- 1Department of Oncology, First People’s Hospital of Guangyuan, Guangyuan, China
- 2Department of Emergency, First Naval Hospital of Southern Theater Command, Zhanjiang, China
The gut microbiome is involved in the absorption and metabolism of host nutrients and modulates the immune response, affecting the efficacy of immunotherapy for cancer. In patients receiving immunotherapy, appropriate modifications of gut microbiota are thought to improve therapeutic response. Of all the factors that influence the gut microbiota, diet is the most influential and modifiable. Healthy dietary patterns as well as some specific dietary components can help the growth of beneficial microbiota in the gut, thereby protecting against cancers and promoting human health. A growing number of researches have confirmed the positive effects of a diet-gut microbiota approach as an adjuvant therapy for cancer, but controversy remains. Here, we summarize the interactions between diet and gut microbes based on previous studies, and discuss the role of gut microbiota-based dietary strategies in tumor immunotherapy, with the potential mechanisms of actions also intensively discussed.
Introduction
The gut microbiota is a highly complex community, it has been described as a virtual organ owing to the myriad of functions it performs, including the production of bioactive metabolites, regulation of immunity, energy homeostasis and protection against pathogens (1). The crosstalk between the host and the gut microbiota generates an intestinal homeostasis beneficial to the host (2). Dysregulation of homeostasis has been implicated in diseases including cancer (3, 4). Meng et al. (5) summarized that gut microbial dysbiosis contributes to cancer susceptibility through multiple pathways, including causing chronic inflammation to promote tumor progression and accelerate invasion and metastasis, triggering innate and adaptive immune responses involved in tumor formation and generating metabolites to promote malignant transformation. Further studies have shown that the microbiota and its associated metabolites are not only closely associated with carcinogenesis by inducing inflammation and immune dysregulation, but also interfere with the efficacy of anticancer drugs (5).
The influence of gut microbiota on various physiological functions depends on the quantity and quality of microorganisms and its metabolic potential, which is determined by many factors (1). Diet, one of the most important influencers of gut microbiota homeostasis, has a major impact on the gut microbiota (6–9). Data indicate that specific nutrients, especially proteins and insoluble fiber, have profound effects on gut microbial community structure, function, and the secretion of metabolites that modulate immune function and multiple metabolic and inflammatory pathways (10, 11). Indeed, diet is also involved in cancer development and prevention. A recent analysis of two large prospective cohort studies showed a potential link between Western diet (WD), gut microbiota and colorectal carcinogenesis, with WD being associated with a higher incidence of pks+ E. coli-rich cancers (12). The World Cancer Research Fund/American Institute for Cancer Research (WCRF/AICR) report also states that the Western diet increases cancer risk and that cancer risk is higher in people on this type of diet. In contrast, consumption of whole grains, dietary fiber and dairy products reduces cancer risk and is associated with better anti-cancer treatment with better quality of life and increased gut microbial population and diversity (13–16). Diet, as a key determinant of microbiota structure, influences the whole gut microbiota mainly by modulating the abundance of specific species and their individual or collective functions (17, 18). Specific dietary patterns elicit different changes in the gut microbiota. How to use diet to regulate gut microbiota so as to affect the development, metastasis and treatment of tumors is one of the most concerned issues in recent years, which requires a deeper understanding of the interaction mechanism between diet and gut microbiota, but the current research on this aspect is insufficient. In this review, we discussed the interaction between diet and microbiota, how diet and microbiota crosstalk affect the occurrence and progression of tumors, and the application of diet and microbiota crosstalk in cancer immunotherapy.
Dietary patterns influencing gut microbiota composition
Increasing evidence suggests that gut microbiota can influence the susceptibility and etiology of cancer (19). Diet can affect this process, it can promote the growth of bacteria with anti-tumor or carcinogenic properties, and can also be metabolized by intestinal bacteria into some bioactive ingredients to prevent cancer, depending on the nutrient composition (20). Population based studies have shown that diet is the main determinant of microbiota variation among individuals (17, 18). Changes in a large number of nutrients in the diet, including fat, protein and carbohydrate, lead to significant changes in human intestinal microbiota (21, 22). The difference of nutrient composition in different dietary patterns results in different intestinal microbial results. Several popular diets, including western diet (WD), Mediterranean diet (MD), ketogenic diet (KD), have been studied for their ability to modulate the intestinal microbiota (Table 1) (23), thus affecting host metabolism and immunity.
Unhealthy dietary patterns can cause gut microbial imbalance, which is related to many diseases (Figure 1). WD is the most common pattern, a diet rich in sugar, salt and/or fat, has been widely recognized as a major factor in the onset of metabolic disorders and associated pathological conditions (24). It can have deleterious effects on the intestinal barrier, leading to leaky gut, gut microbial dysbiosis, and altered metabolites, further contributing to local inflammation and the presence of Lipopolysaccharide (LPS) in the bloodstream, which can lead to systemic endotoxemia and chronic inflammation (24). The WD has a profound impact on the diversity and populations of gut microbial species (25), which is characterized by a significant decrease in intestinal microbial diversity and an increase in the proportion of harmful bacteria. Animal models have shown that WD patterns result in decreased levels of Bacteroidetes and increased levels of both Proteobacteria and Firmicute(26). A significant increase in the abundance of Proteobacteria, Bilophila wadsworthia, was also reported in another study (27). A recent cross-sectional and longitudinal study also confirmed that long-term western dietary patterns can reduce the diversity and function of intestinal microorganisms (28). With the increase of western dietary time, bacterial strains from Prevotella are replaced by dominant strains from Bacteroides, and the proportion of Bacteroides to Prevotella increases 10 times.
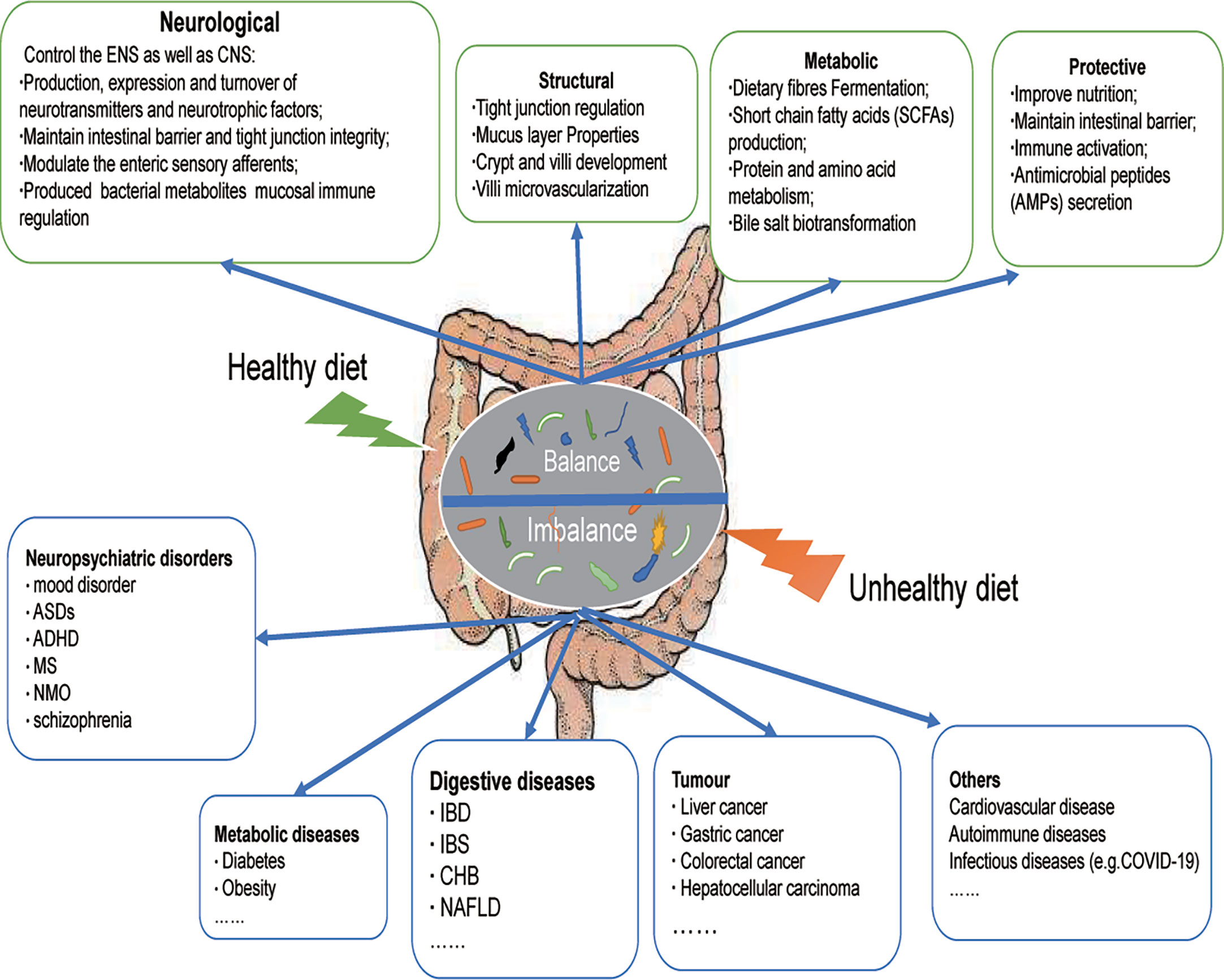
Figure 1 Dietary modulation of the gut microbiota. A healthy diet can maintain the balance of intestinal microecology, and is beneficial for the gut microbiota to exert positive effects on the host (neurological, structural, metabolic, protective). An unhealthy diet disrupts the balance of the host gut microecology and participates in the occurrence and development of many diseases, including neuropsychiatric disorders, metabolic diseases, digestive diseases, tumor, and others. ASDs, Autistic Spectrum Disorder; ADHD, Attention deficit and hyperactivity disorder; MS, Multiple Sclerosis; NMO, neuromyelitis optica; IBD, Inflammatory bowel disease, IBS, Irritable Bowel Syndrome; CHB, Chronic hepatitis B; NAFLD, Nonalcoholic fatty liver disease.
There are studies showing that people on a WD pattern exhibit a considerably higher Firmicutes : Bacteroidetes ratio, less antimicrobial paneth activity, higher concentrations of proinflammatory cytokines, including interferon (IFN)-γ, tumor necrosis factor (TNF)-α, interleukins (IL)-1β and IL-6 (29), and bacterial translocation leading to endotoxemia (30). These changes will lead to an increased risk of many cancers (31) and affect the treatment of tumors (32). For example, a low fiber, high-fat diet can lead to F. nucleatum levels increased significantly (33), which had a strong correlation with colorectal cancer (34). F. nucleatum can activate E-cadherin/β-catenin signal transduction promotes colorectal carcinogenesis and enhances tumor immune evasion by creating a proinflammatory microenvironment conducive to colorectal cancer development through recruitment of immunosuppressive myeloid cells and suppression of natural killer (NK) cell function (35–37). Meanwhile, it is also associated with a lower density of tumor infiltrating CD3+T cells (38), thereby limiting the role of immunotherapy for colorectal cancer, which may be one of the breakthroughs to enhance the efficacy of immunotherapy in the future.
A healthy dietary pattern benefits the stabilization of the intestinal internal environment and increases intestinal microbial diversity (Figure 1), including MD and KD. The MD consists mainly of cereals, nuts, legumes, vegetables, and fruits, with a moderate consumption of poultry and fish (39). The MD is now a popular healthy eating pattern that not only improves overall health, but also reduces the risk of non-communicable diseases (NCDs) (40, 41). MD-based nutritional intervention trials have clarified their possible mechanisms in the host, including the following: reduction of serum lipid levels; prevention of oxidative stress, inflammation and platelet aggregation; regulation of hormones and growth factors associated with cancer development; amino acid restriction-induced inhibition of nutrient-sensing pathways; and regulation of microbial metabolism to promote the normal function of host metabolism(42). Of these, effects on microbes are considered to be key factors in the prevention of NCDs, including cancer.
Studies have shown that the intake of a MD can significantly reduce the incidence of many diseases, such as neurodegenerative diseases (43), cancer (44), obesity (45), type 2 diabetes (46), inflammatory diseases (47) and cardiovascular disease (CVD) (48). This may be mediated by the anti-inflammatory potential of the MD while also being associated with unique gut microbiota alterations caused by the MD (49), namely increased abundances of Bacteroidetes and Clostridia, and decreased abundances of Proteobacteria and Firmicutes (47, 50). This alteration in the gut microbiota favors the production of high levels of short chain fatty acids (SCFAs), including acetate, propionate and butyrate, which can suppress the development of inflammatory, autoimmune, and allergic diseases (51).
A clinical trial (NCT02118857) (50) has shown a significant association between MD and increased levels of fecal SCFAs, Prevotella and some fiber-degrading Firmicutes, and consumption of MD has been associated with a beneficial microbiome related metabolic profile. This microbial signature elicited by MD has also been suggested to have an effect on prevent cancer (41), and interestingly this effect is not restricted to the gut but also affects cancer risk in organs outside the gut by modulating the local microbiota (52, 53). Data from the EPIC study (52) suggest that MD represents the best food model for cancer prevention and is associated with a reduced risk of gastrointestinal cancers, and data from the MOLI-SANI study (54) found that the MD model reduced levels of inflammatory markers such as C-reactive protein (CRP), white blood cells, platelet count and granulocyte/lymphocyte ratio, the latter being associated with poorer cancer prognosis and is an independent predictor of tumour growth, progression and metastatic processes (55). Recently, Shivley et al. (53) showed that MD intake resulted in a 10-fold increase in the abundance of mammary Lactobacilli (negative regulators of breast cancer) compared to the WD, accompanied by increased levels of mammary bile acid metabolites and reduced reactive oxygen metabolites. In conclusion, the MD pattern seems to be associated with features related to the beneficial microbiota inside and outside the gut, and more studies are needed to seek the specific role of its effect on the gut microbiota.
The KD, a high-fat, low carbohydrate diet (56), may promote metabolic health and the prevention of cancer and other NCDs through a variety of mechanisms, including (1) reduced insulin levels; (2) enhanced oxidation of mitochondrial substrates leading to sustained mild elevation of mitochondrial reactive oxygen species production and antioxidant adaptation; and (3) specific antioxidant and anti-inflammatory effects of the ketone body β-hydroxybutyrate as histone deacetylase (HDAC) inhibitors (57). In cancer, lowering circulating glucose levels and inducing ketosis leaves cancer cells lacking energy while normal cells adapt their metabolism to use ketone bodies and survive. In addition, by lowering glucose, levels of insulin and insulin-like growth factor, which are important drivers of cancer cell proliferation, are reduced. In most preclinical tumour models, KDs have been shown to inhibit glycolysis and proliferation of cancer cells(58, 59) and may be used as an adjuvant therapy for cancer (58).
One of the key players in the mechanism of action of the KD may be the gut microbiota. But its effect on the gut microbiota is poorly understood and only limited studies have shown a link between the KD and the gut microbiota. The possible effects of KD on gut microbes have been summarized in reviews by Fan et al. (60) and Paoli et al. (61). However, the ultimate role of the microbiota in mediating the anti-tumour effects of KD has not been investigated to date. Overall, the main effects of the KD on the gut microbiota were as follows: the changes of intestinal microflora α-diversity and richness decreased, beneficial bacteria increased and pathogenic bacteria decreased, metabolic substances such as SCFA increased/decreased, hydrogen sulfide (H2S) increased and lactic acid decreased (61, 62).Indeed, KD have paradoxical effects on the gut microbiota, in a recent study, the fecal microbiological profile of individuals with GLUT1 deficiency syndrome after KD treatment showed significantly increased levels of Desulfovibrio spp, a group of bacteria thought to contribute to the exacerbation of intestinal mucosal inflammation caused by fat intake (63, 64). Therefore, KD may have different efficacy in altering the composition of the gut microbiota.
In addition, the gut microbiome also includes fungi and viruses. The gut viral community includes eukaryotic viruses and phages (65), which can be influenced by diet and have the potential to affect host function by interacting with gut bacteria and/or altering gene expression in the host (66). The study (67) has shown that malnutrition is associated with enterovirus-associated mortality. Diets deficient in nutrients (e.g., vitamin D, zinc) increase susceptibility to enteroviruses (68, 69). However, there is a lack of corresponding studies on how different dietary patterns affect enteroviruses. Similarly, many species of fungi have been detected in the human gut and are influenced by environmental factors (70). Nagpal R, et al. (71) found that a modified Mediterranean ketogenic diet (MMKD) has a broad effect on fungal diversity and modulates mycobacterial biomes related to fungal-bacterial co-regulatory networks. Previous study (72) also showed a direct association between diet and fungi, such as Methanobrevibacter and Candida were positively associated with a diet high in carbohydrates but negatively associated with a diet high in amino acids, proteins and fatty acids. Limited evidence suggests that Candida albicans may interact with immune cells in the host gut through pathways associated with Dectin-1, Toll-like receptor 2 (TLR2), and TLR4, and disrupt fungal-host-microbiota interactions (73). However, the relationship between diet and fungal communities (and function), and the underlying mechanisms remain unclear (74).
Role of the gut microbiota in diet
Gut microbiota play an important role in human metabolism and health. The close interaction between gut microbiota and food intake not only helps to degrade food nutrients, but also can synthesize a variety of nutrients for human use (75).
Recently, a systematic review (76) of the effects of gut microbiota on nutrients and non-nutrients demonstrates that gut microbiota plays a key role in substrate metabolism, especially in the breakdown and transformation of the dietary substrates examined. Gut microbiota is related to energy homeostasis, it plays an important role in energy absorption, storage and consumption from diet. Gut microbiota interacts with the gut environment, breaking down undigested polysaccharides, producing monosaccharides and SCFAs, and allowing the host to recover energy from other undigested dietary matrices. Gut microbes also affect energy balance by regulating the metabolites produced by gene expression (77–81).
The absorption of nutrients mainly occurs in the duodenum and upper jejunum. The undigested dietary components reach the large intestine and are fermented by the intestinal anaerobic microbial community to produce a wide range of metabolites, which reflects the significant biochemical capacity of the microbial community (82, 83). The main fermentation products of healthy adults are gases and organic acids, especially three SCFAs: acetate, propionate and butyrate (84). SCFAs plays an important role in human body. In addition to providing nutrients to enterocytes, SCFAs act as signaling molecules that bind to G protein-coupled receptors (GPR) 41 and GPR43 on the surface of intestinal epithelial and immune cells, regulate the secretion of pro-inflammatory cytokines such as IL-18, and act on the central nervous system to regulate food intake and energy expenditure via glucagon-like peptide 1 (GLP1) and peptide tyrosine-tyrosine (PYY). In addition, SCFAs act as HDAC inhibitors in immune cells and adipocytes, regulating transcription in these cells through chromatin status (22). The production of SCFAs is produced by the fermentation of intestinal specific bacteria using nutrients that cannot be directly absorbed by the host in the diet. The change of bacterial composition will change the distribution of SCFAs in feces.
Early studies have shown that the colonic microbiota has a considerable ability to hydrolyze protein, transforming the dietary protein and endogenous protein from host enzyme, mucin and exfoliated intestinal cells into short peptides, amino acids and derivatives, short chain and branched fatty acids and gases, which is conducive to the rapid absorption of nutrients and the excretion of harmful substances (85). It was confirmed that Bacteroides and Propionibacterium were the main proteolytic bacteria in fecal samples (85). Dai et al. (86) also showed that intestinal bacteria can transform free amino acids into peptides, which contributes a lot to the metabolism and bioavailability of amino acids in mammalian intestine. Gut microorganisms can degrade undigested luminal proteins and peptides in amino acids. Although these amino acids cannot be absorbed by colon epithelium to a large extent, they are precursors of many end products of metabolism, such as SCFAs and organic acids (87), which affect the metabolism of the body. This indicates that intestinal microorganisms can not only absorb and degrade some amino acids, but also synthesize amino acids, suggesting that amino acid exchange between microbial population and host can be carried out in two directions, although it is not clear yet (87).
In addition, studies in the past 40 years have found that intestinal microbiota can synthesize some vitamins, especially vitamin K and B, including biotin, cobalamine, folate, niacin, pantothenic acid, pyridoxine, riboflavin and thiamine (88). And their vitamin synthesis pathway patterns complement each other (89), and there is cross feeding between intestinal microorganisms (90).In short, undigested carbohydrates and proteins in the diet can be fermented by intestinal bacteria to produce SCFAs and gases (CO2, CH4), which are further absorbed or excreted by the colon and account for 10% - 30% of the total food energy supply. Therefore, intestinal flora can enhance human intestinal digestion ability and regulation of energy balance play an important role. Intestinal bacteria rich in enzymes are also necessary for the synthesis of vitamins, and play an important role in the absorption of minerals (91). It can be seen that the reciprocal relationship with intestinal microorganisms may provide a variety of beneficial nutritional functions for the host, including the degradation and detoxification of dyspepsia food, as well as the synthesis of essential nutrients (92).
The role of the gut microbiota in nutrition can be broadly divided into two categories: one is the indirect effect on regulatory pathway, the other is the direct effect on digestion process and nutrition supply (93). Some bacterial species, such as Lactobacillus plantarum or Acetobacter, can promote growth by activating the insulin pathway, and partially compensate for the harmful effects of malnutrition (94, 95). In addition, gut microbes can directly promote the nutrient supply to the host and largely affect the metabolism of lipids and sugars (96). Further studies have shown that gut microbes can increase peptidase activity or provide secondary metabolites, vitamins and amino acids (97–99). On the other hand, the composition of gut microbiota is affected by diet. Different diets seem to have the most profound effect on the quality of the gut microbiota. A healthy diet is rich and comprehensive in nutrients, which helps to promote the diversity and functionality of the gut flora so that it can effectively serve its host (1).
In summary, there is an interaction between nutrients and the gut microbiota in ways that can be direct or indirect, by providing or not providing some of the dietary components required for microbial growth, or by affecting host metabolism and immune responses, or by disrupting the protective function of the gut barrier (Figure 2) (100).
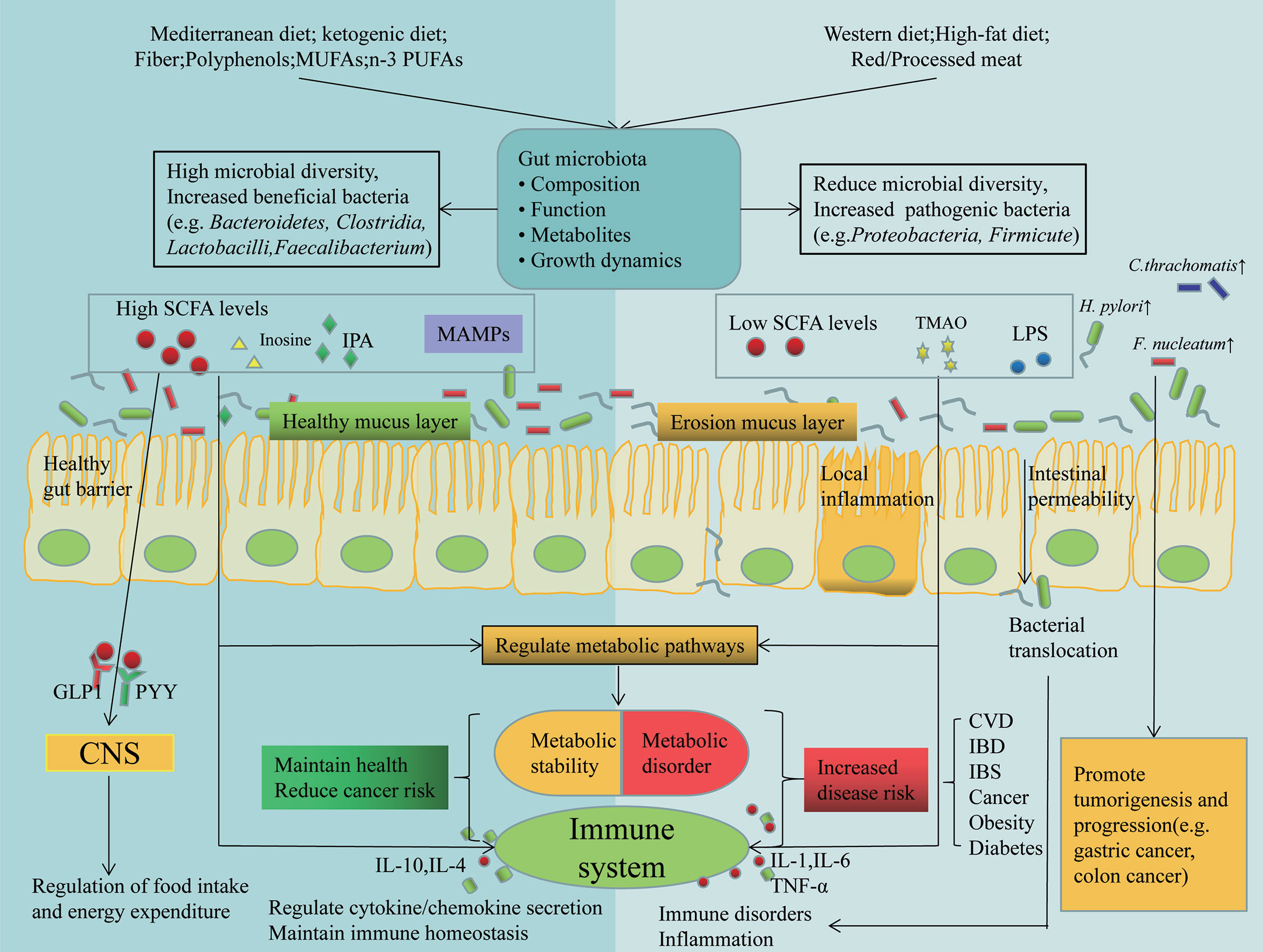
Figure 2 Dietary and gut microbial interactions regulate host immunity and metabolism. Different dietary patterns affect the composition of gut microbes and their metabolites, which are involved in the pathogenesis of many diseases by affecting host metabolism and immunity through different mechanisms. Also alterations in gut microbes in turn affect nutrition. Some tumor associated bacterascites (e.g. H. pylori, F. nucleatum and C. thrachomatis), promote tumorigenesis and progression. GLP1, glucagon-like peptide 1; PYY, peptide tyrosine-tyrosine; IPA, indolepropionic acid; MAMPs, Metabolism-Associated Molecular Patterns; TMAO, Trimetlylamine oxide.
Dietary and gut microbial interactions in cancer immunotherapy
Gut microbes and diet tamper with each other to perform many functions in the host, including synthesis and regulation of bioactive compounds, host metabolic regulation and host immune regulation(22). Interactions between diet and gut microbiota may influence cancer development by altering host metabolism and the immune system (101), as well as shaping cancer immune surveillance and immunotherapeutic responses (102, 103).
Gut microbiota dysbiosis and microbiota-associated metabolites play a key role in tumorigenesis and immunotherapy. SCFAs are major metabolites produced by the fermentation of insoluble dietary fiber by intestinal microorganisms, which serve as energy substrates linking dietary patterns and gut microbiota (104) and play a key role in intestinal microbial immune and metabolic networks affecting the activity of immune and tumor cells (105)(Figure 3), with possible mechanisms including: 1) inhibition of HDAC activity and calcium-regulated phosphatase-mediated activation of nuclear factor of activated T cells 3 (NFATc3) transcription factor, thereby blocking tumor cell proliferation(106) and activating cell cycle inhibitor p21 via GPR43, downregulating inhibitor of apoptosis proteins (IAP) inhibitor, inhibiting cancer cell proliferation, and inducing apoptosis (107). 2) Inducing CD8+T cell ID2 expression via IL-12 signaling thereby directly enhancing the antitumor cytotoxicity of CD8+T, while promoting the expression of effector molecules such as IFN-γ and TNF-α and enhancing the antitumor effects of cytotoxic T lymphocytes (CTL) (108). 3) SCFA provides energy to B cells, memory T cells and effector T cells by regulating metabolic pathways (e.g. glycolysis, TCA cycle and β-oxidation) to enhance the efficacy of immune checkpoint inhibitors (ICI) (109–111). And that changes in nutrient composition can modulate the antitumor effects of SCFA by affecting gut microbes. For example, the WD, on the one hand, slows down mucus growth and increases the permeability of the colonic mucus barrier, leading to a shift in intestinal flora and causing changes in the metabolic and immune system, and on the other hand, the intestinal microbial community changes, with a gradual decrease in SCFA-producing bacteria (e.g., Bifidobacteria) and an increase in Firmicutes phylum (112), resulting in a decrease in SCFA secretion, leading to a disruption of intestinal immune homeostasis and promoting inflammation as well as the expression of cancer-related genes (101). Fiber intake increases the number of bacteria (Bifidobacterium and Lactobacillus) that produce SCFAs (113), a change that increases SCFA production, inhibits HDACi, enhances the anti-inflammatory phenotype in colonic macrophages and dendritic cells (DC) by activating GPR109a, and regulates regulatory T cell (Tregs) function to suppress inflammation and maintain intestinal immune homeostasis (101), thereby reducing cancer risk. Marine omega-3 fatty acid intake resulted in increased abundance of Lactobacillus and Bifidobacterium and decreased F. nucleatum and LPS-producing bacteria (114), contributing to the maintenance of intestinal barrier integrity, reduced oxidative stress and decreased inflammation. In addition, SCFA-producing bacteria, such as Bifidobacterium and Faecalibacterium, promote antitumor immunity and facilitate cancer immunotherapy, making patients more sensitive to immunotherapy responses (115, 116). However, the level of SCFAs seems to be very important in inducing its function. Evidence shows that the anti-inflammatory effect of SCFAs is dose-dependent (117). Qi Hui et al. (118) found that when butyrate concentration is 20 μmol, the levels of IL-6 and IL-1βwere significantly reduced, while the concentration was 2 μmol has no effect. Coutzac et al. (119) also found that Tregs increased at a butyrate concentration of 50 μmol or 100 μmol, but not at a concentration of 10 μmol. The effect of SCFAs on Th1/Th17 cells only occurs at relatively high concentrations (120). When SCFAs increases in vivo for a long time, which is higher than the physiological level, it will cause T cell reaction disorder, produce Th1 and Th17 cells, and thus induce tissue inflammation (121).
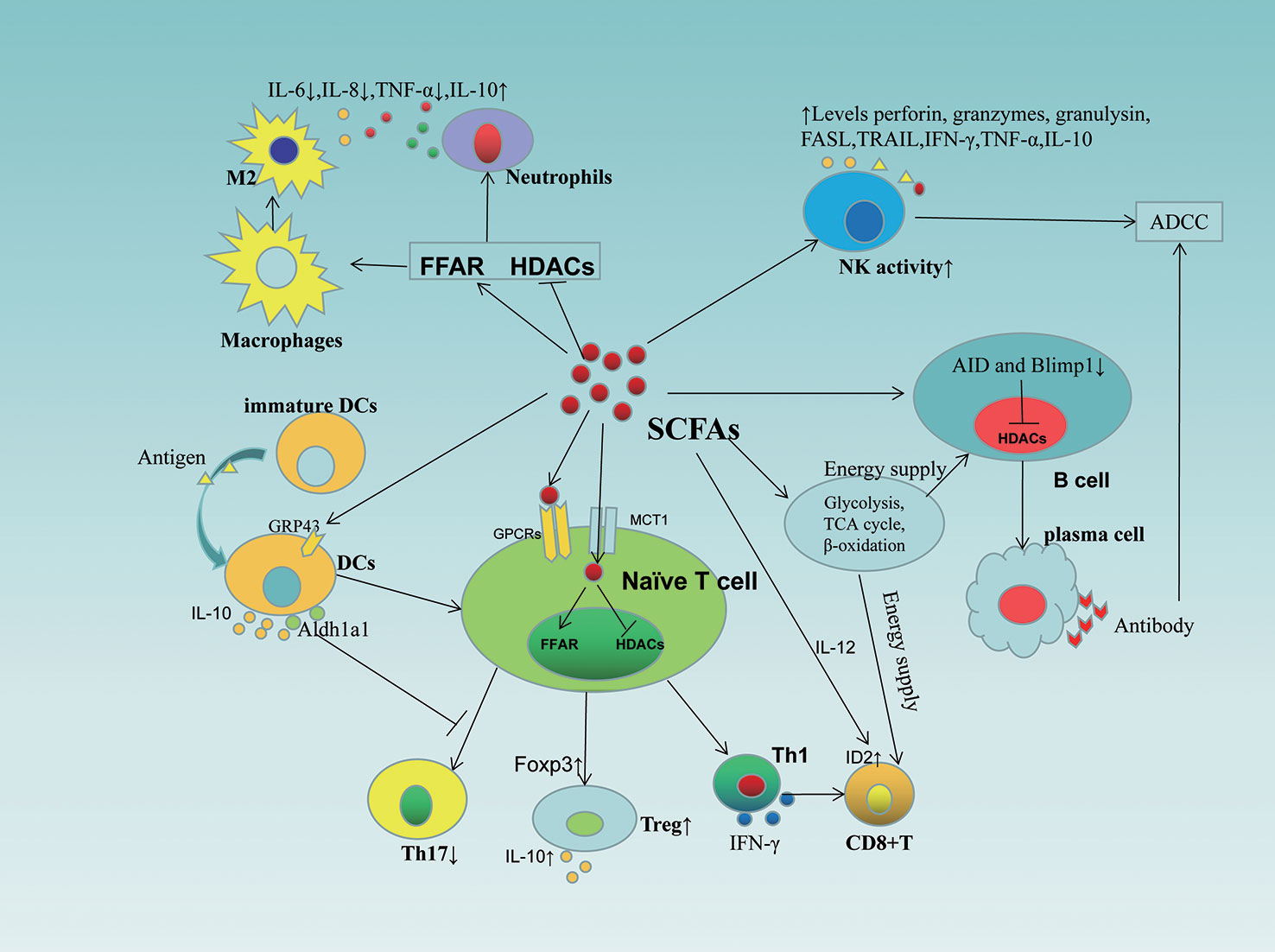
Figure 3 Role of SCFAs in immune cells. SCFAs affect the activity, differentiation and function of immune cells (including T cells, B cells, NK cells, DCs, macrophages, neutrophils) through different mechanisms. SCFAs, short-chain fatty acids; GPCRs, G-protein-coupled receptors; HDACs, inhibit histone deacetylases; FFAR, free fatty acid receptor; ADCC, antibody dependent cell-mediated cytotoxicity; IL, interleukins; MCT1, monocarboxylate transporters 1; FASL, Fas Ligand; TRAIL, tumour necrosis factor-related apoptosis-inducing ligand; NK, natural killer cells; DC, dendritic cell; Aldh1a1, aldehyde dehydrogenases 1 family member a1; AID, activation-induced cytidine deaminase.
Inosine, a purine metabolite of Akkermansia muciniphila and Bifidobacterium pseudolongum, plays an important role in improving the efficacy of ICI (122). On the one hand, inosine enhances the ability of tumor cells to present tumor antigens, thus enabling cytotoxic immune cells to readily recognize and kill tumor cells (105). On the other hand, it stimulates phosphorylation of cAMP response element binding protein (pCREB) through the inosine-A2AR-cAMP-PKA signaling pathway, upregulates IL-12Rβ2 and IFN-γ transcription, and promotes the differentiation and accumulation of Th1 cells in the tumor microenvironment (TME), thus enhancing the potency of ICI (122). Furthermore, when glucose is restricted, inosine serves as an alternative carbon source for CD8+T cells and alleviates the restriction of CD8+T lymphocyte energy metabolism in tumor cells (123).
LPS is a major component of the outer membrane of Gram-negative bacteria and is recognized by the host immune system, and the release of large amounts of LPS causes an uncontrolled immune response (124). Animal experiment (125) showed that LPS-mediated chronic inflammation induced immunosuppressive microenvironment enhanced tumorigenesis. Meanwhile, LPS enhanced the invasive potential of cancer cells by activating the stromal cell-derived factor 1α (SDF-1α)/CXCR4 axis and the onset of epithelial-mesenchymal transition (EMT) through the NF-κB signaling pathway, thus contributing to enhanced cell migration and invasion (126). In addition, the study (127) found that LPS was abundant in CRC tissues and was associated with a low response to anti-PD-L1 mAb treatment. However, LPS has also been shown to be an effective immune booster with potential anti-tumor capabilities. Previous animal trials (128) observed that intravenous LPS completely abrogated immunogenic tumors, but subsequent phase I/II clinical trials conducted were not as effective (129, 130). Further studies have shown that a change in the route of administration (e.g., intratumoral injection) may be more effective in treating cancer and can lead to tumor regression (131). Stronger protective anti-tumor immunity was observed in mice receiving LPS pretreated tumor cells, which was achieved by activating antigen-specific CD8+ T cell responses and reducing MDSCs (132). The use of LPS to activate strong pro-inflammatory responses induced by TLRs to break tumor-associated immune tolerance is also one of the mechanisms to enhance antitumor responses (133). LPS, as an agonist of TLR4, is able to activate anticancer immune responses through the TLR4 pathway, including induction of maturation and migration of DCs, activation of macrophages, B cells, NK cells, and cytotoxic T cells (133).
In addition to SCFA,inosine and LPS, other metabolites affect the antitumor immune response, including anacardic acid and Bile acid (105). The metabolite anacardic acid of Bacteroides caccae induces macrophage activation through phosphorylation of ERK1/2, JNK, P38 kinase and NF-κB, which activates innate immunity (134) and induces the production of neutrophil extracellular traps (NET), promoting the production of tumor-infiltrating immune cells by macrophages, NK cells and T lymphocytes to regulate adaptive and antitumor immunity (135, 136). Bile acid inhibits Th17 cell differentiation (137), attenuates immunostimulatory properties when acting on DCs, thereby inducing Foxp3 expression, upregulating Treg numbers, and promoting immune escape (138), to the detriment of antitumor immunity. However, paradoxically, it has been shown that gut microbiota-mediated bile acid metabolism increases the abundance of CXCR6+NKT cells in the liver and exerts antitumor effects in hepatocellular carcinoma (139). This suggests that there are differences in the roles played by gut microbial metabolites in different scenarios, and continuing to explore the role of these metabolites in different cancers will be one of the future research directions.
Another potential mechanism for this is the reprogramming of TME immunity by the gut microbiota through binding to innate and/or adaptive immune cells (105). For instance, Bifidobacterium bifidum increases DC activation, thereby improving tumour-specific CD8+T-cell responses and restoring anti-PD-L1 therapeutic efficacy (140). Concurrently, CD47-based immunotherapy is promoted in a stimulator of interferon genes (STING) signaling and IFN-I-dependent manner (141). Baeteroides fragilis enhances the antitumor effects of CTLA-4 blockade by triggering DC maturation and stimulating IL-12-dependent Th1 cell immune responses (142). Besides, Baeteroides fragilis induces macrophage phenotypic polarization to M1 and upregulates cellular expression of CD80 and CD86, promoting innate immunity (143). Lactobacillus plantarum effectively increases the expression of natural cytotoxicity receptor (NCR) proteins and promotes NK cell activation to trigger innate immunity (144). These regulatory effects of gut microbes are modulated by dietary factors. It was found that a high-salt diet increased intestinal permeability and intra-tumoral Bifidobacterium localization, which enhanced NK cell activation to induce anti-tumour immunity (145). High-fiber diet-induced microbial alterations derived from STING agonists induced IFN-I signaling via intra-tumour monocytes, shifted mononuclear phagocytes to anti-tumour macrophages (Macs) and triggered monocyte-IFN-I-NK-DC crosstalk, which enhanced anti-tumour responses and ICI efficacy (146).
Earlier studies have shown that the gut microbiota can stimulate anti-tumor immune responses by regulating CD8+T cells (147), Th1 (148) and tumor-associated myeloid cells (149). Bifidobacterium, Enterococcus, faecalibacterium, Ruminococcus and Clostridium promote CD8+ T lymphocyte infiltration in tumor tissues. Firmicutes and Actinobacteria enhance the activation of CD56+CD8+ T cells in peripheral blood of ICI responders (150, 151). Bifidobacterium pseudolongum and Bacteroides fragilis stimulated Th1 immune response to enhance ICI efficacy (122, 142). Faecaliberium increased the proportion of CD4+ T cells and decreased the proportion of Treg cells in peripheral blood (152). In addition, a study has isolated a consortium of 11 bacterial strains from healthy human donor feces that were able to induce an increased ratio of IFNγ+CD8+T cells in a manner dependent on CD103+DCs and major histocompatibility class (MHC) Ia molecules, enhancing the therapeutic effect of immune checkpoint inhibitors (153).
In addition, gut microbes play an important role in enhancing immunogenicity. On the one hand, gut microbiota can enhance ICI responses by acting on UBA6 on the surface of tumor cells, thus directly enhancing the innate immunogenicity of tumor cells. On the other hand, the gut microbiota can promote the efficacy of ICI by providing tumor cross-antigens to indirectly increase the immunogenicity of tumor cells (153–155). The effect of intestinal microorganisms on cancer immunotherapy is under further study (Table 2).
We already know that there is a strong link between diet, gut microbial composition and the outcome of immunotherapy (23). In view of this, many dietary therapies based on gut microbiota have emerged for the treatment of oncology patients. In recent years, attention has been paid to dietary therapies for tumor patients, i.e., the popular “ cancer specific “ diets. One of the mechanisms underlying these dietary patterns is alteration of the gut microbiota (156). Fasting is a dieting pattern that animal models confirm to be beneficial for some types of cancer, and one of its major mechanisms is mediated by gut microbes. Such as every-other-day fasting (EODF) leads to increased levels of Firmicutes and increased SCFAs production, while also reducing the abundance of potentially pathogenic Proteobacteria and concomitantly increasing Akkermansia muciniphila levels (157, 158). A diet low in carbohydrates and rich in fiber, which reduces the growth of pro-inflammatory microorganisms such as Bacteroides acidifaciens, Escherichia coli, Ruminococcus gnavus, and Clostridium cocleatum, increases the growth of anti-inflammatory microorganisms such as Lachnospiraceae (159). Recent studies have shown that higher microbial alpha diversity and abundance of the Ruminococcaceae family and the genus Faecalibacterium in patients with adequate dietary fiber intake enhanced the effect of immunotherapy, with patients having significantly longer Progression-free survival (PFS), and that the effect on treatment efficacy depended on the microbiota, fiber-fermenting Ruminococcaceae family of bacteria may promote fiber-fighting tumor immunity by influencing the pathways of T cell activation and T cell aggregation in tumors, including inducible T cell costimulator (ICOS)-expressing CD8+ and CD4+ T cells (160). The KD can lead to enhanced anticancer immune response by inhibiting lactic acid-mediated tumor immunosuppression and myeloid-derived suppressor cells (MDSC) expression in tumor-bearing mice (161) and has significant efficacy in tumor chemotherapy and radiotherapy (162), but the effect on immunotherapy remains to be determined (163). In addition, some nutrients can influence multiple mechanisms such as cell signaling, apoptosis and immune system regulation, and strongly regulate the composition of the gut microbiota, which plays a key role in maintaining gut homeostasis and is associated with the regulation of host inflammatory and immune responses (164, 165). From this point on, the diet-gut microbiota axis has a potential role in tumorigenesis, immunomodulation and therapy, and perhaps intervention with nutrition and microbes based on standard antineoplastic therapies may have better efficacy.
There are many dietary strategies available to modulate the composition or metabolic/immune activity of the human gut microbiota to enhance the antitumor immune response (166). Supplementation of some micronutrients and vitamins also favors anticancer immune responses, and their effects on immune function have been summarized in detail (REF (167)). Probiotics, prebiotics and polyphenols are the most established (Table 3). Their use is also considered as a potential anti-cancer alternative or adjuvant therapy (168). Polyphenolic compounds, which are widely found in fruits, vegetables and grains, have potential benefits for human health. Dietary polyphenols exert probiotic-like effects that help maintain a healthy gut and reduce inflammation levels by stimulating the growth of beneficial bacteria and inhibiting the development of pathogenic microorganisms (169, 170), with a potential role in the treatment of cancer. For example, resveratrol could enhance the anti-cancer immunity of tumor-bearing mice by promoting the accumulation of effector CD8+T cells and monocyte MDSCs and suppressing the number of tumor-derived CD4+CD25+Tregs and CD8+T cells suppressor granulocyte MDSCs (171, 172). Curcumin contributes to the treatment of inflammatory bowel disease by inhibiting NF-κB activation and inducing mucosal Treg cells and inducing changes in intestinal microbial structure and fecal SCFAs levels (173), which has potential therapeutic effects in some inflammatory tumors. To date, no trials have reported the efficacy of polyphenols on immunotherapy, but polyphenols have immunomodulatory activity and can counteract inflammatory processes in the tumor microenvironment by regulating the production of cytokines and chemokines in turn (174), making them an effective adjunct to future cancer immunotherapy.
The combination of cancer immunotherapy with probiotics is recognized by many researchers because probiotics not only complement immunotherapy, but can improve the safety and reduce the side effects of cancer treatment (175), thanks to the ability of probiotics to alleviate dysbiosis and improve anti-cancer immunity (163). For example, Lactobacillus rhamnosus GG can inhibit the expression of inflammatory proteins NF-κB-p65, COX-2 and TNF-α (176). Lactobacillus plantarum prolongs survival of tumor-bearing mice by enhancing effector CD8+T cell function, CD4+T cell differentiation, and NK cell intratumoral infiltration (177). The combination of Lactobacillus acidophilus NCFM and Bifidobacterium lactis BI-04 resulted in enrichment of butyrate-producing bacteria (e.g., Clostridium and Fasciola) in the colonic mucosa, as well as a reduction in CRC-associated genera, including Fusobacterium and Peptostreptococcus (178).
Animal assays (179) showed that Lactobacillus acidophilus cell lysates enhanced the antitumor activity of anti-CTL antigen-4 blocking antibody (CTLA-4 mAb) in model mice, which was attributed to an increase in CD8+ T cells, an increase in effector memory T cells (CD44+CD8+CD62L+), and a decrease in Treg (CD4+CD25+Foxp3+) and M2 macrophages (F4/80+CD206+) in the tumor microenvironment, along with a partial restoration of CRC-associated dysbiosis. Previous studies have also reported that Bifidobacterium bifidum promotes antitumor responses by enhancing dendritic cell function and promoting T cell-mediated metabolism (140, 180). Supplementation of E. coli Nissle 1917 to tumor-bearing mice also improved the efficacy of galunisertib by enhancing tumor-specific effector T cell infiltration and dendritic cell activation to alleviate the immunosuppressive tumor microenvironment, thereby enhancing the therapeutic effects of galunisertib for tumor growth inhibition and metastasis suppression (181). Recently, Shi et al. (182) reported that oral administration of Akkermansia muciniphila (AKK) to tumor-bearing mice significantly enhanced the therapeutic efficacy of IL-2-based immunotherapy. The mechanism may be through activation of Amuc, an outer membrane protein of toll-like receptor 2 (TLR2) signaling, resulting in effective tumor regression. In addition to the role of probiotics themselves, probiotic derivatives also contribute to tumor immunotherapy. Kawanabe-Matsuda et al. (183) reported that microbial exopolysaccharide produced by Lactobacillus delbrueckii subsp. bulgaricus OLL1073R-1 (EPS-R1) induced an increase in CCR6+ CD8+T cells and production of IFN-γ in Peyer’s patches, accompanied by the expression of a large number of immune response genes, maintained T cell function, and improved the tumor microenvironment, enhancing the antitumor effects of anti-CTLA-4 or anti-PD-1 monoclonal antibodies against CCL20-expressing tumors. However, other studies have yielded conflicting results, with some preclinical models finding reduced frequency of cytotoxic T cells, increased tumorigenesis, and impaired antitumor immunity in the tumor microenvironment of probiotic-treated mice (160, 184). Therefore, the effects of different probiotics on immunity and cancer immunotherapy responses need to be more carefully investigated.
In addition, the recent developed fecal microbiota transplantation (FMT) as an effective way to modulate gut microbiota composition and diversity has been shown to improve the response to ICIs (185). FMT of cancer patients responding to ICIs into sterile or antibiotic-treated mice improved the antitumor effects of PD-1 blockers (186) (29097494). In melanoma patients, gut microbial diversity was richer in ICI-responding patients compared to non-responders. After transplantation of responder colonies in germ-free recipient mice, response to PD-L1 treatment improved, and further analysis revealed higher densities of peripheral CD4+T and CD8+ T cells, upregulation of PD-L1 in the tumor microenvironment, significant enrichment of innate effector cells, and suppressor myeloid cells was reduced (115). In another study in metastatic melanoma, transplantation of feces from responding patients into germ-free mice was shown to improve tumor control, enhance T-cell response, and improve the efficacy of anti-PD-L1 therapy (116). Two recent clinical trials (150, 151) demonstrated that FMT of ICI responder origin altered the gut microbiome of non-responders and reprogrammed TME to overcome resistance to PD-1 blockade. In conclusion, FMT modulates gut microbes in dialogue with the immune system and could be a potential approach for immunotherapy of several cancers.
Conclusions
Diet, as one of the key influences on gut microbes, plays an important role in the composition of gut microbes, depending on the nutritional composition of different dietary patterns. Diet interacts with gut microbes and they are involved in cancer development and treatment by influencing processes such as host metabolism and immunity. There is established evidence that dietary strategies based on the crosstalk between diet and gut microbes have a potentially beneficial role in cancer immunotherapy. However, the information we currently have is insufficient to fully determine how different diets affect the effectiveness of immunotherapy, and more detailed studies are needed to examine how different diets affect certain bacterial species in the gut microbiota to better determine their impact on the effectiveness of immunotherapy (Table 4). Undoubtedly, dietary interventions based on diet-gut microbiota interactions will be an exciting prospect for application.
Author contributions
Conceptualization, XW; writing—original draft preparation, XW and SG; writing—review and editing, XW and SG. All authors contributed to the article and approved the submitted version.
Conflict of interest
The authors declare that the research was conducted in the absence of any commercial or financial relationships that could be construed as a potential conflict of interest.
Publisher’s note
All claims expressed in this article are solely those of the authors and do not necessarily represent those of their affiliated organizations, or those of the publisher, the editors and the reviewers. Any product that may be evaluated in this article, or claim that may be made by its manufacturer, is not guaranteed or endorsed by the publisher.
References
1. Mills S, Stanton C, Lane JA, Smith GJ, Ross RP. Precision nutrition and the microbiome, part I: Current state of the science. Nutrients (2019) 11(4):923. doi: 10.3390/nu11040923
2. Panebianco C, Potenza A, Andriulli A, Pazienza V. Exploring the microbiota to better understand gastrointestinal cancers physiology. Clin Chem Lab Med (2018) 56(9):1400–12. doi: 10.1515/cclm-2017-1163
3. DeGruttola AK, Low D, Mizoguchi A, Mizoguchi E. Current understanding of dysbiosis in disease in human and animal models. Inflammation Bowel Dis (2016) 22(5):1137–50. doi: 10.1097/MIB.0000000000000750
4. Roy S, Trinchieri G. Microbiota: a key orchestrator of cancer therapy. Nat Rev Cancer (2017) 17(5):271–85. doi: 10.1038/nrc.2017.13
5. Meng C, Bai C, Brown TD, Hood LE, Tian Q. Human gut microbiota and gastrointestinal cancer. Genomics Proteomics Bioinf (2018) 16(1):33–49. doi: 10.1016/j.gpb.2017.06.002
6. David LA, Maurice CF, Carmody RN, Gootenberg DB, Button JE, Wolfe BE, et al. Diet rapidly and reproducibly alters the human gut microbiome. Nature (2014) 505(7484):559–63. doi: 10.1038/nature12820
7. Agostoni C, Kim KS. Nutrition and the microbiome 2015. Pediatr Res (2015) 77(1-2):113–4. doi: 10.1038/pr.2014.195
8. Moelling K. Nutrition and the microbiome. Ann N Y Acad Sci (2016) 1372(1):3–8. doi: 10.1111/nyas.13039
9. Grier A, Qiu X, Bandyopadhyay S, Holden-Wiltse J, Kessler HA, Gill AL, et al. Impact of prematurity and nutrition on the developing gut microbiome and preterm infant growth. Microbiome (2017) 5(1):158. doi: 10.1186/s40168-017-0377-0
10. Muegge BD, Kuczynski J, Knights D, Clemente JC, Gonzalez A, Fontana L, et al. Diet drives convergence in gut microbiome functions across mammalian phylogeny and within humans. Science (2011) 332(6032):970–4. doi: 10.1126/science.1198719
11. Clemente JC, Ursell LK, Parfrey LW, Knight R. The impact of the gut microbiota on human health: an integrative view. Cell (2012) 148(6):1258–70. doi: 10.1016/j.cell.2012.01.035
12. Arima K, Zhong R, Ugai T, Zhao M, Haruki K, Akimoto N, et al. Western-Style diet, pks island-carrying escherichia coli, and colorectal cancer: Analyses from two Large prospective cohort studies. Gastroenterology (2022) 163(4):862–74. doi: 10.1053/j.gastro.2022.06.054
13. Montagnese C, Porciello G, Vitale S, Palumbo E, Crispo A, Grimaldi M, et al. Quality of life in women diagnosed with breast cancer after a 12-month treatment of lifestyle modifications. Nutrients (2020) 13(1):136. doi: 10.3390/nu13010136
14. Pellegrini M, Ippolito M, Monge T, Violi R, Cappello P, Ferrocino I, et al. Gut microbiota composition after diet and probiotics in overweight breast cancer survivors: a randomized open-label pilot intervention trial. Nutrition (2020) 74:110749. doi: 10.1016/j.nut.2020.110749
15. Porciello G, Montagnese C, Crispo A, Grimaldi M, Libra M, Vitale S, et al. Mediterranean Diet and quality of life in women treated for breast cancer: A baseline analysis of DEDiCa multicentre trial. PloS One (2020) 15(10):e0239803. doi: 10.1371/journal.pone.0239803
16. Gioxari A, Tzanos D, Kostara C, Papandreou P, Mountzios G, Skouroliakou M. Mediterranean Diet implementation to protect against advanced lung cancer index (ALI) rise: Study design and preliminary results of a randomised controlled trial. Int J Environ Res Public Health (2021) 18(7):3700. doi: 10.3390/ijerph18073700
17. Falony G, Joossens M, Vieira-Silva S, Wang J, Darzi Y, Faust K, et al. Population-level analysis of gut microbiome variation. Science (2016) 352(6285):560–4. doi: 10.1126/science.aad3503
18. Zhernakova A, Kurilshikov A, Bonder MJ, Tigchelaar EF, Schirmer M, Vatanen T, et al. Population-based metagenomics analysis reveals markers for gut microbiome composition and diversity. Science (2016) 352(6285):565–9. doi: 10.1126/science.aad3369
19. Garrett WS. Cancer and the microbiota. Science (2015) 348(6230):80–6. doi: 10.1126/science.aaa4972
20. Tao J, Li S, Gan RY, Zhao CN, Meng X, Li HB. Targeting gut microbiota with dietary components on cancer: Effects and potential mechanisms of action. Crit Rev Food Sci Nutr (2020) 60(6):1025–37. doi: 10.1080/10408398.2018.1555789
21. Singh RK, Chang HW, Yan D, Lee KM, Ucmak D, Wong K, et al. Influence of diet on the gut microbiome and implications for human health. J Transl Med (2017) 15(1):73. doi: 10.1186/s12967-017-1175-y
22. Kolodziejczyk AA, Zheng D, Elinav E. Diet-microbiota interactions and personalized nutrition. Nat Rev Microbiol (2019) 17(12):742–53. doi: 10.1038/s41579-019-0256-8
23. Szczyrek M, Bitkowska P, Chunowski P, Czuchryta P, Krawczyk P, Milanowski J. Diet, microbiome, and cancer immunotherapy-a comprehensive review. Nutrients (2021) 13(7):2217. doi: 10.3390/nu13072217
24. Garcia-Montero C, Fraile-Martinez O, Gomez-Lahoz AM, Pekarek L, Castellanos AJ, Noguerales-Fraguas F, et al. Nutritional components in Western diet versus Mediterranean diet at the gut microbiota-immune system interplay. implications for health and disease. Nutrients (2021) 13(2):699. doi: 10.3390/nu13020699
25. Gentile CL, Weir TL. The gut microbiota at the intersection of diet and human health. Science (2018) 362(6416):776–80. doi: 10.1126/science.aau5812
26. Hildebrandt MA, Hoffmann C, Sherrill-Mix SA, Keilbaugh SA, Hamady M, Chen YY, et al. High-fat diet determines the composition of the murine gut microbiome independently of obesity. Gastroenterology (2009) 137(5):1716–1724 e1711-1712. doi: 10.1053/j.gastro.2009.08.042
27. Devkota S, Wang Y, Musch MW, Leone V, Fehlner-Peach H, Nadimpalli A, et al. Dietary-fat-induced taurocholic acid promotes pathobiont expansion and colitis in Il10-/- mice. Nature (2012) 487(7405):104–8. doi: 10.1038/nature11225
28. Vangay P, Johnson AJ, Ward TL, Al-Ghalith GA, Shields-Cutler RR, Hillmann BM, et al. US Immigration westernizes the human gut microbiome. Cell (2018) 175(4):962–972 e910. doi: 10.1016/j.cell.2018.10.029
29. Guo X, Li J, Tang R, Zhang G, Zeng H, Wood RJ, et al. High fat diet alters gut microbiota and the expression of paneth cell-antimicrobial peptides preceding changes of circulating inflammatory cytokines. Mediators Inflammation (2017) 2017:9474896. doi: 10.1155/2017/9474896
30. Araujo JR, Tomas J, Brenner C, Sansonetti PJ. Impact of high-fat diet on the intestinal microbiota and small intestinal physiology before and after the onset of obesity. Biochimie (2017) 141:97–106. doi: 10.1016/j.biochi.2017.05.019
31. Steck SE, Murphy EA. Dietary patterns and cancer risk. Nat Rev Cancer (2020) 20(2):125–38. doi: 10.1038/s41568-019-0227-4
32. Plotti F, Terranova C, Luvero D, Bartolone M, Messina G, Feole L, et al. Diet and chemotherapy: The effects of fasting and ketogenic diet on cancer treatment. Chemotherapy (2020) 65(3-4):77–84. doi: 10.1159/000510839
33. O'Keefe SJ, Li JV, Lahti L, Ou J, Carbonero F, Mohammed K, et al. Fat, fibre and cancer risk in African americans and rural africans. Nat Commun (2015) 6:6342. doi: 10.1038/ncomms7342
34. Mehta RS, Nishihara R, Cao Y, Song M, Mima K, Qian ZR, et al. Association of dietary patterns with risk of colorectal cancer subtypes classified by fusobacterium nucleatum in tumor tissue. JAMA Oncol (2017) 3(7):921–7. doi: 10.1001/jamaoncol.2016.6374
35. Kostic AD, Chun E, Robertson L, Glickman JN, Gallini CA, Michaud M, et al. Fusobacterium nucleatum potentiates intestinal tumorigenesis and modulates the tumor-immune microenvironment. Cell Host Microbe (2013) 14(2):207–15. doi: 10.1016/j.chom.2013.07.007
36. Rubinstein MR, Wang X, Liu W, Hao Y, Cai G, Han YW. Fusobacterium nucleatum promotes colorectal carcinogenesis by modulating e-cadherin/beta-catenin signaling via its FadA adhesin. Cell Host Microbe (2013) 14(2):195–206. doi: 10.1016/j.chom.2013.07.012
37. Gur C, Ibrahim Y, Isaacson B, Yamin R, Abed J, Gamliel M, et al. Binding of the Fap2 protein of fusobacterium nucleatum to human inhibitory receptor TIGIT protects tumors from immune cell attack. Immunity (2015) 42(2):344–55. doi: 10.1016/j.immuni.2015.01.010
38. Mima K, Sukawa Y, Nishihara R, Qian ZR, Yamauchi M, Inamura K, et al. Fusobacterium nucleatum and T cells in colorectal carcinoma. JAMA Oncol (2015) 1(5):653–61. doi: 10.1001/jamaoncol.2015.1377
39. Estruch R, Ros E, Salas-Salvado J, Covas MI, Corella D, Aros F, et al. Primary prevention of cardiovascular disease with a Mediterranean diet supplemented with extra-virgin olive oil or nuts. N Engl J Med (2018) 378(25):e34. doi: 10.1056/NEJMoa1800389
40. Del Chierico F, Vernocchi P, Dallapiccola B, Putignani L. Mediterranean Diet and health: food effects on gut microbiota and disease control. Int J Mol Sci (2014) 15(7):11678–99. doi: 10.3390/ijms150711678
41. Ostan R, Lanzarini C, Pini E, Scurti M, Vianello D, Bertarelli C, et al. Inflammaging and cancer: a challenge for the Mediterranean diet. Nutrients (2015) 7(4):2589–621. doi: 10.3390/nu7042589
42. Tosti V, Bertozzi B, Fontana L. Health benefits of the Mediterranean diet: Metabolic and molecular mechanisms. J Gerontol A Biol Sci Med Sci (2018) 73(3):318–26. doi: 10.1093/gerona/glx227
43. Karstens AJ, Tussing-Humphreys L, Zhan L, Rajendran N, Cohen J, Dion C, et al. Associations of the Mediterranean diet with cognitive and neuroimaging phenotypes of dementia in healthy older adults. Am J Clin Nutr (2019) 109(2):361–8. doi: 10.1093/ajcn/nqy275
44. Schwingshackl L, Schwedhelm C, Galbete C, Hoffmann G. Adherence to Mediterranean diet and risk of cancer: An updated systematic review and meta-analysis. Nutrients (2017) 9(10):1063. doi: 10.3390/nu9101063
45. Lopez-Legarrea P, Fuller NR, Zulet MA, Martinez JA, Caterson ID. The influence of Mediterranean, carbohydrate and high protein diets on gut microbiota composition in the treatment of obesity and associated inflammatory state. Asia Pac J Clin Nutr (2014) 23(3):360–8. doi: 10.6133/apjcn.2014.23.3.16
46. Salas-Salvado J, Bullo M, Babio N, Martinez-Gonzalez MA, Ibarrola-Jurado N, Basora J, et al. Reduction in the incidence of type 2 diabetes with the Mediterranean diet: results of the PREDIMED-reus nutrition intervention randomized trial. Diabetes Care (2011) 34(1):14–9. doi: 10.2337/dc10-1288
47. Marlow G, Ellett S, Ferguson IR, Zhu S, Karunasinghe N, Jesuthasan AC, et al. Transcriptomics to study the effect of a Mediterranean-inspired diet on inflammation in crohn's disease patients. Hum Genomics (2013) 7:24. doi: 10.1186/1479-7364-7-24
48. Estruch R, Ros E, Salas-Salvado J, Covas MI, Corella D, Aros F, et al. Primary prevention of cardiovascular disease with a Mediterranean diet. N Engl J Med (2013) 368(14):1279–90. doi: 10.1056/NEJMoa1200303
49. Mitsou EK, Kakali A, Antonopoulou S, Mountzouris KC, Yannakoulia M, Panagiotakos DB, et al. Adherence to the Mediterranean diet is associated with the gut microbiota pattern and gastrointestinal characteristics in an adult population. Br J Nutr (2017) 117(12):1645–55. doi: 10.1017/S0007114517001593
50. De Filippis F, Pellegrini N, Vannini L, Jeffery IB, La Storia A, Laghi L, et al. High-level adherence to a Mediterranean diet beneficially impacts the gut microbiota and associated metabolome. Gut (2016) 65(11):1812–21. doi: 10.1136/gutjnl-2015-309957
51. Thorburn AN, Macia L, Mackay CR. Diet, metabolites, and "western-lifestyle" inflammatory diseases. Immunity (2014) 40(6):833–42. doi: 10.1016/j.immuni.2014.05.014
52. Bamia C, Lagiou P, Buckland G, Grioni S, Agnoli C, Taylor AJ, et al. Mediterranean Diet and colorectal cancer risk: results from a European cohort. Eur J Epidemiol (2013) 28(4):317–28. doi: 10.1007/s10654-013-9795-x
53. Shively CA, Register TC, Appt SE, Clarkson TB, Uberseder B, Clear KYJ, et al. Consumption of Mediterranean versus Western diet leads to distinct mammary gland microbiome populations. Cell Rep (2018) 25(1):47–56 e43. doi: 10.1016/j.celrep.2018.08.078
54. Bonaccio M, Pounis G, Cerletti C, Donati MB, Iacoviello L, de Gaetano G, et al. Mediterranean Diet, dietary polyphenols and low grade inflammation: results from the MOLI-SANI study. Br J Clin Pharmacol (2017) 83(1):107–13. doi: 10.1111/bcp.12924
55. Reuter S, Gupta SC, Chaturvedi MM, Aggarwal BB. Oxidative stress, inflammation, and cancer: how are they linked? Free Radic Biol Med (2010) 49(11):1603–16. doi: 10.1016/j.freeradbiomed.2010.09.006
56. Baranano KW, Hartman AL. The ketogenic diet: uses in epilepsy and other neurologic illnesses. Curr Treat Options Neurol (2008) 10(6):410–9. doi: 10.1007/s11940-008-0043-8
57. Miller VJ, Villamena FA, Volek JS. Nutritional ketosis and mitohormesis: Potential implications for mitochondrial function and human health. J Nutr Metab (2018) 2018:5157645. doi: 10.1155/2018/5157645
58. Klement RJ. Beneficial effects of ketogenic diets for cancer patients: a realist review with focus on evidence and confirmation. Med Oncol (2017) 34(8):132. doi: 10.1007/s12032-017-0991-5
59. Weber DD, Aminazdeh-Gohari S, Kofler B. Ketogenic diet in cancer therapy. Aging (Albany NY) (2018) 10(2):164–5. doi: 10.18632/aging.101382
60. Fan Y, Wang H, Liu X, Zhang J, Liu G. Crosstalk between the ketogenic diet and epilepsy: From the perspective of gut microbiota. Mediators Inflamm 2019 (2019) 8373060. doi: 10.1155/2019/8373060
61. Paoli A, Mancin L, Bianco A, Thomas E, Mota JF, Piccini F. Ketogenic diet and microbiota: Friends or enemies? Genes (Basel) (2019) 10(7):534. doi: 10.3390/genes10070534
62. Xie G, Zhou Q, Qiu CZ, Dai WK, Wang HP, Li YH, et al. Ketogenic diet poses a significant effect on imbalanced gut microbiota in infants with refractory epilepsy. World J Gastroenterol (2017) 23(33):6164–71. doi: 10.3748/wjg.v23.i33.6164
63. Ramm-Pettersen A, Nakken KO, Haavardsholm KC, Selmer KK. GLUT1-deficiency syndrome: Report of a four-generation Norwegian family with a mild phenotype. Epilepsy Behav (2017) 70(Pt A):1–4. doi: 10.1016/j.yebeh.2017.02.016
64. Tagliabue A, Ferraris C, Uggeri F, Trentani C, Bertoli S, de Giorgis V, et al. Short-term impact of a classical ketogenic diet on gut microbiota in GLUT1 deficiency syndrome: A 3-month prospective observational study. Clin Nutr ESPEN (2017) 17:33–7. doi: 10.1016/j.clnesp.2016.11.003
65. Carding SR, Davis N, Hoyles L. Review article: the human intestinal virome in health and disease. Aliment Pharmacol Ther (2017) 46(9):800–15. doi: 10.1111/apt.14280
66. Mihindukulasuriya KA, Mars RAT, Johnson AJ, Ward T, Priya S, Lekatz HR, et al. Multi-omics analyses show disease, diet, and transcriptome interactions with the virome. Gastroenterology (2021) 161(4):1194–1207 e1198. doi: 10.1053/j.gastro.2021.06.077
67. Tickell KD, Sharmin R, Deichsel EL, Lamberti LM, Walson JL, Faruque ASG, et al. The effect of acute malnutrition on enteric pathogens, moderate-to-severe diarrhoea, and associated mortality in the global enteric multicenter study cohort: a post-hoc analysis. Lancet Glob Health (2020) 8(2):e215–24. doi: 10.1016/S2214-109X(19)30498-X
68. Bucak IH, Ozturk AB, Almis H, Cevik MO, Tekin M, Konca C, et al. Is there a relationship between low vitamin d and rotaviral diarrhea? Pediatr Int (2016) 58(4):270–3. doi: 10.1111/ped.12809
69. Afolabi OF, Saka AO, Ojuawo A, Biliaminu SA. Serum zinc levels of hospitalized children with acute diarrhea differ by the isolated viruses. Int J Health Sci (Qassim) (2019) 13(5):4–10.
70. Hallen-Adams HE, Suhr MJ. Fungi in the healthy human gastrointestinal tract. Virulence (2017) 8(3):352–8. doi: 10.1080/21505594.2016.1247140
71. Nagpal R, Neth BJ, Wang S, Mishra SP, Craft S, Yadav H. Gut mycobiome and its interaction with diet, gut bacteria and alzheimer's disease markers in subjects with mild cognitive impairment: A pilot study. EBioMedicine (2020) 59:102950. doi: 10.1016/j.ebiom.2020.102950
72. Hoffmann C, Dollive S, Grunberg S, Chen J, Li H, Wu GD, et al. Archaea and fungi of the human gut microbiome: correlations with diet and bacterial residents. PloS One (2013) 8(6):e66019. doi: 10.1371/journal.pone.0066019
73. Li H, Miao MX, Jia CL, Cao YB, Yan TH, Jiang YY, et al. Interactions between candida albicans and the resident microbiota. Front Microbiol (2022) 13:930495. doi: 10.3389/fmicb.2022.930495
74. Li J, Chen D, Yu B, He J, Zheng P, Mao X, et al. Fungi in gastrointestinal tracts of human and mice: from community to functions. Microb Ecol (2018) 75(4):821–9. doi: 10.1007/s00248-017-1105-9
75. Valdes AM, Walter J, Segal E, Spector TD. Role of the gut microbiota in nutrition and health. BMJ (2018) 361:k2179. doi: 10.1136/bmj.k2179
76. Shortt C, Hasselwander O, Meynier A, Nauta A, Fernandez EN, Putz P, et al. Systematic review of the effects of the intestinal microbiota on selected nutrients and non-nutrients. Eur J Nutr (2018) 57(1):25–49. doi: 10.1007/s00394-017-1546-4
77. Achour L, Nancey S, Moussata D, Graber I, Messing B, Flourie B. Faecal bacterial mass and energetic losses in healthy humans and patients with a short bowel syndrome. Eur J Clin Nutr (2007) 61(2):233–8. doi: 10.1038/sj.ejcn.1602496
78. Jumpertz R, Le DS, Turnbaugh PJ, Trinidad C, Bogardus C, Gordon JI, et al. Energy-balance studies reveal associations between gut microbes, caloric load, and nutrient absorption in humans. Am J Clin Nutr (2011) 94(1):58–65. doi: 10.3945/ajcn.110.010132
79. Ziemer CJ, Kerr BJ, Weber TE, Arcidiacono S, Morrison M, Ragauskas A. Effects of feeding fiber-fermenting bacteria to pigs on nutrient digestion, fecal output, and plasma energy metabolites. J Anim Sci (2012) 90(11):4020–7. doi: 10.2527/jas.2012-5193
80. Tims S, Derom C, Jonkers DM, Vlietinck R, Saris WH, Kleerebezem M, et al. Microbiota conservation and BMI signatures in adult monozygotic twins. ISME J (2013) 7(4):707–17. doi: 10.1038/ismej.2012.146
81. Rahat-Rozenbloom S, Fernandes J, Gloor GB, Wolever TM. Evidence for greater production of colonic short-chain fatty acids in overweight than lean humans. Int J Obes (Lond) (2014) 38(12):1525–31. doi: 10.1038/ijo.2014.46
82. Flint HJ, Scott KP, Louis P, Duncan SH. The role of the gut microbiota in nutrition and health. Nat Rev Gastroenterol Hepatol (2012) 9(10):577–89. doi: 10.1038/nrgastro.2012.156
83. Marcobal A, Kashyap PC, Nelson TA, Aronov PA, Donia MS, Spormann A, et al. A metabolomic view of how the human gut microbiota impacts the host metabolome using humanized and gnotobiotic mice. ISME J (2013) 7(10):1933–43. doi: 10.1038/ismej.2013.89
84. Louis P, Hold GL, Flint HJ. The gut microbiota, bacterial metabolites and colorectal cancer. Nat Rev Microbiol (2014) 12(10):661–72. doi: 10.1038/nrmicro3344
85. Macfarlane GT, Cummings JH, Allison C. Protein degradation by human intestinal bacteria. J Gen Microbiol (1986) 132(6):1647–56. doi: 10.1099/00221287-132-6-1647
86. Dai ZL, Zhang J, Wu G, Zhu WY. Utilization of amino acids by bacteria from the pig small intestine. Amino Acids (2010) 39(5):1201–15. doi: 10.1007/s00726-010-0556-9
87. Davila AM, Blachier F, Gotteland M, Andriamihaja M, Benetti PH, Sanz Y, et al. Intestinal luminal nitrogen metabolism: role of the gut microbiota and consequences for the host. Pharmacol Res (2013) 68(1):95–107. doi: 10.1016/j.phrs.2012.11.005
88. Hill MJ. Intestinal flora and endogenous vitamin synthesis. Eur J Cancer Prev (1997) 6(Suppl 1):S43–45. doi: 10.1097/00008469-199703001-00009
89. Magnusdottir S, Ravcheev D, de Crecy-Lagard V, Thiele I. Systematic genome assessment of b-vitamin biosynthesis suggests co-operation among gut microbes. Front Genet (2015) 6:148. doi: 10.3389/fgene.2015.00148
90. Rowland I, Gibson G, Heinken A, Scott K, Swann J, Thiele I, et al. Gut microbiota functions: metabolism of nutrients and other food components. Eur J Nutr (2018) 57(1):1–24. doi: 10.1007/s00394-017-1445-8
91. Wang HH, Wen FQ, Wei JR. [Research advances in the relationship between childhood malnutrition and gut microbiota]. Zhongguo Dang Dai Er Ke Za Zhi (2016) 18(11):1188–93. doi: 10.7499/j.issn.1008-8830.2016.11.026
92. Douglas AE. The microbial dimension in insect nutritional ecology. Funct Ecol (2009) 23(1):38–47. doi: 10.1111/j.1365-2435.2008.01442.x
93. Henry Y, Overgaard J, Colinet H. Dietary nutrient balance shapes phenotypic traits of drosophila melanogaster in interaction with gut microbiota. Comp Biochem Physiol A Mol Integr Physiol (2020) 241:110626. doi: 10.1016/j.cbpa.2019.110626
94. Shin SC, Kim SH, You H, Kim B, Kim AC, Lee KA, et al. Drosophila microbiome modulates host developmental and metabolic homeostasis via insulin signaling. Science (2011) 334(6056):670–4. doi: 10.1126/science.1212782
95. Matos RC, Schwarzer M, Gervais H, Courtin P, Joncour P, Gillet B, et al. D-alanylation of teichoic acids contributes to lactobacillus plantarum-mediated drosophila growth during chronic undernutrition. Nat Microbiol (2017) 2(12):1635–47. doi: 10.1038/s41564-017-0038-x
96. Wong AC, Dobson AJ, Douglas AE. Gut microbiota dictates the metabolic response of drosophila to diet. J Exp Biol (2014) 217(Pt 11):1894–901. doi: 10.1242/jeb.101725
97. Erkosar B, Storelli G, Mitchell M, Bozonnet L, Bozonnet N, Leulier F. Pathogen virulence impedes mutualist-mediated enhancement of host juvenile growth via inhibition of protein digestion. Cell Host Microbe (2015) 18(4):445–55. doi: 10.1016/j.chom.2015.09.001
98. Yamada R, Deshpande SA, Bruce KD, Mak EM, Ja WW. Microbes promote amino acid harvest to rescue undernutrition in drosophila. Cell Rep (2015) 10(6):865–72. doi: 10.1016/j.celrep.2015.01.018
99. Sannino DR, Dobson AJ, Edwards K, Angert ER, Buchon N. The drosophila melanogaster gut microbiota provisions thiamine to its host. mBio (2018) 9(2):e00155–18. doi: 10.1128/mBio.00155-18
100. Verduci E, Carbone MT, Borghi E, Ottaviano E, Burlina A, Biasucci G. Nutrition, microbiota and role of gut-brain axis in subjects with phenylketonuria (PKU): A review. Nutrients (2020) 12(11):3319. doi: 10.3390/nu12113319
101. Song M, Chan AT. Environmental factors, gut microbiota, and colorectal cancer prevention. Clin Gastroenterol Hepatol (2019) 17(2):275–89. doi: 10.1016/j.cgh.2018.07.012
102. Sepich-Poore GD, Zitvogel L, Straussman R, Hasty J, Wargo JA, Knight R. The microbiome and human cancer. Science (2021) 371(6536):eabc4552. doi: 10.1126/science.abc4552
103. Park EM, Chelvanambi M, Bhutiani N, Kroemer G, Zitvogel L, Wargo JA. Targeting the gut and tumor microbiota in cancer. Nat Med (2022) 28(4):690–703. doi: 10.1038/s41591-022-01779-2
104. Hou H, Chen D, Zhang K, Zhang W, Liu T, Wang S, et al. Gut microbiota-derived short-chain fatty acids and colorectal cancer: Ready for clinical translation? Cancer Lett (2022) 526:225–35. doi: 10.1016/j.canlet.2021.11.027
105. Lu Y, Yuan X, Wang M, He Z, Li H, Wang J, et al. Gut microbiota influence immunotherapy responses: mechanisms and therapeutic strategies. J Hematol Oncol (2022) 15(1):47. doi: 10.1186/s13045-022-01273-9
106. Zagato E, Pozzi C, Bertocchi A, Schioppa T, Saccheri F, Guglietta S, et al. Endogenous murine microbiota member faecalibaculum rodentium and its human homologue protect from intestinal tumour growth. Nat Microbiol (2020) 5(3):511–24. doi: 10.1038/s41564-019-0649-5
107. Santoni M, Piva F, Conti A, Santoni A, Cimadamore A, Scarpelli M, et al. Re: Gut microbiome influences efficacy of PD-1-based immunotherapy against epithelial tumors. Eur Urol (2018) 74(4):521–2. doi: 10.1016/j.eururo.2018.05.033
108. Luu M, Riester Z, Baldrich A, Reichardt N, Yuille S, Busetti A, et al. Microbial short-chain fatty acids modulate CD8(+) T cell responses and improve adoptive immunotherapy for cancer. Nat Commun (2021) 12(1):4077. doi: 10.1038/s41467-021-24331-1
109. Kim M, Qie Y, Park J, Kim CH. Gut microbial metabolites fuel host antibody responses. Cell Host Microbe (2016) 20(2):202–14. doi: 10.1016/j.chom.2016.07.001
110. Trompette A, Gollwitzer ES, Pattaroni C, Lopez-Mejia IC, Riva E, Pernot J, et al. Dietary fiber confers protection against flu by shaping Ly6c(-) patrolling monocyte hematopoiesis and CD8(+) T cell metabolism. Immunity (2018) 48(5):992–1005 e1008. doi: 10.1016/j.immuni.2018.04.022
111. Bachem A, Makhlouf C, Binger KJ, de Souza DP, Tull D, Hochheiser K, et al. Microbiota-derived short-chain fatty acids promote the memory potential of antigen-activated CD8(+) T cells. Immunity (2019) 51(2):285–297 e285. doi: 10.1016/j.immuni.2019.06.002
112. Schroeder BO, Birchenough GMH, Stahlman M, Arike L, Johansson MEV, Hansson GC, et al. Bifidobacteria or fiber protects against diet-induced microbiota-mediated colonic mucus deterioration. Cell Host Microbe (2018) 23(1):27–40 e27. doi: 10.1016/j.chom.2017.11.004
113. So D, Whelan K, Rossi M, Morrison M, Holtmann G, Kelly JT, et al. Dietary fiber intervention on gut microbiota composition in healthy adults: a systematic review and meta-analysis. Am J Clin Nutr (2018) 107(6):965–83. doi: 10.1093/ajcn/nqy041
114. Yang B, Wang FL, Ren XL, Li D. Biospecimen long-chain n-3 PUFA and risk of colorectal cancer: a meta-analysis of data from 60,627 individuals. PloS One (2014) 9(11):e110574. doi: 10.1371/journal.pone.0110574
115. Gopalakrishnan V, Spencer CN, Nezi L, Reuben A, Andrews MC, Karpinets TV, et al. Gut microbiome modulates response to anti-PD-1 immunotherapy in melanoma patients. Science (2018) 359(6371):97–103. doi: 10.1126/science.aan4236
116. Matson V, Fessler J, Bao R, Chongsuwat T, Zha Y, Alegre ML, et al. The commensal microbiome is associated with anti-PD-1 efficacy in metastatic melanoma patients. Science (2018) 359(6371):104–8. doi: 10.1126/science.aao3290
117. Schiweck C, Edwin Thanarajah S, Aichholzer M, Matura S, Reif A, Vrieze E, et al. Regulation of CD4(+) and CD8(+) T cell biology by short-chain fatty acids and its relevance for autoimmune pathology. Int J Mol Sci (2022) 23(15):8272. doi: 10.3390/ijms23158272
118. Sam QH, Ling H, Yew WS, Tan Z, Ravikumar S, Chang MW, et al. The divergent immunomodulatory effects of short chain fatty acids and medium chain fatty acids. Int J Mol Sci (2021) 22(12):6453. doi: 10.3390/ijms22126453
119. Coutzac C, Jouniaux JM, Paci A, Schmidt J, Mallardo D, Seck A, et al. Systemic short chain fatty acids limit antitumor effect of CTLA-4 blockade in hosts with cancer. Nat Commun (2020) 11(1):2168. doi: 10.1038/s41467-020-16079-x
120. Park J, Kim M, Kang SG, Jannasch AH, Cooper B, Patterson J, et al. Short-chain fatty acids induce both effector and regulatory T cells by suppression of histone deacetylases and regulation of the mTOR-S6K pathway. Mucosal Immunol (2015) 8(1):80–93. doi: 10.1038/mi.2014.44
121. Park J, Goergen CJ, HogenEsch H, Kim CH. Chronically elevated levels of short-chain fatty acids induce T cell-mediated ureteritis and hydronephrosis. J Immunol (2016) 196(5):2388–400. doi: 10.4049/jimmunol.1502046
122. Mager LF, Burkhard R, Pett N, Cooke NCA, Brown K, Ramay H, et al. Microbiome-derived inosine modulates response to checkpoint inhibitor immunotherapy. Science (2020) 369(6510):1481–9. doi: 10.1126/science.abc3421
123. Wang T, Gnanaprakasam JNR, Chen X, Kang S, Xu X, Sun H, et al. Inosine is an alternative carbon source for CD8(+)-t-cell function under glucose restriction. Nat Metab (2020) 2(7):635–47. doi: 10.1038/s42255-020-0219-4
124. Garcia-Vello P, Di Lorenzo F, Zucchetta D, Zamyatina A, De Castro C, Molinaro A. Lipopolysaccharide lipid a: A promising molecule for new immunity-based therapies and antibiotics. Pharmacol Ther (2022) 230:107970. doi: 10.1016/j.pharmthera.2021.107970
125. Liu CH, Chen Z, Chen K, Liao FT, Chung CE, Liu X, et al. Lipopolysaccharide-mediated chronic inflammation promotes tobacco carcinogen-induced lung cancer and determines the efficacy of immunotherapy. Cancer Res (2021) 81(1):144–57. doi: 10.1158/0008-5472.CAN-20-1994
126. Liu WT, Jing YY, Yan F, Han ZP, Lai FB, Zeng JX, et al. LPS-induced CXCR4-dependent migratory properties and a mesenchymal-like phenotype of colorectal cancer cells. Cell Adh Migr (2017) 11(1):13–23. doi: 10.1080/19336918.2015.1134404
127. Song W, Tiruthani K, Wang Y, Shen L, Hu M, Dorosheva O, et al. Trapping of lipopolysaccharide to promote immunotherapy against colorectal cancer and attenuate liver metastasis. Adv Mater (2018) 30(52):e1805007. doi: 10.1002/adma.201805007
128. Berendt MJ, North RJ, Kirstein DP. The immunological basis of endotoxin-induced tumor regression. requirement for a pre-existing state of concomitant anti-tumor immunity. J Exp Med (1978) 148(6):1560–9. doi: 10.1084/jem.148.6.1560
129. Engelhardt R, Mackensen A, Galanos C. Phase I trial of intravenously administered endotoxin (Salmonella abortus equi) in cancer patients. Cancer Res (1991) 51(10):2524–30.
130. Otto F, Schmid P, Mackensen A, Wehr U, Seiz A, Braun M, et al. Phase II trial of intravenous endotoxin in patients with colorectal and non-small cell lung cancer. Eur J Cancer (1996) 32A(10):1712–8. doi: 10.1016/0959-8049(96)00186-4
131. Chicoine MR, Won EK, Zahner MC. Intratumoral injection of lipopolysaccharide causes regression of subcutaneously implanted mouse glioblastoma multiforme. Neurosurgery (2001) 48(3):607–14. doi: 10.1097/00006123-200103000-00032. discussion 614-605.
132. Li Y, Shen G, Nie W, Li Z, Sang Y, Zhang B, et al. Irradiated tumor cells of lipopolysaccharide stimulation elicit an enhanced anti-tumor immunity. J Cancer Res Clin Oncol (2014) 140(11):1815–23. doi: 10.1007/s00432-014-1721-4
133. Shetab Boushehri MA, Lamprecht A. TLR4-based immunotherapeutics in cancer: A review of the achievements and shortcomings. Mol Pharm (2018) 15(11):4777–800. doi: 10.1021/acs.molpharmaceut.8b00691
134. Gnanaprakasam JNR, Estrada-Muniz E, Vega L. The anacardic 6-pentadecyl salicylic acid induces macrophage activation via the phosphorylation of ERK1/2, JNK, P38 kinases and NF-kappaB. Int Immunopharmacol (2015) 29(2):808–17. doi: 10.1016/j.intimp.2015.08.038
135. Hollands A, Corriden R, Gysler G, Dahesh S, Olson J, Raza Ali S, et al. Natural product anacardic acid from cashew nut shells stimulates neutrophil extracellular trap production and bactericidal activity. J Biol Chem (2016) 291(27):13964–73. doi: 10.1074/jbc.M115.695866
136. Erpenbeck L, Schon MP. Neutrophil extracellular traps: protagonists of cancer progression? Oncogene (2017) 36(18):2483–90. doi: 10.1038/onc.2016.406
137. Paik D, Yao L, Zhang Y, Bae S, D'Agostino GD, Zhang M, et al. Human gut bacteria produce Tau(Eta)17-modulating bile acid metabolites. Nature (2022) 603(7903):907–12. doi: 10.1038/s41586-022-04480-z
138. Campbell C, McKenney PT, Konstantinovsky D, Isaeva OI, Schizas M, Verter J, et al. Bacterial metabolism of bile acids promotes generation of peripheral regulatory T cells. Nature (2020) 581(7809):475–9. doi: 10.1038/s41586-020-2193-0
139. Ma C, Han M, Heinrich B, Fu Q, Zhang Q, Sandhu M, et al. Gut microbiome-mediated bile acid metabolism regulates liver cancer via NKT cells. Science (2018) 360(6391):eaan5931. doi: 10.1126/science.aan5931
140. Sivan A, Corrales L, Hubert N, Williams JB, Aquino-Michaels K, Earley ZM, et al. Commensal bifidobacterium promotes antitumor immunity and facilitates anti-PD-L1 efficacy. Science (2015) 350(6264):1084–9. doi: 10.1126/science.aac4255
141. Shi Y, Zheng W, Yang K, Harris KG, Ni K, Xue L, et al. Intratumoral accumulation of gut microbiota facilitates CD47-based immunotherapy via STING signaling. J Exp Med (2020) 217(5):e20192282. doi: 10.1084/jem.20192282
142. Vetizou M, Pitt JM, Daillere R, Lepage P, Waldschmitt N, Flament C, et al. Anticancer immunotherapy by CTLA-4 blockade relies on the gut microbiota. Science (2015) 350(6264):1079–84. doi: 10.1126/science.aad1329
143. Deng H, Li Z, Tan Y, Guo Z, Liu Y, Wang Y, et al. A novel strain of bacteroides fragilis enhances phagocytosis and polarises M1 macrophages. Sci Rep (2016) 6:29401. doi: 10.1038/srep29401
144. Qiu Y, Jiang Z, Hu S, Wang L, Ma X, Yang X. Lactobacillus plantarum enhanced IL-22 production in natural killer (NK) cells that protect the integrity of intestinal epithelial cell barrier damaged by enterotoxigenic escherichia coli. Int J Mol Sci (2017) 18(11):2409. doi: 10.3390/ijms18112409
145. Rizvi ZA, Dalal R, Sadhu S, Kumar Y, Kumar S, Gupta SK, et al. High-salt diet mediates interplay between NK cells and gut microbiota to induce potent tumor immunity. Sci Adv (2021) 7(37):eabg5016. doi: 10.1126/sciadv.abg5016
146. Lam KC, Araya RE, Huang A, Chen Q, Di Modica M, Rodrigues RR, et al. Microbiota triggers STING-type I IFN-dependent monocyte reprogramming of the tumor microenvironment. Cell (2021) 184(21):5338–5356 e5321. doi: 10.1016/j.cell.2021.09.019
147. Paulos CM, Wrzesinski C, Kaiser A, Hinrichs CS, Chieppa M, Cassard L, et al. Microbial translocation augments the function of adoptively transferred self/tumor-specific CD8+ T cells via TLR4 signaling. J Clin Invest (2007) 117(8):2197–204. doi: 10.1172/JCI32205
148. Viaud S, Saccheri F, Mignot G, Yamazaki T, Daillere R, Hannani D, et al. The intestinal microbiota modulates the anticancer immune effects of cyclophosphamide. Science (2013) 342(6161):971–6. doi: 10.1126/science.1240537
149. Iida N, Dzutsev A, Stewart CA, Smith L, Bouladoux N, Weingarten RA, et al. Commensal bacteria control cancer response to therapy by modulating the tumor microenvironment. Science (2013) 342(6161):967–70. doi: 10.1126/science.1240527
150. Baruch EN, Youngster I, Ben-Betzalel G, Ortenberg R, Lahat A, Katz L, et al. Fecal microbiota transplant promotes response in immunotherapy-refractory melanoma patients. Science (2021) 371(6529):602–9. doi: 10.1126/science.abb5920
151. Davar D, Dzutsev AK, McCulloch JA, Rodrigues RR, Chauvin JM, Morrison RM, et al. Fecal microbiota transplant overcomes resistance to anti-PD-1 therapy in melanoma patients. Science (2021) 371(6529):595–602. doi: 10.1126/science.abf3363
152. Chaput N, Lepage P, Coutzac C, Soularue E, Le Roux K, Monot C, et al. Baseline gut microbiota predicts clinical response and colitis in metastatic melanoma patients treated with ipilimumab. Ann Oncol (2017) 28(6):1368–79. doi: 10.1093/annonc/mdx108
153. Tanoue T, Morita S, Plichta DR, Skelly AN, Suda W, Sugiura Y, et al. A defined commensal consortium elicits CD8 T cells and anti-cancer immunity. Nature (2019) 565(7741):600–5. doi: 10.1038/s41586-019-0878-z
154. Zitvogel L, Ayyoub M, Routy B, Kroemer G. Microbiome and anticancer immunosurveillance. Cell (2016) 165(2):276–87. doi: 10.1016/j.cell.2016.03.001
155. Balachandran VP, Luksza M, Zhao JN, Makarov V, Moral JA, Remark R, et al. Identification of unique neoantigen qualities in long-term survivors of pancreatic cancer. Nature (2017) 551(7681):512–6. doi: 10.1038/nature24462
156. Klement RJ, Pazienza V. Impact of different types of diet on gut microbiota profiles and cancer prevention and treatment. Medicina (Kaunas) (2019) 55(4):84. doi: 10.3390/medicina55040084
157. Li G, Xie C, Lu S, Nichols RG, Tian Y, Li L, et al. Intermittent fasting promotes white adipose browning and decreases obesity by shaping the gut microbiota. Cell Metab (2017) 26(4):672–685 e674. doi: 10.1016/j.cmet.2017.08.019
158. Zheng X, Zhou K, Zhang Y, Han X, Zhao A, Liu J, et al. Food withdrawal alters the gut microbiota and metabolome in mice. FASEB J (2018) 32(9):4878–88. doi: 10.1096/fj.201700614R
159. Panebianco C, Adamberg K, Adamberg S, Saracino C, Jaagura M, Kolk K, et al. Engineered resistant-starch (ERS) diet shapes colon microbiota profile in parallel with the retardation of tumor growth in In vitro and In vivo pancreatic cancer models. Nutrients (2017) 9(4):331. doi: 10.3390/nu9040331
160. Spencer CN, McQuade JL, Gopalakrishnan V, McCulloch JA, Vetizou M, Cogdill AP, et al. Dietary fiber and probiotics influence the gut microbiome and melanoma immunotherapy response. Science (2021) 374(6575):1632–40. doi: 10.1126/science.aaz7015
161. Husain Z, Huang Y, Seth P, Sukhatme VP. Tumor-derived lactate modifies antitumor immune response: effect on myeloid-derived suppressor cells and NK cells. J Immunol (2013) 191(3):1486–95. doi: 10.4049/jimmunol.1202702
162. Woolf EC, Syed N, Scheck AC. Tumor metabolism, the ketogenic diet and beta-hydroxybutyrate: Novel approaches to adjuvant brain tumor therapy. Front Mol Neurosci (2016) 9:122. doi: 10.3389/fnmol.2016.00122
163. Lau HCH, Sung JJ, Yu J. Gut microbiota: impacts on gastrointestinal cancer immunotherapy. Gut Microbes (2021) 13(1):1–21. doi: 10.1080/19490976.2020.1869504
164. Bultman SJ. Interplay between diet, gut microbiota, epigenetic events, and colorectal cancer. Mol Nutr Food Res (2017) 61(1). doi: 10.1002/mnfr.201500902
165. De Almeida CV, de Camargo MR, Russo E, Amedei A. Role of diet and gut microbiota on colorectal cancer immunomodulation. World J Gastroenterol (2019) 25(2):151–62. doi: 10.3748/wjg.v25.i2.151
166. Soldati L, Di Renzo L, Jirillo E, Ascierto PA, Marincola FM, De Lorenzo A. The influence of diet on anti-cancer immune responsiveness. J Transl Med (2018) 16(1):75. doi: 10.1186/s12967-018-1448-0
167. Maggini S, Pierre A, Calder PC. Immune function and micronutrient requirements change over the life course. Nutrients (2018) 10(10):1532. doi: 10.3390/nu10101531
168. Hendler R, Zhang Y. Probiotics in the treatment of colorectal cancer. Medicines (Basel) (2018) 5(3):101. doi: 10.3390/medicines5030101
169. Ju S, Ge Y, Li P, Tian X, Wang H, Zheng X, et al. Dietary quercetin ameliorates experimental colitis in mouse by remodeling the function of colonic macrophages via a heme oxygenase-1-dependent pathway. Cell Cycle (2018) 17(1):53–63. doi: 10.1080/15384101.2017.1387701
170. Mileo AM, Nistico P, Miccadei S. Polyphenols: Immunomodulatory and therapeutic implication in colorectal cancer. Front Immunol (2019) 10:729. doi: 10.3389/fimmu.2019.00729
171. Chen L, Yang S, Liao W, Xiong Y. Modification of antitumor immunity and tumor microenvironment by resveratrol in mouse renal tumor model. Cell Biochem Biophys (2015) 72(2):617–25. doi: 10.1007/s12013-015-0513-z
172. Zhao Y, Shao Q, Zhu H, Xu H, Long W, Yu B, et al. Resveratrol ameliorates Lewis lung carcinoma-bearing mice development, decreases granulocytic myeloid-derived suppressor cell accumulation and impairs its suppressive ability. Cancer Sci (2018) 109(9):2677–86. doi: 10.1111/cas.13720
173. Ohno M, Nishida A, Sugitani Y, Nishino K, Inatomi O, Sugimoto M, et al. Nanoparticle curcumin ameliorates experimental colitis via modulation of gut microbiota and induction of regulatory T cells. PloS One (2017) 12(10):e0185999. doi: 10.1371/journal.pone.0185999
174. Ghiringhelli F, Rebe C, Hichami A, Delmas D. Immunomodulation and anti-inflammatory roles of polyphenols as anticancer agents. Anticancer Agents Med Chem (2012) 12(8):852–73. doi: 10.2174/187152012802650048
175. Mego M, Chovanec J, Vochyanova-Andrezalova I, Konkolovsky P, Mikulova M, Reckova M, et al. Prevention of irinotecan induced diarrhea by probiotics: A randomized double blind, placebo controlled pilot study. Complement Ther Med (2015) 23(3):356–62. doi: 10.1016/j.ctim.2015.03.008
176. Banna GL, Torino F, Marletta F, Santagati M, Salemi R, Cannarozzo E, et al. Lactobacillus rhamnosus GG: An overview to explore the rationale of its use in cancer. Front Pharmacol (2017) 8:603. doi: 10.3389/fphar.2017.00603
177. Hu J, Wang C, Ye L, Yang W, Huang H, Meng F, et al. Anti-tumour immune effect of oral administration of lactobacillus plantarum to CT26 tumour-bearing mice. J Biosci (2015) 40(2):269–79. doi: 10.1007/s12038-015-9518-4
178. Hibberd AA, Lyra A, Ouwehand AC, Rolny P, Lindegren H, Cedgard L, et al. Intestinal microbiota is altered in patients with colon cancer and modified by probiotic intervention. BMJ Open Gastroenterol (2017) 4(1):e000145. doi: 10.1136/bmjgast-2017-000145
179. Zhuo Q, Yu B, Zhou J, Zhang J, Zhang R, Xie J, et al. Lysates of lactobacillus acidophilus combined with CTLA-4-blocking antibodies enhance antitumor immunity in a mouse colon cancer model. Sci Rep (2019) 9(1):20128. doi: 10.1038/s41598-019-56661-y
180. Wang F, Yin Q, Chen L, Davis MM. Bifidobacterium can mitigate intestinal immunopathology in the context of CTLA-4 blockade. Proc Natl Acad Sci U.S.A. (2018) 115(1):157–61. doi: 10.1073/pnas.1712901115
181. Shi L, Sheng J, Wang M, Luo H, Zhu J, Zhang B, et al. Combination therapy of TGF-beta blockade and commensal-derived probiotics provides enhanced antitumor immune response and tumor suppression. Theranostics (2019) 9(14):4115–29. doi: 10.7150/thno.35131
182. Shi L, Sheng J, Chen G, Zhu P, Shi C, Li B, et al. Combining IL-2-based immunotherapy with commensal probiotics produces enhanced antitumor immune response and tumor clearance. J Immunother Cancer (2020) 8(2):e000973. doi: 10.1136/jitc-2020-000973
183. Kawanabe-Matsuda H, Takeda K, Nakamura M, Makino S, Karasaki T, Kakimi K, et al. Dietary lactobacillus-derived exopolysaccharide enhances immune-checkpoint blockade therapy. Cancer Discovery (2022) 12(5):1336–55. doi: 10.1158/2159-8290.CD-21-0929
184. Arthur JC, Gharaibeh RZ, Uronis JM, Perez-Chanona E, Sha W, Tomkovich S, et al. VSL3 probiotic modifies mucosal microbial composition but does not reduce colitis-associated colorectal cancer. Sci Rep (2013) 3:2868. doi: 10.1038/srep02868
185. Chen D, Wu J, Jin D, Wang B, Cao H. Fecal microbiota transplantation in cancer management: Current status and perspectives. Int J Cancer (2019) 145(8):2021–31. doi: 10.1002/ijc.32003
Keywords: diet, gut microbiota, immunity, cancer, immunotherapy
Citation: Wang X and Geng S (2023) Diet-gut microbial interactions influence cancer immunotherapy. Front. Oncol. 13:1138362. doi: 10.3389/fonc.2023.1138362
Received: 05 January 2023; Accepted: 16 March 2023;
Published: 24 March 2023.
Edited by:
Giandomenico Roviello, University of Firenze, ItalyReviewed by:
Sajad Karampoor, Iran University of Medical Sciences, IranAftab Alam, University at Buffalo, United States
Copyright © 2023 Wang and Geng. This is an open-access article distributed under the terms of the Creative Commons Attribution License (CC BY). The use, distribution or reproduction in other forums is permitted, provided the original author(s) and the copyright owner(s) are credited and that the original publication in this journal is cited, in accordance with accepted academic practice. No use, distribution or reproduction is permitted which does not comply with these terms.
*Correspondence: Shitao Geng, MjY5MzQ0MDI3NEBxcS5jb20=