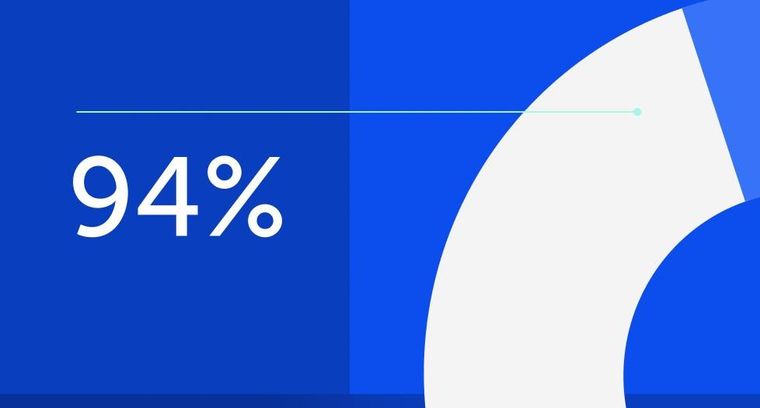
94% of researchers rate our articles as excellent or good
Learn more about the work of our research integrity team to safeguard the quality of each article we publish.
Find out more
REVIEW article
Front. Oncol., 27 April 2023
Sec. Thoracic Oncology
Volume 13 - 2023 | https://doi.org/10.3389/fonc.2023.1153319
This article is part of the Research TopicApplication of Nanotechnology in Diagnosis and/or Therapy of Non-Small Cell Lung CancerView all 5 articles
Non-small cell lung cancer (NSCLC) is a prominent etiology of cancer-related mortality. The heterogeneous nature of this disease impedes its accurate diagnosis and efficacious treatment. Consequently, constant advancements in research are imperative in order to comprehend its intricate nature. In addition to currently available therapies, the utilization of nanotechnology presents an opportunity to enhance the clinical outcomes of NSCLC patients. Notably, the burgeoning knowledge of the interaction between the immune system and cancer itself paves the way for developing novel, emerging immunotherapies for treating NSCLC in the early stages of the disease. It is believed that with the novel engineering avenues of nanomedicine, there is a possibility to overcome the inherent limitations derived from conventional and emerging treatments, such as off-site drug cytotoxicity, drug resistance, and administration methods. Combining nanotechnology with the convergence points of current therapies could open up new avenues for meeting the unmet needs of NSCLC treatment.
Globally, the prevalence and mortality rates of cancer are increasing, with lung cancer constituting the most frequently diagnosed form of the disease, accounting for 11.6% of all reported cases. In the United States, it is anticipated that approximately 236,740 fresh cases of lung cancer will emerge in 2022, culminating in approximately 130,180 fatalities (1, 2). Lung cancer continues to be the primary cause of cancer-related deaths worldwide, responsible for 18.4% of all cancer mortalities. This results in a substantial societal burden and economic loss (2, 3). Approximately 80% of lung cancer fatalities are attributed to smoking. Other significant risk factors for the development of lung cancer include exposure to radon and asbestos, prolonged and cumulative inhalation of air pollution, particularly emissions of polycyclic aromatic hydrocarbons (PAHs), as well as a personal or familial history of lung cancer (4, 5). Lung cancer can be broadly classified into two main types: non-small cell lung cancer (NSCLC) and small cell lung cancer (SCLC), with NSCLC being the more prevalent type (6). The survival rates for both non-small cell lung cancer (NSCLC) and small cell lung cancer (SCLC) in the metastatic stage are notably low, with a mere 4% 5-year survival rate (7, 8). There are three sub-types of NSCLC, which are classified according to the type of lung cells. These are adenocarcinomas, which arise from abnormal lung cells; squamous cell carcinomas, which arise in the bronchi; and large cell carcinomas, which arise peripherally. They all behave in the same way and respond to treatment in the same way (6). Comprehending the prognosis and attributes of lung cancer enables clinicians to provide the most efficacious therapeutic intervention. An array of therapeutic modalities are available, such as surgical intervention, radiotherapy, chemotherapy, targeted therapy, and immunotherapy, which are prescribed based on the stage of the disease, histological subtype, cellular morphology, and clinical status. However, researchers in clinical practice and clinical trials persistently emphasize the crucial aspects of novel therapies, particularly the profound effectiveness and minimal incidence of adverse events (9–11). A myriad of immunotherapeutics has obtained commendable results in the treatment of various cancers, they also meet some daunting challenges such as low water solubility, poor pharmacokinetic profiles, less absorption, less accumulation in the tumor site, less bioactivity after prolonged circulation, and enhanced immune-mediated off-target toxicity (12, 13). As far as we are aware, nanotechnology has the potential to address these issues by utilizing its advantageous characteristics, thus achieving the expected level of clinical benefits (14–17). Based on a comprehensive understanding of the tumor microenvironment, intelligent and stimuli-responsive nanocarriers are being developed to leverage the advantages of acidic pH, hypoxia, enhanced adenosine-triphosphate (ATP) synthesis, altered redox state of cancer cells, and other relevant factors (18). Nanoparticles have been shown to amplify the advantages of cancer immunotherapy by (1) affording protection to antigens and adjuvants; (2) delivering them simultaneously to the antigen-presenting cells (APCs); (3) reprogramming the microenvironment to resume immune surveillance.
A diverse array of nanoscale drug delivery systems (DDS) are presently being investigated, encompassing liposomes, protein-based nanocarriers, inorganic carriers, and polymeric nanoparticles. The utilization of tailored nanoparticles to manage cancer could represent the next stage in enhancing the effectiveness of conventional therapies, which tend to lack specificity (19–21). With the advent of targeted nanosystems (e.g., functionalized magnetic nanoparticles), targeted DDS platform interventions for NSCLC have shown great promise (22). Targeted functionalized magnetic nanoparticles (MNPs) not only improve the precision of chemotherapy but also result in enhanced pharmacological activity and reduced adverse events. Furthermore, they offer desirable advantages as drug delivery vectors (22, 23), including improved drug stability, drug solubility, and high drug loading capacity (24). A number of peptides and polymers have been added to MNPs to facilitate drug delivery or improve their bio-compatibility (25, 26). Researchers have developed a potential cancer treatment method that targets tumor-related blood vessels by targeting nanoparticle-based avenues to surrogates of angiogenesis, such as receptors for vascular endothelial growth factor (VEGF) or integrins (27, 28). Various nanoparticle-based therapies have been explored as potential treatments for metastatic NSCLC, including albumin-bound paclitaxel nanoformulations (Abraxane®). However, the clinical benefits of such therapies are not yet clear, and several challenges remain in translating them into clinical practice (29). Recently, significant progress has been made in the field of cancer nanotherapy, particularly in the exploration of magnetic nanoplatforms for the treatment of NSCLC (29). In this study, the focus will mainly be on the application of nanoparticles in the immunotherapy treatment of NSCLC, taking into account their potential for anti-tumor and anti-progression effects, as well as the potential of nanomedicine-based technology. Furthermore, various translational studies will be explored and discussed regarding the utilization of nanomedicine-based technology and biomarkers in NSCLC immunotherapy.
There is considerable evidence that checkpoint blockade drugs, which primarily inhibit adaptive immunity, are effective and have manageable side effects, including intestinal and pulmonary toxicity and autoimmune sequelae in patients (29). Although new immunotherapeutic approaches aimed at enhancing adaptive responses through immunostimulatory pathways have been met with many concerns regarding their safety profile, these concerns have hindered their successful implementation (29). Nanomedicine offers a promising solution to overcome the limitations of conventional drug delivery methods and maintain the ability to target specific tissues or cell types. It involves the formulation of drugs within carrier materials that are smaller than 100 nanometers. By using nanocarriers, many immunotherapies can be made safer and more effective. The conversion of immunotherapy into nanoparticles comprising of lipids, polymers, or other materials has resulted in changes in systemic exposure, facilitated tumor accumulation, improved innate immune compartments, and modified single-cell signaling (Figure 1). As a result of such approaches, drug development becomes more complex, but these technical difficulties can be easily overcome by numerous clinical-stage companies (30–32). The original aim of using nanomedicine-based drug formulations was to improve the pharmacokinetics and safety profiles of chemotherapy, as well as to increase the accumulation of drugs within tumors (Table 1). The potential for improved efficacy of cancer immunotherapy can be attributed to the ability of nanomaterials to accumulate immune-drugs within cancer cells or around the tumor microenvironment (TME). Furthermore, nanomaterials offer new mechanisms of action for immunotherapeutic agents, including the ability to display ligands on immune cells, modulate the delivery of cell-impermeable compounds intracellularly, and adjust the pattern and timing of drug release or activation.
Figure 1 The mechanisms of nanoparticles medicine in NSCLC immunotherapy. (A) Classes of nanoparticles. Mainly, there are three classes of nanoparticles: polymeric, inorganic, lipid-based nanoparticles. The polymeric nanoparticles are precise control of particle characteristics, payload flexibility and with easy surface modification. The inorganic nanoparticles are unique, electrical, magnetic, optical properties. They can be visualized in size, structure, geometry and they are suited for theranostic applications. The lipid-based nanoparticles are of formulation simplicity, high bioactivity and payload flexibility. (B) Nanoparticles loading ICIs monoclonal antibody in NSCLC treatment. Administration of ICIs leads to the activation of tumor-specific T cells (always CD8+ T cells) activation by blocking PD-1 and CTLA-4 on T cells, and PD-L1 and CD80/86 on APC/tumor cells as well. The use of ICIs along will over-stimulate the immune system and increase the potential of off-target effects which is the source of systematic AEs. Specially, nanoparticles loading ICIs can lead to a high local concentration of ICIs on the tumor region while reducing the off-target effects from ICIs. NSCLC, non-small cell lung cancer; ICIs, immune checkpoint inhibitors; PD-1, programmed cell death 1; CTLA-4, cytotoxic T-lymphocyte–associated antigen; PD-L1, programmed cell death ligand 1; APC, antigen processing cell; AEs, adverse events.
In the case of immunogenic cell death (ICD), ATP and high mobility group protein B1 (HMHB1) known as extracellular molecular patterns are released, along with calreticulin and heat shock protein 90 (HSP90) being exposed to the surface (33). These factors contribute to the recognition of tumor antigens by antigen-presenting cells and their subsequent activation. Nanomedicine formulations can be used to promote ICD because they help to accumulate cytotoxic agents within tumor cells.
Furthermore, the direct association between nanomaterials and external energy sources permits the up-regulation of ICD in response to radiotherapy and magnetic hyperthermia therapy etc (33). The utilization of nanoparticle-based formulations of chemotherapeutic agents that promote immunogenic cell death (ICD) can augment anti-tumor immunity by improving drug delivery to tumor cells. In comparison to free doxorubicin, doxil treatment resulted in increased intratumoral CD8+ T cell infiltration, reduced regulatory T cells (Tregs), and increased CD80 expression by myeloid cells. Currently, the combination of doxil and checkpoint blockade is being investigated in metastatic breast cancer patients. Moreover, a novel approach to improve doxorubicin delivery and enhance ICD involves the covalent linkage of synthetic high-density lipoprotein (HDL) nanodiscs to nanoparticles using an acid-labile linkage (33).
The use of radiation can be regarded as a component of combination immunotherapies (33). Although radiation therapy can cause irreversible DNA damage to cancer cells, it can also trigger the cyclic AMP synthase stimulator of interferon genes (cGAS-STING) pathway, which generates pro-inflammatory cytokines and thereby induces innate and adaptive anti-tumor immunity (33). A high radiation dose could result in attenuated STING activation due to DNA exonuclease TERX1-dependent degradation of cytosolic DNA (33). The immunosuppressive immune cells attacking the tumor can also blunt an initial successful pro-inflammatory radiotherapy (33). In some preclinical studies, radiotherapy has been shown to activate tumor-resident T cells, which have a greater resistance to radiotherapy than circulating T cells (33). The intratumoral administration of nanoparticles post-radiotherapy facilitated the identification of protein antigens that were released from the cancer cells undergoing cell death induced by the nanoparticles. Subsequently, these protein antigens were transported through the lymphatic vessels and were taken up by phagocytic professional antigen-presenting cells located in the draining lymph node (33). By engaging directly with the ICD, radioenhancers can likewise be employed as nanomedicines to improve the effects of ionising radiation (33). Utilizing externally applied alternating magnetic fields to activate paramagnetic iron oxide nanoparticles within the tumor microenvironment (TME), localized hyperthermia can be generated. Preclinical models of glioma, colon cancer, and melanoma have demonstrated the effectiveness of this approach (34, 35), this method encourages CD8+ T cell-mediated immunological responses. Nanoparticle-mediated hyperthermia can induce the production of various pro-inflammatory cytokines within the treated tumor and activated dendritic cells (DCs). In a preclinical study using a dog model, an oral melanoma growing alone was successfully treated with radiation, a virus-like particle adjuvant, and magnetic nanoparticle-induced hyperthermia (36). Numerous nanomaterials have also been employed to increase the effectiveness of combination therapies, either by integrating drugs with synergistic modes of action to produce supra-additive effects, or by encapsulating multiple medications in a single particle to ensure co-delivery to target cells (37, 38). Nanoparticles were prepared using a phospholipid shell containing dihydroartemisinin, a second drug that produces reactive oxygen species (ROS). In preclinical models of colorectal adenocarcinoma, this combination therapy-loaded nanoparticle significantly increased the incidence of ICD in tumors and showed synergistic effects with anti-PD-L1 therapy (39). Phase I clinical trials have just begun for these nanoscale coordination polymer formulations that contain unidentified TME modulating compounds. Additionally, nanoparticles may be made to interact with outside energy sources and carry immunostimulatory agents. When combined with infrared light irradiation, inorganic nanoparticles coated with lipid-anchored photosensitizers enhanced calreticulin exposure on tumor cells and immune cell infiltration in mouse breast tumors (39). In murine models of colon cancer, a single nanoparticle was formulated to encapsulate oxaliplatin, which enabled the combination of photodynamic therapy and chemotherapy to induce ICD, leading to the regression of both irradiated primary tumors and unirradiated secondary tumors (39).
Additionally, T cells and natural killer cells receive costimulatory signals. Many important immunoregulatory receptor interactions occur at cell-cell interfaces. By presenting ligands on the surface of particles at physiologically relevant sizes, nanomedicine offers the potential to mimic such an interface. Compared to equivalent concentrations of soluble anti-CD137 antibodies, agonistic anti-CD137 antibodies conjugated to liposome surfaces were ten times more effective at activating CD8+ T cells (39). Due to the limited capacity of each individual nanoparticle to emit only one signal, as well as its relatively small size which is insufficient to elicit significant receptor cross-linking, the activation of T cells is suboptimal when magnetic nanoparticles of 30 nm diameter, containing immobilized peptide MHC molecules, are coupled with anti-CD28 antibody nanoparticles (39). Activation, proliferation, and the induction of effector functions in T cells are all strongly enhanced when a magnetic field is employed to cause nanoparticle clustering. Additionally, particles can provide several ligands to concurrently target different cell types or bind multiple receptors on target immune cells. Nanoparticles comprising anti-programmed cell death 1 (anti-PD-1) as well as anti-OX40 antibodies improved T-cell activation and therapeutic effectiveness in mice with melanoma and breast cancer when compared to therapy with the same drugs given as a straightforward medication combination (39).
Nanoparticles have been demonstrated to present clusters of death-inducing ligands to circulating tumor cells via the surface of immune cells. Liposomes conjugated with recombinant E-selectin and tumor necrosis factor-related apoptosis-inducing ligand (TRAIL) on their surface enable T cells, monocytes, and natural killer cells to attach to them, allowing for the delivery of clustered TRAIL to circulating tumor cells. When these death ligands are activated, tumor cells are killed (39). TRAIL-presenting nanoparticles have been demonstrated to prevent tumor metastasis and prolong survival in both murine and human xenograft tumor models (40, 41). Lipid nanoparticles have been employed to transport a small molecule inhibitor of macrophage colony-stimulating factor 1 receptor (CSF1R) signaling, in conjunction with signal-regulatory protein-α (SIRPα) blocking antibodies, for the purpose of reprogramming tumor-associated macrophages (TAMs) from an immunosuppressive state to a tumoricidal state in both murine and human xenograft tumor models (42). This study highlights the enhanced effectiveness of the antibody, which can be attributed to its dual role as both a targeting molecule and a direct therapeutic agent by inhibiting SIRPα signaling. Thus, nanomedicine formulations have the potential to modify various immunotherapies targeting cell surface receptors’ signaling to augment their anti-cancer efficacy.
Nanomaterials have been widely employed in medicine to facilitate the direct delivery of drugs into the cytosol. However, hydrophilic and charged substances like nucleic acids, which are taken up by cells via endocytosis, often get trapped in the endolysosomal pathway. As a substitute for natural viruses, nanomaterials have been extensively studied to facilitate the entry of nucleic acids and other drugs into the cytosol. The delivery of substances that activate cytosolic danger sensor proteins, RNA, or DNA encoding immunomodulatory proteins is crucial in the context of cancer immunotherapy. Recently, it has been demonstrated that cyclic dinucleotide agonists of STING may be delivered to the cytoplasm using polymersomes (42). Upon intracellular uptake, polymersomes undergo endosomal acidification, leading to the rupture of endosomal membranes and the release of their STING agonist payload into the cytoplasm. The intratumoral administration of these nanomaterials increased the potency of cyclic dinucleotides by more than 100-fold and demonstrated increased anti-malarial efficacy in melanoma models. Furthermore, a liposomal formulation of cyclic dinucleotides containing pH-responsive lipids was shown to enhance STING activation (42). Alternative approaches to using nanomaterials to activate danger signal pathways involve using synthetic polymers. One recent study has demonstrated the use of pH-responsive block copolymer micelles that can rupture endosomes and directly engage with STING to activate downstream signaling (42). Furthermore, the functionalization of nanoparticles with immunostimulatory agents, such as toll-like receptors (TLRs) agonists, has been shown to be effective. These immunostimulants are absorbed more efficiently by myeloid and antigen-presenting cells compared to free TLR ligands, resulting in heightened immunostimulation (43, 44).
Further control of immunostimulatory or immunosuppressive pathways may be possible through delivery of nucleic acid cargoes encoding therapeutic proteins (45). Oxaliplatin chemotherapy combined with systemic injection of a lipid- and protamine-based nanoparticle expressing a PD-L1 trap protein reduced tumor development in a metastatic colon cancer model (45). Intratumoral administration of lipid nanoparticles that express mRNA for the cytokines IL-23 and IL-36γ, along with the T-cell costimulator OX40L, have led to in situ vaccination and CD8+ T-cell dependent tumor regression in both colon cancer and melanoma models. This approach is currently being investigated in clinical trials (45). Furthermore, the delivery of anti-cancer therapy through surface-engineered nanoparticles targeting tumor cells has the potential to mitigate unexpected effects on off-site targets, while increasing the therapeutic concentration at the site of action, efficacy, and safety for treating lung cancer. By co-delivering therapeutic agents at the optimal time and location using smart nanotools, a simultaneous effect on multiple signaling pathways can be achieved, thus avoiding or combating resistance and preventing undesirable effects. This is the underlying theoretical basis for using designed nanoparticles (46–48). Preclinical and clinical trials have demonstrated significantly improved progression-free survival (PFS) in patients with epidermal growth factor receptor (EGFR)-mutant non-small cell lung cancer (NSCLC) who receive a combination of EGFR tyrosine kinase inhibitors (EGFR-TKIs) and angiogenic therapy. Nonetheless, the use of this combination therapy has been associated with an elevated incidence of grade 3-5 adverse reactions (49–53). Nanomedicine strategies can be utilized to enhance the targeted delivery of combination therapy to the site of action, leading to improved treatment outcomes and reduced incidence of adverse events. Innovative nanomedicine approaches offer the potential to interact with tumor cells through diverse mechanisms, thereby overcoming the limitations associated with conventional antibody-drug conjugates (54, 55). Molecular targeting has also shown great potential in cancer treatment, and their combinations with conventional chemotherapy have improved PFS in a phase III clinical trial with EGFR-mutant NSCLC patients (56).
Nanomedicine has the potential to address the limitations associated with co-delivery of combined therapies by providing a targeted delivery approach for high concentrations of anticancer drugs at the site of action. After absorption from the gastrointestinal tract and distribution throughout the body, EGFR-TKIs interact with the EGFR signaling pathways of many normal cells, affecting proliferation, differentiation, migration, and apoptosis. The use of nanomedicines for targeted drug delivery could help resolve these issues by (1) improving the pharmacokinetic profile of the drug, (2) enhancing tumor targeting potential and localization at the tumor site, (3) minimizing off-site targets and side effects, (4) reducing or reversing multidrug resistance mechanisms, and (5) inhibiting acquired resistance and sensitizing tumor cells to EGFR-TKIs through synergistic action against various anti-tumor targets (57, 58). In addition, the use of nanotechnology as a tool for targeted delivery may offer improvements in the efficacy of anticancer drugs and help to identify beneficial synergistic combinations for treating different subtypes of lung cancer. Nanomedicines can facilitate (1) multivalent targeting and co-delivery of agents to endothelial cells, tumor cells, and the tumor microenvironment; (2) the delivery of large payloads of active substances with diverse physicochemical properties; and (3) the limitation of resistance mechanisms (59). Nanotherapy has the potential to revolutionize the field of clinical lung cancer treatment by reducing the risk of therapeutic failure caused by non-coordinated co-delivery of therapeutic agents and off-target side effects. Despite significant advancements, precise control over the in vivo trajectories of nanosystems is yet to be achieved.
Immunotherapy dosing schedules can have a significant impact on treatment efficacy in preclinical models. Nanoparticles have long been employed to slow down the fast clearance of medicines that might otherwise enter tissues or the bloodstream, and they may have a similar function in immunotherapy (45). To accurately manage the timing of medication release, a nanomedicine formulation can also be made to interact with outside energy sources like heat or light. A TLR9 agonist called CpG-containing DNA complexed with near-infrared light-activatable nanoparticles has been used by researchers to establish the viability of this strategy (45). By controlling the time and place of immunostimulation, as well as the signalling of immunostimulatory biologics, nanotechnology enables more accurate use of current immunotherapy techniques.
The cornerstones of NSCLC treatment in recent times have been surgery, chemotherapy, as well as radiation, but their efficiency has gradually decreased over time and their adverse effects have compelled study into other strategies. Overall survival rates for NSCLC patients have plateaued with the advent of molecularly targeted treatments in conjunction with chemotherapy (60–62). The next generation of medications that will enhance patients’ overall response to NSCLC treatment should be developed as a result of using immunotherapy in conjunction with gene therapy.
As evidenced by the growing number of clinical trials (63, 64), immunotherapies have recently become more focused (Table 2). There are several ways in which the immune system is stimulated by these treatments, and these treatments vary according to the patient’s genetic and epigenetic alterations. Based on histological findings, the immune system was assumed to have little or no capacity to respond to tumors. However, the idea of immunosurveillance demonstrates that a tumor may be identified and addressed at an early stage of development by both the innate and adaptive immune systems (65, 66). Tumors continue to develop defence mechanisms, an immunosuppressive TME, and ways to elude the actions of natural killer (NK) cells, CD8+ T cells, CD4+ T cells, and macrophages despite immunosurveillance. The hypothesis of immunoediting, which has three stages—elimination, equilibrium, and escape—can be used to explain how the immune system and the tumor communicate (66). Immunotherapy’s primary objective is to make tumor-infiltrating immune cells more sensitive to the elimination process. A number of approaches are being investigated to achieve this goal, including the design of vaccines, the modification of immune cells, and the inhibition of mechanisms that allow tumors to evade detection.
Table 2 Key clinical trials investigating efficacy and safety of nanomaterials for lung cancer immunotherapy.
In some preclinical studies, nanoplatforms have been developed for cancer immunotherapy. Moon and his colleagues created a stable and uniform lipoprotein nanodisc, which included phospholipids and apolipoprotein mimetic peptides consisting of 22 amino acids, for delivering neoantigen vaccines to the lymph nodes that drain tumors. These nanodiscs stimulated a potent T cell immune response against tumors, which led to the eradication of established tumors and inhibited the growth of metastatic tumors in murine lungs (67). Nanotechnology and nanomaterials have been utilized to enhance molecularly targeted immunomodulation. This is because molecular targeting drugs have been found to initiate immune responses through various mechanisms, such as aiding in antigen presentation by antigen-presenting cells (APC), promoting T cell infiltration in the tumor region, triggering natural killer (NK) cells, instigating immunogenic cell death (ICD) in tumor cells, and reducing the number of myeloid-derived suppressor cells (MDSCs), regulatory T cells (Treg), and tumor-associated macrophages (TAMs) in the tumor region (68). The A549 tumor xenograft experiment demonstrated a significant antitumor effect, concomitant with the robust induction of innate and adaptive immune responses. The infiltration levels of both CD8+ and CD4+ T cells were augmented, and NK cells were activated, concomitant with the reduction of myeloid-derived suppressor cells (MDSCs) and regulatory T cells expressing the Foxp3 transcription factor (Foxp3+ Tregs), known for their role in immune tolerance (69). Numerous studies are still underway to investigate the potential of immunomodulatory nanomedicine in lung cancer immunotherapy, and we anticipate a surge in their applications in the near future (70–72).
The currently favored treatment modalities being employed are immune checkpoint inhibitors (ICIs) (61, 73). These medications target the immune system’s control mechanisms for lymphocyte activation. The immune checkpoint mechanisms CTLA-4 and PD-1 have undergone substantial research and analysis. T cell activation is inhibited by both mechanisms in a different manner and at different levels (74). By utilizing CTLA-4-dependent mechanisms, the immune system is prevented from overreacting in the early stages of activation. Antigen presentation causes T lymphocytes to become activated, and this is when CTLA-4 is expressed on the cell surface. The receptor engages in interaction with CD80 and CD86, which are expressed on antigen-presenting cells’ membranes (APCs). Through the production of numerous cytokines as a result of this interaction, the TME inhibits and boosts the immune response. Antibodies having a high recognition specificity for CTLA-4 are given as part of a treatment with CTLA-4 inhibitors to stop the protein from binding to CD80/86 ligands[68]. On the other hand, during the effector phase of the innate specific immune response, the PD-1/PD-L1 pathway downregulates the immune response in the late stages. PD-1 is expressed on the membrane of T/B cells and natural killer cells as a result of its activation. In healthy systems, the interaction of this protein with PD-L1 and PD-L2 ligands can lower T cell activation and hence avoid an autoimmune response. But in the TME, tumor cells either produce these ligands on their surfaces or enable other immune cells to express them by activating molecules like IFN-γ. The TME can restrict T cell activation, proliferation, survival, and effector activities in addition to dampening the immune response. For instance, the introduction of anti-PD-1 antibodies blocks the protein’s interaction with PD-L1 and PD-L2, blocking the pathway’s downregulation. The PD-L1 protein is sequestered, which also prevents T-cell activation, and thus prevents the effects of other medications. Due to the possibility of PD-1 engagement with PD-L2, the downregulation of the pathway is limited; thus, the former strategy reduces the immune-related adverse events associated with this therapy. The predominant form of administration for an inhibitor of the PD-1/PD-L1 and CTLA-4 pathways is immunoglobulin G. (IgG). After rigorous clinical studies, several ICIs have been licensed for the treatment of NSCLC (75, 76). ICIs are useful for individuals with advanced cancer or as a second-line therapy, according to clinical trials. Pembrolizumab and atezolizumab have both been administered alone, however chemotherapy is the setting in which they are most frequently utilized (46, 77–79). Clinical data to date reveal that ICIs only assist a small percentage of patients and have limited response rates, despite their powerful antitumor properties (80). Recent research has demonstrated that the administration of these compounds along with chemotherapeutic drugs that are known to influence immune function increases the anti-tumor activity of ICIs. Preclinical investigations have indicated that the amalgamation of ICIs with nanoparticle-mediated chemotherapy results in favorable outcomes in mouse tumor models. A former study described the formulation and development of a drug delivery system comprised of high-density synthetic lipoprotein (sHDL) nanodiscs for chemotherapeutic agents. Through the implementation of this type of platform for delivery, various chemotherapy drugs can be safely and effectively released, and the immune response can be enhanced by inhibiting the PD-1/PD-L1 pathway (67, 81).
ICIs can be used in conjunction with drug-loaded nanoparticles in addition to co-administration with chemotherapy (82). One of the best methods now available is nanoparticle-assisted photodynamic and thermal therapy (82). Photothermal therapy (PTT) is a minimally invasive approach that utilizes nanomaterials to release vibrational energy and ablate cancer cells in localized malignancies. PTT is an alternative to checkpoint inhibitors and has the advantage of allowing tumors to overcome their adaptive immune evasion mechanisms through combined therapy (82). The strategy involves the use of iron oxide (Fe3O4 superparticles) along with magnetic resonance imaging (MRI) particles that have already been approved by the FDA, contained within spheres of the copolymer mPEG-poly(lactide-co-glycolide) (PLGA). Additionally, the immune adjuvant Toll-like receptor 7 (TLR7) is encapsulated with the magnetic nanoparticles to promote an organised immune response against tumors. The effectiveness of this approach has been demonstrated through direct tumor elimination during NIR irradiation, and activation of dendritic cells, by combining three FDA-approved components with PD-L1 ICIs (83).
In recent years, several types of vaccines have been developed into therapeutic vaccines (83). By enhancing humoral and cellular T-cell immune responses, these vaccines were then developed to cure a disease. Through the detection of altered proteins that are expressed in an abnormal manner by tumor cells, the concept of an anti-cancer vaccine was developed. These treat disease by enhancing the humoral and cellular responses of the immune system, primarily T cells. Tumor vaccines are developed by identifying mutated proteins that are abnormally expressed by tumor cells. The immune system identifies these as tumor-associated antigens (TAAs) and categorizes them as expressed fetal antigens (FAs), and further categorizes them as expression of detail antigens (always absent in healthy adults) and overexpression of normal proteins (84–86). The idea behind therapeutic vaccinations is to train the immune system to recognise and react to specific antigens. For the therapy of NSCLC, many vaccine techniques have been examined. These comprise whole-cell vaccines (87–89), protein- and peptide-based vaccines (90, 91), comprise mRNA vaccines (92, 93) and vaccinations based on the whole cells (Table 3).
Along with whole tumor cell vaccinations, one of the earliest and most promising approaches to treating cancer is the use of proteins or peptides. However, peptide vaccines are limited by: (1) the low immunogenicity of cancer antigens alone, which requires the co-administration of an adjuvant to stimulate the immune response (84, 85). (2) Additionally, the possibility of an autoimmune reaction being set off is increased by the absence of proteins that are uniquely expressed in cancer cells. Antigenic proteins contain complicated glycosylation patterns and are challenging to purify, in addition to safety issues. The bench to bedside approach is hampered by this. Utilizing peptides enhances stability and selectivity while lowering the negative immunological reactions brought on by using full proteins. However, it has been demonstrated that a variety of protein vaccines are efficient therapies for NSCLC. A3 (MAGE-A3) is a melanoma-associated antigen that is nearly exclusively expressed on various types of tumor cells. Although these outcomes are not therapeutically applicable, MAGE-A3 treatment in conjunction with immune response-enhancing adjuvants has been shown to provide favorable results (94). Mucinous glycoprotein-1 (MUC1), another well-studied protein linked with tumors, is used as an antigen in the TG4010 vaccination. On the other hand, the use of a 25-amino-acid MUC1 peptide has demonstrated outstanding outcomes (95, 96), but a vaccination based on the whole MUC1 protein has failed to obtain substantial favorable results in numerous clinical trials (94). The issue of peptide stabilization and preservation can be resolved by enclosing peptides within lipid particles, which can also enhance their uptake by antigen-presenting cells (APCs). Furthermore, these vehicles can be decorated with immunopotentiators such as immune cell-targeting adjuvants or ligands (97).
As a result of testing the expression of proteins from injected mRNAs, mRNA vaccines appeared in the early 1990s (97). Initial research on vaccines containing genetic material had mainly focused on DNA. This could be attributed to the higher stability of DNA when compared to RNA. However, the development of drug delivery nanosystems and the safety of mRNA regarding mutagenicity and internalization accessibility have shifted the focus towards mRNA (97, 98). Transfecting cells with mRNA can trigger the immune response in various ways. One of them is by training antigen-presenting cells (APCs) to recognise the encoded antigens and activate humoral and cellular immune responses through the use of mRNA that encodes one or more tumor-associated antigens (99). Due to the drug’s outstanding effectiveness and safety profile, more clinical trials are now being conducted. One of the most promising vaccines now on the market is RNActive® CV9201. It is made up of a combination of five NSCLC-associated antigens that prompt an immune response either after dendritic cells have been collected, transfected, and given to the patient, or after the patient has received mRNA directly (99). Over 65% of patients with NSCLC were able to establish an immunological response to the vaccination, according to findings from a phase Ib clinical study of an mRNA vaccine. Forty-eighty percent of patients had distinct antigen-specific humoral responses, despite the fact that the degree of the reaction elicited varied (99). The mechanism of action of the CV9201 vaccine involves the formation of a complex between mRNA and protamine, which is a cationic protein that can bind to harmful substances and facilitate their internalisation by cells. In vivo studies using this protein-based nanoparticle have shown promising results, inducing activation of the adaptive immune response (99). In spite of this, it is worth mentioning that, since it is autologous, its application to the healthcare system is not affordable. In other studies, EVs such as exosomes were found to be the most promising in the development of cancer vaccines (99). As a result of these vesicles, both mRNA-based antigens can be released directly into the body to induce an immune response, as well as embryonic cells can be co-cultured with antigen-loaded exosomes in vitro in order to mature and then be injected to patients (99). Additionally, to increase the immunogenicity of vaccines, these vesicles are being created via membrane decorating with viral fusion proteins or Toll-like receptor ligands (99).
A liposomal cancer vaccine (L-BLP25) was established by Oncothyreon Canada Inc., where the antigen tecemotide (carcinoma-associated human MUC-1) and an adjuvant 3-O-Deacyl-4’-monophosphoryl lipid A (MPL) were integrated into the lipid bilayer made up of 1,2-dipalmitoyl-sn-glycero-3-phosphocholine (DPPC), 1,2-dimyristoyl-sn-glycero-3-phospho-(1’-rac-glycerol) (DMPG), and cholesterol. Among many global clinical trials, a phase IIb trial (NCT00157209) with patients with patients with stage IIIB or IV NSCLC demonstrated an increment of 4.2 months in median survival in the L-BLP25-administration group compared to the cohort receiving best supportive care only. In a subcluster comprising patients with stage IIIB loco-regional NSCLC, an enhancement of 17.3 months in median survival was observed, where the treatment group showed 49% 3-year survival rate over 27% of best supportive care group (100).
More light should be shed on the tailoring of patient-oriented cancer immunotherapy in concordance with the eventful and changeful dynamics of microenvironment, which will help determine the timing and dosing of the therapeutic schedule. Another phase III trial revealed that the concurrent chemoradiotherapt with liposomal tecemotide vaccine (NCT00409188) improved the survival to 9 months, which hints at the importance of the timing of combinatorial therapy (101).
The creation of novel cancer therapies continues to be hampered by innate and acquired drug resistance (99). Additionally, the short-term effectiveness of traditional therapies like chemotherapy may be compromised. Recent years have seen a rise in interest in gene therapy modification as a method of making tumor cells more drug-responsive. One of the most promising methods is to employ RNA to inhibit the production of certain proteins implicated in tumor resistance. On the basis of tumor abnormalities, silencing RNAs (siRNAs) or long non-coding RNAs (lncRNAs) have been transfected into tumor cells with encouraging results (102, 103). SiRNA administration has been notably investigated as a sensitizing treatment and a gene knockdown technique to reduce the expression of genes associated with cell growth and death. The signaling system, target of rapamycin (mTOR), which controls cell growth and metabolism by inhibiting apoptosis, has been extensively studied (104). The previous studies have revealed that due to their highly positive charge, serene encapsulation in polyplexes allows for high transfer rates. A current nanodrug delivery system exhibits exceptional endosomal escape ability, enabling siRNA delivery to the cytosol (105–107).
Furthermore, to enhance the effectiveness of treatments and prevent resistance mechanisms of tumors, it is imperative to restrict the development and metastatic capabilities of lung cancer while also sensitizing tumor cells. One of the most significant processes in NSCLC is the epithelial-mesenchymal transition (EMT). EMT is the process through which epithelial cells change into mesenchymal cells by losing their adherence and gaining the ability to differentiate and regenerate (108). This process raises the risk of tumor development and metastatic dissemination in cancer patients. Therefore, EMT must be regulated. By attaching to the 3’ untranslated region (UTR) of the target mRNA, microRNA (miRNA), a single-stranded short non-coding RNA, can be used to regulate gene expression (108). This stops the loss of epithelial cell-specific markers and proteins. Conversion can also be thwarted by other kinds of genetic material. In a prior work, siRNA was enclosed in an antibody-conjugated gelatin nanoparticle to block the expression of AXL. They were able to increase the tumor suppressor activity of the p53 pathway, decrease the expression of EMT proteins, and decrease the activity of mTOR.
In addition to inhibiting the expression of certain proteins, repair of the genome has become an increasingly popular treatment option for non-small cell lung cancer (NSCLC). By using CRISPR/Cas9 technology, it is possible to edit genes and open a wide range of possibilities (108). The method primarily involves the use of single-guide RNA-directed Cas9. As a result of the enzyme cleaving the DNA at the point of interest, a modification, deletion, or replacement of the DNA sequence can be accomplished. In light of this, the CRISPR/Cas9 system is widely applicable to the knockout of oncogenes and the study of genes associated with tumor suppression as well as genes associated with resistance.
In summary, advances in nanotechnology have led to a plethora of treatment options for NSCLC. Combining nanomedicine with therapy has resulted in substantial improvements in both clinical benefit and toxicity. The objectives of nanodelivery systems include achieving optimal drug concentration in target cell populations, managing drug release, and improving long-term effects. There exist cutting-edge therapeutic methods such as siRNA, mRNA, and gene editing that have demonstrated effectiveness as anti-cancer techniques. These methods have been seamlessly integrated with nanotechnology and are expected to be made available to patients in the near future due to parallel advancements. Nevertheless, due to a lack of comprehensive understanding of the specific interactions between nanoparticles and biomolecules, designing effective trials is challenging. Moreover, further research is necessary to fully comprehend the issue of tumor nanoparticle permeability caused by insufficient EPR delivery efficiency.
QL had full access to all of the data in the manuscript and take responsibility for the integrity of the data and the accuracy of the data analyses. All authors read and approved the final manuscript. Concept and design: All authors. Acquisition, analysis, or interpretation of the data: All authors. Drafting of the manuscript: LP, QX, SY, YZ, HW, YL, and QL. Critical revision of the manuscript for important intellectual content: LP, QX, LC, YH, JY, KP, and QL. Supervision: LP and QL. All authors contributed to the article.
The authors declare that the research was conducted in the absence of any commercial or financial relationships that could be construed as a potential conflict of interest.
All claims expressed in this article are solely those of the authors and do not necessarily represent those of their affiliated organizations, or those of the publisher, the editors and the reviewers. Any product that may be evaluated in this article, or claim that may be made by its manufacturer, is not guaranteed or endorsed by the publisher.
1. Siegel RL, Miller KD, Wagle NS, Jemal A. Cancer statistics, 2023. CA: A Cancer J Clin (2023) 73:17–48. doi: 10.3322/caac.21763
2. Siegel RL, Miller KD, Fuchs HE, Jemal A. Cancer statistics, 2022. CA: A Cancer J Clin (2022) 72:7–33. doi: 10.3322/caac.21708
3. Bray F, Ferlay J, Soerjomataram I, Siegel RL, Torre LA, Jemal A. Global cancer statistics 2018: GLOBOCAN estimates of incidence and mortality worldwide for 36 cancers in 185 countries. CA: A Cancer J Clin (2018) 68:394–424. doi: 10.3322/caac.21492
4. Thai AA, Solomon BJ, Sequist LV, Gainor JF, Heist RS. Lung cancer. Lancet (2021) 398:535–54. doi: 10.1016/S0140-6736(21)00312-3
5. Herbst RS, Morgensztern D, Boshoff C. The biology and management of non-small cell lung cancer. Nature (2018) 553:446–54. doi: 10.1038/nature25183
6. Molina JR, Yang P, Cassivi SD, Schild SE, Adjei AA. Non-small cell lung cancer: epidemiology, risk factors, treatment, and survivorship. Mayo Clin Proc (2008) 83:584–94. doi: 10.1016/S0025-6196(11)60735-0
7. Boloker G, Wang C, Zhang J. Updated statistics of lung and bronchus cancer in united states (2018). J Thorac Dis (2018) 10:1158–61. doi: 10.21037/jtd.2018.03.15
8. Siegel RL, Miller KD, Jemal A. Cancer statistics, 2018. CA: A Cancer J Clin (2018) 68:7–30. doi: 10.3322/caac.21442
9. Gridelli C, Rossi A, Carbone DP, Guarize J, Karachaliou N, Mok T, et al. Non-small-cell lung cancer. Nat Rev Dis Primers (2015) 1:15009. doi: 10.1038/nrdp.2015.48
10. Beadsmoore CJ, Screaton NJ. Classification, staging and prognosis of lung cancer. Eur J Radiol (2003) 45:8–17. doi: 10.1016/S0720-048X(02)00287-5
11. Scott WJ, Howington J, Feigenberg S, Movsas B, Pisters K. Treatment of non-small cell lung cancer stage I and stage II: ACCP evidence-based clinical practice guidelines (2nd edition). Chest (2007) 132:234s–42s. doi: 10.1378/chest.07-1378
12. Gordon JR, Ma Y, Churchman L, Gordon SA, Dawicki W. Regulatory dendritic cells for immunotherapy in immunologic diseases. Front Immunol (2014) 5:7. doi: 10.3389/fimmu.2014.00007
13. Shen Y, Hao T, Ou S, Hu C, Chen L. Applications and perspectives of nanomaterials in novel vaccine development. Medchemcomm (2018) 9:226–38. doi: 10.1039/C7MD00158D
14. Mukherjee A, Madamsetty VS, Mukherjee S. Emerging trends in immunomodulatory nanomaterials toward cancer therapy. Synthesis Lectures Biomed Eng (2021) 16:i–84. doi: 10.1007/978-3-031-01669-1
15. Mukherjee A, Paul M, Mukherjee S. Recent progress in the theranostics application of nanomedicine in lung cancer. Cancers (Basel) (2019) 11. doi: 10.3390/cancers11050597
16. Madamsetty VS, Paul MK, Mukherjee A, Mukherjee S. Functionalization of nanomaterials and their application in melanoma cancer theranostics. ACS Biomater Sci Eng (2020) 6:167–81. doi: 10.1021/acsbiomaterials.9b01426
17. Mukherjee S, Madamsetty VS, Bhattacharya D, Roy Chowdhury S, Paul MK, Mukherjee A. Recent advancements of nanomedicine in neurodegenerative disorders theranostics. Adv Funct Mater (2020) 30:2003054. doi: 10.1002/adfm.202003054
18. Ovais M, Mukherjee S, Pramanik A, Das D, Mukherjee A, Raza A, et al. Designing stimuli-responsive upconversion nanoparticles that exploit the tumor microenvironment. Adv Mater (2020) 32:2000055. doi: 10.1002/adma.202000055
19. Shi J, Kantoff PW, Wooster R, Farokhzad OC. Cancer nanomedicine: progress, challenges and opportunities. Nat Rev Cancer (2017) 17:20–37. doi: 10.1038/nrc.2016.108
20. Nam J, Son S, Park KS, Zou W, Shea LD, Moon JJ. Cancer nanomedicine for combination cancer immunotherapy. Nat Rev Mater (2019) 4:398–414. doi: 10.1038/s41578-019-0108-1
21. Irvine DJ, Dane EL. Enhancing cancer immunotherapy with nanomedicine. Nat Rev Immunol (2020) 20:321–34. doi: 10.1038/s41577-019-0269-6
22. Lombardo D, Kiselev MA, Caccamo MT. Smart nanoparticles for drug delivery application: development of versatile nanocarrier platforms in biotechnology and nanomedicine. J Nanomater (2019) 2019:3702518. doi: 10.1155/2019/3702518
23. Aslan B, Ozpolat B, Sood AK, Lopez-Berestein G. Nanotechnology in cancer therapy. J Drug Target (2013) 21:904–13. doi: 10.3109/1061186X.2013.837469
24. Ganapathe LS, Mohamed MA, Mohamad Yunus R, Berhanuddin DD. Magnetite (Fe3O4) nanoparticles in biomedical application: from synthesis to surface functionalisation. Magnetochemistry (2020) 6:68. doi: 10.3390/magnetochemistry6040068
25. Liang N, Liu L, Li P, Xu Y, Hou Y, Peng J, et al. Efficient isolation and quantification of circulating tumor cells in non-small cell lung cancer patients using peptide-functionalized magnetic nanoparticles. J Thorac Dis (2020) 12:4262–73. doi: 10.21037/jtd-20-1026A
26. Li R-N, Da X-H, Li X, Lu Y-S, Gu F-F, Liu Y. Functionalized magnetic nanoparticles for drug delivery in tumor therapy*. Chin Phys B (2021) 30:017502. doi: 10.1088/1674-1056/abb3e6
27. Barr MP, O'Byrne KJ, Al-Sarraf N, Gray SG. VEGF-mediated cell survival in non-small-cell lung cancer: implications for epigenetic targeting of VEGF receptors as a therapeutic approach. Epigenomics (2015) 7:897–910. doi: 10.2217/epi.15.51
28. Chen Y, Wang X, Liu T, Zhang DS, Wang Y, Gu H, et al. Highly effective antiangiogenesis via magnetic mesoporous silica-based siRNA vehicle targeting the VEGF gene for orthotopic ovarian cancer therapy. Int J Nanomed (2015) 10:2579–94. doi: 10.2147/ijn.s78774"10.2147/IJN.S78774
29. Yuan DM, Lv YL, Yao YW, Miao XH, Wang Q, Xiao XW, et al. Efficacy and safety of abraxane in treatment of progressive and recurrent non-small cell lung cancer patients: a retrospective clinical study. Thorac Cancer (2012) 3:341–7. doi: 10.1111/j.1759-7714.2012.00113.x
30. Bommareddy PK, Shettigar M, Kaufman HL. Integrating oncolytic viruses in combination cancer immunotherapy. Nat Rev Immunol (2018) 18:498–513. doi: 10.1038/s41577-018-0014-6
31. Neri D. Antibody-cytokine fusions: versatile products for the modulation of anticancer immunity. Cancer Immunol Res (2019) 7:348–54. doi: 10.1158/2326-6066.CIR-18-0622
32. Kureshi R, Bahri M, Spangler JB. Reprogramming immune proteins as therapeutics using molecular engineering. Curr Opin Chem Eng (2018) 19:27–34. doi: 10.1016/j.coche.2017.12.003
33. Krysko DV, Garg AD, Kaczmarek A, Krysko O, Agostinis P, Vandenabeele P. Immunogenic cell death and DAMPs in cancer therapy. Nat Rev Cancer (2012) 12:860–75. doi: 10.1038/nrc3380
34. Toraya-Brown S, Sheen MR, Zhang P, Chen L, Baird JR, Demidenko E, et al. Local hyperthermia treatment of tumors induces CD8(+) T cell-mediated resistance against distal and secondary tumors. Nanomedicine (2014) 10:1273–85. doi: 10.1016/j.nano.2014.01.011
35. Yanase M, Shinkai M, Honda H, Wakabayashi T, Yoshida J, Kobayashi T. Antitumor immunity induction by intracellular hyperthermia using magnetite cationic liposomes. Jpn J Cancer Res (1998) 89:775–82. doi: 10.1111/j.1349-7006.1998.tb03283.x
36. Hoopes PJ, Wagner RJ, Duval K, Kang K, Gladstone DJ, Moodie KL, et al. Treatment of canine oral melanoma with nanotechnology-based immunotherapy and radiation. Mol Pharm (2018) 15:3717–22. doi: 10.1021/acs.molpharmaceut.8b00126
37. Chen Y, Xia R, Huang Y, Zhao W, Li J, Zhang X, et al. An immunostimulatory dual-functional nanocarrier that improves cancer immunochemotherapy. Nat Commun (2016) 7:13443. doi: 10.1038/ncomms13443
38. Park J, Wrzesinski SH, Stern E, Look M, Criscione J, Ragheb R, et al. Combination delivery of TGF-β inhibitor and IL-2 by nanoscale liposomal polymeric gels enhances tumor immunotherapy. Nat Mater (2012) 11:895–905. doi: 10.1038/nmat3355
39. Duan X, Chan C, Han W, Guo N, Weichselbaum RR, Lin W. Immunostimulatory nanomedicines synergize with checkpoint blockade immunotherapy to eradicate colorectal tumors. Nat Commun (2019) 10:1899. doi: 10.1038/s41467-019-09221-x
40. Jyotsana N, Zhang Z, Himmel LE, Yu F, King MR. Minimal dosing of leukocyte targeting TRAIL decreases triple-negative breast cancer metastasis following tumor resection. Sci Adv (2019) 5:eaaw4197. doi: 10.1126/sciadv.aaw4197
41. Nair PM, Flores H, Gogineni A, Marsters S, Lawrence DA, Kelley RF, et al. Enhancing the antitumor efficacy of a cell-surface death ligand by covalent membrane display. Proc Natl Acad Sci (2015) 112:5679–84. doi: 10.1073/pnas.1418962112
42. Kulkarni A, Chandrasekar V, Natarajan SK, Ramesh A, Pandey P, Nirgud J, et al. A designer self-assembled supramolecule amplifies macrophage immune responses against aggressive cancer. Nat BioMed Eng (2018) 2:589–99. doi: 10.1038/s41551-018-0254-6
43. Guan C, Chernyak N, Dominguez D, Cole L, Zhang B, Mirkin CA. RNA-Based immunostimulatory liposomal spherical nucleic acids as potent TLR7/8 modulators. Small (2018) 14:e1803284. doi: 10.1002/smll.201803284
44. Radovic-Moreno AF, Chernyak N, Mader CC, Nallagatla S, Kang RS, Hao L, et al. Immunomodulatory spherical nucleic acids. Proc Natl Acad Sci (2015) 112:3892–7. doi: 10.1073/pnas.1502850112
45. Pastor F, Berraondo P, Etxeberria I, Frederick J, Sahin U, Gilboa E, et al. An RNA toolbox for cancer immunotherapy. Nat Rev Drug Discov (2018) 17:751–67. doi: 10.1038/nrd.2018.132
46. Zhou X, Shi K, Hao Y, Yang C, Zha R, Yi C, et al. Advances in nanotechnology-based delivery systems for EGFR tyrosine kinases inhibitors in cancer therapy. Asian J Pharm Sci (2020) 15:26–41. doi: 10.1016/j.ajps.2019.06.001
47. Gurunathan S, Kang M-H, Qasim M, Kim J-H. Nanoparticle-mediated combination therapy: two-in-One approach for cancer. Int J Mol Sci (2018) 19:3264. doi: 10.3390/ijms19103264
48. Uchibori K, Inase N, Araki M, Kamada M, Sato S, Okuno Y, et al. Brigatinib combined with anti-EGFR antibody overcomes osimertinib resistance in EGFR-mutated non-small-cell lung cancer. Nat Commun (2017) 8:14768. doi: 10.1038/ncomms14768
49. Chen F, Chen N, Yu Y, Cui J. Efficacy and safety of epidermal growth factor receptor (EGFR) inhibitors plus antiangiogenic agents as first-line treatments for patients with advanced EGFR-mutated non-small cell lung cancer: a meta-analysis. Front Oncol (2020) 10. doi: 10.3389/fonc.2020.00904
50. Naumov GN, Nilsson MB, Cascone T, Briggs A, Straume O, Akslen LA, et al. Combined vascular endothelial growth factor receptor and epidermal growth factor receptor (EGFR) blockade inhibits tumor growth in xenograft models of EGFR inhibitor resistance. Clin Cancer Res (2009) 15:3484–94. doi: 10.1158/1078-0432.CCR-08-2904
51. Herbst RS, Ansari R, Bustin F, Flynn P, Hart L, Otterson GA, et al. Efficacy of bevacizumab plus erlotinib versus erlotinib alone in advanced non-small-cell lung cancer after failure of standard first-line chemotherapy (BeTa): a double-blind, placebo-controlled, phase 3 trial. Lancet (2011) 377:1846–54. doi: 10.1016/S0140-6736(11)60545-X
52. Stinchcombe TE, Jänne PA, Wang X, Bertino EM, Weiss J, Bazhenova L, et al. Effect of erlotinib plus bevacizumab vs erlotinib alone on progression-free survival in patients with advanced EGFR-mutant non–small cell lung cancer: a phase 2 randomized clinical trial. JAMA Oncol (2019) 5:1448–55. doi: 10.1001/jamaoncol.2019.1847
53. Saito H, Fukuhara T, Furuya N, Watanabe K, Sugawara S, Iwasawa S, et al. Erlotinib plus bevacizumab versus erlotinib alone in patients with EGFR-positive advanced non-squamous non-small-cell lung cancer (NEJ026): interim analysis of an open-label, randomised, multicentre, phase 3 trial. Lancet Oncol (2019) 20:625–35. doi: 10.1016/S1470-2045(19)30035-X
54. Barok M, Joensuu H, Isola J. Trastuzumab emtansine: mechanisms of action and drug resistance. Breast Cancer Res (2014) 16:209. doi: 10.1186/bcr3621
55. White BE, White MK, Adhvaryu H, Makhoul I, Nima ZA, Biris AS, et al. Nanotechnology approaches to addressing HER2-positive breast cancer. Cancer Nanotechnol (2020) 11:12. doi: 10.1186/s12645-020-00068-2
56. Hosomi Y, Morita S, Sugawara S, Kato T, Fukuhara T, Gemma A, et al. Gefitinib alone versus gefitinib plus chemotherapy for non-Small-Cell lung cancer with mutated epidermal growth factor receptor: NEJ009 study. J Clin Oncol (2020) 38:115–23. doi: 10.1200/JCO.19.01488
57. Zhang T, Wan B, Zhao Y, Li C, Liu H, Lv T, et al. Treatment of uncommon EGFR mutations in non-small cell lung cancer: new evidence and treatment. Trans Lung Cancer Res (2019) 8:302–16. doi: 10.21037/tlcr.2019.04.12
58. Han W, Shi L, Ren L, Zhou L, Li T, Qiao Y, et al. A nanomedicine approach enables co-delivery of cyclosporin a and gefitinib to potentiate the therapeutic efficacy in drug-resistant lung cancer. Signal Transduction Targeted Ther (2018) 3:16. doi: 10.1038/s41392-018-0019-4
59. In GK, Nieva J. Emerging chemotherapy agents in lung cancer: nanoparticles therapeutics for non-small cell lung cancer. Trans Cancer Res (2015) 4:340–55. doi: 10.3978/j.issn.2218-676X.2015.08.05
60. Huang CY, Ju DT, Chang CF, Muralidhar Reddy P, Velmurugan BK. A review on the effects of current chemotherapy drugs and natural agents in treating non-small cell lung cancer. Biomed (Taipei) (2017) 7:23. doi: 10.1051/bmdcn/2017070423
61. Langer CJ. Emerging immunotherapies in the treatment of non–small cell lung cancer (NSCLC): the role of immune checkpoint inhibitors. Am J Clin Oncol (2015) 38:422–30. doi: 10.1097/COC.0000000000000059
62. Davies M. New modalities of cancer treatment for NSCLC: focus on immunotherapy. Cancer Manag Res (2014) 6:63–75. doi: 10.2147/CMAR.S57550
63. Gkolfinopoulos S, Mountzios G. Recent clinical trials of immunotherapy in non-small-cell lung cancer. Immunotherapy (2019) 11:461–6. doi: 10.2217/imt-2019-0021
64. Malhotra J, Jabbour SK, Aisner J. Current state of immunotherapy for non-small cell lung cancer. Transl Lung Cancer Res (2017) 6:196–211. doi: 10.21037/tlcr.2017.03.01
65. Kim R, Emi M, Tanabe K. Cancer immunoediting from immune surveillance to immune escape. Immunology (2007) 121:1–14. doi: 10.1111/j.1365-2567.2007.02587.x
66. Swann JB, Smyth MJ. Immune surveillance of tumors. J Clin Invest (2007) 117:1137–46. doi: 10.1172/JCI31405
67. Kuai R, Ochyl LJ, Bahjat KS, Schwendeman A, Moon JJ. Designer vaccine nanodiscs for personalized cancer immunotherapy. Nat Mater (2017) 16:489–96. doi: 10.1038/nmat4822
68. Li Z, Liu Y, Fang X, Shu Z. Nanomaterials enhance the immunomodulatory effect of molecular targeted therapy. Int J Nanomed (2021) 16:1631–61. doi: 10.2147/IJN.S290346
69. Domvri K, Petanidis S, Anestakis D, Porpodis K, Bai C, Zarogoulidis P, et al. Dual photothermal MDSCs-targeted immunotherapy inhibits lung immunosuppressive metastasis by enhancing T-cell recruitment. Nanoscale (2020) 12:7051–62. doi: 10.1039/D0NR00080A
70. Lahiri A, Maji A, Potdar PD, Singh N, Parikh P, Bisht B, et al. Lung cancer immunotherapy: progress, pitfalls, and promises. Mol Cancer (2023) 22:40. doi: 10.1186/s12943-023-01740-y
71. Lu L, Zhan M, Li X-Y, Zhang H, Dauphars DJ, Jiang J, et al. Clinically approved combination immunotherapy: current status, limitations, and future perspective. Curr Res Immunol (2022) 3:118–27. doi: 10.1016/j.crimmu.2022.05.003
72. Duchemann B, Remon J, Naigeon M, Cassard L, Jouniaux JM, Boselli L, et al. Current and future biomarkers for outcomes with immunotherapy in non-small cell lung cancer. Transl Lung Cancer Res (2021) 10:2937–54. doi: 10.21037/tlcr-20-839
73. Herzberg B, Campo MJ, Gainor JF. Immune checkpoint inhibitors in non-small cell lung cancer. Oncol (2017) 22:81–8. doi: 10.1634/theoncologist.2016-0189
74. Buchbinder EI, Desai A. CTLA-4 and PD-1 pathways: similarities, differences, and implications of their inhibition. Am J Clin Oncol (2016) 39:98. doi: 10.1097/COC.0000000000000239
75. Kazandjian D, Suzman DL, Blumenthal G, Mushti S, He K, Libeg M, et al. FDA Approval summary: nivolumab for the treatment of metastatic non-small cell lung cancer with progression on or after platinum-based chemotherapy. Oncol (2016) 21:634–42. doi: 10.1634/theoncologist.2015-0507
76. Sul J, Blumenthal GM, Jiang X, He K, Keegan P, Pazdur R. FDA Approval summary: pembrolizumab for the treatment of patients with metastatic non-small cell lung cancer whose tumors express programmed death-ligand 1. Oncol (2016) 21:643–50. doi: 10.1634/theoncologist.2015-0498
77. Bakhtiary Z, Barar J, Aghanejad A, Saei AA, Nemati E, Ezzati Nazhad Dolatabadi J, et al. Microparticles containing erlotinib-loaded solid lipid nanoparticles for treatment of non-small cell lung cancer. Drug Dev Ind Pharm (2017) 43:1244–53. doi: 10.1080/03639045.2017.1310223
78. Han W, Shi L, Ren L, Zhou L, Li T, Qiao Y, et al. A nanomedicine approach enables co-delivery of cyclosporin a and gefitinib to potentiate the therapeutic efficacy in drug-resistant lung cancer. Signal Transduction Targeted Ther (2018) 3:1–10. doi: 10.1038/s41392-018-0019-4
79. Gravara LD, Battiloro C, Cantile R, Letizia A, Vitiello F, Montesarchio V, et al. Chemotherapy and/or immune checkpoint inhibitors in NSCLC first-line setting: what is the best approach? Future Med (2020) 9:LMT22. doi: 10.2217/lmt-2019-0018
80. Topalian SL, Hodi FS, Brahmer JR, Gettinger SN, Smith DC, McDermott DF, et al. Safety, activity, and immune correlates of anti–PD-1 antibody in cancer. New Engl J Med (2012) 366:2443–54. doi: 10.1056/NEJMoa1200690
81. Kuai R, Yuan W, Son S, Nam J, Xu Y, Fan Y, et al. Elimination of established tumors with nanodisc-based combination chemoimmunotherapy. Sci Adv (2018) 4:eaao1736. doi: 10.1126/sciadv.aao1736
82. Deng H, Zhang Z. The application of nanotechnology in immune checkpoint blockade for cancer treatment. J Controlled Release (2018) 290:28–45. doi: 10.1016/j.jconrel.2018.09.026
83. Ge R, Liu C, Zhang X, Wang W, Li B, Liu J, et al. Photothermal-activatable Fe3O4 superparticle nanodrug carriers with PD-L1 immune checkpoint blockade for anti-metastatic cancer immunotherapy. ACS Appl Mater Interfaces (2018) 10:20342–55. doi: 10.1021/acsami.8b05876
84. Banchereau J, Palucka K. Cancer vaccines on the move. Nat Rev Clin Oncol (2018) 15:9–10. doi: 10.1038/nrclinonc.2017.149
85. Cuppens K, Vansteenkiste J. Vaccination therapy for non-small-cell lung cancer. Curr Opin Oncol (2014) 26:165–70. doi: 10.1097/CCO.0000000000000052
86. Cortés-Jofré M, Rueda JR, Asenjo-Lobos C, Madrid E, Bonfill Cosp X. Drugs for preventing lung cancer in healthy people. Cochrane Database Syst Rev (2020) 3:Cd002141. doi: 10.1002/14651858.CD002141.pub3
87. Xia L, Schrump DS, Gildersleeve JC. Whole-cell cancer vaccines induce large antibody responses to carbohydrates and glycoproteins. Cell Chem Biol (2016) 23:1515–25. doi: 10.1016/j.chembiol.2016.10.012
88. Ward S, Casey D, Labarthe M-C, Whelan M, Dalgleish A, Pandha H, et al. Immunotherapeutic potential of whole tumor cells. Cancer Immunol Immunother (2002) 51:351–7. doi: 10.1007/s00262-002-0286-2
89. Hirschowitz EA, Mullins A, Prajapati D, Baeker T, Kloecker G, Foody T, et al. Pilot study of 1650-G: a simplified cellular vaccine for lung cancer. J Thorac Oncol (2011) 6:169–73. doi: 10.1097/JTO.0b013e3181fb5c22
90. Peled N, Oton AB, Hirsch FR, Bunn P. MAGE A3 antigen-specific cancer immunotherapeutic. Immunotherapy (2009) 1:19–25. doi: 10.2217/1750743X.1.1.19
91. Wada S, Yada E, Ohtake J, Sasada T. Personalized peptide vaccines for cancer therapy: current progress and state of the art. Expert Rev Precis Med Drug Dev (2017) 2:371–81. doi: 10.1080/23808993.2017.1403286
92. Sebastian M, Von Boehmer L, Zippelius A, Mayer F, Reck M, Atanackovic D, et al. Messenger RNA vaccination in NSCLC: findings from a phase I/IIa clinical trial. J Clin Oncol (2011) 29:2584–4. doi: 10.1200/jco.2011.29.15_suppl.2584
93. Sebastian M, Papachristofilou A, Weiss C, Früh M, Cathomas R, Hilbe W, et al. Phase ib study evaluating a self-adjuvanted mRNA cancer vaccine (RNActive®) combined with local radiation as consolidation and maintenance treatment for patients with stage IV non-small cell lung cancer. BMC Cancer (2014) 14:1–10. doi: 10.1186/1471-2407-14-748
94. Vansteenkiste J, Zielinski M, Linder A, Dahabreh J, Gonzalez EE, Malinowski W, et al. Adjuvant MAGE-A3 immunotherapy in resected non–small-cell lung cancer: phase II randomized study results. J Clin Oncol (2013) 31:2396–403. doi: 10.1200/JCO.2012.43.7103
95. Butts C, Murray N, Maksymiuk A, Goss G, Marshall E, Soulières D, et al. Randomized phase IIB trial of BLP25 liposome vaccine in stage IIIB and IV non–small-cell lung cancer. J Clin Oncol (2005) 23:6674–81. doi: 10.1200/JCO.2005.13.011
96. Sangha R. Butts c. l-BLP25: a peptide vaccine strategy in non–small cell lung cancer. Clin Cancer Res (2007) 13:4652s–4s. doi: 10.1158/1078-0432.CCR-07-0213
97. Midoux P, Pichon C. Lipid-based mRNA vaccine delivery systems. Expert Rev Vaccines (2015) 14:221–34. doi: 10.1586/14760584.2015.986104
98. Fiedler K, Lazzaro S, Lutz J, Rauch S, Heidenreich R. mRNA cancer vaccines. Curr Strategies Cancer Gene Ther (2016) 61–85. doi: 10.1007/978-3-319-42934-2_5
99. Pardi N, Hogan M, Porter F, Weissman D. mRNAvaccines–anewerainvaccinology. NatRev Drug Discov (2017) 17:261–79. doi: 10.1038/nrd.2017.243
100. Butts C, Maksymiuk A, Goss G, Soulières D, Marshall E, Cormier Y, et al. Updated survival analysis in patients with stage IIIB or IV non-small-cell lung cancer receiving BLP25 liposome vaccine (L-BLP25): phase IIB randomized, multicenter, open-label trial. J Cancer Res Clin Oncol (2011) 137:1337–42. doi: 10.1007/s00432-011-1003-3
101. Mitchell P, Thatcher N, Socinski MA, Wasilewska-Tesluk E, Horwood K, Szczesna A, et al. Tecemotide in unresectable stage III non-small-cell lung cancer in the phase III START study: updated overall survival and biomarker analyses. Ann Oncol (2015) 26:1134–42. doi: 10.1093/annonc/mdv104
102. Lu K-h, Li W, Liu X-h, Sun M, Zhang M-l, Wu W-q, et al. Long non-coding RNA MEG3 inhibits NSCLC cells proliferation and induces apoptosis by affecting p53 expression. BMC Cancer (2013) 13:1–11. doi: 10.1186/1471-2407-13-461
103. Zhang Y, Schwerbrock NM, Rogers AB, Kim WY, Huang L. Codelivery of VEGF siRNA and gemcitabine monophosphate in a single nanoparticle formulation for effective treatment of NSCLC. Mol Ther (2013) 21:1559–69. doi: 10.1038/mt.2013.120
104. Fumarola C, Bonelli MA, Petronini PG, Alfieri RR. Targeting PI3K/AKT/mTOR pathway in non small cell lung cancer. Biochem Pharmacol (2014) 90:197–207. doi: 10.1016/j.bcp.2014.05.011
105. Dosta P, Ramos V, Borrós S. Stable and efficient generation of poly(β-amino ester)s for RNAi delivery. Mol Syst Design Eng (2018) 3:677–89. doi: 10.1039/C8ME00006A
106. Dosta P, Segovia N, Cascante A, Ramos V, Borrós S. Surface charge tunability as a powerful strategy to control electrostatic interaction for high efficiency silencing, using tailored oligopeptide-modified poly (beta-amino ester) s (PBAEs). Acta Biomater (2015) 20:82–93. doi: 10.1016/j.actbio.2015.03.029
107. Segovia N, Dosta P, Cascante A, Ramos V, Borrós S. Oligopeptide-terminated poly(β-amino ester)s for highly efficient gene delivery and intracellular localization. Acta Biomater (2014) 10:2147–58. doi: 10.1016/j.actbio.2013.12.054
Keywords: non-small cell lung cancer, immunotherapy, nanomedicine, nanotechnology, combined therapy
Citation: Peng L, Xu Q, Yin S, Zhang Y, Wu H, Liu Y, Chen L, Hu Y, Yuan J, Peng K and Lin Q (2023) The emerging nanomedicine-based technology for non-small cell lung cancer immunotherapy: how far are we from an effective treatment. Front. Oncol. 13:1153319. doi: 10.3389/fonc.2023.1153319
Received: 29 January 2023; Accepted: 11 April 2023;
Published: 27 April 2023.
Edited by:
Yi Zhang, Cedars Sinai Medical Center, United StatesReviewed by:
Yinsong Zhu, University of California, United StatesCopyright © 2023 Peng, Xu, Yin, Zhang, Wu, Liu, Chen, Hu, Yuan, Peng and Lin. This is an open-access article distributed under the terms of the Creative Commons Attribution License (CC BY). The use, distribution or reproduction in other forums is permitted, provided the original author(s) and the copyright owner(s) are credited and that the original publication in this journal is cited, in accordance with accepted academic practice. No use, distribution or reproduction is permitted which does not comply with these terms.
*Correspondence: Qin Lin, bGluNDgyNDc3QDE2My5jb20=
Disclaimer: All claims expressed in this article are solely those of the authors and do not necessarily represent those of their affiliated organizations, or those of the publisher, the editors and the reviewers. Any product that may be evaluated in this article or claim that may be made by its manufacturer is not guaranteed or endorsed by the publisher.
Research integrity at Frontiers
Learn more about the work of our research integrity team to safeguard the quality of each article we publish.