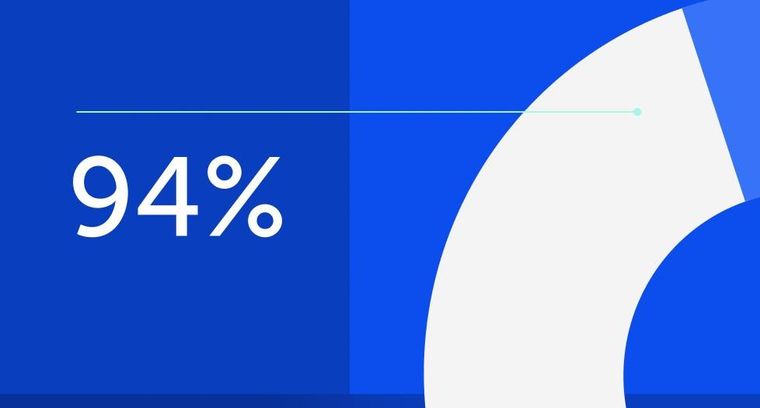
94% of researchers rate our articles as excellent or good
Learn more about the work of our research integrity team to safeguard the quality of each article we publish.
Find out more
REVIEW article
Front. Oncol., 06 March 2024
Sec. Cancer Immunity and Immunotherapy
Volume 14 - 2024 | https://doi.org/10.3389/fonc.2024.1334915
The dry root of the soybean plant Astragalus membranaceus (Fisch) Bge. var. mongholicus (Bge) Hsiao or A. membranaceus (Fisch) Bge, Astragali Radix (AR) has a long medicinal history. Astragalus polysaccharide (APS), the natural macromolecule that exhibits immune regulatory, anti-inflammatory, anti-tumor, and other pharmacological activities, is an important active ingredient extracted from AR. Recently, APS has been increasingly used in cancer therapy owing to its anti-tumor ability as it prevents the progression of prostate, liver, cervical, ovarian, and non-small-cell lung cancer by suppressing tumor cell growth and invasion and enhancing apoptosis. In addition, APS enhances the sensitivity of tumors to antineoplastic agents and improves the body’s immunity. This macromolecule has prospects for broad application in tumor therapy through various pathways. In this article, we present the latest progress in the research on the anti-tumor effects of APS and its underlying mechanisms, aiming to provide novel theoretical support and reference for its use in cancer therapy.
According to the 2020 Global Cancer Statistical Report, cancer is the second leading cause of death globally, with an increase in incidence in recent years (1, 2). Malignant tumors are the primary reason for chronic noninfectious disease-related deaths and are the chief obstacle to a better life expectancy (3). Both gene mutation and epigenetic changes are important factors that lead to cancer occurrence and progression (4). Epigenetics refers to the reversible and heritable changes in gene function without changes in the nuclear DNA. Epigenetic changes include histone modification, DNA methylation, chromatin remodeling, and non-coding RNA regulation, which can affect the expression and silencing of oncogenes and tumor suppressor genes, respectively (4–7). Therefore, epigenetic regulation offers a promising direction and strategy for the development of anti-tumor therapeutics. However, as the pathogenesis of cancer remains unclear, the development of effective treatment methods is limited. Currently, cancer treatment primarily involves surgical resection, radiotherapy, chemotherapy, or a combination of two or all three approaches. During treatment, both cancerous and normal cells are killed, triggering a series of adverse reactions and toxic side effects with varying degrees of impact on prognosis and patients’ quality of life (QOL). Recently, with advancements in tumor microenvironment research, immunotherapy has emerged as a promising therapeutic approach (8, 9). Tumor immunotherapies, such as the use of immune checkpoint inhibitors (targeting programmed cell death protein-1, programmed cell death ligand-1, cytotoxic T lymphocyte-associated antigen 4, etc.) and adoptive cell transfer (such as chimeric antigen receptor T-cell therapy, T cell receptor-engineered T cells therapy, tumor-infiltrating lymphocytes therapy, etc.) (10–13), have demonstrated promising outcomes; however, their efficacy must be validated using clinical trial.
Traditional Chinese medicine has been essential in tumor prevention and management, with significant advantages, particularly in improving and managing clinical symptoms, enhancing the efficacy of anti-cancer drugs, reducing the toxicity of radio- and chemotherapies, enhancing the patient’s immunity and QOL, and effectively prolonging survival time (14), making it a critical strategy for tumor prevention and management. Astragali Radix (AR), a common traditional Chinese herbal medicine used in China for over 2,000 years, is also extensively used in numerous countries and has been included in the pharmacopeias of the United States, Japan, and South Korea (15). Medical research shows that AR exhibits various therapeutic activities, including anti-tumor (16–19), anti-aging (20), immune regulatory (21), anti-fibrosis (22), antibacterial (23), antiviral (24, 25), and anti-radiation (26) effects. Astragalus polysaccharide (APS), a major active component of AR (27–30), is extensively used in medicine as it demonstrates beneficial activities and low toxicity (31).
APS is a mixture of macromolecules, mainly composed of glucans (both water-soluble and water-insoluble) and heteropolysaccharides (mostly water-soluble and acidic), with molecular weights ranging from 8.7×103 to 4.8×106 Da (31–34). According to recent pharmacological studies, APS has a wide spectrum of biological activities, such as anti-inflammatory (35–37), immune regulation (38, 39), anti-fiber (40), anti-radiation (41, 42), anti-aging (21), anti-metabolic disorders (43), protective effects on the cardiovascular system (44, 45), anti-diabetes (46), anti-tumor (47, 48), and anti-infection (49).
Owing to its high efficacy and low toxicity, the role and value of APS in the management of malignancies have garnered increasing research attention in recent years. The anti-cancer mechanism of APS involves inducing the apoptosis of tumor cells by regulating various pathways; inhibiting proliferation, migration, and invasion of tumor cells; regulating immune function and autophagy; and enhancing the efficacy of chemotherapeutic or targeted drugs by reducing their toxicity. However, a comprehensive understanding of how APS works has not been put into perspective. In this study, the anti-tumor mechanisms of APS and its targets are comprehensively reviewed to render a new theoretical basis for cancer treatment.
Apoptosis, also referred to as programmed cell death, plays a key role in maintaining internal environment homeostasis (50, 51). Induction of cell death is a major mechanism underlying the activity of anti-tumor drugs (52).
The Bcl-2 family contains a class of molecules involved in apoptosis-associated pathway modulation. Bcl-2 is an anti-apoptosis gene highly expressed in various tumors (53, 54), whereas Bcl-2-associated X (Bax) is a pro-apoptotic gene (55). Huang et al. proposed that APS induces H22 (a hepatocellular cancer [HCC] cell line) apoptosis by downregulating Bcl-2 and upregulating Bax expression (56). Similarly, Lv et al. reported that after APS treatment, the apoptosis of HepG2 cells is accelerated (57). Specifically, APS decreased the levels of Bcl-2, β-catenin, c-myc, and Cyclin D1 in cells, suggesting that the mechanism of tumor suppression may be related to the inhibition of Bcl-2 expression by downregulating the Wnt/β-catenin signaling pathway. Xie et al. provided additional data demonstrating that APS effectively inhibited the growth of MDA-MB-231 (a human breast cancer [BC] cell line) graft tumor (58). In terms of mechanism, APS concentration-dependently increased Bax protein expression and decreased Bcl-2 protein expression, thus inducing MDA-MB-231 apoptosis.
miRNAs, endogenous non-coding small RNAs that are critical in regulating almost all signaling pathways in eukaryotic cells (59), have recently been found to participate in tumor occurrence and progression by regulating apoptotic signaling pathways (60–63). miR-27a is over-expressed in various tumor cells (64–66). Guo et al. found that APS significantly and dose-dependently reduced miR-27a levels in cells, subsequently upregulating the expression of the tumor suppressor gene FBXW7, thereby inhibiting OV-90 and SKOV-3 proliferation and significantly increasing apoptosis (67). miR-133a, which inhibits cancer cell growth in multiple tumors, is considered a tumor suppressor molecule (68–70). According to Chu et al., after APS treatment, the apoptosis rate of human osteosarcoma MG63 cells increased owing to the upregulation of miR-133a and inactivation of the JNK signaling pathways (71).
Extrinsic apoptosis, one of the major pathways of apoptosis, is mediated by death receptors on the cell surface (72). The binding of Fas ligands to Fas receptors or tumor necrosis factor (TNF) receptors to TNF ligands is the primary way of initiating this apoptotic pathway (73–75). Fas and its ligands, a class of important apoptosis-inducing molecules, exist in various tumor cells (76, 77). In an in vitro study of colon cancer CD133+/CD44+ cells, Li and Shen found that APS can induce apoptosis by activating the Fas death receptor pathway. Specifically, APS increases Fas expression and induces apoptosis in a concentration-dependent manner (78).
The p53 protein is a vital tumor suppressor, and its loss of function is a prerequisite for cancer development (79). In various cancers, p53 is a dominant force promoting apoptosis, cell cycle arrest, and DNA repair (80–83). Zhang et al. showed that APS could activate p53 and p21 and inhibit the expression of Notch1 and Notch3 in vitro, ultimately inhibiting cell proliferation and promoting their apoptosis (84) (Table 1).
Tumors promote abnormal cell proliferation and metabolic activity by disrupting the regulation of growth-promoting signals (85); therefore, suppressing tumor cell proliferation is a vital strategy in the treatment of tumors.
Cell cycle regulation is coordinated by a complex network of interactions between enzymes, cytokines, and cell cycle signaling pathways. This regulation is essential for cell proliferation, growth, and repair (86). Abnormal regulation of the cell proliferation cycle is a major cause of tumor initiation (87). In an in vitro experiment, by inhibiting the JAK2/STAT3 pathway, Liu et al. found that APS induced the cell cycle of bladder cancer UM-UC-3 to stop in the G0/G1 phase, thus inhibiting its proliferation (88). Additionally, Yu et al. provided new evidence demonstrating that APS promotes mouse solid tumor S180 cell apoptosis in a dose-dependent manner through S-phase arrest (89). An in vitro study of APS against the proliferation of HCC cells established that APS increased the G1 phase arrest of human hepatoma HepG2 cells, enhanced their autophagic activity, suppressed proliferation, and enhanced apoptosis by inhibiting the AKT axis (90). Yan et al. showed that APS could inhibit the proliferation of colon cancer SW620 cells through cell cycle arrest, with G2/M phase arrest playing a dominant role (91).
Abnormal activation of cell signal transduction pathways is closely related to the occurrence and development of malignant tumors (92, 93). In an in vitro experiment, APS was found to inhibit RT4 and T24 proliferation and migration by inducing the accumulation of Fe2+ and malondialdehyde in cells, and ferrostatin, an iron ptosis inhibitor, reversed this reaction (94). Furthermore, APS was found to reduce GPX4 expression, inhibit the activity of the light chain subunit SLC7A11 (xCT), and promote the formation of BECN1-xCT complex by activating AMPK/BECN1 signaling. These findings demonstrated the ability of APS to inhibit urothelial carcinoma progression by inducing iron ptosis. According to Guo et al., APS attenuated the proliferative and invading capacities of prostate cancer cells (PC3 and DU145) in vitro and inhibited PC3 xenograft growth in vivo, time- and dose-dependently (95). Moreover, APS significantly inhibited tumor development by upregulating miR-138-5p expression and inhibiting SIRT1 and SREBP1 expression. Furthermore, APS significantly suppresses HeLa cell growth, invasion, and migration (96). This may be achieved by increasing SHP2 and SOCS3 protein levels in cells and inhibiting JAK-STAT pathway overactivation. Wu et al. demonstrated that APS could control the proliferation of lung cancer cells (A549 and NCI-H358 cells) by inhibiting the NF-κB signaling pathway (97) (Table 2).
Tumor invasion and metastases are strongly correlated with adverse prognoses, and the invasion of tumor cells is a prerequisite for metastases. Tumor metastasis is a complicated process involving multiple stages, genes, and gene products. Inhibiting tumor metastasis can prevent tumor cell spread to other body sites, thereby mitigating tumor progression (98).
Multiple studies have linked EMT to tumor progression, invasion, and metastases (99, 100). Yang et al. found through in vitro experiments that APS significantly prevents human BC cells, MCF-7 and Mda-MB-231, from invasion and migration (101). Further research demonstrated the ability of APS to inhibit BC cell invasiveness and migration by regulating the Wnt/β-catenin axis. In addition, macrophage migration inhibitory factor (MIF), a pro-inflammatory factor that is critical in the onset and progression of intestinal, breast, and prostate carcinoma among other malignant tumors (102–104), induces EMT in cancer cells (105, 106). Liao et al. found that an injectable preparation of APS (PG2) dose-dependently inhibited the migratory and invasive activities of lung adenoma A549 cells (107). For specific performance, APS treatment led to reduced EMT markers (vimentin, AXL) and MIF levels in cells.
miRNAs contribute to the proliferation, invasion, and migration of tumor cells by regulating gene transcription (108) and play a key regulatory role in the pathological process of various human tumors. In vitro experiments utilizing A549 and NCI-H1299 by Tao et al. substantiated that APS treatment markedly attenuated the migration and invasiveness of non-small-cell lung cancer (NSCLC) cells compared to that seen with the control, and the underlying mechanism may be related to the APS-related increase in cellular miR-195-5p levels (109). Notably, the increased expression of miR-133a in cells after APS treatment effectively prevents the proliferation, migration, and invasion of prostate cancer cells (DU145) (110).
Tumor angiogenesis is the direct path of tumor cell metastasis (111). VEGF can promote tumor progression in patients with cancer by regulating angiogenesis in cancer tissues (112, 113). Recent studies have shown that the downregulation of VEGF is a positive signal during tumor therapy (114). Zhao et al. reported that APS inhibits Lewis lung cancer growth and metastasis in mice by significantly reducing VEGF and EGFR expression in cancerous tissues (115). Additionally, in vitro studies by Tang and Li demonstrated that APS inhibits the metastasis of gastric cancer cells (SGC7901) induced by vascular endothelial cells (HUVECs) (116) (Table 3).
APS is a mixture of hydrophilic macromolecules (117) that cannot easily penetrate cell membranes, with only a small portion absorbed through the intercellular space (118), limiting its clinical application. Nano-drug delivery systems are highly selective and can deliver drugs to specific sites to enhance therapeutic effects and reduce adverse reactions (119, 120). Therefore, they show great potential for the development and application of anti-tumor drug delivery to improve the therapeutic effects.
Selenium nanoparticles, by virtue of having high bioavailability, potent bioactivity, and low toxicity (121–123), exert significant inhibitory effects on various malignant tumors (124–126). Ji et al. prepared a novel functionalized nanocomposite using alcohol-soluble APS and selenium nanoparticles and found that it was effective in suppressing HepG2 proliferation and accelerating apoptosis by triggering S-phase arrest, thereby stimulating ΔΨm (mitochondrial membrane potential) depletion, increasing the Bax/Bcl-2 ratio, and promoting intracellular reactive oxygen species accumulation (127). Jiao et al. developed selenium nanoparticles modified with macromolecular weight APS and observed positive results in hepatoma treatment, as indicated by the induction of apoptosis and inhibition of proliferation of HepG2 cells (128). The mechanism involved may be related to the increasing S-phase block, the significant enhancement of Bax levels, and the marked reduction of Bcl-2 levels and ΔΨm value. Studies have shown that the selenium nanoparticles modified by APS are cytotoxic to MCF-7 cells. This cytotoxicity is achieved by the induction of apoptosis through the mitochondrial pathway and the activation of autophagy at an early stage and inhibiting it at a late stage (129). Moreover, Huang et al. successfully constructed APS superparamagnetic iron-oxide nanocomposites and demonstrated that they could effectively induce M1 polarization of mouse monocytic macrophage RAW264.7 and improve the killing ability of macrophages against HepG2 cells in vitro. Furthermore, no inhibitory effect on macrophage proliferation was observed (130).
Resistance to chemotherapy is the primary reason for treatment failure and poor prognoses. Therefore, focusing on the mechanism of drug resistance and inhibiting it in tumor cells is essential to reducing drug resistance and optimizing effectiveness while improving patient survival rates.
CDDP is widely used as an initial medication in cancer therapy owing to its exceptional ability to combat cancer and its broad spectrum of effectiveness against various cancer types. However, the prolonged utilization of this drug may result in the development of resistance, thereby restricting its practical implementation in clinical settings. The PI3K/AKT axis is crucial for tumorigenesis and progression (131). As indicated by Gong et al., APS reversed the acquired CDDP resistance in melanoma cell lines B16 in vivo by inhibiting the PD-L1/PI3K/AKT axis (132). Liu and Chen showed that APS was able to overcome the resistance of A549/CDDP cells to CDDP in vitro (133). Subsequent investigations revealed that APS can decrease the ΔΨm values and Bcl-2, p-PI3K, P-gp, and p-AKT levels while elevating Bax expression. This finding implies that the potential mechanism of action could encompass the inhibition of the PI3K/AKT pathway and stimulation of the mitochondrial apoptosis pathway. Lu et al. further demonstrated that APS combined with CDDP effectively inhibits proliferation, migration, and EMT progression of CDDP-resistant SW620 cells by inhibiting miR-10b-5p expression and upregulating AGPAT3 expression (134). The combination of APS and CDDP synergistically inhibits the invasion and metastasis of CNE-1 (a human nasopharyngeal carcinoma cell line). It is associated with the induction of G0/G1 and S phase arrest, downregulation of MMP-9 expression, and upregulation of p53 expression (135). Li et al. observed that APS could enhance the sensitivity of SKOV3 ovarian cancer cells to CDDP treatment by activating the mitochondrial apoptosis pathway and JNK1/2 signaling pathway (136).
In addition to CDDP, the application of APS can potentially augment the chemosensitivity of tumors to other medications. Li et al. showed that APS enhanced the sensitivity of HCC cells to doxorubicin chemotherapy and induced cancer cell apoptosis (137). In vitro tests have shown that by downregulating OGT (O-GlcNAc transferase) and upregulating OGA (O-GlcNAc transferase) expression in Hep3B cells, APS reduced O-GlcNAcylation and intensified endoplasmic reticulum stress responses. According to in vivo experiments, APS combined with doxorubicin inhibited xenograft tumor growth in mice, suggesting that APS can potentially be an optional sensitizer in HCC chemotherapy. A previous study demonstrated that APS effectively reversed the resistance of lung adenocarcinoma (PC9 and HCC827) cells to gefitinib by inhibiting the PD-L1/SREBP-1/EMT axis (138). In a mouse BC model, Bao et al. (139) found that APS effectively alleviated paclitaxel-induced cytotoxicity in mouse monocyte–macrophage RAW264.7 cells. The mechanism for this action is attributable to changes in the cell cycle and apoptosis. When studying the role of APS plus apatinib in human pancreatic cancer cell (ASPC-1 and PANC-1) proliferation and apoptosis, Wu et al. found that the combination therapy contributed to higher cell migration and invasion inhibition rates and apoptosis and lowered p-AKT, MMP-9, and p-ERK levels than those in the control group (140), suggesting that APS plus apatinib is a promising strategy for treating pancreatic cancer (Table 4).
The body’s immune system, through various pathways, identifies and removes mutated tumor cells under normal circumstances, which inhibits tumor cell growth to some extent. Recent evidence has indicated that immune suppression in the microenvironment before tumor metastasis is a key link in the initiation of tumor metastasis (141). Owing to its strong immunomodulating properties (142), APS can enhance the body’s immunity to treat various cancers.
Bamodu et al. reported that APS can downregulate the expression of interleukin (IL)-6/10, markedly increase the M1/M2 macrophage polarization ratio, contribute to the functional maturity of DC, enhance T cell-medicated anti-tumoral immune responses, improve the accuracy of tumor cell killing, and inhibit the growth of tumor cells (143). Wei et al. reported that, through the Notch signaling pathway, APS significantly promoted the production of cytokines such as IL-6 and TNF-α; increased the iNOS levels and polarization rate of M1/M2 macrophages; activated M1 macrophages; and inhibited M2 macrophages, thereby enhancing the killing and phagocytosis of tumor 4T1 cells and the inhibition of tumor growth and metastasis (144). Li et al. observed that the presence of APS can elevate the percentage of M1 macrophages within liver cancer tissues while simultaneously reducing the proportion of M2 macrophages, thereby inhibiting the growth of liver cancer tumor cells (145). Furthermore, APS activates the release of NO and TNF-α by macrophages, thus reinforcing the suppressive and killing impact of the immune system on MCF-7 BC cells (146).
Ding et al. showed that APS inhibits tumor growth in melanoma-bearing mice (147). Specifically, by regulating the composition of the intestinal flora and altering fecal metabolites, APS reduces the MDSC (Myeloid-derived suppressor cell) count, downregulates IL-10, arginase-1, and TGF-β expression, and decreases the immunosuppressive activity of MDSCs in mice with melanoma, thereby enhancing the killing ability of CD8+ T cells on tumors. Yu et al. prepared a novel APS using water at 4°C (148) and demonstrated that it activated anti-tumoral immune responses and enhanced anaerobic metabolism in the solid tumor microenvironment through the HIF-1 axis, ultimately promoting mouse S180 (a cancer cell line) apoptosis (89). He et al. injected APS into HCC BALB/c mice (100, 200, and 400 mg/kg per day for 12 consecutive days) and observed increased CD8+ T cell count, decreased PD-L1 levels, and reduced tumor size, weight, and volume. Furthermore, by upregulating miR-133a-3p and downregulating MSN, APS attenuated PD-L1-mediated immunosuppression, thereby suppressing tumors (149). Chang et al. found that APS downregulates PD-L1 protein levels by inhibiting the AKT/mToR/p70S6K axis, thereby enhancing the immune capacity of 4T1 (mouse BC) and CT26 (mouse colorectal cancer) cells (150) (Table 5).
Chang et al. revealed that APS enhances immune responses in 4T1 and CT26 tumor-bearing mice by downregulating PD-L1 protein levels by inhibiting the AKT/mTOR/p70S6K axis (140).
Lipid metabolism is a major pathway of cellular energy metabolism. Abnormal lipid metabolism can promote tumor progression (151, 152), which is an indicator of human cancer (153).
Lipid metabolism is a complex regulatory process that provides energy, lipid chains, and a large amount of fats required for the formation of new cell membranes for rapidly dividing and proliferating cancer cells (154). Abnormal lipid metabolism is often accompanied by the anomalous overexpression of related enzymes (155), abnormal transcription of related non-coding RNA (156), and activation of carcinogenic signaling pathways (157). Cholesterol and its metabolites are signaling molecules that promote tumor development (158). Triglycerides are closely related to the growth of various tumor cells (159, 160). APS effectively lowers cholesterol and triglycerides (161, 162).
As indicated by a recent study, APS inhibits prostate cancer growth and lipid metabolism in vivo and in vitro (95). By upregulating miR-138-5p, APS significantly suppressed SIRT1 and SREBP1 expression, decreased cholesterol and triglyceride levels in PC3 and DU145, and attenuated cell proliferation. Therefore, the role played by APS in mediating lipid metabolism is important for the prevention of cancer progression.
Autophagy refers to the biological process by which cells undergo intracellular degradation via lysosomes to protect cell integrity and maintain homeostasis under the influence of external environmental stimuli and metabolic pressures (163, 164). It is strongly linked to carcinogenesis and progression of various cancers and plays a dual role in the tumor process (165–167).
Zhi et al. found that after APS treatment, the expression of LC3B-II/I was significantly increased in colorectal cancer HCT-116 cells, while the expression levels of p-PI3K/PI3K, p-AKT/AKT, p-mTOR/mTOR, and p62 were significantly decreased (168). Therefore, they proposed that APS can induce autophagy in colorectal cancer cells by inhibiting the PI3K/AKT/mTOR axis and the development of cancer cells. The proportions of Beclin1 and LC3B in EC109 esophageal cancer cells increased significantly after APS treatment (169). Based on these findings, Chang et al. believed that the anti-tumor mechanism of APS was related to the enhancement of autophagy induced by APS. Li and Shen found that APS elevated caspase-9, caspase-3, and Bax protein levels, decreased Bcl-2 protein expression, and inhibited CD133 and CD44 co-positive colon cancer stem cell proliferation time- and concentration-dependently (78). Moreover, APS concentration-dependently induced apoptosis and this effect was reversed by an autophagy antagonist. These results suggest that APS can inhibit proliferation and promote apoptosis by inducing autophagy in colon cancer stem cells. However, given the dual role of autophagy in tumorigenesis and cancer progression, its exact mechanism in cancers requires further exploration.
The elucidation of the efficacy, safety, and tolerability of drugs administered to patients is the main focus of a clinical trial.
PG2 has recently been found to normalize the neutrophils-to-lymphocytes ratio in patients with lung cancer on combined immune checkpoint inhibitor therapy, suggesting that APS could be used to supplement anti-tumor agents (170). A trial of PG2 plus cytokine-induced killer cells for advanced NSCLC demonstrated that PG2 notably increased the proportion of peripheral blood CD4+ and CD3+ T lymphocytes (171), thus significantly improving patients’ functional status and relieving symptoms of qi deficiency, with remarkable clinical safety. A clinical study investigating PG2 plus gefitinib therapy for advanced lung cancer showed markedly higher serum CD3+, CD4+, and CD4+/CD8+ cell counts in the observation group than in the control group after treatment, accompanied by a better QOL (higher KPS scores) and fewer toxic and side effects (172). This study demonstrated that PG2 combined with gefitinib for advanced NSCLC can improve patients’ immunity, alleviate toxicity and side effects, and improve patients’ overall QOL. Zheng et al. showed that PG2 has a protective effect on the bone marrow, which can reduce the myelotoxicity of platinum-containing drugs combined with other drugs in patients with NSCLC and improve patient tolerance to chemotherapy (173).
Concurrent radiotherapy and chemotherapy are standard therapies for patients with advanced head and neck squamous cell carcinoma; however, the resulting complications can affect patient QOL. Moreover, its efficacy is low. Relapse and metastases are found in half of the head and neck squamous cell carcinoma cases, leading to a 5-year survival rate lesser than 40% (174). Hsieh et al. showed that although compared to the placebo group, patients administered PG2 did not show any difference in their tumor response rate, disease-specific survival rate, or overall survival rate, their QOL was significantly improved as indicated by the reduction in pain and improvement in appetite (175) (Table 6).
Although these results are encouraging, owing to the limited number of patients and the influence of other potential factors, more comprehensive research is needed to ensure the optimal use of APS and its clinical safety profile in cancer therapy.
The rising morbidity and mortality due to cancer in recent years have posed a serious threat to human health; however, its pathogenesis has not been thoroughly understood. The effectiveness of existing therapies is limited, and complete tumor treatment is difficult. Radiotherapy and chemotherapy not only cause pain and reduce the QOL but also increase the economic burden on patients. Currently, effective agents with low toxicity and few side effects need to be explored for treatment or adjuvant therapy.
As a natural product, APS has high medicinal value. It has multi-pathway, multi-target, and multi-level anti-cancer mechanisms. These include inducing apoptosis; regulating tumor cell autophagy; inhibiting tumor cell proliferation, invasion, and metastasis; tumor-related inflammatory microenvironment; and synergism with anticancer drugs (Figure 1). These mechanisms have broad research prospects, which warrant in-depth research.
Figure 1 Anti-tumor pathways mediated by APS. APS, Astragalus polysaccharides; SIRT1, Silent mating type information regulation 2 homolog- 1; AMPK, Adenosine monophosphate activated protein kinase; SREBP1, Recombinant Sterol Regulatory Element Binding Transcription Factor 1; BECN1,Beclin-1; xCT, Light chain subunit SLC7A11; GPX4,Glutathione peroxidase 4; JAK, Janus kinase; STAT, Signal transducer of activators of transcription; SHP2, SH2 domain-containing protein tyrosine phosphatase 2; SOCS3, Suppressor of cy-tokine signaling proteins; AKT, Protein kinase B; NF-κB, Nuclear factor kappa-B; p53, p53 protein; p21, p21 protein; Cyt-c, Cytochrome c; Bcl-2, B-cell lymphoma-2; Bax, Bcl-2-associated X; FBXW7, F-box/WD repeat-containing protein 7; JNK, c-Jun N-terminal kinases; EMT, Epithelial-mesenchymal transition; MIF, Macrophage migration inhibitor factor; VEGF, Vascular endothelial growth factor; EGFR, Epidermal growth factor receptor; p63, p63 protein; PI3K, Phosphatidylinositol-3-kinase; mTOR, Mammalian rapamycin target protein; LC3B, Microtubule-associated protein 1 light chain 3 Beta.
Despite its promising benefits, the study of APS has some limitations. First, the potential mechanisms and causality of its active ingredients in complex cell signaling pathways are not comprehensively understood, and consistent models and evaluation criteria, particularly high-quality and large-sample clinical data, are lacking. Second, the literature survey showed that the APS dose used in related studies varied significantly. Therefore, the safe and effective dose of APS in follow-up studies should be determined, and a relatively safe regimen developed. Moreover, current research on APS is primarily limited to in vitro cell experiments and rodent models, and its optimal dose and safety need to be verified. Additionally, because APS is a hydrophilic macromolecular mixture, its bioavailability is low, and the combination of APS and nanocarriers can overcome this obstacle to achieve targeted drug delivery, effectively reduce drug dosage, and improve bioavailability. However, research on APS-modified nanocarriers to improve the specific targeting of tumor tissues is lacking.
In conclusion, APS has great potential in cancer therapy, particularly as nanoparticles obtained by APS processing. To establish the effectiveness of APS in clinical treatment and its broader anti-tumor mechanism and, thus, provide a more efficacious treatment for patients with cancer, further experimental exploration and research are essential.
QY: Writing – original draft, Investigation. DM: Investigation, Writing – review & editing. QZ: Project administration, Supervision, Writing – review & editing. JW: Data curation, Supervision, Writing – review & editing.
The author(s) declare that financial support was received for the research, authorship, and/or publication of this article. Ding Yuanqing National Famous Traditional Chinese Medicine Expert Inheritance Workshop.
The authors declare that the research was conducted in the absence of any commercial or financial relationships that could be construed as a potential conflict of interest.
All claims expressed in this article are solely those of the authors and do not necessarily represent those of their affiliated organizations, or those of the publisher, the editors and the reviewers. Any product that may be evaluated in this article, or claim that may be made by its manufacturer, is not guaranteed or endorsed by the publisher.
1. Fitzmaurice C, Allen C, Barber RM, Barregard L, Bhutta ZA, Brenner H, et al. Global, regional, and national cancer incidence, mortality, years of life lost, years lived with disability, and disability-adjusted life-years for 32 cancer groups, 1990 to 2015: A systematic analysis for the global burden of disease study. JAMA Oncol. (2017) 3:524–48. doi: 10.1001/jamaoncol.2016.5688
2. Sung H, Ferlay J, Siegel RL, Laversanne M, Soerjomataram I, Jemal A, et al. Global cancer statistics 2020: globocan estimates of incidence and mortality worldwide for 36 cancers in 185 countries. CA Cancer J Clin. (2021) 71:209–49. doi: 10.3322/caac.21660
3. Siegel RL, Miller KD, Wagle NS, Jemal A. Cancer statistics, 2023. CA Cancer J Clin. (2023) 73:17–48. doi: 10.3322/caac.21763
4. Yang Q, Chen Y, Guo R, Dai Y, Tang L, Zhao Y, et al. Interaction of Ncrna and epigenetic modifications in gastric cancer: focus on histone modification. Front Oncol. (2021) 11:822745. doi: 10.3389/fonc.2021.822745
5. Cavalli G, Heard E. Advances in epigenetics link genetics to the environment and disease. Nature. (2019) 571:489–99. doi: 10.1038/s41586-019-1411-0
6. Nacev BA, Jones KB, Intlekofer AM, Yu JSE, Allis CD, Tap WD, et al. The epigenomics of sarcoma. Nat Rev Cancer. (2020) 20:608–23. doi: 10.1038/s41568-020-0288-4
7. Chen Y, Long W, Yang L, Zhao Y, Wu X, Li M, et al. Functional peptides encoded by long non-coding Rnas in gastrointestinal cancer. Front Oncol. (2021) 11:777374. doi: 10.3389/fonc.2021.777374
8. Belli C, Trapani D, Viale G, D’Amico P, Duso BA, Della Vigna P, et al. Targeting the microenvironment in solid tumors. Cancer Treat Rev. (2018) 65:22–32. doi: 10.1016/j.ctrv.2018.02.004
9. Xiang S, Li J, Shen J, Zhao Y, Wu X, Li M, et al. Identification of prognostic genes in the tumor microenvironment of hepatocellular carcinoma. Front Immunol. (2021) 12:653836. doi: 10.3389/fimmu.2021.653836
10. Zhao Q, Jiang Y, Xiang S, Kaboli PJ, Shen J, Zhao Y, et al. Engineered Tcr-T cell immunotherapy in anticancer precision medicine: pros and cons. Front Immunol. (2021) 12:658753. doi: 10.3389/fimmu.2021.658753
11. Kakimi K, Karasaki T, Matsushita H, Sugie T. Advances in personalized cancer immunotherapy. Breast Cancer. (2017) 24:16–24. doi: 10.1007/s12282-016-0688-1
12. Pawłowska A, Suszczyk D, Okła K, Barczyński B, Kotarski J, Wertel I. Immunotherapies based on Pd-1/Pd-L1 pathway inhibitors in ovarian cancer treatment. Clin Exp Immunol. (2019) 195:334–44. doi: 10.1111/cei.13255
13. Solinas C, Aiello M, De Silva P, Gu-Trantien C, Migliori E, Willard-Gallo K. Targeting Pd-1 in cancer: biological insights with a focus on breast cancer. Crit Rev Oncol Hematol. (2019) 142:35–43. doi: 10.1016/j.critrevonc.2019.07.011
14. Zhang Y, Hou W, Lin H. Clinical research results and reflections on Tcm treatment of Malignant tumors. J Tradit Chin Med. (2014) 55:523–5. doi: 10.13288/j.11-2166/r.2014.06.021
15. Su HF, Shaker S, Kuang Y, Zhang M, Ye M, Qiao X. Phytochemistry and cardiovascular protective effects of Huang-Qi (Astragali Radix). Med Res Rev. (2021) 41:1999–2038. doi: 10.1002/med.21785
16. Zhang SY, Zhang J, Sun ZY, Chen XY, Xia TH, Yang SZ, et al. Effect of astragalus injection on the proliferation of hela cells in cervical cancer. Chin J Clin Pharmacol. (2020) 36:975–8. doi: 10.13699/j.cnki.1001-6821.2020.08.011
17. Li S, Sun Y, Huang J, Wang B, Gong Y, Fang Y, et al. Anti-tumor effects and mechanisms of astragalus membranaceus (Am) and its specific immunopotentiation: status and prospect. J Ethnopharmacol. (2020) 258:112797. doi: 10.1016/j.jep.2020.112797
18. Wu Y, Zhang X, Li Z, Yan H, Qin J, Li T. Formononetin inhibits human bladder cancer cell proliferation and invasiveness via regulation of Mir-21 and Pten. Food Funct. (2017) 8:1061–6. doi: 10.1039/C6FO01535B
19. Zhang J, Liu L, Wang J, Ren B, Zhang L, Li W. Formononetin, an isoflavone from Astragalus membranaceus inhibits proliferation and metastasis of ovarian cancer cells. J Ethnopharmacol. (2018) 221:91–9. doi: 10.1016/j.jep.2018.04.014
20. Berezutsky MA, Durnova NA, Vlasova IA. [Experimental and clinical studies of mechanisms of the anti-aging effects of chemical compounds in astragalus membranaceus (Review).]. Adv Gerontol. (2019) 32:702–10.
21. Liu P, Zhao H, Luo Y. Anti-aging implications of Astragalus membranaceus (Huangqi): A well-known Chinese tonic. Aging Dis. (2017) 8:868–86. doi: 10.14336/ad.2017.0816
22. Yu M, Shi J, Sheng M, Gao K, Zhang L, Liu L, et al. Astragalus inhibits epithelial-to-mesenchymal transition of peritoneal mesothelial cells by down-regulating B-catenin. Cell Physiol Biochem. (2018) 51:2794–813. doi: 10.1159/000495972
23. Huang P, Lu X, Yuan B, Liu T, Dai L, Liu Y, et al. Astragaloside iv alleviates E. Coli-caused peritonitis via upregulation of neutrophil influx to the site of infection. Int Immunopharmacol. (2016) 39:377–82. doi: 10.1016/j.intimp.2016.08.011
24. Liang Y, Zhang Q, Zhang L, Wang R, Xu X, Hu X. Astragalus membranaceus treatment protects Raw264.7 cells from influenza virus by regulating G1 phase and the Tlr3-mediated signaling pathway. Evid Based Complement Alternat Med. (2019) 2019:2971604. doi: 10.1155/2019/2971604
25. Shang L, Qu Z, Sun L, Wang Y, Liu F, Wang S, et al. Astragaloside iv inhibits adenovirus replication and apoptosis in A549 cells in vitro. J Pharm Pharmacol. (2011) 63:688–94. doi: 10.1111/j.2042-7158.2011.01258.x
26. Wen W, Chen J, Ding L, Luo X, Zheng X, Dai Q, et al. Astragaloside exerts anti-photoaging effects in Uvb-induced premature senescence of rat dermal fibroblasts through enhanced autophagy. Arch Biochem Biophys. (2018) 657:31–40. doi: 10.1016/j.abb.2018.09.007
27. Tian QE, Li HD, Yan M, Cai HL, Tan QY, Zhang WY. Astragalus polysaccharides can regulate cytokine and P-glycoprotein expression in H22 tumor-bearing mice. World J Gastroenterol. (2012) 18:7079–86. doi: 10.3748/wjg.v18.i47.7079
28. Li Y, Guo S, Zhu Y, Yan H, Qian DW, Wang HQ, et al. Comparative analysis of twenty-five compounds in different parts of Astragalus Membranaceus var. Mongholicus and Astragalus membranaceus by Uplc-Ms/Ms. J Pharm Anal. (2019) 9:392–9. doi: 10.1016/j.jpha.2019.06.002
29. Ma XQ, Shi Q, Duan JA, Dong TT, Tsim KW. Chemical analysis of Radix astragali (Huangqi) in China: A comparison with its adulterants and seasonal variations. J Agric Food Chem. (2002) 50:4861–6. doi: 10.1021/jf0202279
30. Guo Z, Lou Y, Kong M, Luo Q, Liu Z, Wu J. A systematic review of phytochemistry, pharmacology and pharmacokinetics on Astragali radix: implications for Astragali radix as a personalized medicine. Int J Mol Sci. (2019) 20:1463. doi: 10.3390/ijms20061463
31. Fu J, Wang Z, Huang L, Zheng S, Wang D, Chen S, et al. Review of the botanical characteristics, phytochemistry, and pharmacology of Astragalus membranaceus (Huangqi). Phytother Res. (2014) 28:1275–83. doi: 10.1002/ptr.5188
32. Chang X, Chen X, Guo Y, Gong P, Pei S, Wang D, et al. Advances in chemical composition, extraction techniques, analytical methods, and biological activity of Astragali radix. Molecules. (2022) 27:1058. doi: 10.3390/molecules27031058
33. Xie JH, Jin ML, Morris GA, Zha XQ, Chen HQ, Yi Y, et al. Advances on bioactive polysaccharides from medicinal plants. Crit Rev Food Sci Nutr. (2016) 56 Suppl 1:S60–84. doi: 10.1080/10408398.2015.1069255
34. Fan XH, Li K, Yang YD, Li HF, Li XQ, Qin XM. Research progress on structural characterization of astragalus polysaccharides. J Shanxi Univ Tradit Chin Med. (2022) 23:260–7. doi: 10.19763/j.cnki.2096-7403.2022.03.22
35. Liao J, Li C, Huang J, Liu W, Chen H, Liao S, et al. Structure characterization of honey-processed astragalus polysaccharides and its anti-inflammatory activity in vitro. Molecules. (2018) 23:168. doi: 10.3390/molecules23010168
36. Dong N, Li X, Xue C, Zhang L, Wang C, Xu X, et al. Astragalus polysaccharides alleviates Lps-induced inflammation via the Nf-Kb/Mapk signaling pathway. J Cell Physiol. (2020) 235:5525–40. doi: 10.1002/jcp.29452
37. Meng Q, Du X, Wang H, Gu H, Zhan J, Zhou Z. Astragalus polysaccharides inhibits cell growth and pro-inflammatory response in Il-1β-stimulated fibroblast-like synoviocytes by enhancement of autophagy via Pi3k/Akt/Mtor inhibition. Apoptosis. (2017) 22:1138–46. doi: 10.1007/s10495-017-1387-x
38. Liu QY, Yao YM, Yu Y, Dong N, Sheng ZY. Astragalus polysaccharides attenuate postburn sepsis via inhibiting negative immunoregulation of Cd4+ Cd25(High) T cells. PloS One. (2011) 6:e19811. doi: 10.1371/journal.pone.0019811
39. Li J, Bao Y, Lam W, Li W, Lu F, Zhu X, et al. Immunoregulatory and anti-tumor effects of polysaccharopeptide and astragalus polysaccharides on tumor-bearing mice. Immunopharmacol Immunotoxicol. (2008) 30:771–82. doi: 10.1080/08923970802279183
40. Zhang R, Xu L, An X, Sui X, Lin S. Astragalus Polysaccharides Attenuate Pulmonary fibrosis by Inhibiting the Epithelial-Mesenchymal transition and Nf-Kb Pathway Activation. Int J Mol Med. (2020) 46:331–9. doi: 10.3892/ijmm.2020.4574
41. Liu Y, Liu F, Yang Y, Li D, Lv J, Ou Y, et al. Astragalus polysaccharide ameliorates ionizing radiation-induced oxidative stress in mice. Int J Biol Macromol. (2014) 68:209–14. doi: 10.1016/j.ijbiomac.2014.05.001
42. Li Q, Wang D, Bai D, Cai C, Li J, Yan C, et al. Photoprotective effect of Astragalus membranaceus polysaccharide on Uva-induced damage in Hacat cells. PloS One. (2020) 15:e0235515. doi: 10.1371/journal.pone.0235515
43. Wang Y, Chen SY, Yang ZM. Effect of astragalus polysaccharide on sweet taste receptor pathway in intestine of rat model induced by high-sugar and high-fat diet. Chin J Exp Tradit Med Form. (2019) 25:64–8. doi: 10.13422/j.cnki.syfjx.20191037
44. Chen W, Xia YP, Chen WJ, Yu MH, Li YM, Ye HY. Improvement of myocardial glycolipid metabolic disorder in diabetic hamster with astragalus polysaccharides treatment. Mol Biol Rep. (2012) 39:7609–15. doi: 10.1007/s11033-012-1595-y
45. Chen W, Li YM, Yu MH. Astragalus polysaccharides inhibited diabetic cardiomyopathy in hamsters depending on suppression of heart chymase activation. J Diabetes Complications. (2010) 24:199–208. doi: 10.1016/j.jdiacomp.2008.12.003
46. Zhang R, Qin X, Zhang T, Li Q, Zhang J, Zhao J. Astragalus polysaccharide improves insulin sensitivity via Ampk activation in 3t3-L1 adipocytes. Molecules. (2018) 23:2711. doi: 10.3390/molecules23102711
47. Lai X, Xia W, Wei J, Ding X. Therapeutic effect of astragalus polysaccharides on hepatocellular carcinoma H22-bearing mice. Dose Response. (2017) 15:1559325816685182. doi: 10.1177/1559325816685182
48. Zhao L, Tan S, Zhang H, Liu P, Tan YZ, Li JC, et al. Astragalus polysaccharides exerts anti-infective activity by inducing human cathelicidin antimicrobial peptide Il-37 in respiratory epithelial cells. Phytother Res. (2018) 32:1521–9. doi: 10.1002/ptr.6080
49. Aglietti RA, Dueber EC. Recent insights into the molecular mechanisms underlying pyroptosis and gasdermin family functions. Trends Immunol. (2017) 38:261–71. doi: 10.1016/j.it.2017.01.003
50. Chui AJ, Okondo MC, Rao SD, Gai K, Griswold AR, Johnson DC, et al. N-terminal degradation activates the Nlrp1b inflammasome. Science. (2019) 364:82–5. doi: 10.1126/science.aau1208
51. Chen XP. Status of anti-tumor research based on nonapoptotic programmed death. Chin J Pharmacol Toxicity. (2019) 33:896–7.
52. Warren CFA, Wong-Brown MW, Bowden NA. Bcl-2 family isoforms in apoptosis and cancer. Cell Death Dis. (2019) 10:177. doi: 10.1038/s41419-019-1407-6
53. Zhang L, Lu Z, Zhao X. Targeting Bcl-2 for cancer therapy. Biochim Biophys Acta Rev Cancer. (2021) 1876:188569. doi: 10.1016/j.bbcan.2021.188569
54. Fitzsimmons L, Cartlidge R, Chang C, Sejic N, Galbraith LCA, Suraweera CD, et al. Ebv Bcl-2 homologue Bhrf1 drives chemoresistance and lymphomagenesis by inhibiting multiple cellular pro-apoptotic proteins. Cell Death Differ. (2020) 27:1554–68. doi: 10.1038/s41418-019-0435-1
55. Jeng PS, Inoue-Yamauchi A, Hsieh JJ, Cheng EH. Bh3-dependent and independent activation of Bax and Bak in mitochondrial apoptosis. Curr Opin Physiol. (2018) 3:71–81. doi: 10.1016/j.cophys.2018.03.005
56. Huang WH, Liao WR, Sun RX. Astragalus polysaccharide induces the apoptosis of human hepatocellular carcinoma cells by decreasing the expression of Notch1. Int J Mol Med. (2016) 38:551–7. doi: 10.3892/ijmm.2016.2632
57. Lv J, Zhu PF, Liu YM, Zeng QL, Yu ZJ. Astragalus polysaccharides downregulates apoptosis in Hepg2 cell through Wnt/B-catenin signaling pathway. Chin Tradit Herbal Drug. (2018) 49:5155–60. doi: 10.7501/j.issn.0253-2670.2018.21.029
58. Xie RD, Sun SB, He JX, Dong JN, Long F. Effect of astragalus polysaccharide on growth and tumor-related apoptosis protein of human breast cancer Mda-Mb-231 transplanted tumor in nude mice. Chin J Exp Tradit Med Form. (2019) 25:37–43. doi: 10.13422/j.cnki.syfjx.20191622
59. Stavast CJ, Erkeland SJ. The non-canonical aspects of micrornas: many roads to gene regulation. Cells. (2019) 8:1465. doi: 10.3390/cells8111465
60. Saliminejad K, Khorram Khorshid HR, Soleymani Fard S, Ghaffari SH. An overview of micrornas: biology, functions, therapeutics, and analysis methods. J Cell Physiol. (2019) 234:5451–65. doi: 10.1002/jcp.27486
61. Guo T, Zhang Y, Qu X, Che X, Li C, Fan Y, et al. Mir-200a enhances trail-induced apoptosis in gastric cancer cells by targeting A20. Cell Biol Int. (2018) 42:506–14. doi: 10.1002/cbin.10924
62. Lv J, Guo T, Qu X, Che X, Li C, Wang S, et al. Pd-L1 under regulation of Mir-429 influences the sensitivity of gastric cancer cells to trail by binding of Egfr. Front Oncol. (2020) 10:1067. doi: 10.3389/fonc.2020.01067
63. Xu K, Han B, Bai Y, Ma XY, Ji ZN, Xiong Y, et al. Mir-451a suppressing Bap31 can inhibit proliferation and increase apoptosis through inducing er stress in colorectal cancer. Cell Death Dis. (2019) 10:152. doi: 10.1038/s41419-019-1403-x
64. Ding L, Zhang S, Xu M, Zhang R, Sui P, Yang Q. Microrna-27a contributes to the Malignant behavior of gastric cancer cells by directly targeting Ph domain and leucine-rich repeat protein phosphatase 2. J Exp Clin Cancer Res. (2017) 36:45. doi: 10.1186/s13046-017-0516-2
65. Luo W, Zhang D, Ma S, Wang C, Zhang Q, Wang H, et al. Mir-27a is highly expressed in H1650 cancer stem cells and regulates proliferation, migration, and invasion. J Cancer Res Ther. (2018) 14:S1004–s11. doi: 10.4103/0973-1482.199450
66. Maghsudlu M, Farashahi Yazd E, Amiriani T. Increased expression of Mir-27a and Mir-24-2 in esophageal squamous cell carcinoma. J Gastrointest Cancer. (2020) 51:227–33. doi: 10.1007/s12029-019-00232-x
67. Guo Y, Zhang Z, Wang Z, Liu G, Liu Y, Wang H. Astragalus polysaccharides inhibit ovarian cancer cell growth via microrna-27a/Fbxw7 signaling pathway. Biosci Rep. (2020) 40:BSR20193396. doi: 10.1042/bsr20193396
68. Moradi S, Kamal A, Aboulkheyr Es H, Farhadi F, Ebrahimi M, Chitsaz H, et al. Pan-cancer analysis of microrna expression profiles highlights micrornas enriched in normal body cells as effective suppressors of multiple tumor types: A study based on Tcga database. PloS One. (2022) 17:e0267291. doi: 10.1371/journal.pone.0267291
69. Kojima S, Chiyomaru T, Kawakami K, Yoshino H, Enokida H, Nohata N, et al. Tumour suppressors Mir-1 and Mir-133a target the oncogenic function of purine nucleoside phosphorylase (Pnp) in prostate cancer. Br J Cancer. (2012) 106:405–13. doi: 10.1038/bjc.2011.462
70. Li W, Chen A, Xiong L, Chen T, Tao F, Lu Y, et al. Mir-133a acts as a tumor suppressor in colorectal cancer by targeting Eif4a1. Tumour Biol. (2017) 39:1010428317698389. doi: 10.1177/1010428317698389
71. Chu Y, Fang Y, Chi J, Li J, Zhang D, Zou Y, et al. Astragalus Polysaccharides Decrease Proliferation, Migration, and Invasion but Increase Apoptosis of Human Osteosarcoma Cells by up-Regulation of Microrna-133a. Braz J Med Biol Res. (2018) 51:e7665. doi: 10.1590/1414-431x20187665
72. Riedl SJ, Salvesen GS. The apoptosome: signalling platform of cell death. Nat Rev Mol Cell Biol. (2007) 8:405–13. doi: 10.1038/nrm2153
73. MacKenzie SH, Clark AC. Targeting cell death in tumors by activating caspases. Curr Cancer Drug Targets. (2008) 8:98–109. doi: 10.2174/156800908783769391
74. Koren E, Fuchs Y. Modes of regulated cell death in cancer. Cancer Discovery. (2021) 11:245–65. doi: 10.1158/2159-8290.Cd-20-0789
75. Li H, Fan J, Zhao Y, Yang J, Xu H, Manthari RK, et al. Calcium alleviates fluoride-induced kidney damage via Fas/Fasl, Tnfr/Tnf, Dr5/trail pathways in rats. Ecotoxicol Environ Saf. (2021) 226:112851. doi: 10.1016/j.ecoenv.2021.112851
76. Müllauer L, Mosberger I, Grusch M, Rudas M, Chott A. Fas ligand is expressed in normal breast epithelial cells and is frequently up-regulated in breast cancer. J Pathol. (2000) 190:20–30. doi: 10.1002/(ISSN)1096-9896
77. Shimoyama M, Kanda T, Liu L, Koyama Y, Suda T, Sakai Y, et al. Expression of fas ligand is an early event in colorectal carcinogenesis. J Surg Oncol. (2001) 76:63–8; discussion 9. doi: 10.1002/1096-9098(200101)76:1<63::AID-JSO1011>3.3.CO;2-3
78. Li CJ, Shen GH. Mechanism of astragalus polysaccharides promote colon cancer stem cell apoptosis byinducing autophagy. Chin J Tradit Chin Med Pharm. (2022) 37:2274–9.
79. Zhang C, Liu J, Xu D, Zhang T, Hu W, Feng Z. Gain-of-function mutant P53 in cancer progression and therapy. J Mol Cell Biol. (2020) 12:674–87. doi: 10.1093/jmcb/mjaa040
80. Zhang X, Qi Z, Yin H, Yang G. Interaction between P53 and Ras signaling controls cisplatin resistance via Hdac4- and Hif-1α-mediated regulation of apoptosis and autophagy. Theranostics. (2019) 9:1096–114. doi: 10.7150/thno.29673
81. Pan F, Lin X, Hao L, Wang T, Song H, Wang R. The critical role of ferroptosis in hepatocellular carcinoma. Front Cell Dev Biol. (2022) 10:882571. doi: 10.3389/fcell.2022.882571
82. Liebl MC, Hofmann TG. The role of P53 signaling in colorectal cancer. Cancers (Basel). (2021) 13:2125. doi: 10.3390/cancers13092125
83. Liu J, Zhang C, Wang X, Hu W, Feng Z. Tumor suppressor P53 cross-talks with trim family proteins. Genes Dis. (2021) 8:463–74. doi: 10.1016/j.gendis.2020.07.003
84. Zhang JX, Han YP, Bai C, Li Q. Notch1/3 and P53/P21 are a potential therapeutic target for Aps-induced apoptosis in non-small cell lung carcinoma cell lines. Int J Clin Exp Med. (2015) 8:12539–47.
85. Hanahan D, Weinberg RA. Hallmarks of cancer: the next generation. Cell. (2011) 144:646–74. doi: 10.1016/j.cell.2011.02.013
86. Sun Y, Liu Y, Ma X, Hu H. The influence of cell cycle regulation on chemotherapy. Int J Mol Sci. (2021) 22:6923. doi: 10.3390/ijms22136923
87. Bonacci T, Emanuele MJ. Dissenting degradation: deubiquitinases in cell cycle and cancer. Semin Cancer Biol. (2020) 67:145–58. doi: 10.1016/j.semcancer.2020.03.008
88. Liu HW, Chen QR, Xiong H, Zhu Y, Zuo L. Astragalus polysaccharides inhabits proliferation, migration and invasion of bladder cancer Umuc3 cells by regulating the Jak2/Stat3 signaling pathway. J Shanxi Med Univ. (2023) 11):1442–8. doi: 10.13753/j.issn.1007-6611.2023.11.002
89. Yu J, Dong XD, Jiao JS, Ji HY, Liu AJ. Antitumor and immunoregulatory activities of a novel polysaccharide from Astragalus membranaceus on S180 tumor-bearing mice. Int J Biol Macromol. (2021) 189:930–8. doi: 10.1016/j.ijbiomac.2021.08.099
90. Du F, Dong LJ. Mechanism of astragalus polysaccharide in inhibiting proliferation of human hepatocellular carcinoma cells. West China J Pharm Sci. (2020) 35:402–6. doi: 10.13375/j.cnki.wcjps.2020.04.012
91. Yan LJ, Hong T, Luo JH, Cao XM, Liu W, Gao Y, et al. Effect of Astragali radix polysaccharides on proliferation and apoptosis of human colon cancer cell line Sw620. Chin J Exp Tradit Med Form. (2017) 23:97–101. doi: 10.13422/j.cnki.syfjx.2017220097
92. Shehzad A, Lee YS. Molecular mechanisms of curcumin action: signal transduction. Biofactors. (2013) 39:27–36. doi: 10.1002/biof.1065
93. Xin P, Xu X, Deng C, Liu S, Wang Y, Zhou X, et al. The role of Jak/Stat signaling pathway and its inhibitors in diseases. Int Immunopharmacol. (2020) 80:106210. doi: 10.1016/j.intimp.2020.106210
94. Tong G, Wang X, Chen S, Jin Y. Astragalus polysaccharide inhibits the development of urothelial carcinoma by activating Ampk signaling to induce Benc1-Xct complex formation. Aging (Albany NY). (2023) 15:9438–52. doi: 10.18632/aging.205007
95. Guo S, Ma B, Jiang X, Li X, Jia Y. Astragalus polysaccharides inhibits tumorigenesis and lipid metabolism through Mir-138-5p/Sirt1/Srebp1 pathway in prostate cancer. Front Pharmacol. (2020) 11:598. doi: 10.3389/fphar.2020.00598
96. Li YQ. Regulation mechanism of astragalus polysaccharides on proliferation, migration and invasion of cervical cancer cells through Jak-Stat signaling pathway. MCH Care China. (2021) 36:1633–6. doi: 10.19829/j.zgfybj.issn.1001-4411.2021.07.054
97. Wu CY, Ke Y, Zeng YF, Zhang YW, Yu HJ. Anticancer activity of astragalus polysaccharide in human non-small cell lung cancer cells. Cancer Cell Int. (2017) 17:115. doi: 10.1186/s12935-017-0487-6
98. Paul CD, Mistriotis P, Konstantopoulos K. Cancer cell motility: lessons from migration in confined spaces. Nat Rev Cancer. (2017) 17:131–40. doi: 10.1038/nrc.2016.123
99. Taki M, Abiko K, Ukita M, Murakami R, Yamanoi K, Yamaguchi K, et al. Tumor immune microenvironment during epithelial-mesenchymal transition. Clin Cancer Res. (2021) 27:4669–79. doi: 10.1158/1078-0432.Ccr-20-4459
100. Kalluri R. Emt: when epithelial cells decide to become mesenchymal-like cells. J Clin Invest. (2009) 119:1417–9. doi: 10.1172/jci39675
101. Yang S, Sun S, Xu W, Yu B, Wang G, Wang H. Astragalus polysaccharide inhibits breast cancer cell migration and invasion by regulating epithelial−Mesenchymal transition via the Wnt/B−Catenin signaling pathway. Mol Med Rep. (2020) 21:1819–32. doi: 10.3892/mmr.2020.10983
102. Conroy H, Mawhinney L, Donnelly SC. Inflammation and cancer: macrophage migration inhibitory factor (Mif)–the potential missing link. Qjm. (2010) 103:831–6. doi: 10.1093/qjmed/hcq148
103. Hertelendy J, Reumuth G, Simons D, Stoppe C, Kim BS, Stromps JP, et al. Macrophage migration inhibitory factor - a favorable marker in inflammatory diseases? Curr Med Chem. (2018) 25:601–5. doi: 10.2174/0929867324666170714114200
104. Guda MR, Rashid MA, Asuthkar S, Jalasutram A, Caniglia JL, Tsung AJ, et al. Pleiotropic role of macrophage migration inhibitory factor in cancer. Am J Cancer Res. (2019) 9:2760–73.
105. Funamizu N, Hu C, Lacy C, Schetter A, Zhang G, He P, et al. Macrophage migration inhibitory factor induces epithelial to mesenchymal transition, enhances tumor aggressiveness and predicts clinical outcome in resected pancreatic ductal adenocarcinoma. Int J Cancer. (2013) 132:785–94. doi: 10.1002/ijc.27736
106. Guo X, Xu S, Gao X, Wang J, Xue H, Chen Z, et al. Macrophage migration inhibitory factor promotes vasculogenic mimicry formation induced by hypoxia via Cxcr4/Akt/Emt pathway in human glioblastoma cells. Oncotarget. (2017) 8:80358–72. doi: 10.18632/oncotarget.18673
107. Liao CH, Yong CY, Lai GM, Chow JM, Cheng CF, Fang CL, et al. Astragalus polysaccharide (Pg2) suppresses macrophage migration inhibitory factor and aggressiveness of lung adenocarcinoma cells. Am J Chin Med. (2020) 48:1491–509. doi: 10.1142/s0192415x20500731
108. Calin GA, Croce CM. Microrna-cancer connection: the beginning of a new tale. Cancer Res. (2006) 66:7390–4. doi: 10.1158/0008-5472.Can-06-0800
109. Tao X, Zhang X, Feng F. Astragalus polysaccharide suppresses cell proliferation and invasion by up-regulation of Mir-195-5p in non-small cell lung cancer. Biol Pharm Bull. (2022) 45:553–60. doi: 10.1248/bpb.b21-00634
110. Zhang XX, Li WH, Chen XM, Liu QM. Study of astragalus polysaccharide inhibits the proliferation, invasion and migration of prostate cancer Du145 cells by up regulating Mir-133a. Chin J Clin Pharmacol. (2023) 39:395–9. doi: 10.13699/j.cnki.1001-6821.2023.03.021
111. Lawrence R, Watters M, Davies CR, Pantel K, Lu YJ. Circulating tumour cells for early detection of clinically relevant cancer. Nat Rev Clin Oncol. (2023) 20:487–500. doi: 10.1038/s41571-023-00781-y
112. Feng X, Shi XMY. Correlations of recurrence of gastric cancer in patients after radical surgery with serum gastrointestinal hormones, vascular endothelial growth factors and serum anti-helicobacter pylori Igg antibody. J buon. (2020) 25:1476–81.
113. Liabeuf D, Oshima M, Stange DE, Sigal M. Stem cells, helicobacter pylori, and mutational landscape: utility of preclinical models to understand carcinogenesis and to direct management of gastric cancer. Gastroenterology. (2022) 162:1067–87. doi: 10.1053/j.gastro.2021.12.252
114. Zhang Y, Brekken RA. Direct and indirect regulation of the tumor immune microenvironment by Vegf. J Leukoc Biol. (2022) 111:1269–86. doi: 10.1002/JLB.5RU0222-082R
115. Zhao L, Zhong Y, Liang J, Gao H, Tang N. Effect of astragalus polysaccharide on the expression of Vegf and Egfr in mice with Lewis transplantable lung cancer. J Coll Physicians Surg Pak. (2019) 29:392–4. doi: 10.29271/jcpsp.2019.04.392
116. Tang YW, Li YT. Effects of astragalus polysaccharides on in vitro cell metastasis of Sgc7901 induced by non-contact co-cultured huvecs cells. Chin Tradit Pat Med. (2020) 42:887–92. doi: 10.3969/j.issn.1001-1528.2020.04.012
117. Jin M, Zhao K, Huang Q, Shang P. Structural features and biological activities of the polysaccharides from Astragalus membranaceus. Int J Biol Macromol. (2014) 64:257–66. doi: 10.1016/j.ijbiomac.2013.12.002
118. Rosenthal R, Günzel D, Finger C, Krug SM, Richter JF, Schulzke JD, et al. The effect of chitosan on transcellular and paracellular mechanisms in the intestinal epithelial barrier. Biomaterials. (2012) 33:2791–800. doi: 10.1016/j.biomaterials.2011.12.034
119. Parhi P, Mohanty C, Sahoo SK. Nanotechnology-based combinational drug delivery: an emerging approach for cancer therapy. Drug Discovery Today. (2012) 17:1044–52. doi: 10.1016/j.drudis.2012.05.010
120. Li X, Yu C, Meng X, Hou Y, Cui Y, Zhu T, et al. Study of double-targeting nanoparticles loaded with Mcl-1 Sirna and dexamethasone for adjuvant-induced arthritis therapy. Eur J Pharm Biopharm. (2020) 154:136–43. doi: 10.1016/j.ejpb.2020.07.009
121. Mal J, Veneman WJ, Nancharaiah YV, van Hullebusch ED, Peijnenburg WJ, Vijver MG, et al. A comparison of fate and toxicity of selenite, biogenically, and chemically synthesized selenium nanoparticles to zebrafish (Danio rerio) embryogenesis. Nanotoxicology. (2017) 11:87–97. doi: 10.1080/17435390.2016.1275866
122. Zhang J, Wang X, Xu T. Elemental selenium at nano size (Nano-se) as a potential chemopreventive agent with reduced risk of selenium toxicity: comparison with se-methylselenocysteine in mice. Toxicol Sci. (2008) 101:22–31. doi: 10.1093/toxsci/kfm221
123. Estevez H, Garcia-Lidon JC, Luque-Garcia JL, Camara C. Effects of chitosan-stabilized selenium nanoparticles on cell proliferation, apoptosis and cell cycle pattern in Hepg2 cells: comparison with other selenospecies. Colloids Surf B Biointerf. (2014) 122:184–93. doi: 10.1016/j.colsurfb.2014.06.062
124. Zhao S, Yu Q, Pan J, Zhou Y, Cao C, Ouyang JM, et al. Redox-responsive mesoporous selenium delivery of doxorubicin targets Mcf-7 cells and synergistically enhances its anti-tumor activity. Acta Biomater. (2017) 54:294–306. doi: 10.1016/j.actbio.2017.02.042
125. Jia X, Liu Q, Zou S, Xu X, Zhang L. Construction of selenium nanoparticles/B-glucan composites for enhancement of the antitumor activity. Carbohydr Polym. (2015) 117:434–42. doi: 10.1016/j.carbpol.2014.09.088
126. Sonkusre P, Cameotra SS. Biogenic selenium nanoparticles induce Ros-mediated necroptosis in Pc-3 cancer cells through Tnf activation. J Nanobiotechnol. (2017) 15:43. doi: 10.1186/s12951-017-0276-3
127. Ji H, Lou X, Jiao J, Li Y, Dai K, Jia X. Preliminary structural characterization of selenium nanoparticle composites modified by astragalus polysaccharide and the cytotoxicity mechanism on liver cancer cells. Molecules. (2023) 28:1561. doi: 10.3390/molecules28041561
128. Jiao J, Yu J, Ji H, Liu A. Synthesis of macromolecular astragalus polysaccharide-nano selenium complex and the inhibitory effects on Hepg2 cells. Int J Biol Macromol. (2022) 211:481–9. doi: 10.1016/j.ijbiomac.2022.05.095
129. Duan Z, Liang M, Yang C, Yan C, Wang L, Song J, et al. Selenium nanoparticles coupling with astragalus polysaccharides exert their cytotoxicities in Mcf-7 cells by inhibiting autophagy and promoting apoptosis. J Trace Elem Med Biol. (2022) 73:127006. doi: 10.1016/j.jtemb.2022.127006
130. Huang QL, Shi MX, Wang JR, Qu D, Liu YP, Chen Y. Preparation and polarization activity research of astragalus polysaccharide-superparamagnetic iron oxide nanocomposite. Acta Pharm Sin. (2023) 58:779–88. doi: 10.16438/j.0513-4870.2022-1059
131. Tan DS, Miller RE, Kaye SB. New perspectives on molecular targeted therapy in ovarian clear cell carcinoma. Br J Cancer. (2013) 108:1553–9. doi: 10.1038/bjc.2013.126
132. Gong Q, Yu H, Ding G, Ma J, Wang Y, Cheng X. Suppression of stemness and enhancement of chemosensibility in the resistant melanoma were induced by astragalus polysaccharide through pd-L1 downregulation. Eur J Pharmacol. (2022) 916:174726. doi: 10.1016/j.ejphar.2021.174726
133. Liu Y, Chen LY. Effect of astragalus polysaccharide on cisplatin resistance of human lung cancer A549/Ddp cells and its mechanism. J Jilin Univ Med Edit. (2020) 46:1162–8 + 348. doi: 10.13481/j.1671-587x.20200609
134. Lu QZ, Luo YY, Wang X, Zhang LL, Chen SH. Astragalus polysaccharide regulates cisplat in resistance in colorectal cancer cells through Mir-10b-5p/Ag Pat3 molecular axis. J Shenyang Pharm Univ. (2022) 39:970–8. doi: 10.14066/j.cnki.cn21-1349/r.2020.0982
135. Yang YL, Lin ZW, He PT, Nie H, Yao QY, Zhang SY. Inhibitory effect of astragalus polysaccharide combined with cisplatin on cell cycle and migration of nasopharyngeal carcinoma cell lines. Biol Pharm Bull. (2021) 44:926–31. doi: 10.1248/bpb.b20-00959
136. Li C, Hong L, Liu C, Min J, Hu M, Guo W. Astragalus polysaccharides increase the sensitivity of Skov3 cells to cisplatin. Arch Gynecol Obstet. (2018) 297:381–6. doi: 10.1007/s00404-017-4580-9
137. Li M, Duan F, Pan Z, Liu X, Lu W, Liang C, et al. Astragalus polysaccharide promotes doxorubicin-induced apoptosis by reducing O-glcnacylation in hepatocellular carcinoma. Cells. (2023) 12:866. doi: 10.3390/cells12060866
138. Wei J, Li Y, Xu B, Yu J. Astragalus polysaccharides reverse gefitinib resistance by inhibiting mesenchymal transformation in lung adenocarcinoma cells. Am J Transl Res. (2020) 12:1640–57.
139. Bao WR, Li ZP, Zhang QW, Li LF, Liu HB, Ma DL, et al. Astragalus polysaccharide rap selectively attenuates paclitaxel-induced cytotoxicity toward Raw 264.7 cells by reversing cell cycle arrest and apoptosis. Front Pharmacol. (2018) 9:1580. doi: 10.3389/fphar.2018.01580
140. Wu J, Wang J, Su Q, Ding W, Li T, Yu J, et al. Traditional Chinese medicine astragalus polysaccharide enhanced antitumor effects of the angiogenesis inhibitor apatinib in pancreatic cancer cells on proliferation, invasiveness, and apoptosis. Onco Targets Ther. (2018) 11:2685–98. doi: 10.2147/ott.S157129
141. Liu Y, Cao X. Characteristics and significance of the pre-metastatic niche. Cancer Cell. (2016) 30:668–81. doi: 10.1016/j.ccell.2016.09.011
142. Du X, Zhao B, Li J, Cao X, Diao M, Feng H, et al. Astragalus polysaccharides enhance immune responses of Hbv DNA vaccination via promoting the dendritic cell maturation and suppressing Treg frequency in mice. Int Immunopharmacol. (2012) 14:463–70. doi: 10.1016/j.intimp.2012.09.006
143. Bamodu OA, Kuo KT, Wang CH, Huang WC, Wu ATH, Tsai JT, et al. Astragalus polysaccharides (Pg2) enhances the M1 polarization of macrophages, functional maturation of dendritic cells, and T cell-mediated anticancer immune responses in patients with lung cancer. Nutrients. (2019) 11:2264. doi: 10.3390/nu11102264
144. Wei W, Li ZP, Bian ZX, Han QB. Astragalus polysaccharide rap induces macrophage phenotype polarization to M1 via the notch signaling pathway. Molecules. (2019) 24:2016. doi: 10.3390/molecules24102016
145. Li C, Pan XY, Ma M, Zhao J, Zhao F, Lv YP. Astragalus polysacharin inhibits hepatocellular carcinoma-like phenotypes in a murine Hcc model through repression of M2 polarization of tumour-associated macrophages. Pharm Biol. (2021) 59:1533–9. doi: 10.1080/13880209.2021.1991384
146. Li W, Song K, Wang S, Zhang C, Zhuang M, Wang Y, et al. Anti-tumor potential of astragalus polysaccharides on breast cancer cell line mediated by macrophage activation. Mater Sci Eng C Mater Biol Appl. (2019) 98:685–95. doi: 10.1016/j.msec.2019.01.025
147. Ding G, Gong Q, Ma J, Liu X, Wang Y, Cheng X. Immunosuppressive activity is attenuated by astragalus polysaccharides through remodeling the gut microenvironment in melanoma mice. Cancer Sci. (2021) 112:4050–63. doi: 10.1111/cas.15078
148. Yu J, Ji H, Yang Z, Liu A. Relationship between structural properties and antitumor activity of astragalus polysaccharides extracted with different temperatures. Int J Biol Macromol. (2019) 124:469–77. doi: 10.1016/j.ijbiomac.2018.11.156
149. He L, Xu K, Niu L, Lin L. Astragalus polysaccharide (Aps) attenuated pd-L1-mediated immunosuppression via the Mir-133a-3p/Msn axis in Hcc. Pharm Biol. (2022) 60:1710–20. doi: 10.1080/13880209.2022.2112963
150. Chang HL, Kuo YH, Wu LH, Chang CM, Cheng KJ, Tyan YC, et al. The extracts of Astragalus membranaceus overcome tumor immune tolerance by inhibition of tumor programmed cell death protein ligand-1 expression. Int J Med Sci. (2020) 17:939–45. doi: 10.7150/ijms.42978
151. Mashima T, Seimiya H, Tsuruo T. De novo fatty-acid synthesis and related pathways as molecular targets for cancer therapy. Br J Cancer. (2009) 100:1369–72. doi: 10.1038/sj.bjc.6605007
152. Menendez JA, Lupu R. Fatty acid synthase and the lipogenic phenotype in cancer pathogenesis. Nat Rev Cancer. (2007) 7:763–77. doi: 10.1038/nrc2222
153. Merino Salvador M, Gómez de Cedrón M, Moreno Rubio J, Falagán Martínez S, Sánchez Martínez R, Casado E, et al. Lipid metabolism and lung cancer. Crit Rev Oncol Hematol. (2017) 112:31–40. doi: 10.1016/j.critrevonc.2017.02.001
154. Zaidi N, Lupien L, Kuemmerle NB, Kinlaw WB, Swinnen JV, Smans K. Lipogenesis and lipolysis: the pathways exploited by the cancer cells to acquire fatty acids. Prog Lipid Res. (2013) 52:585–9. doi: 10.1016/j.plipres.2013.08.005
155. Pope ED 3rd, Kimbrough EO, Vemireddy LP, Surapaneni PK, Copland JA 3rd, Mody K. Aberrant lipid metabolism as a therapeutic target in liver cancer. Expert Opin Ther Targets. (2019) 23:473–83. doi: 10.1080/14728222.2019.1615883
156. Cheng L, Zhu Y, Han H, Zhang Q, Cui K, Shen H, et al. Microrna-148a deficiency promotes hepatic lipid metabolism and hepatocarcinogenesis in mice. Cell Death Dis. (2017) 8:e2916. doi: 10.1038/cddis.2017.309
157. Yi M, Li J, Chen S, Cai J, Ban Y, Peng Q, et al. Emerging role of lipid metabolism alterations in cancer stem cells. J Exp Clin Cancer Res. (2018) 37:118. doi: 10.1186/s13046-018-0784-5
158. Xu H, Zhou S, Tang Q, Xia H, Bi F. Cholesterol metabolism: new functions and therapeutic approaches in cancer. Biochim Biophys Acta Rev Cancer. (2020) 1874:188394. doi: 10.1016/j.bbcan.2020.188394
159. Yarla N, Madka V, Rao C. Targeting triglyceride metabolism for colorectal cancer prevention and therapy. Curr Drug Targets. (2022) 23:628–35. doi: 10.2174/1389450122666210824150012
160. Wang JY, Yuan YY, Zhang K, Sun X, Bu X, Dong J, et al. Ndrg2 inhibits tumorigenesis of hepatocellular carcinoma by regulating metabolism of phospholipids and triglyceride: A metabonomic analysis. J South Med Univ. (2022) 42:1765–73.
161. Zhuang Q, Ye X, Shen S, Cheng J, Shi Y, Wu S, et al. Astragalus polysaccharides ameliorate diet-induced gallstone formation by modulating synthesis of bile acids and the gut microbiota. Front Pharmacol. (2021) 12:701003. doi: 10.3389/fphar.2021.701003
162. Cheng Y, Tang K, Wu S, Liu L, Qiang C, Lin X, et al. Astragalus polysaccharides lowers plasma cholesterol through mechanisms distinct from statins. PloS One. (2011) 6:e27437. doi: 10.1371/journal.pone.0027437
163. He C, Klionsky DJ. Regulation mechanisms and signaling pathways of autophagy. Annu Rev Genet. (2009) 43:67–93. doi: 10.1146/annurev-genet-102808-114910
164. Klionsky DJ, Abdel-Aziz AK, Abdelfatah S, Abdellatif M, Abdoli A, Abel S, et al. Guidelines for the use and interpretation of assays for monitoring autophagy (4th edition)(1). Autophagy. (2021) 17:1–382. doi: 10.1080/15548627.2020.1797280
165. Yoshii SR, Mizushima N. Monitoring and measuring autophagy. Int J Mol Sci. (2017) 18:1865. doi: 10.3390/ijms18091865
166. Li X, He S, Ma B. Autophagy and autophagy-related proteins in cancer. Mol Cancer. (2020) 19:12. doi: 10.1186/s12943-020-1138-4
167. Li XF, Chen DP, Ouyang FZ, Chen MM, Wu Y, Kuang DM, et al. Increased autophagy sustains the survival and pro-tumourigenic effects of neutrophils in human hepatocellular carcinoma. J Hepatol. (2015) 62:131–9. doi: 10.1016/j.jhep.2014.08.023
168. Zhi Q, Zhang N, Feng GL, Sun WY, Zhao YY, Yang SF. Study on the mechanism of astragalus polysaccharides regulating autophagy of colorectal cancer cells based on Pi3k/Akt/Mtor signaling pathway. Tianjin Med J. (2023) 51:240–5. doi: 10.11958/20220463
169. Chang MZ, Zhang SY, Hao YJ, Zhang SL, Zhang XL. Astragalus polysaccharide inhibits the proliferation of esophageal cancer ec109 cells by inducing cell autophagy. Cent South Pharm. (2022) 20:856–62. doi: 10.7539/j.issn.1672-2981.2022.04.021
170. Tsao SM, Wu TC, Chen J, Chang F, Tsao T. Astragalus polysaccharide injection (Pg2) normalizes the neutrophil-to-lymphocyte ratio in patients with advanced lung cancer receiving immunotherapy. Integr Cancer Ther. (2021) 20:1534735421995256. doi: 10.1177/1534735421995256
171. Zhang Y, Jia YJ, Li XJ, Wang L, Du MN, Zhang XX. Clinical observation on astragalus polysaccharide injection combined with Cik cells treating Nsclc patients with qi deficiency syndrome. Chin Tradit Herbal Drug. (2018) 49:1647–51. doi: 10.7501/j.issn.0253-2670.2018.07.024
172. Liu DL, Gao FF, Wang MM, Li HT. Effects of astragalus polysaccharide injection combined with gefitinib on immune function, quality of life and toxicity of advanced lung cancer. Modern J Integr Tradit Chin West Med. (2018) 27:4049–51. doi: 10.3969/j.issn.1008-8849.2018.36.016
173. Zheng ZP, Yang WB, Li N, Bai YJ, Xing SY, Shao Q, et al. Clinical observation on injection of astragalus polysaccharides protecting marrow suppression of non-small-cell lung cancer after chemotherapy. Chin Tradit Herbal Drugs. (2013) 44:208–9. doi: 10.7501/j.issn.0253-2670.2013.02.018
174. Gildener-Leapman N, Ferris RL, Bauman JE. Promising systemic immunotherapies in head and neck squamous cell carcinoma. Oral Oncol. (2013) 49:1089–96. doi: 10.1016/j.oraloncology.2013.09.009
175. Hsieh CH, Lin CY, Hsu CL, Fan KH, Huang SF, Liao CT, et al. Incorporation of astragalus polysaccharides injection during concurrent chemoradiotherapy in advanced pharyngeal or laryngeal squamous cell carcinoma: preliminary experience of a phase II double-blind, randomized trial. J Cancer Res Clin Oncol. (2020) 146:33–41. doi: 10.1007/s00432-019-03033-8
Keywords: Astragalus polysaccharides, anti-tumor therapy, cancer, anti-tumor mechanism, apoptosis, autophagy
Citation: Yang Q, Meng D, Zhang Q and Wang J (2024) Advances in research on the anti-tumor mechanism of Astragalus polysaccharides. Front. Oncol. 14:1334915. doi: 10.3389/fonc.2024.1334915
Received: 09 November 2023; Accepted: 22 February 2024;
Published: 06 March 2024.
Edited by:
Tao Yi, Hong Kong Baptist University, Hong Kong SAR, ChinaReviewed by:
Yang Li, Baylor College of Medicine, United StatesCopyright © 2024 Yang, Meng, Zhang and Wang. This is an open-access article distributed under the terms of the Creative Commons Attribution License (CC BY). The use, distribution or reproduction in other forums is permitted, provided the original author(s) and the copyright owner(s) are credited and that the original publication in this journal is cited, in accordance with accepted academic practice. No use, distribution or reproduction is permitted which does not comply with these terms.
*Correspondence: Qinyuan Zhang, emhhbmdxaW55dWFuMTk3MUAxMjYuY29t; Jin Wang, enl3YW5namluQDE2My5jb20=
Disclaimer: All claims expressed in this article are solely those of the authors and do not necessarily represent those of their affiliated organizations, or those of the publisher, the editors and the reviewers. Any product that may be evaluated in this article or claim that may be made by its manufacturer is not guaranteed or endorsed by the publisher.
Research integrity at Frontiers
Learn more about the work of our research integrity team to safeguard the quality of each article we publish.