- 1State Key Laboratory for Managing Biotic and Chemical Threats to the Quality and Safety of Agro-products, Ningbo University, Ningbo, China
- 2Laboratory of Biochemistry and Molecular Biology, School of Marine Sciences, Ningbo University, Ningbo, China
- 3Key Laboratory of Applied Marine Biotechnology of Ministry of Education, Ningbo University, Ningbo, China
Breast cancer (BC) is a common malignancy that mainly occurred in women and it has become the most diagnosed cancer annually since 2020. Berberine (BBR), an alkaloid extracted from the Berberidacea family, has been found with broad pharmacological bioactivities including anti-inflammatory, anti-diabetic, anti-hypertensive, anti-obesity, antidepressant, and anticancer effects. Mounting evidence shows that BBR is a safe and effective agent with good anticancer activity against BC. However, its detailed underlying mechanism in BC treatment remains unclear. Here, we will provide the evidence for BBR in BC therapy and summarize its potential mechanisms. This review briefly introduces the source, metabolism, and biological function of BBR and emphasizes the therapeutic effects of BBR against BC via directly interacting with effector proteins, transcriptional regulatory elements, miRNA, and several BBR-mediated signaling pathways. Moreover, the novel BBR-based therapeutic strategies against BC improve biocompatibility and water solubility, and the efficacies of BBR are also briefly discussed. Finally, the status of BBR in BC treatment and future research directions is also prospected.
Introduction
Breast cancer (BC) is one of the most common cancers in women and is characterized by a malignant proliferation of mammary tissue (1–3). In 2020, BC has overtaken lung cancer and become the leading diagnosed and death-causing cancer among women all over the world (4–7). BC is a heterogeneous disease and it can be classified into different subtypes based on diverse molecular biomarkers (8). According to the statuses of four molecular biomarkers, namely, estrogen receptor (ER), progesterone receptor (PR), human epidermal growth factor receptor 2 (HER2), and Ki67, BC can be classified into four main intrinsic subtypes: luminal A, luminal B, HER2-enriched, and triple-negative (1, 8). Luminal A subtype is characterized with ER+/PR+/HER2-/Ki-67low biomarkers and low recurrence risk and favorable prognosis. Luminal B subtype is characterized with ER+/PR-/HER2+/Ki-67high biomarkers. HER2-enriched is the third subtype of BC characterized with ER-/PR-/HER2+ biomarkers. Triple-negative BC (TNBC) is the subtype of BC with ER-/PR-/HER2- biomarkers and the poorest prognosis in clinical studies (7–11).
Currently, there are many methods comprehensively and extensively used in BC therapy. Surgery, radiotherapy, and chemotherapy are the most common therapeutic strategies used to treat all the subtypes of BC in clinic, but most patients would develop drug resistance or relapse later. For the moment, endocrine or single-targeted therapy only has been approved to treat non-TNBC in clinic, and most of them showed poor prognosis and recurrence due to tumor metastasis and drug resistance (11–13), suggesting that a portion of patients develop medicine resistance and experience severe side effects after Western medicine treatment (14). For TNBC, although many novel target therapeutic strategies have been identified and their modulators exhibited good anti-BC activities in vitro and in vivo, none of them have been approved in clinic due to poor efficacy in clinical trials and potent side effects (11). Therefore, it is extremely eager to seek a more effective conservative and multi-target therapeutic agent that could treat cancer without residual symptoms to patients. Traditional Chinese medicine (TCM) as a therapeutic strategy for varieties of diseases has been used for thousands of years and shows remarkable validation in many diseases. With the development of standardization of TCM, some extracted monomers also show excellent efficacy against different diseases. Among them, alkaloid berberine (BBR) exhibited good efficacy against BC. Here, we summarize the source and biological function of BBR, action mechanisms, and novel therapeutic strategies using BBR against BC. Moreover, the strategies to improve the efficacies of BBR against human BC are also briefly discussed.
The overview of BBR
BBR, a pentacyclic isoquinoline compound with a relative molecular weight of 336.37 (Figure 1), is a bioalkaloid initially found in the rhizome, bark, and other structures of Chinese herb Coptis chinensis Franch, and some berberis plants mainly including Berberis aristate DC., Berberis darwinii Hook, and Berberis vulgaris L (15–17). The later study demonstrated that BBR could be also extracted from some other plants (18). With the aid of UDG glucuronosyltransferase and cytochrome (19), BBR could be transformed into four major types of metabolites: berberrubine, demethyleneberberine, thalifendine, and jatrorrhizine in the liver and intestine in vivo (20, 21). Interestingly, the type and quantity of metabolites the metabolites are different in distinct species (22).
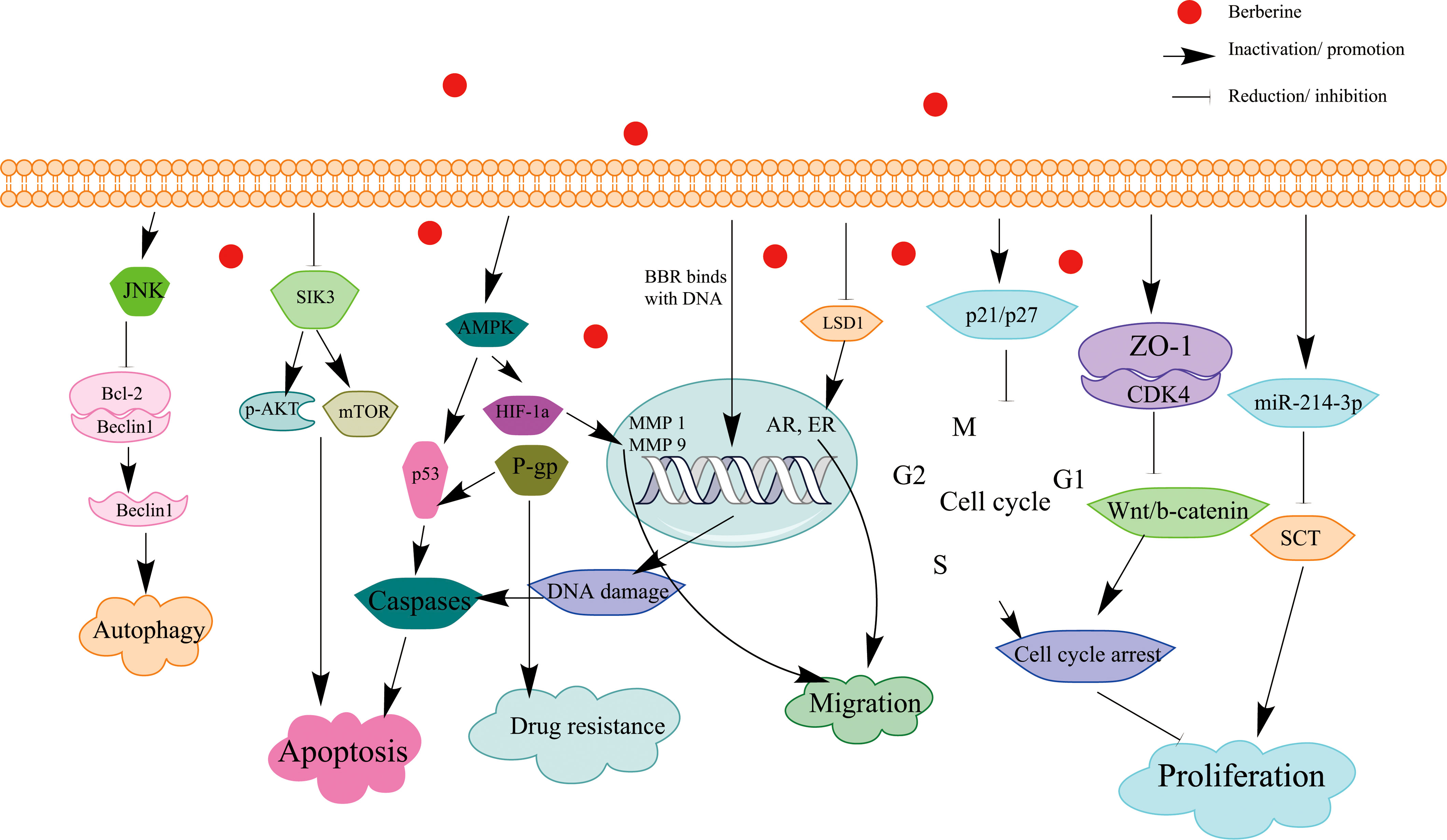
Figure 1 Schematic illustration of the effect of BBR on signaling pathways of apoptosis and cell cycle arrest in BC.
BBR, a traditional Chinese medicine, has been used to clear heat and detoxify toxins, promote blood circulation and remove blood stasis, and dispel dampness and cold for over one thousand years (23). Recently, a substantial body of studies showed that BBR has diverse pharmacological effects including anti-hypertensive, anti-oxidative, anti-inflammatory, anti-diabetic, immunosuppressive (24), anti-cardiovascular (21), and neuroprotective activities (3, 6, 25, 26). Therefore, BBR was widely used to treat lots of infectious, metabolic, cardiovascular, and neurological diseases. Notably, BBR also could prevent and treat some different cancers triggered by gene mutations and agricultural hazard factors (27). The mounting evidence suggested that BBR inhibited proliferation as well as induced apoptosis and cell cycle arrest in different cancer cell lines without significant cytotoxicity effect on most non-malignant epithelial cell lines (28–31). Due to its good activity to prevent, inhibit, and reverse the progression of varieties of cancers, BBR has received remarkable interest as a potential anticancer agent in the treatment of many cancers, especially for BC (32).
The molecular targets of BBR against BC
BBR is a multi-target drug that has been proved effective in treating many cancers and inflammatory diseases. It has been found to combat different cancers via binding to diverse molecular targets due to the heterogeneity among different cancers or different subtypes of same cancer. In BC, BBR has also been found to directly bind several target proteins and DNA sequences to exhibit its anticancer activity.
SIK3
Salt-inducible kinases 3 (SIK3) is an oncogene that plays an important role in BC cells (33). The overexpression of SIK3 promotes BC cell proliferation and growth via regulating the cell cycle (34). Meanwhile, BBR is found to function as a SIK3 inhibitor through binding its ATP-binding pocket via hydrogen bonding. Further study found that BBR exhibited its anticancer activity partially via binding to SIK3 and inducing cell cycle arrest at G1/S cell cycle arrest and apoptosis in BC cells (35).
Ephrin-B2
Ephrin-B2, a single transmembrane cell surface protein (36), is one of the membrane-bound ligands of the eph receptor family (37). The binding of eph receptor and ephrin-B2 in the membrane activates ephrin-B2 signaling (38). This signaling promotes the cancer cell survival and migration via conveying both receptor-expressing cells and ligand-expressing cells (39–41). Ma et al. identified that BBR could directly bind ephrin-B2 and significantly reduce ephrin-B2 level and its downstream proteins (42). The result showed that BBR inhibits the BC survival and migration via targeting ephrin-B2 (42).
LSD1
Histone lysine demethylase 1 (LSD1) is a histone demethylase selectively eliminating the methyl group from histone H3 at H3K4me1/2 and H3K9me1/2 and modulating the transcriptional repression and activation of downstream genes (43, 44). Meanwhile, BC metastasis is associated with the phosphorylation of LSD1 (45). Several studies demonstrated that the stability and degradation of LSD1 could be regulated by ubiquitination and phosphorylation modifications (46). To be specific, reducing ubiquitination levels by deubiquitinase or raising phosphorylation of LSD1 by kinases could inhibit its degradation and increase its stability via reducing its ubiquitination level (47). Given that LSD1 could also function as a coactivator via demethylating H3K9me1/2 and activating transcription by interacting with androgen receptor (AR) and ER, two receptors that mediated the BC progression (48, 49), it is identified as a promising target for BC therapy (49). As a mainly bioactive product of C. chinensis Franch, BBR has exhibited its anticancer activity via directly binding to LSD1, inducing the accumulation H3K9me1/2 and suppressing downstream suppressor genes in BC (50–52). Hence, BBR might exhibit its anticancer effect via partially targeting LSD1 in BC.
DNA, TATA box, and poly(A) tails
The nitrogen atom at the 7-position of BBR has a positive charge and this exceptional structure could strongly bind with DNA sequences with negative charge (Figure 1) (53, 54). The binding between BBR and DNA would lead to DNA damage in cancer cells via regulating the cellular DNA topoisomerase activity (55). Caspase-3 and caspase-9 are proteolytic enzymes that are activated by apoptotic factors, including some target cellular enzymes such as Fas ligand (FasL). BBR could induce the expressions of these apoptotic factors to activate caspase-9 and caspase-3 (56). DNA damage response (DDR) induces cell cycle arrest and apoptosis through the caspase-9 and caspase-3 or Fas/FasL signaling pathway when DNA damage cannot be successfully repaired in time (55, 57) and promotes autophagy to demonstrate its qualities to suppress tumor (58, 59). Therefore, BBR-mediated DDR of cancer cells is also a prospective protein treatment.
Additionally, TATA box is an element that takes part in the process of gene transcription (60). Some results indicated that DNA TATA box and mRNA poly (A) tails played a vital part in the modulation of gene expression, and they are the first and second mainly important targets of BBR to regulate gene transcript (61). In the mechanism, the binding between BBR and TATA box/mRNA poly(A) tails would alter the spatial conformation of these DNA/RNA sequences (60, 61), regulate the transcription of downstream genes, and thus inhibit the BC progression (62). Thus, TATA boxes and poly(A) tails also partially contribute to the anti-BC activity of BBR.
MicroRNA (miR)-214-3p and SCT
MiR-214-3p is a tumor suppressor functioning by inducing cell apoptosis and G2/M arrest of BC cells (63). Secretin (SCT) is an oncogene with an anti-proliferative effect in normal cells but a proliferation-stimulating activity in cancer cells (64). BBR was also found to exhibit its antitumor effects in MCF-7 and MDA-MB-231 BC cells via upregulating tumor suppressor microRNA (miR)-214-3p and reducing SCT level (63, 64). Further study showed that BBR exhibited its anti-BC activities via directly binding to targeted binding sites within both miR-214-3p and SCT (65). In addition, BBR could raise the miR-214-3p expression and reduce SCT level via β-catenin–mediated inactivation of telomerase activity (66).
The BBR-mediated pathways of against BC
BBR suppresses proliferation and migration of BC cells via ZO-1 mediated Wnt/β-catenin signaling pathway
Zonula occludence-1 (ZO-1) is a key molecule tightly attached to other proteins, such as Wnt/β-catenin. Wnt/β-catenin is a membrane-linked protein that could be activated via dissociating from the membrane. The activation of Wnt/β-catenin signaling promotes tumor cell proliferation and migration (67). ZO-1 was found to be activated by BBR, which lead to enhancing the binding of ZO-1 and the cyclin-dependent kinase 4 (CDK4) and reducing CDK4 entry into the nucleus (68, 69). Then, the free Wnt/β-catenin level was also downregulated, which suppresses cancer cell proliferation via induced cell cycle arrest (70, 71). The present study also demonstrated that BBR inhibited the proliferation and migration of BC cells partially via inactivating the ZO-1–mediated Wnt/β-catenin signaling pathway.
BBR induced cell cycle arrest of BC cells through upregulating p21 and p27
P21 and p27 are the key CDK inhibitors (CDKIs) that induce apoptosis and cell cycle arrest in varieties of cells (72). The increase of mRNA and protein expression levels of p21 and p27 is beneficial to inhibit the expressions of diverse cyclins including cyclin D1, cyclin E, cyclin K2, cyclin K4, and cyclin K6 (73). The CDKIs would modulate cell cycle arrest in the G1 phase and induce apoptosis in different cancer cells.
Several studies proved that BBR could modulate different tumor suppressor genes (including p21 and p27) to induce cytotoxicity in BC cells, and reducing the two protein levels strongly clocks up the growth of different tumor types including BC (73). Further study indicated that BBR could induce G1 phase arrest and thus inhibit cell proliferation via upregulating p21 and p27 (74).
BBR sensitizes chemical agents and overcomes drug resistance of BC cells via activating AMPK signaling
Adenosine monophosphate–activated kinase (AMPK), a sensor of energy status, plays an extremely primary part in cellular energy homeostasis and regulates the drug resistance of BC (75, 76). It is activated by hypoxia to compensate for the oxygen reduced by mitochondria respiration (77). Hypoxia-inducible factor-1alpha (HIF-1α), the primary regulator of cell response to hypoxia (78, 79), has the function to increase proliferation and drug resistance of some cancers including BC via regulating metabolic enzymes (80, 81). P-glycoprotein (P-gp) is a critical obstacle to reduce drug accumulation in cancer eradication (82). As a tumor suppressor protein (83), p53 can be activated by being modified by the phosphorylation of multiple protein kinases at multiple sites (84). Interestingly, HIF-1α, P-gp, and p53 are downstream genes of AMPK signaling, and BBR exhibits its anti-BC activity via AMPK-mediated reducing level of HIF-1α and P-gp and phosphorylating p53 (85, 86).
Doxorubicin (DOX) is the most frequently used chemical agent to treat BC in clinic. However, its effectiveness is often reduced in a hypoxic environment (87). Pan et al. found that the activation of AMPK signaling was responsible for lowering the sensitivity of BC cells to DOX. To be specific, AMPK was activated by a hypoxic environment, which upregulated the phosphorylated AMPK (p-AMPK) and HIF-1α. Then, HIF-1α raised P-gp level and thus improved the DOX sensitivity of BC cells. BBR also could overcome DOX resistance via AMPK in a time- and dose-dependent manner. Further study showed that low-dose BBR enhanced cytotoxicity and sensitized DOX sensitivity in vivo via inhibiting the AMPK pathway, whereas high-dose BBR would restrain the activation of AMPK and affect HIF-1α downregulation, which induces p53 activation that led to cell death and apoptosis (88). In conclusion, BBR enhanced sensitivity to chemical agents and drug resistance of BC mainly through activating AMPK signaling cascades (89).
BBR inhibits TPA-induced PKC-α signaling and thus reduces the levels of MMP-1 and MMP-9 in BC
Phorbol ester 12-O-tetradecanoylphorbol-13-acetate (TPA) was found to promote tumor invasion and migration in BC (90). Matrix metalloproteinases (MMPs) are key enzymes that could regulate the cellular microenvironment (91). Matrix metalloproteinase-1 (MMP-1) is the principal LCC-secreted factor that enhances the tumor-promoting traits (92). Matrix metalloproteinase-9 (MMP-9) is an enzyme which belongs to the MMP family, and its activity was related with different stages of carcinoma progression (93, 94). Several studies showed that the MMP-9 level is positively correlated with a higher tumor grade in BC tissue (95), and TPA mediated tumor invasion and migration by upregulating expression of MMP-1 and MMP-9 in BC cells (96, 97). Protein kinases C (PKCs) are a set of serine/threonine kinases involved in the tumor progression in BC (98). Protein kinase C (PKC-α) can be activated by TPA and promote MMP-1 and MMP-9 expressions (99). Meanwhile, it may be elevated in patients with lower ER levels. Further studies indicated that the TPA dose-dependently enhanced the expression levels of MMP-1 and MMP-9 via inducing phosphorylation of PKC-α (99), and BBR administration could suppress TPA-induced proliferation and formation of BC cells via blocking PKC-α/MMP signaling (100, 101). The result demonstrated that BBR also exhibited its anticancer activity via inhibiting the abnormal expressions of MMP-1 and MMP-9 during invasion and proliferation of cancer (102, 103). Here, PKC-α/MMPs signing may be also a potential signaling that mediated the anticancer activity of BBR.
BBR-based combined strategies for BC treatment
BBR and curcumin
Curcumin (CUR) is a natural phenolic product distilled from the rhizome of Curcuma Longa L. and its main functions are similar to BBR (104). Both natural products are famous for their multiple pharmacological properties such as anticancer and anti-inflammation activities (105, 106). Many studies revealed that CUR and BBR exhibited outstanding anticancer properties with low toxicity in multiple cancer types (69, 107). Apoptosis and autophagy have been shown to interrelate by many complex mechanisms (108). Clinical analysis revealed that CUR and BBR have the pharmacological capability to mediate autophagic and apoptosis in multiple cancer cells via diverse signaling pathways, such as p-Jun N-terminal kinase (JNK) signaling, Beclin1/Bcl-2 signaling, and ERK signaling.
JNK is found to mediate autophagy in response to cell stresses in cellulo (109). Beclin1, a protein only consisting of Bcl-2 homology 3 domain, is also a vital initiator of autophagy (110). Bcl-2 and Bax belong to Bcl-2 family proteins. Bcl-2 protein is anti-apoptotic, whereas Bax protein is pro-apoptotic (111). Extracellular signal–regulated protein kinases (EKRs) play important roles in cell proliferation and apoptosis, and they could block apoptotic and induce proliferation by enhancing the expressions of pro-survival genes and reducing associated genes, such as Bcl-2 and Bax (112). Mounting evidence showed that the JNK activation increased phosphorylated Bcl-2 level and dissociation of the Beclin1/Bcl-2 complex (109). Then, the dissociated Beclin1 would induce autophagy. He et al. confirmed that combined therapy using CUR and BBR increases phosphorylated Bcl-2 via activating the JNK pathway (108, 113, 114).
ERK is a signaling regulator modulating the proliferation, growth, and survival in most of cell lines (114). However, its activation is also found to mediate cell death in some cell types (115). CUR or BBR treated alone has been found to activate ERK signaling via phosphorylating ERK in many cancer cells, whereas their combined use significantly enhanced ERK phosphorylation and then raised Bax level and reduced the expression of Bcl-2. Meanwhile, the upregulated Bax/Bcl-2 ratio would inhibit the proliferation of cancer cells via inducing apoptosis (11).
All the results strongly suggested that the combined treatment using BBR and CUR significantly improved anticancer efficacy via inducing autophagy and apoptosis of BC cells through the JNK/Beclin1/Bcl-2 pathway and ERK signaling. This combined strategy may be a promising medicine to treat BC.
BBR and emodin
Emodin (EMO) is a natural anthraquinone compound extracted from several medicinal plants and it has been found to exhibit anticancer effects in BC by regulating several signaling pathways (116). SIK3 belongs to the AMP-activated protein kinase family, and it is required for the mTOR/AKT signaling pathway to mediate the proliferation of BC cells. Serine/threonine kinase (AKT), a regulator modulating the cell cycle progression and survival, is also a factor involved in anticancer effect in BC cells (117). The activation of AKT signaling contributes to tumor progression and drug resistance in various types of cancer (118). The mammalian target of rapamycin (mTOR), a highly conserved kinase, is an important regulatory factor to control translation and proliferation in different cancer cell lines. SIK3 is overexpressed and enhances the phosphorylation of AKT in the BC. Then, the phosphorylated AKT is activated, promotes G1/S cell cycle progression, and finally leads to cell proliferation and survival of cancer cells (119). BBR and EMO were identified as potent SIK3 inhibitors in BC via directly binding to the ATP binding pocket of SIK3 by hydrogens. One of the possible mechanisms was that BBR and EMO inhibited the expression of SIK3 via inactivating the AKT signaling pathway and inducing G1/S cell cycle arrest and apoptosis of BC cells (30). In addition, some results revealed that the combined therapy using BBR and EMO significantly decreased the phosphorylation of AKT compared with either a single treatment in BC cells (35). It is worth mentioning that the combined therapy did not affect the growth of non-tumorigenic cells, suggesting that this strategy may be an effective method to treat BC.
Cancer cells consume a lot of glucose to maintain their persistent proliferation, and mTOR-induced aerobic glycolysis provides the source for this process (120). mTOR is also a kinase regulated by SIK3 and plays crucial roles in BC proliferation. Phosphorylation of p70 S6 kinase 1 (p-S6K1) and phosphorylated E4 binding protein 1 (p4EBP1) are two downstream targets of mTOR, and SIK3 regulates the mTOR signalling pathway and promotes the proliferation of BC cells via promoting phosphorylation of them at residues T389 and T37/46, respectively (63, 121). Meanwhile, EMO and BBR alone or combined administration could inhibit mTOR signaling and growth cell cycle arrest, and apoptosis of BC cells via downregulating phosphorylation levels of p-S6K1 (T389) and p4EBP1(T37/46) (35). Current results suggested that the combination of BBR and EMO inhibits proliferation and growth in BC cells. To sum up, BBR and EMO suppress growth and proliferation through the inactivation of SIK3-induced mTOR and AKT signaling pathways.
Conclusions
BBR has exhibited distinct pharmacological activities in different diseases. In this mini-review, the source and biological function of BBR have been summarized, and the anticancer effects of BBR on BC and its underline mechanisms have been also systematically described. The review demonstrated that BBR exhibited its anti-BC effects through binding to the effector proteins/miRNA/DNA regulatory sequences and thus inhibiting multiple cancer-related signaling (Figure 1). Notably, there are several reported molecular targets for BBR in other cancers such as receptor retinoid X receptor alpha (RXRα) (71), protein tyrosine phosphatase 1B (PTP1B) (122), TNF receptor–associated factor 6 (TRAF6) (123), and angiotensin-converting enzyme (ACE), and these proteins also play important roles in BC; further studies are necessary to investigate the roles of these targets in BBR-mediated anti-BC effects. BBR not only could directly bind to oncogenes ephrin-B2, SIK3, and LSD1 and inactivated their functions in BC but also modulated the transcription of some cancer-related genes via directly binding to their cis-acting elements. In addition, BBR has been found to inhibit cancer cell proliferation and invasion via several signaling pathways (Table 1).
Although BBR exhibited potential anti-BC activity in preclinical studies, there are several predicaments to overcome before it was been advanced into clinical treatment against BC. Firstly, several studies showed that BBR exhibited low toxicity to healthy cells and human beings (126, 127). It could also lead to some adverse events, such as constipation and nausea (128, 129). In addition, some patients injected with BBR through intramuscular and intravenous had presented allergic reactions (130). Moreover, the poor oral bioavailability and low water solubility of BBR could reduce its anticancer activities (131). Currently, several strategies have been introduced to improve the efficacy of BBR against BC and reduce side effects in vivo. Combined therapy using BBR and other natural compounds is proved to significantly improve the anti-BC activity of BBR via inducing more effective apoptosis and reducing the dose of BBR (65, 103). Apart from combined therapy, conjugating BBR with other chemical agents is also an efficient strategy to improve the anticancer activity of BBR for BC therapy (132, 133). Qin et al. found that a conjugator via linker BBR to platinum (II) complex significantly improved the in vitro and in vivo anti-BC activity (134). In addition, drug delivery using nanocarriers is widely used to improve cell penetration, biocompatibility, and in vivo efficacy, and this method is also an applicant for improving bioavailability and the efficacy of BBR against BC (135–137). Moreover, chemical modification is also a potent strategy to improve cell penetration, and water soluble anti-tumor activity of BBR and several BBR derivatives have been designed and exhibited better anti-BC efficacy than their lead compound BBR (132, 138–141).
In conclusion, although some studies showed that BBR induced apoptosis of some BC cell lines and sensitized BC cells to chemotherapy via interfering with some pathways, the detailed mechanisms of the BBR are unclear, and more potential anti-tumor pathways or targets are yet to clarify due to the heterogeneity of BC. Further research would make a thorough inquiry regarding the clinical effect of BBR and whether the combined therapy/nanocarriers/chemical modifications could show a more valid effect on patients and reduce the side effects. Meanwhile, it is imperative to consider the treatment cycle and degree of BBR in clinic. In short, further studies are warranted to define the therapeutic role of BBR as an anticancer drug in BC.
Author contributions
X-DZ: Writing-Original draft preparation. L-JC and C-YL: Prepared figures and Tables. X-YX, Y-JL, FT, and M-HZ: Resources, Conceptualization, Investigation and Validation, Writing reviewing and editing. DZ, G-JY and JC: Supervision, Funding acquisition, Conceptualization, Writing reviewing and editing. The authors contributed to the data preparation and drafted and revised the manuscript. All authors contributed to the article and approved the submitted version.
Funding
This work is supported by the National Natural Science Foundation of China (31972821), the General Scientific Research Project of Education of Zhejiang Province (Y202147351), the Starting Research Fund of Ningbo University (421912073), Student Research and Innovation Program of Ningbo University (2022SRIP1818), and State Key Laboratory for Managing Biotic and Chemical Threats to the Quality and Safety of Agro-products (2010DS700124-ZZ2008).
Conflict of interest
The authors declare that the research was conducted in the absence of any commercial or financial relationships that could be construed as a potential conflict of interest.
Publisher’s note
All claims expressed in this article are solely those of the authors and do not necessarily represent those of their affiliated organizations, or those of the publisher, the editors and the reviewers. Any product that may be evaluated in this article, or claim that may be made by its manufacturer, is not guaranteed or endorsed by the publisher.
References
1. Siegel RL, Miller KD, Fuchs HE, Jemal A. Cancer statistics, (2022). CA Cancer J Clin (2022) 72:7–33. doi: 10.3322/caac.21708
2. Cheng SS, Yang GJ, Wang WH, Song YQ, Ko CN, Han QB, et al. Identification of a cytisine-based EED-EZH2 protein-protein interaction inhibitor preventing metastasis in triple-negative breast cancer cells. Acta Materia Med (2022) 1:197–211. doi: 10.15212/AMM-2022-0006
3. Yang GJ, Ko CN, Zhong HJ, Leung CH, Ma DL. Structure-based discovery of a selective KDM5A inhibitor that exhibits anti-cancer activity via inducing cell cycle arrest and senescence in breast cancer cell lines. Cancers (2019) 11(1):92. doi: 10.3390/cancers11010092
4. Cheng SS, Qu YQ, Wu J, Yang GJ, Liu H, Wang W, et al. Inhibition of the CDK9–cyclin T1 protein–protein interaction as a new approach against triple-negative breast cancer. Acta Pharm Sin B (2022) 12:1390–405. doi: 10.1016/j.apsb.2021.10.024
5. Cheng SS, Yang GJ, Wang WH, Ma DL, Leung CH. Discovery of a tetrahydroisoquinoline-based CDK9-cyclin T1 protein–protein interaction inhibitor as an anti-proliferative and anti-migration agent against triple-negative breast cancer cells. Genes Dis (2021). doi: 10.1016/j.gendis.2021.06.005
6. Yang GJ, Wu J, Miao L, Zhu MH, Zhou QJ, Lu XJ, et al. Pharmacological inhibition of KDM5A for cancer treatment. Eur J Med Chem (2021) 226:113855. doi: 10.1016/j.ejmech.2021.113855
7. Yang GJ, Zhu MH, Lu XJ, Liu YJ, Lu JF, Leung CH, et al. The emerging role of KDM5A in human cancer. J Hematol Oncol (2021) 14:30. doi: 10.1186/s13045-021-01041-1
8. Chen L, Zeng T, Pan X, Zhang YH, Huang T, Cai YD. Identifying methylation pattern and genes associated with breast cancer subtypes. Int J Mol Sci (2019) 20(17):4269. doi: 10.3390/ijms20174269
9. Yang GJ, Zhong HJ, Ko CN, Wong SY, Vellaisamy K, Ye M, et al. Identification of a rhodium(iii) complex as a Wee1 inhibitor against TP53-mutated triple-negative breast cancer cells. Chem Commun (2018) 54:2463–6. doi: 10.1039/c7cc09384e
10. 7Yang GJ, Wang WH, Mok SWF, Wu C, Law BYK, Miao XM, et al. Selective inhibition of lysine-specific demethylase 5A (KDM5A) using a rhodium(III) complex for triple-negative breast cancer therapy. Angew Chem Int Ed Engl (2018) 57:13091–5. doi: 10.1002/anie.201807305
11. Yang GJ, Song YQ, Wang WH, Han QB, Ma DL, Leung CH, et al. An optimized BRD4 inhibitor effectively eliminates NF-κB-driven triple-negative breast cancer cells. Bioorg Chem (2021) 114:105158. doi: 10.1016/j.bioorg.2021.105158
12. Sun Y, Wang W, Tong Y. Berberine inhibits proliferative ability of breast cancer cells by reducing metadherin. J Exp Clin Res (2019) 25:9058–66. doi: 10.12659/MSM.914486
13. Tellez-Gabriel M, Knutsen E, Perander M. Current status of circulating tumor cells, circulating tumor DNA, and exosomes in breast cancer liquid biopsies. Int J Mol Sci (2020) 21(24):9457. doi: 10.3390/ijms21249457
14. Patil J, Kim J, Jayaprakasha GK. Berberine induces apoptosis in breast cancer cells (MCF-7) through mitochondrial-dependent pathway. Eur J Pharmacol (2010) 645:70–8. doi: 10.1016/j.ejphar.2010.07.037
15. Hesari A, Ghasemi F, Cicero AFG, Mohajeri M, Rezaei O, Hayat SMG, et al. Berberine: A potential adjunct for the treatment of gastrointestinal cancers? J Cell Biochem (2018) 119:9655–63. doi: 10.1002/jcb.27392
16. Zhang L, Wu X, Yang R, Chen F, Liao Y, Zhu Z, et al. Effects of berberine on the gastrointestinal microbiota. Front Cell Infect Microbiol (2020) 10:588517. doi: 10.3389/fcimb.2020.588517
17. Ikram M. A review on the chemical and pharmacological aspects of genus berberis. Planta Med (1975) 28:353–8. doi: 10.1055/s-0028-1097869
18. Barzegar E, Fouladdel S, Movahhed TK, Atashpour S, Ghahremani MH, Ostad SN, et al. Effects of berberine on proliferation, cell cycle distribution and apoptosis of human breast cancer T47D and MCF7 cell lines. Iran J Basic Med Sci (2015) 18:334–42.
19. Wang K, Feng X, Chai L, Cao S, Qiu F. The metabolism of berberine and its contribution to the pharmacological effects. Drug Metab Rev (2017) 49:139–57. doi: 10.1080/03602532.2017.1306544
20. Qiu F, Zhu Z, Kang N, Piao S, Qin G, Yao X. Isolation and identification of urinary metabolites of berberine in rats and humans. Drug Metab Dispos (2008) 36:2159–65. doi: 10.1124/dmd.108.021659
21. Fatahian A, Haftcheshmeh SM, Azhdari S, Farshchi HK, Nikfar B, Momtazi-Borojeni AA. Promising anti-atherosclerotic effect of berberine: Evidence from in vitro, in vivo, and clinical studies. Rev Physiol Biochem Pharmacol (2020) 178:83–110. doi: 10.1007/112_2020_42
22. Jiang X, Jiang Z, Jiang M, Sun Y. Berberine as a potential agent for the treatment of colorectal cancer. Front Med (2022) 9:886996. doi: 10.3389/fmed.2022.886996
23. Habtemariam S. Berberine and inflammatory bowel disease: A concise review. Pharmacol Res (2016) 113:592–9. doi: 10.1016/j.phrs.2016.09.041
24. Li YH, Xiao HT, Hu DD, Fatima S, Lin CY, Mu HX. Berberine ameliorates chronic relapsing dextran sulfate sodium-induced colitis in C57BL/6 mice by suppressing Th17 responses. Pharmacol Res (2016) 110:227–39. doi: 10.1016/j.phrs.2016.02.010
25. Palma TV, Lenz LS, Bottari NB, Pereira A, Schetinger MRC, Morsch VM, et al. Berberine induces apoptosis in glioblastoma multiforme U87MG cells via oxidative stress and independent of AMPK activity. Mol Biol Rep (2020) 47:4393–400. doi: 10.1007/s11033-020-05500-9
26. Imanshahidi M, Hosseinzadeh H. Pharmacological and therapeutic effects of berberis vulgaris and its active constituent, berberine. Phytother Res (2008) 22:999–1012. doi: 10.1002/ptr.2399
27. Pazhang Y, Ahmadian S, Javadifar N, Shafiezadeh M. COX-2 and survivin reduction may play a role in berberine-induced apoptosis in human ductal breast epithelial tumor cell line. Int Soc Oncodev Biol Med (2012) 33:207–14. doi: 10.1007/s13277-011-0263-5
28. Du J, Sun Y, Lu YY, Lau E, Zhao M, Zhou QM, et al. Berberine and evodiamine act synergistically against human breast cancer MCF-7 cells by inducing cell cycle arrest and apoptosis. Anticancer Res (2017) 37:6141–51. doi: 10.21873/anticanres.12063
29. Hou Q, Tang X, Liu H, Yang Y, Jing X, Xiao Q, et al. Berberine induces cell death in human hepatoma cells in vitro by downregulating CD147. Cancer Sci (2011) 102:1287–92. doi: 10.1111/j.1349-7006.2011.01933.x
30. Kuo HP, Chuang TC, Yeh MH, Hsu SC, Way TD, Chen PY, et al. Growth suppression of HER2-overexpressing breast cancer cells by berberine via modulation of the HER2/PI3K/Akt signaling pathway. J Agric Food Chem (2011) 59:8216–24. doi: 10.1021/jf2012584
31. Kuo CL, Chou CC, Yung BY. Berberine complexes with DNA in the berberine-induced apoptosis in human leukemic HL-60 cells. Cancer Lett (1995) 93:193–200. doi: 10.1016/0304-3835(95)03809-b
32. Rauf A, Abu-Izneid T, Khalil AA, Imran M, Shah ZA, Emran TB, et al. Berberine as a potential anticancer agent: A comprehensive review. Molecules (2021) 26:7368. doi: 10.3390/molecules26237368
33. Alotaibi D, Amara S, Johnson TL, Tiriveedhi V. Potential anticancer effect of prostratin through SIK3 inhibition. Oncol Lett (2018) 15:3252–8. doi: 10.3892/ol.2017.7674
34. Charoenfuprasert S, Yang YY, Lee YC, Chao KC, Chu PY, Lai CR, et al. Identification of salt-inducible kinase 3 as a novel tumor antigen associated with tumorigenesis of ovarian cancer. Oncogene (2011) 30:3570–84. doi: 10.1038/onc.2011.77
35. Ponnusamy L, Kothandan G, Manoharan R. Berberine and emodin abrogates breast cancer growth and facilitates apoptosis through inactivation of SIK3-induced mTOR and AKT signaling pathway. Biochim Biophys Acta Mol Basis Dis (2020) 1866:165897. doi: 10.1016/j.bbadis.2020.165897
36. Chen II, Caprioli A, Ohnuki H, Kwak H, Porcher C, Tosato G. EphrinB2 regulates the emergence of a hemogenic endothelium from the aorta. Sci Rep (2016) 6:27195. doi: 10.1038/srep27195
37. Dravis C, Henkemeyer M. Ephrin-b reverse signaling controls septation events at the embryonic midline through separate tyrosine phosphorylation-independent signaling avenues. Dev Bio (2011) 355:138–51. doi: 10.1016/j.ydbio.2011.04.020
38. Abéngozar MA, de Frutos S, Ferreiro S, Soriano J, Perez-Martinez M, Olmeda D, et al. Blocking ephrinB2 with highly specific antibodies inhibits angiogenesis, lymphangiogenesis, and tumor growth. Blood (2012) 119:4565–76. doi: 10.1182/blood-2011-09-380006
39. Genander M, Frisén J. Ephrins and eph receptors in stem cells and cancer. Curr Opin Cell Biol (2010) 22:611–6. doi: 10.1016/j.ceb.2010.08.005
40. Poliakov A, Cotrina M, Wilkinson DG. Diverse roles of eph receptors and ephrins in the regulation of cell migration and tissue assembly. Dev Cell (2004) 7:465–80. doi: 10.1016/j.devcel.2004.09.006
41. Yang D, Jin C, Ma H, Huang M, Shi GP, Wang J, et al. EphrinB2/EphB4 pathway in postnatal angiogenesis: a potential therapeutic target for ischemic cardiovascular disease. Angiogenesis (2016) 19:297–309. doi: 10.1007/s10456-016-9514-9
42. Ma W, Zhu M, Zhang D, Yang L, Yang T, Li X, et al. Berberine inhibits the proliferation and migration of breast cancer ZR-75-30 cells by targeting ephrin-B2. Phytomedicine (2017) 25:45–51. doi: 10.1016/j.phymed.2016.12.013
43. Laurent B, Ruitu L, Murn J, Hempel K, Ferrao R, Xiang Y, et al. A specific LSD1/KDM1A isoform regulates neuronal differentiation through H3K9 demethylation. Mol Cell (2015) 57:957–70. doi: 10.1016/j.molcel.2015.01.010
44. Yang GJ, Lei PM, Wong SY, Ma DL, Leung CH. Pharmacological inhibition of LSD1 for cancer treatment. Molecules (2018) 23(12):3194. doi: 10.3390/molecules23123194
45. Feng J, Xu G, Liu J, Zhang N, Li L, Ji J, et al. Phosphorylation of LSD1 at Ser112 is crucial for its function in induction of EMT and metastasis in breast cancer. Breast Cancer Res Treat (2016) 159:443–56. doi: 10.1007/s10549-016-3959-9
46. Shi YJ, Matson C, Lan F, Iwase S, Baba T, Shi Y. Regulation of LSD1 histone demethylase activity by its associated factors. Mol Cell (2005) 19:857–64. doi: 10.1016/j.molcel.2005.08.027
47. Piao L, Suzuki T, Dohmae N, Nakamura Y, Hamamoto R. SUV39H2 methylates and stabilizes LSD1 by inhibiting polyubiquitination in human cancer cells. Oncotarget (2015) 6:16939–50. doi: 10.18632/oncotarget.4760
48. Metzger E, Wissmann M, Yin N, Müller JM, Schneider R, Peters AH, et al. LSD1 demethylates repressive histone marks to promote androgen-receptor-dependent transcription. Nature (2005) 437:436–9. doi: 10.1038/nature04020
49. Song Y, Zhang H, Yang X, Shi Y, Yu B. Annual review of lysine-specific demethylase 1 (LSD1/KDM1A) inhibitors in 2021. Eur J Med Chem (2022) 228:114042. doi: 10.1016/j.ejmech.2021.114042
50. Fu D, Li J, Yu B. Annual review of LSD1/KDM1A inhibitors in 2020. Eur J Med Chem (2021) 214:113254. doi: 10.1016/j.ejmech.2021.113254
51. Lim S, Janzer A, Becker A, Zimmer A, Schüle R, Buettner R, et al. Lysine-specific demethylase 1 (LSD1) is highly expressed in ER-negative breast cancers and a biomarker predicting aggressive biology. Carcinogenesis (2010) 31:512–20. doi: 10.1093/carcin/bgp324
52. Zwergel C, Stazi G, Mai A, Valente S. Trends of LSD1 inhibitors in viral infections. Future Med Chem (2018) 10(10):1133–6. doi: 10.4155/fmc-2018-0065
53. Lu JJ, Bao JL, Chen XP, Huang M, Wang YT. Alkaloids isolated from natural herbs as the anticancer agents. Evid Based Complement Alternat Med (2012) 2012:485042. doi: 10.1155/2012/485042
54. Roudini L, NayebZadeh Eidgahi N, Rahimi HR, Saberi MR, Amiri Tehranizadeh Z, Beigoli S. Determining the interaction behavior of calf thymus DNA with berberine hydrochloride in the presence of linker histone: a biophysical study. J Biomol Struct (2020) 38:364–81. doi: 10.1080/07391102.2019.1574240
55. Bhadra K, Kumar GS. Therapeutic potential of nucleic acid-binding isoquinoline alkaloids: binding aspects and implications for drug design. Med Res Rev (2011) 31:821–62. doi: 10.2174/1389557515666150909144425
56. Zhao Y, Jing Z, Lv J, Zhang Z, Lin J, Cao X, et al. Berberine activates caspase-9/cytochrome c-mediated apoptosis to suppress triple-negative breast cancer cells in vitro and in vivo. BioMed Pharmacother (2017) 95:18–24. doi: 10.1016/j.biopha.2017.08.045
57. Czarny P, Pawlowska E, Bialkowska-arzecha J, Kaarniranta K, Blasiak J. Autophagy in DNA damage response. Int J Mol Sci (2015) 16:2641–62. doi: 10.3390/ijms16022641
58. Diao L, Tang N, Zhang C, Cheng JG, Zhang ZH, Wang SY, et al. Avermectin induced DNA damage to the apoptosis and autophagy in human lung epithelial A549 cells. Ecotoxicol Environ Saf (2021) 215:112129. doi: 10.1016/j.ecoenv.2021.112129
59. Rodriguez-Rocha H, Garcia-Garcia A, Panayiotidis M, Franco R. DNA Damage and autophagy. Mutat Res (2011) 711:158–66. doi: 10.1016/j.mrfmmm.2011.03.007
60. Parra-Marín O, López-Pacheco K, Hernández R, López-Villaseñor I. The highly diverse TATA box-binding proteins among protists: A review. Mol Biochem Parasito (2020) 239:111312. doi: 10.1016/j.molbiopara.2020.111312
61. Yuan ZY, Lu X, Lei F, Chai YS, Wang YG, Jiang JF, et al. TATA boxes in gene transcription and poly (A) tails in mRNA stability: New perspective on the effects of berberine. Sci Rep (2015) 5:18326. doi: 10.1038/srep18326
62. Wang Y, Kheir MM, Chai Y, Hu J, Xing D, Lei F, et al. Comprehensive study in the inhibitory effect of berberine on gene transcription, including TATA box. PloS One (2011) 6:e23495. doi: 10.1371/journal.pone.0023495
63. Vander Heiden MG, Cantley LC, Thompson CB. Understanding the warburg effect: the metabolic requirements of cell proliferation. Science (2009) 324:1029–33. doi: 10.1126/science.1160809
64. Kang S, Kim B, Kang HS, Jeong G, Bae H, Lee H, et al. SCTR regulates cell cycle-related genes toward anti-proliferation in normal breast cells while having pro-proliferation activity in breast cancer cells. Int J Oncol (2015) 47:1923–31. doi: 10.3892/ijo.2015.3164
65. Qin CX, Yang XQ, Jin GC, Zhan ZY. LncRNA TSLNC8 inhibits proliferation of breast cancer cell through the miR-214-3p/FOXP2 axis. Eur Rev Med Pharmacol Sci (2019) 23:8440–8. doi: 10.26355/eurrev_201910_19156
66. Han LC, Wang H, Niu FL, Yan JY, Cai HF. Effect miR-214-3p on proliferation and apoptosis of breast cancer cells by targeting survivin protein. Eur Rev Med Pharmacol Sci (2019) 23:7469–74. doi: 10.26355/eurrev_201909_18856
67. Tang B, Luo H, Xie S, Pan C, Fu J. Deubiquitination of TNKS1 regulates wnt/β-catenin to affect the expression of USP25 to promote the progression of glioma. Dis Markers (2022) 2022:9087190. doi: 10.1155/2022/9087190
68. Liu J, He C, Zhou K, Wang J, Kang JX. Coptis extracts enhance the anticancer effect of estrogen receptor antagonists on human breast cancer cells. Biochem Biophys Res Commun (2009) 378:174–8. doi: 10.1016/j.bbrc.2008.10.169
69. Jabbarzadeh Kaboli P, Rahmat A, Ismail P, Ling KH. Targets and mechanisms of berberine, a natural drug with potential to treat cancer with special focus on breast cancer. Eur J Pharmacol (2014) 740:584–95. doi: 10.1016/j.ejphar.2014.06.025
70. Dian L, Xu Z, Sun Y, Li J, Lu H, Zheng M, et al. Berberine alkaloids inhibit the proliferation and metastasis of breast carcinoma cells involving wnt/β-catenin signaling and EMT. Phytochemistry (2022) 200:113217. doi: 10.1016/j.phytochem.2022.113217
71. Ruan H, Zhan YY, Hou J, Xu B, Chen B, Tian Y, et al. Berberine binds RXRα to suppress β-catenin signaling in colon cancer cells. Oncogene (2017) 36:6906–18. doi: 10.1038/onc.2017.296
72. Mittal A, Tabasum S, Singh RP. Berberine in combination with doxorubicin suppresses growth of murine melanoma B16F10 cells in culture and xenograft. Phytomedicine (2014) 21:340–7. doi: 10.1016/j.phymed.2013.09.002
73. Caldon CE, Daly RJ, Sutherland RL, Musgrove EA. Cell cycle control in breast cancer cells. J Cell Biochem (2006) 97:261–74. doi: 10.1002/jcb.20690
74. Phoon YP, Chivukula IV, Tsoi YL, Kanatani S, Uhlén P, Kuiper R, et al. Notch activation in the mouse mammary luminal lineage leads to ductal hyperplasia and altered partitioning of luminal cell subtypes. Exp Cell Res (2020) 395:112156. doi: 10.1016/j.yexcr.2020.112156
75. Ponnusamy L, Natarajan SR, Thangaraj K, Manoharan R. Therapeutic aspects of AMPK in breast cancer: Progress, challenges, and future directions. Biochim Biophys Acta Rev Cancer (2020) 1874:188379. doi: 10.1016/j.bbcan.2020.188379
76. Grahame HD. AMP-activated protein kinase: an energy sensor that regulates all aspects of cell function. Genes Dev (2011) 25:1895–908. doi: 10.1101/gad.17420111
77. Kim HS, Wannatung T, Lee S, Yang WK, Chung SH, Lim JS, et al. Quercetin enhances hypoxia-mediated apoptosis via direct inhibition of AMPK activity in HCT116 colon cancer. Program Cell Death (2012) 17:938–49. doi: 10.1007/s10495-012-0719-0
78. Zinkernagel AS, Johnson RS, Nizet V. Hypoxia inducible factor (HIF) function in innate immunity and infection. Mol Med (2007) 85:1339–46. doi: 10.1007/s00109-007-0282-2
79. Chen XY, Wang QL, Huang WX, Li LW, Liu LC, Yang Y, et al. Application of serum exosomal hypoxia-inducible factor-1 alpha (HIF-1α) as potential circulating biomarker for bacterial peritonitis. Bioengineered (2021) 13(2): 1975–87. doi: 10.1080/21655979.2021.2006866
80. Ai Z, Lu Y, Qiu S, Fan Z. Overcoming cisplatin resistance of ovarian cancer cells by targeting HIF-1-regulated cancer metabolism. Cancer Lett (2016) 373:36–44. doi: 10.1016/j.canlet.2016.01.009
81. Shan JZ, Xuan YY, Zhang Q, Huang JJ. Ursolic acid sensitized colon cancer cells to chemotherapy under hypoxia by inhibiting MDR1 through HIF-1α. J Zhejiang Univ Sci B (2016) 17:672–82. doi: 10.1631/jzus.B1600266
82. Joshi P, Vishwakarma R, Bharate S. Natural alkaloids as p-gp inhibitors for multidrug resistance reversal in cancer. Eur J Med Chem (2017) 138:273–92. doi: 10.1016/j.ejmech.2017.06.047
83. Sobhani N, Roviello G, D'Angelo A, Roudi R, Neeli PK, Generali D. p53 antibodies as a diagnostic marker for cancer: A meta-analysis. Molecules (2021) 26(20):6215. doi: 10.3390/molecules26206215
84. Wallace NA, Robinson K, Galloway DA. Beta human papillomavirus E6 expression inhibits stabilization of p53 and increases tolerance of genomic instability. J Virol (2014) 88:6112–27. doi: 10.1128/JVI.03808-13
85. Li W, Hua B, Saud SM, Lin H, Hou W, Matter MS, et al. Berberine regulates AMP-activated protein kinase signaling pathways and inhibits colon tumorigenesis in mice. Mol Carcinog (2015) 54:1096–109. doi: 10.1002/mc.22179
86. Yue P, Dan S, Yawei Z, Zhang F, Zheng X, Tan Y, et al. Berberine reverses hypoxia-induced chemoresistance in breast cancer through the inhibition of AMPK- HIF-1α. Int J Biol Sci (2017) 13(6):794–803. doi: 10.7150/ijbs.18969
87. Huang CC, Chia WT, Chung MF, Lin KJ, Hsiao CW, Jin C, et al. An implantable depot that can generate oxygen in situ for overcoming hypoxia-induced resistance to anticancer drugs in chemotherapy. J Am Chem Soc (2016) 138:5222–5. doi: 10.1021/jacs.6b01784
88. Pan Y, Zhang F, Zhao Y, Shao D, Zheng X, Chen Y, et al. Berberine enhances chemosensitivity and induces apoptosis through dose-orchestrated AMPK signaling in breast cancer. J Cancer (2017) 8:1679–89. doi: 10.7150/jca.19106
89. Pan Y, Zhang Y, Chen Q, Tao X, Liu J, Xiao GG. CTAB enhances chemo-sensitivity through activation of AMPK signaling cascades in breast cancer. Front Pharmacol (2019) 10:843. doi: 10.3389/fphar.2019.00843
90. Schlingemann J, Hess J, Wrobel G, Breitenbach U, Gebhardt C, Steinlein P. Profile of gene expression induced by the tumour promotor TPA in murine epithelial cells. Int J Cancer (2003) 104:699–708. doi: 10.1002/ijc.11008
91. Techasen A, Namwat N, Loilome W, Bungkanjana P, Khuntikeo N, Puapairoj A, et al. Tumor necrosis factor-α (TNF-α) stimulates the epithelial-mesenchymal transition regulator snail in cholangiocarcinoma. Med Oncol (2012) 29:3083–91. doi: 10.1007/s12032-012-0305-x
92. Javadian M, Gharibi T, Shekari N, Abdollahpour-Alitappeh M, Mohammadi A, Hossieni A. The role of microRNAs regulating the expression of matrix metalloproteinases (MMPs) in breast cancer development, progression, and metastasis. J Cell Physiol (2019) 234:5399–412. doi: 10.1002/jcp.27445
93. Gabasa M, Radisky ES, Ikemori R, Bertolini G, Arshakyan M, Hockla A, et al. MMP-1 drives tumor progression in large cell carcinoma of the lung through fibroblast senescence. Cancer Lett (2021) 507:1–12. doi: 10.1016/j.canlet.2021.01.028
94. Liao Y, Zhu E, Zhou W. Ox-LDL aggravates the oxidative stress and inflammatory responses of THP-1 macrophages by reducing the inhibition effect of miR-491-5p on. MMP-9. Front Cardiovasc Med (2021) 8:697236. doi: 10.3389/fcvm.2021.697236
95. Zeng ZS, Guillem JG. Colocalisation of matrix metalloproteinase-9-mRNA and protein in human colorectal cancer stromal cells. Br J Cancer (1996) 74:1161–7. doi: 10.1038/bjc.1996.511
96. Ranogajec I, Jakić-Razumović J, Puzović V, Gabrilovac J. Prognostic value of matrix metalloproteinase-2 (MMP-2), matrix metalloproteinase-9 (MMP-9) and aminopeptidase N/CD13 in breast cancer patients. Med Oncol (2012) 29:561–9. doi: 10.1007/s12032-011-9984-y
97. Kim S, Choi JH, Kim JB, Nam SJ, Yang JH, Kim JH, et al. Berberine suppresses TNF-alpha-induced MMP-9 and cell invasion through inhibition of AP-1 activity in MDA-MB-231 human breast cancer cells. Molecules (2008) 13:2975–85. doi: 10.3390/molecules13122975
98. Hartjen P, Medom BK, Reinholz M, Borowski P, Baier A. Regulation of the biochemical function of motif VI of HCV NTPase/helicase by the conserved phe-loop. Biochimie (2009) 91:252–60. doi: 10.1016/j.biochi.2008.09.007
99. Kim S, Han J, Lee SK, Choi MY, Kim J, Lee J, et al. Berberine suppresses the TPA-induced MMP-1 and MMP-9 expressions through the inhibition of PKC-α in breast cancer cells. J Surg Res (2012) 176:e21–29. doi: 10.1016/j.jss.2011.11.1041
100. Gupta AK, Galoforo SS, Berns CM, Martinez AA, Corry PM, Guan KL, et al. Elevated levels of ERK2 in human breast carcinoma MCF-7 cells transfected with protein kinase c alpha. Cell Prolif (1996) 29:655–63. doi: 10.1111/j.1365-2184.1996.tb00979.x
101. Kim S, Kim Y, Kim JE, Cho KH, Chung JH. Berberine inhibits TPA-induced MMP-9 and IL-6 expression in 582 normal human keratinocytes. Phytomedicine (2008) 15:340–47. doi: 10.1016/j.phymed.2007.09.011
102. Hamsa TP, Kuttan G. Berberine inhibits pulmonary metastasis through down-regulation of MMP in metastatic B16F-10 melanoma cells. Phytother Res (2012) 26:568–78. doi: 10.1002/ptr.3586
103. Ma L, Zhang L, Wang B, Wei YY, Liu JY, Zhang LJ. Berberine inhibits chlamydia pneumoniae infection-induced vascular smooth muscle cell migration through downregulating MMP-3 and MMP-9 via PI3K. Eur J Pharmacol (2015) 755:102–9. doi: 10.1016/j.ejphar.2015.02.039
104. Ghosh S, Banerjee S, Sil PC. The beneficial role of curcumin on inflammation, diabetes and neurodegenerative disease: A recent update. Food Chem Toxicol (2015) 83:111–24. doi: 10.1016/j.fct.2015.05.022
105. Kumar A, Ekavali, Chopra K, Mukherjee M, Pottabathini R, Dhull DK. Current knowledge and pharmacological profile of berberine: An update. Eur J Pharmacol (2015) 761:288–97. doi: 10.1016/j.ejphar.2015.05.068
106. Yan K, Zhang C, Feng J, Hou L, Yan L, Zhou Z, et al. Induction of G1 cell cycle arrest and apoptosis by berberine in bladder cancer cells. Eur J Pharmacol (2011) 661:1–7. doi: 10.1016/j.ejphar.2011.04.021
107. Park W, Amin AR, Chen ZG, Shin DM. New perspectives of curcumin in cancer prevention. Cancer Prev Res (2013) 6:387–400. doi: 10.1158/1940-6207.CAPR-12-0410
108. He W, Wang Q, Srinivasan B, Xu J, Padilla MT, Li Z, et al. A JNK-mediated autophagy pathway that triggers c-IAP degradation and necroptosis for anticancer chemotherapy. Oncogene (2014) 33:3004–13. doi: 10.1038/onc.2013.256
109. Wei Y, Pattingre S, Sinha S, Bassik M, Levine B. JNK1-mediated phosphorylation of bcl-2 regulates starvation-induced autophagy. Mol Cell (2008) 30:678–88. doi: 10.1016/j.molcel.2008.06.001
110. Oberstein A, Jeffrey PD, Shi Y. Crystal structure of the bcl-XL-Beclin 1 peptide complex: Beclin 1 is a novel BH3-only protein. J Biol Chem (2007) 282:13123–32. doi: 10.1074/jbc.M700492200
111. Ashkenazi A. Targeting the extrinsic apoptosis pathway in cancer. Cytokine Growth Factor Rev (2008) 19:325–31. doi: 10.1016/j.cytogfr.2008.04.001
112. Kolch W. Coordinating ERK/MAPK signalling through scaffolds and inhibitors. Nat Rev Mol Cell Biol (2005) 6:827–37. doi: 10.1038/nrm1743
113. Thompson SJ, Loftus LT, Ashley MD, Meller R. Ubiquitin-proteasome system as a modulator of cell fate. Curr Opin Pharmacol (2008) 8:90–5. doi: 10.1016/j.coph.2007.09.010
114. Basu A, Haldar S. Signal-induced site specific phosphorylation targets bcl-2 to the proteasome pathway. Int J Oncol (2002) 21:597–601. doi: 10.3892/ijo.21.3.597
115. Cagnol S, Chambard JC. ERK and cell death: mechanisms of ERK-induced cell death–apoptosis, autophagy and senescence. FEBS J (2010) 277:2–21. doi: 10.1111/j.1742-4658.2009.07366.x
116. Thacker PC, Karunagaran D. Curcumin and emodin down-regulate TGF-β signaling pathway in human cervical cancer cells. PloS One (2015) 10:e0120045. doi: 10.1371/journal.pone.0120045
117. Fu L, Chen W, Guo W, Wang J, Tian Y, Shi D, et al. Berberine targets AP-2/hTERT, NF-κB/COX-2, HIF-1α/VEGF and cytochrome-c/caspase signaling to suppress human cancer cell growth. PloS One (2013) 8:e69240. doi: 10.1371/journal.pone.0069240
118. Hua H, Zhang H, Chen J, Wang J, Liu J, Jiang Y. Targeting AKT in cancer for precision therapy. J Hematol Oncol (2021) 14:128. doi: 10.1186/s13045-021-01137-8
119. Amara S, Majors C, Roy B, Hill S, Rose KL, Myles EL, et al. Critical role of SIK3 in mediating high salt and IL-17 synergy leading to breast cancer cell proliferation. PloS One (2017) 12:e0180097. doi: 10.1371/journal.pone.0180097
120. Chen JL, Chen F, Zhang TT, Liu NF. Suppression of SIK1 by miR-141 in human ovarian cancer cell lines and tissues. Int J Mol Med (2016) 37:1601–10. doi: 10.3892/ijmm.2016.2553
121. Ponnusamy L, Manoharan R. Distinctive role of SIK1 and SIK3 isoforms in aerobic glycolysis and cell growth of breast cancer through the regulation of p53 and mTOR signaling pathways. Biochim Biophys Acta Mol Cell Res (2021) 1868:118975. doi: 10.1016/j.bbamcr.2021.118975
122. Choi J, Ali M, Jung H, Oh S, Choi R, Kim E. Protein tyrosine phosphatase 1B inhibitory activity of alkaloids from rhizoma coptidis and their molecular docking studies. J Ethnopharmacol (2015) 171:28–36. doi: 10.1016/j.jep.2015.05.020
123. Ye F, Zhou Q, Tian L, Lei F, Feng D. The protective effect of berberine hydrochloride on LPS−induced osteoclastogenesis through inhibiting TRAF6−Ca2+−calcineurin−NFATcl signaling pathway. Mol Med Rep (2017) 16:6228–33. doi: 10.3892/mmr.2017.7338
124. Chai YS, Yuan ZY, Lei F, Wang YG, Hu J, Du F, et al. Inhibition of retinoblastoma mRNA degradation through poly (A) involved in the neuroprotective effect of berberine against cerebral ischemia. PloS One (2014) 9:e90850. doi: 10.1371/journal.pone.0090850
125. Lin YS, Chiu YC, Tsai YH, Tsai YF, Wang JY, Tseng LM, et al. Different mechanisms involved in the berberine-induced antiproliferation effects in triple-negative breast cancer cell lines. J Cell Biochem (2019) 120:13531–44. doi: 10.1002/jcb.28628
126. Xiong RG, Huang SY, Wu SX, Zhou DD, Yang ZJ, Saimaiti A, et al. Anticancer effects and mechanisms of berberine from medicinal herbs: An update review. Molecules (2022) 27(14):4523. doi: 10.3390/molecules27144523
127. Och A, Podgórski R, Nowak R. Biological activity of berberine-a summary update. Toxins (2020) 12(11):713. doi: 10.3390/toxins12110713
128. Fang S, Guo S, Du S, Cao Z, Yang Y, Su X, et al. A systematic review and meta-analysis. J Ethnopharmacol (2022) 282:114617. doi: 10.1016/j.jep.2021.114617
129. Mirzaee F, Razmjouei P, Shahrahmani H, Vafisani F, Najaf Najafi M, Ghazanfarpour M. The effect and safety of berberine on polycystic ovary syndrome: a systematic review. J Obstet Gynaecol (2021) 41:684–9. doi: 10.1080/01443615.2020.1787964
130. Hu S, Zhao R, Liu Y, Chen J, Zheng Z, Wang S. Preventive and therapeutic roles of berberine in gastrointestinal cancers. BioMed Res Int (2019) 2019:6831520. doi: 10.1155/2019/6831520
131. Bhanumathi R, Vimala K, Shanthi K, Thangaraj R, Kannan S. Bioformulation of silver nanoparticles as berberine carrier cum anticancer agent against breast cancer. New J Chem (2017) 41:14466–77. doi: 10.1039/C7NJ02531A
132. Singh IP, Mahajan S. Berberine and its derivatives: a patent review (2009 - 2012). Expert Opin Ther Pat (2013) 23:215–31. doi: 10.1517/13543776.2013.746314
133. Bhanumathi R, Manivannan M, Thangaraj R, Kannan S. Drug-carrying capacity and anticancer effect of the folic acid- and berberine-loaded silver nanomaterial to regulate the AKT-ERK pathway in breast cancer. ACS Omega (2018) 3:8317–28. doi: 10.1021/acsomega.7b01347
134. Qin QP, Wang ZF, Huang XL, Tan MX, Luo ZH, Wang SL, et al. Two telomerase-targeting pt(ii) complexes of jatrorrhizine and berberine derivatives induce apoptosis in human bladder tumor cells. Dalton Trans (2019) 48:15247–54. doi: 10.1039/c9dt02381j
135. Zheng X, Zhao Y, Jia Y, Shao D, Zhang F, Sun M, et al. Biomimetic co-assembled nanodrug of doxorubicin and berberine suppresses chemotherapy-exacerbated breast cancer metastasis. Biomaterials (2021) 271:120716. doi: 10.1016/j.biomaterials.2021.120716
136. Ziasarabi P, Sahebkar A, Ghasemi F. Evaluation of the effects of nanomicellar curcumin, berberine, and their combination with 5-fluorouracil on breast cancer cells. Adv Exp Med Biol (2021) 1328:21–35. doi: 10.1007/978-3-030-73234-9_3
137. Chiu CF, Fu RH, Hsu SH, Yu YA, Yang SF, Tsao TC, et al. Delivery capacity and anticancer ability of the berberine-loaded gold nanoparticles to promote the apoptosis effect in breast cancer. Cancers (2021) 13(21):5317. doi: 10.3390/cancers13215317
138. Chang JM, Wu JY, Chen SH, Chao WY, Chuang HH, Kam KH, et al. 9-o-terpenyl-substituted berberrubine derivatives suppress tumor migration and increase anti-human non-small-cell lung cancer activity. Int J Mol Sci (2021) 22(18):9864. doi: 10.3390/ijms22189864
139. Pierpaoli E, Arcamone A, Buzzetti F, Salvatore C, Geroni C, Piacenza F, et al. Antitumor effect of novel berberine derivatives in breast cancer cells. Biofactors (2013) 39:672–9. doi: 10.1002/biof.1440
140. Liu J, Huang X, Liu D, Ji K, Tao C, Zhang R, et al. Demethyleneberberine induces cell cycle arrest and cellular senescence of NSCLC cells via c-Myc/HIF-1α pathway. Phytomedicine (2021) 91:153678. doi: 10.1016/j.phymed.2021.153678
Keywords: Breast cancer, berberine, apoptosis, autophagic, cell cycle arrest
Citation: Zhong X-D, Chen L-J, Xu X-Y, Liu Y-J, Tao F, Zhu M-H, Li C-Y, Zhao D, Yang G-J and Chen J (2022) Berberine as a potential agent for breast cancer therapy. Front. Oncol. 12:993775. doi: 10.3389/fonc.2022.993775
Received: 25 July 2022; Accepted: 09 August 2022;
Published: 02 September 2022.
Edited by:
Hui Jia, Shenyang Medical College, ChinaReviewed by:
Madhuri Sharon, Walchand Centre for Research in Naynotechnology and Bionanotechnolog, IndiaYi Zhu, Chengdu University of Traditional Chinese Medicine, China
Copyright © 2022 Zhong, Chen, Xu, Liu, Tao, Zhu, Li, Zhao, Yang and Chen. This is an open-access article distributed under the terms of the Creative Commons Attribution License (CC BY). The use, distribution or reproduction in other forums is permitted, provided the original author(s) and the copyright owner(s) are credited and that the original publication in this journal is cited, in accordance with accepted academic practice. No use, distribution or reproduction is permitted which does not comply with these terms.
*Correspondence: Guan-Jun Yang, champion2014@126.com; Jiong Chen, jchen1975@163.com; Dan Zhao, zhaodan2@nbu.edu.cn