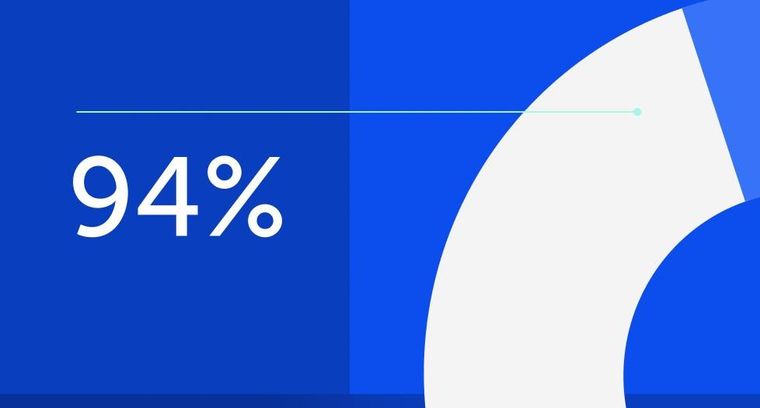
94% of researchers rate our articles as excellent or good
Learn more about the work of our research integrity team to safeguard the quality of each article we publish.
Find out more
REVIEW article
Front. Oncol., 01 February 2024
Sec. Gastrointestinal Cancers: Colorectal Cancer
Volume 13 - 2023 | https://doi.org/10.3389/fonc.2023.1344328
This article is part of the Research TopicThe microbiome in the development of gastrointestinal diseasesView all 17 articles
Gastrointestinal (GI) cancers constitute more than 33% of new cancer cases worldwide and pose a considerable burden on public health. There exists a growing body of evidence that has systematically recorded an upward trajectory in GI malignancies within the last 5 to 10 years, thus presenting a formidable menace to the health of the human population. The perturbations in GI microbiota may have a noteworthy influence on the advancement of GI cancers; however, the precise mechanisms behind this association are still not comprehensively understood. Some bacteria have been observed to support cancer development, while others seem to provide a safeguard against it. Recent studies have indicated that alterations in the composition and abundance of microbiomes could be associated with the progression of various GI cancers, such as colorectal, gastric, hepatic, and esophageal cancers. Within this comprehensive analysis, we examine the significance of microbiomes, particularly those located in the intestines, in GI cancers. Furthermore, we explore the impact of microbiomes on various treatment modalities for GI cancer, including chemotherapy, immunotherapy, and radiotherapy. Additionally, we delve into the intricate mechanisms through which intestinal microbes influence the efficacy of GI cancer treatments.
Gastrointestinal (GI) malignancies constitute approximately one-third of all newly diagnosed cancer cases globally and pose a significant public health challenge. Colorectal cancer (CRC), Gastric cancer (GC), liver cancer, and esophageal cancer are the most commonly observed GI malignancies across the globe (1, 2). Since GI malignancies have been on the rise over the past 5 to 10 years, there is a severe health risk to people due to this trend. The past decade has witnessed the substantiation of the role played by genetic and epigenetic factors in the development of cancer. This has been achieved through the extensive genomic and transcriptome sequencing endeavors undertaken by multiple multinational research initiatives (3). According to current studies, 2.7 million individuals worldwide die from GI cancer yearly, with 4 million cases diagnosed worldwide (4–6). Although GI cancers display a diverse array of biological attributes, several shared risk factors have been discerned. These include pro-tumor genetic mutations, excessive intake of alcohol, smoking, adherence to the Western diet, exposure to radioactive stimuli, and disturbance of the GI microbiota’s homeostasis (7). Furthermore, the disruption of the typical GI environment has been associated with the onset of GI malignancies due to the emergence of pro-tumoral fibrosis and the occurrence of significantly potent local or systemic inflammatory and immunological reactions (8–10). In addition to these risk factors, certain disorders are strongly linked to the origin of GI cancers. For instance, it has been found that GI cancer and diabetes are related. One of the most used anti-hyperglycemic medications, metformin, has been demonstrated to lower the incidence rate of GI malignancies in diabetic individuals (11, 12). The comprehension of the role of bacteria in cancer development is significantly restricted compared to the knowledge we have about viruses causing oncogenesis. However, it is feasible to consider that gaining a better understanding of the long-lasting effects of changes in the composition of the GI microbiota may have the potential to contribute to the progress of preventive strategies against cancer. Moreover, bacteria have the potential to indirectly facilitate the process of carcinogenesis through the alteration of both systemic and local immune reactions. These immune responses play a crucial role in progressing GI tract malignancies (13). The GI tract of humans harbors a vast number of microorganisms that work in conjunction with the host to uphold both wellness and illness. The intricate web of interactions between the GI microbiome and the host gives rise to intimate connections that span various components of human physiology, such as the metabolic, immunological, and neuroendocrine systems (14). These creatures are dynamic and subject to influences from medications, food, lifestyle, genetics, and the environment (15). Researchers have discovered that the influence of gut microorganisms extends beyond the confines of the intestines, affecting a range of conditions including pancreatic disease and hepatic disease, in addition to intestinal diseases such as Inflammatory bowel disease (IBD) and CRC (16). Shortly following the moment of birth, the microbiota initiates the process of establishing residence within the GI tract, subsequently maintaining its presence throughout the entirety of an individual’s lifespan (17). However, it can vary dynamically in response to nutrition, environmental stresses, lifestyle choices, antibiotics, and other medications (18). Increasing proof suggests that the microbial population residing in the digestive system holds immense potential to thwart the growth of cancerous cells while also possessing the ability to enhance the potency of chemotherapy and immunotherapy treatments (19). The gut microbiota is accountable for producing short-chain fatty acids (SCFAs), which bestow advantageous effects on the human body. These SCFAs are generated through the metabolic breakdown of dietary fiber, as well as the synthesis of vitamins B and K2. Additionally, the gut microbiota metabolizes various chemicals, such as sterols and exogenous substances, while also playing a role in regulating immunological function (20).
This study aimes to investigate the primary impacts of intestinal microbiota on the initiation and advancement of GI cancers, along with the potential utilization of these microorganisms as a sophisticated approach to discern and manage these ailments, as expounded upon in this comprehensive analysis.
The analysis of the microbial populations found in various human environments, such as the GI tract, mouth, skin, and vaginal area, requires applying advanced sequencing techniques that can process large amounts of data (21). The utilization of sophisticated sequencing methodologies, encompassing amplicon and shotgun metagenomic sequencing, has significantly transformed our comprehension of the human microbiome by delineating the bacteria linked to either optimal well-being or pathological conditions (22). When the physical condition of an individual is in a state of good health, the gut microbiota engages continuously and regularly with the host organism to sustain a state of balance within the intestines (23). However, maintaining such balance is challenging since the host’s genetic makeup and several exogenous variables, including nutritional consumption and antibiotic usage, have a direct impact on the microbiome (24–26). Dysbiosis, the alteration in both composition and functionality within the microbiome, can occur when there is a persistent disturbance in the stability of the microbial community. This alteration may cause various disorders, including cancer (27, 28). In a dysbiotic microbiome, various pathogenic occurrences are encountered, including a modification in the assortment of microorganisms, a decrease in beneficial commensals, and the proliferation of pathobionts. All of these occurrences can impact the formation of tumors, either in the vicinity of the GI tract or at a more remote location, such as the pancreas and liver (29, 30). The GI system harbors the highest abundance of commensal microorganisms among all the regions of the human body. Variable parts of the digestive system have varying levels of commensal microbial diversity and abundance (31). While a multitude of bacteria belonging to the phyla Firmicutes, Proteobacteria, Actinobacteria, Firmicutes, Bacteroidetes, and are frequently observed within the gut, certain bacterial species seem to be confined to specific regions (31, 32). Each area or organ’s microbial population is related to host characteristics, including pH, oxygen saturation, bile acids, and nutritional bioavailability (33, 34).
The thorough analysis of microbial populations in the host’s environment has been extensively explained as a result of the rapid advancements achieved in next-generation high-throughput sequencing (NGS) (35, 36). Dysbiosis leads to the stimulation of inflammatory components within the GI mucous membranes, which encompasses the liberation of nitric oxide (NO), the presence of oxidative stress, the creation and excretion of pro-inflammatory cytokines, as well as the activation of cyclooxygenase 2 (COX-2). Dysbiosis also causes microecological alterations (27, 37). The detrimental effects of microbial metabolites on extra-intestinal organs can manifest in various pathways, such as the gut-liver axis and the gut-brain axis, thereby impairing their optimal functioning (38, 39). Dysbiosis is believed to be most comprehensible when viewed through carcinogenesis, representing a continuous divergence of the host microbiota from a state of harmony and equilibrium that supports and, or upholds various cancer phenotypes (40, 41). The maintenance of well-balanced gut microbiota is crucial for promoting a healthy lifestyle. At the same time, an imbalance in this microbial community, known as dysbiosis, can lead to inflammatory consequences that accelerate cancer progression (42).
Despite an increasingly widespread acceptance of colonoscopy screening, colorectal cancer (CRC) remains the third most commonly occurring cancer and the primary contributor to cancer-related deaths in both male and female populations within the United States (43). In 2019, a forecast indicated that there would be an estimated 51,020 deaths and approximately 145,600 fresh instances of CRC. Additionally, while the occurrence and fatality rate of CRC has experienced a gradual decrease in the past few decades in individuals aged 65 and above, a distinct trend has emerged among individuals under 50, for whom conventional screening methods have not been recommended (44). The evolution of CRC has been comprehensively examined over recent decades via migration and prospective cohort studies, illustrating the significant influence of nutritional and lifestyle determinants (43). According to estimations, it has been noted that modifiable risk factors, namely excessive weight or obesity, excessive alcohol consumption, smoking, high consumption of red meat, physical inactivity, and inadequate intake of dietary fiber, whole grains, or other beneficial nutrients, play a significant role in approximately 50% to 60% of newly reported cases of CRC in the US (43). The microbiome, which encompasses bacteria, viruses, fungi, and an array of diverse organisms, possesses the ability to regulate the condition of well-being, and modifications to it can contribute to the emergence and progression of ailments. There exists an increasing corpus of scholarly investigation indicating that alterations in the constitution of the intestinal microbiota contribute to the genesis and progression of CRC using the impact of environmental factors that pose a risk (45, 46). This could be the case due to the microbiome’s effect on metabolism and immune response (47). Hence, manipulating the intestinal microbial community could potentially serve as a constituent of strategies aimed at averting CRC (48, 49). Research has demonstrated discrepancies in the composition of the intestinal microbiomes between individuals afflicted with CRC and those deemed healthy (controls). Additionally, certain microbial species have been identified as exhibiting increased or diminished presence within the gut microbiomes of CRC patients. Therefore, to improve screening techniques, alterations in the microbiome can be utilized as biomarkers in the early detection of CRC (49). The colon is home to 70% of the human microbiome (50). Individuals who encounter antibiotics early on exhibit an increased propensity to develop colorectal adenoma during their later years (51). The microbiota in the GI tract plays a crucial role in converting the dietary components into metabolites that can either promote or suppress the growth of tumors. The development of CRC is subsequently influenced by these metabolites (52). Over 2000 different bacteria species are thought to exist in the human gut (53, 54).
According to two separate investigations, tumor specimens had more Fusobacterium DNA and RNA sequences than non-tumor ones (55, 56). Similar relationships have been discovered in several studies, including numerous cohorts of CRC patients worldwide (57, 58). Fusobacterium nucleatum (F. nucleatum) has been associated with more advanced stages of disease, an increased likelihood of recurrence, and shorter periods of survival for patients, thus presenting compelling evidence of its potential causal role in CRC (59) (Figure 1). It is found in 10%–15% of tumors. Furthermore, F. nucleatum levels in tumor tissue have been linked to reduced T-cell infiltration, corroborating studies that claim F. nucleatum inhibits the anti-tumor immune response (43). F. nucleatum has been linked to distinct clinical and molecular characteristics in epidemiological investigations involving individuals with CRC or precancerous lesions. The aforementioned characteristics encompass the presence of anatomical positioning on the right side, mutations in the BRAF gene, and heightened levels of hypermutation alongside microsatellite instability (58, 60, 61). The described attributes of serrated neoplasia imply that F. nucleatum might have a part to play in developing CRC through the serrated pathway. Research has established a connection between F. nucleatum and the consensus molecular subtype 1 of CRC (62). This particular subtype is distinguished by an excessive expression of the immune system and the presence of microsatellite instability (63–65). More recently, in paired main tumors and distant metastases from CRC patients, virtually identical, live Fusobacterium strains were discovered in similar relative abundances. Fusobacterium thus seems to be a crucial part of the tumor microenvironment (66).
Enterotoxigenic Bacteroides fragilis (ETBF), a significant pathogen that releases virulence factors to advance CRC, generates B. fragilis toxin or fragilysin, thereby inciting an adverse immune reaction (67) (Figure 1). Colitis characterized by a robust, selective colonic Stat3 activation and a selective Th17 response was seen in mice treated with ETBF (68). Notably, a study by Chung et al. (69) provided more evidence that BFTs focus on the epithelial cells of the colon to instigate an immune response within the mucosal lining. This, in turn, triggers a series of inflammatory reactions that require the activation of IL-17, NF-kB, and Stat3. The molecular pathways of ETBF-induced adaptive immunity modification in CRC were further characterized by other researchers. Geis and colleagues (70) demonstrated that the presence of regulatory T cells in the local microenvironment resulted in a decrease in the quantity of IL2, thereby enabling the proliferation of Th17 cells, which is essential for the promotion of ETBF-induced CRC. Exosome miR-149-3p produced by colon cells after ETBF treatment also promotes Th17 differentiation (67). Consequences of long-term ETBF infection and inflammation include carcinogenesis. According to an animal study, BFT was required for the impact of ETBF infection, which increased colonic inflammation and enhanced AOM/DSS-induced CRC (71). An IL-17-driven monocytic-MDSC-dominant immunological profile was shown to be related to ETBF-triggered CRC by Thiele et al. (72), indicating that ETBF infection encourages MDSC-mediated immune suppression. B. fragilis emerged as the sole species consistently exhibiting higher levels in the intestinal microbiomes of individuals diagnosed with CRC across various geographical regions. This finding was established through a comprehensive meta-analysis encompassing four case-control studies that examined the metagenomes of CRC patients (73).
There is growing proof that pks+ Escherichia coli (E. coli) can produce virulence factors that control the development and progression of CRC (74) (Figure 1). A cancer-related pathogen that often infects CRC patients and expresses the polyketide synthase (pks) gene Colibactin, a hybrid peptide-polyketide cytotoxin that E. coli produces, induces DNA double-strand breaks and activates the DNA damage checkpoint mechanism in eukaryotic cells (75, 76). The involvement of colibactin in CRC has been shown by recent research. Anaphase bridge development, G2/M cell cycle stoppage, chromosomal aberration, and instability are all signs of the DNA damage response that even brief exposure to pks+ E. coli causes in mammalian epithelial cells (76–78). Cougnoux and colleagues (79), on the other hand, showed that acceleration of AOM-DSS-induced CRC by pks+ E. coli is facilitated by the stimulation of growth factor-secreting senescent cells, which is achieved through the alteration of p53 SUMOylation. Consequently, this modification encourages the proliferation of uninfected cells. Colibactin-producing E. coli also alters the immunological milieu, decreasing antitumor T-cell response and causing immunotherapy resistance and their effects on DNA damage (80).
An anerobic bacteria called Peptostreptococcus >anaerobius (P. anaerobius) often lives in the mouth cavity. The bacterium P. anaerobius, which has been recently identified, was observed to have a higher occurrence rate among individuals diagnosed with CRC in comparison to those who were in good health (81, 82) (Figure 1). This remarkable finding was unearthed by employing the cutting-edge technique of shotgun metagenomic sequencing on fecal samples coupled with the exact 16S ribosomal RNA sequencing method on mucosal samples (83, 84). Subsequent examinations of functional nature revealed that P. anaerobius expedited the progression of AOM-induced CRC by augmenting the synthesis of cholesterol through the activation of TLR2/TLR4 signaling, thereby bolstering the proliferation of CRC cells (85, 86). Moreover, investigations on the profiling of the microbiome in the oral cavity have revealed that there are variations in the quantities of different components of the oral biofilm, including Parvimonas, Haemophilus, Alloprevotella, Prevotella, Lachnoanaerobaculum, Streptococcus, and Neisseria, between patients with CRC and control subjects (87, 88). The development of CRC has been associated with varying gene expression patterns in the mucosal surfaces of different bacteria. Notably, inquiries that have examined samples derived from individuals with colonic neoplasia and controls have discovered analogous networks of oral bacteria that exist on both the oral and colonic mucosal surfaces (88).
In light of the crucial function that the gut microbiota fulfills in CRC, extensive investigations have been conducted to unravel the secrets of regulating gut dysbiosis to avert or combat this ailment (89). Several tactics have been suggested, such as fecal microbial transplantation (FMT), dietary changes, and antibiotic treatment. At present, FMT has demonstrated efficacy in the management of recurrent Clostridium difficile infection. Nevertheless, the utilization of FMT in animals remains limited to the prevention and treatment of CRC (90–94). FMT is only marginally beneficial in a preventative situation, though. A more likely method of controlling the microbiota to prevent CRC is dietary intervention. Recent research has revealed how food and the microbiota interact to cause CRC (95). For instance, high-fat diet-fed mice showed a considerable change in the makeup of their intestinal microbial and reduced gut barrier function, confirming the theory that high fat causes CRC by encouraging microbial dysbiosis (96). Contrarily, dietary fiber can promote the proliferation of advantageous commensals, which produce metabolites such as Short-chain fatty acids (SCFAs) linked to tumor-suppressing properties (97). Intriguingly, ETBF-induced CRC in AOM-DSS mouse models was suppressed by high salt diets, suggesting that the effect of diet may depend on the situation (98, 99).
Current research suggests that the makeup of microorganisms in the GI system, referred to as the intestinal microbiome, can impact the body’s response to different cancer therapies, including immune checkpoint blockade (ICB) therapy and chemotherapy. The study involved comparing samples of rectal cancer that were locally advanced, with and without treatment for F. nucleatum. The results showed that F. nucleatum persistence after neoadjuvant chemoradiotherapy (nCRT) is connected to increased recurrence rates and the inhibition of immune cytotoxicity (100). Time and time again, numerous studies have discovered that F. nucleatum was more commonly present in patients with CRC who experienced a recurrence following chemotherapy (101). Additionally, it was observed that F. nucleatum targeted microRNAs, as well as the innate immunological signaling pathways TLR4 and MYD88, to stimulate the defense autophagy pathway and counteract the response to chemotherapy (102, 103). These findings imply that pathogenic microorganisms may not only influence colorectal carcinogenesis but also enhance treatment resistance (104). Contrarily, much research has also surfaced, demonstrating that gut commensal bacteria can enhance ICB treatment by activating antitumor T cells (105–107).
Gastric cancer (GC) holds a prominent position as the fourth leading contributor to cancer-related mortality globally. Furthermore, it also ranks as the fifth most commonly detected form of cancer (108). Male rates are two times higher than female rates. Eastern Asia has the highest incidence rates, approximately 26,000 fresh instances and 11,000 fatalities from GC manifest annually within the US. The overall 5-year survival rate for GC is considerably low, standing at 32.4%. This is probably because, in the United States, up to 62% of instances of GC are diagnosed at late stages, which are linked to lower overall survival rates than localized illness (109). GC arises as a result of a multifaceted interplay involving the genetic composition of the host, various environmental factors (e.g., alcohol consumption, smoking, excessive intake of salt and meat, and inadequate consumption of vegetables and fruits), as well as microbial elements (e.g., the presence of Helicobacter pylori (H. pylori) infection and the composition of the intestinal microbiota). The persistent activation of the immune system resulting from the intestinal microbiota of the host has been associated with long-term inflammation and altered interactions between the host’s epithelium and microorganisms, which have been associated with GC (110).
The standard oxyntic (corpus) region of the human stomach, characterized by a low pH and an acidic milieu, is a barrier against the proliferation of commensal organisms and potentially detrimental pathogens originating from the upper and lower GI tracts. These regions serve as the primary abode for the vast majority of the microorganisms that comprise the body’s microbiota. These microorganisms are primarily found in the large and small intestines, as well as the oral cavity (111). The false conclusion was reached due to several factors: the inadequate achievement in isolating and cultivating gastric microbiota, the absence of rapid and non-invasive diagnostic tests, and the emergence of microarray and next-generation sequencing technology, which have focused on the bacterial 16S ribosomal RNA (16S rRNA) as the primary target for accurate taxonomy and phylogeny identifications (112).
In 1982, Marshall and Warren discovered the significant revelation that H. pylori was the underlying factor responsible for both peptic ulcers and gastritis, thereby drawing attention to the possibility of stomach infection leading to cancer development (113). This particular microorganism, after recent discovery, has been categorized as a type I carcinogen and is projected to have an impact on over 50% of the global populace. In a tiny proportion of the afflicted population (2%), this infection results in a predictable step-by-step pattern of illness progression that, if discovered in time, can be reversed (114). The CagA protein, one of the cag pathogenicity islands, is a mechanism through which H. pylori infection can cause cancer (115, 116). Depending on the modifications after translation, CagA is initially introduced into the cell through the Type IV secretion system. Subsequently, it assumes a pathogenic role by stimulating the activation of SHP2, Abl, or Src kinases within the enclosure of GC (114). The EPIYA motif, which is distinguished by the existence of residues such as proline, isoleucine, glutamate, tyrosine, and alanine, functions as the site for phosphorylation within the CagA protein and may display discrepancies depending on the particular strain of H. pylori (117, 118). In addition, H. pylori can generate peptidoglycan within the cellular environment of the host, thereby augmenting the synthesis of IL-8 and cox, alongside other pro-inflammatory cytokines (119, 120). Consequently, this leads to the persistence of chronic inflammation and ultimately facilitates the emergence of cancer. It has been additionally established that H. pylori releases VacA toxin. This substance can potentially diminish T-cell responses and facilitate the formation of lesions with minimal opposition from the immune system (114, 121). Today, H. pylori may be detected via a quick urease test, a polymerase chain reaction test, a histological study of biopsy specimens, and a serological test. Infection with H. pylori typically develops in childhood and persists throughout the host’s life without antibiotic therapy. The transmission of bacteria can occur through direct contact between individuals, either through oral-oral or fecal-oral pathways (122). H. pylori is believed to persistently inhabit approximately half of the global populace, with approximately 15% of individuals afflicted by this pathogen subsequently progressing to the development of gastric ulcers (123). H. pylori employs flagella to facilitate its entry into the gastric mucosa, seeking refuge from the stomach’s highly acidic milieu. It is essential to acknowledge that a substantial percentage, surpassing 20%, of H. pylori variants adhere to the exterior of gastric epithelial cells (122). The ability of H. pylori to securely attach to the gastric epithelial cells is facilitated by the implementation of adhesion molecules, including the outer inflammatory protein A (OipA), sialic acid-binding adhesin (SabA), and adherence-associated lipoproteins (AlpA/B) (124).
For many years, H. pylori has been thought of as the predominant, if not the only, bacteria that can live in the stomach’s acid environment and encourage gastric carcinogenesis (111). However, new data from 16SrRNA sequencing showed that non- H. pylori strains co-occurred in both H. pylori + and H. pylori - persons with GC (125). Additionally, accumulating evidence indicates that the term “dysbiosis” best describes how the microbiome gradually changes throughout the development of GC (126, 127).
Probiotics, traditionally limited to their use as food additives (such as in yogurt), have been revolutionized by advanced methods like fecal microbiota transplantation (FMT). These techniques have introduced the concept of therapeutically restoring eubiotics in GI illnesses, thereby transforming the field entirely (128). However, there hasn’t been any research done yet on the treatment or prevention of GC in humans. Notably, an international expert council has questioned FMT practices due to inconsistent results and the need for standardization and safety (129, 130).
Proton pump inhibitors (PPIs) are widely used, which has increased interest in how they may interact with the stomach flora. PPI use over an extended period decreases stomach acid output, encouraging bacterial proliferation because of increased pH brought on by H. pylori. PPIs thus have a significant influence on the variety and abundance of bacteria (131–133). For instance, significant levels of Bifidobacteriaceae from the oral cavity (Bifidobacterium dentium, Scardovia inopinata, and Parascardovia denticolens) were found in human stomachs with hypochlorhydria in gastritis patients on omeprazole (134, 135). PPIs also boost the number of organisms that may colonize the mouth, such as Clostridiales, Streptophyta, Veillonella, Fusobacterium, Leptotrichia, Oribacterium, Porphyromonas, Prevotella, Capnocytophaga, Granulicatella, Campylobacter, and Bulleidia (133, 136). Nonsteroidal anti-inflammatory drugs (NSAIDs) and PPIs can have adverse side effects on the GI mucosa, although taking probiotic strains along with them might lessen these effects. Bifidobacterium, as an exemplification, safeguards mice from the occurrence of stomach ulcers caused by aspirin, while Lactobacillus plantarum, derived from green tea, has curative properties against gastric ulcers induced by alcohol (137–139). In mice given the PPIs rabeprazole or vonoprazan, oral Lactobacillus johnsonii supplementation reduced indomethacin-induced minor intestine damage (140).
Large-scale clinical trials and field investigations have provided substantial evidence in favor of the cancer-preventive effects of H. pylori eradication. However, the emergence of antibiotic resistance poses a significant challenge, as does the disturbance of the gut microbiota and the impact of H. pylori on various other disease states, including asthma and esophageal cancer (141). Various microorganisms linked to stomach illness were found in a recent randomized controlled clinical investigation one year after H. pylori elimination (142). Probiotic Roseburia, Faecalibacterium prausnitzii, and Sphingomonas were decreased, whereas Streptococcus anginosus, Acinetobacter lwoffii, and Ralstonia were enriched. Also linked to chronic gastritis were oral bacteria (Streptococcus, Peptostreptococcus, Prevotella, Rothia, Parvimonas, Granulicatella). Other researchers who conducted endoscopic ablation of early GC in individuals experiencing a deficiency of beneficial microorganisms, such as Ralstonia, Faecalibacterium, Blautia, Methylobacterium, and Megamonas, observed a prolonged presence of dysbiosis in patients after the eradication of H. pylori (143, 144). The restoration of beneficial GI microbiota, such as Bifidobacterium, Lactobacillus, Lachnoclostridium, and Blautia, has been observed in young asymptomatic individuals following the eradication of H. pylori. Additionally, the presence of pathogenic Alistipes has been found to decrease (145, 146).
Liver cancer is the primary reason for cancer deaths, and its prevalence is rising yearly (147). About 90% of initial liver malignancies are hepatocellular carcinomas (HCC), a significant worldwide health issue (148). Several factors increase the risk of HCC, including chronic hepatitis B and C infections, alcoholism, metabolic liver disease (particularly nonalcoholic fatty liver disease), and exposure to food toxins like aristolochic acid and aflatoxins (149). The World Health Organisation predicts that by 2030, this illness will claim the lives of more than a million individuals (150). Sorafenib, an inhibitor of multiple kinases that has received approval for the management of hepatic carcinoma, occupies the position of primary therapeutic modality for advanced HCC. It has been shown to improve overall survival significantly, but it cannot stop the progression of the disease because of the emergence of resistance to antiproliferative therapies (151). The early detection and treatment of HCC contribute to the enhancement of its prognosis, which is also observed in the majority of disease processes. The most optimal opportunity to detect a medical condition at an early stage is by closely monitoring individuals with a heightened likelihood of developing the disease. This group includes both those who have cirrhosis of any kind and those who carry the hepatitis B virus (152). According to the 2012 NCCN recommendations, individuals at a heightened risk should undergo hepatic ultrasonography and AFP testing on a semiannual to annual basis. Per the 2012 recommendations of the National Comprehensive Cancer Network (NCCN), individuals classified as high-risk should undergo hepatic ultrasonography and AFP testing every six to twelve months (152, 153). The correlation between the presence of microorganisms causing infection and the onset of cancer has been recognized for a significant duration. Among the various mechanisms that contribute to this association, the chronic inflammation induced by infection is considered to be an important causative factor. Emerging evidence indicates that the resulting gut dysbiosis, characterized by an imbalanced state of intestinal microbial composition linked to illness, is responsible for the carcinogenic implications of these microbial stimuli. Consequently, this dysbiosis triggers chronic inflammation and, ultimately, the development of cancer (154). However, it is imperative to acknowledge that not all microorganisms are harmful. A plethora of commensal bacteria have a crucial function in fostering the development of the host’s immune system (155, 156). The host’s state of health is influenced by the constituent member types (pathogenic or commensal) and abundance arrangement (dysbiotic or eubiotic) of the intestinal microbial. Numerous investigations have effectively highlighted the crucial functions that gut bacteria play in the development of HCC (154, 157). The bidirectional interplay between the GI tract and the hepatic organ transpires via the portal vein, a conduit that expedites the passage of diverse entities originating from the gastrointestinal system, including nourishing compounds, metabolic byproducts of microorganisms, and constituents of said microorganisms, to the hepatic entity (158, 159). Once in the bile duct, these substances go from the liver to the gut. As a result of this enterohepatic circulation, the liver is constantly exposed to substances that originate from the gut (160). Furthermore, the association linking the gut and the liver, commonly called the “gut-liver axis,” has garnered increasing attention from researchers due to its pivotal role in preserving liver homeostasis and averting the onset of ailments (161, 162). One common finding in several liver illnesses is that tight connections between adjacent intestinal epithelial cells are impaired with increasing intestinal permeability, indicating that substances coming from the gut have an impact on liver function (160, 163). Furthermore, microbial dysbiosis in the lower GI tract and small intestinal bacterial overgrowth (SIBO) are linked to liver injury (164, 165). This finding implies that the bile produced by a healthy liver, along with other liver-derived compounds, contributes to the probiotic status of the gut microbiota (165). The liver is commonly perceived as an organ devoid of immunological function, instead playing a pivotal role in various metabolic processes, energy source storage, and detoxification (166, 167). The organ can also be perceived as a highly responsive component of the immune system, serving as a dwelling place for various immune cells such as Kupfer cells, natural killer (NK)/NKT cells, and T and B lymphocytes. Additionally, it harbors stromal cells such as liver sinusoidal endothelial cells (LSECs) and hepatic stellate cells (HSCs), which possess the ability to release cytokines and various other substances that can interact with immune cells (160).
Hepatitis is a liver inflammation that can either go away independently or progress into a dangerous illness that causes cirrhosis or HCC. Hepatitis B and C virus infections typically result in chronic hepatitis, and viral infections are the primary cause of hepatitis worldwide (168). Both viruses cause host immune responses for clearance after infecting hepatocytes. To stop viral replication, nucleoside (or nucleotide) analogs (NAs) are frequently used to treat viral hepatitis. In addition, the mature gut microbiota is necessary for quick HBV clearance via efficient host immune boosting (154). The gut microbiota of individuals with cirrhosis caused by HBV exhibited notable distinctions compared to the gut microbiota of healthy control subjects, as indicated by a study conducted using advanced next-generation sequencing technology (169, 170). Specifically, certain bacterial species such as Clostridium, Prevotella, Veillonella, and Streptococcus displayed higher prevalence levels, whereas Alistipes and Eubacterium were found to be less frequently observed (171). The presence of oral microorganisms in higher quantities indicates that the transfer of microbes from the mouth to the gut is a prevailing occurrence among individuals with cirrhosis (171). The diversity of microorganisms in the GI tract, assessed using the Shannon and Simpson indices, declined in individuals with cirrhosis and recovered to a level comparable to that of healthy individuals in patients with HBV-related HCC (172, 173). In addition, the diversity decreased more in early hepatitis B patients than in intermediate cases, but not considerably, and both were lower than in healthy controls (174). Collectively, the variety of gut bacteria appears to decline during the early stages of HBV infection and then recover to a level comparable to that of healthy individuals as the liver disease advances. The aberrant bile acid production and composition, which compromises the bile acids’ antimicrobial defenses and enables the transfer of oral species, are thought to contribute to these gut microbial changes linked to HBV infection (154, 175).
Alcohol liver disease (ALD), characterized by alcoholic hepatitis, alcoholic fatty liver disease, and alcoholic cirrhosis, represents a significant contributing factor to various liver ailments (176, 177). Alcohol’s metabolic byproducts are held responsible for the adverse effects of alcohol abuse. Alcohol undergoes oxidation within the hepatocyte, primarily resulting in the formation of acetaldehyde through the activity of alcohol dehydrogenase. Meanwhile, there is a limited production of reactive oxygen species (ROS). Prolonged exposure to acetaldehyde and ROS can lead to hepatotoxicity and carcinogenicity within the liver (178). Although the liver is the primary site of alcohol metabolism, intestinal enzymes and microorganisms are also capable of doing so (179, 180). Therefore, drinking too much alcohol increases luminal acetaldehyde and ROS, which disturbs the gut ecology by affecting gut barrier function and promoting gut dysbiosis, which alter the makeup of the gut’s microbial population (181–183). As substantiation, the presence of alcoholic hepatitis resulted in a decrease in Akkermansia in individuals with severe illness in comparison to individuals who were in good health, and this decrease was even more pronounced (184, 185). Mice that underwent an FMT from individuals suffering from acute hepatitis and alcoholism exhibited increased levels of Bacteroides, Butyricimonas, Alistipes, Bilophila, and Clostridium XIVa compared to mice that did not receive an FMT (186, 187). Additionally, the presence of alcoholic hepatitis resulted in an elevation of bacterial DNA levels in the bloodstream when compared to individuals who do not consume alcohol. This increase was characterized by a decrease in DNA from Bacteroidetes and an increase in DNA from Fusobacteria (188).
It is estimated that approximately 80-100 million individuals in the United States, constituting around 25% of the adult population, are believed to be affected by non-alcoholic fatty liver disease (NAFLD). The primary etiology of chronic hepatic ailment presently observed worldwide is NAFLD (189–191). Hepatic steatosis, a condition characterized by the accumulation of fat in the liver exceeding 5% of its overall weight, can be attributed to an excessive intake of alcohol. Abdominal imaging data suggests that the global prevalence of NAFLD is expected to reach 25%, with the African continent experiencing the lowest majority at 13.5% and the Middle East observing the highest at 31.8%. Non-alcoholic steatohepatitis (NASH) emerges in approximately 30% of individuals diagnosed with NAFLD (192). NASH can potentially progress from a state of simple steatosis to the more severe conditions of cirrhosis or HCC, or it may experience a decline in its condition (192, 193). It has been shown that NAFLD causes lipid metabolism to be dysregulated, resulting in the loss of CD4+ T cells and subsequently encouraging the development of hepatocarcinogenesis (194, 195). Similar to this, IgA+ cells that have accumulated in the livers of NASH patients with fibrosis help to promote hepatocarcinogenesis by inhibiting CD8+ T cell activation (196, 197). The importance of the intestinal metagenome in the etiopathogenesis of NAFLD has also been emphasized by recent findings (192). The initial step in establishing the etiological link between gut bacteria and NAFLD was replicating the hepatic changes associated with the disease in mice utilizing co-housing and FMT trials (198, 199). Dysbiosis, in turn, can lead to the development of metabolic disorders, including metabolic syndrome, obesity, Type 2 Diabetes Mellitus (T2DM), and NAFLD (200, 201). Increased Enterobacteriaceae was shown to be one of the characteristics that predicted NAFLD-cirrhosis, reflecting its significant involvement in the development of NAFLD (169). Two strains of Enterobacteriaceae, which belong to the Klebsiella pneumonia family, were fortuitously discovered to produce ethanol among Chinese individuals affected by NAFLD internally. This finding offers valuable knowledge regarding the development of NAFLD in individuals who do not consume alcohol (202). When compared to NASH cirrhosis, individuals with NAFLD-related HCC had lower levels of Akkermansia and Bifidobacterium species, indicating that gut dysbiosis may worsen the development of NAFLD to hepatocarcinogenesis (203, 204).
Esophageal cancer, acknowledged as a highly prevalent form of malignancy worldwide, is projected to have approximately 604,100 novel occurrences in 2020 (205). Nearly 80% of occurrences of this malignant tumor are found in less developed areas, which bear a disproportionately heavy burden. A discrepancy exists in the occurrence and mortality rates between males and females, with males representing approximately 70% of reported cases, resulting in a 2 to 5-fold difference (206). Moreover, the likelihood of developing esophageal cancer increases as individuals grow older, particularly in middle-aged and older demographics (207). In conjunction with the worldwide phenomenon of population growth and aging, the escalating prevalence of risk factors such as alcohol and tobacco consumption, inadequate dietary habits, sedentary lifestyles, and obesity is contributing to the rapid escalation of esophageal cancer globally (208, 209). esophageal cancer comes in two forms: esophageal squamous cell carcinoma (ESCC, also known as SCC) and esophageal adenocarcinoma (AC). AC is more typical in affluent nations, while ESCC is more widespread in East Asia, Southern Africa, East Africa, and Southern Europe (205). Based on the kind of cell from which cancer arises, SCC and AC exhibit significant differences in carcinogenesis (210). In terms of incidence during the previous forty years, AC has been shown to surpass SCC by a wide margin (108). SCC primarily impacts the upper and middle regions of the thoracic esophagus, arising from the squamous cells present within the mucosal lining of the esophagus. AC commences in the epithelial cells, most in the inferior thoracic esophagus (210). Although little is known about the esophageal microbiome, it is recognized that it is not a sterile portion of the digestive system (211). In the esophagus, food passes through quickly, likely limiting the prevalence of microorganisms. However, the pH in healthy people is very steady (about 7), which is ideal for various microbes. The esophagus is home to certain microbes, according to microbiome analysis (212, 213). It is worth mentioning that the composition of microorganisms inhabiting the lower, middle, and upper regions of the esophagus is indistinguishable (214). Most of the esophageal microbiome comprises six phyla, namely Bacteroides, Firmicutes, Fusobacteria, Proteobacteria, Actinobacteria, and TM7 (215, 216). There is a varied microbial community seen among the Gram-positive bacteria. Particularly, the esophagus of healthy people has the highest concentration of the Streptococcus genus (217). In addition, the esophagus also harbors Prevotella and Veillonella (211). The microbiome is changed by esophageal disorders. It is possible to identify esophageal illnesses by the unbalanced changes in the esophageal microbiome (218, 219). Recent research has expanded our understanding of the connection between changes in the gut microbiota and the development of esophageal cancer. It has been proposed that this connection may be essential for the creation and growth of tumors (220). Blackett et al. (221) showed that individuals with Barrett’s esophagus and gastroesophageal reflux disease (GERD) had significantly higher concentrations of Campylobacter. It is believed that campylobacter causes the esophageal mucosa to become inflamed, followed by epithelial metaplasia, which finally results in malignant transformation (222). Elliott and colleagues (223) discovered that certain strains of Lactobacillus are concentrated within tumors in roughly 50% of AC patients and that microbial diversity diminishes in AC while relative Lactobacillus fermentum abundance rises. Zaidi et al. (224) found that AC contains large amounts of E. coli. Additionally, there was a significant increase in the expression of several Toll-like receptors (TLR1, 3, 6, 7, and 9) within the neoplastic tissue of a rat model mimicking AC. Etiological investigations have elucidated that H. pylori has the potential to mitigate the occurrence of AC through the suppression of gastric acid secretion, thereby reducing the likelihood of reflux esophagitis while also modulating the quantity of T cells (225). H. pylori, on the other hand, has been shown to cause GERD to manifest. Several studies have established a correlation between Tannerella forsythia and an increased likelihood of AC. Conversely, symbiotic Streptococcus pneumoniae and Neisseria have been associated with a decreased risk of AC. Notably, the enrichment of Porphyromonas gingivalis (P. gingivalis) has been identified as a significant risk factor for ESCC, as highlighted by various investigations (226–230). P. gingivalis induces the process of epithelial-mesenchymal transformation (EMT) using the transforming-growth-factor (TGF)-dependent Smad/YAP/TAZ signaling pathway, and also triggers the activation of the nuclear factor (NF)-B signaling pathway, thereby stimulating the proliferation and metastasis of ESCC cells (231, 232). It has been proven that the microbiome of the esophagus contains viral DNA from the Gammapapillomavirus, Betaherpesvirus, and Gammaherpesvirus. With the discovery of Papillomavirus (HPV) DNA from esophageal neoplasia, the probability of developing ESCC was increased in the presence of EBV and HPV infections (233). Fungi infections with inflammation are common in esophageal cancer patients, which may suggest a possible link with the development of esophageal cancer (233). In research by Deng et al. (234) comprising 23 esophageal cancer patients and 23 matched healthy persons, the gut microbiome was examined. By 16S rRNA gene sequencing, the gut microbiota was examined from fresh stool samples. When the strain was considered, it was shown that esophageal cancer patients had much larger amounts of Actinobacteria and Firmicutes and lower levels of Bacteroidetes than healthy people. According to scientists, individuals with esophageal cancer had lower levels of bacteria that produce SCFAs while having higher levels of bacteria that produce lipopolysaccharides (LPS) (234, 235). The significance of the pool of SCFAs ought to be underscored as it possesses various benefits, including its ability to mitigate inflammation and enhance the structural integrity of the intestinal barrier. It is of utmost importance to acknowledge that anaerobic microorganisms located in the distal regions of the GI tract synthesize butyrate, thereby suggesting that it may exert a substantial influence on the pathogenesis of neoplastic growths within this system, encompassing esophageal carcinoma (236). In a study conducted on patients with ESCC, it was discovered that the presence of Fusobacteria, Bacteroidetes, and Spirochaetes was notably reduced (n=18) (176). The high-fat diets (HFD) negatively impact the bile acids composition and the gut flora. According to research in mice, the modifications in bile acid composition brought on by HFD may aid in the emergence of Barrett’s esophagus and esophageal cancer (205).
Bacteria are the predominant microorganisms found throughout the GI tract (237). The impact of specific species or the combined bacteriome on GI cancers has been extensively researched (238). However, in recent times, the presence of viruses, fungi, archaea, and microscopic eukaryotes in the GI tract has been confirmed due to the progress made in sequencing technology and biotechnology (233) (Figure 2).
Viruses exhibit a comparatively reduced presence in the gut when compared to bacteria, yet they have been identified as constituents of the enduring commensal microbial consortium within the GI tract (230, 239–241). Viruses have a notable impact on GI cancers. The human virome, encompassing the entirety of viruses found within the human body, is a vital component of the human microbiota and aids in the preservation of tissue equilibrium (242). Bacteriophages have been predominantly recognized within the microbiome, where they are ascribed to various functions. The functions encompassed within this domain encompass the regulation of bacterial populations through the cyclic processes of phages, namely lysogenic and lytic. The proportions of lytic and lysogenic phages are said to have a relationship with the bacteriome and are linked to the overall health condition of an individual (241, 243). Lysogenic bacteriophages might also play a role in the targeted establishment of bacteria and improving the fitness of host bacteria through the exchange of genetic material within the GI tract (243, 244). The involvement of viruses in the development of GI cancers is evident as they impact the abundance of these viruses, infect the cells of the epithelium, or alter the composition of the bacterium (245). A multi-cohort study was conducted in which fecal samples were analyzed using shotgun metagenomics to investigate the virome shift in patients with CRC compared to healthy individuals. Additional examination revealed that there was a fluctuation observed in the colon bacteriophages, with variations evident in both the early and late stages of CRC (246, 247). The analysis conducted subsequently revealed that there was a variation in the displacement of the colon bacteriophages between the initial and advanced stages of CRC (248, 249). Epsilon15likevirus, Betabaculus virus, Punalikevirus, and Mulikevirus exhibited a noteworthy augmentation in CRC individuals, thereby being linked to escalated intensity and fatality rates. It has been suggested that viruses belonging to the eukaryotic colon could potentially disrupt the balance of the immune system and trigger modifications in the DNA via mechanisms that are dependent on the presence of the virus (250). Indeed, there is a growing body of evidence suggesting that infections caused by eukaryotic viruses are linked to an elevated risk of CRC (251). In cancerous tissues of CRC patients, there was a presence of viral infections including HPV, human herpesviruses, human polyomaviruses, human bocavirus, and Inoue-Melnick virus in comparison to the surrounding normal tissues (252, 253). In the same manner that tumor tissues of CRC patients exhibited the presence of viral DNAs, similar findings were observed in the GC tissues. The well-documented role of Epstein-Barr virus (EBV) as an etiological agent in the development of GC further supports this observation. EBV-positive gastric carcinoma is distinguished by distinct genomic abnormalities and clinicopathological characteristics (254, 255). After being infected, the EBV incorporates its DNA into the host organism. Subsequently, it manifests latent protein and disrupts DNA methylation through the influence of miRNA under the presence of the latent protein. This process ultimately leads to the development of EBV-positive GC (255, 256). The prevalence of human cytomegalovirus (HCMV) is extensive within human populations, encompassing a range of infectious microorganisms. HCMV has been documented to endure within the host for extended durations after the initial infection (257). Recent research has placed greater emphasis on the connections between HCMV and several types of malignancies, such as glioblastoma, breast cancer, GC, and CRC (258). HCMV was observed to exhibit a greater presence in GC tissue compared to the surrounding normal tissues, thus suggesting its potential involvement in the development of carcinogenesis and potentially facilitating the lymphatic metastasis process in GC (259). Furthermore, HCMV has also been documented to elicit disturbances in the GI tract, such as inflammatory bowel disease, ulceration, erosion of the cell wall, and hemorrhage in the mucosal lining (258). Reports have also been documented regarding the identification of viruses, particularly bacteriophages, within the esophageal microbiome. DNA viruses including betaherpesvirus, gammaherpesvirus, and gammapapillomavirus were also found (20, 260). Due to their primary focus on bacteria, it is conceivable that the correlation between the virome of the esophagus and adenocarcinoma could be elucidated in investigations involving larger groups of subjects. In addition, it has been reported that infections caused by EBV and HPV are associated with a heightened susceptibility to ESCC (261). Latent gammaherpesvirus 68 infection in a mouse model exhibited the capacity to induce persistent immune system stimulation, thereby safeguarding against pathogenic infection caused by Listeria monocytogenes (262, 263).
Fungi have established their presence as inhabitants of the GI tract of individuals in good health, although their composition is primarily influenced by lifestyle factors, particularly dietary choices (264). These microorganisms have been observed to exist within the gastric compartment, colon, pancreas, and esophagus, albeit in a significantly smaller ratio when compared to bacteria (265, 266). Recent research is commencing to unveil the significance of fungi in the GI tract. A variety of fungi such as Candida, Cryptococcus, Saccharomyces, Malassezia, Debaryomyces, Cladosporium, Trichosporon, and Galactomyces have been documented as inhabiting the gastrointestinal tract of healthy individuals (264). Fungi play a crucial role in sustaining the equilibrium of the GI tract. Furthermore, they have been demonstrated to possess functions in systemic immunity, regulation of inflammatory reactions, and protection against infectious agents (267). Fungi are purported to stimulate the activation of T helper 17 cells, which play a crucial role in safeguarding the host against infections. Moreover, these cells contribute to the development of secondary lymphoid organs and the fine-tuning of the host’s immune and inflammatory responses (268, 269). Fungal species, namely Candida albicans, Saccharomyces cerevisiae, and Candida glabrata, have been detected in the esophagus of individuals in a non-pathogenic state (270). Candida and Phialemonium demonstrate the capacity to endure the harsh acidic conditions prevalent within the ecosystem, specifically within the gastric fluids (271, 272). The ratio between Basidiomycota and Ascomycota was found to be imbalanced in patients with CRC, similar to other diseases affecting the intestines. In individuals with CRC, an elevation in the population of Malasseziomycetes fungi and a decrease in the abundance of Saccharomycetes fungi were noted (273, 274). The composition of fungal genera including Aspergillus, Rhodotorula, Kwoniella, Pseudogymnoascus, Malassezia, Talaromyces, Moniliophthora, Debaryomyces, Pneumocystis, and Nosemia experienced changes in CRC cases, a finding that was confirmed in separate cohorts of Chinese and European populations (275). In an experimental mouse model investigating esophageal cancer, the administration of oral fungi Cladosporium cladosporiodes was found to enhance the severity of ESCC. Interestingly, this detrimental effect was effectively counteracted by treatment with antifungal agents (276). Additionally, infections caused by the C. albicans fungus were documented in patients suffering from ESCC (277). The presence of an imbalance in gastric fungi was observed in individuals with GC. The profile of the fungal community (mycobiome) in GC patients exhibited notable differences, including a decrease in diversity, compared to the control group. Candida and Alternaria exhibited an increased concentration in the GC, whereas Thermomyces and Saitozyma experienced a decrease in abundance within the GC (278, 279). Similar to other GI microbiome members, alterations in the resident mycobiome that impair their functioning, manipulation of the whole microbiome, or infection by specific pathogenic fungus species may all influence GI malignancies (280, 281).
The progress in the field of sequencing and the analytical methods used in bioinformatics have facilitated the examination of archaea, a group that has received less attention in comparison to bacteria, viruses, and fungi within the intestinal ecosystem (282). Archaea represent a distinctive assemblage of prokaryotic organisms characterized by their lack of D-glycerol, esters, fatty acids, and peptidoglycan (283, 284). Owing to their cellular composition, these organisms were recognized for their ability to colonize harsh habitats such as those characterized by high temperatures, alkaline conditions, acidic conditions, and high salinity levels (285). Archaea were commonly presumed to inhabit environments characterized by severe ecological conditions, nevertheless, recent investigations have ascertained their presence in mesophilic conditions comprising human skin, oral cavity, nasal passages, vaginal region, and the GI tract (286). In the GI tract, there have been documented occurrences of both methanogenic archaea and haloarchaea, with their respective proportions being subject to variation dependent on the individual (287). Methanogenic archaea facilitate the reduction of carbon dioxide through the process of methanogenesis, occurring in the GI tract during nutrient digestion. This metabolic activity effectively assists in the elimination of hydrogen from the gut (288, 289). Colonic archaea have also been found to play a role in the elimination of trimethylamine (TMA) from the GI tract. TMA is generated as a byproduct during the degradation of choline mediated by colon microorganisms, and its presence has been linked to elevated probabilities of atherogenesis and the development of cardiovascular ailments (290, 291). The activation of antigen-specific adaptive immune responses by Archaea is a phenomenon that should not be overlooked, as it has the potential to play a crucial role in maintaining immune homeostasis within the GI tract (292). Distinct groups of archaea in the colon were observed in individuals with CRC and colorectal adenoma in comparison to those who were in good health, thus suggesting a modification during the various phases of the development of cancer (293). The presence of Archaea has also been linked to the emergence of IBD, anorexia, and anaerobic abscesses (294, 295) (Table 1).
It is widely established that systemic chemotherapies impact both healthy GI tract cells and cancer cells. The microbiome will undoubtedly experience a disturbance, thus resulting in dysbiosis, which refers to an interruption in the typical makeup of the microbiome. Chemotherapy has been demonstrated to possess a wide-ranging impact on the microbiota, leading to a reduction in the variety of microorganisms. This reduction is accompanied by an elevation in the abundance of Firmicutes and a decline in Bacteroidetes (324). Moreover, gram-negative bacteria tend to increase while gram-positive bacteria decrease due to chemotherapy treatment. Even though various chemotherapy regimens may have distinct effects on the composition of the GI microbiota, this assertion remains valid (324, 325). The gut microbiota controls toxicity, anticancer effects, and medication effectiveness to control host reactions to chemotherapy medicines (326). The TIMER mechanistic paradigm presents an opportunity to alter the connection between the GI microbiota and chemotherapeutic medications using immunomodulation, translocation, enzyme degradation, metabolism, and ecological variation (327). Yamamura and colleagues (328) have discovered a significant association between the intratumoral DNA of F. nucleatum and the response of patients’ ESCC to neoadjuvant treatment. Liu et al. (384) have found that through autophagy, the intracellular bacterium F. nucleatum provides chemoresistance to ESCC cells. By inducing the activation of autophagy and enhancing the expression of autophagy-associated genes, F. nucleatum specifically acts upon the TLR4/MyD88 signaling pathway, leading to a reduction in the levels of miRNA-4802 and miRNA-18a. Consequently, this molecular modulation results in developing resistance to chemotherapy in CRC. In patients with CRC who are undergoing adjuvant chemotherapy, it has been observed that the presence of F. nucleatum infection leads to a reduction in the effectiveness of 5-Fluorouracil (5-FU) treatment by regulating the baculoviral IAP repeat containing 3 (BIRC3) through the TLR4/NF-B signaling pathway (329, 385). In a mouse CRC model, Mycoplasm hyorhinis may metabolize gemcitabine into inactive 2’, 2’-difluoro deoxyuridine via the CDDL gene (330). Furthermore, it has been established that the majority of the bacteria in PDAC are Gammaproteobacteria, which possess the CDDL gene necessary for the metabolization of gemcitabine. Ciprofloxacin can counteract this impact (331). Different commensal microbiota can alter the tumor microenvironment, impacting how well conventional chemotherapy works. By lowering the generation of ROS, the lack of Lactobacillus reduces the cytotoxicity of oxaliplatin (332). In one study, Chinese patients receiving FOLFOX treatment for low-lying rectal tumors had their gut microbiomes examined by fecal samples. It has been demonstrated that FOLFOX reduces the variety of the whole microbiome, and intriguingly, this diversity was reduced in patients who reacted to the FOLFOX rather than in nonresponders (333–335). Lactobacillus rhamnosus, a probiotic, improved the effectiveness of capecitabine against mouse GC growth (336). Cyclophosphamide (CTX) inhibits several immunological signaling cascades to produce its anticancer action (337). Preclinical research has revealed that some bacterial species, such as Enterococcus hirae (E. hirae), are necessary for CTX-induced immunological activation. To activate the host immune response, CTX causes the bacteria to relocate to the spleen and lymph nodes. The anti-tumor action of CTX is likewise dependent on E. hirae and Barnesiella intestinihominis, according to further investigations (338, 339). By controlling antitumor cytotoxic CD8+ T cell responses and stimulating the IL-12 signaling pathway, butyrate may increase the effectiveness of oxaliplatin (340). According to prospective research, individuals with locally advanced rectal cancer (LARC) may benefit from using the gut microbiota as possible biomarkers to gauge how well they respond to neoadjuvant chemotherapy and radiation (341).
The identification of immunotherapy, a therapeutic approach that harnesses the immune system of the body to tackle cancer, has emerged as a highly promising domain in the realm of cancer therapy (342). In the treatment of GI cancers, especially those that exhibit microsatellite instability (MSI-H), the utilization of immune checkpoint inhibitors (ICIs) like anticytotoxic T lymphocyte-associated protein 4 (CTLA-4) and anti-programmed cell death 1 (PD1) antibodies is being implemented (343–345). While dysbiosis can establish a connection between the microbiome and carcinogenesis, it is also plausible that a microbiome in good health possesses substantial potential to bolster antitumor immunity (346, 347). It has been proven that the therapeutic agents pembrolizumab and nivolumab, which function as inhibitors of PD-1, exhibit enhanced clinical efficacy in the prevalence of Akkermansia muciniphila and Bifidobacterium (348, 349). B. fragilis and Bacteroides thetaiotaomicron are also linked to the effectiveness of anti-CTLA-4 antibodies like ipilimumab (350). As the FMT from individuals who responded to ICI and those who did not into mice was carried out, it is noteworthy to observe that the microbiome of ICI responders exhibited a sustained augmentation of the anti-PD1 effects compared to the nonresponders. This observation highlights the intrinsic capability of the microbiome to stimulate the immune response against tumors (351). The interactions between ICI and microbiome highlight the crucial role that the host microbiome and tumor microenvironment may have in forecasting the response to treatment (351, 352). The potential impact of the microbiome on the effectiveness of ICIs suggests that it could also have a substantial role in regulating immune-related adverse events (iRAEs) associated with ICIs (353, 354). In the context of a practical inquiry, individuals afflicted with severe iRAEs exhibited heightened incidences of Streptococcus, Faecalibacterium, and Stenotrophomonas (355, 356). The concept of harnessing the GI microbiota to enhance the production of the anti-inflammatory compound known as butyrate by the gut microbiota to prevent colitis induced by ICI has already been discussed (357). The utilization of antibiotics to eliminate microbiota appears to diminish the efficacy of immunotherapy (358). In fibrosarcoma, melanoma, and CRC mice models, a combination of ampicillin, colistin, and streptomycin was demonstrated to impede the inhibition of CTLA-4 and subsequently revive the growth of tumors (359). A recent investigation discovered that in mice subjected to anti-CTLA-4 treatment, the administration of Bifidobacterium potentially diminishes autoimmune adversities. However, the absence of vancomycin exacerbates immunotherapy-induced colitis (360). The significant microorganisms that serve as predictive biomarkers for immunotherapy response were identified thanks to these studies. A study conducted on a rat colon adenocarcinoma model has identified a group of 11 bacterial strains that could potentially enhance the efficacy of immunotherapy (361). It’s interesting to note that probiotics have been examined as adjuvants in cancer therapies. In a murine model of CRC, the administration of cell lysates derived from Lactobacillus acidophilus in conjunction with a monoclonal antibody targeting CTLA-4 induced a substantial augmentation in CD8+ T lymphocytes, specifically the effector memory subset, along with a noteworthy reduction in regulatory T cells (Tregs). Additionally, the synergistic combination recovered animals with CRC-induced dysbiosis and reduced the aberrant abundance of Proteobacteria in the tumor microenvironment (362). Therefore, using immunotherapy in concert with probiotics may considerably aid the development of innovative therapeutic methods against CRC (363, 364) (Figure 3).
Uncertainty persists over how gut microbiota controls the effectiveness of radiotherapy. Radiotherapy can augment the overall immune response regulated by the immune system in addition to the cytotoxicity of tumors (365) (Figure 3). In addition to causing tumor cell death, local irradiation can boost systemic immunity and inflammation. The therapeutic utility, however, was limited due to adverse outcomes, including bystander effects on adjacent cells, genomic instability, and alterations to commensal microorganisms (366). Research indicates that the microbiota residing in the GI tract could potentially exert a notable influence on the efficacy of radiation therapy (367, 368). The inhibition of apoptosis in cancer cells and the prevention of local immunocyte infiltration were observed when comparing germ-free mice to conventional mice with radiation. The implications of these findings suggest that the commensal microbiota could potentially have a positive impact on the regulation of the body’s reaction to radiotherapy treatment (369, 370). In experimental mice and humans getting radiotherapy, the gut flora is destroyed, which may lead to colitis and diarrhea partially mediated by IL-1β (371). Intestinal cell apoptosis and intestinal barrier function degradation are further side effects of radiotherapy that might result in intestinal inflammation (372). Further investigation revealed that angiopoietin-like 4 (ANGPTL4), a protein lipoprotein lipase inhibitor, plays a crucial role in radiotherapy damage resistance (373). Streptococcus, Lactobacillus, and Bifidobacterium spp stimulated the expression of ANGPTL4 to shield germ-free mice and regular mice from the harmful effects of irradiation (369). Additionally, butyrate, a widely recognized advantageous microbial byproduct, was demonstrated to enhance the efficacy of radiation in preclinical patient-derived CRC organoid models, suggesting the potential utilization of butyrate in combination with other therapies for cancer management (374). Additionally, a clinical investigation showed that formulations including Lactobacillus casei, L. acidophilus, and B. bifidum might reduce the intestinal adverse effects of radiation exposure (375, 376). Lactobacillus rhamnosus, although it possesses the ability to facilitate the recovery of radiation-induced damage to the intestinal mucosa, induce mesenchymal stem cell pre-migration via the TLR2 pathway, and protect the regular intestinal cavity, its effect on the preservation of transplanted tumor tissue is minimal (387). Additional research has revealed that the radioprotective properties of the microflora are mediated by SCFAs, particularly propionate, and specific tryptophan metabolites generated by the microbiota (377). These results offer a possible therapeutic target for reducing radiotherapy-related side effects and alleviating radiation-induced harm (Table 2).
Gastrointestinal (GI) cancer constitutes one of the new cancer cases worldwide and imposes a significant burden on public health, thus presenting a major threat to human population health. Disturbances in the gastrointestinal microbiota may have a significant impact on the development of gastrointestinal cancers. Some bacteria have been found to support the development of cancer, while others appear to protect against it. Studies have shown that changes in the composition and abundance of microbiomes can be associated with the development of various gastrointestinal cancers, such as colon, stomach, liver, and esophageal cancers. In this study, we examine the importance of gut microbiomes in gastrointestinal cancers and their impact on various gastrointestinal cancer treatments, including chemotherapy, immunotherapy, and radiotherapy. The information in this article paves the way for researchers in the field of cancer and microbiome.
AY: Data curation, Writing – original draft. HA: Methodology, Project administration, Supervision, Writing – review & editing.
The author(s) declare that no financial support was received for the research, authorship, and/or publication of this article.
The authors declare that the research was conducted in the absence of any commercial or financial relationships that could be construed as a potential conflict of interest.
All claims expressed in this article are solely those of the authors and do not necessarily represent those of their affiliated organizations, or those of the publisher, the editors and the reviewers. Any product that may be evaluated in this article, or claim that may be made by its manufacturer, is not guaranteed or endorsed by the publisher.
1. Łukaszewicz-Zając M, Pączek S, Mroczko B. A disintegrin and metalloproteinase (ADAM) family—Novel biomarkers of selected gastrointestinal (GI) Malignancies? Cancers (2022) 14(9):2307. doi: 10.3390/cancers14092307
2. Mahjoor M, Afkhami H, Najafi M, Nasr A, Khorrami S. The role of microRNA-30c in targeting interleukin 6, as an inflammatory cytokine, in the mesenchymal stem cell: a therapeutic approach in colorectal cancer. J Cancer Res Clin Oncol (2023) 149(7):3149–60. doi: 10.1007/s00432-022-04123-w
4. Griffin-Sobel JP. Gastrointestinal cancers: screening and early detection. In: Seminars in Oncology Nursing. WB Saunders: Elsevier, (2017).
5. Meyerhardt JA, Tepper JE, Venook AP. Special series: Advances in GI cancer. J Clin Oncol (2015) 33(16):1717. doi: 10.1200/JCO.2015.60.8661
6. Ferlay J, Soerjomataram I, Dikshit R, Eser S, Mathers C, Rebelo M, et al. Cancer incidence and mortality worldwide: sources, methods and major patterns in GLOBOCAN 2012. Int J Cancer (2015) 136(5):E359–86. doi: 10.1002/ijc.29210
7. Shao B-Z, Chai N-L, Yao Y, Li J-P, Law HKW, Linghu E-Q, et al. Autophagy in gastrointestinal cancers. Front Oncol (2022) 12:975758. doi: 10.3389/fonc.2022.975758
8. Zhang B, Wu Q, Li B, Wang D, Wang L, Zhou YL. m 6 A regulator-mediated methylation modification patterns and tumor microenvironment infiltration characterization in gastric cancer. Mol Cancer (2020) 19:1–21. doi: 10.1186/s12943-020-01170-0
9. Schmitt M, Greten FR. The inflammatory pathogenesis of colorectal cancer. Nat Rev Immunol (2021) 21(10):653–67. doi: 10.1038/s41577-021-00534-x
10. Lu Y, Li Y, Liu Q, Tian N, Du P, Zhu F, et al. MondoA–thioredoxin-interacting protein axis maintains regulatory T-cell identity and function in colorectal cancer microenvironment. Gastroenterology (2021) 161(2):575–591.e16. doi: 10.1053/j.gastro.2021.04.041
11. Khezri MR, Malekinejad H, Majidi-Zolbanin N, Ghasemnejad-berenji M. Anticancer potential of metformin: Focusing on gastrointestinal cancers. Cancer Chemotherapy Pharmacol (2021) 87:587–98. doi: 10.1007/s00280-021-04256-8
12. You JH, Song SO, Kang MJ, Cho YY, Kim SW, Suh SH, et al. Metformin and gastrointestinal cancer development in newly diagnosed type 2 diabetes: a population-based study in Korea. Clin Trans Gastroenterol (2020) 11(11). doi: 10.14309/ctg.0000000000000254
13. Grochowska M, Perlejewski K, Laskus T, Radkowski M. The role of gut microbiota in gastrointestinal tract cancers. Archivum Immunologiae Therapiae Experimentalis (2022) 70(1):7. doi: 10.1007/s00005-021-00641-6
14. Chen C-C, Liou J-M, Lee Y-C, Hong T-C, El-Omar EM, Wu M-S. The interplay between Helicobacter pylori and gastrointestinal microbiota. Gut Microbes (2021) 13(1):1909459. doi: 10.1080/19490976.2021.1909459
15. Schmidt TS, Raes J, Bork P. The human gut microbiome: from association to modulation. Cell (2018) 172(6):1198–215. doi: 10.1016/j.cell.2018.02.044
16. Tong Y, Gao H, Qi Q, Liu X, Li J, Gao J, et al. High fat diet, gut microbiome and gastrointestinal cancer. Theranostics (2021) 11(12):5889. doi: 10.7150/thno.56157
17. Fan Y, Pedersen O. Gut microbiota in human metabolic health and disease. Nat Rev Microbiol (2021) 19(1):55–71. doi: 10.1038/s41579-020-0433-9
18. Wei M-Y, Shi S, Liang C, Meng QC, Hua J, Zhang YY, et al. The microbiota and microbiome in pancreatic cancer: more influential than expected. Mol Cancer (2019) 18(1):1–15. doi: 10.1186/s12943-019-1008-0
19. Gacesa R, Kurilshikov A, Vich Vila A, Sinha T, Klaassen MA, Bolte LA, et al. Environmental factors shaping the gut microbiome in a Dutch population. Nature (2022) 604(7907):732–9. doi: 10.1038/s41586-022-04567-7
20. Liu Y, Baba Y, Ishimoto T, Gu X, Zhang J, Nomoto D, et al. Gut microbiome in gastrointestinal cancer: a friend or foe? Int J Biol Sci (2022) 18(10):4101. doi: 10.7150/ijbs.69331
21. Consortium HMP. Structure, function and diversity of the healthy human microbiome. Nature (2012) 486(7402):207–14. doi: 10.1038/nature11234
22. Knight R, Vrbanac A, Taylor BC, Aksenov A, Callewaert C, Debelius J, et al. Best practices for analysing microbiomes. Nat Rev Microbiol (2018) 16(7):410–22. doi: 10.1038/s41579-018-0029-9
23. Yeoh YK, Zuo T, Lui GC, Zhang F, Liu Q, Li AY, et al. Gut microbiota composition reflects disease severity and dysfunctional immune responses in patients with COVID-19. Gut (2021) 70(4):698–706. doi: 10.1136/gutjnl-2020-323020
24. Kurilshikov A, Medina-Gomez C, Bacigalupe R, Radjabzadeh D, Wang J, Demirkan A, et al. Large-scale association analyses identify host factors influencing human gut microbiome composition. Nat Genet (2021) 53(2):156–65. doi: 10.1038/s41588-020-00763-1
25. Cotillard A, Kennedy SP, Kong LC, Prifti E, Pons N, Le Chatelier E, et al. Dietary intervention impact on gut microbial gene richness. Nature (2013) 500(7464):585–8. doi: 10.1038/nature12480
26. Rothschild D, Weissbrod O, Barkan E, Kurilshikov A, Korem T, Zeevi D, et al. Environment dominates over host genetics in shaping human gut microbiota. Nature (2018) 555(7695):210–5. doi: 10.1038/nature25973
27. Stolfi C, Maresca C, Monteleone G, Laudisi F. Implication of intestinal barrier dysfunction in gut dysbiosis and diseases. Biomedicines (2022) 10(2):289. doi: 10.3390/biomedicines10020289
28. Lloyd-Price J, Abu-Ali G, Huttenhower C. The healthy human microbiome. Genome Med (2016) 8(1):1–11. doi: 10.1186/s13073-016-0307-y
29. Levy M, Kolodziejczyk AA, Thaiss CA, Elinay E. Dysbiosis and the immune system. Nat Rev Immunol (2017) 17(4):219–32. doi: 10.1038/nri.2017.7
30. Matson V, Chervin CS, Gajewski TF. Cancer and the microbiome—influence of the commensal microbiota on cancer, immune responses, and immunotherapy. Gastroenterology (2021) 160(2):600–13. doi: 10.1053/j.gastro.2020.11.041
31. Martinez-Guryn K, Leone V, Chang EB. Regional diversity of the gastrointestinal microbiome. Cell Host Microbe (2019) 26(3):314–24. doi: 10.1016/j.chom.2019.08.011
32. Dicks LM. Gut bacteria and neurotransmitters. Microorganisms (2022) 10(9):1838. doi: 10.3390/microorganisms10091838
33. Friedman ES, Bittinger K, Esipova TV, Hou L, Chau L, Jiang J, et al. Microbes vs. chemistry in the origin of the anaerobic gut lumen. Proc Natl Acad Sci (2018) 115(16):4170–5. doi: 10.1073/pnas.1718635115
34. Olm MR, Crits-Christoph A, Bouma-Gregson K, Firek BA, Morowitz MJ, Banfield JF. inStrain profiles population microdiversity from metagenomic data and sensitively detects shared microbial strains. Nat Biotechnol (2021) 39(6):727–36. doi: 10.1038/s41587-020-00797-0
35. Karygianni L, Ren Z, Koo H, Thurnheer T. Biofilm matrixome: extracellular components in structured microbial communities. Trends Microbiol (2020) 28(8):668–81. doi: 10.1016/j.tim.2020.03.016
36. Church DL, Cerutti L, Gürtler A, Griener T, Zelazny A, Emler S. Performance and application of 16S rRNA gene cycle sequencing for routine identification of bacteria in the clinical microbiology laboratory. Clin Microbiol Rev (2020) 33(4):10.1128/cmr. 00053–19. doi: 10.1128/CMR.00053-19
37. Rothhammer V, Borucki DM, Tjon EC, Takenaka MC, Chao CC, Ardura-Fabregat A, et al. Microglial control of astrocytes in response to microbial metabolites. Nature (2018) 557(7707):724–8. doi: 10.1038/s41586-018-0119-x
38. Moran MA, Kujawinski EB, Schroer WF, Amin SA, Bates NR, Bertrand EM, et al. Microbial metabolites in the marine carbon cycle. Nat Microbiol (2022) 7(4):508–23. doi: 10.1038/s41564-022-01090-3
39. Schwabe RF, Greten TF. Gut microbiome in HCC–Mechanisms, diagnosis and therapy. J Hepatol (2020) 72(2):230–8. doi: 10.1016/j.jhep.2019.08.016
40. De Pessemier B, Grine L, Debaere M, Maes A, Paetzold B, Callewaert C. Gut–skin axis: current knowledge of the interrelationship between microbial dysbiosis and skin conditions. Microorganisms (2021) 9(2):353. doi: 10.3390/microorganisms9020353
41. Scott AJ, Alexander JL, Merrifield CA, Cunningham D, Jobin C, Brown R, et al. International Cancer Microbiome Consortium consensus statement on the role of the human microbiome in carcinogenesis. Gut (2019) 68(9):1624–32. doi: 10.1136/gutjnl-2019-318556
42. Helmink BA, Khan MAW, Hermann A, Gopalakrishnan V, Wargo JA. The microbiome, cancer, and cancer therapy. Nat Med (2019) 25(3):377–88. doi: 10.1038/s41591-019-0377-7
43. Song M, Chan AT, Sun J. Influence of the gut microbiome, diet, and environment on risk of colorectal cancer. Gastroenterology (2020) 158(2):322–40. doi: 10.1053/j.gastro.2019.06.048
44. Siegel RL, Miller KD, Fedewa SA, Ahnen DJ, Meester RG, Barzi A, et al. Colorectal cancer statistics, 2017. CA: Cancer J Clin (2017) 67(3):177–93. doi: 10.3322/caac.21395
45. Song M, Chan AT. Environmental factors, gut microbiota, and colorectal cancer prevention. Clin Gastroenterol Hepatol (2019) 17(2):275–89. doi: 10.1016/j.cgh.2018.07.012
46. André T, Shiu KK, Kim TW, Jensen BV, Jensen LH, Punt C, et al. Pembrolizumab in microsatellite-instability–high advanced colorectal cancer. New Engl J Med (2020) 383(23):2207–18. doi: 10.1056/NEJMoa2017699
47. Bolyen E, Rideout JR, Dillon MR, Bokulich NA, Abnet CC, Al-Ghalith GA, et al. Reproducible, interactive, scalable and extensible microbiome data science using QIIME 2. Nat Biotechnol (2019) 37(8):852–7. doi: 10.1038/s41587-019-0209-9
48. Biller LH, Schrag D. Diagnosis and treatment of metastatic colorectal cancer: a review. Jama (2021) 325(7):669–85. doi: 10.1001/jama.2021.0106
49. Roy S, Trinchieri G. Microbiota: a key orchestrator of cancer therapy. Nat Rev Cancer (2017) 17(5):271–85. doi: 10.1038/nrc.2017.13
50. Abdill RJ, Adamowicz EM, Blekhman R. Public human microbiome data are dominated by highly developed countries. PloS Biol (2022) 20(2):e3001536. doi: 10.1371/journal.pbio.3001536
51. Fukunaga S, Nakano D, Kawaguchi T, Eslam M, Ouchi A, Nagata T, et al. Non-obese MAFLD is associated with colorectal adenoma in health check examinees: a multicenter retrospective study. Int J Mol Sci (2021) 22(11):5462. doi: 10.3390/ijms22115462
52. Bultman SJ. Interplay between diet, gut microbiota, epigenetic events, and colorectal cancer. Mol Nutr Food Res (2017) 61(1):1500902. doi: 10.1002/mnfr.201500902
53. Klünemann M, Andrejev S, Blasche S, Mateus A, Phapale P, Devendran S, et al. Bioaccumulation of therapeutic drugs by human gut bacteria. Nature (2021) 597(7877):533–8. doi: 10.1038/s41586-021-03891-8
54. Almeida A, Mitchell AL, Boland M, Forster SC, Gloor GB, Tarkowska A, et al. A new genomic blueprint of the human gut microbiota. Nature (2019) 568(7753):499–504. doi: 10.1038/s41586-019-0965-1
55. Chen S, Zhang L, Li M, Zhang Y, Sun M, Wang L, et al. Fusobacterium nucleatum reduces METTL3-mediated m6A modification and contributes to colorectal cancer metastasis. Nat Commun (2022) 13(1):1248. doi: 10.1038/s41467-022-28913-5
56. Xu C, Fan L, Lin Y, Shen W, Qi Y, Zhang Y, et al. Fusobacterium nucleatum promotes colorectal cancer metastasis through miR-1322/CCL20 axis and M2 polarization. Gut Microbes (2021) 13(1):1980347. doi: 10.1080/19490976.2021.1980347
57. Flanagan L, Schmid J, Ebert M, Soucek P, Kunicka T, Liska V, et al. Fusobacterium nucleatum associates with stages of colorectal neoplasia development, colorectal cancer and disease outcome. Eur J Clin Microbiol Infect Dis (2014) 33:1381–90. doi: 10.1007/s10096-014-2081-3
58. Tahara T, Yamamoto E, Suzuki H, Maruyama R, Chung W, Garriga J, et al. Fusobacterium in colonic flora and molecular features of colorectal carcinoma. Cancer Res (2014) 74(5):1311–8. doi: 10.1158/0008-5472.CAN-13-1865
59. Zhang Y, Zhang L, Zheng S, Li M, Xu C, Jia D, et al. Fusobacterium nucleatum promotes colorectal cancer cells adhesion to endothelial cells and facilitates extravasation and metastasis by inducing ALPK1/NF-κB/ICAM1 axis. Gut Microbes (2022) 14(1):2038852. doi: 10.1080/19490976.2022.2038852
60. Ito M, Kanno S, Nosho K, Sukawa Y, Mitsuhashi K, Kurihara H, et al. Association of Fusobacterium nucleatum with clinical and molecular features in colorectal serrated pathway. Int J Cancer (2015) 137(6):1258–68. doi: 10.1002/ijc.29488
61. Huang G, Chen J, Zhou J, Xiao S, Zeng W, Xia J, et al. Epigenetic modification and BRAF gene mutation in thyroid carcinoma. Cancer Cell Int (2021) 21(1):1–14. doi: 10.1186/s12935-021-02405-w
62. Wang H, Luo K, Guan Z, Li Z, Xiang J, Ou S, et al. Identification of the crucial role of CCL22 in F. nucleatum-related colorectal tumorigenesis that correlates with tumor microenvironment and immune checkpoint therapy. Front Genet (2022) 13:811900. doi: 10.3389/fgene.2022.811900
63. Rashtak S, Rego R, Sweetser SR, Sinicrope FA. Sessile serrated polyps and colon cancer prevention. Cancer Prev Res (2017) 10(5):270–8. doi: 10.1158/1940-6207.CAPR-16-0264
64. Purcell R, Visnovska M, Biggs PJ, Schmeier S, Frizelle FA. Distinct gut microbiome patterns associate with consensus molecular subtypes of colorectal cancer. Sci Rep (2017) 7(1):11590. doi: 10.1038/s41598-017-11237-6
65. Guinney J, Dienstmann R, Wang X, De Reynies A, Schlicker A, Soneson C, et al. The consensus molecular subtypes of colorectal cancer. Nat Med (2015) 21(11):1350–6. doi: 10.1038/nm.3967
66. Bullman S, Pedamallu CS, Sicinska E, Clancy TE, Zhang X, Cai D, et al. Analysis of Fusobacterium persistence and antibiotic response in colorectal cancer. Science (2017) 358(6369):1443–8. doi: 10.1126/science.aal5240
67. Cao Y, Wang Z, Yan Y, Ji L, He J, Xuan B, et al. Enterotoxigenic Bacteroides fragilis promotes intestinal inflammation and Malignancy by inhibiting exosome-packaged miR-149-3p. Gastroenterology (2021) 161(5):1552–1566.e12. doi: 10.1053/j.gastro.2021.08.003
68. Allen J, Rosendahl Huber A, Pleguezuelos-Manzano C, Puschhof J, Wu S, Wu X, et al. Colon Tumors in Enterotoxigenic Bacteroides fragilis (ETBF)-colonized mice do not display a unique mutational signature but instead possess host-dependent alterations in the APC gene. Microbiol Spectr (2022) 10(3):e01055–22. doi: 10.1128/spectrum.01055-22
69. Chung L, Orberg ET, Geis AL, Chan JL, Fu K, Shields CE, et al. Bacteroides fragilis toxin coordinates a pro-carcinogenic inflammatory cascade via targeting of colonic epithelial cells. Cell Host Microbe (2018) 23(2):203–214.e5. doi: 10.1016/j.chom.2018.01.007
70. Geis AL, Fan H, Wu X, Wu S, Huso DL, Wolfe JL, et al. Regulatory T-cell response to enterotoxigenic Bacteroides fragilis colonization triggers IL17-dependent colon carcinogenesis. Cancer Discovery (2015) 5(10):1098–109. doi: 10.1158/2159-8290.CD-15-0447
71. Hwang S, Lee CG, Jo M, Park CO, Gwon SY, Hwang S, et al. Enterotoxigenic Bacteroides fragilis infection exacerbates tumorigenesis in AOM/DSS mouse model. Int J Med Sci (2020) 17(2):145. doi: 10.7150/ijms.38371
72. Orberg ET, Fan H, Tam AJ, Dejea CM, Shields CD, Wu S, et al. The myeloid immune signature of enterotoxigenic Bacteroides fragilis-induced murine colon tumorigenesis. Mucosal Immunol (2017) 10(2):421–33. doi: 10.1038/mi.2016.53
73. Dai Z, Coker OO, Nakatsu G, Wu WK, Zhao L, Chen Z, et al. Multi-cohort analysis of colorectal cancer metagenome identified altered bacteria across populations and universal bacterial markers. Microbiome (2018) 6(1):1–12. doi: 10.1186/s40168-018-0451-2
74. Arima K, Zhong R, Ugai T, Zhao M, Haruki K, Akimoto N, et al. Western-Style diet, PKs island-carrying Escherichia coli, and colorectal cancer: analyses from two large prospective cohort studies. Gastroenterology (2022) 163(4):862–74. doi: 10.1053/j.gastro.2022.06.054
75. Silpe JE, Wong JWH, Owen SV, Baym M, Balskus EP. The bacterial toxin colibactin triggers prophage induction. Nature (2022) 603(7900):315–20. doi: 10.1038/s41586-022-04444-3
76. Iftekhar A, Berger H, Bouznad N, Heuberger J, Boccellato F, Dobrindt U, et al. Genomic aberrations after short-term exposure to colibactin-producing E. coli transform primary colon epithelial cells. Nat Commun (2021) 12(1):1003. doi: 10.1038/s41467-021-21162-y
77. Dougherty MW, Jobin C. Shining a light on colibactin biology. Toxins (2021) 13(5):346. doi: 10.3390/toxins13050346
78. Velilla JA, Volpe MR, Kenney GE, Walsh RMJ, Balskus EP, Gaudet R. Structural basis of colibactin activation by the ClbP peptidase. Nat Chem Biol (2023) 19(2):151–8. doi: 10.1038/s41589-022-01142-z
79. Cougnoux A, Dalmasso G, Martinez R, Buc E, Delmas J, Gibold L, et al. Bacterial genotoxin colibactin promotes colon tumour growth by inducing a senescence-associated secretory phenotype. Gut (2014) 63(12):1932–42. doi: 10.1136/gutjnl-2013-305257
80. Lopès A, Billard E, Casse AH, Villéger R, Veziant J, Roche G, et al. Colibactin-positive Escherichia coli induce a procarcinogenic immune environment leading to immunotherapy resistance in colorectal cancer. Int J Cancer (2020) 146(11):3147–59. doi: 10.1002/ijc.32920
81. Moon J, Kim H, Lee D, Seo J. Development of a novel endolysin, PanLys. 1, for the specific inhibition of Peptostreptococcus anaerobius. Anim Bioscience (2023) 36(8):1285. doi: 10.5713/ab.22.0454
82. Gu J, Lv X, Li W, Li G, He X, Zhang Y, et al. Deciphering the Mechanism of Peptostreptococcus anaerobius induced Chemoresistance in Colorectal Cancer: The Important Roles of MDSCs Recruitment and EMT Activation. Front Immunol (2023) 14:1230681. doi: 10.3389/fimmu.2023.1230681
83. Feng Q, Liang S, Jia H, Stadlmayr A, Tang L, Lan Z, et al. Gut microbiome development along the colorectal adenoma–carcinoma sequence. Nat Commun (2015) 6(1):6528. doi: 10.1038/ncomms7528
84. Yu J, Feng Q, Wong SH, Zhang D, Liang Qy, Qin Y, et al. Metagenomic analysis of faecal microbiome as a tool towards targeted non-invasive biomarkers for colorectal cancer. Gut (2017) 66(1):70–8. doi: 10.1136/gutjnl-2015-309800
85. Long X, Wong CC, Tong L, Chu ES, Ho Szeto C, Go MY, et al. Peptostreptococcus anaerobius promotes colorectal carcinogenesis and modulates tumour immunity. Nat Microbiol (2019) 4(12):2319–30. doi: 10.1038/s41564-019-0541-3
86. Tsoi H, Chu ES, Zhang X, Sheng J, Nakatsu G, Ng SC, et al. Peptostreptococcus anaerobius induces intracellular cholesterol biosynthesis in colon cells to induce proliferation and causes dysplasia in mice. Gastroenterology (2017) 152(6):1419–1433.e5. doi: 10.1053/j.gastro.2017.01.009
87. Yang Y, Cai Q, Shu X-O, Steinwandel MD, Blot WJ, Zheng W, et al. Prospective study of oral microbiome and colorectal cancer risk in low-income and African American populations. Int J Cancer (2019) 144(10):2381–9. doi: 10.1002/ijc.31941
88. Flemer B, Warren RD, Barrett MP, Cisek K, Das A, Jeffery IB, et al. The oral microbiota in colorectal cancer is distinctive and predictive. Gut (2018) 67(8):1454–63. doi: 10.1136/gutjnl-2017-314814
89. Ma L, Ni Y, Wang Z, Tu W, Ni L, Zhuge F, et al. Spermidine improves gut barrier integrity and gut microbiota function in diet-induced obese mice. Gut Microbes (2020) 12(1):1832857. doi: 10.1080/19490976.2020.1832857
90. Korpela K, Helve O, Kolho KL, Saisto T, Skogberg K, Dikareva E, et al. Maternal fecal microbiota transplantation in cesarean-born infants rapidly restores normal gut microbial development: a proof-of-concept study. Cell (2020) 183(2):324–334.e5. doi: 10.1016/j.cell.2020.08.047
91. Wong SH, Zhao L, Zhang X, Nakatsu G, Han J, Xu W, et al. Gavage of fecal samples from patients with colorectal cancer promotes intestinal carcinogenesis in germ-free and conventional mice. Gastroenterology (2017) 153(6):1621–1633.e6. doi: 10.1053/j.gastro.2017.08.022
92. Li L, Li X, Zhong W, Yang M, Xu M, Sun Y, et al. Gut microbiota from colorectal cancer patients enhances the progression of intestinal adenoma in Apcmin/+ mice. EBioMedicine (2019) 48:301–15. doi: 10.1016/j.ebiom.2019.09.021
93. Kim HS, Whon TW, Sung H, Jeong YS, Jung ES, Shin NR, et al. Longitudinal evaluation of fecal microbiota transplantation for ameliorating calf diarrhea and improving growth performance. Nat Commun (2021) 12(1):161. doi: 10.1038/s41467-020-20389-5
94. DeFilipp Z, Bloom PP, Torres Soto M, Mansour MK, Sater MR, Huntley MH, et al. Drug-resistant E. coli bacteremia transmitted by fecal microbiota transplant. New Engl J Med (2019) 381(21):2043–50. doi: 10.1056/NEJMoa1910437
95. O'keefe SJ. Diet, microorganisms and their metabolites, and colon cancer. Nat Rev Gastroenterol Hepatol (2016) 13(12):691–706. doi: 10.1038/nrgastro.2016.165
96. Yang J, Wei H, Zhou Y, Szeto CH, Li C, Lin Y, et al. High-fat diet promotes colorectal tumorigenesis through modulating gut microbiota and metabolites. Gastroenterology (2022) 162(1):135–149.e2. doi: 10.1053/j.gastro.2021.08.041
97. Makki K, Deehan EC, Walter J, Bäckhed F. The impact of dietary fiber on gut microbiota in host health and disease. Cell Host Microbe (2018) 23(6):705–15. doi: 10.1016/j.chom.2018.05.012
98. Li J, Zhu Y, Yang L, Wang Z. Effect of gut microbiota in the colorectal cancer and potential target therapy. Discover Oncol (2022) 13(1):51. doi: 10.1007/s12672-022-00517-x
99. Hwang S, Yi HC, Hwang S, Jo M, Rhee K-J. Dietary salt administration decreases enterotoxigenic Bacteroides fragilis (ETBF)-promoted tumorigenesis via inhibition of colonic inflammation. Int J Mol Sci (2020) 21(21):8034. doi: 10.3390/ijms21218034
100. Serna G, Ruiz-Pace F, Hernando J, Alonso L, Fasani R, Landolfi S, et al. Fusobacterium nucleatum persistence and risk of recurrence after preoperative treatment in locally advanced rectal cancer. Ann Oncol (2020) 31(10):1366–75. doi: 10.1016/j.annonc.2020.06.003
101. Liu H, Du J, Chao S, Li S, Cai H, Zhang H, et al. Fusobacterium nucleatum promotes colorectal cancer cell to acquire stem cell-like features by manipulating lipid droplet-mediated numb degradation. Advanced Sci (2022) 9(12):2105222. doi: 10.1002/advs.202105222
102. Gao Y, Bi D, Xie R, Li M, Guo J, Liu H, et al. Fusobacterium nucleatum enhances the efficacy of PD-L1 blockade in colorectal cancer. Signal transduction targeted Ther (2021) 6(1):398. doi: 10.1038/s41392-021-00795-x
103. McIlvanna E, Linden GJ, Craig SG, Lundy FT, James JA. Fusobacterium nucleatum and oral cancer: a critical review. BMC Cancer (2021) 21:1–11. doi: 10.1186/s12885-021-08903-4
104. Huang P, Wong CC. Microbiome in Colorectal Cancer. In: Microbiome in Gastrointestinal Cancer. Springer Singapore: Springer, (2023). p. 23–39.
105. DeStefano Shields CE, White JR, Chung L, Wenzel A, Hicks JL, Tam AJ, et al. Bacterial-driven inflammation and mutant BRAF expression combine to promote murine colon tumorigenesis that is sensitive to immune checkpoint therapy. Cancer Discovery (2021) 11(7):1792–807. doi: 10.1158/2159-8290.CD-20-0770
106. Owens JA, Saeedi BJ, Naudin CR, Hunter-Chang S, Barbian ME, Eboka RU, et al. Lactobacillus rhamnosus GG orchestrates an antitumor immune response. Cell Mol Gastroenterol Hepatol (2021) 12(4):1311–27. doi: 10.1016/j.jcmgh.2021.06.001
107. Mager LF, Burkhard R, Pett N, Cooke NC, Brown K, Ramay H, et al. Microbiome-derived inosine modulates response to checkpoint inhibitor immunotherapy. Science (2020) 369(6510):1481–9. doi: 10.1126/science.abc3421
108. Sung H, Ferlay J, Siegel RL, Laversanne M, Soerjomataram I, Jemal A, et al. Global cancer statistics 2020: GLOBOCAN estimates of incidence and mortality worldwide for 36 cancers in 185 countries. CA: Cancer J Clin (2021) 71(3):209–49. doi: 10.3322/caac.21660
109. Xia JY, Aadam AA. Advances in screening and detection of gastric cancer. J Surg Oncol (2022) 125(7):1104–9. doi: 10.1002/jso.26844
110. Nasr R, Shamseddine A, Mukherji D, Nassar F, Temraz S. The crosstalk between microbiome and immune response in gastric cancer. Int J Mol Sci (2020) 21(18):6586. doi: 10.3390/ijms21186586
111. Serrano C, Harris PR, Smith PD, Bimczok D. Interactions between H. pylori and the gastric microbiome: Impact on gastric homeostasis and disease. Curr Opin Physiol (2021) 21:57–64. doi: 10.1016/j.cophys.2021.04.003
112. Elinav E, Garrett WS, Trinchieri G, Wargo J. The cancer microbiome. Nat Rev Cancer (2019) 19(7):371–6. doi: 10.1038/s41568-019-0155-3
113. Tshibangu-Kabamba E, Yamaoka Y. Helicobacter pylori infection and antibiotic resistance—from biology to clinical implications. Nat Rev Gastroenterol Hepatol (2021) 18(9):613–29. doi: 10.1038/s41575-021-00449-x
114. Sexton RE, Al Hallak MA, Diab M, Azmi AS. Gastric cancer: a comprehensive review of current and future treatment strategies. Cancer Metastasis Rev (2020) 39:1179–203. doi: 10.1007/s10555-020-09925-3
115. Rick JR, Goldman M, Semino-Mora C, Liu H, Olsen C, Rueda-Pedraza E, et al. In situ expression of cagA and risk of gastroduodenal disease in Helicobacter pylori infected children. J Pediatr Gastroenterol Nutr (2010) 50(2):167. doi: 10.1097/MPG.0b013e3181bab326
116. Hatakeyama M. Oncogenic mechanisms of the Helicobacter pylori CagA protein. Nat Rev Cancer (2004) 4(9):688–94. doi: 10.1038/nrc1433
117. Jones KR, Joo YM, Jang S, Yoo YJ, Lee HS, Chung IS, et al. Polymorphism in the CagA EPIYA motif impacts development of gastric cancer. J Clin Microbiol (2009) 47(4):959–68. doi: 10.1128/JCM.02330-08
118. Kim KE. Gastric cancer in Korean Americans: risks and reductions Vol. 13. Korean and Korean-American: Korean and Korean-American studies bulletin, (2003). p. 84.
119. Hooi JK, Lai WY, Ng WK, Suen MM, Underwood FE, Tanyingoh D, et al. Global prevalence of Helicobacter pylori infection: systematic review and meta-analysis. Gastroenterology (2017) 153(2):420–9. doi: 10.1053/j.gastro.2017.04.022
120. Fallone CA, Chiba N, van Zanten SV, Fischbach L, Gisbert JP, Hunt RH, et al. The Toronto consensus for the treatment of Helicobacter pylori infection in adults. Gastroenterology (2016) 151(1):51–69.e14. doi: 10.1053/j.gastro.2016.04.006
121. Chen Y-H, Tsai WH, Wu HY, Chen CY, Yeh WL, Chen YH, et al. Probiotic lactobacillus. spp. act against Helicobacter pylori-induced inflammation. J Clin Med (2019) 8(1):90. doi: 10.3390/jcm8010090
122. Alipour M. Molecular mechanism of Helicobacter pylori-induced gastric cancer. J gastrointestinal Cancer (2021) 52:23–30. doi: 10.1007/s12029-020-00518-5
123. Bravo D, et al. Helicobacter pylori in human health and disease: Mechanisms for local gastric and systemic effects. World J Gastroenterol (2018) 24(28):3071. doi: 10.3748/wjg.v24.i28.3071
124. Fagoonee S, Pellicano R. Helicobacter pylori: molecular basis for colonization and survival in gastric environment and resistance to antibiotics. A short review. Infect Dis (2019) 51(6):399–408. doi: 10.1080/23744235.2019.1588472
125. Li Q, Yu H. The role of non-H. pylori bacteria in the development of gastric cancer. Am J Cancer Res (2020) 10(8):2271.
126. Zhang Y, Zhang S, Li B, Luo Y, Gong Y, Jin X, et al. Gut microbiota dysbiosis promotes age-related atrial fibrillation by lipopolysaccharide and glucose-induced activation of NLRP3-inflammasome. Cardiovasc Res (2022) 118(3):785–97. doi: 10.1093/cvr/cvab114
127. Ling Z, Zhu M, Yan X, Cheng Y, Shao L, Liu X, et al. Structural and functional dysbiosis of fecal microbiota in Chinese patients with Alzheimer's disease. Front Cell Dev Biol (2021) 8:634069. doi: 10.3389/fcell.2020.634069
128. Ng SC, Kamm MA, Yeoh YK, Chan PK, Zuo T, Tang W, et al. Scientific frontiers in faecal microbiota transplantation: joint document of Asia-Pacific Association of Gastroenterology (APAGE) and Asia-Pacific Society for Digestive Endoscopy (APSDE). Gut (2020) 69(1):83–91. doi: 10.1136/gutjnl-2019-319407
129. Xu H-M, Huang HL, Xu J, He J, Zhao C, Peng Y, et al. Cross-talk between butyric acid and gut microbiota in ulcerative colitis following fecal microbiota transplantation. Front Microbiol (2021) 12:658292. doi: 10.3389/fmicb.2021.658292
130. Xu H-M, Huang HL, Zhou YL, Zhao HL, Xu J, Shou DW, et al. Fecal microbiota transplantation: a new therapeutic attempt from the gut to the brain. Gastroenterol Res Pract (2021) 2021. doi: 10.1155/2021/6699268
131. Bruno G, Zaccari P, Rocco G, Scalese G, Panetta C, Porowska B, et al. Proton pump inhibitors and dysbiosis: Current knowledge and aspects to be clarified. World J Gastroenterol (2019) 25(22):2706. doi: 10.3748/wjg.v25.i22.2706
132. Malfertheiner P, Kandulski A, Venerito M. Proton-pump inhibitors: understanding the complications and risks. Nat Rev Gastroenterol Hepatol (2017) 14(12):697–710. doi: 10.1038/nrgastro.2017.117
133. Durán C, Ciucci S, Palladini A, Ijaz UZ, Zippo AG, Sterbini FP, et al. Nonlinear machine learning pattern recognition and bacteria-metabolite multilayer network analysis of perturbed gastric microbiome. Nat Commun (2021) 12(1):1926. doi: 10.1038/s41467-021-22135-x
134. Duysburgh C, Abbeele PVd, Franckenstein D, Westphal M, Kuchinka-Koch A, Marzorati M. Co-administration of lactulose crystals with amoxicillin followed by prolonged lactulose treatment promotes recovery of the human gut microbiome in vitro. Antibiotics (2022) 11(7):962. doi: 10.3390/antibiotics11070962
135. Huang Y, Sheth RU, Zhao S, Cohen LA, Dabaghi K, Moody T, et al. High-throughput microbial culturomics using automation and machine learning. Nat Biotechnol (2023) p:1–10. doi: 10.1038/s41587-023-01674-2
136. Parsons BN, Ijaz UZ, D’Amore R, Burkitt MD, Eccles R, Lenzi L, et al. Comparison of the human gastric microbiota in hypochlorhydric states arising as a result of Helicobacter pylori-induced atrophic gastritis, autoimmune atrophic gastritis and proton pump inhibitor use. PloS Pathog (2017) 13(11):e1006653. doi: 10.1371/journal.ppat.1006653
137. Baker M, Perazella MA. NSAIDs in CKD: are they safe? Am J Kidney Dis (2020) 76(4):546–57. doi: 10.1053/j.ajkd.2020.03.023
138. Hsieh S-Y, Lian YZ, Lin IH, Yang YC, Tinkov AA, Skalny AV, et al. Combined Lycium barbarum polysaccharides and C-phycocyanin increase gastric Bifidobacterium relative abundance and protect against gastric ulcer caused by aspirin in rats. Nutr Metab (2021) 18(1):1–16. doi: 10.1186/s12986-020-00538-9
139. Park H, Cho D, Huang E, Seo JY, Kim WG, Todorov SD, et al. Amelioration of alcohol induced gastric ulcers through the administration of Lactobacillus plantarum APSulloc 331261 isolated from green tea. Front Microbiol (2020) 11:420. doi: 10.3389/fmicb.2020.00420
140. Nadatani Y, Watanabe T, Suda W, Nakata A, Matsumoto Y, Kosaka S, et al. Gastric acid inhibitor aggravates indomethacin-induced small intestinal injury via reducing Lactobacillus johnsonii. Sci Rep (2019) 9(1):17490. doi: 10.1038/s41598-019-53559-7
141. Burgermeister E, Ebert MP. Microbiome in Gastric Cancer. In: Microbiome in Gastrointestinal Cancer, vol. Singapore: Springer Nature Singapore, (2023). p. 41–66.
142. Sung JJ, Coker OO, Chu E, Szeto CH, Luk ST, Lau HC, et al. Gastric microbes associated with gastric inflammation, atrophy and intestinal metaplasia 1 year after Helicobacter pylori eradication. Gut (2020) 69(9):1572–81. doi: 10.1136/gutjnl-2019-319826
143. Yang J, Wang J, Zhou H, Wang Y, Huang H, Jin H, et al. Endoscopic radiofrequency ablation plus a novel oral 5-fluorouracil compound versus radiofrequency ablation alone for unresectable extrahepatic cholangiocarcinoma. Gastrointestinal endoscopy (2020) 92(6):1204–1212.e1. doi: 10.1016/j.gie.2020.04.075
144. Watanabe T, Nadatani Y, Suda W, Higashimori A, Otani K, Fukunaga S, et al. Long-term persistence of gastric dysbiosis after eradication of Helicobacter pylori in patients who underwent endoscopic submucosal dissection for early gastric cancer. Gastric Cancer (2021) 24:710–20. doi: 10.1007/s10120-020-01141-w
145. Beukema M, Faas MM, de Vos P. The effects of different dietary fiber pectin structures on the gastrointestinal immune barrier: impact via gut microbiota and direct effects on immune cells. Exp Mol Med (2020) 52(9):1364–76. doi: 10.1038/s12276-020-0449-2
146. He C, Peng C, Wang H, Ouyang Y, Zhu Z, Shu X, et al. The eradication of Helicobacter pylori restores rather than disturbs the gastrointestinal microbiota in asymptomatic young adults. Helicobacter (2019) 24(4):e12590. doi: 10.1111/hel.12590
147. Anwanwan D, Singh SK, Singh S, Saikam V, Singh R. Challenges in liver cancer and possible treatment approaches. Biochim Biophys Acta (BBA)-Reviews Cancer (2020) 1873(1):188314. doi: 10.1016/j.bbcan.2019.188314
148. Li Y, Zhang R, Xu Z, Wang Z. Advances in nanoliposomes for the diagnosis and treatment of liver cancer. Int J nanomedicine (2022) p:909–25. doi: 10.2147/IJN.S349426
149. Yang JD, Hainaut P, Gores GJ, Amadou A, Plymoth A, Roberts LR. A global view of hepatocellular carcinoma: trends, risk, prevention and management. Nat Rev Gastroenterol Hepatol (2019) 16(10):589–604. doi: 10.1038/s41575-019-0186-y
150. Bray F, Laversanne M, Weiderpass E, Soerjomataram I. The ever-increasing importance of cancer as a leading cause of premature death worldwide. Cancer (2021) 127(16):3029–30. doi: 10.1002/cncr.33587
151. Galicia-Moreno M, Silva-Gomez JA, Lucano-Landeros S, Santos A, Monroy-Ramirez HC, Armendariz-Borunda J. Liver cancer: therapeutic challenges and the importance of experimental models. Can J Gastroenterol Hepatol (2021) 2021. doi: 10.1155/2021/8837811
152. Ganesan R, Yoon SJ, Suk KT. Microbiome and metabolomics in liver cancer: scientific technology. Int J Mol Sci (2022) 24(1):537. doi: 10.3390/ijms24010537
153. Gao YX, Yang TW, Yin JM, Yang PX, Kou BX, Chai MY, et al. Progress and prospects of biomarkers in primary liver cancer. Int J Oncol (2020) 57(1):54–66. doi: 10.3892/ijo.2020.5035
154. Wu C-Y, Tseng C-H. Microbiota and liver cancer. Microbiome Gastrointestinal Cancer (2023) p:67–90. doi: 10.1007/978-981-19-4492-5_5
155. Gahlawat G, Choudhury AR. A review on the biosynthesis of metal and metal salt nanoparticles by microbes. RSC Adv (2019) 9(23):12944–67. doi: 10.1039/C8RA10483B
156. Zhao Q, Elson CO. Adaptive immune education by gut microbiota antigens. Immunology (2018) 154(1):28–37. doi: 10.1111/imm.12896
157. Hsu BB, Plant IN, Lyon L, Anastassacos Fm, Way JC, Silver PA. In situ reprogramming of gut bacteria by oral delivery. Nat Commun (2020) 11(1):5030. doi: 10.1038/s41467-020-18614-2
158. Kang H, You HJ, Lee G, Lee SH, Yoo T, Choi M, et al. Interaction effect between NAFLD severity and high carbohydrate diet on gut microbiome alteration and hepatic de novo lipogenesis. Gut Microbes (2022) 14(1):2078612. doi: 10.1080/19490976.2022.2078612
159. Sheng W, Ji G, Zhang L. The effect of lithocholic acid on the gut-liver axis. Front Pharmacol (2022) 13:910493. doi: 10.3389/fphar.2022.910493
160. Ohtani N, Hara E. Gut-liver axis-mediated mechanism of liver cancer: a special focus on the role of gut microbiota. Cancer Sci (2021) 112(11):4433–43. doi: 10.1111/cas.15142
161. Rao Y, Kuang Z, Li C, Guo S, Xu Y, Zhao D, et al. Gut Akkermansia muciniphila ameliorates metabolic dysfunction-associated fatty liver disease by regulating the metabolism of L-aspartate via gut-liver axis. Gut Microbes (2021) 13(1):1927633. doi: 10.1080/19490976.2021.1927633
162. Liu J, Wu A, Cai J, She Z-G, Li H. The contribution of the gut-liver axis to the immune signaling pathway of NAFLD. Front Immunol (2022) 13:968799. doi: 10.3389/fimmu.2022.968799
163. Crabb DW, Im GY, Szabo G, Mellinger JL, Lucey MR. Diagnosis and treatment of alcohol-associated liver diseases: 2019 practice guidance from the American Association for the Study of Liver Diseases. Hepatology (2020) 71(1):306–33. doi: 10.1002/hep.30866
164. Liu C, Makrinioti H, Saglani S, Bowman M, Lin LL, Camargo CA Jr, et al. Microbial dysbiosis and childhood asthma development: Integrated role of the airway and gut microbiome, environmental exposures, and host metabolic and immune response. Front Immunol (2022) 13:1028209. doi: 10.3389/fimmu.2022.1028209
165. Ohtani N, Kawada N. Role of the gut–liver axis in liver inflammation, fibrosis, and cancer: a special focus on the gut microbiota relationship. Hepatol Commun (2019) 3(4):456–70. doi: 10.1002/hep4.1331
166. Lin L, Yan L, Liu Y, Qu C, Ni J, Li H. The burden and trends of primary liver cancer caused by specific etiologies from 1990 to 2017 at the global, regional, national, age, and sex level results from the global burden of disease study 2017. Liver Cancer (2020) 9(5):563–82. doi: 10.1159/000508568
167. Albillos A, De Gottardi A, Rescigno M. The gut-liver axis in liver disease: Pathophysiological basis for therapy. J Hepatol (2020) 72(3):558–77. doi: 10.1016/j.jhep.2019.10.003
168. Zhang L, Zi L, Kuang T, Wang K, Qiu Z, Wu Z, et al. Investigating causal associations among gut microbiota, metabolites, and liver diseases: a Mendelian randomization study. Front Endocrinol (2023) 14. doi: 10.3389/fendo.2023.1159148
169. Caussy C, Tripathi A, Humphrey G, Bassirian S, Singh S, Faulkner C, et al. A gut microbiome signature for cirrhosis due to nonalcoholic fatty liver disease. Nat Commun (2019) 10(1):1406. doi: 10.1038/s41467-019-09455-9
170. Ramachandran P, Dobie R, Wilson-Kanamori JR, Dora EF, Henderson BE, Luu NT, et al. Resolving the fibrotic niche of human liver cirrhosis at single-cell level. Nature (2019) 575(7783):512–8. doi: 10.1038/s41586-019-1631-3
171. Qin N, Pons N, Batto JM, Kennedy SP, Leonard P, Yuan C, et al. Alterations of the human gut microbiome in liver cirrhosis. Nature (2014) 513(59-64):762. doi: 10.1038/nature13568
172. Ren Z, Li A, Jiang J, Zhou L, Yu Z, Lu H, et al. Gut microbiome analysis as a tool towards targeted non-invasive biomarkers for early hepatocellular carcinoma. Gut (2019) 68(6):1014–23. doi: 10.1136/gutjnl-2017-315084
173. Liu Q, Li F, Zhuang Y, Xu J, Wang J, Mao X, et al. Alteration in gut microbiota associated with hepatitis B and non-hepatitis virus related hepatocellular carcinoma. Gut Pathog (2019) 11(1):1–13. doi: 10.1186/s13099-018-0281-6
174. Wang J, Wang Y, Zhang X, Liu J, Zhang Q, Zhao Y, et al. Gut microbial dysbiosis is associated with altered hepatic functions and serum metabolites in chronic hepatitis B patients. Front Microbiol (2017) 8:2222. doi: 10.3389/fmicb.2017.02222
175. Chakaroun RM, Massier L, Kovacs P. Gut microbiome, intestinal permeability, and tissue bacteria in metabolic disease: perpetrators or bystanders? Nutrients (2020) 12(4):1082. doi: 10.3390/nu12041082
176. Singal AK, Mathurin P. Diagnosis and treatment of alcohol-associated liver disease: a review. Jama (2021) 326(2):165–76. doi: 10.1001/jama.2021.7683
177. Kushner T, Cafardi J. Chronic liver disease and COVID-19: alcohol use disorder/alcohol-associated liver disease, nonalcoholic fatty liver disease/nonalcoholic steatohepatitis, autoimmune liver disease, and compensated cirrhosis. Clin Liver Dis (2020) 15(5):195. doi: 10.1002/cld.974
179. Cederbaum AI. Alcohol metabolism. Clinics liver Dis (2012) 16(4):667–85. doi: 10.1016/j.cld.2012.08.002
180. Bishehsari F, Magno E, Swanson G, Desai V, Voigt RM, Forsyth CB, et al. Alcohol and gut-derived inflammation. Alcohol research: Curr Rev (2017) 38(2):163.
181. Dunagan M, et al. Acetaldehyde disrupts tight junctions in Caco-2 cell monolayers by a protein phosphatase 2A-dependent mechanism. The American Journal: American Journal of Physiology-Gastrointestinal and Liver Physiology (2012) 303:G1356–64.
182. Wang Y, Tong J, Chang B, Wang B, Zhang D, Wang B. Effects of alcohol on intestinal epithelial barrier permeability and expression of tight junction−associated proteins. Mol Med Rep (2014) 9(6):2352–6. doi: 10.3892/mmr.2014.2126
183. Mutlu EA, Gillevet PM, Rangwala H, Sikaroodi M, Naqvi A, Engen PA, et al. Colonic microbiome is altered in alcoholism. The American Journal: American Journal of Physiology-Gastrointestinal and Liver Physiology (2012) 302, G966–78.
184. Smirnova E, Puri P, Muthiah MD, Daitya K, Brown R, Chalasani N, et al. Fecal microbiome distinguishes alcohol consumption from alcoholic hepatitis but does not discriminate disease severity. Hepatology (2020) 72(1):271–86. doi: 10.1002/hep.31178
185. Lang S, Fairfied B, Gao B, Duan Y, Zhang X, Fouts DE, et al. Changes in the fecal bacterial microbiota associated with disease severity in alcoholic hepatitis patients. Gut Microbes (2020) 12(1):1785251. doi: 10.1080/19490976.2020.1785251
186. Wilson BC, Vatanen T, Jayasinghe TN, Leong KS, Derraik JG, Albert BB, et al. Strain engraftment competition and functional augmentation in a multi-donor fecal microbiota transplantation trial for obesity. Microbiome (2021) 9(1):107. doi: 10.1186/s40168-021-01060-7
187. Zhang T, Lu G, Zhao Z, Liu Y, Shen Q, Li P, et al. Washed microbiota transplantation vs. manual fecal microbiota transplantation: clinical findings, animal studies and in vitro screening. Protein Cell (2020) 11(4):251–66. doi: 10.1007/s13238-019-00684-8
188. Puri P, Liangpunsakul S, Christensen JE, Shah VH, Kamath PS, Gores GJ, et al. The circulating microbiome signature and inferred functional metagenomics in alcoholic hepatitis. Hepatology (2018) 67(4):1284–302. doi: 10.1002/hep.29623
189. Farzanegi P, Dana A, Ebrahimpoor Z, Asadi M, Azarbayjani MA. Mechanisms of beneficial effects of exercise training on non-alcoholic fatty liver disease (NAFLD): Roles of oxidative stress and inflammation. Eur J sport Sci (2019) 19(7):994–1003. doi: 10.1080/17461391.2019.1571114
190. Perumpail BJ, Khan MA, Yoo ER, Cholankeril G, Kim D, Ahmed A. Clinical epidemiology and disease burden of nonalcoholic fatty liver disease. World J Gastroenterol (2017) 23(47):8263. doi: 10.3748/wjg.v23.i47.8263
191. Younossi ZM, Koenig AB, Abdelatif D, Fazel Y, Henry L, Wymer M. Global epidemiology of nonalcoholic fatty liver disease—meta-analytic assessment of prevalence, incidence, and outcomes. Hepatology (2016) 64(1):73–84. doi: 10.1002/hep.28431
192. Vallianou N, Christodoulatos GS, Karampela I, Tsilingiris D, Magkos F, Stratigou T, et al. Understanding the role of the gut microbiome and microbial metabolites in non-alcoholic fatty liver disease: current evidence and perspectives. Biomolecules (2021) 12(1):56. doi: 10.3390/biom12010056
193. Sydor S, Best J, Messerschmidt I, Manka P, Vilchez-Vargas R, Brodesser S, et al. Altered microbiota diversity and bile acid signaling in cirrhotic and noncirrhotic NASH-HCC. Clin Trans Gastroenterol (2020) 161(10):bqaa134. doi: 10.14309/ctg.0000000000000131
194. DiStefano JK. NAFLD and NASH in postmenopausal women: implications for diagnosis and treatment. Endocrinology (2020) 161(10):bqaa134. doi: 10.1210/endocr/bqaa134
195. Younossi Z, Anstee QM, Marietti M, Hardy T, Henry L, Eslam M, et al. Global burden of NAFLD and NASH: trends, predictions, risk factors and prevention. Nat Rev Gastroenterol Hepatol (2018) 15(1):11–20. doi: 10.1038/nrgastro.2017.109
196. Dudek M, Pfister D, Donakonda S, Filpe P, Schneider A, Laschinger M, et al. Auto-aggressive CXCR6+ CD8 T cells cause liver immune pathology in NASH. Nature (2021) 592(7854):444–9. doi: 10.1038/s41586-021-03233-8
197. Romero FA, Jones CT, Xu Y, Fenaux M, Halcomb RL. The race to bash NASH: emerging targets and drug development in a complex liver disease. J medicinal Chem (2020) 63(10):5031–73. doi: 10.1021/acs.jmedchem.9b01701
198. Jackson DN, Theiss AL. Gut bacteria signaling to mitochondria in intestinal inflammation and cancer. Gut Microbes (2020) 11(3):285–304. doi: 10.1080/19490976.2019.1592421
199. Rengarajan S, Vivio EE, Parkes M, Peterson DA, Roberson ED, Newberry RD, et al. Dynamic immunoglobulin responses to gut bacteria during inflammatory bowel disease. Gut Microbes (2020) 11(3):405–20. doi: 10.1080/19490976.2019.1626683
200. Vallianou N, Stratigou T, Christodulatos GS, Dalamaga M. Understanding the role of the gut microbiome and microbial metabolites in obesity and obesity-associated metabolic disorders: current evidence and perspectives. Curr Obes Rep (2019) 8:317–32. doi: 10.1007/s13679-019-00352-2
201. Hoozemans J, Brauw Md, Nieuwdorp M, Gerder V. Gut microbiome and metabolites in patients with NAFLD and after bariatric surgery: a comprehensive review. Metabolites (2021) 11(6):353. doi: 10.3390/metabo11060353
202. Yuan J, Chen C, Cui J, Lu J, Yan C, Wei X, et al. Fatty liver disease caused by high-alcohol-producing Klebsiella pneumoniae. Cell Metab (2019) 30(4):675–688.e7. doi: 10.1016/j.cmet.2019.08.018
203. Aron-Wisnewsky J, Vigliotti C, Witjes J, Le P, Holleboom AG, Verheij J, et al. Gut microbiota and human NAFLD: disentangling microbial signatures from metabolic disorders. Nat Rev Gastroenterol Hepatol (2020) 17(5):279–97. doi: 10.1038/s41575-020-0269-9
204. Carotti S, Aquilano K, Zalfa F, Ruggiero S, Valentini F, Zingariello M, et al. Lipophagy impairment is associated with disease progression in NAFLD. Front Physiol (2020) 11:850. doi: 10.3389/fphys.2020.00850
205. Sharma T, Gupta A, Chauhan R, Bhat AA, Nisar S, Hashem S, et al. Cross-talk between the microbiome and chronic inflammation in esophageal cancer: Potential driver of oncogenesis. Cancer Metastasis Rev (2022) 41(2):281–99. doi: 10.1007/s10555-022-10026-6
206. Kamangar F, Nasrollahzadeh D, Safiri S, Sepanlou SG, Fitzmaurice C, Ikuta KS, et al. The global, regional, and national burden of oesophageal cancer and its attributable risk factors in 195 countries and territories, 1990–2017: a systematic analysis for the Global Burden of Disease Study 2017. Lancet Gastroenterol Hepatol (2020) 5(6):582–97. doi: 10.1016/S2468-1253(20)30007-8
207. Asombang AW, Chishinga N, Nkhoma A, Chipaila J, Nsokolo B, Manda-Mapalo M, et al. Systematic review and meta-analysis of esophageal cancer in Africa: epidemiology, risk factors, management and outcomes. World J Gastroenterol (2019) 25(31):4512. doi: 10.3748/wjg.v25.i31.4512
208. Lagergren J, Smyth E, Cunningham D, Lagergren P. Oesophageal cancer. Lancet (2017) 390(10110):2383–96. doi: 10.1016/S0140-6736(17)31462-9
209. Uhlenhopp DJ, Then EO, Sunkara T, Gaduputi V. Epidemiology of esophageal cancer: update in global trends, etiology and risk factors. Clin J Gastroenterol (2020) 13(6):1010–21. doi: 10.1007/s12328-020-01237-x
210. Liu CQ, Ma YL, Qin Q, Wang PH, Luo Y, Xu PF, et al. Epidemiology of esophageal cancer in 2020 and projections to 2030 and 2040. Thorac Cancer (2023) 14(1):3–11. doi: 10.1111/1759-7714.14745
211. Laserna-Mendieta E, FitzGerald JA, Ollala JM, Bernardo D, Claesson MJ, Lucendo AJ. Esophageal microbiome in active eosinophilic esophagitis and changes induced by different therapies. Sci Rep (2021) 11(1):7113. doi: 10.1038/s41598-021-86464-z
212. Tarazi M, Chidambaram S, Markar SR. Risk factors of esophageal squamous cell carcinoma beyond alcohol and smoking. Cancers (2021) 13(5):1009. doi: 10.3390/cancers13051009
213. Hasan A, Hasan LK, Schnabl B, Greytak M, Yadlapati R. Microbiome of the aerodigestive tract in health and esophageal disease. Digestive Dis Sci (2021) 66:12–8. doi: 10.1007/s10620-020-06720-6
214. Ma SD, Guccione C, Linnemeyer K, Weissbrod PA, Curtius K, Yadlapati RH. Oral microbiome diversity between patients with normal versus elevated salivary pepsin levels and its implication in gastroesophageal reflux disease. Foregut (2023) 3(1):131–4. doi: 10.1177/26345161221127439
215. Bilello J, Okereke I. Impact of environmental and pharmacologic changes on the upper gastrointestinal microbiome. Biomedicines (2021) 9(6):617. doi: 10.3390/biomedicines9060617
216. Yeh CC, Chen CC, Chen CC, Han ML, Wu JF, Wang HP, et al. Characteristics of the esophageal microbiome in patients with achalasia and its changes before and after peroral endoscopic myotomy: A pilot study. J Gastroenterol Hepatol (2023) 38(8):1307–15. doi: 10.1111/jgh.16192
217. Corning B, Copland AP, Frye JW. The esophageal microbiome in health and disease. Curr Gastroenterol Rep (2018) 20:1–7. doi: 10.1007/s11894-018-0642-9
218. Bernardot L, Roman S, Barret M, Vitton V, Wallenhorst T, Pioche M, et al. Efficacy of per-oral endoscopic myotomy for the treatment of non-achalasia esophageal motor disorders. Surg Endoscopy (2020) 34:5508–15. doi: 10.1007/s00464-019-07348-y
219. Lv J, Guo L, Liu J-J, Zhao H-P, Zhang J, Wang J-H. Alteration of the esophageal microbiota in Barrett's esophagus and esophageal adenocarcinoma. World J Gastroenterol (2019) 25(18):2149. doi: 10.3748/wjg.v25.i18.2149
220. Zhou J, Sun S, Luan S, Xiao X, Yang Y, Mao C, et al. Gut microbiota for esophageal cancer: role in carcinogenesis and clinical implications. Front Oncol (2021) 11:717242. doi: 10.3389/fonc.2021.717242
221. Blackett K, Siddhi SS, Cleary S, Steed H, Miller MH, Macfarlane S, et al. Oesophageal bacterial biofilm changes in gastro-oesophageal reflux disease, Barrett's and oesophageal carcinoma: association or causality? Alimentary Pharmacol Ther (2013) 37(11):1084–92. doi: 10.1111/apt.12317
222. Kaakoush NO, Castaño-Rodríguez N, Man SM, Mitchell HM. Is Campylobacter to esophageal adenocarcinoma as Helicobacter is to gastric adenocarcinoma? Trends Microbiol (2015) 23(8):455–62. doi: 10.1016/j.tim.2015.03.009
223. Elliott DRF, Walker AW, O'Donovan M, Parkhill J, Fitzgerald RC. A non-endoscopic device to sample the oesophageal microbiota: a case-control study. Lancet Gastroenterol Hepatol (2017) 2(1):32–42. doi: 10.1016/S2468-1253(16)30086-3
224. Zaidi AH, Kelly LA, Kreft RE, Barlek M, Omstead AN, Matsui D, et al. Associations of microbiota and toll-like receptor signaling pathway in esophageal adenocarcinoma. BMC Cancer (2016) 16(1):1–10. doi: 10.1186/s12885-016-2093-8
225. Wang Z, Shaheen NJ, Whiteman DC, Anderson LA, Vaughan TL, Corley DA, et al. Helicobacter pylori infection is associated with reduced risk of Barrett's esophagus: an analysis of the Barrett's and esophageal adenocarcinoma consortium. Off J Am Coll Gastroenterology| ACG (2018) 113(8):1148–55. doi: 10.1038/s41395-018-0070-3
226. Howden CW. H. pylori and barrett's Esophagus: Implications for Populations and Practice. American College of Gastroenterology (2018) p. 1119–20.
227. Chen X, Winckler B, Lu M, Cheng H, Yuan Z, Yang Y, et al. Oral microbiota and risk for esophageal squamous cell carcinoma in a high-risk area of China. PloS One (2015) 10(12):e0143603. doi: 10.1371/journal.pone.0143603
228. Gao S, Li S, Ma Z, Liang S, Shan T, Zhang M, et al. Presence of Porphyromonas gingivalis in esophagus and its association with the clinicopathological characteristics and survival in patients with esophageal cancer. Infect Agents Cancer (2016) 11:1–9. doi: 10.1186/s13027-016-0049-x
229. Peters BA, Wu J, Pei Z, Yang L, Purdue MP, Freedman ND, et al. Oral microbiome composition reflects prospective risk for esophageal cancers. Cancer Res (2017) 77(23):6777–87. doi: 10.1158/0008-5472.CAN-17-1296
230. Deshpande NP, Riordan SM, Castaño-Rodríguez N, Wilkins MR, Kaakoush NO. Signatures within the esophageal microbiome are associated with host genetics, age, and disease. Microbiome (2018) 6:1–14. doi: 10.1186/s40168-018-0611-4
231. Meng F, Li R, Ma L, Liu L, Lai X, Yang D, et al. Porphyromonas gingivalis promotes the motility of esophageal squamous cell carcinoma by activating NF-κB signaling pathway. Microbes infection (2019) 21(7):296–304. doi: 10.1016/j.micinf.2019.01.005
232. Sun T, Wu Z, Wang X, Wang Y, Hu X, Qin W, et al. LNC942 promoting METTL14-mediated m6A methylation in breast cancer cell proliferation and progression. Oncogene (2020) 39(31):5358–72. doi: 10.1038/s41388-020-1338-9
233. Coker OO. Non-bacteria microbiome (virus, fungi, and archaea) in gastrointestinal cancer. Microbiome Gastrointestinal Cancer (2023), 91–106. doi: 10.1007/978-981-19-4492-5_6
234. Deng Y, Tang D, Hou P, Shen W, Li H, Wang T, et al. Dysbiosis of gut microbiota in patients with esophageal cancer. Microbial Pathogenesis (2021) 150:104709. doi: 10.1016/j.micpath.2020.104709
235. Huang J, Koulaouzidis A, Marlicz W, Lok V, Chu C, Ngai CH, et al. Global burden, risk factors, and trends of esophageal cancer: an analysis of cancer registries from 48 countries. Cancers (2021) 13(1):141. doi: 10.3390/cancers13010141
236. Guan X, Li W, Meng H. A double-edged sword: Role of butyrate in the oral cavity and the gut. Mol Oral Microbiol (2021) 36(2):121–31. doi: 10.1111/omi.12322
237. Nejman D, Livyatan I, Fuks G, Gavert N, Zwang Y, Geller LT, et al. The human tumor microbiome is composed of tumor type–specific intracellular bacteria. Science (2020) 368(6494):973–80. doi: 10.1126/science.aay9189
238. Weng M-T, Chiu Y-T, Wei P-Y, Chiang C-W, Fang H-L, Wei S-C, et al. Microbiota and gastrointestinal cancer. J Formosan Med Assoc (2019) 118:S32–41. doi: 10.1016/j.jfma.2019.01.002
239. Coker OO, Dai Z, Nie Y, Zhao G, Cao L, Nakatsu G, et al. Mucosal microbiome dysbiosis in gastric carcinogenesis. Gut (2018) 67(6):1024–32. doi: 10.1136/gutjnl-2017-314281
240. Beller L, Matthijnssens J. What is (not) known about the dynamics of the human gut virome in health and disease. Curr Opin Virol (2019) 37:52–7. doi: 10.1016/j.coviro.2019.05.013
241. Shkoporov AN, Clooney AG, Sutton TD, Ryan FJ, Daly KM, Nolan JA, et al. The human gut virome is highly diverse, stable, and individual specific. Cell Host Microbe (2019) 26(4):527–541.e5. doi: 10.1016/j.chom.2019.09.009
242. Li Q, Oduro PK, Guo R, Li R, Leng L, Kong X, et al. Oncolytic viruses: Immunotherapy drugs for gastrointestinal Malignant tumors. Front Cell Infection Microbiol (2022) 12:921534. doi: 10.3389/fcimb.2022.921534
243. Santiago-Rodriguez TM, Hollister EB. Human virome and disease: high-throughput sequencing for virus discovery, identification of phage-bacteria dysbiosis and development of therapeutic approaches with emphasis on the human gut. Viruses (2019) 11(7):656. doi: 10.3390/v11070656
244. Chen Q, Dharmaraj T, Cai PC, Burgener EB, Haddock NL, Spakowitz AJ, et al. Bacteriophage and bacterial susceptibility, resistance, and tolerance to antibiotics. Pharmaceutics (2022) 14(7):1425. doi: 10.3390/pharmaceutics14071425
245. Garten RJ, Davis CT, Russell CA, Shu B, Lindstrom S, Balish A, et al. Antigenic and genetic characteristics of swine-origin 2009 A (H1N1) influenza viruses circulating in humans. science (2009) 325(5937):197–201. doi: 10.1126/science.1176225
246. Klein M, Gögenur I, Ingeholm P, Njor SH, Iversen LH, Emmertsen KJ, et al. Validation of the Danish colorectal cancer group (DCCG. Dk) database–on behalf of the Danish colorectal cancer group. Colorectal Dis (2020) 22(12):2057–67. doi: 10.1111/codi.15352
247. Di Saverio S, Pata F, Gallo G, Carrano F, Scorza A, Sileri P, et al. Coronavirus pandemic and colorectal surgery: practical advice based on the Italian experience. Colorectal Dis (2020) 22(6):625–34. doi: 10.1111/codi.15056
248. Gaertner WB, Burgess PL, Davids JS, Lightner AL, Shogan BD, Sun MY, et al. The American Society of Colon and Rectal Surgeons clinical practice guidelines for the management of anorectal abscess, fistula-in-ano, and rectovaginal fistula. Dis Colon Rectum (2022) 65(8):964–85. doi: 10.1097/DCR.0000000000002473
249. Kucejko RJ, Holleran TJ, Stein DE, Poggio JL. How soon should patients with colon cancer undergo definitive resection? Dis Colon Rectum (2020) 63(2):172–82. doi: 10.1097/DCR.0000000000001525
250. Massimino L, Lovisa S, Lamparelli LA, Danese S, Ungaro F. Gut eukaryotic virome in colorectal carcinogenesis: Is that a trigger? Comput Struct Biotechnol J (2021) 19:16–28. doi: 10.1016/j.csbj.2020.11.055
251. Emlet C, Ruffin M, Lamendella R. Enteric virome and carcinogenesis in the gut. Digestive Dis Sci (2020) 65(3):852–64. doi: 10.1007/s10620-020-06126-4
252. Dalal N, Jalandra R, Bayal N, Yadav AK, Harshulika Sharma M, et al. Gut microbiota-derived metabolites in CRC progression and causation. J Cancer Res Clin Oncol (2021) 147:3141–55. doi: 10.1007/s00432-021-03729-w
253. Hossain MS, Karuniawati H, Jairoun AA, Urbi Z, Ooi DJ, John A, et al. Colorectal cancer: a review of carcinogenesis, global epidemiology, current challenges, risk factors, preventive and treatment strategies. Cancers (2022) 14(7):1732. doi: 10.3390/cancers14071732
254. Bjornevik K, Cortese M, Healy BC, Kuhle J, Mina MJ, Leng Y, et al. Longitudinal analysis reveals high prevalence of Epstein-Barr virus associated with multiple sclerosis. Science (2022) 375(6578):296–301. doi: 10.1126/science.abj8222
255. Sun K, Jia K, Lv H, Wang SQ, Wu Y, Lei H, et al. EBV-positive gastric cancer: current knowledge and future perspectives. Front Oncol (2020) 10:583463. doi: 10.3389/fonc.2020.583463
256. Ok CY, Papathomas TG, Medeiros LJ, Young KH. EBV-positive diffuse large B-cell lymphoma of the elderly. Blood J Am Soc Hematol (2013) 122(3):328–40. doi: 10.1182/blood-2013-03-489708
257. Favacho AR, Cintra EA, Coelho LCBB, Linhares MIS. In vitro activity evaluation of Parkia pendula seed lectin against human cytomegalovirus and herpes virus 6. Biologicals (2007) 35(3):189–94. doi: 10.1016/j.biologicals.2006.09.005
258. Lv Y-l, Han FF, An ZL, Jia Y, Xuan LL, Gong LL, et al. Cytomegalovirus infection is a risk factor in gastrointestinal cancer: A cross-sectional and meta-analysis study. Intervirology (2020) 63(1-6):10–6. doi: 10.1159/000506683
259. Zhang L, Guo G, Xu J, Sun X, Chen W, Jin J, et al. Human cytomegalovirus detection in gastric cancer and its possible association with lymphatic metastasis. Diagn Microbiol Infect Dis (2017) 88(1):62–8. doi: 10.1016/j.diagmicrobio.2017.02.001
260. Bernard R, et al. Association between oral microbiome and esophageal diseases: a state-of-the-art review. Digestive Dis (2022) 40(3):345–54. doi: 10.1159/000517736
261. Xu W, et al. Viruses, other pathogenic microorganisms and esophageal cancer. Gastrointestinal tumors (2015) 2(1):2–13. doi: 10.1159/000380897
262. Bedford T, Riley S, Barr IG, Broor S, Chadha M, Cox NJ, et al. Global circulation patterns of seasonal influenza viruses vary with antigenic drift. Nature (2015) 523(7559):217–20. doi: 10.1038/nature14460
263. Barton ES, White DW, Cathelyn JS, Brett-McClellan KA, Engle M, Diamond MS, et al. Herpesvirus latency confers symbiotic protection from bacterial infection. Nature (2007) 447(7142):326–9. doi: 10.1038/nature05762
264. Hallen-Adams HE, Suhr MJ. Fungi in the healthy human gastrointestinal tract. Virulence (2017) 8(3):352–8. doi: 10.1080/21505594.2016.1247140
265. Rajapaksha P, Elbourne A, Gangadoo S, Brown R, Cozzolino D, Chapman J. A review of methods for the detection of pathogenic microorganisms. Analyst (2019) 144(2):396–411. doi: 10.1039/C8AN01488D
266. Franco-Duarte R, Černáková L, Kadam S, Kaushik K, Salehi B, Bevilacqua A, et al. Advances in chemical and biological methods to identify microorganisms—from past to present. Microorganisms (2019) 7(5):130. doi: 10.3390/microorganisms7050130
267. Perez JC. Fungi of the human gut microbiota: Roles and significance. Int J Med Microbiol (2021) 311(3):151490. doi: 10.1016/j.ijmm.2021.151490
268. Fiers WD, Gao IH, Iliev ID. Gut mycobiota under scrutiny: fungal symbionts or environmental transients? Curr Opin Microbiol (2019) 50:79–86. doi: 10.1016/j.mib.2019.09.010
269. Lücking R, Aime MC, Robbertse B, Miller AN, Ariyawansa HA, Aoki T, et al. Unambiguous identification of fungi: where do we stand and how accurate and precise is fungal DNA barcoding? IMA fungus (2020) 11(1):1–32. doi: 10.1186/s43008-020-00033-z
270. Garcia-Rubio R, de Oliveira HC, Rivera J, Trevijano-Contador N. The fungal cell wall: Candida, Cryptococcus, and Aspergillus species. Front Microbiol (2020) 10:2993. doi: 10.3389/fmicb.2019.02993
271. Berkow EL, Lockhart SR. Fluconazole resistance in Candida species: a current perspective. Infection Drug resistance (2017) p:237–45. doi: 10.2147/IDR.S118892
272. Arendrup MC, Patterson TF. Multidrug-resistant Candida: epidemiology, molecular mechanisms, and treatment. J Infect Dis (2017) 216(suppl_3):S445–S45. doi: 10.1093/infdis/jix131
273. Zhu L, Song J, Zhou J-L, Si J, Cui B-K. Species diversity, phylogeny, divergence time, and biogeography of the genus Sanghuangporus (Basidiomycota). Front Microbiol (2019) 10:812. doi: 10.3389/fmicb.2019.00812
274. Gressler M, Löhr NA, Schäfer T, Lawrinowitz S, Seibold PS, Hoffmeister D. Mind the mushroom: natural product biosynthetic genes and enzymes of Basidiomycota. Natural product Rep (2021) 38(4):702–22. doi: 10.1039/D0NP00077A
275. Coker OO, Nakatsu G, Dai RZ, Wu WK, Wong SH, Ng SC, et al. Enteric fungal microbiota dysbiosis and ecological alterations in colorectal cancer. Gut (2019) 68(4):654–62. doi: 10.1136/gutjnl-2018-317178
276. Zhu F, Willette-Brown J, Song NY, Lomada D, Song Y, Xue L, et al. Autoreactive T cells and chronic fungal infection drive esophageal carcinogenesis. Cell Host Microbe (2017) 21(4):478–493.e7. doi: 10.1016/j.chom.2017.03.006
277. Rautemaa R, Hietanen J, Niissalo S, Pirinen S, Perheentupa J. Oral and oesophageal squamous cell carcinoma–a complication or component of autoimmune polyendocrinopathy-candidiasis-ectodermal dystrophy (APECED, APS-I). Oral Oncol (2007) 43(6):607–13. doi: 10.1016/j.oraloncology.2006.07.005
278. Chu Z-Q, Zhang K-C, Chen L. Neutrophil extracellular traps in gastrointestinal cancer. World J Gastroenterol (2021) 27(33):5474. doi: 10.3748/wjg.v27.i33.5474
279. Zhong M, Xiong Y, Zhao J, Gao Z, Ma J, Wu Z, et al. Candida albicans disorder is associated with gastric carcinogenesis. Theranostics (2021) 11(10):4945. doi: 10.7150/thno.55209
280. Czerw A, Religioni U, Banaś T. Perception of cancer in patients diagnosed with the most common gastrointestinal cancers. BMC Palliative Care (2020) 19(1):1–11. doi: 10.1186/s12904-020-00650-w
281. Harvey JB, Phan LH, Villarreal OE, Bowser JL. CD73's potential as an immunotherapy target in gastrointestinal cancers. Front Immunol (2020) 11:508. doi: 10.3389/fimmu.2020.00508
282. Karimi B, Terrat S, Dequiedt S, Saby NP, Horrigue W, Lelièvre M, et al. Biogeography of soil bacteria and archaea across France. Sci Adv (2018) 4(7):eaat1808. doi: 10.1126/sciadv.aat1808
283. Takahashi S, Tomita J, Nishioka K, Hisada T, Nishijima M. Development of a prokaryotic universal primer for simultaneous analysis of Bacteria and Archaea using next-generation sequencing. PloS One (2014) 9(8):e105592. doi: 10.1371/journal.pone.0105592
284. Spang A, Eme L, Saw JH, Caceres EF, Zaremba-Niedzwiedzka K, Lombard J, et al. Asgard archaea are the closest prokaryotic relatives of eukaryotes. PloS Genet (2018) 14(3):e1007080. doi: 10.1371/journal.pgen.1007080
285. Eme L, Spang A, Lombard J, Stairs CW, Ettema TJG. Archaea and the origin of eukaryotes. Nat Rev Microbiol (2017) 15(12):711–23. doi: 10.1038/nrmicro.2017.133
286. Lurie-Weinberger MN, Gophna U. Archaea in and on the human body: health implications and future directions. PloS Pathog (2015) 11(6):e1004833. doi: 10.1371/journal.ppat.1004833
287. Kim JY, Whon TW, Lim MY, Kim YB, Kim N, Kwon MS, et al. The human gut archaeome: identification of diverse haloarchaea in Korean subjects. Microbiome (2020) 8(1):1–17. doi: 10.1186/s40168-020-00894-x
288. Shi X, Gao G, Tian J, Wang XC, Jin X, Jin P. Symbiosis of sulfate-reducing bacteria and methanogenic archaea in sewer systems. Environ Int (2020) 143:105923. doi: 10.1016/j.envint.2020.105923
289. Roccarina D, Lauritano EC, Gabrielli M, Franceschi F, Ojetti V, Gasbarrini A. The role of methane in intestinal diseases. Off J Am Coll Gastroenterology| ACG (2010) 105(6):1250–6. doi: 10.1038/ajg.2009.744
290. Chen S-C, Musat N, Lechtenfeld OJ, Paschke H, Schmidt M, Said N, et al. Anaerobic oxidation of ethane by archaea from a marine hydrocarbon seep. Nature (2019) 568(7750):108–11. doi: 10.1038/s41586-019-1063-0
291. Moissl-Eichinger C, Pausan M, Taffner J, Berg G, Bang C, Schmitz R. Archaea are interactive components of complex microbiomes. Trends Microbiol (2018) 26(1):70–85. doi: 10.1016/j.tim.2017.07.004
292. Santoro AE, Richter RA, Dupont CL. Planktonic marine archaea. Annu Rev Mar Sci (2019) 11:131–58. doi: 10.1146/annurev-marine-121916-063141
293. Liu Y, Makarova KS, Huang WC, Wolf YI, Nikolskaya AN, Zhang X, et al. Expanded diversity of Asgard archaea and their relationships with eukaryotes. Nature (2021) 593(7860):553–7. doi: 10.1038/s41586-021-03494-3
294. Krupovic M, Cvirkaite-Krupovic V, Iranzo J, Prangishvili D, Koonin EV. Viruses of archaea: structural, functional, environmental and evolutionary genomics. Virus Res (2018) 244:181–93. doi: 10.1016/j.virusres.2017.11.025
295. Dombrowski N, Lee J-H, Williams TA, Offre P, Spang A. Genomic diversity, lifestyles and evolutionary origins of DPANN archaea. FEMS Microbiol Lett (2019) 366(2):fnz008. doi: 10.1093/femsle/fnz008
296. Tran HN, Thu TN, Nguyen PH, Vo CN, Doan KV, Nguyen Ngoc Minh C, et al. Tumour microbiomes and Fusobacterium genomics in Vietnamese colorectal cancer patients. NPJ Biofilms Microbiomes (2022) 8(1):87. doi: 10.1038/s41522-022-00351-7
297. Tajima Y, Okuda S, Hanai T, Hiro J, Masumori K, Koide Y, et al. Differential analysis of microbiomes in mucus and tissues obtained from colorectal cancer patients. Sci Rep (2022) 12(1):18193. doi: 10.1038/s41598-022-21928-4
298. Wang H, Zhang K, Wu L, Qin Q, He Y. Prediction of pathogenic factors in dysbiotic gut microbiomes of colorectal cancer patients using reverse microbiomics. Front Oncol (2022) 12:882874. doi: 10.3389/fonc.2022.882874
299. Kim SH, Lim YJ. The role of microbiome in colorectal carcinogenesis and its clinical potential as a target for cancer treatment. Intestinal Res (2022) 20(1):31–42. doi: 10.5217/ir.2021.00034
300. Ren L, Ye J, Zhao B, Sun J, Cao P, Yang Y. The role of intestinal microbiota in colorectal cancer. Front Pharmacol (2021) 12:674807. doi: 10.3389/fphar.2021.674807
301. Fratila TD, Ismaiel A, Dumitrascu DL. Microbiome modulation in the prevention and management of colorectal cancer: a systematic review of clinical interventions. Med Pharm Rep (2023) 96(2):131. doi: 10.15386/mpr-2526
302. Negrut RL, Cote A, Maghiar AM. Exploring the potential of oral microbiome biomarkers for colorectal cancer diagnosis and prognosis: A systematic review. Microorganisms (2023) 11(6):1586. doi: 10.3390/microorganisms11061586
303. Eastmond AK, Shetty C, Rizvi SM, Sharaf J, Williams KA, Tariq M, et al. A systematic review of the gastrointestinal microbiome: A game changer in colorectal cancer. Cureus (2022) 14(8). doi: 10.7759/cureus.28545
304. Lauka L, Reitano E, Carra MC, Gaiani F, Gavriilidis P, Brunetti F, et al. Role of the intestinal microbiome in colorectal cancer surgery outcomes. World J Surg Oncol (2019) 17(1):1–12. doi: 10.1186/s12957-019-1754-x
305. Xiang Y, Zhang C, Wang J, Cheng Y, Wang L, Tong Y, et al. Identification of host gene-microbiome associations in colorectal cancer patients using mendelian randomization. J Trans Med (2023) 21(1):1–15. doi: 10.1186/s12967-023-04335-9
306. Zwezerijnen-Jiwa FH, Apewokin S, Alenghat T, Ollberding NJ. A systematic review of microbiome-derived biomarkers for early colorectal cancer detection. Neoplasia (2023) 36:100868. doi: 10.1016/j.neo.2022.100868
307. Kharofa J, Apewokin S, Alenghat T, Ollberding NJ. Metagenomic analysis of the fecal microbiome in colorectal cancer patients compared to healthy controls as a function of age. Cancer Med (2023) 12(3):2945–57. doi: 10.1002/cam4.5197
308. Yang J, Zhou X, Liu X, Ling Z, Ji F. Role of the gastric microbiome in gastric cancer: from carcinogenesis to treatment. Front Microbiol (2021) 12:641322. doi: 10.3389/fmicb.2021.641322
309. Aviles-Jimenez F, Vazquez-Jimenez F, Medrano-Guzman R, Mantilla A, Torres J. Stomach microbiota composition varies between patients with non-atrophic gastritis and patients with intestinal type of gastric cancer. Sci Rep (2014) 4(1):4202. doi: 10.1038/srep04202
310. Stewart OA, Wu F, Chen Y. The role of gastric microbiota in gastric cancer. Gut Microbes (2020) 11(5):1220–30. doi: 10.1080/19490976.2020.1762520
311. Engstrand L, Graham DY. Microbiome and gastric cancer. Digestive Dis Sci (2020) 65:865–73. doi: 10.1007/s10620-020-06101-z
312. Schulz C, Schütte K, Mayerle J, Malfertheiner P. The role of the gastric bacterial microbiome in gastric cancer: Helicobacter pylori and beyond. Ther Adv Gastroenterol (2019) 12:1756284819894062. doi: 10.1177/1756284819894062
313. Gantuya B, El Serag HB, Matsumoto T, Ajami NJ, Uchida T, Oyuntsetseg K, et al. Gastric mucosal microbiota in a Mongolian population with gastric cancer and precursor conditions. Alimentary Pharmacol Ther (2020) 51(8):770–80. doi: 10.1111/apt.15675
314. Wang L, Xin Y, Zhou J, Tian Z, Liu C, Yu X, et al. Gastric mucosa-associated microbial signatures of early gastric cancer. Front Microbiol (2020) 11:1548. doi: 10.3389/fmicb.2020.01548
315. Yan L, Chen Y, Chen F, Tao T, Hu Z, Wang J, et al. Effect of Helicobacter pylori eradication on gastric cancer prevention: updated report from a randomized controlled trial with 26.5 years of follow-up. Gastroenterology (2022) 163(1):154–162.e3. doi: 10.1053/j.gastro.2022.03.039
316. Moreno-Gonzalez M, Beraza N. The role of the microbiome in liver cancer. Cancers (2021) 13(10):2330. doi: 10.3390/cancers13102330
317. Zhou A, Tang L, Zeng S, Lei Y, Yang S, Tang B. Gut microbiota: a new piece in understanding hepatocarcinogenesis. Cancer Lett (2020) 474:15–22. doi: 10.1016/j.canlet.2020.01.002
318. Hartmann P, Chu H, Duan Y, Schnabl B. Gut microbiota in liver disease: too much is harmful, nothing at all is not helpful either. Am J Physiology-Gastrointestinal Liver Physiol (2019) 316(5):G563–73. doi: 10.1152/ajpgi.00370.2018
319. Abe K, Takahashi A, Ohira H. Microbiota and liver. Liver Systemic Dis (2016) p:25–34. doi: 10.1007/978-4-431-55790-6_2
320. Moreira C, Figueiredo C, Ferreira RM. The role of the microbiota in esophageal cancer. Cancers (2023) 15(9):2576. doi: 10.3390/cancers15092576
321. Shen W, Tang D, Deng Y, Li H, Wang T, Wan P, et al. Association of gut microbiomes with lung and esophageal cancer: a pilot study. World J Microbiol Biotechnol (2021) 37:1–16. doi: 10.1007/s11274-021-03086-3
322. Park CH, Lee SK. Exploring esophageal microbiomes in esophageal diseases: a systematic review. J Neurogastroenterol Motil (2020) 26(2):171. doi: 10.5056/jnm19240
323. Lv W, Zuo J, Wang Y, Fan Z, Feng L, Wang L, et al. The microbial characteristics of esophageal squamous cell carcinoma (ESCC) and healthy subjects. Alexandria, Virginia: American Society of Clinical Oncology, (2020).
324. Wei L, Wen X-S, Xian CJ. Chemotherapy-induced intestinal microbiota dysbiosis impairs mucosal homeostasis by modulating toll-like receptor signaling pathways. Int J Mol Sci (2021) 22(17):9474. doi: 10.3390/ijms22179474
325. Rizvi NA, Cho BC, Reinmuth N, Lee KH, Luft A, Ahn MJ, et al. Durvalumab with or without tremelimumab vs standard chemotherapy in first-line treatment of metastatic non–small cell lung cancer: the MYSTIC phase 3 randomized clinical trial. JAMA Oncol (2020) 6(5):661–74. doi: 10.1001/jamaoncol.2020.0237
326. Mao J, Wang D, Long J, Yang X, Lin J, Song Y, et al. Gut microbiome is associated with the clinical response to anti-PD-1 based immunotherapy in hepatobiliary cancers. J Immunotherapy Cancer (2021) 9(12). doi: 10.1136/jitc-2021-003334
327. Alexander JL, Wilson ID, Teare J, Marchesi JR, Nicholson JK, Kinross JM. Gut microbiota modulation of chemotherapy efficacy and toxicity. Nat Rev Gastroenterol Hepatol (2017) 14(6):356–65. doi: 10.1038/nrgastro.2017.20
328. Yamamura K, Izumi D, Kandimalla R, Sonohara F, Baba Y, Yoshida N, et al. Intratumoral Fusobacterium nucleatum levels predict therapeutic response to neoadjuvant chemotherapy in esophageal squamous cell carcinoma. Clin Cancer Res (2019) 25(20):6170–9. doi: 10.1158/1078-0432.CCR-19-0318
329. Yu T, Guo F, Yu Y, Sun T, Ma D, Han J, et al. Fusobacterium nucleatum promotes chemoresistance to colorectal cancer by modulating autophagy. Cell (2017) 170(3):548–563.e16. doi: 10.1016/j.cell.2017.07.008
330. Rohwer N, Kühl AA, Ostermann AI, Hartung NM, Schebb NH, Zopf D, et al. Effects of chronic low-dose aspirin treatment on tumor prevention in three mouse models of intestinal tumorigenesis. Cancer Med (2020) 9(7):2535–50. doi: 10.1002/cam4.2881
331. Xiong G, Liu C, Yang G, Feng M, Xu J, Zhao F, et al. Long noncoding RNA GSTM3TV2 upregulates LAT2 and OLR1 by competitively sponging let-7 to promote gemcitabine resistance in pancreatic cancer. J Hematol Oncol (2019) 12:1–18. doi: 10.1186/s13045-019-0777-7
332. Zheng D, Liwinski T, Elinav E. Interaction between microbiota and immunity in health and disease. Cell Res (2020) 30(6):492–506. doi: 10.1038/s41422-020-0332-7
333. Nagourney RA, Evans S, Tran PH, Nagourney AJ, Sugarbaker PH. Colorectal cancer cells from patients treated with FOLFOX or CAPOX are resistant to oxaliplatin. Eur J Surg Oncol (2021) 47(4):738–42. doi: 10.1016/j.ejso.2020.09.017
334. Al-Toubah T, Morse B, Pelle E, Strosberg J. Efficacy of FOLFOX in patients with aggressive pancreatic neuroendocrine tumors after prior capecitabine/temozolomide. oncologist (2021) 26(2):115–9. doi: 10.1002/onco.13611
335. Vora KB, Parikh A. Gastrointestinal cancers and the link to the microbiome. Adv Oncol (2023). doi: 10.1016/j.yao.2023.01.013
336. Rahimi AM, Nabavizadeh F, Ashabi G, Halimi S, Rahimpour M, Vahedian J, et al. Probiotic Lactobacillus rhamnosus supplementation improved capecitabine protective effect against gastric cancer growth in male BALB/c mice. Nutr Cancer (2021) 73(10):2089–99. doi: 10.1080/01635581.2020.1832237
337. Hawkins DS, Chi YY, Anderson JR, Tian J, Arndt CA, Bomgaars L, et al. Addition of vincristine and irinotecan to vincristine, dactinomycin, and cyclophosphamide does not improve outcome for intermediate-risk rhabdomyosarcoma: a report from the Children’s Oncology Group. J Clin Oncol (2018) 36(27):2770. doi: 10.1200/JCO.2018.77.9694
338. Viaud S, Saccheri F, Mignot G, Yamazaki T, Daillère R, Hannani D, et al. The intestinal microbiota modulates the anticancer immune effects of cyclophosphamide. science (2013) 342(6161):971–6. doi: 10.1126/science.1240537
339. Daillère R, Vétizou M, Waldschmitt N, Yamazaki T, Isnard C, Poirier-Colame V, et al. Enterococcus hirae and Barnesiella intestinihominis facilitate cyclophosphamide-induced therapeutic immunomodulatory effects. Immunity (2016) 45(4):931–43. doi: 10.1016/j.immuni.2016.09.009
340. He Y, Fu L, Li Y, Wang W, Gong M, Zhang J, et al. Gut microbial metabolites facilitate anticancer therapy efficacy by modulating cytotoxic CD8+ T cell immunity. Cell Metab (2021) 33(5):988–1000.e7. doi: 10.1016/j.cmet.2021.03.002
341. Yi Y, Shen L, Shi W, Xia F, Zhang H, Wang Y, et al. Gut microbiome components predict response to neoadjuvant chemoradiotherapy in patients with locally advanced rectal cancer: a prospective, longitudinal study. Clin Cancer Res (2021) 27(5):1329–40. doi: 10.1158/1078-0432.CCR-20-3445
342. Miao L, Zhang Y, Huang L. mRNA vaccine for cancer immunotherapy. Mol Cancer (2021) 20(1):1–23. doi: 10.1186/s12943-021-01335-5
343. Bose M, Mukherjee P. Potential of anti-MUC1 antibodies as a targeted therapy for gastrointestinal cancers. Vaccines (2020) 8(4):659. doi: 10.3390/vaccines8040659
344. Sheikh M, Poustchi H, Pourshams A, Khoshnia M, Gharavi A, Zahedi M, et al. Household fuel use and the risk of gastrointestinal cancers: the Golestan cohort study. Environ Health Perspect (2020) 128(6):067002. doi: 10.1289/EHP5907
345. Lumish MA, Cercek A. Practical considerations in diagnosing and managing early-onset GI cancers. J Clin Oncol (2022) 40(24):2662–80. doi: 10.1200/JCO.21.02708
346. Sharma VK, Singh TG, Garg N, Dhiman S, Gupta S, Rahman MH, et al. Dysbiosis and Alzheimer’s disease: a role for chronic stress? Biomolecules (2021) 11(05):678. doi: 10.3390/biom11050678
347. Xu K, Gao X, Xia G, Chen M, Zeng N, Wang S, et al. Rapid gut dysbiosis induced by stroke exacerbates brain infarction in turn. Gut (2021) 70(8):1486–94. doi: 10.1136/gutjnl-2020-323263
348. Sehgal K, Gill RR, Widick P, Bindal P, McDonald DC, Shea M, et al. Association of performance status with survival in patients with advanced non–small cell lung cancer treated with pembrolizumab monotherapy. JAMA network Open (2021) 4(2):e2037120–e2037120. doi: 10.1001/jamanetworkopen.2020.37120
349. Shiu K-K, Andre T, Kim TW, Jensen BV, Jensen LH, Punt CJ, et al. KEYNOTE-177: Phase III randomized study of pembrolizumab versus chemotherapy for microsatellite instability-high advanced colorectal cancer. American Society of Clinical Oncology (2021).
350. Vétizou M, Pitt JM, Daillère R, Lepage P, Waldschmitt N, Flament C, et al. Anticancer immunotherapy by CTLA-4 blockade relies on the gut microbiota. Science (2015) 350(6264):1079–84. doi: 10.1126/science.aad1329
351. Liu W, Ma F, Sun B, Liu Y, Tang H, Luo J, et al. Intestinal microbiome associated with immune-related adverse events for patients treated with anti-PD-1 inhibitors, a real-world study. Front Immunol (2021) 12:756872. doi: 10.3389/fimmu.2021.756872
352. Dougan M, Wang Y, Rubio-Tapia A, Lim JK. AGA clinical practice update on diagnosis and management of immune checkpoint inhibitor colitis and hepatitis: expert review. Gastroenterology (2021) 160(4):1384–93. doi: 10.1053/j.gastro.2020.08.063
353. Grieneisen L, Dasari M, Gould TJ, Björk JR, Grenier JC, Yotova V, et al. Gut microbiome heritability is nearly universal but environmentally contingent. Science (2021) 373(6551):181–6. doi: 10.1126/science.aba5483
354. Liu Y-X, Qin Y, Chen T, Lu M, Qian X, Guo X, et al. A practical guide to amplicon and metagenomic analysis of microbiome data. Protein Cell (2021) 12(5):315–30. doi: 10.1007/s13238-020-00724-8
355. Siddiqui BA, Gheeya JS, Goswamy R, Bathala TK, Surasi DS, Gao J, et al. Durable responses in patients with genitourinary cancers following immune checkpoint therapy rechallenge after moderate-to-severe immune-related adverse events. J immunotherapy Cancer (2021) 9(7). doi: 10.1136/jitc-2021-002850
356. Wang A, Xu Y, Fei Y, Wang M. The role of immunosuppressive agents in the management of severe and refractory immune-related adverse events. Asia-Pacific J Clin Oncol (2020) 16(4):201–10. doi: 10.1111/ajco.13332
357. Chang AE, Golob JL, Schmidt TM, Peltier DC, Lao CD, Tewari M. Targeting the gut microbiome to mitigate immunotherapy-induced colitis in cancer. Trends Cancer (2021) 7(7):583–93. doi: 10.1016/j.trecan.2021.02.005
358. Douafer H, Andrieu V, Phanstiel O, Brunel JM. Antibiotic adjuvants: make antibiotics great again! J medicinal Chem (2019) 62(19):8665–81. doi: 10.1021/acs.jmedchem.8b01781
359. Knezevich SR, McFadden DE, Tao W, Lim JF, Sorensen PHB. A novel ETV6-NTRK3 gene fusion in congenital fibrosarcoma. Nat Genet (1998) 18(2):184–7. doi: 10.1038/ng0298-184
360. Wang F, Yin Q, Chen L, Davis MM. Bifidobacterium can mitigate intestinal immunopathology in the context of CTLA-4 blockade. Proc Natl Acad Sci (2018) 115(1):157–61. doi: 10.1073/pnas.1712901115
361. Tanoue T, Morita S, Plichta DR, Skelly AN, Suda W, Sugiura Y, et al. A defined commensal consortium elicits CD8 T cells and anti-cancer immunity. Nature (2019) 565(7741):600–5. doi: 10.1038/s41586-019-0878-z
362. Zhuo Q, Yu B, Zhou J, Zhang J, Zhang R, Xie J, et al. Lysates of Lactobacillus acidophilus combined with CTLA-4-blocking antibodies enhance antitumor immunity in a mouse colon cancer model. Sci Rep (2019) 9(1):20128. doi: 10.1038/s41598-019-56661-y
363. DePeaux K, Delgoffe GM. Metabolic barriers to cancer immunotherapy. Nat Rev Immunol (2021) 21(12):785–97. doi: 10.1038/s41577-021-00541-y
364. Murciano-Goroff YR, Warner AB, Wolchok JD. The future of cancer immunotherapy: microenvironment-targeting combinations. Cell Res (2020) 30(6):507–19. doi: 10.1038/s41422-020-0337-2
365. Uribe-Herranz M, Rafail S, Beghi S, Gil-de-Gómez L, Verginadis I, Bittinger K, et al. Gut microbiota modulate dendritic cell antigen presentation and radiotherapy-induced antitumor immune response. J Clin Invest (2020) 130(1):466–79. doi: 10.1172/JCI124332
366. Wei J, Montalvo-Ortiz W, Yu L, Krasco A, Ebstein S, Cortez C, et al. Sequence of αPD-1 relative to local tumor irradiation determines the induction of abscopal antitumor immune responses. Sci Immunol (2021) 6(58):eabg0117. doi: 10.1126/sciimmunol.abg0117
367. Gomaa EZ. Human gut microbiota/microbiome in health and diseases: a review. Antonie Van Leeuwenhoek (2020) 113(12):2019–40. doi: 10.1007/s10482-020-01474-7
368. Zmora N, Suez J, Elinav E. You are what you eat: diet, health and the gut microbiota. Nat Rev Gastroenterol Hepatol (2019) 16(1):35–56. doi: 10.1038/s41575-018-0061-2
369. Hong J, Fang J-Y. Gut microbiota impacts on the efficacy of anticancer treatment of colorectal cancer. In: Microbiome in gastrointestinal cancer. Springer (2023). p. 237–49.
370. Uberoi A, Bartow-McKenney C, Zheng Q, Flowers L, Campbell A, Knight SA, et al. Commensal microbiota regulates skin barrier function and repair via signaling through the aryl hydrocarbon receptor. Cell Host Microbe (2021) 29(8):1235–1248.e8. doi: 10.1016/j.chom.2021.05.011
371. Gerassy-Vainberg S, Blatt A, Danin-Poleg Y, Gershovich K, Sabo E, Nevelsky A, et al. Radiation induces proinflammatory dysbiosis: transmission of inflammatory susceptibility by host cytokine induction. Gut (2018) 67(1):97–107. doi: 10.1136/gutjnl-2017-313789
372. Ferreira MR, Muls A, Dearnaley DP, Andreyev JN. Microbiota and radiation-induced bowel toxicity: lessons from inflammatory bowel disease for the radiation oncologist. Lancet Oncol (2014) 15(3):e139–47. doi: 10.1016/S1470-2045(13)70504-7
373. Saito S, Kitabatake M, Ouji-Sageshima N, Ogawa T, Oda A, Nishimura T, et al. Angiopoietin-like 4 is a critical regulator of fibroblasts during pulmonary fibrosis development. Am J Respir Cell Mol Biol (2023) 69(3):328–39. doi: 10.1165/rcmb.2022-0304OC
374. Park M, Kwon J, Shin HJ, Moon SM, Kim SB, Shin US, et al. Butyrate enhances the efficacy of radiotherapy via FOXO3A in colorectal cancer patient−derived organoids. Int J Oncol (2020) 57(6):1307–18. doi: 10.3892/ijo.2020.5132
375. Liao H, Liu S, Wang H, Su H, Liu Z. Enhanced antifungal activity of bovine lactoferrin-producing probiotic Lactobacillus casei in the murine model of vulvovaginal candidiasis. BMC Microbiol (2019) 19:1–13. doi: 10.1186/s12866-018-1370-x
376. Lai H-H, Chiu C-H, Kong M-S, Chang C-J, Chen C-C. Probiotic Lactobacillus casei: effective for managing childhood diarrhea by altering gut microbiota and attenuating fecal inflammatory markers. Nutrients (2019) 11(5):1150. doi: 10.3390/nu11051150
377. Guo H, Chou WC, Lai Y, Liang K, Tam JW, Brickey WJ, et al. Multi-omics analyses of radiation survivors identify radioprotective microbes and metabolites. Science (2020) 370(6516):eaay9097. doi: 10.1126/science.aay9097
378. Goc J, Sonnenberg GF. Harnessing microbiota to improve immunotherapy for gastrointestinal cancers. Cancer Immunol Res (2022) 10(11):1292–8. doi: 10.1158/2326-6066.CIR-22-0164
379. Liu J, Lin Z, Zhao Y. Intestinal microbiomes in gastrointestinal cancer. Highlights Science Eng Technol (2023) 36:269–75. doi: 10.54097/hset.v36i.5683
380. Wan X, Song M, Wang A, Zhao Y, Wei Z, Lu Y. Microbiome crosstalk in immunotherapy and antiangiogenesis therapy. Front Immunol (2021) 12:747914. doi: 10.3389/fimmu.2021.747914
381. Liang H, Jo JH, Zhang Z, MacGibeny MA, Han J, Proctor DM, et al. Predicting cancer immunotherapy response from gut microbiomes using machine learning models. Oncotarget (2022) 13:876. doi: 10.18632/oncotarget.28252
382. Peng Z, Cheng S, Kou Y, Wang Z, Jin R, Hu H, et al. The gut microbiome is associated with clinical response to anti–PD-1/PD-L1 immunotherapy in gastrointestinal cancer. Cancer Immunol Res (2020) 8(10):1251–61. doi: 10.1158/2326-6066.CIR-19-1014
383. Zhou H, Yuan Y, Wang H, Xiang W, Li S, Zheng H, et al. Gut microbiota: a potential target for cancer interventions. Cancer Manage Res (2021) p:8281–96. doi: 10.2147/CMAR.S328249
384. Liu Y, Baba Y, Ishimoto T, Tsutsuki H, Zhang T, Nomoto D, et al. Fusobacterium nucleatum confers chemoresistance by modulating autophagy in oesophageal squamous cell carcinoma. Br J Cancer (2021) 124(5):963–74. doi: 10.1038/s41416-020-01198-5
385. Zhang S, Yang Y, Weng W, Guo B, Cai G, Ma Y, et al. Fusobacterium nucleatum promotes chemoresistance to 5-fluorouracil by upregulation of BIRC3 expression in colorectal cancer. J Exp Clin Cancer Res (2019) 38:1–13. doi: 10.1186/s13046-018-0985-y
386. Reis Ferreira M, Andreyev HJ, Mohammed K, Truelove L, Gowan SM, Li J, et al. Microbiota-and radiotherapy-induced gastrointestinal side-effects (MARS) study: a large pilot study of the microbiome in acute and late-radiation enteropathy. Clin Cancer Res (2019) 25(21):6487–500. doi: 10.1158/1078-0432.CCR-19-0960
387. Riehl TE, Alvarado D, Ee X, Zuckerman A, Foster L, Kapoor V, et al. Lactobacillus rhamnosus GG protects the intestinal epithelium from radiation injury through release of lipoteichoic acid, macrophage activation and the migration of mesenchymal stem cells. Gut (2019) 68(6):1003–13. doi: 10.1136/gutjnl-2018-316226
Keywords: gastrointestinal cancer, gut microbiome, microbiota, cancer therapy, inflammation
Citation: Yarahmadi A and Afkhami H (2024) The role of microbiomes in gastrointestinal cancers: new insights. Front. Oncol. 13:1344328. doi: 10.3389/fonc.2023.1344328
Received: 01 December 2023; Accepted: 20 December 2023;
Published: 01 February 2024.
Edited by:
Ralf Weiskirchen, RWTH Aachen University, GermanyReviewed by:
Neha Nanda, Harvard Medical School, United StatesCopyright © 2024 Yarahmadi and Afkhami. This is an open-access article distributed under the terms of the Creative Commons Attribution License (CC BY). The use, distribution or reproduction in other forums is permitted, provided the original author(s) and the copyright owner(s) are credited and that the original publication in this journal is cited, in accordance with accepted academic practice. No use, distribution or reproduction is permitted which does not comply with these terms.
*Correspondence: Hamed Afkhami, aGFtZWRhZmtoYW1pNzBAZ21haWwuY29t
Disclaimer: All claims expressed in this article are solely those of the authors and do not necessarily represent those of their affiliated organizations, or those of the publisher, the editors and the reviewers. Any product that may be evaluated in this article or claim that may be made by its manufacturer is not guaranteed or endorsed by the publisher.
Research integrity at Frontiers
Learn more about the work of our research integrity team to safeguard the quality of each article we publish.