- 1Third Hospital of Shanxi Medical University, Shanxi Bethune Hospital, Shanxi Academy of Medical Sciences, Tongji Shanxi Hospital, Taiyuan, China
- 2Department of Hepatobiliary Surgery, Shanxi Bethune Hospital, Shanxi Academy of Medical Sciences, Tongji Shanxi Hospital, Third Hospital of Shanxi Medical University, Taiyuan, China
- 3Hepatic Surgery Center, Institute of Hepato-Pancreato-Biliary Surgery, Tongji Hospital, Tongji Medical College, Huazhong University of Science and Technology, Wuhan, China
Actin is the most abundant and highly conserved cytoskeletal protein present in all eukaryotic cells. Remodeling of the actin cytoskeleton is controlled by a variety of actin-binding proteins that are extensively involved in biological processes such as cell motility and maintenance of cell shape. LIM domain and actin-binding protein 1 (LIMA1), as an important actin cytoskeletal regulator, was initially thought to be a tumor suppressor frequently downregulated in epithelial tumors. Importantly, the deficiency of LIMA1 may be responsible for dysregulated cytoskeletal dynamics, altered cell motility and disrupted cell-cell adhesion, which promote tumor proliferation, invasion and migration. As research progresses, the roles of LIMA1 extend from cytoskeletal dynamics and cell motility to cell division, gene regulation, apical extrusion, angiogenesis, cellular metabolism and lipid metabolism. However, the expression of LIMA1 in malignant tumors and its mechanism of action have not yet been elucidated, and many problems and challenges remain to be addressed. Therefore, this review systematically describes the structure and biological functions of LIMA1 and explores its expression and regulatory mechanism in malignant tumors, and further discusses its clinical value and therapeutic prospects.
1 Introduction
Actin-binding proteins mediate the assembly of actin monomers into distinct filamentous structures. Actin filaments assemble into a variety of networks and bundles that interconnect to form the actin cytoskeleton (1). This is a highly dynamic structure and the dynamic rearrangement of the actin cytoskeleton is fundamental to support a wide range of cellular behaviors. It plays a key role in cell biological processes including cell polarity, adhesion and migration, cell division, intracellular transport and endocytosis (2, 3). Recent studies showed that LIM structural domain proteins are associated with mediating cytoskeletal homeostasis and coordinating these cellular behaviors (4). LIM domain and actin-binding protein 1 (LIMA1) is an actin-binding cytoskeletal protein containing a LIM structural domain. It is subcellularly localized to actin stress fibers and focal adhesion plaques (5, 6). The amino-terminal and carboxy-terminal ends of LIMA1 are located lateral to the central LIM structural domain. They are present in at least two actin-binding domains that are capable of cross-linking and stabilizing cytoskeletal filaments and promoting stress fiber formation (7). This ensures cytoskeletal homeostasis and maintains integration and coordination between different actin regulatory pathways to support dynamic cellular behaviors.
LIMA1 is also known as epithelial protein lost in neoplasms (EPLIN) and sterol regulatory element binding protein 3 (SREBP3). It was initially identified as a differentially expressed gene in oral epithelial cell carcinogenesis using cDNA differential analysis (8). Subsequently, Maul et al. (9) first described and identified LIMA1 as a novel cytoskeletal protein. LIMA1 exists as two distinct isoforms: LIMA1-α containing 600 amino acids and LIMA1-β containing 759 amino acids (10). The amino acid structural domain sequence of LIMA1 is unique. The LIM domain-containing protein as an interaction site for specific signal transduction proteins can form two closely spaced zinc-binding subdomains and allow LIMA1 to dimerize on its own or bind to other proteins (5, 11). Due to the importance of LIMA1 in regulating actin cytoskeleton dynamics and its potential involvement in cadherin-mediated cell adhesion (12), the loss of LIMA1 from cancer cells may affect cell behavior and further enhance invasive characteristics. LIMA1 deficiency may be responsible for dysregulated cytoskeletal dynamics, altered cell motility and disrupted cell-cell adhesion, which can promote tumor proliferation, invasion, and migration (13).
Recent studies, including those from our laboratory, demonstrate a broader role for LIMA1 in controlling cancer cell behaviors. The effects of LIMA1 also extend from cell migration and cytoskeleton dynamics to cell cycle, gene regulation, angiogenesis, and lipid metabolism, among others, providing new ideas for future exploration of cancer treatment strategies (14). In this paper, the latest research progress on the molecular characteristics, biological functions and regulatory mechanisms of LIMA1 could be expounded. It focuses on the roles and significance of LIMA in various cancers and provides insights into its future research prospects.
2 The characterization and functions of LIMA1
2.1 The structure of LIMA1
Current studies have identified the organization of the human LIMA1 gene, which is located on chromosome 12q13.12 and has a sequence length of 107,733 bp (5, 10). It consists of 11 exons spanning 100 kb and 10 introns. The LIMA1 gene is structured with two independent promoter regions. The DNA sequence at the transcription start site stimulates the expression of the promoter reporter gene constructs (13). Under the protection of 5’ RACE and S1 nuclease, the transcription start site of LIMA1-α mRNA is approximately 50 kb downstream near the end of intron 3 and is positioned before exon 4 and contains 4-11 exons. Similarly, the transcription start site of LIMA1-β mRNA is located near the start of exon 1 of the gene and contains all 11 exons (10) (Figure 1). The amino acid sequences of these two isoforms are characterized by the presence of a centrally located LIM structural domain. The LIM structural domain is a cysteine-rich double zinc finger structural domain derived from the three homologous structural domain-containing transcription factors Lin-11, Isl-1 and Mec-3 (15, 16). The N-terminal and C-terminal sides of the LIM structural domain of LIMA1 can promote parallel formation of actin filament structures by cross-linking and binding actin filaments (7) (Figure 2).
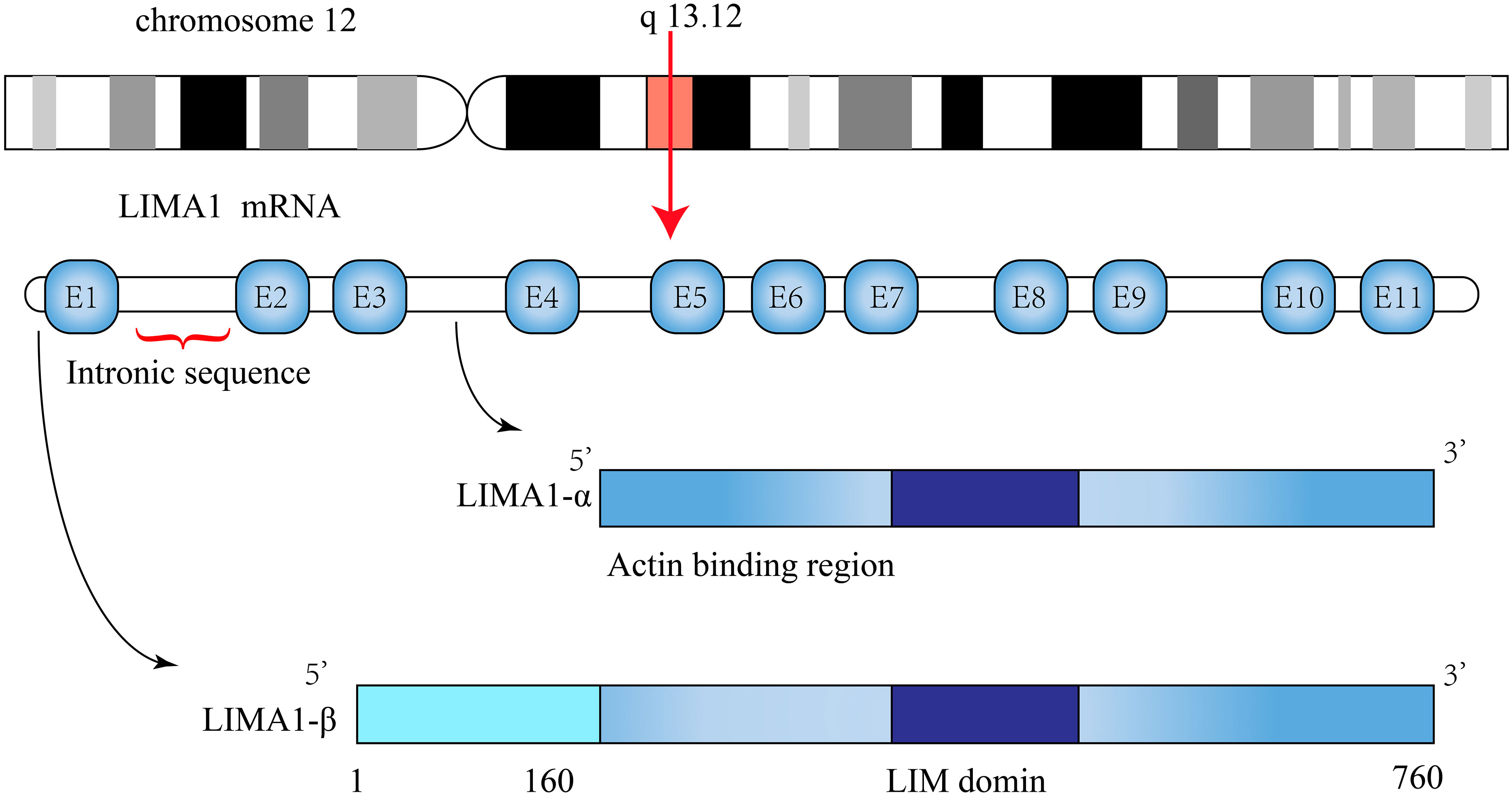
Figure 1 Schematic representation of LIMA1 structure. The LIMA1 gene is located on chromosome 12 and consists of 11 exons and 10 introns. Its protein contains two isoforms, a LIMA1-α of 600 amino acids and a LIMA1-β of 759 amino acids.
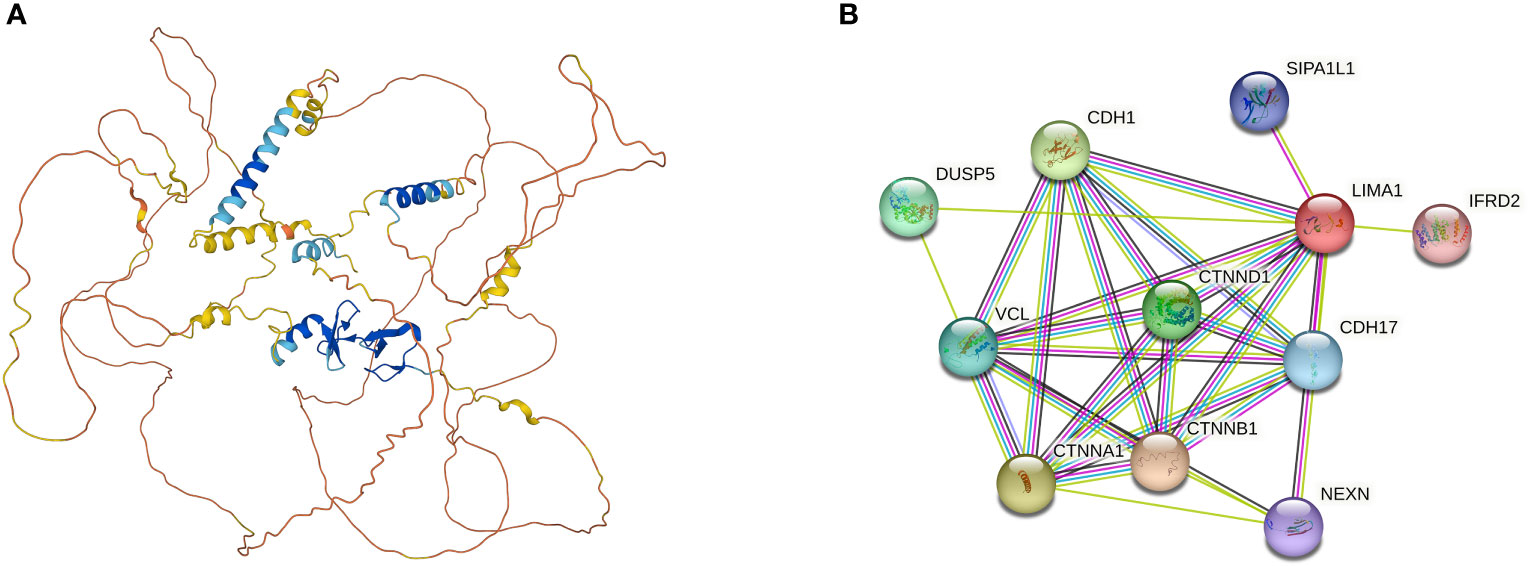
Figure 2 (A) Three-dimensional structure of LIMA1 (PDB ID=2Y), from AlphaFold Protein Structure Database (https://alphafold.ebi.ac.uk/). LIMA1 contains a LIM structural domain in the center and multiple actin-binding domains at the lateral end, which is capable of cross-linking and stabilizing a network of stabilized actin filaments. (B) Potential molecules and functional proteins interacting with LIMA1, which can be derived from the search tool STRING (http://www.string-db.org/) for retrieving interacting genes or proteins. DUSP5, Dual Specificity Phosphatase 5; CDH1, Cadherin 1; VCL, Vinculin; CTNND1, Catenin Delta 1; CTNNA1, Catenin Alpha 1; CTNNB1, Catenin Beta 1; SIPA1L1, Signal Induced Proliferation Associated 1 Like 1; CDH17, Cadherin 17; NEXN, Nexilin F-Actin Binding Protein; IFRD2, Interferon Related Developmental Regulator 2.
Sequence analysis found that LIMA1-α and LIMA1-β isoforms are conserved across species (10). In subsequent working study, Maul et al. (17) isolated and characterized mouse and zebrafish LIMA1 homologous to humans. Akin to the human gene, both 593 aa LIMA1-α and 753 aa LIMA1-β isoforms were found in mouse, showing 77% and 75% identity with human isoforms (5, 17). In contrast, there was only one form in zebrafish, namely 629 aa LIMA1. The overall similarity between zebrafish and human amino acid sequences was not significant. Wang et al. (18) revealed two isoforms of the porcine LIMA1 gene with different expression patterns in muscle development and maintenance. LIMA1-α was preferentially expressed in developing skeletal muscles. Especially in the early stages, LIMA1-α played an important role in the process of muscle cell morphology and growth. LIMA1-β was not expressed during muscle development and only weak expression was detected in adult muscles (13, 18). Recent studies indicated that LIMA1-β regulated the distribution of OB-cadherin, which was involved in the assembly of cadherin-catenin protein complexes in osteoblasts, and affected bone formation (19).
2.2 The functions of LIMA1
2.2.1 Controlling for actin dynamics
LIMA1 controls actin dynamics by stabilizing the actin filament network. The expression of LIMA1 increases the number and size of actin stress fibers and inhibits active Rac-induced membrane folding (6). Furthermore, LIMA1 inhibits actin filament nucleation and F-actin depolymerization by mediating the actin-related protein complex 2/3 (Arp2/3) (13). Moreover, Han et al. (7) showed that LIMA1 was a novel extracellular signal-regulated kinase (ERK)/mitogen-activated protein kinase (MAPK) substrate and that ERK responded, both in vitro and in vivo, to LIMA1 Ser360, Ser602, and Ser692 phosphorylation. ERK-mediated phosphorylation of LIMA1 decreased C-terminal region binding activity to actin leading to reorganization of actin filaments and enhanced cell motility. In addition, Song et al. (20) found that LIMA1 was bound to the actin cytoskeleton and inhibited anchorage-dependent growth of transformed NIH3T3 cells. LIMA1 exhibited a patchy pattern of distribution in the cytoplasm of Ras-transformed cells and activated Ras prevented or altered LIMA1 co-localization with actin stress fibers (21). These changes in subcellular localization of LIMA1 may represent a non-specific outcome of transformation, which frequently altered cell morphology and cytoskeleton. In this process, LIMA1-α is transcriptionally regulated by G-actin and megakaryoblastic acute leukemia (MAL)/myocardin related transcription factor (MRTF) coactivators. It is sensitive to drugs that can stabilize the inhibitory actin MAL complex and non-polymerizable actin mutants (22).
LIMA1 binds to α-catenin and mediates the interaction of the cadherin-catenin complex with F-actin, stabilizing the actin fibers and establishing the adhesion bands at the cell-cell junctions (12). Epithelial cells remodel their junctional structure by responding to mechanical forces. E-cadherin acts as a mechanosensitive regulator of this process of epithelial cell junctional remodeling and can interact with LIMA1 and Vinculin to maintain the zonula adherens (23). Chen et al. (24) identified the substrate of human cell division cycle 14A (CDC14A), the actin regulator LIMA1, by phosphorylated proteomics and the biotin identification proximity assay. LIMA1 phosphorylation regulated by ERK and CDC14A had shown reduced enrichment of α/β-catenin at the cell-cell junctions and downregulation of E-cadherin. Actin kinetic activity was reduced by local regulation of actin rearrangement.
In addition, the actin-stabilizing protein leucine zipper protein 1 (LUZP1) is localized to actin and microtubule structures (25). LIMA1 regulates actin and actin-related protein (myosin Va and ARP2/3) levels by interacting with LUZP1. It also regulates cell migration and ciliogenesis, which is associated with cancer development (26).
2.2.2 Regulation of cell adhesion and metastasis
Cells attach to the network of pre-adsorbed proteins in the extracellular matrix (ECM) or to neighboring cells by interacting with specialized molecules on the cell surface (27). This process is referred to as cell adhesion. Regulation of cell-cell interactions through adhesion junctions is largely dependent on the interaction mediated by E-cadherin and the assembly of a large number of adapter proteins with filamentous actin bundles (28).
LIMA1 acts as an adapter for adhesion junctions and localizes to the integrin adhesion sites of cells in a particularly interesting new cysteine-histidine rich protein 1 (PINCH-1) regulation-dependent manner (29). Depletion of LIMA1 induces the proliferation and migration of keratin-forming cells on collagen and fibronectin both in vivo and in vitro. Tsurumi et al. (30) showed high expression of the actin cross-linking protein LIMA1 in glomerular thylakoid cells. LIMA1 was concentrated in peripheral actin bundles at local adhesions and formed protein complexes with paxillin. Platelet derived growth factor (PDGF) regulated the interaction of LIMA1 with paxillin by inducing the mitogen-activated protein kinase (MEK)-ERK cascade and also induces subcellular localization of LIMA1 from local adhesion to peripheral folds (13, 30). In addition, ubiquitination of Rab40b-Cullin5 regulates LIMA1 localization and promotes cell migration and invasion by altering adhesion patch and cytoskeletal dynamics (31). Zhang et al. (32) showed that epidermal growth factor (EGF) activated phosphorylation, ubiquitination, and degradation of LIMA1 through an ERK1/2-dependent signaling cascade response. Point mutations in serine residues (serine362 and serine604) of LIMA1 have made it resistant to EGF-induced protein degradation.
Subsequent studies confirmed that LIMA1 was a direct transcriptional target of p53 (14, 33). Knockdown of LIMA1 significantly enhanced cancer cell invasion and partially reversed the p53-induced metastasis of cancer cells (33). It can be speculated that LIMA1 may be a novel prognostic predictor and therapeutic target for tumors. P73, a member of the p53 family, was shown to induce cell cycle arrest and apoptosis, negatively regulating cancer progression (34). Steder et al. (35) found that N-terminal truncation of the P73 gene family produced DNp73, which was frequently upregulated in high-grade tumors. DNp73 inhibited the repression of LIMA1 by interfering with p73, leading to the disruption of intercellular adhesion junctions, which further activated insulin like IGF1R-PKB/STAT3 signaling pathway to initiate the invasion and metastasis cascade response.
2.2.3 Dynamic maintenance of the epithelial mesenchymal transition
Epithelial mesenchymal transition (EMT) is the process by which epithelial cells lose apical-basal polarity and intercellular adhesion and then transform to invasive mesenchymal cells (36). During the transition, proteins characteristic of the epithelial phenotype such as E-cadherin, cytokeratins, or occludin are absent, while N-cadherin, vimentin, or fibronectin are upregulated due to the acquisition of mesenchymal cell characteristics (37). Zhang et al. (32) and Zhitnyak et al. (38) revealed that the actin-binding protein LIMA1 maintained the stability of the circumferential actin bundle, and that EGF induced EMT and increased invasive potential through phosphorylated degradation of LIMA1 leading to active remodeling of the actin cytoskeleton and disruption of intercellular adhesion. It is well known that the regulation of EMT requires the involvement of multiple signaling pathways, including the activation of Wnt/β-catenin, TGF-β, and Notch, among other pathways (39), leading to altered cell morphology, increase in cell motility, and enhanced secretion of growth factors or proteins (40). Subsequently, it was reported that DNp73 exhibited an EMT-like phenotype through dependent downregulation of LIMA1 with loss of E-cadherin and Slug (35). This led to the loss of apical cell polarization and stable cell adhesion, allowing the cells to acquire the ability to invade to promote metastatic potential.
Recent studies support that LIMA1 may regulate the epithelial to mesenchymal transition. Most of these studies suggest that LIMA1 deficiency appears to contribute to the development of EMT and metastasis of cancer cells. LIMA1 is a negative regulator of EMT and invasiveness in prostate cancers, inhibiting E-cadherin, activating β-catenin signaling pathway and enhancing chemoresistance (41, 42). Similarly, LIMA1 depletion was found to promote EMT and induced actin cytoskeleton remodeling in breast cancers (43) and epithelial ovarian cancers (44) significantly enhancing the migration and invasion of epithelial cancer cells both in vivo and in vitro. And another study found that the expression of LIMA1 was upregulated in head and neck tumors, driving invasion and metastasis by activating tumor-associated pathways such as PI3K-AKT and JAK-STAT signaling pathways to promote the EMT process (45). It is considered likely that DNA demethylation of the LIMA1 promoter region results in different expression levels of LIMA1 in HNSC.
For future studies on LIMA1 regulation of EMT, we propose constructive and rational research themes. It is possible to quantitatively assess the role of LIMA1 in EMT, explore the molecular mechanisms of LIMA1 regulation of EMT, establish LIMA1 targeting factors and explore their interactions with LIMA1. These studies can predict the cancer biomarkers and patient prognosis associated with LIMA1 and EMT, and help identify novel drugs and therapeutic approaches for cancers.
2.2.4 Modification of the epithelial defense against cancer
During the initial stages of oncogenic mutations, individual cells within the epithelium may undergo transformation (46). Normal epithelial cells can identify the presence of transformed cells and eliminate newly emerging transformed cells by competition, which is known as epithelial defense against cancer (EDAC) (47). Recent studies have shown that transformed cells of RasV12 are extruded from the tip by neighboring epithelial cells (48). This process is the most basic epithelial defense against cancer and involves various non-cellular autonomous changes in normal and transformed cells; however, this is unrelated to the anti-tumor properties of the immune system. The molecular mechanisms behind this phenomenon remain largely elusive. Ohoka et al. (21) found that caveolin-1 (Cav-1) and LIMA1 accumulated in the apical and outer membrane structural domains and in the cytoplasmic matrix of RasV12 transformed cells. LIMA1 acted mainly upstream of Cav-1 to regulate the non-cellular, autonomous activation of myosin II and PKA in RasV12 transformed cells (21, 48). This resulted in extrusion of RasV12 transformed cells from the apical part of the normal epithelial cell monolayer.
In addition, Saitoh et al. (49) showed that Rab5-mediated endocytosis was enhanced in RasV12-transformed cells disrupting cell-to-cell adhesion based on E-cadherin through the dependent regulation of LIMA1. This process affected the accumulation of filamentous proteins in neighboring normal cells, which acted as mechano-sensors exerting the physical forces required for apical extrusion. Importantly, LIMA1 co-accumulated with plectins, microtubules and intermediate filaments in RasV12 transformed cells and positively regulated the apical elimination of transformed cells from the epithelium in a synergistic manner (50). Kasai et al. (51) showed that paxillin induced the acetylation of microtubulin by linking the plectin-LIMA1 complex. It inhibited histone deacetylase 6 (HDAC6) activity, partially rescuing the inhibitory effect of paxillin knockdown on apical extrusion in RasV12 cells (50, 51). In future studies, there is still a need to reveal the mechanism of cytoskeletal organization mechanism of apical extrusion in transformed cells to provide new avenues for establishing novel cancer prevention and treatment.
2.2.5 Promotion of the endothelial cell formation and angiogenesis
Angiogenesis is the process by which endothelial cells form new blood vessels from pre-existing vessels (52). This process includes many complex steps, including the activation, proliferation and migration of endothelial cells, the vascular rings formed by endothelial cell tubes, and the generation of neovascularization and basement membranes (53). Importantly, adherent junctions are required for the remodeling of cellular junctions and the maintenance of vascular endothelial integrity.
The vascular endothelial cadherin (VE-cadherin) is an endothelial cell-specific expressed protein, also known as cadherin 5. VE-cadherin mediates adhesive junctions between adjacent vascular endothelial cells by connecting chain proteins, including α-catenin, β-catenin and γ-catenin, to the actin cytoskeleton (54). Chervin-Pétinot et al. (55) previously presented evidence that LIMA1 co-localized with α-catenin of endothelial cells in the actin cortical loop. LIMA1 attached the VE-cadherin and catenin complexes to the actin cytoskeleton by interacting with α-catenin and actin filaments. It also strengthened cell-cell cohesion by recruiting vinculin, while enhancing endothelial adhesion junctions (55). In addition, ARP2/3 complex dynamically competes with VE-cadherin and a/β-catenin to bind actin filaments (56). This has been shown to be critical in the formation and maintenance of endothelial cell adhesion and integrity mediated by cadherin/catenin complexes.
Subsequently, Hofer et al. (57) used the fluorescent live cell imaging system to explore endothelial cell junctional dynamics under the static and shear stress conditions. By using fluorescent tags (mCherry and EGFP) with self-labelling tags (Halo and SNAP), it was demonstrated that VE-cadherin tagged with EGFP maintained cytoarchitectonic integrity during shear stress-induced junctional remodeling (57). And that two isoforms of LIMA1 were shown to be localized at the cell junctions of vascular endothelial cells (54, 57). However, whether these isoforms have different functions at the cell junctions remains to be investigated. Subsequently, Smith et al. (58) preliminarily verified that LIMA1 subtypes were dependent on stimulation both in vivo and in vitro. The LIMA1-α expression was increased in endothelial cells during the growth phase; this modulates subcellular localization somewhat and controls protrusion dynamics as driven by actin (58). LIMA1-α controlled the plasma membrane protrusion directly by interacting with the Arp2/3 complex and junction-associated intermittent lamellipodia (JAIL), to increase cell migration and cell junction adhesion (5, 6, 56). The LIMA1-β expression was higher in endothelial cells exposed to aortic endothelium and endothelial cells with high shear stress compared to the vena cava endothelium (58). The magnitude of the LIMA1-β expression in endothelial cells correlates with hemodynamics and acts to induce and stabilize stress fibers. In addition, some investigators conducted in vitro studies based on tumor vascular endothelial cells and in vivo studies based on animal models (59, 60).
Angiogenesis plays a crucial role in almost all stages of cancer growth, aggressiveness and metastasis. Tumor angiogenesis has been reported to be a hallmark of carcinogenesis (61). Sanders et al. (59) found that overexpression of LIMA1-α could regulate endothelial cell migration, stromal adhesion and formation of new vascular-like structures in vitro, and retard tumor formation in vivo. It is evident that LIMA1-α has anti-angiogenic effects. Notably, there is was more substantial interaction between LIMA1-α and ERK in endothelial angiogenesis. ERK inhibitors could rescue the tubule formation in HECV cells caused by the overexpression of LIMA1-α (7, 59). Liang et al. (60) also showed that miR-93-5p enhanced the migration and angiogenesis of human umbilical vein endothelial cells (HUVEC) through the downregulation of LIMA1 based on in vitro and in vivo studies. LIMA1 plays a disruptive role in angiogenesis during cancer development to a certain extent, thus providing a new theoretical basis for the molecular regulation mechanism of the tumor angiogenesis process.
2.2.6 Modulation of the inflammatory response
Endothelial cells are considered key regulators of the inflammatory response. When exposed to the proinflammatory cytokines IL-1β, TNF-α, and IFN-γ, it could lead to local breaks at endothelial cell-cell junctions and exacerbated endothelial barrier dysfunction during the inflammatory response (62). Maucher et al. (63) demonstrated significant changes in the miRNA expression profile of endothelial cells in response to the stimulation by inflammatory cytokines, which may be involved in endothelial permeability regulation. Among them, miR-29a-3p, miR-29b-3p and miR-155-5p expression was significantly increased and suppressed the adhesion protein expression in endothelial cells after transcription. Importantly, the target genes of these miRNAs included β-catenin, p120-catenin and LIMA1, which are key mediators of endothelial cell adhesion junctions (14, 63). These results provide new insights into the dysfunction of the inflammation-induced endothelial barrier and the mechanism of cancer progression.
2.2.7 Maintaining the stability of cell division
Cell division is a fundamental process required for cell proliferation and DNA replication in the majority of organisms, which ensures relative genetic stability (64). During cytokinesis, actin-myosin II contractile loops and septal filaments act synergistically to generate the oval groove and control the contraction of microfilaments constricting it (65). The oval groove is gradually deepened to drive cell membrane invasion causing the parental cells to divide into two daughter cells to complete cytoplasmic division.
Chircop et al. (66) discovered that the actin-binding protein LIMA1 was localized in the oval groove during cytoplasmic division. LIMA1 recruited myosin II, septin 2, small GTP ases, and RhoA to locally accumulate in the oval groove to maintain its formation and contractile ring activity (67). The membrane protein supervillin co-localized with endogenous myosin II and LIMA1 in the oval sulcus and synergistically exerted the functions in regulating actin and microtubule motility (14, 67). Notably, deletion of supervillin could lead to an increase in the number of binucleated and multinucleated cells. Subsequently, Sundvold et al. (68) found that LIMA1 maintained proper cell division by recruiting ACAT-related protein required for viability 1 (Arv1) to the oval groove and driving efficient contraction of the actomyosin ring during the mitotic telomere phase. Both LIMA1 and Arv1 are required for efficient accumulation of myosin at the oval groove. Given that LIMA1 is frequently lost in multiple cancers (13), deletion of LIMA1 affects the recruitment of key cytoplasmic splitting proteins at the oval groove, which leads to the formation of multinucleated cells and increases genomic instability and oncogenicity.
2.2.8 Control of the membrane dynamics
Membrane dynamics is an important component of cellular metabolic processes (69). It includes contraction of the oval groove during cell division, tubularization of plasma membrane receptors, enhancement of laminar lipid formation, cell migration and invasion, and endocytosis during membrane protein sorting and transport (70). These processes are dependent on the structural and functional interconnection of the cell membrane with the cytoskeleton.
As the actin-binding protein, LIMA1 is a key effector molecule mediating pluripotency control of membrane dynamics and cellular metabolism. Duethorn et al. (71) revealed that LIMA1 was ectopically expressed in mouse and human pluripotent stem cells. The LIMA1 expression was transcriptionally controlled by naive pluripotency circuits and could inhibit the membrane vesicle formation (71). As that LIMA1 is required for normal mitochondrial energy in embryonic stem cells and is essential for solid tumor growth.
2.2.9 Regulation of lipid metabolism
Lipid metabolism is an important and complex biochemical reaction in the metabolic processes of human body (72). When the synthesis, decomposition, digestion, absorption, and transport of lipid substances in the body are abnormal, it will cause either too much or too little lipid to be present in each tissue resulting in abnormal lipid metabolism (73). Particularly, cholesterol is an important lipid in the process of lipid metabolism, which synthesizes cholesteryl esters under specific conditions and attaches to the walls of blood vessels and in the liver. High levels of low-density lipoprotein cholesterol (LDL-C) are strongly associated with an increased risk of myocardial infarction and death from vascular diseases due to increased blood cholesterol (74).
Cholesterol variability is controlled by genetic variability. Zhang et al. (75) verified that LIMA1-K306fs SNV was responsible for the low LDL-C variant by Sanger sequencing. The LIMA1 gene mutation was found to be a rare shift mutation in Chinese families with genetically low LDL-C Kazakhs (75). This was the first time that LIMA1 variability was proposed as a newly identified genetic player in the mechanisms controlling cholesterol homeostasis (76). The mutation resulted in low serum LDL-C concentrations and reduced cholesterol absorption in the intestine. Subsequently, Lim et al. (77) similarly demonstrated that cholesterol absorption is reduced with intestinal-specific deficiency of LIMA1 and that inhibition of LIMA1 could reduce LDL-C cholesterol levels. LIMA1 and niemann-pick c1-like 1 (NPC1L1) interactions are required during cholesterol absorption (77). Based on mouse models and tests on human samples, researches had shown that LIMA1 regulated cholesterol transport by recruiting myosin Vb to NPC1L1 to promote efficient intestinal absorption of cholesterol (75, 77). In addition, Su et al. (78) identified genes associated with low disease prevalence based on phenomic, genomic, and metabolomic features, including APOA5, LPL, HIF1A, LIMA1, and others. The association between these genes and lipid metabolism and inflammation is particularly striking, but the exact mechanisms remain unclear.
In the future, it is necessary to study in depth the detailed mechanisms by which LIMA1 controls cholesterol variability to add the meaningful mechanistic insights into the biology of cholesterol absorption. This can provide new therapeutic targets for hypercholesterolemia and abnormal lipid metabolism.
3 Roles of LIMA1 in cancer development and progression
The roles and regulatory mechanisms of LIMA1 gene have received a great deal of scientific attention in a variety of malignancies. This is consistent with the findings that LIMA1 contributes to the maintenance of the epithelial cytoskeleton, supports cell-cell junctions, and regulates lipid metabolism and angiogenesis (6, 14) (Table 1). LIMA1 deficiency promotes the disassembly of adhesion-catenin complexes and redistribution of cadherin-catenin complex components (11, 12): it induces remodeling of the actin cytoskeleton and activation of β-catenin signaling, which promotes epithelial to mesenchymal morphology and enhances plasticity and migration of cancer cells (31, 32). Overexpression of LIMA1 has been shown to be effective in manipulating tumor characteristics such as reducing cell growth and cell motility and rendering cells less aggressive (41, 42).
Recent studies have shown that the LIMA1 gene exhibits frequent downregulation and loss in cancers (Figure 3), and it can be involved in the development of malignancy through various mechanisms (13) (Figure 4). LIMA1 is associated with the progression and metastasis of various solid tumors including oral cancer (8), esophageal cancer (79), gastric cancer (80), prostate cancer (11, 41, 42, 81), breast cancer (43), ovarian cancer (44), and head and neck squamous cell carcinoma (45, 46), as well as osteosarcoma (82) and lymphoma (83, 84) (Table 2). Moreover, LIMA1 particularly plays a crucial role in biological processes such as tumor proliferation, apoptosis, migration, invasion, drug resistance, immune response (79, 80). This suggests that LIMA1 is expected to be a potential prognostic biological marker and therapeutic target for tumor therapy. Therefore, there is an urgent need to further explore the molecular mechanisms by which LIMA1 regulates tumor progression.
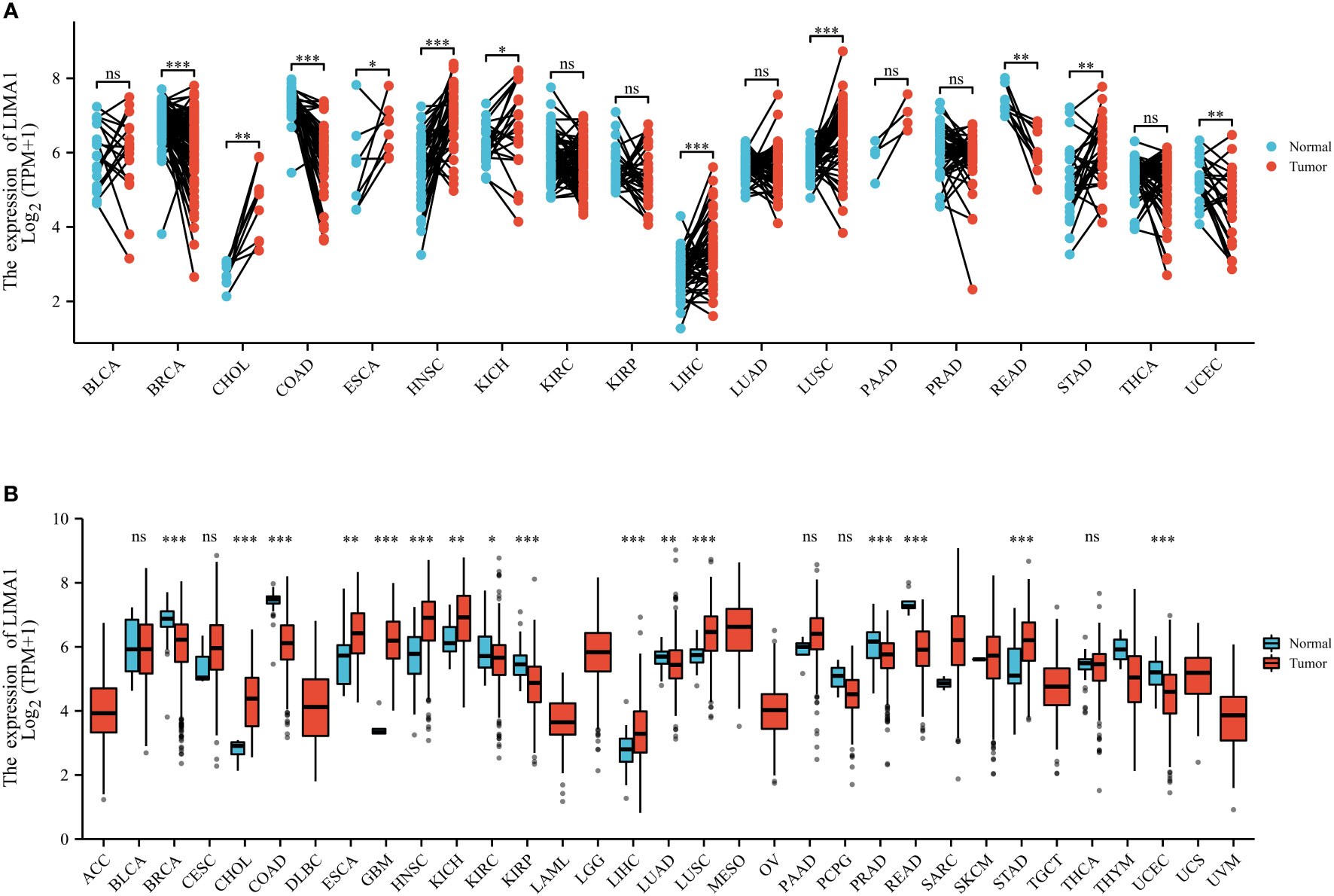
Figure 3 LIMA1 expression patterns in pan-cancer. (A) LIMA1 expression levels in 33 different types of cancer and non-cancer samples based on The Cancer Genome Atlas Database (TCGA) (https://portal.gdc.cancer.gov/). (B) LIMA1 expression levels in cancer tissues and its paired normal tissues based on TCGA dataset. *p < 0.05, **p < 0.01, ***p < 0.001. "ns" means that there is no statistical difference between the two comparison groups.
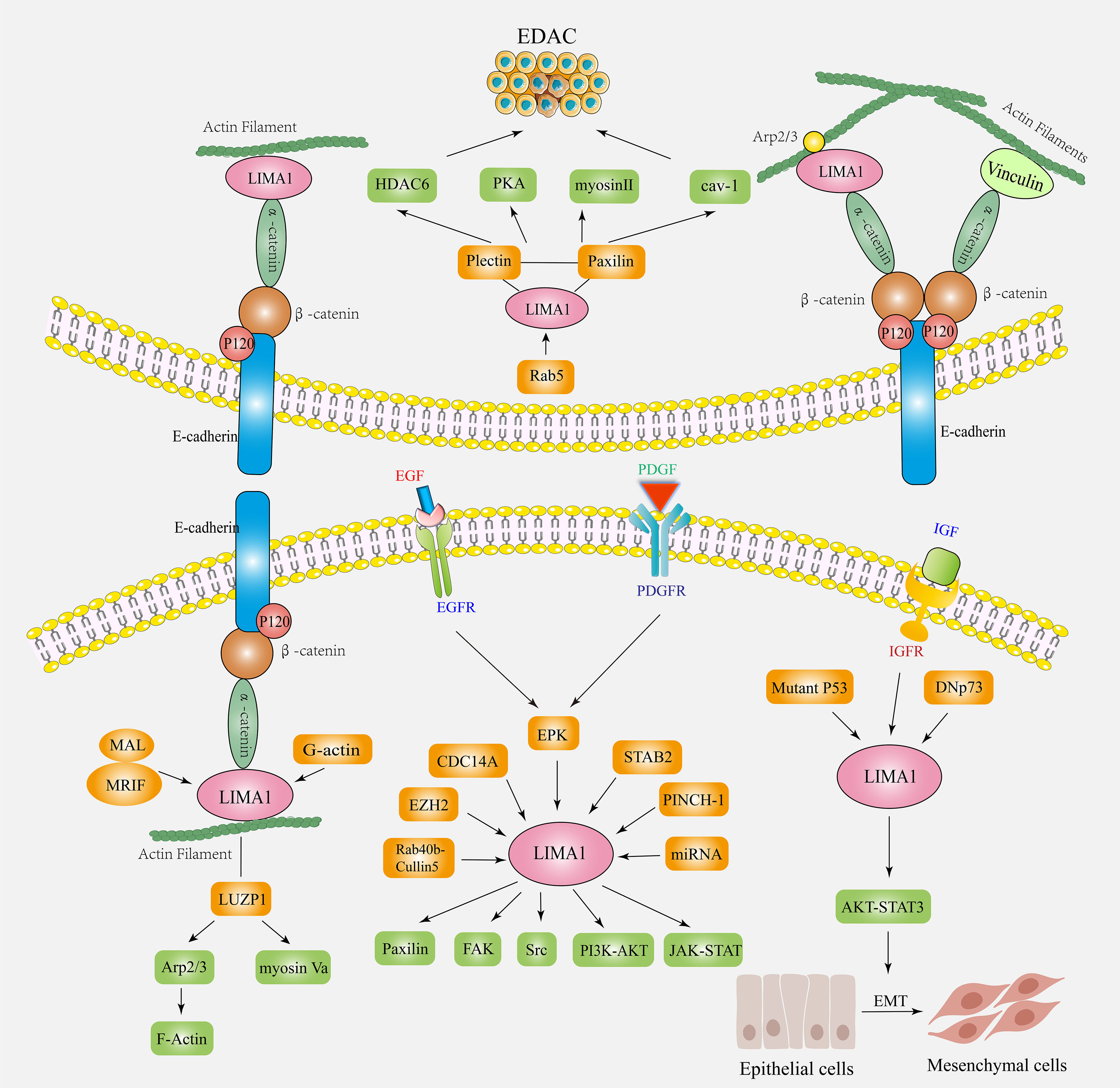
Figure 4 The biological functions and mechanisms of action of LIMA1 in cancers. LIMA1 couples with α-catenin protein, mediates the interaction of cadherin-catenin protein complex with actin. It controls actin dynamics and cell-cell junctions by stabilizing the actin filament networks. LIMA1 acts as a transcriptional target for a variety of factors (ERK, EZH2, CDC14A, Rab40b-Cullin5, STAB2, PINCH-1, miRNA, etc.) by regulating downstream signaling factors (paxillin, plectin, FAK, Src, Cav-1, Myosin II, PKA, etc.) to affect the plasticity and migration ability of cancer cells. LIMA1 deficiency induces the remodeling of the regulatory actin cytoskeleton and activation of signaling pathways, promoting EMT and EDAC, and affects tumor invasion and metastasis.
4 Expression of LIMA1 and its regulatory mechanisms in digestive system tumors
Digestive system cancers are one of the most common leading causes of cancer deaths worldwide, with high morbidity and mortality (85). It is difficult to detect cancers at an early stage due to lack of effective biomarkers (86). Therefore, identification of potentially effective biomarkers can help in the early diagnosis of such cancers. The current research hot-spots on LIMA1 in digestive system tumors are mainly focused on esophageal cancer (79) and gastric cancer (80). While our laboratory has validated the regulatory mechanism of LIMA1 with liver cancer. LIMA1 is expected to be a new biomarker for tumor diagnosis, prognostic biomarker and targeted therapy to improve survival of cancer patients in the future.
4.1 LIMA1 and esophageal cancer
Esophageal cancer is the most aggressive of all malignancies of the gastrointestinal tract. Esophageal cancer is prone to recurrence and metastasis, and the current five-year survival rate for patients remains low due to its high morbidity and mortality (87). Liu et al. (79) used the quantitative polymerase chain reaction (q-PCR) method to determine the aberrant expression of LIMA1-α transcripts in human esophageal tissues (tumor, paraneoplastic, and normal). It was also shown that overexpression of LIMA1-α reduced the aggressiveness of the esophageal cancer cell line KYSE150 and attenuated the rate of cell invasion and growth in vitro. This is consistent with previous studies showing that LIMA1-α expression is frequently downregulated or lost in a variety of different cancer cell lines, including oral cancer cells (8), prostate cancer cells (11, 41), and breast cancer cell lines (43). There was a negative correlation between LIMA1-α expression levels and tumor grade, lymph node status, tumor stage, and whether patients remained disease-free or died from cancers (79). Combined with the findings of LIMA1-α inhibition of cell growth and invasion, it was found that LIMA1-α downregulation has a predictive value. This highlights the potential of LIMA1 as a prognostic indicator and that this molecule may act as a protective factor.
4.2 LIMA1 and gastric cancer
Gastric cancer is one of the most common and highly aggressive malignancies worldwide. It accounts for more than 1 million new cases each year and remains the third leading cause of cancer death (88). Surgical resection is the best option for early-stage gastric cancer, while chemotherapy is mainly used in the intermediate and late stages of the disease (89), however, there are many reports of treatment failure in gastric cancer due to chemotherapy resistance. Combined with the low rate of early diagnosis, limited treatment and tumor heterogeneity, the prognosis of gastric cancer remains poor.
Gong et al. (80) explored the correlation between LIMA1 transcript expression and patient clinicopathological factors and its importance in neoadjuvant chemotherapy (NAC) responsiveness through two gastric cancer cohorts collected from Beijing Cancer Hospital. Additional studies have shown that the effect of NAC plays a key role in the prognosis of gastric cancer patients (90). Initially larger gastric cancer cohort to assess the association between LIMA1 expression and clinico-pathological features and prognosis. A second smaller cohort containing patients receiving NAC was evaluated to explore the role of LIMA1 in response to chemotherapy (14, 80). LIMA1 negatively regulates biological function in gastric cancers requiring NAC and may promote longer overall survival. Moreover, LIMA1 inhibits deep tumor infiltration and promotes differentiation, playing an important role in chemotherapy responsiveness. It is suggested that LIMA1 may be a potential prognostic indicator for gastric cancer.
Therefore, further studies and larger cohorts are necessary in the future the better to understand the role of LIMA1 in gastric cancer, particularly regarding its involvement in chemoresistance and therapeutic response. This will provide new targets for biomarkers or therapeutic strategies related to LIMA1 in gastric cancer.
4.3 LIMA1 and hepatocellular carcinoma
Hepatocellular carcinoma (HCC) is a major global problem, with viral hepatitis B and C infection, alcohol abuse and metabolic disorders being multiple risk factors for the development of cirrhosis and HCC (91). Due to the lack of symptoms in the early stages of HCC, most patients are diagnosed with metastasis in the late stages of the disease (92). Therefore, the need to find promising biomarkers for HCC diagnosis is urgent.
Existing studies suggest that LIMA1 plays an inhibitory role in most malignant tumors, but some studies has been reported that LIMA1 exerts an oncogenic effect in the head and neck tumors (41–43, 45, 81). However, the role and regulatory mechanisms of LIMA1 in hepatocellular carcinoma are still not well understood, and its biological function and clinical value need to be further explored. Increasing research evidences imply that LIMA1 affects cancer progression by regulating important biological processes such as actin dynamics, cell adhesion and metastasis, angiogenesis and lipid metabolism (5, 13, 14). There are no published articles on the effect of LIMA1 on the malignant phenotype of hepatocellular carcinoma. Our study found that the LIMA1 was highly expressed in hepatocellular carcinoma tissues and cell lines. Overexpression of LIMA1 enhanced the migration and invasion of hepatocellular carcinoma cells, which conflicted with our previous knowledge of LIMA1. Subsequently, we will focus on the specific mechanisms of how LIMA1 regulates HCC, which may provide novel therapeutic targets for the treatment of HCC patients.
5 Expression of LIMA1 and its regulatory mechanisms in urinary system tumors
Research on LIMA1 in urologic system tumors is currently focused on prostate cancer. Prostate cancer (PCa) is the most common type of solid tumor in men and the second most common cause of cancer-related deaths in men worldwide (93). Survival of PCa patients depends on early disease diagnosis and effective treatment options, however, lack of specificity to serum prostate specific antigen has been shown to lead to overdiagnosis and overtreatment of PCa (94). A growing number of studies have shown consistent results that LIMA1 is a negative regulator of EMT and aggressiveness, and LIMA1 is negatively correlated with PCa progression (11, 41, 42, 81).
Sanders et al. (41) cloned the full-length human LIMA1 cDNA gene into an expression vector and transfected the human prostate cancer cell line PC-3. Overexpression of LIMA1 in PCa resulted in reduced growth potential both in vitro and in vivo, and decreased cell invasiveness and extracellular matrix adhesion in multiple model assays. In addition, Collins et al. (42) generated LIMA1-α overexpression models and confirmed that overexpression of LIMA1-α reduced cell growth, migration and invasion and affected transcription and protein expression of paxillin, focal adhesion kinase (FAK) and tyrosine protein kinase (Src). Several earlier studies found that LIMA1 was associated with cell-cell adhesion through the interaction of cadherin-catenin complexes bound to F-actin (6, 13). Studies have also suggested a possible link between this molecule and Paxillin and a possible role in regulating cell adhesion to the extracellular matrix (51). Thus, it is evident that these studies confirmed the importance of LIMA1 in prostate cancer and further highlighted the importance of LIMA1 in regulating the growth and aggressiveness of prostate cancer cells.
Subsequently, Zhang et al. (11) identified a significant downregulation of LIMA1 after EMT by quantitative proteomics using an experimental model of prostate cancer metastasis. Biochemical and functional analyses showed that LIMA1 was a negative regulator of EMT and PCa cell invasiveness (13). LIMA1 downregulation significantly could disrupt epithelial architecture, induce actin cytoskeleton remodeling, affect specific gene expression profiles, and activate the pro-EMT program (95). In recent years, Wu et al. (81) established androgen-repressed prostate cancer (ARCaP) cells with temporary or permanent knockdown of LIMA1 to determine the function of LIMA1 in PCa EMT and aggressiveness. The use of the ARCaP EMT model was demonstrated by the understanding of LIMA1 biology and the ARCaP model in the discovery of new drugs for the prevention and treatment of prostate cancer metastasis.
Future studies will need to focus on the generation and efficacy of recombinant forms of LIMA1 for the treatment of prostate cancer both in vitro and in vivo, thus facilitating the identification of new “druggable” therapeutic targets for the treatment of metastatic prostate cancer to help design new therapeutic strategies.
6 The expression of LIMA1 and its regulatory mechanisms in breast and reproductive system tumors
6.1 LIMA1 and breast cancer
Breast cancer is the most common malignancy that threatens the health of women worldwide. The incidence of breast cancer is increasing year-on-year, but the prognosis for patients remains pessimistic (96). Jiang et al. (43) first identified downregulation of the LIMA1-α expression in breast cancer cells and tissues. The LIMA1-α expression was associated with reduced growth of breast cancer cells both in vitro and in vivo and inhibited cell migration and invasiveness by relying on the ERK1/2 signaling pathway. Previous studies have shown that LIMA1 acts as a link between the E-Cadherin-β-Catenin -α-Catenin complex and actin filaments to maintain epithelial phenotype, stabilize the actin cytoskeletal network, and maintain functional epithelial junctions (23, 31). EGF phosphorylates LIMA1 thereby leading to LIMA1 degradation. Furthermore, there is a clear clinical correlation between the LIMA1 expression and tumor grade, lymph node status and tumor stage of breast cancers (43). It is evident that LIMA1 is a negative regulator of breast cancer cell migration. Upregulation of LIMA1 leads to the suppression of cell invasion ability, migration ability and growth rate in vitro and in vivo. Thus, it is hypothesized that LIMA1 is an important prognostic indicator and could be considered as an important target during targeted breast cancer therapy in the future.
6.2 LIMA1 and epithelial ovarian cancer
Epithelial ovarian cancer (EOC) is one of the most lethal gynecological malignancies. It is diagnosed at the late stage in most women which explains the poor prognosis of this malignancy (97). Liu et al. (44) used reverse transcription-polymerase chain reaction (RT-PCR) and immune-histochemistry (IHC) methods to validate the downregulation of the LIMA1-α expression in human ovarian cancer tissues and cell lines at the mRNA and protein levels. Compared with control cells, the knockdown of LIMA1-α caused a significant increase in ovarian cancer cell growth, adhesion, invasion and migration ability. The inhibitory effect of LIMA1 on ovarian cancer cell growth is in line with findings in breast cancer (43), prostate cancer (41, 42), esophageal cancer (79), and endothelial cell lines (54, 55). This was the first article to confirm the LIMA1-α expression in human epithelial ovarian cancer tissues and its effect on the biological behavior of epithelial ovarian cancer cell lines (44).The precise molecular mechanism by which LIMA1-α inhibits the epithelial ovarian cancer phenotype remains unknown. However, relevant studies have provided conclusive evidence that reduction of LIMA1 has the potential to regulate cancer cell migration and invasion by disrupting cell-cell adhesion of adherens junctions, reducing E-cadherin expression and enhancing the EMT-like phenotype (31, 35). These findings suggest that prevention of LIMA1 degradation or partial restoration of the LIMA1 expression may be a novel strategy for the treatment of invasive ovarian cancer growth and metastasis. LIMA1 has the potential to be used as a prognostic predictor and this molecule, in patients with epithelial ovarian cancer, acts as a protective factor.
7 Expression of LIMA1 and its regulatory mechanisms in the head and neck tumors
Oral cancer is the most common tumor of the head and neck region and is the sixth most common tumor worldwide (98). The incidence of oral cancer has increased significantly due to the oncogenic effect of human papillomavirus (HPV). It has one of the highest mortality rates and this has remained the case for more than 20 years (99). Given its difficulty in early diagnosis, susceptibility to metastasis, and poor prognosis, many studies aimed to find more sensitive, specific, and valuable tumor markers. LIMA1 was initially found to be a downregulated gene in oral cancer (8). Subsequently, Wirsing et al. (100) studied prognostic markers (CALML5, CD59, and LIMA1) selected from pathological profiles in patients with head and neck cancers. They also performed an unbiased analysis of these prognostic markers at the mRNA and protein levels in 121 oral cancer patients. Only CALML5 showed significant prognostic value, while the prognostic value of CD59 and LIMA1 could not be validated in this cohort, emphasizing the necessity to assess the head and neck cancer specificity by subgroup analysis (100).
Notably, another study has identified LIMA1 overexpression in the head and neck squamous cell carcinoma (HNSC) (45). High expression of LIMA1 is associated with carcinogenesis and predicts a poor prognosis, especially in HPV-negative and TP53-mutated HNSC. HPV is involved in 25% of HNSC cases and is strongly associated with prolonged survival in HPV-positive patients (45). The increased levels of TP53 mutation may lead to dysfunction of this gene, which has been shown to have oncogenic effects in several cancer types (101). It has been reported that TP53-induced LIMA1 inhibits cell invasion and that TP53 mutations lead to upregulation of LIMA1 expression levels, leading to EMT and further driving tumor invasion and metastasis (33, 45). DNA demethylation of the LIMA1 promoter region may affect its expression upregulation, positively correlating with tumor metastasis, angiogenesis, and EMT. These could illustrate the heterogeneity of LIMA1 expression and the diversity of its functions, and more detailed studies of specific regulatory mechanisms are needed in the future to provide new insights for the development of new biomarkers and personalized cancer therapy.
8 Expression of LIMA1 and its regulatory mechanisms in other tumors
8.1 LIMA1 and osteosarcoma
Osteosarcoma is the most common primary malignancy among bone tumors, with peak incidence concentrated in children and adolescents (102). Osteosarcoma has a high propensity for local invasion and metastasis (103). Seong et al. (82) identified genes differentially regulated by special AT-rich-binding protein 2 (SATB2), including LIMA1, by microarray analysis. Silencing LIMA1 expression resulted in reduced cell adhesion and increased stress fibers in knockdown SATB2 cells compared with control cells, partially rescuing the reduced invasive phenotype of knockdown SATB2. It is evident that SATB2-mediated invasion may be due to interference with LIMA1 expression, which is a key mediator of SATB2-regulated osteosarcoma invasion. In addition, the novel finding indicated that LIMA1 regulated paxillin levels and phosphorylation (82) and phosphorylated paxillin was known to affect variations of adherent patches (30, 51). These suggest that LIMA1 may regulate osteosarcoma invasion by regulating cell adhesion and adherent patch changes.
In future work, there is a need to explore in depth the transcriptional regulatory mechanisms of SATB2 regulatory genes and signaling pathways, including LIMA1 that control the effect of the actin cytoskeleton on motility and invasion. This may help to discover targeting proteins for metastatic osteosarcoma and other cancers with high expression of SATB2.
8.2 LIMA1 and mucosa-associated lymphoid tissue lymphomas
Mucosa-associated Lymphoid Tissue (MALT) lymphoma is a relatively inert non-hodgkin lymphoma (NHL) containing B cells (104). Its pathogenesis progresses in association with chronic inflammation (105). A gene fusion between apoptosisinhibitor-2 (API2) on chromosome 11 and the mucosa-associated lymphoid tissue translocation gene 1 (MALT1) on chromosome 18 formed the API2-MALT1 oncogenic chimera (106). This is the most common chromosomal translocation in MALT lymphomas and has disrupted the function of the MALT1 gene. Using an efficient strategy related to tandem mass spectrometry, Nie et al. (83) identified LIMA1 as a novel interactor and substrate for API2-MALT1 chimeric cystathionase. Interestingly, it was also shown that API2-MALT1-mediated protein hydrolysis produced a LIM domain-only containing oncogenic characteristic fragments both in vivo and in vitro (83), implying that API2-MALT1 converted LIMA1 into an oncogenic LIM domain-only-like protein in MALT lymphoma.
The oncogenicity of B cells was observed through LIMA1-α cleavage and RNA deletion that can be mediated by API2-MALT1 (107). This suggests that LIMA1-α functions as a putative tumor suppressor in B cells akin to its inhibitory function in epithelial cells. Overall, specific inhibition of the interaction between API2 and LIMA1 may facilitate the development of targeted therapies against API2-MALT1 positive lymphomas.
8.3 LIMA1 and diffuse large B-cell lymphoma
Diffuse large B-cell lymphoma (DLBCL) is the most common subtype of aggressive lymphoma. DLBCL is frequently found in most cases of NHL that are aggressive or moderate to highly malignant (108). The pathology of DLBCL is characterized by a diffuse growth of large lymphocytes and a characteristic distribution of tumor cells in the perivascular space (109).
Based on miRNA analysis of DLBCL, Kwanhian et al. (110) identified microRNA-142 (miR-142) as the human microRNA gene that was consistently mutated in 20% of cases with primary DLBCL. Deletion of miR-142 in knockout mice has been reported to result in dysregulated lymphangiogenesis and immunodeficiency (111). Menegatti et al. (84) validated the effect of miR-142 inactivation on protein expression in DLBCL by CRISPR/Cas9 knockdown of miR-142 in DLBCL cell lines BJAB and SUDHL4. MiR-142 knockdown induced a consistent upregulation of genes or proteins that were oncogenic and a downregulation of genes or proteins associated with immunodeficiency responses required for MHC-I presentation. Among them, CCNB1, LIMA1, and TFRC were identified as potential targets and novel targets for miR-142 knockdown cell lines (84). The role of miRNAs to influence cellular transcriptional regulatory networks was further enhanced.
9 Conclusions and future prospects
Notably, using human tumor specimens as the “gold standard”, the EPLIN expression was found to be negatively correlated with clinical lymph node metastasis in a variety of solid tumors (Table 3). The role of LIMA1 in malignancy progression has expanded from a tumor suppressor that acts primarily in early disease stages to a metastasis suppressor that may act in late stages to prevent and delay the invasion and spread of primary cancer cells.
With the deepening of the LIMA1 gene research, LIMA1 has been recognized as being actively involved in cancer cell signaling, possibly through multiple protein interactions associated with cancer progression, in addition to its function as a structural protein. Moreover, LIMA1 is also involved in apical elimination, cilia growth, cholesterol uptake, cellular metabolism, angiogenesis, and endothelial cell dynamics. This provides an additional avenue to explore the implications and mechanisms of LIMA1 in a broader context. Expanding our exploration of multiple areas of this important molecule, LIMA1, in the future will increase its therapeutic potential and help design new metastatic disease prevention and treatment.
Author contributions
XW and CZ drafted and revised the original draft preparation. HS and JY revised the manuscript. XZ and YY collected relevant papers. LZ and JH reviewed the article. XW designed tables and charts. All authors contributed to the article and approved the submitted version.
Funding
This article was supported by the National Natural Science Foundation of China (Grant No. 82073090), Human Resources and Social Security Department System of Shanxi Province (Grant No. 20210001), Research Project Supported by Shanxi Scholarship Council of China (Grant No. 2021-116), Shanxi ‘136’ Leading Clinical Key Specialty (Grant No. 2019XY002), and Shanxi Provincial Key Laboratory of Hepatobiliary and Pancreatic Diseases (under construction).
Conflict of interest
The authors declare that the research was conducted in the absence of any commercial or financial relationships that could be construed as a potential conflict of interest.
Publisher’s note
All claims expressed in this article are solely those of the authors and do not necessarily represent those of their affiliated organizations, or those of the publisher, the editors and the reviewers. Any product that may be evaluated in this article, or claim that may be made by its manufacturer, is not guaranteed or endorsed by the publisher.
Glossary
References
1. Yin LM, Schnoor M, Jun CD. Structural characteristics, binding partners and related diseases of the calponin homology (CH) domain. Front Cell Dev Biol (2020) 8:342. doi: 10.3389/fcell.2020.00342
2. Davidson AJ, Wood W. Unravelling the actin cytoskeleton: a new competitive edge? Trends Cell Biol (2016) 26:569–76. doi: 10.1016/j.tcb.2016.04.001
3. Lappalainen P, Kotila T, Jegou A, Romet-Lemonne G. Biochemical and mechanical regulation of actin dynamics. Nat Rev Mol Cell Biol (2022) 23:836–52. doi: 10.1038/s41580-022-00508-4
4. Smith MA, Hoffman LM, Beckerle MC. LIM proteins in actin cytoskeleton mechanoresponse. Trends Cell Biol (2014) 24:575–83. doi: 10.1016/j.tcb.2014.04.009
5. Collins RJ, Jiang WG, Hargest R, Mason MD, Sanders AJ. EPLIN: a fundamental actin regulator in cancer metastasis? Cancer Metastasis Rev (2015) 34:753–64. doi: 10.1007/s10555-015-9595-8
6. Maul RS, Song Y, Amann KJ, Gerbin SC, Pollard TD, Chang DD. EPLIN regulates actin dynamics by cross-linking and stabilizing filaments. J Cell Biol (2003) 160:399–407. doi: 10.1083/jcb.200212057
7. Han MY, Kosako H, Watanabe T, Hattori S. Extracellular signal-regulated kinase/mitogen-activated protein kinase regulates actin organization and cell motility by phosphorylating the actin cross-linking protein EPLIN. Mol Cell Biol (2007) 27:8190–204. doi: 10.1128/MCB.00661-07
8. Chang DD, Park NH, Denny CT, Nelson SF, Pe M. Characterization of transformation related genes in oral cancer cells. Oncogene (1998) 16:1921–30. doi: 10.1038/sj.onc.1201715
9. Maul RS, Chang DD. EPLIN, epithelial protein lost in neoplasm. Oncogene (1999) 18:7838–41. doi: 10.1038/sj.onc.1203206
10. Chen S, Maul RS, Kim HR, Chang DD. Characterization of the human EPLIN (Epithelial protein lost in neoplasm) gene reveals distinct promoters for the two EPLIN isoforms. Gene (2000) 248:69–76. doi: 10.1016/s0378-1119(00)00144-x
11. Zhang S, Wang X, Osunkoya AO, Iqbal S, Wang Y, Chen Z, et al. EPLIN downregulation promotes epithelial-mesenchymal transition in prostate cancer cells and correlates with clinical lymph node metastasis. Oncogene (2011) 30:4941–52. doi: 10.1038/onc.2011.199
12. Abe K, Takeichi M. EPLIN mediates linkage of the cadherin catenin complex to f-actin and stabilizes the circumferential actin belt. Proc Natl Acad Sci USA (2008) 105:13–9. doi: 10.1073/pnas.0710504105
13. Wu D. Epithelial protein lost in neoplasm (EPLIN): beyond a tumor suppressor. Genes Dis (2017) 4:100–7. doi: 10.1016/j.gendis.2017.03.002
14. Zeng J, Jiang WG, Sanders AJ. Epithelial protein lost in neoplasm, EPLIN, the cellular and molecular prospects in cancers. Biomolecules (2021) 11(7):1038. doi: 10.3390/biom11071038
15. Huang Z, Yu C, Yu L, Shu H, Zhu X. The roles of FHL3 in cancer. Front Oncol (2022) 12:887828. doi: 10.3389/fonc.2022.887828
16. Wei X, Zhang H. Four and a half LIM domains protein 1 can be as a double-edged sword in cancer progression. Cancer Biol Med (2020) 17:270–81. doi: 10.20892/j.issn.2095-3941.2019.0420
17. Maul RS, Sachi GC, Chang DD. Characterization of mouse epithelial protein lost in neoplasm (EPLIN) and comparison of mammalian and zebrafish EPLIN. Gene (2001) 262:155–60. doi: 10.1016/s0378-1119(00)00540-0
18. Wang H, Wang H, Zhu Z, Yang S, Feng S, Li K. Characterization of porcine EPLIN gene revealed distinct expression patterns for the two isoforms. Anim Biotechnol (2007) 18:101–8. doi: 10.1080/10495390600864660
19. Miyazaki S, Funamoto T, Sekimoto T, Kurogi S, Ohta T, Nagai T, et al. EPLINbeta is involved in the assembly of cadherin-catenin complexes in osteoblasts and affects bone formation. Acta Histochem Cytochem (2022) 55:99–110. doi: 10.1267/ahc.22-00027
20. Song Y, Maul RS, Gerbin CS, Chang DD. Inhibition of anchorage-independent growth of transformed NIH3T3 cells by epithelial protein lost in neoplasm (EPLIN) requires localization of EPLIN to actin cytoskeleton. Mol Biol Cell (2002) 13:1408–16. doi: 10.1091/mbc.01-08-0414
21. Ohoka A, Kajita M, Ikenouchi J, Yako Y, Kitamoto S, Kon S, et al. EPLIN is a crucial regulator for extrusion of RasV12-transformed cells. J Cell Sci (2015) 128:781–9. doi: 10.1242/jcs.163113
22. Leitner L, Shaposhnikov D, Descot A, Hoffmann R, Posern G. Epithelial protein lost in neoplasm alpha (Eplin-alpha) is transcriptionally regulated by G-actin and MAL/MRTF coactivators. Mol Cancer (2010) 9:60. doi: 10.1186/1476-4598-9-60
23. Taguchi K, Ishiuchi T, Takeichi M. Mechanosensitive EPLIN-dependent remodeling of adherens junctions regulates epithelial reshaping. J Cell Biol (2011) 194:643–56. doi: 10.1083/jcb.201104124
24. Chen NP, Uddin B, Hardt R, Ding W, Panic M, Lucibello I, et al. Human phosphatase CDC14A regulates actin organization through dephosphorylation of epithelial protein lost in neoplasm. Proc Natl Acad Sci U S A (2017) 114:5201–6. doi: 10.1073/pnas.1619356114
25. Goncalves J. LUZP1: a new player in the actin-microtubule cross-talk. Eur J Cell Biol (2022) 101:151250. doi: 10.1016/j.ejcb.2022.151250
26. Goncalves J, Sharma A, Coyaud E, Laurent E, Raught B, Pelletier L. LUZP1 and the tumor suppressor EPLIN modulate actin stability to restrict primary cilia formation. J Cell Biol (2020) 219(7):e201908132. doi: 10.1083/jcb.201908132
27. Duraivelan K, Samanta D. Emerging roles of the nectin family of cell adhesion molecules in tumour-associated pathways. Biochim Biophys Acta Rev Cancer (2021) 1876:188589. doi: 10.1016/j.bbcan.2021.188589
28. Honig B, Shapiro L. Adhesion protein structure, molecular affinities, and principles of cell-cell recognition. Cell (2020) 181:520–35. doi: 10.1016/j.cell.2020.04.010
29. Karakose E, Geiger T, Flynn K, Lorenz-Baath K, Zent R, Mann M, et al. The focal adhesion protein PINCH-1 associates with EPLIN at integrin adhesion sites. J Cell Sci (2015) 128:1023–33. doi: 10.1242/jcs.162545
30. Tsurumi H, Harita Y, Kurihara H, Kosako H, Hayashi K, Matsunaga A, et al. Epithelial protein lost in neoplasm modulates platelet-derived growth factor-mediated adhesion and motility of mesangial cells. Kidney Int (2014) 86:548–57. doi: 10.1038/ki.2014.85
31. Linklater ES, Duncan ED, Han KJ, Kaupinis A, Valius M, Lyons TR, et al. Rab40-Cullin5 complex regulates EPLIN and actin cytoskeleton dynamics during cell migration. J Cell Biol (2021) 220(7):e202008060. doi: 10.1083/jcb.202008060
32. Zhang S, Wang X, Iqbal S, Wang Y, Osunkoya AO, Chen Z, et al. Epidermal growth factor promotes protein degradation of epithelial protein lost in neoplasm (EPLIN), a putative metastasis suppressor, during epithelial-mesenchymal transition. J Biol Chem (2013) 288:1469–79. doi: 10.1074/jbc.M112.438341
33. Ohashi T, Idogawa M, Sasaki Y, Tokino T. P53 mediates the suppression of cancer cell invasion by inducing LIMA1/EPLIN. Cancer Lett (2017) 390:58–66. doi: 10.1016/j.canlet.2016.12.034
34. Nguyen D, Yang K, Chiao L, Deng Y, Zhou X, Zhang Z, et al. Inhibition of tumor suppressor p73 by nerve growth factor receptor via chaperone-mediated autophagy. J Mol Cell Biol (2020) 12:700–12. doi: 10.1093/jmcb/mjaa017
35. Steder M, Alla V, Meier C, Spitschak A, Pahnke J, Furst K, et al. DNp73 exerts function in metastasis initiation by disconnecting the inhibitory role of EPLIN on IGF1R-AKT/STAT3 signaling. Cancer Cell (2013) 24:512–27. doi: 10.1016/j.ccr.2013.08.023
36. Nowak E, Bednarek I. Aspects of the epigenetic regulation of EMT related to cancer metastasis. Cells-Basel (2021) 10(12):3435. doi: 10.3390/cells10123435
37. Bracken CP, Goodall GJ. The many regulators of epithelial-mesenchymal transition. Nat Rev Mol Cell Biol (2022) 23:89–90. doi: 10.1038/s41580-021-00442-x
38. Zhitnyak IY, Rubtsova SN, Litovka NI, Gloushankova NA. Early events in actin cytoskeleton dynamics and e-Cadherin-Mediated cell-cell adhesion during epithelial-mesenchymal transition. Cells-Basel (2020) 9(3):578. doi: 10.3390/cells9030578
39. Gundamaraju R, Lu W, Paul MK, Jha NK, Gupta PK, Ojha S, et al. Autophagy and EMT in cancer and metastasis: who controls whom? Biochim Biophys Acta Mol Basis Dis (2022) 1868:166431. doi: 10.1016/j.bbadis.2022.166431
40. Pastushenko I, Blanpain C. EMT transition states during tumor progression and metastasis. Trends Cell Biol (2019) 29:212–26. doi: 10.1016/j.tcb.2018.12.001
41. Sanders AJ, Martin TA, Ye L, Mason MD, Jiang WG. EPLIN is a negative regulator of prostate cancer growth and invasion. J Urol (2011) 186:295–301. doi: 10.1016/j.juro.2011.03.038
42. Collins RJ, Morgan LD, Owen S, Ruge F, Jiang WG, Sanders AJ. Mechanistic insights of epithelial protein lost in neoplasm in prostate cancer metastasis. Int J Cancer (2018) 143:2537–50. doi: 10.1002/ijc.31786
43. Jiang WG, Martin TA, Lewis-Russell JM, Douglas-Jones A, Ye L, Mansel RE. Eplin-alpha expression in human breast cancer, the impact on cellular migration and clinical outcome. Mol Cancer (2008) 7:71. doi: 10.1186/1476-4598-7-71
44. Liu R, Martin TA, Jordan NJ, Ruge F, Ye L, Jiang WG. Epithelial protein lost in neoplasm-alpha (EPLIN-alpha) is a potential prognostic marker for the progression of epithelial ovarian cancer. Int J Oncol (2016) 48:2488–96. doi: 10.3892/ijo.2016.3462
45. Ma W, Liao Y, Gao Z, Zhu W, Liu J, She W. Overexpression of LIMA1 indicates poor prognosis and promotes epithelial-mesenchymal transition in head and neck squamous cell carcinoma. Clin Med Insights Oncol (2022) 16:1363230453. doi: 10.1177/11795549221109493
46. Kon S, Fujita Y. Cell competition-induced apical elimination of transformed cells, EDAC, orchestrates the cellular homeostasis. Dev Biol (2021) 476:112–6. doi: 10.1016/j.ydbio.2021.03.015
47. Pothapragada SP, Gupta P, Mukherjee S, Das T. Matrix mechanics regulates epithelial defence against cancer by tuning dynamic localization of filamin. Nat Commun (2022) 13:218. doi: 10.1038/s41467-021-27896-z
48. Tanimura N, Fujita Y. Epithelial defense against cancer (EDAC). Semin Cancer Biol (2020) 63:44–8. doi: 10.1016/j.semcancer.2019.05.011
49. Saitoh S, Maruyama T, Yako Y, Kajita M, Fujioka Y, Ohba Y, et al. Rab5-regulated endocytosis plays a crucial role in apical extrusion of transformed cells. Proc Natl Acad Sci USA (2017) 114:E2327–36. doi: 10.1073/pnas.1602349114
50. Kadeer A, Maruyama T, Kajita M, Morita T, Sasaki A, Ohoka A, et al. Plectin is a novel regulator for apical extrusion of RasV12-transformed cells. Sci Rep (2017) 7:44328. doi: 10.1038/srep44328
51. Kasai N, Kadeer A, Kajita M, Saitoh S, Ishikawa S, Maruyama T, et al. The paxillin-plectin-EPLIN complex promotes apical elimination of RasV12-transformed cells by modulating HDAC6-regulated tubulin acetylation. Sci Rep (2018) 8:2097. doi: 10.1038/s41598-018-20146-1
52. Shi X, Dong X, Young S, Chen AM, Liu X, Zheng Z, et al. The impact of angiogenesis inhibitors on survival of patients with small cell lung cancer. Cancer Med (2019) 8:5930–8. doi: 10.1002/cam4.2462
53. Rochefort P, Chabaud S, Pierga JY, Tredan O, Brain E, Bidard FC, et al. Soluble VE-cadherin in metastatic breast cancer: an independent prognostic factor for both progression-free survival and overall survival. Br J Cancer (2017) 116:356–61. doi: 10.1038/bjc.2016.427
54. Anderson TS, Wooster AL, Piersall SL, Okpalanwaka IF, Lowe DB. Disrupting cancer angiogenesis and immune checkpoint networks for improved tumor immunity. Semin Cancer Biol (2022) 86:981–96. doi: 10.1016/j.semcancer.2022.02.009
55. Chervin-Petinot A, Courcon M, Almagro S, Nicolas A, Grichine A, Grunwald D, et al. Epithelial protein lost in neoplasm (EPLIN) interacts with alpha-catenin and actin filaments in endothelial cells and stabilizes vascular capillary network. Vitro J Biol Chem (2012) 287:7556–72. doi: 10.1074/jbc.M111.328682
56. Abu TA, Schnittler HJ. Dynamics between actin and the VE-cadherin/catenin complex: novel aspects of the ARP2/3 complex in regulation of endothelial junctions. Cell Adh Migr (2014) 8:125–35. doi: 10.4161/cam.28243
57. Hofer I, Schimp C, Taha M, Seebach J, Aldirawi M, Cao J, et al. Advanced methods for the investigation of cell contact dynamics in endothelial cells using florescence-based live cell imaging. J Vasc Res (2018) 55:350–64. doi: 10.1159/000494933
58. Taha M, Aldirawi M, Marz S, Seebach J, Odenthal-Schnittler M, Bondareva O, et al. EPLIN-alpha and -beta isoforms modulate endothelial cell dynamics through a spatiotemporally differentiated interaction with actin. Cell Rep (2019) 29:1010–26. doi: 10.1016/j.celrep.2019.09.043
59. Sanders AJ, Ye L, Mason MD, Jiang WG. The impact of EPLINalpha (Epithelial protein lost in neoplasm) on endothelial cells, angiogenesis and tumorigenesis. Angiogenesis (2010) 13:317–26. doi: 10.1007/s10456-010-9188-7
60. Liang L, Zhao L, Zan Y, Zhu Q, Ren J, Zhao X. MiR-93-5p enhances growth and angiogenesis capacity of HUVECs by down-regulating EPLIN. Oncotarget (2017) 8:107033–43. doi: 10.18632/oncotarget.22300
61. Ghalehbandi S, Yuzugulen J, Pranjol M, Pourgholami MH. The role of VEGF in cancer-induced angiogenesis and research progress of drugs targeting VEGF. Eur J Pharmacol (2023) 949:175586. doi: 10.1016/j.ejphar.2023.175586
62. Tubon I, Zannoni A, Bernardini C, Salaroli R, Bertocchi M, Mandrioli R, et al. In vitro anti-inflammatory effect of salvia sagittata ethanolic extract on primary cultures of porcine aortic endothelial cells. Oxid Med Cell Longev (2019) 2019:6829173. doi: 10.1155/2019/6829173
63. Maucher D, Schmidt B, Schumann J. Loss of endothelial barrier function in the inflammatory setting: indication for a cytokine-mediated post-transcriptional mechanism by virtue of upregulation of miRNAs miR-29a-3p, miR-29b-3p, and miR-155-5p. Cells (2021) 10(11):2843. doi: 10.3390/cells10112843
64. Rizzelli F, Malabarba MG, Sigismund S, Mapelli M. The crosstalk between microtubules, actin and membranes shapes cell division. Open Biol (2020) 10:190314. doi: 10.1098/rsob.190314
65. Gupta VK, Chaudhuri O. Mechanical regulation of cell-cycle progression and division. Trends Cell Biol (2022) 32:773–85. doi: 10.1016/j.tcb.2022.03.010
66. Chircop M, Oakes V, Graham ME, Ma MP, Smith CM, Robinson PJ, et al. The actin-binding and bundling protein, EPLIN, is required for cytokinesis. Cell Cycle (2009) 8:757–64. doi: 10.4161/cc.8.5.7878
67. Smith TC, Fang Z, Luna EJ. Novel interactors and a role for supervillin in early cytokinesis. Cytoskeleton (Hoboken) (2010) 67:346–64. doi: 10.1002/cm.20449
68. Sundvold H, Sundvold-Gjerstad V, Malerod-Fjeld H, Haglund K, Stenmark H, Malerod L. Arv1 promotes cell division by recruiting IQGAP1 and myosin to the cleavage furrow. Cell Cycle (2016) 15:628–43. doi: 10.1080/15384101.2016.1146834
69. McNiven MA, Ridley AJ. Focus on membrane dynamics. Trends Cell Biol (2006) 16:485–6. doi: 10.1016/j.tcb.2006.08.010
70. Carlton JG, Jones H, Eggert US. Membrane and organelle dynamics during cell division. Nat Rev Mol Cell Biol (2020) 21:151–66. doi: 10.1038/s41580-019-0208-1
71. Duethorn B, Groll F, Rieger B, Drexler H, Brinkmann H, Kremer L, et al. Lima1 mediates the pluripotency control of membrane dynamics and cellular metabolism. Nat Commun (2022) 13:610. doi: 10.1038/s41467-022-28139-5
72. Cao Y. Adipocyte and lipid metabolism in cancer drug resistance. J Clin Invest (2019) 129:3006–17. doi: 10.1172/JCI127201
73. Matey-Hernandez ML, Williams F, Potter T, Valdes AM, Spector TD, Menni C. Genetic and microbiome influence on lipid metabolism and dyslipidemia. Physiol Genomics (2018) 50:117–26. doi: 10.1152/physiolgenomics.00053.2017
74. Hoogeveen RC, Ballantyne CM. Residual cardiovascular risk at low LDL: remnants, lipoprotein(a), and inflammation. Clin Chem (2021) 67:143–53. doi: 10.1093/clinchem/hvaa252
75. Zhang YY, Fu ZY, Wei J, Qi W, Baituola G, Luo J, et al. A LIMA1 variant promotes low plasma LDL cholesterol and decreases intestinal cholesterol absorption. Science (2018) 360:1087–92. doi: 10.1126/science.aao6575
76. Donato LJ. LIMA1: a newly identified player in the field of cholesterol control. Clin Chem (2018) 64:1792–3. doi: 10.1373/clinchem.2018.294645
77. Lim GB. LIMA1 variant influences cholesterol absorption. Nat Rev Cardiol (2018) 15:502. doi: 10.1038/s41569-018-0054-4
78. Su MW, Chang CK, Lin CW, Ling SJ, Hsiung CN, Chu HW, et al. Blood multiomics reveal insights into population clusters with low prevalence of diabetes, dyslipidemia and hypertension. PloS One (2020) 15:e229922. doi: 10.1371/journal.pone.0229922
79. Liu Y, Sanders AJ, Zhang L, Jiang WG. EPLIN-alpha expression in human oesophageal cancer and its impact on cellular aggressiveness and clinical outcome. Anticancer Res (2012) 32:1283–9. doi: 10.1016/j.canlet.2016.12.034
80. Gong W, Zeng J, Ji J, Jia Y, Jia S, Sanders AJ, et al. EPLIN expression in gastric cancer and impact on prognosis and chemoresistance. Biomolecules (2021) 11(4):547. doi: 10.3390/biom11040547
81. Wu D, Osunkoya AO, Kucuk O. Epithelial protein lost in neoplasm (EPLIN) and prostate cancer: lessons learned from the ARCaP model. Am J Clin Exp Urol (2021) 9:264–76. doi: 10.1016/j.gendis.2017.03.002
82. Seong BK, Lau J, Adderley T, Kee L, Chaukos D, Pienkowska M, et al. SATB2 enhances migration and invasion in osteosarcoma by regulating genes involved in cytoskeletal organization. Oncogene (2015) 34:3582–92. doi: 10.1038/onc.2014.289
83. Nie Z, Du MQ, McAllister-Lucas LM, Lucas PC, Bailey NG, Hogaboam CM, et al. Conversion of the LIMA1 tumour suppressor into an oncogenic LMO-like protein by API2-MALT1 in MALT lymphoma. Nat Commun (2015) 6:5908. doi: 10.1038/ncomms6908
84. Menegatti J, Nakel J, Stepanov YK, Caban KM, Ludwig N, Nord R, et al. Changes of protein expression after CRISPR/Cas9 knockout of miRNA-142 in cell lines derived from diffuse Large b-cell lymphoma. Cancers (Basel) (2022) 14(20):5031. doi: 10.3390/cancers14205031
85. Wang J, Ma X, Ma Z, Ma Y, Wang J, Cao B. Research progress of biomarkers for immune checkpoint inhibitors on digestive system cancers. Front Immunol (2022) 13:810539. doi:10.3389/fimmu.2022.810539
86. Zhang Y, Huang S, Yang G, Zou L, Huang X, Liu S. The role of miRNAs during endoplasmic reticulum stress induced apoptosis in digestive cancer. J Cancer (2021) 12:6787–95. doi: 10.7150/jca.62352
87. Lopes N, Correia MP, Henrique R, Jeronimo C. Epigenetic alterations in oesophageal cancer: expression and role of the involved enzymes. Int J Mol Sci (2020) 21(10):3522. doi: 10.3390/ijms21103522
88. Xie J, Fu L, Jin L. Immunotherapy of gastric cancer: past, future perspective and challenges. Pathol Res Pract (2021) 218:153322. doi: 10.1016/j.prp.2020.153322
89. Machlowska J, Baj J, Sitarz M, Maciejewski R, Sitarz R. Gastric cancer: epidemiology, risk factors, classification, genomic characteristics and treatment strategies. Int J Mol Sci (2020) 21(11):4012. doi: 10.3390/ijms21114012
90. Jiang L, Ma Z, Ye X, Kang W, Yu J. Clinicopathological factors affecting the effect of neoadjuvant chemotherapy in patients with gastric cancer. World J Surg Oncol (2021) 19:44. doi: 10.1186/s12957-021-02157-x
91. Fujiwara N, Friedman SL, Goossens N, Hoshida Y. Risk factors and prevention of hepatocellular carcinoma in the era of precision medicine. J Hepatol (2018) 68:526–49. doi: 10.1016/j.jhep.2017.09.016
92. Couri T, Pillai A. Goals and targets for personalized therapy for HCC. Hepatol Int (2019) 13:125–37. doi: 10.1007/s12072-018-9919-1
93. Lorenc T, Klimczyk K, Michalczewska I, Slomka M, Kubiak-Tomaszewska G, Olejarz W. Exosomes in prostate cancer diagnosis, prognosis and therapy. Int J Mol Sci (2020) 21(6):2118. doi: 10.3390/ijms21062118
94. Teng M, Zhou S, Cai C, Lupien M, He HH. Pioneer of prostate cancer: past, present and the future of FOXA1. Protein Cell (2021) 12:29–38. doi: 10.1007/s13238-020-00786-8
95. Brill-Karniely Y, Dror D, Duanis-Assaf T, Goldstein Y, Schwob O, Millo T, et al. Triangular correlation (TrC) between cancer aggressiveness, cell uptake capability, and cell deformability. Sci Adv (2020) 6:x2861. doi: 10.1126/sciadv.aax2861
96. Liang Y, Zhang H, Song X, Yang Q. Metastatic heterogeneity of breast cancer: molecular mechanism and potential therapeutic targets. Semin Cancer Biol (2020) 60:14–27. doi: 10.1016/j.semcancer.2019.08.012
97. Yang WH, Huang Z, Wu J, Ding CC, Murphy SK, Chi JT. A TAZ-ANGPTL4-NOX2 axis regulates ferroptotic cell death and chemoresistance in epithelial ovarian cancer. Mol Cancer Res (2020) 18:79–90. doi: 10.1158/1541-7786.MCR-19-0691
98. Chattopadhyay I, Verma M, Panda M. Role of oral microbiome signatures in diagnosis and prognosis of oral cancer. Technol Cancer Res Treat (2019) 18:1078135002. doi: 10.1177/1533033819867354
99. Warnakulasuriya S, Kerr AR. Oral cancer screening: past, present, and future. J Dent Res (2021) 100:1313–20. doi: 10.1177/00220345211014795
100. Wirsing AM, Bjerkli IH, Steigen SE, Rikardsen O, Magnussen SN, Hegge B, et al. Validation of selected head and neck cancer prognostic markers from the pathology atlas in an oral tongue cancer cohort. Cancers (Basel) (2021) 13(10):2387. doi: 10.3390/cancers13102387
101. Hu J, Cao J, Topatana W, Juengpanich S, Li S, Zhang B, et al. Targeting mutant p53 for cancer therapy: direct and indirect strategies. J Hematol Oncol (2021) 14:157. doi: 10.1186/s13045-021-01169-0
102. Corre I, Verrecchia F, Crenn V, Redini F, Trichet V. The osteosarcoma microenvironment: a complex but targetable ecosystem. Cells-Basel (2020) 9(4):976. doi: 10.3390/cells9040976
103. Chen C, Xie L, Ren T, Huang Y, Xu J, Guo W. Immunotherapy for osteosarcoma: fundamental mechanism, rationale, and recent breakthroughs. Cancer Lett (2021) 500:1–10. doi: 10.1016/j.canlet.2020.12.024
104. Marcelis L, Tousseyn T, Sagaert X. MALT lymphoma as a model of chronic inflammation-induced gastric tumor development. Curr Top Microbiol Immunol (2019) 421:77–106. doi: 10.1007/978-3-030-15138-6_4
105. Kiesewetter B, Raderer M. Immunomodulatory treatment for mucosa-associated lymphoid tissue lymphoma (MALT lymphoma). Hematol Oncol (2020) 38:417–24. doi: 10.1002/hon.2754
106. Izumi K, Nishikori M, Yuan H, Otsuka Y, Nakao K, Takaori-Kondo A. Establishment and characterization of a MALT lymphoma cell line carrying an API2-MALT1 translocation. Genes Chromosomes Cancer (2020) 59:517–24. doi: 10.1002/gcc.22855
107. Dotlic S, Gasparov S, Lovric E, Dominis M, Korac P. Is it possible to overcome antiapoptotic API2/MALT1 events in tumor b-cells by influencing tregs in MALT lymphoma? Med Hypotheses (2012) 79:500–3. doi: 10.1016/j.mehy.2012.07.001
108. Miao Y, Medeiros LJ, Li Y, Li J, Young KH. Genetic alterations and their clinical implications in DLBCL. Nat Rev Clin Oncol (2019) 16:634–52. doi: 10.1038/s41571-019-0225-1
109. Modi D, Potugari B, Uberti J. Immunotherapy for diffuse large b-cell lymphoma: current landscape and future directions. Cancers (Basel) (2021) 13(22):5827. doi: 10.3390/cancers13225827
110. Kwanhian W, Lenze D, Alles J, Motsch N, Barth S, Doll C, et al. MicroRNA-142 is mutated in about 20% of diffuse large b-cell lymphoma. Cancer Med (2012) 1:141–55. doi: 10.1002/cam4.29
Keywords: actin cytoskeleton, LIMA1, cells migration and metastatic, EMT, angiogenesis, cancer progression
Citation: Wang X, Zhang C, Song H, Yuan J, Zhang X, Yuan Y, Zhang L and He J (2023) Characterization of LIMA1 and its emerging roles and potential therapeutic prospects in cancers. Front. Oncol. 13:1115943. doi: 10.3389/fonc.2023.1115943
Received: 04 December 2022; Accepted: 02 May 2023;
Published: 19 May 2023.
Edited by:
Michelle L. Matter, Tulane University, United StatesReviewed by:
Yukio Ago, Hiroshima University, JapanAntonina Alexandrova, Russian Cancer Research Center NN Blokhin, Russia
Nicholas Anagnou, Biomedical Research Foundation of the Academy of Athens (BRFAA), Greece
Copyright © 2023 Wang, Zhang, Song, Yuan, Zhang, Yuan, Zhang and He. This is an open-access article distributed under the terms of the Creative Commons Attribution License (CC BY). The use, distribution or reproduction in other forums is permitted, provided the original author(s) and the copyright owner(s) are credited and that the original publication in this journal is cited, in accordance with accepted academic practice. No use, distribution or reproduction is permitted which does not comply with these terms.
*Correspondence: Jiefeng He, hejiefeng2005@163.com; Lei Zhang, zhangl7803@126.com
†These authors have contributed equally to this work and share first authorship