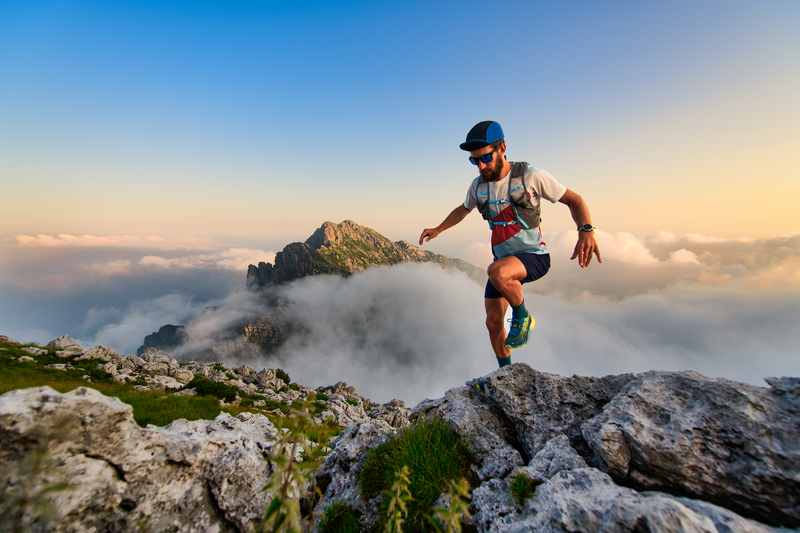
95% of researchers rate our articles as excellent or good
Learn more about the work of our research integrity team to safeguard the quality of each article we publish.
Find out more
REVIEW article
Front. Oncol. , 07 November 2023
Sec. Cancer Molecular Targets and Therapeutics
Volume 13 - 2023 | https://doi.org/10.3389/fonc.2023.1257985
Epithelial-mesenchymal transition (EMT) is a cellular reprogramming process that converts epithelial cells into mesenchymal-like cells with migratory and invasive capabilities. The initiation and regulation of EMT is closely linked to a range of transcription factors, cell adhesion molecules and signaling pathways, which play a key role in cancer metastasis and drug resistance. The regulation of ferroptosis is intricately linked to various cell death pathways, intracellular iron homeostasis, and the protein network governing iron supply and storage. The ability of ferroptosis to disrupt cancer cells and overcome drug resistance lies in its control of intracellular iron ion levels. EMT process can promote the accumulation of iron ions, providing conditions for ferroptosis. Conversely, ferroptosis may impact the regulatory network of EMT by modulating transcription factors, signaling pathways, and cell adhesion molecules. Thus, ferroptosis related genes and signaling pathways and oxidative homeostasis play important roles in the regulation of EMT. In this paper, we review the role of ferroptosis related genes and their signaling pathways in regulating cancer EMT to better understand the crosstalk mechanism between ferroptosis and EMT, aiming to provide better therapeutic strategies for eradicating cancer cells and overcoming drug resistance.
Recently, the incidence of cancer has increased dramatically, making it the second most common cause of death in the world. EMT (EMT) is a major cause of cancer metastasis and characterized by proliferation, division, invasion and migration. Therefore, there is a need for an effective EMT treatment strategy, which can be broadly classified into surgical and nonsurgical approaches (1). Most conventional nonsurgical treatments are closely related to apoptosis, particularly those associated with the cystine aspartate protease (caspase) family (2). However, the cancer cells exhibit apoptosis resistance due to the negative effects of over-expression of protein and the escape of apoptosis due to the mutation of apoptosis and multiple drug resistance, and resulting in loss of therapeutic efficacy of conventional chemotherapy (3). Thus, there is an urgent need to develop new anticancer tools to overcome these limitations and improve cancer outcomes.
In 2012, a novel programmed cell death form distinct from necrosis and apoptosis, called ferroptosis, was discovered (4). Ferroptosis is a complicated biological process, which involves the disruption of iron metabolism and the accumulation of ROS (ROS). It can be controlled through co-regulatory mechanisms and specific signaling pathways, where cell-to-cell contact plays a crucial role in ferroptosis regulation (5). Recent research indicates that ROS, DNA damage and metabolic reprogramming are needed to activate lipid peroxidation and ferroptosis (6). The main reasons for ferroptosis are the abnormal increase of iron-dependent lipid free radicals and the imbalance of oxidation-reduction homeostasis (7). The oxidation-reduction basis of ferroptosis is primarily achieved through the inactivation of cellular antioxidant defense systems, Among them are cystine/glutamate reverse transport system Xc−, glutathione (GSH), glutathione peroxidase 4 (GPX4), and FSP1 (8). system Xc−, which is a protein carrier for small molecule in ferroptosis, is made up of the regulatory subunit solute carrier family 3 member 2 (SLC3A2) and the catalytic subunit solute carrier family 7 member 11 (SLC7A11). It helps extracellular cystine to enter the cell membrane and synthesize GSH via ATP-dependent enzymes such as glutamate cysteine ligase and GSH synthetase. It also exports intracellular glutamate to the extracellular fluid through the system Xc− to maintain and protect normal cell function (9). SLC7A11 is regulated by a variety of factors, such as TP53, NFE2L2, and BRCA1-associated protein 1, Mucin 1, as well as cell-surface associated proteins or autophagy-associated genes, for example, BECN1. Inhibition of SLC7A11 pathway may be an important upstream mechanism for ferroptosis. Thus, the survival and growth of cancer cells are largely dependent on the transport activity of the system Xc−, which makes them a potential target for the development of anticancer drugs. GSH, a powerful antioxidant, is critical in inhibiting the formation of peroxides and the reduction of ROS and reactive nitrogen in cell membranes. As a cofactor and substrate of GPX4, it can prevent the lipid peroxidation in the biological membrane. Lipid peroxidases are responsible for the death of cells and serious oxidative damage (10). In addition, FSP1, a newly developed antioxidant, activates coenzyme Q10 to remove lipid peroxidation. Therefore, lipid peroxidation and ferroptosis can be inhibited at the same time (11). During ferroptosis, membrane damage occurs. But cells can reduce the degree of damage to the membrane by activating GSH, coenzyme Q10, Nuclear factor erythroid 2 (Nrf2) and membrane repair pathway (ESCRT-III pathway) (12). Furthermore, GPX4, a phospholipid peroxidase, plays an important role in reducing phospholipid peroxides and inhibiting the reaction between phospholipid peroxides and labile iron pools in cells through depletion of GSH. This, in turn, terminates the free radical-driven amplification reaction. Therefore, GPX4 not only acts as anti-lipid peroxidation, but also acts as a downstream regulator that inhibits ferroptosis (13). Erastin and RSL3 are two typical inducers of ferroptosis. Erastin triggers ferroptosis by inhibiting the system Xc−, leading to cysteine depletion and insufficient synthesis of GSH, disrupting the function of GPX4 (14). Recent studies have also shown that certain drug-resistant cancer cells with characteristics of EMT are highly sensitive to ferroptosis inducing drugs, providing a new research area for targeted therapy (15).
In the course of EMT, epithelial cells lose their apical-basal polarity and cell-cell adhesion, and acquire spindle-shaped mesenchymal shapes to gain the ability to migrate and invade, which are closely related to the invasion, migration and metastasis of cancer (16). During the course of EMT, the cells lost their epithelial cell markers, such as E-cadherin (CDH1), cytokeratins, and occludin, and upregulates N-cadherin, vimentin, fibronectin, β1 and β3 integrins (17). EMT is related to core transcription factors, epigenetic regulation, post-transcriptional regulation, and gene mutation. ZEB1/2, SNAIL1/2 and TWIST1/2 are key transcription factors (18). Epigenetic modification plays an important role in EMT through regulation of transcription factors. There is a positive correlation between high methylation of CDH1 and EMT (19). CDH1 is a subtype of epithelial cell specific calcium binding proteins (E-cadherin), which is expressed in epithelial cells. As a tumor suppressor gene, E-cadherin plays a role in regulating cell polarity, differentiation, migration, and stem cell properties (20). Furthermore, ZEB1/2 binds to the E-box regulatory gene sequence in the promoter to repress CDH1 transcription and activate the expression of mesenchymal phenotypes such as wave proteins, thereby increasing cell proliferation and metastasis (20). ZEB1 also regulates the expression of CDH1 via epigenetic regulation, which is critical to EMT via MAPK/ERK, JAK/STAT3, PI3K/AKT, and so on (18). The expression of SNAIL is up-regulated in recurrent tumors by binding to E box on CDH1 promoter and inhibiting CDH1 transcription (20). TWIST can decrease the expression of E-cadherin, and enhance the expression of Vimentin, N-cadherin, which may be related to the prognosis of cancer (21). Some non-coding RNAs, such as miR-200 and miR-205, regulate the expression of EMT-induced transcription factors, which can inhibit ZEB1/2 expression. Inhibition of miR-200c or high expression of ZEB1 may lead to poor prognosis in various cancers, including breast and ovarian carcinoma. In the regulation of EMT, long non-coding RNAs (lncRNAs), for example, TGF-β-activated lncRNA (lncRNA-ATB) and lncRNA-PNUTS. They can act as sponges for miR-200 and miR-205, preventing them from inhibiting the expression of EMT-inducing transcription factors (22). TGF-β, a well-defined epithelial cell inhibiting growth factor, can induce EMT, resulting in the loss of E-cadherin and the proliferation of mesenchymal markers, such as N-cadherin (23). Mutations of TGF-β can cause cancer cells to proliferate uncontrollably. Successfully promoting the migration and metastasis of cancer cells by regulating the expression of EMT transcription factors. Therefore, these transcription factors can serve as therapeutic targets in cancer treatment. It is hoped that further study of these mechanisms will lead to a new breakthrough in the treatment of cancer and improve the prognosis of cancer patients.
Ferroptosis is closely related to EMT, which are important biological processes associated with the growth of cancer. The latest research indicates that drug-resistant cancer cells that have Patients undergoing EMT are more susceptible to ferroptosis, indicating that they are more prone to being killed by ferroptosis inducers compared to non-drug-resistant cancer cells (15). It also suggests that ferroptosis may inhibit EMT progression in cancer.
Understanding the interaction of EMT with ferroptosis is critical to overcoming the challenges of cancer therapy (3). It is imperative to find new treatments to fill the gaps in current therapeutic strategies and to improve the overall outcome of patients. Future studies should explore the molecular mechanisms and interactions of EMT and ferroptosis in order to advance the field of cancer therapy.
Recently, it has been demonstrated that high mesenchymal status is closely related to GPX signaling. GPX4 is involved in the induction of ferroptosis in cancer cells. The EMT-mediated transcription factor ZEB1 regulates and induces EMT via TGF-β/Smad, Furthermore, it can promote ferroptosis through direct inhibition of the activity of GPX4 and activation of the PPARγ (PPARγ) pathway (24). TGF-β can promote EMT of cancer cells by inducing ZEB1 and enhancing GPX4 inhibitor activity (25). Metadherin (MTDH), a newly discovered cancer-related antigen, promotes EMT, invasion and metastasis in a wide range of cancers, including breast and colon. It downregulates E-cadherin expression through activation of Wnt/β-catenin, MAPK and PI3K/AKT (26). MTDH, as an RNA binding protein and is also a positive regulator of EMT, can make cells more sensitive to ferroptosis by decreasing GPX4, SLC3A2, and reducing cysteine, GSH and increasing glutamate (24). The regulation of MDTH-GPX4/SLC3A2-GSH axis may contribute to the improvement of EMT susceptibility to ferroptosis. Moreover, it has been shown that targeting GPX4 can inhibit the growth and metastasis of epithelial-derived carcinoma by inhibiting the proliferation of steroid regulatory element-binding protein (27). Furthermore, Jiang et al. (28) have shown that targeting GPX4 might be a useful strategy to overcome the first generation NSCLL. Loss of GPX4 may result in the death of iron in the refractory carcinoma cells due to various cancers and therapies. Thus, targeting GPX4 or inducing ferroptosis might be an effective therapeutic strategy to overcome drug resistance.
It has been shown that targeting GPX4 or inducing ferroptosis may be an effective way to overcome drug resistance. Targeted regulation of GPX4 is promising as a novel approach to cancer therapy. The further study of the role of GPX4 in the development and therapy of cancer will contribute to a better understanding and application of this strategy.
The CD44-dependent increase in iron endocytosis promotes iron-dependent demethylase activity, which promotes the expression of genes associated with EMT signaling, thereby sensitising cancer cells to iron transfer (29). Data from these preclinical studies suggest that EMT may confer sensitivity to ferroptosis-based treatments.
Nrf2 is a transcription factor with a basic leucine zipper domain that partially supports tumor cell proliferation by activating the oxidative stress response (30). rf2 regulates ferroptosis directly or indirectly by regulating the content of GPX4, Intracellular free iron level, the regeneration of coenzyme NAD (P) H and mitochondrial function (14). NF2 has an adverse effect on ferroptosis by increasing the expression of Fe and ROS, such as quinoline oxidoreductase 1 and hemolymph 1(HO-1) (31). The Kelch-like ECH-associated protein 1 (Keap1) regulates the activity of Nrf2 in the cytoplasm, which is critical for ubiquitination and proteasome degradation (30). Using a protein–protein interaction (PPI) network, It has been shown that Nrf2 regulates ferroptosis by directly influencing the GPX4 and PPARγ pathways, and regulates the level of iron ions via Nrf2/HO-1 (14). High stromal states make many cancer cells less sensitive to ferroptosis, while drug-resistant stromal states help cells to avoid ferroptosis by regulating lipid peroxidation (24). Moreover, GPX4 inhibition leads to intracellular iron-mediated peroxidation, inducing ferroptosis. Therefore, ferroptosis induction can effectively eliminate EMT in cancer (32). The Keap1/Nrf2/HO-1 signaling pathway has been found to play an important role in the regulation of ferroptosis and EMT (33).
Nrf2 binds directly to the anti-oxidation reaction element region of the system Xc− Subunit promoter, and promotes the expression of the system Xc− (34). Inhibiting Nrf2 or over-expression of Keap1 reduces the level of system Xc−, whereas over-expression of system Xc− has no effect on Nrf2 and Keap1. Thus, the system Xc− may be a potential downstream target of Nrf2 (35). Atorvastatin inhibits the system Xc−/GPX4/Nrf2 axis by interfering with the antioxidant system and promoting ferroptosis. Inhibition of system Xc−, GSH depletion, and increased oxidative stress all result in the occurrence of EMT. Therefore, the control of the system Xc− can inhibit the EMT, thereby reducing the metastasis and resistance of the cancer (36).
Nrf2 plays a key role in the regulation of EMT. Studying Nrf2, as well as its downstream targets, will help to better understand the mechanisms involved and guide the development of new therapeutic strategies. Deep study of Nrf2’s function and regulation network will be helpful to enhance the efficacy of cancer therapy, particularly in overcoming therapeutic resistance and inhibiting metastasis.
BACH1 is a hemoglobin-binding transcription factor, which plays an important role in the regulation of oxidative stress, hemoglobin, and iron metabolism (37). BACH1 regulates EMT through modulation of intercellular adhesion genes, such as claudin3 and claudin4. It also regulates the transcription factors, including FOXA1 and Snail2, which may be involved in the EMT, and form a direct effect on the EMT. BACH1 inhibits the expression of CDH1 and promotes EMT, whereas FOXA1 acts as an intermediary between BACH1 and E-cadherin (38). In addition, BACH1 directly activates Snail2, which is a typical transcription factor that activates EMT through inhibition of adhesion and promotion of stem cells (38). Moreover, BACH1 can promote ferroptosis through inhibition of GSH pathway and instability of iron metabolism (37). Through the activation of Hippo signaling pathway, E-cadherin-mediated cell contact can inhibit ferroptosis, thus decreasing the activity of YAP, the transcription coactivator, caused by ferroptosis (39). YAP is activated during EMT initiation, resulting in increased susceptibility to ferroptosis. Since BACH1 can inhibit E-cadherin expression and cell adhesion, it can be used to inhibit E-cadherin-Hippo-YAP pathway via CYC-GSH-GPX4 to promote ferroptosis (37).
BACH1 can also inhibit Nrf2 signaling pathway by binding to Nrf2. Deletion of BACH1 increased expression of Nrf2-regulated genes, especially HO-1 (40). BACH1 promotes the increase of unstable iron content in the cell, whereas Nrf2 inhibits an increase in intracellular iron content. Increasing the ratio of BACH1/Nrf2 leads to ferroptosis (41). Therefore, BACH1 can be used to link ferroptosis to EMT, leading to a deeper linkage.
BACH1 plays an important role in the regulation of EMT. Further research on the function and regulation of BACH1 will help us better understand the interplay between EMT and ferroptosis and provide guidance for the development of new therapeutic strategies. At the same time, the BACH1/Nrf2 regulatory networks are being studied, and their potential applications in cancer therapy are discussed.
ROS are highly reactive molecules that are important regulators of signaling pathways. They are tumorigenic in nature and act as secondary messengers in the cellular signaling cascade to induce and maintain the oncogenic phenotype of cells as well as sustain cancer development by inducing DNA damage and promoting the proliferation, survival, and migration of tumor cells (42). ROS acts as a signaling molecule capable of triggering different types of cell death, including ferroptosis, which requires ROS accumulation throughout the process, thereby disrupting cell membrane integrity (4). A study has reported that ROS generation is enhanced during EMT (43). In general, ROS are produced via a series of specialized enzyme complexes or as a by-product of redox reactions. Furthermore, in the Fenton reaction, ROS are produced in lysosomes and unstable iron pools where ferritin phagocytosis occurs. Ferroptosis induction involves ROS production, and ferritin phagocytosis-mediated ROS production contributes to EMT inhibition. Therefore, the relationship between EMT and ferroptosis and ROS production should be elucidated. Dithiocarbamate derivatives are a class of iron-chelating agents with anticancer properties, including the ability to induce ferritinophagy (44). Deng et al. (33) reported that 2,2’-dipyridylketone hydrazone dithiocarbamate (DpdtbA) is an antagonist of TGF-β1-induced EMT by the generation of ROS induced by ferritinophagately. In addition, DpdtbA treatment led to the removal of GPX4 and the system Xc−, which increased lipid peroxidation, indicating that DpdtbA mimics the role of the ferroptosis inducer Erastin in the induction of ferroptosis (44). Experimental results also showed that Fer-1 was able to counteract the regulation of DpdtbA on Vimentin and E-cadherin, suggesting that the ferroptosis induction was involved in suppressing EMT (33). Nevertheless, cancer cells thrive in hypoxic environments, and DpdtbA induces EMT suppression under these conditions, possibly associated with activation of P53, PHD2/Hif1α pathways (45).
ROS plays an important role in the development of cancer and may affect EMT and ferroptosis. As iron chelators, dithiocarbamate derivatives, such as DpdtbA, can interfere with the generation of ROS induced by ferritinophagy and may inhibit EMT. Understanding this mechanism will be helpful in the development of cancer metastasis and drug resistance, and in the development of therapeutic strategies. The exploration of ROS, EMT, and ferroptosis signaling pathways will provide a better understanding and strategy for cancer therapy.
P53 is a tumor suppressor protein and a key and complex ROS regulatory factor in cancer development. It has both antioxidant and pro-oxidant functions (45). It has both antioxidant and pro-oxidant functions. P53-inducible ROS may induce cell death via apoptosis and ferroptosis, and its anti-oxidative activity may contribute to the inhibition of the growth of cancer by preventing DNA damage (46). Activated P53 binds to SLC7A11’s promoter region, inhibits its transcription activity, decreases the intracellular cysteine and GSH concentrations, and enhances the accumulation of ROS, Indirect inhibition of the activity of GPX4, resulting in the formation of ferroptosis in cells (47). Studies of cambogia (an iron chelator) have demonstrated that it can markedly increase the levels of lipid ROS in TGF-β1 induced melanoma cells, Regulation of oxidative stress by activating P53/SLC7A11/GPX4 signaling pathway, induction of ferroptosis and inhibition of EMT, And thus block the invasion and metastasis of the tumor (48). The PI3K/AKT/mTOR pathway is critical for the growth and survival of cells, and the inhibition of this pathway may increase the therapeutic effect of ferroptosis. There is growing evidence that the AKT/mTOR pathway is involved in the regulation of critical processes like EMT, motility, and metastasis of cancer cells (49, 50). The AKT/mTOR pathway is downstream of P53, sActivation of P53 results in AKT and mTOR downregulation. It was found that the EMT reversal induced by 2,2 ‘-dipyridyketone hydrazine dithiocarbamate was related to P53/AKT/mTOR pathway (23). Thus, through modulation of P53/PI3K/AKT/mTOR signaling pathway, it is possible to regulate the susceptibility of cancer cells to ferroptosis.
P53 plays an important role as a regulator of ROS in the development of cancer. It influences the proliferation, survival and EMT of cancer cells through induction of ROS and regulation of PI3K/AKT/mTOR. Deep Research on the mechanism of P53 in regulating ROS, ferroptosis, and EMT will help to better understand the molecular mechanisms underlying cancer progression and provide new insights into the development of therapeutic strategies that target these pathways.
Nrf2 is an important factor in the regulation of ROS. When ROS levels increase, the degradation of Nrf2 is disrupted by Keap1, allowing Nrf2 to stabilize and enter the cell nucleus to activate a protective antioxidant response (51). By inducing iron engulfment within ferritin, the activation of Nrf2 results in the up-regulation of HO-1, which is advantageous to induce ferroptosis (52). These results suggest that Keap1/Nrf2/HO-1 can regulate the EMT and ferroptosis processes. Generally speaking, the intensity of ROS induced by ferritin engulfment has a great effect on the occurrence of ferroptosis and progression of EMT, and also influences the activation of Keap1/Nrf2/HO-1 (53).
Deep research on the role of Nrf2 in the regulation of ROS, ferroptosis, and EMT may help to understand the complexity of cancer development and therapy. As one of the regulatory mechanisms produced by ROS, iron sequestration plays an important role not only in the induction of ferroptosis, but also in the regulation of EMT and activation of Keap1/Nrf2/HO-1 pathway through the influence of ROS. Therefore, further research on the mechanism of Keap1/Nrf2/HO-1 signaling pathway will contribute to the development of new therapeutic strategies, especially in the treatment of cancer.
The Wnt/β-catenin signaling pathway plays an important role in the development and progression of cancer by regulating cell proliferation and apoptosis. When Wnt ligands (such as Wnt3a and Wnt1) are secreted, they bind to Frizzled receptors and low-density lipoprotein receptors, thereby blocking the pathway of β-catenin degradation and activating downstream gene transcription Cyclin D1, and WISP (54). These genes are activated to promote the EMT, thus triggering the growth and metastasis of the tumor (55). In the activation process of the Wnt signaling pathway, β-catenin enters the cell nucleus and binds to transcription factors TCF/LEF. TCF4 plays an important role in Wnt/β-catenin signaling pathway (56). It is suggested that the activation of Wnt/β-catenin signaling pathway enhances TCF4 expression and promotes GPX4 transcription via β-catenin/TCF4. Thus, the formation of ROS, and the inhibition of ferroptosis in gastric carcinoma cells were inhibited (57). The Wnt signaling pathway plays an important role in the regulation of the progression of EMT and ferroptosis. At present, however, there is no evidence to support the specific association of Wnt signaling with ferroptosis and EMT. Therefore, further study on the role of ferroptosis in the regulation of EMT via Wnt signaling pathway will contribute to a better understanding of the interaction between the two pathways in cancer growth and metastasis.
Autophagy is a cellular self-digestive process that maintains cellular homeostasis and function by degrading and recycling harmful or ageing substances within the cell. Recent studies have found that autophagy is closely related not only to cell survival and death, but also to some important biological processes such as ferroptosis and EMT (58).
ROS-induced autophagy is a process that gives expression to key regulators of ferritin degradation and transferrin receptor 1 during ferroptosis, which has been suggested by recent studies to be a process of autophagic cell death that can be termed autophagy-dependent ferroptosis (59, 60). Firstly, autophagy affects the occurrence of ferroptosis by regulating ROS generation (Figure 1). Studies have shown that autophagy can remove excessive intracellular iron ions and reduce the reaction between iron ions and ROS, thereby reducing the amount of ROS generation and thus alleviating the oxidative stress state of cells (61). In addition, autophagy can also reduce intracellular ROS generation by removing sources of ROS generation, such as mitochondria and endoplasmic reticulum. Thus, autophagy can prevent ferroptosis by regulating ROS generation (62).
Figure 1 Effects of autophagy on ferroptosis and EMT (By Figdraw).
Secondly, autophagy can also influence the onset of ferroptosis by regulating the clearance of ROS. It was found that autophagy can remove intracellular ROS and reduce oxidative stress in cells (63). Autophagy reduces intracellular ROS content by degrading ROS-generating sources such as mitochondria and transporting ROS to lysosomes for degradation through the autophagosome membrane. In addition, autophagy can enhance ROS scavenging by regulating the expression and activity of antioxidant enzymes. Thus, autophagy may also inhibit ferroptosis by regulating ROS scavenging (64).
In addition, autophagy is also closely related to the occurrence of EMT (Figure 1). Recent studies have shown that autophagy can inhibit EMT by degrading and regulating the expression of EMT transcription factors such as Snail, Twist and ZEB (65). In addition, autophagy can also influence the occurrence of EMT by removing intracellular aging substances and harmful substances and maintaining cellular homeostasis and function (66).
Taken together, ROS is involved in various response pathways affecting tumorigenesis, and a comprehensive understanding of the regulation of different cellular responses can facilitate the clinical application of ROS or ROS-regulated pathways to formulate and improve the shortcomings of current cancer therapies.
Therefore, the above ferroptosis related genes and their signaling pathways can be regulated to modulate EMT occurrence in cancer cells (Figure 2), providing more strategies for tumor treatment.
Significant progress has been made in the field of tumor treatment in recent years, including the increasing use of targeted drugs and immunotherapy. However, the problem of tumor drug resistance remains challenging. Therefore, reversing or inhibiting EMT has become a potential cancer treatment strategy to suppress the migration and metastasis of tumor cells to distant sites. Resistant cancer cells, upon transition to a mesenchymal state, become resistant to apoptosis induced by conventional therapies but are highly sensitive to ferroptosis inducers due to changes in their metabolic state. By inhibiting or activating ferroptosis related genes and their signaling pathways, the regulation of EMT by ferroptosis can be achieved, thereby improving the prognosis and treatment outcomes of cancer and providing new strategies to address clinically challenging cancers.
Further research on the interaction mechanism between ferroptosis and EMT not only helps us gain a deeper understanding of the mechanisms of tumor metastasis and recurrence but also provides new strategies for tumor treatment. Exploring and confirming the deeper relationship between these two processes can help us better grasp the diversity and complexity of tumor development, thus guiding clinical practice and the development of treatment strategies. Ferroptosis and EMT have significant implications in cancer treatment. Reversing or inhibiting EMT and modulating genes and signaling pathways related to ferroptosis can bring about breakthroughs in tumor treatment. Further research will help uncover the interplay mechanisms between these two processes and provide a theoretical basis for the development of more effective cancer treatment strategies.
WM: Writing – original draft. ZZ: Funding acquisition, Resources, Writing – review & editing. LS: Writing – review & editing. QW: Writing – review & editing. WF: Writing – review & editing. YT: Writing – review & editing. YH: Writing – review & editing. YW: Writing – review & editing.
The author(s) declare financial support was received for the research, authorship, and/or publication of this article. This work was supported by the Gansu Provincial Science and Technology Department Key Research and Development Project, Grant Number: 22YF7FA098, Gansu Province Outstanding Graduate Student “Innovation Star” Project, Grant Number: 2022CXZX-744, Lanzhou City Talent Innovation and Entrepreneurship Project in Gansu Province, Grant Number:2019-RC-119, and 2021 Gansu Province Higher Education Innovation Fund Project, Grant Number:2021B-228.
Thanks to all the authors for their contribution to this study.
The authors declare that the research was conducted in the absence of any commercial or financial relationships that could be construed as a potential conflict of interest.
All claims expressed in this article are solely those of the authors and do not necessarily represent those of their affiliated organizations, or those of the publisher, the editors and the reviewers. Any product that may be evaluated in this article, or claim that may be made by its manufacturer, is not guaranteed or endorsed by the publisher.
1. Robertson AG, Rendina LM. Gadolinium theranostics for the diagnosis and treatment of cancer. Chem Soc Rev (2021) 50(7):4231–44. doi: 10.1039/d0cs01075h
2. Fulda S, Debatin KM. Extrinsic versus intrinsic apoptosis pathways in anticancer chemotherapy. Oncogene (2006) 25(34):4798–811. doi: 10.1038/sj.onc.1209608
3. Majidinia M, Mirza-Aghazadeh-Attari M, Rahimi M, Mihanfar A, Karimian A, Safa A, et al. Overcoming multidrug resistance in cancer: Recent progress in nanotechnology and new horizons. IUBMB Life (2020) 72(5):855–71. doi: 10.1002/iub.2215
4. Dixon SJ, Lemberg KM, Lamprecht MR, Skouta R, Zaitsev EM, Gleason CE, et al. Ferroptosis: an iron-dependent form of nonapoptotic cell death. Cell (2012) 149(5):1060–72. doi: 10.1016/j.cell.2012.03.042
5. Dietrich C, Hofmann TG. Ferroptosis meets cell-cell contacts. Cells (2021) 10(9):2462. doi: 10.3390/cells10092462
6. Li C, Zhang Y, Liu J, Kang R, Klionsky DJ, Tang D. Mitochondrial DNA stress triggers autophagy-dependent ferroptotic death. Autophagy (2021) 17(4):948–60. doi: 10.1080/15548627.2020.1739447
7. Chen JJ, Galluzzi L. Fighting resilient cancers with iron. Trends Cell Biol (2018) 28(2):77–8. doi: 10.1016/j.tcb.2017.11.007
8. Ebrahimi N, Adelian S, Shakerian S, Afshinpour M, Chaleshtori SR, Rostami N, et al. Crosstalk between ferroptosis and the epithelial-mesenchymal transition: Implications for inflammation and cancer therapy. Cytokine Growth Factor Rev (2022) 64:33–45. doi: 10.1016/j.cytogfr.2022.01.006
9. Mao H, Zhao Y, Li H, Lei L. Ferroptosis as an emerging target in inflammatory diseases. Prog Biophys Mol Biol (2020) 155:20–8. doi: 10.1016/j.pbiomolbio.2020.04.001
10. Das UN. Saturated fatty acids, MUFAs and PUFAs regulate ferroptosis. Cell Chem Biol (2019) 26(3):309–11. doi: 10.1016/j.chembiol.2019.03.001
11. Bersuker K, Hendricks JM, Li Z, Magtanong L, Ford B, Tang PH, et al. The CoQ oxidoreductase FSP1 acts parallel to GPX4 to inhibit ferroptosis. Nature (2019) 575(7784):688–92. doi: 10.1038/s41586-019-1705-2
12. Dai E, Meng L, Kang R, Wang X, Tang D. ESCRT-III-dependent membrane repair blocks ferroptosis. Biochem Biophys Res Commun (2020) 522(2):415–21. doi: 10.1016/j.bbrc.2019.11.110
13. Jiang X, Stockwell BR, Conrad M. Ferroptosis: mechanisms, biology and role in disease. Nat Rev Mol Cell Biol (2021) 22(4):266–82. doi: 10.1038/s41580-020-00324-8
14. Song X, Long D. Nrf2 and ferroptosis: A new research direction for neurodegenerative diseases. Front Neurosci (2020) 14:267. doi: 10.3389/fnins.2020.00267
15. Viswanathan VS, Ryan MJ, Dhruv HD, Gill S, Eichhoff OM, Seashore-Ludlow B, et al. Dependency of a therapy-resistant state of cancer cells on a lipid peroxidase pathway. Nature (2017) 547(7664):453–7. doi: 10.1038/nature23007
16. Pastushenko I, Blanpain C. EMT transition states during tumor progression and metastasis. Trends Cell Biol (2019) 29(3):212–26. doi: 10.1016/j.tcb.2018.12.001
17. Lu W, Kang Y. Epithelial-mesenchymal plasticity in cancer progression and metastasis. Dev Cell (2019) 49(3):361–74. doi: 10.1016/j.devcel.2019.04.010
18. Dongre A, Weinberg RA. New insights into the mechanisms of epithelial-mesenchymal transition and implications for cancer. Nat Rev Mol Cell Biol (2019) 20(2):69–84. doi: 10.1038/s41580-018-0080-4
19. Huang Y, Hong W, Wei X. The molecular mechanisms and therapeutic strategies of EMT in tumor progression and metastasis. J Hematol Oncol (2022) 15(1):129. doi: 10.1186/s13045-022-01347-8
20. Serrano-Gomez SJ, Maziveyi M, Alahari SK. Regulation of epithelial-mesenchymal transition through epigenetic and post-translational modifications. Mol Cancer (2016) 15(1):1–14. doi: 10.1186/s12943-016-0502-x
21. Dave N, Guaita-Esteruelas S, Gutarra S, Frias À, Beltran M, Peiró S, et al. Functional cooperation between snail1 and twist in the regulation of ZEB1 expression during epithelial to mesenchymal transition. J Biol Chem (2011) 286(14):12024–32. doi: 10.1074/jbc.M110.168625
22. Grelet S, Link LA, Howley B, Obellianne C, Palanisamy V, Gangaraju VK, et al. A regulated PNUTS mRNA to lncRNA splice switch mediates EMT and tumour progression. Nat Cell Biol (2017) 19(9):1105–15. doi: 10.1038/ncb3595
23. Xu Z, Feng J, Li Y, Guan D, Chen H, Zhai X, et al. The vicious cycle between ferritinophagy and ROS production triggered EMT inhibition of gastric cancer cells was through p53/AKT/mTor pathway. Chem Biol Interact (2020) 328:109196. doi: 10.1016/j.cbi.2020.109196
24. Liu X, Zhang Y, Wu X, Xu F, Ma H, Wu M, et al. Targeting ferroptosis pathway to combat therapy resistance and metastasis of cancer. Front Pharmacol (2022) 13:909821. doi: 10.3389/fphar.2022.909821
25. Lee J, You JH, Kim MS, Roh JL. Epigenetic reprogramming of epithelial-mesenchymal transition promotes ferroptosis of head and neck cancer. Redox Biol (2020) 37:101697. doi: 10.1016/j.redox.2020.101697
26. Dhiman G, Srivastava N, Goyal M, Rakha E, Lothion-Roy J, Mongan NP, et al. Metadherin: A therapeutic target in multiple cancers. Front Oncol (2019) 9:349. doi: 10.3389/fonc.2019.00349
27. Fukuda M, Ogasawara Y, Hayashi H, Okuyama A, Shiono J, Inoue K, et al. Down-regulation of glutathione peroxidase 4 in oral cancer inhibits tumor growth through SREBP1 signaling. Anticancer Res (2021) 41(4):1785–92. doi: 10.21873/anticanres.14944
28. Jiang XM, Xu YL, Yuan LW, Zhang LL, Huang MY, Ye ZH, et al. TGFβ2-mediated epithelial-mesenchymal transition and NF-κB pathway activation contribute to osimertinib resistance. Acta Pharmacol Sin (2021) 42(3):451–9. doi: 10.1038/s41401-020-0457-8
29. Müller S, Sindikubwabo F, Cañeque T, Lafon A, Versini A, Lombard B, et al. CD44 regulates epigenetic plasticity by mediating iron endocytosis. Nat Chem (2020) 12(10):929–38. doi: 10.1038/s41557-020-0513-5
30. Taguchi K, Yamamoto M. The KEAP1-NRF2 system as a molecular target of cancer treatment. Cancers (Basel) (2020) 13(1):46. doi: 10.3390/cancers13010046
31. Lei P, Bai T, Sun Y. Mechanisms of ferroptosis and relations with regulated cell death: A review. Front Physiol (2019) 10:139. doi: 10.3389/fphys.2019.00139
32. Hangauer MJ, Viswanathan VS, Ryan MJ, Bole D, Eaton JK, Matov A, et al. Drug-tolerant persister cancer cells are vulnerable to GPX4 inhibition. Nature (2017) 551(7679):247–50. doi: 10.1038/nature24297
33. Guan D, Zhou W, Wei H, Wang T, Zheng K, Yang C, et al. Ferritinophagy-mediated ferroptosis and activation of keap1/nrf2/HO-1 pathway were conducive to EMT inhibition of gastric cancer cells in action of 2,2'-di-pyridineketone hydrazone dithiocarbamate butyric acid ester. Oxid Med Cell Longevity (2022) 2022:3920664. doi: 10.1155/2022/3920664
34. Carpi-Santos R, Calaza KC. Alterations in system x(c)(-) expression in the retina of type 1 diabetic rats and the role of Nrf2. Mol Neurobiol (2018) 55(10):7941–8. doi: 10.1007/s12035-018-0961-8
35. Fan Z, Wirth AK, Chen D, Wruck CJ, Rauh M, Buchfelder M, et al. Nrf2-Keap1 pathway promotes cell proliferation and diminishes ferroptosis. Oncogenesis (2017) 6(8):e371. doi: 10.1038/oncsis.2017.65
36. Sun L, Dong H, Zhang W, Wang N, Ni N, Bai X, et al. Lipid peroxidation, GSH depletion, and SLC7A11 inhibition are common causes of EMT and ferroptosis in A549 cells, but different in specific mechanisms. DNA Cell Biol (2021) 40(2):172–83. doi: 10.1089/dna.2020.5730
37. Nishizawa H, Matsumoto M, Shindo T, Saigusa D, Kato H, Suzuki K, et al. Ferroptosis is controlled by the coordinated transcriptional regulation of glutathione and labile iron metabolism by the transcription factor BACH1. J Biol Chem (2020) 295(1):69–82. doi: 10.1074/jbc.RA119.009548
38. Sato M, Matsumoto M, Saiki Y, Alam M, Nishizawa H, Rokugo M, et al. BACH1 promotes pancreatic cancer metastasis by repressing epithelial genes and enhancing epithelial-mesenchymal transition. Cancer Res (2020) 80(6):1279–92. doi: 10.1158/0008-5472.CAN-18-4099
39. Wu J, Minikes AM, Gao M, Bian H, Li Y, Stockwell BR, et al. Intercellular interaction dictates cancer cell ferroptosis via NF2-YAP signalling. Nature (2019) 572(7769):402–6. doi: 10.1038/s41586-019-1426-6
40. Zhang H, Zhou L, Davies KJA, Forman HJ. Silencing Bach1 alters aging-related changes in the expression of Nrf2-regulated genes in primary human bronchial epithelial cells. Arch Biochem Biophys (2019) 672:108074. doi: 10.1016/j.abb.2019.108074
41. Nishizawa H, Yamanaka M, Igarashi K. Ferroptosis: regulation by competition between NRF2 and BACH1 and propagation of the death signal. FEBS J (2023) 290(7):1688–704. doi: 10.1111/febs.16382
42. Renaudin X. Reactive oxygen species and DNA damage response in cancer. Int Rev Cell Mol Biol (2021) 364:139–61. doi: 10.1016/bs.ircmb.2021.04.001
43. Chatterjee R, Chatterjee J. ROS and oncogenesis with special reference to EMT and stemness. Eur J Cell Biol (2020) 99(2-3):151073. doi: 10.1016/j.ejcb.2020.151073
44. Li L, Li H, Li Y, Feng J, Guan D, Zhang Y, et al. Ferritinophagy-mediated ROS production contributed to proliferation inhibition, apoptosis, and ferroptosis induction in action of mechanism of 2-pyridylhydrazone dithiocarbamate acetate. Oxid Med Cell Longevity (2021) 2021:5594059. doi: 10.1155/2021/5594059
45. Guan D, Li C, Li Y, Li Y, Wang G, Gao F, et al. The DpdtbA induced EMT inhibition in gastric cancer cell lines was through ferritinophagy-mediated activation of p53 and PHD2/hif-1alpha pathway. J Inorg Biochem (2021) 218:111413. doi: 10.1016/j.jinorgbio.2021.111413
46. Jiang L, Kon N, Li T, Wang SJ, Su T, Hibshoosh H, et al. Ferroptosis as a p53-mediated activity during tumour suppression. Nature (2015) 520(7545):57–62. doi: 10.1038/nature14344
47. Kang R, Kroemer G, Tang D. The tumor suppressor protein p53 and the ferroptosis network. Free Radic Biol Med (2019) 133:162–8. doi: 10.1016/j.freeradbiomed.2018.05.074
48. Wang M, Li S, Wang Y, Cheng H, Su J, Li Q. Gambogenic acid induces ferroptosis in melanoma cells undergoing epithelial-to-mesenchymal transition. Toxicol Appl Pharmacol (2020) 401:115110. doi: 10.1016/j.taap.2020.115110
49. Li Y, Wang T, Sun Y, Huang T, Li C, Fu Y, et al. p53-mediated PI3K/AKT/mTOR pathway played a role in ptox(Dpt)-induced EMT inhibition in liver cancer cell lines. Oxid Med Cell Longevity (2019) 2019:2531493. doi: 10.1155/2019/2531493
50. Yi J, Zhu J, Wu J, Thompson CB, Jiang X. Oncogenic activation of PI3K-AKT-mTOR signaling suppresses ferroptosis via SREBP-mediated lipogenesis. Proc Natl Acad Sci United States America (2020) 117(49):31189–97. doi: 10.1073/pnas.2017152117
51. Cheung EC, Vousden KH. The role of ROS in tumour development and progression. Nat Rev Cancer (2022) 22(5):280–97. doi: 10.1038/s41568-021-00435-0
52. Chang LC, Chiang SK, Chen SE, Yu YL, Chou RH, Chang WC. Heme oxygenase-1 mediates BAY 11-7085 induced ferroptosis. Cancer Lett (2018) 416:124–37. doi: 10.1016/j.canlet.2017.12.025
53. Feng J, Li C, Xu R, Li Y, Hou Q, Feng R, et al. DpdtC-induced EMT inhibition in MGC-803 cells was partly through ferritinophagy-mediated ROS/p53 pathway. Oxid Med Cell Longevity (2020) 2020:9762390. doi: 10.1155/2020/9762390
54. Singh S, Nagalakshmi D, Sharma KK, Ravichandiran V. Natural antioxidants for neuroinflammatory disorders and possible involvement of Nrf2 pathway: A review. Heliyon (2021) 7(2):e06216. doi: 10.1016/j.heliyon.2021.e06216
55. Garlapati P, Ling J, Chiao PJ, Fu J. Circular RNAs regulate cancer-related signaling pathways and serve as potential diagnostic biomarkers for human cancers. Cancer Cell Int (2021) 21(1):317. doi: 10.1186/s12935-021-02017-4
56. Doumpas N, Lampart F, Robinson MD, Lentini A, Nestor CE, Cantu C, et al. TCF/LEF dependent and independent transcriptional regulation of Wnt/beta-catenin target genes. EMBO J (2019) 38(2):e98873. doi: 10.15252/embj.201798873
57. Wang Y, Zheng L, Shang W, Yang Z, Li T, Liu F, et al. Wnt/β-catenin signaling confers ferroptosis resistance by targeting GPX4 in gastric cancer. Cell Death Differ (2022) 29(11):2190–202. doi: 10.1038/s41418-022-01008-w
58. Liang C, Xi-Xi X, Yun-Xiang S, Qiu-Hua X, Yang-Yong L, Yuan-Sen H, et al. Surfactin inhibits Fusarium graminearum by accumulating intracellular ROS and inducing apoptosis mechanisms. World J Microbiol Biotechnol (2023) 39(12):340. doi: 10.1007/s11274-023-03790-2
59. Park E, Chung SW. ROS-mediated autophagy increases intracellular iron levels and ferroptosis by ferritin and transferrin receptor regulation. Cell Death Dis (2019) 10(11):822. doi: 10.1038/s41419-019-2064-5
60. Pierzynowska K, Rintz E, Gaffke L, Węgrzyn G. Ferroptosis and its modulation by autophagy in light of the pathogenesis of lysosomal storage diseases. Cells (2021) 10(2):365. doi: 10.3390/cells10020365
61. Zeb A, Choubey V, Gupta R, Kuum M, Safiulina D, Vaarmann A, et al. A novel role of KEAP1/PGAM5 complex: ROS sensor for inducing mitophagy. Redox Biol (2021) 48:102186. doi: 10.1016/j.redox.2021.102186
62. Zhang Y, Kong Y, Ma Y, Ni S, Wikerholmen T, Xi K, et al. Loss of COPZ1 induces NCOA4 mediated autophagy and ferroptosis in glioblastoma cell lines. Oncogene (2021) 40(8):1425–39. doi: 10.1038/s41388-020-01622-3
63. Colella B, Faienza F, Di Bartolomeo S. EMT regulation by autophagy: A new perspective in glioblastoma biology. Cancers (Basel) (2019) 11(3). doi: 10.3390/cancers11030312
64. Su X, Song C, He Z, Song Q, Meng L, Dong C, et al. Ambra1 in exosomes secreted by HK-2 cells damaged by supersaturated oxalate induce mitophagy and autophagy-ferroptosis in normal HK-2 cells to participate in the occurrence of kidney stones. Biochim Biophys Acta Mol Cell Res (2023) 1871(1):119604. doi: 10.1016/j.bbamcr.2023.119604
65. Chu C, Wang X, Yang C, Chen F, Shi L, Xu W, et al. Neutrophil extracellular traps drive intestinal microvascular endothelial ferroptosis by impairing Fundc1-dependent mitophagy. Redox Biol (2023) 67:102906. doi: 10.1016/j.redox.2023.102906
66. Das A, Chakrabarty S, Nag D, Paul S, Ganguli A, Chakrabarti G. Heavy water (D(2)O) induces autophagy-dependent apoptotic cell death in non-small cell lung cancer A549 cells by generating reactive oxygen species (ROS) upon microtubule disruption. Toxicol Vitro: Int J Assoc BIBRA (2023) 93:105703. doi: 10.1016/j.tiv.2023.105703
Keywords: ferroptosis, epithelial-mesenchymal transition, chemoresistance, mechanism, malignancy
Citation: Mu W, Zhou Z, Shao L, Wang Q, Feng W, Tang Y, He Y and Wang Y (2023) Advances in the relationship between ferroptosis and epithelial–mesenchymal transition in cancer. Front. Oncol. 13:1257985. doi: 10.3389/fonc.2023.1257985
Received: 13 July 2023; Accepted: 24 October 2023;
Published: 07 November 2023.
Edited by:
Liling Tang, Chongqing University, ChinaReviewed by:
Yi Zhang, Chongqing University of Technology, ChinaCopyright © 2023 Mu, Zhou, Shao, Wang, Feng, Tang, He and Wang. This is an open-access article distributed under the terms of the Creative Commons Attribution License (CC BY). The use, distribution or reproduction in other forums is permitted, provided the original author(s) and the copyright owner(s) are credited and that the original publication in this journal is cited, in accordance with accepted academic practice. No use, distribution or reproduction is permitted which does not comply with these terms.
*Correspondence: Zubang Zhou, enpieGpoQDEyNi5jb20=
Disclaimer: All claims expressed in this article are solely those of the authors and do not necessarily represent those of their affiliated organizations, or those of the publisher, the editors and the reviewers. Any product that may be evaluated in this article or claim that may be made by its manufacturer is not guaranteed or endorsed by the publisher.
Research integrity at Frontiers
Learn more about the work of our research integrity team to safeguard the quality of each article we publish.