- 1Institute of Medical Science, University of Toronto, Toronto, ON, Canada
- 2Department of Radiation Oncology, Odette Cancer Centre, Sunnybrook Hospital, Toronto, ON, Canada
- 3Division of Neurosurgery, St. Michael’s Hospital, University of Toronto, Toronto, ON, Canada
Intracranial metastatic disease is a serious complication of cancer, treated through surgery, radiation, and targeted therapies. The central role of radiation therapy makes understanding the radioresistance of metastases a priori a key interest for prognostication and therapeutic development. Although historically defined clinic-radiographically according to tumour response, developments in new techniques for delivering radiation treatment and understanding of radioprotective mechanisms led to a need to revisit the definition of radioresistance in the modern era. Factors influencing radioresistance include tumour-related factors (hypoxia, cancer stem cells, tumour kinetics, tumour microenvironment, metabolic alterations, tumour heterogeneity DNA damage repair, non-coding RNA, exosomes, methylomes, and autophagy), host-related factors (volume effect & dose-limiting non-cancerous tissue, pathophysiology, and exosomes), technical factors, and probabilistic factors (cell cycle and random gravity of DNA damage). Influences on radioresistance are introduced and discussed in the context of brain metastases.
1 Introduction
1.1 Intracranial metastatic disease and radiotherapy
Intracranial metastatic disease (IMD) is a serious complication of cancer and the most common type of brain tumour (1), estimated to affect roughly 2% of all patients with cancer and compromise 12% of all metastatic sites (2). IMD has a poor prognosis, which is affected by factors such as the extent of systemic disease, number of intracranial metastases, performance status and age (1, 3). Treatments for IMD include surgery, radiation and target therapies (4). Radiation therapy (RT) holds a central role in IMD treatment and why understanding the radioresistance of a metastases a priori is of key interest in both prognostication and therapeutic development.
1.2 History of radiobiology and radioresistance
The effects seen from RT result principally from direct or indirect DNA damage (5). Direct ionising radiation damage results from DNA ionisation or excitation, leading to a base change. Indirect ionising radiation damage occurs more frequently from RT and occurs when the energy from the RT is transferred to other cellular atoms (namely water) and produces free radicals, which can then damage the DNA (5).
Historically, radioresistance has been defined clinic-radiographically according to tumour response following treatment with a maximum tolerable dose (6). However the radiation dose was limited by conventional techniques. In particular for brain metastases the treatment was whole brain radiation treatment (WBRT), and the “maximum” dose applied was far lower than what can be achieved with modern conformal stereotactic techniques. Therefore, the development and increasing use of stereotactic radiosurgery (SRS), which allows a higher maximum tolerable dose because of decreased radiation exposure beyond the target, has brought into question the validity of these classical definitions. There is a need to revisit the definition of radioresistance in the modern era.
Lester J. Peters, in his keynote address from the 1980s, identified four main categories of clinical radioresistance: tumour-related factors, host-related factors, technical factors, and probabilistic factors (6). Tumour-related factors include hypoxia, number of clonogenic cells, tumour kinetics and intrinsic radioresistance. Host-related factors include the volume effect (complications based on the volume irritated), dose-limiting non-cancerous tissue, pathophysiological factors and host defences. Technical factors include geographic miss of treatment and errors in dose delivery. Lastly, probabilistic radioresistance, as RT relies on damaged DNA to produce an effect, speaks to the randomness inherent in the production of DNA damage (6). Since then, additional factors that affect radioresistance have been identified, including the tumour microenvironment (TME), metabolic alterations, cancer stem cells (CSCs), tumour heterogeneity, microRNAs, cell cycle stage, DNA damage repair pathways, exosomes, methylation state and autophagy (7–9). Ultimately, many of these factors are interconnected and influence each other through various mechanisms and sub-pathways (Figure 1).
2 Tumour-related factors
Peters identified hypoxia, the number of clonogenic cells, tumour kinetics and intrinsic radioresistance as the tumour-related factors causing radioresistance. Work performed since has further characterised the mechanisms in which tumours develop radioresistant phenotypes (6). The broad term of intrinsic radioresistance, which had initially been defined as “radioresistance manifested by asynchronous well-oxygenated populations of tumour cells cultured in-vitro” and had focused on DNA repair mechanisms (6), now also includes factors such as the TME, metabolic alterations, cancer stem cells (which had been previously termed clonogenic cancer cells), tumour heterogeneity, DNA damage repair, non-coding RNAs, exosomes, methylation state and autophagy.
2.1 Hypoxia
Indirect DNA damage inflicted by RT is primarily mediated by reactive oxygen species (ROSs), including O2-, H2O2, and OH (8). The ionising effect of RT is enhanced by normoxia (the “oxygen effect”) (5). The “oxygen fixation hypothesis” asserts that DNA damage incurred by free radicals is made irreparable when free molecular oxygen is available (5, 10). In many brain metastases, however, the TME is marked by regions of relative hypoxia (11). Hypoxia in tumours occurs in a gradient in relation to capillary penetration, leading to levels of cellular oxygenation ranging from normoxia to anoxic necrosis (5, 12). This gradient can result in varied responses to RT (5). Acute cellular hypoxia may occur regardless of cell proximity to the vasculature, adding a layer of temporal hypoxia that may alter treatment response (13).
Further, hypoxia may induce many pro-proliferative or adaptive pathways that can enhance radioresistance. Activation of hypoxia-inducible factor-1 (HIF-1), for example, may result in multiple cell-level changes, such as in energy metabolism, cell cycle, epithelial-to-mesenchymal transition (EMT), DNA damage response, autophagy, epigenetics and cytoprotection (11), that can support cell survival in hostile microenvironments, all while aiding in the maintenance of a stem-like state (14). Chronic hypoxia and vascular remodelling can also cause endothelial and oligodendrocytic cell death, resulting in demyelination and defective microenvironment function (15). In studies evaluating hypoxia in lung cancer-derived brain metastases, hypoxia was found to be a considerable yet heterogeneous feature.
2.2 Clonogenic/Cancer stem cells
Clonogenic cells are defined as cells that are capable of regenerating the tumour within which they are found (6). More recently, this property has been proposed to reside within cancer stem cells (CSCs). CSCs have also been shown to possess intrinsic resistance to cytotoxic injury and cell death (16) and, as such, may serve as a reservoir for tumour recurrence following RT (17). CSCs employ a variety of radioresistance mechanisms to survive radiation-associated injury (17). Relative to non-CSCs, CSCs have an enhanced capacity for DNA repair and defences against ROS-mediated DNA damage (18).
CSCs have been identified to express many molecular mediators of radioresistance, including components of the ERK, VEGF, glycolysis, WNT/β-catenin, Notch, JAK2 and PI3K/Akt/mTOR pathways (19, 20). Markers for CSC radioresistance include CD133, CD24-/low/CD44+, CD29, CD44, CD45, CD3, CD20, CD10, glycophorin A, CD64, CD 326, and ALDH1 (19). CSCs have also been found to be capable of undergoing the epithelial-mesenchymal transition (EMT), which has been linked to the development of metastatic disease (14).
2.3 Tumour kinetics
Due to radiation treatments being delivered through fractions over a timeframe, tumour cells are given opportunities to replicate between treatments (6). The more active the tumour kinetic profile (i.e. growth rate), the more ‘recuperation’ can occur between treatments (21). Relative to fractionated WBRT, SRS delivers a high radiation dose in a single treatment (or a few high dose per fraction treatments). Intracranial progression in those with IMD is common in the timeframe between diagnosis and the day of SRS treatment, with an interval growth in the index lesion (largest lesion at diagnosis) of a minimum of 3mm in roughly 60% of the patients (22).
Tumour kinetics of IMD can vary based on the primary tumour type. A study investigating apparent diffusion coefficient (ADC) in brain metastases pre- and post-SRS, found that ADC was higher pre- and post-SRS in brain metastases relative to healthy brain tissue, while no significant difference in ADC pre-SRS was observed between samples of different primaries. Interestingly, post-SRS, lung metastases ADC decreased, breast metastases ADC increased, and genitourinary metastases ADC did not significantly change (23). Differences in IMD kinetics have been found to be a useful prognostic indicator for overall survival in patients receiving SRS for IMD, with a study finding the hazard ratio per 1% change in brain metastases size/day between diagnostic and stereotactic MRI to be 1.32 (95% CI 1.06-1.65) (24).
2.4 Tumour microenvironment
The TME plays a role in tumour phenotype and overall cancer outcomes while modulating factors such as hypoxia and influencing the behaviour of tumour cells (13). Influences can come from cross-talking cancer cells, immune cells, vasculature, structural tissue, and more (13). Many TME components are mixed in with the cancer cells, with varying spatial architecture of the TME between lesions (25). Unbalanced cancer cell proliferation leads to unbalanced oxygen demands, which neo-vasculature attempts to respond to (13). Neo-vasculature is developed in response to proinflammatory signals such as vascular endothelial growth factor (VEGF), secreted by tumour cells (26). This vasculature provides oxygen and nutrients but rarely permeates the tumour, meaning the aforementioned hypoxic gradient remains in the presence of neo-vasculature (13).
Tumour-associated macrophages (TAMs) have been well characterised for their prevalence and association with cancers, their ability to promote tumour growth, and their role in enhancing radioresistance (13). Cyclooxygenase-2 (Cox-2) can be produced in cells such as macrophages, producing prostaglandin E2 (PGE2), which can lead to the upregulation of early growth response1 (Egr1) transcription factor and Id1 expression, increasing resistance to radiation-induced DNA damage (27). It has also been suggested that irradiation of macrophages induces high expression of tumour necrosis factor-α (TNF-α), promoting angiogenesis and cell survival (28).
Studies on Cancer-Associated Fibroblasts (CAFs) and RT have reported a poorer prognosis following RT when increased levels of CAFs are detected (13). Factors secreted by CAFs reported to induce radioresistance include epidermal growth factor (EGF), fibroblast growth factor 4 (FGF4), and insulin-like growth factor 2 (IGF2) (29). These factors confer survival and protection signals to elude radiation-induced cell death (29).
Targeting the post-irradiation inflammatory process could potentially aid in the complete elimination of cancer cells by reducing the long-term fibrotic presence (30). Post-irradiation upregulation of NF-KB is associated with the expression of inflammatory cytokines and enzymes within the TME, such as IL-β/6/8, Cox-2, and granulocyte-macrophage colony-stimulating factor (30).
The brain is a somewhat unique structure in the body, composed of distinct tissue types (e.g. astrocytes, neurons, microglia), leading to an equally unique extracellular matrix (ECM) and TME (31, 32). Studies have found a loss of PTEN expression in tumour cells when in the brain, determining that microRNA containing exosomes released by astrocytes lead to this PTEN suppression (33). PTEN suppression aids in metastatic growth (32). Additionally, a study investigating the cellular architecture of IMD by analysing stromal composition observed that IMD from different cancer types presented with similar metastatic niches. All cases observed were mainly located in the brain’s cortex; however, major differences in immune composition were observed (34).
2.5 Metabolic alterations
Metabolic changes within cancer are a well-classified staple of the disease. The Warburg effect, through the promotion of glycolysis and synthesis of NADPH through the pentose phosphate pathway, provides a source of electrons for antioxidant systems, ultimately decreasing cellular ROSs (8, 35). Other glucose metabolism-related factors associated with radioresistance include the GLUT1 membrane protein (36). This protein has a high affinity for glucose, allowing for higher transport rates under normal physiological conditions (37).
Lipid metabolism also plays a role in cellular radioresistance (38, 39). Increased fatty acid oxidation (FAO) reduces fatty acid accumulation following RT (39). FAO products such as FAO-derived acetyl-CoA also aid in radioresistance through pathways enhancing anti-phagocytosis in radioresistant cells (39). Apart from lipid and glucose metabolism, radiosensitisation of cancer cells was also observed in a study where glutamine metabolism was targeted by inhibition of GLS and MYC (40).
Cellular metabolism in the brain primarily uses glucose as the desired energy source (41). A study on breast cancer IMD found an increase in the expression of enzymes involved with glycolysis, oxidative phosphorylation, and the tricarboxylic acid cycle when oxygenated and increased activation of the redox-balancing pentose phosphate pathway and glutathione pathway (42). Additionally, in the absence of glucose, brain metastatic breast cancer cells increased oxidations of glutamine and branched amino acids and gluconeogenesis to allow for non-oxidative pentose pathway synthesis of purine (43). Another study on breast cancer IMD, had found that the fatty acid binding protein 7 (FABP7) supports cellular glycolytic phenotype and storage of lipid droplets. This regulator aids in IMD development and initial survival within the brain microenvironment (44).
2.6 Tumour heterogeneity
The problem of progenitor cancer cells remaining after treatment can become exacerbated when heterogeneous populations of cancer cells reside within the same tumour. tumour heterogeneity spans a broad spectrum, including differences in molecular, cellular, genetic, and spatial features of cells (45). In a study on murine glioma radioresistance, single-cell transcriptomic analysis identified distinct radioresistant and radiosensitive groups. Of the radioresistant groups, one was highly proliferative, the other was not (12). Studies have found that tumour heterogeneity relies on the number of CSCs and that cell-cell and cell-matrix interactions lead to the tumour heterogeneity needed for growth (46).
A study performing a transcriptomic analysis during a study on breast cancer metastasis to the brain and liver, was able to generate 50 intratumoural expression-based subclusters for overall transcriptomic analysis across all tumour cells (47), exemplifying the sheer quantity of intratumoural heterogeneity possible within IMD. IMDs often have clinically relevant genotypic and phenotypic changes that occur during the process of metastases, which can contribute to observed heterogeneity. Performing whole-exome sequencing analysis between matched primaries and brain metastases, using 83 samples from 11 different primaries, a study found 53% of cases contained potentially clinically relevant alterations detected in the brain metastases not present in the matched primary (48).
2.7 DNA damage repair
DNA damage repair serves as a main mechanism for radioresistance with mechanisms including non-homologous end joining (NHEJ), homologous recombination (HR), single strand annealing (SSA), mismatch repair (MMR), nucleotide excision repair (NER), and base excision repair (BER) (10, 17). Of these, only NHEJ and HR repair double-strand breaks, with HR being less error-prone by using the homologous sequence of the damaged DNA’s respective sister chromatid as a template (10). A key part of DNA damage response is sensing and responding to damage effectively. Sensors transduce the DNA damage signal and sometimes directly recruit DNA damage repair proteins to the site of damage (49).
Studies have found frequent overexpression of BARD1 and RAD51 in IMD from breast cancer relative to both matched primary tumours and unlinked systemic metastases. These genes function to repair double-strand breaks, ultimately protecting IMD from permanent ROS DNA damage (50). Another group investigating DNA damage response gene signatures in colorectal brain metastases found that IMD presented with deficiencies in HR and MMR relative to the colorectal cancer primaries (51). These two studies demonstrate differences in DNA damage repair between primary cancers and their IMD, which may ultimately influence radioresistance profiles between primaries and IMD.
2.8 Non-coding RNAs
Non-coding RNAs, such as microRNAs modulate gene expression following transcription (8). MicroRNAs do not directly confer radioresistance or radiosensitivity, but by modulating gene expression, some microRNAs influence radioresistance through their involvement with related signalling pathways (52).
Long non-coding RNAs (lncRNAs) have been found to play an important role in cancer radioresistance. LncRNAs play roles in transcription, chromatin remodelling, and biological behaviour within the cell (53, 54). A study investigating 23 lncRNAs in 11 cancer types found 13 lncRNAs downregulated and 3 upregulated related to radiosensitivity, and 11 lncRNAs upregulated related to radioresistance (55). Notable lncRNA targets or pathways included PSEN1, EGFR, p53, MAPK1, and ERK. An expanded list of significant non-coding RNA and their targets, including those identified by the aforementioned study, (55) are listed in Table 1.
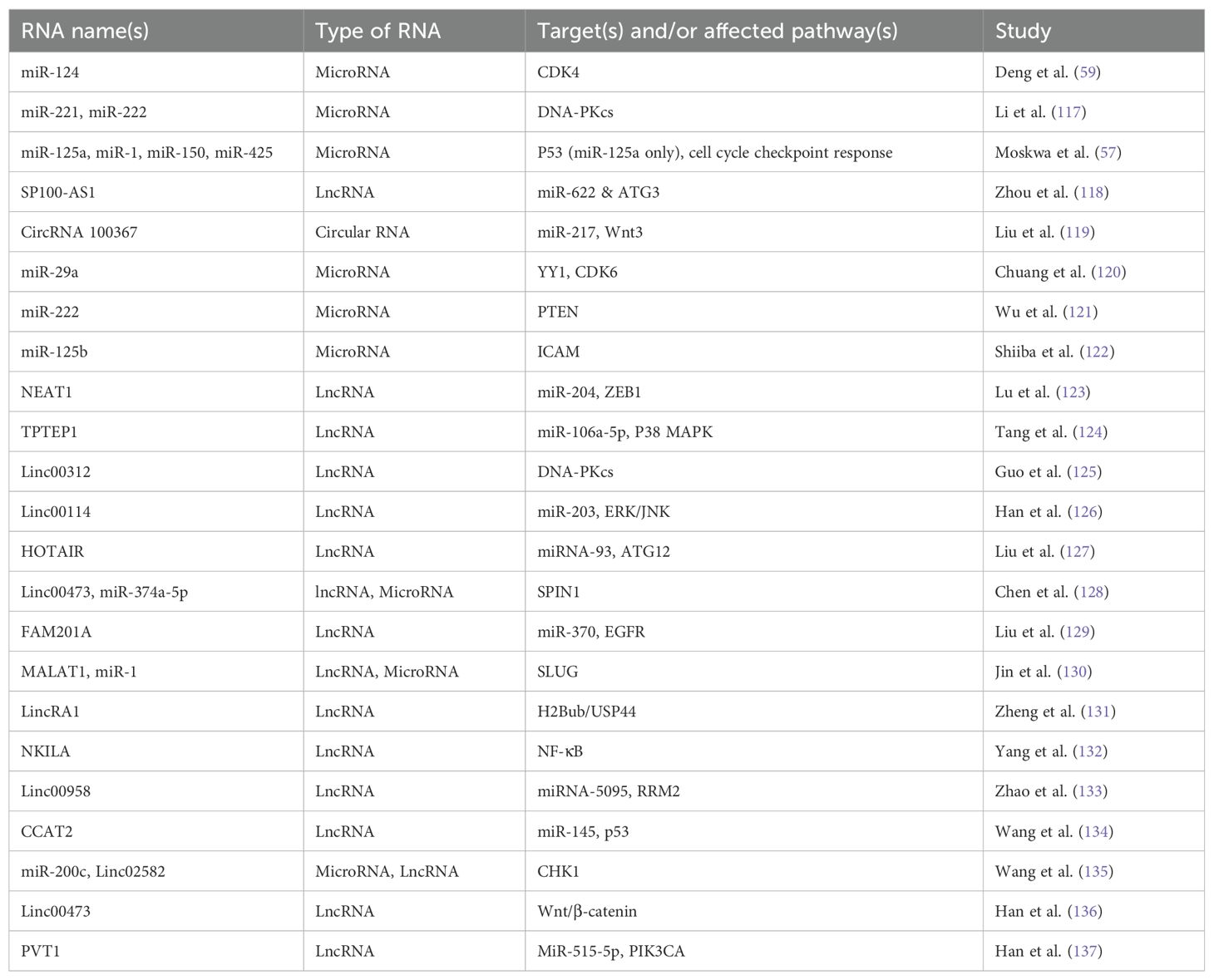
Table 1. Radioresistance/radiosensitivity related non-coding RNA names, type, target, affected pathway, and study.
Of the previously identified non-coding RNAs involved in response to radiation, few studies have identified their involvement in IMD. Circular RNA circBCBM1 modulates miR-125a and, through inhibiting miR-125a activity, promotes breast cancer metastasising to the brain (56). Interestingly, in a study on miRs, which induce radioresistance in glioblastomas, overexpression of miR-125a promoted radioresistance (57). A study comparing microRNA signatures in brain metastases compared to primary non-small cell lung cancer found notable upregulation of miR-124-3p and downregulation of miR-150-5p in brain metastases relative to the primary (58). miR-124 has been identified to promote radiosensitisation of glioma cells through targeting CDK4, and overexpression of miR150 induces radioresistance through cell-cycle checkpoint upregulation (57, 59).
2.9 Exosomes
Exosomes released by cells contain components such as mRNA, proteins, DNA fragments, lipids, and non-coding RNAs, playing roles in many cancer attributes, such as cell-cell communication, progression, interactions with the stroma and TME, and signalling pathway activation (7). While under stress, exosome qualities change, with studies that have found exosomes released from radiation-treated cancer cells enhanced the migration of cells which received them (60), and it has been indicated that exosomes aid in establishing pre-metastatic niches (61). Radiation-induced exosomes play a pivotal role in characterising cellular radioresistance (62). Studies on DNA damage and exosome secretion in cancer cells have found that p53 activation in response to DNA damage can increase exosome production and secretion (63, 64).
Exosomes maintain multiple roles within IMD, ranging from aiding in the initiation of metastasis by aiding in the destruction of tight junctions and modulating cell migration (65, 66) to being associated with intracranial failure following RT (67). A study on lung cancer patients with IMD receiving whole brain RT analysed circulating exosome integrins, where they found that levels of integrin β3 were associated with survival and local intracranial control (67).
2.10 Methylomes
Following RT, the methylomic landscape of cells can be altered, potentially promoting treatment efficacy or causing radioresistance (68). Differences have been found between the methylomes of radiosensitive and radioresistant cancers, as well as differences between the methylomes of intrinsic and adaptive radioresistance (68). When comparing the methylome of differentially responding non-small cell lung cancer, a study found hypermethylation was typically identified in the promotor regions of tumour suppressor genes and hypomethylation in specific genes; hypermethylation was found to be more prevalent in radioresistant cells (69).
Studies have identified and validated differentially methylated genes between radioresistant and radiosensitive non-small cell lung cancer phenotypes, including the cell survival and antioxidant-related genes SERPINB5, S100A6, CAT, and BNC1 (69). Studies in oral cancer have found additional radioresistant methylation markers with the hypermethylation of FHIT in radioresistant cells (70).
A study found that YTHDF3, which promotes breast cancer brain metastases through various pathways is dependent on m6A methylation (71). Furthermore, a study on DNA methylation of prostate cancer IMD, found aberrant methylation within the IMD to be associated with the activity of the PRC2 complex as well as their mutational background. Ultimately, their results suggested a specific reprogramming requirement within primary tumours to form IMD (72).
2.11 Autophagy
Acting as a cellular recycling mechanism allows autophagy to confer both radioresistance and chemoresistance. Ultimately, this cellular self-preservation mechanism allows cellular population regrowth following treatments and harsh conditions (9). For example, the glucose-related protein GRP94 has direct implications in promoting IMD through the activation of pro-survival autophagy pathways (73). These studies further identify autophagy as a cause of IMD and a target for treatment.
2.12 Related therapeutic approaches
When targeting hypoxia, various approaches are being investigated, including hypoxia-activated prodrugs (HAPs), hyperbaric oxygen breathing, allosteric haemoglobin modifiers, oxygen diffusion aide molecules, and oxygen transport agents (74). With the importance of HIF-1 to hypoxia and overall tumour phenotype, it has also been identified as a target for drugs such as vorinostat and benzopyranyl triazole (75) (Figure 2A).
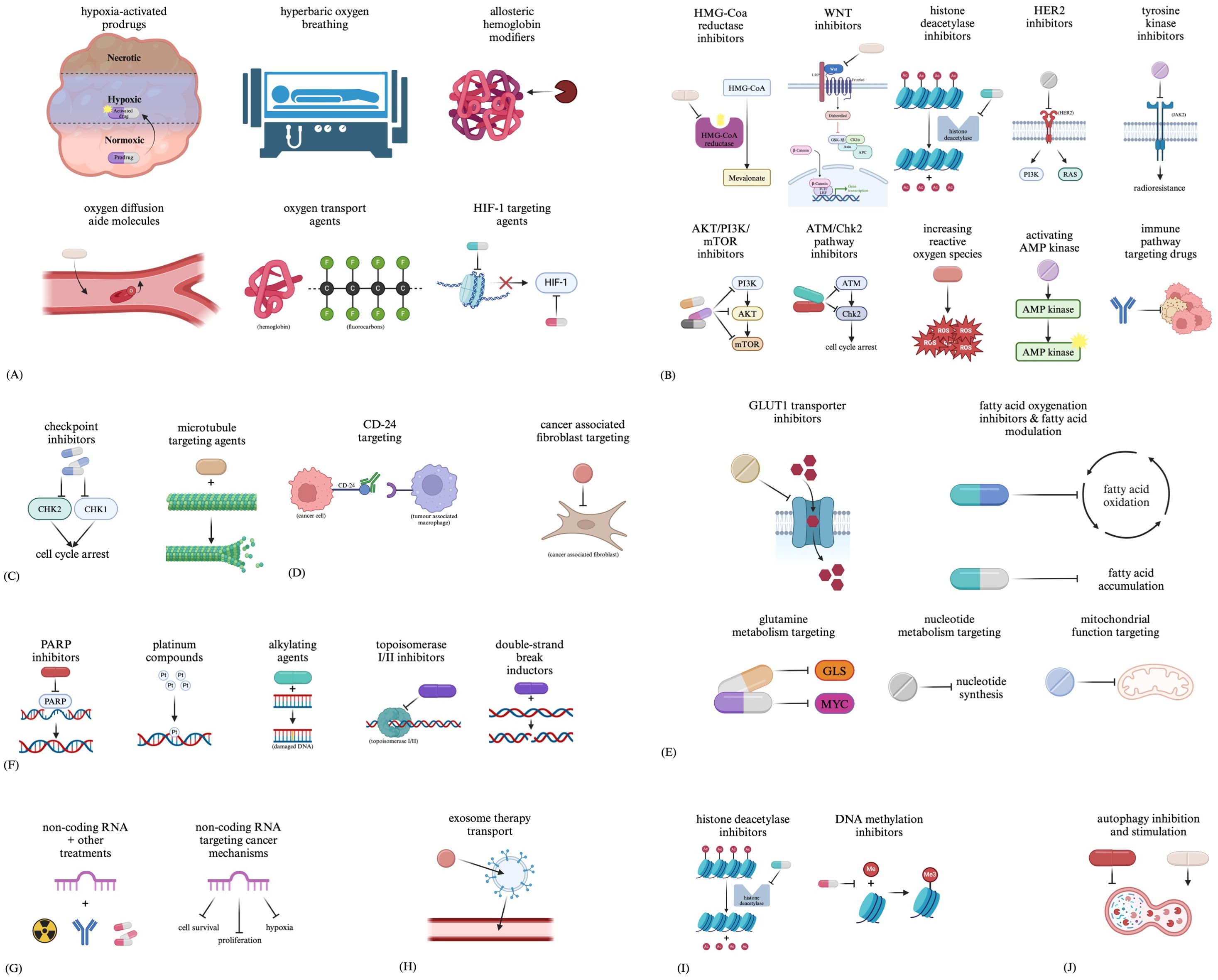
Figure 2. Therapeutic approaches related to (A) hypoxia, (B) CSCs, (C) the cell cycle, (D) the tumour microenvironment, (E) metabolic changes, (F) DNA damage repair, (G) non-coding RNAs, (H) exosomes, (I) methylation and (J) autophagy.
Targeted treatment of CSCs proves challenging due to the heterogeneity between subclones and how the tumour reacts to initial treatment (76). Although preclinical, papers review approaches under investigation for targeting CSCs in cancers such as acute myeloid leukaemia, including inhibition of HMG-Coa reductase, WNT, histone deacetylases, HER2, AKT/PI3K/mTOR, the ATM/Chk2 pathway; increasing reactive oxygen species, activating AMP kinase, and drugs targeting immune pathways (76). As tyrosine kinase pathways such as JAK2 have been implicated in CSC radioresistance, tyrosine kinase inhibitors may prove to be an additional radiosensitiser (Figure 2B).
Main targets for treatment aimed at the cell cycle include the cell cycle checkpoint kinases CHK1 and CHK2 (77). Additionally, many types of drugs can lead to cell cycle arrest, such as microtubule-targeting agents, indirectly altering the tumour kinetics of the affected cells (Figure 2C).
The TME is another target for therapies (78), including treatments such as immunotherapies with targets such as CD-24 to interfere with tumour cell and TAM interactions (79). Drugs in clinical trials targeting CAFs are also being developed, including small molecules aimed at preventing CAF activation, reprogramming CAFs, interfering with CAF-mediated signalling, and additional CAF-related functions (80) (Figure 2D).
Targets such as the GLUT1 transporter have been identified in many studies developing drugs to target this metabolic process (81–85). Aside from glycolysis-targeting agents, drugs aimed at targeting fatty acid synthesis and oxidation, glutamine metabolism, nucleotide metabolism, and mitochondrial function are all being investigated (81) (Figure 2E).
Drug types such as PARP inhibitors, platinum compounds, alkylating agents, topoisomerase I/II inhibitors, and double-strand break inductors have all been developed to target DNA repair. These drugs function by either indirectly targeting DNA damage repair by causing more DNA damage than can be repaired or directly targeting DNA damage repair by interfering with necessary mechanisms for repair (86) (Figure 2F).
Some studies have already begun researching targeting non-coding RNAs to act as sensitisers for other treatments (87). Additionally, non-coding RNAs have also been identified as potential therapies which could be used to target various cancerous processes (88) (Figure 2G).
Due to their ability to carry molecules between cells, strong tumour tropism, low toxicity and immunogenicity, and ability to travel throughout the body, exosomes are being investigated not as targets for treatments but as potential carriers for therapies such as biomolecules and chemical agents (89–91) (Figure 2H).
With the prominence of histone deacetylase (HDAC) inhibitors such as Vorinostat (92), along with DNA methylation inhibitors (93), modification of epigenetics and gene expression of cancer cells is also becoming a more prevalent route for novel cancer-treating drugs (Figure 2I).
Targeting treatment towards autophagy can prove difficult due to its opposing roles in both cancer progression and inhibition, leading to the proposal of interventions for inhibiting and stimulating autophagy (94). Drugs such as chloroquine and hydroxychloroquine are autophagy-inhibiting drugs (94), whereas stimulation of autophagy can generally occur through cellular stress (94), with molecules such as the semisynthetic vitamin E derivative alpha-tocopheryloxyacetic acid stimulating tumour autophagy (95). Additionally, a study reviewing the regulation of autophagy in intracranial tumours describes repurposing antidepressants with autophagy-modulating functions to induce cytotoxic effects through the dysregulation of autophagy pathways (96) (Figure 2J).
3 Host-related factors
Aside from the tumour’s inherent factors modulating response to radiation treatment, the indiscriminate damaging nature of RT leads to factors related to the host influencing the treatment parameters that can be used. This can be seen through factors such as the volume effect, dose-limiting non-cancerous tissue, and additional pathophysiological factors.
3.1 Volume effect and dose limiting non-cancerous tissue
The volume effect describes the increase in acute and chronic side effects of RT as treatment volume increases, namely due to larger amounts of non-cancerous tissue being irradiated (97). This problem can be manifested differently in different tissues, with various toxicities observed based on surrounding tissues (98). The significance of the brain and all of its functions makes minimisation and avoidance of neural toxicities an important consideration when planning neurological RT treatments.
Toxicities in the brain following RT can range from acute toxicities, such as fatigue, to more severe toxicities, such as cognitive impairment, necrosis of CNS tissue and disruption of endocrine function (98). Differences in precision and dosages between SRS and WBRT ultimately translate into different manifestations of toxicities. Techniques such as hippocampal-avoidance WBRT (HA-WBRT) have been developed to reduce cognitive impairment in patients receiving WBRT (99). The efficacy of HA-WBRT as a cognition-sparing approach has however, been questioned, with some studies finding no significant benefit to cognition relative to conventional WBRT (99). WBRT doses tend to range between 20 and 40 Gy, delivered in 5 to 20 fractions, with relatively lower precision (100), whereas SRS delivers high precision (accuracy of 1mm) and high radiation dosage in a single treatment (101).
In a study with patients with 1-3 IMD lesions, randomised to either SRS + WBRT or SRS alone to test differences in cognitive deterioration between the groups, SRS alone had less cognitive decline following 3 months relative to SRS + WBRT (102). In a separate study on long-term outcomes of patients with brain metastases randomised to either receive SRS or WBRT, less cognitive deterioration among long-term survivors was observed in those received SRS relative to those who received WBRT, with clinical meaningfulness with the association of late cognitive deterioration and WBRT (103).
Due to the different levels of precision and, ultimately, toxicities caused by these different treatments, dose limitations are placed to protect the surrounding tissue. Although doses being delivered are protective to surrounding tissue, if a tumour is presenting as radioresistant, these protective doses are likely insufficient to damage the tumour.
3.2 Pathophysiological factors
It has been well characterised how comorbidities can influence outcomes in cancer patients (104). Radioresistance of cancer is an additional outcome which can be influenced by comorbidities/pathophysiological factors such as anaemia and age (6). Examples include how patients with Fanconi Anemia tend to be relatively radiosensitive due to disruptions in DNA damage repair (105), and how elderly patients (both healthy and cancer patients) tend to be more radiosensitive in general (106).
3.3 Exosomes
Exosome transfer from stromal cells to breast cancer cells has been identified, with RNA within these exosomes modulated radioresistance within the breast cancer cells by regulating the STAT1/NOTCH3 pathway (107). Additionally, the microRNA miR-208a, which can be transported by exosomes, can modulate radiosensitivity and proliferation of lung cancer cells through targeting p21 and altering the AKT/mTOR pathway (108).
4 Technical factors
There are several considerations with respect to technical factors that can impact how we interpret outcomes. For example, doses can be prescribed in various ways, leading to homogeneous or inhomogeneous dose distributions, and there can be variation in the definition of the gross target volume (GTV), clinical target volume (CTV) and planning target volume (PTV). Even the technology used can impact biological effects on the tumour; for example, a study has found transcriptomic differences in SRS-treated oligo brain metastases between those receiving treatment through Gamma-knife (GK) and linear accelerator (LINAC) modalities (109). Ultimately what is most critical is that the tumour is appropriately visualised and targeted.
Current treatment planning for RT requires physicians to determine and contour the area to be treated based on their treatment planning computed tomography (CT) scan, which is typically fused to a magnetic resonance image (MRI). However, multimodal images are often used to accurately determine the GTV, and these can include positron emission tomography (PET) scans. In treating brain metastases, it is generally accepted that the MRI used for treatment planning should not be older than 7 to 14 days by the time of the first treatment delivery in order to reduce the risk of geographic miss, and the geometric fidelity has to be checked as that alone can lead to targeting errors (110). With technical advances in radiotherapy systems, real-time imaging is increasingly being demanded with MRI to verify the position of the target before each fraction. The MR LINAC represents a technical feat such that a MRI and a LINAC are combined, allowing for such verification and real-time on line adaptive radiotherapy (111, 112). This technology is relevant in the brain, as Tan reported on significant dynamics in the surgical cavity CTV for brain metastases treated during a 5-day course of hypofractionated stereotactic RT (113). Furthermore, there is increasing evidence of dynamic changes for high-grade glioma during a course of treatment that could otherwise result in geographic miss should the tumour not be visualised when applying small margins (114, 115).
5 Probabilistic factors
Due to DNA being the target of RT, the gravity of the damage incurred is associated with a level of probability. Additionally, although highly related to tumour kinetics, the stage of the cell cycle of any given cell within a tumour is another matter of probability.
5.1 Random gravity of DNA damage
RT is an untargeted treatment type, with no mechanism available to target the sections of DNA to be damaged. Because of this, probability dictates if sections being damaged are genes essential for cell survival or clinically irrelevant regions.
5.2 Cell cycle
Through differences in sister chromatid proximity and DNA damage repair pathways active at different stages in the cell cycle, discrepancies in radioresistance occur in a stage-dependent manner (10). Generally, the late S phase and G2 phase are radioresistant due to the proximity of sister chromatids during these phases, allowing for the less error-prone DNA damage repair mechanism of homologous recombination (10). Asynchronous cell cycles provide another layer of tumour heterogeneity, which can lead to additional discrepancies in RT response within the tumour (5).
6 Summary of radioresistance in brain metastases
Akin to how additional hallmarks of cancer had been identified since Douglas Hanahan and Robert A. Weinberg’s initial work (116), the same can be said for mechanisms of radioresistance, with many new potential mechanisms for radioresistance being identified since Peters et al.’s keynote address. Across the four categories of tumour-related factors, host-related factors, technical factors, and probabilistic factors, 19 general mechanisms for radioresistance have been identified. These include hypoxia, clonogenic/cancer stem cells, tumour kinetics, the tumour microenvironment, metabolic alterations, tumour heterogeneity, DNA damage repair, non-coding RNAs, exosomes, methylomes and autophagy as tumour-related factors; pathophysiological factors, and the volume effect and dose limiting-non-cancerous tissue as the host related factors; the machine used, technique applied and planning strategy as the technical factors; and random gravity of DNA damage and the cell cycle as probabilistic factors. Due to the broad spectrum of each mechanism, although therapeutic approaches have been identified to target many of these factors, further investigation on their application to promote radiosensitisation will be necessary. In the context of brain metastases, these general mechanisms are relevant and play potential roles in observable radioresistance. With the variety of primary cancers that may lead to brain metastases, influential differences between primary cancer types may result in significant differences in some of these mechanisms, ultimately leading to different levels of radioresistance across brain metastases between patients. With a wide variety of these mechanisms and their application to brain metastases manifesting from different primary cancer types, studies investigating these mechanisms in brain metastases with the context of their cancer type of origin would further our understanding of radioresistance in brain metastases.
Author contributions
AY: Conceptualization, Investigation, Writing – original draft, Writing – review & editing. AS: Investigation, Validation, Writing – original draft, Writing – review & editing. SD: Conceptualization, Investigation, Supervision, Validation, Writing – original draft, Writing – review & editing.
Funding
The author(s) declare financial support was received for the research, authorship, and/or publication of this article. SD received funding from the Keenan Chair in Surgery.
Acknowledgments
Figure 2 was created in BioRender.
Conflict of interest
SD served as provincial lead for CNS Cancers at Ontario Health Cancer Care Ontario. SD was on the advisory board of the Subcortical Surgery Group. SD received research funding from Synaptive and VPIX Medical. SD receives royalties from Oxford University Press. SD was a speaker for the American Association of Neurological Surgeons and Congress of Neurological Surgeons. AS: Elekta/Elekta AB: Research grant, Consultant, Honorarium for past educational seminars, Travel Expenses. Varian: Honorarium for past educational seminars. BrainLab: Research grant, Consultant, Honorarium for educational seminars, Travel Expenses. AstraZeneca: Honorarium for educational seminars. ISRS: President of the International Stereotactic Radiosurgery Society ISRS. Seagen Inc: Honorarium for education seminars and research grants. Cerapedics: Honorarium for educational seminars, Travel Expenses. CarboFIX: Honorarium for educational seminars. Servier: Honorarium for educational seminars.
The remaining author declares that the research was conducted in the absence of any commercial or financial relationships that could be construed as a potential conflict of interest.
The author(s) declared that they were an editorial board member of Frontiers, at the time of submission. This had no impact on the peer review process and the final decision.
Publisher’s note
All claims expressed in this article are solely those of the authors and do not necessarily represent those of their affiliated organizations, or those of the publisher, the editors and the reviewers. Any product that may be evaluated in this article, or claim that may be made by its manufacturer, is not guaranteed or endorsed by the publisher.
References
1. Gállego Pérez-Larraya J, Hildebrand J. Chapter 77 - Brain metastases††This chapter is dedicated to the memory of Professor Jerzy Hildebrand, in: Handbook of clinical neurology (2014). Available online at: https://www.sciencedirect.com/science/article/pii/B9780702040887000778 (Accessed 2024 Jan 15).
2. Cagney DN, Martin AM, Catalano PJ, Redig AJ, Lin NU, Lee EQ, et al. Incidence and prognosis of patients with brain metastases at diagnosis of systemic Malignancy: a population-based study. Neuro Oncol. (2017) 19:1511–21. doi: 10.1093/neuonc/nox077
3. Ostrom QT, Wright CH, Barnholtz-Sloan JS. Chapter 2 - Brain metastases: epidemiology, in: Handbook of clinical neurology (2018). Elsevier. Available online at: https://www.sciencedirect.com/science/article/pii/B9780128111611000025 (Accessed 2024 Jan 15).
4. Matzenauer M, Vrana D, Melichar B. Treatment of brain metastases. Biomed Papers. (2016) 160:484–90. doi: 10.5507/bp.2016.058
5. Hall EJ, Giaccia AJ. Radiobiology for the radiologist. 8th edition. Wolters Kluwer: Philadelphia Baltimore New York London Buenos Aires (2019). p. 597.
6. Peters LJ, Withers HR, Thames HD, Fletcher GH. Keynote address—the problem: Tumor radioresistance in clinical radiotherapy. Int J Radiat Oncology Biology Physics. (1982) 8:101–8. doi: 10.1016/0360-3016(82)90392-3
7. Ni J, Bucci J, Malouf D, Knox M, Graham P, Li Y. Exosomes in cancer radioresistance. Front Oncol. (2019) 9:869. doi: 10.3389/fonc.2019.00869
8. Ali MY, Oliva CR, Noman ASM, Allen BG, Goswami PC, Zakharia Y, et al. Radioresistance in glioblastoma and the development of radiosensitizers. Cancers (Basel). (2020) 12:2511. doi: 10.3390/cancers12092511
9. Taylor MA, Das BC, Ray SK. Targeting autophagy for combating chemoresistance and radioresistance in glioblastoma. Apoptosis. (2018) 23:563–75. doi: 10.1007/s10495-018-1480-9
10. Goto Y, Koyasu S, Kobayashi M, Harada H. The emerging roles of the ubiquitination/deubiquitination system in tumor radioresistance regarding DNA damage responses, cell cycle regulation, hypoxic responses, and antioxidant properties: Insight into the development of novel radiosensitizing strategies. Mutat Research/Fundamental Mol Mech Mutagenesis. (2017) 803–805:76–81. doi: 10.1016/j.mrfmmm.2017.07.007
11. Kabakov AE, Yakimova AO. Hypoxia-induced cancer cell responses driving radioresistance of hypoxic tumors: approaches to targeting and radiosensitizing. Cancers (Basel). (2021) 13:1102. doi: 10.3390/cancers13051102
12. Corroyer-Dulmont A, Valable S, Fantin J, Chatre L, Toutain J, Teulier S, et al. Multimodal evaluation of hypoxia in brain metastases of lung cancer and interest of hypoxia image-guided radiotherapy. Sci Rep. (2021) 11:11239. doi: 10.1038/s41598-021-90662-0
13. Suwa T, Kobayashi M, Nam JM, Harada H. Tumor microenvironment and radioresistance. Exp Mol Med. (2021) 53:1029–35. doi: 10.1038/s12276-021-00640-9
14. Marie-Egyptienne DT, Lohse I, Hill RP. Cancer stem cells, the epithelial to mesenchymal transition (EMT) and radioresistance: Potential role of hypoxia. Cancer Letters. (2013) 341:63–72. doi: 10.1016/j.canlet.2012.11.019
15. Kelley K, Knisely J, Symons M, Ruggieri R. Radioresistance of brain tumors. Cancers (Basel). (2016) 8:42. doi: 10.3390/cancers8040042
16. Nassar D, Blanpain C. Cancer stem cells: basic concepts and therapeutic implications. Annu Rev Pathol Mech Dis. (2016) 11:47–76. doi: 10.1146/annurev-pathol-012615-044438
17. Schulz A, Meyer F, Dubrovska A, Borgmann K. Cancer stem cells and radioresistance: DNA repair and beyond. Cancers (Basel). (2019) 11:862. doi: 10.3390/cancers11060862
18. Rycaj K, Tang DG. Cancer stem cells and radioresistance. Int J Radiat Biol. (2014) 90:615–21. doi: 10.3109/09553002.2014.892227
19. Chang L, Graham P, Hao J, Ni J, Deng J, Bucci J, et al. Cancer stem cells and signaling pathways in radioresistance. Oncotarget. (2015) 7:11002–17. doi: 10.18632/oncotarget.v7i10
20. Park SY, Lee CJ, Choi JH, Kim JH, Kim JW, Kim JY, et al. The JAK2/STAT3/CCND2 Axis promotes colorectal Cancer stem cell persistence and radioresistance. J Exp Clin Cancer Res. (2019) 38:399. doi: 10.1186/s13046-019-1405-7
21. Tubiana M. Tumor cell proliferation kinetics and tumor growth rate. Acta Oncologica. (1989) 28:113–21. doi: 10.3109/02841868909111193
22. Nicholls LW, Pinkham MB, Bernard A, Lusk R, Watkins T, Hall B, et al. Radiological kinetics of brain metastases and clinical implications for patients treated with stereotactic radiosurgery. Clin Oncol. (2019) 31:34–40. doi: 10.1016/j.clon.2018.09.005
23. Madamesila J, Ploquin N, Faruqi S, Tchistiakova E. Investigating diffusion patterns of brain metastases pre- and post-stereotactic radiosurgery: a feasibility study. BioMed Phys Eng Express. (2021) 7:065004. doi: 10.1088/2057-1976/ac1d89
24. Lee SF, Yip PL, Chan OL, Lee VWY, Wong A, Choi HCW. Brain metastasis growth kinetics: A novel prognosticator for stereotactic radiotherapy. Clin Oncol. (2023) 35:e328–35. doi: 10.1016/j.clon.2023.02.012
25. Fu T, Dai LJ, Wu SY, Xiao Y, Ma D, Jiang YZ, et al. Spatial architecture of the immune microenvironment orchestrates tumor immunity and therapeutic response. J Hematol Oncol. (2021) 14:98. doi: 10.1186/s13045-021-01103-4
26. Jarosz-Biej M, Smolarczyk R, Cichoń T, Kułach N. Tumor microenvironment as A “Game changer” in cancer radiotherapy. Int J Mol Sci. (2019) 20:3212. doi: 10.3390/ijms20133212
27. Cook PJ, Thomas R, Kingsley PJ, Shimizu F, Montrose DC, Marnett LJ, et al. Cox-2-derived PGE2 induces Id1-dependent radiation resistance and self-renewal in experimental glioblastoma. Neuro Oncol. (2016) 18:1379–89. doi: 10.1093/neuonc/now049
28. Meng Y, Beckett MA, Liang H, Mauceri HJ, van Rooijen N, Cohen KS, et al. Blockade of TNFα signaling in tumor-associated macrophages: a new radiosensitizing strategy. Cancer Res. (2010) 70:1534–43. doi: 10.1158/0008-5472.CAN-09-2995
29. Chu TY, Yang JT, Huang TH, Liu HW. Crosstalk with cancer-associated fibroblasts increases the growth and radiation survival of cervical cancer cells. rare. (2014) 181:540–7. doi: 10.1667/RR13583.1
30. Barker HE, Paget JTE, Khan AA, Harrington KJ. The tumour microenvironment after radiotherapy: mechanisms of resistance and recurrence. Nat Rev Cancer. (2015) 15:409–25. doi: 10.1038/nrc3958
31. Wang Y, Chen R, Wa Y, Ding S, Yang Y, Liao J, et al. Tumor immune microenvironment and immunotherapy in brain metastasis from non-small cell lung cancer. Front Immunol. (2022) 13:829451. doi: 10.3389/fimmu.2022.829451
32. Quail DF, Joyce JA. The microenvironmental landscape of brain tumors. Cancer Cell. (2017) 31:326–41. doi: 10.1016/j.ccell.2017.02.009
33. Zhang L, Zhang S, Yao J, Lowery FJ, Zhang Q, Huang WC, et al. Microenvironment-induced PTEN loss by exosomal microRNA primes brain metastasis outgrowth. Nature. (2015) 527:100–4. doi: 10.1038/nature15376
34. Gonzalez H, Mei W, Robles I, Hagerling C, Allen BM, Okholm TLH, et al. Cellular architecture of human brain metastases. Cell. (2022) 185:729–45. doi: 10.1016/j.cell.2021.12.043
35. Tang L, Wei F, Wu Y, He Y, Shi L, Xiong F, et al. Role of metabolism in cancer cell radioresistance and radiosensitization methods. J Exp Clin Cancer Res. (2018) 37:87. doi: 10.1186/s13046-018-0758-7
36. Kunkel M, Moergel M, Stockinger M, Jeong JH, Fritz G, Lehr HA, et al. Overexpression of GLUT-1 is associated with resistance to radiotherapy and adverse prognosis in squamous cell carcinoma of the oral cavity. Oral Oncol. (2007) 43:796–803. doi: 10.1016/j.oraloncology.2006.10.009
37. Chung FY, Huang MY, Yeh CS, Chang HJ, Cheng TL, Yen LC, et al. GLUT1 gene is a potential hypoxic marker in colorectal cancer patients. BMC Cancer. (2009) 9:241. doi: 10.1186/1471-2407-9-241
38. Wang C, Zheng W, Yao D, Chen Q, Zhu L, Zhang J, et al. Upregulation of DNA metabolism-related genes contributes to radioresistance of glioblastoma. Hum Gene Ther Clin Dev. (2019) 30:74–87. doi: 10.1089/humc.2018.251
39. An D, Zhai D, Wan C, Yang K. The role of lipid metabolism in cancer radioresistance. Clin Transl Oncol. (2023) 25:2332–49. doi: 10.1007/s12094-023-03134-4
40. Mukha A, Kahya U, Dubrovska A. Targeting glutamine metabolism and autophagy: the combination for prostate cancer radiosensitization. Autophagy. (2021) 17:3879–81. doi: 10.1080/15548627.2021.1962682
41. Tyagi A, Wu SY, Watabe K. Metabolism in the progression and metastasis of brain tumors. Cancer Lett. (2022) 539:215713. doi: 10.1016/j.canlet.2022.215713
42. Chen EI, Hewel J, Krueger JS, Tiraby C, Weber MR, Kralli A, et al. Adaptation of energy metabolism in breast cancer brain metastases. Cancer Res. (2007) 67:1472–86. doi: 10.1158/0008-5472.CAN-06-3137
43. Chen J, Lee HJ, Wu X, Huo L, Kim SJ, Xu L, et al. Gain of glucose-independent growth upon metastasis of breast cancer cells to the brain. Cancer Res. (2015) 75:554–65. doi: 10.1158/0008-5472.CAN-14-2268
44. Cordero A, Kanojia D, Miska J, Panek WK, Xiao A, Han Y, et al. FABP7 is a key metabolic regulator in HER2+ breast cancer brain metastasis. Oncogene. (2019) 38:6445–60. doi: 10.1038/s41388-019-0893-4
45. Ou A, Yung WKA, Majd N. Molecular mechanisms of treatment resistance in glioblastoma. Int J Mol Sci. (2020) 22:351. doi: 10.3390/ijms22010351
46. Olivares-Urbano MA, Griñán-Lisón C, Marchal JA, Núñez MI. CSC radioresistance: A therapeutic challenge to improve radiotherapy effectiveness in cancer. Cells. (2020) 9:1651. doi: 10.3390/cells9071651
47. Zou Y, Ye F, Kong Y, Hu X, Deng X, Xie J, et al. The single-cell landscape of intratumoral heterogeneity and the immunosuppressive microenvironment in liver and brain metastases of breast cancer. Adv Sci (Weinh). (2022) 10:2203699. doi: 10.1002/advs.202203699
48. Brastianos PK, Carter SL, Santagata S, Cahill DP, Taylor-Weiner A, Jones RT, et al. Genomic characterization of brain metastases reveals branched evolution and potential therapeutic targets. Cancer Discovery. (2015) 5:1164–77. doi: 10.1158/2159-8290.CD-15-0369
49. Huang RX, Zhou PK, damage response signaling pathways DNA. and targets for radiotherapy sensitization in cancer. Signal Transduct Target Ther. (2020) 5:60. doi: 10.1038/s41392-020-0150-x
50. Woditschka S, Evans L, Duchnowska R, Reed LT, Palmieri D, Qian Y, et al. DNA double-strand break repair genes and oxidative damage in brain metastasis of breast cancer. J Natl Cancer Inst. (2014) 106:dju145. doi: 10.1093/jnci/dju145
51. Sun J, Wang C, Zhang Y, Xu L, Fang W, Zhu Y, et al. Genomic signatures reveal DNA damage response deficiency in colorectal cancer brain metastases. Nat Commun. (2019) 10:3190. doi: 10.1038/s41467-019-10987-3
52. Ahmad P, Sana J, Slavik M, Slampa P, Smilek P, Slaby O. MicroRNAs involvement in radioresistance of head and neck cancer. Dis Markers. (2017) 2017:8245345. doi: 10.1155/2017/8245345
53. Fang Y, Fullwood MJ. Roles, functions, and mechanisms of long non-coding RNAs in cancer. Genomics Proteomics Bioinf. (2016) 14:42–54. doi: 10.1016/j.gpb.2015.09.006
54. Li J, Tian H, Yang J, Gong Z. Long noncoding RNAs regulate cell growth, proliferation, and apoptosis. DNA Cell Biol. (2016) 35:459–70. doi: 10.1089/dna.2015.3187
55. Xie Y, Han J, Xie K, Gou Q. LncRNAs as biomarkers for predicting radioresistance and survival in cancer: a meta-analysis. Sci Rep. (2022) 12:18494. doi: 10.1038/s41598-022-21785-1
56. Fu B, Liu W, Zhu C, Li P, Wang L, Pan L, et al. Circular RNA circBCBM1 promotes breast cancer brain metastasis by modulating miR-125a/BRD4 axis. Int J Biol Sci. (2021) 17:3104–17. doi: 10.7150/ijbs.58916
57. Moskwa P, Zinn PO, Choi YE, Shukla SA, Fendler W, Chen CC, et al. A functional screen identifies miRs that induce radioresistance in glioblastomas. Mol Cancer Res. (2014) 12:1767–78. doi: 10.1158/1541-7786.MCR-14-0268
58. Tsakonas G, Koulouris A, Kazmierczak D, Botling J, Ortiz-Villalon C, Nord H, et al. Matched Analyses of Brain Metastases versus Primary Non-Small Cell Lung Cancer Reveal a Unique microRNA Signature. Int J Mol Sci. (2022) 24:193. doi: 10.3390/ijms24010193
59. Deng X, Ma L, Wu M, Zhang G, Jin C, Guo Y, et al. miR-124 radiosensitizes human glioma cells by targeting CDK4. J Neurooncol. (2013) 114:263–74. doi: 10.1007/s11060-013-1179-2
60. Arscott WT, Tandle AT, Zhao S, Shabason JE, Gordon IK, Schlaff CD, et al. Ionizing radiation and glioblastoma exosomes: implications in tumor biology and cell migration. Transl Oncol. (2013) 6:638–48. doi: 10.1593/tlo.13640
61. Grange C, Tapparo M, Collino F, Vitillo L, Damasco C, Deregibus MC, et al. Microvesicles released from human renal cancer stem cells stimulate angiogenesis and formation of lung premetastatic niche. Cancer Res. (2011) 71:5346–56. doi: 10.1158/0008-5472.CAN-11-0241
62. Yang Z, Zhong W, Yang L, Wen P, Luo Y, Wu C. The emerging role of exosomes in radiotherapy. Cell Commun Signal. (2022) 20:171. doi: 10.1186/s12964-022-00986-1
63. Lehmann BD, Paine MS, Brooks AM, McCubrey JA, Renegar RH, Wang R, et al. Senescence-associated exosome release from human prostate cancer cells. Cancer Res. (2008) 68:7864–71. doi: 10.1158/0008-5472.CAN-07–6538
64. Lespagnol A, Duflaut D, Beekman C, Blanc L, Fiucci G, Marine JC, et al. Exosome secretion, including the DNA damage-induced p53-dependent secretory pathway, is severely compromised in TSAP6/Steap3-null mice. Cell Death Differ. (2008) 15:1723–33. doi: 10.1038/cdd.2008.104
65. Zhou W, Fong MY, Min Y, Somlo G, Liu L, Palomares MR, et al. Cancer-secreted miR-105 destroys vascular endothelial barriers to promote metastasis. Cancer Cell. (2014) 25:501–15. doi: 10.1016/j.ccr.2014.03.007
66. Wei L, Wang G, Yang C, Zhang Y, Chen Y, Zhong C, et al. MicroRNA-550a-3-5p controls the brain metastasis of lung cancer by directly targeting YAP1. Cancer Cell Int. (2021) 21:491. doi: 10.1186/s12935-021-02197-z
67. Chen GY, Cheng JCH, Chen YF, Yang JCH, Hsu FM. Circulating exosomal integrin β3 is associated with intracranial failure and survival in lung cancer patients receiving cranial irradiation for brain metastases: A prospective observational study. Cancers (Basel). (2021) 13:380. doi: 10.3390/cancers13030380
68. Cabrera-Licona A, Pérez-Añorve IX, Flores-Fortis M, del Moral-Hernández O, González-dela RCH, Suárez-Sánchez R, et al. Deciphering the epigenetic network in cancer radioresistance. Radiotherapy Oncol. (2021) 159:48–59. doi: 10.1016/j.radonc.2021.03.012
69. Kim EH, Park AK, Dong SM, Ahn JH, Park WY. Global analysis of CpG methylation reveals epigenetic control of the radiosensitivity in lung cancer cell lines. Oncogene. (2010) 29:4725–31. doi: 10.1038/onc.2010.223
70. Lin HY, Hung SK, Lee MS, Chiou WY, Huang TT, Tseng CE, et al. DNA methylome analysis identifies epigenetic silencing of FHIT as a determining factor for radiosensitivity in oral cancer: an outcome-predicting and treatment-implicating study. Oncotarget. (2015) 6:915–34. doi: 10.18632/oncotarget.2821
71. Chang G, Shi L, Ye Y, Shi H, Zeng L, Tiwary S, et al. YTHDF3 induces the translation of m6A-enriched gene transcripts to promote breast cancer brain metastasis. Cancer Cell. (2020) 38:857–871.e7. doi: 10.1016/j.ccell.2020.10.004
72. Gallon J, Rodriguez-Calero A, Benjak A, Akhoundova D, Maletti S, Amstutz U, et al. DNA methylation landscapes of prostate cancer brain metastasis are shaped by early driver genetic alterations. Cancer Res. (2023) 83:1203–13. doi: 10.1158/0008-5472.CAN-22-2236
73. Santana-Codina N, Muixí L, Foj R, Sanz-Pamplona R, Badia-Villanueva M, Abramowicz A, et al. GRP94 promotes brain metastasis by engaging pro-survival autophagy. Neuro Oncol. (2020) 22:652–64. doi: 10.1093/neuonc/noz198
74. Graham K, Unger E. Overcoming tumor hypoxia as a barrier to radiotherapy, chemotherapy and immunotherapy in cancer treatment. Int J Nanomedicine. (2018) 13:6049–58. doi: 10.2147/IJN.S140462
75. Albadari N, Deng S, Li W. The transcriptional factors HIF-1 and HIF-2 and their novel inhibitors in cancer therapy. Expert Opin Drug Discovery. (2019) 14:667–82. doi: 10.1080/17460441.2019.1613370
76. Krause M, Dubrovska A, Linge A, Baumann M. Cancer stem cells: Radioresistance, prediction of radiotherapy outcome and specific targets for combined treatments. Advanced Drug Delivery Rev. (2017) 109:63–73. doi: 10.1016/j.addr.2016.02.002
77. Babiker HM, McBride A, Cooke LS, Mahadevan D. Therapeutic potential of investigational CHK-1 inhibitors for the treatment of solid tumors. Expert Opin Investigational Drugs. (2017) 26:1063–72. doi: 10.1080/13543784.2017.1360275
78. Yang M, Li J, Gu P, Fan X. The application of nanoparticles in cancer immunotherapy: Targeting tumor microenvironment. Bioact Mater. (2020) 6:1973–87. doi: 10.1016/j.bioactmat.2020.12.010
79. Li C, Xu X, Wei S, Jiang P, Xue L, Wang J. Tumor-associated macrophages: potential therapeutic strategies and future prospects in cancer. J Immunother Cancer. (2021) 9:e001341. doi: 10.1136/jitc-2020-001341
80. Hu D, Li Z, Zheng B, Lin X, Pan Y, Gong P, et al. Cancer-associated fibroblasts in breast cancer: Challenges and opportunities. Cancer Commun (Lond). (2022) 42:401–34. doi: 10.1002/cac2.12291
81. Stine ZE, Schug ZT, Salvino JM, Dang CV. Targeting cancer metabolism in the era of precision oncology. Nat Rev Drug Discovery. (2022) 21:141–62. doi: 10.1038/s41573-021-00339-6
82. Zhang C, Liu Z, Wang F, Zhang B, Zhang X, Guo P, et al. Nanomicelles for GLUT1-targeting hepatocellular carcinoma therapy based on NADPH depletion. Drug Deliv. (2022) 30:2162160. doi: 10.1080/10717544.2022.2162160
83. Ma P, Wei G, Chen J, Jing Z, Wang X, Wang Z. GLUT1 targeting and hypoxia-activating polymer-drug conjugate-based micelle for tumor chemo-thermal therapy. Drug Deliv. (2021) 28:2256–67. doi: 10.1080/10717544.2021.1992039
84. Meng Y, Xu X, Luan H, Li L, Dai W, Li Z, et al. The progress and development of GLUT1 inhibitors targeting cancer energy metabolism. Future Medicinal Chem. (2019) 11:2333–52. doi: 10.4155/fmc-2019-0052
85. Shen S, Du M, Liu Q, Gao P, Wang J, Liu S, et al. Development of GLUT1-targeting alkyl glucoside-modified dihydroartemisinin liposomes for cancer therapy. Nanoscale. (2020) 12:21901–12. doi: 10.1039/D0NR05138A
86. Huang R, Zhou PK. DNA damage repair: historical perspectives, mechanistic pathways and clinical translation for targeted cancer therapy. Signal Transduct Target Ther. (2021) 6:254. doi: 10.1038/s41392-021-00648-7
87. Yang M, Schneekloth JS. Targeting non-coding RNA sensitizes cancer cells to drugs. Trends Pharmacol Sci. (2019) 40:447–8. doi: 10.1016/j.tips.2019.04.017
88. Kara G, Calin GA, Ozpolat B. RNAi-based therapeutics and tumor targeted delivery in cancer. Advanced Drug Delivery Rev. (2022) 182:114113. doi: 10.1016/j.addr.2022.114113
89. Lin Z, Wu Y, Xu Y, Li G, Li Z, Liu T. Mesenchymal stem cell-derived exosomes in cancer therapy resistance: recent advances and therapeutic potential. Mol Cancer. (2022) 21:179. doi: 10.1186/s12943-022-01650-5
90. Zhang M, Hu S, Liu L, Dang P, Liu Y, Sun Z, et al. Engineered exosomes from different sources for cancer-targeted therapy. Signal Transduct Target Ther. (2023) 8:124. doi: 10.1038/s41392-023-01382-y
91. Wang Y, Jiang M, Zheng X, He Y, Ma X, Li J, et al. Application of exosome engineering modification in targeted delivery of therapeutic drugs. Biochem Pharmacol. (2023) 215:115691. doi: 10.1016/j.bcp.2023.115691
92. McClure JJ, Li X, Chou CJ. Chapter six - advances and challenges of HDAC inhibitors in cancer therapeutics, in: Advances in cancer research (2018). Academic Press. Available online at: https://www.sciencedirect.com/science/article/pii/S0065230X18300150 (Accessed 2024 May 17).
93. Da Costa EM, McInnes G, Beaudry A, Raynal NJM. DNA methylation–targeted drugs. Cancer J. (2017) 23:270. doi: 10.1097/PPO.0000000000000278
94. Levy JMM, Towers CG, Thorburn A. Targeting autophagy in cancer. Nat Rev Cancer. (2017) 17:528–42. doi: 10.1038/nrc.2017.53
95. Li Y, Hahn T, Garrison K, Cui ZH, Thorburn A, Thorburn J, et al. The vitamin E analog alpha-TEA stimulates tumor autophagy and enhances antigen cross-presentation. Cancer Res. (2012) 72:3535–45. doi: 10.1158/0008-5472.CAN-11-3103
96. Petrosyan E, Fares J, Cordero A, Rashidi A, Arrieta VA, Kanojia D, et al. Repurposing autophagy regulators in brain tumors. Int J Cancer. (2022) 151:167–80. doi: 10.1002/ijc.v151.2
97. Hopewell JW, Trott KR. Volume effects in radiobiology as applied to radiotherapy. Radiotherapy Oncol. (2000) 56:283–8. doi: 10.1016/S0167-8140(00)00236-X
98. Wang K, Tepper JE. Radiation therapy-associated toxicity: Etiology, management, and prevention. CA: A Cancer J Clin. (2021) 71:437–54. doi: 10.3322/caac.21689
99. Shang W, Yao H, Sun Y, Mu A, Zhu L, Li X. Preventive effect of hippocampal sparing on cognitive dysfunction of patients undergoing whole-brain radiotherapy and imaging assessment of hippocampal volume changes. BioMed Res Int. (2022) 2022:4267673. doi: 10.1155/2022/4267673
100. Perlow HK, Dibs K, Liu K, Jiang W, Rajappa P, Blakaj DM, et al. Whole-brain radiation therapy versus stereotactic radiosurgery for cerebral metastases. Neurosurg Clinics North America. (2020) 31:565–73. doi: 10.1016/j.nec.2020.06.006
101. Yanagihara TK, Saadatmand HJ, Wang TJC. Reevaluating stereotactic radiosurgery for glioblastoma: new potential for targeted dose-escalation. J Neurooncol. (2016) 130:397–411. doi: 10.1007/s11060-016-2270-2
102. Brown PD, Jaeckle K, Ballman KV, Farace E, Cerhan JH, Anderson SK, et al. Effect of radiosurgery alone vs radiosurgery with whole brain radiation therapy on cognitive function in patients with 1 to 3 brain metastases. JAMA. (2016) 316:401–9. doi: 10.1001/jama.2016.9839
103. Palmer JD, Klamer BG, Ballman KV, Brown PD, Cerhan JH, Anderson SK, et al. Association of long-term outcomes with stereotactic radiosurgery vs whole-brain radiotherapy for resected brain metastasis. JAMA Oncol. (2022) 8:1809–15. doi: 10.1001/jamaoncol.2022.5049
104. Panigrahi G, Ambs S. How comorbidities shape cancer biology and survival. Trends Cancer. (2021) 7:488–95. doi: 10.1016/j.trecan.2020.12.010
105. Beddok A, Krieger S, Castera L, Stoppa-Lyonnet D, Thariat J. Management of Fanconi Anemia patients with head and neck carcinoma: Diagnosis and treatment adaptation. Oral Oncol. (2020) 108:104816. doi: 10.1016/j.oraloncology.2020.104816
106. Schuster B, Ellmann A, Mayo T, Auer J, Haas M, Hecht M, et al. Rate of individuals with clearly increased radiosensitivity rise with age both in healthy individuals and in cancer patients. BMC Geriatr. (2018) 18:105. doi: 10.1186/s12877-018-0799-y
107. Boelens MC, Wu TJ, Nabet BY, Xu B, Qiu Y, Yoon T, et al. Exosome transfer from stromal to breast cancer cells regulates therapy resistance pathways. Cell. (2014) 159:499–513. doi: 10.1016/j.cell.2014.09.051
108. Tang Y, Cui Y, Li Z, Jiao Z, Zhang Y, He Y, et al. Radiation-induced miR-208a increases the proliferation and radioresistance by targeting p21 in human lung cancer cells. J Exp Clin Cancer Res. (2016) 35:7. doi: 10.1186/s13046-016-0285-3
109. Shireman JM, White Q, Agrawal N, Ni Z, Chen G, Zhao L, et al. Genomic analysis of human brain metastases treated with stereotactic radiosurgery under the phase-II clinical trial (NCT03398694) reveals DNA damage repair at the peripheral tumor edge. medRxiv. (2023) ;2023:04.15.23288491. doi: 10.1093/neuonc/noad179.0294
110. Seymour ZA, Fogh SE, Westcott SK, Braunstein S, Larson DA, Barani IJ, et al. Interval from imaging to treatment delivery in the radiation surgery age: how long is too long. Int J Radiat OncologyBiologyPhysics. (2015) 93:126–32. doi: 10.1016/j.ijrobp.2015.05.001
111. Westerhoff JM, Daamen LA, Christodouleas JP, Blezer ELA, Choudhury A, Westley RL, et al. Safety and tolerability of online adaptive high-field magnetic resonance-guided radiotherapy. JAMA Netw Open. (2024) 7:e2410819. doi: 10.1001/jamanetworkopen.2024.10819
112. Tseng CL, Chen H, Stewart J, Lau AZ, Chan RW, Lawrence LSP, et al. High grade glioma radiation therapy on a high field 1.5 Tesla MR-Linac - workflow and initial experience with daily adapt-to-position (ATP) MR guidance: A first report. Front Oncol. (2022) 12:1060098. doi: 10.3389/fonc.2022.1060098
113. Tan H, Stewart J, Ruschin M, Wang MH, Myrehaug S, Tseng CL, et al. Inter-fraction dynamics during post-operative 5 fraction cavity hypofractionated stereotactic radiotherapy with a MR LINAC: a prospective serial imaging study. J Neurooncol. (2022) 156:569–77. doi: 10.1007/s11060-021-03938-w
114. Tseng CL, Zeng KL, Mellon EA, Soltys SG, Ruschin M, Lau AZ, et al. Evolving concepts in margin strategies and adaptive radiotherapy for glioblastoma: A new future is on the horizon. Neuro Oncol. (2024) 26:S3–16. doi: 10.1093/neuonc/noad258
115. Stewart J, Sahgal A, Lee Y, Soliman H, Tseng CL, Detsky J, et al. Quantitating interfraction target dynamics during concurrent chemoradiation for glioblastoma: A prospective serial imaging study. Int J Radiat Oncol Biol Phys. (2021) 109:736–46. doi: 10.1016/j.ijrobp.2020.10.002
116. Hanahan D. Hallmarks of cancer: new dimensions. Cancer Discovery. (2022) 12:31–46. doi: 10.1158/2159-8290.CD-21-1059
117. Li W, Guo F, Wang P, Hong S, Zhang C. miR-221/222 confers radioresistance in glioblastoma cells through activating akt independent of PTEN status. Curr Mol Med. (2014) 14:185–95. doi: 10.2174/1566524013666131203103147
118. Zhou Y, Shao Y, Hu W, Zhang J, Shi Y, Kong X, et al. A novel long noncoding RNA SP100-AS1 induces radioresistance of colorectal cancer via sponging miR-622 and stabilizing ATG3. Cell Death Differ. (2023) 30:111–24. doi: 10.1038/s41418-022-01049-1
119. Liu J, Xue N, Guo Y, Niu K, Gao L, Zhang S, et al. CircRNA_100367 regulated the radiation sensitivity of esophageal squamous cell carcinomas through miR-217/Wnt3 pathway. Aging (Albany NY). (2019) 11:12412–27. doi: 10.18632/aging.102580
120. Chuang PC, Chen PT, Wang CC, Su WH, Chen YH, Huang EY. MicroRNA-29a manifests multifaceted features to intensify radiosensitivity, escalate apoptosis, and revoke cell migration for palliating radioresistance-enhanced cervical cancer progression. Int J Mol Sci. (2022) 23:5524. doi: 10.3390/ijms23105524
121. Wu W, Chen X, Yu S, Wang R, Zhao R, Du C. microRNA-222 promotes tumor growth and confers radioresistance in nasopharyngeal carcinoma by targeting PTEN. Mol Med Rep. (2018) 17:1305–10. doi: 10.3892/mmr.2017.7931
122. Shiiba M, Shinozuka K, Saito K, Fushimi K, Kasamatsu A, Ogawara K, et al. MicroRNA-125b regulates proliferation and radioresistance of oral squamous cell carcinoma. Br J Cancer. (2013) 108:1817–21. doi: 10.1038/bjc.2013.175
123. Lu Y, Li T, Wei G, Liu L, Chen Q, Xu L, et al. The long non-coding RNA NEAT1 regulates epithelial to mesenchymal transition and radioresistance in through miR-204/ZEB1 axis in nasopharyngeal carcinoma. Tumor Biol. (2016) 37:11733–41. doi: 10.1007/s13277-015-4773-4
124. Tang T, Wang LX, Yang ML, Zhang RM. lncRNA TPTEP1 inhibits stemness and radioresistance of glioma through miR-106a-5p-mediated P38 MAPK signaling. Mol Med Rep. (2020) 22:4857–67. doi: 10.3892/mmr.2020.11542
125. Guo Z, Wang YH, Xu H, Yuan CS, Zhou HH, Huang WH, et al. LncRNA linc00312 suppresses radiotherapy resistance by targeting DNA-PKcs and impairing DNA damage repair in nasopharyngeal carcinoma. Cell Death Dis. (2021) 12:69. doi: 10.1038/s41419-020-03302-2
126. Han YY, Liu K, Xie J, Li F, Wang Y, Yan B. LINC00114 promoted nasopharyngeal carcinoma progression and radioresistance in vitro and in vivo through regulating ERK/JNK signaling pathway via targeting miR-203. Eur Rev Med Pharmacol Sci. (2020) 24:2491–504. doi: 10.1038/bjc.2013.175
127. Liu Y, Chen X, Chen X, Liu J, Gu H, Fan R, et al. Long non-coding RNA HOTAIR knockdown enhances radiosensitivity through regulating microRNA-93/ATG12 axis in colorectal cancer. Cell Death Dis. (2020) 11:175. doi: 10.1038/s41419-020-2268-8
128. Chen W, Zhang Y, Wang H, Pan T, Zhang Y, Li C. LINC00473/miR-374a-5p regulates esophageal squamous cell carcinoma via targeting SPIN1 to weaken the effect of radiotherapy. J Cell Biochem. (2019) 120:14562–72. doi: 10.1002/jcb.v120.9
129. Liu AM, Zhu Y, Huang ZW, Lei L, Fu SZ, Chen Y. Long noncoding RNA FAM201A involves in radioresistance of non-small-cell lung cancer by enhancing EGFR expression via miR-370. Eur Rev Med Pharmacol Sci. (2019) 23:5802–14. doi: 10.26355/eurrev_201907_18319
130. Jin C, Yan B, Lu Q, Lin Y, Ma L. The role of MALAT1/miR-1/slug axis on radioresistance in nasopharyngeal carcinoma. Tumor Biol. (2016) 37:4025–33. doi: 10.1007/s13277-015-4227-z
131. Zheng J, Wang B, Zheng R, Zhang J, Huang C, Zheng R, et al. Linc-RA1 inhibits autophagy and promotes radioresistance by preventing H2Bub1/USP44 combination in glioma cells. Cell Death Dis. (2020) 11:758. doi: 10.1038/s41419-020-02977-x
132. Yang T, Li S, Liu J, Yin D, Yang X, Tang Q. lncRNA-NKILA/NF-κB feedback loop modulates laryngeal cancer cell proliferation, invasion, and radioresistance. Cancer Med. (2018) 7:2048–63. doi: 10.1002/cam4.2018.7.issue-5
133. Zhao H, Zheng GH, Li GC, Xin L, Wang YS, Chen Y, et al. Long noncoding RNA LINC00958 regulates cell sensitivity to radiotherapy through RRM2 by binding to microRNA-5095 in cervical cancer. J Cell Physiol. (2019) 234:23349–59. doi: 10.1002/jcp.v234.12
134. Wang M, Wang L, He X, Zhang J, Zhu Z, Zhang M, et al. lncRNA CCAT2 promotes radiotherapy resistance for human esophageal carcinoma cells via the miR−145/p70S6K1 and p53 pathway. Int J Oncol. (2020) 56:327–36. doi: 10.3892/ijo.2019.4929
135. Wang B, Zheng J, Li R, Tian Y, Lin J, Liang Y, et al. Long noncoding RNA LINC02582 acts downstream of miR-200c to promote radioresistance through CHK1 in breast cancer cells. Cell Death Dis. (2019) 10:764. doi: 10.1038/s41419-019-1996-0
136. Han PB, Ji XJ, Zhang M, Gao LY. Upregulation of lncRNA LINC00473 promotes radioresistance of HNSCC cells through activating Wnt/β-catenin signaling pathway. Eur Rev Med Pharmacol Sci. (2018) 22:7305–13. doi: 10.26355/eurrev_201811_16267
Keywords: radiotherapy, cancer, brain, metastases, radioresistance
Citation: Youssef A, Sahgal A and Das S (2024) Radioresistance and brain metastases: a review of the literature and applied perspective. Front. Oncol. 14:1477448. doi: 10.3389/fonc.2024.1477448
Received: 08 August 2024; Accepted: 09 October 2024;
Published: 30 October 2024.
Edited by:
Ira Ida Skvortsova, Innsbruck Medical University, AustriaReviewed by:
Connor Kinslow, Columbia University, United StatesSatoru Osuka, University of Alabama at Birmingham, United States
Copyright © 2024 Youssef, Sahgal and Das. This is an open-access article distributed under the terms of the Creative Commons Attribution License (CC BY). The use, distribution or reproduction in other forums is permitted, provided the original author(s) and the copyright owner(s) are credited and that the original publication in this journal is cited, in accordance with accepted academic practice. No use, distribution or reproduction is permitted which does not comply with these terms.
*Correspondence: Sunit Das, c3VuaXQuZGFzQHV0b3JvbnRvLmNh