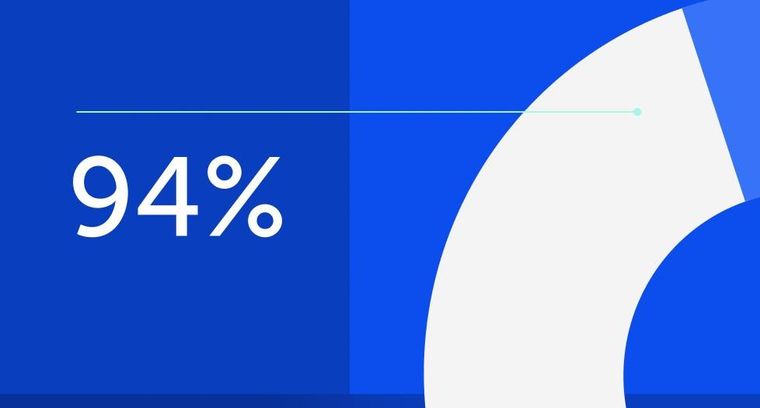
94% of researchers rate our articles as excellent or good
Learn more about the work of our research integrity team to safeguard the quality of each article we publish.
Find out more
MINI REVIEW article
Front. Oncol., 06 September 2023
Sec. Hematologic Malignancies
Volume 13 - 2023 | https://doi.org/10.3389/fonc.2023.1193978
This article is part of the Research TopicReviews in Hematologic Malignancies: 2023View all 15 articles
Hematologic malignancies comprise a diverse range of blood, bone marrow, and organ-related disorders that present significant challenges due to drug resistance, relapse, and treatment failure. Cancer-associated fibroblasts (CAFs) represent a critical component of the tumor microenvironment (TME) and have recently emerged as potential therapeutic targets. In this comprehensive review, we summarize the latest findings on the roles of CAFs in various hematologic malignancies, including acute leukemia, multiple myeloma, chronic lymphocytic leukemia, myeloproliferative neoplasms, and lymphoma. We also explore their involvement in tumor progression, drug resistance, and the various signaling pathways implicated in their activation and function. While the underlying mechanisms and the existence of multiple CAF subtypes pose challenges, targeting CAFs and their associated pathways offers a promising avenue for the development of innovative treatments to improve patient outcomes in hematologic malignancies.
Hematologic malignancies encompass a diverse array of blood, bone marrow, and organ-related disorders. Presently, leukemias and lymphomas can be treated using drugs or drug combinations, such as chemotherapy, targeted therapies, immunotherapy, immune checkpoint inhibitors, and chimeric antigen receptor-T (CAR-T) cells. These treatments have significantly enhanced patient prognoses. However, emerging drug resistance poses a major challenge, leading to relapse and treatment failure (1).
CAFs constitute the largest proportion of stromal cells in the tumor microenvironment (TME) (2). The origin of CAFs remains a subject of debate, with fibroblasts and mesenchymal stem cells (MSCs) from bone marrow (BM) and adipose tissue reservoirs believed to be their primary source (3). No specific markers exist for CAFs, although elevated alpha-smooth muscle actin (αSMA) expression is considered indicative of activated CAFs (4). Exhibiting enhanced proliferative and migratory capabilities, CAFs significantly influence tumor progression (5). Numerous studies have established the critical role of CAFs in solid tumors such as pancreatic, breast, colon, gastric, and liver malignancies (6–10). Further research has also explored targeting mechanisms like the TGFβ signaling pathway and the JAK/STAT signaling pathway (11, 12). The significance of CAFs in tumor progression and drug resistance is increasingly acknowledged, making them a focal point of recent research. Promisingly, several CAF-targeting therapies have entered clinical trials (5, 13).
The intricate interplay between CAFs and cancer cells is crucial for their interaction and is evident in hematologic tumors as well (Figure 1). For instance, bone marrow stromal cells can adopt CAF phenotypes, with the latter secreting various cytokines to stimulate tumor cell growth, infiltration, and endosteal niche reconstruction. Concurrently, TME remodeling provides tumor stem cells additional time for clonal reproduction, resulting in the continuous emergence of new genetic mutations that drive disease progression. This CAF-mediated remodeling also contributes to drug resistance, relapse, and tumor cell progression. In this article, we provide a comprehensive review of recent literature and summarize the roles of CAFs in hematologic tumors, as well as their potential value in disease treatment.
Figure 1 The crosstalk between CAF origins, CAFs and hematological malignancies. CAFs can emanate from a wide array of origins, encompassing mesenchymal stem cells, normal fibroblasts, myofibroblasts, endothelial cells, adipocyte pericytes, monocytes and macrophages, each exhibiting distinct phenotypes. The crosstalk between CAFs and hematological malignancies plays an important role in the development of blood cancer. Hematological malignancies are capable of facilitating the conversion of these diverse CAF origins into activated CAFs via numerous paracrine pathways. Subsequently, these activated CAFs can enhance the malignant phenotype of hematological malignancies through paracrine routes.
AL is characterized by the abnormal differentiation and proliferation of hematopoietic stem cells, which impedes normal hematopoiesis (14). Zhai et al. found that the presence of abundant reticulin fibers was associated with poor outcomes in acute myeloid leukemia (AML) (15). Their study showed that CAFs expressing elevated levels of FSP1, αSMA, or FAP protein were extensively distributed within the bone marrow (BM) of AML patients. They also proposed that CAFs could potentially shield leukemia cell lines (THP-1/K-562) from chemotherapy (15). By targeting growth differentiation factor-15 (GDF15) or suppressing GDF15 expression, the sensitivity of leukemic cells to chemotherapy increased, suggesting that GDF15 secretion by CAFs may play a crucial role in mediating the chemoprotective effects of CAFs (15).
Pan et al. carried out a series of investigations on CAFs in B-cell acute lymphoblastic leukemia (B-ALL) (16). They discovered that in newly diagnosed and relapsed B-ALL patients, bone marrow mononuclear cells had a higher percentage of CAF markers αSMA and FAP (16). Additionally, when BM-MSCs were co-cultured with leukemia cells, they adopted a CAF phenotype, which led to increased production of tumor-promoting growth factors and reduced daunorubicin-induced damage to B-ALL cells (16). Notably, while the chemoprotective effects of MSCs and CAFs on B-ALL were somewhat similar, CAFs proved to be more effective than MSCs in promoting the aggressiveness of B-ALL cells (16).
Subsequent research indicated that the overexpression of TGF-β plays a critical role in promoting the differentiation of BM-MSCs into CAFs, which may be dependent on the SDF-1/CXCR4 pathway (16, 17). The TGF-β receptor inhibitor LY2109761 and the CXCR4 antagonist AMD3100 both reduce CAF activation, offering a novel approach for chemotherapeutic regimens in AL (16–18). Li et al. isolated and cultured the first fibroblast tumor cell line, HXWMF-1, derived from CAFs in a 6-year-old B-ALL patient. They found compelling evidence that leukemic cells could potentially induce the malignant transformation of CAFs in a BALB/c nude mouse model (19).
MM is a disorder characterized by malignant plasma cell proliferation. The TME plays a substantial role in MM pathophysiology by secreting various cytokines that promote plasma cell survival, proliferation, and treatment resistance (20). Notably, the expression of CAF markers (FSP1, αSMA, FAP) in the bone marrow (BM) of patients with active MM was significantly higher (21). MM cells were found to induce CAF proliferation and enhance MM cell adhesion, proliferation, and apoptosis inhibition (21). The interaction between the two may be mediated through the SDF-1α/CXCR4 axis and integrins (21). Ciavarella et al. discovered that the activation levels of CAFs in MM patients at different clinical stages correlated with the expression of the fibrinolytic system (22). Compared to patients in the quiescent phase, CAFs in active MM patients exhibited higher transcriptional levels of u-PAR and u-PA. Selectively silencing u-PAR significantly suppressed CAF phenotype and function (22). Meanwhile, Kanehira et al. demonstrated that lysophosphatidic acid receptors 1 and 3 influenced the transition of MSCs to CAF differentiation, resulting in distinct outcomes (23).
Several targeted therapies for MM have emerged, but most have encountered drug resistance. For example, bortezomib, the first protease inhibitor approved by the FDA for MM treatment, has demonstrated limited efficacy in most patients due to the development of drug resistance (24). Several studies have investigated the vital role CAFs play in this issue. In vitro experiments indicated that CAFs from bortezomib-resistant patients inhibited bortezomib-induced apoptosis in MM cells. It is well-known that cellular autophagy contributes to drug resistance. When bortezomib-resistant CAFs are exposed to bortezomib, the autocrine TGF-pathway, which fosters autophagy, may become activated. Conversely, using TβR-I/II inhibitors to block Smad2/3 and autophagic pathways may help counteract MM resistance (25). CAR-T treatments targeting BCMA can detect and eradicate malignant plasma cells in MM patients, making them a promising therapeutic option. A study by Sakemura et al. revealed through ex vivo experiments that MM-CAFs inhibited antigen-specific proliferation of BCMA CAR-T cells via TGF-β secretion, consequently dampening their anti-myeloma activity (26). Simultaneously, targeting both MM cells and their CAFs with CAR-T cells reduced drug resistance development and slowed tumor progression, suggesting a new treatment approach (26).
CLL is a cancer characterized by the uncontrolled growth of mature lymphocytes in the blood, bone marrow, lymph nodes, and spleen (27). In the context of CLL, CAFs play a critical role in disease progression and interaction with the tumor microenvironment. CLL cells have the ability to activate the AKT pathway and stimulate the proliferation of MSCs via platelet-derived growth factor (PDGF) receptors (28). Furthermore, both bone marrow-derived MSCs and endothelial cells (ECs) can adopt a CAF phenotype when exposed to CLL-derived exosomes (29). These exosomes contain various molecular signals that can influence the behavior of recipient cells. Recent research has shown that CLL cells can trigger the transformation of BM-MSCs into CAFs by releasing exosomes containing miR-146a, which in turn inhibits USP16 (30). Additionally, a significant presence of αSMA(+) stromal cells was identified in infiltrating lymph nodes, further confirming the existence of numerous CAFs in CLL patients (29). CAFs play a crucial role in shaping the CLL microenvironment by influencing various immune cell functions. They release cytokines and chemokines that contribute to T cell and myeloid cell immunosuppression, and activate the AKT and NF-κB pathways, all of which promote tumor progression (31).
MPNs are malignant diseases characterized by excessive proliferation within the myeloid lineage, and αSMA, a CAF marker, is significantly elevated in MPN patients (32). Research suggests that αSMA expression levels influence the self-renewal and differentiation potential of MSCs, indicating a possible connection between αSMA expression and MPN development and prognosis (33). Primary myelofibrosis (PMF) is an MPN subtype characterized by progressive myelofibrosis. The development of myelofibrotic processes in PMF is currently believed to be associated with excessive stimulation of MSCs by growth factors (34).
In MPNs, lysyl oxidase (LOX), a stromal cross-linking protein, contributes to increased bone marrow stromal deposition. The use of a LOX inhibitor (BAPN) to decrease reticulin fibers supports LOX’s role in myelofibrosis development (35). LOXL2 expression is found to be elevated in MPN patients, especially those with PMF (36). Higher levels of LOXL2 may contribute to MPN progression by modulating the function of peripheral stromal cells that display a cancer-associated fibroblast phenotype (36). Furthermore, LOXL2 is considered a key factor in driving the differentiation of mesenchymal stem cells (MSCs) into CAFs (37). These discoveries provide novel perspectives for targeted MPN treatments. Simtuzumab, a monoclonal antibody that inhibits LOXL2, is currently being tested in phase II clinical trials (38).
In PMF patients, there is a significant expansion of clonal tumorigenic fibroblasts, a particular type of CAFs, which are functionally different from normal fibroblasts. This difference may be associated with JAK2 signaling, and these fibroblasts contribute to the progression of myelofibrosis (34). On the other hand, the fibroblast differentiation inhibitor SAP (PRM-151) substantially increases the survival rate of NSG mice transplanted with PMF bone marrow cells and reduces the development of myelofibrosis (34). Longhitano et al. found that exposure to IGFBP-6 leads to an increased expression of CAF markers (αSMA, FAP, TGF-β) in HS5 cells. Their research suggests that IGFBP-6 triggers the differentiation of MSCs into CAFs and indicates a connection between the IGFBP-6/SHH/TLR4 axis and alterations in the PMF microenvironment. This offers new perspectives on the pathogenesis of fibrosis in PMF patients (39).
Lymphoma is the most prevalent hematologic malignancy, divided mainly into non-Hodgkin’s lymphoma (90%) and Hodgkin’s lymphoma (10%) (40). CAF-like cells and their precursors are present in secondary lymphoid organs (SLOs) before lymphoma onset, playing a crucial role in the progression of malignancies. For example, fibroblastic reticular cells (FRCs) form the structural foundation of SLOs and are essential for organ development, T and B cell compartmentalization, and adaptive immune response involvement. This provides a supportive microenvironment for the proliferation of malignant B cells (41). Numerous studies indicate that CAFs can aid lymphocyte survival by enhancing glycolysis (42, 43). Metabolic analyses have shown that elevated concentrations of CAF-secreted pyruvate decrease intracellular ROS production in primary lymphoma cells, augment tumor cell dependence on the citric acid cycle, and boost tumor cell survival (44). Furthermore, CAFs modulate the expression of the pyrimidine transporter protein ENT2 in tumor cells by secreting exosomes containing miR-4717-5p, resulting in chemoresistance (43).
Diffuse large B-cell lymphoma (DLBCL), the most prevalent lymphoma type, triggers the activation process of CAFs. Activated CAFs display a compensatory suppressive response by increasing PD-L1 expression and reducing the lytic-killing activity of CD8 T cells against tumor cells (45, 46). These findings offer a fresh perspective on the disease’s initiation. Two CAF subtypes have been identified in adult T-cell leukemia/lymphoma (ATLL): CAFs/EGRhigh and CAFs/EGRlow. CAFs in ATLL were found to significantly contribute to CD4 T-cell proliferation
(47). Additionally, CAF/EGRhigh influences CD8 and NKT cell expansion through EGFR (47). These findings suggest potential avenues for targeted therapy.
CAFs have emerged as critical contributors to hematologic malignancies, influencing tumor progression and drug resistance. While the underlying mechanisms of CAF activation and function in hematologic malignancies are not yet fully understood, recent research has highlighted several potential therapeutic targets and pathways (48). Targeting CAFs and their associated pathways could provide an innovative approach to treating hematologic malignancies and enhancing patient outcomes. As research into the interplay between CAFs and hematologic malignancies continues, there is a promising prospect for developing novel treatments that target CAFs and improve clinical outcomes for patients.
Table 1 provides a summary of the latest research advancements in CAF-related targets and pathways within the context of hematologic malignancies.
In recent years, the understanding of cancer biology has expanded, leading to the identification of various cellular and molecular players involved in tumor progression. One such player is CAFs, which have been implicated in the progression of solid tumors. However, their role in blood cancers remains underexplored. In this discussion, we will delve into the potential involvement of CAFs in blood cancers and evaluate their suitability as a promising therapeutic target.
To begin, it is essential to understand the role of CAFs in the tumor microenvironment. CAFs are key stromal cells that modulate the extracellular matrix, support angiogenesis, and produce a myriad of growth factors and cytokines. These actions contribute to the tumor-promoting milieu, ultimately enhancing cancer cell survival, proliferation, and metastasis. Given their critical role in solid tumors, it is plausible to assume that CAFs may have similar functions in blood cancers.
Blood cancers, such as leukemia, lymphoma, and myeloma, arise from the malignant transformation of cells in the blood, bone marrow, or lymphatic system. Although these cancers lack the solid tumor architecture, they still interact with the surrounding microenvironment, which may include CAFs. For instance, interactions between leukemia cells and bone marrow stromal cells, including fibroblasts, have been reported to support leukemia cell survival and contribute to therapeutic resistance. This suggests that CAFs could be critical players in the pathogenesis of blood cancers.
Targeting CAFs as a therapeutic strategy in blood cancers may have several advantages. First, as stromal cells, CAFs are genetically more stable than cancer cells, making them less likely to develop resistance to targeted therapies. Second, by disrupting the crosstalk between CAFs and cancer cells, the tumor-promoting microenvironment could be altered, potentially enhancing the efficacy of existing treatments. Finally, targeting CAFs may have a synergistic effect when combined with other therapies, leading to improved clinical outcomes.
However, it is important to consider the challenges and limitations associated with targeting CAFs in blood cancers. One of the primary challenges lies in the heterogeneity of CAFs, as they can originate from various cell types and exhibit diverse phenotypes and functions. This complexity may hinder the development of specific CAF-targeted therapies and could necessitate the identification of common signaling pathways or markers that can be targeted across different CAF subpopulations (4, 5). Cancer boasts a multifaceted biological composition and structure, encompassing cancerous cells, stromal cells, and the extracellular matrix (49). Historically, the majority of treatments have primarily aimed at cancer cells themselves (49). However, recent research has shed light on the significant influence the TME has on the behavior of cancer cells and their response to therapies (49, 50). Notably, CAFs, which constitute the most prevalent type of stromal cells within the TME, play a crucial yet understated role in the inception, progression, and metastasis of cancer (49). Consequently, focusing research on TME and CAF markers has emerged as a pivotal component of innovative strategies for the design and discovery of next-generation cancer drugs (49). However, unlike the case with solid tumors, the study of the tumor microenvironment and CAF markers in fibroblasts associated with hematological malignancies is still in its early stages (49, 51, 52). This remains an important area for future exploration and research.
Another challenge is the potential for off-target effects, given that CAFs share similarities with normal fibroblasts. Developing therapies that selectively target CAFs without affecting healthy fibroblasts is essential to minimize adverse side effects. Furthermore, the dynamic nature of the tumor microenvironment and the reciprocal interactions between CAFs and cancer cells may result in compensatory mechanisms that limit the efficacy of CAF-targeted therapies. Therefore, understanding the molecular mechanisms underlying these interactions is crucial for the development of effective treatment strategies (53).
In light of these challenges, future research should focus on elucidating the molecular and cellular mechanisms that govern CAFs’ involvement in blood cancers. High-throughput screening technologies, such as single-cell RNA sequencing, could provide valuable insights into the heterogeneity of CAF populations and identify potential therapeutic targets (54–56). Additionally, the development of advanced in vitro and in vivo models that more closely mimic the tumor microenvironment will be essential for evaluating the safety and efficacy of novel CAF-targeted therapies. Moreover, the potential synergistic effects of combining CAF-targeted therapies with other treatment modalities, such as chemotherapy, immunotherapy, and targeted therapies, should be investigated. This combinatorial approach may help overcome potential resistance mechanisms and improve clinical outcomes for patients with blood cancers.
In summary, the targeting of CAFs in blood cancers presents a promising therapeutic strategy, but it is not without challenges. Future research should address the limitations and obstacles associated with CAF-targeted therapies and explore the potential benefits of combining these treatments with existing therapies. By deepening our understanding of CAFs’ role in blood cancers and overcoming the hurdles associated with their targeting, we may be able to unlock new, more effective treatment options for patients suffering from these malignancies.
Investigating and writing the manuscript, ZYD and RUS. Editing and revising, WKH and ROS. Literature reading and review, LT. Project administration, YW and XYZ. All authors contributed to the article and approved the submitted version.
The project was funded by the Science and Technology Innovation Project for Nanjing Overseas Chinese (YW, No. B42), the Incubation Program of National Natural Science Foundation of Jiangsu Provincial People’s Hospital (YW, No. 39) and Jiangsu Province “Double-Creation Doctorate” Talent Program (YW).
The authors declare that the research was conducted in the absence of any commercial or financial relationships that could be construed as a potential conflict of interest.
All claims expressed in this article are solely those of the authors and do not necessarily represent those of their affiliated organizations, or those of the publisher, the editors and the reviewers. Any product that may be evaluated in this article, or claim that may be made by its manufacturer, is not guaranteed or endorsed by the publisher.
1. Auberger P, Tamburini-Bonnefoy J, Puissant A, MDPI. Drug resistance in hematological Malignancies. Switzerland: MDPI (2020).
2. Chen X, Song E. Turning foes to friends: targeting cancer-associated fibroblasts. Nat Rev Drug Discovery (2019) 18(2):99–115. doi: 10.1038/s41573-018-0004-1
3. De Wever O, Van Bockstal M, Mareel M, Hendrix A, Bracke M. Carcinoma-associated fibroblasts provide operational flexibility in metastasis. In: Seminars in cancer biology. Elsevier, Amsterdam (2014).
4. Biffi G, Tuveson DA. Diversity and biology of cancer-associated fibroblasts. Physiol Rev (2021) 101(1):147–76. doi: 10.1152/physrev.00048.2019
5. Saw PE, Chen J, Song E. Targeting CAFs to overcome anticancer therapeutic resistance. Trends Cancer (2022) 8:527–55. doi: 10.1016/j.trecan.2022.03.001
6. Richards KE, Zeleniak AE, Fishel ML, Wu J, Littlepage LE, Hill R. Cancer-associated fibroblast exosomes regulate survival and proliferation of pancreatic cancer cells. Oncogene (2017) 36(13):1770–8. doi: 10.1038/onc.2016.353
7. Wen S, Hou Y, Fu L, Xi L, Yang D, Zhao M, et al. Cancer-associated fibroblast (CAF)-derived IL32 promotes breast cancer cell invasion and metastasis via integrin β3–p38 MAPK signalling. Cancer Lett (2019) 442:320–32. doi: 10.1016/j.canlet.2018.10.015
8. Herrera M, Berral-González A, López-Cade I, Galindo-Pumariño C, Bueno-Fortes S, Martín-Merino M, et al. Cancer-associated fibroblast-derived gene signatures determine prognosis in colon cancer patients. Mol Cancer (2021) 20(1):1–6. doi: 10.1186/s12943-021-01367-x
9. Li X, Sun Z, Peng G, Xiao Y, Guo J, Wu B, et al. Single-cell RNA sequencing reveals a pro-invasive cancer-associated fibroblast subgroup associated with poor clinical outcomes in patients with gastric cancer. Theranostics (2022) 12(2):620. doi: 10.7150/thno.60540
10. Song M, He J, Pan QZ, Yang J, Zhao J, Zhang YJ, et al. Cancer-associated fibroblast-mediated cellular crosstalk supports hepatocellular carcinoma progression. Hepatology (2021) 73(5):1717–35. doi: 10.1002/hep.31792
11. Sahai E, Astsaturov I, Cukierman E, DeNardo DG, Egeblad M, Evans RM, et al. A framework for advancing our understanding of cancer-associated fibroblasts. Nat Rev Cancer (2020) 20(3):174–86. doi: 10.1038/s41568-019-0238-1
12. Vicent S, SaylesL C, Vaka D, Khatri P, Gevaert O, Chen R, et al. Cross-species functional analysis of cancer-associated fibroblasts identifies a critical role for Clcf1 and Il-6 in non–small cell lung cancer in vivo. Cancer Res (2012) 72(22):5744–56. doi: 10.1158/0008-5472.CAN-12-1097
13. Van Cutsem E, Tempero MA, Sigal D, Oh D-Y, Fazio N, Macarulla T, et al. Randomized phase III trial of pegvorhyaluronidase alfa with nab-paclitaxel plus gemcitabine for patients with hyaluronan-high metastatic pancreatic adenocarcinoma. J Clin Oncol (2020) 38(27):3185. doi: 10.1200/JCO.20.00590
14. Devine SM, Larson RA. Acute leukemia in adults: recent developments in diagnosis and treatment. CA: Cancer J Clin (1994) 44(6):326–52. doi: 10.3322/canjclin.44.6.326
15. Zhai Y, Zhang J, Wang H, Lu W, Liu S, Yu Y, et al. Growth differentiation factor 15 contributes to cancer-associated fibroblasts-mediated chemo-protection of AML cells. J Exp Clin Cancer Res (2016) 35(1):1–12. doi: 10.1186/s13046-016-0405-0
16. Pan C, Liu P, Ma D, Zhang S, Ni M, Fang Q, et al. Bone marrow mesenchymal stem cells in microenvironment transform into cancer-associated fibroblasts to promote the progression of B-cell acute lymphoblastic leukemia. Biomedicine Pharmacotherapy (2020) 130:110610. doi: 10.1016/j.biopha.2020.110610
17. Xu Y, Tabe Y, Jin L, Watt J, McQueen T, Ohsaka A, et al. TGF-beta receptor kinase inhibitor LY2109761 reverses the anti-apoptotic effects of TGF-beta1 in myelo-monocytic leukaemic cells co-cultured with stromal cells. Br J Haematol (2008) 142(2):192–201. doi: 10.1111/j.1365-2141.2008.07130.x
18. Cooper TM, Sison EAR, Baker SD, Li L, Ahmed A, Trippett T, et al. A phase 1 study of the CXCR4 antagonist plerixafor in combination with high-dose cytarabine and etoposide in children with relapsed or refractory acute leukemias or myelodysplastic syndrome: A Pediatric Oncology Experimental Therapeutics Investigators’ Consortium study (POE 10-03). Pediatr Blood Cancer (2017) 64(8):e26414. doi: 10.1002/pbc.26414
19. Li Y, Gu L. Establishment and characterization of HXWMF-1: the first mouse fibroblastic tumor cell line derived from leukemia-associated fibroblasts. Cancer Cell Int (2021) 21(1):1–10. doi: 10.1186/s12935-021-01870-7
20. Roccaro AM, Sacco A, Maiso P, Azab AK, Tai Y-T, Reagan M, et al. BM mesenchymal stromal cell–derived exosomes facilitate multiple myeloma progression. J Clin Invest (2013) 123(4):1542–55. doi: 10.1172/JCI66517
21. Frassanito MA, Rao L, Moschetta M, Ria R, Di Marzo L, De Luisi A, et al. Bone marrow fibroblasts parallel multiple myeloma progression in patients and mice: in vitro and in vivo studies. Leukemia (2014) 28(4):904–16. doi: 10.1038/leu.2013.254
22. Ciavarella S, Laurenzana A, De Summa S, Pilato B, Chillà A, Lacalamita R, et al. u-PAR expression in cancer associated fibroblast: new acquisitions in multiple myeloma progression. BMC Cancer (2017) 17(1):1–11. doi: 10.1186/s12885-017-3183-y
23. Kanehira M, Fujiwara T, Nakajima S, Okitsu Y, Onishi Y, Fukuhara N, et al. A lysophosphatidic acid receptors 1 and 3 axis governs cellular senescence of mesenchymal stromal cells and promotes growth and vascularization of multiple myeloma. Stem Cells (2017) 35(3):739–53. doi: 10.1002/stem.2499
24. Ruschak AM, Slassi M, Kay LE, Schimmer AD. Novel proteasome inhibitors to overcome bortezomib resistance. J Natl Cancer Institute (2011) 103(13):1007–17. doi: 10.1093/jnci/djr160
25. Frassanito M, De Veirman K, Desantis V, Di Marzo L, Vergara D, Ruggieri S, et al. Halting pro-survival autophagy by TGFβ inhibition in bone marrow fibroblasts overcomes bortezomib resistance in multiple myeloma patients. Leukemia (2016) 30(3):640–8. doi: 10.1038/leu.2015.289
26. Sakemura R, Hefazi M, Siegler EL, Cox MJ, Larson DP, Hansen MJ, et al. Targeting cancer-associated fibroblasts in the bone marrow prevents resistance to CART-cell therapy in multiple myeloma. Blood J Am Soc Hematol (2022) 139(26):3708–21. doi: 10.1182/blood.2021012811
27. Hallek M, Shanafelt TD, Eichhorst B. Chronic lymphocytic leukaemia. Lancet (2018) 391(10129):1524–37. doi: 10.1016/S0140-6736(18)30422-7
28. Raffaghello L, Vacca A, Pistoia V, Ribatti D. Cancer associated fibroblasts in hematological Malignancies. Oncotarget (2015) 6(5):2589. doi: 10.18632/oncotarget.2661
29. Paggetti J, Haderk F, Seiffert M, Janji B, Distler U, Ammerlaan W, et al. Exosomes released by chronic lymphocytic leukemia cells induce the transition of stromal cells into cancer-associated fibroblasts. Blood J Am Soc Hematol (2015) 126(9):1106–17. doi: 10.1182/blood-2014-12-618025
30. Yang Y, Li J, Geng Y. Exosomes derived from chronic lymphocytic leukaemia cells transfer miR-146a to induce the transition of mesenchymal stromal cells into cancer-associated fibroblasts. J Biochem (2020) 168(5):491–8. doi: 10.1093/jb/mvaa064
31. van Attekum MH, Eldering E, Kater AP. Chronic lymphocytic leukemia cells are active participants in microenvironmental cross-talk. haematologica (2017) 102(9):1469. doi: 10.3324/haematol.2016.142679
32. Schmitt-Graeff AH, Nitschke R, Zeiser R. The hematopoietic niche in myeloproliferative neoplasms. Mediators Inflammation (2015) 2015. doi: 10.1155/2015/347270
33. Talele NP, Fradette J, Davies JE, Kapus A, Hinz B. Expression of α-smooth muscle actin determines the fate of mesenchymal stromal cells. Stem Cell Rep (2015) 4(6):1016–30. doi: 10.1016/j.stemcr.2015.05.004
34. Verstovsek S, Manshouri T, Pilling D, Bueso-Ramos CE, Newberry KJ, Prijic S, et al. Role of neoplastic monocyte-derived fibrocytes in primary myelofibrosis. J Exp Med (2016) 213(9):1723–40. doi: 10.1084/jem.20160283
35. Eliades A, Papadantonakis N, Bhupatiraju A, Burridge KA, Johnston-Cox HA, Migliaccio AR, et al. Control of megakaryocyte expansion and bone marrow fibrosis by lysyl oxidase. J Biol Chem (2011) 286(31):27630–8. doi: 10.1074/jbc.M111.243113
36. Xu N, Li Y, Zhou X, Li L, Lu Q, Liu Q, et al. LOXL2 highly-expressed induce the transition of stromal cells into cancer-associated fibroblasts which maybe involve in myeloproliferative neoplasms progession. Washington, DC: American Society of Hematology. (2015).
37. Liu X, Xu N, Pan C, Zhou X, Liu Q, Feng R, et al. Emerging potential roles of lysyl-oxidase-like 2 stimulates cancer associated fibroblasts promoting myelofibrosis of myeloproliferative neoplasms. Washington, DC: American Society of Hematology. (2016).
38. Verstovsek S, Savona MR, Mesa RA, Dong H, Maltzman JD, Sharma S, et al. A phase 2 study of simtuzumab in patients with primary, post-polycythaemia vera or post-essential thrombocythaemia myelofibrosis. Br J haematology (2017) 176(6):939–49. doi: 10.1111/bjh.14501
39. Longhitano L, Tibullo D, Vicario N, Giallongo C, La Spina E, Romano A, et al. IGFBP-6/sonic hedgehog/TLR4 signalling axis drives bone marrow fibrotic transformation in primary myelofibrosis. Aging (Albany NY) (2021) 13(23):25055. doi: 10.18632/aging.203779
40. Mugnaini EN, Ghosh N. Lymphoma. Primary Care: Clinics Office Pract (2016) 43(4):661–75. doi: 10.1016/j.pop.2016.07.012
41. Höpken UE, Rehm A. Targeting the tumor microenvironment of leukemia and lymphoma. Trends Cancer (2019) 5(6):351–64. doi: 10.1016/j.trecan.2019.05.001
42. Aoki T, Shimada K, Sakamoto A, Sugimoto K, Morishita T, Kojima Y, et al. Emetine elicits apoptosis of intractable B-cell lymphoma cells with MYC rearrangement through inhibition of glycolytic metabolism. Blood (2016) 128(22):3019. doi: 10.1182/blood.V128.22.3019.3019
43. Kunou S, Shimada K, Takai M, Sakamoto A, Aoki T, Hikita T, et al. Exosomes secreted from cancer-associated fibroblasts elicit anti-pyrimidine drug resistance through modulation of its transporter in Malignant lymphoma. Oncogene (2021) 40(23):3989–4003. doi: 10.1038/s41388-021-01829-y
44. Sakamoto A, Kunou S, Shimada K, Tsunoda M, Aoki T, Iriyama C, et al. Pyruvate secreted from patient-derived cancer-associated fibroblasts supports survival of primary lymphoma cells. Cancer Sci (2019) 110(1):269–78. doi: 10.1111/cas.13873
45. Apollonio B, Jarvis P, Phillips B, Kuhnl A, Salisbury J, Zacharioudakis G, et al. Diffuse large B-cell lymphoma remodels the fibroblastic reticular network that acquires aberrant immunosuppressive capabilities; implications for the regulation of anti-tumor immunity in the immuno-oncology era. Blood (2018) 132:675. doi: 10.1182/blood-2018-99-116409
46. Apollonio B, Jarvis P, Phillips B, Kuhnl A, Salisbury J, Zacharioudakis G, et al. Diffuse large B-cell lymphoma (DLBCL) tumor cells reprogram lymphatic fibroblasts into cancer-associated fibroblasts (CAFs) that contribute to tumor microenvironment (TME)-driven immune privilege. Blood (2015) 126(23):1474. doi: 10.1182/blood.v126.23.1474.1474
47. Joo E-H, Bae JH, Park J, Bang YJ, Han J, Gulati N, et al. Deconvolution of adult T-cell leukemia/lymphoma with single-cell RNA-seq using frozen archived skin tissue reveals new subset of cancer-associated fibroblast. Front Immunol (2022) 13. doi: 10.3389/fimmu.2022.856363
48. Glabman RA, Choyke PL, Sato N. Cancer-associated fibroblasts: tumorigenicity and targeting for cancer therapy. Cancers (Basel) (2022) 14(16). doi: 10.3390/cancers14163906
49. Dzobo K, Dandara C. Broadening drug design and targets to tumor microenvironment? Cancer-associated fibroblast marker expression in cancers and relevance for survival outcomes. OMICS (2020) 24(6):340–51. doi: 10.1089/omi.2020.0042
50. Dzobo K. Taking a full snapshot of cancer biology: deciphering the tumor microenvironment for effective cancer therapy in the oncology clinic. OMICS (2020) 24(4):175–9. doi: 10.1089/omi.2020.0019
51. Rimal R, Desai P, Daware R, Hosseinnejad A, Prakash J, Lammers T, et al. Cancer-associated fibroblasts: Origin, function, imaging, and therapeutic targeting. Adv Drug Delivery Rev (2022) 189:114504. doi: 10.1016/j.addr.2022.114504
52. Dzobo K, Senthebane DA, Dandara C. The tumor microenvironment in tumorigenesis and therapy resistance revisited. Cancers (Basel) (2023) 15(2). doi: 10.3390/cancers15020376
53. Gu L, Liao P, Liu H. Cancer-associated fibroblasts in acute leukemia. Front Oncol (2022) 12:1022979. doi: 10.3389/fonc.2022.1022979
54. Lavie D, Ben-Shmuel A, Erez N, Scherz-Shouval R. Cancer-associated fibroblasts in the single-cell era. Nat Cancer (2022) 3(7):793–807. doi: 10.1038/s43018-022-00411-z
55. Luo H, Xia X, Huang L-B, An H, Cao M, Kim GD, et al. Pan-cancer single-cell analysis reveals the heterogeneity and plasticity of cancer-associated fibroblasts in the tumor microenvironment. Nat Commun (2022) 13(1):6619. doi: 10.1038/s41467-022-34395-2
Keywords: cancer associated fibroblast (CAF), hematologic malignancies, crosstalk, chemoresistance, therapeutic target
Citation: Ding Z, Shi R, Hu W, Tian L, Sun R, Wu Y and Zhang X (2023) Cancer-associated fibroblasts in hematologic malignancies: elucidating roles and spotlighting therapeutic targets. Front. Oncol. 13:1193978. doi: 10.3389/fonc.2023.1193978
Received: 27 March 2023; Accepted: 14 August 2023;
Published: 06 September 2023.
Edited by:
Giuseppe Maurizio Campo, University of Messina, ItalyReviewed by:
Kevin Dzobo, University of Cape Town, South AfricaCopyright © 2023 Ding, Shi, Hu, Tian, Sun, Wu and Zhang. This is an open-access article distributed under the terms of the Creative Commons Attribution License (CC BY). The use, distribution or reproduction in other forums is permitted, provided the original author(s) and the copyright owner(s) are credited and that the original publication in this journal is cited, in accordance with accepted academic practice. No use, distribution or reproduction is permitted which does not comply with these terms.
*Correspondence: Rong Sun, ZG9jdG9yc3Vucm9uZzgxOEAxNjMuY29t; Yang Wu, c3VyZ2VvbnlhbmdAb3V0bG9vay5jb20=; Xiaoyan Zhang, ODc3MzA3Mzc1QHFxLmNvbQ==
†These authors have contributed equally to this work
Disclaimer: All claims expressed in this article are solely those of the authors and do not necessarily represent those of their affiliated organizations, or those of the publisher, the editors and the reviewers. Any product that may be evaluated in this article or claim that may be made by its manufacturer is not guaranteed or endorsed by the publisher.
Research integrity at Frontiers
Learn more about the work of our research integrity team to safeguard the quality of each article we publish.