- 1Laboratory of Vascular Surgery, Affiliated Hospital of Guangdong Medical University, Zhanjiang, Guangdong, China
- 2Department of Breast Surgery, Affiliated Hospital of Guangdong Medical University, Zhanjiang, Guangdong, China
Cancer cells can alter their metabolism to meet energy and molecular requirements due to unfavorable environments with oxygen and nutritional deficiencies. Therefore, metabolic reprogramming is common in a tumor microenvironment (TME). Aryl hydrocarbon receptor (AhR) is a ligand-activated nuclear transcription factor, which can be activated by many exogenous and endogenous ligands. Multiple AhR ligands can be produced by both TME and tumor cells. By attaching to various ligands, AhR regulates cancer metabolic reprogramming by dysregulating various metabolic pathways, including glycolysis, lipid metabolism, and nucleotide metabolism. These regulated pathways greatly contribute to cancer cell growth, metastasis, and evading cancer therapies; however, the underlying mechanisms remain unclear. Herein, we review the relationship between TME and metabolism and describe the important role of AhR in cancer regulation. We also focus on recent findings to discuss the idea that AhR acts as a receptor for metabolic changes in tumors, which may provide new perspectives on the direction of AhR research in tumor metabolic reprogramming and future therapeutic interventions.
1 Introduction
Cancer is the leading cause of death worldwide, an important barrier to life expectancy, and a cause of considerable economic burden on patients (1). Cancer is caused due to oncogene activation and the loss of cancer suppressors, which causes metabolic reprogramming to provide metabolites and energy needed by the cancer cells to sustain tumorigenesis and survival (2). Under normoxic conditions, cells obtain energy via glycolysis and mitochondrial oxidative phosphorylation, whereas under hypoxic conditions, with compromised mitochondrial function, cells mainly rely on glycolysis (3). The infinite proliferation of cancer cells necessitates urgent requirements for more energy, resulting in an imbalance between the production and consumption of energy and oxygen and a hypoxic environment, followed by a series of dysregulations in various metabolic pathways (4).
Recent studies have revealed considerable differences between the metabolic profiles of cancer and normal tissues, with cancer cells exhibiting varying degrees of alteration in metabolic pathways, including glycolysis, the tricarboxylic acid (TCA) cycle, amino acid, nucleotide, and lipid metabolism. Tumor cells undergo adaptive changes in their metabolic characteristics, a process known as metabolic reprogramming, in response to several interacting factors, including a harsh tumor microenvironment (TME) caused by fast tumor cell proliferation (5). In addition to markedly altered glucose metabolism, tumor cells differ greatly from normal cells regarding nucleotide production and utilization (6); for example, among the three breast cancer subtypes, triple-negative breast cancer (TNBC) has shown the most robust nucleotide biosynthesis compared with that shown by normal breast tissue (7, 8). These metabolic changes and various reactions depend not only on the cancer subtype but also on how the cancer cells interact with the intricate surrounding milieu (9). A particular heterogeneity of cancer cells may result from the changes in cellular metabolic pathways, together with the molecules produced and inefficient oxygen supply, forming the unfavorable TME, which in turn regulates the proliferation and invasion of cancer cells (10). In summary, tumor metabolic reprogramming is a notable contributor to a distinctive TME.
Reportedly, in glioma cells, aryl hydrocarbon receptor (AhR) is activated by kynurenine (Kyn), which is generated by indoleamine 2,3-dioxygenase (IDO), causing tumor-associated macrophage (TAM) accumulation in the TME. Mounting evidence suggests that Kyn-triggered AhR helps tumor cells avoid the immune system (11, 12). In patients with melanoma, high levels of IDO-1 and Kyn have been linked to immunosuppression (13). Although the precise measurement of Kyn in cutaneous melanoma is unknown, it is estimated to be >50 µM in the TME and approximately 2–8 µM in the plasma (depending on the disease type) (14–18). Furthermore, excess reactive oxygen species (ROS) from the TME may trigger the synthesis of antioxidant proteins by activating AhR, enabling tumor cell response to the TME (19). In summary, AhR is an important target for enhancing tumor adaptation, tumor immune evasion, and monitoring changes in the TME.
AhR is a ligand-activated transcription factor that is most commonly associated with xenobiotic ligand metabolism (20). Previous research has discovered that AhR has a crucial role in lipid metabolism, nucleotide de novo synthesis, and tumor glycolysis (21–23). AhR ligands include environmentally toxic chemicals, such as 2,3,7,8-tetrachlorodibenzo-p-dioxin (dioxin), and exogenous and endogenous ligands, such as 2-(1’H-indole-3’-carbonyl)-thiazole-4-carboxylic acid methyl ester (ITE) and various tryptophan metabolites (24, 25). Previous studies have shown that AhR ligands may exhibit agonistic or antagonistic activities (26–28). AhR can cause metabolic modifications in cancer cells via the regulation of glycolysis and lipid metabolism by interacting with different ligands (29). However, the causal relationship between AhR and metabolic reprogramming in cancer remains unclear.
ROS are inevitable byproducts of intracellular metabolism (30) and are generated due to active mitochondrial metabolism (31). ROS mediate oxidative stress; however, the true cause is not the formation of ROS but rather the imbalance in space and time between ROS production and detoxification (32). Oncogene activation, upregulation of the phosphoinositide 3-kinase signaling pathway, and hypoxia cause mitochondria in the cancer cells to produce ROS at a higher rate (33–35), producing a harsh TME. Low ROS concentrations, particularly that of H2O2, favorably control cellular growth and adaptation to metabolic stress (36). Thus, antioxidants are beneficial for tumor cell proliferation and can be targeted to inhibit antioxidants to prevent cancer cell proliferation, tumorigenesis, and metastasis (37). Uric acid (UA), a byproduct of purine metabolism, exhibits antioxidant properties (38) that are likely to have prognostic implications for patients with cancer. Reportedly, AhR can mediate ROS generation (39–41). Interestingly, increased ROS levels in the TME can trigger the production of antioxidant proteins by activating AhR, thereby shielding breast cancer cells from oxidative stress (19). Cancer cells that undergo metabolic reprogramming inevitably produce excess ROS, resulting in a harsh TME. AhR detects ROS and mediates their generation. Hence, AhR, TME, metabolic reprogramming, and ROS are intertwined and maintain a dynamic equilibrium.
Presently, many studies have shown that AhR is involved in the process of cancer metabolic reprogramming by binding with different ligands and that novel interventions targeting AhR may have notable clinical value. In this review, we highlight AhR signaling pathways in the TME and their contributions to tumor survival and invasion. Additionally, we describe the important role of AhR in the regulation of cancer cell metabolism and relevant pathways, which may be used to better understand the potential of anticancer therapies.
2 Clinical applications of metabolic reprogramming in tumors
The TME, a crucial location for cancer cell metabolism, is critical for AhR to regulate tumor metabolic reprogramming. Many studies have focused on the relationship between tumor cells and their microenvironments. TME is composed of both cancerous and non-cancerous cells that control tumor growth, progression, and resistance to cancer treatment (42). TME includes extracellular matrix, endothelial cells, cancer-associated fibroblasts, adipocytes, and TAMs. It has been demonstrated that the chemical carcinogen 3-methylcholanthrene activates AhR in CAF, which accelerates the development of breast cancer (43). Kyn-activated AhR produced by CAFs isolated from tumors is associated with tumor drug resistance, and it has been suggested that targeted inhibition of AhR may be a new strategy for the treatment of malignant tumors (44, 45). There is evidence that CAF is associated with tumor metabolism (46), and interestingly, AhR can be activated by small molecules produced in metabolism (47). In glioblastoma, kyn activates AhR in TAM to regulate its function and T-cell immunity, correlating with poor tumor prognosis (11). Tryptophan-derived metabolites can aid immune escape from tumors by activating the AhR of TAM, and expression of the AhR in TAM has a profound impact on tumor growth and TME (48). In TME, the expression of AhR in CAF and TAM is closely related to metabolism and tumor progression.
TME studies have mainly focused on metabolic reprogramming, which is considered one of the key factors promoting cancer development (49). The dysregulation of tumor cell metabolism results in hypoxia, an acidic TME, and the depletion of sensitive metabolites in the cells (50), which enables the invasion of effector T cells to compete with the tumor for metabolites and compromise their function (51, 52). Cellular carcinogenesis causes metabolic pathways to be dysregulated, and these altered metabolic pathways, in turn, provide cancer cells with a better chance of survival under hypoxic environments and confer them the ability to proliferate and survive at a high rate. Reportedly, metabolic byproducts of cancer cells can regulate the function of tumor-infiltrating immune cells and offer numerous benefits. For example, lactic acid released by cancer cells through glycolysis promotes the polarization of immune cells toward an immunosuppressive phenotype (53, 54). Changes in the metabolism of cancer cells also exert a considerable effect on other TME components, such as non-cancerous cells, in the microenvironment, which can stimulate the migration of cancer cells and mediate pro-cancerous activities (9). Because cancer cells grow much faster than normal cells because of metabolic reprogramming (55), metabolic reprogramming and the TME have become popular research topics. Studies on tumor heterogeneity have focused on the development of immunosuppression by tumor cells in response to glucose competition with normal tissues and the increased release of lactate from the microenvironment following metabolism (56, 57). Tumor metabolism is controllable, and clinical therapy that reprograms the TME greatly affects the chemotherapy, radiation, and targeted therapy on tumor cells. Through the use of nano-delivery technologies, the T cell activator anti-CD28-coupled aryl hydrocarbon receptor (AhR) inhibitor (CH223191) can be encapsulated to modify the tumor immune milieu and successfully prevent tumor cell metastasis (58).
There have been some successful attempts at reprogramming tumor metabolism worldwide. A meta-analysis showed that the overexpression of glucose transporter protein 1 (GLUT1) in solid cancers is linked to the poor prognosis of many tumor types, suggesting that direct GLUT1 targeting could be a promising treatment strategy for solid cancers (59). Serine biosynthesis is frequently increased in various cancer cells, and overexpressed genes are involved in nucleotide synthesis, antioxidant defense, and methylation responses in breast cancer cells (60, 61). Fatty acid synthase (FASN) is an oncogene and is involved in cancer-associated metabolic reprogramming, and FASN-targeted drugs are in clinical development and trials (62). In addition to recent studies on glucose, amino acid, and lipid metabolisms, the study of nucleotide metabolism and its metabolites in the TME metabolic reprogramming has been a hot spot. Recent research demonstrated that purine metabolism in the TME causes heterogeneity in macrophages (63), and that proline isomerase of CAF in TME targets pancreatic ductal adenocarcinoma synergetically with gemcitabine, the antipyrimidine metabolizing medication, to facilitate the elimination of the tumor by immunochemotherapy (64). It’s interesting to note that changes in AhR expression are correspond to susceptibility and resistance to the clinical antimetabolic chemotherapy medicines gemcitabine and cytarabine, which are currently widely utilized (65, 66).
The synthesis of nucleotides and deoxyribonucleotides is the first metabolic route to be extensively studied and successfully targeted in cancer therapy (67). Deoxyribonucleoside triphosphates (dNTPs), which are necessary for DNA replication and transmission of the entire genome to the next generation, are required by all dividing cells during the S phase of the cell cycle. Additionally, compared with normal cells, tumor cells that are highly replicating and proliferating have more active nucleotide metabolism. Targeting DNA replication is another early cancer therapeutic strategy (68). Early identified antimetabolic chemotherapeutic agents include folate antagonists, pyrimidines, and purines such as methotrexate, cytarabine, and fluorouracil. However, these interfere with DNA replication and synthesis and greatly damage autologous cells, searching for specific targets is an urgent problem. Both remedial and de novo synthesis can provide nucleotides for cellular needs, and remedial synthesis serves as the main route for nucleotide acquisition in healthy cells. In a recent study, breast cancer cells undergoing lung metastasis were found to have considerably higher levels of phosphoribosyl pyrophosphate synthase 2 (PRPS2), which is a crucial gene for de novo nucleotide synthesis. This gene leads to the production of more cyclic guanosine monophosphate (cGMP), which in turn activates the cGMP-dependent protein kinase G and downstream mitogen-activated protein kinase (MAPK) pathways, thereby increasing tumor stemness. Tumor stemness can be considerably reduced and lung metastasis can be prevented in breast cancer cells by silencing the PRPS2 gene to block de novo nucleotide synthesis. The metabolic signature of metastatic breast cancer cells is accelerated by nucleotide synthesis from scratch, and its metabolites can modify signaling pathways to support breast cancer stemness and metastasis (69) (Figure 1).
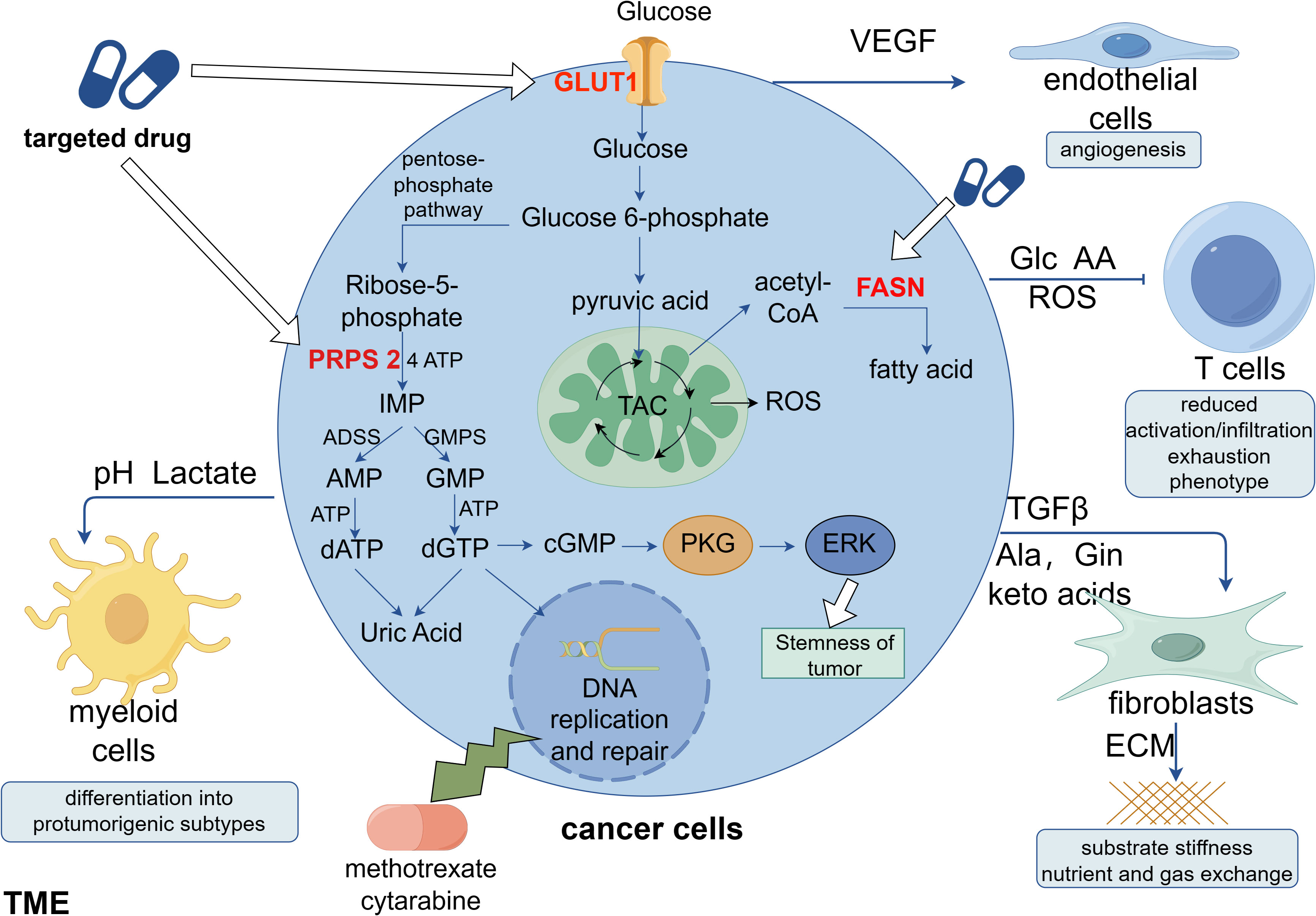
Figure 1 Clinical applications of metabolic reprogramming in tumors. Cancer cells can secrete a variety of factors to stimulate non-cancer cells in the TME, favoring their own proliferation, migration and immune escape. Studies indicate that new targets for anti-tumor metabolism are likely to include phosphoribosyl pyrophosphate synthase 2 (PRPS 2), glucose transporter protein 1 (GLUT1), and fatty acid synthase (FASN). Among these, it has been demonstrated that the nucleotide de novo synthesis-related enzyme PRPS 2 is linked to the stemness of breast cancer, and its efficient suppression will prevent lung metastases of breast cancer. Targeting nucleotide de novo synthesis has significant promise since, in contrast to cancer cells, normal cells obtain nucleotides through remedial synthesis. This is because ab initio nucleotide synthesis consumes a considerable quantity of ATP.
Nucleotide biosynthesis requires a large amount of energy, as shown by the purine synthesis pathway, which requires five adenosine triphosphate molecules, two glutamine and formic acid molecules, and one glycine, aspartic acid, and carbon dioxide molecule to produce one hypoxanthine nucleotide molecule (70). Metabolic reprogramming is crucial in the fight against tumors because the energy metabolism of malignant cells differs greatly from that of normal cells. Many studies have revealed that AhR regulates metabolic pathways and ROS of tumors to varying degrees, offering notable potential for antitumor research (19, 21, 23, 40, 41, 71–73). Recent studies have shown that ROS are involved in metabolic regulation by activating the ribotoxic stress response (RSR) in cellular models (74).
3 Role of AhR in tumor progression
AhR functions as a complex metabolic regulator and transcription factor in most cancer cells. AhR is a ligand-activated receptor for aryl hydrocarbons. When activated from its dormant state in the cytoplasm, AhR translocates to the nucleus and activates the transcription of its target genes (75). In tumor immune escape, AhR plays a key role in the immunosuppressive phenotype of the TME in cancer cells and is closely related to human amino acid metabolism as a sensor of tryptophan metabolites and is also a powerful immune system regulator (48). AhR activation enhances tumor aggressiveness, reduces cluster of differentiation (CD)8 T cell (76) and macrophage (48) antitumor immunity, and aids tumor cells in evading immune responses (77). In summary, AhR overexpression helps tumor cells evade the immune system by sending inhibitory signals to the immune cells through the TME.
In addition to its role in tumor immune evasion, AhR may also play a crucial role in the growth of tumor cells by controlling the cell cycle. In liver cancer, AhR activation prevents tumor cells from entering the G0/G1 phase, which reduces DNA replication and prevents cell proliferation. High levels of tetraploidy have been associated with an increased risk of tumor formation (78), and the existing evidence shows that tumors typically contain chromosomes close to the tetraploid, and the uncontrolled proliferation of tetraploid cells trigger tumor formation (79). Sustained DNA damage has been observed in AhR-deficient hepatocytes, which is detrimental to the entry of cells into the tetraploid phase of proliferation. AhR, as a tumor suppressor, can be activated by controlling the expression of inflammatory cytokines, DNA damage, and cell proliferation (78). In a study, AhR mutant mice showed increased development of liver tumors, and AhR agonists suppressed cholesterol regulatory element-binding protein (SREBP)2 and stopped tumor progression in mice (28). Studies on hepatocellular carcinoma have shown that the activation of AhR can have an oncogenic effect, as opposed to immune escape. Thus, it is evident that the regulatory activities of AhR play intricate roles in tumors and are closely linked to tryptophan metabolism (80).
It is worth investigating whether AhR activation promotes or hinders tumor formation, and studies on breast cancer have readily demonstrated this paradox. When AhR was knocked down by short interfering RNA in two different types of breast cancer cells, BT474 and MDA-MB-468, its regulatory effect on cell proliferation was enhanced in BT474 cells, whereas no effects were detected on the proliferation of MDA-MB-468 cells (81). Animal and cellular studies on the breast cancer cell line MCF-7 have shown that AhR expression is not necessary for mammary carcinogenesis (82) and that the MCF-7 cell proliferation is unaffected by AhR deficiency (83). In contrast, AhR overexpression promotes MCF-7 cell proliferation (84). Furthermore, whether AhR promotes or inhibits malignancy in TNBC MDA-MB-231 cells remains debatable (85–87). According to previous studies, ligands for AhR can either be exogenous or endogenous, and they can function in an agonistic or antagonistic manner (88). Thus, AhR expression alone plays various roles in breast cancer depending on the ligand to which it binds and its effects on different cell types. This suggests that AhR may serve as a relay for pro- or oncogenic factors, and its binding to various AhR ligands helps control the growth and migration of breast cancer cells. Protein kinase A complexes of AhR and nuclear factor-κB (NF-κB) RelB can attach to chemokine-specific binding sites to activate inflammatory factors (89). This promotes the proliferation and migration of breast cancer cells. Additionally, AhR can interact with NF-κB RelA and lead to an increase in cellular-master regulator of cell cycle entry and proliferative metabolism (Myc) levels in MCF-7 cells, which further triggers carcinogenesis (90).
Epithelial-mesenchymal transition (EMT) is the process that epithelial cells lose their polarized shape and acquire the capacity of migration and invasion. It occurs in the early stages of tumor metastasis and is considered to be an important cause of cancer metastasis (91). EMT biomarkers such as waveform protein, N-calmodulin, and MMP 9 are overexpressed in cancer and involved in the promotion of cancer metastasis (92). Many studies have shown that AhR activity leads to loss of cell contact inhibition and alterations in extracellular matrix remodeling, and it is also found that AhR plays critical role in EMT induction (93–95). According to Dai et al., kyn stimulates AhR in renal cell carcinoma to promote infiltration, migration, and EMT progression (96). MMP, a biomarker of EMT, is a family of zinc-dependent endoproteases which could degrade the extracellular matrix to promote cell proliferation and migration (97). MMP could also affect the TME of malignant tumors and support EMT by inducing invasive and metastatic tendencies of cancer cells (98). In esophageal cancer cells, knockdown of AhR gene inhibits tumor progression by down-regulating the expression levels of MMP 1, MMP 2, and MMP 9 (99). Different thyroid carcinoma cell types are promoted to express MMP1, MMP2, and MMP9 differently by kyn-activated AhR (95). TCDD-activated AhR upregulates MMP9 expression activity in a variety of malignant tumors (100–104). As a result, the activation of AhR in different tumors demonstrated a consistent promotion of MMPs, which therefore affect EMT and the further tumor progression.
In addition to MMP, other markers of EMT are also widely studied. TDO 2 regulates metastasis and invasion of hepatocellular carcinoma by activating the Kyn-AhR pathway to promote EMT in cancer cells (104). In thyroid carcinogenesis cells, kyn activates AhR expression, then promotes an increase in fibronectin, SLUG, and N-calmodulin and a decrease in E-calmodulin, which resulted in increased cell invasion and motility. The authors also found that AhR has a close correlation with EMT, with AhR being essential in managing the immunosuppressive milieu, it therefore triggers the development of both EMT and an immunosuppressive TME (95). 3,3’-Diindolylmethane could reverse the EMT process by regulating AhR inhibition of the EGFR/RhoA/ROCK 1/NF-κB/COX 2/PGE 2 pathway, it is reported that 3,3’-Diindolylmethane down-regulated the expression of mesenchymal cell markers including β-Catenin, Vimentin, and Slug, and upregulated the epithelial cell marker Claudin-1 (105). TCDD-activated AhR also promotes EMT in ovarian cancer cells (106), and the current study demonstrated that both ligand-activated AhR promote EMT. However, direct overexpression of AhR showed different regulating roles in different cancers. In lung cancer cells, cells overexpressing AhR exhibited lower cell mobility, high expression of E-cadherin and low expression of waveform proteins (biomarkers associated with EMT), suggesting that higher AhR expression are associated with lower cell motility (107). Studies on gastric cancer cells indicated that ROS mediates the KYNU-kyn-AhR signaling pathway to influence EMT capacity (108). Direct overexpression of AhR leads to the opposite effect, however, the role of ligand-activated AhR in the EMT of malignancies is better established. Therefore, AhR has its potential to be the receptor for metabolic changes in tumors.
Although intricate processes are involved, AhR plays a crucial role in tumorigenesis. The activation of the tryptophan 2,3-dioxygenase-2 (TDO2)–Kyn–AhR pathway promoted liver metastases of colon cancer in a mouse model by aiding in immune evasion and maintaining stemness (109). As AhR can bind to various ligands, we can deduce that it plays various roles in malignancies. However, because the diverse roles of AhR impede a thorough investigation of its mechanisms, it is imperative to identify a broad path for future research on the involvement of AhR in carcinogenesis and development.
4 Effect of different ligand-activated AhR on tumor glucose metabolism
Cancer cells support their metabolism through glycolysis, both aerobic glycolysis (also called the Warburg effect) and anaerobic glycolysis, to provide biosynthetic molecules and energy for the survival and development of cancer cells (55); therefore, robust glycolysis is considered a hallmark of cancer metabolism. However, the effects of AhR on augmented glycolysis remain unclear. Most cells receive sufficient energy to maintain cellular activity through efficient oxidative phosphorylation of glucose in normoxic environments; however, in hypoxic environments, glucose can only be used to produce energy through inefficient anaerobic glycolysis (110, 111). Interestingly, increased glycolytic metabolism can indicate that cancer cells are actively reproducing because glycolysis serves as the primary energy source for cancer cells under both normoxic and hypoxic conditions (55). Numerous studies have suggested that AhR may be an important regulator of glycolytic gene expression and glycolytic endpoints (21, 112–116).
Reportedly, TDO2 enhances the Kyn pathway (KP), thereby producing excess Kyn, which further activates AhR and upregulates CXC chemokine ligand 5 (CXCL5). CXCL5 recruits TAM into the TME and promotes TAM polarization and abundance, leading to active glycolysis in cancer cells and thereby promoting cancer cell proliferation and survival. AhR modulates glucose absorption and total glycolytic flux, in addition to upregulating CXCL5 by affecting several glycolytic genes, including GLUT1, hexokinase (HK) 1/2, and phosphofructokinase-liver type (23). The activation of the cytokine/IDO/Kyn/AhR pathway in pancreatic cancer cells can shield them from inflammation in the hostile TME and facilitate their adaptation to it (117).
Recently, it was discovered that AhR binds to lncRNA and actively controls the expression of the glycolytic enzyme gene hexokinase 2 (HK2), stimulating glycolytic metabolism and accelerating tumor growth (72) (Figure 2). Another study found that HK2 is a transcriptional target of AhR, and over-expression of HK2 could in turn alter AhR gene expression and modulate its activity (118). Additionally, in oncogene MYC-expressing rat fibroblast cells, AhR deletion has been shown to reduce intracellular glucose and pyruvate as well as the expression of enzymes associated to glycolysis and tricarboxylic acid (21). In addition, AhR activated by 2,3’,4,4’,5-pentachlorobiphenyl (PCB118) increased ROS production by up-regulating nicotinamide adenine dinucleotide phosphate (NADPH) oxidase. In the last stage of the glycolytic process, ROS can further upregulate the expression level of the rate-limiting enzyme M2-type pyruvate kinase (PKM2) to improve glucose metabolism in tumor cells (71).
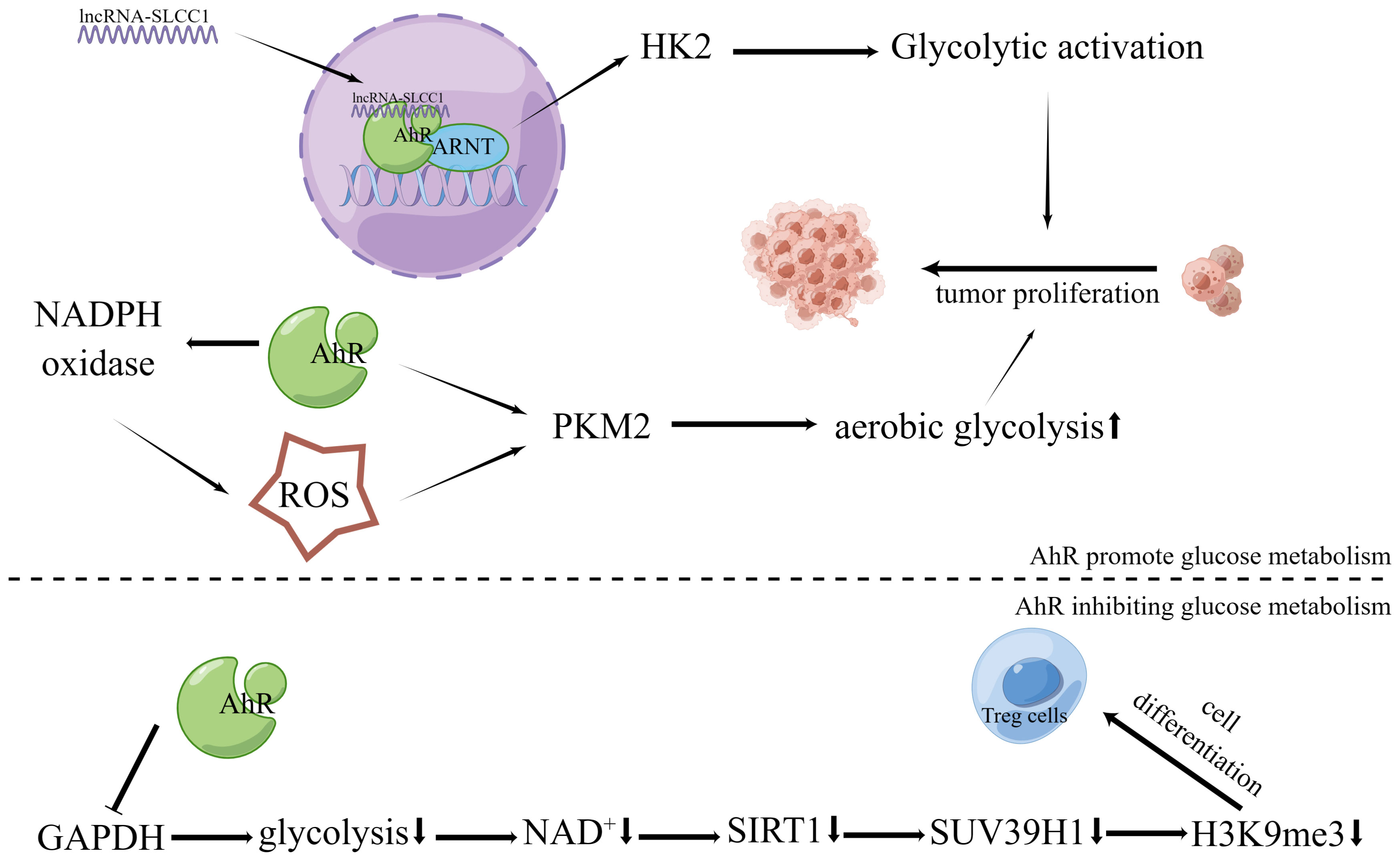
Figure 2 Effects of AhR in tumor energy metabolism. The lncRNA-SLCC1 binds to the AhR and increases the expression of HK2, which activates glycolytic metabolism and promotes tumor growth. As a result of AhR’s activation of NADPH oxidase and subsequent upregulation in ROS production, the expression level of PKM2, the rate-limiting enzyme of aerobic glycolysis, is further increased. This boosts the aerobic glycolysis of tumor cells and encourages the growth of tumor cells via suppressing GAPDH and preventing glycolysis, AhR activation promotes Treg development via controlling the NAD+/SIRT1/SUV39H1/H3K9me3 signaling pathway.
Reduced glucose uptake and mitochondrial activity are correlated with downregulated GLUTs due to 2,3,7,8-tetrachlorodibenzo-p-dioxin (TCDD)-activated AhR, which impacts glucose transport and utilization in pluripotent embryonal cancer cells (119). While assays for glycolytic products have revealed a considerable reduction in glycolytic flux, AhR activation has been shown to decrease the rate of glucose uptake and the formation of pyruvate and lactate (115). The glycolytic process considerably decreased in the colon cells after activation of AhR by Norisoboldine, indicating that AhR activation interferes with the glycolytic pathway by suppressing the production of glyceraldehyde-3-phosphate dehydrogenase (GAPDH) (120) (Figure 2). Consequently, AhR showed important regulating roles in the control of glucose metabolism by binding with different ligands. In addition to glycolysis, cancer cells harness lipid metabolism to obtain the energy and molecules needed for proliferation, survival, invasion, and adaptation to the TME (121).
5 Potential regulatory mechanisms of AhR in tumor lipid metabolism
Lipids are involved in energy metabolism and are important components of the cell membranes and secondary messengers. Because tumors have high metabolic demands and large fatty acids consumption, therefore, cancer cells are different from normal cells in terms of how they absorbing exogenous fatty acids and producing endogenous fatty acids. Specialized transporter proteins are necessary for the effective passage of exogenous FA across the plasma membrane. Tumors exhibit a significant increase in the gene and protein expression levels of these fatty acid transporter proteins; of them the most well studied protein is CD 36, which is commonly referred to as fatty acid translocase (FAT) (122, 123). AhR activation was linked to increased CD36 expression, and siAhR efficiently reduced lipoxin A4-induced CD36 overexpression and lipid uptake (124).
In healthy tissues, only hepatocytes and adipocytes are capable of de novo lipogenesis; however, cancer cells also have the ability to reactivate this anabolic process (125). Key regulators of adipogenesis, including sterol regulatory element binding protein (SREBP), acetyl coenzyme A carboxylase (ACC), FASN and stearoyl coenzyme A desaturase 1 (SCD-1), are detected to be significantly up-regulated in various human cancers (125–128). According to further analysis, we found that these enzymes connected to de novo adipogenesis can be effectively regulated by AhR. The expression level of SCD-1 can be attenuated by inhibiting the expression of AhR (129). Indole-3-acetic acid (IAA)-activated AhR negatively regulates SREBP-1c and FASN (130). In colon tumor cells, inhibition of AhR activity decreases the expression levels of SCD-1, a key enzyme of the biosynthetic pathway, and SREBP, a transcriptional regulator of adipogenesis, to restrict cancer cell proliferation in a cell-specific manner (73). Although there has been very limit research on AhR’s role in tumor lipid metabolism, it is found that AhR influences many of the genes that are up-regulated in tumors and are linked to lipid metabolism regulation, including CD36, SCD-1, SREBP, and FASN. Therefore, AhR has great potential in regulating tumor lipid metabolism and worth more further investigation.
6 AhR, UA, and ROS are jointly involved in the adaptive regulation of TME by tumors
By integrating metabolomics and transcriptomics, it has been discovered that AhR regulates MYC expression to modulate glycolysis and de novo pyrimidine production in the cells. Metabolomics data also showed a decrease in uridine monophosphate (UMP), and AhR silencing resulted in decreased expression and translation of genes related to pyrimidine ab initio synthesis (21). No previous study has reported whether purine metabolism in nucleic acids is related to AhR, however, we found that AhR may play important roles in regulating nucleotide metabolism.
UA is a metabolite of purines, and a large number of clinical studies have shown that UA is associated with tumorigenesis and progression and has received wide attention. Breast cancer cell proliferation and migration have been reported to be affected by increased de novo nucleotide synthesis, and UA was used to assess the prognosis of patients with breast cancer and as a feedback regulator of signaling pathways (69). Serum hyperuric acid (SUA) has been shown to affect the course of treatment and prognosis of patients with cancer, and prospective studies have reported that SUA increases the probability of cancer-induced mortality (131–134). In contrast, clinical evidence from some regions suggests that UA exhibits an anticancer effect due to its antioxidant activity (135–138), and fundamental research revealed that UA exerts anticancer effects by promoting dendritic cell maturation, which thereafter triggers an immune rejection response against tumors (139). Reportedly, UA exerts antioxidant effects in extracellular environments but exerts pro-oxidant effects in intracellular environments. UA may function as an antioxidant, scavenge oxygen free radicals, and reduce the production of carcinogenic ROS, which increases the mutation rate of cells, thereby increasing the risk of carcinogenesis, indicating that targeting UA may lower the risk of cancer (38, 140). However, by functioning as a pro-oxidant, UA may penetrate normal cells and contribute to cancer progression by enhancing tumor cell proliferation, migration, and survival through ROS and inflammatory stress (141).
According to certain theories, AhR can regulate the transcription of some CYPs, which can mediate AhR to generate ROS, and AhR is crucial for the burst of ROS that occurs following reoxygenation (142). After activation, AhR translocates from its inhibitor proteins to the nucleus and actively forms heterodimers with ARNT (143, 144). The transcription of genes, including CYP1A, NADPH oxidase 2, P40phox, and P47phox, is stimulated by the binding of these complex proteins to dioxin- or xenobiotic-responsive elements, which is a key step in the development of oxidative stress-mediated breast cancer (145). An imbalance between ROS production and antioxidant scavenging activity induces oxidative stress (146, 147). Additionally, it has been reported that pentachlorobiphenyl stimulates NADPH oxidase via AhR, thereby increasing ROS generation (71). Cell cycle protein-dependent kinase inhibitor 1 B (p27kip1) coordinates cell cycle progression by inhibiting cyclin-dependent kinase complexes (148). AhR was established as a direct regulator of p27Kip1 transcription. AhR activation in hepatocellular carcinoma upregulates p27kip1, which suppresses hepatocellular carcinoma cell proliferation (149) (Figure 3). Recently, it has been found that ROS can activate RSR signaling to participate in the metabolic regulation of cells (74).
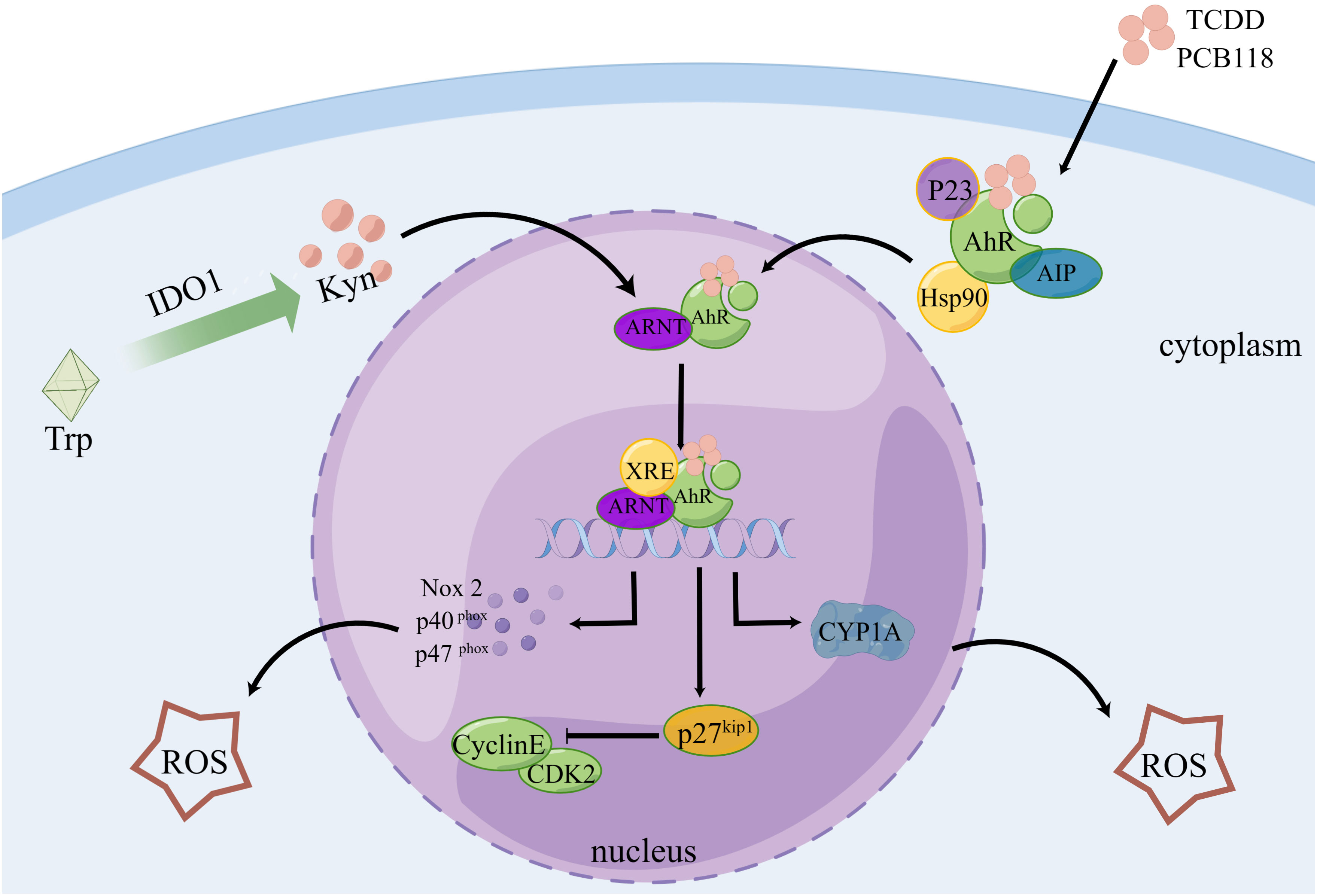
Figure 3 AhR controls the cell cycle and produces ROS. Following TCDD and PCB118 activation of the AhR complex with P23, AIP, Hsp90, etc., AhR is transported to the nucleus where it binds to ARNT to form a heterodimer. This heterodimer then binds to XRE to activate the expression of XRE-controlled genes like CYP1A, Nox2, P27Kip1, etc. ROS are regulated by CYP1A, Nox2, P40, P47, and other proteins, and P27Kip1 can block the cell cycle. Additionally, Kyn, a byproduct of IDO1-controlled tryptophan metabolism, plays a role in AhR regulation.
Indirect oxidative damage to DNA and free radicals in the cellular and mitochondrial dNTP pools can result from redox regulation dysfunction and increased ROS levels (150), and the incorporation of these oxidized nucleotides into DNA synthesis can result in mismatches, mutations, and cell death (151, 152). Eukaryotic cells have two functionally distinct dNTP pools. The smaller pool is used for mitochondrial DNA replication and is available throughout the cell cycle, whereas the other pool is used for genomic DNA replication and repair in the nucleus and is available primarily during the S phase (67). The human MutT homolog 1 (MTH1) protein is required for the effective survival of cancer cells, but not for normal noncancerous cells. When oxidized dNTPs are present in cancer cells due to oxidative stress and excessive ROS under harsh conditions, MTH1 overexpression prevents DNA damage induced by oxidized nucleotides during replication. Owing to the strict redox control in normal cells than in cancer cells, normal cells may appear less dependent on MTH 1 activity (153). In summary, MTH1 activity facilitates the alleviation of DNA damage in tumor cells via AhR-mediated ROS production (Figure 4).
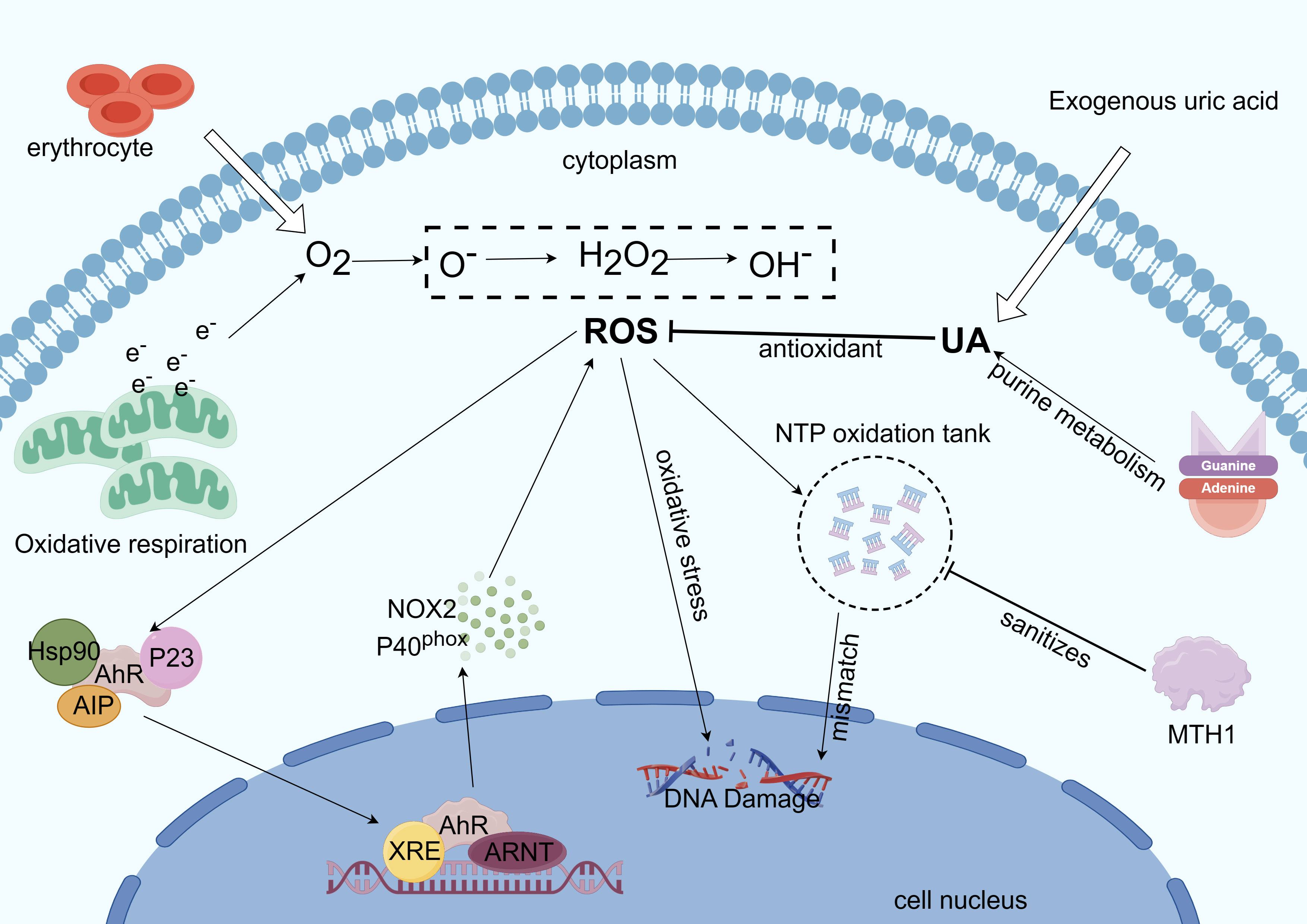
Figure 4 Mechanism of AhR involvement in intracellular ROS regulation. Excess ROS is produced by mitochondrial metabolism when electrons are overworked (32). ROS also form NTP oxidation pools, dope oxidized dNTPs, and cause oxidative stress, which damages DNA during tumor cell replication. ROS can also trigger the transcription of genes like CYPs and NOX2 to react with the ROS (19). Reactive oxygen species generation is suppressed by UA’s antioxidant action. Tumor cell growth benefits from base mismatch reduction, mutation reduction, and purification of the NTP oxidation pool—all of which are achieved by MTH1 (153).
7 Conclusion and perspective
In conclusion, AhR is crucial in the regulation of cellular metabolism, especially in tumor metabolic reprogramming. Many study has been focus on glucose metabolism, but no unified conclusions are achieved on whether AhR upregulation promotes or inhibits glucose metabolism (23, 72). In terms of lipid metabolism, the involvement of AhR in tumor lipid metabolism has been reported only in colon cancer (73). However, we found that AhR pays significant roles in the regulation of genes related to lipid metabolism changes in a variety of cancer cells. These findings highlight the pivotal role of AhR in metabolic control. Unfortunately, no great advancement has been made in research on nucleotide metabolism in initial clinical tumor therapy trials targeting AhR.
Many studies have revealed that AhR may control ROS in the TME, creating an environment favorable for tumor growth and migration, and even affecting cancer treatment (41). From the above, we can draw various conclusions. The regulatory function of AhR in cell glucose, lipid, and nucleotide metabolism is undeniably present and can aid tumor immune escape by modifying immune cells in the TME. While tumor metabolic reprogramming helps cancer cells proliferate quickly, it also unavoidably produces a harsh TME, as indicated by increased ROS levels. Although excess ROS accumulation is harmful to cell proliferation, AhR can detect excess ROS and trigger the production of antioxidant proteins, thereby shielding cancer cells from oxidative stress. The effects of ROS on tumor cells can also be mitigated by MTH1-mediated cleansing of oxidized dNTPs (153). UA, the final byproduct of human purine metabolism, exhibits antioxidant properties (38) and reduces intracellular ROS, which in turn reduces AhR activation and downregulates P27kip1, promoting tumor cell cycle and progression (Figure 4). In conclusion, AhR has great potential to regulate tumor metabolic reprogramming by sensing changes in the TME.
Author contributions
ZW: Writing – original draft. YZ: Funding acquisition, Writing – review & editing. ZL: Writing – review & editing. MH: Writing – review & editing. XS: Funding acquisition, Supervision, Writing – review & editing.
Funding
The author(s) declare financial support was received for the research, authorship, and/or publication of this article. The present study was supported by the Characteristic Innovation Projects of Universities in Guangdong Province (grant no. 2021KTSCX037) and Zhanjiang Science and Technology Project (grant nos. 2020A06004, 2021A05056 and 2022A01190).
Acknowledgments
We thank Figdraw (www.figdraw.com) for figure drawing.
Conflict of interest
The authors declare that the research was conducted in the absence of any commercial or financial relationships that could be construed as a potential conflict of interest.
Publisher’s note
All claims expressed in this article are solely those of the authors and do not necessarily represent those of their affiliated organizations, or those of the publisher, the editors and the reviewers. Any product that may be evaluated in this article, or claim that may be made by its manufacturer, is not guaranteed or endorsed by the publisher.
References
1. Sung H, Ferlay J, Siegel RL, Laversanne M, Soerjomataram I, Jemal A, et al. Global cancer statistics 2020: GLOBOCAN estimates of incidence and mortality worldwide for 36 cancers in 185 countries. CA Cancer J Clin. (2021) 71:209–49. doi: 10.3322/caac.21660
2. Boroughs LK, DeBerardinis RJ. Metabolic pathways promoting cancer cell survival and growth. Nat Cell Biol. (2015) 17:351–9. doi: 10.1038/ncb3124
3. Wang Q, Wang P, Qin Z, Yang X, Pan B, Nie F, et al. Altered glucose metabolism and cell function in keloid fibroblasts under hypoxia. Redox Biol. (2021) 38:101815. doi: 10.1016/j.redox.2020.101815
4. Pavlova NN, Zhu J, Thompson CB. The hallmarks of cancer metabolism: Still emerging. Cell Metab. (2022) 34:355–77. doi: 10.1016/j.cmet.2022.01.007
5. Faubert B, Solmonson A, DeBerardinis RJ. Metabolic reprogramming and cancer progression. Science. (2020) 368:eaaw5473. doi: 10.1126/science.aaw5473
6. Liu T, Wang Z, Ye L, Duan Y, Jiang H, He H, et al. Nucleus-exported CLOCK acetylates PRPS to promote de novo nucleotide synthesis and liver tumour growth. Nat Cell Biol. (2023) 25:273–84. doi: 10.1038/s41556-022-01061-0
7. More TH, RoyChoudhury S, Christie J, Taunk K, Mane A, Santra MK, et al. Metabolomic alterations in invasive ductal carcinoma of breast: A comprehensive metabolomic study using tissue and serum samples. Oncotarget. (2018) 9:2678–96. doi: 10.18632/oncotarget.23626
8. Tayyari F, Gowda GAN, Olopade OF, Berg R, Yang HH, Lee MP, et al. Metabolic profiles of triple-negative and luminal A breast cancer subtypes in African-American identify key metabolic differences. Oncotarget. (2018) 9:11677–90. doi: 10.18632/oncotarget.24433
9. Dias AS, Almeida CR, Helguero LA, Duarte IF. Metabolic crosstalk in the breast cancer microenvironment. Eur J Cancer. (2019) 121:154–71. doi: 10.1016/j.ejca.2019.09.002
10. Bahrami A, Hassanian SM, Khazaei M, Hasanzadeh M, Shahidsales S, Maftouh M, et al. The therapeutic potential of targeting tumor microenvironment in breast cancer: rational strategies and recent progress. J Cell Biochem. (2018) 119:111–22. doi: 10.1002/jcb.26183
11. Takenaka MC, Gabriely G, Rothhammer V, Mascanfroni ID, Wheeler MA, Chao C-C, et al. Control of tumor-associated macrophages and T cells in glioblastoma via AHR and CD39. Nat Neurosci. (2019) 22:729–40. doi: 10.1038/s41593-019-0370-y
12. McKay ZP, Brown MC, Gromeier M. Aryl hydrocarbon receptor signaling controls CD155 expression on macrophages and mediates tumor immunosuppression. J Immunol. (2021) 206:1385–94. doi: 10.4049/jimmunol.2000792
13. Campesato LF, Budhu S, Tchaicha J, Weng C-H, Gigoux M, Cohen IJ, et al. Blockade of the AHR restricts a Treg-macrophage suppressive axis induced by L-Kynurenine. Nat Commun. (2020) 11:4011. doi: 10.1038/s41467-020-17750-z
14. Chen Y, Guillemin GJ. Kynurenine pathway metabolites in humans: disease and healthy States. Int J Tryptophan Res. (2009) 2:1–19. doi: 10.4137/ijtr.s2097
15. Adams S, Teo C, McDonald KL, Zinger A, Bustamante S, Lim CK, et al. Involvement of the kynurenine pathway in human glioma pathophysiology. PloS One. (2014) 9:e112945. doi: 10.1371/journal.pone.0112945
16. Shu-Chuin C, Fanidi A, Ueland PM, Relton C, Midttun O, Vollset SE, et al. Circulating biomarkers of tryptophan and the kynurenine pathway and lung cancer risk. Cancer epidemiology Biomarkers prevention : Publ Am Assoc Cancer Research cosponsored by Am Soc Prev Oncol. (2014) 23:461–8. doi: 10.1158/1055-9965.EPI-13-0770
17. Rad Pour S, Morikawa H, Kiani NA, Yang M, Azimi A, Shafi G, et al. Exhaustion of CD4+ T-cells mediated by the kynurenine pathway in melanoma. Sci Rep. (2019) 9:12150. doi: 10.1038/s41598-019-48635-x
18. Venkateswaran N, Lafita-Navarro MC, Hao Y-H, Kilgore JA, Perez-Castro L, Braverman J, et al. MYC promotes tryptophan uptake and metabolism by the kynurenine pathway in colon cancer. Genes Dev. (2019) 33:1236–51. doi: 10.1101/gad.327056.119
19. Kubli SP, Bassi C, Roux C, Wakeham A, Göbl C, Zhou W, et al. AhR controls redox homeostasis and shapes the tumor microenvironment in BRCA1-associated breast cancer. Proc Natl Acad Sci U.S.A. (2019) 116:3604–13. doi: 10.1073/pnas.1815126116
20. Murray IA, Patterson AD, Perdew GH. Aryl hydrocarbon receptor ligands in cancer: friend and foe. Nat Rev Cancer. (2014) 14:801–14. doi: 10.1038/nrc3846
21. Lafita-Navarro MC, Perez-Castro L, Zacharias LG, Barnes S, DeBerardinis RJ, Conacci-Sorrell M. The transcription factors aryl hydrocarbon receptor and MYC cooperate in the regulation of cellular metabolism. J Biol Chem. (2020) 295:12398–407. doi: 10.1074/jbc.AC120.014189
22. Huang T, Song J, Gao J, Cheng J, Xie H, Zhang L, et al. Adipocyte-derived kynurenine promotes obesity and insulin resistance by activating the AhR/STAT3/IL-6 signaling. Nat Commun. (2022) 13:3489. doi: 10.1038/s41467-022-31126-5
23. Lee R, Li J, Li J, Wu C-J, Jiang S, Hsu W-H, et al. Synthetic essentiality of tryptophan 2,3-dioxygenase 2 in APC-mutated colorectal cancer. Cancer Discovery. (2022) 12:1702–17. doi: 10.1158/2159-8290.CD-21-0680
24. Opitz CA, Litzenburger UM, Sahm F, Ott M, Tritschler I, Trump S, et al. An endogenous tumour-promoting ligand of the human aryl hydrocarbon receptor. Nature. (2011) 478:197–203. doi: 10.1038/nature10491
25. Piwarski SA, Thompson C, Chaudhry AR, Denvir J, Primerano DA, Fan J, et al. The putative endogenous AHR ligand ITE reduces JAG1 and associated NOTCH1 signaling in triple negative breast cancer cells. Biochem Pharmacol. (2020) 174:113845. doi: 10.1016/j.bcp.2020.113845
26. Ikuta T, Kurosumi M, Yatsuoka T, Nishimura Y. Tissue distribution of aryl hydrocarbon receptor in the intestine: Implication of putative roles in tumor suppression. Exp Cell Res. (2016) 343:126–34. doi: 10.1016/j.yexcr.2016.03.012
27. Han H, Davidson LA, Hensel M, Yoon G, Landrock K, Allred C, et al. Loss of aryl hydrocarbon receptor promotes colon tumorigenesis in apcS580/+; krasG12D/+ Mice. Mol Cancer research : MCR. (2021) 19:771–83. doi: 10.1158/1541-7786.MCR-20-0789
28. Chen W, Wen L, Bao Y, Tang Z, Zhao J, Zhang X, et al. Gut flora disequilibrium promotes the initiation of liver cancer by modulating tryptophan metabolism and up-regulating SREBP2. Proc Natl Acad Sci U.S.A. (2022) 119:e2203894119. doi: 10.1073/pnas.2203894119
29. Ye G, Gao H, Zhang X, Liu X, Chen J, Liao X, et al. Aryl hydrocarbon receptor mediates benzo[a]pyrene-induced metabolic reprogramming in human lung epithelial BEAS-2B cells. Sci Total Environ. (2021) 756:144130. doi: 10.1016/j.scitotenv.2020.144130
30. Skulachev VP. Role of uncoupled and non-coupled oxidations in maintenance of safely low levels of oxygen and its one-electron reductants. Q Rev Biophys. (1996) 29:169–202. doi: 10.1017/s0033583500005795
31. Boveris A, Chance B. The mitochondrial generation of hydrogen peroxide. General properties and effect of hyperbaric oxygen. Biochem J. (1973) 134:707–16. doi: 10.1042/bj1340707
32. Andreyev AY, Kushnareva YE, Starkov AA. Mitochondrial metabolism of reactive oxygen species. Biochem (Mosc). (2005) 70:200–14. doi: 10.1007/s10541-005-0102-7
33. Irani K, Xia Y, Zweier JL, Sollott SJ, Der CJ, Fearon ER, et al. Mitogenic signaling mediated by oxidants in Ras-transformed fibroblasts. Science. (1997) 275:1649–52. doi: 10.1126/science.275.5306.1649
34. Chandel NS, Maltepe E, Goldwasser E, Mathieu CE, Simon MC, Schumacker PT. Mitochondrial reactive oxygen species trigger hypoxia-induced transcription. Proc Natl Acad Sci U.S.A. (1998) 95:11715–20. doi: 10.1073/pnas.95.20.11715
35. Cheung EC, Lee P, Ceteci F, Nixon C, Blyth K, Sansom OJ, et al. Opposing effects of TIGAR- and RAC1-derived ROS on Wnt-driven proliferation in the mouse intestine. Genes Dev. (2016) 30:52–63. doi: 10.1101/gad.271130.115
36. Finkel T. From sulfenylation to sulfhydration: what a thiolate needs to tolerate. Sci Signal. (2012) 5:pe10. doi: 10.1126/scisignal.2002943
37. Weinberg F, Hamanaka R, Wheaton WW, Weinberg S, Joseph J, Lopez M, et al. Mitochondrial metabolism and ROS generation are essential for Kras-mediated tumorigenicity. Proc Natl Acad Sci U.S.A. (2010) 107:8788–93. doi: 10.1073/pnas.1003428107
38. Ames BN, Cathcart R, Schwiers E, Hochstein P. Uric acid provides an antioxidant defense in humans against oxidant- and radical-caused aging and cancer: a hypothesis. Proc Natl Acad Sci U.S.A. (1981) 78:6858–62. doi: 10.1073/pnas.78.11.6858
39. Ren F, Ji C, Huang Y, Aniagu S, Jiang Y, Chen T. AHR-mediated ROS production contributes to the cardiac developmental toxicity of PM2.5 in zebrafish embryos. Sci Total Environ. (2020) 719:135097. doi: 10.1016/j.scitotenv.2019.135097
40. Huang Y, Zhang J, Tao Y, Ji C, Aniagu S, Jiang Y, et al. AHR/ROS-mediated mitochondria apoptosis contributes to benzo[a]pyrene-induced heart defects and the protective effects of resveratrol. Toxicology. (2021) 462:152965. doi: 10.1016/j.tox.2021.152965
41. Zhan Y, Zhang Z, Liu Y, Fang Y, Xie Y, Zheng Y, et al. NUPR1 contributes to radiation resistance by maintaining ROS homeostasis via AhR/CYP signal axis in hepatocellular carcinoma. BMC Med. (2022) 20:365. doi: 10.1186/s12916-022-02554-3
42. Arcucci A, Ruocco MR, Granato G, Sacco AM, Montagnani S. Cancer: an oxidative crosstalk between solid tumor cells and cancer associated fibroblasts. BioMed Res Int. (2016) 2016:4502846. doi: 10.1155/2016/4502846
43. Cirillo F, Lappano R, Bruno L, Rizzuti B, Grande F, Guzzi R, et al. AHR and GPER mediate the stimulatory effects induced by 3-methylcholanthrene in breast cancer cells and cancer-associated fibroblasts (CAFs). J Exp Clin Cancer Res. (2019) 38:335. doi: 10.1186/s13046-019-1337-2
44. Chen L-B, Zhu S-P, Liu T-P, Zhao H, Chen P-F, Duan Y-J, et al. Cancer associated fibroblasts promote renal cancer progression through a TDO/kyn/ahR dependent signaling pathway. Front Oncol. (2021) 11:628821. doi: 10.3389/fonc.2021.628821
45. Feng H, Cao B, Peng X, Wei Q. Cancer-associated fibroblasts strengthen cell proliferation and EGFR TKIs resistance through aryl hydrocarbon receptor dependent signals in non-small cell lung cancer. BMC Cancer. (2022) 22:764. doi: 10.1186/s12885-022-09877-7
46. Domingo-Vidal M, Whitaker-Menezes D, Martos-Rus C, Tassone P, Snyder CM, Tuluc M, et al. Cigarette smoke induces metabolic reprogramming of the tumor stroma in head and neck squamous cell carcinoma. Mol Cancer Res. (2019) 17:1893–909. doi: 10.1158/1541-7786.MCR-18-1191
47. Gutiérrez-Vázquez C, Quintana FJ. Regulation of the immune response by the aryl hydrocarbon receptor. Immunity. (2018) 48:19–33. doi: 10.1016/j.immuni.2017.12.012
48. Hezaveh K, Shinde RS, Klötgen A, Halaby MJ, Lamorte S, Ciudad MT, et al. Tryptophan-derived microbial metabolites activate the aryl hydrocarbon receptor in tumor-associated macrophages to suppress anti-tumor immunity. Immunity. (2022) 55:324–340.e8. doi: 10.1016/j.immuni.2022.01.006
50. Hanahan D, Weinberg RA. Hallmarks of cancer: the next generation. Cell. (2011) 144:646–74. doi: 10.1016/j.cell.2011.02.013
51. Scharping NE, Menk AV, Moreci RS, Whetstone RD, Dadey RE, Watkins SC, et al. The tumor microenvironment represses T cell mitochondrial biogenesis to drive intratumoral T cell metabolic insufficiency and dysfunction. Immunity. (2016) 45:374–88. doi: 10.1016/j.immuni.2016.07.009
52. Najjar YG, Menk AV, Sander C, Rao U, Karunamurthy A, Bhatia R, et al. Tumor cell oxidative metabolism as a barrier to PD-1 blockade immunotherapy in melanoma. JCI Insight. (2019) 4:e124989. doi: 10.1172/jci.insight.124989
53. Angelin A, Gil-de-Gómez L, Dahiya S, Jiao J, Guo L, Levine MH, et al. Foxp3 reprograms T cell metabolism to function in low-glucose, high-lactate environments. Cell Metab. (2017) 25:1282–1293.e7. doi: 10.1016/j.cmet.2016.12.018
54. Chen P, Zuo H, Xiong H, Kolar MJ, Chu Q, Saghatelian A, et al. Gpr132 sensing of lactate mediates tumor-macrophage interplay to promote breast cancer metastasis. Proc Natl Acad Sci U.S.A. (2017) 114:580–5. doi: 10.1073/pnas.1614035114
55. Weinhouse S. On respiratory impairment in cancer cells. Science. (1956) 124:267–9. doi: 10.1126/science.124.3215.267
56. Wang JX, Choi SYC, Niu X, Kang N, Xue H, Killam J, et al. Lactic acid and an acidic tumor microenvironment suppress anticancer immunity. Int J Mol Sci. (2020) 21:8363. doi: 10.3390/ijms21218363
57. Kumagai S, Koyama S, Itahashi K, Tanegashima T, Lin Y-T, Togashi Y, et al. Lactic acid promotes PD-1 expression in regulatory T cells in highly glycolytic tumor microenvironments. Cancer Cell. (2022) 40:201–218.e9. doi: 10.1016/j.ccell.2022.01.001
58. Han Z, Gong C, Li J, Guo H, Chen X, Jin Y, et al. Immunologically modified enzyme-responsive micelles regulate the tumor microenvironment for cancer immunotherapy. Mater Today Bio. (2022) 13:100170. doi: 10.1016/j.mtbio.2021.100170
59. Wang J, Ye C, Chen C, Xiong H, Xie B, Zhou J, et al. Glucose transporter GLUT1 expression and clinical outcome in solid tumors: a systematic review and meta-analysis. Oncotarget. (2017) 8:16875–86. doi: 10.18632/oncotarget.15171
60. Mattaini KR, Sullivan MR, Vander Heiden MG. The importance of serine metabolism in cancer. J Cell Biol. (2016) 214:249–57. doi: 10.1083/jcb.201604085
61. Yang M, Vousden KH. Serine and one-carbon metabolism in cancer. Nat Rev Cancer. (2016) 16:650–62. doi: 10.1038/nrc.2016.81
62. Menendez JA, Lupu R. Fatty acid synthase (FASN) as a therapeutic target in breast cancer. Expert Opin Ther Targets. (2017) 21:1001–16. doi: 10.1080/14728222.2017.1381087
63. Li S, Yu J, Huber A, Kryczek I, Wang Z, Jiang L, et al. Metabolism drives macrophage heterogeneity in the tumor microenvironment. Cell Rep. (2022) 39:110609. doi: 10.1016/j.celrep.2022.110609
64. Koikawa K, Kibe S, Suizu F, Sekino N, Kim N, Manz TD, et al. Targeting Pin1 renders pancreatic cancer eradicable by synergizing with immunochemotherapy. Cell. (2021) 184:4753–4771.e27. doi: 10.1016/j.cell.2021.07.020
65. Jia Y, Guo J, Zhao Y, Zhang Z, Shi L, Fang Y, et al. AHR signaling pathway reshapes the metabolism of AML/MDS cells and potentially leads to cytarabine resistance. Acta Biochim Biophys Sin (Shanghai). (2021) 53:492–500. doi: 10.1093/abbs/gmab017
66. Stukas D, Jasukaitiene A, Bartkeviciene A, Matthews J, Maimets T, Teino I, et al. Targeting AHR increases pancreatic cancer cell sensitivity to gemcitabine through the ELAVL1-DCK pathway. Int J Mol Sci. (2023) 24:13155. doi: 10.3390/ijms241713155
67. Mathews CK. Deoxyribonucleotide metabolism, mutagenesis and cancer. Nat Rev Cancer. (2015) 15:528–39. doi: 10.1038/nrc3981
68. Mathews CK. DNA synthesis as a therapeutic target: the first 65 years. FASEB J. (2012) 26:2231–7. doi: 10.1096/fj.12-0602ufm
69. Lv Y, Wang X, Li X, Xu G, Bai Y, Wu J, et al. Nucleotide de novo synthesis increases breast cancer stemness and metastasis via cGMP-PKG-MAPK signaling pathway. PloS Biol. (2020) 18:e3000872. doi: 10.1371/journal.pbio.3000872
70. Pedley AM, Benkovic SJ. A new view into the regulation of purine metabolism: the purinosome. Trends Biochem Sci. (2017) 42:141–54. doi: 10.1016/j.tibs.2016.09.009
71. Liang W, Zhang Y, Song L, Li Z. 2,3’4,4’,5-Pentachlorobiphenyl induces hepatocellular carcinoma cell proliferation through pyruvate kinase M2-dependent glycolysis. Toxicol Lett. (2019) 313:108–19. doi: 10.1016/j.toxlet.2019.06.006
72. Yan T, Shen C, Jiang P, Yu C, Guo F, Tian X, et al. Risk SNP-induced lncRNA-SLCC1 drives colorectal cancer through activating glycolysis signaling. Signal Transduct Target Ther. (2021) 6:70. doi: 10.1038/s41392-020-00446-7
73. Karasová M, Procházková J, Tylichová Z, Fedr R, Ciganek M, Machala M, et al. Inhibition of aryl hydrocarbon receptor (AhR) expression disrupts cell proliferation and alters energy metabolism and fatty acid synthesis in colon cancer cells. Cancers (Basel). (2022) 14:4245. doi: 10.3390/cancers14174245
74. Snieckute G, Ryder L, Vind AC, Wu Z, Arendrup FS, Stoneley M, et al. ROS-induced ribosome impairment underlies ZAKα-mediated metabolic decline in obesity and aging. Science. (2023) 382:eadf3208. doi: 10.1126/science.adf3208
75. Shinde R, McGaha TL. The aryl hydrocarbon receptor: connecting immunity to the microenvironment. Trends Immunol. (2018) 39:1005–20. doi: 10.1016/j.it.2018.10.010
76. Liu Y, Liang X, Dong W, Fang Y, Lv J, Zhang T, et al. Tumor-repopulating cells induce PD-1 expression in CD8+ T cells by transferring kynurenine and ahR activation. Cancer Cell. (2018) 33:480–494.e7. doi: 10.1016/j.ccell.2018.02.005
77. Sadik A, Somarribas Patterson LF, Öztürk S, Mohapatra SR, Panitz V, Secker PF, et al. IL4I1 is a metabolic immune checkpoint that activates the AHR and promotes tumor progression. Cell. (2020) 182:1252–1270.e34. doi: 10.1016/j.cell.2020.07.038
78. Fan Y, Boivin GP, Knudsen ES, Nebert DW, Xia Y, Puga A. The aryl hydrocarbon receptor functions as a tumor suppressor of liver carcinogenesis. Cancer Res. (2010) 70:212–20. doi: 10.1158/0008-5472.CAN-09-3090
79. Weaver BAA, Cleveland DW. The role of aneuploidy in promoting and suppressing tumors. J Cell Biol. (2009) 185:935–7. doi: 10.1083/jcb.200905098
80. Sári Z, Mikó E, Kovács T, Jankó L, Csonka T, Lente G, et al. Indolepropionic acid, a metabolite of the microbiome, has cytostatic properties in breast cancer by activating AHR and PXR receptors and inducing oxidative stress. Cancers (Basel). (2020) 12:2411. doi: 10.3390/cancers12092411
81. Zhang S, Lei P, Liu X, Li X, Walker K, Kotha L, et al. The aryl hydrocarbon receptor as a target for estrogen receptor-negative breast cancer chemotherapy. Endocr Relat Cancer. (2009) 16:835–44. doi: 10.1677/ERC-09-0054
82. Spink BC, Bennett JA, Lostritto N, Cole JR, Spink DC. Expression of the aryl hydrocarbon receptor is not required for the proliferation, migration, invasion, or estrogen-dependent tumorigenesis of MCF-7 breast cancer cells. Mol Carcinog. (2013) 52:544–54. doi: 10.1002/mc.21889
83. Yoshioka H, Hiromori Y, Aoki A, Kimura T, Fujii-Kuriyama Y, Nagase H, et al. Possible aryl hydrocarbon receptor-independent pathway of 2,3,7,8-tetrachlorodibenzo-p-dioxin-induced antiproliferative response in human breast cancer cells. Toxicol Lett. (2012) 211:257–65. doi: 10.1016/j.toxlet.2012.04.005
84. Luecke-Johansson S, Gralla M, Rundqvist H, Ho JC, Johnson RS, Gradin K, et al. A molecular mechanism to switch the aryl hydrocarbon receptor from a transcription factor to an E3 ubiquitin ligase. Mol Cell Biol. (2017) 37:e00630–16. doi: 10.1128/MCB.00630-16
85. Hall JM, Barhoover MA, Kazmin D, McDonnell DP, Greenlee WF, Thomas R. Activation of the aryl-hydrocarbon receptor inhibits invasive and metastatic features of human breast cancer cells and promotes breast cancer cell differentiation. S. Mol Endocrinol. (2010) 24:359–69. doi: 10.1210/me.2009-0346
86. Gd G, Br B, Hc M, Ml F, Se E. Knockdown of aberrantly upregulated aryl hydrocarbon receptor reduces tumor growth and metastasis of MDA-MB-231 human breast cancer cell line. Int J Cancer. (2013) 133:2769–80. doi: 10.1002/ijc.28297
87. Goode G, Pratap S, Eltom SE. Depletion of the aryl hydrocarbon receptor in MDA-MB-231 human breast cancer cells altered the expression of genes in key regulatory pathways of cancer. PloS One. (2014) 9:e100103. doi: 10.1371/journal.pone.0100103
88. Baker JR, Sakoff JA, McCluskey A. The aryl hydrocarbon receptor (AhR) as a breast cancer drug target. Med Res Rev. (2020) 40:972–1001. doi: 10.1002/med.21645
89. Vogel CFA, Sciullo E, Li W, Wong P, Lazennec G, Matsumura F. RelB, a new partner of aryl hydrocarbon receptor-mediated transcription. Mol Endocrinol. (2007) 21:2941–55. doi: 10.1210/me.2007-0211
90. Kim DW, Gazourian L, Quadri SA, Romieu-Mourez R, Sherr DH, Sonenshein GE. The RelA NF-kappaB subunit and the aryl hydrocarbon receptor (AhR) cooperate to transactivate the c-myc promoter in mammary cells. Oncogene. (2000) 19:5498–506. doi: 10.1038/sj.onc.1203945
91. Giannelli G, Koudelkova P, Dituri F, Mikulits W. Role of epithelial to mesenchymal transition in hepatocellular carcinoma. J Hepatol. (2016) 65:798–808. doi: 10.1016/j.jhep.2016.05.007
92. Yang S, Liu Y, Li M-Y, Ng CSH, Yang S-L, Wang S, et al. FOXP3 promotes tumor growth and metastasis by activating Wnt/β-catenin signaling pathway and EMT in non-small cell lung cancer. Mol Cancer. (2017) 16:124. doi: 10.1186/s12943-017-0700-1
93. Pierre S, Chevallier A, Teixeira-Clerc F, Ambolet-Camoit A, Bui L-C, Bats A-S, et al. Aryl hydrocarbon receptor-dependent induction of liver fibrosis by dioxin. Toxicol Sci. (2014) 137:114–24. doi: 10.1093/toxsci/kft236
94. Song L, Guo L, Li Z. Molecular mechanisms of 3,3’4,4’,5-pentachlorobiphenyl-induced epithelial-mesenchymal transition in human hepatocellular carcinoma cells. Toxicol Appl Pharmacol. (2017) 322:75–88. doi: 10.1016/j.taap.2017.03.003
95. Moretti S, Nucci N, Menicali E, Morelli S, Bini V, Colella R, et al. The aryl hydrocarbon receptor is expressed in thyroid carcinoma and appears to mediate epithelial-mesenchymal-transition. Cancers (Basel). (2020) 12:145. doi: 10.3390/cancers12010145
96. Dai G, Chen X, He Y. The gut microbiota activates ahR through the tryptophan metabolite kyn to mediate renal cell carcinoma metastasis. Front Nutr. (2021) 8:712327. doi: 10.3389/fnut.2021.712327
97. Cui N, Hu M, Khalil RA. Biochemical and biological attributes of matrix metalloproteinases. Prog Mol Biol Transl Sci. (2017) 147:1–73. doi: 10.1016/bs.pmbts.2017.02.005
98. Smith BN, Bhowmick NA. Role of EMT in metastasis and therapy resistance. J Clin Med. (2016) 5:17. doi: 10.3390/jcm5020017
99. Zhu P, Zhou K, Lu S, Bai Y, Qi R, Zhang S. Modulation of aryl hydrocarbon receptor inhibits esophageal squamous cell carcinoma progression by repressing COX2/PGE2/STAT3 axis. J Cell Commun Signal. (2020) 14:175–92. doi: 10.1007/s12079-019-00535-5
100. Haque M, Francis J, Sehgal I. Aryl hydrocarbon exposure induces expression of MMP-9 in human prostate cancer cell lines. Cancer Lett. (2005) 225:159–66. doi: 10.1016/j.canlet.2004.11.043
102. Peng T-L, Chen J, Mao W, Song X, Chen M-H. Aryl hydrocarbon receptor pathway activation enhances gastric cancer cell invasiveness likely through a c-Jun-dependent induction of matrix metalloproteinase-9. BMC Cell Biol. (2009) 10:27. doi: 10.1186/1471-2121-10-27
103. Ishida M, Mikami S, Kikuchi E, Kosaka T, Miyajima A, Nakagawa K, et al. Activation of the aryl hydrocarbon receptor pathway enhances cancer cell invasion by upregulating the MMP expression and is associated with poor prognosis in upper urinary tract urothelial cancer. Carcinogenesis. (2010) 31:287–95. doi: 10.1093/carcin/bgp222
104. Li L, Wang T, Li S, Chen Z, Wu J, Cao W, et al. TDO2 promotes the EMT of hepatocellular carcinoma through kyn-ahR pathway. Front Oncol. (2020) 10:562823. doi: 10.3389/fonc.2020.562823
105. Zhu P, Yu H, Zhou K, Bai Y, Qi R, Zhang S. 3,3’-Diindolylmethane modulates aryl hydrocarbon receptor of esophageal squamous cell carcinoma to reverse epithelial-mesenchymal transition through repressing RhoA/ROCK1-mediated COX2/PGE2 pathway. J Exp Clin Cancer Res. (2020) 39:113. doi: 10.1186/s13046-020-01618-7
106. Therachiyil L, Krishnankutty R, Ahmad F, Mateo JM, Uddin S, Korashy HM. Aryl hydrocarbon receptor promotes cell growth, stemness like characteristics, and metastasis in human ovarian cancer via activation of PI3K/akt, β-catenin, and epithelial to mesenchymal transition pathways. Int J Mol Sci. (2022) 23:6395. doi: 10.3390/ijms23126395
107. Tsai C-H, Li C-H, Cheng Y-W, Lee C-C, Liao P-L, Lin C-H, et al. The inhibition of lung cancer cell migration by AhR-regulated autophagy. Sci Rep. (2017) 7:41927. doi: 10.1038/srep41927
108. Xu H, Hu C, Wang Y, Shi Y, Yuan L, Xu J, et al. Glutathione peroxidase 2 knockdown suppresses gastric cancer progression and metastasis via regulation of kynurenine metabolism. Oncogene. (2023) 42:1994–2006. doi: 10.1038/s41388-023-02708-4
109. Miyazaki T, Chung S, Sakai H, Ohata H, Obata Y, Shiokawa D, et al. Stemness and immune evasion conferred by the TDO2-AHR pathway are associated with liver metastasis of colon cancer. Cancer Sci. (2022) 113:170–81. doi: 10.1111/cas.15182
110. Balaban RS. Regulation of oxidative phosphorylation in the mammalian cell. Am J Physiol. (1990) 258:C377–389. doi: 10.1152/ajpcell.1990.258.3.C377
111. Vander Heiden MG, Cantley LC, Thompson CB. Understanding the Warburg effect: the metabolic requirements of cell proliferation. Science. (2009) 324:1029–33. doi: 10.1126/science.1160809
112. Mascanfroni ID, Takenaka MC, Yeste A, Patel B, Wu Y, Kenison JE, et al. Metabolic control of type 1 regulatory T cell differentiation by AHR and HIF1-α. Nat Med. (2015) 21:638–46. doi: 10.1038/nm.3868
113. Nault R, Fader KA, Ammendolia DA, Dornbos P, Potter D, Sharratt B, et al. Dose-dependent metabolic reprogramming and differential gene expression in TCDD-elicited hepatic fibrosis. Toxicol Sci. (2016) 154:253–66. doi: 10.1093/toxsci/kfw163
114. Prokopec SD, Houlahan KE, Sun RX, Watson JD, Yao CQ, Lee J, et al. Compendium of TCDD-mediated transcriptomic response datasets in mammalian model systems. BMC Genomics. (2017) 18:78. doi: 10.1186/s12864-016-3446-z
115. Sutter CH, Olesen KM, Bhuju J, Guo Z, Sutter TR. AHR regulates metabolic reprogramming to promote SIRT1-dependent keratinocyte differentiation. J Invest Dermatol. (2019) 139:818–26. doi: 10.1016/j.jid.2018.10.019
116. Diani-Moore S, Marques Pedro T, Rifkind AB. Organ-specific effects on glycolysis by the dioxin-activated aryl hydrocarbon receptor. PloS One. (2020) 15:e0243842. doi: 10.1371/journal.pone.0243842
117. Leja-Szpak A, Góralska M, Link-Lenczowski P, Czech U, Nawrot-Porąbka K, Bonior J, et al. The opposite effect of L-kynurenine and ahr inhibitor ch223191 on apoptotic protein expression in pancreatic carcinoma cells (Panc-1). Anticancer Agents Med Chem. (2019) 19:2079–90. doi: 10.2174/1871520619666190415165212
118. Watzky M, Huard S, Juricek L, Dairou J, Chauvet C, Coumoul X, et al. Hexokinase 2 is a transcriptional target and a positive modulator of AHR signalling. Nucleic Acids Res. (2022) 50:5545–64. doi: 10.1093/nar/gkac360
119. Tonack S, Kind K, Thompson JG, Wobus AM, Fischer B, Santos AN. Dioxin affects glucose transport via the arylhydrocarbon receptor signal cascade in pluripotent embryonic carcinoma cells. Endocrinology. (2007) 148:5902–12. doi: 10.1210/en.2007-0254
120. Lv Q, Wang K, Qiao S, Yang L, Xin Y, Dai Y, et al. Norisoboldine, a natural AhR agonist, promotes Treg differentiation and attenuates colitis via targeting glycolysis and subsequent NAD+/SIRT1/SUV39H1/H3K9me3 signaling pathway. Cell Death Dis. (2018) 9:258. doi: 10.1038/s41419-018-0297-3
121. Bian X, Liu R, Meng Y, Xing D, Xu D, Lu Z. Lipid metabolism and cancer. J Exp Med. (2021) 218:e20201606. doi: 10.1084/jem.20201606
122. Su X, Abumrad NA. Cellular fatty acid uptake: a pathway under construction. Trends Endocrinol Metab. (2009) 20:72–7. doi: 10.1016/j.tem.2008.11.001
123. Koundouros N, Poulogiannis G. Reprogramming of fatty acid metabolism in cancer. Br J Cancer. (2020) 122:4–22. doi: 10.1038/s41416-019-0650-z
124. Yao L, Wang C, Zhang X, Peng L, Liu W, Zhang X, et al. Hyperhomocysteinemia activates the aryl hydrocarbon receptor/CD36 pathway to promote hepatic steatosis in mice. Hepatology. (2016) 64:92–105. doi: 10.1002/hep.28518
125. Röhrig F, Schulze A. The multifaceted roles of fatty acid synthesis in cancer. Nat Rev Cancer. (2016) 16:732–49. doi: 10.1038/nrc.2016.89
126. Santos CR, Schulze A. Lipid metabolism in cancer. FEBS J. (2012) 279:2610–23. doi: 10.1111/j.1742-4658.2012.08644.x
127. Geng F, Cheng X, Wu X, Yoo JY, Cheng C, Guo JY, et al. Inhibition of SOAT1 suppresses glioblastoma growth via blocking SREBP-1-mediated lipogenesis. Clin Cancer Res. (2016) 22:5337–48. doi: 10.1158/1078-0432.CCR-15-2973
128. Shimano H, Sato R. SREBP-regulated lipid metabolism: convergent physiology - divergent pathophysiology. Nat Rev Endocrinol. (2017) 13:710–30. doi: 10.1038/nrendo.2017.91
129. Rojas IY, Moyer BJ, Ringelberg CS, Tomlinson CR. Reversal of obesity and liver steatosis in mice via inhibition of aryl hydrocarbon receptor and altered gene expression of CYP1B1, PPARα, SCD1, and osteopontin. Int J Obes (Lond). (2020) 44:948–63. doi: 10.1038/s41366-019-0512-z
130. Krishnan S, Ding Y, Saedi N, Choi M, Sridharan GV, Sherr DH, et al. Gut microbiota-derived tryptophan metabolites modulate inflammatory response in hepatocytes and macrophages. Cell Rep. (2018) 23:1099–111. doi: 10.1016/j.celrep.2018.03.109
131. Levine W, Dyer AR, Shekelle RB, Schoenberger JA, Stamler J. Serum uric acid and 11.5-year mortality of middle-aged women: findings of the Chicago Heart Association Detection Project in Industry. J Clin Epidemiol. (1989) 42:257–67. doi: 10.1016/0895-4356(89)90061-9
132. Strasak AM, Rapp K, Hilbe W, Oberaigner W, Ruttmann E, Concin H, et al. Serum uric acid and risk of cancer mortality in a large prospective male cohort. Cancer Causes Control. (2007) 18:1021–9. doi: 10.1007/s10552-007-9043-3
133. Yiu A, Van Hemelrijck M, Garmo H, Holmberg L, Malmström H, Lambe M, et al. Circulating uric acid levels and subsequent development of cancer in 493,281 individuals: findings from the AMORIS Study. Oncotarget. (2017) 8:42332–42. doi: 10.18632/oncotarget.16198
134. Yang J, Wang Y, Zhao Q, Zhang X, Wang X, Qin X, et al. Association of serum uric acid with increased risk of cancer among hypertensive Chinese. Int J Cancer. (2017) 141:112–20. doi: 10.1002/ijc.30731
135. Glantzounis GK, Tsimoyiannis EC, Kappas AM, Galaris DA. Uric acid and oxidative stress. Curr Pharm Des. (2005) 11:4145–51. doi: 10.2174/138161205774913255
136. Lawal AO, Kolude B, Adeyemi BF. Serum uric Acid levels in oral cancer patients seen at tertiary institution in Nigeria. Ann Ib Postgrad Med. (2012) 10:9–12. doi: 10.4103/1119-3077.94093
137. Horsfall LJ, Nazareth I, Petersen I. Serum uric acid and the risk of respiratory disease: a population-based cohort study. Thorax. (2014) 69:1021–6. doi: 10.1136/thoraxjnl-2014-205271
138. Taghizadeh N, Vonk JM, Boezen HM. Serum uric acid levels and cancer mortality risk among males in a large general population-based cohort study. Cancer Causes Control. (2014) 25:1075–80. doi: 10.1007/s10552-014-0408-0
139. Hu D-E, Moore AM, Thomsen LL, Brindle KM. Uric acid promotes tumor immune rejection. Cancer Res. (2004) 64:5059–62. doi: 10.1158/0008-5472.CAN-04-1586
140. Yan S, Zhang P, Xu W, Liu Y, Wang B, Jiang T, et al. Serum uric acid increases risk of cancer incidence and mortality: A systematic review and meta-analysis. Mediators Inflammation. (2015) 2015:764250. doi: 10.1155/2015/764250
141. Fini MA, Elias A, Johnson RJ, Wright RM. Contribution of uric acid to cancer risk, recurrence, and mortality. Clin Transl Med. (2012) 1:16. doi: 10.1186/2001-1326-1-16
142. Zangar RC, Davydov DR, Verma S. Mechanisms that regulate production of reactive oxygen species by cytochrome P450. Toxicol Appl Pharmacol. (2004) 199:316–31. doi: 10.1016/j.taap.2004.01.018
143. Casado FL, Singh KP, Gasiewicz TA. The aryl hydrocarbon receptor: regulation of hematopoiesis and involvement in the progression of blood diseases. Blood Cells Mol Dis. (2010) 44:199–206. doi: 10.1016/j.bcmd.2010.01.005
144. Quintana FJ. Regulation of central nervous system autoimmunity by the aryl hydrocarbon receptor. Semin Immunopathol. (2013) 35:627–35. doi: 10.1007/s00281-013-0397-1
146. Kajarabille N, Latunde-Dada GO. Programmed cell-death by ferroptosis: antioxidants as mitigators. Int J Mol Sci. (2019) 20:4968. doi: 10.3390/ijms20194968
147. Jakubczyk K, Dec K, Kałduńska J, Kawczuga D, Kochman J, Janda K. Reactive oxygen species - sources, functions, oxidative damage. Pol Merkur Lekarski. (2020) 48:124–7.
148. Elson DJ, Nguyen BD, Wood R, Zhang Y, Puig-Sanvicens V, Kolluri SK. The cyclin-dependent kinase inhibitor p27Kip1 interacts with the aryl hydrocarbon receptor and negatively regulates its transcriptional activity. FEBS Lett. (2022) 596:2056–71. doi: 10.1002/1873-3468.14434
149. Kolluri SK, Weiss C, Koff A, Göttlicher M. p27(Kip1) induction and inhibition of proliferation by the intracellular Ah receptor in developing thymus and hepatoma cells. Genes Dev. (1999) 13:1742–53. doi: 10.1101/gad.13.13.1742
150. Luo M, He H, Kelley MR, Georgiadis MM. Redox regulation of DNA repair: implications for human health and cancer therapeutic development. Antioxid Redox Signal. (2010) 12:1247–69. doi: 10.1089/ars.2009.2698
151. Ichikawa J, Tsuchimoto D, Oka S, Ohno M, Furuichi M, Sakumi K, et al. Oxidation of mitochondrial deoxynucleotide pools by exposure to sodium nitroprusside induces cell death. DNA Repair (Amst). (2008) 7:418–30. doi: 10.1016/j.dnarep.2007.11.007
152. Oka S, Ohno M, Tsuchimoto D, Sakumi K, Furuichi M, Nakabeppu Y. Two distinct pathways of cell death triggered by oxidative damage to nuclear and mitochondrial DNAs. EMBO J. (2008) 27:421–32. doi: 10.1038/sj.emboj.7601975
Keywords: aryl hydrocarbon receptor, cancer, metabolic reprogramming, metabolism, tumor microenvironment
Citation: Wang Z, Zhang Y, Liao Z, Huang M and Shui X (2024) The potential of aryl hydrocarbon receptor as receptors for metabolic changes in tumors. Front. Oncol. 14:1328606. doi: 10.3389/fonc.2024.1328606
Received: 06 November 2023; Accepted: 29 January 2024;
Published: 16 February 2024.
Edited by:
Nadia Judith Jacobo-Herrera, National Institute of Medical Sciences and Nutrition Salvador Zubirán, MexicoReviewed by:
Eric Hanse, University of California, Irvine, United StatesAmir Shadboorestan, Tarbiat Modares University, Iran
Rridha Oueslati, University Carthage - Sciences Faculty of Bizerte, Tunisia
Copyright © 2024 Wang, Zhang, Liao, Huang and Shui. This is an open-access article distributed under the terms of the Creative Commons Attribution License (CC BY). The use, distribution or reproduction in other forums is permitted, provided the original author(s) and the copyright owner(s) are credited and that the original publication in this journal is cited, in accordance with accepted academic practice. No use, distribution or reproduction is permitted which does not comply with these terms.
*Correspondence: Xiaorong Shui, c2h1aXhpYW9yQDEyNi5jb20=