- Department of Endocrinology, The First Affiliated Hospital of Yangtze University, Jingzhou, China
Anaplastic thyroid cancer (ATC) is the most aggressive type of thyroid cancer. While ATC is rare, its mortality is high. Standard treatments, such as surgery, radiotherapy, and chemotherapy, have demonstrated limited efficacy in managing ATC. However, the advent of immunotherapy has significantly improved the prognosis for patients with ATC. Immunotherapy effectively targets and eliminates tumor cells by using the power of the body’s immune cells. The neoantigen is an atypical protein generated by somatic mutation, is exclusively observed in neoplastic cells, and is devoid of central tolerance. Neoantigens exhibit enhanced specificity towards tumor cells and display robust immunogenic properties. Currently, neoantigen therapy is primarily applied in immune checkpoint inhibitors and cellular immunotherapy, encompassing adoptive immunotherapy and tumor vaccines. This study discusses the mechanism, tumor microenvironment, clinical trials, adverse events, limitations and future directions associated with ATC immunotherapy.
1 Introduction
Also known as undifferentiated thyroid carcinoma, anaplastic thyroid carcinoma (ATC) is the most malignant thyroid cancer and one of the most aggressive solid tumors (1). ATC accounts for <2% of thyroid cancers, but >40% of patients have large primary tumors, extrathyroidal spread, and local and distant metastases at the time of diagnosis (2). The median survival after initial diagnosis is approximately four months, and one in five patients survives >12 months. Furthermore, the 5-year survival rate is close to 0% (3). The standard ATC treatment includes surgery, radiotherapy, and chemotherapy. However, ATC is extremely malignant, and the effect of treatment is limited due to its invasiveness, lack of differentiation, and chemoresistance (4, 5). While ATC features gene mutations the incidence rate is lower than that of differentiated thyroid cancer. The signaling pathways in ATC are mainly MAPK, PI3K–AKT–mTOR, and JAK–STAT, which contain important targets such as RET and EGFR. Targeted drugs such as sorafenib, lenvatinib, dabrafenib, and trametinib have exerted some effects, but drug resistance is the main issue that should be solved (6). Therefore, safer and more effective treatments are needed to improve the prognosis of patients with ATC. The use of immunotherapy to treat solid tumors, including ATC, has become a promising treatment option (7). Immunotherapy has developed rapidly in recent years and yielded significant results in reducing disease recurrence and prolonging patient survival. Moreover, various levels of combined therapy can synergistically combat tumors and minimize side effects. This article reviews the current immune mechanism and immunotherapy of ATC.
2 ATC immunotherapy mechanisms
2.1 Fundamentals of immunotherapy
Tumor immunotherapy uses the body’s immune cells to eliminate tumor cells, with T cells being the main effector cells. Tumor elimination by T cells begins with the recognition of tumor antigens (8), which are self-molecules altered by gene mutation, protein truncation, protein misfolding, or abnormal post-translational modification (9). Tumor antigens are divided into tumor-associated antigens (TAAs) and tumor-specific antigens (TSAs) (10).TAAs are macromolecules found on the surface of tumor cells and can also be found in normal tissues. Nevertheless, TAAs are highly expressed in tumors, most of which have central tolerance and weak immunogenicity (11). TSAs, or neoantigens, are not expressed in normal somatic cells and do not exhibit central tolerance. TSAs are more specific to tumor cells and have strong immunogenicity. Neoantigens can be presented on the cell surface and subsequently recognized by T cells under the action of major histocompatibility complex (MHC) molecules, causing T cell activation and promoting T cell-mediated attack and removal of tumor cells (12). Neoantigens contribute to tumor-specific immune responses and have been used as targets for novel, precise, and personalized tumor immunotherapy (13).Currently, neoantigen therapy is mainly used in immune checkpoint inhibitors (ICIs) and cellular immunotherapy (adoptive immunotherapy and tumor vaccines).
2.2 Relationship between tumor microenvironment and ATC
The tumor microenvironment (TME) refers to the environment required for tumor cell appearance and development and contains tumor cells, extracellular matrix, immune cells, and the cytokines, metabolites, and exosomes they release (14). Different organs have biologically unique microenvironments, and different thyroid cancer types also have greatly differing immunological TMEs (15). Immune cells in the ATC TME mainly consist of tumor-associated macrophages (TAMs), tumor-associated mast cells (MCs), dendritic cells (DCs), natural killer cells (NKs), cytotoxic T cells (CTLs), and regulatory T cells (Tregs) (16). However, some scientists believe that ATC only involves cancer cells, macrophages, and vascular endothelial cells and almost no other cells (17). Due to the low prevalence and low possibility of surgery for ATC, most of the current studies address the role of the phenotypic characteristics of tumor-associated immune cells in the pathogenesis or progression of papillary thyroid carcinoma, whereas there are few studies on ATC.
Thyroid cancer has an extremely wide range of TAM density, with TAM density being the highest in ATC (18). TAMs can directly promote tumor occurrence, development, and metastasis by releasing various inflammatory factors, growth factors, and matrix proteases. TAMs can also indirectly promote tumor progression by mediating tumor angiogenesis and immunosuppression (19, 20). TAMs are mainly divided into M0, M1, and M2 types (21). M0 TAMS are usually dormant, while the proinflammatory M1 TAMs mainly produce cytokines such as tumor necrosis factor-α (TNF-α) and interleukin-1 (IL-1), which inhibit and kill tumor cells. The anti-inflammatory M2 TAMs produce factors such as IL-10 and IL-13, which promote tumor formation and development (22). In ATC, TAMs account for >50% of nuclear cells, and the M2 type is predominant (17). M2-like TAMs accelerate ATC cell metastasis by activating the insulin receptor (IR)-A/insulin-like growth factor 1 receptor (IGF1R)-mediated PI3K–AKT–mTOR pathway and upregulating IGF-1/IGF-2 (23). It is also believed that only ATC contains a branched TAM network to promote ATC invasion through metabolic and nutritional functions (17).
The ATC microenvironment has significantly increased tumor-infiltrating lymphocytes (TILs) as compared with normal thyroid tissues, and most of them are CD8+T cells (24). However, only one investigator demonstrated that CD8+ T cells enhance their killing effect on ATC cells by secreting granzyme, TNF-α, and interferon-γ (IFN-γ) (25). In patients with ATC, NKs kill tumor cells by inducing apoptosis via the release of cytolytic granules (26). MCs are the first immune cells recruited during inflammation and are associated with ATC aggressiveness (27). ATC has a higher number of MCs than other forms of thyroid cancer. By producing IL-6, TNF-α, and CXC chemokine ligand 8 (CXCL8)/IL-8, MCs aid epithelial–mesenchymal transition in ATC cells (28). Galectin-9 aids MC support of ATC cell adhesion, angiogenesis, metastasis, and tumor immune escape (29). Most of the body’s professional antigen-presenting cells are termed DCs, yet the amount of DCs in ATC is much lower than that of other thyroid cancers (30, 31).
Soluble mediators such as cytokines and chemokines are mainly released by tumor-infiltrating immune cells and can also be released by cancer cells. ATC cells secrete CXCL9 and CXCL10, which are chemotactic to T cells (24). IL-4 and IL-10 upregulate the anti-apoptotic proteins B-cell lymphoma-2 (Bcl-2) and Bcl-xL to promote ATC cell progression and chemotherapy resistance (32). CXC chemokine receptor 4 (CXCR4) is involved in the local invasion and distant metastasis of ATC cells mediated by stromal cell-derived factor (33). The transcription factor CREB3L1 activates the extracellular matrix signaling pathway, maintains the fibroblast-like characteristics of ATC cells, reshapes the tumor interstitial microenvironment, and drives ATC malignant transformation (34). ATC is also linked to elevated levels of other cytokines, including IL-6, IL-1, granulocyte colony-stimulating factor, macrophage colony-stimulating factor, CXCL8, and CXCR4 (35, 36). One obvious characteristic of ATC is the high percentage of immunosuppressive cytokines (24). By altering the immunological microenvironment, immunotherapy can reinstate the tumor-killing capacity of anti-tumor immune cells (37).
Single-cell RNA sequencing (scRNA-seq) is a novel technology that enables comprehensive analysis of the cell composition and transcriptional phenotype of malignant cells and surrounding immune cells by examining the transcriptome information of individual cells (38). In one study, scholars used scRNA-seq to distinguish tumor cells from normal cells based on differences in copy number (39). They estimated that the average prediction accuracy for identifying tumor cells was 97%. Lu sequenced the single-cell RNA of ATC patients and classified the cell types (40). They divided the cells into eight main types and found that in patients with ATC, the number of endothelial cells decreased significantly, while the number of myeloid cells increased significantly. Furthermore, they also discovered that ATC cells exhibited overexpression of mesenchymal and glial genes. Compared with papillary thyroid carcinoma, the number of M2 macrophages in ATC significantly increased, while the number of M1 macrophages decreased. In ATC tumors, the total depleted cells account for more than 50% of the T cell population. It is also found that TRα1, as a transcription factor, plays a role in inhibiting tumor growth through various signaling pathways. ScRNA-seq revealed that the induction of PAX8 by TRα1 transformed the cell landscape of ATC from one state to another through a transcription program (41). ScRNA-Seq not only deepens our understanding of ATC cell composition and heterogeneity but also offers new insights for personalized therapy and the development of molecular markers.
3 Application of immunotherapy in ATC
3.1 Immune checkpoint inhibitors
Immune cells have cell surface receptors known as ICIs, which control T lymphocyte activation and effector activities (42). The most well-characterized ICIs are programmed cell death protein 1 (PD-1) and cytotoxic T lymphocyte antigen 4 (CTLA-4). The other ICIs include lymphocyte activation gene 3, T-cell immunoglobulin and immunoreceptor tyrosine-based inhibitory motif domain, T-cell immunoglobulin domain and mucin domain 3 (43). A trial of the PD-1 inhibitor spartalizumab in patients with advanced/metastatic ATC reported an overall response rate of 19%, including a complete response of 7% and a partial response of 12%. PD-L1-positive individuals had a higher response rate (29%) than PD-L1-negative patients (0%) (44). An objective response rate of 16% and a 1-year survival rate of 38% were noted for ATC treated with pembrolizumab or nivolumab (45). However, only one patient in a trial using stereotactic body radiation therapy and the CTLA-4 inhibitor tremelimumab or the PD-L1 inhibitor durvalumab for metastatic ATC lived for more than a year (46).Furthermore, additional research reported the efficacy of pembrolizumab and spartalizumab for patients with ATC, which demonstrated the anti-tumor action of ICIs in ATC management (47, 48).
Before starting immunotherapy, most of the patients in these trials receiving ICIs underwent surgery, radiation, chemotherapy, and even targeted therapy. Six patients with metastatic ATC who had not responded to radiation, chemotherapy, or radioiodine therapy were included in a trial evaluating the combination of lenvatinib and pembrolizumab. The results demonstrated that 66% of the patients had a full response, 16% had stable illness, and 16% had progressing disease. The median progression-free survival for all patients was 16.5 months. Half of the ATC patients were still receiving therapy at the time of data cutoff, with treatment durations of 1–40 months (49). Following surgery, a 67-year-old patient with ATC was treated with the PD-1 inhibitor sintilimab in conjunction with the antiangiogenic drug anlotinib. The patient demonstrated notable tumor shrinkage and an 18.3-month maintained remission (50). After receiving combination therapy consisting of the anti-PD-1 antibody camrelizumab and the multitargeted kinase inhibitor famitinib, a patient with locally advanced unresectable ATC underwent postoperative radiotherapy and complete surgical resection after computed tomography demonstrated a partial lesion response after three cycles. The patient demonstrated a very good quality of life about 24 months after diagnosis (51). Another study reported that three patients with ATC died within six months despite treatment with surgery, radiation, and chemotherapy in addition to pembrolizumab (52). Based on the aforementioned trials, it is believed that the treatment effect might also be connected to the duration of treatment overlap. Hence, more research is needed to fully understand the synergistic mechanism and tolerance of combined treatments. Currently, there are several reports on ongoing ATC studies involving PD-1/PD-L1 inhibitor (NCT05453799, and NCT05119296), or PD-1 combined with other treatment (NCT04171622, NCT04675710, NCT05696548, NCT04238624, NCT05659186, NCT03181100, NCT04400474, and NCT04579757). Please refer to Tables 1, 2 for a summary of trials.
Adverse effects are unavoidable when using ICIs. According to NCT02404441, grade 1 or 2 diarrhea, pruritus, exhaustion, and fever were the most frequent treatment-related adverse effects in patients with advanced/metastatic ATC receiving spartalizumab (44). The most common grade 2–4 adverse events were hypertension, fatigue, weight loss/anorexia, oral mucositis, diarrhea, joint/muscle pain, and hand-foot syndrome in six patients with metastatic ATC who were treated with combined lenvatinib and pembrolizumab after all other forms of surgery, chemotherapy, or radiotherapy had failed. However, the lenvatinib-induced adverse effects necessitated treatment stopping in two patients (49). One patient receiving pembrolizumab treatment developed severe grade 4 colitis (bloody stools, severe dehydration, and hypotension), necessitating extensive fluid resuscitation and systemic steroids (53). Despite the ICI adverse effects reported in several studies, most of them can be alleviated with symptomatic therapy. Therefore, ICIs have emerged as a new tool in ATC treatment.
3.2 Adoptive immunotherapy
Adoptive immunotherapy involves injecting immune cells with anti-tumor activity to either directly kill or activate the body’s immune response to kill tumor cells. It includes TILs, T cell receptor engineered T cells (TCR-T), chimeric antigen receptor T cell therapy (CAR-T), and NK therapy. TIL therapy was effective in treating numerous solid tumors, including melanoma, breast, cervical, head and neck, stomach, liver, esophagus, and lung cancers (54). The effect of autologous TILs (LT-145 or LN-145-S1) on patients with ATC and some other cancers is being studied in NCT03449108. As an alternative to TIL therapy, T cells can be isolated from peripheral blood and targeted to tumor cells by transferring synthetic TCR or CAR using gene therapy techniques (55). TCR-T involves transfecting TCR α- and β-chain genes into T cells, which can recognize TSAs. This results in structural alterations in the TCR antigen-binding region of the T cells, enabling them to specifically recognize the corresponding tumor antigens. T lymphocytes expressing specific TCRs can recognize human leukocyte antigen (HLA)–peptide complexes on the surface of tumor cells. Antigenic stimulation signals are transmitted by phosphorylating the intracellular region of the immune receptor tyrosine activation motifs, which activates the T cell immune response (56). Patients with colorectal cancer, synovial sarcoma, and metastatic melanoma demonstrated a notable clinical response to TCR-T. CAR overcomes some of the limitations of TCR. However, the success of CAR-T therapy has been concentrated in hematologic tumors, whereas only partial progression has been observed in solid tumors to date (57, 58). Ongoing studies are evaluating the safety and tolerability of AIC2 CAR-T cells in patients with relapsed/refractory poorly differentiated thyroid cancer and ATC (NCT04420754). Animal studies have demonstrated that adoptive cell therapy based on NKs significantly inhibited metastatic growth in ATC lung metastasis model mice (59). An ongoing study (NCT05194709) is evaluating the efficacy, safety, and pharmacokinetics of anti-5T4 CAR-NK cells in patients with advanced solid tumors, and the NCT03415100 study is evaluating the safety and feasibility of CAR-NK cell therapy targeting NKG2D ligands in patients with metastatic solid tumors.
TIL-treated patients with advanced cutaneous melanoma experienced many adverse events, such as thrombocytopenia, fever, chills, neutropenia, and tachycardia (60). In colorectal cancer, TCR-T causes severe transient inflammatory colitis (61). The most common adverse effects of CAR-T in other tumors are cytokine release syndrome and immune effector cell-associated neurotoxicity syndrome (62). Grade 3 cytokine release syndrome also occurred in two patients in a phase I study of CD33 CAR-NK cells in patients with relapsed or refractory acute myeloid leukemia (63). However, the adverse effects of adoptive immunotherapy in ATC are unclear and require further clarification.
3.3 Tumor vaccines
Tumor vaccines can stimulate the body to generate active specific immunity against tumor cells or cells or molecules in the TME that promote tumor growth. ATC typically has a high mutation burden, and most of the neoantigens in ATC can be used for tumor vaccines. Thus, tumor vaccines might be more effective in ATC (64). The current ATC vaccines mainly include DC vaccines and oncolytic virus (OV) vaccines. Triiodothyronine can enhance DC ability to stimulate cytotoxic T-cell responses, thereby enhancing anti-tumor responses (65). DC vaccines have been successfully used to treat medullary thyroid carcinoma, and the treatment of thyroid cancer might be more effective than that for other tumors (66). OVs use natural or genetically modified viruses to specifically infect and lyse cancer cells but do not harm normal cells. The anti-cancer activities of OVs are derived from multimodal cancer-killing mechanisms. First, OVs infect and replicate in cancer cells, inducing tumor cell lysis and releasing infectious viral progeny that spreads to the surrounding tumor cells. Second, OV-mediated oncolysis of tumor cells initiates the release of tumor-associated antigens, cellular danger-associated molecular pattern signals, and cytokines that promote the maturation of antigen-presenting cells and activate antigen-specific CD4 and CD8 T cell responses. Third, OVs can specifically infect and destroy tumor vascular endothelial cells and stromal cells and destroy tumor blood vessels by promoting endostatin and angiostatin production (67). Furthermore, OVs induced cell death and tumor regression in cultured ATC cells and mouse models and modulated the ATC microenvironment to shift the M2-type TAMs to the proinflammatory M1 phenotype (68, 69). Although adverse events such as fever, injection site reactions, headache, and vomiting occurred in patients with glioma and melanoma treated with the OV vaccine, they were all tolerable (70). Nevertheless, patients with ATC have not received a tumor vaccine. It is expected that the development of DC And OV vaccines will become a means of treating ATC (Figure 1).
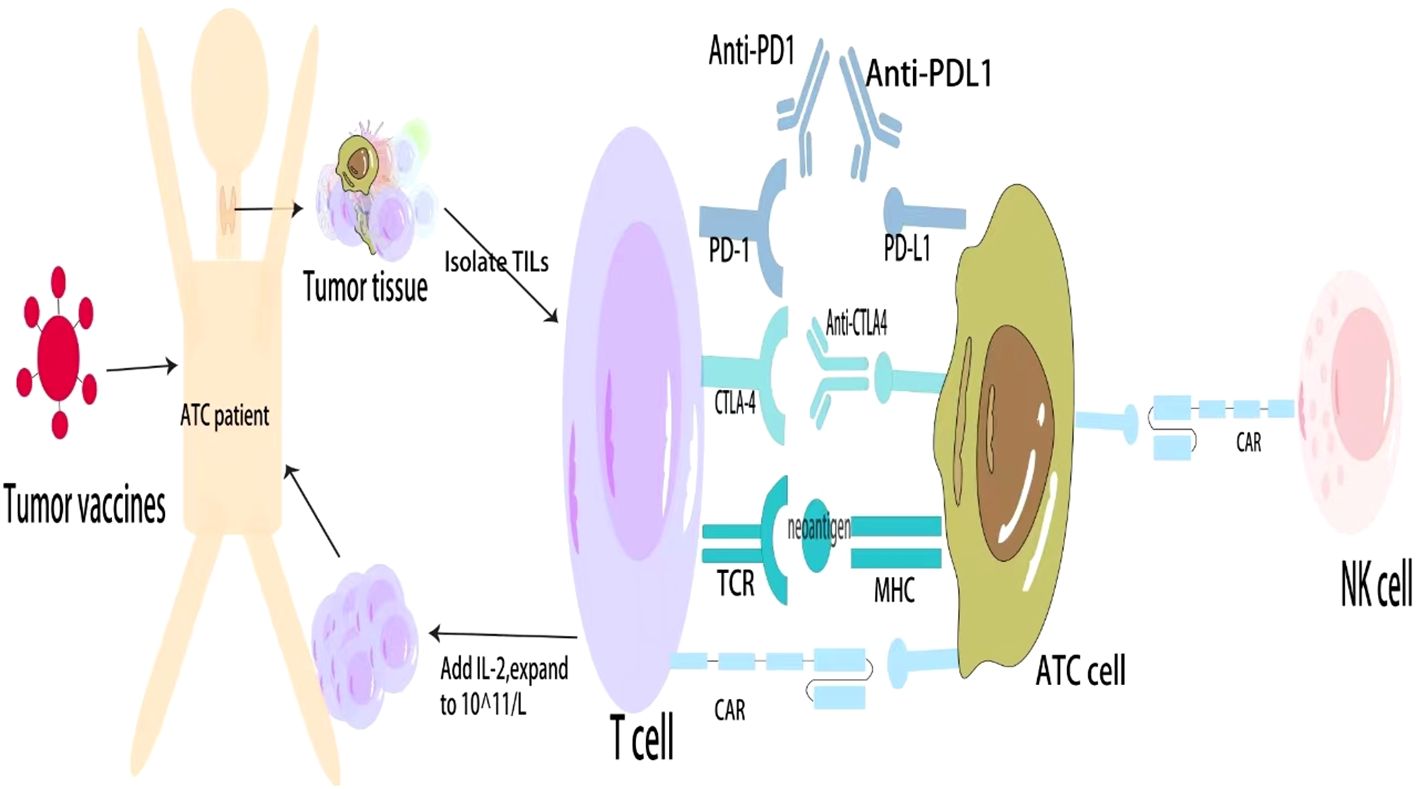
Figure 1 General approaches to immunotherapy for ATC. ATC, Anaplastic thyroid cancer; PD, Programmed cell death protein; CTLA, Cytotoxic T lymphocyte antigen; TCR, T cell receptor; CAR, Chimeric antigen receptor; MHC, Major histocompatibility complex; TILs, Tumor-infiltrating lymphocytes; NKs, Natural killer cells.
4 Application limitations and future directions of ATC immunotherapy
Immunotherapy is a promising treatment for patients with ATC, but some clinical management issues persist. ICIs have had tremendous success, but many patients do not respond to them (71). The cell preparation, expansion, and infusion of TILs are complex which can take a long time for patients (72). TCR is limited by human HLA and must match HLA to be effective, whereas CAR-T can recognize extracellular antigens presented independently of HLA (73). However, CAR-T therapy is subject to inadequate infiltration of CAR-T cells, poor proliferation and durability, toxicity control, and an immunosuppressive microenvironment (74). The NK survival rate and cytotoxicity are significantly reduced by the CAR-binding epitope location and its distance from the surface of CAR-NK cells and by NK sensitivity to freeze-thawing (75). DC vaccines are complex and expensive to produce, require ex vivo expansion, maturation, and activation, and have a short half-life in vivo (76). Furthermore, the OV vaccines are subject to drug resistance, and the pharmacokinetics, quality control, and detection methods require further investigation (77, 78).
Immunotherapy is the fourth major therapy for cancer treatment and has achieved good results. However, considering the abovementioned adverse effects and limitations, further development and optimization are required. As the effectiveness of a single treatment is currently restricted, surgery, radiotherapy, chemotherapy, targeted therapy, and immunotherapy can be combined to varying degrees to maximize the advantages of each treatment. Aiming to alleviate symptoms, inhibit tumor cell proliferation, and promote tumor cell apoptosis. Oncolytic herpes simplex virus and BRAF inhibitors enhanced the immune-mediated anti-tumor effect in animal tests, hence improving the survival rate of the ATC mouse model (79). ICIs, targeted therapies, and CAR-T combination therapy demonstrated significant synergistic effects in rectal cancer, non-small cell lung cancer, liver cancer, breast cancer, hematologic neoplasms, and other cancers (80). When the two promising treatments are used in combination, several unknowns remain to be investigated, such as the relative dose, timing, design, and means of overcoming mitigating factors (81). Better preclinical models that more effectively recapitulate the intricate interactions of human immune cells are required to increase the success rate of clinical translation. Given the large presence of TAMs in the ATC microenvironment, targeting TAMs might be a potential ATC treatment. Modifying macrophages with CAR might aid in overcoming immunosuppressive cytokines and upregulate antigen presentation. CAR-M can home in on tumor tissue, have immunity to immunosuppressive TMEs, and lack an immune exhaustion process similar to those of T cells and NKs. Thus, CAR-M might be another potential cell treatment technique (82). CAR-M has been effectively used in animal trials to treat ovarian and breast cancer (83, 84).
5 Conclusion
Currently, several studies indicated that immunotherapy can prolong the survival time of patients with ATC, and combining different therapy modalities has greater efficacy than a single-arm treatment. Combination therapy can impede and regulate tumor growth, lower the risk of adverse effects, and exert synergistic anti-tumor effects. Due to the advancements in understanding the mechanisms of immune action, immunotherapy may progress rapidly, which will be excellent news for most patients with cancer.
Author contributions
JC: Writing – original draft. ZX: Writing – review & editing. HW: Writing – review & editing.
Funding
The author(s) declare financial support was received for the research, authorship, and/or publication of this article. This study was funded by the National Natural Science Foundation of China 82070809.
Conflict of interest
The authors declare that the research was conducted in the absence of any commercial or financial relationships that could be construed as a potential conflict of interest.
Publisher’s note
All claims expressed in this article are solely those of the authors and do not necessarily represent those of their affiliated organizations, or those of the publisher, the editors and the reviewers. Any product that may be evaluated in this article, or claim that may be made by its manufacturer, is not guaranteed or endorsed by the publisher.
References
1. Pacini F, Castagna MG, Brilli L, Pentheroudakis G. Thyroid cancer: ESMO Clinical Practice Guidelines for diagnosis, treatment and follow-up. Ann Oncol Off J Eur Soc Med Oncol. (2012) 23 Suppl 7:vii110–9. doi: 10.1093/annonc/mds230
2. Maniakas A, Dadu R, Busaidy NL, Wang JR, Ferrarotto R, Lu C, et al. Evaluation of overall survival in patients with anaplastic thyroid carcinoma, 2000-2019. JAMA Oncol. (2020) 6:1397–404. doi: 10.1001/jamaoncol.2020.3362
3. Garcia-Alvarez A, Hernando J, Carmona-Alonso A, Capdevila J. What is the status of immunotherapy in thyroid neoplasms? Front Endocrinol. (2022) 13:929091. doi: 10.3389/fendo.2022.929091
4. Ferrari SM, Elia G, Ragusa F, Ruffilli I, La Motta C, Paparo SR, et al. Novel treatments for anaplastic thyroid carcinoma. Gland Surg. (2020) 9:S28–s42. doi: 10.21037/gs
5. Saini S, Tulla K, Maker AV, Burman KD, Prabhakar BS. Therapeutic advances in anaplastic thyroid cancer: a current perspective. Mol Cancer. (2018) 17:154. doi: 10.1186/s12943-018-0903-0
6. Yuan J, Guo Y. Targeted therapy for anaplastic thyroid carcinoma: advances and management. Cancers. (2022) 15:179. doi: 10.3390/cancers15010179
7. Pham T, Roth S, Kong J, Guerra G, Narasimhan V, Pereira L, et al. An update on immunotherapy for solid tumors: A review. Ann Surg Oncol. (2018) 25:3404–12. doi: 10.1245/s10434-018-6658-4
8. Wu Z, Li S, Zhu X. The mechanism of stimulating and mobilizing the immune system enhancing the anti-tumor immunity. Front Immunol. (2021) 12:682435. doi: 10.3389/fimmu.2021.682435
9. Meeusen E, Lim E, Mathivanan S. Secreted tumor antigens - immune biomarkers for diagnosis and therapy. Proteomics. (2017) 17:1600442. doi: 10.1002/pmic.201600442
10. Song Q, Zhang CD, Wu XH. Therapeutic cancer vaccines: From initial findings to prospects. Immunol Lett. (2018) 196:11–21. doi: 10.1016/j.imlet.2018.01.011
11. Yarchoan M, Johnson BA 3rd, Lutz ER, Laheru DA, Jaffee EM. Targeting neoantigens to augment antitumour immunity. Nat Rev Cancer. (2017) 17:209–22. doi: 10.1038/nrc.2016.154
12. Zhang Z, Lu M, Qin Y, Gao W, Tao L, Su W, et al. Neoantigen: A new breakthrough in tumor immunotherapy. Front Immunol. (2021) 12:672356. doi: 10.3389/fimmu.2021.672356
13. Chen F, Zou Z, Du J, Su S, Shao J, Meng F, et al. Neoantigen identification strategies enable personalized immunotherapy in refractory solid tumors. J Clin Invest. (2019) 129:2056–70. doi: 10.1172/JCI99538
14. Labani-Motlagh A, Ashja-Mahdavi M, Loskog A. The tumor microenvironment: A milieu hindering and obstructing antitumor immune responses. Front Immunol. (2020) 11:940. doi: 10.3389/fimmu.2020.00940
15. Fidler IJ. The pathogenesis of cancer metastasis: the 'seed and soil' hypothesis revisited. Nat Rev Cancer. (2003) 3:453–8. doi: 10.1038/nrc1098
16. Menicali E, Guzzetti M, Morelli S, Moretti S, Puxeddu E. Immune landscape of thyroid cancers: new insights. Front Endocrinol. (2020) 11:637826. doi: 10.3389/fendo.2020.637826
17. Caillou B, Talbot M, Weyemi U, Pioche-Durieu C, Al Ghuzlan A, Bidart JM, et al. Tumor-associated macrophages (TAMs) form an interconnected cellular supportive network in anaplastic thyroid carcinoma. PloS One. (2011) 6:e22567. doi: 10.1371/journal.pone.0022567
18. Jung KY, Cho SW, Kim YA, Kim D, Oh BC, Park DJ, et al. Cancers with higher density of tumor-associated macrophages were associated with poor survival rates. J Pathol Trans Med. (2015) 49:318–24. doi: 10.4132/jptm.2015.06.01
19. Li Y, Jia Y, Xu Y, Li K. DMF activates NRF2 to inhibit the pro-invasion ability of TAMs in breast cancer. Front Oncol. (2021) 11:706448. doi: 10.3389/fonc.2021.706448
20. Mantovani A, Allavena P, Marchesi F, Garlanda C. Macrophages as tools and targets in cancer therapy. Nat Rev Drug Discov. (2022) 21:799–820. doi: 10.1038/s41573-022-00520-5
21. MacDonald L, Jenkins J, Purvis G, Lee J, Franco AT. The thyroid tumor microenvironment: potential targets for therapeutic intervention and prognostication. Hormones Cancer. (2020) 11:205–17. doi: 10.1007/s12672-020-00390-6
22. Chen Y, Song Y, Du W, Gong L, Chang H, Zou Z. Tumor-associated macrophages: an accomplice in solid tumor progression. J Biomed Sci. (2019) 26:78. doi: 10.1186/s12929-019-0568-z
23. Lv J, Liu C, Chen FK, Feng ZP, Jia L, Liu PJ, et al. M2−like tumour−associated macrophage−secreted IGF promotes thyroid cancer stemness and metastasis by activating the PI3K/AKT/mTOR pathway. Mol Med Rep. (2021) 24:604. doi: 10.3892/mmr
24. Chakraborty S, Carnazza M, Jarboe T, DeSouza N, Li XM, Moscatello A, et al. Disruption of cell-cell communication in anaplastic thyroid cancer as an immunotherapeutic opportunity. Adv Exp Med Biol. (2021) 1350:33–66. doi: 10.1007/978-3-030-83282-7_2
25. Wang X, Zhang Y, Zheng J, Yao C, Lu X. LncRNA UCA1 attenuated the killing effect of cytotoxic CD8 + T cells on anaplastic thyroid carcinoma via miR-148a/PD-L1 pathway. Cancer Immunol Immunother CII. (2021) 70:2235–45. doi: 10.1007/s00262-020-02753-y
26. Yin M, Di G, Bian M. Dysfunction of natural killer cells mediated by PD-1 and Tim-3 pathway in anaplastic thyroid cancer. Int Immunopharmacol. (2018) 64:333–9. doi: 10.1016/j.intimp.2018.09.016
27. Visciano C, Liotti F, Prevete N, Cali G, Franco R, Collina F, et al. Mast cells induce epithelial-to-mesenchymal transition and stem cell features in human thyroid cancer cells through an IL-8-Akt-Slug pathway. Oncogene. (2015) 34:5175–86. doi: 10.1038/onc.2014.441
28. Veschi V, Verona F, Lo Iacono M, D'Accardo C, Porcelli G, Turdo A, et al. Cancer stem cells in thyroid tumors: from the origin to metastasis. Front Endocrinol. (2020) 11:566. doi: 10.3389/fendo.2020.00566
29. Hou Y, Wang Q, Su L, Zhu Y, Xiao Y, Feng F. Increased tumor-associated mast cells facilitate thyroid cancer progression by inhibiting CD8+ T cell function through galectin-9. Braz J Med Biol Res = Rev Bras pesquisas medicas e biologicas. (2023) 56:e12370. doi: 10.1590/1414-431x2023e12370
30. Mellman I, Steinman RM. Dendritic cells: specialized and regulated antigen processing machines. Cell. (2001) 106:255–8. doi: 10.1016/S0092-8674(01)00449-4
31. Ugolini C, Basolo F, Proietti A, Vitti P, Elisei R, Miccoli P, et al. Lymphocyte and immature dendritic cell infiltrates in differentiated, poorly differentiated, and undifferentiated thyroid carcinoma. Thyroid Off J Am Thyroid Assoc. (2007) 17:389–93. doi: 10.1089/thy.2006.0306
32. Stassi G, Todaro M, Zerilli M, Ricci-Vitiani L, Di Liberto D, Patti M, et al. Thyroid cancer resistance to chemotherapeutic drugs via autocrine production of interleukin-4 and interleukin-10. Cancer Res. (2003) 63:6784–90.
33. Hwang JH, Hwang JH, Chung HK, Kim DW, Hwang ES, Suh JM, et al. CXC chemokine receptor 4 expression and function in human anaplastic thyroid cancer cells. J Clin Endocrinol Metab. (2003) 88:408–16. doi: 10.1210/jc.2002-021381
34. Pan Z, Xu T, Bao L, Hu X, Jin T, Chen J, et al. CREB3L1 promotes tumor growth and metastasis of anaplastic thyroid carcinoma by remodeling the tumor microenvironment. Mol Cancer. (2022) 21:190. doi: 10.1186/s12943-022-01658-x
35. Polymeris A, Kogia C, Ioannidis D, Lilis D, Drakou M, Maounis N, et al. Excessive leukocytosis leading to a diagnosis of aggressive thyroid anaplastic carcinoma: A case report and relevant review. Eur Thyroid J. (2020) 9:162–8. doi: 10.1159/000506767
36. Liu Q, Sun W, Zhang H. Roles and new insights of macrophages in the tumor microenvironment of thyroid cancer. Front Pharmacol. (2022) 13:875384. doi: 10.3389/fphar.2022.875384
37. Lv B, Wang Y, Ma D, Cheng W, Liu J, Yong T, et al. Immunotherapy: reshape the tumor immune microenvironment. Front Immunol. (2022) 13:844142. doi: 10.3389/fimmu.2022.844142
38. Wang T, Shi J, Li L, Zhou X, Zhang H, Zhang X, et al. Single-cell transcriptome analysis reveals inter-tumor heterogeneity in bilateral papillary thyroid carcinoma. Front Immunol. (2022) 13:840811. doi: 10.3389/fimmu.2022.840811
39. Gao R, Bai S, Henderson YC, Lin Y, Schalck A, Yan Y, et al. Delineating copy number and clonal substructure in human tumors from single-cell transcriptomes. Nat Biotechnol. (2021) 39:599–608. doi: 10.1038/s41587-020-00795-2
40. Lu L, Wang JR, Henderson YC, Bai S, Yang J, Hu M, et al. Anaplastic transformation in thyroid cancer revealed by single-cell transcriptomics. J Clin Invest. (2023) 133:e169653. doi: 10.1172/JCI169653
41. Hwang E, Doolittle WKL, Zhu YJ, Zhu X, Zhao L, Yu Y, et al. Thyroid hormone receptor α1: a novel regulator of thyroid cancer cell differentiation. Oncogene. (2023) 42:3075–86. doi: 10.1038/s41388-023-02815-2
42. Li X, Shao C, Shi Y, Han W. Lessons learned from the blockade of immune checkpoints in cancer immunotherapy. J Hematol Oncol. (2018) 11:31. doi: 10.1186/s13045-018-0578-4
43. Luo Y, Yang YC, Shen CK, Ma B, Xu WB, Wang QF, et al. Immune checkpoint protein expression defines the prognosis of advanced thyroid carcinoma. Front Endocrinol. (2022) 13:859013. doi: 10.3389/fendo.2022.859013
44. Capdevila J, Wirth LJ, Ernst T, Ponce Aix S, Lin CC, Ramlau R, et al. PD-1 blockade in anaplastic thyroid carcinoma. J Clin Oncol Off J Am Soc Clin Oncol. (2020) 38:2620–7. doi: 10.1200/JCO.19.02727
45. Hatashima A, Archambeau B, Armbruster H, Xu M, Shah M, Konda B, et al. An evaluation of clinical efficacy of immune checkpoint inhibitors for patients with anaplastic thyroid carcinoma. Thyroid Off J Am Thyroid Assoc. (2022) 32:926–36. doi: 10.1089/thy.2022.0073
46. Lee NY, Riaz N, Wu V, Brinkman T, Tsai CJ, Zhi W, et al. A pilot study of durvalumab (MEDI4736) with tremelimumab in combination with image-guided stereotactic body radiotherapy in the treatment of metastatic anaplastic thyroid cancer. Thyroid Off J Am Thyroid Assoc. (2022) 32:799–806. doi: 10.1089/thy.2022.0050
47. Sukari A, Kukreja G, Nagasaka M, Shukairy MK, Yoo G, Lin HS, et al. The role of immune checkpoint inhibitors in anaplastic thyroid cancer (Case Series). Oral Oncol. (2020) 109:104744. doi: 10.1016/j.oraloncology.2020.104744
48. Shih SR, Chen KH, Lin KY, Yang PC, Chen KY, Wang CW, et al. Immunotherapy in anaplastic thyroid cancer: Case series. J Formosan Med Assoc = Taiwan yi zhi. (2022) 121:1167–73. doi: 10.1016/j.jfma.2022.01.003
49. Dierks C, Seufert J, Aumann K, Ruf J, Klein C, Kiefer S, et al. Combination of lenvatinib and pembrolizumab is an effective treatment option for anaplastic and poorly differentiated thyroid carcinoma. Thyroid Off J Am Thyroid Assoc. (2021) 31:1076–85. doi: 10.1089/thy.2020.0322
50. Gui L, Liu S, Zhang Y, Shi Y. A remarkable and durable response to sintilimab and anlotinib in the first-line treatment of an anaplastic thyroid carcinoma without targetable genomic alterations: A case report. OncoTargets Ther. (2021) 14:2741–6. doi: 10.2147/OTT.S305196
51. Yang S, Ji D, Xue F, Chen T, Wang Y, Ji Q. Neoadjuvant famitinib and camrelizumab, a new combined therapy allowing surgical resection of the primary site for anaplastic thyroid carcinoma. Cancer Rep (Hoboken NJ). (2023) 6:e1770. doi: 10.1002/cnr2.1770
52. Chintakuntlawar AV, Yin J, Foote RL, Kasperbauer JL, Rivera M, Asmus E, et al. A phase 2 study of pembrolizumab combined with chemoradiotherapy as initial treatment for anaplastic thyroid cancer. Thyroid Off J Am Thyroid Assoc. (2019) 29:1615–22. doi: 10.1089/thy.2019.0086
53. Spalart V, Legius B, Segers K, Coolen J, Maes B, Decoster L. Dramatic response to first line single agent pembrolizumab in anaplastic thyroid carcinoma. Case Rep Endocrinol. (2019) 2019:9095753. doi: 10.1155/2019/9095753
54. Hendry S, Salgado R, Gevaert T, Russell PA, John T, Thapa B, et al. Assessing tumor-infiltrating lymphocytes in solid tumors: A practical review for pathologists and proposal for a standardized method from the international immuno-oncology biomarkers working group: part 2: TILs in melanoma, gastrointestinal tract carcinomas, non-small cell lung carcinoma and mesothelioma, endometrial and ovarian carcinomas, squamous cell carcinoma of the head and neck, genitourinary carcinomas, and primary brain tumors. Adv anatomic Pathol. (2017) 24:311–35. doi: 10.1097/PAP.0000000000000161
55. Bear AS, Fraietta JA, Narayan VK, O'Hara M, Haas NB. Adoptive cellular therapy for solid tumors. American Society of Clinical Oncology educational book. American Society of Clinical Oncology. Annual Meeting (2021) 41:57–65. doi: 10.1200/EDBK_321115
56. Shafer P, Kelly LM, Hoyos V. Cancer therapy with TCR-engineered T cells: current strategies, challenges, and prospects. Front Immunol. (2022) 13:835762. doi: 10.3389/fimmu.2022.835762
57. Haslauer T, Greil R, Zaborsky N, Geisberger R. CAR T-cell therapy in hematological Malignancies. Int J Mol Sci. (2021) 22:8996. doi: 10.3390/ijms22168996
58. Martinez M, Moon EK. CAR T cells for solid tumors: new strategies for finding, infiltrating, and surviving in the tumor microenvironment. Front Immunol. (2019) 10:128. doi: 10.3389/fimmu.2019.00128
59. Zhu L, Li XJ, Kalimuthu S, Gangadaran P, Lee HW, Oh JM, et al. Natural killer cell (NK-92MI)-based therapy for pulmonary metastasis of anaplastic thyroid cancer in a nude mouse model. Front Immunol. (2017) 8:816. doi: 10.3389/fimmu.2017.00816
60. Pillai M, Jiang Y, Lorigan PC, Thistlethwaite FC, Thomas M, Kirillova N, et al. Clinical feasibility and treatment outcomes with nonselected autologous tumor-infiltrating lymphocyte therapy in patients with advanced cutaneous melanoma. Am J Cancer Res. (2022) 12:3967–84.
61. Parkhurst MR, Yang JC, Langan RC, Dudley ME, Nathan DA, Feldman SA, et al. T cells targeting carcinoembryonic antigen can mediate regression of metastatic colorectal cancer but induce severe transient colitis. Mol Ther J Am Soc Gene Ther. (2011) 19:620–6. doi: 10.1038/mt.2010.272
62. Thompson JA, Schneider BJ, Brahmer J, Achufusi A, Armand P, Berkenstock MK, et al. Management of immunotherapy-related toxicities, version 1.2022, NCCN clinical practice guidelines in oncology. J Natl Compr Cancer Network JNCCN. (2022) 20:387–405. doi: 10.6004/jnccn.2022.0020
63. Huang R, Wen Q, Zhang X. CAR-NK cell therapy for hematological Malignancies: recent updates from ASH 2022. J Hematol Oncol. (2023) 16:35. doi: 10.1186/s13045-023-01435-3
64. Shin E, Koo JS. Cell component and function of tumor microenvironment in thyroid cancer. Int J Mol Sci. (2022) 23:12578. doi: 10.3390/ijms232012578
65. Alamino VA, Montesinos MM, Rabinovich GA, Pellizas CG. The thyroid hormone triiodothyronine reinvigorates dendritic cells and potentiates anti-tumor immunity. Oncoimmunology. (2016) 5:e1064579. doi: 10.1080/2162402X.2015.1064579
66. Stift A, Sachet M, Yagubian R, Bittermann C, Dubsky P, Brostjan C, et al. Dendritic cell vaccination in medullary thyroid carcinoma. Clin Cancer Res an Off J Am Assoc Cancer Res. (2004) 10:2944–53. doi: 10.1158/1078-0432.CCR-03-0698
67. Tian Y, Xie D, Yang L. Engineering strategies to enhance oncolytic viruses in cancer immunotherapy. Signal Transduct Target Ther. (2022) 7:117. doi: 10.1038/s41392-022-00951-x
68. Jiang K, Song C, Kong L, Hu L, Lin G, Ye T, et al. Recombinant oncolytic Newcastle disease virus displays antitumor activities in anaplastic thyroid cancer cells. BMC Cancer. (2018) 18:746. doi: 10.1186/s12885-018-4522-3
69. Passaro C, Borriello F, Vastolo V, Di Somma S, Scamardella E, Gigantino V, et al. The oncolytic virus dl922-947 reduces IL-8/CXCL8 and MCP-1/CCL2 expression and impairs angiogenesis and macrophage infiltration in anaplastic thyroid carcinoma. Oncotarget. (2016) 7:1500–15. doi: 10.18632/oncotarget.v7i2
70. Cui C, Wang X, Lian B, Ji Q, Zhou L, Chi Z, et al. OrienX010, an oncolytic virus, in patients with unresectable stage IIIC-IV melanoma: a phase Ib study. J Immunother Cancer. (2022) 10:e004307. doi: 10.1136/jitc-2021-004307
71. Wang S, Xie K, Liu T. Cancer immunotherapies: from efficacy to resistance mechanisms - not only checkpoint matters. Front Immunol. (2021) 12:690112. doi: 10.3389/fimmu.2021.690112
72. Wang S, Sun J, Chen K, Ma P, Lei Q, Xing S, et al. Perspectives of tumor-infiltrating lymphocyte treatment in solid tumors. BMC Med. (2021) 19:140. doi: 10.1186/s12916-021-02006-4
73. Szeto C, Lobos CA, Nguyen AT, Gras S. TCR recognition of peptide-MHC-I: rule makers and breakers. Int J Mol Sci. (2020) 22:68. doi: 10.3390/ijms22010068
74. Jung IY, Narayan V, McDonald S, Rech AJ, Bartoszek R, Hong G, et al. BLIMP1 and NR4A3 transcription factors reciprocally regulate antitumor CAR T cell stemness and exhaustion. Sci Trans Med. (2022) 14:eabn7336. doi: 10.1126/scitranslmed.abn7336
75. Wang W, Jiang J, Wu C. CAR-NK for tumor immunotherapy: Clinical transformation and future prospects. Cancer Lett. (2020) 472:175–80. doi: 10.1016/j.canlet.2019.11.033
76. Li L, Goedegebuure SP, Gillanders WE. Preclinical and clinical development of neoantigen vaccines. Ann Oncol Off J Eur Soc Med Oncol. (2017) 28:xii11–xii7. doi: 10.1093/annonc/mdx681
77. Raja J, Ludwig JM, Gettinger SN, Schalper KA, Kim HS. Oncolytic virus immunotherapy: future prospects for oncology. J Immunother Cancer. (2018) 6:140. doi: 10.1186/s40425-018-0458-z
78. Shalhout SZ, Miller DM, Emerick KS, Kaufman HL. Therapy with oncolytic viruses: progress and challenges. Nat Rev Clin Oncol. (2023) 20:160–77. doi: 10.1038/s41571-022-00719-w
79. Crespo-Rodriguez E, Bergerhoff K, Bozhanova G, Foo S, Patin EC, Whittock H, et al. Combining BRAF inhibition with oncolytic herpes simplex virus enhances the immune-mediated antitumor therapy of BRAF-mutant thyroid cancer. J Immunother Cancer. (2020) 8:e000698. doi: 10.1136/jitc-2020-000698
80. Li B, Jin J, Guo D, Tao Z, Hu X. Immune checkpoint inhibitors combined with targeted therapy: the recent advances and future potentials. Cancers. (2023) 15:2858. doi: 10.3390/cancers15102858
81. Bayat Mokhtari R, Homayouni TS, Baluch N, Morgatskaya E, Kumar S, Das B, et al. Combination therapy in combating cancer. Oncotarget. (2017) 8:38022–43. doi: 10.18632/oncotarget.v8i23
82. Chen Y, Yu Z, Tan X, Jiang H, Xu Z, Fang Y, et al. CAR-macrophage: A new immunotherapy candidate against solid tumors. Biomed Pharmacother = Biomedecine pharmacotherapie. (2021) 139:111605. doi: 10.1016/j.biopha.2021.111605
83. Klichinsky M, Ruella M, Shestova O, Lu XM, Best A, Zeeman M, et al. Human chimeric antigen receptor macrophages for cancer immunotherapy. Nat Biotechnol. (2020) 38:947–53. doi: 10.1038/s41587-020-0462-y
Keywords: anaplastic thyroid cancer, immunotherapy, tumor microenvironment, immune checkpoint inhibitors, cellular immunotherapy
Citation: Chen J, Xiao Z and Wu H (2024) Research progress of immunotherapy against anaplastic thyroid cancer. Front. Oncol. 14:1365055. doi: 10.3389/fonc.2024.1365055
Received: 03 January 2024; Accepted: 13 March 2024;
Published: 26 March 2024.
Edited by:
Laura Senovilla, Spanish National Research Council (CSIC), SpainReviewed by:
Yuan Cao, 960th Hospital of the PLA, ChinaDmitry Aleksandrovich Zinovkin, Gomel State Medical University, Belarus
Copyright © 2024 Chen, Xiao and Wu. This is an open-access article distributed under the terms of the Creative Commons Attribution License (CC BY). The use, distribution or reproduction in other forums is permitted, provided the original author(s) and the copyright owner(s) are credited and that the original publication in this journal is cited, in accordance with accepted academic practice. No use, distribution or reproduction is permitted which does not comply with these terms.
*Correspondence: Hongyan Wu, anpzbmZtemt6eEAxNjMuY29t
†These authors have contributed equally to this work