- Drug Discovery Biology, Monash Institute of Pharmaceutical Sciences, Monash University, Parkville, VIC, Australia
The tumor microenvironment influences cancer progression and response to treatments, which ultimately impacts the survival of patients with cancer. The sympathetic nervous system (SNS) is a core component of solid tumors that arise in the body. In addition to influencing cancer progression, a role for the SNS in the effectiveness of cancer treatments is beginning to emerge. This review explores evidence that the SNS impairs chemotherapy efficacy. We review findings of studies that evaluated the impact of neural ablation on chemotherapy outcomes and discuss plausible mechanisms for the impact of neural signaling on chemotherapy efficacy. We then discuss implications for clinical practice, including opportunities to block neural signaling to improve response to chemotherapy.
Introduction
The tumor microenvironment was long thought to be a bystander in cancer progression but is now recognized to play an active role in regulating cancer progression (1). Cancer cells interact with stromal and immune cells in the tumor microenvironment, actively sculpting the environment to favor cancer progression (1). Multiple compartments of the tumor microenvironment regulate both treatment efficacy and resistance (2, 3). For example, variation in the immune profile of individual tumors predict treatment outcomes, where high T cell and low macrophage abundance in the tumor has been linked to better response to chemotherapy in patients (4, 5). Moreover, vascular integrity within the tumor can affect chemotherapy distribution within the tumor (6, 7). Whilst much is known about how the vasculature and immune landscape of tumors contributes to treatment response or resistance, the role of tumor-associated nerves in modulating these effects is only beginning to be elucidated.
The peripheral nervous system is comprised of the autonomic (sympathetic and parasympathetic) and somatic nervous systems. The sympathetic and parasympathetic nervous systems regulates physiological functions of organs, while sensory neurons transmit internal and external sensory cues (temperature, touch, mechanical and noxious stimuli) to the brain, and regulate signaling in innervated organs through neuropeptide release (8, 9). Tumors that arise in the body (outside the central nervous system) may be innervated by more than one neural subtype (10–15), each of which has been shown to play an active role in cancer progression by either regulating cancer cell function or shaping the tumor microenvironment to favor cancer progression, as reviewed previously (16).
In addition to modulating cancer progression, recent studies provide evidence that the sympathetic nervous system (SNS) also regulates the response to chemotherapy treatment. The SNS is activated by stimuli that challenge organismal homeostasis, inducing a fight-or-flight stress response (17). Activation of the SNS induces the release of catecholaminergic neurotransmitters including norepinephrine (or noradrenaline) from nerve endings and epinephrine (or adrenaline) from the adrenal gland (18). Neurotransmitter ligation of G-protein coupled adrenergic receptors shifts gene expression to modulate the behavior of diverse cell types in the tumor microenvironment. The β2-adrenergic receptor (β2AR) subtype is expressed by tumor cells, endothelial cells, and immune cells, and preclinical studies from the last two decades show that β2AR signaling drives tumor growth and metastasis and suppresses anti-cancer immunity in various cancer types (19–33), as previously reviewed (34). More recently, evidence for the role of other adrenergic receptor subtypes (α1AR, α2AR, β1AR and β3AR) in regulating cancer progression is emerging (35–38). As described below, evidence for SNS regulation of chemotherapy response has focused on the role of β2AR, and the role of the other adrenergic receptors on chemotherapy response is yet to be explored. Therefore, here we focus on evidence that SNS signaling through β2AR modulates chemotherapy response.
Targeting tumor-associated nerves improves response to chemotherapy
Expanding on well characterized roles for the SNS in cancer progression (16, 39), the application of tools from neuroscience and pharmacology to the field of cancer biology has begun to elucidate a role for the SNS in response to cancer treatments including chemotherapy. Evidence that SNS signaling can impact the effectiveness of chemotherapy comes from studies that target different aspects of SNS signaling including tumor-associated nerves, neurotrophins that support these nerves, and the receptor signaling activated by SNS neurotransmitters.
In mouse models of triple-negative breast cancer (TNBC) and pancreatic ductal adenocarcinoma, localized SNS denervation of the tumor microenvironment using surgery or neurotoxin prior to chemotherapy treatment were shown to improve chemotherapy control of metastasis and increase overall survival (23, 28) (Table 1). In a mouse model of TNBC, ablation of sympathetic nerves within the mammary fatpad using 6-hydroxydopamine reduced metastasis progression after treatment with the anthracycline chemotherapy doxorubicin (23) (Figure 1). In contrast, anthracycline chemotherapy had no effect on metastasis in mice with intact sympathetic innervation at the site of the primary tumor. Denervation of the primary tumor had no effect on doxorubicin control of primary tumor growth, nor did denervation of the primary tumor have any effect on metastasis in mice that were not treated with chemotherapy. These findings indicate that neural signaling may modulate the response to chemotherapy by affecting the invasive properties of tumor cells rather than by enhancing the effects of chemotherapy on tumor cell killing (23). Similarly, resection of the nerve bundles that supply the pancreas (around the celiac and superior mesenteric arteries) in mice with established pancreas tumors improved the response to subsequent treatment with gemcitabine by doubling overall survival compared to mice with intact nerves (28). As the effect of nerve resection on survival was not evaluated in mice not treated with gemcitabine, it is possible that improved survival was due to an additive effect of chemotherapy and denervation, rather than an effect of neural ablation on gemcitabine efficacy. Additionally, this study did not confirm that the resected nerve bundle specifically contained sympathetic nerve fibers, hence ablation of other neuronal subtypes present in the nerve bundles may contribute to the observed improvement in survival outcomes (28). Nonetheless, these studies suggest that neural signaling may impair the effect of chemotherapy.
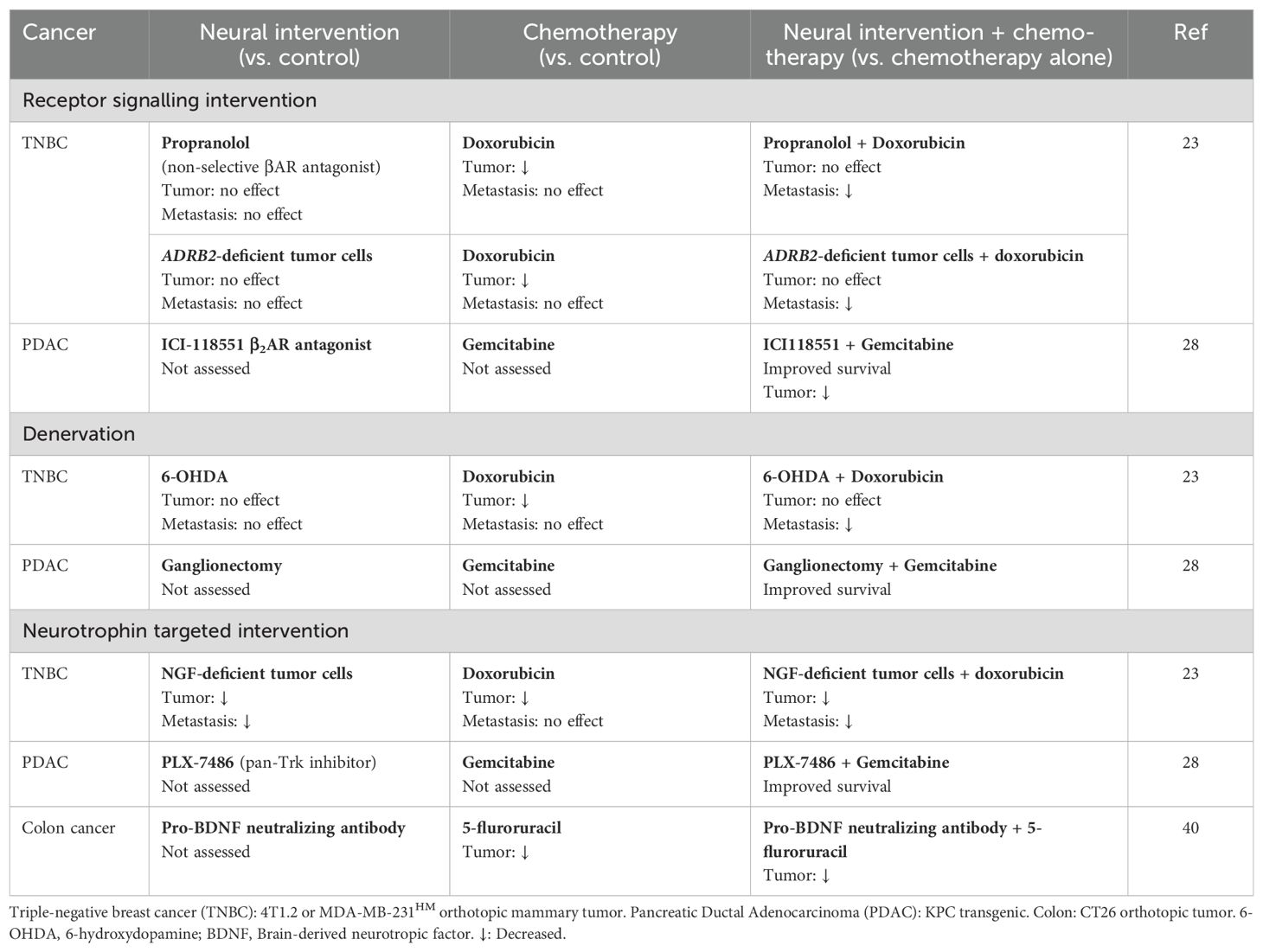
Table 1. Neural-targeted interventions modulate cancer progression and response to chemotherapy in mouse models of cancer.
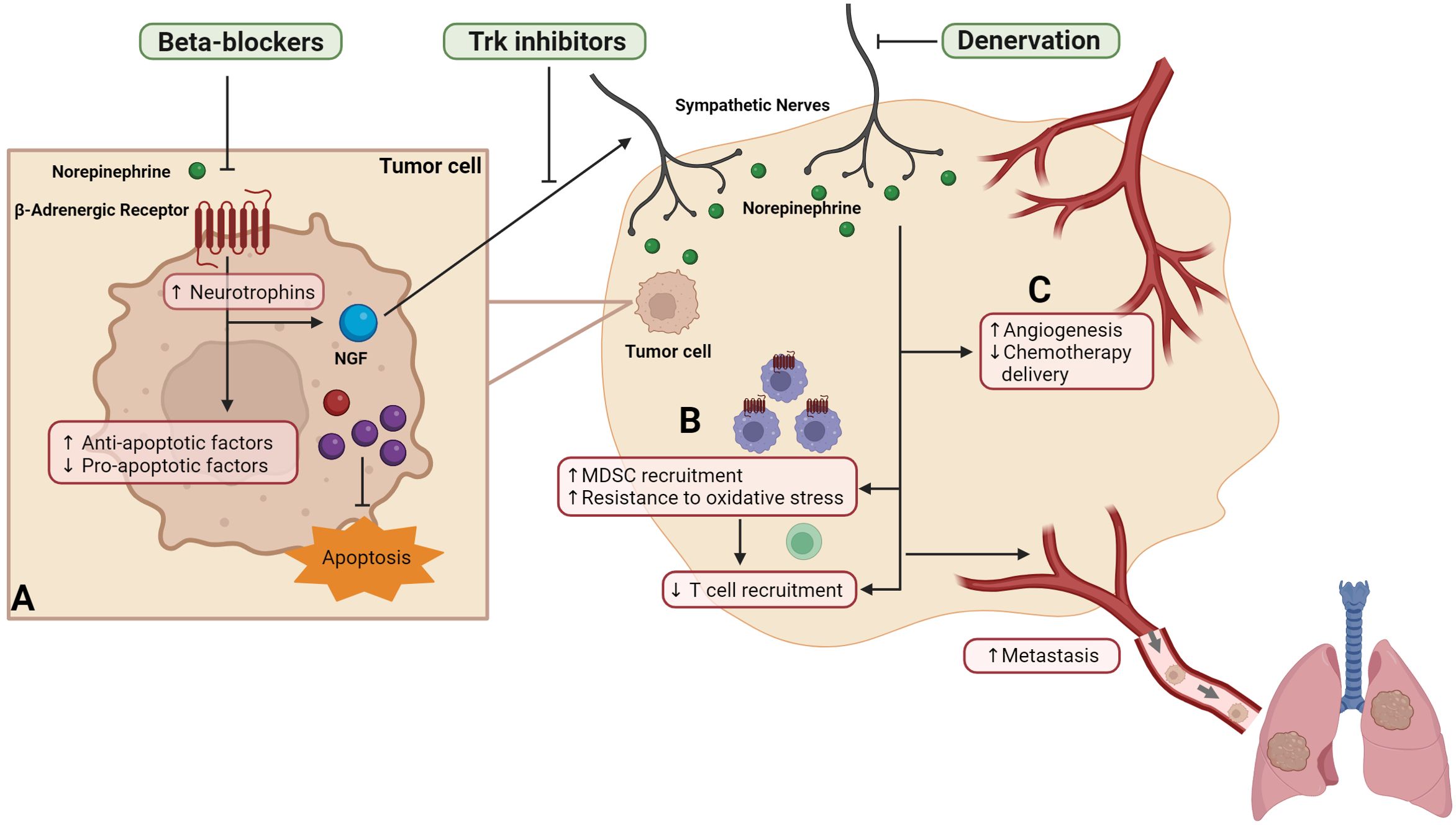
Figure 1. Strategies that block SNS signaling during chemotherapy improve cancer-related outcomes. Sympathetic signaling acts on the multiple components of the tumor microenvironment to drive cancer cell dissemination, including (A) tumor cells to increase transcription of neurotrophins including nerve growth factor (NGF) and shift the balance of pro- and anti-apoptotic factors to reduce apoptosis, (B) immune cells to increase recruitment of myeloid derived suppressor cells (MDSC) to the tumor, increase MDSC resistance to oxidative stress, and reduced cytotoxic T cells recruitment, and (C) blood and lymph vasculature to reduce drug delivery and increase pathways of tumor cell escape. Preclinical studies showed that interventions that target sympathetic nervous system, including surgical or chemical denervation, Trk inhibitors or beta-blockers reduce metastasis and improve survival.
Studies that targeted neurotrophin support of tissue innervation also provide evidence that neural signaling impacts chemotherapy response. Neurotrophins including nerve growth factor (NGF) support the growth and maintenance of sympathetic neurons (40). Within tumors, neurotrophins are synthesized by cancer cells and could increase tumor innervation (41, 42). Blocking neurotrophin signaling using a pan-tropomyosin receptor kinase (Trk) inhibitor during treatment with gemcitabine was shown to improve survival of mice with pancreatic cancer compared with treatment with gemcitabine alone (28) (Table 1). Similarly, blocking pro-brain derived neurotrophic factor using a neutralizing antibody during treatment with 5-fluorouracil reduced growth associated protein-43-positive tumor innervation and resulted in smaller colorectal tumors than treatment with the chemotherapy alone (41) (Table 1). In addition to being expressed by neurons, TrkA and p75 neurotrophin receptors (p75NTR) are expressed by tumor cells and NGF activation of these receptors promotes tumor cell proliferation (28, 43). Therefore, it will be important to determine whether improved outcomes of neurotrophin targeted strategies that have been observed in preclinical studies are due to an effect on chemotherapy efficacy or a result of limiting cancer cell proliferation during treatment.
When activated, SNS nerves release neurotransmitters that bind to adrenergic receptors in target tissues. Evaluation of clinical cancer samples shows wide variation in levels of adrenergic receptors on tumor cells (44, 45). A number of studies that examined the prognostic potential of β2AR expression found that high tumor cell β2AR was associated with worse survival outcomes in various cancer types (32, 46–50). A plausible mechanism for this effect may be through modulation of treatment efficacy. For example, in a study of HER2+ breast cancer, high levels of tumor cell β2AR prior to neoadjuvant treatment with anthracycline-containing chemotherapy and trastuzumab was associated with decreased pathological complete response (46). While not all tumors express high β2AR at diagnosis, there is evidence that β2AR levels (and therefore sensitivity to SNS activation) may be increased by chemotherapy (23). Anthracycline drugs including doxorubicin and epirubicin were shown to increase β2AR transcription in mouse models of TNBC and in patient samples, thereby sensitizing tumor cells to SNS neurotransmitter signaling (23). These findings suggest that chemotherapy treatment could potentially affect the tumor response to future treatment by modulating sensitivity to neural signaling.
In line with these clinical observations, studies that investigated pharmacological blockade of βAR suggest that the SNS impacts chemotherapy outcomes. β-adrenergic antagonists, also known as beta-blockers, are commonly used to treat cardiovascular diseases and a range of other conditions (51). In mice with TNBC, treatment with the beta-blocker propranolol significantly improved doxorubicin-mediated control of metastatic growth, independent of an effect on the primary tumor (23) (Table 1). Genetic knockout of β2AR from tumor cells replicated the effect of beta-blockade: tumors derived from β2AR-deficient tumor cells had reduced metastasis following doxorubicin administration compared to tumors derived from β2AR-expressing tumor cells (23). Other chemotherapy drugs may also be improved by blocking neural signaling. In an immunocompromised mouse model of TNBC, propranolol-mediated blockade of SNS signaling during treatment with paclitaxel and 5-fluorouracil improved survival of mice (determined by primary tumor burden) (52), and treating mice with pancreatic tumor with the selective β2-blocker ICI-118551 during treatment with gemcitabine slowed primary tumor growth and increased the overall survival time compared treatment with gemcitabine chemotherapy alone (28) (Table 1).
Retrospective clinical studies that investigated cancer-related outcomes in patients who were treated with beta-blockers at the time of cancer diagnosis or treatment also support a role for βAR signaling in improving clinical outcomes. Beta-blockers are used to treat cardiovascular disease including arrhythmias and heart failure, glaucoma, and are used for migraine prophylaxis (53). As a consequence of the widespread use of beta-blocker drugs, a significant proportion of adults who are diagnosed with cancer will (co-incidentally) be prescribed a beta-blocker during cancer treatment. A recent retrospective study using both hospital and population cohorts, found that beta-blocker use at the time of treatment with chemotherapy was associated with better metastasis-free survival in women with TNBC compared to no use of beta-blockers (23). Similar findings were reported in another cohort of women with TNBC treated with chemotherapy (54). These studies did not distinguish between use of cardio-selective versus non-selective beta-blockers. While beta-blocker use at diagnosis has been associated with improved disease-free survival and/or overall survival in other cancer types (55–63), those studies did not consider interactions with standard cancer treatments such as chemotherapy. Nonetheless, as beta-blocker use for cardiovascular indications tends to be long-term, it is likely that beta-blockers were used concurrently with cancer treatment in those cancer cohorts. In future clinical analyses it will be important to investigate if the beneficial effects of beta-blockers on cancer outcomes is due to an interaction with chemotherapy.
Mechanisms for neural effects on chemotherapy treatment
The effects of SNS activation on chemotherapy response may be due to effects on tumor cells, or components of the tumor microenvironment (Figure 1). While mechanisms of chemotherapy drugs vary, their ultimate goal is to induce apoptosis in cancer cells. In addition, optimal chemotherapeutic effects may be dependent on induction of a tumor-targeted immune response and drug access to the tumor. Neural signaling may impact the effects of chemotherapy by acting at each of these levels.
Beta-adrenergic signaling desensitizes cancer cells to the cytotoxic effects of chemotherapy, an effect that is prevented with beta-blockers (24, 52, 64–67). This has been demonstrated for different chemotherapy drugs including cisplatin, paclitaxel, and vincristine, and in cell lines derived from different cancer types including breast, ovarian, pancreatic cancer and neuroblastoma (24, 52, 64–67). Several mechanisms have been proposed, including modulation of the apoptotic molecular machinery and DNA damage response by βAR signaling (Figure 1A) (24, 63, 65, 67). Preclinical studies have shown that βAR signaling inactivated pro-apoptotic protein BAD and p53, and increased anti-apoptotic protein BCL-XL, BCL-2 and MCL1 in pancreatic cancer cells (24). βAR signaling can also increase dual specificity phosphatase 1 protein which inhibited paclitaxel-induced JNK/c-Jun-dependent apoptotic signaling pathway (64). βAR signaling has been implicated in promoting DNA damage, thus driving G1 cell cycle arrest in breast cancer cells which may contribute to the lack of paclitaxel efficacy in the presence of βAR signaling (66). In contrast, blockade of βAR signaling using propranolol increased levels of the pro-apoptotic protein p53 in breast cancer cells in vitro; and a similar observation was reported in a single patient who was treated with propranolol before surgical resection of tumor (68). These findings indicate that blockade of βAR signaling may improve cytotoxic effects of chemotherapy. Supporting this hypothesis, co-administration of propranolol increased tumor sensitivity to docetaxel or pro-apoptotic therapy Apo2L/TRAIL, resulting in smaller tumors compared to mice that were treated with either therapy alone (24, 64).
The finding that blocking sympathetic neural signaling improve chemotherapy control of metastasis without impacting primary tumor burden in vivo or cancer cell proliferation in vitro (23), suggests that beta-blockade may improve chemotherapy efficacy via alternative mechanisms besides targeting chemotherapy-induced apoptosis. Emerging evidence suggests that neural signaling can impair chemotherapy through effects on immune cells (69)(Figure 1B). An immune response is required for the optimal effect of some chemotherapy drugs (70, 71). For example, the anthracycline drug doxorubicin increased proliferation of tumor-specific CD8+ T cells in tumor-draining lymph nodes and T cell deletion reduced the effectiveness of chemotherapy in mice (71). Moreover, leukocyte infiltration into tumors predicts recurrence-free and overall cancer survival in TNBC patients who received adjuvant chemotherapy (5). However, myeloid-derived suppressor cells (MDSC) that are prevalent in the tumor can dampen CD8 immune response (72), which may affect chemotherapy efficacy. Recently, it was shown that activation of βAR signaling in MDSC inhibited doxorubicin-induced apoptosis by inducing metabolic reprogramming, thus making the MDSC more resistant to doxorubicin-induced oxidative stress (Figure 1B) (69). Consequently, propranolol blockade of βAR signaling promoted MDSC cell death and reduced MDSC abundance in tumors, which was associated with increased survival of mice with lymphoma compared to treatment with doxorubicin or propranolol alone (69). Neural signaling through βAR has also been shown to regulate recruitment, expansion and function of other immune cell populations. For example, βAR signaling promotes recruitment of other myeloid cell populations to tumors, while reducing recruitment of functional CD8+ T cells (29, 73–76). Additionally, βAR signaling can modulates T cell motility by regulating tissue oxygen levels, thus affecting T cell priming and expansion of tumor-specific T cells (19). Neural signaling also impairs T cell priming and thus cytotoxicity by downregulating the antigen presentation capacity of dendritic cells (20, 77). Notably, most of the immune cells regulated by neural signaling through βAR have been implicated in modulation of chemotherapy efficacy (4, 70, 71, 78–81). Therefore, future studies are needed to examine if these immune cells are also targeted by βAR signaling in the context of chemotherapy treatment. These studies will provide important insights into how blocking neural signaling can improve the anti-cancer immune response, which may have significant implications for clinical practice with the recent shift towards addition of immunotherapy to chemotherapy regimens.
Neural regulation of tumor vasculature may also impact the effect of chemotherapy (Figure 1C). The tumor vasculature serves as an important route to ensure effective drug delivery (6, 7). Physiological SNS activation by restraint stress has been shown to dysregulate blood and lymph vasculature within tumors via βAR-signaling (29, 30, 60, 82). βAR-induced remodeling of vasculature in tumor occurs through both direct effects on endothelial cells and indirect effects through immune cells and tumor cells (29, 30, 33, 60, 82). Genetic deletion of Adrb2, which encodes β2AR, in endothelial cells significantly increased oxidative metabolism in endothelial cells, thus reducing angiogenesis, vascular density, and ultimately progression of prostatic intraepithelial neoplasia in a transgenic model of prostate cancer (33). Other studies have shown that tumor-associated macrophages are required for βAR-induced vascular remodeling by inducing production of VEGF-A and VEGF-C by tumor cells (29, 60). βAR signaling can also affect tumor cells directly by upregulating transcription and production of VEGF-A, leading to vascular remodeling in tumors (30, 82, 83). As tumor vasculature is a critical component of the tumor microenvironment that impacts chemotherapy efficacy (80, 81, 84), βAR-induced remodeling of tumor vasculature may contribute to the effects of neural signaling on chemotherapy efficacy. Therefore, to fully understand how neural signaling modulates the effects of chemotherapy, it will be important for future studies to define how the SNS regulates multiple aspects of the tumor microenvironment including immune and vasculature contributions to treatment response.
Discussion: clinical implications and future directions
SNS control of chemotherapy efficacy suggests that strategies that target neural signaling may be leveraged to improve outcomes for patients with cancer. Pharmacological beta-blockade could be rapidly translated to help patients as beta-blockers are widely available, inexpensive, and well-tolerated drugs that could be readily combined with existing treatments including chemotherapy. Early-stage clinical trials for the use of beta-blockers are promising. Phase II window of opportunity studies have shown that the beta-blocker propranolol reduces biomarkers of invasion and inflammation and is well tolerated by patients with cancer (85–87). Feasibility trials have demonstrated that propranolol may be safely combined with neoadjuvant chemotherapy (88, 89) and used in the perioperative period (85, 86). Additional insights may come from several ongoing single arm trials (NCT04005365, NCT02641314, NCT03108300, NCT02897986) in other cancer types, and early evidence suggests that beta-blockers may improve progression-free survival in cancer patients (90).
To best design future clinical trials for evaluation of beta-blocker use in combination with existing cancer therapies, we need to understand which treatment regimens can be optimally enhanced by blocking sympathetic neural signaling. To address this, a number of key questions remain to be addressed. Firstly, does the SNS broadly impair chemotherapy, or are effects limited to drugs with specific mechanisms of action? In the context of TNBC, observations from a hospital cohort and mechanistic preclinical studies suggest that beta-blockers may optimally improve anthracycline-containing regimens (23, 54). However, in vitro and in vivo studies across TNBC, neuroblastoma, pancreatic cancer, and osteosarcoma, suggest that beta-blockers may improve the effect of taxanes, 5-fluorouracil, vincristine, gemcitabine and cisplatin (28, 52, 91, 92). Therefore, it will be important to determine if the benefit of blocking neural signaling depends on the distinctive biology of different cancer types.
Second, consideration of the receptor that mediates the effects of SNS activation will determine the type of beta-blocker used in future clinical trials. The mechanistic studies described above emphasize a role for β2AR in the effects of neural signaling on chemotherapy (23, 69). This raises the possibility that non-selective beta-blockers may be more effective in the chemotherapy treatment context than β1AR-targeted cardio-selective beta-blockers. Beyond the treatment context, some epidemiological analyses support this concept (55, 57, 93), while other studies that did not distinguish between beta-blocker subtypes have found improved cancer-related outcomes (59, 62, 94). It will be also important to consider the timing of beta-blockade. Previous phase II trials were designed to block SNS signaling in the peri-operative period (85, 86), and emerging studies suggest that beta-blockade during neoadjuvant chemotherapy treatment may improve long term relapse-free and overall survival (23, 54). Recent findings that SNS signaling through α2AR may have anti-tumor effects by modulating the immune response, has led to suggestions that drugs such as clonidine may be effective in cancer (36). However, perioperative clonidine use during resection surgery for lung or breast cancer was found to have no association with cancer-related survival (95). Therefore, further research on the role of α2AR in chemotherapy response is needed. Third, does SNS activity affect other cancer treatment modalities, such as immunotherapy, radiotherapy, or targeted therapies? Preclinical studies suggest that this is likely, indicating that beta-blockade may have benefit across different cancer treatments. Physiological SNS activation by exposure to cold temperature housing impaired the therapeutic effects of immune checkpoint inhibitors in mouse models of breast cancer, melanoma and lymphoma, and this effect was phenocopied by βAR agonism and blocked by beta-blockade (20, 76, 96). Moreover, preclinical studies found that beta-blockade improved radiotherapy-induced reduction of primary tumor growth and enhanced an abscopal response in a non-irradiated second primary tumor, suggesting that βAR signaling impairs the efficacy of radiotherapy (97, 98). Other targeted therapies including trastuzumab, erlotinib, and the anti-angiogenic drug sunitinib are impaired by βAR signaling (46, 82, 99, 100). Mechanistic studies suggest that βAR activation induced oncogenic PI3K/Akt/mTOR signaling or LKB1/mTOR signaling, which impaired the response to trastuzumab and erlotinib, respectively (46, 100), while βAR upregulation of angiogenic factors VEGF, IL-8, and IL-6 may impair the effects of sunitinib (82, 99). Expanding our understanding of the impact of βAR signaling on other treatment modalities, beside chemotherapy, will help to guide strategic translation of neural-targeted intervention strategies including beta-blockers.
Beyond evaluation of the SNS, an understanding of the role of other peripheral neural subtypes may provide additional opportunities to enhance cancer treatment response. A growing body of work shows that sensory neurons induce immunosuppression in tumors through the actions of neuropeptide signaling (12, 14). Future research to evaluate the impact of sensory neural regulation of anti-cancer immunity may identify opportunities to improve treatment modalities that rely on a functional immune response including chemotherapy and immunotherapy. Drugs that target sensory neuropeptide signaling are already used clinically to treat migraine (gepant drugs) and nausea (pitant drugs) (101, 102), and may provide a strategy to target sensory signaling in tumors. The parasympathetic nervous system modulates anti-inflammatory pathways (103), suggesting it could be leveraged to slow cancer. However, as parasympathetic neural signaling has been implicated in tumor cell dissemination in prostate cancer (15), further research is needed. Generalized nerve blocking drugs also offer a strategy to target multiple neural subtypes within the tumor. Supporting evidence for this approach comes from a prospective clinical trial that administered lidocaine around the tumor immediately prior to surgery and found a small but significant improvement in disease-free and overall survival (104).
Opportunities for clinical translation are not limited to pharmacological strategies, with several other techniques currently being used to modulate neural signaling. While not currently used for cancer, denervation may allow targeted ablation of neurons to a specific organ. This approach is utilized to treat resistant hypertension using sympathetic denervation of the renal system via catheter (105). Additionally, implantable bioelectric devices that use electric stimulation to modulate vagus nerve signaling are currently being developed for the treatment of rheumatoid arthritis and Crohn’s Disease (106–108), suggesting that a similar approach can be used to target cancers that are regulated by the vagus nerve.
Finally, targeting neural signaling during the period of cancer treatment may have additional benefits beyond control of cancer progression. The non-selective β-blocker carvedilol is currently used to treat cardiotoxicity induced by anthracycline chemotherapy and trastuzumab (109). Carvedilol has been shown to reduce primary tumor growth and metastasis by modulating tumor cell invasion (59), raising the possibility that carvedilol could be administered at the time of conventional cancer treatment to both slow cancer progression and prevent treatment-induced cardiotoxic side-effects. Drugs that target neurotrophic signaling may be another strategy for multifaceted effects. For example, anti-NGF antibodies have been evaluated for treatment of pain (110), suggesting that they could reduce cancer-associated pain in addition to targeting the sympathetic and sensory nervous systems in the tumor microenvironment. As the NGF targeted drug tanezumab failed FDA approval for treatment of osteoarthritis (111), there may be commercial interest in repurposing this drug for treatment of cancer.
Conclusions
Cancer is a stressful experience which elevates SNS flight-or-flight signaling in patients. In addition, cancer treatment increases tumor sensitivity to SNS signaling (23). Our understanding of how neural signaling regulates cancer treatment efficacy is expanding and will support the discovery of targeted neural interventions that may be leveraged to enhance outcomes of standard cancer treatments.
Author contributions
AM: Conceptualization, Writing – original draft, Writing – review & editing. ES: Conceptualization, Supervision, Writing – original draft, Writing – review & editing. AC: Conceptualization, Supervision, Writing – original draft, Writing – review & editing.
Funding
The author(s) declare financial support was received for the research, authorship, and/or publication of this article. ES and AC are supported by a grant from the National Health and Medical Research Council (2020851), and ES is supported by a grant from the National Breast Cancer Foundation IIRS-20-025.
Conflict of interest
The authors declare that the research was conducted in the absence of any commercial or financial relationships that could be construed as a potential conflict of interest.
Publisher’s note
All claims expressed in this article are solely those of the authors and do not necessarily represent those of their affiliated organizations, or those of the publisher, the editors and the reviewers. Any product that may be evaluated in this article, or claim that may be made by its manufacturer, is not guaranteed or endorsed by the publisher.
References
1. de Visser KE, Joyce JA. The evolving tumor microenvironment: From cancer initiation to metastatic outgrowth. Cancer Cell. (2023) 41:374–403. doi: 10.1016/j.ccell.2023.02.016
2. Junttila MR, de Sauvage FJ. Influence of tumour micro-environment heterogeneity on therapeutic response. Nature. (2013) 501:346–54. doi: 10.1038/nature12626
3. Wu P, Gao W, Su M, Nice EC, Zhang W, Lin J, et al. Adaptive mechanisms of tumor therapy resistance driven by tumor microenvironment. Front Cell Dev Biol. (2021) 9. doi: 10.3389/fcell.2021.641469
4. DeNardo DG, Brennan DJ, Rexhepaj E, Ruffell B, Shiao SL, Madden SF, et al. Leukocyte complexity predicts breast cancer survival and functionally regulates response to chemotherapy. Cancer Discovery. (2011) 1:54–67. doi: 10.1158/2159-8274.CD-10-0028
5. Loi S, Drubay D, Adams S, Pruneri G, Francis PA, Lacroix-Triki M, et al. Tumor-infiltrating lymphocytes and prognosis: A pooled individual patient analysis of early-stage triple-negative breast cancers. J Clin Oncol. (2019) 37:559–69. doi: 10.1200/JCO.18.01010
6. Nakasone ES, Askautrud HA, Kees T, Park JH, Plaks V, Ewald AJ, et al. Imaging tumor-stroma interactions during chemotherapy reveals contributions of the microenvironment to resistance. Cancer Cell. (2012) 21:488–503. doi: 10.1016/j.ccr.2012.02.017
7. Primeau AJ, Rendon A, Hedley D, Lilge L, Tannock IF. The distribution of the anticancer drug Doxorubicin in relation to blood vessels in solid tumors. Clin Cancer Res. (2005) 11:8782–8. doi: 10.1158/1078-0432.CCR-05-1664
8. Scott-Solomon E, Boehm E, Kuruvilla R. The sympathetic nervous system in development and disease. Nat Rev Neurosci. (2021) 22:685–702. doi: 10.1038/s41583-021-00523-y
9. Talbot S, Foster SL, Woolf CJ. Neuroimmunity: physiology and pathology. Annu Rev Immunol. (2016) 34:421–47. doi: 10.1146/annurev-immunol-041015-055340
10. Bae G, Kim H-S, Won K, Kim G, Sung J-Y, Lim S-J. Lower sympathetic nervous system density and β-adrenoreceptor expression are involved in gastric cancer progression. Anticancer Res. (2019) 39:231–6. doi: 10.21873/anticanres.13102
11. Li D, Hu LN, Zheng SM, La T, Wei LY, Zhang XJ, et al. High nerve density in breast cancer is associated with poor patient outcome. FASEB Bioadv. (2022) 4:391–401. doi: 10.1096/fba.2021-00147
12. Balood M, Ahmadi M, Eichwald T, Ahmadi A, Majdoubi A, Roversi K, et al. Nociceptor neurons affect cancer immunosurveillance. Nature. (2022) 611:405–12. doi: 10.1038/s41586-022-05374-w
13. Le TT, Payne SL, Buckwald MN, Hayes LA, Parker SR, Burge CB, et al. Sensory nerves enhance triple-negative breast cancer invasion and metastasis via the axon guidance molecule PlexinB3. NPJ Breast Cancer. (2022) 8:116. doi: 10.1038/s41523-022-00485-z
14. McIlvried LA, Atherton MA, Horan NL, Goch TN, Scheff NN. Sensory neurotransmitter calcitonin gene-related peptide modulates tumor growth and lymphocyte infiltration in oral squamous cell carcinoma. Adv Biol (Weinh). (2022) 6:e2200019. doi: 10.1002/adbi.202200019
15. Magnon C, Hall SJ, Lin J, Xue X, Gerber L, Freedland SJ, et al. Autonomic nerve development contributes to prostate cancer progression. Science. (2013) 341:1236361. doi: 10.1126/science.1236361
16. Winkler F, Venkatesh HS, Amit M, Batchelor T, Demir IE, Deneen B, et al. Cancer neuroscience: State of the field, emerging directions. Cell. (2023) 186:1689–707. doi: 10.1016/j.cell.2023.02.002
17. Goldstein DS. Sympathetic nervous system. In: Fink G, editor. Encyclopedia of stress, 2nd ed. Academic Press, New York (2007). p. 697–703.
18. Paravati S, Rosani A, Warrington SJ. Physiology, catecholamines. Treasure Island (FL: StatPearls (2024).
19. Devi S, Alexandre YO, Loi JK, Gillis R, Ghazanfari N, Creed SJ, et al. Adrenergic regulation of the vasculature impairs leukocyte interstitial migration and suppresses immune responses. Immunity. (2021) 54(6):1219-30. doi: 10.1016/j.immuni.2021.03.025
20. Nissen MD, Sloan EK, Mattarollo SR. [amp]]beta;-adrenergic signaling impairs antitumor CD8(+) T-cell responses to B-cell lymphoma immunotherapy. Cancer Immunol Res. (2018) 6:98–109. doi: 10.1158/2326-6066.CIR-17-0401
21. Qiao G, Bucsek MJ, Winder NM, Chen M, Giridharan T, Olejniczak SH, et al. [amp]]beta;-Adrenergic signaling blocks murine CD8(+) T-cell metabolic reprogramming during activation: a mechanism for immunosuppression by adrenergic stress. Cancer Immunol Immunother. (2019) 68:11–22. doi: 10.1007/s00262-018-2243-8
22. Qiao G, Chen M, Mohammadpour H, MacDonald CR, Bucsek MJ, Hylander BL, et al. Chronic adrenergic stress contributes to metabolic dysfunction and an exhausted phenotype in T cells in the tumor microenvironment. Cancer Immunol Res. (2021) 9:651–64. doi: 10.1158/2326-6066.CIR-20-0445
23. Chang A, Botteri E, Gillis RD, Lofling L, Le CP, Ziegler AI, et al. Beta-blockade enhances anthracycline control of metastasis in triple-negative breast cancer. Sci Transl Med. (2023) 15:eadf1147. doi: 10.1126/scitranslmed.adf1147
24. Eng JW, Reed CB, Kokolus KM, Pitoniak R, Utley A, Bucsek MJ, et al. Housing temperature-induced stress drives therapeutic resistance in murine tumour models through beta2-adrenergic receptor activation. Nat Commun. (2015) 6:6426. doi: 10.1038/ncomms7426
25. Inbar S, Neeman E, Avraham R, Benish M, Rosenne E, Ben-Eliyahu S. Do stress responses promote leukemia progression? An animal study suggesting a role for epinephrine and prostaglandin-E2 through reduced NK activity. PloS One. (2011) 6:e19246. doi: 10.1371/journal.pone.0019246
26. Kim-Fuchs C, Le CP, Pimentel MA, Shackleford D, Ferrari D, Angst E, et al. Chronic stress accelerates pancreatic cancer growth and invasion: a critical role for beta-adrenergic signaling in the pancreatic microenvironment. Brain Behav Immun. (2014) 40:40–7. doi: 10.1016/j.bbi.2014.02.019
27. Lamkin DM, Sloan EK, Patel AJ, Chiang BS, Pimentel MA, Ma JC, et al. Chronic stress enhances progression of acute lymphoblastic leukemia via beta-adrenergic signaling. Brain Behav Immun. (2012) 26:635–41. doi: 10.1016/j.bbi.2012.01.013
28. Renz BW, Takahashi R, Tanaka T, Macchini M, Hayakawa Y, Dantes Z, et al. beta2 adrenergic-neurotrophin feedforward loop promotes pancreatic cancer. Cancer Cell. (2018) 34:863–7. doi: 10.1016/j.ccell.2018.10.010
29. Sloan EK, Priceman SJ, Cox BF, Yu S, Pimentel MA, Tangkanangnukul V, et al. The sympathetic nervous system induces a metastatic switch in primary breast cancer. Cancer Res. (2010) 70:7042–52. doi: 10.1158/0008-5472.CAN-10-0522
30. Thaker PH, Han LY, Kamat AA, Arevalo JM, Takahashi R, Lu C, et al. Chronic stress promotes tumor growth and angiogenesis in a mouse model of ovarian carcinoma. Nat Med. (2006) 12:939–44. doi: 10.1038/nm1447
31. Chang A, Le CP, Walker AK, Creed SJ, Pon CK, Albold S, et al. [amp]]beta;2-Adrenoceptors on tumor cells play a critical role in stress-enhanced metastasis in a mouse model of breast cancer. Brain Behav Immun. (2016) 57:106–15. doi: 10.1016/j.bbi.2016.06.011
32. Kurozumi S, Kaira K, Matsumoto H, Hirakata T, Yokobori T, Inoue K, et al. Beta2-Adrenergic receptor expression is associated with biomarkers of tumor immunity and predicts poor prognosis in estrogen receptor-negative breast cancer. Breast Cancer Res Treat. (2019) 177:603–10. doi: 10.1007/s10549-019-05341-6
33. Zahalka AH, Arnal-Estape A, Maryanovich M, Nakahara F, Cruz CD, Finley LWS, et al. Adrenergic nerves activate an angio-metabolic switch in prostate cancer. Science. (2017) 358:321–6. doi: 10.1126/science.aah5072
34. Chang A, Sloan EK, Antoni MH, Knight JM, Telles R, Lutgendorf SK. Biobehavioral pathways and cancer progression: insights for improving well-being and cancer outcomes. Integr Cancer Ther. (2022) 21:15347354221096081. doi: 10.1177/15347354221096081
35. Broso F, Gatto P, Sidarovich V, Ambrosini C, De Sanctis V, Bertorelli R, et al. Alpha-1 adrenergic antagonists sensitize neuroblastoma to therapeutic differentiation. Cancer Res. (2023) 83:2733–49. doi: 10.1158/0008-5472.CAN-22-1913
36. Zhu J, Naulaerts S, Boudhan L, Martin M, Gatto L, Van den Eynde BJ. Tumour immune rejection triggered by activation of α2-adrenergic receptors. Nature. (2023) 618:607–15. doi: 10.1038/s41586-023-06110-8
37. Globig A-M, Zhao S, Roginsky J, Maltez VI, Guiza J, Avina-Ochoa N, et al. The β1-adrenergic receptor links sympathetic nerves to T cell exhaustion. Nature. (2023) 622:383–92. doi: 10.1038/s41586-023-06568-6
38. Bruno G, Nastasi N, Subbiani A, Boaretto A, Ciullini Mannurita S, Mattei G, et al. [amp]]beta;3-adrenergic receptor on tumor-infiltrating lymphocytes sustains IFN-γ-dependent PD-L1 expression and impairs anti-tumor immunity in neuroblastoma. Cancer Gene Ther. (2023) 30:890–904. doi: 10.1038/s41417-023-00599-x
39. Demir IE, Mota Reyes C, Alrawashdeh W, Ceyhan GO, Deborde S, Friess H, et al. Future directions in preclinical and translational cancer neuroscience research. Nat Cancer. (2021) 1:1027–31. doi: 10.1038/s43018-020-00146-9
40. Glebova NO, Ginty DD. Heterogeneous requirement of NGF for sympathetic target innervation in vivo. J Neurosci. (2004) 24:743–51. doi: 10.1523/JNEUROSCI.4523-03.2004
41. Jiang CC, Marsland M, Wang Y, Dowdell A, Eden E, Gao F, et al. Tumor innervation is triggered by endoplasmic reticulum stress. Oncogene. (2022) 41:586–99. doi: 10.1038/s41388-021-02108-6
42. Pundavela J, Roselli S, Faulkner S, Attia J, Scott RJ, Thorne RF, et al. Nerve fibers infiltrate the tumor microenvironment and are associated with nerve growth factor production and lymph node invasion in breast cancer. Mol Oncol. (2015) 9:1626–35. doi: 10.1016/j.molonc.2015.05.001
43. Descamps S, Toillon RA, Adriaenssens E, Pawlowski V, Cool SM, Nurcombe V, et al. Nerve growth factor stimulates proliferation and survival of human breast cancer cells through two distinct signaling pathways. J Biol Chem. (2001) 276:17864–70. doi: 10.1074/jbc.M010499200
44. Powe DG, Voss MJ, Habashy HO, Zanker KS, Green AR, Ellis IO, et al. Alpha- and beta-adrenergic receptor (AR) protein expression is associated with poor clinical outcome in breast cancer: an immunohistochemical study. Breast Cancer Res Treat. (2011) 130:457–63. doi: 10.1007/s10549-011-1371-z
45. Rains SL, Amaya CN, Bryan BA. Beta-adrenergic receptors are expressed across diverse cancers. Oncoscience. (2017) 4:95–105. doi: 10.18632/oncoscience.v4i7-8
46. Liu D, Yang Z, Wang T, Yang Z, Chen H, Hu Y, et al. beta2-AR signaling controls trastuzumab resistance-dependent pathway. Oncogene. (2016) 35:47–58. doi: 10.1038/onc.2015.58
47. Shimizu A, Kaira K, Mori K, Kato M, Shimizu K, Yasuda M, et al. Prognostic significance of β2-adrenergic receptor expression in Malignant melanoma. Tumor Biol. (2016) 37:5971–8. doi: 10.1007/s13277-015-4420-0
48. Kaira K, Kamiyoshihara M, Kawashima O, Endoh H, Imaizumi K, Sugano M, et al. Prognostic impact of beta2 adrenergic receptor expression in surgically resected pulmonary pleomorphic carcinoma. Anticancer Res. (2019) 39:395–403. doi: 10.21873/anticanres.13125
49. Ogawa H, Kaira K, Motegi Y, Yokobori T, Takada T, Kato R, et al. Prognostic significance of beta2-adrenergic receptor expression in patients with surgically resected colorectal cancer. Int J Clin Oncol. (2020) 25:1137–44. doi: 10.1007/s10147-020-01645-6
50. Yazawa T, Kaira K, Shimizu K, Shimizu A, Mori K, Nagashima T, et al. Prognostic significance of beta2-adrenergic receptor expression in non-small cell lung cancer. Am J Transl Res. (2016) 8:5059–70.
51. do Vale GT, Ceron CS, Gonzaga NA, Simplicio JA, Padovan JC. Three generations of β-blockers: history, class differences and clinical applicability. Curr Hypertens Rev. (2019) 15:22–31. doi: 10.2174/1573402114666180918102735
52. Pasquier E, Street J, Pouchy C, Carre M, Gifford AJ, Murray J, et al. beta-blockers increase response to chemotherapy via direct antitumour and anti-angiogenic mechanisms in neuroblastoma. Br J Cancer. (2013) 108:2485–94. doi: 10.1038/bjc.2013.205
54. Melhem-Bertrandt A, Chavez-Macgregor M, Lei X, Brown EN, Lee RT, Meric-Bernstam F, et al. Beta-blocker use is associated with improved relapse-free survival in patients with triple-negative breast cancer. J Clin Oncol. (2011) 29:2645–52. doi: 10.1200/JCO.2010.33.4441
55. Spilsbury K, Tuesley KM, Pearson SA, Coory MD, Donovan P, Steer CB, et al. Perioperative beta-blocker supply and survival in women with epithelial ovarian cancer and a history of cardiovascular conditions. J Clin Oncol. (2023) 41:266–75. doi: 10.1200/JCO.22.00097
56. Yap A, Lopez-Olivo MA, Dubowitz J, Pratt G, Hiller J, Gottumukkala V, et al. Effect of beta-blockers on cancer recurrence and survival: a meta-analysis of epidemiological and perioperative studies. Br J Anaesth. (2018) 121:45–57. doi: 10.1016/j.bja.2018.03.024
57. Stoer NC, Bouche G, Pantziarka P, Sloan EK, Andreassen BK, Botteri E. Use of non-cancer drugs and survival among patients with pancreatic adenocarcinoma: a nationwide registry-based study in Norway. Acta Oncol. (2021) 60:1146–53. doi: 10.1080/0284186X.2021.1953136
58. Udumyan R, Montgomery S, Fang F, Almroth H, Valdimarsdottir U, Ekbom A, et al. Beta-blocker drug use and survival among patients with pancreatic adenocarcinoma. Cancer Res. (2017) 77:3700–7. doi: 10.1158/0008-5472.CAN-17-0108
59. Gillis RD, Botteri E, Chang A, Ziegler AI, Chung NC, Pon CK, et al. Carvedilol blocks neural regulation of breast cancer progression in vivo and is associated with reduced breast cancer mortality in patients. Eur J Cancer. (2021) 147:106–16. doi: 10.1016/j.ejca.2021.01.029
60. Le CP, Nowell CJ, Kim-Fuchs C, Botteri E, Hiller JG, Ismail H, et al. Chronic stress in mice remodels lymph vasculature to promote tumour cell dissemination. Nat Commun. (2016) 7:10634. doi: 10.1038/ncomms10634
61. Ahl R, Matthiessen P, Fang X, Cao Y, Sjolin G, Lindgren R, et al. [amp]]beta;-blockade in rectal cancer surgery: A simple measure of improving outcomes. Ann Surg. (2020) 271:140–6. doi: 10.1097/SLA.0000000000002970
62. Lu H, Liu X, Guo F, Tan S, Wang G, Liu H, et al. Impact of beta-blockers on prostate cancer mortality: a meta-analysis of 16,825 patients. Onco Targets Ther. (2015) 8:985–90. doi: 10.2147/OTT
63. Zahalka AH, Fram E, Lin W, Mohn L, Frenette PS, Agalliu I, et al. Use of beta-blocker types and risk of incident prostate cancer in a multiethnic population. Urol Oncol. (2020) 38:794.e11–794.e16. doi: 10.1016/j.urolonc.2020.03.024
64. Kang Y, Nagaraja AS, Armaiz-Pena GN, Dorniak PL, Hu W, Rupaimoole R, et al. Adrenergic stimulation of DUSP1 impairs chemotherapy response in ovarian cancer. Clin Cancer Res. (2016) 22:1713–24. doi: 10.1158/1078-0432.CCR-15-1275
65. Porcelli L, Garofoli M, Di Fonte R, Fucci L, Volpicella M, Strippoli S, et al. The β-adrenergic receptor antagonist propranolol offsets resistance mechanisms to chemotherapeutics in diverse sarcoma subtypes: a pilot study. Sci Rep. (2020) 10:10465. doi: 10.1038/s41598-020-67342-6
66. Reeder A, Attar M, Nazario L, Bathula C, Zhang A, Hochbaum D, et al. Stress hormones reduce the efficacy of paclitaxel in triple negative breast cancer through induction of DNA damage. Br J Cancer. (2015) 112:1461–70. doi: 10.1038/bjc.2015.133
67. Zhang M, Chen F, Sun X, Huang Y, Zeng Y, Chen J, et al. Sympathetic β2-adrenergic receptor blockade overcomes docetaxel resistance in prostate cancer. Biochem Biophys Res Commun. (2023) 657:69–79. doi: 10.1016/j.bbrc.2023.03.046
68. Montoya A, Varela-Ramirez A, Dickerson E, Pasquier E, Torabi A, Aguilera R, et al. The beta adrenergic receptor antagonist propranolol alters mitogenic and apoptotic signaling in late stage breast cancer. BioMed J. (2019) 42:155–65. doi: 10.1016/j.bj.2019.02.003
69. Daneshmandi S, Choi JE, Yan Q, MacDonald CR, Pandey M, Goruganthu M, et al. Myeloid-derived suppressor cell mitochondrial fitness governs chemotherapeutic efficacy in hematologic Malignancies. Nat Commun. (2024) 15:2803. doi: 10.1038/s41467-024-47096-9
70. Ma Y, Aymeric L, Locher C, Mattarollo SR, Delahaye NF, Pereira P, et al. Contribution of IL-17-producing gamma delta T cells to the efficacy of anticancer chemotherapy. J Exp Med. (2011) 208:491–503. doi: 10.1084/jem.20100269
71. Mattarollo SR, Loi S, Duret H, Ma Y, Zitvogel L, Smyth MJ. Pivotal role of innate and adaptive immunity in anthracycline chemotherapy of established tumors. Cancer Res. (2011) 71:4809–20. doi: 10.1158/0008-5472.CAN-11-0753
72. Li K, Shi H, Zhang B, Ou X, Ma Q, Chen Y, et al. Myeloid-derived suppressor cells as immunosuppressive regulators and therapeutic targets in cancer. Signal Transduction Targeted Ther. (2021) 6:362. doi: 10.1038/s41392-021-00670-9
73. Mohammadpour H, MacDonald CR, Qiao G, Chen M, Dong B, Hylander BL, et al. beta2 adrenergic receptor-mediated signaling regulates the immunosuppressive potential of myeloid-derived suppressor cells. J Clin Invest. (2019) 129:5537–52. doi: 10.1172/JCI129502
74. Armaiz-Pena GN, Gonzalez-Villasana V, Nagaraja AS, Rodriguez-Aguayo C, Sadaoui NC, Stone RL, et al. Adrenergic regulation of monocyte chemotactic protein 1 leads to enhanced macrophage recruitment and ovarian carcinoma growth. Oncotarget. (2015) 6:4266–73. doi: 10.18632/oncotarget.v6i6
75. Jiang W, Li Y, Li ZZ, Sun J, Li JW, Wei W, et al. Chronic restraint stress promotes hepatocellular carcinoma growth by mobilizing splenic myeloid cells through activating β-adrenergic signaling. Brain Behav Immun. (2019) 80:825–38. doi: 10.1016/j.bbi.2019.05.031
76. Bucsek MJ, Qiao G, MacDonald CR, Giridharan T, Evans L, Niedzwecki B, et al. beta-adrenergic signaling in mice housed at standard temperatures suppresses an effector phenotype in CD8(+) T cells and undermines checkpoint inhibitor therapy. Cancer Res. (2017) 77:5639–51. doi: 10.1158/0008-5472.CAN-17-0546
77. Hervé J, Dubreil L, Tardif V, Terme M, Pogu S, Anegon I, et al. [amp]]beta;2-Adrenoreceptor agonist inhibits antigen cross-presentation by dendritic cells. J Immunol. (2013) 190:3163–71. doi: 10.4049/jimmunol.1201391
78. Apetoh L, Ghiringhelli F, Tesniere A, Obeid M, Ortiz C, Criollo A, et al. Toll-like receptor 4-dependent contribution of the immune system to anticancer chemotherapy and radiotherapy. Nat Med. (2007) 13:1050–9. doi: 10.1038/nm1622
79. Ghiringhelli F, Apetoh L, Tesniere A, Aymeric L, Ma Y, Ortiz C, et al. Activation of the NLRP3 inflammasome in dendritic cells induces IL-1beta-dependent adaptive immunity against tumors. Nat Med. (2009) 15:1170–8. doi: 10.1038/nm.2028
80. Hughes R, Qian BZ, Rowan C, Muthana M, Keklikoglou I, Olson OC, et al. Perivascular M2 macrophages stimulate tumor relapse after chemotherapy. Cancer Res. (2015) 75:3479–91. doi: 10.1158/0008-5472.CAN-14-3587
81. Karagiannis GS, Pastoriza JM, Wang Y, Harney AS, Entenberg D, Pignatelli J, et al. Neoadjuvant chemotherapy induces breast cancer metastasis through a TMEM-mediated mechanism. Sci Transl Med. (2017) 9(397):eaan0026. doi: 10.1126/scitranslmed.aan0026
82. Liu J, Deng GH, Zhang J, Wang Y, Xia XY, Luo XM, et al. The effect of chronic stress on anti-angiogenesis of sunitinib in colorectal cancer models. Psychoneuroendocrinology. (2015) 52:130–42. doi: 10.1016/j.psyneuen.2014.11.008
83. Lutgendorf SK, Cole S, Costanzo E, Bradley S, Coffin J, Jabbari S, et al. Stress-related mediators stimulate vascular endothelial growth factor secretion by two ovarian cancer cell lines. Clin Cancer Res. (2003) 9:4514–21.
84. Alishekevitz D, Gingis-Velitski S, Kaidar-Person O, Gutter-Kapon L, Scherer SD, Raviv Z, et al. Macrophage-induced lymphangiogenesis and metastasis following paclitaxel chemotherapy is regulated by VEGFR3. Cell Rep. (2016) 17:1344–56. doi: 10.1016/j.celrep.2016.09.083
85. Hiller JG, Cole SW, Crone EM, Byrne DJ, Shackleford DM, Pang JB, et al. Preoperative beta-blockade with propranolol reduces biomarkers of metastasis in breast cancer: A phase II randomized trial. Clin Cancer Res. (2020) 26:1803–11. doi: 10.1158/1078-0432.CCR-19-2641
86. Shaashua L, Shabat-Simon M, Haldar R, Matzner P, Zmora O, Shabtai M, et al. Perioperative COX-2 and β-adrenergic blockade improves metastatic biomarkers in breast cancer patients in a phase-II randomized trial. Clin Cancer Res. (2017) 23:4651–61. doi: 10.1158/1078-0432.CCR-17-0152
87. Knight JM, Rizzo JD, Hari P, Pasquini MC, Giles KE, D’Souza A, et al. Propranolol inhibits molecular risk markers in HCT recipients: a phase 2 randomized controlled biomarker trial. Blood Adv. (2020) 4:467–76. doi: 10.1182/bloodadvances.2019000765
88. Hopson MB, Lee S, Accordino M, Trivedi M, Maurer M, Crew KD, et al. Phase II study of propranolol feasibility with neoadjuvant chemotherapy in patients with newly diagnosed breast cancer. Breast Cancer Res Treat. (2021) 188(2):427-32. doi: 10.21203/rs.3.rs-162839/v1
89. Ramondetta LM, Hu W, Thaker PH, Urbauer DL, Chisholm GB, Westin SN, et al. Prospective pilot trial with combination of propranolol with chemotherapy in patients with epithelial ovarian cancer and evaluation on circulating immune cell gene expression. Gynecol Oncol. (2019) 154:524–30. doi: 10.1016/j.ygyno.2019.07.004
90. De Giorgi V, Grazzini M, Benemei S, Marchionni N, Botteri E, Pennacchioli E, et al. Propranolol for off-label treatment of patients with melanoma: results from a cohort study. JAMA Oncol. (2018) 4:e172908. doi: 10.1001/jamaoncol.2017.2908
91. Pasquier E, Ciccolini J, Carre M, Giacometti S, Fanciullino R, Pouchy C, et al. Propranolol potentiates the anti-angiogenic effects and anti-tumor efficacy of chemotherapy agents: implication in breast cancer treatment. Oncotarget. (2011) 2:797–809. doi: 10.18632/oncotarget.v2i10
92. Solernó LM, Sobol NT, Gottardo MF, Capobianco CS, Ferrero MR, Vásquez L, et al. Propranolol blocks osteosarcoma cell cycle progression, inhibits angiogenesis and slows xenograft growth in combination with cisplatin-based chemotherapy. Sci Rep. (2022) 12:15058. doi: 10.1038/s41598-022-18324-3
93. Barron TI, Connolly RM, Sharp L, Bennett K, Visvanathan K. Beta blockers and breast cancer mortality: A population-based study. J Clin Oncol. (2011) 29:2635–44. doi: 10.1200/JCO.2010.33.5422
94. Botteri E, Munzone E, Rotmensz N, Cipolla C, De Giorgi V, Santillo B, et al. Therapeutic effect of beta-blockers in triple-negative breast cancer postmenopausal women. Breast Cancer Res Treat. (2013) 140:567–75. doi: 10.1007/s10549-013-2654-3
95. Forget P, Berliere M, Poncelet A, De Kock M. Effect of clonidine on oncological outcomes after breast and lung cancer surgery. Br J Anaesth. (2018) 121:103–4. doi: 10.1016/j.bja.2018.04.020
96. Kokolus KM, Zhang Y, Sivik JM, Schmeck C, Zhu J, Repasky EA, et al. Beta blocker use correlates with better overall survival in metastatic melanoma patients and improves the efficacy of immunotherapies in mice. Oncoimmunology. (2018) 7:e1405205. doi: 10.1080/2162402X.2017.1405205
97. Chen M, Qiao G, Hylander BL, Mohammadpour H, Wang XY, Subjeck JR, et al. Adrenergic stress constrains the development of anti-tumor immunity and abscopal responses following local radiation. Nat Commun. (2020) 11:1821. doi: 10.1038/s41467-020-15676-0
98. MacDonald CR, Bucsek MJ, Qiao G, Chen M, Evans L, Greenberg DJ, et al. Adrenergic receptor signaling regulates the response of tumors to ionizing radiation. Radiat Res. (2019) 191:585–9. doi: 10.1667/RR15193.1
99. Deng GH, Liu J, Zhang J, Wang Y, Peng XC, Wei YQ, et al. Exogenous norepinephrine attenuates the efficacy of sunitinib in a mouse cancer model. J Exp Clin Cancer Res. (2014) 33:21. doi: 10.1186/1756-9966-33-21
100. Nilsson MB, Sun H, Diao L, Tong P, Liu D, Li L, et al. Stress hormones promote EGFR inhibitor resistance in NSCLC: Implications for combinations with β-blockers. Sci Trans Med. (2017) 9:eaao4307. doi: 10.1126/scitranslmed.aao4307
101. Moreno-Ajona D, Villar-Martinez MD, Goadsby PJ. New generation gepants: migraine acute and preventive medications. J Clin Med. (2022) 11(6):1656. doi: 10.3390/jcm11061656
102. Ramirez-Garcia PD, Retamal JS, Shenoy P, Imlach W, Sykes M, Truong N, et al. A pH-responsive nanoparticle targets the neurokinin 1 receptor in endosomes to prevent chronic pain. Nat Nanotechnol. (2019) 14:1150–9. doi: 10.1038/s41565-019-0568-x
103. Bloom O, Tracey KJ, Pavlov VA. Exploring the vagus nerve and the inflammatory reflex for therapeutic benefit in chronic spinal cord injury. Curr Opin Neurol. (2022) 35:249–57. doi: 10.1097/WCO.0000000000001036
104. Badwe RA, Parmar V, Nair N, Joshi S, Hawaldar R, Pawar S, et al. Effect of peritumoral infiltration of local anesthetic before surgery on survival in early breast cancer. J Clin Oncol. (2023) 41:3318–28. doi: 10.1200/JCO.22.01966
105. Sesa-Ashton G, Nolde JM, Muente I, Carnagarin R, Lee R, Macefield VG, et al. Catheter-based renal denervation: 9-year follow-up data on safety and blood pressure reduction in patients with resistant hypertension. Hypertension. (2023) 80:811–9. doi: 10.1161/HYPERTENSIONAHA.122.20853
106. Koopman FA, Chavan SS, Miljko S, Grazio S, Sokolovic S, Schuurman PR, et al. Vagus nerve stimulation inhibits cytokine production and attenuates disease severity in rheumatoid arthritis. Proc Natl Acad Sci. (2016) 113:8284–9. doi: 10.1073/pnas.1605635113
107. D’Haens G, Eberhardson M, Cabrijan Z, Danese S, Berg Rvd, Löwenberg M, et al. Neuroimmune modulation through vagus nerve stimulation reduces inflammatory activity in crohn’s disease patients: A prospective open-label study. J Crohn’s Colitis. (2023) 17:1897–909. doi: 10.1093/ecco-jcc/jjad151
108. Genovese MC, Gaylis NB, Sikes D, Kivitz A, Lewis Horowitz D, Peterfy C, et al. Safety and efficacy of neurostimulation with a miniaturised vagus nerve stimulation device in patients with multidrug-refractory rheumatoid arthritis: a two-stage multicentre, randomised pilot study. Lancet Rheumatol. (2020) 2:e527–38. doi: 10.1016/S2665-9913(20)30172-7
109. Kheiri B, Abdalla A, Osman M, Haykal T, Chahine A, Ahmed S, et al. Meta-analysis of carvedilol for the prevention of anthracycline-induced cardiotoxicity. Am J Cardiol. (2018) 122:1959–64. doi: 10.1016/j.amjcard.2018.08.039
110. Schmelz M, Mantyh P, Malfait AM, Farrar J, Yaksh T, Tive L, et al. Nerve growth factor antibody for the treatment of osteoarthritis pain and chronic low-back pain: mechanism of action in the context of efficacy and safety. Pain. (2019) 160:2210–20. doi: 10.1097/j.pain.0000000000001625
Keywords: cancer, sympathetic nervous system, chemotherapy, beta-blocker, metastasis, neuron
Citation: Manoleras AV, Sloan EK and Chang A (2024) The sympathetic nervous system shapes the tumor microenvironment to impair chemotherapy response. Front. Oncol. 14:1460493. doi: 10.3389/fonc.2024.1460493
Received: 06 July 2024; Accepted: 30 August 2024;
Published: 24 September 2024.
Edited by:
Aparna Jayachandran, Fiona Elsey Cancer Research Institute, AustraliaReviewed by:
Bernhard Riedel, Peter MacCallum Cancer Centre, AustraliaAli H Zahalka, University of Texas Southwestern Medical Center, United States
Copyright © 2024 Manoleras, Sloan and Chang. This is an open-access article distributed under the terms of the Creative Commons Attribution License (CC BY). The use, distribution or reproduction in other forums is permitted, provided the original author(s) and the copyright owner(s) are credited and that the original publication in this journal is cited, in accordance with accepted academic practice. No use, distribution or reproduction is permitted which does not comply with these terms.
*Correspondence: Aeson Chang, YWVzb24uY2hhbmdAbW9uYXNoLmVkdQ==