- Department of Neurosurgery, Xijing Hospital, Fourth Military Medical University, Xi’an, China
Angiogenic growth factors (AGFs) are a class of secreted cytokines related to angiogenesis that mainly include vascular endothelial growth factors (VEGFs), stromal-derived factor-1 (SDF-1), platelet-derived growth factors (PDGFs), fibroblast growth factors (FGFs), transforming growth factor-beta (TGF-β) and angiopoietins (ANGs). Accumulating evidence indicates that the role of AGFs is not only limited to tumor angiogenesis but also participating in tumor progression by other mechanisms that go beyond their angiogenic role. AGFs were shown to be upregulated in the glioma microenvironment characterized by extensive angiogenesis and high immunosuppression. AGFs produced by tumor and stromal cells can exert an immunomodulatory role in the glioma microenvironment by interacting with immune cells. This review aims to sum up the interactions among AGFs, immune cells and cancer cells with a particular emphasis on glioma and tries to provide new perspectives for understanding the glioma immune microenvironment and in-depth explorations for anti-glioma therapy.
1 Introduction
Angiogenic growth factors (AGFs) are a series of secreted cytokines that plays crucial roles in angiogenesis by interacting with their corresponding receptors. AGFs mainly include vascular endothelial growth factors (VEGFs), stromal-derived factor-1 (SDF-1), platelet-derived growth factors (PDGFs), fibroblast growth factors (FGFs), transforming growth factor-beta (TGF-beta) and angiopoietins (ANGs). Angiogenesis is one of the important hallmarks of tumors, in which process AGFs play essential roles (1). However, accumulating evidence indicates that the function of AGFs is not only limited to tumor angiogenesis but also involved in tumor progression via multiple mechanisms that go beyond their angiogenic role (2–6). In the tumor microenvironment (TME), immune cells reprogram and express immunosuppressive phenotypes that leads to escaping of tumor cells from host immune surveillance and attack, in which process AGFs can be involved (7, 8). Anti-angiogenic therapy by targeting AGFs to normalize tumor vessels has also been found to improve anti-tumor immunity. Combination of anti-angiogenic drugs and immunotherapy has become a canonical treatment for hepatocellular carcinoma, non-small cell lung cancer and renal cell carcinoma, but still, it has not always been successful (8–10). A better understanding of the tangled interplay among AGFs, immune cells and cancer cells in the TME is urgently needed to shed lights on finding new anti-tumor therapeutic avenues.
Glioma accounts for about 80% of all intracranial malignance (11). The 5-year survival rate for patients with glioblastoma (GBM) which is the most aggressive form of glioma is only 6.9% (12). Angiogenesis in glioma and GBM is particularly obvious among all tumors (13, 14). Anti-angiogenic therapy by targeting AGFs or their receptors has become a hot topic in the treatment of glioma during the past decades (15, 16). This therapy differs from the traditional anti-tumor therapies. It aims to inhibit tumor growth rather than directly attack tumor cells. Unfortunately, most clinical trials of anti-angiogenic treatment to glioma ended with patients showing serious side effects or no significant benefits (16, 17). Therefore, the anti-angiogenic treatment of glioma should be considered from a more comprehensive perspective than just focusing on the vessels. The microenvironment of glioma contains tumor cells, glial cells, immune cells, neurons, vasculature and extracellular matrix. The glioma microenvironment generates a pro-tumor dynamic with significant angiogenesis and immunosuppression (18). The innate immune system including natural killer (NK) cells, macrophages, microglia and neutrophils and the adaptive immune system together establish a well-regulated immunity for proper brain function (19). Besides angiogenesis, glioma exhibits strong immunosuppressive properties of the microenvironment (20, 21). The expression of most AGFs, represented by VEGFs, were significantly upregulated in glioma (22, 23).
Aberrantly expressed AGFs interacts with immune cells by binding to the related receptors that expressed could be one of the explanations of immunosuppression exhibited in the glioma microenvironment (24, 25). A comprehensive summary of the interactions between AGFs, immune cells and tumor cells in the glioma microenvironment is necessary, which may help us to better understand the connections and provide new perspectives for deeper explorations on anti-glioma treatment. In this article, we review the relations and interplay among AGFs, immune cells and tumor cells with a special focus on the glioma microenvironment (Figure 1).
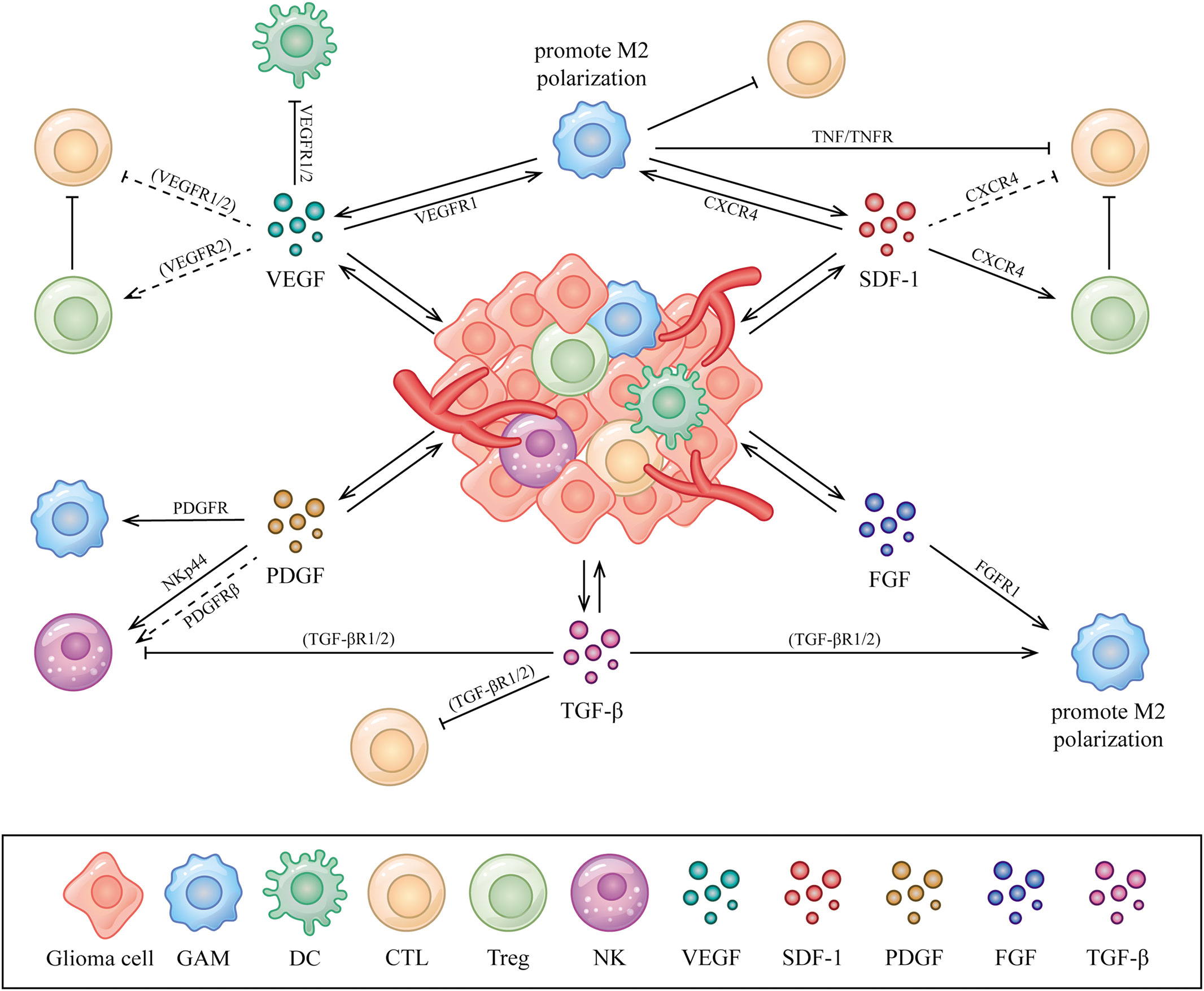
Figure 1 Interactions between AGFs, immune cells and tumor cells in the glioma microenvironment. AGFs including VEGFs, PDGFs, SDF-1, FGFs and TGF-β secreted by glioma or stromal cells could be upregulated in the glioma microenvironment, which acts on various immune cells including GAMs, DCs, T cells and NK cells to perform immunomodulatory functions mainly as immunosuppression. The directional arrow indicates effect of promoting. The suppressive arrow indicates effect of inhibiting. The dashed line indicates speculated associations that have not been confirmed in the glioma microenvironment but in other conditions.
2 VEGF
Vascular endothelial growth factors (VEGFs), alternatively referred to as vascular permeability factors, are a protein family related to angiogenesis. VEGFA, VEGFB, VEGFC, VEGFD, VEGFE (viral VEGF), VEGFF (snake venom VEGF), placental growth factor (PlGF) and endocrine gland-derived vascular endothelial growth factor (EG-VEGF) together construct VEGF family (26). This family of growth factors correspond to a variety of receptors including the tyrosine kinase receptors, which are named as VEGFR1-3 respectively, together with neuropilin and co-receptors of heparan sulfate proteoglycan families (27). VEGFA/VEGFR2 is the most potent combination in angiogenesis. VEGFC and VEGFD bind primarily to VEGFR3 to induce lymph angiogenesis and developmental angiogenesis. In contrast, VEGFR1 primarily acts to attenuate angiogenic signaling (28). However, VEGFR1 and VEGFR3 can play an alternative role in promoting angiogenesis when VEGFR2 pathway is reduced (29). In physiological conditions, VEGF is expressed in early embryos, various vascular tissues, heart, kidney, skeletal muscle, endocrine glands and other tissues, which play major roles in angiogenesis, vessel permeability and extracellular matrix degeneration.
In the course of tumorigenesis, VEGF is primarily derived from tumor cells and can also be secreted by smooth muscle cells, keratinocytes, neutrophils, platelets, macrophages, endothelial cells and fibroblasts (30, 31). VEGF expression can be elevated by activation of oncogenes such as Ras, stimulation of certain cytokines and growth factors including PDGF, epidermal growth factor (EGF), tumor necrosis factor-α, interleukin-1, interleukin-6 and HIF-1 (32). One of the typical pathological features of GBM is abundant angiogenesis which is associated with VEGF closely. A recent study reported that the expression level of VEGF is an independent risk factor for glioma prognosis (33). Studies have found that GBM cells express and secrete VEGFA protein, which was regulated by protein disulfide isomerase A4 in GBM cells (34, 35). Another study of GBM has shown that VEGFA was dramatically overexpressed while no significant change of VEGFB expression was detected in GBM patients (36). However, anti-VEGF therapy alone has not shown significant advantages in glioma treatment in patients. More and more emphasis on the function of VEGF of its immune effect has been shown. VEGFA was considered as a core negative gene affecting immune activity in the GBM microenvironment (37). VEGFRs are expressed both on the surface of immune cells and vascular endothelial cells. In GBM tissues, VEGFR1 is mainly expressed on tumor cells and tumor-associated macrophages. VEGFR2 is expressed mostly on tumor stem cells, regulatory T cells (Tregs) and vessel endothelial cells (38, 39). Due to the ability to interact with VEGF receptors expressed on immune cells, VEGF is able to exert great influences in the glioma immune microenvironment (40).
The primarily discovered immunosuppressive function of VEGF is to inhibit dendritic cell (DC) maturation, which causes less tumor antigen presentation and results in a potential immune avoidance of the tumor (41). VEGFR1 and VEGFR2 are expressed on DCs. VEGFR1 can regulate maturation of DC while VEGFR2 can control the function of mature DC (42, 43). It has been confirmed that VEGFR1 is a main receptor for VEGF-related suppression of DC maturation. When VEGF and PlGF binds to VEGFR1, the downstream signal transmission leads to blockade of the nuclear factor kappa-B (NF-κB) activation in hematopoietic progenitor cells of bone marrow which in turn affects the early stage of bone marrow/DC differentiation (42, 43). Researchers injected GL261 cells overexpressing Vector or VEGFC into the striatum of WT mice and confirmed that tumor-associated meningeal lymphangiogenesis are enhanced by VEGFC, which facilitates transportation of DCs from brain tumors to deep cervical lymph nodes (dCLNs) through CCL21/CCR7 signaling pathway. VEGFC overexpression in tumors induces a stronger immune response followed by the application of anti-PD-1 or anti-CTLA-4 treatment, in which the tumors displayed decreased tumor volumes and tumor weight and showed longer survival compared to the Vector group in the mice (44). In addition, it has been demonstrated that the association of anti-angiogenic therapeutic regimen with DC vaccination suppressed glioma progression in rats via stimulating immune response, suppressing glioma stem-cell-like cell development and inhibiting angiogenesis-related protein expressions such as ICAM-1, VCAM and VEGFs (45).
Tumor-associated macrophages (TAMs) perform a considerable function in the immune microenvironment of cancer. In glioma, they are named as glioma-associated microglia and/or macrophages (GAMs). GAMs usually contain macrophages recruited from circulating monocytes and microglia which arises from myeloid progenitors and resides in the brain for a long time (46). GAMs exhibited significant diversity as well as plasticity and showed distinctive phenotypes, which are ascribed to inflammatory (M1) or alternative (M2) polarized secretory forms (47). M1-type macrophages can increase the number of activated NK cells, while M2-type macrophages not only can inhibit CD8+ T lymphocyte proliferation but also upregulate the inhibitory receptor expression of CTLA-4 and PD-1 (48). In a similar way, M2-type GAMs, the major type of GAM, resulted in tumor progression by producing anti-inflammatory cytokines and growth factors, which inhibit the host immune reaction (49). VEGFA/VEGFR1 is one of the most important ligand-receptor pairs associated with recruitment of monocytes/macrophages to form GAMs in a specific TME regions around glioma. Macrophages in gliomas were differentiated from VEGF producing monocytes. The number of macrophages was positively correlated with VEGF expression (50). Meanwhile, macrophages upregulate VEGFR1 expression and promote formation of GAMs (51). Under ischemia, hypoxia or in the absence of nutrients, VEGF expression are stimulated in macrophages in tumors (52). Through activation of PI3K/Akt/Nrf2 pathway in GBM cells, VEGF produced by M2 GAMs promotes GBM cell stemness, proliferation, epithelial-mesenchymal transition (EMT), and temozolomide resistance (51, 53). VEGF downregulation caused by mitochondrial damage in tumor cells resulted in an increased rate of M1/M2 macrophages both in vivo and in vitro, which enhances the TAM effects to strengthen the immunity to against tumors in the microenvironment (54).
Tregs which express VEGFR2 on their surfaces are highly suppressive, which play a vital role in immune tolerance to tumors (38). Both Treg recruitment and cytotoxic T lymphocyte (CTL) depletion can happen at the same time under the joint action of VEGF and VEGFR1/2 (55, 56). A study revealed that VEGFA blockade can enhance T-cell transfer therapy by increasing the amount of pmel-1 T cell infiltration in a melanoma mouse model (57). Bevacizumab, an anti-VEGF humanized murine monoclonal antibody, is effective in inhibiting GAMs and Tregs and increasing CTL infiltration, thereby restores the supportive immune microenvironment in glioblastoma (58). The relationship between VEGF and T cells in glioma is also reflected in the blood vessel normalization of VEGF blockade, which enhances the effectiveness of CAR-T therapy in glioblastoma models in mice (59). Moreover, VEGFRs peptide vaccination can activate CTLs which ultimately kill tumor cells, endothelial cells and Tregs expressing VEGFR1/2 in primary glioblastomas patients in clinical trial (60).
In summary, VEGFs in glioma are mostly acting as immunosuppressive factors in the microenvironment, which help tumor cells to avoid immune surveillance and immune cell killing. VEGFs possess regulatory effects in the glioma immune microenvironment involving inhibition of DC maturation, amassment of GAMs and controlling infiltration of T lymphocytes. It is worth noting that not all types of VEGFs cause immunosuppression in the glioma microenvironment. VEGFC, for example, can enhance radiotherapy efficacy and anti-tumor immunity in gliomas when combined with VEGFR2. This effect was ascribed to the CCL21-dependent CD8+ T cell activation and DC trafficking (61). Tumor-associated dendritic cells (TA-DCs) suppress anti-tumor immune responses, but when treated with inflammatory molecules, TA-DCs gain the ability to reactivate T cells (62).
3 SDF-1
Stromal-derived factor-1 (SDF-1), also referred to C-X-C motif ligand 12 (CXCL12), is a primitive and conserved chemokine (63). SDF-1 is an acidic protein that can be expressed under both physiological and pathological conditions by diverse cells such as bone marrow cells, epithelial cells, endothelial cells and tumor cells. It is currently believed that there are three SDF-1 isoforms named as SDF-1α/β/γ respectively, among which SDF-1α showed the strongest effects on vessel sprouting and permeability (64). The major receptor of SDF-1 is C-X-C Motif Chemokine Receptor 4 (CXCR4), belonging to the family of G protein-coupled receptor (GPCR) which traverses the plasma membrane seven times. CXCR4 is widely distributed in smooth muscle cell precursors, endothelium precursor cells, endothelial cells, immature and mature hematopoietic cells, and also astrocytes, microglia and neurons in the central nervous system (CNS) of adults (65, 66). SDF-1 directly participates in angiogenesis by recruiting endothelial progenitor cells through coupling to CXCR4 on endothelial cells (67). SDF-1 may also promote angiogenesis indirectly, by stimulating endothelial cells to secrete proangiogenic cytokines such as CXCL1, CXCL8 and VEGF (68–70).
In glioma, studies of SDF-1 functions in promoting angiogenesis are relatively comprehensive, whereas here we mainly discuss the role of SDF-1 in the glioma immune microenvironment and its direct effects on glioma progression. SDF-1 and CXCR4 were the most frequently expressed mRNA identified in 31 human astrocytic neoplasms (71). Enhanced SDF-1 and CXCR4 expression was observed not only in low-grade glioma such as oligoastrocytomas and astrogliomas, but also in areas of core necrosis and marginal infiltration of glioblastoma (71–75). CXCR4 is widely expressed on the surface of a variety of leukocytes, such as monocytes, macrophages, T cells, B cells, eosinophils and neutrophils. Thus, SDF-1 can participate in immune regulation such as leukocyte migration, leukocyte homing and lymphocyte recycling (65).
In the glioma microenvironment, SDF-1 is mainly derived from glioma cells and microglia and binds to CXCR4+ cells including microglia, macrophages, monocytes, endothelial cells and glioma cells themselves to initiate G-protein subunit dissociation, and the subsequent stimulation of mitogen-activated protein kinase (MAPK), phosphoinositide 3-kinase (PI3K) and phospholipase C (PLC) (63, 76–80). Independent of G proteins, it has been demonstrated that Janus kinase (JAK)2 and JAK3 pathways are activated by SDF-1-CXCR4, which allows the signal transducer and activator of transcription (STAT) molecule recruitment and activation to initiate transcription of multiple cancer-associated genes (80–83). A large number of aberrantly activated STATs, especially STAT3 and STAT5, were found to be actively involved in tumorigenesis, immune surveillance escaping and self-immunotolerance of tumor cells in glioma (83, 84).
Glioma cell released SDF-1 causes GAMs that expresses CXCR4 to polarize towards a M2-like phenotype which is anti-inflammatory and pro-tumorigenic (85). Specifically, a study on zebrafish showed that SDF-1β/CXCR-4b signaling is required for macrophage infiltration and microglia differentiation in the brain (86). As an important part of the glioma immune microenvironment, M2 type of GAMs are functioning in inhibiting T cells, promoting glioma cell EMT and invasion (85, 87, 88). When SDF-1 binds to macrophages or CD8+ T cells that express CXCR4, tumor necrosis factors (TNFs) and tumor necrosis factor receptors (TNF-Rs) will be secreted respectively to exert immunosuppressive function by delivering pro-apoptotic signals to T cells (89). In a syngeneic murine glioma model, both the number of Tregs and the expression of CXCR4 showed time-dependent upregulation, which may be one of the reasons for immune escape of glioma cells (90). The above processes result in local immunosuppression in the glioma microenvironment which leads to glioma progression ultimately. In glioma mice, combined therapy of anti-CXCR4 and anti-PD-1 reduced immunosuppressive leukocytes counts, advanced the CD4+/CD8+ T cell ratio and raised the amount of pro-inflammatory cytokines (91).
SDF-1 can directly promote glioma aggression. It is identified that SDF-1α activates focal adhesion kinase (FAK) and proline-rich tyrosine kinase 2 (Pyk2) signaling pathways to promote invadopodia formation which enhances glioma cell invasiveness by a study including 20 human glioblastoma specimens (76). In the optic pathway glioma, SDF-1 is expressed by numerous brain-derived cells including endothelial cells, entrapped axons and infiltrating microglia, which enhances optic glioma cell survival via CXCR4 receptor presented on glioma cells, whereas blocking CXCR4 inhibited glioma development in vivo (92, 93). Notably, the aggressiveness of the hepatocellular carcinoma is enhanced under the effect of ionizing radiation through increasing SDF-1 expression by epigenetic regulation (94). X-ray irradiation as a component of the normative therapeutic regimens for GBM (95) was frequently shown to enhance SDF-1 expression. Enlightening by this phenomenon, it would be of significance to clarify whether radiotherapy (RT) induces epigenetic regulation of the SDF-1 promoter to increase the expression, thereby increases glioblastoma invasiveness. Thus, attentions regarding to the potential disadvantages of RT need to be brought to clinicians and researchers, and individualized treatment by SDF-1 inhibition could be a potential solution.
CXCR4 and CXCR7 as receptors for SDF-1 are broadly involved in tumorigenesis (96). Dysregulated SDF-1-CXCR4/CXCR7 signaling has been observed in a diversity of tumor types involving gliomas (97). SDF-1-CXCR4/CXCR7 signaling shows both pro- and anti-inflammatory effects in tumors. On one hand, SDF-1 can mediate plasmacytoid DC trafficking to the tumor region and Tregs homing to the bone marrow microenvironment (98). On the other, it can promote the entry of immune cells with inhibitory functions like immature DC into the TME while rejecting immune effector cells (99). Current studies showed that Tregs with CXCR4 overexpression can be seen in several types of cancers which could explain why Tregs are recruited by SDF-1 at the site of tumorigenesis. However, this has not been confirmed in glioma. Upregulation of CXCR4 expression has also been seen in glioblastoma-associated T cells, but whether glioblastoma employ a similar mechanism to affect T cell infiltration remains unknown (100).
4 PDGF
The platelet-derived growth factor (PDGF) family contains four PDGFs (PDGFA, PDGFB, PDGFC and PDGFD) which forms five different disulphide-linked dimers (PDGF-AA, PDGF-BB, PDGF-CC, PDGF-DD and PDGF-AB) and two tyrosine kinases receptors (PDGF receptor α and β) (101). PDGF triggers the receptor kinase activity by driving the dimer formation from monomeric PDGFRs. PDGFRαα can be stimulated by all types of active ligand molecules except for PDGF-DD. PDGFRαβ can be stimulated by PDGF-AB, PDGF-BB and PDGF-CC whilst PDGFRββ can be stimulated by PDGF-BB or PDGF-DD (102). The activated PDGFRs initiates PI3K/AKT/mTOR, RAS/MAPK/ERK and JAK/STAT3 signaling pathways, which guide cells to survive, proliferate or migrate. Signaling is eventually terminated by internalization and degradation of the active PDGFR dimers (103). PDGFs/PDGFRs are expressed in various cell types under physiological conditions. In normal conditions, PDGFs are produced by megakaryocytes and preserved in platelets, and are released from disintegrated platelets when blood clots. PDGFs can also be secreted by diverse cells such as osteoblasts, fibroblasts, vascular smooth muscle cells, endothelial cells, glial cells and neurons (104). Different PDGF isoforms exert diverse functions. These functions include but are not limited to promoting organ development, wound healing and inducing macrophage recruitment (105). In tumors, PDGFs were shown to modulate tumor microenvironment, tumor growth and metastasis by targeting stromal cells, vascular endothelial cells and malignant cells (106). Human glioma expresses all PDGFs and PDGFRs. PDGFRα is found to be expressed mostly in glioma cells, whereas PDGFRβ is expressed mainly by the stromal cells (107).
PDGFs/PDGFRs are closely relevant to the physiopathological processes of glioma cells and tumor microenvironment. The interaction between PDGFs and GAMs promotes glioma cell migration as well as creating an immunosuppressive milieu of the TME. PDGFs were shown to increase monocyte and macrophage infiltration and promote inflammation in gliomas. In a mouse glioma model, high-grade glioma was developed in connection with intratumoral macrophages infiltration, in which mice PDGFB was expressed under the control of glioneuronal-specific nestin promoter (108). In another study, by immunohistochemical stainings on human pediatric high-grade gliomas (HGG) tissues, PDGFB/PDGFRβ was found to be strongly associated with IBA 1+, which suggested a possible role of the PDGF signaling in the infiltration of TAM (IBA 1+) (109). PDGFD was shown to promote neuroinflammation and enhances macrophage infiltration by an in vivo study of the mouse after intracerebral hemorrhage (110). Matrix metalloproteinases (MMPs) are important enzymes involved in monocytes/macrophages infiltration. PDGFC and PDGFD were showed to stimulate monocyte migration by expressing MMPs (MMP-2 and MMP-9) in an in vitro study (111). PDGFs released by microglia can also affect glioma cell migration. A study on glioma mouse model showed that M2-polarized microglia rather than M2-polarized bone marrow-derived macrophage is the reason for the elevated expression of PDGFRβ on glioma cells and their increased ability of migration (107). Furthermore, another study showed that PDGFB as well as SDF-1α released by microglia are key factors that may induce the formation of invadopodia and stimulate cell migration by activating Pyk2 and FAK kinases in human glioblastoma (76).
NK cells are known to kill tumor cells through several pathways, among which the most important way is to identify surface marker molecules on target cells and induce apoptosis through secretion of cytotoxins and cytokines. Recently an important study showed that PDGFD can inhibit tumor growth by inducing immunoreceptor tyrosine-based activation motif (ITAM) signaling via NKp44, a NK cell receptor, which leads to the generation of anti-tumoral factors from NK cells (112). Further study reported that PDGFD contributes to IL-15-mediated NK cell survival other than its effector function via PDGFRβindependently from NKp44 (113). A recent study has confirmed that the transcriptional profile of NK cells stimulated by PDGFD can predict a better prognosis of patients with low-grade glioma, suggesting that NK cells may be more conducive to against tumors under the action of PDGF (114). These studies indicate that PDGFD not only promotes tumor growth and stromal response but also activates innate immune systems in response to tumors. Therapeutics that selectively targeting PDGFD pathway in NK cells may provide a new aspect in the immunotherapies (113).
In addition, PDGF-AB is found to inhibit dendritic cell maturation by upregulating C-type lectin-like receptor 2 (CLEC2), which can further induce the formation of Tregs. PDGFB is shown to be associated with CD4+ T cell inhibition by inducing jumonji domain-containing protein 6 in patients with chronic hepatitis B infection (115).
At present, PDGF/PDGFR inhibitors have not achieved satisfactory results in the treatment of glioma (116, 117). Although forementioned evidence suggested that PDGFs play important roles in inflammatory cell activation and migration and some signaling pathways in certain cells have been explored, the specific mechanisms in the context of glioma remains largely unclear. Studies on how PDGFs/PDGFRs interacts with NK cells and regulatory T cells in glioma microenvironment may be worth to know.
5 TGF-β
Transforming growth factor-beta (TGF-β) indicates a large superfamily which contains three TGF-β isoforms (TGF-β1, -β2 and -β3), as well as Activins, Nodal, bone morphogenetic proteins (BMPs), the growth differentiation factors (GDFs) and the müllerian inhibiting substance (MIS) (118). As a multifunctional polypeptide cytokine, TGF-β plays important roles in angiogenesis, cell proliferation, wound healing and immune regulation by binding to a hetero-tetramer of TGF-β1 and TGF-β2 receptor serine/threonine kinases (119, 120). TGF-β is classified as AGFs because of its pro-angiogenic effect through stimulating endothelial cell and cancer-associated fibroblast proliferation, migration and sprouting both directly or indirectly (121, 122).
Hereby we mainly review the function of TGF-β in immunity specially in the glioma microenvironment. TGF-β effects as tumor inhibitors at the beginning but promotes tumor progression in the late stages of tumorigenesis (123). The increased TGF-β expression is associated with higher malignant degree and poorer prognosis of glioma patients (124, 125). TGF-β is able to affect the activity of immunocytes to regulate immune responses in the TME (126). In malignant gliomas, especially in GBM, TGF-β can be released by tumor cells, Tregs, M2-type GAM and myeloid-derived suppressor cells (MDSC) (127–129). TGF-β has been reported to inhibit the anti-tumor immunoreaction and modulate the properties of both GBM cells and the stromal cells in TME (129, 130). In glioma patients with different WHO grades, TGF-β1 and TGF-β2 were found to be the major TGF-β isoforms secreted by glioma cells which are able to downregulate cellular adhesion molecule (CAM) expression on GBM endothelial cells to prevent T cell infiltration (131). TGF-β2 has been observed to mitigate the recognition of glioma cells by CD4+ T lymphocytes through reducing the expression of HLA-DR antigen on human glioma cells (132). One study on GBM patients reported that TGF-β helps GBM cells to escape from the recognition of immune effector cells by downregulating the NKG2D which is one of the receptors expressed on NK cells and CD8+ T cells (133). In another study, TGF-β1 can inhibit the NK cell recognition and killing of glioblastoma stem cells through TGF-βR1/2 and the Smad2/3 phosphorylation in NK cells (127). In addition, in the presence of TGF-β1 in vitro, GAMs can be recruited and polarized to the M2-phonotype by Smad and PI3K/AKT signaling pathway (134, 135).
As a multifunctional cytokine, TGF-β supports tumor progression in general and contributes to generating an immunosuppressive microenvironment in glioma. Overexpression of TGF-β and its effects on anti-tumor immune responses by interacting with T cells and NK cells have been recognized in GBM (130). Notably, higher cytoplasmic TGF-β1 levels were found to be related to longer survival of patients with astrocytoma (136). This phenomenon may need to be considered when employing TGF-β related therapy on glioma patients.
6 FGF
Fibroblast growth factors (FGFs) were originally discovered and named for its function of promoting fibroblast growth. It is a family consists of twenty-two members (FGFs1–23), of which the mouse FGF15 is an ortholog of human FGF19 and ten of these factors were identified in the brain (137, 138). Similar to other growth factors, FGFs exert their effects through activation of four specific receptors named fibroblast growth factor receptor (FGFR) 1-4, which are receptor tyrosine kinases (RTKs) (139). FGFs are essential for embryonic development, cellular proliferation, angiogenesis, and tissue repair (140, 141). Thus, FGF family is also considered as AGFs. Overexpression of FGFs/FGFRs or continuous activation of FGFRs caused by chromosomal translocations has been found in a variety of tumors leading to up-regulation of downstream signaling involving NF-κB, STAT, RAS-MAPK, PI3K-AKT and phospholipase Cγ (PLCγ) pathways (142). Sustained activation of the above signaling pathways eventually leads to uncontrollable cell division, angiogenesis and EMT (143).
FGFs are differentially expressed in different types of tumors and play important roles through paracrine or endocrine signaling. In glioma tissues, higher levels of FGF1 and FGF2 were detected compared to normal brain tissues (144). It was reported that mutations of the FGFR1 kinase domain were found in human GBM tissue and the expression of FGFR1, FGFR3 and FGFR4 was increased in glioma (145–148). In contrast, FGFR2 expression is low or undetectable in high-grade astrocytomas whereas it is abundantly expressed in normal brain tissues (149). A recent study revealed that FGFR1 on macrophages are activated by glioma cell-derived FGF20, which inhibits β-catenin degradation through phosphorylating glycogen synthase kinase 3β (GSK3β) and subsequently suppresses macrophage polarization to the M1 phonotypes in vitro (150). In addition, studies show that FGF2 originated from TAMs in MC38 tumors plays a significant role for the transition of TAMs to a pro-tumorigenic M2 phenotype (151). FGF signaling pathway including FGFR1, FGFR4 and FGFR23 was shown to be involved in recruiting immunosuppressive cells, regulating M2 polarization of GAMs and T cell exhaustion in gliomas (152). These findings may serve as a basis for targeting FGF signaling to regulate immune response of gliomas. Nevertheless, the effects of other FGFs on the immune microenvironment of gliomas are currently unclear.
7 Angiopoietins
Angiopoietins (ANGs) are a group of angiogenic growth factors belonging to the angiopoietin family, which consists of ANG1-4, the vascular endothelial-protein tyrosine phosphatase (VE-PTP) and the associated receptor tyrosine kinase Tie1 and Tie2 which are mainly expressed on the surface of endothelial cells and hematopoietic cells (153–155). ANG1 and ANG2 are the most studied factors functioning in regulating vascular integrity in an opposite way (156). The ratio of ANG2/1 was increased in mesenchymal cells from GBM specimens compared to normal human cerebrovascular pericytes (157). Upregulation of ANG2 expression was observed in GBM mouse model (158). The abnormal expression of ANG2 is considered to be the other major cause besides VEGF for the massive heterogeneous angiogenesis happened in gliomas (159). In addition, ANG2 has been shown to promote cell proliferation, invasiveness and malignant transformation of gliomas both in vitro and in mouse models (160, 161). In the immune aspects, ANG2 is considered as immunosuppressive in tumors by recruiting MDSC, regulatory T cells and monocytes (162).
Based on the study by analyzing the TCGA cohort, ANG2 was shown to be involved in immune regulation in TME by interacting with neutrophils, macrophages, NK cells and mast cells (163). In some kinds of solid tumors, angiopoietins were reported to be associated with immune cells including TAMs, NK cells and neutrophils, but whether it effects in a similar way in the glioma microenvironment requires further confirmation (164, 165). Available evidences showed that Tie2 was expressed in immunocytes including DCs, TAMs, T lymphocytes, neutrophils and mast cells, combining with the findings that ANG2 was expressed aberrantly in gliomas, provide us a feasible theoretical basis for further studying the ANG and immune cell interactions in the glioma microenvironment (62, 166–169).
8 Concluding remarks and perspectives
Angiogenesis is one of the hallmarks of cancer (1). Gliomas, particularly high-grade gliomas including GBM, exhibits enhanced angiogenesis and immunosuppression (170, 171). Standard treatment fails to improve glioma patient survival in an efficient way, when combining with anti-angiogenic therapy, the clinical results were still unsatisfactory (172, 173). After a period of usage, resistance was shown in anti-angiogenic therapy which develops compensatory pathways to maintain glioma angiogenesis and growth (16, 174).
In recent years, a number of anti-vascular treatment regimens targeting AGFs or AGF receptors have been proposed in glioma. The therapeutic agents targeting VRGF are the mostly studied. Bevacizumab (BEV), the only VEGF-targeting drug approved by the US Food and Drug Administration (FDA) for GBM patients, has not been shown to improve overall survival (OS) in Phase III clinical trial unlike the results from the colorectal cancer trials which acquired significant effects in OS improvement (16, 175). As well, BEV also showed no significant effects in another Phase II clinical trial for patients with WHO grade 2 and grade 3 gliomas (176). Incidentally, the AGF receptors such as VEGFR, PDGFR, FGFR and TIE are all belong to the receptor tyrosine kinases (RTKs). Imatinib, which is the first kinase inhibitor received FDA approval in 2001 has been widely used in the treatment of leukemia and gastrointestinal tumors (177–179). Whereas, the efficacy of imatinib in the clinical treatment of glioma needs further validation (180–182). In addition, AMD3000 as a CXCR4 (SDF-1 receptor) antagonist, has been shown to have therapeutic potential in glioma in vivo and in vitro. It can ultimately improve the glioma immune microenvironment by reducing CXCR4+ monocyte myeloid-derived suppressor cell infiltration and inducing immunogenic cell death (183). Such therapies are often proposed to target tumor angiogenesis initially. Worth to note that due to the existence of blood-brain barrier, appropriate drug carriers could be considered to achieve better therapeutic effects when applying anti-AGFs and related therapy (184). Significantly, anti-AGFs or their corresponding receptors with combined therapeutic methods, for example immunotherapy, may be helpful for glioma treatment.
AGFs were significantly upregulated in gliomas (22). In the glioma microenvironment, there is evidence showing upregulations of VEGFs, SDF-1, PDGFs, TGF-β, FGFs and ANGs that we have reviewed, which lead to pathological effects such as tumor growth and dysfunction of blood vessels. Besides angiogenesis, the function of AGFs in tumor aggression is also reflected in immune regulation (115). Studies have shown that many of these AGFs can be the important causes of immunosuppression in the glioma microenvironment. Combination of anti-angiogenic drugs and immunotherapy has become one of the standard therapeutic regimens for multiple cancers (8–10). At present, the role of AGFs and their corresponding receptors in glioma immune microenvironment have not been comprehensively studied. Elucidating the interplay between AGFs and immunocytes in the glioma microenvironment can be a crucial step for improving the anti-glioma therapy. Here we summarize the AGF receptors expressed on immune cells and patient glioma cells, which could be of certain significance for the research of AGFs in the immune microenvironment of gliomas (Table 1).
With the deepening in neuroimmunology research, the effect of AGFs on immune cells in the glioma microenvironment attracted attentions (221). It is worth noting that TAM content varies in different types of gliomas, in which higher levels of TAM was detected in high-grade gliomas (versus low-grade), recurrent GBM (versus primary), and IDH-WT GBM (versus IDH-mutant) (222). Therefore, it is most likely that there is a certain correlation between the prognosis of glioma with TAM whose recruitment and function can be influenced by AGFs to some extent. We noticed that the AGF receptors expressed on microglia, as a type of resident and unique macrophages comprising 10% of brain cells in the CNS (223), are mostly the same as those expressed on macrophages. Nevertheless, we observed that there are more types of FGFRs on microglia than macrophages which may deserve further explorations. Up to now, researchers have refined the different origins of macrophages in the CNS to be monocyte-derived macrophage-derived TAMs (TAM-MDM) and microglia derived TAMs (TAM-MG). It has been found that the two types of cells tend to show different characteristics in distribution and function, which would be meaningful for the GAM study (222). In addition, it has been confirmed that mature myeloid-derived mast cells can enter the CNS from the periphery, which can be used as a prognostic factor for glioma patients. The mechanisms of how mast cells affect glioma immune microenvironment is still unclear (224–226). Worth to note, besides common immune cells, endothelial cells have also been confirmed to directly participate in immunosuppression in the microenvironment of glioma (227, 228).
In exploration of anti-angiogenic treatment, the effects on immune regulation should be considered. Besides the AGFs discussed in this review, it is worth mentioning that angiogenesis-associated factors such as sonic hedgehog (Shh), matrix metalloproteinases (MMPs) and microRNAs (miRNAs) may also play a part in the glioma immune microenvironment. Studies on the regulation of AGFs in the glioma immune microenvironment will help further understanding the disease and may reveal potential clinical treatment for gliomas.
Author contributions
ZG: Conceptualization, Writing – original draft, Writing – review & editing, Visualization. QZ: Visualization, Writing – original draft. WL: Supervision, Writing – review & editing. XJ: Supervision, Writing – review & editing, Conceptualization, Funding acquisition. YZ: Conceptualization, Funding acquisition, Supervision, Writing – review & editing, Project administration, Writing – original draft.
Funding
This work was supported by the National Natural Science Foundation of China (No. 82303425 and No. 82171458), Xijing Hospital (XJZT21CM07 and XJZT21J06), and Xi’an Science and Technology Bureau Fund (22YXYJ0153).
Acknowledgments
We would like to thank all the staff of the Department of Neurosurgery in Xijing Hospital for their support. Thanks to the foundations that we have been granted.
Conflict of interest
The authors declare that the research was conducted in the absence of any commercial or financial relationships that could be construed as a potential conflict of interest.
Publisher’s note
All claims expressed in this article are solely those of the authors and do not necessarily represent those of their affiliated organizations, or those of the publisher, the editors and the reviewers. Any product that may be evaluated in this article, or claim that may be made by its manufacturer, is not guaranteed or endorsed by the publisher.
References
1. Hanahan D. Hallmarks of cancer: new dimensions. Cancer Discovery (2022) 12(1):31–46. doi: 10.1158/2159-8290.Cd-21-1059
2. Singhal M, Augustin HG. Beyond angiogenesis: exploiting angiocrine factors to restrict tumor progression and metastasis. Cancer Res (2020) 80(4):659–62. doi: 10.1158/0008-5472.Can-19-3351
3. Zhang Q, Agoston AT, Pham TH, Zhang W, Zhang X, Huo X, et al. Acidic bile salts induce epithelial to mesenchymal transition via vegf signaling in non-neoplastic barrett's cells. Gastroenterology (2019) 156(1):130–44.e10. doi: 10.1053/j.gastro.2018.09.046
4. Oh SI, Jeong H, Park HS, Choi KA, Hwang I, Lee J, et al. Activation of cxcl12-cxcr4 signalling induces conversion of immortalised embryonic kidney cells into cancer stem-like cells. Artif cells nanomed Biotechnol (2020) 48(1):1303–13. doi: 10.1080/21691401.2020.1841783
5. Sisto M, Lisi S. Towards a unified approach in autoimmune fibrotic signalling pathways. Int J Mol Sci (2023) 24(10):9060. doi: 10.3390/ijms24109060
6. Rani V, Prabhu A. Combining angiogenesis inhibitors with radiation: advances and challenges in cancer treatment. Curr Pharm Des (2021) 27(7):919–31. doi: 10.2174/1381612826666201002145454
7. Kumar V, Stewart J. Immunometabolic reprogramming, another cancer hallmark. Front Immunol (2023) 14:1125874. doi: 10.3389/fimmu.2023.1125874
8. Hack SP, Zhu AX, Wang Y. Augmenting anticancer immunity through combined targeting of angiogenic and pd-1/pd-L1 pathways: challenges and opportunities. Front Immunol (2020) 11:598877. doi: 10.3389/fimmu.2020.598877
9. Salani F, Genovesi V, Vivaldi C, Massa V, Cesario S, Bernardini L, et al. Primary resistance to immunotherapy-based regimens in first line hepatocellular carcinoma: perspectives on jumping the hurdle. Cancers (Basel) (2022) 14(19):4896. doi: 10.3390/cancers14194896
10. Li L, Wen Q, Ding R. Therapeutic targeting of vegf and/or tgf-B to enhance anti-pd-(L)1 therapy: the evidence from clinical trials. Front Oncol (2022) 12:905520. doi: 10.3389/fonc.2022.905520
11. Schaff LR, Mellinghoff IK. Glioblastoma and other primary brain malignancies in adults: A review. Jama (2023) 329(7):574–87. doi: 10.1001/jama.2023.0023
12. Ostrom QT, Price M, Neff C, Cioffi G, Waite KA, Kruchko C, et al. Cbtrus statistical report: primary brain and other central nervous system tumors diagnosed in the United States in 2015-2019. Neuro-oncology (2022) 24(Suppl 5):v1–v95. doi: 10.1093/neuonc/noac202
13. Crivii CB, Boşca AB, Melincovici CS, Constantin AM, Mărginean M, Dronca E, et al. Glioblastoma microenvironment and cellular interactions. Cancers (Basel) (2022) 14(4):1092. doi: 10.3390/cancers14041092
14. Rosińska S, Gavard J. Tumor vessels fuel the fire in glioblastoma. Int J Mol Sci (2021) 22(12):6514. doi: 10.3390/ijms22126514
15. Tan AC, Ashley DM, López GY, Malinzak M, Friedman HS, Khasraw M. Management of glioblastoma: state of the art and future directions. CA: Cancer J Clin (2020) 70(4):299–312. doi: 10.3322/caac.21613
16. Pellerino A, Bruno F, Soffietti R, Rudà R. Antiangiogenic therapy for malignant brain tumors: does it still matter? Curr Oncol Rep (2023) 25(7):777–85. doi: 10.1007/s11912-023-01417-1
17. Srivastava R, Dodda M, Zou H, Li X, Hu B. Tumor niches: perspectives for targeted therapies in glioblastoma. Antioxid Redox Signal (2023). doi: 10.1089/ars.2022.0187
18. Yuile A, Wei JQ, Mohan AA, Hotchkiss KM, Khasraw M. Interdependencies of the neuronal, immune and tumor microenvironment in gliomas. Cancers (Basel) (2023) 15(10):2856. doi: 10.3390/cancers15102856
19. Castellani G, Croese T, Peralta Ramos JM, Schwartz M. Transforming the understanding of brain immunity. Sci (New York NY) (2023) 380(6640):eabo7649. doi: 10.1126/science.abo7649
20. Murota Y, Tabu K, Taga T. Cancer stem cell-associated immune microenvironment in recurrent glioblastomas. Cells (2022) 11(13):2054. doi: 10.3390/cells11132054
21. Rafii S, Kandoussi S, Ghouzlani A, Naji O, Reddy KP, Ullah Sadiqi R, et al. Deciphering immune microenvironment and cell evasion mechanisms in human gliomas. Front Oncol (2023) 13:1135430. doi: 10.3389/fonc.2023.1135430
22. Groblewska M, Mroczko B. Pro- and antiangiogenic factors in gliomas: implications for novel therapeutic possibilities. Int J Mol Sci (2021) 22(11):6126. doi: 10.3390/ijms22116126
23. Saker Z, Rizk M, Bahmad HF, Nabha SM. Targeting angiogenic factors for the treatment of medulloblastoma. Curr Treat Options Oncol (2022) 23(6):864–86. doi: 10.1007/s11864-022-00981-1
24. Bikfalvi A, da Costa CA, Avril T, Barnier JV, Bauchet L, Brisson L, et al. Challenges in glioblastoma research: focus on the tumor microenvironment. Trends Cancer (2023) 9(1):9–27. doi: 10.1016/j.trecan.2022.09.005
25. Jain S, Chalif EJ, Aghi MK. Interactions between anti-angiogenic therapy and immunotherapy in glioblastoma. Front Oncol (2021) 11:812916. doi: 10.3389/fonc.2021.812916
26. Melincovici CS, Boşca AB, Şuşman S, Mărginean M, Mihu C, Istrate M, et al. Vascular endothelial growth factor (Vegf) - key factor in normal and pathological angiogenesis. ROmanian J morphol embryol = Rev roumaine morphologie embryologie (2018) 59(2):455–67.
27. Simons M, Gordon E, Claesson-Welsh L. Mechanisms and regulation of endothelial vegf receptor signalling. Nat Rev Mol Cell Biol (2016) 17(10):611–25. doi: 10.1038/nrm.2016.87
28. Li P, Ferrara N. Vascular heterogeneity: vegf receptors make blood vessels special. J Exp Med (2022) 219(3):e20212539. doi: 10.1084/jem.20212539
29. Karaman S, Paavonsalo S, Heinolainen K, Lackman MH, Ranta A, Hemanthakumar KA, et al. Interplay of vascular endothelial growth factor receptors in organ-specific vessel maintenance. J Exp Med (2022) 219(3):e20210565. doi: 10.1084/jem.20210565
30. Geindreau M, Bruchard M, Vegran F. Role of cytokines and chemokines in angiogenesis in a tumor context. Cancers (Basel) (2022) 14(10):2446. doi: 10.3390/cancers14102446
31. Hoeres T, Wilhelm M, Smetak M, Holzmann E, Schulze-Tanzil G, Birkmann J. Immune cells regulate vegf signalling via release of vegf and antagonistic soluble vegf receptor-1. Clin Exp Immunol (2018) 192(1):54–67. doi: 10.1111/cei.13090
32. Ghalehbandi S, Yuzugulen J, Pranjol MZI, Pourgholami MH. The role of vegf in cancer-induced angiogenesis and research progress of drugs targeting vegf. Eur J Pharmacol (2023) 949:175586. doi: 10.1016/j.ejphar.2023.175586
33. Pang H, Dang X, Ren Y, Zhuang D, Qiu T, Chen H, et al. 3d-asl perfusion correlates with vegf expression and overall survival in glioma patients: comparison of quantitative perfusion and pathology on accurate spatial location-matched basis. J magnetic resonance Imaging JMRI (2019) 50(1):209–20. doi: 10.1002/jmri.26562
34. Tu Z, Wang C, Hu Q, Tao C, Fang Z, Lin L, et al. Protein disulfide-isomerase A4 confers glioblastoma angiogenesis promotion capacity and resistance to anti-angiogenic therapy. J Exp Clin Cancer Res CR (2023) 42(1):77. doi: 10.1186/s13046-023-02640-1
35. Szulzewsky F, Pelz A, Feng X, Synowitz M, Markovic D, Langmann T, et al. Glioma-associated microglia/macrophages display an expression profile different from M1 and M2 polarization and highly express gpnmb and spp1. PloS One (2015) 10(2):e0116644. doi: 10.1371/journal.pone.0116644
36. Biterge-Sut B. A comprehensive analysis of the angiogenesis-related genes in glioblastoma multiforme vs. brain lower grade glioma. Arquivos neuro-psiquiatria (2020) 78(1):34–8. doi: 10.1590/0004-282x20190131
37. Rao C, Jin J, Lu J, Wang C, Wu Z, Zhu Z, et al. A multielement prognostic nomogram based on a peripheral blood test, conventional mri and clinical factors for glioblastoma. Front Neurol (2022) 13:822735. doi: 10.3389/fneur.2022.822735
38. Suzuki H, Onishi H, Wada J, Yamasaki A, Tanaka H, Nakano K, et al. Vegfr2 is selectively expressed by foxp3high cd4+ Treg. Eur J Immunol (2010) 40(1):197–203. doi: 10.1002/eji.200939887
39. Yao X, Ping Y, Liu Y, Chen K, Yoshimura T, Liu M, et al. Vascular endothelial growth factor receptor 2 (Vegfr-2) plays a key role in vasculogenic mimicry formation, neovascularization and tumor initiation by glioma stem-like cells. PloS One (2013) 8(3):e57188. doi: 10.1371/journal.pone.0057188
40. Turkowski K, Brandenburg S, Mueller A, Kremenetskaia I, Bungert AD, Blank A, et al. Vegf as a modulator of the innate immune response in glioblastoma. Glia (2018) 66(1):161–74. doi: 10.1002/glia.23234
41. Gabrilovich DI, Chen HL, Girgis KR, Cunningham HT, Meny GM, Nadaf S, et al. Production of vascular endothelial growth factor by human tumors inhibits the functional maturation of dendritic cells. Nat Med (1996) 2(10):1096–103. doi: 10.1038/nm1096-1096
42. Dikov MM, Ohm JE, Ray N, Tchekneva EE, Burlison J, MoGhanaki D, et al. Differential roles of vascular endothelial growth factor receptors 1 and 2 in dendritic cell differentiation. J Immunol (Baltimore Md 1950) (2005) 174(1):215–22. doi: 10.4049/jimmunol.174.1.215
43. Mimura K, Kono K, Takahashi A, Kawaguchi Y, Fujii H. Vascular endothelial growth factor inhibits the function of human mature dendritic cells mediated by vegf receptor-2. Cancer immunol immunother CII (2007) 56(6):761–70. doi: 10.1007/s00262-006-0234-7
44. Hu X, Deng Q, Ma L, Li Q, Chen Y, Liao Y, et al. Meningeal lymphatic vessels regulate brain tumor drainage and immunity. Cell Res (2020) 30(3):229–43. doi: 10.1038/s41422-020-0287-8
45. Zhou X, Liao Y, Li H, Zhao Z, Liu Q. Dendritic cell vaccination enhances antiangiogenesis induced by endostatin in rat glioma. J Cancer Res Ther (2016) 12(1):198–203. doi: 10.4103/0973-1482.151430
46. Sampson JH, Gunn MD, Fecci PE, Ashley DM. Brain immunology and immunotherapy in brain tumours. Nat Rev Cancer (2020) 20(1):12–25. doi: 10.1038/s41568-019-0224-7
47. Richard SA. The pivotal immunoregulatory functions of microglia and macrophages in glioma pathogenesis and therapy. J Oncol (2022) 2022:8903482. doi: 10.1155/2022/8903482
48. Pan Y, Yu Y, Wang X, Zhang T. Tumor-associated macrophages in tumor immunity. Front Immunol (2020) 11:583084. doi: 10.3389/fimmu.2020.583084
49. Tamura R, Tanaka T, Yamamoto Y, Akasaki Y, Sasaki H. Dual role of macrophage in tumor immunity. Immunotherapy (2018) 10(10):899–909. doi: 10.2217/imt-2018-0006
50. Caverzán MD, Beaugé L, Oliveda PM, Cesca González B, Bühler EM, Ibarra LE. Exploring monocytes-macrophages in immune microenvironment of glioblastoma for the design of novel therapeutic strategies. Brain Sci (2023) 13(4):542. doi: 10.3390/brainsci13040542
51. Zhang G, Tao X, Ji B, Gong J. Hypoxia-driven M2-polarized macrophages facilitate cancer aggressiveness and temozolomide resistance in glioblastoma. Oxid Med Cell Longevity (2022) 2022:1614336. doi: 10.1155/2022/1614336
52. Colegio OR, Chu NQ, Szabo AL, Chu T, Rhebergen AM, Jairam V, et al. Functional polarization of tumour-associated macrophages by tumour-derived lactic acid. Nature (2014) 513(7519):559–63. doi: 10.1038/nature13490
53. Yang YP, Chien CS, Yarmishyn AA, Chan MS, Lee AC, Chen YW, et al. Musashi-1 regulates mif1-mediated M2 macrophage polarization in promoting glioblastoma progression. Cancers (Basel) (2021) 13(8):1799. doi: 10.3390/cancers13081799
54. Yao Y, Tao J, Lyu J, Chen C, Huang Y, Zhou Z. Enhance mitochondrial damage by nuclear export inhibition to suppress tumor growth and metastasis with increased antitumor properties of macrophages. ACS Appl Mater Interfaces (2023) 15(17):20774–87. doi: 10.1021/acsami.3c02305
55. Motz GT, Santoro SP, Wang LP, Garrabrant T, Lastra RR, Hagemann IS, et al. Tumor endothelium fasl establishes a selective immune barrier promoting tolerance in tumors. Nat Med (2014) 20(6):607–15. doi: 10.1038/nm.3541
56. Ishikawa E, Miyazaki T, Takano S, Akutsu H. Anti-angiogenic and macrophage-based therapeutic strategies for glioma immunotherapy. Brain tumor Pathol (2021) 38(3):149–55. doi: 10.1007/s10014-021-00402-5
57. ShriMali RK, Yu Z, Theoret MR, Chinnasamy D, Restifo NP, Rosenberg SA. Antiangiogenic agents can increase lymphocyte infiltration into tumor and enhance the effectiveness of adoptive immunotherapy of cancer. Cancer Res (2010) 70(15):6171–80. doi: 10.1158/0008-5472.Can-10-0153
58. Tamura R, Tanaka T, Ohara K, Miyake K, Morimoto Y, Yamamoto Y, et al. Persistent restoration to the immunosupportive tumor microenvironment in glioblastoma by bevacizumab. Cancer Sci (2019) 110(2):499–508. doi: 10.1111/cas.13889
59. Dong X, Ren J, Amoozgar Z, Lee S, Datta M, Roberge S, et al. Anti-vegf therapy improves egfr-viii-car-T cell delivery and efficacy in syngeneic glioblastoma models in mice. J immunother Cancer (2023) 11(3):e005583. doi: 10.1136/jitc-2022-005583
60. Tamura R, Morimoto Y, Kosugi K, Sato M, Oishi Y, Ueda R, et al. Clinical and histopathological analyses of vegf receptors peptide vaccine in patients with primary glioblastoma - a case series. BMC Cancer (2020) 20(1):196. doi: 10.1186/s12885-020-6589-x
61. Zhou C, Ma L, Xu H, Huo Y, Luo J. Meningeal lymphatics regulate radiotherapy efficacy through modulating anti-tumor immunity. Cell Res (2022) 32(6):543–54. doi: 10.1038/s41422-022-00639-5
62. Osterbur J, Sprague L, Muccioli M, Pate M, Mansfield K, McGinty J, et al. Adhesion to substrates induces dendritic cell endothelization and decreases immunological response. Immunobiology (2013) 218(1):64–75. doi: 10.1016/j.imbio.2012.02.003
63. Groblewska M, Litman-Zawadzka A, Mroczko B. The role of selected chemokines and their receptors in the development of gliomas. Int J Mol Sci (2020) 21(10):3704. doi: 10.3390/ijms21103704
64. Chang CW, Seibel AJ, Avendano A, Cortes-Medina MG, Song JW. Distinguishing specific cxcl12 isoforms on their angiogenesis and vascular permeability promoting properties. Advanced healthc mater (2020) 9(4):e1901399. doi: 10.1002/adhm.201901399
65. Teicher BA, Fricker SP. Cxcl12 (Sdf-1)/cxcr4 pathway in cancer. Clin Cancer Res (2010) 16(11):2927–31. doi: 10.1158/1078-0432.Ccr-09-2329
66. Bajetto A, Bonavia R, Barbero S, Piccioli P, Costa A, Florio T, et al. Glial and neuronal cells express functional chemokine receptor cxcr4 and its natural ligand stromal cell-derived factor 1. J Neurochem (1999) 73(6):2348–57. doi: 10.1046/j.1471-4159.1999.0732348.x
67. Ahn GO, Brown JM. Role of endothelial progenitors and other bone marrow-derived cells in the development of the tumor vasculature. Angiogenesis (2009) 12(2):159–64. doi: 10.1007/s10456-009-9135-7
68. Owen JL, Mohamadzadeh M. Macrophages and chemokines as mediators of angiogenesis. Front Physiol (2013) 4:159. doi: 10.3389/fphys.2013.00159
69. Liang Z, Brooks J, Willard M, Liang K, Yoon Y, Kang S, et al. Cxcr4/cxcl12 axis promotes vegf-mediated tumor angiogenesis through akt signaling pathway. Biochem Biophys Res Commun (2007) 359(3):716–22. doi: 10.1016/j.bbrc.2007.05.182
70. Singh AK, Arya RK, Trivedi AK, Sanyal S, Baral R, Dormond O, et al. Chemokine receptor trio: cxcr3, cxcr4 and cxcr7 crosstalk via cxcl11 and cxcl12. Cytokine Growth factor Rev (2013) 24(1):41–9. doi: 10.1016/j.cytogfr.2012.08.007
71. Bajetto A, Barbieri F, Dorcaratto A, Barbero S, Daga A, Porcile C, et al. Expression of cxc chemokine receptors 1-5 and their ligands in human glioma tissues: role of cxcr4 and sdf1 in glioma cell proliferation and migration. Neurochem Int (2006) 49(5):423–32. doi: 10.1016/j.neuint.2006.03.003
72. Calatozzolo C, Maderna E, Pollo B, Gelati M, Marras C, Silvani A, et al. Prognostic value of cxcl12 expression in 40 low-grade oligodendrogliomas and oligoastrocytomas. Cancer Biol Ther (2006) 5(7):827–32. doi: 10.4161/cbt.5.7.2838
73. Oh JW, Drabik K, Kutsch O, Choi C, Tousson A, Benveniste EN. Cxc chemokine receptor 4 expression and function in human astroglioma cells. J Immunol (Baltimore Md 1950) (2001) 166(4):2695–704. doi: 10.4049/jimmunol.166.4.2695
74. Rempel SA, Dudas S, Ge S, Gutiérrez JA. Identification and localization of the cytokine sdf1 and its receptor, cxc chemokine receptor 4, to regions of necrosis and angiogenesis in human glioblastoma. Clin Cancer Res (2000) 6(1):102–11.
75. Zagzag D, Esencay M, Mendez O, Yee H, Smirnova I, Huang Y, et al. Hypoxia- and vascular endothelial growth factor-induced stromal cell-derived factor-1alpha/cxcr4 expression in glioblastomas: one plausible explanation of Scherer's structures. Am J Pathol (2008) 173(2):545–60. doi: 10.2353/ajpath.2008.071197
76. Nuñez RE, del Valle MM, Ortiz K, Almodovar L, Kucheryavykh L. Microglial cytokines induce invasiveness and proliferation of human glioblastoma through pyk2 and fak activation. Cancers (Basel) (2021) 13(24):6160. doi: 10.3390/cancers13246160
77. Kioi M, Vogel H, Schultz G, Hoffman RM, Harsh GR, Brown JM. Inhibition of vasculogenesis, but not angiogenesis, prevents the recurrence of glioblastoma after irradiation in mice. J Clin Invest (2010) 120(3):694–705. doi: 10.1172/jci40283
78. Wang SC, Hong JH, Hsueh C, Chiang CS. Tumor-secreted sdf-1 promotes glioma invasiveness and tam tropism toward hypoxia in a murine astrocytoma model. Lab investigation; J Tech Methods Pathol (2012) 92(1):151–62. doi: 10.1038/labinvest.2011.128
79. Hughes CE, Nibbs RJB. A guide to chemokines and their receptors. FEBS J (2018) 285(16):2944–71. doi: 10.1111/febs.14466
80. Janssens R, Struyf S, Proost P. The unique structural and functional features of cxcl12. Cell Mol Immunol (2018) 15(4):299–311. doi: 10.1038/cmi.2017.107
81. Ahr B, Denizot M, Robert-Hebmann V, Brelot A, Biard-Piechaczyk M. Identification of the cytoplasmic domains of cxcr4 involved in jak2 and stat3 phosphorylation. J Biol Chem (2005) 280(8):6692–700. doi: 10.1074/jbc.M408481200
82. Vila-Coro AJ, Rodríguez-Frade JM, Martín De Ana A, Moreno-Ortíz MC, Martínez AC, Mellado M. The chemokine sdf-1alpha triggers cxcr4 receptor dimerization and activates the jak/stat pathway. FASEB J (1999) 13(13):1699–710. doi: 10.1096/fasebj.13.13.1699
83. Luwor RB, Stylli SS, Kaye AH. The role of stat3 in glioblastoma multiforme. J Clin Neurosci (2013) 20(7):907–11. doi: 10.1016/j.jocn.2013.03.006
84. Swiatek-MaChado K, Kaminska B. Stat signaling in glioma cells. Adv Exp Med Biol (2020) 1202:203–22. doi: 10.1007/978-3-030-30651-9_10
85. Roesch S, Rapp C, Dettling S, Herold-Mende C. When immune cells turn bad-tumor-associated microglia/macrophages in glioma. Int J Mol Sci (2018) 19(2):436. doi: 10.3390/ijms19020436
86. Chia K, Mazzolini J, Mione M, Sieger D. Tumor initiating cells induce cxcr4-mediated infiltration of pro-tumoral macrophages into the brain. Elife (2018) 7:e31918. doi: 10.7554/eLife.31918
87. Gjorgjevski M, Hannen R, Carl B, Li Y, Landmann E, Buchholz M, et al. Molecular profiling of the tumor microenvironment in glioblastoma patients: correlation of microglia/macrophage polarization state with metalloprotease expression profiles and survival. Biosci Rep (2019) 39(6):BSR20182361. doi: 10.1042/bsr20182361
88. Walentynowicz KA, Ochocka N, Pasierbinska M, Wojnicki K, Stepniak K, Mieczkowski J, et al. In search for reliable markers of glioma-induced polarization of microglia. Front Immunol (2018) 9:1329. doi: 10.3389/fimmu.2018.01329
89. Gagliardi F, Narayanan A, Reni M, Franzin A, Mazza E, Boari N, et al. The role of cxcr4 in highly malignant human gliomas biology: current knowledge and future directions. Glia (2014) 62(7):1015–23. doi: 10.1002/glia.22669
90. Grauer OM, Nierkens S, Bennink E, Toonen LW, Boon L, Wesseling P, et al. Cd4+Foxp3+ Regulatory T cells gradually accumulate in gliomas during tumor growth and efficiently suppress antiglioma immune responses in vivo. Int J Cancer (2007) 121(1):95–105. doi: 10.1002/ijc.22607
91. Wu A, Maxwell R, Xia Y, Cardarelli P, Oyasu M, Belcaid Z, et al. Combination anti-cxcr4 and anti-pd-1 immunotherapy provides survival benefit in glioblastoma through immune cell modulation of tumor microenvironment. J neuro-oncol (2019) 143(2):241–9. doi: 10.1007/s11060-019-03172-5
92. Warrington NM, Gianino SM, Jackson E, Goldhoff P, Garbow JR, Piwnica-Worms D, et al. Cyclic amp suppression is sufficient to induce gliomagenesis in a mouse model of neurofibromatosis-1. Cancer Res (2010) 70(14):5717–27. doi: 10.1158/0008-5472.Can-09-3769
93. Codrici E, Popescu I-D, Tanase C, Enciu A-M. Friends with benefits: chemokines, glioblastoma-associated microglia/macrophages, and tumor microenvironment. Int J Mol Sci (2022) 23(5):2509. doi: 10.3390/ijms23052509
94. Ahn HJ, Hwang SY, Nguyen NH, Lee IJ, Lee EJ, Seong J, et al. Radiation-induced cxcl12 upregulation via histone modification at the promoter in the tumor microenvironment of hepatocellular carcinoma. Mol Cells (2019) 42(7):530–45. doi: 10.14348/molcells.2019.2280
95. Kazda T, Dziacky A, Burkon P, Pospisil P, Slavik M, Rehak Z, et al. Radiotherapy of glioblastoma 15 years after the landmark stupp's trial: more controversies than standards? Radiol Oncol (2018) 52(2):121–8. doi: 10.2478/raon-2018-0023
96. Fan H, Wang W, Yan J, Xiao L, Yang L. Prognostic significance of cxcr7 in cancer patients: A meta-analysis. Cancer Cell Int (2018) 18:212. doi: 10.1186/s12935-018-0702-0
97. Santagata S, Ieranò C, Trotta AM, Capiluongo A, Auletta F, Guardascione G, et al. Cxcr4 and cxcr7 signaling pathways: A focus on the cross-talk between cancer cells and tumor microenvironment. Front Oncol (2021) 11:591386. doi: 10.3389/fonc.2021.591386
98. Nagarsheth N, Wicha MS, Zou W. Chemokines in the cancer microenvironment and their relevance in cancer immunotherapy. Nat Rev Immunol (2017) 17(9):559–72. doi: 10.1038/nri.2017.49
99. Portella L, Bello AM, Scala S. Cxcl12 signaling in the tumor microenvironment. Adv Exp Med Biol (2021) 1302:51–70. doi: 10.1007/978-3-030-62658-7_5
100. Kollis PM, Ebert LM, Toubia J, Bastow CR, Ormsby RJ, Poonnoose SI, et al. Characterising distinct migratory profiles of infiltrating T-cell subsets in human glioblastoma. Front Immunol (2022) 13:850226. doi: 10.3389/fimmu.2022.850226
101. Fredriksson L, Li H, Eriksson U. The pdgf family: four gene products form five dimeric isoforms. Cytokine Growth factor Rev (2004) 15(4):197–204. doi: 10.1016/j.cytogfr.2004.03.007
102. Heldin CH, Lennartsson J, Westermark B. Involvement of platelet-derived growth factor ligands and receptors in tumorigenesis. J Internal Med (2018) 283(1):16–44. doi: 10.1111/joim.12690
104. Demoulin JB, Montano-Almendras CP. Platelet-derived growth factors and their receptors in normal and malignant hematopoiesis. Am J Blood Res (2012) 2(1):44–56.
105. Roskoski R Jr. The role of small molecule platelet-derived growth factor receptor (Pdgfr) inhibitors in the treatment of neoplastic disorders. Pharmacol Res (2018) 129:65–83. doi: 10.1016/j.phrs.2018.01.021
106. Cao Y. Multifarious functions of pdgfs and pdgfrs in tumor growth and metastasis. Trends Mol Med (2013) 19(8):460–73. doi: 10.1016/j.molmed.2013.05.002
107. Wallmann T, Zhang XM, Wallerius M, Bolin S, Joly AL, Sobocki C, et al. Microglia induce pdgfrb expression in glioma cells to enhance their migratory capacity. iScience (2018) 9:71–83. doi: 10.1016/j.isci.2018.10.011
108. Kong LY, Wu AS, Doucette T, Wei J, Priebe W, Fuller GN, et al. Intratumoral mediated immunosuppression is prognostic in genetically engineered murine models of glioma and correlates to immunotherapeutic responses. Clin Cancer Res (2010) 16(23):5722–33. doi: 10.1158/1078-0432.Ccr-10-1693
109. Ross JL, Chen Z, Herting CJ, Grabovska Y, Szulzewsky F, Puigdelloses M, et al. Platelet-derived growth factor beta is a potent inflammatory driver in paediatric high-grade glioma. Brain J Neurol (2021) 144(1):53–69. doi: 10.1093/brain/awaa382
110. Yang P, Manaenko A, Xu F, Miao L, Wang G, Hu X, et al. Role of pdgf-D and pdgfr-B in neuroinflammation in experimental ich mice model. Exp Neurol (2016) 283(Pt A):157–64. doi: 10.1016/j.expneurol.2016.06.010
111. Wågsäter D, Zhu C, Björck HM, Eriksson P. Effects of pdgf-C and pdgf-D on monocyte migration and mmp-2 and mmp-9 expression. Atherosclerosis (2009) 202(2):415–23. doi: 10.1016/j.atherosclerosis.2008.04.050
112. Barrow AD, Edeling MA, Trifonov V, Luo J, Goyal P, Bohl B, et al. Natural killer cells control tumor growth by sensing a growth factor. Cell (2018) 172(3):534–48.e19. doi: 10.1016/j.cell.2017.11.037
113. Ma S, Tang T, Wu X, Mansour AG, Lu T, Zhang J, et al. Pdgf-D-pdgfrβ Signaling enhances il-15-mediated human natural killer cell survival. Proc Natl Acad Sci United States America (2022) 119(3):e2114134119. doi: 10.1073/pnas.2114134119
114. Sun Y, Sedgwick AJ, Palarasah Y, Mangiola S, Barrow AD. A transcriptional signature of pdgf-dd activated natural killer cells predicts more favorable prognosis in low-grade glioma. Front Immunol (2021) 12:668391. doi: 10.3389/fimmu.2021.668391
115. Khan KA, Kerbel RS. Improving immunotherapy outcomes with anti-angiogenic treatments and vice versa. Nat Rev Clin Oncol (2018) 15(5):310–24. doi: 10.1038/nrclinonc.2018.9
116. Batchelor TT, Gerstner ER, Ye X, Desideri S, Duda DG, Peereboom D, et al. and phase ii studies of tandutinib, an oral platelet-derived growth factor receptor-B Tyrosine kinase inhibitor, in patients with recurrent glioblastoma. Neuro-oncology (2016) 19(4):567–75. doi: 10.1093/neuonc/now185
117. Brar HK, Jose J, Wu Z, Sharma M. Tyrosine kinase inhibitors for glioblastoma multiforme: challenges and opportunities for drug delivery. Pharmaceutics (2022) 15(1):59. doi: 10.3390/pharmaceutics15010059
118. Tzavlaki K, Moustakas A. Tgf-B Signaling. Biomolecules (2020) 10(3):487. doi: 10.3390/biom10030487
119. Chen PY, Qin L, Simons M. Tgfβ Signaling pathways in human health and disease. Front Mol Biosci (2023) 10:1113061. doi: 10.3389/fmolb.2023.1113061
120. Birch JL, Coull BJ, Spender LC, Watt C, Willison A, Syed N, et al. Multifaceted transforming growth factor-beta (Tgfβ) signalling in glioblastoma. Cell signal (2020) 72:109638. doi: 10.1016/j.cellsig.2020.109638
121. Ali S, Rehman MU, Yatoo AM, Arafah A, Khan A, Rashid S, et al. Tgf-B Signaling pathway: therapeutic targeting and potential for anti-cancer immunity. Eur J Pharmacol (2023) 947:175678. doi: 10.1016/j.ejphar.2023.175678
122. Liu S, Ren J, Ten Dijke P. Targeting tgfβ Signal transduction for cancer therapy. Signal Transduct Target Ther (2021) 6(1):8. doi: 10.1038/s41392-020-00436-9
123. Villar VH, Subotički T, Đikić D, Mitrović-Ajtić O, Simon F, Santibanez JF. Transforming growth factor-B1 in cancer immunology: opportunities for immunotherapy. Adv Exp Med Biol (2023) 1408:309–28. doi: 10.1007/978-3-031-26163-3_17
124. Bruna A, Darken RS, Rojo F, Ocaña A, Peñuelas S, Arias A, et al. High tgfbeta-smad activity confers poor prognosis in glioma patients and promotes cell proliferation depending on the methylation of the pdgf-B gene. Cancer Cell (2007) 11(2):147–60. doi: 10.1016/j.ccr.2006.11.023
125. Kaminska B, Cyranowski S. Recent advances in understanding mechanisms of tgf beta signaling and its role in glioma pathogenesis. Adv Exp Med Biol (2020) 1202:179–201. doi: 10.1007/978-3-030-30651-9_9
126. Chan MK, Chan EL, Ji ZZ, Chan AS, Li C, Leung KT, et al. Transforming growth factor-B Signaling: from tumor microenvironment to anticancer therapy. Explor targeted anti-tumor Ther (2023) 4(2):316–43. doi: 10.37349/etat.2023.00137
127. Shaim H, Shanley M, Basar R, Daher M, Gumin J, Zamler DB, et al. Targeting the Av integrin/tgf-B Axis improves natural killer cell function against glioblastoma stem cells. J Clin Invest (2021) 131(14):e142116. doi: 10.1172/jci142116
128. Liu S, Zhang C, Wang B, Zhang H, Qin G, Li C, et al. Regulatory T cells promote glioma cell stemness through tgf-B-nf-Kb-il6-stat3 signaling. Cancer immunol immunother CII (2021) 70(9):2601–16. doi: 10.1007/s00262-021-02872-0
129. Gong L, Ji L, Xu D, Wang J, Zou J. Tgf-B Links glycolysis and immunosuppression in glioblastoma. Histol Histopathol (2021) 36(11):1111–24. doi: 10.14670/hh-18-366
130. Nana AW, Yang PM, Lin HY. Overview of transforming growth factor B Superfamily involvement in glioblastoma initiation and progression. Asian Pacific J Cancer Prev APJCP (2015) 16(16):6813–23. doi: 10.7314/apjcp.2015.16.16.6813
131. Lohr J, Ratliff T, Huppertz A, Ge Y, Dictus C, Ahmadi R, et al. Effector T-cell infiltration positively impacts survival of glioblastoma patients and is impaired by tumor-derived tgf-B. Clin Cancer Res (2011) 17(13):4296–308. doi: 10.1158/1078-0432.Ccr-10-2557
132. Zuber P, Kuppner MC, De Tribolet N. Transforming growth factor-beta 2 down-regulates hla-dr antigen expression on human malignant glioma cells. Eur J Immunol (1988) 18(10):1623–6. doi: 10.1002/eji.1830181023
133. Crane CA, Han SJ, Barry JJ, Ahn BJ, Lanier LL, Parsa AT. Tgf-beta downregulates the activating receptor nkg2d on nk cells and cd8+ T cells in glioma patients. Neuro-oncology (2010) 12(1):7–13. doi: 10.1093/neuonc/nop009
134. Wu A, Wei J, Kong LY, Wang Y, Priebe W, Qiao W, et al. Glioma cancer stem cells induce immunosuppressive macrophages/microglia. Neuro-oncology (2010) 12(11):1113–25. doi: 10.1093/neuonc/noq082
135. Li D, Zhang Q, Li L, Chen K, Yang J, Dixit D, et al. B2-microglobulin maintains glioblastoma stem cells and induces M2-like polarization of tumor-associated macrophages. Cancer Res (2022) 82(18):3321–34. doi: 10.1158/0008-5472.Can-22-0507
136. Mabrouk GM, Ali EM, El-Rehany MA, El-Samoly HM. Tgf-beta1, tnf-alpha and cytochrome C in human astrocytic tumors: A short-term follow up and correlation with survival. Clin Biochem (2007) 40(3-4):255–60. doi: 10.1016/j.clinbiochem.2006.09.009
137. Angelin B, Larsson TE, Rudling M. Circulating fibroblast growth factors as metabolic regulators–a critical appraisal. Cell Metab (2012) 16(6):693–705. doi: 10.1016/j.cmet.2012.11.001
138. Reuss B, von Bohlen und Halbach O. Fibroblast growth factors and their receptors in the central nervous system. Cell Tissue Res (2003) 313(2):139–57. doi: 10.1007/s00441-003-0756-7
139. Szymczyk J, Sluzalska KD, Materla I, Opalinski L, Otlewski J, Zakrzewska M. Fgf/fgfr-dependent molecular mechanisms underlying anti-cancer drug resistance. Cancers (Basel) (2021) 13(22):5796. doi: 10.3390/cancers13225796
140. Lu J, Jiang L, Chen Y, Lyu K, Zhu B, Li Y, et al. The functions and mechanisms of basic fibroblast growth factor in tendon repair. Front Physiol (2022) 13:852795. doi: 10.3389/fphys.2022.852795
141. Farooq M, Khan AW, Kim MS, Choi S. The role of fibroblast growth factor (Fgf) signaling in tissue repair and regeneration. Cells (2021) 10(11):3242. doi: 10.3390/cells10113242
142. Jing Q, Wang Y, Liu H, Deng X, Jiang L, Liu R, et al. Fgfs: crucial factors that regulate tumour initiation and progression. Cell proliferation (2016) 49(4):438–47. doi: 10.1111/cpr.12275
143. Chen L, Zhang Y, Yin L, Cai B, Huang P, Li X, et al. Fibroblast growth factor receptor fusions in cancer: opportunities and challenges. J Exp Clin Cancer Res CR (2021) 40(1):345. doi: 10.1186/s13046-021-02156-6
144. Klimaschewski L, Claus P. Fibroblast growth factor signalling in the diseased nervous system. Mol Neurobiol (2021) 58(8):3884–902. doi: 10.1007/s12035-021-02367-0
145. Rand V, Huang J, Stockwell T, Ferriera S, Buzko O, Levy S, et al. Sequence survey of receptor tyrosine kinases reveals mutations in glioblastomas. PNAS (2005) 102(40):14344–9. doi: 10.1073/pnas.0507200102
146. Yamaguchi F, Saya H, Bruner JM, Morrison RS. Differential expression of two fibroblast growth factor-receptor genes is associated with malignant progression in human astrocytomas. Proc Natl Acad Sci United States America (1994) 91(2):484–8. doi: 10.1073/pnas.91.2.484
147. Jimenez-Pascual A, Mitchell K, Siebzehnrubl FA, Lathia JD. Fgf2: A novel druggable target for glioblastoma? Expert Opin Ther Targets (2020) 24(4):311–8. doi: 10.1080/14728222.2020.1736558
148. Gabler L, Jaunecker CN, Katz S, van Schoonhoven S, Englinger B, Pirker C, et al. Fibroblast growth factor receptor 4 promotes glioblastoma progression: A central role of integrin-mediated cell invasiveness. Acta neuropathologica Commun (2022) 10(1):65. doi: 10.1186/s40478-022-01363-2
149. Ohashi R, Matsuda Y, Ishiwata T, Naito Z. Downregulation of fibroblast growth factor receptor 2 and its isoforms correlates with a high proliferation rate and poor prognosis in high-grade glioma. Oncol Rep (2014) 32(3):1163–9. doi: 10.3892/or.2014.3283
150. Cai X, Tao W, Li L. Glioma cell-derived fgf20 suppresses macrophage function by activating B-catenin. Cell signal (2022) 89:110181. doi: 10.1016/j.cellsig.2021.110181
151. Im JH, Buzzelli JN, Jones K, Franchini F, Gordon-Weeks A, Markelc B, et al. Fgf2 alters macrophage polarization, tumour immunity and growth and can be targeted during radiotherapy. Nat Commun (2020) 11(1):4064. doi: 10.1038/s41467-020-17914-x
152. Su J, Ma Q, Long W, Tang H, Wu C, Luo M, et al. Lctl is a prognostic biomarker and correlates with stromal and immune infiltration in gliomas. Front Oncol (2019) 9:1083. doi: 10.3389/fonc.2019.01083
153. Akwii RG, Mikelis CM. Targeting the angiopoietin/tie pathway: prospects for treatment of retinal and respiratory disorders. Drugs (2021) 81(15):1731–49. doi: 10.1007/s40265-021-01605-y
154. Chowdhury TA, Koceja C, Eisa-Beygi S, Kleinstiver BP, Kumar SN, Lin CW, et al. Temporal and spatial post-transcriptional regulation of zebrafish tie1 mrna by long noncoding rna during brain vascular assembly. Arterioscler Thromb Vasc Biol (2018) 38(7):1562–75. doi: 10.1161/atvbaha.118.310848
155. Thomas M, Augustin HG. The role of the angiopoietins in vascular morphogenesis. Angiogenesis (2009) 12(2):125–37. doi: 10.1007/s10456-009-9147-3
156. Hu B, Cheng SY. Angiopoietin-2: development of inhibitors for cancer therapy. Curr Oncol Rep (2009) 11(2):111–6. doi: 10.1007/s11912-009-0017-3
157. Balaziova E, Vymola P, Hrabal P, Mateu R, Zubal M, Tomas R, et al. Fibroblast activation protein expressing mesenchymal cells promote glioblastoma angiogenesis. Cancers (Basel) (2021) 13(13):3304. doi: 10.3390/cancers13133304
158. Leiss L, Mutlu E, Øyan A, Yan T, Tsinkalovsky O, Sleire L, et al. Tumour-associated glial host cells display a stem-like phenotype with a distinct gene expression profile and promote growth of gbm xenografts. BMC Cancer (2017) 17(1):108. doi: 10.1186/s12885-017-3109-8
159. Wang F, Li C, Han F, Chen L, Zhu L. Bmal1 may be involved in angiogenesis and peritumoral cerebral edema of human glioma by regulating vegf and ang2. Aging (2021) 13(22):24675–85. doi: 10.18632/aging.203708
160. Qi L, Wang ZY, Shao XR, Li M, Chen SN, Liu XQ, et al. Isl2 modulates angiogenesis through transcriptional regulation of angpt2 to promote cell proliferation and malignant transformation in oligodendroglioma. Oncogene (2020) 39(37):5964–78. doi: 10.1038/s41388-020-01411-y
161. Kawashima T, Yashiro M, Kasashima H, Terakawa Y, Uda T, Nakajo K, et al. Oligodendrocytes up-regulate the invasive activity of glioblastoma cells via the angiopoietin-2 signaling pathway. Anticancer Res (2019) 39(2):577–84. doi: 10.21873/anticanres.13150
162. Fukumura D, Kloepper J, Amoozgar Z, Duda DG, Jain RK. Enhancing cancer immunotherapy using antiangiogenics: opportunities and challenges. Nat Rev Clin Oncol (2018) 15(5):325–40. doi: 10.1038/nrclinonc.2018.29
163. Yang S, Zou X, Li J, Yang H, Zhang A, Zhu Y, et al. Immunoregulation and clinical significance of neutrophils/nets-angpt2 in tumor microenvironment of gastric cancer. Front Immunol (2022) 13:1010434. doi: 10.3389/fimmu.2022.1010434
164. Park J, Kim JT, Lee SJ, Kim JC. The anti-inflammatory effects of angiogenin in an endotoxin induced uveitis in rats. Int J Mol Sci (2020) 21(2):413. doi: 10.3390/ijms21020413
165. Sheng J, Xu Z. Three decades of research on angiogenin: A review and perspective. Acta Biochim Biophys Sin (2016) 48(5):399–410. doi: 10.1093/abbs/gmv131
166. Cassetta L, Pollard JW. A timeline of tumour-associated macrophage biology. Nat Rev Cancer (2023) 23(4):238–57. doi: 10.1038/s41568-022-00547-1
167. Park HR, Shiva A, Cummings P, Kim S, Kim S, Lee E, et al. Angiopoietin-2-dependent spatial vascular destabilization promotes T-cell exclusion and limits immunotherapy in melanoma. Cancer Res (2023) 83(12):1968–83. doi: 10.1158/0008-5472.Can-22-2838
168. Lemieux C, Maliba R, Favier J, Théorêt JF, Merhi Y, Sirois MG. Angiopoietins can directly activate endothelial cells and neutrophils to promote proinflammatory responses. Blood (2005) 105(4):1523–30. doi: 10.1182/blood-2004-09-3531
169. Brunckhorst MK, Wang H, Lu R, Yu Q. Angiopoietin-4 promotes glioblastoma progression by enhancing tumor cell viability and angiogenesis. Cancer Res (2010) 70(18):7283–93. doi: 10.1158/0008-5472.Can-09-4125
170. Ni L, Sun P, Zhang S, Qian B, Chen X, Xiong M, et al. Transcriptome and single-cell analysis reveal the contribution of immunosuppressive microenvironment for promoting glioblastoma progression. Front Immunol (2022) 13:1051701. doi: 10.3389/fimmu.2022.1051701
171. Muir M, Gopakumar S, Traylor J, Lee S, Rao G. Glioblastoma multiforme: novel therapeutic targets. Expert Opin Ther Targets (2020) 24(7):605–14. doi: 10.1080/14728222.2020.1762568
172. Xiao Q, Yang S, Ding G, Luo M. Anti-vascular endothelial growth factor in glioblastoma: A systematic review and meta-analysis. Neurological Sci (2018) 39(12):2021–31. doi: 10.1007/s10072-018-3568-y
173. Wick W, Gorlia T, Bendszus M, Taphoorn M, Sahm F, Harting I, et al. Lomustine and bevacizumab in progressive glioblastoma. New Engl J Med (2017) 377(20):1954–63. doi: 10.1056/NEJMoa1707358
174. Shamshiripour P, Hajiahmadi F, Lotfi S, Esmaeili NR, Zare A, Akbarpour M, et al. Next-generation anti-angiogenic therapies as a future prospect for glioma immunotherapy; from bench to bedside. Front Immunol (2022) 13:859633. doi: 10.3389/fimmu.2022.859633
175. Prager GW, Taieb J, Fakih M, Ciardiello F, Van Cutsem E, Elez E, et al. Trifluridine-tipiracil and bevacizumab in refractory metastatic colorectal cancer. New Engl J Med (2023) 388(18):1657–67. doi: 10.1056/NEJMoa2214963
176. Reijneveld JC, Machingura A, Coens C, Taphoorn MJB, Taal W, Clement PM, et al. Health-Related Quality-of-Life Results from the Randomised Phase Ii Tavarec Trial on Temozolomide with or without Bevacizumab in 1p/19q Intact First-Recurrence World Health Organization grade 2 and 3 Glioma (European Organization for Research and Treatment of Cancer 26091). Eur J Cancer (2023) 190:112946. doi: 10.1016/j.ejca.2023.112946
177. Cohen P, Cross D, Jänne PA. Kinase drug discovery 20 years after imatinib: progress and future directions. Nat Rev Drug Discovery (2021) 20(7):551–69. doi: 10.1038/s41573-021-00195-4
178. Senapati J, Sasaki K, Issa GC, Lipton JH, Radich JP, Jabbour E, et al. Management of chronic myeloid leukemia in 2023 - common ground and common sense. Blood Cancer J (2023) 13(1):58. doi: 10.1038/s41408-023-00823-9
179. Li B, Chen H, Yang S, Chen F, Xu L, Li Y, et al. Advances in immunology and immunotherapy for mesenchymal gastrointestinal cancers. Mol Cancer (2023) 22(1):71. doi: 10.1186/s12943-023-01770-6
180. Sautter L, Hofheinz R, Tuettenberg J, Grimm M, Vajkoczy P, Groden C, et al. Open-label phase ii evaluation of imatinib in primary inoperable or incompletely resected and recurrent glioblastoma. Oncology (2020) 98(1):16–22. doi: 10.1159/000502483
181. Lu J, Hu Y, Qian R, Zhang Y, Yang X, Luo P. Enhanced proliferation inhibition and apoptosis in glioma cells elicited by combination of irinotecan and imatinib. Eur J Pharmacol (2020) 874:173022. doi: 10.1016/j.ejphar.2020.173022
182. Jaeckle KA, Anderson SK, Twohy EL, Dixon JG, Giannini C, Jenkins R, et al. Phase I-ii trial of imatinib mesylate (Gleevec; sti571) in treatment of recurrent oligodendroglioma and mixed oligoastrocytoma. North central cancer treatment group study N0272 (Alliance/Ncctg). J neuro-oncol (2019) 143(3):573–81. doi: 10.1007/s11060-019-03194-z
183. Alghamri MS, Banerjee K, Mujeeb AA, Mauser A, Taher A, Thalla R, et al. Systemic delivery of an adjuvant cxcr4-cxcl12 signaling inhibitor encapsulated in synthetic protein nanoparticles for glioma immunotherapy. ACS nano (2022) 16(6):8729–50. doi: 10.1021/acsnano.1c07492
184. Lu J, Gao X, Wang S, He Y, Ma X, Zhang T, et al. Advanced strategies to evade the mononuclear phagocyte system clearance of nanomaterials. Exploration (2023) 3(1):20220045. doi: 10.1002/exp.20220045
185. Hamrah P, Chen L, Cursiefen C, Zhang Q, Joyce NC, Dana MR. Expression of vascular endothelial growth factor receptor-3 (Vegfr-3) on monocytic bone marrow-derived cells in the conjunctiva. Exp eye Res (2004) 79(4):553–61. doi: 10.1016/j.exer.2004.06.028
186. Tian Z, Zhang P, Li X, Jiang D. Analysis of immunogenic cell death in ascending thoracic aortic aneurysms based on single-cell sequencing data. Front Immunol (2023) 14:1087978. doi: 10.3389/fimmu.2023.1087978
187. Thepmalee C, Panya A, Junking M, Chieochansin T, YenchitsOmanus PT. Inhibition of il-10 and tgf-B Receptors on dendritic cells enhances activation of effector T-cells to kill cholangiocarcinoma cells. Hum Vaccin Immunother (2018) 14(6):1423–31. doi: 10.1080/21645515.2018.1431598
188. Schoppmann SF, Birner P, Stöckl J, Kalt R, Ullrich R, Caucig C, et al. Tumor-associated macrophages express lymphatic endothelial growth factors and are related to peritumoral lymphangiogenesis. Am J Pathol (2002) 161(3):947–56. doi: 10.1016/s0002-9440(10)64255-1
189. Cui F, Xu Z, Hu J, Lv Y. Spindle pole body component 25 and platelet-derived growth factor mediate crosstalk between tumor-associated macrophages and prostate cancer cells. Front Immunol (2022) 13:907636. doi: 10.3389/fimmu.2022.907636
190. Maldonado LAG, Nascimento CR, Rodrigues Fernandes NA, Silva ALP, D'Silva NJ, Rossa C Jr. Influence of tumor cell-derived tgf-B on macrophage phenotype and macrophage-mediated tumor cell invasion. Int J Biochem Cell Biol (2022) 153:106330. doi: 10.1016/j.biocel.2022.106330
191. Yu X, Buttgereit A, Lelios I, Utz SG, Cansever D, Becher B, et al. The cytokine tgf-B Promotes the development and homeostasis of alveolar macrophages. Immunity (2017) 47(5):903–12.e4. doi: 10.1016/j.immuni.2017.10.007
192. Takase N, Koma Y, Urakawa N, Nishio M, Arai N, Akiyama H, et al. Ncam- and fgf-2-mediated fgfr1 signaling in the tumor microenvironment of esophageal cancer regulates the survival and migration of tumor-associated macrophages and cancer cells. Cancer Lett (2016) 380(1):47–58. doi: 10.1016/j.canlet.2016.06.009
193. Lisi L, Pia Ciotti GM, Chiavari M, Ruffini F, Lacal PM, Graziani G, et al. Vascular endothelial growth factor receptor 1 in glioblastoma−Associated microglia/macrophages. Oncol Rep (2020) 43(6):2083–92. doi: 10.3892/or.2020.7553
194. Esposito E, Hayakawa K, Ahn BJ, Chan SJ, Xing C, Liang AC, et al. Effects of ischemic post-conditioning on neuronal vegf regulation and microglial polarization in a rat model of focal cerebral ischemia. J Neurochem (2018) 146(2):160–72. doi: 10.1111/jnc.14337
195. Shin YJ, Riew TR, Park JH, Pak HJ, Lee MY. Expression of vascular endothelial growth factor-C (Vegf-C) and its receptor (Vegfr-3) in the glial reaction elicited by human mesenchymal stem cell engraftment in the normal rat brain. J Histochem Cytochem (2015) 63(3):170–80. doi: 10.1369/0022155414564218
196. Wang Z, Song K, Zhao W, Zhao Z. Dendritic cells in tumor microenvironment promoted the neuropathic pain via paracrine inflammatory and growth factors. Bioengineered (2020) 11(1):661–78. doi: 10.1080/21655979.2020.1771068
197. Jia X, Zhang A, Li Z, Peng X, Tian X, Gao F. Activation of spinal pdgfrβ in microglia promotes neuronal autophagy via P38 mapk pathway in morphine-tolerant rats. J Neurochem (2021) 158(2):373–90. doi: 10.1111/jnc.15383
198. Yaqubi M, Groh AMR, Dorion MF, Afanasiev E, Luo JXX, Hashemi H, et al. Analysis of the microglia transcriptome across the human lifespan using single cell rna sequencing. J Neuroinflamm (2023) 20(1):132. doi: 10.1186/s12974-023-02809-7
199. Kuroda E, Nishimura K, Kawanishi S, Sueyoshi M, Ueno F, Toji Y, et al. Mouse bone marrow-derived microglia-like cells secrete transforming growth factor-B1 and promote microglial Aβ Phagocytosis and reduction of brain Aβ. Neuroscience (2020) 438:217–28. doi: 10.1016/j.neuroscience.2020.05.004
200. Parthasarathy G, Pattison MB, Midkiff CC. The fgf/fgfr system in the microglial neuroinflammation with borrelia burgdorferi: likely intersectionality with other neurological conditions. J Neuroinflamm (2023) 20(1):10. doi: 10.1186/s12974-022-02681-x
201. Fu X, Chen H, Han S. C16 peptide and angiopoietin-1 protect against lps-induced bv-2 microglial cell inflammation. Life Sci (2020) 256:117894. doi: 10.1016/j.lfs.2020.117894
202. Shin JY, Yoon IH, Kim JS, Kim B, Park CG. Vascular endothelial growth factor-induced chemotaxis and il-10 from T cells. Cell Immunol (2009) 256(1-2):72–8. doi: 10.1016/j.cellimm.2009.01.006
203. Renault C, Veyrenche N, Mennechet F, Bedin AS, Routy JP, Van de Perre P, et al. Th17 cd4+ T-cell as a preferential target for hiv reservoirs. Front Immunol (2022) 13:822576. doi: 10.3389/fimmu.2022.822576
204. Marie JC, Liggitt D, Rudensky AY. Cellular mechanisms of fatal early-onset autoimmunity in mice with the T cell-specific targeting of transforming growth factor-beta receptor. Immunity (2006) 25(3):441–54. doi: 10.1016/j.immuni.2006.07.012
205. Etori K, Tanaka S, Tamura J, Hattori K, Kagami SI, Nakamura J, et al. Fibroblast growth factor receptor 1 as a potential marker of terminal effector peripheral T helper cells in rheumatoid arthritis patients. Rheumatol (Oxford England) (2023). doi: 10.1093/rheumatology/kead220
206. Leplina O, Smetanenko E, Tikhonova M, Batorov E, Tyrinova T, Pasman N, et al. Binding of the placental growth factor to vegf receptor type 1 modulates human T cell functions. J Leukocyte Biol (2020) 108(3):1013–24. doi: 10.1002/JLB.2A0420-723RR
207. Voron T, Colussi O, Marcheteau E, Pernot S, Nizard M, Pointet AL, et al. Vegf-a modulates expression of inhibitory checkpoints on cd8+ T cells in tumors. J Exp Med (2015) 212(2):139–48. doi: 10.1084/jem.20140559
208. Lee JY, Park S, Min WS, Kim HJ. Restoration of natural killer cell cytotoxicity by vegfr-3 inhibition in myelogenous leukemia. Cancer Lett (2014) 354(2):281–9. doi: 10.1016/j.canlet.2014.08.027
209. Viel S, Marçais A, Guimaraes FS, Loftus R, Rabilloud J, Grau M, et al. Tgf-B Inhibits the activation and functions of nk cells by repressing the mtor pathway. Sci Signaling (2016) 9(415):ra19. doi: 10.1126/scisignal.aad1884
210. Massena S, Christoffersson G, Vågesjö E, Seignez C, Gustafsson K, Binet F, et al. Identification and characterization of vegf-a-responsive neutrophils expressing cd49d, vegfr1, and cxcr4 in mice and humans. Blood (2015) 126(17):2016–26. doi: 10.1182/blood-2015-03-631572
211. Genkel V, Dolgushin I, Baturina I, Savochkina A, Nikushkina K, Minasova A, et al. Associations between Circulating Vegfr2(Hi)-Neutrophils and Carotid Plaque Burden in Patients Aged 40-64 without Established Atherosclerotic Cardiovascular Disease. J Immunol Res (2022) 2022:1539935. doi: 10.1155/2022/1539935
212. Schernberg A, Blanchard P, Chargari C, Deutsch E. Neutrophils, a candidate biomarker and target for radiation therapy? Acta Oncol (Stockholm Sweden) (2017) 56(11):1522–30. doi: 10.1080/0284186x.2017.1348623
213. Marone G, Varricchi G, Loffredo S, Granata F. Mast cells and basophils in inflammatory and tumor angiogenesis and lymphangiogenesis. Eur J Pharmacol (2016) 778:146–51. doi: 10.1016/j.ejphar.2015.03.088
214. Baumgarten P, Blank AE, Franz K, Hattingen E, Dunst M, Zeiner P, et al. Differential expression of vascular endothelial growth factor a, its receptors vegfr-1, -2, and -3 and co-receptors neuropilin-1 and -2 does not predict bevacizumab response in human astrocytomas. Neuro-oncology (2016) 18(2):173–83. doi: 10.1093/neuonc/nov288
215. Paugh BS, Broniscer A, Qu C, Miller CP, Zhang J, Tatevossian RG, et al. Genome-wide analyses identify recurrent amplifications of receptor tyrosine kinases and cell-cycle regulatory genes in diffuse intrinsic pontine glioma. J Clin Oncol (2011) 29(30):3999–4006. doi: 10.1200/jco.2011.35.5677
216. Li Y, Yu H, Ma Q, Wei M, Liu X, Qi Y, et al. Si-pdgfrβ-loaded exosomes suppress the progression of glioma by inhibiting the oxidative associated pi3k/akt/ezh2 signaling pathway. Oxid Med Cell Longevity (2022) 2022:5081439. doi: 10.1155/2022/5081439
217. Deng L, Zheng W, Dong X, Liu J, Zhu C, Lu D, et al. Chemokine receptor cxcr7 is an independent prognostic biomarker in glioblastoma. Cancer Biomarkers sect A Dis Markers (2017) 20(1):1–6. doi: 10.3233/cbm-151430
218. Kjellman C, Olofsson SP, Hansson O, Von Schantz T, Lindvall M, Nilsson I, et al. Expression of tgf-beta isoforms, tgf-beta receptors, and smad molecules at different stages of human glioma. Int J Cancer (2000) 89(3):251–8. doi: 10.1002/1097-0215(20000520)89:3<251::aid-ijc7>3.0.co;2-5
219. Loilome W, Joshi AD, ap Rhys CM, Piccirillo S, Vescovi AL, Gallia GL, et al. Glioblastoma cell growth is suppressed by disruption of fibroblast growth factor pathway signaling. J neuro-oncol (2009) 94(3):359–66. doi: 10.1007/s11060-009-9885-5
220. Lee OH, Xu J, Fueyo J, Fuller GN, Aldape KD, Alonso MM, et al. Expression of the receptor tyrosine kinase tie2 in neoplastic glial cells is associated with integrin beta1-dependent adhesion to the extracellular matrix. Mol Cancer Res MCR (2006) 4(12):915–26. doi: 10.1158/1541-7786.Mcr-06-0184
221. Mamuladze T, Kipnis J. Type 2 immunity in the brain and brain borders. Cell Mol Immunol (2023). doi: 10.1038/s41423-023-01043-8
222. Silvin A, Qian J, Ginhoux F. Brain macrophage development, diversity and dysregulation in health and disease. Cell Mol Immunol (2023). doi: 10.1038/s41423-023-01053-6
223. Hammond TR, Dufort C, Dissing-Olesen L, Giera S, Young A, Wysoker A, et al. Single-cell rna sequencing of microglia throughout the mouse lifespan and in the injured brain reveals complex cell-state changes. Immunity (2019) 50(1):253–71.e6. doi: 10.1016/j.immuni.2018.11.004
224. Berriat F, Lobsiger CS, Boillée S. The contribution of the peripheral immune system to neurodegeneration. Nat Neurosci (2023) 26(6):942–54. doi: 10.1038/s41593-023-01323-6
225. Xie Q, Liu T, Zhang X, Ding Y, Fan X. Construction of a telomere-related gene signature to predict prognosis and immune landscape for glioma. Front Endocrinol (2023) 14:1145722. doi: 10.3389/fendo.2023.1145722
226. Chen R, Wu W, Liu T, Zhao Y, Wang Y, Zhang H, et al. Large-scale bulk rna-seq analysis defines immune evasion mechanism related to mast cell in gliomas. Front Immunol (2022) 13:914001. doi: 10.3389/fimmu.2022.914001
227. Huang H, Langenkamp E, Georganaki M, Loskog A, Fuchs PF, Dieterich LC, et al. Vegf suppresses T-lymphocyte infiltration in the tumor microenvironment through inhibition of nf-Kb-induced endothelial activation. FASEB J (2015) 29(1):227–38. doi: 10.1096/fj.14-250985
Keywords: angiogenic growth factor, immune cell, glioma, immune modulation, tumor microenvironment
Citation: Ge Z, Zhang Q, Lin W, Jiang X and Zhang Y (2023) The role of angiogenic growth factors in the immune microenvironment of glioma. Front. Oncol. 13:1254694. doi: 10.3389/fonc.2023.1254694
Received: 07 July 2023; Accepted: 28 August 2023;
Published: 13 September 2023.
Edited by:
Wei Zhang, Capital Medical University, ChinaReviewed by:
Di Yu, Uppsala University, SwedenMeng Zheng, Henan University, China
Hua Huang, Uppsala University, Sweden
Copyright © 2023 Ge, Zhang, Lin, Jiang and Zhang. This is an open-access article distributed under the terms of the Creative Commons Attribution License (CC BY). The use, distribution or reproduction in other forums is permitted, provided the original author(s) and the copyright owner(s) are credited and that the original publication in this journal is cited, in accordance with accepted academic practice. No use, distribution or reproduction is permitted which does not comply with these terms.
*Correspondence: Yanyu Zhang, eWFueXUuemhhbmdAZm1tdS5lZHUuY24=; eWFueXUuemhhbmdAaG90bWFpbC5jb20=; Xiaofan Jiang, amlhbmd4ZkBmbW11LmVkdS5jbg==