acute promyelocytic leukemia cells. It sensitises these cancer cells to FAS ligand-death receptor signalling (51). Moreover, Resveratol (RSV), is a polyphenolic natural product that attracted great interest mainly due to its anticarcinogenic, anti-inflammatory, and cardioprotective properties (56). RSV has structural similarities with tyrosine kinases that are known inhibitors of GLUT1 (57). In cancer cells it is observed that resveratrol inhibited the uptake of glucose, favouring the anticancer action. The type of cell death observed in ovarian cancer cells treated with RSV has been reported as apoptosis or autophagy (58). Thus these inhibitors of GLUT transporters are prototypes for further development of anticancer therapeutics targeting GLUT mediated glucose transport and glucose metabolism (Figure 2).
SGLT-2 inhibitors are a new class of antidiabetic drugs which act by decreasing the active reverse transport of glucose by SGLT-2 in renal tubule. SGLT-2 inhibitors reduce glucose absorption, induce glycosuria which lowers plasma glucose independent of insulin. Preclinical and in vitro cell studies have confirmed that SGLT-2 inhibitors have anti-proliferative effects of certain cancers including liver, pancreatic, bowel, lung and breast cancers (59). One proposed mechanism of action of SGLT-2 inhibitors is the inhibitory effect of the production of ATP, which causes the activation of the AMPK pathway in cancer cells. In turn, this SGLT-2 inhibitor induced AMPK activation results in inhibition of mTOR (60). mTOR signalling is important in cell growth and metabolism and is dysregulated in cancer pathophysiology (61). This inhibition of mTOR results in initiation of apoptosis (62).
The metabolism, proliferation, growth and survival of cancer cells is also largely dependent on signalling through the serine/threonine-protein kinase (AKT) and phosphoinositide-3-kinase (PI3K) pathways (63). The cells in the AKT pathway are those which are most frequently activated and proliferate and survive in the cancer pathway. Agents that target PI3K are being researched in clinical trials and there is upcoming evidence that expression of PI3K causes an increased dependence of glycolysis in turn implying that drugs targeting the PI3K pathway would interfere with glucose metabolism (24).
Finally, there has been evidence to suggest that microRNAs regulate cancer metabolism that evince a higher rate of metabolism of glucose. MicroRNAs are a family of non-coding RNAs that are not translated into proteins but control gene expression either at pre- or post-transcriptional stages. Moreover, they have been shown to be involved in multiple biological processes, one of which is glucose metabolism, mediated by targeting enzymes, transcription factors, oncogenes or tumour suppressors (64). P53 is a tumour suppressor gene, meaning that it functions to inhibit the growth of tumours, cell cycle progression, apoptosis and DNA damage response (65). A number of microRNAs regulate p53. The mechanism of p53 is that it inhibits aerobic glycolysis by suppressing expression of some GLUTs and other molecules. As a result, the dysregulation of p53 that occurs in many malignancies leads to pentose phosphate pathway or aerobic glycolysis (66). MiR-504 has been shown to downregulate p53 and increases tumourigenicity (67).
To sum up, previous studies have confirmed that glucose does play a critical role in the cancer metabolic pathways through various mechanisms described above. A better understanding of how cancer cells rely on systemic glucose metabolism for initiation and progression will hopefully lead to novel targeting modalities for cancer treatment. As discussed, there is new research into how bones regulate whole-body glucose metabolism therefore there could be a potential link between the skeletal system affecting cancers. This will be discussed in further detail in the next section of this hypothesis paper.
Role of bones in regulating systemic glucose metabolism: key mechanisms and mediators
The human adult skeleton consists of 213 bones, not including the sesamoid bones. Bones vary in size, shape and strength to respond to the demands of the motor tasks.
Bones are made up of osteoid matrix and hydroxyapatite [Ca10(PO4)6(OH)2] crystal but they also contain water, non-collagenous and collagenous proteins, lipids and specialised bone cells (68). Bone mineral, in the form of hydroxyapatite crystals, is an essential store of calcium and phosphate crucial for mineral homeostasis and provides the skeleton with mechanical rigidity and compressive strength.
The musculoskeletal system constantly undergoes remodelling to replace old and damaged bone to maintain bone integrity and mineral homeostasis (69). The bone remodelling cycle consists of five key co-ordinated steps; activation, resorption, reversal, formation and termination which takes approximately 120 to 200 days in cortical and trabecular bone, respectively (70). Osteoclasts are cells that break down and resorb bone, and osteoblasts are cells that form new bone tissue (71). During the bone remodelling cycle, osteoclastic resorption is tightly coupled with osteoblastic bone formation (72). The remodelling cycle has two key pathways receptor activator of NF-kB (RANK)/receptor activator of NF-kB ligand (RANKL)/osteoprotegerin (OPG) and wingless-related integration site (Wnt) that transduce systemically and locally produced signals (73). These pathways are important in determining the timing and balance of bone resorption and formation to ensure its tight regulation (74). Bone remodelling also plays an important role in maintaining plasma calcium homeostasis (75). The regulation of bone remodelling is both systemic and local. The major systemic regulators include parathyroid hormone (PTH), calcitriol, growth hormone, glucocorticoids, thyroid hormones, and sex hormones. Others such as insulin-like growth factors (IGFs), prostaglandins, tumour growth factor-beta (TGF-beta), bone morphogenetic proteins (BMP), and cytokines are also crucial (76). This cycle is a life-long process and is imperative for preserving bone integrity and strength.
Osteoblasts are known to release the hormone osteocalcin, promoting whole-body glucose homeostasis (Figure 3). Moreover, uncarboxylated osteocalcin has less affinity for hydroxyapatite, readily promoting its release into circulation, and it is responsible for multiple endocrine functions (77). A pre-clinical study demonstrated that mice lacking in osteocalcin were obese, hyperglycaemic, hypoinsulinemic with reduced insulin secretion and sensitivity. They had reduced islet size and beta cell mass, suggesting a link between bone and regulation of energy metabolism (78). Conversely, one study using osteocalcin deficient mice showed no impact on glucose metabolism and muscle mass in mice (79). Notwithstanding, osteoblasts express the Esp gene, coding for protein tyrosine phosphatase (PTP), which controls glucose metabolism by Esp negatively regulating osteocalcin by producing its inactive form. Pancreas-specific PTP depleted mice demonstrate impaired glucose tolerance and glucose-stimulated insulin secretion when tested with high fat feeding (Table 1). Mice lacking PTP in osteoblasts demonstrated an increase in bone resorption and insulin sensitivity (80). Pre-clinical studies highlighted that mice without Esp had increased uncarboxylated osteocalcin resulting in increased osteoblast signalling and whole-body glucose metabolism (81, 82). However this is contradicted by a study (83) suggesting that mice without Esp, appeared hypoglycaemic and severely sensitive to insulin. Interestingly, osteoblasts express copious amounts of insulin receptor and respond to insulin by upregulating the expression of anabolic bone markers, increasing glucose uptake along with several other functions (81). There has been evidence to show that localised insulin quickens bone fracture healing and formation in vivo in comparison to patients with Type 1 diabetes whom are associated with low bone mass and early onset of bone diseases (84).
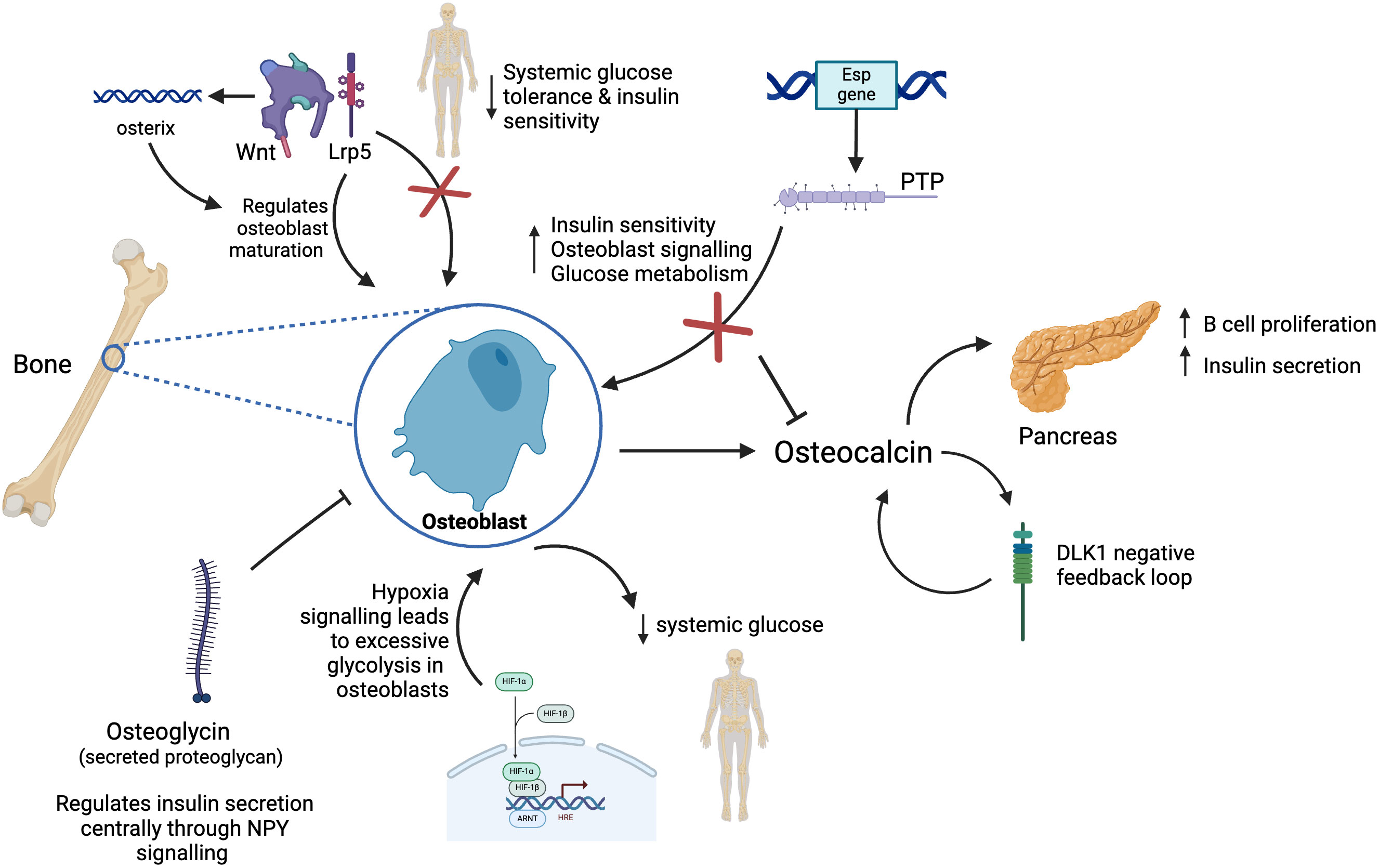
Figure 3. Model of new endocrine functions of bone. Osteoblasts in bone release the hormone osteocalcin which is responsible for multiple endocrine functions including acting on the pancreas to increase insulin secretion and beta cell proliferation. Osteocalcin controls the production of DKL1 which works as a negative feedback system preventing osteocalcin induced hypoglycaemia. Osteoblasts express Esp gene which codes for PTP, in turn this controls glucose metabolism by Esp negatively regulating osteocalcin resulting in reduced bone resorption and insulin secretion. Osteoglycin inhibits osteoblast activity and regulates glucose homeostasis through NPY. Wnt signalling through Lrp5 signalling regulates osteoblast maturation and whole-body glucose metabolism. Activation of Wnt pathway stimulates production of osterix, a transcription factor further promoting osteoblast differentiation. Hypoxia signalling through HIF-1 leads to excessive glycolysis in osteoblasts, thus reducing systemic levels of glucose.
When mammalian cells are exposed to hypoxia in tissue culture, they increase glycolysis, a response thought to be cell-intrinsic. This is driven by the post-translational stabilisation of the hypoxia-inducible factor HIF-1 (85, 86). Under normal oxygen conditions, HIF-1 is continuously produced, but its levels are regulated post-translationally. Prolyl hydroxylase enzymes hydroxylate specific proline residues on the HIF-1α subunit, leading to recognition by the von Hippel-Lindau tumour suppressor (pVHL) and subsequent ubiquitination, targeting HIF-1α for degradation (87, 88). A study on the role of bones in glucose metabolism found that excessive glycolysis in osteoblast lineage cells that is induced by hypoxia signalling increases the use of glucose within the skeleton, thus reducing systemic levels of glucose (89). A pre-clinical study in mice showed that mice with the induced deletion of the hypoxia signalling pathway component von Hippel-Lindau (VHL) in skeletal osteolineage cells led to high bone mass of mice but also hypoglycaemia and increased glucose tolerance not accounted for by osteocalcin or insulin. In vitro and in vivo data indicated that VHL deficient osteoblasts had increased glucose uptake and glycolysis associated with hypoxia inducible factor (HIF) - target gene expression, resembling the Warburg effect that affects cancer cells (90). The improved metabolism in VHL mutant mice (90) is associated with increased tumour growth and metastasis (91). HIF signalling activation also increases bone mass likely through multiple key downstream effecters including vascular endothelial growth factor (VEGF) that mediates coupled osteo-angiogenic responses in bone. Local HIF activation in bone cells triggers increased glycolysis, which is associated with strongly enhanced osteoblastic glucose consumption (92). Studies with human patients with bone metastases from adenocarcinoma lung cancer (stage IV) showed that the level of glucose uptake in metastatic lesions inversely correlated with blood glucose levels and glycated haemoglobin values (HBa1C) (90). Overall, literature published supports a link between osteoblast-secreted osteocalcin and systemic glucose regulation (Figure 3).
Wnt pathways are a group of signal transduction pathways that send signals from proteins through cell surface receptors. The processes that it controls include body axis patterning, cell fate specification, cell proliferation and cell migration in bone, heart and muscle (93). Moreover, Wnt impacts bone homeostasis through β-catenin dependent and independent pathways. Activation of Wnt/β-catenin stimulates expression of transcription factor, osterix, promoting osteoblast differentiation (94). A study on glycogen synthase kinase 3β, a negative regulator of Wnt, showed that its deletion decreased circulating glucose secondary to increased insulin sensitivity. The blood glucose levels of these mutant mice had switched from low to high suggesting insulin resistance which is what occurs in feature Type 2 diabetes (95). This was supported by a study conducted by Yao et al. who showed that whole-body metabolism is regulated by osteocalcin and, specifically, through Wnt/β-catenin signalling pathway in osteoblasts (96). Moreover, another study by Kim et al. concluded that Wnt signalling through low-density lipoprotein-related receptor 5 (Lrp5) coreceptor regulates osteoblast maturation and whole-body glucose metabolism. Mice lacking Lrp5 in osteoblasts had hyperglycaemia, reduced glucose tolerance and insulin sensitivity reinforcing a link between the Wnt signalling pathway and osteoblast insulin signalling suggesting it could influence whole-body glucose homeostasis (97). A pre-clinical study also highlighted the role of delta like-1 (DLK1) in bone, showing that uncarboxylated osteocalcin controls production of DLK1 which works as a negative feedback system preventing osteocalcin induced hypoglycaemia (98). These studies provide further evidence of the role of bone cells in regulating whole-body glucose levels.
Osteoglycin, coded by the OGN gene and a secreted proteoglycan is expressed in a variety of organs and has an impact on bone formation, tumourigenesis amongst many other roles (99). Osteoglycin inhibits osteoblast activity whilst feeding back to increase whole-body homeostasis acting centrally via neuropeptide Y (NPY) neurons. Periods of low osteoglycin, seen in obesity, reduce food intake and insulin sensitivity, thus increasing serum glucose availability. A study showed that NPY signalling directly through peripheral Y1 receptors within osteoblasts controls insulin secretion and regulates glucose homeostasis (100). Another study shows that this central NPY signalling initiated process leads to subsequent osteoblastic Y1 signalling increasing osteoglycin secretion when energy balance is low (101). This study also uses an osteoglycin knockout mice model showed that osteoglycin acts to supress bone formation by supressing osteoblastic activity. It also feeds back to help increase whole body energy balance by acting centrally on hypothalamic NPY-ergic neurons to increase food intake as well as by improving glucose uptake through modulation of insulin secretion and insulin action at target tissue such as muscle. Conversely, the low levels of circulating osteoglycin seen during obesity act dually to both reduce food intake and reduce insulin responsiveness, glucose uptake, and, as a consequence, increase blood glucose. The shift in blood glucose in turn increases the energy available to bone formation through increased glucose uptake into early osteoblasts, further enabling the skeleton to adapt to increases in body weight. The study also showed that in humans post gastric surgery, as a model of negative energy balance, osteoglycin is associated with lower body mass index (BMI) and lean mass as well as changes in weight and glucose levels. This highlights the importance of Y1 receptor and osteoglycin in bone in the regulation of glucose homeostasis. Moreover, it identifies a role for osteoglycin in facilitating the matching of bone growth to alterations in energy status. This is further shown in a commentary of a study on osteoglycin, linking bone and energy homeostasis (102). In the study, researchers induced obesity in mice with a high fat diet. The authors reported that obese mice had decreased circulating levels of osteoglcyin compared with control mice fed normal chow. This effect was negatively associated with bone mass, which suggests that osteoglycin promotes alterations in bone mass following changes in body weight during intervals of high-fat feeding. Moreover, the role of osteoglycin was further demonstrated in 22 patients who had underwent weight loss through dietary programme or gastric surgery (102). Before weight loss, there was a negative correlation between osteoglycin levels and BMI, and a positive correlation between osteoglycin levels and lean mass. After the intervention, patients who had undergone substantial weight loss had a considerable increase in levels of osteoglycin, which correlated with reduced blood levels of glucose. Therefore, osteoglycin in humans is linked with regulating glucose metabolism during changes in energy homeostasis, which supports the findings in mice (102).
Overall, the mechanistic and clinical studies outlined above had clear outcomes confirming the role of bones in regulating whole-body glucose metabolism through a variety of pathways. These studies are beneficial since they untangle complex disease mechanisms and propose new avenues for research into understanding the initiation and progression of human systemic disease, and emerging therapeutic avenues that consider the skeletal system as an important player in the regulation of systemic glucose metabolism.
Known and emerging links between cancer, systemic glucose, and bones as endocrine tissues
Malignant cells support tumour growth by adapting to metabolic changes. The link between whole-body glucose metabolism and tumourigenesis has attracted great interest from researchers and clinicians. The following studies discuss the emerging research on the links between cancer, systemic glucose and bones as endocrine tissues.
Previously published studies showed that osteoprogenitor cells (OPCs) are found in hypoxic environments in the bone marrow and that activation of HIF signalling in these cells increases bone mass, but also favours breast cancer metastasis to local bones (91). HIF signalling in osteoblast lineage cells also promotes breast cancer growth and dissemination remotely, in lungs and in other distant tissues from bones (91). These results indicate that that loss of bone homeostasis through alterations of the bone anabolism could affect breast cancer progression and present the skeleton as an important organ of the tumour macroenvironment. They also suggest that targeting the bone microenvironment could limit systemic tumour growth and dissemination in breast cancer.
Osteoglycin, as previously mentioned, is a protein that takes part in the development of the bone extracellular matrix and influences many processes including tumourigenesis (112). Several studies have shown that osteoglycin plays an important role in creating the cancer microenvironment and that it is in fact regulated by the p53, a tumour suppressor gene (113). Moreover, its role in controlling tumour cell proliferation by either increasing or inhibiting cancer growth exhibiting both protumourigenic and antitumourigenic properties have been identified in different cancers, including colorectal, breast and ovarian cancers (114, 115). Particularly in colorectal cancer, osteoglycin has been involved in enhancing the T lymphocyte infiltration (116). These data indicates that osteoglycin could be involved in regulating the tumour microenvironment and may be a useful therapeutic target in oncology. However, there is limited evidence on osteogylcin’s role in other tumours and in glucose homeostasis, further research on how bone-mediated systemic glucose homeostasis can impact cancer development and progression is needed (116). Notwithstanding, an in vivo study conducted in mice highlighted that short-term starvation, therefore glucose restriction, enhances chemotherapy drugs efficacy, promotes tumour deceleration and increases cancer-free survival in melanoma, glioma and breast cancer. This was due to limited toxicity in normal cells, reinforcing the utility of targeting glucose pathways for cancer therapies (117). To this regard, modulating systemic glucose via sodium-glucose co-transporter 2 (SGLT-2) inhibitor drugs, which target the mammalian target of rapamycin (mTOR) signalling pathway, reduced tumour cell proliferation and induction of breast cell apoptosis (118). In addition, Canagliflozin (an SGLT-2 inhibitor), also showed antiproliferative effects and induction of apoptosis through the mTOR signalling pathway in pancreatic cancers (119) (Figure 4).
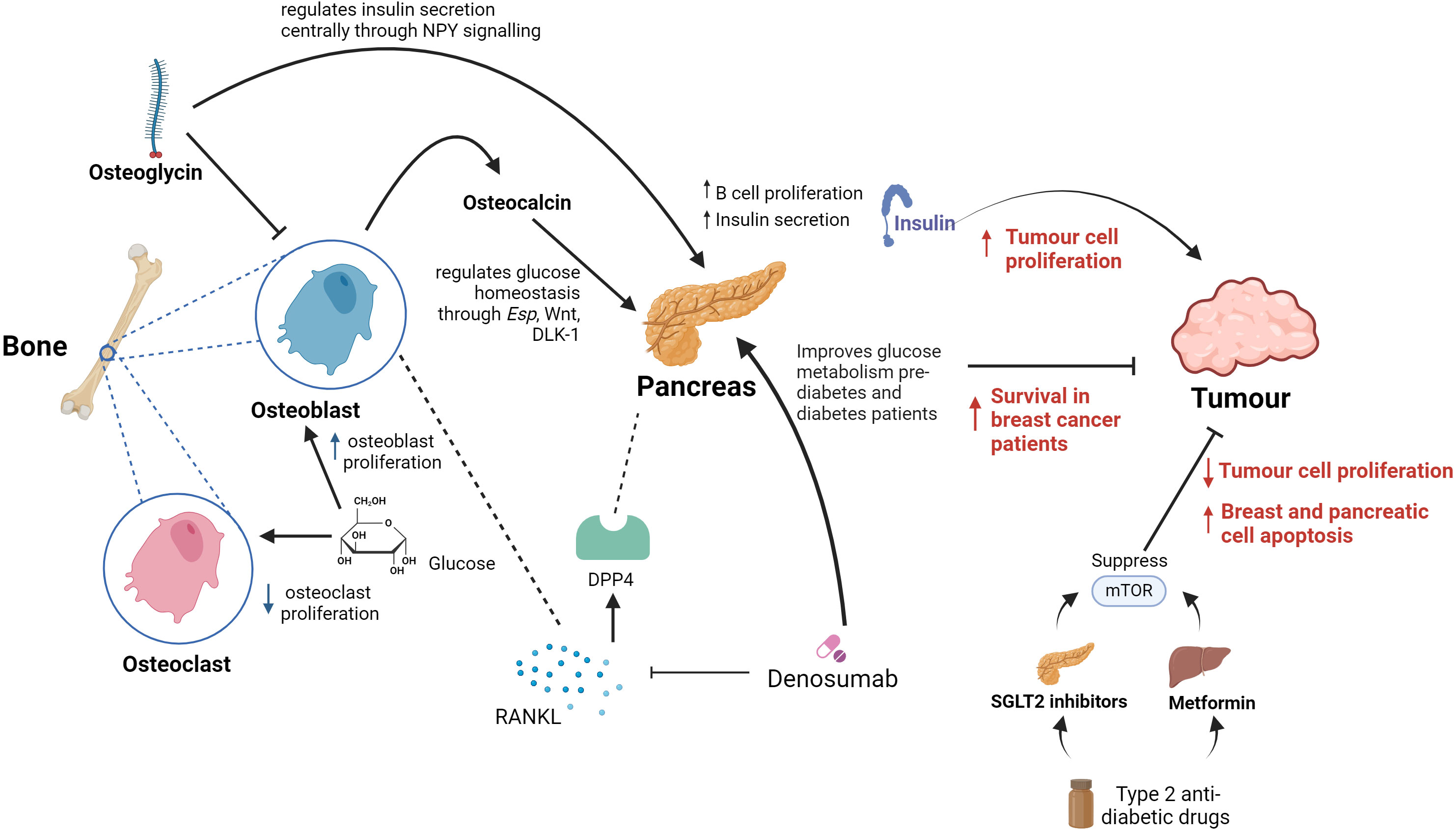
Figure 4. Summary of proposed key links between bones, systemic glucose and cancer. In bone, osteoclasts and osetoclasts are affected by glucose metabolism. Glucose causes reduced osteoclast proliferation and increased osteoblast proliferation. Osteoblasts in bone release the hormone osteocalcin, which is responsible for multiple endocrine functions including acting on the pancreas to increase insulin secretion and beta cell proliferation. Osteoglycin inhibits osteoblast activity and regulates glucose homeostasis through NPY. Denosumab inhibits the RANKL receptor which is found in osteoclasts in bone and leads to increased survival in breast cancer patients. It also improves glucose metabolism in pre-diabetes and diabetes patients. DPP4 is a well-known enzyme involved in glucose homeostasis, including via pancreatic action, that is known to be an osteoclast-derived protein. Therefore, DPP4 is an important link between RANKL and systemic glucose metabolism homeostasis. Metformin and SGLT2 inhibitors used in the treatment of diabetes supress mTOR which has shown to reduce tumour cell proliferation and increase breast and pancreatic cell apoptosis.
Classic drugs that target critical metabolic control points for aerobic glycolysis may therefore act as an opportunity in treating cancer (Figure 4). This is due to the fact that high levels of insulin in the bloodstream increase mitosis and tumour cell growth and proliferation (120). There is a volume of research suggesting that the drugs that are used to treat metabolic diseases such as Type 2 diabetes could be utilised for cancer therapy. A retrospective clinical study has found that metformin, a widespread anti-diabetic drug, may offer an element of cancer prevention as well as better outcomes when used in combination with other cancer therapies (121). Potential anticancer properties of metformin are thought to be based on two different mechanisms. First, insulin is a growth factor and exerts mitogenic properties mediated through insulin-like growth factor receptors and insulin receptors. Metformin decreases insulin resistance and lowers circulating insulin levels, leading to decreased signalling on insulin-like growth factor receptors and insulin receptors (122). Metformin functions by targeting the enzyme activated protein kinase (AMPK) which encourages muscles to take glucose up from the blood and inhibits transcription of the gene responsible for glycogenesis in liver cells. A recent study has eluded that the upstream regulator of AMPK is a protein kinase known as LKB1 which is a well-known tumour suppressor. Moreover, metformin inhibits mTOR activity through activation of LBK1 and in turn AMPK, ultimately reducing protein synthesis and angiogenesis (123). Furthermore, a separate study has demonstrated that using new antiglycolytic agents such as dharichloroacetate have been tested for monotherapy in solid glycolytic tumours such as hepatocellular carcinoma by inducing cellular apoptosis and decrease cancer cell proliferation, improving survival in animal models (124).
Studies have shown that the long term use of insulin, in particular in individuals with Type 2 diabetes, has an increase in cancer risk. A study has shown that long term use of insulin, is associated with increased risk of ovarian cancer (122). Moreover another study suggests that insulin therapy, especially with certain analogs like insulin glargine, is linked to an increased risk of breast cancer. Previous studies have suggested that breast epithelial cells exposed to insulin are at risk for transformation in a stepwise carcinogenesis process (125). More specifically, insulin has been shown to activate members of the insulin-like growth factor (IGF) receptor family to inhibit apoptosis and subsequently prolong the survival of these transformed breast tissue cells (125). This mechanism suggests that insulin not only facilitates glucose uptake but may also support a tumour-friendly microenvironment, thereby affecting whole-body glucose metabolism and contributing to cancer progression (126). This interplay between insulin, glucose metabolism, and cancer risk underscores the importance of monitoring insulin therapy and metabolic health in cancer-prone populations. Moreover, future research should focus on elucidating the mechanisms by which insulin and glucose metabolism influence cancer development and exploring the efficacy of metabolic-targeting therapies in reducing cancer risk and improving patient outcomes.
Previous data show that once tumour cells disseminate to the bone marrow, there is increased chance of relapse of cancer resulting in particularly a poor prognosis in early stage breast cancer (127). In breast cancer bone metastases, tumour cells secrete cytokines and growth factors causing stroma cells and osteoblasts to secrete RANK-ligand (RANKL). This leads to increased osteoclast differentiation which in turn stimulate tumour cell proliferation and tumour cell survival (128). Another study shows that breast and prostate cancer cells not only express RANKL but also upregulate RANKL expression by osteoblasts and bone marrow stromal cells (129, 130). Numerous experimental models of bone metastasis have shown that RANKL antagonists prevent tumour-associated osteolysis and significantly reduced skeletal tumour burden (131). Animal models that mimic advanced prostate, breast, or non-small cell lung cancer, representing both osteolytic and osteoblastic skeletal lesions, have demonstrated that the RANKL inhibitors, RANK-Fc or OPG-Fc,were effective in preventing or delaying of bone metastases and reducing progression of tumours in the skeleton (132). This was observed in a model of carcinogen and hormone-induced breast cancer, which demonstrated that RANKL inhibition with RANK-Fc significantly delayed mammary tumour formation in transgenic mice and almost completely blocked tumour formation in wild-type mice (133). Denosumab is a drug which is a human monoclonal antibody and is used in the treatment of osteoporosis and bone metastases (134). It inhibits RANKL, which is involved in the bone remodelling cycle, and has resulted in disease free survival in patients with breast cancer (135) (Figure 4). Previous studies have also shown that inhibiting RANKL with denosumab improved glucose metabolism parameters in pre-diabetic and diabetic patients (136).
In the RANKL pathway, OPG is an inhibitor of RANKL, which enhances osteoclasts apoptosis (137). High OPG levels are associated with impaired glucose tolerance (IGT) which could be as a result of insulin levels decreasing in patients with IGT and the similar roles between OPG and insulin in blocking osteoclastogenesis (138–140). Based on OPG’s function, Denosumab, could improve glucose entry into the muscle through increasing insulin sensitivity (141). Denosumab was also proven to decrease dipeptidilpeptidase-4 (DPP4) serum concentrations and increase glucagon-like peptide-1 (GLP-1) in subjects with IGT, demonstrating a crucial role in glucose and insulin metabolism (142). DPP4 is a well-known enzyme involved in glucose homeostasis that is known to be an osteoclast-derived protein (117). Therefore, DPP4 is an important link between RANKL and systemic glucose metabolism homeostasis.
However, it must be understood that RANKL participates in other tissues. Studies show that RANK/RANKL system plays an essential role in developmental maturation and functional maintenance of the immune system (143, 144). Studies of mice with heart failure have also shown a high and persistent expression of the RANK, RANKL, and OPG genes in the ischemic and nonischaemic areas of the heart (145). Furthermore, intravenous post-infarction anti-RANKL treatments in mice have shown to reduce infarct size and cardiac neutrophil infiltration (146). In addition, in muscles, high levels of RANKL expression generates poor glucose uptake, leading to alterations in muscle metabolism (141). In the liver RANKL induces insulin resistance by promoting inflammation, whereas in the pancreas RANKL produces a hyperglycaemic state by decreasing insulin production and augments glucagon production which could lead to β-cell dysfunction (141, 142). The main sources of RANKL are macrophages that have infiltrated the bone, pancreas, liver and muscles, which is a signal for the inflammatory NF-κB pathway, linking it to impaired IGT (147–149). Studies have shown that metformin decreased RANKL activity and its expression in the bone and liver, however the molecular mechanisms are not fully understood (150, 151).
Drug clinical trials have shown that systemic glucose homeostasis can be regulated both by using classic glucose metabolism modulation (e.g. anti-diabetic drugs) or by using emerging glucose metabolic modulation by bone signalling (e.g. RANKL). Both have demonstrated impact on cancer progression, patients’ survival and disease outcomes. This adds plausibility to the hypothesis that bone metabolism could influence systemic glucose homeostasis with potential consequences for cancer progression and treatment.
Challenges, conclusions and future perspectives
Glucose metabolism plays a role in cancer development and progression through many different mechanisms. Moreover, bone metabolism affects whole-body glucose homeostasis and in turn could be hypothesised that it can take part in cancer progression and treatment. However, further studies focussing on addressing the hypothesis of how bones impact glucose systemically, and how this changes the disease course in oncology need to be carried out. Understanding these dynamics is critical for developing integrated therapies that target both bone health and metabolic alterations in cancer patients. Furthermore, modulating pathways that influence bone remodelling may provide new avenues for controlling cancer metastasis to bone. For example, drugs that target energy metabolism, like metformin used in patients with diabetes, impact osteoblast or osteoclast activity, and in turn this can affect cancer progression. Moreover, inhibitors and activators of osteoblast differentiation and bone formation for example Wnt inhibitors, Esp and osteocalcin can alter bone formation and in turn, energy metabolism. Additionally, inhibitors of GLUT transporters could also be a target for modulating glucose metabolism, and in turn be used for anticancer therapies. Understanding the additional role of these drugs is important since this knowledge could ultimately be used to develop treatments to slow cancer cell growth and cause tumour death.
The exploitation of potentially reprogrammed glucose metabolism to target cancer cells either by classically targeting systemic glucose metabolism or bone glucose metabolism provides new worthwhile therapeutic targets and avenues. Future treatment of cancer patients should consider a more holistic approach where bone health and systemic glucose metabolism are important factors to be appraised and, when possible, modulated alongside conventional cancer therapy for specific tumour types (e.g. radiotherapy, chemotherapy, and targeted immunotherapy). Advances in understanding interactions between osteoblasts, osteoclasts, and bone metastatic cancer cells will aid in controlling and ultimately preventing cancer cell metastasis to bone.
Data availability statement
The original contributions presented in the study are included in the article/supplementary material. Further inquiries can be directed to the corresponding author.
Author contributions
RR: Writing – original draft. AT: Writing – review & editing.
Funding
The author(s) declare financial support was received for the research, authorship, and/or publication of this article. AT was funded by the BHF (FS/19/34/34354), she is a recipient of a Wellcome Trust Technology Development Award (221295/Z/20/Z) and a Chan Zuckerberg Initiative DAF grant number 2020-225273, an advised fund of Silicon Valley Community Foundation (grant DOI https://doi.org/10.37921/690910twdfoo).
Conflict of interest
The authors declare that the research was conducted in the absence of any commercial or financial relationships that could be construed as a potential conflict of interest.
Publisher’s note
All claims expressed in this article are solely those of the authors and do not necessarily represent those of their affiliated organizations, or those of the publisher, the editors and the reviewers. Any product that may be evaluated in this article, or claim that may be made by its manufacturer, is not guaranteed or endorsed by the publisher.
References
1. Foldager CB, Bendtsen M, Bünger C. PET scanning for evaluation of bone metabolism. Acta orthopaedica. (2009) 80:737–9. doi: 10.3109/17453670903487040
2. Vandamme D, Fitzmaurice W, Kholodenko B, Kolch W. Systems medicine: helping us understand the complexity of disease. QJM: Int J Med. (2013) 106:891–5. doi: 10.1093/qjmed/hct163
3. Guntur AR, Rosen CJ. Bone as an endocrine organ. Endocrine practice: Off J Am Coll Endocrinol Am Assoc Clin Endocrinologists. (2012) 18:758–62. doi: 10.4158/EP12141.RA
4. Hadjidakis DJ, Androulakis II. Bone remodeling. Ann N Y Acad Sci. (2006) 1092:385–96. doi: 10.1196/annals.1365.035
5. Donat A, Knapstein PR, Jiang S, Baranowsky A, Ballhause TM, Frosch KH, et al. Glucose metabolism in osteoblasts in healthy and pathophysiological conditions. Int J Mol Sci. (2021) 22:6–13. doi: 10.3390/ijms22084120
6. Mafra da Costa A, Hernandes ICP, Weiderpass E, Soerjomataram I, Fregnani J. Cancer statistics over time in northwestern são paulo state, Brazil: incidence and mortality. Cancer Epidemiol Biomarkers Prev. (2022) 31:707–14. doi: 10.1158/1055-9965.Epi-21-0842
7. Siegel RL, Miller KD, Wagle NS, Jemal A. Cancer statistics, 2023. CA Cancer J Clin. (2023) 73:17–48. doi: 10.3322/caac.21763
8. Jiang W, Rixiati Y, Zhao B, Li Y, Tang C, Liu J. Incidence, prevalence, and outcomes of systemic Malignancy with bone metastases. J Orthopaedic Surg. (2020) 28:2309499020915989. doi: 10.1177/2309499020915989
9. Vasseur A, Kiavue N, Bidard F-C, Pierga J-Y, Cabel L. Clinical utility of circulating tumor cells: an update. Mol Oncol. (2021) 15:1647–66. doi: 10.1002/1878-0261.12869
10. D'Antonio C, Passaro A, Gori B, Del Signore E, Migliorino MR, Ricciardi S, et al. Bone and brain metastasis in lung cancer: recent advances in therapeutic strategies. Ther Adv Med Oncol. (2014) 6:101–14. doi: 10.1177/1758834014521110
11. Röcken M. Early tumor dissemination, but late metastasis: insights into tumor dormancy. J Clin Invest. (2010) 120:1800–3. doi: 10.1172/jci43424
12. Yin JJ, Pollock CB, Kelly K. Mechanisms of cancer metastasis to the bone. Cell Res. (2005) 15:57–62. doi: 10.1038/sj.cr.7290266
13. Svensson E, Christiansen CF, Ulrichsen SP, Rørth MR, Sørensen HT. Survival after bone metastasis by primary cancer type: a Danish population-based cohort study. BMJ Open. (2017) 7:e016022. doi: 10.1136/bmjopen-2017-016022
14. Selvaggi G, Scagliotti GV. Management of bone metastases in cancer: a review. Crit Rev Oncol Hematol. (2005) 56:366. doi: 10.1016/j.critrevonc.2005.03.011
15. Bussard KM, Gay CV, Mastro AM. The bone microenvironment in metastasis; what is special about bone? Cancer Metastasis Rev. (2008) 27:41–4. doi: 10.1007/s10555-007-9109-4
16. Ottewell PD. The role of osteoblasts in bone metastasis. J Bone Oncol. (2016) 5:124–7. doi: 10.1016/j.jbo.2016.03.007
17. Kinder M, Chislock E, Bussard KM, Shuman L, Mastro AM. Metastatic breast cancer induces an osteoblast inflammatory response. Exp Cell Res. (2008) 314:173–83. doi: 10.1016/j.yexcr.2007.09.021
18. Shupp AB, Kolb AD, Mukhopadhyay D, Bussard KM. Cancer metastases to bone: concepts, mechanisms, and interactions with bone osteoblasts. Cancers (Basel). (2018) 10:4–5. doi: 10.3390/cancers10060182
19. Jehn CF, Diel IJ, Overkamp F, Kurth A, Schaefer R, Miller K, et al. Management of metastatic bone disease algorithms for diagnostics and treatment. Anticancer Res. (2016) 36:2631–7. doi: 10.1016/j.critrevonc.2005.03.011
20. Adekola K, Rosen ST, Shanmugam M. Glucose transporters in cancer metabolism. Curr Opin Oncol. (2012) 24:650–4. doi: 10.1097/CCO.0b013e328356da72
21. Koppenol WH, Bounds PL, Dang CV. Otto Warburg's contributions to current concepts of cancer metabolism. Nat Rev Cancer. (2011) 11:325–37. doi: 10.1038/nrc3038
22. Liberti MV, Locasale JW. The warburg effect: how does it benefit cancer cells? Trends Biochem Sci. (2016) 41:211–8. doi: 10.1016/j.tibs.2015.12.001
23. Chen L-Q, Cheung LS, Feng L, Tanner W, Frommer WB. Transport of sugars. Annu Rev Biochem. (2015) 84:865–94. doi: 10.1146/annurev-biochem-060614-033904
24. Vander Heiden MG, Cantley LC, Thompson CB. Understanding the Warburg effect: the metabolic requirements of cell proliferation. Science. (2009) 324:1029–33. doi: 10.1126/science.1160809
25. Rodríguez-Enríquez S, Marín-Hernández A, Gallardo-Pérez JC, Moreno-Sánchez R. Kinetics of transport and phosphorylation of glucose in cancer cells. J Cell Physiol. (2009) 221:552–9. doi: 10.1002/jcp.21885
26. Anderson NM, Mucka P, Kern JG, Feng H. The emerging role and targetability of the TCA cycle in cancer metabolism. Protein Cell. (2018) 9:216–37. doi: 10.1007/s13238-017-0451-1
27. Akram M. Citric acid cycle and role of its intermediates in metabolism. Cell Biochem Biophys. (2014) 68:475–8. doi: 10.1007/s12013-013-9750-1
28. Marquez J, Flores J, Kim AH, Nyamaa B, Nguyen ATT, Park N, et al. Rescue of TCA cycle dysfunction for cancer therapy. J Clin Med. (2019) 8:1–3, 11. doi: 10.3390/jcm8122161
29. Eniafe J, Jiang S. The functional roles of TCA cycle metabolites in cancer. Oncogene. (2021) 40:3351–63. doi: 10.1038/s41388-020-01639-8
30. Bardella C, Pollard PJ, Tomlinson I. SDH mutations in cancer. Biochim Biophys Acta. (2011) 1807:1432–43. doi: 10.1016/j.bbabio.2011.07.003
31. Wu J-Y, Huang T-W, Hsieh Y-T, Wang Y-F, Yen C-C, Lee G-L, et al. Cancer-derived succinate promotes macrophage polarization and cancer metastasis via succinate receptor. Mol Cell. (2020) 77:213–227.e215. doi: 10.1016/j.molcel.2019.10.023
32. Launonen V, Vierimaa O, Kiuru M, Isola J, Roth S, Pukkala E, et al. Inherited susceptibility to uterine leiomyomas and renal cell cancer. Proc Natl Acad Sci U.S.A. (2001) 98:3387–92. doi: 10.1073/pnas.051633798
33. Ylisaukko-oja SK, Cybulski C, Lehtonen R, Kiuru M, Matyjasik J, Szymañska A, et al. Germline fumarate hydratase mutations in patients with ovarian mucinous cystadenoma. Eur J Hum Genet. (2006) 14:880–3. doi: 10.1038/sj.ejhg.5201630
34. Xiao M, Yang H, Xu W, Ma S, Lin H, Zhu H, et al. Inhibition of α-KG-dependent histone and DNA demethylases by fumarate and succinate that are accumulated in mutations of FH and SDH tumor suppressors. Genes Dev. (2012) 26:1326–38. doi: 10.1101/gad.191056.112
35. Deng D, Yan N. GLUT, SGLT, and SWEET: Structural and mechanistic investigations of the glucose transporters. Protein Sci. (2016) 25:546–58. doi: 10.1002/pro.2858
36. Zambrano A, Molt M, Uribe E, Salas M. Glut 1 in cancer cells and the inhibitory action of resveratrol as A potential therapeutic strategy. Int J Mol Sci. (2019) 20:4. doi: 10.3390/ijms20133374
37. Thorens B, Mueckler M. Glucose transporters in the 21st century. Am J Physiol Endocrinol Metab. (2010) 298:E141–145. doi: 10.1152/ajpendo.00712.2009
38. Luo XM, Zhou SH, Fan J. Glucose transporter-1 as a new therapeutic target in laryngeal carcinoma. J Int Med Res. (2010) 38:1885–92. doi: 10.1177/147323001003800601
39. Carvalho KC, Cunha IW, Rocha RM, Ayala FR, Cajaíba MM, Begnami MD, et al. GLUT1 expression in Malignant tumors and its use as an immunodiagnostic marker. Clinics (Sao Paulo). (2011) 66:965–72. doi: 10.1590/s1807-59322011000600008
40. Parente P, Coli A, Massi G, Mangoni A, Fabrizi MM, Bigotti G. Immunohistochemical expression of the glucose transporters Glut-1 and Glut-3 in human Malignant melanomas and benign melanocytic lesions. J Exp Clin Cancer Res. (2008) 27:34. doi: 10.1186/1756-9966-27-34
41. Okcu O, Sen B, Ozturk C, Guvendi GF, Bedir R. GLUT-1 expression in breast cancer. Turk Patoloji Derg. (2022) 38:114–21. doi: 10.5146/tjpath.2021.01557
42. Reinicke K, Sotomayor P, Cisterna P, Delgado C, Nualart F, Godoy A. Cellular distribution of Glut-1 and Glut-5 in benign and Malignant human prostate tissue. J Cell Biochem. (2012) 113:553–62. doi: 10.1002/jcb.23379
43. Lidgren A, Bergh A, Grankvist K, Rasmuson T, Ljungberg B. Glucose transporter-1 expression in renal cell carcinoma and its correlation with hypoxia inducible factor-1 alpha. BJU Int. (2008) 101:480–4. doi: 10.1111/j.1464-410X.2007.07238.x
44. Medina Villaamil V, Aparicio Gallego G, Valbuena Rubira L, García Campelo R, Valladares-Ayerbes M, Grande Pulido E, et al. Fructose transporter GLUT5 expression in clear renal cell carcinoma. Oncol Rep. (2011) 25:315–23. doi: 10.3892/or.2010.1096
45. Aparicio LM, Villaamil VM, Calvo MB, Rubira LV, Rois JM, Valladares-Ayerbes M, et al. Glucose transporter expression and the potential role of fructose in renal cell carcinoma: A correlation with pathological parameters. Mol Med Rep. (2010) 3:575–80. doi: 10.3892/mmr_00000300
46. Bryant NJ, Govers R, James DE. Regulated transport of the glucose transporter GLUT4. Nat Rev Mol Cell Biol. (2002) 3:267–77. doi: 10.1038/nrm782
47. McBrayer SK, Cheng JC, Singhal S, Krett NL, Rosen ST, Shanmugam M. Multiple myeloma exhibits novel dependence on GLUT4, GLUT8, and GLUT11: implications for glucose transporter-directed therapy. Blood. (2012) 119:4686–97. doi: 10.1182/blood-2011-09-377846
48. Shin E, Koo JS. Glucose metabolism and glucose transporters in breast cancer. Front Cell Dev Biol. (2021) 9:728759. doi: 10.3389/fcell.2021.728759
49. Garrido P, Osorio FG, Morán J, Cabello E, Alonso A, Freije JMP, et al. Loss of GLUT4 induces metabolic reprogramming and impairs viability of breast cancer cells. J Cell Physiol. (2015) 230:191–8. doi: 10.1002/jcp.24698
50. Tarn C, Skorobogatko YV, Taguchi T, Eisenberg B, von Mehren M, Godwin AK. Therapeutic effect of imatinib in gastrointestinal stromal tumors: AKT signaling dependent and independent mechanisms. Cancer Res. (2006) 66:5477–86. doi: 10.1158/0008-5472.Can-05-3906
51. Wood TE, Dalili S, Simpson CD, Hurren R, Mao X, Saiz FS, et al. A novel inhibitor of glucose uptake sensitizes cells to FAS-induced cell death. Mol Cancer Ther. (2008) 7:3546–55. doi: 10.1158/1535-7163.Mct-08-0569
52. Chan DA, Sutphin PD, Nguyen P, Turcotte S, Lai EW, Banh A, et al. Targeting GLUT1 and the warburg effect in renal cell carcinoma by chemical synthetic lethality. Sci Trans Med. (2011) 3:94ra70–0. doi: 10.1126/scitranslmed.3002394
53. Wu K-H, Ho C-T, Chen Z-F, Chen L-C, Whang-Peng J, Lin T-N, et al. The apple polyphenol phloretin inhibits breast cancer cell migration and proliferation via inhibition of signals by type 2 glucose transporter. J Food Drug Anal. (2018) 26:221–31. doi: 10.1016/j.jfda.2017.03.009
54. Liu Y, Cao Y, Zhang W, Bergmeier S, Qian Y, Akbar H, et al. A small-molecule inhibitor of glucose transporter 1 downregulates glycolysis, induces cell-cycle arrest, and inhibits cancer cell growth in vitro and in vivo. Mol Cancer Ther. (2012) 11:1672–82. doi: 10.1158/1535-7163.Mct-12-0131
55. Pliszka M, Szablewski L. Glucose transporters as a target for anticancer therapy. Cancers. (2021) 13:4184. doi: 10.3390/cancers13164184
56. Bishayee A. Cancer prevention and treatment with resveratrol: from rodent studies to clinical trials. Cancer Prev Res. (2009) 2:409–18. doi: 10.1158/1940-6207.Capr-08-0160
57. Vera JC, Reyes AM, Velásquez FV, Rivas CI, Zhang RH, Strobel P, et al. Direct inhibition of the hexose transporter GLUT1 by tyrosine kinase inhibitors. Biochemistry. (2001) 40:777–90. doi: 10.1021/bi001660j
58. Opipari AW Jr., Tan L, Boitano AE, Sorenson DR, Aurora A, Liu JR. Resveratrol-induced autophagocytosis in ovarian cancer cells. Cancer Res. (2004) 64:696–703. doi: 10.1158/0008-5472.Can-03-2404
59. Dutka M, Bobiński R, Francuz T, Garczorz W, Zimmer K, Ilczak T, et al. SGLT-2 inhibitors in cancer treatment-mechanisms of action and emerging new perspectives. Cancers (Basel). (2022) 14:1–2. doi: 10.3390/cancers14235811
60. Zhou J, Zhu J, Yu SJ, Ma HL, Chen J, Ding XF, et al. Sodium-glucose co-transporter-2 (SGLT-2) inhibition reduces glucose uptake to induce breast cancer cell growth arrest through AMPK/mTOR pathway. BioMed Pharmacother. (2020) 132:110821. doi: 10.1016/j.biopha.2020.110821
61. Saxton RA, Sabatini DM. mTOR signaling in growth, metabolism, and disease. Cell. (2017) 168:960–76. doi: 10.1016/j.cell.2017.02.004
62. Villani LA, Smith BK, Marcinko K, Ford RJ, Broadfield LA, Green AE, et al. The diabetes medication Canagliflozin reduces cancer cell proliferation by inhibiting mitochondrial complex-I supported respiration. Mol Metab. (2016) 5:1048–56. doi: 10.1016/j.molmet.2016.08.014
63. Kumar A, Rajendran V, Sethumadhavan R, Purohit R. AKT kinase pathway: a leading target in cancer research. ScientificWorldJournal. (2013) 2013:756134. doi: 10.1155/2013/756134
64. Taefehshokr S, Taefehshokr N, Hemmat N, Hajazimian S, Isazadeh A, Dadebighlu P, et al. The pivotal role of MicroRNAs in glucose metabolism in cancer. Pathol - Res Pract. (2021) 217:153314. doi: 10.1016/j.prp.2020.153314
65. Cheung EC, Vousden KH. The role of p53 in glucose metabolism. Curr Opin Cell Biol. (2010) 22:186–91. doi: 10.1016/j.ceb.2009.12.006
66. Feng Z, Levine AJ. The regulation of energy metabolism and the IGF-1/mTOR pathways by the p53 protein. Trends Cell Biol. (2010) 20:427–34. doi: 10.1016/j.tcb.2010.03.004
67. Hu W, Chan CS, Wu R, Zhang C, Sun Y, Song JS, et al. Negative Regulation of Tumor Suppressor p53 by MicroRNA miR-504. Mol Cell. (2010) 38:689–99. doi: 10.1016/j.molcel.2010.05.027
68. Feng X. Chemical and biochemical basis of cell-bone matrix interaction in health and disease. Curr Chem Biol. (2009) 3:189–96. doi: 10.2174/187231309788166398
69. Boyce BF, Yao Z, Xing L. Osteoclasts have multiple roles in bone in addition to bone resorption. Crit Rev Eukaryot Gene Expr. (2009) 19:171–80. doi: 10.1615/critreveukargeneexpr.v19.i3.10
70. Agerbaek MO, Eriksen EF, Kragstrup J, Mosekilde L, Melsen F. A reconstruction of the remodelling cycle in normal human cortical iliac bone. Bone Miner. (1991) 12:101–12. doi: 10.1016/0169-6009(91)90039-3
71. Caetano-Lopes J, Canhão H, Fonseca JE. Osteoblasts and bone formation. Acta Reumatol Port. (2007) 32:103–10.
72. Parfitt AM. Bone remodeling and bone loss: understanding the pathophysiology of osteoporosis. Clin Obstetrics Gynecology. (1987) 30:789–811. doi: 10.1097/00003081-198712000-00004
73. Clevers H, Nusse R. Wnt/β-catenin signaling and disease. Cell. (2012) 149:1192–205. doi: 10.1016/j.cell.2012.05.012
74. Boyce BF, Xing L. Biology of RANK, RANKL, and osteoprotegerin. Arthritis Res Ther. (2007) 9 Suppl 1:S1. doi: 10.1186/ar2165
75. Siddiqui JA, Partridge NC. Physiological bone remodeling: systemic regulation and growth factor involvement. Physiology. (2016) 31:233–45. doi: 10.1152/physiol.00061.2014
76. Crockett JC, Rogers MJ, Coxon FP, Hocking LJ, Helfrich MH. Bone remodelling at a glance. J Cell Sci. (2011) 124:991–8. doi: 10.1242/jcs.063032
77. Jaschke N, Sipos W, Hofbauer LC, Rachner TD, Rauner M. Skeletal endocrinology: where evolutionary advantage meets disease. Bone Res. (2021) 9:4. doi: 10.1038/s41413-021-00149-x
78. Lee NK, Sowa H, Hinoi E, Ferron M, Ahn JD, Confavreux C, et al. Endocrine regulation of energy metabolism by the skeleton. Cell. (2007) 130:456–69. doi: 10.1016/j.cell.2007.05.047
79. Diegel CR, Hann S, Ayturk UM, Hu JCW, Lim K-E, Droscha CJ, et al. An osteocalcin-deficient mouse strain without endocrine abnormalities. PloS Genet. (2020) 16:e1008361. doi: 10.1371/journal.pgen.1008361
80. Wang Y-N, Liu S, Jia T, Feng Y, Xu X, Zhang D. T cell protein tyrosine phosphatase in glucose metabolism. [Review. Front Cell Dev Biol. (2021) 9. doi: 10.3389/fcell.2021.682947
81. Ferron M, Wei J, Yoshizawa T, Del Fattore A, DePinho RA, Teti A, et al. Insulin signaling in osteoblasts integrates bone remodeling and energy metabolism. Cell. (2010) 142:296–308. doi: 10.1016/j.cell.2010.06.003
82. Clemens TL, Karsenty G. The osteoblast: an insulin target cell controlling glucose homeostasis. J Bone Mineral Res. (2011) 26:677–80. doi: 10.1002/jbmr.321
83. Brennan-Speranza TC, Conigrave AD. Osteocalcin: an osteoblast-derived polypeptide hormone that modulates whole body energy metabolism. Calcified Tissue Int. (2015) 96:1–10. doi: 10.1007/s00223-014-9931-y
84. Paglia DN, Wey A, Breitbart EA, Faiwiszewski J, Mehta SK, Al-Zube L, et al. Effects of local insulin delivery on subperiosteal angiogenesis and mineralized tissue formation during fracture healing. J Orthopaedic Res. (2013) 31:783–91. doi: 10.1002/jor.22288
85. Semenza GL, Jiang BH, Leung SW, Passantino R, Concordet JP, Maire P, et al. Hypoxia response elements in the aldolase A, enolase 1, and lactate dehydrogenase A gene promoters contain essential binding sites for hypoxia-inducible factor 1. J Biol Chem. (1996) 271:32529–37. doi: 10.1074/jbc.271.51.32529
86. Pouysségur J, Dayan F, Mazure NM. Hypoxia signalling in cancer and approaches to enforce tumour regression. Nature. (2006) 441:437–43. doi: 10.1038/nature04871
87. Jaakkola P, Mole DR, Tian YM, Wilson MI, Gielbert J, Gaskell SJ, et al. Targeting of HIF-alpha to the von Hippel-Lindau ubiquitylation complex by O2-regulated prolyl hydroxylation. Science. (2001) 292:468–72. doi: 10.1126/science.1059796
88. Maxwell PH, Wiesener MS, Chang GW, Clifford SC, Vaux EC, Cockman ME, et al. The tumour suppressor protein VHL targets hypoxia-inducible factors for oxygen-dependent proteolysis. Nature. (1999) 399:271–5. doi: 10.1038/20459
89. Greenhill C. Role of bone in glucose metabolism. Nat Rev Endocrinol. (2018) 14:191–1. doi: 10.1038/nrendo.2018.25
90. Dirckx N, Tower RJ, Mercken EM, Vangoitsenhoven R, Moreau-Triby C, Breugelmans T, et al. Vhl deletion in osteoblasts boosts cellular glycolysis and improves global glucose metabolism. J Clin Invest. (2018) 128:1087–105. doi: 10.1172/jci97794
91. Devignes C-S, Aslan Y, Brenot A, Devillers A, Schepers K, Fabre S, et al. HIF signaling in osteoblast-lineage cells promotes systemic breast cancer growth and metastasis in mice. Proc Natl Acad Sci. (2018) 115:E992–E1001. doi: 10.1073/pnas.1718009115
92. Valle-Tenney R, Melis S, Maes C. Hypoxia signaling in bone physiology and energy metabolism. Curr Opin Endocrine Metab Res. (2023) 32:100473. doi: 10.1016/j.coemr.2023.100473
93. Komiya Y, Habas R. Wnt signal transduction pathways. Organogenesis. (2008) 4:68–75. doi: 10.4161/org.4.2.5851
94. Kobayashi Y, Uehara S, Udagawa N, Takahashi N. Regulation of bone metabolism by Wnt signals. J Biochem. (2016) 159:387–92. doi: 10.1093/jb/mvv124
95. Gillespie JR, Bush JR, Bell GI, Aubrey LA, Dupuis H, Ferron M, et al. GSK-3β Function in bone regulates skeletal development, whole-body metabolism, and male life span. Endocrinology. (2013) 154:3702–18. doi: 10.1210/en.2013-1155
96. Yao Q, Yu C, Zhang X, Zhang K, Guo J, Song L. Wnt/β-catenin signaling in osteoblasts regulates global energy metabolism. Bone. (2017) 97:175–83. doi: 10.1016/j.bone.2017.01.028
97. Kim SP, Frey JL, Li Z, Goh BC, Riddle RC. Lack of lrp5 signaling in osteoblasts sensitizes male mice to diet-induced disturbances in glucose metabolism. Endocrinology. (2017) 158:3805–16. doi: 10.1210/en.2017-00657
98. Abdallah BM, Ditzel N, Laborda J, Karsenty G, Kassem M. DLK1 regulates whole-body glucose metabolism: A negative feedback regulation of the osteocalcin-insulin loop. Diabetes. (2015) 64:3069–80. doi: 10.2337/db14-1642
99. Nulali J, Zhan M, Zhang K, Tu P, Liu Y, Song H. Osteoglycin: an ECM factor regulating fibrosis and tumorigenesis. Biomolecules. (2022) 12:1674. doi: 10.3390/biom12111674
100. Lee NJ, Nguyen AD, Enriquez RF, Luzuriaga J, Bensellam M, Laybutt R, et al. NPY signalling in early osteoblasts controls glucose homeostasis. Mol Metab. (2015) 4:164–74. doi: 10.1016/j.molmet.2014.12.010
101. Lee NJ, Ali N, Zhang L, Qi Y, Clarke I, Enriquez RF, et al. Osteoglycin, a novel coordinator of bone and glucose homeostasis. Mol Metab. (2018) 13:30–44. doi: 10.1016/j.molmet.2018.05.004
102. Leong I. Osteoglycin — linking bone and energy homeostasis. Nat Rev Endocrinol. (2018) 14:379–9. doi: 10.1038/s41574-018-0036-y
103. Guntur AR, Rosen CJ. Bone as an endocrine organ. Endocr Pract. (2012) 18:758–62. doi: 10.4158/ep12141.Ra
104. Semenza GL. Targeting HIF-1 for cancer therapy. Nat Rev Cancer. (2003) 3:721–32. doi: 10.1038/nrc1187
105. Park HK, Ahima RS. Physiology of leptin: energy homeostasis, neuroendocrine function and metabolism. Metabolism. (2015) 64:24–34. doi: 10.1016/j.metabol.2014.08.004
106. Pereira S, Cline DL, Glavas MM, Covey SD, Kieffer TJ. Tissue-specific effects of leptin on glucose and lipid metabolism. Endocrine Rev. (2020) 42:1–28. doi: 10.1210/endrev/bnaa027
107. Zoch ML, Clemens TL, Riddle RC. New insights into the biology of osteocalcin. Bone. (2016) 82:42–9. doi: 10.1016/j.bone.2015.05.046
108. Karsenty G. Osteocalcin: A multifaceted bone-derived hormone. Annu Rev Nutr. (2023) 43:55–71. doi: 10.1146/annurev-nutr-061121-091348
109. Tanaka K, Matsumoto E, Higashimaki Y, Katagiri T, Sugimoto T, Seino S, et al. Role of osteoglycin in the linkage between muscle and bone. J Biol Chem. (2012) 287:11616–28. doi: 10.1074/jbc.M111.292193
110. Kaelin WG Jr. Molecular basis of the VHL hereditary cancer syndrome. Nat Rev Cancer. (2002) 2:673–82. doi: 10.1038/nrc885
111. Esen E, Chen J, Karner CM, Okunade AL, Patterson BW, Long F. WNT-LRP5 signaling induces Warburg effect through mTORC2 activation during osteoblast differentiation. Cell Metab. (2013) 17:745–55. doi: 10.1016/j.cmet.2013.03.017
112. Bhowmick NA, Neilson EG, Moses HL. Stromal fibroblasts in cancer initiation and progression. Nature. (2004) 432:332–7. doi: 10.1038/nature03096
113. Tasheva ES. Analysis of the promoter region of human mimecan gene. Biochim Biophys Acta (BBA) - Gene Structure Expression. (2002) 1575:123–9. doi: 10.1016/S0167-4781(02)00245-2
114. Wang Y, Ma Y, Lü B, Xu E, Huang Q, Lai M. Differential expression of mimecan and thioredoxin domain–containing protein 5 in colorectal adenoma and cancer: A proteomic study. Exp Biol Med. (2007) 232:1152–9. doi: 10.3181/0701-rm-8
115. Xu T, Zhang R, Dong M, Zhang Z, Li H, Zhan C, et al. Osteoglycin (OGN) inhibits cell proliferation and invasiveness in breast cancer via PI3K/akt/mTOR signaling pathway. OncoTargets Ther. (2019) 12:10639–50. doi: 10.2147/OTT.S222967
116. Hu X, Li Y-Q, Li Q-G, Ma Y-L, Peng J-J, Cai S-J. Osteoglycin-induced VEGF inhibition enhances T lymphocytes infiltrating in colorectal cancer. EBioMedicine. (2018) 34:35–45. doi: 10.1016/j.ebiom.2018.07.021
117. Lee C, Raffaghello L, Brandhorst S, Safdie FM, Bianchi G, Martin-Montalvo A, et al. Fasting cycles retard growth of tumors and sensitize a range of cancer cell types to chemotherapy. Sci Transl Med. (2012) 4:124ra127. doi: 10.1126/scitranslmed.3003293
118. Kennedy SP, O'Neill M, Cunningham D, Morris PG, Toomey S, Blanco-Aparicio C, et al. Preclinical evaluation of a novel triple-acting PIM/PI3K/mTOR inhibitor, IBL-302, in breast cancer. Oncogene. (2020) 39:3028–40. doi: 10.1038/s41388-020-1202-y
119. Xu D, Zhou Y, Xie X, He L, Ding J, Pang S, et al. Inhibitory effects of canagliflozin on pancreatic cancer are mediated via the downregulation of glucose transporter−1 and. Int J Oncol. (2020) 57:1223–33. doi: 10.3892/ijo.2020.5120
120. Saraei P, Asadi I, Kakar MA, Moradi-Kor N. The beneficial effects of metformin on cancer prevention and therapy: a comprehensive review of recent advances. Cancer Manag Res. (2019) 11:3295–313. doi: 10.2147/cmar.S200059
121. Evans JM, Donnelly LA, Emslie-Smith AM, Alessi DR, Morris AD. Metformin and reduced risk of cancer in diabetic patients. Bmj. (2005) 330:1304–5. doi: 10.1136/bmj.38415.708634.F7
122. Bodmer M, Becker C, Meier C, Jick SS, Meier CR. Use of metformin and the risk of ovarian cancer: A case–control analysis. Gynecologic Oncol. (2011) 123:200–4. doi: 10.1016/j.ygyno.2011.06.038
123. Rêgo DF, Pavan LM, Elias ST, De Luca Canto G, Guerra EN. Effects of metformin on head and neck cancer: a systematic review. Oral Oncol. (2015) 51:416–22. doi: 10.1016/j.oraloncology.2015.01.007
124. Dunbar EM, Coats BS, Shroads AL, Langaee T, Lew A, Forder JR, et al. Phase 1 trial of dichloroacetate (DCA) in adults with recurrent Malignant brain tumors. Investigational New Drugs. (2014) 32:452–64. doi: 10.1007/s10637-013-0047-4
125. Wu JW, Azoulay L, Majdan A, Boivin J-F, Pollak M, Suissa S. Long-term use of long-acting insulin analogs and breast cancer incidence in women with type 2 diabetes. J Clin Oncol. (2017) 35:3647–53. doi: 10.1200/jco.2017.73.4491
127. Bidard FC, Vincent-Salomon A, Gomme S, Nos C, de Rycke Y, Thiery JP, et al. Disseminated tumor cells of breast cancer patients: a strong prognostic factor for distant and local relapse. Clin Cancer Res. (2008) 14:3306–11. doi: 10.1158/1078-0432.Ccr-07-4749
128. Steger GG, Bartsch R. Denosumab for the treatment of bone metastases in breast cancer: evidence and opinion. Ther Adv Med Oncol. (2011) 3:233–43. doi: 10.1177/1758834011412656
129. Thomas RJ, Guise TA, Yin JJ, Elliott J, Horwood NJ, Martin TJ, et al. Breast cancer cells interact with osteoblasts to support osteoclast formation. Endocrinology. (1999) 140:4451–8. doi: 10.1210/endo.140.10.7037
130. Fizazi K, Yang J, Peleg S, Sikes CR, Kreimann EL, Daliani D, et al. Prostate cancer cells-osteoblast interaction shifts expression of growth/survival-related genes in prostate cancer and reduces expression of osteoprotegerin in osteoblasts. Clin Cancer Res. (2003) 9:2587–97.
131. Roodman GD, Dougall WC. RANK ligand as a therapeutic target for bone metastases and multiple myeloma. Cancer Treat Rev. (2008) 34:92–101. doi: 10.1016/j.ctrv.2007.09.002
132. Canon JR, Roudier M, Bryant R, Morony S, Stolina M, Kostenuik PJ, et al. Inhibition of RANKL blocks skeletal tumor progression and improves survival in a mouse model of breast cancer bone metastasis. Clin Exp Metastasis. (2008) 25:119–29. doi: 10.1007/s10585-007-9127-1
133. Gonzalez-Suarez E, Jacob AP, Jones J, Miller R, Roudier-Meyer MP, Erwert R, et al. RANK ligand mediates progestin-induced mammary epithelial proliferation and carcinogenesis. Nature. (2010) 468:103–7. doi: 10.1038/nature09495
134. Chen Y, Zhu J, Zhou Y, Peng J, Wang B. Efficacy and safety of denosumab in osteoporosis or low bone mineral density postmenopausal women. [Systematic review. Front Pharmacol. (2021) 12:588095. doi: 10.3389/fphar.2021.588095
135. Gnant M, Pfeiler G, Steger GG, Egle D, Greil R, Fitzal F, et al. Adjuvant denosumab in postmenopausal patients with hormone receptor-positive breast cancer (ABCSG-18): disease-free survival results from a randomised, double-blind, placebo-controlled, phase 3 trial. Lancet Oncol. (2019) 20:339–51. doi: 10.1016/s1470-2045(18)30862-3
136. Pacheco-Soto BT, Elguezabal-Rodelo RG, Porchia LM, Torres-Rasgado E, Pérez-Fuentes R, Gonzalez-Mejia ME. Denosumab improves glucose parameters in patients with impaired glucose tolerance: a systematic review and meta-analysis. J Drug Assess. (2021) 10:97–105. doi: 10.1080/21556660.2021.1989194
137. Kostenuik PJ. Osteoprotegerin and RANKL regulate bone resorption, density, geometry and strength. Curr Opin Pharmacol. (2005) 5:618–25. doi: 10.1016/j.coph.2005.06.005
138. Duan P, Yang M, Wei M, Liu J, Tu P. Serum osteoprotegerin is a potential biomarker of insulin resistance in chinese postmenopausal women with prediabetes and type 2 diabetes. Int J Endocrinol. (2017) 2017:8724869. doi: 10.1155/2017/8724869
139. Cantley J, Ashcroft FM. Q&A: insulin secretion and type 2 diabetes: why do β-cells fail? BMC Biol. (2015) 13:6. doi: 10.1186/s12915-015-0140-6
140. Jeffcoate WJ, Game F, Cavanagh PR. The role of proinflammatory cytokines in the cause of neuropathic osteoarthropathy (acute Charcot foot) in diabetes. Lancet. (2005) 366:2058–61. doi: 10.1016/s0140-6736(05)67029-8
141. Bonnet N, Bourgoin L, Biver E, Douni E, Ferrari S. RANKL inhibition improves muscle strength and insulin sensitivity and restores bone mass. J Clin Invest. (2019) 129:3214–23. doi: 10.1172/jci125915
142. Weivoda MM, Chew CK, Monroe DG, Farr JN, Atkinson EJ, Geske JR, et al. Identification of osteoclast-osteoblast coupling factors in humans reveals links between bone and energy metabolism. Nat Commun. (2020) 11:87. doi: 10.1038/s41467-019-14003-6
143. van Dam PA, Verhoeven Y, Trinh XB. The non-bone-related role of RANK/RANKL signaling in cancer. Adv Exp Med Biol. (2020) 1277:53–62. doi: 10.1007/978-3-030-50224-9_3
144. Rao S, Cronin SJF, Sigl V, Penninger JM. RANKL and RANK: from mammalian physiology to cancer treatment. Trends Cell Biol. (2018) 28:213–23. doi: 10.1016/j.tcb.2017.11.001
145. Slavic S, Andrukhova O, Ford K, Handschuh S, Latic N, Reichart U, et al. Selective inhibition of receptor activator of NF-κB ligand (RANKL) in hematopoietic cells improves outcome after experimental myocardial infarction. J Mol Med (Berl). (2018) 96:559–73. doi: 10.1007/s00109-018-1641-x
146. Carbone F, Crowe LA, Roth A, Burger F, Lenglet S, Braunersreuther V, et al. Treatment with anti-RANKL antibody reduces infarct size and attenuates dysfunction impacting on neutrophil-mediated injury. J Mol Cell Cardiol. (2016) 94:82–94. doi: 10.1016/j.yjmcc.2016.03.013
147. Esser N, Legrand S, Piette J, Scheen A, Paquot N. Inflammation as a link between obesity, metabolic syndrome and type 2 diabetes. Diabetes Res Clin Pract. (2014) 105:141–3. doi: 10.1016/j.diabres.2014.04.006
148. Huang R, Wang X, Zhou Y, Xiao Y. RANKL-induced M1 macrophages are involved in bone formation. Bone Res. (2017) 5:17019. doi: 10.1038/boneres.2017.19
149. Kiechl S, Wittmann J, Giaccari A, Knoflach M, Willeit P, Bozec A, et al. Blockade of receptor activator of nuclear factor-κB (RANKL) signaling improves hepatic insulin resistance and prevents development of diabetes mellitus. Nat Med. (2013) 19:358–63. doi: 10.1038/nm.3084
150. Liu L, Zhang C, Hu Y, Peng B. Protective effect of metformin on periapical lesions in rats by decreasing the ratio of receptor activator of nuclear factor kappa B ligand/osteoprotegerin. J Endod. (2012) 38:943–7. doi: 10.1016/j.joen.2012.03.010
Keywords: glucose, bone, metabolism, cancer, systemic
Citation: Ronghe R and Tavares AAS (2024) The skeleton: an overlooked regulator of systemic glucose metabolism in cancer? Front. Oncol. 14:1481241. doi: 10.3389/fonc.2024.1481241
Received: 15 August 2024; Accepted: 22 October 2024;
Published: 11 November 2024.
Edited by:
Cecilia Ana Suarez, National Scientific and Technical Research Council (CONICET), ArgentinaCopyright © 2024 Ronghe and Tavares. This is an open-access article distributed under the terms of the Creative Commons Attribution License (CC BY). The use, distribution or reproduction in other forums is permitted, provided the original author(s) and the copyright owner(s) are credited and that the original publication in this journal is cited, in accordance with accepted academic practice. No use, distribution or reproduction is permitted which does not comply with these terms.
*Correspondence: Rucha Ronghe, czE5MDQ5NDZAZWQuYWMudWs=