- 1School of Medicine, University of Limerick, Limerick, Ireland
- 2Health Research Institute, University of Limerick, Limerick, Ireland
- 3Lambe Institute for Translational Research, University of Galway, Galway, Ireland
- 4School of Allied Health, University of Limerick, Limerick, Ireland
- 5Science Foundation Ireland Research Centre in Pharmaceuticals (SSPC), University of Limerick, Limerick, Ireland
- 6Department of Chemical Sciences, Bernal Institute, University of Limerick, Limerick, Ireland
Introduction: The extracellular matrix (ECM) has been heavily implicated in the development and progression of cancer. We have previously shown that Annexin A2 is integral in the migration and invasion of breast cancer cells and in the clinical progression of ER-negative breast cancer, processes which are highly influenced by the surrounding tumor microenvironment and ECM.
Methods: We investigated how modulations of the ECM may affect the role of Annexin A2 in MDA-MB-231 breast cancer cells using western blotting, immunofluorescent confocal microscopy and immuno-precipitation mass spectrometry techniques.
Results: We have shown that the presence of collagen-I, the main constituent of the ECM, increases the post-translational phosphorylation of Annexin A2 and subsequently causes the translocation of Annexin A2 to the extracellular surface. In the presence of collagen-I, we identified fibronectin as a novel interactor of Annexin A2, using mass spectrometry analysis. We then demonstrated that reducing Annexin A2 expression decreases the degradation of fibronectin by cancer cells and this effect on fibronectin turnover is increased according to collagen-I abundance.
Discussion: Our results suggest that Annexin A2's role in promoting cancer progression is mediated by collagen-I and Annexin A2 maybe a therapeutic target in the bi-directional cross-talk between cancer cells and ECM remodeling that supports metastatic cancer progression.
1 Introduction
The study of cancer has evolved over decades to encompass not only malignant cells, but also those cells’ neighbors and surroundings, known as the tumor microenvironment (TME). Apart from stromal and immune cells, the principal component of the TME is the extracellular matrix (ECM). Comprised of a diverse array of proteoglycans and fibrillar proteins, the ECM can be thought of as a 3-dimenstional scaffold which gives structure and physical support to cells and tissues. A tangible paradigm shift has occurred in our understanding of the ECM, as we now understand it is not simply an inert bystander in homeostasis, but rather it is a dynamic and active component in maintaining appropriate cellular signaling pathways and tissue integrity (1). This dynamicity can be hijacked in the malignant process, wherein the normal levels of organization between tissue and stroma become disrupted as tumor cells aberrantly remodel, dysregulate and invade their microenvironment. An over-expression of ECM proteins and ECM remodeling enzymes are found in a variety of solid cancer types (2, 3) often leading to the creation of a desmoplastic TME. This is characterized by the increased deposition and cross-linking of fibrotic ECM proteins, such as collagens, in particular collagen Type I (collagen-I) (4, 5).
In human breast cancers, a desmoplastic or fibrotic reaction, triggered by altered deposition and remodeling of collagen-I, is often the first noticeable sign of breast cancer development and is a common characteristic of the disease with pathological consequence (6). Previous work by our lab has shown that the presence of collagen-I and fibronectin increases both the proliferation and migration of breast cancer cells (7). Collagen abundance and fibre density has been associated with breast tumor growth (8, 9), spread to the lymph nodes (10) and lung metastases (11). Furthermore, high mammographic breast density, a characteristic strongly associated with increased collagen abundance (12, 13), is linked to a 4–6 fold increase in breast cancer risk, to metastatic progression and to overall poor prognosis (13–17).
TME dysregulation is multifactorial and, in its essence, results from aberrant inside-out and outside-in signaling between cancer cells and their surroundings. One such mediator for this TME-cancer cell cross-talk is Annexin A2 due to its transient intra- to extra-cellular cycling and its role in ECM degradation. The translocation of Annexin A2 is regulated by its binding to S100A10 and by its post-translational modifications (18, 19). Phosphorylation of Annexin A2 at Tyr24 (aka Tyr23) by the Src family of kinases, promotes Annexin A2 expression on the cell surface (18, 20–23). Conversely, phosphorylation at Ser26 (aka Ser25) by Protein Kinase C (PKC) inhibits Annexin A2 surface expression by interrupting its binding to S100A10 (23–25). Once on the extracellular surface, Annexin A2 plays a role in ECM remodeling by acting as a co-receptor for plasminogen and tissue-type plasminogen activator (t-PA), thus promoting the localized activation of plasmin, a serine protease known to degrade ECM proteins such as fibronectin and laminin (26–29). Activated plasmin also functions to release sequestered growth factors in the TME and further activate matrix metalloproteases (MMPs), triggering a proteolytic cascade, which increases the invasive capacity of cancer cells (30–32). Despite these molecular insights and a number of links between Annexin A2 phosphorylation and cancer, there is scant information regarding the physiological regulation and implications of this in the context of the TME.
Our previous work revealed Annexin A2 to be a newly synthesized protein in the growth factor induced migration and invasion of breast cancer cells. Further to this, we showed that Annexin A2’s expression is required to support the proliferation and migration of cells in vitro. In patients, we found Annexin A2 expression to be higher in aggressive, ER negative subtypes of breast cancer and to be associated with a higher risk of metastatic progression (33). In addition to our findings, several other studies have linked Annexin A2 to cancer progression as reviewed in (19, 34, 35). Similarly, ECM dysregulation, including desmoplasia and increased collagen expression has been also linked to the metastatic process (36, 37). Consequently, we wanted to investigate the potential relationship between ECM dysregulation and Annexin A2 expression as both represent hallmarks of aggressive cancer progression (35, 38). Considering Annexin A2’s well documented role in the activation of ECM remodeling proteases, this led to our rational to investigate the relationship here as a potential explanation for our previous findings on Annexin A2’s role in metastatic processes and patient prognosis.
2 Materials and methods
2.1 Cell culture
Human breast cancer cell lines MDA-MB-231 were purchased from the ECACC culture collection (Sigma Aldrich, Wicklow, Ireland). Cell lines were routinely tested upon freezing and thawing for mycoplasma contamination using PCR. Cells were maintained at 37°C, 5% CO2 humidified incubator in DMEM-high glucose supplemented with 10% fetal bovine serum, 5% l-glutamine and 5% penicillin/streptomycin (all obtained from Sigma Aldrich).
2.2 Extracellular matrix coating of tissue culture surface
All matrix proteins were diluted in sterile 1 X PBS. Concentrations used were as follows: Collagen-I = 1.8 µg/cm2, fibronectin = 20µg/cm2. Following coating, plates were incubated at 37°C for 4 hours before the excess coating was aspirated off and cells plated onto the surface.
2.3 Cell lysis, SDS PAGE and western blotting
The cells were lysed in 1% NP-40 lysis buffer then quantified and denatured with SDS loading buffer and boiled for 5 min. Lysates were separated on 12% SDS acrylamide gels and subsequently transferred to nitrocellulose membrane. Membranes were blocked using 5% BSA for 1 h at room temperature then probed with corresponding primary antibodies at 4°C overnight. Dilutions of antibodies were as follows: Annexin A2 1:1000 (Abcam, Cambridge UK), Annexin A2 1:1000 (BD Bioscience), B-Actin 1:1000 (Sigma), phospo-Tyr24-Annexin A2 1:250 (Santa Cruz Biotechnology, Heidelberg, GE) GAPDH 1:1000 (Sigma) Na/K-ATPase 1:500 (Cell Signaling, Beverly, MA), Fibronectin 1:100, (Santa Cruz). IRdye700- or IRdye800-conjugated secondary antibodies, were then coupled to the primary antibody for 1 h at room temperature. Protein bands were detected using the Odyssey Sc (LI-COR, Cambridge, UK) and quantified using Image Studio 5.2 (LI-COR).
2.4 Surface and sub-cellular fractionation
To separate cytosolic and membrane fractions of cellular lysates, cells were washed with cold PBS and suspended in buffer containing 320 mM Sucrose, 10 mM TRIS-HCL pH 7.4, 10 mM EDTA and 10 mM EGTA before homogenization using a 26-G needle. Differential centrifugation was then used to isolate subcellular fractions, wherein lysates were spun at 800 × g for 10 minutes to remove nuclei, at 16000 x g for 40 minutes to isolate the cytosolic fraction and then again at 16000 x g for 40 minutes to pellet the membrane fraction. Resulting protein fractions were quantified using the Bradford Assay (B6916 Sigma Aldrich). Following protein quantification, SDS-PAGE separation, and western blotting, GAPDH and Na/K ATPase were used as cytosolic and membrane markers respectively.
To elute cell surface proteins, cells were washed with ice-cold PBS before being incubated, rocking at room temperature with 0.53mM EDTA-PBS for 10 mins, as described in (39) EDTA wash was then collected and subjected to acetone precipitation to concentrate protein amount before being quantified and separated using SDS-PAGE and western blotting. Ponceau staining of total protein was used as a loading control.
2.5 Immunoprecipitation
For Annexin A2 immunoprecipitation, cell lysates were pre-cleared with protein G agarose beads (Sigma Aldrich) for 1 hour with gentle rotation at 4°C. Cleared lysates were then transferred in a new tube for incubation with agarose beads and primary anti-Annexin A2 antibody (Rabbit, ab41803, Abcam) overnight at 4°C with gentle rotation. The beads were washed with lysis buffer and boiled with 2x SDS loading buffer at 100°C for 5 minutes before separation using SDS-PAGE.
For fibronectin immunoprecipitation, protein G agarose beads were incubated with anti-FN for 1 hour, rotating at 4°C. Beads were then spun down and added to purified fibronectin-PBS solution for 3 hours, rotating at 4°C. Beads were then washed with lysis buffer and added to MDA-MB-231 cell lysate, and incubated overnight, rotating at 4°C. The beads were washed with lysis buffer and boiled with 2x SDS loading buffer at 100°C for 5 minutes before separation using SDS-PAGE. For all experiments, a control/blank IP was prepared with all the reagents, but without the antibody, to account for non-specific binding.
2.6 Mass spectrometry analysis
Following SDS-PAGE, gel lanes containing protein bands stained with Coomassie were excised from the gel and transported to Mass Spectrometry and Proteomics Facility, University of St Andrews, Fife, for analysis as described in (33). Briefly, gel chunks were subjected to trypsin digestion, peptides were separated by nano-LC utilizing an Eksigent two-dimensional LC NanoLC system (Eksigent/Applied Biosystems Sciex, MA, USA) interfaced with a QStar XL mass spectrometer (Applied Biosystems Sciex, MA, USA). Data sets were searched against the NCBInr 20190208 database using MASCOT software (Matrix Science, MA, USA) under the following parameters: maximum one missed cleavage of trypsin digestion, carbamidomethyl (C) as a fixed modification, oxidation (M) as a variable modification, a peptide mass tolerance of ± 20 ppm and a fragment mass tolerance of ±0.05 Da. Only scores higher than the significance threshold (p < 0.05) were reported.
2.7 Functional and interaction analysis
Prior to functional analysis, protein lists were first reduced using the cut-off of 4 or more peptide matches. Protein-protein interaction networks were probed using STRING v11 (40), visualized using Cytoscape (V3.8.2) with StringApp (41) and analysed using CentiScape app (42). Proteins were then analyzed using the PANTHER Overrepresentation Test (V14.1) (43) with the Fisher’s exact test to calculate p-value and Benjamini–Hochberg procedure to calculate false discovery rate (FDR). FDR cut-off was <0.05. In the case of Cellular Component sub-ontology analysis, REVIGO was used (http://revigo.irb.hr/), at a similarity cut-off of 0.9, to reduce the quantity of redundant and overlapping terms (44). Overrepresented GO terms were then visualized as a network using Cytoscape. Centrality of nodes was assessed using CentiScaPe.
2.8 Immunofluorescence of extracellular surface protein
MDA-MB-231 cells were plated at 10,000 cells per well in 96-well Greiner ScreenStar (Cruinn, Dublin, IE) plates and incubated for 24 hours. The cells were then fixed with 4% PFA at room temperature for 10 minutes before PFA was removed and the cells washed with PBS. Fixation was then quenched by the addition of 50mM NH4CL for 1 hour. Cells were not subjected to a permeabilizing agent to maintain membrane integrity and to restrict antibody staining to the extracellular surface. The non-permeabilized cells were then blocked using 2% donkey serum (DS) – PBS. Primary antibodies were then applied, diluted in 10% DS-PBS and incubated overnight at 4°C. The next day, wells were washed three times using PBS, before secondary antibodies and counterstains were added, diluted in 10% DS-PBS. After 1 hour, cells were washed three times with PBS before being covered with PBS for imaging. Plates were then imaged using ImageXpress confocal microscope (Molecular Devices, CA, USA) equipped with metaXpress software. Images were acquired using the 50 μm slit confocal at 20X magnification. 9 sites per well were acquired. Exposure times, focus and offset were maintained for all wells in a plate. Images were then exported and analyzed using Cell Profiler vers.3.1.9 (Broad Institute, US) (45). Within this, to identify primary objects (nuclei) a global threshold strategy, with the Otsu method was used. Secondary objects (cells) were then identified using the Propagation method, under the same threshold parameters.
2.9 Use of publically available expression & clinical data
cBioportal (www.cbioportal.org) (46, 47) was used to export expression data and clinical characteristics (as of October 2021) from the Breast Invasive Carcinoma (TCGA, PanCancer Atlas) study. The following search parameters were used: Selected Studies: Breast Invasive Carcinoma (TCGA, PanCancer Atlas) (1084 total samples), Select Genomic Profiles: All, Select Patient/Case Set: All samples (1804). Genes: ANXA2 & COL1A1. Phosphoprotein site level expression data by CPTAC (TMT, Log2ratio) for Tyr 24 of Annexin A2 and COL1A1 mRNA Expression, [RSEM, log2(value + 1)] for 98 samples were plotted against each other using Graphpad Prism and Spearman’s correlation.
3 Results
3.1 Collagen-I triggers the post-translational phosphorylation of Annexin A2 and subsequent membrane and extracellular translocation in breast cancer cells
Using immunoblot analysis we firstly investigated how the expression and regulation of Annexin A2 in breast cancer cells is affected by the presence of collagen-I. Collagen-I was chosen as it is the most abundant constituent of the ECM and is linked to breast cancer proliferation, migration and metastasis (7, 9, 11, 48). We were interested to see that, in the presence of collagen-I, the phosphorylation of Annexin A2 at Tyr24 is dramatically increased, as illustrated in Figure 1A. The protein levels of total Annexin A2 were unchanged. Furthermore, gene expression analysis of ANXA2 mRNA levels shows this increase in phosphorylated Annexin A2 is not a result of increasing total levels of Annexin A2 (Supplementary Figure 1), in fact, collagen-I appears to decrease levels of ANXA2 mRNA. This suggests a possible regulation at a translational level, potentially through Annexin A2 phosphorylation promoting the formation of the Annexin A2-S100A10 heterotetrameric complex, in which the proteins have been reported to exert a reciprocal co-stabilization effect on each other’s protein levels (49–51). Moreover, in applying our observation to publically available patient data [Breast Invasive Carcinoma- TCGA, PanCancer Atlas (47)] using cBioportal (46, 52), we can see that there is a low but statistically significant positive correlation between the mRNA expression of COL1A1 and the level of phosphorylation of Annexin A2 at Tyr 24 (Spearman’s correlation, r = 0.2429, p = 0.0160). As seen in Figure 1A (ii) this suggests the trend observed in in vitro could hold true in patients, pending further investigation from these results, we can conclude that although collagen does not increase the expression of Annexin A2, it does appear to regulate an important post-translational modification of Annexin A2 (23).
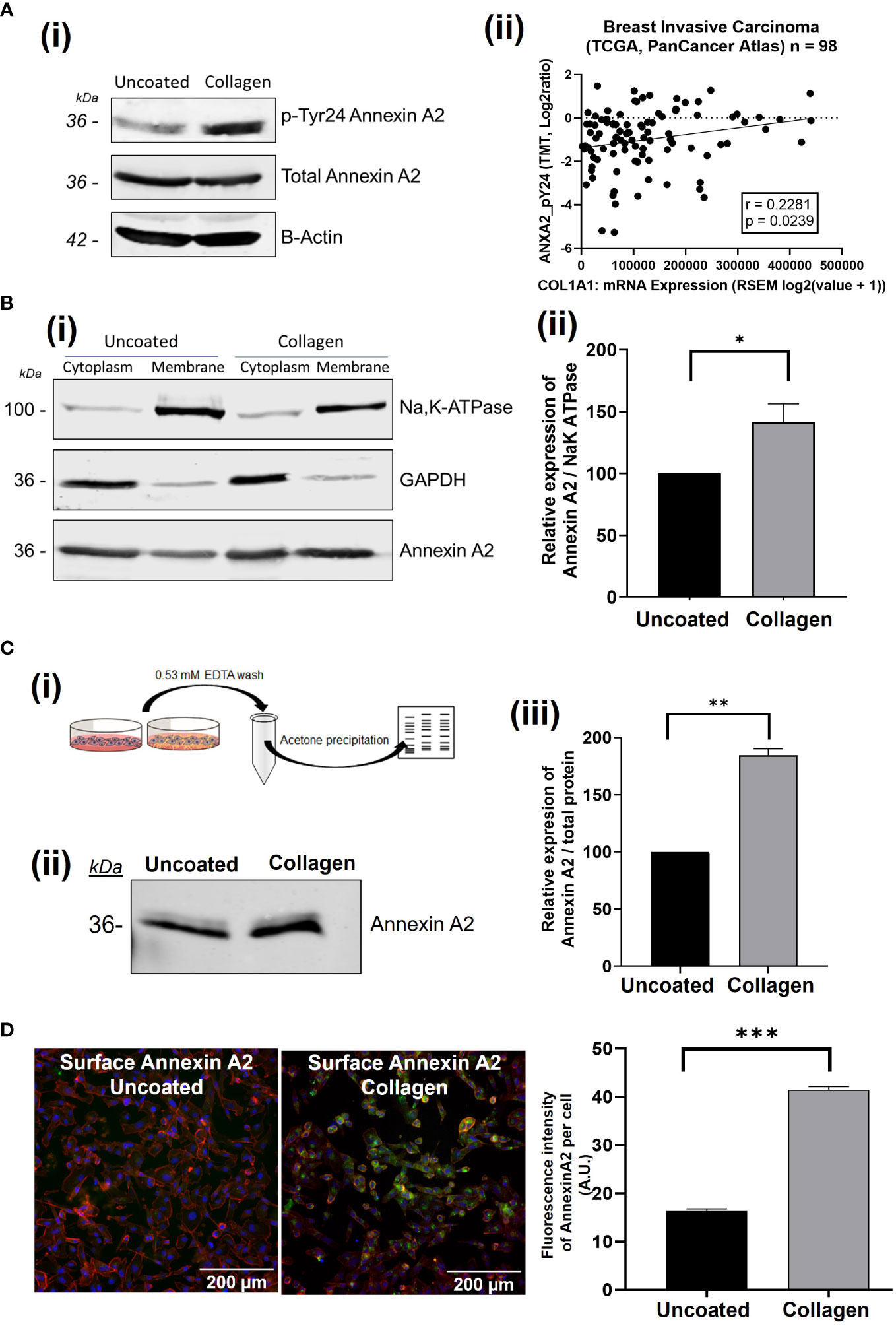
Figure 1 The presence of collagen-I induces the phosphorylation of Annexin A2 at Tyr 24 and translocation to the extracellular membrane. (A) MDA-MB-231 cells were grown on uncoated or collagen-I coated plates for 24 hours before western blot analysis. Representative blot and corresponding graph showing the increase in AnxA2 phosphorylation at Tyr24 (n=3). (ii): Scatterplot indicating a significant correlation the relationship between phospho-Tyr24-Annexin A2 levels and COL1A1 expression in breast cancer patient samples from the “Breast Invasive Carcinoma (TCGA, PanCancer Atlas)” study (Spearman’s correlation, r = 0.2429, p = 0.0160, n=98). (B) (i) Subcellular fractionation and western blot analysis shows an increase in Annexin A2 at the cell membrane in the presence of collagen-I. GAPDH and Na/K-ATPase were used as markers for the cytoplasm and the membrane respectively. (ii) Densitometry analysis of Annexin A2 protein bands normalized against Na/K-ATPase and expressed as a percentage of Annexin A2 level in uncoated control, measured using Image Studio software. Data displayed as mean +/- SEM. (students T-test, *p < 0.05, n=4) (C) (i) Workflow of surface elute quantification. (ii) Western blot showing increased Annexin A2 in the surface elutes of cells plated on collagen-I. (iii) Protein amounts were quantified via densitometry of Annexin A2 protein bands normalized against total protein/ponceau staining and expressed as a percentage of Annexin A2 level in uncoated control. Data displayed as mean +/- SEM. (Student’s T-test, *p < 0.05, n=2). (D) Non-permeabilized cells plated on uncoated or collagen-I coated plastic were surface stained for Annexin A2, and counterstained with Hoechst (nuclei) and TRITC phalloidin (actin cytoskeleton), before being imaged and analyzed using Cell Profiler. Analysis of the fluorescence intensity per cell shows a higher fluorescence thus higher surface Annexin A2 expression when cells are plated on collagen for 24 hours. Data displayed as mean integrated fluorescence per cell ± SEM (p = <0.0001, n=3, approx. 3200 cells analyzed per plate). *p ≤ 0.05, **p ≤ 0.01, ***p ≤ 0.001.
Phosphorylation of Annexin A2 at Tyr24 has been shown to modulate AnxA2-S100A10 complex formation and extracellular translocation, as well as cytoskeletal rearrangement and epithelial to mesenchymal transition (18, 21, 53–56). Thus, we investigated how this collagen-I mediated phosphorylation of Annexin A2 affects its location within the cell using a variety of methods. Firstly, using subcellular fractionation to separate whole cell lysates into cytosolic and membrane fractions, we showed that when cells are plated on collagen-I, more Annexin A2 is found within the membrane fraction than cells on uncoated plastic. (Figure 1B, Student’s T-test, p = 0.0356, n=3).
As Annexin A2 relies on Ca2+ for its membrane phospholipid binding properties, the addition of a chelating agent such as EDTA can disrupt this binding and elute Annexin A2 from the surface of cells. We next wanted to test whether the presence of collagen-I would affect the amount of Annexin A2 eluted. To do this, cells were plated on either uncoated or collagen-I coated 10cm2 plates, incubated for 24 hours then washed with EDTA-PBS. As this wash was extremely dilute, we carried out an acetone precipitation to concentrate the protein amount before running on 12% SDS-PAGE and western blotting. As shown in Figure 1C, a greater amount of Annexin A2 protein was eluted from the cell surface when the cells were plated on collagen-I (Student’s T-test, p = 0.0042, n=2). The amount of Annexin A2 was normalized to total protein content using ponceau staining and Image studio software.
To further confirm this movement of Annexin A2 to the extracellular surface of cells when in contact with collagen-I we next employed immunofluorescent microscopy. Cells were plated on either uncoated or collagen-I coated wells for 24 hours before being fixed and surface stained for the presence of Annexin A2. The omission of a permeabilization agent, which penetrates the cell membrane, allowed us to label the extracellular Annexin A2. Following acquisition, images were imported into Cell Profiler for single cell fluorescence analysis, with the workflow outlined in Supplementary Figure 2. Comparison of the fluorescence intensity of Annexin A2-A488 surface staining showed that non-permeabilized cells plated on collagen expressed more surface Annexin A2 than non-permeabilized cells plated on plastic. Statistical analysis shows the mean fluorescence of cells on collagen-I is significantly higher than cell on plastic (Figure 1D), Student’s t test, p = <0.0001, n=3). These results strongly suggest, through three independent experimental methods, that the presence of collagen-I triggers the cell surface localization of Annexin A2 in breast cancer cells.
3.2 The identification of candidate Annexin A2 binding proteins using interactome analysis
Following our discovery of the role collagen-I plays in the post-translational phosphorylation and localization of Annexin A2, our next step was to investigate the functional consequences of this relationship in cancer cells. To achieve this, we investigated Annexin A2 interactors and how this is altered when cells are in contact with collagen-I. Protein-protein interaction analysis is a well-known method of investigating protein function and signal transduction within cells (57). To investigate the interactions of Annexin A2 in our cells, we carried out an Annexin A2 affinity pull-down, followed by a mass spectrometry screen as illustrated in Figure 2A. The proteins identified in the “Blank” or no antibody, negative control sample were subtracted from the “Uncoated” and “Collagen” binding partners lists to account for unspecific binding and contaminates. This resulted in lists of 357 proteins in the “Uncoated” sample and 378 proteins in the “Collagen” sample being identified as candidate Annexin A2 binding proteins. Each protein was assigned its corresponding HGNC gene id to avoid any duplicates, protein aliases or Microsoft Excel errors being included (58).
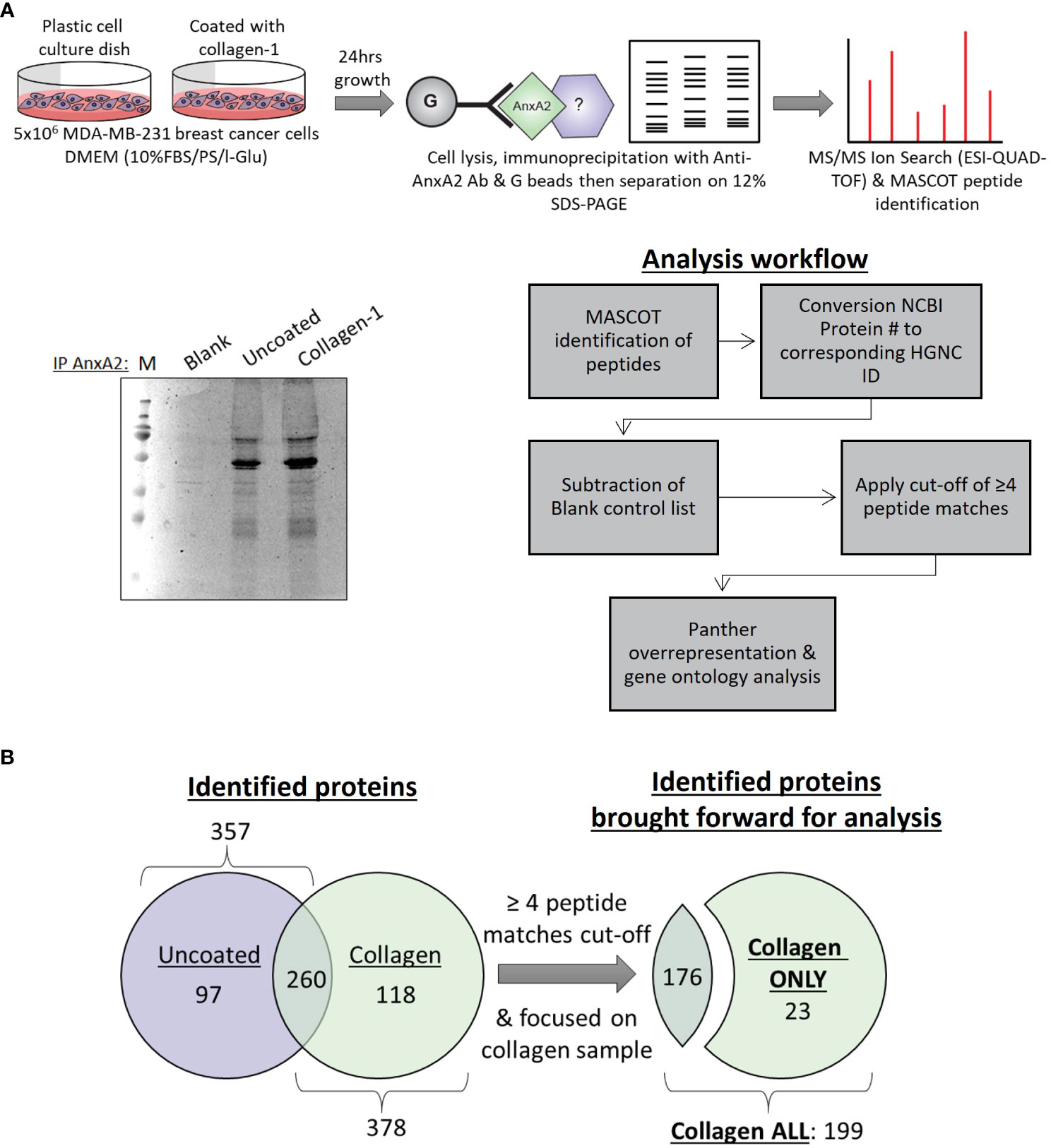
Figure 2 Mass spectrometry analysis of Annexin A2 binding partners with and without the presence of collagen-I (A) Optimization and setup of a workflow to determine Annexin A2 binding partners and how this is affected by the presence of collagen-I. (B) Lists of identified proteins from “Uncoated” (n=357) and “Collagen” (n=378) samples were truncated according to the cut-off of ≥ 4 peptide matches, producing “Collagen ONLY” (n=23) and “Collagen ALL” (n=199) lists for subsequent analysis.
In both lists, Annexin A2 was one of the most highly scored proteins, giving us confidence in the reliability and quality of our experimental approach and adding credence to our subsequent findings. Furthermore, previously reported binding partners of Annexin A2 such as Ahnak (59, 60) and RACK1(aka GNB2L1) (61, 62) were also identified and highly scored within our lists further adding to experimental validity. To ensure the validity of identified proteins and to maintain a high level on confidence in our findings, only proteins with ≥ 4 peptide matches were included in subsequent analysis from here on, as displayed in Figure 2B.
Due to our discovery of the role of collagen-I in regulating the post-translational modification and location of Annexin A2, we assessed the overlap between proteins identified as Annexin A2 interactors when cells are plated on collagen-I (referred to as “Collagen ALL”) and proteins known to be involved in the ECM. This was achieved by comparing with the MatrisomeDB human in-silico matrisome dataset, which is a curated collection of proteomic data from 17 studies on the ECM (63). The comparison (Figure 3A), revealed a discrete and interconnected network of proteins, centered around the ECM protein fibronectin (FN1). Analysis of this network using Centiscape (42) showed FN1 to have the maximum values of centrality parameters including: eccentricity, closeness, centroid, betweenness, stress and radiality, suggesting a central regulatory role of FN1 within this network (Figure S3). To further probe the specific effect of collagen-I on Annexin A2, we next evaluated the proteins which were only identified in the collagen-I sample (i.e., subtracting those found in the intersection with “Uncoated” control), hereafter named “Collagen ONLY”, (Supplementary Table 2). When we investigate the protein-protein interactions within this list using String, we can see that nearly every node is in an interconnected network, again surrounding fibronectin as a central node, even without the addition of Annexin A2 as a central bait node (Figure 3B). This suggests a functional relationship or pathway between these potential Annexin A2 binding partners, with fibronectin as a central interactor. Furthermore, analysis of fibronectin’s position of centrality within the network revealed high values for a number of parameters, including radiality (protein is easily central to the regulation of other proteins but with the possibility to be irrelevant for few other proteins), stress (protein is highly relevant in connecting regulatory molecules and heavily involved in cellular processes) and betweenness (likely crucial to maintain functionality and coherence of signaling mechanisms) (Supplementary Figure 3A) (42, 64). In addition, a scatter plot of node centrality highlights the nodes of most relevance in the upper right quadrant as FN1, followed by UBC and RPS15 (Supplementary Figure 3B). To understand the functional consequences of collagen-I’s effect on the regulation of Annexin A2, we further analyzed the “Collagen ONLY” list of proteins, using the PANTHER overrepresentation test (43). As depicted in Figure 3C the most significantly enriched function was cell adhesion molecule binding (GO:0050839), with 8 of the identified proteins being associated with this GO term (Desmoplakin, Thrombospondin-1, DExH-Box Helicase 29, Heat Shock Protein Family A (Hsp70) Member 8, Fibronectin, Tight junction protein ZO-2, Ataxin-2-like protein and Cytoskeleton-associated protein 5). The Gene Ontology term “cell adhesion molecule binding” is defined as interacting selectively and non-covalently with a cell adhesion molecule or adhesive extracellular matrix constituent (65). This again points to fibronectin as an important interactor of Annexin A2 within the context of ECM regulation. Furthermore, several extracellular associated cellular compartments were found to be significantly overrepresented within the “Collagen ONLY” list (Figure 3D). This was of great interest to our study due to our focus on the relationship between Annexin A2 and the extracellular environment, particularly the ECM. This result suggests that when cells are in contact with collagen-I, Annexin A2 is more likely to be involved in ECM binding, in particular fibronectin. This is consistent with our observations that in this state, Annexin A2 is phosphorylated at Tyr 24 and is also translocated to the extracellular membrane.
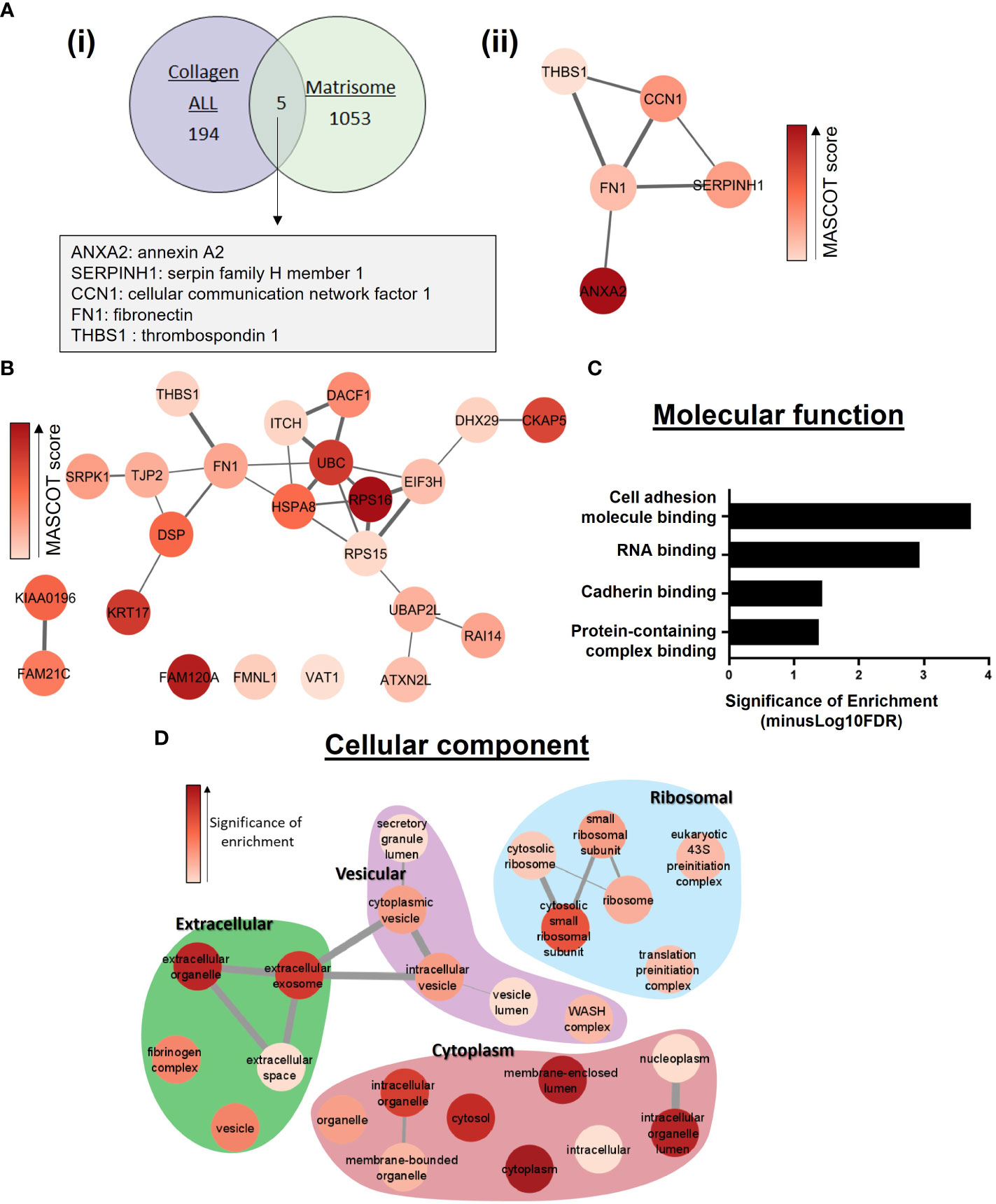
Figure 3 Functional enrichment analysis of proteins identified as Annexin A2 binding partners in the presence of collagen-I. (A) (i) Comparison of the overlap between identified proteins within “collagen-I ALL” list and constituents of the extracellular matrix, derived from the MatrisomeDB (63) (ii) Protein-protein interaction network of the 5 proteins identified in (i). Interactions were assessed with String (40) and visualized using the StringApp in Cytoscape set at 0.4 confidence level (41). Node coloured indicates MASCOT (MS/MS) score. Edge line thickness denotes the confidence of association between protein nodes. (B) Protein-protein interaction network of the 23 proteins identified in the “collagen-I only” list. Assessed as in (A) (ii), with 0.3 confidence level. (C) Functional enrichment analysis of Annexin A2 binding partners in “collagen-I ONLY” was carried out using the PANTHER overrepresentation test (43). Molecular Function overrepresentation test shows cell adhesion molecule binding to be the most significantly enriched term in the list of identified proteins. (D) Cellular Compartments overrepresentation test shows a wide range of significantly overrepresented GO terms, including several extracellular associated terms.
3.3 Reduction of Annexin A2 expression decreases the ability of breast cancer cells to degrade fibronectin and this ability is influenced by the presence of collagen-I
Given the results of our mass spectrometry analysis identifying fibronectin as a novel Annexin A2 binding partner of central importance (Figure 3B), we decided to further investigate the relationship between the two proteins. We first tested their binding directly by carrying out a fibronectin baited immunoprecipitation of Annexin A2, verifying the binding between the two proteins (Figure 4A; Supplementary Figure 4). Having confirmed the interaction between Annexin A2 and fibronectin, we next examined its functional consequence. It is reported that when Annexin A2 becomes phosphorylated and translocated to the extracellular surface, it acts as an activation platform for plasmin, a protease known to directly degrade ECM proteins including fibronectin (27, 66, 67). We hypothesized that Annexin A2 could regulate the degradation of fibronectin, as a known interactor of both plasminogen and its activators (27, 29), and a novel interactor of fibronectin. To test our hypothesis, we assessed the direct effect of Annexin A2 expression on the degradation of fibronectin using a modified gelatin degradation assay (68) and high-throughput immunofluorescent imaging. Cells were transfected with siANXA2 or Negative control siRNA and grown for 72 hours to allow the knockdown to take effect (Figure 4B). Cells were then plated on uncoated wells, fibronectin coated wells or on wells coated with various percentages of fibronectin and collagen-I combinations. Degradation of fibronectin was detected as a localized loss of fluorescence (as illustrated in Figure 4E; Supplementary Figure 5). To quantify this, Cell Profiler was used to identify cells within each image, and the mean fluorescence intensity of fibronectin staining per cell was then measured.
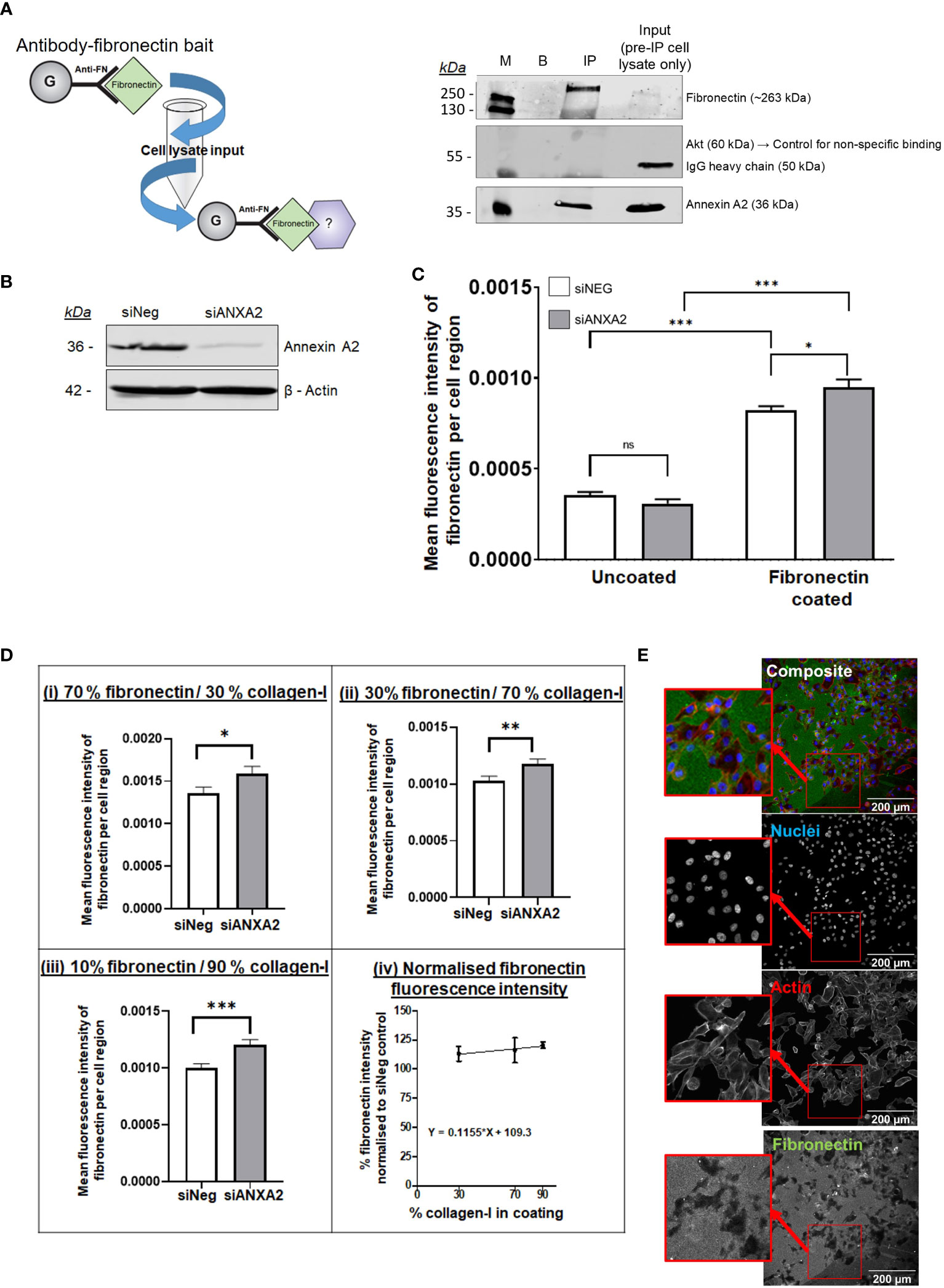
Figure 4 Annexin A2 is capable of binding to fibronectin and reduction in Annexin A2 expression reduces the ability of cells to degrade fibronectin, an ability that is further influenced by the presence of collagen-I. (A) Immunoprecipitation of Annexin A2 binding to Fibronectin. Pull-down bait of exogenous purified fibronectin was prepared and incubated with MDA-MB-231 cell lysate. Western blot demonstrating the binding between fibronectin and Annexin A2 using fibronectin immunoprecipitation in MDA-MB-231 lysate. M= Protein size marker, B= Blank/No Ab control, IP= Anti-FN coupled with purified fibronectin then cell lysate, input= MDA-MB-231 cell lysate used for IP before incubation with FN bait. (B) Representative blot showing efficacy of Annexin A2 siRNA knockdown. (C) 72 hours after transfection, cells were plated in wells coated with fibronectin and then incubated for 24 hours before being stained for Fibronectin. Fibronectin coating correlated with a significant increase in mean fluorescence intensity per cell. For cells on fibronectin coated plastic, KD of Annexin A2 resulted in increased fibronectin staining intensity, showing a decreased ability of cells to degrade fibronectin. (Students T test, *p < 0.05, ***p <0.0001, n =3) (D) For cells on defined mixtures of fibronectin and collagen-I coatings, KD of Annexin A2 resulted in increased fibronectin staining intensity, the difference between KD and siNeg control increased as the proportion of collagen-I was increased. (Mann-Whitney U, *p < 0.05, **p < 0.001, ***p < 0.0001, n =3) (E) Representative immunofluorescent images of fibronectin degradation quantification. ns, not significant.
From Figure 4C, we can see that coating the wells with fibronectin gives a large increase in the measured fluorescence compared to uncoated wells, giving us confidence that our approach was appropriate to quantify fibronectin abundance. Cells with reduced Annexin A2 expression had significantly lower ability to locally degrade fibronectin, as demonstrated by a marked increase in mean fluorescence within each cell area (Student’s T test, p = 0.0110). From this we can conclude that a reduction in Annexin A2 reduces the cells ability to degrade fibronectin, likely via Annexin A2’s known role in plasmin activation.
We next examined the effect of plating cells on defined mixtures of collagen-I (COL) and fibronectin (FN) to assess whether the presence of collagen-I could influence the Annexin A2 mediated digestion of fibronectin (Figure 4D). Using majority fibronectin coating of 70% FN/30%COL, we can see that at these proportions, the reduction of Annexin A2 expression causes a significant increase in measured fibronectin abundance (Mann-Whitney U test, p = 0.0276). This effect continues as the loss of Annexin A2 consistently reduces the average fluorescence of stained fibronectin for 30% and 10% fibronectin coating (Figure 4D (iv)). Remarkably, the significance of this difference actually increases when the coating mixture is predominantly collagen-I, suggesting a more dramatic effect with more collagen-I present. This can be seen in Figure 4D for 30%FN/70%COL (Mann- Whitney U, p = 0.0052) and for 10%FN/70%COL (Mann-Whitney U, p = 0.0006). To compare the result of ANXA2 knockdown across the different coating ratios, the fluorescence intensity of fibronectin in the ANXA2 knockdown condition was normalized relative to the fluorescence intensity of fibronectin in the negative control siRNA condition for each plate. As illustrated in Figure 4D (v), the relative % increase in fibronectin fluorescence intensity does increase slightly as the ratio of collagen-I within the coating increases, as measured by a positive value for the slope of the line. This suggests that Annexin A2 expression has a dominant effect on the cell’s ability to degrade fibronectin. When Annexin A2 is knocked down, the cells’ ability to degrade fibronectin does not increase with increase in collagen-I abundance. These results suggest the effect on the cells ability to degrade fibronectin is dependent on high expression of Annexin A2 rather than merely collagen-I abundance.
4 Discussion
In this study, we have shown that the post-translational modification, localization and functional interactions of Annexin A2 are strongly influenced by the ECM, in particular, collagen-I, the most abundant collagen type in the body (69, 70). We firstly observed that collagen-I stimulates the phosphorylation of Annexin A2 at Tyr 24 and, accordingly, also triggers the translocation of Annexin A2 to the extracellular membrane. High collagen-I conditions are pertinent in the study of breast cancer as collagen-I is often overexpressed and associated with poor clinical outcomes (2, 6, 36, 71). This post-translational modification at Tyr24 is of particular interest in malignancy due to the early discovery of phosphorylated Annexin A2 in transformed cells (72, 73) and more recent observations of increased Tyr24 phosphorylation levels in cancer (74). Furthermore, a number of studies have linked phosphorylation of Annexin A2 to the promotion of EMT and metastatic cell behaviors in a variety cancer types (21, 53, 54, 61, 75–78). Our observed link between Tyr24 and collagen-I is particularly interesting given the independent observations that both Tyr24 phosphorylation (21) and collagen-I (7) can increase cell migration. This suggests Annexin A2 may be integral in the pathways contributing to collagen-I associated malignancy and cell migration. Moreover, the use of a specific imaging marker targeted to phospho-ANXA2 on a variety of solid tumor types has shown Annexin A2 is highly phosphorylated in the TME, with particular localization at the invasive tumor front (56). In addition, this study showed both cancer cells and cancer associated fibroblasts express phosphorylated Annexin A2, whereas normal fibroblasts do not. Given the integral role of cancer associated fibroblasts in the production of a desmoplastic TME, and the aberrant remodeling of ECM proteins such as collagen-I and fibronectin, our observations that collagen triggers the phosphorylation of Annexin A2 provides potential explanation for the specific upregulation of phospho-Annexin A2 at Tyr 24 on tumor cells and within the TME seen in the study by Shen et al. (56). Furthermore, our evidence of a link between collagen abundance and the activation of this pro-metastatic modification of Annexin A2 goes some way to explaining our previous findings of Annexin A2’s integral role in breast cancer metastasis and the associated ECM dysregulation seen in the disease (33).
We next conducted a protein interactome analysis which yielded an extensive list of potential binding partners of Annexin A2. Knowing collagen-I triggers the phosphorylation and translocation of Annexin A2, we hypothesized that investigating Annexin A2 interactors under these conditions may provide insight into the functional consequences of this regulation. Of note within the list of proteins found bound to Annexin A2 only when cells are plated on collagen-I (Table S1) is Keratin 17, which was previously discovered to be a novel binding partner of Annexin A2 in a study by Chung et al. (79). This study found that when Keratin-17 expression is silenced, the phosphorylation of Annexin A2 at Tyr 24 is reduced, with the authors hypothesizing that keratin filaments act as a scaffold to regulate the subcellular location of Annexin and facilitate its phosphorylation. This may provide an explanation for our observation that Keratin-17 is bound to Annexin A2 only when our cells are plated on collagen-I as this is when we see an increase in Tyr24 phosphorylation. Once again, this agreement of our findings with the published literature provides strong validity for our approach and hypothesis that the collagen-I mediated regulation of Annexin A2 affects its interactome, with potential functional consequences.
Thus, in attempting to elucidate the effect of collagen-I on Annexin A2 function, we assessed the specific proteins found bound to Annexin A2 in this condition, particularly in terms of the ECM. This revealed a network of interacting proteins with fibronectin, an ECM constituent and glycoprotein, emerging as a central and important node. Furthermore, gene ontology enrichment analysis revealed cell adhesion molecule binding, also known as adhesive extracellular matrix constituents, to be the most significantly overrepresented function with our identified list, an ontology of which fibronectin is a member. Although not expressed in healthy adult breast tissue, fibronectin expression is increased in breast tumor stroma (80) and has been linked to the promotion of proliferation, angiogenesis and metastasis of breast cancer cells (81, 82). To our knowledge, the interaction between Annexin A2 and fibronectin has not been characterized. Using String (40) to search for published evidence of PPIs, one study was found that suggested Annexin A2 as a potential interactor due to co-precipitation with associated α5β1 integrin complexes (83). Furthermore, inhibition of Annexin A2 interactions using a specific targeted peptide reduces the adhesion of cells to fibronectin (84). Given the role of Annexin A2 in promoting breast cancer progression in vivo and in patients evidenced by us (33) and several others, coupled with our observation that proteins involved in cell adhesion to the ECM, including fibronectin, are enriched in our list of Annexin A2 binding partners, it is reasonable to hypothesize that the collagen–I regulation of Annexin A2 may influence its role in degradation and remodeling of the ECM.
A critical hallmark of malignancy is its capacity to spread and invade neighboring tissue. This metastatic ability is facilitated by the proteolytic cleavage of ECM proteins that make up physiological barriers like the basement membrane, upon intra- and extravasation (85–87). It is well evidenced that cell-surface Annexin A2 promotes the localized activation of plasmin to regulate vascular fibrinolysis and neoangiogenesis (32, 88–92). Plasmin, a serine protease, has been shown to degrade fibronectin both in vitro (93, 94) and in vivo (95, 96). Within the context of cancer, plasmin can proteolytically remodel the cancer associated ECM (including fibronectin), creating a path which enhances cancer cell escape from the primary site (26, 97, 98). Thus, to characterize the implications of Annexin A2 binding to fibronectin, we examined whether Annexin A2 expression specifically affects the degradation of fibronectin by breast cancer cells, something that has not been directly investigated before. Remarkably, we saw that knockdown of Annexin A2 did in fact impair the fibronectin degradation ability of MDA-MB-231 breast cancer cells. This is of critical relevance in breast cancer progression as fibronectin turnover has been shown to increase the metastatic capacity of tumor cells (99–101). Furthermore, the proteolytic cleavage of fibronectin by plasmin creates fibronectin degradation products that further enhance the migration and invasion of tumor cells, through their interaction with cell surface integrin receptors (97, 98). This suggests Annexin A2 has an integral role in the degradation of fibronectin by cancer cells and modulating Annexin A2 activity could be a viable therapeutic strategy, in the yet unsuccessful targeting of plasmin-mediated cancer cell dissemination (102, 103). Further investigation of this mechanism using known pharmacological inhibitors of Annexin A2 phosphorylation, such as Src targeting agents (61) or a combination of phospho-null or phospho-memetic expressing cells in 3-dimensional culture or in vivo models may provide insight into future clinical targeting potential.
Taken together, our work shows that the regulatory effect of collagen-I on the phosphorylation and subsequent localization of Annexin A2, and in enhancing Annexin A2’s pro-tumorigenic role in the degradation of fibronectin. Bearing in mind the association of collagen-I over-expression with migration, invasion, breast cancer metastasis and poor survival (36), we can hypothesize that collagen-I’s influence on Annexin A2 phosphorylation and the subsequent degradation of fibronectin, may be one of the many ways in which the ECM can promote cancer progression. Furthermore, these findings go some way to explain the molecular mechanisms and associated biological processes that Annexin A2 phosphorylation mediates in the TME, and how these are plausibly connected to aggressive disease and cancer metastasis, as seen in our previous work, and that of others (21, 31, 33, 35, 61).
5 Conclusions
Ultimately, we have shown that the phosphorylation, location and functionality of Annexin A2 in cancer is regulated by the TME. In addition, we have greatly increased our knowledge of the function of Annexin A2 in breast cancer cells, through interactome studies, contributing to the overall understanding of this protein. Our work demonstrates that Annexin A2 has a distinct role in mediating the dynamic reciprocity between the ECM and cancer cells. The expression, localization and interactions of Annexin A2 allow it to function as a bi-directional negotiator between the extracellular environment, the cancer cell and back out to the TME. Our findings suggest a mechanism for the poor clinical outcomes associated with a desmoplastic TME as we hypothesize that the high expression of collagen-I seen in desmoplasia can increase the invasive capacity of breast cancer cells via its regulation of Annexin A2. Further investigations with additional cancer cell lines, primary cancer cells and in vivo models are necessary to identify specific clinical disease manifestations in which Annexin A2 and collagen-I interactions influence the cancer phenotype and could be targeted in the prevention of cancer progression. Our previous findings showing the association of Annexin A2 expression with metastatic progression, combined with our new observations of how Annexin A2’s role is heavily influenced by the ECM points to the interaction between Annexin A2 and collagen-I as a target of interest in cancer progression. Future studies to unpick the precise molecular mechanism and highlight targetable nodes within this pathway are needed to translate these findings to breast cancer patients. Given the major role of ECM degradation in cell migration and invasion, this is an obvious avenue of investigation for anti-cancer therapies. However, due to the poor results to date from clinical trials of proteolysis inhibitors such as Batimastat, Amiloride and Upamostat (102–104), it is clear a new and perhaps indirect, strategy is needed in preventing cancer metastasis. Thus, we suggest the targeting of Annexin A2 to prevent its translocation or protein interactions may be an approach to halting the ECM and TME mediated progression of breast cancer.
Data availability statement
The proteomics data presented in the study are deposited in the MassIVE ProteoSAFe repository, accession number MSV000093084, available at: https://doi.org/doi:10.25345/C50R9MF2R. Further inquiries can be directed to the corresponding authors.
Ethics statement
Ethical approval was not required for the studies on humans as only commercially available established cell lines were used and publicly available data from anonymised online databases was used. No animal subjects were used in this study.
Author contributions
AM: Conceptualization, Formal analysis, Investigation, Methodology, Visualization, Writing – original draft. JN: Formal analysis, Visualization, Writing – review & editing. ROC: Formal analysis, Visualization, Writing – review & editing. AL: Conceptualization, Supervision, Writing – review & editing. JA: Project administration, Writing – review & editing. PK: Conceptualization, Funding acquisition, Supervision, Writing – original draft. KM: Conceptualization, Formal analysis, Funding acquisition, Investigation, Methodology, Supervision, Visualization, Writing – original draft.
Funding
The author(s) declare financial support was received for the research, authorship, and/or publication of this article. This work was supported by grants received from the Irish Research Council, Grant GOIPG/2016/1547 (to AM), the Mid-Western Cancer Foundation (to PK) and Science Foundation Ireland Infrastructure Programme; Opportunity Fund (to KM).
Acknowledgments
We are grateful to our colleagues in the Laboratory of Cellular and Molecular Biology for helpful discussions and critical review.
Conflict of interest
The authors declare that the research was conducted in the absence of any commercial or financial relationships that could be construed as a potential conflict of interest.
Publisher’s note
All claims expressed in this article are solely those of the authors and do not necessarily represent those of their affiliated organizations, or those of the publisher, the editors and the reviewers. Any product that may be evaluated in this article, or claim that may be made by its manufacturer, is not guaranteed or endorsed by the publisher.
Supplementary material
The Supplementary Material for this article can be found online at: https://www.frontiersin.org/articles/10.3389/fonc.2023.1270436/full#supplementary-material
References
1. Xu R, Boudreau A, Bissell MJ. Tissue architecture and function: dynamic reciprocity via extra- and intra-cellular matrices. Cancer Metastasis Rev (2009) 28(1-2):167–76. doi: 10.1007/s10555-008-9178-z
2. Lu P, Weaver VM, Werb Z. The extracellular matrix: a dynamic niche in cancer progression. J Cell Biol (2012) 196(4):395–406. doi: 10.1083/jcb.201102147
3. Kim J, Tanner K. Recapitulating the tumor ecosystem along the metastatic cascade using 3D culture models. Front Oncol (2015) 5:170. doi: 10.3389/fonc.2015.00170
4. Walker RA. The complexities of breast cancer desmoplasia. Breast Cancer Res (2001) 3(3):143–5. doi: 10.1186/bcr287
5. Sasser AK, Hall BM. Desmoplasia. In: Schwab M, editor. Encyclopedia of Cancer. Berlin, Heidelberg: Springer Berlin Heidelberg (2014). p. 1344–7.
6. Kauppila S, Stenback F, Risteli J, Jukkola A, Risteli L. Aberrant type I and type III collagen gene expression in human breast cancer in vivo. J Pathol (1998) 186(3):262–8. doi: 10.1002/(SICI)1096-9896(1998110)186:3<262::AID-PATH191>3.0.CO;2-3
7. Nolan J, Mahdi AF, Dunne CP, Kiely PA. Collagen and fibronectin promote an aggressive cancer phenotype in breast cancer cells but drive autonomous gene expression patterns. Gene (2020) 761:145024. doi: 10.1016/j.gene.2020.145024
8. Provenzano PP, Inman DR, Eliceiri KW, Keely PJ. Matrix density-induced mechanoregulation of breast cell phenotype, signaling and gene expression through a FAK-ERK linkage. Oncogene (2009) 28(49):4326–43. doi: 10.1038/onc.2009.299
9. Lyons TR, O’Brien J, Borges VF, Conklin MW, Keely PJ, Eliceiri KW, et al. Postpartum mammary gland involution drives progression of ductal carcinoma in situ through collagen and COX-2. Nat Med (2011) 17(9):1109–15. doi: 10.1038/nm.2416
10. Kakkad SM, Solaiyappan M, Argani P, Sukumar S, Jacobs LK, Leibfritz D, et al. Collagen I fiber density increases in lymph node positive breast cancers: pilot study. J BioMed Opt (2012) 17(11):116017. doi: 10.1117/1.JBO.17.11.116017
11. Provenzano PP, Inman DR, Eliceiri KW, Knittel JG, Yan L, Rueden CT, et al. Collagen density promotes mammary tumor initiation and progression. BMC Med (2008) 6:11. doi: 10.1186/1741-7015-6-11
12. Li T, Sun L, Miller N, Nicklee T, Woo J, Hulse-Smith L, et al. The association of measured breast tissue characteristics with mammographic density and other risk factors for breast cancer. Cancer Epidemiol Biomarkers Prev (2005) 14(2):343–9. doi: 10.1158/1055-9965.EPI-04-0490
13. Boyd NF, Martin LJ, Yaffe MJ, Minkin S. Mammographic density and breast cancer risk: current understanding and future prospects. Breast Cancer Res (2011) 13(6):223. doi: 10.1186/bcr2942
14. Ursin G, Hovanessian-Larsen L, Parisky YR, Pike MC, Wu AH. Greatly increased occurrence of breast cancers in areas of mammographically dense tissue. Breast Cancer Res (2005) 7(5):R605–8. doi: 10.1186/bcr1260
15. McCormack VA, dos Santos Silva I. Breast density and parenchymal patterns as markers of breast cancer risk: a meta-analysis. Cancer Epidemiol Biomarkers Prev (2006) 15(6):1159–69. doi: 10.1158/1055-9965.EPI-06-0034
16. Maskarinec G, Pagano IS, Little MA, Conroy SM, Park SY, Kolonel LN. Mammographic density as a predictor of breast cancer survival: the Multiethnic Cohort. Breast Cancer Res (2013) 15(1):R7. doi: 10.1186/bcr3378
17. Huo CW, Waltham M, Khoo C, Fox SB, Hill P, Chen S, et al. Mammographically dense human breast tissue stimulates MCF10DCIS.com progression to invasive lesions and metastasis. Breast Cancer Res (2016) 18(1):1–13. doi: 10.1186/s13058-016-0767-4
18. Deora AB, Kreitzer G, Jacovina AT, Hajjar KA. An annexin 2 phosphorylation switch mediates p11-dependent translocation of annexin 2 to the cell surface. J Biol Chem (2004) 279(42):43411–8. doi: 10.1074/jbc.M408078200
19. Wang T, Wang Z, Niu R, Wang L. Crucial role of Anxa2 in cancer progression: highlights on its novel regulatory mechanism. Cancer Biol Med (2019) 16(4):671–87. doi: 10.20892/j.issn.2095-3941.2019.0228
20. Valapala M, Vishwanatha JK. Lipid raft endocytosis and exosomal transport facilitate extracellular trafficking of annexin A2. J Biol Chem (2011) 286(35):30911–25. doi: 10.1074/jbc.M111.271155
21. Zheng L, Foley K, Huang L, Leubner A, Mo G, Olino K, et al. Tyrosine 23 phosphorylation-dependent cell-surface localization of annexin A2 is required for invasion and metastases of pancreatic cancer. PloS One (2011) 6(4):e19390. doi: 10.1371/journal.pone.0019390
22. Valapala M, Maji S, Borejdo J, Vishwanatha JK. Cell surface translocation of annexin A2 facilitates glutamate-induced extracellular proteolysis. J Biol Chem (2014) 289(23):15915–26. doi: 10.1074/jbc.M113.511550
23. Grindheim AK, Saraste J, Vedeler A. Protein phosphorylation and its role in the regulation of Annexin A2 function. Biochim Biophys Acta Gen Subj (2017) 1861(11 Pt A):2515–29. doi: 10.1016/j.bbagen.2017.08.024
24. He KL, Sui G, Xiong H, Broekman MJ, Huang B, Marcus AJ, et al. Feedback regulation of endothelial cell surface plasmin generation by PKC-dependent phosphorylation of annexin A2. J Biol Chem (2011) 286(17):15428–39. doi: 10.1074/jbc.M110.185058
25. Yang W, Mei FC, Cheng X. EPAC1 regulates endothelial annexin A2 cell surface translocation and plasminogen activation. FASEB J (2018) 32(4):2212–22. doi: 10.1096/fj.201701027R
26. Deryugina EI, Quigley JP. Cell surface remodeling by plasmin: a new function for an old enzyme. J BioMed Biotechnol (2012) 2012:564259. doi: 10.1155/2012/564259
27. Valls MD, Soldado M, Arasa J, Perez-Aso M, Williams AJ, Cronstein BN, et al. Annexin A2-mediated plasminogen activation in endothelial cells contributes to the proangiogenic effect of adenosine A2A receptors. Front Pharmacol (2021) 12. doi: 10.3389/fphar.2021.654104
28. Ling Q, Jacovina AT, Deora A, Febbraio M, Simantov R, Silverstein RL, et al. Annexin II regulates fibrin homeostasis and neoangiogenesis in vivo. J Clin Invest (2004) 113(1):38–48. doi: 10.1172/JCI19684
29. Liu W, Hajjar KA. The annexin A2 system and angiogenesis. Biol Chem (2016) 397(10). doi: 10.1515/hsz-2016-0166
30. Gouri A, Dekaken A, El Bairi K, Aissaoui A, Laabed N, Chefrour M, et al. Plasminogen activator system and breast cancer: potential role in therapy decision making and precision medicine. biomark Insights (2016) 11:105–11. doi: 10.4137/BMI.S33372
31. Sharma MR, Koltowski L, Ownbey RT, Tuszynski GP, Sharma MC. Angiogenesis-associated protein annexin II in breast cancer: selective expression in invasive breast cancer and contribution to tumor invasion and progression. Exp Mol Pathol (2006) 81(2):146–56. doi: 10.1016/j.yexmp.2006.03.003
32. Sharma M, Ownbey RT, Sharma MC. Breast cancer cell surface annexin II induces cell migration and neoangiogenesis via tPA dependent plasmin generation. Exp Mol Pathol (2010) 88(2):278–86. doi: 10.1016/j.yexmp.2010.01.001
33. Mahdi AF, Malacrida B, Nolan J, McCumiskey ME, Merrigan AB, Lal A, et al. Expression of annexin A2 promotes cancer progression in estrogen receptor negative breast cancers. Cells (2020) 9(7):1582. doi: 10.3390/cells9071582
34. Christensen M, Høgdall CK, Jochumsen KM, Høgdall EVS. Annexin A2 and cancer: A systematic review. Int J Oncol (2017) 52(1):5–18. doi: 10.3892/ijo.2017.4197
35. Sharma MC. Annexin A2 (ANX A2): An emerging biomarker and potential therapeutic target for aggressive cancers. Int J Cancer (2019) 144(9):2074–81. doi: 10.1002/ijc.31817
36. Xu S, Xu H, Wang W, Li S, Li H, Li T, et al. The role of collagen in cancer: from bench to bedside. J Transl Med (2019) 17(1):309. doi: 10.1186/s12967-019-2058-1
37. Bourgot I, Primac I, Louis T, Noël A, Maquoi E. Reciprocal interplay between fibrillar collagens and collagen-binding integrins: implications in cancer progression and metastasis. Front Oncol (2020) 10. doi: 10.3389/fonc.2020.01488
38. Kaushik S, Pickup MW, Weaver VM. From transformation to metastasis: deconstructing the extracellular matrix in breast cancer. Cancer Metastasis Rev (2016) 35(4):655–67. doi: 10.1007/s10555-016-9650-0
39. Mai J, Finley RL, Waisman DM, Sloane BF. Human procathepsin B interacts with the annexin II tetramer on the surface of tumor cells. J Biol Chem (2000) 275(17):12806–12. doi: 10.1074/jbc.275.17.12806
40. Szklarczyk D, Gable AL, Lyon D, Junge A, Wyder S, Huerta-Cepas J, et al. STRING v11: protein-protein association networks with increased coverage, supporting functional discovery in genome-wide experimental datasets. Nucleic Acids Res (2019) 47(D1):D607–D13. doi: 10.1093/nar/gky1131
41. Doncheva NT, Morris JH, Gorodkin J, Jensen LJ. Cytoscape StringApp: network analysis and visualization of proteomics data. J Proteome Res (2019) 18(2):623–32. doi: 10.1021/acs.jproteome.8b00702
42. Scardoni G, Petterlini M, Laudanna C. Analyzing biological network parameters with CentiScaPe. Bioinformatics (2009) 25(21):2857–9. doi: 10.1093/bioinformatics/btp517
43. Mi H, Muruganujan A, Ebert D, Huang X, Thomas PD. PANTHER version 14: more genomes, a new PANTHER GO-slim and improvements in enrichment analysis tools. Nucleic Acids Res (2019) 47(D1):D419–D26. doi: 10.1093/nar/gky1038
44. Supek F, Bosnjak M, Skunca N, Smuc T. REVIGO summarizes and visualizes long lists of gene ontology terms. PloS One (2011) 6(7):e21800. doi: 10.1371/journal.pone.0021800
45. McQuin C, Goodman A, Chernyshev V, Kamentsky L, Cimini BA, Karhohs KW, et al. CellProfiler 3.0: Next-generation image processing for biology. PloS Biol (2018) 16(7):e2005970. doi: 10.1371/journal.pbio.2005970
46. Cerami E, Gao J, Dogrusoz U, Gross BE, Sumer SO, Aksoy BA, et al. The cBio cancer genomics portal: an open platform for exploring multidimensional cancer genomics data. Cancer Discovery (2012) 2(5):401–4. doi: 10.1158/2159-8290.CD-12-0095
47. Hoadley KA, Yau C, Hinoue T, Wolf DM, Lazar AJ, Drill E, et al. Cell-of-origin patterns dominate the molecular classification of 10,000 tumors from 33 types of cancer. Cell (2018) 173(2):291–304.e6. doi: 10.1016/j.cell.2018.03.022
48. Henriksen K, Karsdal MA, Karsdal MA. Chapter 1 - type I collagen. In: Biochemistry of Collagens, Laminins and Elastin (Second Edition). Academic Press (2016). p. 1–11.
49. He KL, Deora AB, Xiong H, Ling Q, Weksler BB, Niesvizky R, et al. Endothelial cell annexin A2 regulates polyubiquitination and degradation of its binding partner S100A10/p11. J Biol Chem (2008) 283(28):19192–200. doi: 10.1074/jbc.M800100200
50. Madureira PA, O’Connell PA, Surette AP, Miller VA, Waisman DM. The biochemistry and regulation of S100A10: a multifunctional plasminogen receptor involved in oncogenesis. J BioMed Biotechnol (2012) 2012:353687. doi: 10.1155/2012/353687
51. Bharadwaj A, Bydoun M, Holloway R, Waisman D. Annexin A2 heterotetramer: structure and function. Int J Mol Sci (2013) 14(3):6259–305. doi: 10.3390/ijms14036259
52. Gao J, Aksoy BA, Dogrusoz U, Dresdner G, Gross B, Sumer SO, et al. Integrative analysis of complex cancer genomics and clinical profiles using the cBioPortal. Sci Signal (2013) 6(269):pl1. doi: 10.1126/scisignal.2004088
53. de Graauw M, Tijdens I, Smeets MB, Hensbergen PJ, Deelder AM, van de Water B. Annexin A2 phosphorylation mediates cell scattering and branching morphogenesis via cofilin Activation. Mol Cell Biol (2008) 28(3):1029–40. doi: 10.1128/MCB.01247-07
54. Rescher U, Ludwig C, Konietzko V, Kharitonenkov A, Gerke V. Tyrosine phosphorylation of annexin A2 regulates Rho-mediated actin rearrangement and cell adhesion. J Cell Sci (2008) 121(Pt 13):2177–85. doi: 10.1242/jcs.028415
55. Yuan J, Yang Y, Gao Z, Wang Z, Ji W, Song W, et al. Tyr23 phosphorylation of Anxa2 enhances STAT3 activation and promotes proliferation and invasion of breast cancer cells. Breast Cancer Res Treat (2017) 164(2):327–40. doi: 10.1007/s10549-017-4271-z
56. Shen D, Xu B, Liang K, Tang R, Sudlow GP, Egbulefu C, et al. Selective imaging of solid tumours via the calcium-dependent high-affinity binding of a cyclic octapeptide to phosphorylated Annexin A2. Nat BioMed Eng (2020) 4(3):298–313. doi: 10.1038/s41551-020-0528-7
57. Hayes S, Malacrida B, Kiely M, Kiely PA. Studying protein-protein interactions: progress, pitfalls and solutions. Biochem Soc Trans (2016) 44(4):994–1004. doi: 10.1042/BST20160092
58. Ziemann M, Eren Y, El-Osta A. Gene name errors are widespread in the scientific literature. Genome Biol (2016) 17(1):177. doi: 10.1186/s13059-016-1044-7
59. Benaud C, Gentil BJ, Assard N, Court M, Garin J, Delphin C, et al. AHNAK interaction with the annexin 2/S100A10 complex regulates cell membrane cytoarchitecture. J Cell Biol (2004) 164(1):133–44. doi: 10.1083/jcb.200307098
60. Jin J, Bhatti DL, Lee KW, Medrihan L, Cheng J, Wei J, et al. Ahnak scaffolds p11/Anxa2 complex and L-type voltage-gated calcium channel and modulates depressive behavior. Mol Psychiatry (2020) 25(5):1035–49. doi: 10.1038/s41380-019-0371-y
61. Fan Y, Si W, Ji W, Wang Z, Gao Z, Tian R, et al. Rack1 mediates tyrosine phosphorylation of Anxa2 by Src and promotes invasion and metastasis in drug-resistant breast cancer cells. Breast Cancer Res (2019) 21(1):66. doi: 10.1186/s13058-019-1147-7
62. Yang Y, Wu N, Wang Z, Zhang F, Tian R, Ji W, et al. Rack1 mediates the interaction of P-glycoprotein with Anxa2 and regulates migration and invasion of multidrug-resistant breast cancer cells. Int J Mol Sci (2016) 17(10):1718. doi: 10.3390/ijms17101718
63. Naba A, Clauser KR, Ding H, Whittaker CA, Carr SA, Hynes RO. The extracellular matrix: Tools and insights for the “omics” era. Matrix Biol (2016) 49:10–24. doi: 10.1016/j.matbio.2015.06.003
64. Scardoni G, Tosadori G, Faizan M, Spoto F, Fabbri F, Laudanna C. Biological network analysis with CentiScaPe: centralities and experimental dataset integration. F1000Research (2015) 3:139. doi: 10.12688/f1000research.4477.2
65. Huntley RP, Sawford T, Mutowo-Meullenet P, Shypitsyna A, Bonilla C, Martin MJ, et al. The GOA database: gene Ontology annotation updates for 2015. Nucleic Acids Res (2015) 43(Database issue):D1057–63. doi: 10.1093/nar/gku1113
66. Lokman NA, Ween MP, Oehler MK, Ricciardelli C. The role of annexin A2 in tumorigenesis and cancer progression. Cancer Microenviron (2011) 4(2):199–208. doi: 10.1007/s12307-011-0064-9
67. Kumari S, Malla R. New insight on the role of plasminogen receptor in cancer progression. Cancer Growth Metastasis (2015) 8:35–42. doi: 10.4137/CGM.S27335
68. Martin KH, Hayes KE, Walk EL, Ammer AG, Markwell SM, Weed SA. Quantitative measurement of invadopodia-mediated extracellular matrix proteolysis in single and multicellular contexts. J Vis Exp (2012) 2012(66):e4119. doi: 10.3791/4119
69. Di Lullo GA, Sweeney SM, Korkko J, Ala-Kokko L, San Antonio JD. Mapping the ligand-binding sites and disease-associated mutations on the most abundant protein in the human, type I collagen. J Biol Chem (2002) 277(6):4223–31. doi: 10.1074/jbc.M110709200
70. Sorushanova A, Delgado LM, Wu Z, Shologu N, Kshirsagar A, Raghunath R, et al. The collagen suprafamily: from biosynthesis to advanced biomaterial development. Adv Mater (2019) 31(1):e1801651. doi: 10.1002/adma.201801651
71. Liu J, Shen JX, Wu HT, Li XL, Wen XF, Du CW, et al. Collagen 1A1 (COL1A1) promotes metastasis of breast cancer and is a potential therapeutic target. Discovery Med (2018) 25(139):211–23.
72. Erikson E, Erikson RL. Identification of a cellular protein substrate phosphorylated by the avian sarcoma virus-transforming gene product. Cell (1980) 21(3):829–36. doi: 10.1016/0092-8674(80)90446-8
73. Radke K, Gilmore T, Martin GS. Transformation by Rous sarcoma virus: a cellular substrate for transformation-specific protein phosphorylation contains phosphotyrosine. Cell (1980) 21(3):821–8. doi: 10.1016/0092-8674(80)90445-6
74. Mohammad HS, Kurokohchi K, Yoneyama H, Tokuda M, Morishita A, Jian G, et al. Annexin A2 expression and phosphorylation are up-regulated in hepatocellular carcinoma. Int J Oncol (2008) 33(6):1157–63. doi: 10.3892/ijo_00000105
75. Wang T, Yuan J, Zhang J, Tian R, Ji W, Zhou Y, et al. Anxa2 binds to STAT3 and promotes epithelial to mesenchymal transition in breast cancer cells. Oncotarget (2015) 6(31):30975–92. doi: 10.18632/oncotarget.5199
76. Cui L, Song J, Wu L, Cheng L, Chen A, Wang Y, et al. Role of Annexin A2 in the EGF-induced epithelial-mesenchymal transition in human CaSki cells. Oncol Lett (2017) 13(1):377–83. doi: 10.3892/ol.2016.5406
77. Rocha MR, Barcellos-de-Souza P, Sousa-Squiavinato ACM, Fernandes PV, de Oliveira IM, Boroni M, et al. Annexin A2 overexpression associates with colorectal cancer invasiveness and TGF-ss induced epithelial mesenchymal transition via Src/ANXA2/STAT3. Sci Rep (2018) 8(1):11285. doi: 10.1038/s41598-018-29703-0
78. Wu M, Sun Y, Xu F, Liang Y, Liu H, Yi Y. Annexin A2 Silencing Inhibits Proliferation and Epithelial-to-mesenchymal Transition through p53-Dependent Pathway in NSCLCs. J Cancer (2019) 10(5):1077–85. doi: 10.7150/jca.29440
79. Chung BM, Murray CI, Van Eyk JE, Coulombe PA. Identification of novel interaction between annexin A2 and keratin 17: evidence for reciprocal regulation. J Biol Chem (2012) 287(10):7573–81. doi: 10.1074/jbc.M111.301549
80. Ioachim E, Charchanti A, Briasoulis E, Karavasilis V, Tsanou H, Arvanitis DL, et al. Immunohistochemical expression of extracellular matrix components tenascin, fibronectin, collagen type IV and laminin in breast cancer: their prognostic value and role in tumour invasion and progression. Eur J Cancer (2002) 38(18):2362–70. doi: 10.1016/S0959-8049(02)00210-1
81. Chen Y, Chen L, Hong D, Chen Z, Zhang J, Fu L, et al. Baicalein inhibits fibronectin-induced epithelial-mesenchymal transition by decreasing activation and upregulation of calpain-2. Cell Death Dis (2019) 10(5):341. doi: 10.1038/s41419-019-1572-7
82. Lin TC, Yang CH, Cheng LH, Chang WT, Lin YR, Cheng HC. Fibronectin in cancer: friend or foe. Cells (2019) 9(1):27. doi: 10.3390/cells9010027
83. Humphries JD, Byron A, Bass MD, Craig SE, Pinney JW, Knight D, et al. Proteomic analysis of integrin-associated complexes identifies RCC2 as a dual regulator of Rac1 and Arf6. Sci Signal (2009) 2(87):ra51. doi: 10.1126/scisignal.2000396
84. Staquicini DI, Rangel R, Guzman-Rojas L, Staquicini FI, Dobroff AS, Tarleton CA, et al. Intracellular targeting of annexin A2 inhibits tumor cell adhesion, migration, and in vivo grafting. Sci Rep (2017) 7(1):4243. doi: 10.1038/s41598-017-03470-w
85. Ludwig T. Local proteolytic activity in tumor cell invasion and metastasis. Bioessays (2005) 27(11):1181–91. doi: 10.1002/bies.20306
86. Duffy MJ, McGowan PM, Gallagher WM. Cancer invasion and metastasis: changing views. J Pathol (2008) 214(3):283–93. doi: 10.1002/path.2282
87. Yilmaz M, Christofori G. Mechanisms of motility in metastasizing cells. Mol Cancer Res (2010) 8(5):629–42. doi: 10.1158/1541-7786.MCR-10-0139
88. Brownstein C, Deora AB, Jacovina AT, Weintraub R, Gertler M, Khan KM, et al. Annexin II mediates plasminogen-dependent matrix invasion by human monocytes: enhanced expression by macrophages. Blood (2004) 103(1):317–24. doi: 10.1182/blood-2003-04-1304
89. Diaz VM, Hurtado M, Thomson TM, Reventos J, Paciucci R. Specific interaction of tissue-type plasminogen activator (t-PA) with annexin II on the membrane of pancreatic cancer cells activates plasminogen and promotes invasion in vitro. Gut (2004) 53(7):993–1000. doi: 10.1136/gut.2003.026831
90. Sharma M, Blackman MR, Sharma MC. Antibody-directed neutralization of annexin II (ANX II) inhibits neoangiogenesis and human breast tumor growth in a xenograft model. Exp Mol Pathol (2012) 92(1):175–84. doi: 10.1016/j.yexmp.2011.10.003
91. Sharma MC, Jain D. Important role of annexin A2 (ANXA2) in new blood vessel development in vivo and human triple negative breast cancer (TNBC) growth. Exp Mol Pathol (2020) 116:104523. doi: 10.1016/j.yexmp.2020.104523
92. Sharma MC, Tuszynski GP, Blackman MR, Sharma M. Long-term efficacy and downstream mechanism of anti-annexinA2 monoclonal antibody (anti-ANX A2 mAb) in a pre-clinical model of aggressive human breast cancer. Cancer Lett (2016) 373(1):27–35. doi: 10.1016/j.canlet.2016.01.013
93. Liotta LA, Goldfarb RH, Brundage R, Siegal GP, Terranova V, Garbisa S. Effect of plasminogen activator (urokinase), plasmin, and thrombin on glycoprotein and collagenous components of basement membrane. Cancer Res (1981) 41(11 Pt 1):4629–36.
94. Horowitz JC, Rogers DS, Simon RH, Sisson TH, Thannickal VJ. Plasminogen activation induced pericellular fibronectin proteolysis promotes fibroblast apoptosis. Am J Respir Cell Mol Biol (2008) 38(1):78–87. doi: 10.1165/rcmb.2007-0174OC
95. Selvarajan S, Lund LR, Takeuchi T, Craik CS, Werb Z. A plasma kallikrein-dependent plasminogen cascade required for adipocyte differentiation. Nat Cell Biol (2001) 3(3):267–75. doi: 10.1038/35060059
96. Tjwa M, Moura R, Moons L, Plaisance S, De Mol M, Jansen S, et al. Fibrinolysis-independent role of plasmin and its activators in the haematopoietic recovery after myeloablation. J Cell Mol Med (2009) 13(11-12):4587–95. doi: 10.1111/j.1582-4934.2008.00521.x
97. Bekes EM, Deryugina EI, Kupriyanova TA, Zajac E, Botkjaer KA, Andreasen PA, et al. Activation of pro-uPA is critical for initial escape from the primary tumor and hematogenous dissemination of human carcinoma cells. Neoplasia (2011) 13(9):806–21. doi: 10.1593/neo.11704
98. Grither WR, Divine LM, Meller EH, Wilke DJ, Desai RA, Loza AJ, et al. TWIST1 induces expression of discoidin domain receptor 2 to promote ovarian cancer metastasis. Oncogene (2018) 37(13):1714–29. doi: 10.1038/s41388-017-0043-9
99. Hunter MC, O’Hagan KL, Kenyon A, Dhanani KC, Prinsloo E, Edkins AL. Hsp90 binds directly to fibronectin (FN) and inhibition reduces the extracellular fibronectin matrix in breast cancer cells. PloS One (2014) 9(1):e86842. doi: 10.1371/journal.pone.0086842
100. Attieh Y, Clark AG, Grass C, Richon S, Pocard M, Mariani P, et al. Cancer-associated fibroblasts lead tumor invasion through integrin-beta3-dependent fibronectin assembly. J Cell Biol (2017) 216(11):3509–20. doi: 10.1083/jcb.201702033
101. Gopal S, Veracini L, Grall D, Butori C, Schaub S, Audebert S, et al. Fibronectin-guided migration of carcinoma collectives. Nat Commun (2017) 8:14105. doi: 10.1038/ncomms14105
102. Wyganowska-Swiatkowska M, Tarnowski M, Murtagh D, Skrzypczak-Jankun E, Jankun J. Proteolysis is the most fundamental property of Malignancy and its inhibition may be used therapeutically (Review). Int J Mol Med (2019) 43(1):15–25. doi: 10.3892/ijmm.2018.3983
103. Lin H, Xu L, Yu S, Hong W, Huang M, Xu P. Therapeutics targeting the fibrinolytic system. Exp Mol Med (2020) 52(3):367–79. doi: 10.1038/s12276-020-0397-x
Keywords: breast cancer, Annexin A2, metastasis, collagen-I, extracellular matrix, tumor microenvironment
Citation: Mahdi AF, Nolan J, O’Connor RÍ, Lowery AJ, Allardyce JM, Kiely PA and McGourty K (2023) Collagen-I influences the post-translational regulation, binding partners and role of Annexin A2 in breast cancer progression. Front. Oncol. 13:1270436. doi: 10.3389/fonc.2023.1270436
Received: 01 August 2023; Accepted: 11 October 2023;
Published: 24 October 2023.
Edited by:
Jixin Dong, University of Nebraska Medical Center, United StatesReviewed by:
Jianmin Zhang, University at Buffalo, United StatesJerome Devy, Université de Reims Champagne-Ardenne, France
Copyright © 2023 Mahdi, Nolan, O’Connor, Lowery, Allardyce, Kiely and McGourty. This is an open-access article distributed under the terms of the Creative Commons Attribution License (CC BY). The use, distribution or reproduction in other forums is permitted, provided the original author(s) and the copyright owner(s) are credited and that the original publication in this journal is cited, in accordance with accepted academic practice. No use, distribution or reproduction is permitted which does not comply with these terms.
*Correspondence: Amira F. Mahdi, YW1pcmEubWFoZGlAdWwuaWU=; Kieran McGourty, a2llcmFuLm1jZ291cnR5QHVsLmll