- 1OncoRay - National Center for Radiation Research in Oncology, Faculty of Medicine and University Hospital Carl Gustav Carus, Technische Universität Dresden and Helmholtz-Zentrum Dresden-Rossendorf, Dresden, Germany
- 2Helmholtz-Zentrum Dresden-Rossendorf, Institute of Radiooncology-OncoRay, Dresden, Germany
- 3German Cancer Consortium (DKTK), Partner Site Dresden and German Cancer Research Center (DKFZ), Heidelberg, Germany
- 4National Center for Tumor Diseases (NCT), Partner Site Dresden: German Cancer Research Center (DKFZ), Heidelberg, Faculty of Medicine and University Hospital Carl Gustav Carus, Technische Universität Dresden, Helmholtz-Zentrum Dresden-Rossendorf (HZDR), Dresden, Germany
The SLC3A2 gene encodes for a cell-surface transmembrane protein CD98hc (4F2). CD98hc serves as a chaperone for LAT1 (SLC7A5), LAT2 (SLC7A8), y+LAT1 (SLC7A7), y+LAT2 (SLC7A6), xCT (SLC7A11) and Asc1 (SLC7A10) providing their recruitment to the plasma membrane. Together with the light subunits, it constitutes heterodimeric transmembrane amino acid transporters. CD98hc interacts with other surface molecules, such as extracellular matrix metalloproteinase inducer CD147 (EMMPRIN) and adhesion receptors integrins, and regulates glucose uptake. In this way, CD98hc connects the signaling pathways sustaining cell proliferation and migration, biosynthesis and antioxidant defense, energy production, and stem cell properties. This multifaceted role makes CD98hc one of the critical regulators of tumor growth, therapy resistance, and metastases. Indeed, the high expression levels of CD98hc were confirmed in various tumor tissues, including head and neck squamous cell carcinoma, glioblastoma, colon adenocarcinoma, pancreatic ductal adenocarcinoma, and others. A high expression of CD98hc has been linked to clinical prognosis and response to chemo- and radiotherapy in several types of cancer. In this mini-review, we discuss the physiological functions of CD98hc, its role in regulating tumor stemness, metastases, and therapy resistance, and the clinical significance of CD98hc as a tumor marker and therapeutic target.
1 Introduction
CD98 heavy chain (CD98hc, or 4F2, 4F2HC, 4T2HC, CD98, CD98HC, MDU1, NACAE) is a type II transmembrane glycoprotein identified in activated lymphocytes (1). It is encoded by the solute carrier family 3 member 2 (SLC3A2) gene in humans. The gene is mapped to the 11q12.3 chromosomal region, and encodes 4 transcript splice variants (according to the RefSeq database). Full-length CD98hc is a glycosylated type II transmembrane protein consisting of 630 amino acid residues (as for the transcript variant 3, NM_002394) and composed of four structural regions: intracellular N-tail (100 - 184 amino acid residues), single transmembrane domain (mapped within 185-205 amino acid residues), domain linker and glycosylated extracellular domain including 206-630 amino acid residues (2). CD98hc serves as a chaperone for six amino acid transporters, providing their recruitment to the plasma membrane. In particular, CD98hc binds with one of the light chains (L-type amino acid transporter 1 (LAT1), LAT2, y+LAT1, y+LAT2, cystine/glutamate antiporter (xCT) and Asc-type amino acid transporter 1 (Asc1) through disulfide bond and electrostatic interactions to form CD98 protein (2–5). In addition, CD98hc modulates intracellular signaling by its direct physical association with cell adhesion receptor integrin β1 (6), or glycoprotein CD147, an essential regulator of lactate and pyruvate transport (7), and regulates glucose uptake (8) that makes CD98hc a multifunctional hub protein. Indeed, lack of CD98hc triggers amino acid and glucose uptake inhibition, glycolysis suppression, decrease in the intracellular levels of nucleotides through the defective pentose phosphate pathway (PPP), oxidative stress, and cell cycle arrest (9–11). Overexpression of CD98hc drives malignant transformation (12, 13) and is associated with progression in different human malignancies. CD98hc and CD98hc binding partners are critical in regulating cancer cell functional properties (Table 1). A high expression of CD98hc has been related to the histopathological features and clinical prognosis in patients with many solid cancers, such as head and neck squamous cell carcinoma (HNSCC), glioma, colon adenocarcinoma, pancreatic ductal adenocarcinoma (PDAC), non-small cell lung cancer (NSCLC) and breast cancer (18, 35–41), and tumor response to conventional therapies, such as chemo- and radiotherapy (11, 42, 43). Thus, CD98hc plays a vital role in both physiological and pathological conditions. This mini-review discusses the role of CD98hc in regulating tumor growth, metastasis, and therapy response. We also highlight the clinical significance of CD98hc as a marker of tumor progression and therapy resistance and a promising therapeutic target to enhance the efficacy of conventional anti-cancer therapy.
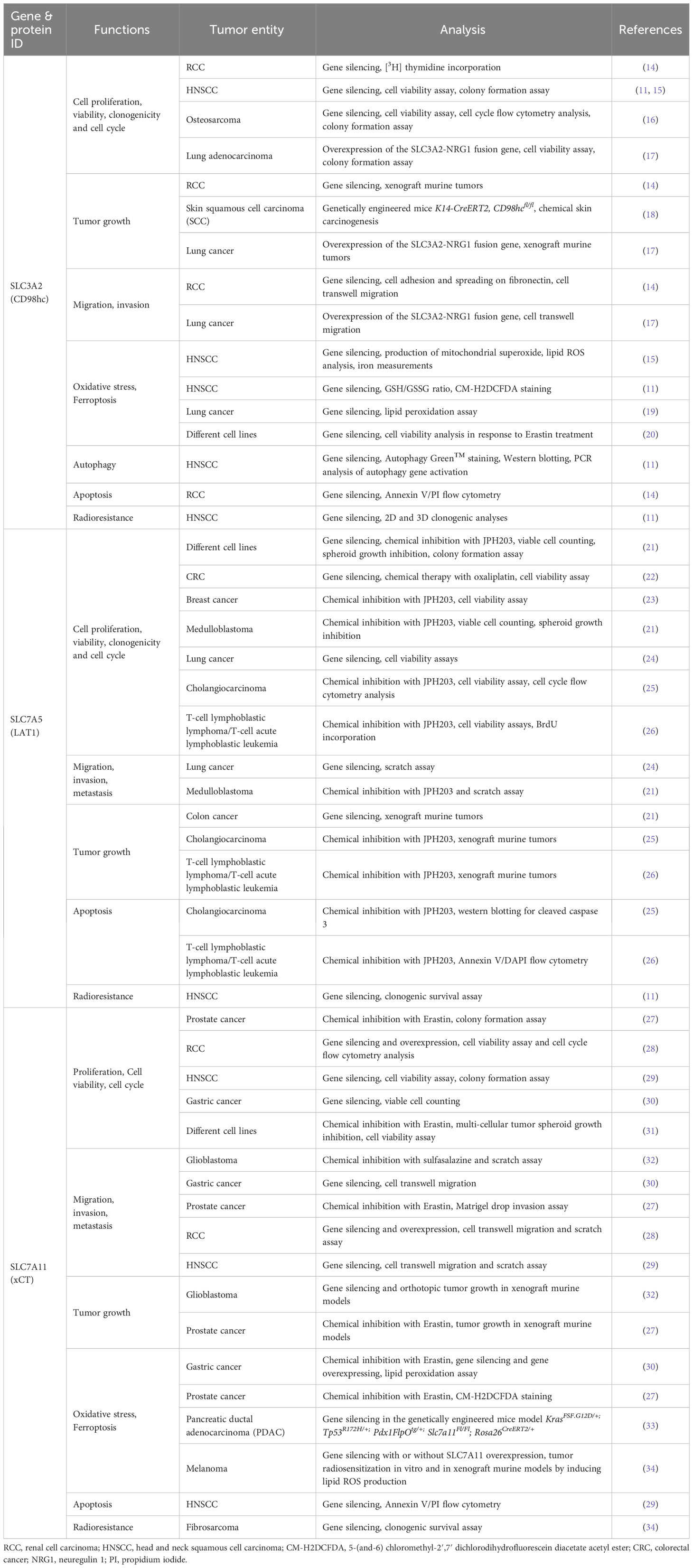
Table 1 The exemplary studies of the roles of CD98hc and CD98hc binding partners in regulating the cancer cell functional properties.
2 Biological functions and signaling mechanisms mediated by CD98hc
Targeted disruption of the CD98hc gene and analysis of the chimeric mice models demonstrated that CD98hc contributes to embryogenesis. During the early stage of embryonic development, embryos that lack CD98hc die shortly after implantation due to defective integrin signaling (44, 45), whereas the amino acid transport regulated by CD98hc becoming indispensable for the embryonic development at later stages (44). Embryonic stem (ES) cell lines without CD98hc expression showed a low ability to spread on fibronectin (FN) or laminin. CD98 expression enables FN matrix assembly through CD98hc–integrin interaction and consequent activation of RhoA-mediating extracellular matrix contraction (46).
CD98hc is expressed in all human organs, especially in placenta, bone marrow, kidney, lung, uterus, and thyroid tissues (47, 48) (Figure 1A), and is essential for the proliferation, survival, and functioning of different types of normal cells, including vascular smooth muscle cells (50), central nervous system (51), skin (52), dental cells (53), and other tissues (https://www.ncbi.nlm.nih.gov/gene/6520). The reduction of CD98hc expression resembles age-related skin alterations in mice models with tamoxifen-inducible epidermis-specific CD98hc knockout. CD98hc deletion in basal keratinocytes inhibits epidermal wound healing and hair growth due to deficient epidermis regeneration by self-renewal, keratinocyte proliferation, and migration. These effects are mediated by defective c-Src/focal adhesion kinase (FAK) signaling, persistent RhoA activation, and reactive oxygen species (ROS) accumulation due to insufficient amino acid (AA) availability (52). Indeed, two core functions of CD98 in different types of cells are to assist in transporting AA into and out of cells and regulate downstream signaling pathways, including extracellular regulated protein kinase (ERK), phosphatidylinositol-3-kinase (PI3K), FAK, Src, and Rho GTP, through its binding to the interacting partners, such as CD147 or integrins (54, 55). CD98hc forms a complex with integrin β1 or β3 subunits, and promotes anchorage independent cell growth and integrin-mediated cell signaling such as phospho-FAK, Akt and mitogen-activated protein kinase (MAPK)/extracellular signal-regulated kinase (ERK) pathways (14, 56, 57). The interaction between CD98hc and integrins is important for the regulation of stemness, proliferation, cell survival, and cancer transformation (14, 56, 57).
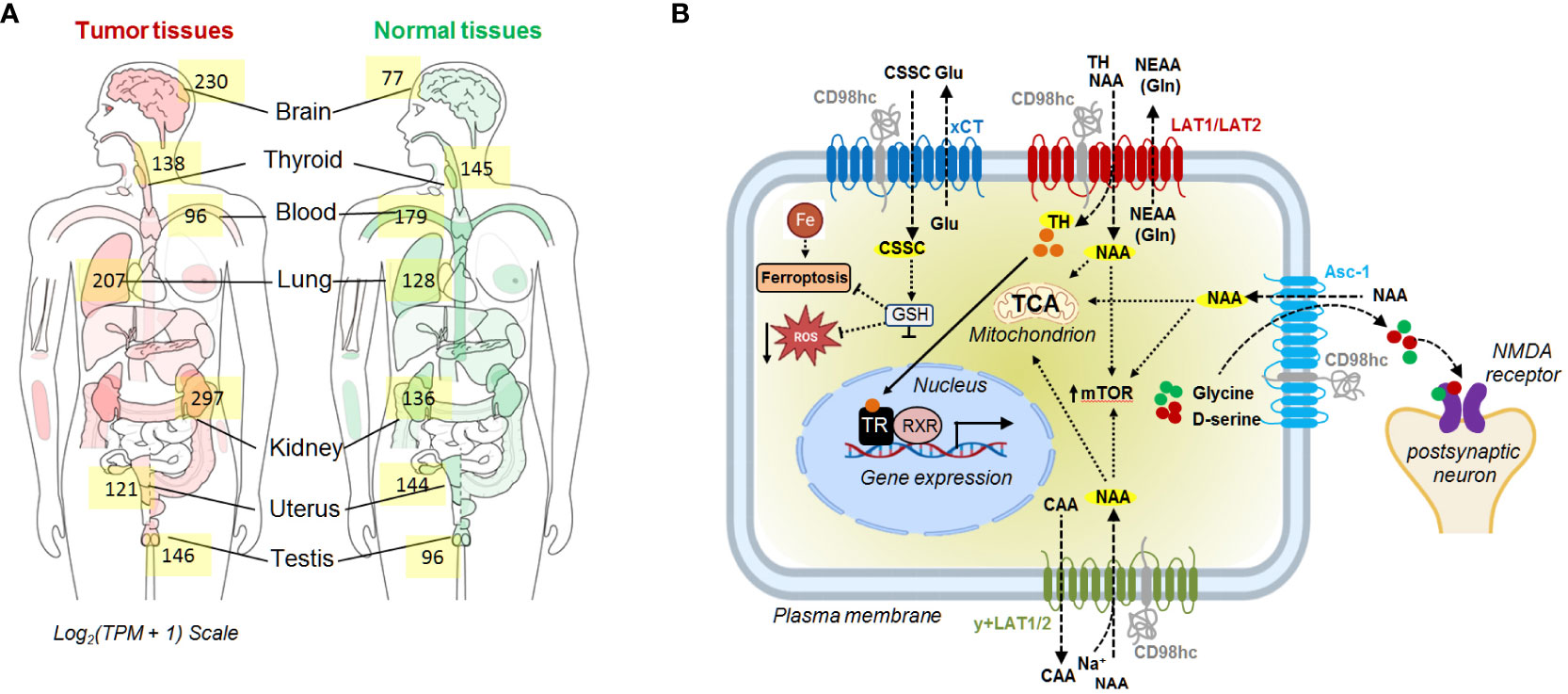
Figure 1 (A) The median expression of SLC3A2 in tumor and normal tissue. The data are obtained using GEPIA 2 (49). (B) Schematic representation of the CD98hc-related transport systems. CAA, cationic amino acids; CSSC, cystine; Gln, glutamine; Glu, glutamate; GSH, glutathione; NAA, neutral amino acids; NEAA, non-essential amino acids; NMDA, N-methyl-D-aspartate receptor; TCA, tricarboxylic acid cycle; TH, thyroid hormones; TR, thyroid hormone receptors; RXR, retinoid X receptor.
Heterodimerization of CD98hc with light subunits of the SLC7 family is essential for their trafficking, membrane topology, stability, and transport activity (2, 58, 59), although CD98hc does not directly contribute to the amino acid transport (2). CD98hc-LAT1 (SLC7A5) and LAT2 (SLC7A8) are responsible for the transport of neutral amino acids, such as tyrosine, phenylalanine, leucine, cysteine, isoleucine, methionine, valine, tryptophan, as well as histidine. (5, 60, 61). In addition, recent studies using cryo–electron microscopy (cryo-EM) shed light on the structural features defining the specificity of LAT2 toward small neutral amino acids and glutamine (5). Another study based on the analysis of the cryo-EM structure of CD98hc-LAT1 heterodimer revealed the presence of four N-glycosylated Asn residues within the extracellular CD98hc domain and confirmed that this glycosylation is not directly involved in the formation of the CD98hc-LAT1 complex (2). Instead, the recent finding suggested the role of this glycosylation in regulating CD98hc stability and trafficking to the plasma membrane and, consequently, LAT1 intracellular distribution and function (62). In agreement with this observation, other studies also demonstrated that knockout of CD98hc expression resulted in the cytoplasmic localization of LAT1 (11, 63). CD98hc-LAT1 and CD98hc-LAT2 mediated amino acid transport is necessary to meet the energetic and nutritional demands, as evidenced by low protein synthesis and proliferation rate in CD98hc knockout cells (10). Furthermore, CD98hc-LAT1 and CD98hc-LAT2 mediate the transport of the amino acid-derived thyroid hormones, mainly 3,3'--diiodothyronine (3,3'-T2) (64), essential for energy metabolism and developmental processes (65, 66). CD98hc-y+LAT1 (SLC7A7) and CD98hc-y+LAT2 (SLC7A6) heterodimers play a role in the efflux of cationic amino acids, such as arginine, lysine, and ornithine in exchange for neutral amino acids and Na+ (67, 68). CD98hc-xCT (SLC7A11) functions as a cystine/glutamate antiporter, which transports cystine into the cell in exchange for glutamate (69). Cystine plays a critical role in cellular antioxidant defense and redox balance by influencing the availability of cysteine, which is a precursor for the synthesis of glutathione (GSH) (70). GSH, as the primary intracellular antioxidant, contributes to neutralizing ROS and protects cells from oxidative damage (70, 71). CD98hc-Asc1 (SLC7A10) regulates the D-serine and glycine transport in the central nervous system. Both D-serine and glycine serve as co-agonists of the N-methyl-D-aspartate (NMDA) receptor (72). Finally, CD98hc is reported to bind GLUT1 and prevent its lysosomal degradation, thereby increasing glucose uptake (8) (Figure 1B). Furthermore, studies using mass-spectrometry based identification of the protein-protein interaction demonstrated that CD98hc interacts with PTPRJ, a receptor protein tyrosine phosphatase regulating CD98hc proteasomal degradation (73), and ASCT2 (SLC1A5) transporter mediating glutamine uptake (74). The mass spectrometry analysis suggested that CD98hc, CD147, monocarboxylate transporters (MCTs), and ASCT2 are part of the cell surface protein complex regulating cell energy metabolism and biosynthesis by the coordinated transport of amino acid and lactate (74). CD147 mediates the membrane localization of CD98hc and the activation of the downstream signaling mechanisms (75). CD147-CD98hc complex regulates various cell functions contributing to cell proliferation (55), metabolism (74), drug resistance (76), and cell aggregation (77) and leading to the activation of several key signaling pathways such as β-catenin (78), β1-integrin (75) and PI3K/AKT (55, 75) (Figure 2).
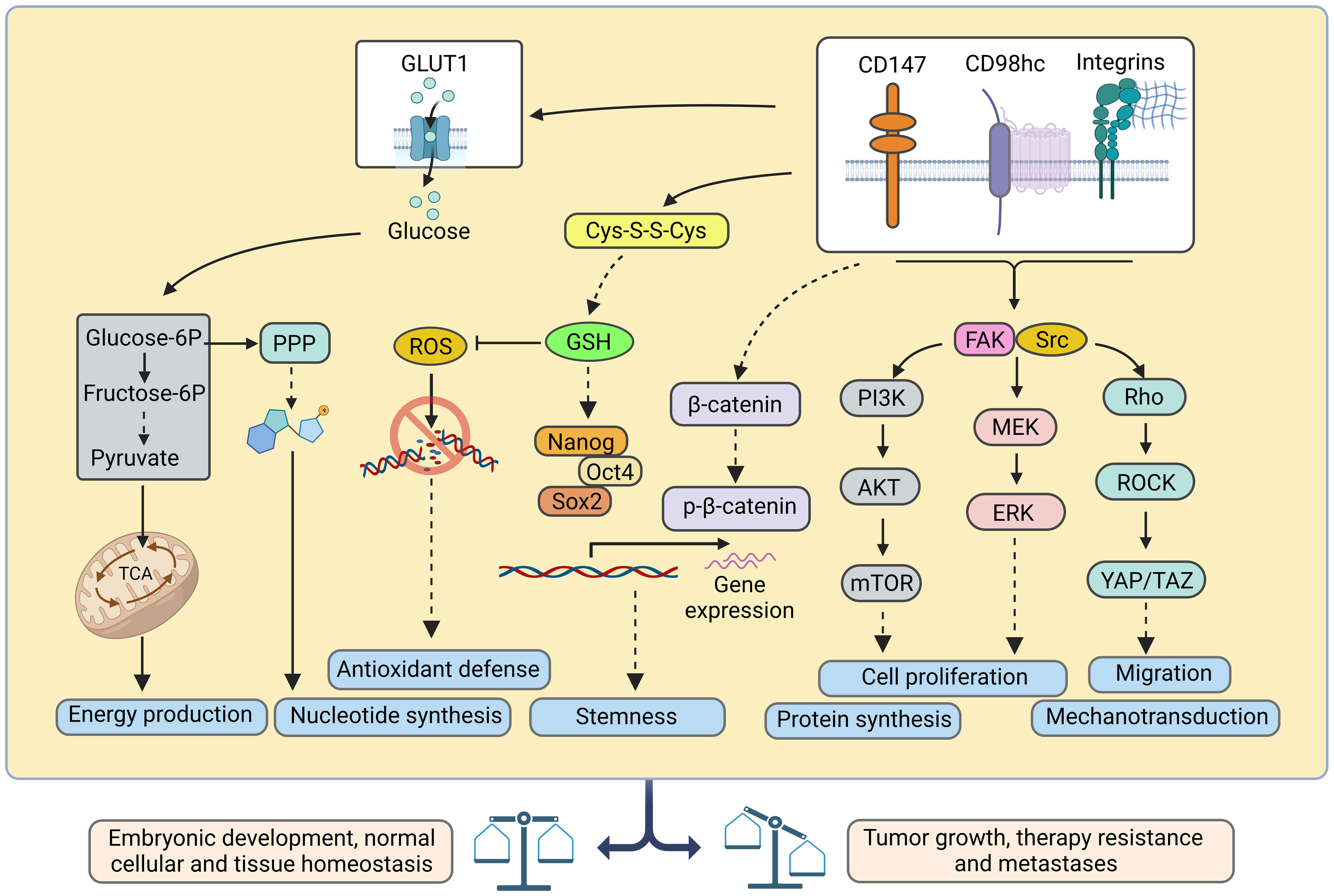
Figure 2 Ideograph of downstream pathways regulated by CD98hc and its partners. CD98hc-dependent pathways are critical for embryonic development and normal cellular and tissue homeostasis, and their deregulation is associated with tumor growth, therapy resistance, and metastases. CD98hc, CD98 heavy chain; Cys-S-S-Cys, cystine; FAK, focal adhesion kinase; ERK, extracellular signal-regulated kinase; GLUT1, glucose transporter type 1; GSH, reduced glutathione; MEK, mitogen-activated protein kinase kinase; mTOR, mammalian target of rapamycin; PI3K, phosphoinositide 3-kinases; PPP, pentose phosphate pathway; ROCK, Rho-associated protein kinase; ROS, reactive oxygen species; TAZ, transcriptional coactivator with PDZ-binding motif; YAP, yes-associated protein 1. Created with BioRender.com.
3 CD98hc as a regulation of immunity
CD98hc is an essential regulator of innate immunity and adaptive immune responses, mediating the functions of T and B lymphocytes and macrophages. In 1981, CD98hc was discovered as a cell surface marker present on the activated human lymphocytes and monocytes (1). The following findings revealed that CD98hc regulates B cell proliferation, spreading, and formation of the antibody-producing plasma cells through the integrin and MAPK/ERK/p27 signaling mechanism (79). Furthermore, the same team later demonstrated that the interaction of CD98hc with integrins regulates T cell proliferation (80), whereas CD98hc loss is associated with impaired antigen-driven T cell clonal expansion in vivo and prevents autoimmune response in murine modes of type I diabetes (80). Interestingly, inhibiting CD98hc on monocytes using anti-CD98 monoclonal antibody was also shown to suppress T cell proliferation (81). Anti-CD98hc antibody was suggested as a possible approach to increase transplantation efficacy since CD98hc deletion in T cells was associated with attenuated lymphocyte migration, low proliferation in response to the alloantigens, and poor allograft infiltration (82). On the other hand, the presence of CD98hc is critical for the control of immune tolerance. Blocking CD98hc and CD147 interaction leads to the degradation of the Foxp3 protein, one of the key transcription factors driving the differentiation and functions of regulatory T cells (Treg). Consequently, Treg cell stability requires cell-cell contact associated with CD98hc-CD147 interaction, sequestering of cyclin-dependent kinase 2 (CDK2) from activation and Foxp3 stabilization (83). Conditional deletion of CD98hc inhibits antigen-presenting and phagocytic activities of macrophages and is associated with decreased p130cas phosphorylation and impaired activation of AKT, ERK, and c-Jun N-terminal kinase (JNK) after treatment with macrophage colony-stimulating factor (M-CSF) and receptor activator of nuclear factor κB ligand (RANKL), the inducers of macrophage differentiation into osteoclasts. As a result, osteoclast formation by peritoneal macrophages isolated from CD98hc-defect mice was severely impaired (84). Nevertheless, CD98hc knockout mice have normal trabecular bones, and the function of CD98hc for in vivo osteoclast formation still needs to be clarified (84). CD98hc and its binding partners integrin β1 and CD147 regulate the actin cytoskeleton and affect monocyte adhesion by activating integrin-mediated signaling (85). CD98hc also serves as a surface receptor to mediate the internalization and trafficking of β-defensin 3 (hBD3), a peptide regulating innate immune response (86). This finding could potentially suggest that CD98hc/hBD2 interplay can play a role in the innate immune surveillance of tumor cells (86, 87).
4 CD98hc as a pathogen entry protein
In 1992, CD98hc was described as a fusion regulatory protein (FRP)-1 (gp80) regulating cell fusion upon Newcastle disease virus (NDV) infection (88). Since then, CD98hc has been shown to mediate many host-pathogen interactions (89). In particular, CD98hc provides an entry by endocytosis for the vaccinia virus (VV) in different in vitro models, including mouse embryonic fibroblasts (MEF) and human HeLa cells (90). CD98hc and VV particles co-localize in plasma membrane lipid rafts of the host cells upon infection, and genetic silencing of CD98hc expression reduced virus entry (90). CD98hc was identified as one of the proteins interacting with mouse norovirus-1 (MNV-1). The infection of mouse macrophages by MNV-1 is reduced after CD98hc depletion (91). Plasmodium vivax is a pathogenic protozoal parasite causing human malaria through the invasion of reticulocytes (92). Plasmodium vivax reticulocyte binding proteins (PvRBP) serve as invasion ligands mediating reticulocyte invasion (92). A recent study identified CD98hc as a reticulocyte-specific receptor for PvRBP2a, providing an additional route for Plasmodium vivax infection (93). Upon Herpes simplex virus 1 (HSV-1) infection, CD98hc and β1 integrin interact with HSV-1 proteins and mediate nucleocytoplasmic transport of perinuclear virions to release viral nucleocapsids into the cytosol (94, 95). CD98hc is also suggested to regulate viral gene expression upon Kaposi’s sarcoma-associated herpesvirus (KSHV) infection (96). Furthermore, CD98hc plays a role in bacterial infection. In particular, CD98hc has been identified as a binding partner for the VirB2 pilus protein of gram-negative coccobacilli Brucella and is essential for bacterial uptake and intracellular replication (97).
5 CD98hc as a promoter of tumor growth and metastases
The high expression levels of CD98hc in tumors compared to normal tissues could serve as indirect evidence for the contribution of CD98hc in tumor development. Pan-cancer analysis showed that CD98hc is highly expressed in many cancer types, including HNSCC, glioblastoma, colon, lung, kidney, pancreatic cancer and melanoma (Figure 3A). Indeed, there is a growing research interest in the and melanoma oncogenic roles of CD98hc. Lose and gain function studies showed CD98hc appears essential for tumor initiation, progression, and metastatic development (12, 13, 18, 76, 99–104). Overexpression of CD98hc in fibroblasts inhibits apoptosis, drives malignant transformation, and induces tumor formation in immunodeficient mice (12, 13, 105). CD98hc boosts cancer cell proliferation and tumor growth by transporting amino acids (10, 11), augmenting the integrin signaling (18, 54), and activation of the mTOR (11, 15, 17), PI3K/AKT (16) and MAPK signaling pathways (104, 106). CD98hc is essential for sustaining glucose uptake, glycolysis, and pentose phosphate pathway (PPP) (8, 10), fueling the Krebs cycle (11) and therefore maintaining cell energy metabolism. CD98hc also promotes the cell cycle by providing raw materials for nucleotide synthesis (10).
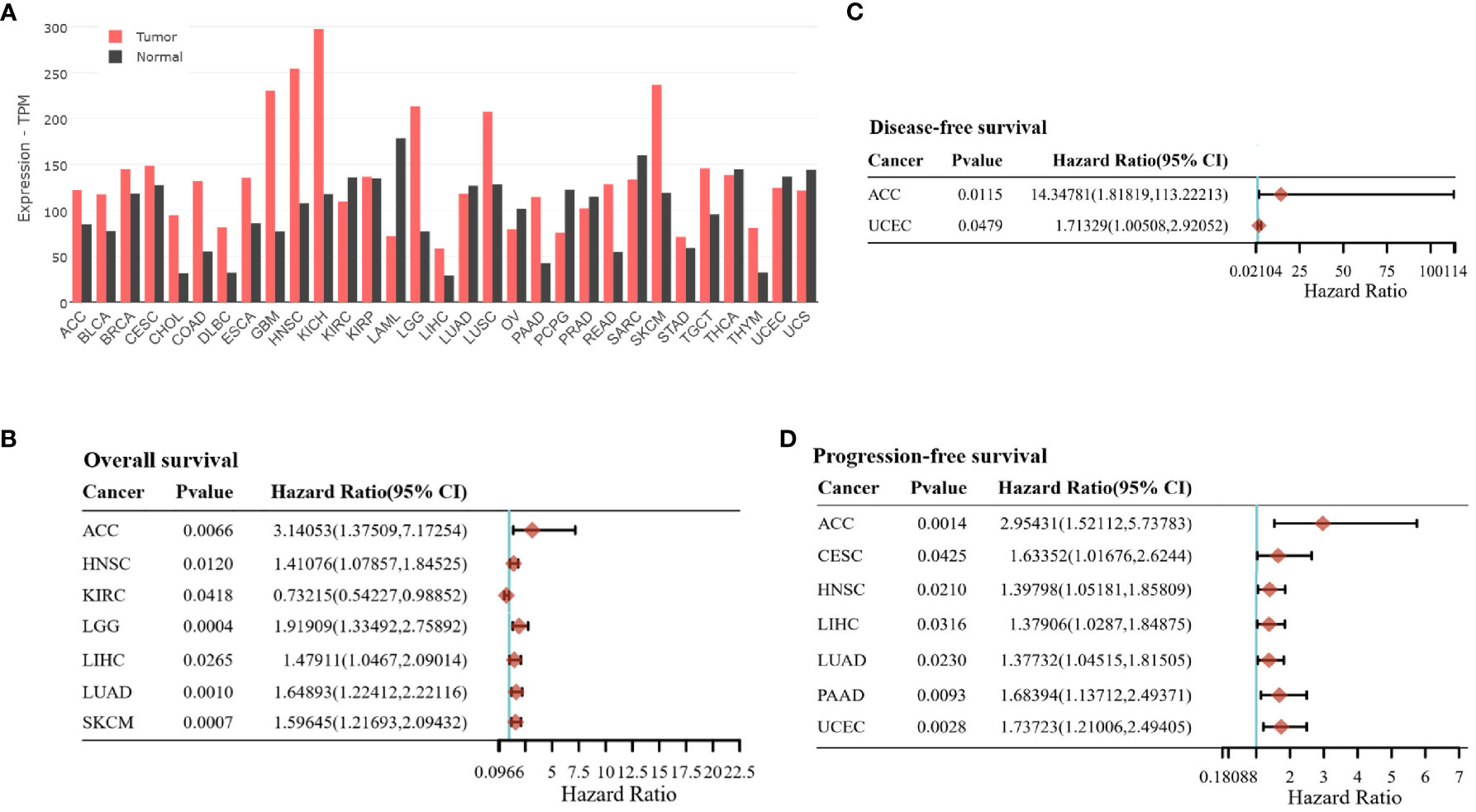
Figure 3 (A) The SLC3A2 gene expression in tumor samples and normal tissues. The data are obtained using GEPIA 2 (49) based on The Cancer Genome Atlas (TCGA) and the Genotype-Tissue Expression (GTEx) datasets. TCGA Study Abbreviations: LAML, Acute Myeloid Leukemia; ACC, Adrenocortical carcinoma; BLCA, Bladder Urothelial Carcinoma; LGG, Brain Lower Grade Glioma; BRCA, Breast invasive carcinoma; CESC, Cervical squamous cell carcinoma and endocervical adenocarcinoma; CHOL, Cholangiocarcinoma; COAD, Colon adenocarcinoma; ESCA:Esophageal carcinoma; GBM:Glioblastoma multiforme; HNSC, Head and Neck squamous cell carcinoma; KICH, Kidney Chromophobe; KIRC:Kidney renal clear cell carcinoma; KIRP:Kidney renal papillary cell carcinoma; LIHC, Liver hepatocellular carcinoma; LUAD:Lung adenocarcinoma; LUSC:Lung squamous cell carcinoma; DLBC, Lymphoid Neoplasm Diffuse Large B-cell Lymphoma; OV, Ovarian serous cystadenocarcinoma; PAAD, Pancreatic adenocarcinoma; PCPG, Pheochromocytoma and Paraganglioma; PRAD, Prostate adenocarcinoma; READ:Rectum adenocarcinoma; SARC:Sarcoma; SKCM, Skin Cutaneous Melanoma; STAD, Stomach adenocarcinoma; TGCT:Testicular Germ Cell Tumors; THYM, Thymoma; THCA, Thyroid carcinoma; UCEC, Uterine Corpus Endometrial Carcinoma; TPM, transcripts per million. (B, C) The association between SLC3A2 gene expression and prognosis profile, including overall survival (B), disease-free survival (C), and progression-free survival (D) across different tumor entities. The data are obtained using the GSCALite platform (98) based on The Cancer Genome Atlas (TCGA) data.
Furthermore, CD98hc is essential for sensing the extracellular matrix (ECM) stiffness and tumor cell migration and invasion. The ECM is made up of fibrous proteins such as collagens, elastin, and glycoproteins, including fibronectin, proteoglycans, and laminin (107). ECM is dynamically regulated in normal and disease conditions (108). Exposure of human bronchial epithelial cells to the diesel exhaust particle (DEP) extract induced expression of CD98hc and upregulation of mRNA levels of matrix metalloproteinase (MMP)-2 (109), a zinc-dependent endopeptidase mediating the degradation of ECM components and tumor cell invasion (110, 111). Consistently, silencing of CD98hc reduced the levels of MMP2 (109). Integrins mediate the communication between ECM and cancer cells through binding to ECM proteins, such as FN and vitronectin (VN) (112). CD98hc promotes tumorigenesis by interacting with integrins β1 and β3 and activating adhesive signals, such as focal adhesion kinase (FAK) regulating actin cytoskeleton dynamics. Acquisition of the aggressive tumor characteristics in clear cell renal cancer (ccRCC) cells is attributed to the CD98hc-integrin binding, whereas silencing of CD98hc decreases tumor cell spreading, migration and proliferation, and tumor growth in vivo (14). CD98 overexpression in the intestinal epithelial cell is associated with activation of the MEK/ERK signaling, increases levels of proinflammatory cytokines, and leads to tumorigenesis (113). Furthermore, a recent study demonstrated that CD98hc promotes colon cancer metastases through the crosstalk of tumor cells and tumor-associated neutrophils (TANs) (106). In particular, colorectal cancer cells secret transforming growth factor beta1 (TGF-β1) to induce neutrophils to become anterior gradient-2 (AGR2) positive TANs (92). TANs secrete AGR2 in the tumor microenvironment. CD98hc, expressed in colorectal cancer (CRC) cells, is the functional receptor for secreted AGR2 and physically interacts with AGR2 via its extracellular region. CD98hc-AGR2 binding leads to increased xCT activity and intracellular level of reduced GSH and promotes CRC liver metastasis through MAPK/ERK and RhoA/Rho-associated protein kinase 2 (ROCK2) pathway (106). The high levels of CD98hc expression in metastatic tissues compared to primary tumors have been found in different types of solid cancers (114, 115), and CD98hc expression in primary tumors is associated with increased metastatic development in HNSCC (43, 116), breast cancer (36), and gastric cancer (117). CD98hc mediates integrin-driven mechanotransduction. Furthermore, CD98hc increases the stiffness of the tumor microenvironment by activation of the actin-Rho/Rho-associated protein kinase (ROCK) and YAP/TAZ signaling, resulting in changes in collagen and fibronectin organization (18). Integrin-mediated mechanotransduction plays a role in Ras-induced development of skin squamous cell carcinoma, and conditional knockout of CD98hc expression in epidermis inhibited chemical skin carcinogenesis mediated by 7,12-dimethylbenz(a)anthracene (DMBA)-induced oncogenic Ras mutations. These findings suggest that CD98hc mediates Ras-induced tumor growth (18). A fusion of CD98hc with other oncogenes affects its functions and pathophysiological role. More than 20% of patients with invasive mucinous adenocarcinoma of the lung were found to have a fusion of CD98hc and a driver oncogene protein Neuregulin 1 (NRG1). CD98hc-NRG1 fusion promotes the proliferation, invasion, migration, and tumor growth of lung cancer cells via the upregulation of PI3K/AKT/mTOR and FAK-Src pathways (97). Furthermore, patients with CD98hc-NRG1 fusions showed significantly lower overall survival and disease-free survival compared to those without gene fusion (17). All these findings indicate that CD98hc regulates cancer cell proliferation and migration via modeling the tumor microenvironment and directly activating the signaling cascades in tumor cells.
One of the tumor hallmarks is metabolic rewiring induced by oncogenic mutations and epigenetic changes. This metabolic reprogramming is often associated with upregulation of ROS levels. Amino acid uptake mediated by CD98hc plays a role in tumor cell protection against oxidative stress (71). In particular, CD98hc is essential to prevent ferroptosis, a form of regulated cell death characterized by the accumulation of lipid peroxides and iron-dependent ROS in cells (118). It is emerging as an essential process in cancer biology, with implications for both cancer development and therapy (31). For example, one of the promising anti-cancer therapeutic strategies via ferroptosis induction is the inhibition of glutathione peroxidase 4 (GPX4), an enzyme catalyzing the peroxide reduction at the expense of GSH and therefore preventing cells from ferroptosis (119). Cystine transporter system Xc- composed of catalytic subunit xCT/SLC7A11 and chaperone subunit CD98hc transfers extracellular cystine into cells and converts it into cysteine, which is then used to synthesize GSH, a crucial ROS scavenger and cofactor of GPX4 to reduce peroxides to corresponding alcohol molecules (120). As a key component of system Xc-, CD98hc has been shown to be a ferroptosis mediator in HNSCC, lung, and prostate cancer (15, 19, 27). Of interest, exosomes of the hepatitis B virus (HBV)-positive hepatocellular carcinoma (HCC) cells induce ferroptosis in tumor-suppressing M1-type macrophages by inhibiting CD98hc expression through exosomal miR-142-3p (121). This finding can explain a depletion of M1-type macrophages in HBV+ HCC tissues (121). Inhibition or downregulation of SLC7A11 (xCT) leads to reduced cystine uptake, resulting in low intracellular GSH levels, accumulation of ROS, and increased susceptibility to ferroptosis (122). Blocking SLC7A11 (xCT) by a small molecule compound, erastin, induces ferroptosis in cancer cells (27, 30, 123). All this evidence indicates that CD98hc and CD98hc-related amino acid transporters play a vital role in fast-growing tumor cells by regulating energy metabolism, biosynthesis, and key oncogenic pathways.
6 CD98hc and CD98hc-related amino acid transporters as potential markers and regulators of cancer stem cells
Cancer stem cells (CSCs) are a population of cancer cells defined by their ability to self-renew and differentiate. CSCs give rise to tumorigenic and non-tumorigenic cell populations and maintain tumor growth (124–126). Cancer cell plasticity represents a significant challenge for target CSCs (127). However, if CSCs are not eradicated during the course of treatment along with non-CSC populations, they might lead to tumor recurrence (128). The subpopulations of CSCs are responsible for tumor cell dissemination and metastatic growth (129). Some CSC populations are also proven to be resistant to the conventional treatment (128, 130, 131). CSC markers were related to the poor clinical prognosis in different types of cancer (124, 128, 132, 133). CD98hc and CD98hc-binding proteins, such as SLC7A5 and SLC7A11, have been characterized as putative CSC markers for several malignancies (134–138) (Table 2). These studies mainly used spherogenicity assays in vitro and CSC-related gene expression analyses to assess the CSC phenotypes, and only some of them employed tumor transplantation assay, a “gold standard” approach for characterizing CSC populations (142). In particular, Martens-de Kemp et al. used limiting dilution analysis and serial transplantation of CD98high and CD98low HNSCC cells to demonstrate that CD98high cells are self-renewing population, and CD98high cell–derived tumors histologically recapitulate the parental tumor tissues (135). Bajaj et al. demonstrated that CD98hc promotes acute myelogenous leukemia (AML) propagation in mice models of disease by maintaining leukemic stem cells through the integrin signaling pathway, and genetic loss of CD98hc or antibody-mediated CD98hc blockage impairs in vivo AML propagation (56). CD98hc increases the incidences of intestinal tumors in mice bearing a mutation in Apc tumor suppressor (113) and in a murine model of colitis-associated CRC (102). On the other hand, the study by Huang et al. based on the transcriptome analysis suggested that CD98hc suppresses CSCs in human cervical carcinoma HeLa cells grown in 3D culture (143). The role of xCT/SLC7A11 in regulating CSCs and its potential targeting is also an actively investigated topic. Immunotargeting of xCT using DNA-based vaccination of mice bearing syngeneic breast tumors inhibits the growth of primary tumors and prevents metastasis formation (138). It was suggested that xCT plays a role in the maintenance of breast CSC cells by regulating GSH production and intracellular redox balance (138). Breast CSCs were found to secrete DKK1 (Dickkopf WNT Signaling Pathway Inhibitor 1), which activates xCT expression in the metastatic cells, protecting them from ferroptosis and increasing metastatic colonization in murine models (139). Similarly, inhibition of xCT by chemical inhibitor erastin targets colorectal CSCs in vitro and in vivo and attenuates their chemoresistance by elevating ROS levels and inducing ferroptosis (140). Stem cell surface marker CD44v6 induces GSH synthesis by stabilizing the xCT expression, thereby reducing the ROS level and promoting drug resistance of cancer cells (144). In triple-negative breast cancer, chemotherapy induces xCT expression and GSH synthesis in a hypoxia-inducible factor (HIF-1)–dependent manner. Increased levels of intracellular GSH induce nuclear translocation of FoxO3a transcription factor and trigger expression of pluripotency factor Nanog. Consequently, Nanog regulates the expression of other pluripotency factors, such as Oct4 and Sox2, and drives tumor cell reprogramming and CSC enrichment (145). Of interest, the Sox2 transcription factor directly binds to the xCT gene promoter, and Sox2-mediated xCT upregulation protects lung CSCs from ferroptosis (146). Inhibition of xCT with chemical drug sulfasalazine induces apoptosis in CD44v-positive HNSCC cells in murine xenograft models and sensitizes tumor cells to the epidermal growth factor receptor (EGFR)-targeted therapy with cetuximab (147). Thus, based on the current literature (Table 2), CD98hc and related amino acid transporters are potential markers for CSCs. Nevertheless, more evidence from the preclinical animal models and patient-derived tissues is warranted to prove that CD98hc and CD98hc-related amino acid transporters serve as markers and regulators of CSCs in different tumor entities.
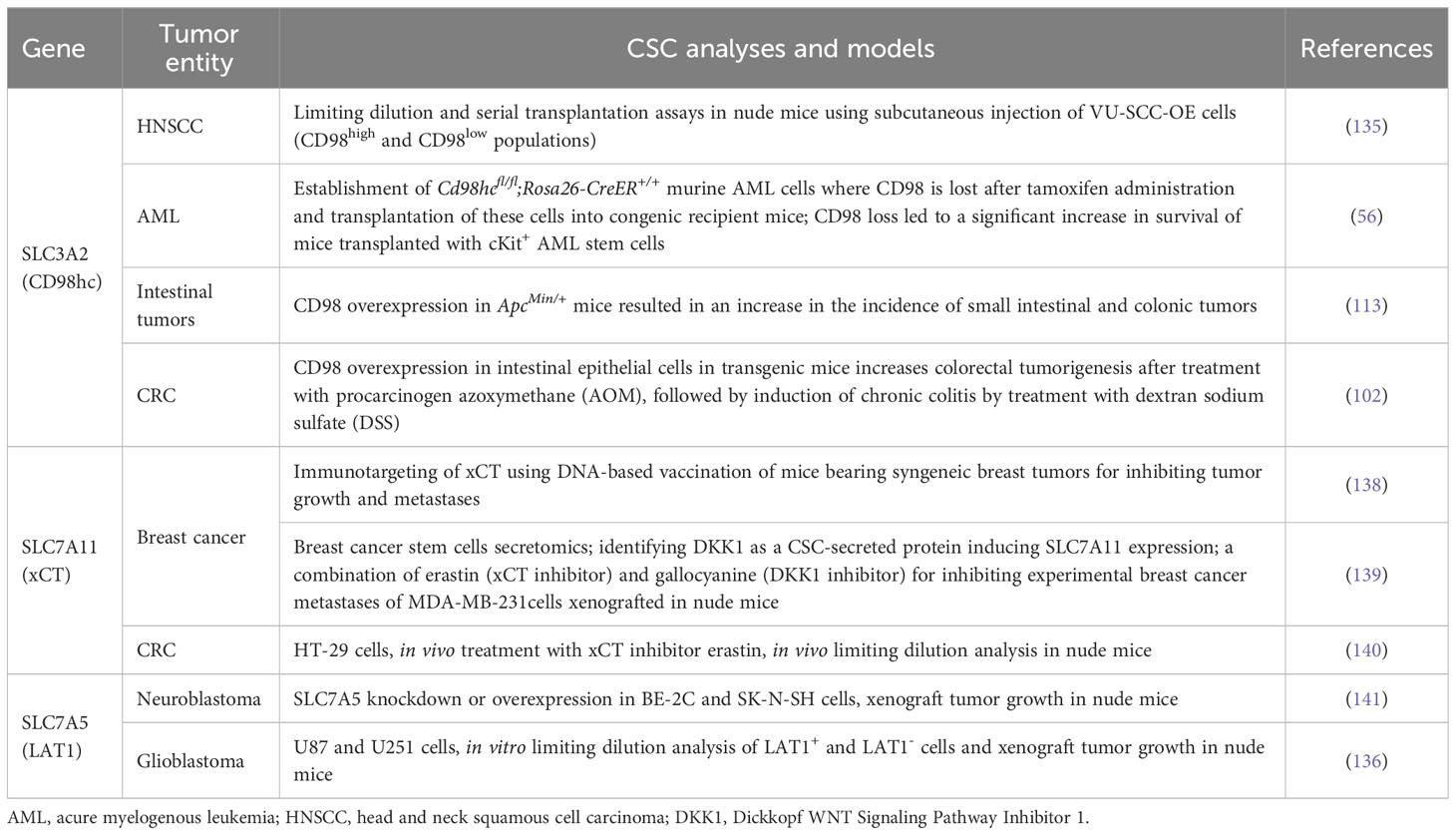
Table 2 CD98hc and its binding partners as cancer stem cell (CSC) markers and regulators (exemplary in vivo studies).
7 Clinical significance of CD98hc and CD98hc-associated proteins as potential prognostic markers and therapeutic targets
CD98hc as an oncogene has been correlated with the poor clinical prognosis of patients with different types of cancer (37, 38, 117, 148–154). Elevated CD98hc expression was identified as a prognostic marker for predicting a worse prognosis in patients with pulmonary pleomorphic carcinoma (PPC) (148), biliary tract cancer (149), gastric cancer (117), breast cancer (151), CRC (154), HNSCC (37, 152, 153) and pancreatic cancer (39) (Table 3). Our previous study also showed that high expression of CD98hc and LAT1 are associated with poor prognoses in patients with HNSCC treated with primary radiochemotherapy (RCTx) or postoperative radiochemotherapy (PORT-C) (11, 43, 116). On the contrary, low CD98hc expression is an independent factor for predicting poor overall survival (OS) and progression-free survival (PFS) for patients with cutaneous angiosarcoma (CA) (156), although the small sample size is a limitation of this study since CA is a rare malignant tumor. Pan-cancer analysis revealed that CD98hc expression is an independent hazard factor for most cancer types (Figures 3B–D).
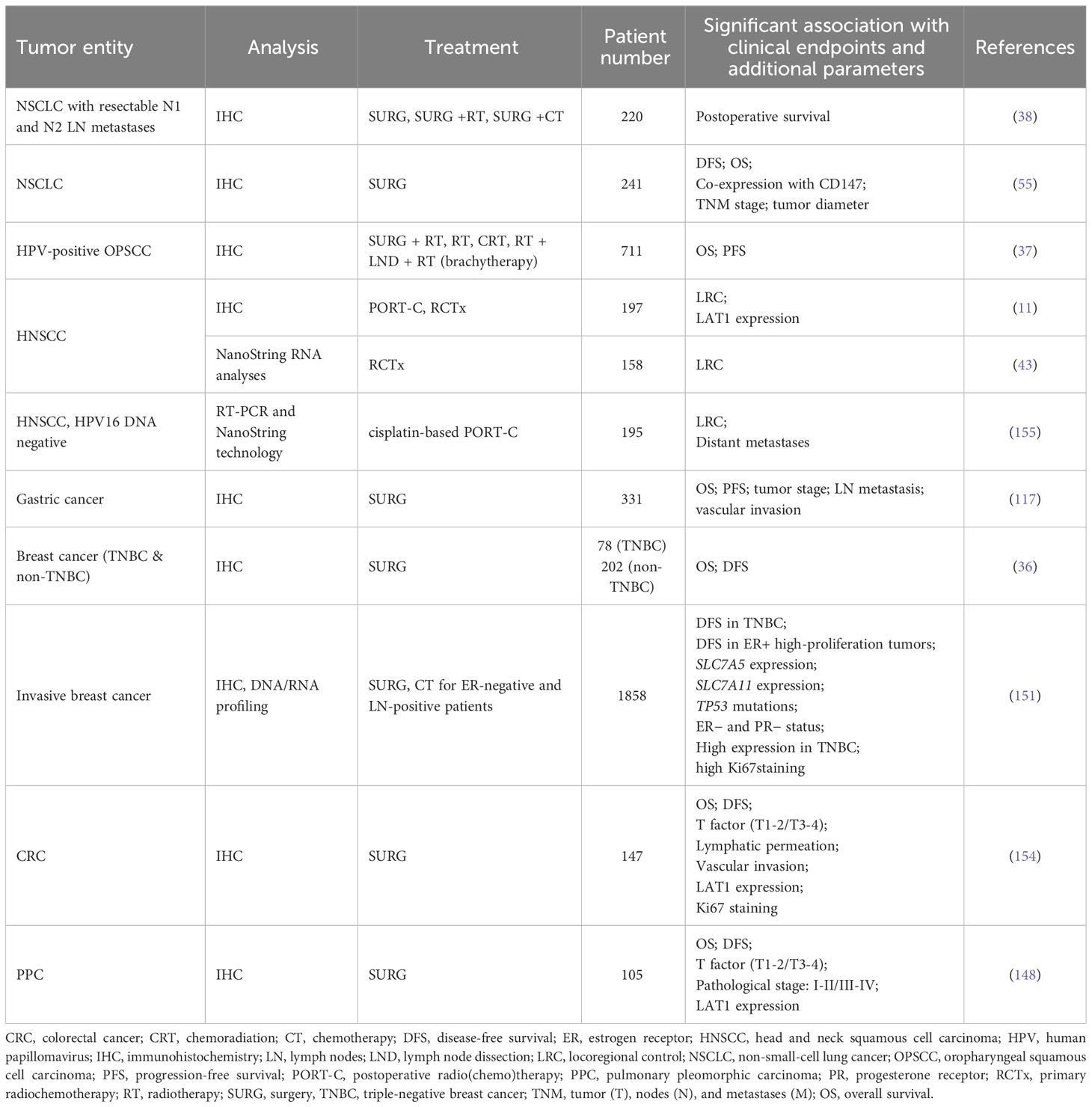
Table 3 Correlation of CD98hc expression with clinical outcomes in patients with malignant diseases (exemplary studies).
CD98hc is a potential target to improve the therapeutic effect of conventional therapies (157–160). We have demonstrated that CD98hc-associated signaling mechanisms, such as mTOR pathway activation, amino acid metabolism, oxidative stress, and DNA repair, play a central role in regulating HNSCC radioresistance (11, 160). Analysis of the expression of geneset associated with T- and B-cell activation in HNSCC revealed that it negatively correlates with SLC3A2 (CD98hc) expression, and therefore, CD98hc could be potentially associated with tumor immune evasion (157). Thus, strategies harnessing the immune system could be critical for the treatment of immunologic “cold” tumors with high CD98hc expression. A recent study from our group confirmed that CD98hc-redirected UniCAR T cells destroy radioresistant HNSCC spheroids (157). Although immune cells, including T cells, also express CD98hc, the expression level is substantially lower than in tumor cells, and the elimination of CD98hc tumor cells occurs before possible UniCAR T cell fratricide (157, 158). Furthermore, CD98hc regulates breast cancer cell sensitivity to anti-estrogen treatment. Co-expression of the CD98hc/LAT1 complex correlates with endocrine therapy resistance in patients with estrogen receptor (ER) positive/human epidermal growth factor receptor-2 (HER2) negative breast cancer. Depletion of both CD98hc and LAT1 mRNA upregulated the sensitivity of breast tumor cells to a selective estrogen receptor modulator tamoxifen (161). A cell polarity protein Scribble (SCRIB) and CD98hc form a quaternary complex with a mammalian homolog of Drosophila protein lethal giant larvae homolog 2 (LLGL2) and LAT1 to promote plasma membrane localization and stabilizing the amino acid transporters and promote cell proliferation and tamoxifen resistance in ER-positive breast cancer cells. Downregulation of CD98hc expression sensitizes tamoxifen-resistant breast cancer cells to tamoxifen under nutrient stress conditions (162). These studies suggest that CD98hc plays an essential role in endocrine therapy resistance.
Although the development of CD98hc as a therapeutic target is mainly at the stage of preclinical laboratory research, accumulating evidence shows the antitumor roles of CD98hc inhibition in a variety of cancer types (144, 145). Antibody-mediated CD98hc blockade deteriorates cell proliferation and tumor growth both in vitro and in vivo (56, 163, 164). Furthermore, immunotargeting of the CD98hc binding partners LAT-1 and xCT also has strong antitumor effects in the murine tumor models (138, 165). In 1986, Yagita et al. found that anti-CD98hc antibody specific for the extracellular domain inhibits lymphocyte proliferation (166). Later, Hayes et al. used in vivo phenotypic screening to identify anti-CD98hc antibodies with the most potent antitumor properties in vivo using different xenograft murine models for Burkitt’s lymphoma, leukemia, and patient-derived lung tumors (163). This study demonstrated that IGN523, a humanized monoclonal antibody, possesses a strong antitumor effect in both hematopoietic malignancies and solid tumors. The molecular mechanisms of the CD98hc-mediated antitumor activities include inhibition of the CD98hc-dependent amino acid transport, induction of antibody-dependent cellular cytotoxicity (ADCC), and increase in the lysosomal permeability leading to cell death (163). It has been evaluated in the early-phase clinical trial for acute myeloid leukemia (NCT02040506). In clinical studies, IGN523 was well tolerated and associated with modest antitumor activity as a single agent, whereas complete or partial responses were not observed for the treated individuals (167). Anti-CD98hc therapy can be potentially tested in solid tumors in the future in combination with standard treatment. Phase I study of another anti-CD98hc antibody, KHK2898 (NCT01516645), was carried out in patients with advanced solid tumors who no longer respond to standard therapy. However, no results were yet posted. A large screening of more than 10,000 monoclonal antibodies raised against multiple myeloma (MM) identified R8H283 antibody specific for the glycosylated form of CD98hc. R8H283 antibody does not bind CD98hc protein on normal hematopoietic cells and possesses a strong anti-MM effect in vitro and in vivo. The molecular mechanism of the R8H283-mediated anti-MM activities was attributed to ADCC and complement-dependent toxicity (164). A study by Tian et al. screened a phage-display library of single-chain variable fragments (scFvs) targeting CD98hc and identified anti-CD98hc antibody with pH-dependent binding and improved antitumor activity and pharmacokinetic properties in the experimental in vivo models (168).
A Phase I study of JPH203, a LAT1 inhibitor (UMIN000016546), in patients with advanced or refractory solid tumors demonstrated that the drug was well tolerated at low doses and had promising anti-tumor activity in patients with CRC and biliary tract cancer (BTC) (169). A phase II study for patients with advanced BTC (UMIN000034080) will provide more information on JPH203 safety and efficacy. Sulfasalazine is a FDA-approved drug primarily used for the treatment of ulcerative colitis (170) and rheumatoid arthritis (171). It also acts as an xCT inhibitor, reducing cystine uptake and glutathione synthesis (172). Sulfasalazine treatment induces ROS production and synergizes with radiation to increase DNA damage and death of glioblastoma cells in vitro (172), inhibits tumor growth in murine models (32), and sensitizes xenograft tumors to radiation (172). Sulfasalazine has been investigated in preclinical and early-phase clinical trials for its safety, drug–drug interactions, and potential anticancer effects for breast and CD44v-positive gastric cancer (173, 174). The treatment of patients with cisplatin refractory gastric cancer with sulfasalazine in combination with cisplatin did not show sufficient antitumor efficacy that could be partially explained by the metabolizing of the orally administered drug in the intestines and, therefore, decreasing its inhibitor potential, suggesting that alternative treatment route should be considered in future studies (174). Another xCT inhibitor, sorafenib, was initially approved by FDA in 2005 for the treatment of advanced renal cell carcinoma (RCC). Since then, it has also been approved for the treatment of hepatocellular carcinoma (HCC) and radio-iodine resistant advanced differentiated thyroid carcinoma (175–177). However, tumors often become drug resistant (178, 179). There are no CD98hc-specific chemical inhibitors available. Based on the results of in silico analysis by the Cancer Therapeutics Response Portal (CTRP), CD98hc can be potentially susceptible to some repurposed inhibitors, such as thioredoxin-1 (Trx-1) inhibitor PX-12, GPX-4 inhibitors ML162 and ML-210, inhibitor of GPX-4 and ferroptosis activator (1S,3R)-RSL3, and PRIMA-1 (P53-dependent reactivation and induction of massive apoptosis) compound (Figure 4).
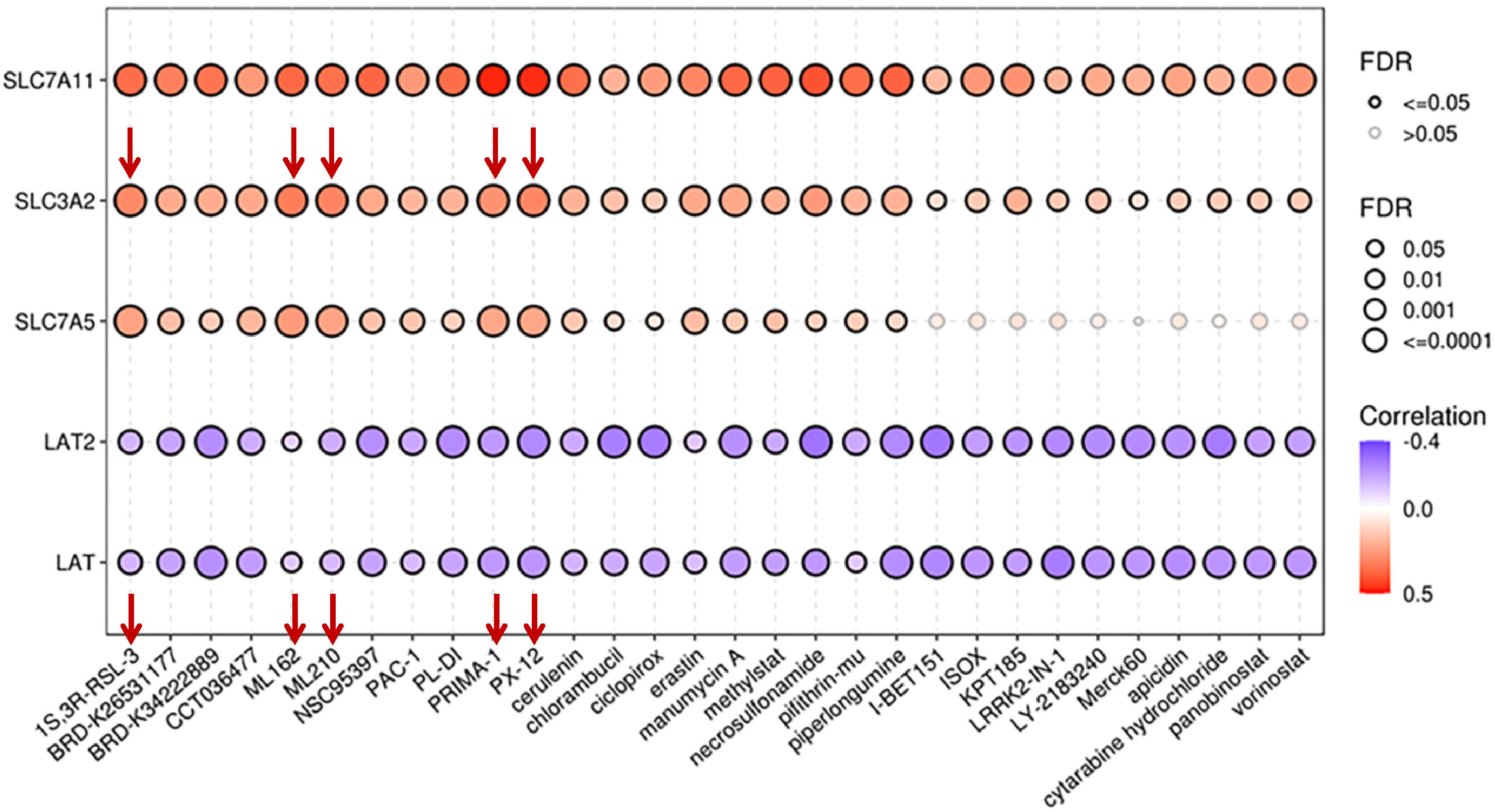
Figure 4 Correlation between drug sensitivity and mRNA expression levels of CD98hc and its binding partners. The data are obtained using the GSCALite platform (98) based on the Cancer Therapeutics Response Portal (CTRP). FDR, false discovery rate.
Anti-CD98hc radiopharmaceuticals are a promising approach for tumor imaging. Deuschle et al. have developed a high-affinity Anticalin, an engineered protein molecule binding CD98hc with a picomolar affinity for non-invasive biomedical imaging (180). Furthermore, compared with existing tumor-specific positron emission tomography (PET) probes, the LAT1-specific PET probe improved specificity for the early-phase diagnostic application and evaluation of tumor therapy response. LAT1-specific PET probe 18F-FIMP has been recently tested in clinics for patients with glioblastoma and demonstrated higher specificity for tumors compared to the 11C-MET and [18F]Fluorodeoxyglucose (18F-FDG) based imaging (181, 182). All this evidence indicates that CD98hc and its binding partners are particularly well-suited targets for diagnostic evaluation and therapeutic intervention in cancers.
8 Future perspectives
An accumulating body of evidence has confirmed the contribution of the CD98hc-mediated mechanisms in cancer initiation and progression. The ongoing clinical studies aim to validate the role of CD98hc as a marker of tumor diagnosis and prognosis. Preclinical studies demonstrated that CD98hc is a potent regulator of tumor cell proliferation, invasion, and therapy resistance and a potential target for cancer treatment. Immunotargeting CD98hc demonstrated promising results in vitro and in vivo. Targeting the CD98hc-binding amino acid transporters is also an additional treatment option, although its antitumor efficacy could be challenged by the previously described transporter plasticity and redundancy (183). Understanding the mechanisms of CD98hc interplay with its partners, such as integrins and CD147, is highly important, and targeting these mechanisms could be another therapeutic option. Although CD98hc-positive cells in HNSCC and oropharyngeal cancer have stem cell properties, it is still a matter of debate whether CD98hc can serve as a marker of CSCs and what its role in the regulation of the tumor self-renewal and differentiation. More evidence from in vivo transplantation assays and patient-derived tumor models would be needed to address these questions. Nevertheless, the levels of CD98hc expression were correlated with clinical outcomes for different tumor entities, suggesting that tumor cells with high CD98hc expression have survival advantages after conventional treatment compared to their counterparts with low CD98hc expression. The ongoing biological studies and further clinical trials for the combination of CD98hc-targeted treatment and conventional therapy, including radiotherapy, might bring breakthroughs in the laboratory research and clinical application of CD98hc in the near future.
Author contributions
XP and AD wrote, edited and approved the final manuscript. All authors contributed to the article and approved the submitted version.
Funding
The research in AD group is supported by German Cancer Aid (Deutsche Krebshilfe), project ID: 70114659 and Deutsche Forschungsgemeinschaft (DFG) #416001651 and DFG SPP 2084: µBONE, #401326337 and #491692296.
Conflict of interest
The authors declare that the research was conducted in the absence of any commercial or financial relationships that could be construed as a potential conflict of interest.
Publisher’s note
All claims expressed in this article are solely those of the authors and do not necessarily represent those of their affiliated organizations, or those of the publisher, the editors and the reviewers. Any product that may be evaluated in this article, or claim that may be made by its manufacturer, is not guaranteed or endorsed by the publisher.
Glossary
References
1. Haynes BF, Hemler ME, Mann DL, Eisenbarth GS, Shelhamer J, Mostowski HS, et al. Characterization of a monoclonal antibody (4F2) that binds to human monocytes and to a subset of activated lymphocytes. J Immunol (1981) 126:1409–14. doi: 10.4049/jimmunol.126.4.1409
2. Lee Y, Wiriyasermkul P, Jin C, Quan L, Ohgaki R, Okuda S, et al. Cryo-EM structure of the human L-type amino acid transporter 1 in complex with glycoprotein CD98hc. Nat Struct Mol Biol (2019) 26:510–7. doi: 10.1038/s41594-019-0237-7
3. Kanai Y, Fukasawa Y, Cha SH, Segawa H, Chairoungdua A, Kim DK, Matsuo H, Kim JY, Miyamoto K, Takeda E, Endou H. Transport properties of a system y+L neutral and basic amino acid transporter. Insights into the mechanisms of substrate recognition. J Biol Chem (2000) 275:20787–93. doi: 10.1074/jbc.M000634200
4. Fotiadis D, Kanai Y, Palacin M. The SLC3 and SLC7 families of amino acid transporters. Mol Aspects Med (2013) 34:139–58. doi: 10.1016/j.mam.2012.10.007
5. Rodriguez CF, Escudero-Bravo P, Diaz L, Bartoccioni P, Garcia-Martin C, Gilabert JG, et al. Structural basis for substrate specificity of heteromeric transporters of neutral amino acids. Proc Natl Acad Sci U.S.A. (2021) 118:1–10. doi: 10.1073/pnas.2113573118
6. Prager GW, Feral CC, Kim C, Han J, Ginsberg MH. CD98hc (SLC3A2) interaction with the integrin beta subunit cytoplasmic domain mediates adhesive signaling. J Biol Chem (2007) 282:24477–84. doi: 10.1074/jbc.M702877200
7. Deora AA, Philp N, Hu J, Bok D, Rodriguez-Boulan E. Mechanisms regulating tissue-specific polarity of monocarboxylate transporters and their chaperone CD147 in kidney and retinal epithelia. Proc Natl Acad Sci U.S.A. (2005) 102:16245–50. doi: 10.1073/pnas.0504419102
8. Ohno H, Nakatsu Y, Sakoda H, Kushiyama A, Ono H, Fujishiro M, et al. 4F2hc stabilizes GLUT1 protein and increases glucose transport activity. Am J Physiol Cell Physiol (2011) 300:C1047–54. doi: 10.1152/ajpcell.00416.2010
9. de la Ballina LR, Cano-Crespo S, Gonzalez-Munoz E, Bial S, Estrach S, Cailleteau L, et al. Amino acid transport associated to cluster of differentiation 98 heavy chain (CD98hc) is at the cross-road of oxidative stress and amino acid availability. J Biol Chem (2016) 291:9700–11. doi: 10.1074/jbc.M115.704254
10. Cano-Crespo S, Chillaron J, Junza A, Fernandez-MIranda G, Garcia J, Polte C, et al. CD98hc (SLC3A2) sustains amino acid and nucleotide availability for cell cycle progression. Sci Rep (2019) 9:14065. doi: 10.1038/s41598-019-50547-9
11. Digomann D, Kurth I, Tyutyunnykova A, Chen O, Lock S, Gorodetska I, et al. The CD98 heavy chain is a marker and regulator of head and neck squamous cell carcinoma radiosensitivity. Clin Cancer Res (2019) 25:3152–63. doi: 10.1158/1078-0432.CCR-18-2951
12. Hara K, Kudoh H, Enomoto T, Hashimoto Y, Masuko T. Malignant transformation of NIH3T3 cells by overexpression of early lymphocyte activation antigen CD98. Biochem Biophys Res Commun (1999) 262:720–5. doi: 10.1006/bbrc.1999.1051
13. Shishido T, Uno S, Kamohara M, Tsuneoka-Suzuki T, Hashimoto Y, Enomoto T, et al. Transformation of BALB3T3 cells caused by over-expression of rat CD98 heavy chain (HC) requires its association with light chain: mis-sense mutation in a cysteine residue of CD98HC eliminates its transforming activity. Int J Cancer (2000) 87:311–6. doi: 10.1002/1097-0215(20000801)87:3<311::AID-IJC1>3.0.CO;2-W
14. Poettler M, Unseld M, Braemswig K, Haitel A, Zielinski CC, Prager GW. CD98hc (SLC3A2) drives integrin-dependent renal cancer cell behavior. Mol Cancer (2013) 12:169. doi: 10.1186/1476-4598-12-169
15. Wu F, Xiong G, Chen Z, Lei C, Liu Q, Bai Y. SLC3A2 inhibits ferroptosis in laryngeal carcinoma via mTOR pathway. Hereditas (2022) 159:6. doi: 10.1186/s41065-022-00225-0
16. Zhu B, Cheng D, Hou L, Zhou S, Ying T, Yang Q. SLC3A2 is upregulated in human osteosarcoma and promotes tumor growth through the PI3K/Akt signaling pathway. Oncol Rep (2017) 37:2575–82. doi: 10.3892/or.2017.5530
17. Shin DH, Lee D, Hong DW, Hong SH, Hwang JA, Lee BI, et al. Oncogenic function and clinical implications of SLC3A2-NRG1 fusion in invasive mucinous adenocarcinoma of the lung. Oncotarget (2016) 7:69450–65. doi: 10.18632/oncotarget.11913
18. Estrach S, Lee SA, Boulter E, Pisano S, Errante A, Tissot FS, et al. CD98hc (SLC3A2) loss protects against ras-driven tumorigenesis by modulating integrin-mediated mechanotransduction. Cancer Res (2014) 74:6878–89. doi: 10.1158/0008-5472.CAN-14-0579
19. Ma L, Zhang X, Yu K, Xu X, Chen T, Shi Y, et al. Targeting SLC3A2 subunit of system X(C)(-) is essential for m(6)A reader YTHDC2 to be an endogenous ferroptosis inducer in lung adenocarcinoma. Free Radic Biol Med (2021) 168:25–43. doi: 10.1016/j.freeradbiomed.2021.03.023
20. He J, Liu D, Liu M, Tang R, Zhang D. Characterizing the role of SLC3A2 in the molecular landscape and immune microenvironment across human tumors. Front Mol Biosci (2022) 9:961410. doi: 10.3389/fmolb.2022.961410
21. Cormerais Y, Pagnuzzi-Boncompagni M, Schrotter S, Giuliano S, Tambutte E, Endou H, et al. Inhibition of the amino-acid transporter LAT1 demonstrates anti-neoplastic activity in medulloblastoma. J Cell Mol Med (2019) 23:2711–8. doi: 10.1111/jcmm.14176
22. Shibasaki Y, Yokobori T, Sohda M, Shioi I, Ozawa N, Komine C, et al. Association of high LAT1 expression with poor prognosis and recurrence in colorectal cancer patients treated with oxaliplatin-based adjuvant chemotherapy. Int J Mol Sci (2023) 24:1–13. doi: 10.3390/ijms24032604
23. Shindo H, Harada-Shoji N, Ebata A, Sato M, Soga T, Miyashita M, et al. Targeting amino acid metabolic reprogramming via L-type amino acid transporter 1 (LAT1) for endocrine-resistant breast cancer. Cancers (Basel) (2021) 13:1–14. doi: 10.3390/cancers13174375
24. Liu Y, Ma G, Liu J, Zheng H, Huang G, Song Q, et al. SLC7A5 is a lung adenocarcinoma-specific prognostic biomarker and participates in forming immunosuppressive tumor microenvironment. Heliyon (2022) 8:e10866. doi: 10.1016/j.heliyon.2022.e10866
25. Yothaisong S, Dokduang H, Anzai N, Hayashi K, Namwat N, Yongvanit P, et al. Inhibition of l-type amino acid transporter 1 activity as a new therapeutic target for cholangiocarcinoma treatment. Tumour Biol (2017) 39:1010428317694545. doi: 10.1177/1010428317694545
26. Rosilio C, Nebout M, Imbert V, Griessinger E, Neffati Z, Benadiba J, et al. L-type amino-acid transporter 1 (LAT1): a therapeutic target supporting growth and survival of T-cell lymphoblastic lymphoma/T-cell acute lymphoblastic leukemia. Leukemia (2015) 29:1253–66. doi: 10.1038/leu.2014.338
27. Ghoochani A, Hsu EC, Aslan M, Rice MA, Nguyen HM, Brooks JD, et al. Ferroptosis inducers are a novel therapeutic approach for advanced prostate cancer. Cancer Res (2021) 81:1583–94. doi: 10.1158/0008-5472.CAN-20-3477
28. Xu F, Guan Y, Xue L, Zhang P, Li M, Gao M, et al. The roles of ferroptosis regulatory gene SLC7A11 in renal cell carcinoma: A multi-omics study. Cancer Med (2021) 10:9078–96. doi: 10.1002/cam4.4395
29. Wu Y, Sun X, Song B, Qiu X, Zhao J. MiR-375/SLC7A11 axis regulates oral squamous cell carcinoma proliferation and invasion. Cancer Med (2017) 6:1686–97. doi: 10.1002/cam4.1110
30. Jiang Y, Cui J, Cui M, Jing R. SLC7A11 promotes the progression of gastric cancer and regulates ferroptosis through PI3K/AKT pathway. Pathol Res Pract (2023) 248:154646. doi: 10.1016/j.prp.2023.154646
31. Dixon SJ, Patel DN, Welsch M, Skouta R, Lee ED, Hayano M, et al. Pharmacological inhibition of cystine-glutamate exchange induces endoplasmic reticulum stress and ferroptosis. Elife (2014) 3:e02523. doi: 10.7554/eLife.02523
32. Tsuchihashi K, Okazaki S, Ohmura M, Ishikawa M, Sampetrean O, Onishi N, et al. The EGF receptor promotes the Malignant potential of glioma by regulating amino acid transport system xc(-). Cancer Res (2016) 76:2954–63. doi: 10.1158/0008-5472.CAN-15-2121
33. Badgley MA, Kremer DM, Maurer HC, DelGiorno KE, Lee HJ, Purohit V, et al. Cysteine depletion induces pancreatic tumor ferroptosis in mice. Science (2020) 368:85–9. doi: 10.1126/science.aaw9872
34. Lang X, Green MD, Wang W, Yu J, Choi JE, Jiang L, et al. Radiotherapy and immunotherapy promote tumoral lipid oxidation and ferroptosis via synergistic repression of SLC7A11. Cancer Discovery (2019) 9:1673–85. doi: 10.1158/2159-8290.CD-19-0338
35. Liu D, An M, Wen G, Xing Y, Xia P. Both in situ and circulating SLC3A2 could be used as prognostic markers for human lung squamous cell carcinoma and lung adenocarcinoma. Cancers (Basel) (2022) 141–14. doi: 10.3390/cancers14215191
36. Ichinoe M, Mikami T, Yanagisawa N, Yoshida T, Hana K, Endou H, et al. Prognostic values of L-type amino acid transporter 1 and CD98hc expression in breast cancer. J Clin Pathol (2021) 74:589–95. doi: 10.1136/jclinpath-2020-206457
37. Rietbergen MM, Martens-de Kemp SR, Bloemena E, Witte BI, Brink A, Baatenburg de Jong RJ, et al. Cancer stem cell enrichment marker CD98: a prognostic factor for survival in patients with human papillomavirus-positive oropharyngeal cancer. Eur J Cancer (2014) 50:765–73. doi: 10.1016/j.ejca.2013.11.010
38. Kaira K, Oriuchi N, Imai H, Shimizu K, Yanagitani N, Sunaga N, et al. CD98 expression is associated with poor prognosis in resected non-small-cell lung cancer with lymph node metastases. Ann Surg Oncol (2009) 16:3473–81. doi: 10.1245/s10434-009-0685-0
39. Bianconi D, Fabian E, Herac M, Kieler M, Thaler J, Prager G, et al. Expression of CD98hc in pancreatic cancer and its role in cancer cell behavior. J Cancer (2022) 13:2271–80. doi: 10.7150/jca.70500
40. Haining Z, Kawai N, Miyake K, Okada M, Okubo S, Zhang X, et al. Relation of LAT1/4F2hc expression with pathological grade, proliferation and angiogenesis in human gliomas. BMC Clin Pathol (2012) 12:4. doi: 10.1186/1472-6890-12-4
41. Nawashiro H, Otani N, Shinomiya N, Fukui S, Ooigawa H, Shima K, et al. L-type amino acid transporter 1 as a potential molecular target in human astrocytic tumors. Int J Cancer (2006) 119:484–92. doi: 10.1002/ijc.21866
42. Xiao B, Viennois E, Chen Q, Wang L, Han MK, Zhang Y, et al. Silencing of intestinal glycoprotein CD98 by orally targeted nanoparticles enhances chemosensitization of colon cancer. ACS Nano (2018) 12:5253–65. doi: 10.1021/acsnano.7b08499
43. Linge A, Lohaus F, Lock S, Nowak A, Gudziol V, Valentini C, et al. HPV status, cancer stem cell marker expression, hypoxia gene signatures and tumour volume identify good prognosis subgroups in patients with HNSCC after primary radiochemotherapy: A multicentre retrospective study of the German Cancer Consortium Radiation Oncology Group (DKTK-ROG). Radiother Oncol (2016) 121:364–73. doi: 10.1016/j.radonc.2016.11.008
44. Sato Y, Heimeier RA, Li C, Deng C, Shi YB. Extracellular domain of CD98hc is required for early murine development. Cell Biosci (2011) 1:7. doi: 10.1186/2045-3701-1-7
45. Tsumura H, Suzuki N, Saito H, Kawano M, Otake S, Kozuka Y, et al. The targeted disruption of the CD98 gene results in embryonic lethality. Biochem Biophys Res Commun (2003) 308:847–51. doi: 10.1016/S0006-291X(03)01473-6
46. Feral CC, Zijlstra A, Tkachenko E, Prager G, Gardel ML, Slepak M, et al. CD98hc (SLC3A2) participates in fibronectin matrix assembly by mediating integrin signaling. J Cell Biol (2007) 178:701–11. doi: 10.1083/jcb.200705090
47. Fagerberg L, Hallström BM, Oksvold P, Kampf C, Djureinovic D, Odeberg J, et al. Analysis of the human tissue-specific expression by genome-wide integration of transcriptomics and antibody-based proteomics. Mol Cell Proteomics (2014) 13(2):397–406. doi: 10.1074/mcp.M113.035600
48. Fukasawa Y, Segawa H, Kim JY, Chairoungdua A, Kim DK, Matsuo H, et al. Identification and characterization of a Na(+)-independent neutral amino acid transporter that associates with the 4F2 heavy chain and exhibits substrate selectivity for small neutral D- and L-amino acids. J Biol Chem (2000) 275:9690–8. doi: 10.1074/jbc.275.13.9690
49. Tang Z, Li C, Kang B, Gao G, Li C, Zhang Z. GEPIA: a web server for cancer and normal gene expression profiling and interactive analyses. Nucleic Acids Res (2017) 45:W98–W102. doi: 10.1093/nar/gkx247
50. Fogelstrand P, Feral CC, Zargham R, Ginsberg MH. Dependence of proliferative vascular smooth muscle cells on CD98hc (4F2hc, SLC3A2). J Exp Med (2009) 206:2397–406. doi: 10.1084/jem.20082845
51. Burdo J, Dargusch R, Schubert D. Distribution of the cystine/glutamate antiporter system xc- in the brain, kidney, and duodenum. J Histochem Cytochem (2006) 54:549–57. doi: 10.1369/jhc.5A6840.2006
52. Boulter E, Estrach S, Errante A, Pons C, Cailleteau L, Tissot F, et al. CD98hc (SLC3A2) regulation of skin homeostasis wanes with age. J Exp Med (2013) 210:173–90. doi: 10.1084/jem.20121651
53. Park JC, Kim YB, Yoon JH, Kim HJ, Kim SM, Kanai Y, et al. Preferential expression of L-type amino acid transporter 1 in ameloblasts during rat tooth development. Anat Histol Embryol (2004) 33:119–24. doi: 10.1111/j.1439-0264.2003.00524.x
54. Feral CC, Nishiya N, Fenczik CA, Stuhlmann H, Slepak M, Ginsberg MH. CD98hc (SLC3A2) mediates integrin signaling. Proc Natl Acad Sci U.S.A. (2005) 102:355–60. doi: 10.1073/pnas.0404852102
55. Fei F, Li X, Xu L, Li D, Zhang Z, Guo X, et al. CD147-CD98hc complex contributes to poor prognosis of non-small cell lung cancer patients through promoting cell proliferation via the PI3K/Akt signaling pathway. Ann Surg Oncol (2014) 21:4359–68. doi: 10.1245/s10434-014-3816-1
56. Bajaj J, Konuma T, Lytle NK, Kwon HY, Ablack JN, Cantor JM, et al. CD98-mediated adhesive signaling enables the establishment and propagation of acute myelogenous leukemia. Cancer Cell (2016) 30:792–805. doi: 10.1016/j.ccell.2016.10.003
57. Henderson NC, Collis EA, Mackinnon AC, Simpson KJ, Haslett C, Zent R, et al. CD98hc (SLC3A2) interaction with beta 1 integrins is required for transformation. J Biol Chem (2004) 279:54731–41. doi: 10.1074/jbc.M408700200
58. Nakamura E, Sato M, Yang H, Miyagawa F, Harasaki M, Tomita K, et al. 4F2 (CD98) heavy chain is associated covalently with an amino acid transporter and controls intracellular trafficking and membrane topology of 4F2 heterodimer. J Biol Chem (1999) 274:3009–16. doi: 10.1074/jbc.274.5.3009
59. Rosell A, Meury M, Alvarez-Marimon E, Costa M, Perez-Cano L, Zorzano A, et al. Structural bases for the interaction and stabilization of the human amino acid transporter LAT2 with its ancillary protein 4F2hc. Proc Natl Acad Sci U.S.A. (2014) 111:2966–71. doi: 10.1073/pnas.1323779111
60. Kanai Y, Segawa H, Miyamoto K, Uchino H, Takeda E, Endou H. Expression cloning and characterization of a transporter for large neutral amino acids activated by the heavy chain of 4F2 antigen (CD98). J Biol Chem (1998) 273:23629–32. doi: 10.1074/jbc.273.37.23629
61. Scalise M, Galluccio M, Console L, Pochini L, Indiveri C. The human SLC7A5 (LAT1): the intriguing histidine/large neutral amino acid transporter and its relevance to human health. Front Chem (2018) 6:243. doi: 10.3389/fchem.2018.00243
62. Console L, Scalise M, Salerno S, Scanga R, Giudice D, De Bartolo L, et al. N-glycosylation is crucial for trafficking and stability of SLC3A2 (CD98). Sci Rep (2022) 12:14570. doi: 10.1038/s41598-022-18779-4
63. Cormerais Y, Giuliano S, LeFloch R, Front B, Durivault J, Tambutte E, et al. Genetic disruption of the multifunctional CD98/LAT1 complex demonstrates the key role of essential amino acid transport in the control of mTORC1 and tumor growth. Cancer Res (2016) 76:4481–92. doi: 10.1158/0008-5472.CAN-15-3376
64. Krause G, Hinz KM. Thyroid hormone transport across L-type amino acid transporters: What can molecular modelling tell us? Mol Cell Endocrinol (2017) 458:68–75. doi: 10.1016/j.mce.2017.03.018
65. Moreno M, Giacco A, Di Munno C, Goglia F. Direct and rapid effects of 3,5-diiodo-L-thyronine (T2). Mol Cell Endocrinol (2017) 458:121–6. doi: 10.1016/j.mce.2017.02.012
66. Friesema EC, Docter R, Moerings EP, Verrey F, Krenning EP, Hennemann G, et al. Thyroid hormone transport by the heterodimeric human system L amino acid transporter. Endocrinology (2001) 142:4339–48. doi: 10.1210/endo.142.10.8418
67. Sperandeo MP, Paladino S, Maiuri L, Maroupulos GD, Zurzolo C, Taglialatela M, et al. A y(+)LAT-1 mutant protein interferes with y(+)LAT-2 activity: implications for the molecular pathogenesis of lysinuric protein intolerance. Eur J Hum Genet (2005) 13:628–34. doi: 10.1038/sj.ejhg.5201376
68. Rotoli BM, Barilli A, Visigalli R, Ferrari F, Dall'Asta V. y+LAT1 and y+LAT2 contribution to arginine uptake in different human cell models: Implications in the pathophysiology of Lysinuric Protein Intolerance. J Cell Mol Med (2020) 24:921–9. doi: 10.1111/jcmm.14801
69. Bridges RJ, Natale NR, Patel SA. System xc(-) cystine/glutamate antiporter: an update on molecular pharmacology and roles within the CNS. Br J Pharmacol (2012) 165:20–34. doi: 10.1111/j.1476-5381.2011.01480.x
70. Forman HJ, Zhang H, Rinna A. Glutathione: overview of its protective roles, measurement, and biosynthesis. Mol Aspects Med (2009) 30:1–12. doi: 10.1016/j.mam.2008.08.006
71. Kahya U, Koseer AS, Dubrovska A. Amino acid transporters on the guard of cell genome and epigenome. Cancers (Basel) (2021) 13:1–33. doi: 10.3390/cancers13010125
72. Mikou A, Cabaye A, Goupil A, Bertrand HO, Mothet JP, Acher FC. Asc-1 transporter (SLC7A10): homology models and molecular dynamics insights into the first steps of the transport mechanism. Sci Rep (2020) 10:3731. doi: 10.1038/s41598-020-60617-y
73. D'Agostino S, Lanzillotta D, Varano M, Botta C, Baldrini A, Bilotta A, et al. The receptor protein tyrosine phosphatase PTPRJ negatively modulates the CD98hc oncoprotein in lung cancer cells. Oncotarget (2018) 9:23334–48. doi: 10.18632/oncotarget.25101
74. Xu D, Hemler ME. Metabolic activation-related CD147-CD98 complex. Mol Cell Proteomics (2005) 4:1061–71. doi: 10.1074/mcp.M400207-MCP200
75. Wu B, Wang Y, Yang XM, Xu BQ, Feng F, Wang B, et al. Basigin-mediated redistribution of CD98 promotes cell spreading and tumorigenicity in hepatocellular carcinoma. J Exp Clin Cancer Res (2015) 34:110. doi: 10.1186/s13046-015-0226-6
76. Yang H, Zou W, Li Y, Chen B, Xin X. Bridge linkage role played by CD98hc of anti-tumor drug resistance and cancer metastasis on cisplatin-resistant ovarian cancer cells. Cancer Biol Ther (2007) 6:942–7. doi: 10.4161/cbt.6.6.4190
77. Guo N, Zhang K, Lv M, Miao J, Chen Z, Zhu P. CD147 and CD98 complex-mediated homotypic aggregation attenuates the CypA-induced chemotactic effect on Jurkat T cells. Mol Immunol (2015) 63:253–63. doi: 10.1016/j.molimm.2014.07.005
78. Santiago-Gomez A, Barrasa JI, Olmo N, Lecona E, Burghardt H, Palacin M, et al. 4F2hc-silencing impairs tumorigenicity of HeLa cells via modulation of galectin-3 and beta-catenin signaling, and MMP-2 expression. Biochim Biophys Acta (2013) 1833:2045–56. doi: 10.1016/j.bbamcr.2013.04.017
79. Cantor J, Browne CD, Ruppert R, Feral CC, Fassler R, Rickert RC, et al. CD98hc facilitates B cell proliferation and adaptive humoral immunity. Nat Immunol (2009) 10:412–9. doi: 10.1038/ni.1712
80. Cantor J, Slepak M, Ege N, Chang JT, Ginsberg MH. Loss of T cell CD98 H chain specifically ablates T cell clonal expansion and protects from autoimmunity. J Immunol (2011) 187:851–60. doi: 10.4049/jimmunol.1100002
81. Diaz LA Jr., Friedman AW, He X, Kuick RD, Hanash SM, Fox DA. Monocyte-dependent regulation of T lymphocyte activation through CD98. Int Immunol (1997) 9:1221–31. doi: 10.1093/intimm/9.9.1221
82. Liu Z, Hou J, Chen J, Tsumura H, Ito M, Ito Y, et al. Deletion of CD98 heavy chain in T cells results in cardiac allograft acceptance by increasing regulatory T cells. Transplantation (2012) 93:1116–24. doi: 10.1097/TP.0b013e31824fd7cd
83. Geng J, Chen R, Yang FF, Lin P, Zhu YM, Fu X, et al. CD98-induced CD147 signaling stabilizes the Foxp3 protein to maintain tissue homeostasis. Cell Mol Immunol (2021) 18:2618–31. doi: 10.1038/s41423-021-00785-7
84. Tsumura H, Ito M, Takami M, Arai M, Li XK, Hamatani T, et al. Conditional deletion of CD98hc inhibits osteoclast development. Biochem Biophys Rep (2016) 5:203–10. doi: 10.1016/j.bbrep.2015.11.023
85. Kim MY, Cho JY. Molecular association of CD98, CD29, and CD147 critically mediates monocytic U937 cell adhesion. Korean J Physiol Pharmacol (2016) 20:515–23. doi: 10.4196/kjpp.2016.20.5.515
86. Colavita I, Nigro E, Sarnataro D, Scudiero O, Granata V, Daniele A, et al. Membrane protein 4F2/CD98 is a cell surface receptor involved in the internalization and trafficking of human beta-Defensin 3 in epithelial cells. Chem Biol (2015) 22:217–28. doi: 10.1016/j.chembiol.2014.11.020
87. Adyns L, Proost P, Struyf S. Role of defensins in tumor biology. Int J Mol Sci (2023) 24:1–16. doi: 10.3390/ijms24065268
88. Ito Y, Komada H, Kusagawa S, Tsurudome M, Matsumura H, Kawano M, et al. Fusion regulation proteins on the cell surface: isolation and characterization of monoclonal antibodies which enhance giant polykaryocyte formation in Newcastle disease virus-infected cell lines of human origin. J Virol (1992) 66:5999–6007. doi: 10.1128/jvi.66.10.5999-6007.1992
89. Vection S, O'Callaghan D, Keriel A. CD98hc in host-pathogen interactions: roles of the multifunctional host protein during infections. FEMS Microbiol Rev (2022) 46:1–10. doi: 10.1093/femsre/fuac023
90. Schroeder N, Chung CS, Chen CH, Liao CL, Chang W. The lipid raft-associated protein CD98 is required for vaccinia virus endocytosis. J Virol (2012) 86:4868–82. doi: 10.1128/JVI.06610-11
91. Bragazzi Cunha J, Wobus CE. Select membrane proteins modulate MNV-1 infection of macrophages and dendritic cells in a cell type-specific manner. Virus Res (2016) 222:64–70. doi: 10.1016/j.virusres.2016.06.001
92. Gupta S, Singh S, Popovici J, Roesch C, Shakri AR, Guillotte-Blisnick M, et al. Targeting a reticulocyte binding protein and duffy binding protein to inhibit reticulocyte invasion by plasmodium vivax. Sci Rep (2018) 8:10511. doi: 10.1038/s41598-018-28757-4
93. Malleret B, El Sahili A, Tay MZ, Carissimo G, Ong ASM, Novera W, et al. Plasmodium vivax binds host CD98hc (SLC3A2) to enter immature red blood cells. Nat Microbiol (2021) 6:991–9. doi: 10.1038/s41564-021-00939-3
94. Hirohata Y, Arii J, Liu Z, Shindo K, Oyama M, Kozuka-Hata H, et al. Herpes simplex virus 1 recruits CD98 heavy chain and beta1 integrin to the nuclear membrane for viral de-envelopment. J Virol (2015) 89:7799–812. doi: 10.1128/JVI.00741-15
95. Arii J. Host and viral factors involved in nuclear egress of herpes simplex virus 1. Viruses (2021) 13:1–17. doi: 10.3390/v13050754
96. Veettil MV, Sadagopan S, Sharma-Walia N, Wang FZ, Raghu H, Varga L, et al. Kaposi's sarcoma-associated herpesvirus forms a multimolecular complex of integrins (alphaVbeta5, alphaVbeta3, and alpha3beta1) and CD98-xCT during infection of human dermal microvascular endothelial cells, and CD98-xCT is essential for the postentry stage of infection. J Virol (2008) 82:12126–44. doi: 10.1128/JVI.01146-08
97. Keriel A, Botella E, Estrach S, Bragagnolo G, Vergunst AC, Feral CC, et al. Brucella intracellular life relies on the transmembrane protein CD98 heavy chain. J Infect Dis (2015) 211:1769–78. doi: 10.1093/infdis/jiu673
98. Liu CJ, Hu FF, Xia MX, Han L, Zhang Q, Guo AY. GSCALite: a web server for gene set cancer analysis. Bioinformatics (2018) 34(21):3771–3772. doi: 10.1093/bioinformatics/bty411
99. Zhou XY, Li JY, Tan JT, HuangLi YL, Nie XC, Xia P. Clinical significance of the CD98hc-CD147 complex in ovarian cancer: a bioinformatics analysis. J Obstet Gynaecol (2023) 43:2188085. doi: 10.1080/01443615.2023.2188085
100. Cantor JM, Ginsberg MH. CD98 at the crossroads of adaptive immunity and cancer. J Cell Sci (2012) 125:1373–82. doi: 10.1242/jcs.096040
101. Ablack JN, Metz PJ, Chang JT, Cantor JM, Ginsberg MH. Ubiquitylation of CD98 limits cell proliferation and clonal expansion. J Cell Sci (2015) 128:4273–8. doi: 10.1242/jcs.178129
102. Nguyen HT, Dalmasso G, Torkvist L, Halfvarson J, Yan Y, Laroui H, et al. CD98 expression modulates intestinal homeostasis, inflammation, and colitis-associated cancer in mice. J Clin Invest (2011) 121:1733–47. doi: 10.1172/JCI44631
103. Huang G, Ma L, Shen L, Lei Y, Guo L, Deng Y, et al. MIF/SCL3A2 depletion inhibits the proliferation and metastasis of colorectal cancer cells via the AKT/GSK-3beta pathway and cell iron death. J Cell Mol Med (2022) 26:3410–22. doi: 10.1111/jcmm.17352
104. Bulus N, Feral C, Pozzi A, Zent R. CD98 increases renal epithelial cell proliferation by activating MAPKs. PloS One (2012) 7:e40026. doi: 10.1371/journal.pone.0040026
105. Hara K, Ueda S, Ohno Y, Tanaka T, Yagi H, Okazaki S, et al. NIH3T3 cells overexpressing CD98 heavy chain resist early G1 arrest and apoptosis induced by serum starvation. Cancer Sci (2012) 103:1460–6. doi: 10.1111/j.1349-7006.2012.02304.x
106. Tian S, Chu Y, Hu J, Ding X, Liu Z, Fu D, et al. Tumour-associated neutrophils secrete AGR2 to promote colorectal cancer metastasis via its receptor CD98hc-xCT. Gut (2022) 71:2489–501. doi: 10.1136/gutjnl-2021-325137
107. Mouw JK, Ou G, Weaver VM. Extracellular matrix assembly: a multiscale deconstruction. Nat Rev Mol Cell Biol (2014) 15:771–85. doi: 10.1038/nrm3902
108. Zhao X, Psarianos P, Ghoraie LS, Yip K, Goldstein D, Gilbert R, et al. Metabolic regulation of dermal fibroblasts contributes to skin extracellular matrix homeostasis and fibrosis. Nat Metab (2019) 1:147–57. doi: 10.1038/s42255-018-0008-5
109. Le Vee M, Jouan E, Lecureur V, Fardel O. Aryl hydrocarbon receptor-dependent up-regulation of the heterodimeric amino acid transporter LAT1 (SLC7A5)/CD98hc (SLC3A2) by diesel exhaust particle extract in human bronchial epithelial cells. Toxicol Appl Pharmacol (2016) 290:74–85. doi: 10.1016/j.taap.2015.11.014
110. Karamanos NK, Theocharis AD, Neill T, Iozzo RV. Matrix modeling and remodeling: A biological interplay regulating tissue homeostasis and diseases. Matrix Biol (2019) 75-76:1–11. doi: 10.1016/j.matbio.2018.08.007
111. Quintero-Fabian S, Arreola R, Becerril-Villanueva E, Torres-Romero JC, Arana-Argaez V, Lara-Riegos J, et al. Role of matrix metalloproteinases in angiogenesis and cancer. Front Oncol (2019) 9:1370. doi: 10.3389/fonc.2019.01370
112. Hynes RO. Integrins: bidirectional, allosteric signaling machines. Cell (2002) 110:673–87. doi: 10.1016/S0092-8674(02)00971-6
113. Nguyen HT, Dalmasso G, Yan Y, Laroui H, Charania M, Ingersoll S, et al. Intestinal epithelial cell-specific CD98 expression regulates tumorigenesis in Apc(Min/+) mice. Lab Invest (2012) 92:1203–12. doi: 10.1038/labinvest.2012.83
114. Kaira K, Oriuchi N, Imai H, Shimizu K, Yanagitani N, Sunaga N, et al. l-type amino acid transporter 1 and CD98 expression in primary and metastatic sites of human neoplasms. Cancer Sci (2008) 99:2380–6. doi: 10.1111/j.1349-7006.2008.00969.x
115. Wang Q, Tiffen J, Bailey CG, Lehman ML, Ritchie W, Fazli L, et al. Targeting amino acid transport in metastatic castration-resistant prostate cancer: effects on cell cycle, cell growth, and tumor development. J Natl Cancer Inst (2013) 105:1463–73. doi: 10.1093/jnci/djt241
116. Linge A, Lock S, Krenn C, Appold S, Lohaus F, Nowak A, et al. Independent validation of the prognostic value of cancer stem cell marker expression and hypoxia-induced gene expression for patients with locally advanced HNSCC after postoperative radiotherapy. Clin Transl Radiat Oncol (2016) 1:19–26. doi: 10.1016/j.ctro.2016.10.002
117. Satoh T, Kaira K, Takahashi K, Takahashi N, Kanai Y, Asao T, et al. Prognostic significance of the expression of CD98 (4F2hc) in gastric cancer. Anticancer Res (2017) 37:631–6. doi: 10.21873/anticanres.11357
118. Hirschhorn T, Stockwell BR. The development of the concept of ferroptosis. Free Radic Biol Med (2019) 133:130–43. doi: 10.1016/j.freeradbiomed.2018.09.043
119. Wang C, Zheng C, Wang H, Shui S, Jin H, Liu G, et al. Dual degradation mechanism of GPX4 degrader in induction of ferroptosis exerting anti-resistant tumor effect. Eur J Med Chem (2023) 247:115072. doi: 10.1016/j.ejmech.2022.115072
120. Du Y, Guo Z. Recent progress in ferroptosis: inducers and inhibitors. Cell Death Discovery (2022) 8:501. doi: 10.1038/s41420-022-01297-7
121. Hu Z, Yin Y, Jiang J, Yan C, Wang Y, Wang D, et al. Exosomal miR-142-3p secreted by hepatitis B virus (HBV)-hepatocellular carcinoma (HCC) cells promotes ferroptosis of M1-type macrophages through SLC3A2 and the mechanism of HCC progression. J Gastrointest Oncol (2022) 13:754–67. doi: 10.21037/jgo-21-916
122. Liu J, Xia X, Huang P. xCT: A critical molecule that links cancer metabolism to redox signaling. Mol Ther (2020) 28:2358–66. doi: 10.1016/j.ymthe.2020.08.021
123. Chen L, Qiao L, Bian Y, Sun X. GDF15 knockdown promotes erastin-induced ferroptosis by decreasing SLC7A11 expression. Biochem Biophys Res Commun (2020) 526:293–9. doi: 10.1016/j.bbrc.2020.03.079
124. Cable J, Pei D, Reid LM, Wang XW, Bhatia S, Karras P, et al. Cancer stem cells: advances in biology and clinical translation-a Keystone Symposia report. Ann N Y Acad Sci (2021) 1506:142–63. doi: 10.1111/nyas.14719
125. Kreso A, Dick JE. Evolution of the cancer stem cell model. Cell Stem Cell (2014) 14:275–91. doi: 10.1016/j.stem.2014.02.006
126. Batlle E, Clevers H. Cancer stem cells revisited. Nat Med (2017) 23:1124–34. doi: 10.1038/nm.4409
127. Perez-Gonzalez A, Bevant K, Blanpain C. Cancer cell plasticity during tumor progression, metastasis and response to therapy. Nat Cancer (2023) 4:1063–82. doi: 10.1038/s43018-023-00595-y
128. Krause M, Dubrovska A, Linge A, Baumann M. Cancer stem cells: Radioresistance, prediction of radiotherapy outcome and specific targets for combined treatments. Adv Drug Delivery Rev (2017) 109:63–73. doi: 10.1016/j.addr.2016.02.002
129. Peitzsch C, Tyutyunnykova A, Pantel K, Dubrovska A. Cancer stem cells: The root of tumor recurrence and metastases. Semin Cancer Biol (2017) 44:10–24. doi: 10.1016/j.semcancer.2017.02.011
130. Chen J, Li Y, Yu TS, McKay RM, Burns DK, Kernie SG, et al. A restricted cell population propagates glioblastoma growth after chemotherapy. Nature (2012) 488:522–6. doi: 10.1038/nature11287
131. Zhou HM, Zhang JG, Zhang X, Li Q. Targeting cancer stem cells for reversing therapy resistance: mechanism, signaling, and prospective agents. Signal Transduct Target Ther (2021) 6:62. doi: 10.1038/s41392-020-00430-1
132. Butof R, Dubrovska A, Baumann M. Clinical perspectives of cancer stem cell research in radiation oncology. Radiother Oncol (2013) 108:388–96. doi: 10.1016/j.radonc.2013.06.002
133. Skvortsov S, Skvortsova II, Tang DG, Dubrovska A. Concise review: prostate cancer stem cells: current understanding. Stem Cells (2018) 36:1457–74. doi: 10.1002/stem.2859
134. Liu YH, Li YL, Shen HT, Chien PJ, Sheu GT, Wang BY, et al. L-type amino acid transporter 1 regulates cancer stemness and the expression of programmed cell death 1 ligand 1 in lung cancer cells. Int J Mol Sci (2021) 22:1–15. doi: 10.3390/ijms222010955
135. Martens-de Kemp SR, Brink A, Stigter-van Walsum M, Damen JM, Rustenburg F, Wu T, et al. CD98 marks a subpopulation of head and neck squamous cell carcinoma cells with stem cell properties. Stem Cell Res (2013) 10:477–88. doi: 10.1016/j.scr.2013.02.004
136. Wang X, Chen J, Liu XH, Zeng XY, Long QY, Liu YH, et al. Evaluation of CD98 light chain-LAT1 as a potential marker of cancer stem-like cells in glioblastoma. Biochim Biophys Acta Mol Cell Res (2022) 1869:119303. doi: 10.1016/j.bbamcr.2022.119303
137. Polewski MD, Reveron-Thornton RF, Cherryholmes GA, Marinov GK, Aboody KS. SLC7A11 overexpression in glioblastoma is associated with increased cancer stem cell-like properties. Stem Cells Dev (2017) 26:1236–46. doi: 10.1089/scd.2017.0123
138. Lanzardo S, Conti L, Rooke R, Ruiu R, Accart N, Bolli E, et al. Immunotargeting of antigen xCT attenuates stem-like cell behavior and metastatic progression in breast cancer. Cancer Res (2016) 76:62–72. doi: 10.1158/0008-5472.CAN-15-1208
139. Wu M, Zhang X, Zhang W, Chiou YS, Qian W, Liu X, et al. Cancer stem cell regulated phenotypic plasticity protects metastasized cancer cells from ferroptosis. Nat Commun (2022) 13:1371. doi: 10.1038/s41467-022-29018-9
140. Xu X, Zhang X, Wei C, Zheng D, Lu X, Yang Y, et al. Targeting SLC7A11 specifically suppresses the progression of colorectal cancer stem cells via inducing ferroptosis. Eur J Pharm Sci (2020) 152:105450. doi: 10.1016/j.ejps.2020.105450
141. Yue M, Jiang J, Gao P, Liu H, Qing G. Oncogenic MYC activates a feedforward regulatory loop promoting essential amino acid metabolism and tumorigenesis. Cell Rep (2017) 21:3819–32. doi: 10.1016/j.celrep.2017.12.002
142. Rycaj K, Tang DG. Cell-of-origin of cancer versus cancer stem cells: assays and interpretations. Cancer Res (2015) 75:4003–11. doi: 10.1158/0008-5472.CAN-15-0798
143. Huang W, Hu H, Zhang Q, Wu X, Wei F, Yang F, et al. Regulatory networks in mechanotransduction reveal key genes in promoting cancer cell stemness and proliferation. Oncogene (2019) 38:6818–34. doi: 10.1038/s41388-019-0925-0
144. Ishimoto T, Nagano O, Yae T, Tamada M, Motohara T, Oshima H, et al. CD44 variant regulates redox status in cancer cells by stabilizing the xCT subunit of system xc(-) and thereby promotes tumor growth. Cancer Cell (2011) 19:387–400. doi: 10.1016/j.ccr.2011.01.038
145. Lu H, Samanta D, Xiang L, Zhang H, Hu H, Chen I, et al. Chemotherapy triggers HIF-1-dependent glutathione synthesis and copper chelation that induces the breast cancer stem cell phenotype. Proc Natl Acad Sci U.S.A. (2015) 112:E4600–9. doi: 10.1073/pnas.1513433112
146. Wang X, Chen Y, Wang X, Tian H, Wang Y, Jin J, et al. Stem cell factor SOX2 confers ferroptosis resistance in lung cancer via upregulation of SLC7A11. Cancer Res (2021) 81:5217–29. doi: 10.1158/0008-5472.CAN-21-0567
147. Yoshikawa M, Tsuchihashi K, Ishimoto T, Yae T, Motohara T, Sugihara E, et al. xCT inhibition depletes CD44v-expressing tumor cells that are resistant to EGFR-targeted therapy in head and neck squamous cell carcinoma. Cancer Res (2013) 73:1855–66. doi: 10.1158/0008-5472.CAN-12-3609-T
148. Kaira K, Kawashima O, Endoh H, Imaizumi K, Goto Y, Kamiyoshihara M, et al. Expression of amino acid transporter (LAT1 and 4F2hc) in pulmonary pleomorphic carcinoma. Hum Pathol (2019) 84:142–9. doi: 10.1016/j.humpath.2018.09.020
149. Kaira K, Sunose Y, Oriuchi N, Kanai Y, Takeyoshi I. CD98 is a promising prognostic biomarker in biliary tract cancer. Hepatobiliary Pancreat Dis Int (2014) 13:654–7. doi: 10.1016/S1499-3872(14)60278-2
150. Guo X, Li H, Fei F, Liu B, Li X, Yang H, et al. Genetic variations in SLC3A2/CD98 gene as prognosis predictors in non-small cell lung cancer. Mol Carcinog (2015) 54 Suppl 1:E52–60. doi: 10.1002/mc.22167
151. El Ansari R, Craze ML, Diez-Rodriguez M, Nolan CC, Ellis IO, Rakha EA, et al. The multifunctional solute carrier 3A2 (SLC3A2) confers a poor prognosis in the highly proliferative breast cancer subtypes. Br J Cancer (2018) 118:1115–22. doi: 10.1038/s41416-018-0038-5
152. Liang J, Sun Z. Overexpression of membranal SLC3A2 regulates the proliferation of oral squamous cancer cells and affects the prognosis of oral cancer patients. J Oral Pathol Med (2021) 50:371–7. doi: 10.1111/jop.13132
153. Toyoda M, Kaira K, Shino M, Sakakura K, Takahashi K, Takayasu Y, et al. CD98 as a novel prognostic indicator for patients with stage III/IV hypopharyngeal squamous cell carcinoma. Head Neck (2015) 37:1569–74. doi: 10.1002/hed.23797
154. Ogawa H, Kaira K, Motegi Y, Yokobori T, Takada T, Katoh R, et al. Role of amino acid transporter expression as a prognostic marker in patients with surgically resected colorectal cancer. Anticancer Res (2019) 39:2535–43. doi: 10.21873/anticanres.13375
155. Linge A, Lock S, Gudziol V, Nowak A, Lohaus F, von Neubeck C, et al. Low cancer stem cell marker expression and low hypoxia identify good prognosis subgroups in HPV(-) HNSCC after postoperative radiochemotherapy: a multicenter study of the DKTK-ROG. Clin Cancer Res (2016) 22:2639–49. doi: 10.1158/1078-0432.CCR-15-1990
156. Shimizu A, Kaira K, Okubo Y, Utsumi D, Yasuda M, Tominaga H, et al. Prognostic impact of LAT1 and CD98 expression in cutaneous angiosarcoma. Neoplasma (2017) 64:283–8. doi: 10.4149/neo_2017_216
157. Koseer AS, Loureiro LR, Jureczek J, Mitwasi N, Gonzalez Soto KE, Aepler J, et al. Validation of CD98hc as a therapeutic target for a combination of radiation and immunotherapies in head and neck squamous cell carcinoma. Cancers (Basel) (2022) 14:1–21. doi: 10.3390/cancers14071677
158. Arndt C, Loureiro LR, Feldmann A, Jureczek J, Bergmann R, Mathe D, et al. UniCAR T cell immunotherapy enables efficient elimination of radioresistant cancer cells. Oncoimmunology (2020) 9:1743036. doi: 10.1080/2162402X.2020.1743036
159. Nachef M, Ali AK, Almutairi SM, Lee SH. Targeting SLC1A5 and SLC3A2/SLC7A5 as a potential strategy to strengthen anti-tumor immunity in the tumor microenvironment. Front Immunol (2021) 12:624324. doi: 10.3389/fimmu.2021.624324
160. DigOmann D, Linge A, Dubrovska A. SLC3A2/CD98hc, autophagy and tumor radioresistance: a link confirmed. Autophagy (2019) 15:1850–1. doi: 10.1080/15548627.2019.1639302
161. Alfarsi LH, El-Ansari R, Craze ML, Masisi BK, Mohammed OJ, Ellis IO, et al. Co-expression effect of SLC7A5/SLC3A2 to predict response to endocrine therapy in oestrogen-receptor-positive breast cancer. Int J Mol Sci (2020) 21:1–15. doi: 10.3390/ijms21041407
162. Saito Y, Matsuda S, Ohnishi N, Endo K, Ashitani S, Ohishi M, et al. Polarity protein SCRIB interacts with SLC3A2 to regulate proliferation and tamoxifen resistance in ER+ breast cancer. Commun Biol (2022) 5:403. doi: 10.1038/s42003-022-03363-3
163. Hayes GM, Chinn L, Cantor JM, Cairns B, Levashova Z, Tran H, et al. Antitumor activity of an anti-CD98 antibody. Int J Cancer (2015) 137:710–20. doi: 10.1002/ijc.29415
164. Hasegawa K, Ikeda S, Yaga M, Watanabe K, Urakawa R, Iehara A, et al. Selective targeting of multiple myeloma cells with a monoclonal antibody recognizing the ubiquitous protein CD98 heavy chain. Sci Transl Med (2022) 14:eaax7706. doi: 10.1126/scitranslmed.aax7706
165. Ueda S, Hayashi H, Miyamoto T, Abe S, Hirai K, Matsukura K, et al. Anti-tumor effects of mAb against L-type amino acid transporter 1 (LAT1) bound to human and monkey LAT1 with dual avidity modes. Cancer Sci (2019) 110:674–85. doi: 10.1111/cas.13908
166. Yagita H, Hashimoto Y. Monoclonal antibodies that inhibit activation and proliferation of lymphocytes. II. Requisite role of the monoclonal antibody-defined antigen systems in activation and proliferation of human and rat lymphocytes. J Immunol (1986) 136:2062–8. doi: 10.4049/jimmunol.136.6.2062
167. Bixby D, Wieduwilt MJ, Akard LP, Khoury HJ, Becker PS, Van EH, et al. A phase I study of IGN523, a novel anti-CD98 monoclonal antibody in patients with relapsed or refractory acute myeloid leukemia (AML). Blood (2015) 126:3809. doi: 10.1182/blood.V126.23.3809.3809
168. Tian X, Liu X, Ding J, Wang F, Wang K, Liu J, et al. An anti-CD98 antibody displaying pH-dependent Fc-mediated tumour-specific activity against multiple cancers in CD98-humanized mice. Nat BioMed Eng (2023) 7:8–23. doi: 10.1038/s41551-022-00956-5
169. Okano N, Naruge D, Kawai K, Kobayashi T, Nagashima F, Endou H, et al. First-in-human phase I study of JPH203, an L-type amino acid transporter 1 inhibitor, in patients with advanced solid tumors. Invest New Drugs (2020) 38:1495–506. doi: 10.1007/s10637-020-00924-3
170. Ko CW, Singh S, Feuerstein JD, Falck-Ytter C, Falck-Ytter Y, Cross RK, et al. AGA clinical practice guidelines on the management of mild-to-moderate ulcerative colitis. Gastroenterology (2019) 156:748–64. doi: 10.1053/j.gastro.2018.12.009
171. James HM, Gillis D, Hissaria P, Lester S, Somogyi AA, Cleland LG, et al. Common polymorphisms in the folate pathway predict efficacy of combination regimens containing methotrexate and sulfasalazine in early rheumatoid arthritis. J Rheumatol (2008) 35:562–71.
172. Sleire L, Skeie BS, Netland IA, Forde HE, Dodoo E, Selheim F, et al. Drug repurposing: sulfasalazine sensitizes gliomas to gamma knife radiosurgery by blocking cystine uptake through system Xc-, leading to glutathione depletion. Oncogene (2015) 34:5951–9. doi: 10.1038/onc.2015.60
173. Wang X, Zhang ZY, Arora S, Hughes L, Wang J, Powers D, et al. Effects of rolapitant administered intravenously or orally on the pharmacokinetics of digoxin (P-glycoprotein substrate) and sulfasalazine (Breast cancer resistance protein substrate) in healthy volunteers. J Clin Pharmacol (2018) 58:202–11. doi: 10.1002/jcph.1005
174. Shitara K, Doi T, Nagano O, Fukutani M, Hasegawa H, Nomura S, et al. Phase 1 study of sulfasalazine and cisplatin for patients with CD44v-positive gastric cancer refractory to cisplatin (EPOC1407). Gastric Cancer (2017) 20:1004–9. doi: 10.1007/s10120-017-0720-y
175. Escudier B, Worden F, Kudo M. Sorafenib: key lessons from over 10 years of experience. Expert Rev Anticancer Ther (2019) 19:177–89. doi: 10.1080/14737140.2019.1559058
176. Cheng AL, Kang YK, Chen Z, Tsao CJ, Qin S, Kim JS, et al. Efficacy and safety of sorafenib in patients in the Asia-Pacific region with advanced hepatocellular carcinoma: a phase III randomised, double-blind, placebo-controlled trial. Lancet Oncol (2009) 10:25–34. doi: 10.1016/S1470-2045(08)70285-7
177. Schneider TC, Abdulrahman RM, Corssmit EP, Morreau H, Smit JW, Kapiteijn E. Long-term analysis of the efficacy and tolerability of sorafenib in advanced radio-iodine refractory differentiated thyroid carcinoma: final results of a phase II trial. Eur J Endocrinol (2012) 167:643–50. doi: 10.1530/EJE-12-0405
178. Tang W, Chen Z, Zhang W, Cheng Y, Zhang B, Wu F, et al. The mechanisms of sorafenib resistance in hepatocellular carcinoma: theoretical basis and therapeutic aspects. Signal Transduct Target Ther (2020) 5:87. doi: 10.1038/s41392-020-0187-x
179. He Y, Luo Y, Huang L, Zhang D, Wang X, Ji J, et al. New frontiers against sorafenib resistance in renal cell carcinoma: From molecular mechanisms to predictive biomarkers. Pharmacol Res (2021) 170:105732. doi: 10.1016/j.phrs.2021.105732
180. Deuschle FC, Morath V, Schiefner A, Brandt C, Ballke S, Reder S, et al. Development of a high affinity Anticalin((R)) directed against human CD98hc for theranostic applications. Theranostics (2020) 10:2172–87. doi: 10.7150/thno.38968
181. Nozaki S, Nakatani Y, Mawatari A, Shibata N, Hume WE, Hayashinaka E, et al. (18)F-FIMP: a LAT1-specific PET probe for discrimination between tumor tissue and inflammation. Sci Rep (2019) 9:15718. doi: 10.1038/s41598-019-52270-x
182. Nozaki S, Nakatani Y, Mawatari A, Hume WE, Wada Y, Ishii A, et al. First-in-human assessment of the novel LAT1 targeting PET probe (18)F-FIMP. Biochem Biophys Res Commun (2022) 596:83–7. doi: 10.1016/j.bbrc.2022.01.099
183. Bröer A, Gauthier-Coles G, Rahimi F, van Geldermalsen M, Dorsch D, Wegener A, Holst J, Bröer S. Ablation of the ASCT2 (SLC1A5) gene encoding a neutral amino acid transporter reveals transporter plasticity and redundancy in cancer cells. J Biol Chem. (2019) 294(11):4012–4026. doi: 10.1074/jbc.RA118.006378
Keywords: CSC: cancer stem cell, CD98hc, therapy resistance, tumor progression, LAT1, xCT, ferroptosis
Citation: Xia P and Dubrovska A (2023) CD98 heavy chain as a prognostic biomarker and target for cancer treatment. Front. Oncol. 13:1251100. doi: 10.3389/fonc.2023.1251100
Received: 30 June 2023; Accepted: 29 August 2023;
Published: 26 September 2023.
Edited by:
Shihori Tanabe, National Institute of Health Sciences (NIHS), JapanReviewed by:
Mariafrancesca Scalise, University of Calabria, ItalyXiang-Min Yang, Fourth Military Medical University, China
Copyright © 2023 Xia and Dubrovska. This is an open-access article distributed under the terms of the Creative Commons Attribution License (CC BY). The use, distribution or reproduction in other forums is permitted, provided the original author(s) and the copyright owner(s) are credited and that the original publication in this journal is cited, in accordance with accepted academic practice. No use, distribution or reproduction is permitted which does not comply with these terms.
*Correspondence: Pu Xia, cHUueGlhQHVrZGQuZGU=; Anna Dubrovska, YS5kdWJyb3Zza2FAaHpkci5kZQ==