Erratum: Clinical insights into nanomedicine and biosafety: advanced therapeutic approaches for common urological cancers
- 1Student Research Committee, School of Advanced Technologies in Medicine, Shahid Beheshti University of Medical Sciences, Tehran, Iran
- 2Reza Radiotherapy and Oncology Center (RROC), Mashhad, Iran
- 3Legal Medicine Organization, Tehran, Iran
- 4School of Medicine, Guilan University of Medical Sciences, Rasht, Iran
- 5School of Medicine, Shahroud University of Medical Sciences, Shahroud, Iran
- 6School of Medicine, Mazandaran University of Medical Sciences, Sari, Iran
- 7Islamic Azad Tehran University of Medical Sciences, Tehran, Iran
- 8Autoimmune Diseases Research Center, Kashan University of Medical Sciences, Kashan, Iran
- 9Coenzyme R Research Institute, Tehran, Iran
- 10Shiraz University of Medical Sciences, Shiraz, Iran
Urological cancers including those of the prostate, bladder, and kidney, are prevalent and often lethal malignancies besides other less common ones like testicular and penile cancers. Current treatments have major limitations like side effects, recurrence, resistance, high costs, and poor quality of life. Nanotechnology offers promising solutions through enhanced diagnostic accuracy, targeted drug delivery, controlled release, and multimodal imaging. This review reflects clinical challenges and nanomedical advances across major urological cancers. In prostate cancer, nanoparticles improve delineation and radiosensitization in radiation therapy, enable fluorescent guidance in surgery, and enhance chemotherapy penetration in metastatic disease. Nanoparticles also overcome bladder permeability barriers to increase the residence time of intravesical therapy and chemotherapy agents. In renal cancer, nanocarriers potentiate tyrosine kinase inhibitors and immunotherapy while gene vectors and zinc oxide nanoparticles demonstrate antiproliferative effects. Across modalities, urological applications of nanomedicine include polymeric, liposomal, and metal nanoparticles for targeted therapy, prodrug delivery, photodynamic therapy, and thermal ablation. Biosafety assessments reveal favorable profiles but clinical translation remains limited, necessitating further trials. In conclusion, nanotechnology holds significant potential for earlier detection, precise intervention, and tailored treatment of urological malignancies, warranting expanded research to transform patient outcomes.
Introduction
Urological malignancies arising in the kidney, bladder, and prostate are prevalent cancers with substantial morbidity and mortality (1). They stand out as prevalent and lethal malignancies within the genitourinary system, and current theranostic strategies for these cancers are deemed inadequate, marked by limitations in sensitivity, specificity, efficacy, safety, and overall quality of life (2).
The drawbacks of current treatments for urological cancers are multifaceted and depend on the type and stage of cancer, as well as the patient’s age, health status, and preferences. Some common drawbacks of current treatments include the high risk of acute and long -term side effects that negatively impact patient well-being. For example, radical surgical procedures may lead to chronic pain, nausea, fatigue, and sexual dysfunction. Similarly, radiation and chemotherapy often cause severe gastrointestinal adverse events and cognitive impairment. Such side effects can be disabling or even life-threatening in elderly patients with comorbidities (1, 3). Additionally, localized urological cancers often recur and progress despite initial treatment. In the metastatic setting, hormone, and chemotherapies provide transient control but eventually spawn lethal therapeutic resistance (4, 5).
From diagnosis to end-of-life care, patients experience cumulative adverse events ranging from acute catheterizations, stomas, and cytopenias to long-term cognitive changes and secondary malignancies. Exorbitant costs further compound the physical and emotional side effects.
Thus, there is an unmet need for new diagnostic and therapeutic paradigms tailored to the unique biology of genitourinary tumors and the sensibilities of affected patients (6, 7).
To overcome these drawbacks, there is a need for novel and personalized approaches to urological cancer management, such as immunotherapy, targeted therapy, precision medicine, and multidisciplinary care. Realizing these opportunities necessitates additional preclinical validations and ethical evaluations to translate nanomedicine into widespread urologic practice responsibly. With concerted efforts across scientific disciplines and institutional infrastructures, nano-enabled care stands to make substantive impacts on the early detection, risk-stratification, and tailored treatment of genitourinary cancers.
Nanotechnology, offering advantages in urological cancer theranostics like enhanced permeability, targeted delivery, controlled release, and multimodal imaging, has explored various nanoparticle (NP) types such as metallic, polymeric, liposomal, and carbon-based NPs. Gold NPs enable a rapid urinary test for bladder cancer (BC), while magnetic NPs are utilized in magnetic resonance imaging and hyperthermia therapy for prostate cancer (PCa). Additionally, polymeric NPs have shown efficacy in chemotherapy and gene therapy for BC (8). However, despite the promising potential of nanotechnology, it is essential to ensure the biosafety of novel treatments. Biosafety refers to the assessment and management of the potential risks of nanomaterials to human health and the environment. Some of the factors that affect the biosafety of nanomaterials include their size, shape, surface charge, chemical composition, biodegradability, biodistribution, and biocompatibility. Therefore, it is essential to conduct rigorous preclinical and clinical studies to evaluate the biosafety of nanomaterials before they can be translated into clinical practice (9, 10). In this review, we aim to provide a comprehensive and updated overview of the role of nanotechnology in urological cancer treatment and the biosafety of previous studies, focusing on clinical relevance and insight. We also will discuss the current challenges and opportunities of nanotechnology in urological cancer, and highlight the future directions and perspectives of this promising field.
Prostate cancer
In 2020, approximately 1.4 million cases of PCa were reported globally, making it the second most frequently identified cancer among men (11). The number of deaths due to PCa in 2020 was 375,000 worldwide. According to the World Health Organization (WHO), PCa is the most often diagnosed male cancer in 112 countries and the leading cancer-related death in 48 countries. Moreover, corresponding socio-economic burden is enormous; PCa treatment costs increase more rapidly than for any other cancer (12).
Current treatment options and their limitations
Localized PCa has various treatment options resulting in controversy among experts and confusion leading to frustration and stress in patients (13).
Surgical prostatectomy is an important treatment option for all risk groups of localized disease and even for selected patients with regional nodal metastasis. Although surgical indications become more limited as medical treatment options for PCa continue to expand, radical prostatectomy remains a crucial option in many patients (14). The improvement of anatomic knowledge and technical methods as well as the advent of laparoscopy and robotic-assisted surgery, have changed the historically morbid and lethal prostatectomy to a rather safe procedure becoming a common treatment approach for localized PCa (15, 16); However, this treatment method is still invasive and faces the risk of complications such as blood loss, postoperative pain, surrounding structures injury, reoperation, infection, urine leak, incontinency, long-time catheterization, anastomotic stricture, thromboembolism, heart attack, and death (16). In addition, there is still no consensus about some technical decisions such as whether to dissect lymphatics or not (17).
Radiation therapy has also undergone significant advancements as the pivotal treatment modality for most cases of local and locally advanced PCa. There is a remarkable increase in the utilization of highly conformal intensity-modulated radiation therapy (IMRT), and image-guided radiation therapy (IGRT), which implements fiducial markers placed in the targeted tissue for daily localization, and stereotactic external beam radiation therapy, irradiating small target volumes with high doses of radiation in few fractions (13). Interstitial brachytherapy is also widely used alone or as an additional radiation boost for localized PCa patients (18).
Although all these methods take effective steps towards increasing the radiation dose to the target and saving healthy organs which is particularly important, when it comes to cancers that occur in the complex anatomical site of the pelvis, various acute and chronic side effects of radiation therapy continue to bother PCa patients, especially in the first four years after treatment (13). There is a wide range of radiation side-effects including acute complications caused by inflammation such as cystitis, urethritis, and proctitis manifesting as frequency, urgency, and discomfort during urination or bowel movements (19). Such side effects force patients to make strict dietary changes and take more medications to control side effects (20). On the other hand, there are long-term complications, like various disorders related to sexual and digestive functions and probable strictures and obstructions, which, despite having a greater impact on the quality of life of patients, are more neglected than acute complications (21, 22).
Other than medical castration and local palliative treatments, systemic treatment for metastatic PCa, specifically metastatic Castration-Resistant Prostate Cancer (mCRPC), includes a few chemotherapeutic options, targeted therapy, and immunotherapy (23). Chemotherapy leads to multiple acute and chronic side effects from gastrointestinal discomfort and hair/skin conditions to serious toxicities such as cytopenia, immune suppression, leukemia, and severe hypersensitivity reactions (24). On many occasions, the severity of toxicities results in dose modification and treatment discontinuation. Immunotherapeutic drugs that engage the patient’s immune system in the battle against malignant cells have drawn enormous attention recently. Although promising in multiple malignancies, immunotherapy provides few options with currently less than significant results for PCa, including programmed cell death protein 1 (PD-1) and cytotoxic T-lymphocyte antigen 4 (CTLA-4) blockage and vaccine-based pharmaceuticals (25, 26). While investigations on the role of immunotherapy in metastatic PCa continue to evolve, concerns are raised regarding a wide and not fully understood range of treatment-related toxicities due to the close connection of the immune system with various organ systems and random events of systemic immune activation caused by the intravenous systemic drug administration (27).
Nuclear medicine and bone-seeking radiolabeled pharmaceuticals have demonstrated encouraging results in metastatic PCa treatment, particularly since the discovery of the prostate-specific membrane antigen (PSMA) protein as a beneficial diagnostic and therapeutic target (28); However, multiple adverse effects, including anemia, changes in blood parameters, liver enzyme elevation, nephrotoxicity, fatigue, nausea, and dry mouth, are reported in a considerable proportion of patients (20).
Androgen deprivation therapy (ADT) is a form of hormonal intervention used as a major component in the treatment of PCa across all risk groups (23). PCa’s proliferation is testosterone dependent whether it is local or metastatic and suppression of the androgen-signaling axis is a key ingredient in PCa management recipe. One routine option that is still recommended in individual metastatic patients is the surgical removal of testicles (orchiectomy); However, medical castration with Luteinizing Hormone-releasing Hormone (LHRH) agonists and antagonists, antiandrogens, and novel enzymatic steroidogenesis inhibitors is the preferred option with manageable duration (19). Castration, regardless of whether it is surgical or medical, often leads to various adverse effects that can be a source of frustration and challenges for patients. These side effects include sexual problems, hot flashes, metabolic disorders, muscle mass decline, sleep disturbances, mental health issues, genitourinary difficulties, bone loss, weight gain, and anemia, all of which can significantly affect the patient’s quality of life. Whether surgical or medical, castration results in significant toxicity which is the major source of challenge and frustration in most cases. These adverse effects include a long list of highly prevalent effects such as sexual problems, hot flashes, metabolic disorders, muscle mass decline, sleep disturbances, mental health issues, genitourinary difficulties, bone loss, weight gain, and anemia, all of which dramatically affects the patient’s quality of life. On the other hand, castration resistance, meaning disease recurrence or progression while sufficient testosterone suppression is evidenced, eventually occurs in all patients treated with ADT (29). This will leave the patient with limited more toxic treatment options such as chemotherapy and immunotherapy. Increasing the accuracy of drug delivery to the cellular level can prevent further development of drug resistance that deprives the patient of the remaining options (30).
Delivery of drugs to malignant cells can significantly reduce side effects in different scenarios of PCa, such as localized, metastatic, or castration resistance. This is the primary topic of discussion in nanomedicine.
Nanotechnology applications in PCa therapy
Nanotechnology has a meaningful role in urological cancers, especially PCa in the field of prevention, diagnosis, and treatment. It can address multiple aspects of treatment drawbacks mentioned above, in nearly every treatment modality. Better chemotherapy drug delivery can cause a higher rate of remission. There are a variety of uses like magnetic, lipid-based, and gold NPs; Progress in imaging can facilitate the diagnosis and NPs can enhance water solubility and accumulation in targeted cells for chemotherapy drugs which causes better therapeutic outcomes (31).
In surgery, the accumulation of nanoparticles in the diseased area, specifically metal nanoparticles such as Gold, can increase the detection accuracy before and during the operation (32, 33). Moreover, the nanoparticle can be further improved via conjugation to specific ligands targeting antigens like PSMA (34), or formulation with ablative agents to perform the treatment on its own (35, 36). In a recent study, Wu et al. developed a Cy-KUE-OA nanoprobe to facilitate PCa surgery via fluorescence guidance. They utilized Glutamate-urea-lysine (KUE) as a promising PSMA ligand and Oleic acid (OA), a monounsaturated fatty acid that is actively accumulated in malignant cells, to deliver a cyanine-based fluorescent dye which is activated after both uptake and infrared radiation (37). This methodology can be performed with various ligand types, vehicles and active substances, taking benefit from the flexibility and structural versatility. Teh et al. incorporated nanoprobe technology in two modalities (38). They reported successful implementation of targeted nano-probes for intraoperative visualization of tumoral cells and postoperative targeted drug delivery in mice models and proposed the potential of this method to improve multimodality treatment in clinical studies.
In radiation therapy, remarkable advances in technology, accuracy and variety of modalities of radiotherapy devices in the last twenty years have coincided with the emergence and expansion of the use of nanotechnology in medicine (39). With the spread of modern complex radiotherapy techniques using highly non-uniform radiation intensity to deliver elegant conformal dose distributions that are especially useful in the treatment of pelvic tumors such as prostate, accurate diagnosis of the tumor area has become much more important comparing to previous 2D and 3D techniques (13). Precise delineation of the tumor is the first key to an optimal treatment planning and radiation delivery resulting in high tumor dose (more than 80 Gy to the planning target volume [PTV] in IMRT) and less organ-at-risk radiation (40, 41). This crucial factor can be improved using fluorescent or metal nanoparticles targeting and accumulating in the malignant cells. Current imaging techniques used for tumor delineation discriminate the tumoral tissue based on differences in contrast, functional attenuation, or magnetic resonance, whilst nanoparticles can detect the malignancy on cellular level. On the other hand, the particles can also act as targeted radiosensitizers (42, 43). Moeendarbari et al. took a step further and incorporated the radioisotope palladium-103 in gold nanoparticles to develop an internal radiation treatment for unresectable tumors and reported a dramatic response of more than 80% tumor shrinkage in PCa xenograft models (44). Currently, ongoing clinical trials are investigating various applications of nanomedicine in treatment with ionizing radiation; However, modern paradigms such as tumor microenvironment and immunogenicity must be taken into consideration before the adoption of these methods into patient treatment (42).
Numerous systemic therapeutics can become incorporated in nanostructures to enhance the effect, target specific cells, and save normal organ-systems (45). Nanomedicine drug delivery systems can increase drug solubility, extend half-life, and regulate drug release by adjusting their chemical and physical properties such as hydrophilicity, charge, size, and the nature of their surface ligands. Moreover, nanomedical drug conjugates can penetrate target cells in PCa via mechanisms mediated by receptors, making them the front line of anti-cancer drug experiments (46). These features become specifically beneficial regarding castration resistant PCa, in which the only effective options left are a number of systemic treatments, the maximum efficiency of which and avoiding discontinuation of treatment due to complications is critical (47). Most PCa deaths are caused by Castration-Resistant Prostate Cancer (CRPC), and CRPC patients are at high risk of developing drug resistance. Previous studies have developed new nanoparticles called lipid-calcium-phosphate arginine-glycine-aspartic acid (LCP-RGD) NPs that can deliver small interfering RNA (siRNA) and Docetaxel (DTX), resulting in significant therapeutic effects. By encapsulating the chemotherapeutic agent, with dioleoyl phosphatidic acid (DOPA) and distearoyl phosphatidylethanolamine-polyethylene glycol (DSPE-PEG), the calcium phosphate core of the NPs can efficiently aggregate the negatively charged siRNA. The synergistic effects of DTX and GRP78 siRNA are associated with changes in the cell cycle, autophagy, and apoptosis following GRP78 silencing, which can enhance the destruction of tumor cells by DTX (47).
In addition to the role of nanomedicine in the conventional treatments mentioned above, a wide range of herbal and complementary treatments can be formulated in nanoparticles, alone or in combination with chemotherapy or immunotherapy, as reviewed in detail by Cherian et al. (48). These complementary medications can further enhance the treatment efficacy or reduce side-effects, without changing the original method, treatment time, or number of administrations. Additional encouraging uses of nanomedicine in the treatment of PCa including gene therapy via delivering mRNA structured with antiproliferative and apoptotic effects, photodynamic therapy using photosensitizing agents, and immunotherapy (Table 1).
Nanotechnology’s impact on PCa treatment extends to advanced targeted therapy and image-guided interventions. Through the use of nanocarriers, therapeutic agents can be precisely delivered to PCa cells, enhancing treatment specificity. Targeted therapy at the molecular level allows for tailored approaches, interfering with specific signaling pathways involved in cancer growth (72, 73). Regardless of the type of treatment, the prognosis varies and is influenced by many factors, but all treatments can affect sexual function and quality of life to different extents. The integration of nanotechnology in targeted therapy and image-guided interventions represents a significant advancement in the field of PCa treatment. By combining therapeutic precision with real-time monitoring, nanotechnology holds the potential to improve treatment outcomes, minimize side effects, and offer a more personalized and effective approach to managing PCa (Table 1 and Figure 1).
Bladder cancer
In 2020, bladder cancer (BC) ranked as the tenth most frequently identified cancer globally, with an estimated 573,000 new cases and 213,000 deaths attributed to the disease (74). The American Urological Association (AUA) provides guidelines for classifying non-muscle invasive bladder cancer (NMIBC) into high-, intermediate-, and low-risk groups, which helps in tailoring treatment and surveillance strategies; both carry significant morbidity rates (75, 76). Despite advancements in treatment, NMIBC is notorious for its high recurrence rates, which can range from 15% to 61% within the first year and between 31% and 78% within five years. The economic impact of managing and treating bladder cancer which is increasing globally is substantial, with costs associated with diagnosis, treatment, and follow-up care contributing to a significant financial burden on healthcare systems (77).
Current treatment options and their limitations
Intravesical therapy has become a mainstay in the management of NMIBC. This approach involves the direct instillation of therapeutic agents into the bladder, targeting residual malignant cells following transurethral resection. The agents used for intravesical therapy include Bacillus Calmette-Guérin (BCG), mitomycin C, and gemcitabine (78). These agents can be used alone or in combination to induce cytotoxic effects or stimulate an immune response against cancer cells. However, the effectiveness of intravesical therapy is often hampered by the bladder’s natural permeability barrier, which limits drug penetration and reduces the duration of drug residence in the bladder, potentially leading to treatment failure (79, 80). Although intravesical treatment is widely utilized as the main tool to prevent recurrence in superficially resected BC, current management of NMIBC is far from sufficient. One-year recurrence rates and 5-year recurrence rates are reported in a range of 15–61% and 31–78% respectively (81). The bladder permeability barrier (BPB) on the urothelial surface decreases substance penetration and the repeating emptying of the drug and its dilution by urine leads to short residence time, unsustainability, low penetration, and failure to release with consistency (82).
To address these challenges, researchers are exploring innovative strategies to enhance the delivery and efficacy of intravesical therapy. These include the development of nanocarriers that can improve drug solubility and retention, magnetic particles that can be directed to tumor sites, and gene therapy approaches that target specific molecular pathways involved in bladder cancer progression (78, 83, 84).
For MIBC, treatment typically involves more aggressive interventions, with radical cystectomy being the standard of care. This surgical procedure entails the complete removal of the bladder and surrounding organs, including the prostate in males and, in females, the anterior exenteration of the bladder, urethra, vaginal wall, and uterus. While radical cystectomy is effective, it is also associated with significant morbidity and impacts on quality of life. Partial cystectomy is rarely feasible and there is often the need for neobladder construction via reconfiguring a portion of the intestine (85, 86).
Although robotic-assisted and laparoscopic surgeries, which are increasingly being adopted in clinical practice, offer less invasive alternatives with the potential for reduced complications and quicker recovery times (87), the invasive nature of the optimal therapeutic surgery still leads to various short- and long-term complications including multiple metabolic and neuromechanical side effects. Replacing the bladder with an absorptive structure results in numerous electrolyte imbalances and vitamin deficiencies which then cause dysfunction in other organ systems (85), as well as long-term complications such as obstructions and strictures. Tumor recurrence is another common consequence in some studies, 86% of which developed within the first 3 years of cystectomy (88). While certain individuals may derive advantages from the seemingly comparable option of bladder-conserving three-modality therapy, involving transurethral resection followed by chemoradiotherapy, this approach comes with its own array of negative outcomes (89), which can be mitigated to a considerable degree through the utilization of nanomedical drug delivery methods.
Radiation therapy, chemotherapy, and immunotherapy serve as adjunctive treatment modalities for both NMIBC and MIBC cases. Local resection along with radiation and chemotherapy is also an option for local MIBC cases who are not selected for radical therapy or have negative features in the pathologic assessment of the surgical tissue (80, 86). However, each modality presents its own set of challenges. Radiation therapy, despite the use of modern techniques such as IMRT and IGRT, may lead to genitourinary and gastrointestinal dysfunction due to challenges in adequately targeting and sparing normal tissues (90). Although chemotherapy and immunotherapy are novel treatment options that have shown promise in clinical trials, they are not yet widely available and have numerous side effects that are neither completely investigated in research nor sufficiently familiar in the clinic (27).
The economic impact of bladder cancer is substantial, with management costs being among the highest for any malignancy. Recurrent disease, the need for ongoing surveillance, and the introduction of novel therapies all contribute to the financial burden of bladder cancer care. In the European Union, bladder cancer treatment accounts for approximately 3% of all cancer costs, representing a significant healthcare expenditure (91).
Enhancements in the biological comprehension of bladder cancer have revealed new potential targets for BC treatment strategies. Among these advancements are immunotherapy, targeted therapy, and antibody-drug conjugates, all of which have demonstrated potential in bettering patient outcomes with bladder cancer (92).
Nanotechnology applications in BC therapy
The clinical management of bladder cancer presents a complex array of challenges. A key issue is the high propensity for tumor recurrence and progression following initial treatment modalities. This complication arises in part from the limitations inherent to current diagnostic techniques, which may fail to accurately detect and characterize the disease state. The ability to reliably distinguish between NMIBC and MIBC is crucial, as these subtypes warrant distinct therapeutic approaches and clinical monitoring strategies. Consequently, there remains a pressing need for enhancements to the existing paradigms governing bladder cancer diagnosis, treatment selection, and post-treatment surveillance in order to improve overall patient outcomes and mitigate the substantial socioeconomic burden imposed by this malignancy (79).
Adhering to a strong variety of uroplakins and transmembrane proteins, the bladder mucosal layer poses a significant barrier to the effective entry of therapeutic medicines into neoplastic tumors (93, 94). Overcoming this biological impedance necessitates innovative therapeutic strategies. In this regard, the burgeoning fields of nanomedicine and biomaterials engineering have made notable strides, holding considerable potential for facilitating targeted intravesical delivery of anticancer agents; a group of researchers, for example, fabricated a poly-(L)-glutamic acid-based nanoparticle system to deliver drugs specifically to bladder cancer cells via intravesical administration. They utilized the lectin WGA as a targeting ligand to facilitate binding and internalization of the nanoparticles by bladder tumor cells, thereby enhancing therapeutic efficacy while overcoming the barrier imposed by the bladder mucosal lining. These cutting-edge approaches may not only enhance drug bioavailability at the tumor site, but could also synergize with and augment the efficacy of existing treatment modalities (95). Nanomedicine and biomaterial-based drug delivery platforms offer innovative approaches to enhance bladder cancer treatment outcomes (96). The unique advantages offered by nanotechnology in cancer treatment applications include improved drug efficacy and/or reduced toxicity, spatial and temporal drug release, enhanced drug stability, solubility, and tumor site accumulation, facilitated delivery of polymeric drugs to intracellular sites of action, codelivery of multiple drugs or therapeutic modalities for combination therapy, improved diffusion of drugs across tight epithelial and endothelial barriers, and sensitive imaging and accurate diagnosis with visible drug delivery or real-time feedback on the in vivo efficacy of a therapeutic agent, advantages that have been described in previous reviews (31, 94).
Expanding the purview beyond this, an investigation demonstrated that nano contrast agents used in conjunction with MRI, proffer a non-invasive modality for monitoring prognosis and therapeutic responses among patients afflicted with bladder cancer, furnishing clinicians with invaluable insights into disease trajectory and therapeutic efficacy (97). Photothermal nanoprobes (PNPs) represent an epochal innovation, endowed with the capacity to discriminate cancerous cells through thermogenic or fluorescent mechanisms under infrared illumination. This pioneering approach portends unprecedented potential for early detection, precise localization, and targeted therapeutic interventions, heralding a paradigm shift in bladder cancer diagnostics and therapeutics. Moreover, PNPs loaded with DOX have exhibited a protracted half-life subsequent to intravenous administration, eclipsing the efficacy of DOX in isolation and potentially obviating the exigency for recurrent dosing, thereby engendering diminished patient burden and heightened treatment compliance (98).
In advancing nanomedicine for bladder cancer, biosafety considerations are paramount. Ensuring the biocompatibility and safety of nanotechnological interventions is imperative for their translation into clinical practice. While nanomedicine holds immense promise, addressing concerns regarding toxicity, immunogenicity, and long-term effects is crucial for its widespread adoption. Rigorous preclinical evaluation and regulatory oversight are essential to ascertain the safety profile of nanomedicine techniques, fostering confidence in their clinical utility.
In Tables 1–3, we review recent nanomedical approaches in bladder cancer as well as other common urological cancers, encompassing polymeric NPs, lipid NPs, metallic NPs, and their applications in drug delivery, imaging, and therapeutic interventions. These advancements exemplify the potential of nanotechnology in revolutionizing bladder cancer management (Figure 2).
Kidney cancer
Renal cancer accounted for an estimated 431,000 new cases globally in 2020, according to the World Health Organization (74) It carries a significant financial cost, which includes both direct medical expenses and indirect expenditures related to disability and loss (137). Renal cell carcinoma (RCC) is the most common type of kidney cancer, accounting for approximately 90% of all renal malignancies (138). The American Joint Committee on Cancer (AJCC) tumor-node-metastasis (TNM) staging system is the most widely utilized for RCC, where stage I and II denote localized tumors, stage III indicates regional lymph node involvement, and stage IV signifies distant metastases (139). Irrespective of disease stage, surgical resection remains the cornerstone of curative treatment for RCC in medically operable patients without contraindications (140).
Current treatment options and their limitations
Surgery forms the cornerstone of treatment for RCC across all stages, whenever feasible for medically eligible patients without contraindications (141). In addition to radical or partial nephrectomy, surgical removal of metastases is an option for those with stage IV disease (142). Furthermore, ablative therapies such as thermal ablation and Stereotactic Body Radiation Therapy (SBRT) are commonly employed to either shrink tumors or eliminate residual malignant cells without the need for invasive surgery (143).
In recent years, there has been widespread adoption of immunotherapy and targeted medications for advanced metastatic and recurrent RCC. Various novel agents are now standard treatments in adjuvant, metastatic, and recurrent settings, either alone or in combination therapy (144). However, it is important to note that each treatment option has its own set of drawbacks and limitations.
Surgical management as a local treatment of RCC, is a complex procedure that needs a high level of expertise, experience, and qualification. It is highly recommended to be done in high-volume centers and under the supervision of a multi-disciplinary team (145). Other than general surgical complications such as blood loss, surgical site infection, anesthesia side effects, and cardiovascular events, the surgical opening is a highly morbid incision, and removing the whole kidney results in an average 35% decrease in glomerular filtration rate (GFR) (85). Although partial excision and minimally invasive approaches are widely adopted to overcome the morbid outcomes correlated with total nephrectomy, these procedures need more expertise and often result in a higher recurrence rate due to the presence of positive surgical margins or even other malignant tumors in the remaining part of the kidney (143).
Thermal ablative techniques including Radiofrequency Ablation (RFA), Cryotherapy, and Microwave Ablation (MWA) are minimally invasive options with surgical, endoscopic, or percutaneous approaches (146). Although these methods are capable of sparing the kidney from surgical approaches, they have a learning curve and a relatively high local failure rate; Moreover, since no pathological specimen is achieved in contrast to the surgical approaches, precise histopathologic diagnosis is not possible. The imaging follow-up of these patients is also a challenge regarding the structural disruption (85, 147). SBRT is a radiation treatment indicating the use of high doses in a few fractions. Despite the ability of dose escalation to overcome the radioresistant nature of RCC being utilized as an ablative treatment in specific indications, it can result in gastrointestinal or genitourinary toxicity sometimes limiting the ultimate delivered dose (148, 149).
In systemic therapy of RCC, the main components are tyrosine kinase inhibitors (TKI) and immunotherapeutic drugs such as anti-PD-1 antibodies and anti-CTLA-4 antibodies. Chemotherapy is not generally used for RCC, except for specific subtypes in an advanced stage (150). TKIs are small molecules that inhibit the binding of adenosine triphosphate (ATP) to growth factor receptors further interrupting the intracellular cascade of phosphorylation and preventing the proliferation of malignant cells (151). Although these medications successfully prevent further tumoral proliferation in adjuvant and metastatic settings, they come with the expense of well-known adverse effects such as hypertension, diarrhea, fatigue, skin rash, and hand-foot syndrome (152).
The advent of immunotherapy dramatically changed treatment recommendations in RCC; However, as these agents alter the immune-related functions in multiple organ systems, they can result in a series of adverse effects such as inflammation in various organs and endocrine disturbances (27).
Nanotechnology applications in kidney cancer therapy
Nanotechnology has surfaced as a hopeful pathway for treating RCC, presenting novel methods to tackle the complexities associated with this cancer. Drug delivery methods, such as the combination of tyrosine kinase inhibitors with thermosensitive liposomes (TKI/TSL) stimulated by focused ultrasound (FUS), have shown effectiveness in enhancing drug accumulation in the tumor area and promoting regression (133). Recent findings challenge the idea that NPs may penetrate tumors through a Cav1-mediated transcellular route rather than the conventional enhanced permeability and retention (EPR) effect. Using a siRNA delivery technology (RGD-MEND), enhanced NP accumulation in vessel-rich cancers, like RCC, was observed. The study proposes a Cav1-mediated transcellular route, not the conventional EPR effect, for NP penetration into tumor vasculature. Experiments suppressing Cav1 with siRNA counteracted enhanced NP delivery, supporting the involvement of a Cav1-induced transcellular route in tumor NP accumulation (134). Exosomes, nano-sized vesicles crucial for intercellular communication, have also garnered attention, with exosomal miR-1 demonstrating significant inhibition of cell proliferation, migration, and invasion in RCC cells (153).
Exosomes, crucial for intercellular communication, involve Polymerase I and Transcript Release Factor (PTRF or CAVIN1), a potential biomarker in cancers. The study investigates the unknown mechanisms regulating exosome-related PTRF secretion. Exogenous and endogenous immunoprecipitation assays reveal UBE2O’s direct interaction and ubiquitination of PTRF. UBE2O inhibits PTRF’s impact on exosome secretion by decreasing caveolae formation. UBE2O decreases overall exosome secretion, leading to reduced PTRF release via exosomes. SDPR (CAVIN2) interacts with both UBE2O and PTRF, promoting PTRF expression in exosomes. The findings suggest that increasing UBE2O expression could be a novel approach for cancer treatment by controlling exosome-related PTRF secretion (154).
Gene therapy has also benefited from nanomedicine, with nanoparticles effectively delivering the AIM2 gene, leading to reduced cell proliferation, migration, and invasion while enhancing apoptosis in RCC cells (155). RCC lacks effective treatments, prompting interest in Absent in Melanoma 2 (AIM2) as a potential therapeutic target. AIM2 expression is significantly decreased in RCC patient specimens and renal carcinoma cell lines. Nanoparticles (H1/pAIM2) effectively deliver the AIM2 gene, increasing its expression and reducing cell proliferation, migration, and invasion while enhancing apoptosis in vitro. In vivo, intertumoral injection of H1/pAIM2 NPs inhibits tumor growth in xenograft mice. The therapeutic efficacy of H1/pAIM2 is associated with enhanced inflammasome activation, suggesting its potential as an efficient therapeutic approach for RCC treatment (155). Additionally, zinc oxide nanoparticles (ZONs) have demonstrated potential in inhibiting the RCC cell proliferation and viability by impeding lipid accumulation, oxidative stress, and reactive oxygen species (ROS) levels while upregulating miR-454-3p which targets ACSL4 (156).
Photothermal therapy (PTT) has also been explored, with an injectable thermosensitive hydrogel containing curcumin-loaded hollow mesoporous organosilica nanoparticles (Cur@HMON@gel) demonstrating sustained and controlled release of curcumin under ultrasound irradiation, generating ROS for sonodynamic tumor therapy (SDT). In vivo studies show that this nano-platform has high biocompatibility and biodegradability, with the potential for clinical translation in solid tumor eradication (132). Furthermore, a combination of the anticancer drug lonidamine (LND) and polydopamine (PDA) loaded onto stellate mesoporous silica nanoparticles (MSNs) and cloaked with RCC membranes (MLP@M) has exhibited enhanced synergistic effects when triggered by an 808 nm laser, improving antiproliferative and tumor-suppressing abilities for RCC treatment (157).
In the realm of image-guided surgery in RCC, extracellular vehicles (EVs) and circulating tumor cells (CTCs) have emerged as potential biomarkers, with optimized isolation protocols and microfluidic devices incorporating photovoltaic-based surface-enhanced Raman spectroscopy (SERS) platforms and shell-isolated nanoparticles (SHINs) enabling sensitive and specific identification of these biomarkers in renal cancer (158, 159). Furthermore, the RUBYchip™, a microfluidic label-free CTC detection platform, has demonstrated a remarkable efficiency of 74.9% in spiking experiments and effective clinical validation in RCC patients, positioning it as a promising tool for CTC detection in RCC management (160). By enabling sensitive and specific detection of biomarkers like EVs and CTCs, nanotechnology is providing powerful tools for image-guided surgery in RCC. These liquid biopsy approaches can reveal key information about the tumor’s presence, location, molecular characteristics, and spread - all of which can inform and guide surgical interventions and treatment strategies for optimal outcomes.
Combination therapies leveraging nanotechnology have emerged as a new approach to address the challenges associated with RCC treatment. One noteworthy development involves the investigation of long non-coding RNA SLERCC, which has demonstrated potential as a therapeutic target due to its tumor-suppressive effects. Plasmid-encapsulated nanomaterials have been explored as a novel avenue for delivering SLERCC-based therapies, offering a targeted and efficient approach to RCC treatment (161). Another breakthrough strategy involves the development of a nanoplatform that combines the tyrosine kinase inhibitor Sorafenib with a CA IX-targeted apoptosis inducer. This innovative platform effectively addresses drug resistance, a major hurdle in RCC treatment, by selectively inhibiting the growth of resistant tumors. Remarkably, this nanoplatform has demonstrated significant inhibition of resistant tumor growth, highlighting its potential as a selective and effective therapeutic approach (128). Additionally, the PH1/pHGFK1 nanoparticle has shown remarkable efficacy in inhibiting RCC growth and enhancing survival in xenografted mice models. This nanoparticle system leverages a synergistic strategy, combining multiple therapeutic modalities to achieve superior antitumor effects. The good results observed with the PH1/pHGFK1 nanoparticle underscore the potential of integrating nanotechnology with synergistic treatment approaches for more effective RCC management (135). In metastatic RCC, a combination of tyrosine kinase inhibitors and gold nanorods with photothermal ablation has showcased a synergistic effect (126), and cuprous oxide nanoparticles (CONPs) have emerged as a potential solution for sunitinib-resistant RCC by disrupting copper transportation and inducing apoptosis (136). These collective findings underscore the diverse and innovative approaches explored in RCC research, with combination therapies leveraging nanotechnology playing a pivotal role in overcoming treatment challenges and improving patient outcomes (Table and Figure 3).
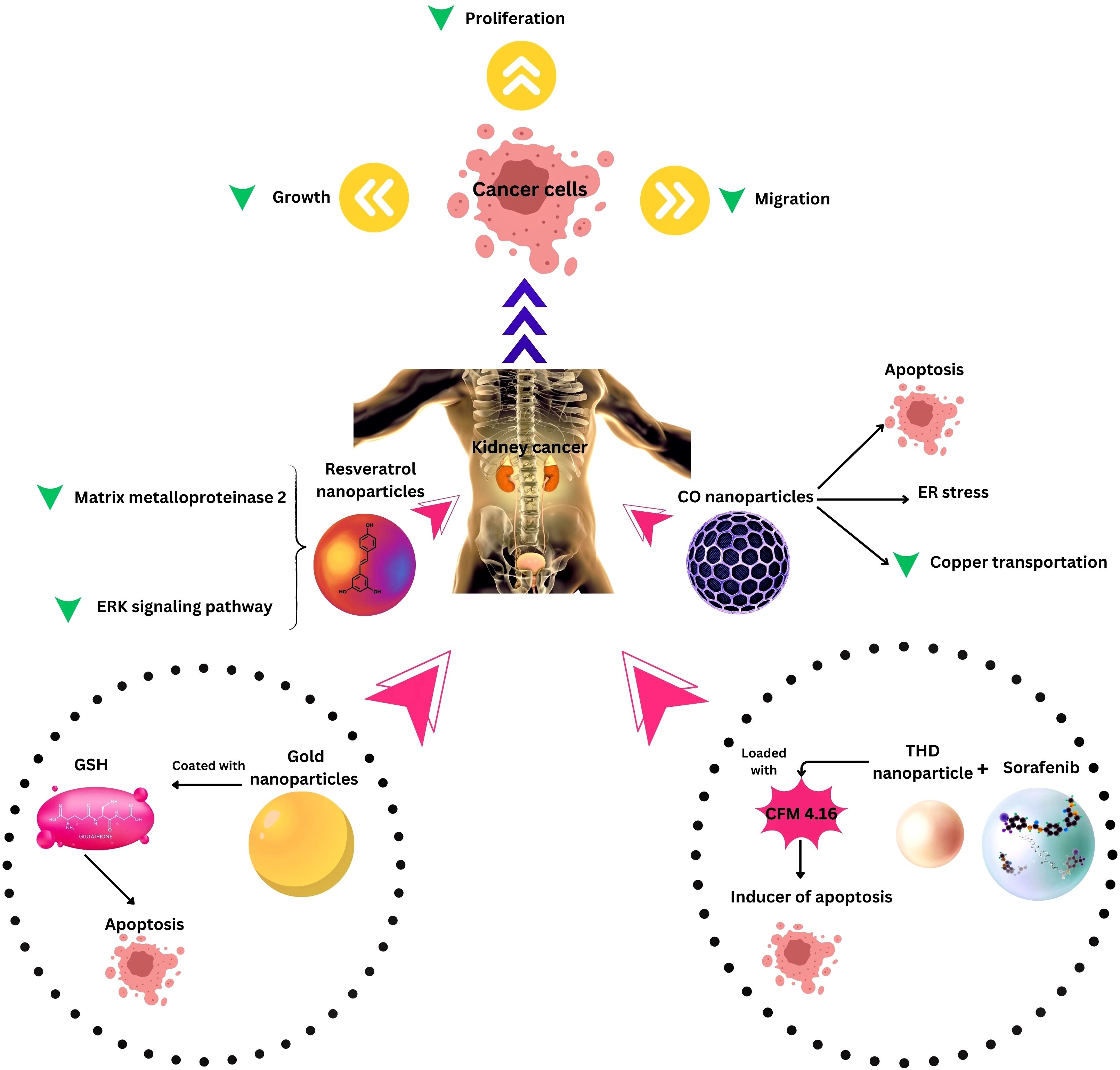
Figure 3. Nanotechnology applications in Kidney Cancer Therapy. ER, Endoplasmic reticulum; THD, Tumor hypoxia directed; ERK, extracellular signal-regulated kinase, CO, Cuprous oxide.
Less common urological cancers and current drawbacks
While prostate and bladder cancers are among the most prevalent urological malignancies, testicular, penile, and urethral cancers, though less common, can have a significant impact on patients’ lives. Testicular cancer, despite its relatively low incidence rate, is the most commonly diagnosed cancer among men aged 15-35 years (162, 163). Penile cancer, a rare malignancy, accounts for approximately 0.4-0.6% of all male cancers, but can have devastating psychological and functional consequences (164, 165). Upper tract urothelial carcinomas, while infrequent, significantly impact affected individuals due to its challenging treatment and potential consequences on quality of life (162, 166).
Current drawbacks in less common urological cancers
Treatment modalities, including surgery, radiation therapy, chemotherapy, and immunotherapy, aim to eradicate or control the disease. Surgical interventions, radiation therapy and chemotherapy regimens, while essential in these disease management, may cause adverse effects such as urinary incontinence, sexual dysfunction, and fatigue, further impacting patients’ physical and emotional well-being. These malignancies not only pose a threat to patients’ physical health but also carry a substantial psychosocial and economic burden, often leading to impaired quality of life, emotional distress, and financial strain (167, 168). Given the unique challenges associated with these cancers, there is an urgent need for innovative therapeutic approaches to improve patient outcomes and alleviate the burden on individuals and healthcare systems.
Nanotechnology applications in less common urological cancers
Nanomedicine has also shown promise in addressing the challenges associated with the treatment of less common urological cancers, including testicular, penile, and urinary tract cancers. In testicular cancer, nanoparticle-based approaches have been explored for targeted drug delivery, improving therapeutic efficacy, and reducing systemic toxicity. Hyaluronic acid (HA)-based nanoparticles have demonstrated enhanced tumor accumulation and superior antitumor activity compared to free cisplatin, a commonly used chemotherapeutic agent (139, 140). Additionally, gold nanoparticles (AuNPs) have been investigated for their ability to induce localized hyperthermia upon near-infrared (NIR) light exposure, leading to cancer cell death through photothermal therapy (PTT) (169, 170). In penile cancer, ultrasmall superparamagnetic iron oxide nanoparticles (USPIOs) have been studied in conjunction with lymphotropic nanoparticle-enhanced MRI, demonstrating higher sensitivity, specificity, and accuracy in detecting lymph node metastases compared to traditional imaging methods (171, 172). For urinary tract cancers, such as bladder cancer, novel nanomaterials like Fe3O4@PDA-VCR-FA SPs and DC-PNM-PTX have shown promising results in photothermal conversion, biocompatibility, and enhanced drug delivery (97, 173, 174). Additionally, nanoparticles like PNPs have been explored for their ability to manifest cancerous cells through heat or fluorescence under infrared light, with longer half-lives compared to conventional chemotherapeutic agents (98). Despite these advancements, further research is necessary to address challenges associated with nanoparticle-based therapies, including biodistribution, toxicity, and large-scale manufacturing, to facilitate their clinical translation for the treatment of less common urological cancers.
Biosafety and limitations of nanomedicine application in the clinic
Nanomedicines have evolved significantly over the years and hold promise in improving early detection, accurate diagnosis, increasing treatment efficacy, and reducing side effects for urological cancers. NPs play a crucial role in the effective delivery of various therapies, including chemotherapy, gene therapy, and immunotherapy drugs, as well as in cancer diagnosis, imaging, and biomarker identification. Some notable nanoparticles that have entered human trials for urological cancers include Superparamagnetic Iron Oxide Nanoparticles (SPIONs) like Ferumoxytol, which is FDA-approved and is used as an intravenous contrast agent for MRI. Clinical studies have evaluated its utility in delineating lymph node metastasis in PCa patients, demonstrating favorable tumor detection with low toxicity (175). Albumin-bound paclitaxel (Abraxane) (176) is another nanoparticle formulation that has garnered attention, with several clinical studies indicating its ability to induce tumor response in metastatic castration-resistant prostate cancer. Notably, Abraxane achieves higher intratumoral paclitaxel concentrations and exhibits a more favorable toxicity profile compared to solvent-based paclitaxel formulations.
A comprehensive inventory profiles over 60 nanoparticle platforms explored across major treatment strategies for prostate, bladder, and renal cancers. These platforms encompass a diverse range of nanostructures, including polymeric, liposomal, gold, and magnetic formulations, designed to encapsulate and deliver chemotherapies, ablation agents, antigens, and genes. These nanoparticles enable targeted drug delivery, photothermal tumor ablation, immunostimulation, and radiosensitization, among other therapeutic modalities. The inventory highlights the favorable safety profiles of most platforms and captures the versatility of nanoparticles in addressing therapeutic gaps through tailored multifunctionality (177, 178).
Despite the promising potential of nanomedicine, the clinical translation of these innovative therapies faces several challenges. Potential toxicity concerns, limited biodistribution, and scalability issues remain significant hurdles that need to be addressed (179, 180). Rigorous preclinical and clinical testing is essential to ensure the safety and efficacy of nanomedicines, and comprehensive regulatory frameworks and guidelines are necessary for their development and approval (181). Furthermore, ongoing research is required to address the limitations and optimize the design, targeting, and delivery of nanomedicines for improved therapeutic outcomes and patient benefit (182, 183).
While significant progress has been made in the field of nanomedicine for urological cancers, further clinical translation and adoption of these innovative therapies are needed to harness their full potential and provide more effective, targeted, and personalized treatment options for patients. Collaboration between researchers, clinicians, and regulatory bodies is crucial to facilitate the successful integration of nanomedicine into clinical practice and improve patient outcomes.
Conclusion
This comprehensive review elucidates nanotechnology’s recent strides and future prospects in confronting major clinical hurdles across common urological malignancies, such as prostate, bladder, and renal cell carcinoma. NPs have emerged as versatile platforms, offering enhanced tumor penetration, controlled drug release, targeted ligand-directed delivery to cancerous cells, and heat-triggered activation. These innovative nanoplatforms, spanning polymeric, liposomal, and metallic constructs, can revolutionize existing and novel therapeutic modalities, ranging from chemotherapy to gene therapy, thereby addressing the diverse therapeutic gaps in urological cancers.
While the inventory of nanoparticle platforms showcases promising potential and favorable safety profiles, the clinical translation of these cutting-edge technologies remains limited, with a majority of research confined to preclinical phases. To bridge this gap and facilitate widespread clinical adoption, rigorous clinical trials are imperative to establish long-term biosafety conclusively. Moreover, comparative effectiveness studies, head-to-head clinical evaluations, and cost-benefit analyses are currently lacking, underscoring the need for a comprehensive review of nanomedicine’s real-world impact.
Addressing these research imperatives through multidisciplinary collaborations among researchers, clinicians, and regulatory bodies can expedite the bench-to-bedside transition and unlock the full potential of nanomedicine in uro-oncology. The future landscape of personalized nanomedicine envisions patients with urological cancers benefiting from integrated diagnostic-therapeutic platforms, offering real-time monitoring, minimized side effects, prevention of recurrence and drug resistance, and preserving the quality of life. The advent of smart multifunctional nanostructures heralds immense promise in transforming patient outcomes across the urological cancer spectrum, ushering in a new era of targeted, effective, and personalized cancer care.
Full Names of nanoparticles in Tables 1–3:
CUR NP, Curcumin nanoparticle; AgNP-PLE, Silver nanoparticle with papaya leaf; RSV-SLN, Resveratrol with solid lipid nanoparticle; MGF-AuNP, Mangiferin functionalized gold nanoparticulate agent; PHB-PEI NP, Polyethyleneimine-functionalized polyhydroxybutyrate nanoparticle; PTX/siRNA NP-Apt, siRNAs and Paclitaxel- Aptamer-Functionalized Shell–Core nanoparticle; LNP, Lipid nanoparticle; ICGNP, Polylactide nanoparticle encapsulating indocyanine green; PEG-b-PNBMA, Polyethylene glycol)-block-poly (4,5-dimethoxy-2-nitrobenzylmethacrylate); mRNA vaccine NP, Adjuvant-pulsed mRNA vaccine nanoparticle; RALA/pDNA nanoparticle, DNA vaccination via incorporating cationic RALA nanoparticle; DTXL/GRP78 siRNA, Docetaxel/Codelivery GRP78 siRNA; GNP+ DTX, Docetaxel with Gold nanoparticle; CuSNP, Copper sulfide nanoparticle; Tf-CRC-SLN, Transferrin curcumin bioconjugated solid lipid nanoparticle; Gen@AuNP, Genistein–gold nanoparticle conjugates; @PCEC NP, PCL-based biodegradable nanoparticle; NLPs-RGD-Cur-ATO, Liposomes (arsenic trioxide/curcumin) modified with RGD peptide; Apt-PEG-siRNA@ZIF-8 nanoplatfrom, Aptamer- poly ethylene glycol- FOR Small interfering RNAs zeolitic imidazolate framework-8; Cy-KUE-OA- PSMA, Self-quenched near-infrared fluorescence probe, Cy-KUE-OA effective on Prostate-specific membrane antigen; AuNP, gold nanoparticle; PSMA-AuNP, Prostate-specific membrane antigen- gold nanoparticle; Pd-Au Nano seeds, Palladium- gold Nanoseed; PLGA NP, Poly-lactide-co-glycolic acid (PLGA) nanoparticle; Poly TTG-SS@DTX NP, Docetaxel -loaded poly-Tetraethylene glycol nanoparticle; YBS-BMS NP-RKC, Nano-photosensitizer with pH-response integrating immunogenic pyroptosis induction and immune checkpoint blockade; Au-TNF NP, Gold nanoparticle conjugated tumor necrosis factor-alpha; PNP, Paclitaxel nanoparticle; HA/CHI NP-aggregated HET, Hyaluronan/chitosan nanoparticle-aggregated heteronemin; Cat-Alg NP, Catechol-functionalized alginate (Cat-Alg) nanoparticle; Au@TNA NP, Gold -Tannic acid nanoparticle; PLZ4 NP, PLZ4 (amino acid sequence: cQDGRMGFc) nanoparticle; AB680@EMVs-aPDL1, Nanocomplexes - CD73 inhibitor (AB680)- Macrophage-derived exosome-mimetic nanovesicles (EMVs)- programmed cell death ligand 1 (aPDL1); GNP-LLO91–99, Gold nanoparticle loaded with the bacterial peptide 91–99 of the listeriolysin O toxin (GNP-LLO91–99) nanovaccines; Modified MSNP, Modified mesoporous silica nanoparticle; Nano papain, Papain nanoparticle; SYMPHONY, Synergistic Immuno Photothermal Nanotherapy; SERS NP, Surface-Enhanced Raman Scattering nanoparticle; CS/PNIPAAm@SWCNTs, (nanocomplexes) Near-Infrared Guided Thermal-Responsive Nanomedicine against Orthotopic Superficial; HG-MNS-DOX, Hydrogels- magnetic nanostructure- doxorubicin; KDM6A-mRNA, Lysine-specific demethylase 6A- mRNA; Au–Ag@PDA NP, Polydopamine-coated branched Au–Ag nanoparticle;FePPy-NH2 NP, Synthesized Fe (III)-doped polyaminopyrrole nanoparticle; UCNP-AuNR, Multifunctional nanoclusters of upconversion - and gold nanorod; Fe3O4-MNP, Fe3O4 magnetic nanoparticle; BCG-CWS, Bacillus Calmette-Guerin - cell wall skeleton; FCS Cu2-xSe NP, Fluorinated chitosan Cu2-xSe nanoparticle; TiO2, Titanium dioxide; CS/PEG NP& CS NP, Chitosan and chitosan/polyethylene glycol nanoparticle; PTX/CS NSs, Paclitaxel/chitosan nanosupensions; Sor-Mag-SLNs, Sorafenib loaded magnetic solid lipid nanoparticle; HSA-AuNR-TKI, Tyrosine kinase inhibitors and gold nanorods in human serum albumin protein; TLR7/8 agonists encapsulated in PLGA NP, TLR 7/8 bi-specific agonists encapsulated in poly(lactide-co-glycolide); CA IX-C4.16 NP, Carbonic anhydrase IX- new class of apoptosis inducer CFM 4.16 nanoparticle; H1-pAIM2/pCAIX, Folate-grafted PEI600-CyD (H1) NP-mediated DNA vaccine melanoma 2 (AIM2)/specific antigen of carbonic anhydrase IX (CAIX); Ser/ICG@Lip, Sertraline Hydrochloride and indocyanine green @Lip; GSH-AuNP, Glutathione (GSH)-modified small-sized gold nanoparticle; Cur@HMON@gel, Curcumin (Cur)-loaded hollow mesoporous organosilica NP @elastic gel matrix; TKI/TSLs, Tyrosine kinase inhibitor–loaded, thermosensitive liposomes; LPs by siVEGFR2, Long-circulating liposomes by the vascular endothelial cell growth factor receptor 2; H1/pHGFK1, First kringle domain of hepatocyte growth factor nanoparticles; CONP, Cuprous oxide nanoparticle.
Author contributions
MF: Writing – original draft. MD: Writing – original draft. SP: Writing – original draft, Validation. SR: Writing – original draft, Supervision. DZ: Writing – original draft, Investigation. BH: Writing – original draft. TO: Writing – original draft. HM: Supervision, Writing – review & editing. RA: Validation, Writing – review & editing.
Funding
The author(s) declare that no financial support was received for the research, authorship, and/or publication of this article.
Conflict of interest
The authors declare that the research was conducted in the absence of any commercial or financial relationships that could be construed as a potential conflict of interest.
Publisher’s note
All claims expressed in this article are solely those of the authors and do not necessarily represent those of their affiliated organizations, or those of the publisher, the editors and the reviewers. Any product that may be evaluated in this article, or claim that may be made by its manufacturer, is not guaranteed or endorsed by the publisher.
References
1. Barber N, Ali A. Urologic Cancers. Exon Publications (2022). doi: 10.36255/exon-publications-urologic-cancers
2. Mourey L. Tumors: urologic cancer. In: Encyclopedia of Gerontology and Population Aging. Springer (2022). p. 5291–301.
3. Zapała Ł, Ślusarczyk A. Recent advances in the treatment of upper urinary tract and bladder cancers. Front Urol. (2013) 3:1300741.
4. Merseburger AS, Burger M. Urologic oncology. Springer International Publishing (2019). doi: 10.1007/978-3-319-42623-5
5. Swami U, McFarland TR, Nussenzveig R, Agarwal N. Advanced prostate cancer: treatment advances and future directions. Trends Cancer. (2020) 6:702–15. doi: 10.1016/j.trecan.2020.04.010
6. Ploussard G, Albrand G, Rozet F, Lang H, Paillaud E, Mongiat-Artus P. Challenging treatment decision-making in older urologic cancer patients. World J Urol. (2014) 32:299–308. doi: 10.1007/s00345-013-1158-4
7. Ramsey SD, Bansal A, Fedorenko CR, Blough DK, Overstreet KA, Shankaran V, et al. Financial insolvency as a risk factor for early mortality among patients with cancer. J Clin Oncol. (2016) 34:980. doi: 10.1200/JCO.2015.64.6620
8. Hu X, Zhang YS, Liu YC, Wang N, Zeng XT, Zhang LL. Emerging photodynamic/sonodynamic therapies for urological cancers: progress and challenges. J Nanobiotechnology. (2022) 20:1–26. doi: 10.1186/s12951-022-01637-w
9. Li J, Wu T, Li S, Chen X, Deng Z, Huang Y. Nanoparticles for cancer therapy: a review of influencing factors and evaluation methods for biosafety. Clin Trans Oncol. (2023), 25: 1–13. doi: 10.1007/s12094-023-03117-5
10. Gavas S, Quazi S, Karpiński TM. Nanoparticles for cancer therapy: current progress and challenges. Nanoscale Res Lett. (2021) 16:173. doi: 10.1186/s11671-021-03628-6
11. Culp MB, Soerjomataram I, Efstathiou JA, Bray F, Jemal A. Recent global patterns in prostate cancer incidence and mortality rates. Eur Urol. (2020) 77:38–52. doi: 10.1016/j.eururo.2019.08.005
12. Bergengren O, Pekala KR, Matsoukas K, Fainberg J, Mungovan SF, Bratt O, et al. 2022 update on prostate cancer epidemiology and risk factors—A systematic review. Eur Urol. (2023). doi: 10.1016/j.eururo.2023.04.021
13. Perez, Zelefsky M. Low-risk prostate cancer. In: Halperin EC, Perez CA, Brady LW, editors. Perez & Brady’s Principles and Practice of Radiation Oncology, 7th edition. LWW (2018).
14. Costello AJ. Considering the role of radical prostatectomy in 21st century prostate cancer care. Nat Rev Urol. (2020) 17:177–88. doi: 10.1038/s41585-020-0287-y
15. Yossepowitch O, Eggener SE, Bianco FJ Jr., Carver BS, Serio A, Scardino PT, et al. Radical prostatectomy for clinically localized, high risk prostate cancer: critical analysis of risk assessment methods. J Urol. (2007) 178:493–9. doi: 10.1016/j.juro.2007.03.105
16. Moretti TBC, Magna LA, Reis LO. Surgical results and complications for open, laparoscopic, and robot-assisted radical prostatectomy: a reverse systematic review. Eur Urol Open Sci. (2022) 44:150–61. doi: 10.1016/j.euros.2022.08.015
17. Touijer KA, Sjoberg DD, Benfante N, Laudone VP, Ehdaie B, Eastham JA, et al. Limited versus extended pelvic lymph node dissection for prostate cancer: a randomized clinical trial. Eur Urol Oncol. (2021) 4:532–9. doi: 10.1016/j.euo.2021.03.006
18. Zaorsky NG, Davis BJ, Nguyen PL, Showalter TN, Hoskin PJ, Yoshioka Y, et al. The evolution of brachytherapy for prostate cancer. Nat Rev Urol. (2017) 14:415–39. doi: 10.1038/nrurol.2017.76
19. Reichert ZR, Udager AM, Palapattu GS, Spratt DE BLK. Cancer of the prostate. In: DeVita VT, Lawrence TS RSA, editors. DeVita, Hellman and Rosenberg’s Cancer Principles and Practice of Oncology, 12th ed. LWW (2023). p. 784–833.
20. Seitzer KE, Seifert R, Kessel K, Roll W, Schlack K, Boegemann M, et al. Lutetium-177 labelled PSMA targeted therapy in advanced prostate cancer: current status and future perspectives. Cancers (Basel). (2021) 13:3715. doi: 10.3390/cancers13153715
21. Ferreira MR, Sands CJ, Li JV, Andreyev JN, Chekmeneva E, Gulliford S, et al. Impact of pelvic radiation therapy for prostate cancer on global metabolic profiles and microbiota-driven gastrointestinal late side effects: A longitudinal observational study. Int J Radiat OncologyBiologyPhysics. (2021) 111:1204–13. doi: 10.1016/j.ijrobp.2021.07.1713
22. Nolsøe AB, Jensen CFS, Østergren PB, Fode M. Neglected side effects to curative prostate cancer treatments. Int J Impot Res. (2021) 33:428–38. doi: 10.1038/s41443-020-00386-4
23. (NCCN®) NCCN. CCN guidelines treatment by cancer type. In: Prostate Cancer (2023). Available at: https://www.nccn.org/professionals/physician_gls/pdf/prostate.pdf.
24. Pranav, Laskar P, Jaggi M, Chauhan SC, Yallapu MM. Biomolecule-functionalized nanoformulations for prostate cancer theranostics. J Adv Res. (2023) 51:197–217. doi: 10.1016/j.jare.2022.11.001
25. Rehman Lu, Nisar MH, Fatima W, Sarfraz A, Azeem N, Sarfraz Z, et al. Immunotherapy for prostate cancer: A current systematic review and patient centric perspectives. J Clin Med. (2023) 12:1446. doi: 10.3390/jcm12041446
26. Fay EK, Graff JN. Immunotherapy in prostate cancer. Cancers (Basel). (2020) 12:1752. doi: 10.3390/cancers12071752
27. Brown TJ, Mamtani R, Bange EM. Immunotherapy adverse effects. JAMA Oncol. (2021) 7:1908. doi: 10.1001/jamaoncol.2021.5009
28. Abusalem M, Martiniova L, Soebianto S, DePalatis L, Ravizzini G. Current status of radiolabeled monoclonal antibodies targeting PSMA for imaging and therapy. Cancers (Basel). (2023) 15:4537. doi: 10.3390/cancers15184537
29. Edmunds K, Tuffaha H, Galvão DA, Scuffham P, Newton RU. Incidence of the adverse effects of androgen deprivation therapy for prostate cancer: a systematic literature review. Supportive Care Cancer. (2020) 28:2079–93. doi: 10.1007/s00520-019-05255-5
30. Chandrasekar T, Yang JC, Gao AC, Evans CP. Mechanisms of resistance in castration-resistant prostate cancer (CRPC). Transl Androl Urol. (2015) 4:365–80.
31. He MH, Chen L, Zheng T, Tu Y, He Q, Fu HL, et al. Potential applications of nanotechnology in urological cancer. Front Pharmacol. (2018) 9:745. doi: 10.3389/fphar.2018.00745
32. Devi RV, Doble M, Verma RS. Nanomaterials for early detection of cancer biomarker with special emphasis on gold nanoparticles in immunoassays/sensors. Biosens Bioelectron. (2015) 68:688–98. doi: 10.1016/j.bios.2015.01.066
33. Mangadlao JD, Wang X, McCleese C, Escamilla M, Ramamurthy G, Wang Z, et al. Prostate-specific membrane antigen targeted gold nanoparticles for theranostics of prostate cancer. ACS Nano. (2018) 12:3714–25. doi: 10.1021/acsnano.8b00940
34. Hara D, Tao W, Totiger TM, Pourmand A, Dogan N, Ford JC, et al. Prostate cancer targeted X-ray fluorescence imaging via gold nanoparticles functionalized with prostate-specific membrane antigen (PSMA). Int J Radiat Oncol Biol Phys. (2021) 111:220–32. doi: 10.1016/j.ijrobp.2021.04.032
35. Jue JS, Coons S, Hautvast G, Thompson SF, Geraats J, Richstone L, et al. Novel automated three-dimensional surgical planning tool and magnetic resonance imaging/ultrasound fusion technology to perform nanoparticle ablation and cryoablation of the prostate for focal therapy. J Endourol. (2022) 36:369–72. doi: 10.1089/end.2021.0266
36. Omiyale OC, Musa M, Otuyalo AI, Gbayisomore TJ, Onikeku DZ, George SD, et al. A review on selenium and gold nanoparticles combined photodynamic and photothermal prostate cancer tumors ablation. Discover Nano. (2023). doi: 10.1186/s11671-023-03936-z
37. Wu LL, Zhao Q, Wang Q, Zhang Q, Yang F, Zheng B, et al. Membrane dual-targeting probes: A promising strategy for fluorescence-guided prostate cancer surgery and lymph node metastases detection. Acta Pharm Sin B. (2023) 13:1204–15. doi: 10.1016/j.apsb.2022.07.018
38. Teh J, Tripathi M, Reichel D, Sagong B, Montoya R, Zhang Y, et al. Intraoperative assessment and postsurgical treatment of prostate cancer tumors using tumor-targeted nanoprobes. Nanotheranostics. (2021) 5:57–72. doi: 10.7150/ntno.50095
39. King RB, McMahon SJ, Hyland WB, Jain S, Butterworth KT, Prise KM, et al. An overview of current practice in external beam radiation oncology with consideration to potential benefits and challenges for nanotechnology. Cancer Nanotechnol. (2017) 8:1–12. doi: 10.1186/s12645-017-0027-z
40. Yorke ED, Chui CS. "Treatment plan optimization: an elusive goal," in advances in medical physics. Medical Physics Publishing (2008) 12:211–35.
41. Tas B. Advanced radiation treatment planning of prostate cancer. In: Prostate Cancer. IntechOpen (2018). doi: 10.5772/intechopen.70249
42. DuRoss AN, Phan J, Lazar AJ, Walker JM, Guimaraes AR, Baas C, et al. Radiotherapy reimagined: Integrating nanomedicines into radiotherapy clinical trials. Wiley Interdiscip Rev Nanomed Nanobiotechnol. (2023) 15:e1867. doi: 10.1002/wnan.1867
43. Luo D, Wang X, Zeng S, Ramamurthy G, Burda C, Basilion JP. Prostate-specific membrane antigen targeted gold nanoparticles for prostate cancer radiotherapy: does size matter for targeted particles? Chem Sci. (2019) 10:8119–28. doi: 10.1039/C9SC02290B
44. Moeendarbari S, Tekade R, Mulgaonkar A, Christensen P, Ramezani S, Hassan G, et al. Theranostic nanoseeds for efficacious internal radiation therapy of unresectable solid tumors. Sci Rep. (2016) 6:20614. doi: 10.1038/srep20614
45. Han X, Huang J, Lin H, Wang Z, Li P, Chen Y. 2D ultrathin MXene-based drug-delivery nanoplatform for synergistic photothermal ablation and chemotherapy of cancer. Adv Healthc Mater. (2018) 7:e1701394. doi: 10.1002/adhm.201701394
46. Zhao J, Zhang C, Wang W, Li C, Mu X, Hu K. Current progress of nanomedicine for prostate cancer diagnosis and treatment. Biomedicine Pharmacotherapy. (2022) 155:113714. doi: 10.1016/j.biopha.2022.113714
47. Zhang X, He Z, Xiang L, Li L, Zhang H, Lin F, et al. Codelivery of GRP78 siRNA and docetaxel via RGD-PEG-DSPE/DOPA/CaP nanoparticles for the treatment of castration-resistant prostate cancer. Drug Des Devel Ther. (2019), 13:1357–72. doi: 10.2147/DDDT
48. Cherian AM, Nair SV, Lakshmanan VK. The role of nanotechnology in prostate cancer theranostic applications. J Nanosci Nanotechnol. (2014) 14:841–52. doi: 10.1166/jnn.2014.9052
49. Tanaudommongkon I, Tanaudommongkon A, Prathipati P, Nguyen JT, Keller ET, Dong X. Curcumin nanoparticles and their cytotoxicity in docetaxel-resistant castration-resistant prostate cancer cells. Biomedicines. (2020) 8:253. doi: 10.3390/biomedicines8080253
50. Singh SP, Mishra A, Shyanti RK, Singh RP, Acharya A. Silver nanoparticles synthesized using Carica papaya leaf extract (AgNPs-PLE) causes cell cycle arrest and apoptosis in human prostate (DU145) cancer cells. Biol Trace Elem Res. (2021) 199:1316–31. doi: 10.1007/s12011-020-02255-z
51. Sharma AN, Upadhyay PK, Dewangan HK. Development, evaluation, pharmacokinetic and biodistribution estimation of resveratrol-loaded solid lipid nanoparticles for prostate cancer targeting. J Microencapsul. (2022) 39:563–74. doi: 10.1080/02652048.2022.2135785
52. Khoobchandani M, Khan A, Katti KK, Thipe VC, Al-Yasiri AY, MohanDoss DKD, et al. Green nanotechnology of MGF-AuNPs for immunomodulatory intervention in prostate cancer therapy. Sci Rep. (2021) 11:16797. doi: 10.1038/s41598-021-96224-8
53. Conte R, Valentino A, Di Cristo F, Peluso G, Cerruti P, Di Salle A, et al. Cationic polymer nanoparticles-mediated delivery of miR-124 impairs tumorigenicity of prostate cancer cells. Int J Mol Sci. (2020) 21:869. doi: 10.3390/ijms21030869
54. Guo Q, Dong Y, Zhang Y, Fu H, Chen C, Wang L, et al. Sequential release of pooled siRNAs and paclitaxel by aptamer-functionalized shell–core nanoparticles to overcome paclitaxel resistance of prostate cancer. ACS Appl Mater Interfaces. (2021) 13:13990–4003. doi: 10.1021/acsami.1c00852
55. Wang J, Zhang Y, Liu C, Zha W, Dong S, Xing H, et al. Multifunctional lipid nanoparticles for protein kinase N3 shRNA delivery and prostate cancer therapy. Mol Pharm. (2022) 19:4588–600. doi: 10.1021/acs.molpharmaceut.2c00244
56. Akkurt MG, Gülsoy M. Polylactide nanoparticles encapsulating indocyanine green for photothermal therapy of prostate cancer cells. Photodiagnosis Photodyn Ther. (2022) 37:102693. doi: 10.1016/j.pdpdt.2021.102693
57. Sun B, Liu J, Kim HJ, Rahmat JNB, Neoh KG, Zhang Y. Light-responsive smart nanocarriers for wirelessly controlled photodynamic therapy for prostate cancers. Acta Biomater. (2023) 171. doi: 10.1016/j.actbio.2023.09.031
58. Islam MA, Rice J, Reesor E, Zope H, Tao W, Lim M, et al. Adjuvant-pulsed mRNA vaccine nanoparticle for immunoprophylactic and therapeutic tumor suppression in mice. Biomaterials. (2021) 266:120431. doi: 10.1016/j.biomaterials.2020.120431
59. Cole G, Ali AA, McErlean E, Mulholland EJ, Short A, McCrudden CM, et al. DNA vaccination via RALA nanoparticles in a microneedle delivery system induces a potent immune response against the endogenous prostate cancer stem cell antigen. Acta Biomater. (2019) 96:480–90. doi: 10.1016/j.actbio.2019.07.003
60. Jackson N, Hill I, Alhussan A, Bromma K, Morgan J, Abousaida B, et al. Dual enhancement in the radiosensitivity of prostate cancer through nanoparticles and chemotherapeutics. Cancer Nanotechnol. (2023) 14:75. doi: 10.1186/s12645-023-00228-0
61. Poudel K, Thapa RK, Gautam M, Ou W, Soe ZC, Gupta B, et al. Multifaceted NIR-responsive polymer-peptide-enveloped drug-loaded copper sulfide nanoplatform for chemo-phototherapy against highly tumorigenic prostate cancer. Nanomedicine. (2019) 21:102042. doi: 10.1016/j.nano.2019.102042
62. Akanda M, Getti G, Nandi U, Mithu MS, Douroumis D. Bioconjugated solid lipid nanoparticles (SLNs) for targeted prostate cancer therapy. Int J Pharm. (2021) 599:120416. doi: 10.1016/j.ijpharm.2021.120416
63. Vodnik VV, Mojić M, Stamenović U, Otoničar M, Ajdžanović V, Maksimović-Ivanić D, et al. Development of genistein-loaded gold nanoparticles and their antitumor potential against prostate cancer cell lines. Materials Sci Engineering: C. (2021) 124:112078. doi: 10.1016/j.msec.2021.112078
64. Yousefnezhad M, Davaran S, Babazadeh M, Akbarzadeh A, Pazoki-Toroudi H. PCL-based nanoparticles for doxorubicin-ezetimibe co-delivery: A combination therapy for prostate cancer using a drug repurposing strategy. BioImpacts. (2023) 13:241–53. doi: 10.34172/bi.2023.24252
65. Khosravani F, Amiri F, Mahmoudi R, Morshedi D, Kobarfard F, Alipour M, et al. RGD-decorated nanoliposomes for combined delivery of arsenic trioxide and curcumin to prostate cancer cells.. Naunyn Schmiedebergs Arch Pharmacol. (2023), 397: 1–11.
66. Saeinasab M, Iranpour S, Hosseini-Giv N, Saljooghi AS, Matin MM. Tumor-targeted delivery of SNHG15 siRNA using a ZIF-8 nanoplatform: Towards a more effective prostate cancer therapy. Int J Biol Macromol. (2024). doi: 10.1016/j.ijbiomac.2024.129233
67. Tabatabaie F, Franich R, Feltis B, Geso M. Oxidative damage to mitochondria enhanced by ionising radiation and gold nanoparticles in cancer cells. Int J Mol Sci. (2022) 23:6887. doi: 10.3390/ijms23136887
68. Schmidt RM, Hara D, Vega JD, Abuhaija MB, Tao W, Dogan N, et al. Quantifying radiosensitization of PSMA-targeted gold nanoparticles on prostate cancer cells at megavoltage radiation energies by Monte Carlo simulation and local effect model. Pharmaceutics. (2022) 14. doi: 10.3390/pharmaceutics14102205
69. Li L, Li R, Li J, Yao J, Zhang Q, Ji Q, et al. Tumor-microenvironment responsive nano-carrier system for therapy of prostate cancer. J Mater Sci Mater Med. (2023) 34. doi: 10.1007/s10856-023-06749-9
70. Wang H, He Z, Gao Y, Feng D, Wei X, Huang Y, et al. Dual-pronged attack: pH-driven membrane-anchored NIR dual-type nano-photosensitizer excites immunogenic pyroptosis and sequester immune checkpoint for enhanced prostate cancer photo-immunotherapy. Advanced Sci. (2023) 10. doi: 10.1002/advs.202302422
71. Shenoi MM, Iltis I, Choi J, Koonce NA, Metzger GJ, Griffin RJ, et al. Nanoparticle delivered vascular disrupting agents (VDAs): use of TNF-alpha conjugated gold nanoparticles for multimodal cancer therapy. Mol Pharm. (2013) 10:1683–94. doi: 10.1021/mp300505w
72. Ashrafizadeh M, Aghamiri S, Tan SC, Zarrabi A, Sharifi E, Rabiee N, et al. Nanotechnological approaches in prostate cancer therapy: integration of engineering and biology. Nano Today. (2022) 45:101532. doi: 10.1016/j.nantod.2022.101532
73. He L, Liu J, Li S, Feng X, Wang C, Zhuang X, et al. Polymer nanoplatforms at work in prostate cancer therapy. Adv Ther (Weinh). (2019) 2:1800122. doi: 10.1002/adtp.201800122
74. Sung H, Ferlay J, Siegel RL, Laversanne M, Soerjomataram I, Jemal A, et al. Global cancer statistics 2020: GLOBOCAN estimates of incidence and mortality worldwide for 36 cancers in 185 countries. CA Cancer J Clin. (2021) 71. doi: 10.3322/caac.21660
75. Lenis AT, Lec PM, Chamie K, Mshs MD. Bladder cancer: A review. JAMA. (2020) 324:1980–91. doi: 10.1001/jama.2020.17598
76. Holzbeierlein JM, Brooke RB, David IC, Sam SH, Rebecca J, Andrew CK, et al. Diagnosis and treatment of non-muscle invasive bladder cancer: AUA/SUO guideline: 2024 amendment. J Urol. (2024). doi: 10.1097/JU.0000000000003846
77. Halaseh SA, Halaseh S, Alali Y, Ashour ME, Alharayzah MJ. A review of the etiology and epidemiology of bladder cancer: all you need to know. Cureus. (2022). doi: 10.7759/cureus.27330
78. Shen Z, Shen T, Wientjes MG, O’Donnell MA, Au JL. Intravesical treatments of bladder cancer: review. Pharm Res. (2008) 25:1500–10. doi: 10.1007/s11095-008-9566-7
79. Chang SS, Boorjian SA, Chou R. Diagnosis and treatment of non-muscle invasive bladder cancer: AUA/SUO guideline. J Urol (2016) 196: 1021–9.
80. (NCCN®) NCCN. CCN guidelines treatment by cancer type. In: Bladder Cancer (2023). Available at: https://www.nccn.org.
81. Teoh JYC, Huang J, Ko WYK, Lok V, Choi P, Ng CF, et al. Global trends of bladder cancer incidence and mortality, and their associations with tobacco use and gross domestic product per capita. Eur Urol. (2020) 78:893–906. doi: 10.1016/j.eururo.2020.09.006
82. Sarfraz M, Qamar S, Rehman MU, Tahir MA, Ijaz M, Ahsan A, et al. Nano-formulation based intravesical drug delivery systems: An overview of versatile approaches to improve urinary bladder diseases. Pharmaceutics. (2022) 14:1909. doi: 10.3390/pharmaceutics14091909
83. Zhang C, Zhao J, Wang W, Geng H, Wang Y, Gao B. Current advances in the application of nanomedicine in bladder cancer. BioMed Pharmacother. (2023) 157:114062. doi: 10.1016/j.biopha.2022.114062
84. Buss JH, Begnini KR, Bender CB, Pohlmann AR, Guterres SS, Collares T, et al. Nano-BCG: A promising delivery system for treatment of human bladder cancer. Front Pharmacol. (2017) 8:977. doi: 10.3389/fphar.2017.00977
85. Feldman AS, Miyamoto DT, Dahl DM, Efstathiou JA LRJ. Cancer of the bladder, ureter, and renal pelvis. In: DeVita VT, Lawrence TS RSA, editors. DeVita, Hellman and Rosenberg’s Cancer Principles and Practice of Oncology, 12th ed. LWW (2023).
86. Witjes JA, Bruins HM, Cathomas R, Compérat EM, Cowan NC, Gakis G, et al. European Association of Urology guidelines on muscle-invasive and metastatic bladder cancer: summary of the 2020 guidelines. Eur Urol. (2021) 79:82–104. doi: 10.1016/j.eururo.2020.03.055
87. Parekh DJ, Reis IM, Castle EP, Gonzalgo ML, Woods ME, Svatek RS, et al. Robot-assisted radical cystectomy versus open radical cystectomy in patients with bladder cancer (RAZOR): an open-label, randomised, phase 3, non-inferiority trial. Lancet. (2018) 391:2525–36. doi: 10.1016/S0140-6736(18)30996-6
88. Stein JP, Lieskovsky G, Cote R, Groshen S, Feng AC, Boyd S, et al. Radical cystectomy in the treatment of invasive bladder cancer: long-term results in 1,054 patients. J Clin Oncol. (2001) 19:666–75. doi: 10.1200/JCO.2001.19.3.666
89. Royce TJ, Feldman AS, Mossanen M, Yang JC, Shipley WU, Pandharipande PV, et al. Comparative effectiveness of bladder-preserving tri-modality therapy versus radical cystectomy for muscle-invasive bladder cancer. Clin Genitourin Cancer. (2019) 17:23–31.e3. doi: 10.1016/j.clgc.2018.09.023
90. Dearnaley D, Syndikus I, Mossop H, Khoo V, Birtle A, Bloomfield D, et al. Conventional versus hypofractionated high-dose intensity-modulated radiotherapy for prostate cancer: 5-year outcomes of the randomised, non-inferiority, phase 3 CHHiP trial. Lancet Oncol. (2016) 17:1047–60. doi: 10.1016/S1470-2045(16)30102-4
91. Leal J, Luengo-Fernandez R, Sullivan R, Witjes JA. Economic burden of bladder cancer across the European union. Eur Urol. (2016) 69. doi: 10.1016/j.eururo.2015.10.024
92. Dobruch J, Oszczudłowski M. Bladder cancer: Current challenges and future directions. Medicina (Lithuania). (2021) 57. doi: 10.3390/medicina57080749
93. GuhaSarkar S, Banerjee R. Intravesical drug delivery: Challenges, current status, opportunities and novel strategies. J Controlled Release. (2010) 148. doi: 10.1016/j.jconrel.2010.08.031
94. Apfelthaler C, Anzengruber M, Gabor F, Wirth M. Poly - (l) - glutamic acid drug delivery system for the intravesical therapy of bladder cancer using WGA as targeting moiety. Eur J Pharm Biopharm. (2017) 115:131–9. doi: 10.1016/j.ejpb.2017.02.016
95. Tong T, Guan Y, Gao Y, Xing C, Zhang S, Jiang D, et al. Smart nanocarriers as therapeutic platforms for bladder cancer. Nano Res. (2021), 15: 1–20.
96. Kolawole OM, Lau WM, Mostafid H, Khutoryanskiy VV. Advances in intravesical drug delivery systems to treat bladder cancer. Int J Pharmaceutics. (2017) 532. doi: 10.1016/j.ijpharm.2017.08.120
97. Sweeney SK, Luo Y, O’Donnell MA, Assouline J. Nanotechnology and cancer: improving real-time monitoring and staging of bladder cancer with multimodal mesoporous silica nanoparticles. Cancer Nanotechnol. (2016) 7:1–18. doi: 10.1186/s12645-016-0015-8
98. Lin TY, Li Y, Liu Q, Chen JL, Zhang H, Lac D, et al. Novel theranostic nanoporphyrins for photodynamic diagnosis and trimodal therapy for bladder cancer. Biomaterials. (2016) 104:339–51. doi: 10.1016/j.biomaterials.2016.07.026
99. Lu Z, Yeh TK, Wang J, Chen L, Lyness G, Xin Y, et al. Paclitaxel gelatin nanoparticles for intravesical bladder cancer therapy. J Urol. (2011) 185:1478–83. doi: 10.1016/j.juro.2010.11.091
100. Huang HH, Kuo SM, Wu YJ, Su JH. Improvement and enhancement of antibladder carcinoma cell effects of heteronemin by the nanosized hyaluronan aggregation. Int J Nanomedicine. (2016), 11: 1237–51. doi: 10.2147/IJN
101. Sahatsapan N, Ngawhirunpat T, Rojanarata T, Opanasopit P, Patrojanasophon P. Catechol-functionalized alginate nanoparticles as Mucoadhesive carriers for Intravesical chemotherapy. AAPS PharmSciTech. (2020) 21:1–9. doi: 10.1208/s12249-020-01752-7
102. Hsu CW, Cheng NC, Liao MY, Cheng TY, Chiu YC. Development of folic acid-conjugated and methylene blue-adsorbed Au@ TNA nanoparticles for enhanced photodynamic therapy of bladder cancer cells. Nanomaterials. (2020) 10:1351. doi: 10.3390/nano10071351
103. Zhu Z, Ma AH, Zhang H, Lin TY, Xue X, Farrukh H, et al. Phototherapy with cancer-specific nanoporphyrin potentiates immunotherapy in bladder cancer. Clin Cancer Res. (2022) 28:4820–31. doi: 10.1158/1078-0432.CCR-22-1362
104. Zhou Q, Ding W, Qian Z, Zhu Q, Sun C, Yu Q, et al. Immunotherapy strategy targeting programmed cell death ligand 1 and CD73 with macrophage-derived mimetic nanovesicles to treat bladder cancer. Mol Pharm. (2021) 18:4015–28. doi: 10.1021/acs.molpharmaceut.1c00448
105. Terán-Navarro H, Zeoli A, Salines-Cuevas D, Marradi M, Montoya N, Gonzalez-Lopez E, et al. Gold glyconanoparticles combined with 91–99 peptide of the bacterial toxin, listeriolysin O, are efficient immunotherapies in experimental bladder tumors. Cancers (Basel). (2022) 14:2413.
106. Shahidi M, Abazari O, Dayati P, Bakhshi A, Zavarreza J, Modarresi MH, et al. Multicomponent siRNA/miRNA-loaded modified mesoporous silica nanoparticles targeted bladder cancer for a highly effective combination therapy. Front Bioeng Biotechnol. (2022) 10:949704. doi: 10.3389/fbioe.2022.949704
107. de Lima CSA, Rial-Hermida MI, de Freitas LF, Pereira-da-Mota AF, Vivero-Lopez M, Ferreira AH, et al. Mucoadhesive gellan gum-based and carboxymethyl cellulose-based hydrogels containing gemcitabine and papain for bladder cancer treatment. Int J Biol Macromol. (2023) 242:124957. doi: 10.1016/j.ijbiomac.2023.124957
108. Liu Y, Maccarini P, Palmer GM, Etienne W, Zhao Y, Lee CT, et al. Synergistic immuno photothermal nanotherapy (SYMPHONY) for the treatment of unresectable and metastatic cancers. Sci Rep. (2017) 7:8606. doi: 10.1038/s41598-017-09116-1
109. Davis RM, Kiss B, Trivedi DR, Metzner TJ, Liao JC, Gambhir SS. Surface-enhanced Raman scattering nanoparticles for multiplexed imaging of bladder cancer tissue permeability and molecular phenotype. ACS Nano. (2018) 12:9669–79. doi: 10.1021/acsnano.8b03217
110. Gao H, Bi Y, Wang X, Wang M, Zhou M, Lu H, et al. Near-infrared guided thermal-responsive nanomedicine against orthotopic superficial bladder cancer. ACS Biomater Sci Eng. (2017) 3:3628–34. doi: 10.1021/acsbiomaterials.7b00405
111. Smilowitz HM, Tarmu LJ, Sanders MM, Taylor JA III, Choudhary D, Xue C, et al. Biodistribution of gold nanoparticles in BBN-induced muscle-invasive bladdercancer in mice. Int J Nanomedicine. (2017), 12: 7937–46. doi: 10.2147/IJN
112. Jaiswal MK, Pradhan L, Vasavada S, De M, Sarma HD, Prakash A, et al. Magneto-thermally responsive hydrogels for bladder cancer treatment: Therapeutic efficacy and in vivo biodistribution. Colloids Surf B Biointerfaces. (2015) 136:625–33. doi: 10.1016/j.colsurfb.2015.09.058
113. Kong N, Zhang R, Wu G, Sui X, Wang J, Kim NY, et al. Intravesical delivery of KDM6A-mRNA via mucoadhesive nanoparticles inhibits the metastasis of bladder cancer. Proc Natl Acad Sci. (2022) 119:e2112696119. doi: 10.1073/pnas.2112696119
114. Zhao X, Qi T, Kong C, Hao M, Wang Y, Li J, et al. Photothermal exposure of polydopamine-coated branched Au–Ag nanoparticles induces cell cycle arrest, apoptosis, and autophagy in human bladder cancer cells. Int J Nanomedicine. (2018), 13: 6413–28. doi: 10.2147/IJN
115. Liu H, Zhang M, Jin H, Tao K, Tang C, Fan Y, et al. Fe (III)-doped Polyaminopyrrole nanoparticle for imaging-guided Photothermal therapy of bladder cancer. ACS Biomater Sci Eng. (2022) 8:502–11. doi: 10.1021/acsbiomaterials.1c01217
116. Cho SK, Su LJ, Mao C, Wolenski CD, Flaig TW, Park W. Multifunctional nanoclusters of NaYF4: Yb3+, Er3+ upconversion nanoparticle and gold nanorod for simultaneous imaging and targeted chemotherapy of bladder cancer. Materials Sci Engineering: C. (2019) 97:784–92. doi: 10.1016/j.msec.2018.12.113
117. Zhang D, Sun P, Li P, Xue A, Zhang X, Zhang H, et al. A magnetic chitosan hydrogel for sustained and prolonged delivery of Bacillus Calmette–Guérin in the treatment of bladder cancer. Biomaterials. (2013) 34:10258–66. doi: 10.1016/j.biomaterials.2013.09.027
118. Masuda H, Nakamura T, Noma Y, Harashima H. Application of BCG-CWS as a systemic adjuvant by using nanoparticulation technology. Mol Pharm. (2018) 15:5762–71. doi: 10.1021/acs.molpharmaceut.8b00919
119. Yoon HY, Yang HM, Kim CH, Goo YT, Hwang GY, Chang IH, et al. Enhanced intracellular delivery of BCG cell wall skeleton into bladder cancer cells using liposomes functionalized with folic acid and Pep-1 peptide. Pharmaceutics. (2019) 11:652. doi: 10.3390/pharmaceutics11120652
120. Zhang S, Li G, Deng D, Dai Y, Liu Z, Wu S. Fluorinated chitosan mediated synthesis of copper selenide nanoparticles with enhanced penetration for second near-infrared photothermal therapy of bladder cancer. Adv Ther (Weinh). (2021) 4:2100043. doi: 10.1002/adtp.202100043
121. Ni W, Li M, Cui J, Xing Z, Li Z, Wu X, et al. 808 nm light triggered black TiO2 nanoparticles for killing of bladder cancer cells. Materials Sci Engineering: C. (2017) 81:252–60. doi: 10.1016/j.msec.2017.08.020
122. Melo MN, Pereira FM, Rocha MA, Ribeiro JG, Junges A, Monteiro WF, et al. Chitosan and chitosan/PEG nanoparticles loaded with indole-3-carbinol: Characterization, computational study and potential effect on human bladder cancer cells. Materials Sci Engineering: C. (2021) 124:112089. doi: 10.1016/j.msec.2021.112089
123. Liu Y, Wang R, Hou J, Sun B, Zhu B, Qiao Z, et al. Paclitaxel/chitosan nanosupensions provide enhanced intravesical bladder cancer therapy with sustained and prolonged delivery of paclitaxel. ACS Appl Bio Mater. (2018) 1:1992–2001. doi: 10.1021/acsabm.8b00501
124. Grillone A, Riva ER, Mondini A, Forte C, Calucci L, Innocenti C, et al. Active targeting of sorafenib: preparation, characterization, and in vitro testing of drug-loaded magnetic solid lipid nanoparticles. Adv Healthc Mater. (2015) 4:1681–90. doi: 10.1002/adhm.201500235
125. Zhu XW, Dong FM, Liu J, Li MS. Resveratrol nanoparticles suppresses migration and invasion of renal cell carcinoma cells by inhibiting matrix metalloproteinase 2 expression and extracellular signal-regulated kinase pathway. J BioMed Nanotechnol. (2022) 18:1001–8. doi: 10.1166/jbn.2022.3310
126. Liu J, Abshire C, Carry C, Sholl AB, Mandava SH, Datta A, et al. Nanotechnology combined therapy: tyrosine kinase-bound gold nanorod and laser thermal ablation produce a synergistic higher treatment response of renal cell carcinoma in a murine model. BJU Int. (2017) 119:342–8. doi: 10.1111/bju.13590
127. Kim H, Niu L, Larson P, Kucaba TA, Murphy KA, James BR, et al. Polymeric nanoparticles encapsulating novel TLR7/8 agonists as immunostimulatory adjuvants for enhanced cancer immunotherapy. Biomaterials. (2018) 164:38–53. doi: 10.1016/j.biomaterials.2018.02.034
128. Alsaab HO, Sau S, Alzhrani RM, Cheriyan VT, Polin LA, Vaishampayan U, et al. Tumor hypoxia directed multimodal nanotherapy for overcoming drug resistance in renal cell carcinoma and reprogramming macrophages. Biomaterials. (2018) 183:280–94. doi: 10.1016/j.biomaterials.2018.08.053
129. Chai D, Shan H, Wang G, Zhang Q, Li H, Fang L, et al. Combining DNA vaccine and AIM2 in H1 nanoparticles exert anti-renal carcinoma effects via enhancing tumor-specific multi-functional CD8+ T-cell responses. Mol Cancer Ther. (2019) 18:323–34. doi: 10.1158/1535-7163.MCT-18-0832
130. Lei Y, Zeng L, Xie S, Fan K, Yu Y, Chen J, et al. Sertraline/ICG-loaded liposome for dual-modality imaging and effective chemo-photothermal combination therapy against metastatic clear cell renal cell carcinoma. Chem Biol Drug Des. (2020) 95:320–31. doi: 10.1111/cbdd.13652
131. Al-Barram LFA. Laser enhancement of cancer cell destruction by photothermal therapy conjugated glutathione (GSH)-coated small-sized gold nanoparticles. Lasers Med Sci. (2021) 36:325–37. doi: 10.1007/s10103-020-03033-y
132. Li C, Zhu P, Xiang H, Jin Y, Lu B, Shen Y, et al. 3D-CEUS tracking of injectable chemo-sonodynamic therapy-enabled mop-up of residual renal cell carcinoma after thermal ablation. Mater Today Bio. (2023) 18:100513. doi: 10.1016/j.mtbio.2022.100513
133. Abshire C, Murad HY, Arora JS, Liu J, Mandava SH, John VT, et al. Focused ultrasound-triggered release of tyrosine kinase inhibitor from thermosensitive liposomes for treatment of renal cell carcinoma. J Pharm Sci. (2017) 106:1355–62. doi: 10.1016/j.xphs.2017.01.027
134. Sakurai Y, Kato A, Harashima H. Involvement of Caveolin-1-mediated transcytosis in the intratumoral accumulation of liposomes. Biochem Biophys Res Commun. (2020) 525:313–8. doi: 10.1016/j.bbrc.2020.02.086
135. Gao X, Jiang P, Zhang Q, Liu Q, Jiang S, Liu L, et al. Peglated-H1/pHGFK1 nanoparticles enhance anti-tumor effects of sorafenib by inhibition of drug-induced autophagy and stemness in renal cell carcinoma. J Exp Clin Cancer Res. (2019) 38:1–15. doi: 10.1186/s13046-019-1348-z
136. Yang Q, Wang YE, Yang Q, Gao Y, Duan X, Fu Q, et al. Cuprous oxide nanoparticles trigger ER stress-induced apoptosis by regulating copper trafficking and overcoming resistance to sunitinib therapy in renal cancer. Biomaterials. (2017) 146:72–85. doi: 10.1016/j.biomaterials.2017.09.008
137. Chien CR, Geynisman DM, Kim B, Xu Y, Shih YCT. Economic burden of renal cell carcinoma—Part I: an updated review. PharmacoEconomics. (2019) 37. doi: 10.1007/s40273-018-0746-y
138. Capitanio U, Montorsi F. Renal cancer. In: The Lancet (2016). doi: 10.1016/S0140-6736(15)00046-X
139. Amin MB, Greene FL, Edge SB, Compton CC, Gershenwald JE, Brookland RK, et al. The Eighth Edition AJCC Cancer Staging Manual: Continuing to build a bridge from a population-based to a more “personalized” approach to cancer staging. CA Cancer J Clin. (2017) 67. doi: 10.3322/caac.21388
140. Ljungberg B, Albiges L, Abu-Ghanem Y, Bedke J, Capitanio U, Dabestani S, et al. European association of urology guidelines on renal cell carcinoma: the 2022 update. Eur Urol. (2022) 82. doi: 10.1016/j.eururo.2022.03.006
141. Campbell S, Uzzo RG, Allaf ME, Bass EB, Cadeddu JA, Chang A, et al. Renal mass and localized renal cancer: AUA guideline. J Urol. (2017) 198:520–9. doi: 10.1016/j.juro.2017.04.100
142. Ouzaid I, Capitanio U, Staehler M, Wood CG, Leibovich BC, Ljungberg B, et al. Surgical metastasectomy in renal cell carcinoma: a systematic review. Eur Urol Oncol. (2019) 2:141–9. doi: 10.1016/j.euo.2018.08.028
143. Green H, Taylor A, Khoo V. Beyond the knife in renal cell carcinoma: A systematic review—To ablate or not to ablate? Cancers (Basel). (2023) 15:3455. doi: 10.3390/cancers15133455
144. (NCCN®) NCCN. CCN guidelines treatment by cancer type. In: Kidney Cancer (2023). Available at: www.nccn.org.
145. Minervini A, Campi R, Lane BR, Cobelli OD, Sanguedolce F, Hatzichristodoulou G, et al. Impact of resection technique on perioperative outcomes and surgical margins after partial nephrectomy for localized renal masses: A prospective multicenter study. J Urology. (2020) 203:496–504. doi: 10.1097/JU.0000000000000591
146. Filippiadis D, Mauri G, Marra P, Charalampopoulos G, Gennaro N, De Cobelli F. Percutaneous ablation techniques for renal cell carcinoma: current status and future trends. Int J Hyperthermia. (2019) 36:21–30. doi: 10.1080/02656736.2019.1647352
147. Klatte T, Kroeger N, Zimmermann U, Burchardt M, Belldegrun AS, Pantuck AJ. The contemporary role of ablative treatment approaches in the management of renal cell carcinoma (RCC): focus on radiofrequency ablation (RFA), high-intensity focused ultrasound (HIFU), and cryoablation. World J Urol. (2014) 32:597–605. doi: 10.1007/s00345-014-1284-7
148. Ponsky L, Lo SS, Zhang Y, Schluchter M, Liu Y, Patel R, et al. Phase I dose-escalation study of stereotactic body radiotherapy (SBRT) for poor surgical candidates with localized renal cell carcinoma. Radiotherapy Oncol. (2015) 117:183–7. doi: 10.1016/j.radonc.2015.08.030
149. Francolini G, Detti B, Ingrosso G, Desideri I, Becherini C, Carta G, et al. Stereotactic body radiation therapy (SBRT) on renal cell carcinoma, an overview of technical aspects, biological rationale and current literature. Crit Rev Oncol Hematol. (2018) 131:24–9. doi: 10.1016/j.critrevonc.2018.08.010
150. Wilson NR, Wiele AJ, Surasi DS, Rao P, Sircar K, Tamboli P, et al. Efficacy and safety of gemcitabine plus doxorubicin in patients with renal medullary carcinoma. Clin Genitourin Cancer. (2021) 19:e401–8. doi: 10.1016/j.clgc.2021.08.007
151. Wehland M, Bauer J, Magnusson NE, Infanger M, Grimm D. Biomarkers for anti-angiogenic therapy in cancer. Int J Mol Sci. (2013) 14:9338–64. doi: 10.3390/ijms14059338
152. Randrup Hansen C, Grimm D, Bauer J, Wehland M, Magnusson NE. Effects and side effects of using sorafenib and sunitinib in the treatment of metastatic renal cell carcinoma. Int J Mol Sci. (2017) 18:461. doi: 10.3390/ijms18020461
153. Yoshino H, Tatarano S, Tamai M, Tsuruda M, Iizasa S, Arima J, et al. Exosomal microRNA-1 and MYO15A as a target for therapy and diagnosis in renal cell carcinoma. Biochem Biophys Res Commun. (2022) 630:71–6. doi: 10.1016/j.bbrc.2022.09.056
154. Cen X, Chen Q, Wang B, Xu H, Wang X, Ling Y, et al. UBE2O ubiquitinates PTRF/CAVIN1 and inhibits the secretion of exosome-related PTRF/CAVIN1. Cell Commun Signal. (2022) 20:191. doi: 10.1186/s12964-022-00996-z
155. Chai D, Liu N, Li H, Wang G, Song J, Fang L, et al. H1/pAIM2 nanoparticles exert anti-tumour effects that is associated with the inflammasome activation in renal carcinoma. J Cell Mol Med. (2018) 22:5670–81. doi: 10.1111/jcmm.13842
156. Zhou X, Cao T. Zinc oxide nanoparticle inhibits tumorigenesis of renal cell carcinoma by modulating lipid metabolism targeting miR-454-3p to repressing metabolism enzyme ACSL4. J Oncol. (2022) 2022:2883404. doi: 10.1155/2022/2883404
157. Chen J, Ren F, Cao W, Wu Z, Ju G, Xiao C, et al. Photothermal therapy enhance the anti-mitochondrial metabolism effect of lonidamine to renal cell carcinoma in homologous-targeted nanosystem. Nanomedicine. (2021) 34:102370. doi: 10.1016/j.nano.2021.102370
158. Zieren RC, Dong L, Pierorazio PM, Pienta KJ, de Reijke TM, Amend SR. Extracellular vesicle isolation from human renal cancer tissue. Med Oncol. (2020) 37:28. doi: 10.1007/s12032-020-1346-1
159. Niciński K, Krajczewski J, Kudelski A, Witkowska E, Trzcińska-Danielewicz J, Girstun A, et al. Detection of circulating tumor cells in blood by shell-isolated nanoparticle - enhanced Raman spectroscopy (SHINERS) in microfluidic device. Sci Rep. (2019) 9:9267.
160. Leitão TP, Corredeira P, Kucharczak S, Rodrigues M, Piairo P, Rodrigues C, et al. Clinical validation of a size-based microfluidic device for circulating tumor cell isolation and analysis in renal cell carcinoma. Int J Mol Sci. (2023) 24. doi: 10.3390/ijms24098404
161. Mao W, Wang K, Zhang W, Chen S, Xie J, Zheng Z, et al. Transfection with Plasmid-Encoding lncRNA-SLERCC nanoparticle-mediated delivery suppressed tumor progression in renal cell carcinoma. J Exp Clin Cancer Res. (2022) 41:252. doi: 10.1186/s13046-022-02467-2
162. Siegel RL, Miller KD, Jemal A. Cancer statistics, 2020. Cancer J Clin. (2020). doi: 10.3322/caac.21590
163. Rosen A, Jayram G, Drazer M, Eggener SE. Global trends in testicular cancer incidence and mortality. Eur Urol. (2011) 60. doi: 10.1016/j.eururo.2011.05.004
164. Barnholtz-Sloan JS, Maldonado JL, Pow-sang J, Guiliano AR. Incidence trends in primary Malignant penile cancer. Urologic Oncology: Semin Original Investigations. (2007) 25. doi: 10.1016/j.urolonc.2006.08.029
165. Maddineni SB, Lau MM, Sangar VK. Identifying the needs of penile cancer sufferers: A systematic review of the quality of life, psychosexual and psychosocial literature in penile cancer. BMC Urol. (2009) 9. doi: 10.1186/1471-2490-9-8
166. Leow JJ, Liu Z, Tan TW, Lee YM, Yeo EK, Chong YL. Optimal management of upper tract urothelial carcinoma: Current perspectives. OncoTargets Ther. (2020) 13. doi: 10.2147/OTT
167. Rossen PB, Pedersen AF, Zachariae R, Von Der Maase H. Health-related quality of life in long-term survivors of testicular cancer. J Clin Oncol. (2009) 27. doi: 10.1200/JCO.2008.19.6931
168. Audenet F, Sfakianos JP. Psychosocial impact of penile carcinoma. Trans Andrology Urol. (2017) 6. doi: 10.21037/tau
169. Huang X, El-Sayed IH, Qian W, El-Sayed MA. Cancer cell imaging and photothermal therapy in the near-infrared region by using gold nanorods. J Am Chem Soc. (2006) 128. doi: 10.1021/ja057254a
170. Liao S, Yue W, Cai S, Tang Q, Lu W, Huang L, et al. Improvement of gold nanorods in photothermal therapy: recent progress and perspective. Front Pharmacol. (2021) 12. doi: 10.3389/fphar.2021.664123
171. Hughes B, Leijte J, Shabbir M, Watkin N, Horenblas S. Non-invasive and minimally invasive staging of regional lymph nodes in penile cancer. World J Urol. (2009) 27. doi: 10.1007/s00345-008-0288-6
172. Feldman AS, McDougal WS, Harisinghani MG. The potential of nanoparticle-enhanced imaging. In: Urologic Oncology: Seminars and Original Investigations. Elsevier (2008). p. 65–73.
173. Pan A, Zhang H, Li Y, Lin TY, Wang F, Lee J, et al. Disulfide-crosslinked nanomicelles confer cancer-specific drug delivery and improve efficacy of paclitaxel in bladder cancer. Nanotechnology. (2016) 27. doi: 10.1088/0957-4484/27/42/425103
174. Tao K, Liu S, Wang L, Qiu H, Li B, Zhang M, et al. Targeted multifunctional nanomaterials with MRI, chemotherapy and photothermal therapy for the diagnosis and treatment of bladder cancer. Biomater Sci. (2020) 8. doi: 10.1039/C9BM01377F
175. Chikhaliwala P, Chandra S. Recent status of the current clinical trials going on for superparamagnetic materials. In: Superparamagnetic Materials for Cancer Medicine. Springer (2023). p. 243–68.
176. Deng X, Huang X, Dong X, Mao G, Xing W. Efficacy and safety of nano-paclitaxel formulation for cancer treatment: evidence from randomized clinical trials. Future Med. (2023). doi: 10.2217/nnm-2023-0080
177. Anselmo AC, Mitragotri S. Nanoparticles in the clinic: An update. Bioeng Transl Med. (2019) 4. doi: 10.1002/btm2.10143
178. Rosenblum D, Joshi N, Tao W, Karp JM, Peer D. Progress and challenges towards targeted delivery of cancer therapeutics. Nat Commun. (2018) 9. doi: 10.1038/s41467-018-03705-y
179. Wolfram J, Suri K, Huang Y, Molinaro R, Borsoi C, Scott B, et al. Evaluation of anticancer activity of celastrol liposomes in prostate cancer cells. J Microencapsul. (2014) 31. doi: 10.3109/02652048.2013.879932
180. Blanco E, Shen H, Ferrari M. Principles of nanoparticle design for overcoming biological barriers to drug delivery. Nat Biotechnol. (2015) 33. doi: 10.1038/nbt.3330
181. Etheridge ML, Campbell SA, Erdman AG, Haynes CL, Wolf SM, McCullough J. The big picture on nanomedicine: The state of investigational and approved nanomedicine products. Nanomedicine: Nanotechnology Biology Med. (2013) 9. doi: 10.1016/j.nano.2012.05.013
182. Shi J, Kantoff PW, Wooster R, Farokhzad OC. Cancer nanomedicine: Progress, challenges and opportunities. Nat Rev Cancer. (2017) 17. doi: 10.1038/nrc.2016.108
Keywords: nanomedicine, nanoparticle, urology, cancer, treatment, clinical, biosafety
Citation: Fattahi MR, Dehghani M, Paknahad S, Rahiminia S, Zareie D, Hoseini B, Oroomi TR, Motedayyen H and Arefnezhad R (2024) Clinical insights into nanomedicine and biosafety: advanced therapeutic approaches for common urological cancers. Front. Oncol. 14:1438297. doi: 10.3389/fonc.2024.1438297
Received: 25 May 2024; Accepted: 29 July 2024;
Published: 13 August 2024.
Edited by:
Hamed Ahmadi, University of Minnesota Medical Center, United StatesReviewed by:
Sayeda Yasmin-Karim, Harvard Medical School, United StatesR. C. Koumar, Yenepoya University, India
Copyright © 2024 Fattahi, Dehghani, Paknahad, Rahiminia, Zareie, Hoseini, Oroomi, Motedayyen and Arefnezhad. This is an open-access article distributed under the terms of the Creative Commons Attribution License (CC BY). The use, distribution or reproduction in other forums is permitted, provided the original author(s) and the copyright owner(s) are credited and that the original publication in this journal is cited, in accordance with accepted academic practice. No use, distribution or reproduction is permitted which does not comply with these terms.
*Correspondence: Hossein Motedayyen, aG1vdGVkYXl5ZW5AZ21haWwuY29t; Reza Arefnezhad, UmV6YS5hcmVmMTM3NEBnbWFpbC5jb20=