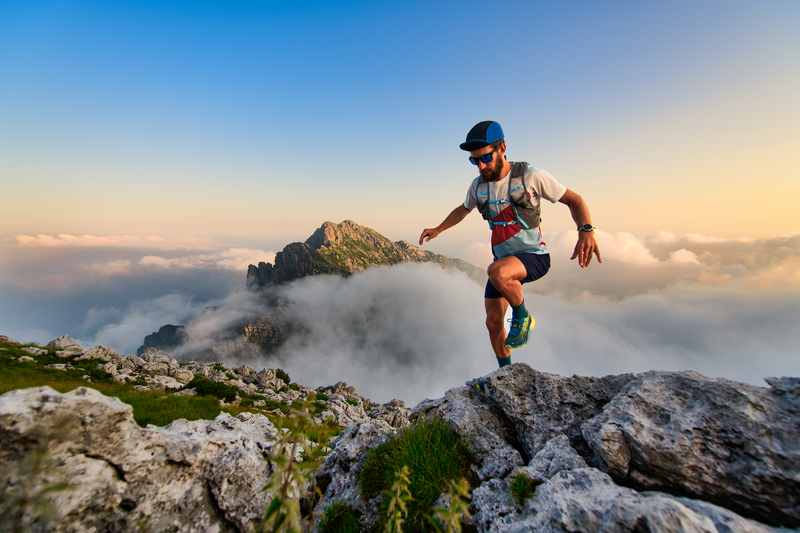
95% of researchers rate our articles as excellent or good
Learn more about the work of our research integrity team to safeguard the quality of each article we publish.
Find out more
REVIEW article
Front. Oncol. , 05 July 2023
Sec. Gastrointestinal Cancers: Hepato Pancreatic Biliary Cancers
Volume 13 - 2023 | https://doi.org/10.3389/fonc.2023.1186659
This article is part of the Research Topic The Role of Tumor Microenvironment in the Development, Treatment and Prognosis of Hepatocellular Carcinoma View all 30 articles
Copper is an essential trace element that acts as a cofactor in various enzyme active sites in the human body. It participates in numerous life activities, including lipid metabolism, energy metabolism, and neurotransmitter synthesis. The proposal of “Cuproptosis” has made copper metabolism-related pathways a research hotspot in the field of tumor therapy, which has attracted great attention. This review discusses the biological processes of copper uptake, transport, and storage in human cells. It highlights the mechanisms by which copper metabolism affects hepatocellular carcinogenesis and metastasis, including autophagy, apoptosis, vascular invasion, cuproptosis, and ferroptosis. Additionally, it summarizes the current clinical applications of copper metabolism-related drugs in antitumor therapy.
Primary liver cancer is a prevalent malignant tumor that ranks as the third most common cause of cancer-related deaths worldwide, and is particularly frequent in East Asia and Southeast Asia (1). Hepatocellular carcinoma (HCC) is the most common type of primary liver cancer, accounting for 75%-85% of cases. Finding new breakthroughs in treatment has been the key to research on HCC, as HCC has a 5-year survival of less than 15% (2). Copper is a widespread metallic element in nature. With the interdisciplinary development, the pathways of copper metabolism in human body are gradually discovered. The redox properties of copper are both beneficial and potentially toxic to cells, which are increasingly found to be involved in cell proliferation and death pathways in a rising number of studies (3). The metallic signal of copper is strongly associated with tumor progression, especially HCC, where the liver is the main organ for copper storage (4). Key enzymes and genes related to copper metabolism have become important directions in the treatment of HCC, while the exploration of copper in the diagnosis, treatment, prognosis and survival analysis of HCC has become a current research topic.
This review aims to provide an overview of the normal physiological metabolic processes of copper, a summary of the mechanisms of copper metabolism involved in the progression of HCC, and a discussion of recent research advances in the treatment of HCC through the regulation of copper homeostasis.
Copper, which is an essential cofactor, is widely found in living organisms in nature. Diets high in copper, such as offal, shellfish, seeds, legumes, vegetables and whole grain cereals, are the main ways in which the body obtains copper, and industrial products may also be an important source of copper in the human body (5). The intestinal epithelium is responsible for the absorption of dietary copper, which is removed through the liver if the level is high in the body and secreted by the bile into the gastrointestinal tract and excreted in the feces. To keep free copper at a low level, copper ions in the body mainly attach to certain proteins or other molecules to ensure normal biochemical processes (6). Since its unstable redox potential, copper homeostasis is essential for cell survival.
Copper is absorbed in the small intestine, with the duodenum serving as its primary absorption site and having an absorption efficiency of up to 60% (7). Copper in the diet is usually present in the form of Cu2+, but only Cu+ can be absorbed and reused. This process is mainly involved by the prostate metal reductase six transmembrane epithelial antigen of the prostate (STEAP) and duodenal cytochrome b (DCYTB) (8, 9). Cu+ is taken up into the cell mediated by copper transporters 1 (CTR1) on the apical side of the enterocyte. Cells can also take minor amounts of Cu2+ up, but the underlying mechanism is not clear. The low affinity copper transporter receptor 2 (CTR2), divalent metal transporter1 (DMT1), and sodium-dependent amino-acid transporters may explain this mechanism as alternative copper uptake pathways (10).
There are two membrane-bound copper-transporting adenosine triphosphatases (ATPases) exist in human cells, ATP7A and ATP7B, both of which play an important role in the digestive tract. ATP7A may promote the efflux of Cu+ from the intestinal epithelium and transport to the circulation (11, 12). ATP7B is primarily responsible for the storage of Cu+ in intracellular vesicles to maintain the copper balance required for normal homeostasis of the intestinal epithelial (13).
Ceruloplasmin (CP) is the main carrier involved in copper transport, and each CP can bind six Cu+ (14). In addition, albumin and histidine are also involved in the transport of copper. Copper is secreted by intestinal epithelial cells into the portal vein circulation and bound with these copper-carrying protein. When Cu+ is transported to the liver, they would be mediated by CTR1 into hepatocytes. In the cytoplasm, Cu+ would be isolated by glutathione (GSH) and stored in metallothioneins (MTs) (11). Both have a high affinity for copper and are rich in thiol groups, which helps to maintain a low quantity of free copper. Usually, liver is one of the main organs storing Cu+, with MT1 and MT2 being the main storage sites for Cu+. But a new study shows that MT3, which is highly expressed in the central nervous system, is one of the major players in copper homeostasis (15).
In addition, a portion of the Cu+ will bind to the copper chaperones and transported to specific organelles to participate in related physiological process. In mitochondria, Cu+ is involved in the respiratory chain and redox pathways by binding to cytochrome c oxidase (CCO). For example, the copper chaperones (COX17, COX19 and COX23) are responsible for transporting Cu+ to the mitochondria, and then which is delivered to CCO by the mitochondrial inner membrane proteins Sco1, Sco2 and COX11.
Antioxidant protein 1 (ATOX1) would transport Cu+ to the Trans-Golgi Network (TGN) and promote the synthesis of copper enzymes such as lysyl oxidase, tyrosinase and copper cyanobactin (16). Except for the liver, ATP7A are expressed in most tissues (16). However, ATP7B are only present in hepatocytes, which pump Cu+ from the cytoplasm into the TGN. When excess copper enters the hepatocyte, endolysosomal vesicles containing ATP7B would transport them to bile duct and drain the excess Cu+ into the bile (17). Therefore, mutations in ATP7A and ATP7B predispose to disorders of copper metabolism, allowing Cu+ to accumulate in cells, which leads to the onset of Menkes’ disease and Wilson disease (18).
In addition, Copper Chaperone (CCS) would also transport Cu+ to superoxide dismutase (SOD) to alleviate oxidative stress and maintain copper homeostasis (19, 20). In the nucleus, Cu+ can be combined with transcription factors and drive gene expression (21). The process of copper absorption and transport in the human body is shown in Figure 1.
Figure 1 The process of copper absorption and transport in the human body. Cu2+ is restored to Cu+ by STEAP and DCYTB in the small intestine. Cu+ is taken up into the cell mediated by CTR1. ATP7B is primarily responsible for the storage of Cu+, and ATP7A may promote the efflux of Cu+ from the intestinal epithelium and transport to the circulation. When Cu+ is transported to the liver, they would be mediated by CTR1 into hepatocytes. Cu+ would be isolated by GSH and stored in MTs. In mitochondria, Cu+ is involved in the respiratory chain and redox pathways by binding to CCO. ATOX1 would transport Cu+ to the TGN and promote the synthesis of copper enzymes. When excess copper enters the hepatocyte, endolysosomal vesicles containing ATP7B would excrete Cu+ into the bile. In addition, CCS would also transport Cu+ to SOD to alleviate oxidative stress. In the nucleus, Cu+ can be combined with transcription factors and drive gene expression.
The role of copper in biological processes has been a hot topic of research for this century. Numerous factors regulate and maintain the body’s intake, transport, and secretion of copper in a dynamic equilibrium. Aberrant copper metabolism or copper-induced cell death can lead to a variety of diseases when copper homeostasis is disrupted in the body. Low level of Cu can impair the function of metal-binding enzymes, while too high level can lead to abnormal cellular functions (4). A number of studies have shown that tumor tissues require higher level of copper to meet the high metabolic demands compared to healthy tissues (22). Elevated copper levels have now been found to be associated with a multitude of malignancies according to research, including breast cancer (23), colorectal cancer (24), lung cancer (25), and gallbladder cancer (26). Copper ions were absorbed through the intestine, arriving in the liver from the portal vein with serum proteins as carriers, and enter the hepatocytes via CTR1, where large amount of copper was stored in the hepatocytes in combination with MT1 and MT2 (27). The liver is the center of Cu storage and transport as a core player in the regulation of systemic Cu homeostasis. The liver cells will be the first to be impacted when there is an abnormality in the copper metabolism. Therefore, Cu is closely associated with the development of liver disease. Wilson disease, as we know it, has a defect in copper processing resulting in high copper level and toxic effects on liver cells (28). Mitochondrial damage induced by copper overload results in other liver lesions in more than half of Wilson disease patients (29). Cirrhosis, the end-stage of many liver diseases, is an essential risk factor for HCC, which also exhibits abnormal accumulation of copper and abnormal distribution of other trace metal elements (30). Both European and Asian cohort studies have shown that elevated copper levels in humans are associated with a high risk of morbidity and a poor prognosis of HCC (31, 32). Cuproptosis-related genes have potential for constructing a prognostic model for HCC (33).
Autophagy is a highly regulated cellular mechanism that aimed at bioenergetic recovery through intracellular destruction and breakdown of dysfunctional cytoplasmic components and recycling of energy (34). Autophagy is a double-edged sword. On the one hand, autophagy can suppress tumors by reducing reactive oxygen species (ROS) and removing damaged organelles and toxic substances from cells. On the other hand, autophagy inhibits tumor cell apoptosis and provides metabolic support to accelerate the growth of HCC cells. High level of Cu+ generates large amounts of ROS during oxidation, which is important for the onset of autophagy (35). Unc-51-like kinase 1 (ULK1/2) is an important initiator of autophagy and is involved in the formation of autophagic vesicles as well as the regulation of the autophagic process. Tsang et al. (36) found that ULK1/2 had a strong affinity for copper. Copper-dependent ULK1/2 activation stimulated autophagic flux, and low intracellular copper (CTR1 depletion, ATP7A overexpression, or copper chelator use) resulted in a decrease of autophagy. In addition, excess copper can also increase autophagic flux by activating the expression of autophagy-related gene 5 (ATG5) (37), Beclin-1 (BECN1) (37)and AMPK-mTOR (38) pathways, as well as accelerate autophagic vesicle formation by mediating TFEB (39) transcription factors. The autophagic targets and pathways associated with copper metabolism in HCC are shown in Figure 2A. It is notable that the accumulation of copper and the activation of autophagy in Wilson disease as well as in HCC occur simultaneously (40–42).
Figure 2 Targets and pathways of autophagy, apoptosis and vascular invasion associated with copper metabolism in HCC. (A) Copper impacts the autophagic pathway of HCC by affecting the formation of phagophore, autophagosome, and lysosome. (B) Copper directly affects the apoptotic pathway of HCC by generating ROS, forming apoptosome, and activating the apoptotic cascade response, and indirectly affects the apoptotic pathway of HCC by regulating ERK1/2 and PD-L1. (C) Copper affects vascular invasion by affecting endothelial cells as well as HCC metastasis.
Apoptosis is a programmed death process that is essential in normal development, tissue repair and immune modulation in the human body (43). The Fenton reaction is one of the most common reactions for copper-catalyzed ROS production, whereby high concentrations of copper-induced ROS lead to mitochondrial dysfunction with accelerated apoptosis (44). Moreover, the oxidation of hydroxyl radicals generated by the Fenton reaction can break down the DNA helix structure, resulting in damage to DNA (45). Mitra et al. (46) found that copper-treated spleen and thymus produced different apoptotic pathways. Recent studies (47) have revealed that mitogen-activated protein kinase kinase 1 (MEK1) in the MAPK-ERK pathway also has a high-affinity copper binding site. High concentrations of Cu+ stimulate MEK1-dependent phosphorylation of ERK1/2, and the use of copper chelators can target MEK and inhibit tumor proliferation (48). Excessive copper production in the liver induces ROS and mitochondrial transmembrane potential changes that activate the intrinsic pathway of p53 cell apoptosis (49). With the activation of P53, cytochrome C (Cyt C) induces caspase9 to initiate the cascade activation of downstream caspase3, which triggers apoptosis (50, 51). Cyt C, Apoptotic protease-activating factor 1 (Apaf-1) and caspase 9 could also combine to form an “apoptosome”, an apoptotic pathway that induces hepatocyte death (50, 52). The proteasome is a multi-enzyme complex consisting of a 20S core and two 19S regulatory particles (53). It has been shown that proteasome can induce Cyt C into cytoplasm and activate caspase cascade reaction to induce apoptosis, while copper complexes show strong inhibitory ability against proteasome, which may become a new target for tumor therapy (54, 55). It is worth noting that Cu activates the IL6/Jack/STAT3 signaling pathway after catalytic EGFR phosphorylation and is involved in the induction of IFNγ-mediated upregulation of PD-L1 expression (56). An overload of Cu leads to immunosuppression, which not only leads to the development of HCC, but is an important issue in the treatment of HCC. The apoptotic targets and pathways associated with copper metabolism in HCC are shown in Figure 2B.
Vascular invasion is an important mode of tumor progression as well as metastasis. Notably, copper is a key point in the angiogenic signaling cascade, and copper overload stimulates tumor neovascular growth and invasion, while copper deficiency hinders neointimal formation (57). It was found that copper could activate the EGFR/ERK/c-fos transduction pathway to induce vascular endothelial growth factor (VEGF) expression in hepatoma cells to promote tumor angiogenesis (58). In a further way, copper can also promote the synthesis of FGF-1 through ATOX1 and superoxide dismutase 1 (SOD1) to affect vascular endothelial function, while increasing the invasive and metastatic capacity of tumor cells by activating lysyl oxidase (LOX) (59–61). Copper can even induce the expression of HIF-1α to promote rapid adaptation of tumor cells to hypoxic conditions when the microenvironment is not suitable for tumor growth (62). Copper has a significant facilitating impact in HCC metastasis and angiogenesis, and copper chelators can be used as targeted agents to limit HCC vascular invasion (63). The targets and pathways of vascular invasion associated with copper metabolism in HCC are shown in Figure 2C.
As early as the 1980s, Halliwell et al. discovered that copper could lead to cell death (64). The mechanism and specific form of copper-induced cell death has long been obscure. In the past, copper ion carriers including disulfiram (DSF) and elesclomol (ES) were considered to have the ability to cause cell death. ES-Cu can bind to ferredoxin 1 (FDX1) and lead to inhibition of Fe-S clusters or act directly on mitochondrial membranes (65), which leads to polarization of the mitochondrial membrane potential and cell death via a ROS-mediated mechanism (66). Similarly, DSF/Cu was also considered to act on the mitochondrial respiratory chain, leading to elevated ROS levels (67). It has only recently been elucidated that cell death induced by copper ion carriers is a copper-induced cell death, independent of the known patterns of cell death. Cuproptosis is a regulated mode of cell death that is distinct from apoptosis and ferroptosis (68). The accumulation of intracellular copper ions acts on the lipid acylation of proteins in the tricarboxylic acid cycle (TCA), triggering proteotoxic stress and inducing cell death (69). Ferredoxin 1 (FDX1), an upstream regulator of protein lipid acylation, has been found to be a key regulator of cuproptosis with significant association with HCC staging and prognosis (70). In contrast to the general population, HCC patients have a much lower level of FDX1 expression, while the low expression of FDX1 suggests a poor prognosis, tumor cells acquire a survival advantage over healthy cells by resisting cuproptosis. In addition, it was found (71) that the cuproptosis-related gene LIPT1 may promote the proliferation and metastasis of HCC, which is a new potential therapeutic target for HCC. It is worth noting that cuproptosis may be an elemental factor in the development of HCC, but experimental studies on cuproptosis are still in their infancy, and most studies have only demonstrated the association of cuproptosis with the prognosis of HCC based on bioinformatic analysis of public databases such as TCGA, ICGC and GEO, so more studies are needed to support this point of view (72–76). The functions of cuproptosis characteristic genes in HCC are shown in Table 1.
Ferroptosis is a recently identified form of cell death characterized by iron accumulation and lipid peroxidation that has emerged as an effective therapeutic target for tumor suppression. We are well known for the fact that sorafenib can inhibit the progression of HCC by inducing ferroptosis (77). However, copper and iron which are common trace elements in human body, the association between copper metabolism and ferroptosis has been rarely reported. GPX4 is a key gene associated with ferroptosis. Exogenous copper promotes GPX4 ubiquitination by directly binding to GPX4 protein cysteines C107 and C148, accelerating GPX4 aggregate formation, and Tax1-binding protein 1 (TAX1BP1) is involved in the transformation breakdown of GPX4, leading to lipid peroxide accumulation and inducing ferroptosis (78). The plasma ceruloplasmin-ferroportin transport system is an active mode of intracellular iron transport in hepatocytes. Shang et al. (79) discovered that high copper level disrupted Cu-Fe homeostasis and that overexpression of CP inhibited ferroptosis induced by erastin and RSL3 in HCC cells. Despite the fact that ionizing radiation can slow tumor cell development by increasing ferroptosis, which is beneficial for patients with advanced HCC that cannot be surgically removed, the efficacy of radiation is hampered by radioresistance (80, 81). Notably, copper metabolism MURR1 domain 10 (COMMD10) is a critical protein in the regulation of radioresistance in HCC, which causes copper aggregation, thereby upregulating the expression of CP and SLC7A11 target genes, reducing lipid peroxidation levels, and inhibiting ferroptosis in HCC (82). Excessive accumulation of free intracellular copper causes cell death, and a similar result occurs when the intracellular copper content is depleted. Copper depletion-mediated metabolic reprogramming leads to mitochondrial perturbations and significant changes in lipids and lipid-like molecules (including increased levels of arachidonic and epinephrine acids), with high levels of ROS and lipid peroxidation strongly inducing ferroptosis (83). Interestingly, DSF/Cu which is combined with copper is an FDA approved clinical anti-alcoholic drug, and Ren et al. found that DSF/Cu can activate ferroptosis and synergize with sorafenib in the fight against HCC (84). There is a direct link between ferroptosis and copper, with copper acting as a crucial factor in the regulation of ferroptosis (Figure 3).
Figure 3 Association of copper metabolism with ferroptosis in HCC. Cu+ regulates the level of ferroptosis in HCC by activating GPX4 or promoting its ubiquitination. CP inhibits ferroptosis by directly inhibiting the iron death inducers Erastin and RSL3.
With the in-depth study of copper metabolism, the effects of copper on human health are becoming clear. How to safely, effectively and rationally use drugs to regulate copper homeostasis to treat diseases has attracted people’s attention. Previous treatments for inherited copper dysregulation diseases (such as Wilson disease, Menkes disease) provide reference for this. In terms of tumors, there are two main ways to regulate copper homeostasis: one is to chelate copper in tumor cells through copper chelates to inhibit copper proliferation; the other is using copper ionophores to increase the copper in tumor tissue to promote cuproptosis.
Copper chelators are commonly used to treat Wilson disease patients to lower their elevated copper in the blood. In the subsequent research, it was observed that anti-angiogenic agents can limit tumor growth and that copper restriction can reduce the levels of angiogenic factors to inhibit the angiogenesis of blood vessels (85, 86). Copper chelating agents have aroused people’s interest in the field of cancer. Researches have shown that copper can affect tumor angiogenesis by affecting HIF-1 (87); participat in tumor proliferation through the transcription factor ATOX1 (21), CCO (88) and MEK1 (89); regulate the Mediator of Cell Mobility protein (MEMO) (90), the Secreted Protein Acidic and Rich in Cysteine (SPARC) (91, 92), the copper binding enzymes LOX (93), to influence tumor spreading. Additionally, it has been documented that copper chelation can also promote ubiquitin mediated degradation of the immune checkpoint PD-L1, such that tumor cells cannot protect themselves from antitumor immune responses by overexpressing PD-L1 (56).Therefore, copper chelators may act on tumors through these pathways. At present, the inhibitory effect of copper chelators on tumor angiogenesis has received much attention. A crucial aspect of HCC is its abundant arterial blood supply. It is ideal for treatment with copper chelators due to this property. We believe that copper chelators have a bright future in the treatment of HCC.
Common copper chelators include tetrathiomolybdate (TM) and trientine. TM is a selective copper chelator which could deplete copper in tumor cells and reduce its bioavailability. TM can inhibit angiogenesis and reduce blood supply to tumor tissues by supressing transcription factors (NF-kB) and reduce tumor proliferative activity by inhibiting mitochondrial CCO function to reduce ATP production (88, 94). In addition, Davis et al. (95) suggested that TM could reduce the expression of glucose transporter 1 (GLUT1) and other glycolytic genes induced by hypoxia in HCC cells to decrease glucose utilization and limit energy acquisition. This shows that TM can limit the production of ATP in tumor cells through mitochondrial tricarboxylic acid cycle and glycolysis. The mechanism of TM for the treatment of HCC is shown in Figure 4. The safety of TM has also been recognized. In a phase II clinical trial, TM was well tolerated (96). Trientine is commonly used in patients who are intolerant to other copper chelators due to severe side effects such as myelosuppression and autoimmune diseases. KADOWAKI et al. (97) suggested that trientine induces tumor apoptosis by activating P38 mitogen-activated protein kinase, which is involved in multiple pathways to inhibit the growth of HCC and other tumors (98–101). A study using trientine in a mouse model of HCC xenotransplantation showed that trientine could limit tumor growth by inhibiting the growth of HCC endothelial cells and blood vessels, and promote the induction of tumor apoptosis (102). It is safer compared to copper chelator D-pen with lower incidence of serious side effects (103). Therefore, we believe that trientine has potential for clinical use in the treatment of HCC.
Figure 4 The mechanism of TM for the treatment of HCC. TM can inhibit angiogenesis and reduce vascular invasion of HCC by inhibiting transcription factors (NF-kB). In addition, it can also reduce the vascular invasion of HCC by inhibiting the tricarboxylic acid cycle and reducing the energy supply of tumor tissue by glycolysis.
In contrast to copper chelators which deplete copper bioavailability in tumor tissue to inhibit cuproplasia with the aim of achieving antitumor effects, copper ionophores increase the intracellular concentration of copper ions in tumor cells. Excessive intracellular copper ion concentration has cytotoxicity as it can lead to the production of a large amount of ROS (104). Additionally, copper ions directly attach to the lipoylated parts of the tricarboxylic acid (TCA) cycle, causing lipoylated protein to aggregate and Fe-S cluster protein to disappear (69). This causes protein toxicity stress, which kills or restricts tumor growth.
Currently, common copper ionophores are elesclomol(ES) and disulfiram(DSF). ES is an injectable, small molecule drug that binds to copper ions in the blood. Tumor cells can effectively absorb this complex. When Cu2+ of this complex enters the mitochondria of cells, it is reduced to Cu+ by mitochondrial protein FDX1. Cu+ will react with molecular oxygen to produce superoxide, which will be disproportionated to generate H2O2. H2O2 can further react with Cu+ to produce more destructive and highly reactive hydroxyl radicals, destroying mitochondria, and restricting the division and proliferation of tumor cells (105). Moreover, ES can also downregulate GSH (106), which is an important component of the intracellular antioxidant system and contributes to the clearance of intracellular ROS (107). Without a doubt, its decrease leads to the rise in intracellular ROS. Additionally, Tsvetkov et al. (65, 69) argued that ES has anti-tumor properties that go beyond merely its capacity to generate ROS in tumor cells. ES was discovered to be able to bind to FDX1, which results in the the aggregation of lipoylated mitochondrial enzymes and a loss of Fe–S proteins, leading to cuprotosis. Since this process requires the involvement of oxygen molecules, this drug is mainly suitable for cancers that are energized by oxidative phosphorylation of mitochondria. Under hypoxic conditions, the energy metabolism of tumors is mainly generated through glycolysis in the cytoplasm rather than the mitochondria, which is often accompanied by increased levels of the lactate dehydrogenase (LDH). At this time, the activity of ES is low. HCC cells have been reported to have a characteristic of significantly increased mitochondrial metabolism (108). This provides a theoretical basis for the use of ES in the treatment of HCC. Another study on carboplatin-resistant HCC cells also showed this feature (109). In terms of safety, ES has few reported side effects in humans and is well tolerated by patients. It was found that while ES was present at concentrations that significantly inhibited tumor cells, it did not enrich for copper ions in human peripheral blood mononuclear cells (105). This also suggests that the risk of side effects from taking this drug is low. The mechanism of elesclomol for the treatment of HCC is shown in Figure 5.
Figure 5 The mechanism of ES for the treatment of HCC. In mitochondria, the complex of ES and Cu2+ binds to FDX1, while Cu2+is reduced to Cu+ by FDX1. Cu+ combines with O2 to generate ROS. FDX1 also promotes the lipoylation of DLAT. The reduced Cu+ binds to lipoylated DLAT to promote its oligomerization, ultimately causing the occurrence of cuproptosis. Additionally, Cu+ prevents FDX1 from stimulating the synthesis of Fe-S.
DSF was used to treat alcohol dependence by inhibiting acetaldehyde dehydrogenase (ALDH). In the 1970s, researchers began seeking clinical evidence of the anti-cancer effects of DSF (110). DSF has anticancer action that is reliant on metal ions and promotes apoptosis while decreasing angiogenesis. In acidic conditions, DSF would be reduced to diethyl dithiocarbamate (DDTC), which may then be coupled with Cu2+ to create DDTC-Cu2+ complexes. There are a number of possible mechanisms of action for DSF and DDTC-Cu2+, including the generation of ROS (111), obstruction of the ubiquitin pathway (112), activation of the MAPK pathway (113), and irreversible inhibition of ALDH (114). In addition, a study by Li et al. (115) demonstrated that DSF in combination with copper could effectively inhibit the metastasis of HCC, suggesting that DSF in combination with copper could restrain the process of epithelial-mesenchymal transition (EMT) and the metastasis of HCC by limiting NF-kB and TGF-Β signaling. Concerning security, DSF has been approved by FDA for application, and its safety is generally acceptable. Current trial phase and validated targets information on copper chelators and copper ionophore for HCC are shown in Table 2.
Table 2 Current trial phase and validated targets information on copper chelators and copper ionophore for HCC.
The demand of tumor cells for copper ions and the transport of copper ions by CTR1 on the surface cause high copper concentration in tumor tissues. This makes copper complexes have the potential to act as tracers. The radioisotope 64Cu has been used for in vivo tumor imaging and therapy. 64Cu has a half-life of 12.7 hours and is suitable for imaging small molecules as well as large molecules such as antibodies and peptides. Its relatively short half-life does not add a radiation burden to patients after imaging studies, which is a advantage that can be applied to positron emission tomography (PET) imaging. Currently, 64CuCl2 has performed well in PET imaging of HCC in animal models (130).
In addition, copper-based nanoparticles have been applied in both photodynamic therapy PDT and photothermal therapy PTT. Huang et al. (131) used copper-cysteamine nanoparticles as photosensitizers for HCC and achieved good therapeutic results. It is notable that the homeostasis of copper ions in the human body is essential, and either its deficiency or its excess can cause human diseases. The long-term use of copper binding compounds, including copper chelators and copper ionophores, may disturb the homeostasis of essential metals, thus resulting in severe side effects. Although copper conjugates exhibit certain selectivity toward tumor cells, their therapeutic window still needs to be enlarged for safer applications. There is a need to develop more rational strategies and new therapeutic modalities to increase targeting to tumor cells, improve efficacy against tumors, as well as mitigate side effects.
With the development of cross-cutting disciplines, the pathways of copper metabolism involved in human activities have been elucidated. Copper is absorbed in the small intestine and stored by vital organs such as the liver. Copper is essential for the regulation of the physiological functions of the liver. Excess copper would be excreted via the biliary tract when copper levels in the body are high. However, the state of cuproplasia is commonly seen in the microenvironment of HCC. Copper overload accelerates the progression of HCC through immunosuppression, vascular invasion, cuproptosis and ferroptosis. Current therapies on copper metabolism in HCC include copper chelators, copper ionophores, etc., all of which have a good safety profile. Currently, copper metabolism research is in a preliminary stage and this therapy is not explicitly recommended in the guidelines despite clear therapeutic effects, so further clinical studies are still urgently needed.
CZ, TL and JY gathered information and designed the review. PS and RJ drew the pictures. LY and WZ critically revised the manuscript. All authors contributed to the article and approved the submitted version.
National Natural Science Foundation of China (81473651); Construction Project of Chinese Medicine Discipline in Henan Province (STG-ZYXKY-2020024); Construction Project of National Famous Chinese Medicine Inheritance Studio (National TCM Office Human Education Letter [2022] No. 245); Henan Provincial Science and Technology Tackling Program Project (202102310495); National Chinese medicine clinical research base business construction research project (No.JDZX2012050). National Science and Technology Major Project of the Ministry of Science and Technology of China (2018ZX10303502).
The authors declare that the research was conducted in the absence of any commercial or financial relationships that could be construed as a potential conflict of interest.
All claims expressed in this article are solely those of the authors and do not necessarily represent those of their affiliated organizations, or those of the publisher, the editors and the reviewers. Any product that may be evaluated in this article, or claim that may be made by its manufacturer, is not guaranteed or endorsed by the publisher.
1. Sung H, Ferlay J, Siegel RL, Laversanne M, Soerjomataram I, Jemal A, et al. Global cancer statistics 2020: globocan estimates of incidence and mortality worldwide for 36 cancers in 185 countries. CA Cancer J Clin (2021) 71(3):209–49. doi: 10.3322/caac.21660
2. Forner A, Reig M, Bruix J. Hepatocellular carcinoma. Lancet (2018) 391(10127):1301–14. doi: 10.1016/s0140-6736(18)30010-2
3. Li Y. Copper homeostasis: emerging target for cancer treatment. IUBMB Life (2020) 72(9):1900–8. doi: 10.1002/iub.2341
4. Ge EJ, Bush AI, Casini A, Cobine PA, Cross JR, DeNicola GM, et al. Connecting copper and cancer: from transition metal signalling to metalloplasia. Nat Rev Cancer (2022) 22(2):102–13. doi: 10.1038/s41568-021-00417-2
5. Bost M, Houdart S, Oberli M, Kalonji E, Huneau JF, Margaritis I. Dietary copper and human health: current evidence and unresolved issues. J Trace Elem Med Biol (2016) 35:107–15. doi: 10.1016/j.jtemb.2016.02.006
6. Morrell A, Tallino S, Yu L, Burkhead JL. The role of insufficient copper in lipid synthesis and fatty-liver disease. IUBMB Life (2017) 69(4):263–70. doi: 10.1002/iub.1613
7. de Romaña DL, Olivares M, Uauy R, Araya M. Risks and benefits of copper in light of new insights of copper homeostasis. J Trace Elem Med Biol (2011) 25(1):3–13. doi: 10.1016/j.jtemb.2010.11.004
8. Knutson MD. Steap proteins: implications for iron and copper metabolism. Nutr Rev (2007) 65(7):335–40. doi: 10.1111/j.1753-4887.2007.tb00311.x
9. McKie AT, Barrow D, Latunde-Dada GO, Rolfs A, Sager G, Mudaly E, et al. An iron-regulated ferric reductase associated with the absorption of dietary iron. Science (2001) 291(5509):1755–9. doi: 10.1126/science.1057206
10. Zimnicka AM, Ivy K, Kaplan JH. Acquisition of dietary copper: a role for anion transporters in intestinal apical copper uptake. Am J Physiol Cell Physiol (2011) 300(3):C588–99. doi: 10.1152/ajpcell.00054.2010
11. Gromadzka G, Tarnacka B, Flaga A, Adamczyk A. Copper dyshomeostasis in neurodegenerative diseases-therapeutic implications. Int J Mol Sci (2020) 21(23):9259. doi: 10.3390/ijms21239259
12. Monty JF, Llanos RM, Mercer JF, Kramer DR. Copper exposure induces trafficking of the menkes protein in intestinal epithelium of Atp7a transgenic mice. J Nutr (2005) 135(12):2762–6. doi: 10.1093/jn/135.12.2762
13. Pierson H, Muchenditsi A, Kim BE, Ralle M, Zachos N, Huster D, et al. The function of atpase copper transporter Atp7b in intestine. Gastroenterology (2018) 154(1):168–80.e5. doi: 10.1053/j.gastro.2017.09.019
14. Pohanka M. Copper and copper nanoparticles toxicity and their impact on basic functions in the body. Bratisl Lek Listy (2019) 120(6):397–409. doi: 10.4149/bll_2019_065
15. Vašák M, Meloni G. Mammalian metallothionein-3: new functional and structural insights. Int J Mol Sci (2017) 18(6):1117. doi: 10.3390/ijms18061117
16. Lutsenko S, Barnes NL, Bartee MY, Dmitriev OY. Function and regulation of human copper-transporting atpases. Physiol Rev (2007) 87(3):1011–46. doi: 10.1152/physrev.00004.2006
17. Linder MC. Copper homeostasis in mammals, with emphasis on secretion and excretion. A Review. Int J Mol Sci (2020) 21(14):4932. doi: 10.3390/ijms21144932
18. Polishchuk EV, Concilli M, Iacobacci S, Chesi G, Pastore N, Piccolo P, et al. Wilson Disease protein Atp7b utilizes lysosomal exocytosis to maintain copper homeostasis. Dev Cell (2014) 29(6):686–700. doi: 10.1016/j.devcel.2014.04.033
19. Bulcke F, Dringen R, Scheiber IF. Neurotoxicity of copper. Adv Neurobiol (2017) 18:313–43. doi: 10.1007/978-3-319-60189-2_16
20. Gralla EB, Valentine JS. Null mutants of saccharomyces cerevisiae Cu,Zn superoxide dismutase: characterization and spontaneous mutation rates. J Bacteriol (1991) 173(18):5918–20. doi: 10.1128/jb.173.18.5918-5920.1991
21. Itoh S, Kim HW, Nakagawa O, Ozumi K, Lessner SM, Aoki H, et al. Novel role of antioxidant-1 (Atox1) as a copper-dependent transcription factor involved in cell proliferation. J Biol Chem (2008) 283(14):9157–67. doi: 10.1074/jbc.M709463200
22. Blockhuys S, Celauro E, Hildesjö C, Feizi A, Stål O, Fierro-González JC, et al. Defining the human copper proteome and analysis of its expression variation in cancers. Metallomics (2017) 9(2):112–23. doi: 10.1039/c6mt00202a
23. Pavithra V, Sathisha TG, Kasturi K, Mallika DS, Amos SJ, Ragunatha S. Serum levels of metal ions in female patients with breast cancer. J Clin Diagn Res (2015) 9(1):BC25–c7. doi: 10.7860/jcdr/2015/11627.5476
24. Stepien M, Jenab M, Freisling H, Becker NP, Czuban M, Tjønneland A, et al. Pre-diagnostic copper and zinc biomarkers and colorectal cancer risk in the European prospective investigation into cancer and nutrition cohort. Carcinogenesis (2017) 38(7):699–707. doi: 10.1093/carcin/bgx051
25. Zhang X, Yang Q. Association between serum copper levels and lung cancer risk: a meta-analysis. J Int Med Res (2018) 46(12):4863–73. doi: 10.1177/0300060518798507
26. Basu S, Singh MK, Singh TB, Bhartiya SK, Singh SP, Shukla VK. Heavy and trace metals in carcinoma of the gallbladder. World J Surg (2013) 37(11):2641–6. doi: 10.1007/s00268-013-2164-9
27. Garza NM, Swaminathan AB, Maremanda KP, Zulkifli M, Gohil VM. Mitochondrial copper in human genetic disorders. Trends Endocrinol Metab (2023) 34(1):21–33. doi: 10.1016/j.tem.2022.11.001
28. Guindi M. Wilson Disease. Semin Diagn Pathol (2019) 36(6):415–22. doi: 10.1053/j.semdp.2019.07.008
29. Fanni D, Fanos V, Gerosa C, Piras M, Dessi A, Atzei A, et al. Effects of iron and copper overload on the human liver: an ultrastructural study. Curr Med Chem (2014) 21(33):3768–74. doi: 10.2174/0929867321666140601163244
30. Poznański J, Sołdacki D, Czarkowska-Pączek B, Bonna A, Kornasiewicz O, Krawczyk M, et al. Cirrhotic liver of liver transplant recipients accumulate silver and Co-accumulate copper. Int J Mol Sci (2021) 22(4):1782. doi: 10.3390/ijms22041782
31. Stepien M, Hughes DJ, Hybsier S, Bamia C, Tjønneland A, Overvad K, et al. Circulating copper and zinc levels and risk of hepatobiliary cancers in europeans. Br J Cancer (2017) 116(5):688–96. doi: 10.1038/bjc.2017.1
32. Fang AP, Chen PY, Wang XY, Liu ZY, Zhang DM, Luo Y, et al. Serum copper and zinc levels at diagnosis and hepatocellular carcinoma survival in the guangdong liver cancer cohort. Int J Cancer (2019) 144(11):2823–32. doi: 10.1002/ijc.31991
33. Wang Y, Zhang Y, Wang L, Zhang N, Xu W, Zhou J, et al. Development and experimental verification of a prognosis model for cuproptosis-related subtypes in hcc. Hepatol Int (2022) 16(6):1435–47. doi: 10.1007/s12072-022-10381-0
34. Jiang Y, Huo Z, Qi X, Zuo T, Wu Z. Copper-induced tumor cell death mechanisms and antitumor theragnostic applications of copper complexes. Nanomedicine (Lond) (2022) 17(5):303–24. doi: 10.2217/nnm-2021-0374
35. Moloney JN, Cotter TG. Ros signalling in the biology of cancer. Semin Cell Dev Biol (2018) 80:50–64. doi: 10.1016/j.semcdb.2017.05.023
36. Tsang T, Posimo JM, Gudiel AA, Cicchini M, Feldser DM, Brady DC. Copper is an essential regulator of the autophagic kinases Ulk1/2 to drive lung adenocarcinoma. Nat Cell Biol (2020) 22(4):412–24. doi: 10.1038/s41556-020-0481-4
37. Wan F, Zhong G, Ning Z, Liao J, Yu W, Wang C, et al. Long-term exposure to copper induces autophagy and apoptosis through oxidative stress in rat kidneys. Ecotoxicol Environ Saf (2020) 190:110158. doi: 10.1016/j.ecoenv.2019.110158
38. Guo H, Ouyang Y, Yin H, Cui H, Deng H, Liu H, et al. Induction of autophagy Via the ros-dependent ampk-mtor pathway protects copper-induced spermatogenesis disorder. Redox Biol (2022) 49:102227. doi: 10.1016/j.redox.2021.102227
39. Peña KA, Kiselyov K. Transition metals activate tfeb in overexpressing cells. Biochem J (2015) 470(1):65–76. doi: 10.1042/bj20140645
40. Tian LY, Smit DJ, Jücker M. The role of Pi3k/Akt/Mtor signaling in hepatocellular carcinoma metabolism. Int J Mol Sci (2023) 24(3):2652. doi: 10.3390/ijms24032652
41. Polishchuk EV, Merolla A, Lichtmannegger J, Romano A, Indrieri A, Ilyechova EY, et al. Activation of autophagy, observed in liver tissues from patients with Wilson disease and from Atp7b-deficient animals, protects hepatocytes from copper-induced apoptosis. Gastroenterology (2019) 156(4):1173–89.e5. doi: 10.1053/j.gastro.2018.11.032
42. Yazdani HO, Huang H, Tsung A. Autophagy: dual response in the development of hepatocellular carcinoma. Cells (2019) 8(2):91. doi: 10.3390/cells8020091
43. Shi Y. Mechanisms of caspase activation and inhibition during apoptosis. Mol Cell (2002) 9(3):459–70. doi: 10.1016/s1097-2765(02)00482-3
44. Zhang F, Li F, Lu GH, Nie W, Zhang L, Lv Y, et al. Engineering magnetosomes for Ferroptosis/Immunomodulation synergism in cancer. ACS Nano (2019) 13(5):5662–73. doi: 10.1021/acsnano.9b00892
45. Gupte A, Mumper RJ. Elevated copper and oxidative stress in cancer cells as a target for cancer treatment. Cancer Treat Rev (2009) 35(1):32–46. doi: 10.1016/j.ctrv.2008.07.004
46. Mitra S, Keswani T, Ghosh N, Goswami S, Datta A, Das S, et al. Copper induced immunotoxicity promote differential apoptotic pathways in spleen and thymus. Toxicology (2013) 306:74–84. doi: 10.1016/j.tox.2013.01.001
47. Turski ML, Brady DC, Kim HJ, Kim BE, Nose Y, Counter CM, et al. A novel role for copper in Ras/Mitogen-activated protein kinase signaling. Mol Cell Biol (2012) 32(7):1284–95. doi: 10.1128/mcb.05722-11
48. Brady DC, Crowe MS, Greenberg DN, Counter CM. Copper chelation inhibits Braf(V600e)-driven melanomagenesis and counters resistance to Braf(V600e) and Mek1/2 inhibitors. Cancer Res (2017) 77(22):6240–52. doi: 10.1158/0008-5472.Can-16-1190
49. Keswani T, Mitra S, Bhattacharyya A. Copper-induced immunotoxicity involves cell cycle arrest and cell death in the liver. Environ Toxicol (2015) 30(4):411–21. doi: 10.1002/tox.21916
50. Jiang X, Wang X. Cytochrome c-mediated apoptosis. Annu Rev Biochem (2004) 73:87–106. doi: 10.1146/annurev.biochem.73.011303.073706
51. Xiong C, Ling H, Hao Q, Zhou X. Cuproptosis: P53-regulated metabolic cell death? Cell Death Differ (2023) 30(4):876–84. doi: 10.1038/s41418-023-01125-0
52. Liu H, Guo H, Jian Z, Cui H, Fang J, Zuo Z, et al. Copper induces oxidative stress and apoptosis in the mouse liver. Oxid Med Cell Longev (2020) 2020:1359164. doi: 10.1155/2020/1359164
53. Bard JAM, Goodall EA, Greene ER, Jonsson E, Dong KC, Martin A. Structure and function of the 26s proteasome. Annu Rev Biochem (2018) 87:697–724. doi: 10.1146/annurev-biochem-062917-011931
54. Li DD, Yagüe E, Wang LY, Dai LL, Yang ZB, Zhi S, et al. Novel copper complexes that inhibit the proteasome and trigger apoptosis in triple-negative breast cancer cells. ACS Med Chem Lett (2019) 10(9):1328–35. doi: 10.1021/acsmedchemlett.9b00284
55. Gałczyńska K, Drulis-Kawa Z, Arabski M. Antitumor activity of Pt(Ii), Ru(Iii) and Cu(Ii) complexes. Molecules (2020) 25(15):3492. doi: 10.3390/molecules25153492
56. Voli F, Valli E, Lerra L, Kimpton K, Saletta F, Giorgi FM, et al. Intratumoral copper modulates pd-L1 expression and influences tumor immune evasion. Cancer Res (2020) 80(19):4129–44. doi: 10.1158/0008-5472.Can-20-0471
57. Urso E, Maffia M. Behind the link between copper and angiogenesis: established mechanisms and an overview on the role of vascular copper transport systems. J Vasc Res (2015) 52(3):172–96. doi: 10.1159/000438485
58. Rigiracciolo DC, Scarpelli A, Lappano R, Pisano A, Santolla MF, De Marco P, et al. Copper activates hif-1α/Gper/Vegf signalling in cancer cells. Oncotarget (2015) 6(33):34158–77. doi: 10.18632/oncotarget.5779
59. Fukai T, Ushio-Fukai M, Kaplan JH. Copper transporters and copper chaperones: roles in cardiovascular physiology and disease. Am J Physiol Cell Physiol (2018) 315(2):C186–c201. doi: 10.1152/ajpcell.00132.2018
60. Wong CC, Tse AP, Huang YP, Zhu YT, Chiu DK, Lai RK, et al. Lysyl oxidase-like 2 is critical to tumor microenvironment and metastatic niche formation in hepatocellular carcinoma. Hepatology (2014) 60(5):1645–58. doi: 10.1002/hep.27320
61. Shanbhag V, Jasmer-McDonald K, Zhu S, Martin AL, Gudekar N, Khan A, et al. Atp7a delivers copper to the lysyl oxidase family of enzymes and promotes tumorigenesis and metastasis. Proc Natl Acad Sci U.S.A. (2019) 116(14):6836–41. doi: 10.1073/pnas.1817473116
62. Martin F, Linden T, Katschinski DM, Oehme F, Flamme I, Mukhopadhyay CK, et al. Copper-dependent activation of hypoxia-inducible factor (Hif)-1: implications for ceruloplasmin regulation. Blood (2005) 105(12):4613–9. doi: 10.1182/blood-2004-10-3980
63. Gaur K, Vázquez-Salgado AM, Duran-Camacho G, Dominguez-Martinez I, Benjamín-Rivera JA, Fernández-Vega L, et al. Iron and copper intracellular chelation as an anticancer drug strategy. Inorganics (Basel) (2018) 6(4):126. doi: 10.3390/inorganics6040126
64. Halliwell B, Gutteridge JM. Oxygen toxicity, oxygen radicals, transition metals and disease. Biochem J (1984) 219(1):1–14. doi: 10.1042/bj2190001
65. Tsvetkov P, Detappe A, Cai K, Keys HR, Brune Z, Ying W, et al. Mitochondrial metabolism promotes adaptation to proteotoxic stress. Nat Chem Biol (2019) 15(7):681–9. doi: 10.1038/s41589-019-0291-9
66. Buccarelli M, D'Alessandris QG, Matarrese P, Mollinari C, Signore M, Cappannini A, et al. Elesclomol-induced increase of mitochondrial reactive oxygen species impairs glioblastoma stem-like cell survival and tumor growth. J Exp Clin Cancer Res (2021) 40(1):228. doi: 10.1186/s13046-021-02031-4
67. Jiao Y, Hannafon BN, Ding WQ. Disulfiram's anticancer activity: evidence and mechanisms. Anticancer Agents Med Chem (2016) 16(11):1378–84. doi: 10.2174/1871520615666160504095040
68. Tang D, Kang R, Berghe TV, Vandenabeele P, Kroemer G. The molecular machinery of regulated cell death. Cell Res (2019) 29(5):347–64. doi: 10.1038/s41422-019-0164-5
69. Tsvetkov P, Coy S, Petrova B, Dreishpoon M, Verma A, Abdusamad M, et al. Copper induces cell death by targeting lipoylated tca cycle proteins. Science (2022) 375(6586):1254–61. doi: 10.1126/science.abf0529
70. Zhang Z, Zeng X, Wu Y, Liu Y, Zhang X, Song Z. Cuproptosis-related risk score predicts prognosis and characterizes the tumor microenvironment in hepatocellular carcinoma. Front Immunol (2022) 13:925618. doi: 10.3389/fimmu.2022.925618
71. Yan C, Niu Y, Ma L, Tian L, Ma J. System analysis based on the cuproptosis-related genes identifies Lipt1 as a novel therapy target for liver hepatocellular carcinoma. J Transl Med (2022) 20(1):452. doi: 10.1186/s12967-022-03630-1
72. Ma YL, Yang YF, Wang HC, Yang CC, Yan LJ, Ding ZN, et al. A novel prognostic scoring model based on copper homeostasis and cuproptosis which indicates changes in tumor microenvironment and affects treatment response. Front Pharmacol (2023) 14:1101749. doi: 10.3389/fphar.2023.1101749
73. Fu J, Wang S, Li Z, Qin W, Tong Q, Liu C, et al. Comprehensive multiomics analysis of cuproptosis-related gene characteristics in hepatocellular carcinoma. Front Genet (2022) 13:942387. doi: 10.3389/fgene.2022.942387
74. Chen Y, Tang L, Huang W, Abisola FH, Zhang Y, Zhang G, et al. Identification of a prognostic cuproptosis-related signature in hepatocellular carcinoma. Biol Direct (2023) 18(1):4. doi: 10.1186/s13062-023-00358-w
75. Zhang G, Sun J, Zhang X. A novel cuproptosis-related lncrna signature to predict prognosis in hepatocellular carcinoma. Sci Rep (2022) 12(1):11325. doi: 10.1038/s41598-022-15251-1
76. Wang G, Xiao R, Zhao S, Sun L, Guo J, Li W, et al. Cuproptosis regulator-mediated patterns associated with immune infiltration features and construction of cuproptosis-related signatures to guide immunotherapy. Front Immunol (2022) 13:945516. doi: 10.3389/fimmu.2022.945516
77. Li Y, Xia J, Shao F, Zhou Y, Yu J, Wu H, et al. Sorafenib induces mitochondrial dysfunction and exhibits synergistic effect with cysteine depletion by promoting hcc cells ferroptosis. Biochem Biophys Res Commun (2021) 534:877–84. doi: 10.1016/j.bbrc.2020.10.083
78. Xue Q, Yan D, Chen X, Li X, Kang R, Klionsky DJ, et al. Copper-dependent autophagic degradation of Gpx4 drives ferroptosis. Autophagy (2023) 19(7):1982–1996. doi: 10.1080/15548627.2023.2165323
79. Shang Y, Luo M, Yao F, Wang S, Yuan Z, Yang Y. Ceruloplasmin suppresses ferroptosis by regulating iron homeostasis in hepatocellular carcinoma cells. Cell Signal (2020) 72:109633. doi: 10.1016/j.cellsig.2020.109633
80. Lei G, Zhang Y, Koppula P, Liu X, Zhang J, Lin SH, et al. The role of ferroptosis in ionizing radiation-induced cell death and tumor suppression. Cell Res (2020) 30(2):146–62. doi: 10.1038/s41422-019-0263-3
81. Krisnawan VE, Stanley JA, Schwarz JK, DeNardo DG. Tumor microenvironment as a regulator of radiation therapy: new insights into stromal-mediated radioresistance. Cancers (Basel) (2020) 12(10):2916. doi: 10.3390/cancers12102916
82. Yang M, Wu X, Hu J, Wang Y, Wang Y, Zhang L, et al. Commd10 inhibits Hif1α/Cp loop to enhance ferroptosis and radiosensitivity by disrupting Cu-fe balance in hepatocellular carcinoma. J Hepatol (2022) 76(5):1138–50. doi: 10.1016/j.jhep.2022.01.009
83. Li F, Wu X, Liu H, Liu M, Yue Z, Wu Z, et al. Copper depletion strongly enhances ferroptosis via mitochondrial perturbation and reduction in antioxidative mechanisms. Antioxidants (Basel) (2022) 11(11):2084. doi: 10.3390/antiox11112084
84. Ren X, Li Y, Zhou Y, Hu W, Yang C, Jing Q, et al. Overcoming the compensatory elevation of Nrf2 renders hepatocellular carcinoma cells more vulnerable to Disulfiram/Copper-induced ferroptosis. Redox Biol (2021) 46:102122. doi: 10.1016/j.redox.2021.102122
85. Brewer GJ, Dick RD, Grover DK, LeClaire V, Tseng M, Wicha M, et al. Treatment of metastatic cancer with tetrathiomolybdate, an anticopper, antiangiogenic agent: phase I study. Clin Cancer Res (2000) 6(1):1–10.
86. Pan Q, Kleer CG, van Golen KL, Irani J, Bottema KM, Bias C, et al. Copper deficiency induced by tetrathiomolybdate suppresses tumor growth and angiogenesis. Cancer Res (2002) 62(17):4854–9.
87. Wu Z, Zhang W, Kang YJ. Copper affects the binding of hif-1α to the critical motifs of its target genes. Metallomics (2019) 11(2):429–38. doi: 10.1039/c8mt00280k
88. Ishida S, Andreux P, Poitry-Yamate C, Auwerx J, Hanahan D. Bioavailable copper modulates oxidative phosphorylation and growth of tumors. Proc Natl Acad Sci U.S.A. (2013) 110(48):19507–12. doi: 10.1073/pnas.1318431110
89. Brady DC, Crowe MS, Turski ML, Hobbs GA, Yao X, Chaikuad A, et al. Copper is required for oncogenic braf signalling and tumorigenesis. Nature (2014) 509(7501):492–6. doi: 10.1038/nature13180
90. MacDonald G, Nalvarte I, Smirnova T, Vecchi M, Aceto N, Dolemeyer A, et al. Memo is a copper-dependent redox protein with an essential role in migration and metastasis. Sci Signal (2014) 7(329):ra56. doi: 10.1126/scisignal.2004870
91. Blockhuys S, Wittung-Stafshede P. Roles of copper-binding proteins in breast cancer. Int J Mol Sci (2017) 18(4):871. doi: 10.3390/ijms18040871
92. Morrissey MA, Jayadev R, Miley GR, Blebea CA, Chi Q, Ihara S, et al. Sparc promotes cell invasion in vivo by decreasing type iv collagen levels in the basement membrane. PLoS Genet (2016) 12(2):e1005905. doi: 10.1371/journal.pgen.1005905
93. Wang TH, Hsia SM, Shieh TM. Lysyl oxidase and the tumor microenvironment. Int J Mol Sci (2016) 18(1):62. doi: 10.3390/ijms18010062
94. Pan Q, Bao LW, Merajver SD. Tetrathiomolybdate inhibits angiogenesis and metastasis through suppression of the nfkappab signaling cascade. Mol Cancer Res (2003) 1(10):701–6.
95. Davis CI, Gu X, Kiefer RM, Ralle M, Gade TP, Brady DC. Altered copper homeostasis underlies sensitivity of hepatocellular carcinoma to copper chelation. Metallomics (2020) 12(12):1995–2008. doi: 10.1039/d0mt00156b
96. Chan N, Willis A, Kornhauser N, Ward MM, Lee SB, Nackos E, et al. Influencing the tumor microenvironment: a phase ii study of copper depletion using tetrathiomolybdate in patients with breast cancer at high risk for recurrence and in preclinical models of lung metastases. Clin Cancer Res (2017) 23(3):666–76. doi: 10.1158/1078-0432.Ccr-16-1326
97. Kadowaki S, Endoh D, Okui T, Hayashi M. Trientine, a copper-chelating agent, induced apoptosis in murine fibrosarcoma cells by activation of the P38 mapk pathway. J Vet Med Sci (2009) 71(11):1541–4. doi: 10.1292/jvms.001541
98. Han J, Sun P. The pathways to tumor suppression Via route P38. Trends Biochem Sci (2007) 32(8):364–71. doi: 10.1016/j.tibs.2007.06.007
99. Iwasa H, Han J, Ishikawa F. Mitogen-activated protein kinase P38 defines the common senescence-signalling pathway. Genes Cells (2003) 8(2):131–44. doi: 10.1046/j.1365-2443.2003.00620.x
100. Liu Z, Ning F, Cai Y, Sheng H, Zheng R, Yin X, et al. The egfr-P38 mapk axis up-regulates pd-L1 through mir-675-5p and down-regulates hla-abc Via hexokinase-2 in hepatocellular carcinoma cells. Cancer Commun (Lond) (2021) 41(1):62–78. doi: 10.1002/cac2.12117
101. Yang Z, Zhang H, Yin M, Cheng Z, Jiang P, Feng M, et al. Neurotrophin3 promotes hepatocellular carcinoma apoptosis through the jnk and P38 mapk pathways. Int J Biol Sci (2022) 18(15):5963–77. doi: 10.7150/ijbs.72982
102. Yoshii J, Yoshiji H, Kuriyama S, Ikenaka Y, Noguchi R, Okuda H, et al. The copper-chelating agent, trientine, suppresses tumor development and angiogenesis in the murine hepatocellular carcinoma cells. Int J Cancer (2001) 94(6):768–73. doi: 10.1002/ijc.1537
103. Walshe JM. Treatment of wilson's disease with trientine (Triethylene tetramine) dihydrochloride. Lancet (1982) 1(8273):643–7. doi: 10.1016/s0140-6736(82)92201-2
104. Xiao Y, Chen DI, Zhang X, Cui Q, Fan Y, Bi C, et al. Molecular study on copper-mediated tumor proteasome inhibition and cell death. Int J Oncol (2010) 37(1):81–7. doi: 10.3892/ijo_00000655
105. Nagai M, Vo NH, Shin Ogawa L, Chimmanamada D, Inoue T, Chu J, et al. The oncology drug elesclomol selectively transports copper to the mitochondria to induce oxidative stress in cancer cells. Free Radic Biol Med (2012) 52(10):2142–50. doi: 10.1016/j.freeradbiomed.2012.03.017
106. Wangpaichitr M, Wu C, You M, Maher JC, Dinh V, Feun LG, et al. N',N'-Dimethyl-N',N'-Bis(Phenylcarbonothioyl) propanedihydrazide (Elesclomol) selectively kills cisplatin resistant lung cancer cells through reactive oxygen species (Ros). Cancers (Basel) (2009) 1(1):23–38. doi: 10.3390/cancers1010023
107. Niu B, Liao K, Zhou Y, Wen T, Quan G, Pan X, et al. Application of glutathione depletion in cancer therapy: enhanced ros-based therapy, ferroptosis, and chemotherapy. Biomaterials (2021) 277:121110. doi: 10.1016/j.biomaterials.2021.121110
108. Zhang Z, Li TE, Chen M, Xu D, Zhu Y, Hu BY, et al. Mfn1-dependent alteration of mitochondrial dynamics drives hepatocellular carcinoma metastasis by glucose metabolic reprogramming. Br J Cancer (2020) 122(2):209–20. doi: 10.1038/s41416-019-0658-4
109. Sun Y, Xu H, Chen X, Li X, Luo B. Inhibition of mitochondrial respiration overcomes hepatocellular carcinoma chemoresistance. Biochem Biophys Res Commun (2019) 508(2):626–32. doi: 10.1016/j.bbrc.2018.11.182
111. Carnero A, Garcia-Mayea Y, Mir C, Lorente J, Rubio IT, ME LL. The cancer stem-cell signaling network and resistance to therapy. Cancer Treat Rev (2016) 49:25–36. doi: 10.1016/j.ctrv.2016.07.001
112. Chen D, Cui QC, Yang H, Dou QP. Disulfiram, a clinically used anti-alcoholism drug and copper-binding agent, induces apoptotic cell death in breast cancer cultures and xenografts Via inhibition of the proteasome activity. Cancer Res (2006) 66(21):10425–33. doi: 10.1158/0008-5472.Can-06-2126
113. Chiba T, Suzuki E, Yuki K, Zen Y, Oshima M, Miyagi S, et al. Disulfiram eradicates tumor-initiating hepatocellular carcinoma cells in ros-P38 mapk pathway-dependent and -independent manners. PLoS One (2014) 9(1):e84807. doi: 10.1371/journal.pone.0084807
114. Wang NN, Wang LH, Li Y, Fu SY, Xue X, Jia LN, et al. Targeting Aldh2 with Disulfiram/Copper reverses the resistance of cancer cells to microtubule inhibitors. Exp Cell Res (2018) 362(1):72–82. doi: 10.1016/j.yexcr.2017.11.004
115. Li Y, Wang LH, Zhang HT, Wang YT, Liu S, Zhou WL, et al. Disulfiram combined with copper inhibits metastasis and epithelial-mesenchymal transition in hepatocellular carcinoma through the nf-Κb and tgf-Β pathways. J Cell Mol Med (2018) 22(1):439–51. doi: 10.1111/jcmm.13334
116. Morisawa A, Okui T, Shimo T, Ibaragi S, Okusha Y, Ono M, et al. Ammonium tetrathiomolybdate enhances the antitumor effects of cetuximab Via the suppression of osteoclastogenesis in head and neck squamous carcinoma. Int J Oncol (2018) 52(3):989–99. doi: 10.3892/ijo.2018.4242
117. Xu M, Casio M, Range DE, Sosa JA, Counter CM. Copper chelation as targeted therapy in a mouse model of oncogenic braf-driven papillary thyroid cancer. Clin Cancer Res (2018) 24(17):4271–81. doi: 10.1158/1078-0432.Ccr-17-3705
118. Mandinova A, Soldi R, Graziani I, Bagala C, Bellum S, Landriscina M, et al. S100a13 mediates the copper-dependent stress-induced release of il-1alpha from both human U937 and murine nih 3t3 cells. J Cell Sci (2003) 116(Pt 13):2687–96. doi: 10.1242/jcs.00471
119. Landriscina M, Bagalá C, Mandinova A, Soldi R, Micucci I, Bellum S, et al. Copper induces the assembly of a multiprotein aggregate implicated in the release of fibroblast growth factor 1 in response to stress. J Biol Chem (2001) 276(27):25549–57. doi: 10.1074/jbc.M102925200
120. Hassouneh B, Islam M, Nagel T, Pan Q, Merajver SD, Teknos TN. Tetrathiomolybdate promotes tumor necrosis and prevents distant metastases by suppressing angiogenesis in head and neck cancer. Mol Cancer Ther (2007) 6(3):1039–45. doi: 10.1158/1535-7163.Mct-06-0524
121. Redman BG, Esper P, Pan Q, Dunn RL, Hussain HK, Chenevert T, et al. Phase ii trial of tetrathiomolybdate in patients with advanced kidney cancer. Clin Cancer Res (2003) 9(5):1666–72.
122. Henry NL, Dunn R, Merjaver S, Pan Q, Pienta KJ, Brewer G, et al. Phase ii trial of copper depletion with tetrathiomolybdate as an antiangiogenesis strategy in patients with hormone-refractory prostate cancer. Oncology (2006) 71(3-4):168–75. doi: 10.1159/000106066
123. Chan N, Willis A, Kornhauser N, Ward MM, Lee SB, Nackos E, et al. Correction: influencing the tumor microenvironment: a phase ii study of copper depletion using tetrathiomolybdate in patients with breast cancer at high risk for recurrence and in preclinical models of lung metastases. Clin Cancer Res (2020) 26(18):5051. doi: 10.1158/1078-0432.Ccr-20-3177
124. Moriguchi M, Nakajima T, Kimura H, Watanabe T, Takashima H, Mitsumoto Y, et al. The copper chelator trientine has an antiangiogenic effect against hepatocellular carcinoma, possibly through inhibition of interleukin-8 production. Int J Cancer (2002) 102(5):445–52. doi: 10.1002/ijc.10740
125. Mammoto T, Jiang A, Jiang E, Panigrahy D, Kieran MW, Mammoto A. Role of collagen matrix in tumor angiogenesis and glioblastoma multiforme progression. Am J Pathol (2013) 183(4):1293–305. doi: 10.1016/j.ajpath.2013.06.026
126. Juarez JC, Betancourt O Jr., Pirie-Shepherd SR, Guan X, Price ML, Shaw DE, et al. Copper binding by tetrathiomolybdate attenuates angiogenesis and tumor cell proliferation through the inhibition of superoxide dismutase 1. Clin Cancer Res (2006) 12(16):4974–82. doi: 10.1158/1078-0432.Ccr-06-0171
127. MacDonagh L, Gallagher MF, Ffrench B, Gasch C, Breen E, Gray SG, et al. Targeting the cancer stem cell marker, aldehyde dehydrogenase 1, to circumvent cisplatin resistance in nsclc. Oncotarget (2017) 8(42):72544–63. doi: 10.18632/oncotarget.19881
128. Ying H, Qin A, Cheng TS, Pavlos NJ, Rea S, Dai K, et al. Disulfiram attenuates osteoclast differentiation in vitro: a potential antiresorptive agent. PLoS One (2015) 10(4):e0125696. doi: 10.1371/journal.pone.0125696
129. Daniel KG, Chen D, Orlu S, Cui QC, Miller FR, Dou QP. Clioquinol and pyrrolidine dithiocarbamate complex with copper to form proteasome inhibitors and apoptosis inducers in human breast cancer cells. Breast Cancer Res (2005) 7(6):R897–908. doi: 10.1186/bcr1322
130. Peng F, Liu J, Wu JS, Lu X, Muzik O. Mouse extrahepatic hepatoma detected on micropet using copper (Ii)-64 chloride uptake mediated by endogenous mouse copper transporter 1. Mol Imaging Biol (2005) 7(5):325–9. doi: 10.1007/s11307-005-0021-4
Keywords: copper, hepatocellular carcinoma, biology, treatment, molecular mechanism, cancer
Citation: Zhou C, Yang J, Liu T, Jia R, Yang L, Sun P and Zhao W (2023) Copper metabolism and hepatocellular carcinoma: current insights. Front. Oncol. 13:1186659. doi: 10.3389/fonc.2023.1186659
Received: 15 March 2023; Accepted: 21 June 2023;
Published: 05 July 2023.
Edited by:
Hui Liu, The University of Hong Kong, Hong Kong SAR, ChinaReviewed by:
Nan Li, Jinan University, ChinaCopyright © 2023 Zhou, Yang, Liu, Jia, Yang, Sun and Zhao. This is an open-access article distributed under the terms of the Creative Commons Attribution License (CC BY). The use, distribution or reproduction in other forums is permitted, provided the original author(s) and the copyright owner(s) are credited and that the original publication in this journal is cited, in accordance with accepted academic practice. No use, distribution or reproduction is permitted which does not comply with these terms.
*Correspondence: Wenxia Zhao, Wmhhby13ZW54aWFAMTYzLmNvbQ==
†These authors have contributed equally to this work
Disclaimer: All claims expressed in this article are solely those of the authors and do not necessarily represent those of their affiliated organizations, or those of the publisher, the editors and the reviewers. Any product that may be evaluated in this article or claim that may be made by its manufacturer is not guaranteed or endorsed by the publisher.
Research integrity at Frontiers
Learn more about the work of our research integrity team to safeguard the quality of each article we publish.