- 1Division of Gastrointestinal, Breast, Pediatric, and General Surgery, Department of Surgery, Shiga University of Medical Science, Otsu, Japan
- 2Shiga University of Medical Science, Otsu, Japan
Ascorbic acid has attracted substantial attention for its potential antitumor effects by acting as an antioxidant in vivo and as a cofactor in diverse enzymatic reactions. However, solid proof of its clinical efficacy against cancer and the mechanism behind its effect have not been established. Moreover, cancer forms cancer-specific microenvironments and interacts with various cells, such as cancer-associated fibroblasts (CAFs), to maintain cancer growth and progression; however, the effect of ascorbic acid on the cancer microenvironment is unclear. This review discusses the effects and mechanisms of ascorbic acid on cancer, including the role of ascorbic acid concentration. In addition, we present future perspectives on the effects of ascorbic acid on cancer cells and the CAF microenvironment. Ascorbic acid has a variety of effects, which contributes to the complexity of these effects. Oral administration of ascorbic acid results in low blood concentrations (<0.2 mM) and acts as a cofactor for antioxidant effects, collagen secretion, and HIFα degradation. In contrast, intravenous treatment achieves large blood concentrations (>1 mM) and has oxidative-promoting actions that exert anticancer effects via reactive oxygen species. Therefore, intravenous administration at high concentrations is required to achieve the desired effects on cancer cells during treatment. Partial data on the effect of ascorbic acid on fibroblasts indicate that it may also modulate collagen secretion in CAFs and impart tumor-suppressive effects. Thus, future studies should verify the effect of ascorbic acid on CAFs. The findings of this review can be used to guide further research and clinical trials.
Introduction
Ascorbic acid, also known as vitamin C, is a low-molecular-weight compound with the chemical formula C6H8O6 and a molecular weight of 176.12 g/mol. It is an essential water-soluble vitamin that cannot be synthesized in the human body (1). Instead, this vitamin must be acquired by consuming food. Inadequate provision of dietary vitamin C can lead to deficiencies such as scurvy (2–4). Ascorbic acid acts in vivo as an antioxidant and cofactor in various enzymatic reactions but has also attracted substantial attention for its potential antitumor effects (5, 6). However, the clinical efficacy of ascorbic acid as an anticancer treatment, and the mechanism behind its effects, have not yet been confirmed.
Cancer maintains its characteristic growth and progression by interacting with surrounding cells, forming a cancer microenvironment composed of various cells. Among these cells, cancer-associated fibroblasts (CAFs) play a significant role in cancer cell proliferation, invasion, and metastasis by providing growth factors and nutrients to cancer cells and reorganizing the extracellular matrix of the peri-cancer stroma (7–11). However, the effect of ascorbic acid on the cancer microenvironment is unclear. Moreover, the heterogeneity phenotype of fibroblasts in the peritumoral stroma of some carcinomas promotes tumor growth (12, 13). Therefore, elucidation of the heterogeneity of fibroblasts is urgently required for the effective destruction of cancer cells.
In this review, we discuss the differences between the antioxidant and oxidant-promoting effects of ascorbic acid, including the role of ascorbic acid concentration. Our current understanding of the concentration-dependent actions and processes of ascorbic acid is also explained. We then provide future perspectives on the antitumor effects of ascorbic acid on cancer cells and its effects on CAFs, which form a key cancer microenvironment.
Administration route and vascular concentration of ascorbic acid
Orally ingested ascorbic acid is absorbed by transporters of sodium-dependent vitamin C transporters (SVCTs) and glucose transporters (GLUTs) in the small intestine and excreted via the kidneys (14). In vivo, ascorbic acid exists as reduced ascorbic acid or oxidized ascorbic acid (dehydroascorbic acid (DHA)), which are respectively taken into cells through SVCTs and GLUTs (15–17). Rat experiments revealed variations between the oral and intravenous administration of ascorbic acid, whereby oral administration of 5 mg/g of body weight did not raise blood ascorbic acid concentrations, but intravenous administration of 5 mg/g boosted ascorbic acid concentrations to approximately 10 mM (18). However, since mice and rats can synthesize ascorbic acid in their bodies (<100 μM), it is necessary to be careful in applying the results of experiments with mice and rats to humans, whose systems cannot synthesize ascorbic acid. In human studies, oral administration of 400 mg or more of ascorbic acid maintained steady-state blood concentrations of 50–80 µM (19), with oral administration of 3 g of ascorbic acid every 4 h increasing the maximum blood concentration to approximately 220 µM. Conversely, intravenous administration of 50 g of ascorbic acid was predicted to increase the maximum blood concentration to approximately 13.4 mM (20). The half-life of ascorbic acid in the blood is 2.0 ± 0.6 h (21). Furthermore, in a report on patients with cancer, ascorbic acid concentrations in the blood reached 20.3–49.0 mM with intravenous administration of 60–70 g/m2 or 1.5 g/kg of ascorbic acid (21–23). In other words, blood concentrations of ascorbic acid vary widely depending on the route of administration. Thus, the pharmacological effects of ascorbic acid resulting from the low concentrations achieved by oral administration (several hundred μM) may differ from those resulting from the high pharmacological concentrations achieved by intravenous administration (>1 mM). As such, the intended administration route of ascorbic acid must be considered. Adverse effects of ascorbic acid include effects on renal function and hemolysis caused by a deficiency of glucose-6-phosphate dehydrogenase (G6PD). Oral doses of more than 1000 mg per day increase renal excretion of urate and oxalate compared to lower doses, so caution should be exercised when administering high doses (19). G6PD is required for the proper function of glutathione peroxidase, especially in erythrocytes (24). However, many clinical trials in which high concentrations of intravenous ascorbic acid were administered as monotherapy or in combination with anticancer agents have shown no serious adverse effects (21, 25–27). Therefore, ascorbic acid is considered a drug with very low toxicity to the human body.
In vivo effects of ascorbic acid
Recent studies have demonstrated that ascorbic acid absorbed in vivo has both antioxidant and oxidant-promoting effects (28, 29). Ascorbic acid also exhibits various physiological effects by catalyzing Fe(II)- and 2-oxoglutarate-dependent dioxygenase reactions (14).
Ascorbic acid and reactive oxygen species
Ascorbic acid degrades reactive oxygen species (ROS) at average blood concentrations of 40–80 μM, reducing low-density lipoprotein oxidation associated with atherosclerosis and lipid oxidation of cell membranes (30–32). However, high pharmacological concentrations of ascorbic acid achieved via intravenous administration produce H2O2 in vivo (18, 33, 34) and then hydroxyl radicals via the Fenton reaction (35). Intravascularly, ROS produced by high concentrations of ascorbic acid are degraded by catalase in serum, whereas extravascularly, ROS accumulate without degradation by ascorbic acid and act as a pro-oxidant. Thus, ascorbic acid is notable for its paradoxical activity, serving as an antioxidant at low doses and a pro-oxidant at high doses (28, 29). In addition, oral administration of ascorbic acid does not reach the same pharmacological concentrations as intravenous treatment (19, 20); therefore, intravenous administration of ascorbic acid is required for pro-oxidant activity to occur. In a rat study, intravenous administration of 0.5 mg/g of ascorbic acid increased the H2O2 concentration in the extracellular fluid from undetectable to 20 μM, and intraperitoneal injection of the same dose increased H2O2 concentration to approximately 5 μM. In contrast, no increase in H2O2 concentration in the extracellular fluid was detected after oral administration of ascorbic acid (18). In addition, in a mouse subcutaneous transplantation model, intraperitoneal administration of 4 mg/g of ascorbic acid increased the H2O2 concentration in the extracellular fluid around the tumor to approximately 150 μM (34, 35).
Ascorbic acid as a cofactor for dioxygenase
Members of the Fe(II) and 2-oxoglutarate-dependent dioxygenase families catalyze many oxidation reactions throughout biology. Ascorbic acid acts as a coenzyme that catalyzes the reactions that produce hydroxylation products using 2-oxoglutarate and oxygen as substrates (36). Particularly well-known are the reactions in collagen (37) and HIFα, which is a master regulator of the cellular hypoxia response pathway (38). The reaction in collagen is mediated by one of the proline hydroxylases, collagen prolyl-4-hydroxylase (C-P4H), which hydroxylates the procollagen proline (37). C-P4H has a high binding capacity to oxygen and is not affected by the oxygen concentration. Conversely, in the reactions in HIFα, ascorbic acid catalyzes two types of reactions: PHD1-3 in proline hydroxylase (38–40) and factor inhibiting HIF-1 (FIH-1) in asparagine hydroxylase (41–43). In the PHD reaction, ascorbic acid degrades HIFα via ubiquitination by pVHL proteins (44–46). In the reaction of FIH-1, it suppresses the interaction with CBP/p300, which is a transcriptional cofactor, and suppresses the transcriptional activity of HIFs (42). These reactions are dependent on the oxygen concentration; thus, ascorbic acid acts as an oxygen sensor in the cell because the reaction is reduced in a hypoxic environment and HIF is not degraded (36). The concentrations of ascorbic acid necessary to sustain enzymatic activity of PHDs and FIH-1 are 140-180 uM and 260 uM, respectively (37) and are well above the steady-state blood concentrations of 40–80 μM, suggesting that these reactions require sufficient blood and tissue concentrations of ascorbic acid (14, 47). Ascorbic acid also acts as a cofactor for ten-eleven translocations (TETs) of DNA hydroxylases; TETs are proteins that convert 5-methylcytosine (mC) to 5-hydroxymethylcytosine (hmC) (48, 49). Ascorbic acid promotes DNA demethylation by accelerating the reaction of TETs (50).
Antitumor effect of ascorbic acid
Ascorbic acid exhibits antitumor effects in various carcinomas (5, 6, 51); however, clinical studies have not yet produced any significant evidence of these effects (52). Ascorbic acid exhibits antitumor effects through ROS-mediated mechanisms and as a cofactor. The mechanisms of ascorbic acid as a cofactor include effects on HIFα via PHDs and FIH-1 and epigenetic effects via DNA demethylases (6, 49). (Table 1) Ascorbic acid can also modulate metabolism and epigenetic gene expression in immune cells as well as cancer cells (64–67). Ascorbic acid is also known to inhibit EMT of tumor cells (58, 59). Here, we discuss the known antitumor effects of ascorbic acid.
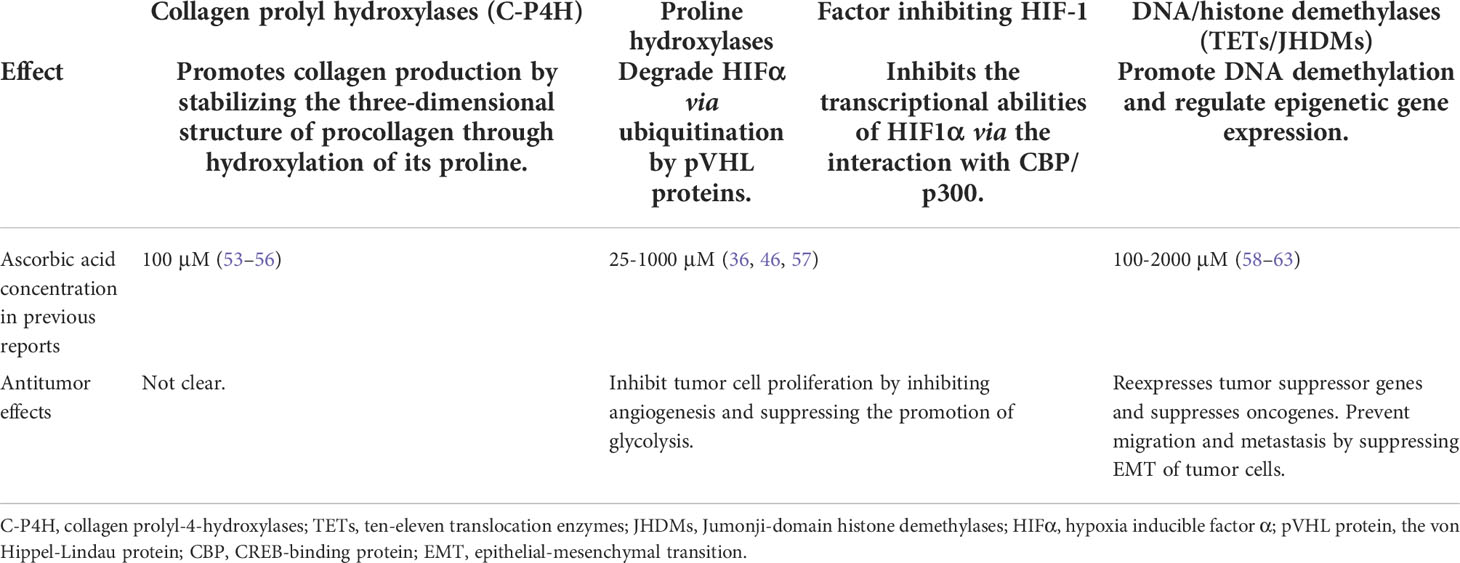
Table 1 The types and effects of Fe (II) and 2-oxoglutaric acid-dependent dioxygenases in which ascorbic acid acts as a cofactor.
ROS-mediated antitumor effects of ascorbic acid
The ROS-mediated mechanism is the most well-known mechanism of the antitumor effect of ascorbic acid in various carcinoma. Intravenous administration of high ascorbic acid concentrations acts as a pro-oxidant in vivo, producing ROS through the Fenton reaction (18). H2O2, a ROS formed outside of the cell, diffuses rapidly inside the cell (68) where it consumes antioxidants such as reduced glutathione and NADPH. In addition, in colorectal cancer with KRAS or BRAF mutations, lung cancer with KRAS mutations, and pancreatic cancer, GLUT1 expression is increased because of an accelerated glycolytic pathway, resulting in higher DHA absorption (69–72). ROS accumulation increases oxidative stress, such as DNA damage, and DNA damage increases PARP activity, thereby decreasing NAD+ levels and limiting glycolytic system processes (73, 74). In addition, GAPDH, an enzyme of the glycolytic system, is inhibited in its enzymatic function by the reversible binding of oxidized glutathione to cystein152, which is reactive to oxidative stress (75). As a result, the glycolytic pathway produces less adenosine triphosphate (ATP), and cells suffer apoptosis. Indeed, in a report of metabolic changes induced by ascorbate in a colon cancer cell line with KRAS or BRAF mutations, metabolomic analysis using LC/MS/MS showed that upstream metabolites in the glycolytic reaction catalyzed by NAD+ and GAPDH were accumulated, whereas downstream metabolites were reduced (74). Because of redox imbalance, cancer cells are susceptible to ROS and the effects of ascorbic acid (76). In conclusion, the pro-oxidant effect of high doses of ascorbic acid induces cell death by generating ROS in cancer cells and limiting ATP generation through the glycolytic pathway.
Conversely, the balance of oxidative stress and antioxidant activity plays a crucial role in tumor development and progression. In melanoma, ROS are overproduced by mitochondria or NADPH oxidase, which promotes tumor development and progression through DNA damage-induced mutation of oncogenes and signal transduction via NF-κB (77, 78). In addition, melanoma acquires metastatic potential due to enhanced production of antioxidant enzymes such as catalase and tolerance to oxidative stress (78, 79). Ascorbic acid has a dual impact on melanoma, with high concentrations triggering cell death and low amounts promoting tumor growth (80).
Despite the above reported antitumor effects of ascorbic acid at high concentrations, the ROS-mediated antitumor effects of ascorbic acid remain insufficient for the following reasons. First, the Fenton reaction-mediated ROS-generating effect of ascorbic acid, which is recognized in vitro, may be inhibited at in vivo concentrations of Fe2+ and Fe3+ (81). Second, in vivo, iron ions are always chelated, so the Fenton reaction may not occur (30). Finally, the inhibitory effect of ascorbic acid on ATP synthesis, even in the presence of PARP inhibitors in vitro, may be exerted by ascorbic acid regardless of the reduction of NAD+ levels by PARP (82). In conclusion, it is possible that in vitro results of the ROS-mediated antitumor effects of ascorbic acid are not compatible with its in vivo mode of action, suggesting that alternative anticancer mechanisms may be involved.
HIFα-mediated antitumor effects of ascorbic acid as a coenzyme
HIFα, which is expressed in many tumors such as melanoma, leukemia, and carcinomas, including colon, pancreatic, and lung cancer (83–87), is involved in angiogenesis and regulation of the glycolytic system, which are crucial processes for cancer growth and progression, suggesting that HIFα may be a novel cancer therapeutic strategy (88, 89). Ascorbic acid is a cofactor for Fe(II)- and 2-oxoglutarate-dependent dioxygenases and has various physiological effects, catalyzing the interaction of PHDs and FIH-1 and degrading the HIFα activity (44–46). Ascorbic acid concentrations in human tumor samples were negatively connected with HIF1α expression in colon cancer, with higher ascorbic acid concentrations associated with prolonged recurrence-free survival (83). In human endometrial tumors, patients with higher ascorbic acid levels in tumors had lower protein expression of HIF1α, VEGF, and GLUT1 and lower malignancy (90). In human pancreatic cancer cell lines, in vitro, low ascorbic acid concentrations (25 μM) reduced HIF1α expression and suppressed tumor growth under hypoxic conditions (57). In a model of subcutaneous lung tumor transplantation in rats, intraperitoneal injection of ascorbic acid (1 g/kg) suppressed HIF1α expression in tumors and decreased tumor growth and vascular density (91). In a mouse model of human B cell lymphoma implanted subcutaneously, oral treatment of ascorbic acid (5 g/L) reduced HIF1α expression and prevented tumor development (92).
Thus, the activity of ascorbic acid as a coenzyme may suppress HIFα expression and activity in tumor cells and may inhibit tumor cell proliferation by inhibiting angiogenesis.
Ascorbic acid regulates epigenomic modifications
Ascorbic acid catalyzes the reaction of DNA hydroxylase TETs and Jumonji C domain-containing histone demethylases (JHMDs), thereby having epigenetic antitumor effects (6, 49, 93). TET is a member of the same family of iron- and 2-oxoglutarate-dependent dioxygenases as PHDs, which convert 5-methylcytosine (5mC) into 5-hydroxymethylcytosine, promoting histone demethylation and contributing to oncogene suppression and the re-expression of tumor suppressor genes (94). In hematologic tumors such as acute myeloid leukemia and myelodysplastic syndromes, loss-of-function mutations in TET2 are known to occur frequently, resulting in decreased and hypermethylated 5hmC. In these hematologic tumors, administration of several hundred μM ascorbic acid has a gene reprogramming effect that restores TET function and increases 5hmC levels, suppressing cell proliferation and promoting myeloid progenitor cell differentiation (60, 94). In malignant melanoma, 5hmC is known to decrease as the disease progresses, and administration of 100 μM ascorbic acid restores 5hmC via TET, induces apoptosis in tumor cells, and shows antitumor effects (61, 62). For colon cancer, administration of 1 mM ascorbic acid has also been reported to increase 5hmC via TET in vitro, showing antitumor effects when combined with an inhibitor of isocitrate dehydrogenase (IDH) mutations (63). JHDMs are histone demethylases that use Fe2 + and α-ketoglutarate as cofactors to demethylate histones and regulate gene expression (95). Isocitrate dehydrogenases (IDH) mutations reduce α-ketoglutarate, a substrate for TETs and JHDMs, and promote DNA methylation in cells with IDH mutations, regulating gene expression that leads to carcinogenesis such as glioma (96). Ascorbic acid is necessary for the proper activity of JHDMs and may correct gene expression that promotes oncogenesis by promoting histone demethylation (93, 97). Essentially, ascorbic acid has antitumor effects by improving the hypermethylation state observed in tumors via TETs and JHDMs, and by reprogramming gene expression.
Ascorbic acid downregulates EMT
Ascorbic acid regulates the epithelial-mesenchymal transition (EMT), which is important for metastatic tumor potential. In vitro, ascorbic acid suppressed EMT in human pancreatic cancer cells by decreasing Snail and increasing E-cadherin at concentrations of 1-1.5 mM (98). Ascorbic acid, in conjunction with 5-azacytidine (5-AZA), a potent DNA methyltransferase inhibitor, regulated EMT inhibition and cell cycle progression in human HCC cells in vitro by suppressing Snail expression via TET (58). Interestingly, ascorbic acid produced two distinct reactions in human breast cancer in vitro. A low dose (100 μM) of ascorbic acid decreased E-cadherin and increased the mesenchymal marker vimentin, while a high dose (2 mM) of ascorbic acid conversely increased E-cadherin and decreased vimentin, reversing TGF-β 1-induced EMT and, as a result, suppressing the formation of lung metastases in vivo (59). Ascorbic acid at concentrations of 1 mM or higher is thought to suppress EMT in tumors, possibly by inhibiting the effect of TGF-β1 or by regulating Snail expression by TETs.
Effects of ascorbic acid on fibroblasts
Ascorbic acid is known to enhance collagen synthesis (99, 100) and wound healing (101), reduce UV-induced damage (102, 103), and exhibit anti-inflammatory effects (104, 105); however, these effects are primarily skin-confined. Recently, the CAF cancer microenvironment has attracted considerable attention (10, 11), although few studies have described the effects of ascorbic acid on CAFs. Here, we describe the effects of ascorbic acid on fibroblasts.
Ascorbic acid and dermal fibroblasts
Ascorbic acid acts as a cofactor for C-P4Hs when taken up by human dermal fibroblasts and promotes collagen production by stabilizing the three-dimensional structure of procollagen through hydroxylation of its proline (4, 106, 107). In vitro, a low concentration of ascorbic acid (100 μM) in human skin fibroblasts increases the expression of type1,3,4 collagen and SVCT2 at the mRNA level (53–55) as well as increasing proliferation (56), suggesting a direct effect on fibroblasts. In human clinical data, oral ascorbic acid intake with exercise stimulation doubled the amino-terminal propeptide of collagen I in the blood, indicating enhanced collagen production (108). In addition, ascorbic acid concentrations as low as 0.17 mM in human skin fibroblasts increase the contractile phenotype of myofibroblasts in the presence of TGF-β1 through enhancement of the expression of TGFb1-responsive genes, but do not increase such a phenotype in the absence of TGF-β1 (109). Ascorbic acid promotes collagen production and proliferation of skin fibroblasts as a coenzyme. Moreover, in these studies, ascorbic acid increases in collagen synthesis and secretion occurred at concentrations as low as several hundred μM.
Ascorbic acid and other fibroblast reports
According to a study on tumor stroma, intraperitoneal administration of ascorbic acid at a high dose of 4 g/kg in an orthotopically transplanted mouse model of human pancreatic cancer resulted in tumor reduction, reduced metastasis, and enhanced tumor stroma due to increased collagen secretion (98). In the 4T1 breast cancer orthotopic model utilizing ascorbic acid-deficient (gulonolactone oxidase knockout mouse) mice, oral administration of ascorbic acid increased type 1 collagen to form a capsule around the tumor, and tumor boundaries were more clearly defined than in the control group (110). Thus, ascorbic acid may increase collagen production in the tumor stroma at both high and low doses. However, it is unknown whether this effect is on tumor cells or CAFs, and further research is needed to determine whether ascorbic acid activates CAFs in the tumor microenvironment and increases collagen production. In contrast, hepatic stellate cells, which are responsible for liver fibrosis, were inhibited in vitro by low doses of ascorbic acid (50-200 M), which decreased intracellular TGF-1 in rat cell lines (111). In a report examining the development of pulmonary fibrosis by paraquat treatment, intraperitoneal administration of 150 mg/kg of ascorbic acid inhibited pulmonary fibrosis in a mouse model by inhibiting inflammatory cell infiltration into the bronchoalveolar lavage fluid, suppressing apoptosis by increasing antioxidant activity in the lung, and inhibiting TGF-β in the lung (112). As a result, ascorbic acid may inhibit fibrosis by inhibiting inflammatory cell infiltration and reduction of TGF-β in tissues. In our study, we also found that in vitro, human pancreatic-derived fibroblasts, whose proliferation is promoted when co-cultured with cancer cells, receive high doses (>1 mM) of ascorbic acid for growth inhibition. (Figure 1) In conclusion, the effects of low and high doses of ascorbic acid on CAFs, such as enhanced collagen production and inhibition of fibrosis development, differ from organ to organ or disease model to disease model and remain unclear.
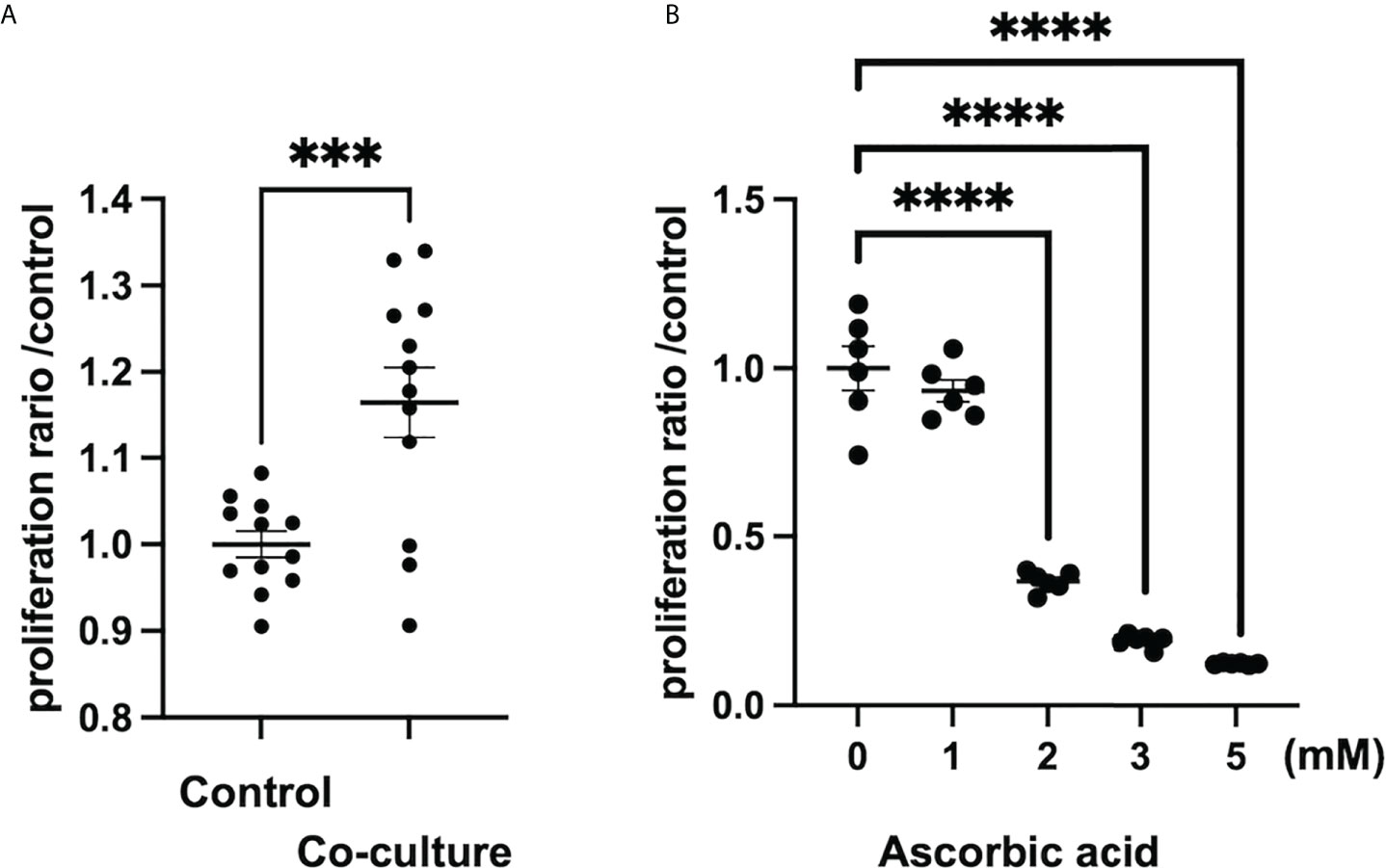
Figure 1 Ascorbic acid reduces the proliferation of human-derived pancreatic fibroblasts (hPFs). (A) Proliferation of hPFs is increased in a co-culture with a pancreatic cancer cell line (MIAPaCa2). ***P < 0.001 versus control, means ± SEM, n = 12. (B) Proliferation of hPFs is dose-dependently reduced by high-dose ascorbic acid treatment. ****P < 0.0001 versus ascorbic acid 0 mM, means ± SEM, n = 6. Statistical analysis was performed by GraphPad Prism 9 and significance was determined by Student’s t-test.
Clinical trials on ascorbic acid
In the 1970s, clinical trials involving ascorbic acid revealed that a small sample of patients treated with intravenous and oral ascorbic acid lived longer than a control group (113, 114). At that time, the mechanism of the antitumor effect of ascorbic acid efficacy remained unclear, and subsequent randomized, double-blind, placebo-controlled trials with oral ascorbic acid failed to demonstrate a survival benefit (115, 116). Therefore, the antitumor effect of ascorbic acid was viewed unfavorably. Multiple mechanisms of ascorbic acid’s antitumor effect were subsequently proven in vitro, along with differences in ascorbic acid blood levels between oral and intravenous administration methods. Furthermore, the fact that blood levels of ascorbic acid were decreased in cancer patients (117, 118) and that the adverse effects associated with ascorbic acid administration were extremely low, led to the expectation that ascorbic acid could be used for therapeutic applications. (Table 2) There were a few scattered case reports showing tumor shrinkage with ascorbic acid treatment (131, 153–158), and there were also reports of antitumor effects in a small number of studies (25, 124, 159, 160). Ascorbic acid in combination with chemotherapeutic agents has also been researched, and some reports of reduced side effects and improved quality of life have been observed (21, 161, 162). In contrast, there have been no large-scale clinical trials that have demonstrated an additional antitumor effect by ascorbic acid (6, 76, 131, 163), and several ongoing clinical trials of ascorbic acid alone or in combination with chemotherapeutic agents for advanced colon cancer, pancreatic cancer, lung cancer, and other malignancies are expected to provide results in the near future (140, 148, 149, 151) (Table 2).
Discussion
Ascorbic acid is a medicine that has been widely investigated and used for a long time; however, its beneficial effects against cancer have not yet been proven by clinical trials. The contrasting in vivo effects of ascorbic acid may explain this. That is, the oxidative-promoting impact at high concentrations is detrimental to cancer cells, whereas the antioxidant effect at low concentrations may promote cancer (164). Because of this paradoxical effect, the administration route of ascorbic acid should be carefully considered. In addition, future research should explain the different activities of multiple dioxylases as cofactors, such as HIFα degradation, immune cell modulation, and epigenetic regulation of gene expression, in relation to the cancer microenvironment (Figure 2).
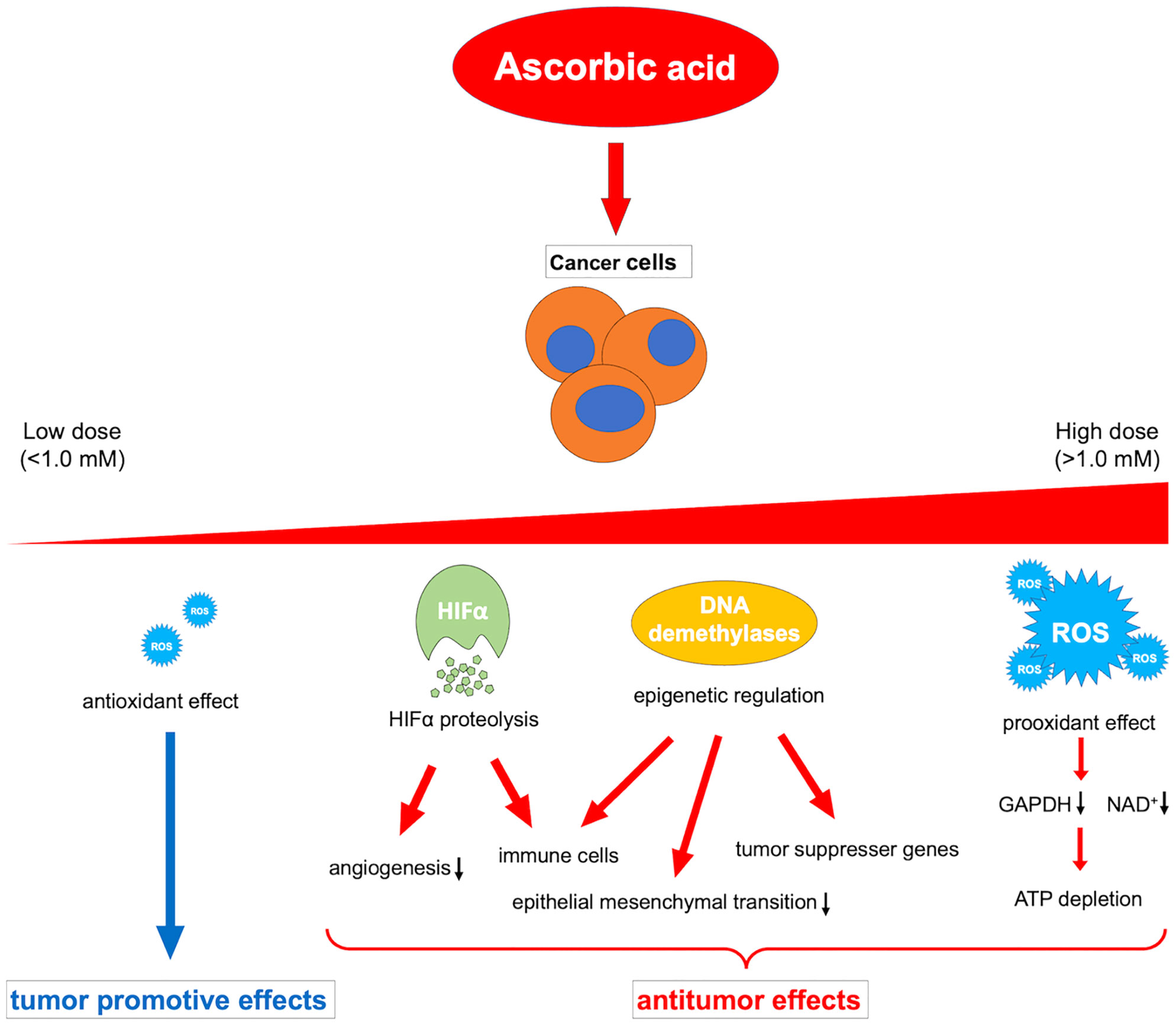
Figure 2 Overview of the various dose-dependent effects of ascorbic acid on cancer. ROS, reactive oxygen species; HIFα, hypoxia-inducible factor-alpha; GAPDH, glyceraldehyde 3-phosphate dehydrogenase; NAD+, nicotinamide adenine dinucleotide.
Additionally, research on the effects of ascorbic acid on CAFs implies the existence of novel therapeutic possibilities. Since the diversity of gene expression in fibroblasts in vivo differs among organs and pathological conditions (165), the effects of ascorbic acid on CAFs are also expected to vary among organs and pathological conditions. One of the potential effects of ascorbic acid may be the inhibition of tumor-promoting CAFs. Tumor-promoting CAFs support cancer growth by supplying cancer cells with nutrients and growth factors (7–11). Moreover, tumor-promoting CAFs control ECM secretion and protease secretion, remodel the ECM, and generate invasive routes necessary for solid tumor invasion (166, 167). Furthermore, in tumors with a high stromal component, such as pancreatic and breast cancer, the stromal fluid pressure in the tumor area increases, reducing drug delivery and indicating resistance to treatment (168, 169). Tumor-promoting CAFs promote cancer through cross-talk functions with cancer cells, ECM remodeling functions, and physical drug barrier functions. Ascorbic acid has an inhibitory effect on fibroblasts through a reduction in TGF at low doses and an inhibitory effect on cell proliferation via a prooxidant effect at higher doses, suggesting that it may have an inhibitory effect on tumor-promoting CAFs.
Conversely, collagen is known to form a barrier that physically obstructs cell migration without protease degradation (170, 171). In a mouse model lacking -SMA-positive fibroblasts, the tumor suppressive effects of CAFs have been demonstrated to induce an undifferentiated tumor phenotype and dramatically reduce survival (172). The increase in cancer stroma, tumor shrinkage, and metastasis inhibition effects of ascorbic acid may be attributed to the activation of tumor suppressive fibroblasts and the formation of collagen barriers that inhibit tumor progression.
Ascorbic acid may also affect CAFs via suppression of HIF1α. Tumor-induced ROS-mediated “pseudo-hypoxia” in CAFs leads to the accumulation of HIF1α and enhanced aerobic glycolysis (173, 174). Furthermore, high expression of HIF1α in CAFs induces protein expression in myofibroblasts in CAFs, and inhibition or knockout of HIF1α improves their phenotype (175). Stimulation by TGF-β or PDGF also suppresses IDH3 expression and decreases 2-oxoglutarate in fibroblasts, resulting in HIF1α accumulation and regulating fibroblast differentiation into CAFs (176). Ascorbic acid may inhibit the accumulation of HIF1α by promoting the reaction of 2-oxoglutarate-dependent dioxygenases such as PHDs and FIH-1, thereby suppressing fibroblast differentiation into CAFs. The JAK1/STAT3 pathway is also an important pathway that maintains actomyosin contractility and the CAF phenotype (177), and methylation of the promoter of protein tyrosine phosphatase non-receptor type 6 (SHP-1), which negatively regulates the JAK/STAT pathway, allowing for sustained signaling (167). For this epigenetic reorganization, DNA demethylase-mediated effects such as TETs of ascorbic acid may be exerted. However, CAFs have an enhanced glycolytic system due to chronic hypoxia in the tumor microenvironment and subsequent epigenetic reorganization by demethylation of HIF1α and promoters of enzymes of the glycolytic system (178), and there may be unexpected epigenetic effects of ascorbic acid that should be clarified in the future. Ascorbic acid may have a tumor suppressive effect by affecting CAFs and reprogramming them into normal fibroblasts. (Figure 3) It is possible that the antitumor effect of ascorbic acid can be improved by examining the method of administration and adapting it to the expression status of HIF in tumors and CAFs. Furthermore, elucidating the effects of ascorbic acid targeting not only tumor cells but also tumor microenvironments such as CAFs may help to reveal further antitumor effects of ascorbic acid.
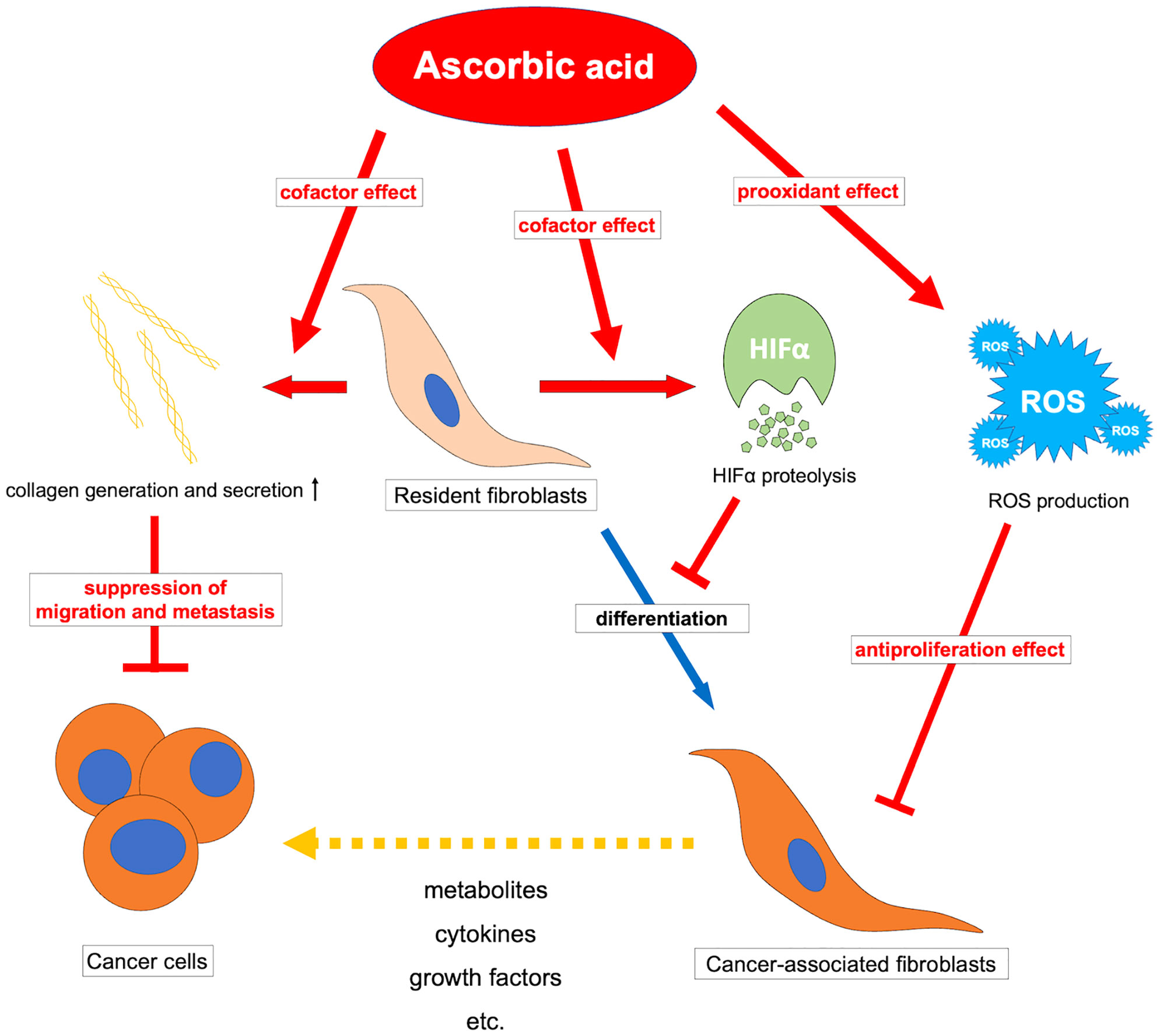
Figure 3 Overview of the antitumor effects of ascorbic acid on cancer-associated fibroblasts. ROS, reactive oxygen species; HIFα, hypoxia-inducible factor-alpha.
Author contributions
TMa and TMi drafted the manuscript, which was subsequently critically revised by MT and SU. All authors contributed to the article and approved the submitted version.
Funding
This work was supported by JSPS KAKENHI (grant Nos. 22K08769).
Acknowledgments
The authors would like to thank Ikuko Arikawa, Yumiko Ito, and Sachiko Sawada for technical assistance with the experiments. Furthermore, we would like to thank Editage (www.editage.jp) for English language editing.
Conflict of interest
The authors declare that the research was conducted in the absence of any commercial or financial relationships that could be construed as a potential conflict of interest.
Publisher’s note
All claims expressed in this article are solely those of the authors and do not necessarily represent those of their affiliated organizations, or those of the publisher, the editors and the reviewers. Any product that may be evaluated in this article, or claim that may be made by its manufacturer, is not guaranteed or endorsed by the publisher.
References
1. Nishikimi M, Koshizaka T, Ozawa T, Yagi K. Occurrence in humans and guinea pigs of the gene related to their missing enzyme l-gulono-gamma-lactone oxidase. Arch Biochem Biophys (1988) 267:842–6. doi: 10.1016/0003-9861(88)90093-8
3. Padayatty SJ, Katz A, Wang Y, Eck P, Kwon O, Lee JH, et al. Vitamin c as an antioxidant: evaluation of its role in disease prevention. J Am Coll Nutr (2003) 22:18–35. doi: 10.1080/07315724.2003.10719272
4. Peterkofsky B. Ascorbate requirement for hydroxylation and secretion of procollagen: relationship to inhibition of collagen synthesis in scurvy. Am J Clin Nutr (1991) 54:1135S–40S. doi: 10.1093/ajcn/54.6.1135s
5. Villagran M, Ferreira J, Martorell M, Mardones L. The role of vitamin c in cancer prevention and therapy: A literature review. Antioxid (Basel) (2021) 10:1894. doi: 10.3390/antiox10121894
6. Böttger F, Vallés-Martí A, Cahn L, Jimenez CR. High-dose intravenous vitamin c, a promising multi-targeting agent in the treatment of cancer. J Exp Clin Cancer Res (2021) 40:343. doi: 10.1186/s13046-021-02134-y
7. Sousa CM, Biancur DE, Wang X, Halbrook CJ, Sherman MH, Zhang L, et al. Pancreatic stellate cells support tumour metabolism through autophagic alanine secretion. Nature (2016) 536:479–83. doi: 10.1038/nature19084
8. Parker SJ, Amendola CR, Hollinshead KER, Yu Q, Yamamoto K, Encarnación-Rosado J, et al. Selective alanine transporter utilization creates a targetable metabolic niche in pancreatic cancer. Cancer Discov (2020) 10:1018–37. doi: 10.1158/2159-8290.CD-19-0959
9. Ikuta D, Miyake T, Shimizu T, Sonoda H, Mukaisho KI, Tokuda A, et al. Fibrosis in metastatic lymph nodes is clinically correlated to poor prognosis in colorectal cancer. Oncotarget (2018) 9:29574–86. doi: 10.18632/oncotarget.25636
10. Sahai E, Astsaturov I, Cukierman E, DeNardo DG, Egeblad M, Evans RM, et al. A framework for advancing our understanding of cancer-associated fibroblasts. Nat Rev Cancer (2020) 20:174–86. doi: 10.1038/s41568-019-0238-1
11. Kalluri R, Zeisberg M. Fibroblasts in cancer. Nat Rev Cancer (2006) 6:392–401. doi: 10.1038/nrc1877
12. Maehira H, Miyake T, Iida H, Tokuda A, Mori H, Yasukawa D, et al. Vimentin expression in tumor microenvironment predicts survival in pancreatic ductal adenocarcinoma: heterogeneity in the fibroblast population. Ann Surg Oncol (2019) 26:4791–804. doi: 10.1245/s10434-019-07891-x
13. Mizutani Y, Kobayashi H, Iida T, Asai N, Masamune A, Hara A, et al. Meflin-positive cancer-associated fibroblasts inhibit pancreatic carcinogenesis. Cancer Res (2019) 79:5367–81. doi: 10.1158/0008-5472.CAN-19-0454
14. Padayatty SJ, Levine M. Vitamin c physiology: the known and the unknown and goldilocks. Oral Dis (2016) 22:463–93. doi: 10.1111/odi.12446
15. Tsukaguchi H, Tokui T, Mackenzie B, Berger UV, Chen XZ, Wang Y, et al. A family of mammalian na+-dependent l-ascorbic acid transporters. Nature (1999) 399:70–5. doi: 10.1038/19986
16. Wang Y, Mackenzie B, Tsukaguchi H, Weremowicz S, Morton CC, Hediger MA. Human vitamin c (L-ascorbic acid) transporter SVCT1. Biochem Biophys Res Commun (2000) 267:488–94. doi: 10.1006/bbrc.1999.1929
17. Muñoz-Montesino C, Peña E, Roa FJ, Sotomayor K, Escobar E, Rivas CI. Transport of vitamin c in cancer. Antioxid Redox Signal (2021) 35:61–74. doi: 10.1089/ars.2020.8166
18. Chen Q, Espey MG, Sun AY, Lee JH, Krishna MC, Shacter E, et al. Ascorbate in pharmacologic concentrations selectively generates ascorbate radical and hydrogen peroxide in extracellular fluid in vivo. Proc Natl Acad Sci USA (2007) 104:8749–54. doi: 10.1073/pnas.0702854104
19. Levine M, Conry-Cantilena C, Wang Y, Welch RW, Washko PW, Dhariwal KR, et al. Vitamin c pharmacokinetics in healthy volunteers: evidence for a recommended dietary allowance. Proc Natl Acad Sci USA (1996) 93:3704–9. doi: 10.1073/pnas.93.8.3704
20. Padayatty SJ, Sun H, Wang Y, Riordan HD, Hewitt SM, Katz A, et al. Vitamin c pharmacokinetics: implications for oral and intravenous use. Ann Intern Med (2004) 140:533–7. doi: 10.7326/0003-4819-140-7-200404060-00010
21. Stephenson CM, Levin RD, Spector T, Lis CG. Phase I clinical trial to evaluate the safety, tolerability, and pharmacokinetics of high-dose intravenous ascorbic acid in patients with advanced cancer. Cancer Chemother Pharmacol (2013) 72:139–46. doi: 10.1007/s00280-013-2179-9
22. Nielsen TK, Højgaard M, Andersen JT, Poulsen HE, Lykkesfeldt J, Mikines KJ. Elimination of ascorbic acid after high-dose infusion in prostate cancer patients: A pharmacokinetic evaluation. Basic Clin Pharmacol Toxicol (2015) 116:343–8. doi: 10.1111/bcpt.12323
23. Hoffer LJ, Levine M, Assouline S, Melnychuk D, Padayatty SJ, Rosadiuk K, et al. Phase I clinical trial of i.v. ascorbic acid in advanced malignancy. Ann Oncol (2008) 19:1969–74. doi: 10.1093/annonc/mdn377
24. Quinn J, Gerber B, Fouche R, Kenyon K, Blom Z, Muthukanagaraj P. Effect of high-dose vitamin c infusion in a glucose-6-Phosphate dehydrogenase-deficient patient. Case Rep Med (2017) 2017:5202606. doi: 10.1155/2017/5202606
25. Monti DA, Mitchell E, Bazzan AJ, Littman S, Zabrecky G, Yeo CJ, et al. Phase I evaluation of intravenous ascorbic acid in combination with gemcitabine and erlotinib in patients with metastatic pancreatic cancer. PLoS One (2012) 7:e29794. doi: 10.1371/journal.pone.0029794
26. Hoffer LJ, Robitaille L, Zakarian R, Melnychuk D, Kavan P, Agulnik J, et al. High-dose intravenous vitamin c combined with cytotoxic chemotherapy in patients with advanced cancer: a phase I-II clinical trial. PLoS One (2015) 10:e0120228. doi: 10.1371/journal.pone.0120228
27. Nauman G, Gray JC, Parkinson R, Levine M, Paller CJ. Systematic review of intravenous ascorbate in cancer clinical trials. Antioxid (Basel) (2018) 7:89. doi: 10.3390/antiox7070089
28. Du J, Cullen JJ, Buettner GR. Ascorbic acid: chemistry, biology and the treatment of cancer. Biochim Biophys Acta (2012) 1826:443–57. doi: 10.1016/j.bbcan.2012.06.003
29. Kaźmierczak-Barańska J, Boguszewska K, Adamus-Grabicka A, Karwowski BT. Two faces of vitamin c-antioxidative and pro-oxidative agent. Nutrients (2020) 12:1501. doi: 10.3390/nu12051501
30. Ďuračková Z. Some current insights into oxidative stress. Physiol Res (2010) 59:459–69. doi: 10.33549/physiolres.931844
31. Santos KLB, Bragança VAN, Pacheco LV, Ota SSB, Aguiar CPO, Borges RS. Essential features for antioxidant capacity of ascorbic acid (vitamin c). J Mol Model (2021) 28:1. doi: 10.1007/s00894-021-04994-9
32. Gale CR, Ashurst HE, Powers HJ, Martyn CN. Antioxidant vitamin status and carotid atherosclerosis in the elderly. Am J Clin Nutr (2001) 74:402–8. doi: 10.1093/ajcn/74.3.402
33. Chen Q, Espey MG, Krishna MC, Mitchell JB, Corpe CP, Buettner GR, et al. Pharmacologic ascorbic acid concentrations selectively kill cancer cells: action as a pro-drug to deliver hydrogen peroxide to tissues. Proc Natl Acad Sci USA (2005) 102:13604–9. doi: 10.1073/pnas.0506390102
34. Chen Q, Espey MG, Sun AY, Pooput C, Kirk KL, Krishna MC, et al. Pharmacologic doses of ascorbate act as a pro-oxidant and decrease growth of aggressive tumor xenografts in mice. Proc Natl Acad Sci USA (2008) 105:11105–9. doi: 10.1073/pnas.0804226105
35. Pawlowska E, Szczepanska J, Blasiak J. Pro- and antioxidant effects of vitamin c in cancer in correspondence to its dietary and pharmacological concentrations. Oxid Med Cell Longev (2019) 2019:7286737. doi: 10.1155/2019/7286737
36. Ozer A, Bruick RK. Non-heme dioxygenases: cellular sensors and regulators jelly rolled into one? Nat Chem Biol (2007) 3:144–53. doi: 10.1038/nchembio863
37. Myllyharju J, Kivirikko KI. Characterization of the iron- and 2-oxoglutarate-binding sites of human prolyl 4-hydroxylase. EMBO J (1997) 16:1173–80. doi: 10.1093/emboj/16.6.1173
38. Bruick RK, McKnight SL. A conserved family of prolyl-4-hydroxylases that modify HIF. Science (2001) 294:1337–40. doi: 10.1126/science.1066373
39. Epstein ACR, Gleadle JM, McNeill LA, Hewitson KS, O’Rourke J, Mole DR, et al. C. elegans EGL-9 and mammalian homologs define a family of dioxygenases that regulate HIF by prolyl hydroxylation. Cell (2001) 107:43–54. doi: 10.1016/S0092-8674(01)00507-4
40. Ivan M, Haberberger T, Gervasi DC, Michelson KS, Günzler V, Kondo K, et al. Biochemical purification and pharmacological inhibition of a mammalian prolyl hydroxylase acting on hypoxia-inducible factor. Proc Natl Acad Sci USA (2002) 99:13459–64. doi: 10.1073/pnas.192342099
41. Hewitson KS, McNeill LA, Riordan MV, Tian YM, Bullock AN, Welford RW, et al. Hypoxia-inducible factor (HIF) asparagine hydroxylase is identical to factor inhibiting HIF (FIH) and is related to the cupin structural family. J Biol Chem (2002) 277:26351–5. doi: 10.1074/jbc.C200273200
42. Lando D, Peet DJ, Gorman JJ, Whelan DA, Whitelaw ML, Bruick RK. FIH-1 is an asparaginyl hydroxylase enzyme that regulates the transcriptional activity of hypoxia-inducible factor. Genes Dev (2002) 16:1466–71. doi: 10.1101/gad.991402
43. Rani S, Roy S, Singh M, Kaithwas G. Regulation of transactivation at c-TAD domain of HIF-1 alpha by factor-inhibiting HIF-1 alpha (FIH-1): A potential target for therapeutic intervention in cancer. Oxid Med Cell Longev (2022) 2022:2407223. doi: 10.1155/2022/2407223
44. Jaakkola P, Mole DR, Tian YM, Wilson MI, Gielbert J, Gaskell SJ, et al. Targeting of HIF-alpha to the von hippel-lindau ubiquitylation complex by O2-regulated prolyl hydroxylation. Science (2001) 292:468–72. doi: 10.1126/science.1059796
45. Cockman ME, Masson N, Mole DR, Jaakkola P, Chang GW, Clifford SC, et al. Hypoxia inducible factor-alpha binding and ubiquitylation by the von hippel-lindau tumor suppressor protein. J Biol Chem (2000) 275:25733–41. doi: 10.1074/jbc.M002740200
46. Kuiper C, Dachs GU, Currie MJ, Vissers MC. Intracellular ascorbate enhances hypoxia-inducible factor (HIF)-hydroxylase activity and preferentially suppresses the HIF-1 transcriptional response. Free Radic Biol Med (2014) 69:308–17. doi: 10.1016/j.freeradbiomed.2014.01.033
47. Lykkesfeldt J, Tveden-Nyborg P. The pharmacokinetics of vitamin c. Nutrients (2019) 11:2412. doi: 10.3390/nu11102412
48. Tahiliani M, Koh KP, Shen Y, Pastor WA, Bandukwala H, Brudno Y, et al. Conversion of 5-methylcytosine to 5-hydroxymethylcytosine in mammalian DNA by MLL partner TET1. Science (2009) 324:930–5. doi: 10.1126/science.1170116
49. Camarena V, Wang G. The epigenetic role of vitamin c in health and disease. Cell Mol Life Sci (2016) 73:1645–58. doi: 10.1007/s00018-016-2145-x
50. Blaschke K, Ebata KT, Karimi MM, Zepeda-Martínez JA, Goyal P, Mahapatra S, et al. Vitamin c induces tet-dependent DNA demethylation and a blastocyst-like state in ES cells. Nature (2013) 500:222–6. doi: 10.1038/nature12362
51. Hamaguchi R, Ito T, Narui R, Morikawa H, Uemoto S, Wada H. Effects of alkalization therapy on chemotherapy outcomes in advanced pancreatic cancer: A retrospective case-control study. In Vivo (2020) 34:2623–9. doi: 10.21873/invivo.12080
52. van Gorkom GNY, Lookermans EL, Van Elssen CHMJ, Bos GMJ. The effect of vitamin c (ascorbic acid) in the treatment of patients with cancer: A systematic review. Nutrients (2019) 11:977. doi: 10.3390/nu11050977
53. Kishimoto Y, Saito N, Kurita K, Shimokado K, Maruyama N, Ishigami A. Ascorbic acid enhances the expression of type 1 and type 4 collagen and SVCT2 in cultured human skin fibroblasts. Biochem Biophys Res Commun (2013) 430:579–84. doi: 10.1016/j.bbrc.2012.11.110
54. Tajima S, Pinnell SR. Ascorbic acid preferentially enhances type I and III collagen gene transcription in human skin fibroblasts. J Dermatol Sci (1996) 11:250–3. doi: 10.1016/0923-1811(95)00640-0
55. Maione-Silva L, de Castro EG, Nascimento TL, Cintra ER, Moreira LC, Cintra BAS, et al. Ascorbic acid encapsulated into negatively charged liposomes exhibits increased skin permeation, retention and enhances collagen synthesis by fibroblasts. Sci Rep (2019) 9:522. doi: 10.1038/s41598-018-36682-9
56. Phillips CL, Combs SB, Pinnell SR. Effects of ascorbic acid on proliferation and collagen synthesis in relation to the donor age of human dermal fibroblasts. J Invest Dermatol (1994) 103:228–32. doi: 10.1111/1523-1747.ep12393187
57. Chen C, Tang P, Yue J, Ren P, Liu X, Zhao X, et al. Effect of siRNA targeting HIF-1alpha combined l-ascorbate on biological behavior of hypoxic MiaPaCa2 cells. Technol Cancer Res Treat (2009) 8:235–40. doi: 10.1177/153303460900800309
58. Sajadian SO, Tripura C, Samani FS, Ruoss M, Dooley S, Baharvand H, et al. Vitamin c enhances epigenetic modifications induced by 5-azacytidine and cell cycle arrest in the hepatocellular carcinoma cell lines HLE and Huh7. Clin Epigenet (2016) 8:46. doi: 10.1186/s13148-016-0213-6
59. Zeng LH, Wang QM, Feng LY, Ke YD, Xu QZ, Wei AY, et al. High-dose vitamin c suppresses the invasion and metastasis of breast cancer cells via inhibiting epithelial-mesenchymal transition. Onco Targets Ther (2019) 12:7405–13. doi: 10.2147/OTT.S222702
60. Mingay M, Chaturvedi A, Bilenky M, Cao Q, Jackson L, Hui T, et al. Vitamin c-induced epigenomic remodelling in IDH1 mutant acute myeloid leukaemia. Leukemia (2018) 32:11–20. doi: 10.1038/leu.2017.171
61. Lian CG, Xu Y, Ceol C, Wu F, Larson A, Dresser K, et al. Loss of 5-hydroxymethylcytosine is an epigenetic hallmark of melanoma. Cell (2012) 150:1135–46. doi: 10.1016/j.cell.2012.07.033
62. Mustafi S, Sant DW, Liu ZJ, Wang G. Ascorbate induces apoptosis in melanoma cells by suppressing clusterin expression. Sci Rep (2017) 7:3671. doi: 10.1038/s41598-017-03893-5
63. Gerecke C, Schumacher F, Berndzen A, Homann T, Kleuser B. Vitamin c in combination with inhibition of mutant IDH1 synergistically activates TET enzymes and epigenetically modulates gene silencing in colon cancer cells. Epigenetics (2020) 15:307–22. doi: 10.1080/15592294.2019.1666652
64. van Gorkom GNY, Klein Wolterink RGJ, Van Elssen CHMJ, Wieten L, Germeraad WTV, Bos GMJ. Influence of vitamin c on lymphocytes: an overview. Antioxid (Basel) (2018) 7:41. doi: 10.3390/antiox7030041
65. Ang A, Pullar JM, Currie MJ, Vissers MCM. Vitamin c and immune cell function in inflammation and cancer. Biochem Soc Trans (2018) 46:1147–59. doi: 10.1042/BST20180169
66. McPherson RC, Konkel JE, Prendergast CT, Thomson JP, Ottaviano R, Leech MD, et al. Epigenetic modification of the PD-1 (Pdcd1) promoter in effector CD4+ T cells tolerated by peptide immunotherapy. Elife (2014) 3:e03416. doi: 10.7554/eLife.03416
67. Minor EA, Court BL, Young JI, Wang G. Ascorbate induces ten-eleven translocation (Tet) methylcytosine dioxygenase-mediated generation of 5-hydroxymethylcytosine. J Biol Chem (2013) 288:13669–74. doi: 10.1074/jbc.C113.464800
68. Antunes F, Cadenas E. Estimation of H2O2 gradients across biomembranes. FEBS Lett (2000) 475:121–6. doi: 10.1016/S0014-5793(00)01638-0
69. Kawada K, Nakamoto Y, Kawada M, Hida K, Matsumoto T, Murakami T, et al. Relationship between 18F-fluorodeoxyglucose accumulation and KRAS/BRAF mutations in colorectal cancer. Clin Cancer Res (2012) 18:1696–703. doi: 10.1158/1078-0432.CCR-11-1909
70. Yun J, Rago C, Cheong I, Pagliarini R, Angenendt P, Rajagopalan H, et al. Glucose deprivation contributes to the development of KRAS pathway mutations in tumor cells. Science (2009) 325:1555–9. doi: 10.1126/science.1174229
71. Sasaki H, Shitara M, Yokota K, Hikosaka Y, Moriyama S, Yano M, et al. Overexpression of GLUT1 correlates with kras mutations in lung carcinomas. Mol Med Rep (2012) 5:599–602. doi: 10.3892/mmr.2011.736
72. Bryant KL, Mancias JD, Kimmelman AC, Der CJ. KRAS: feeding pancreatic cancer proliferation. Trends Biochem Sci (2014) 39:91–100. doi: 10.1016/j.tibs.2013.12.004
73. Alano CC, Garnier P, Ying W, Higashi Y, Kauppinen TM, Swanson RA. NAD+ depletion is necessary and sufficient for poly(ADP-ribose) polymerase-1-mediated neuronal death. J Neurosci (2010) 30:2967–78. doi: 10.1523/JNEUROSCI.5552-09.2010
74. Yun J, Mullarky E, Lu C, Bosch KN, Kavalier A, Rivera K, et al. Vitamin c selectively kills KRAS and BRAF mutant colorectal cancer cells by targeting GAPDH. Science (2015) 350:1391–6. doi: 10.1126/science.aaa5004
75. Hwang NR, Yim SH, Kim YM, Jeong J, Song EJ, Lee Y, et al. Oxidative modifications of glyceraldehyde-3-phosphate dehydrogenase play a key role in its multiple cellular functions. Biochem J (2009) 423:253–64. doi: 10.1042/BJ20090854
76. Ngo B, Van Riper JM, Cantley LC, Yun J. Targeting cancer vulnerabilities with high-dose vitamin c. Nat Rev Cancer (2019) 19:271–82. doi: 10.1038/s41568-019-0135-7
77. Brar SS, Kennedy TP, Whorton AR, Sturrock AB, Huecksteadt TP, Ghio AJ, et al. Reactive oxygen species from NAD(P)H:quinone oxidoreductase constitutively activate NF-kappaB in malignant melanoma cells. Am J Physiol Cell Physiol (2001) 280:C659–76. doi: 10.1152/ajpcell.2001.280.3.C659
78. Sagwal SK, Bekeschus S. ROS pleiotropy in melanoma and local therapy with physical modalities. Oxid Med Cell Longev (2021) 2021:6816214. doi: 10.1155/2021/6816214
79. Baldi A, Lombardi D, Russo P, Palescandolo E, De Luca A, Santini D, et al. Ferritin contributes to melanoma progression by modulating cell growth and sensitivity to oxidative stress. Clin Cancer Res (2005) 11:3175–83. doi: 10.1158/1078-0432.CCR-04-0631
80. Yang G, Yan Y, Ma Y, Yang Y. Vitamin c at high concentrations induces cytotoxicity in malignant melanoma but promotes tumor growth at low concentrations. Mol Carcinog (2017) 56:1965–76. doi: 10.1002/mc.22654
81. Murayama Y, Kashiyagura R, Ohmomi E, Ishida Y, Shinada T, Satoh T. Fe2+ as a physiological and selective inhibitor of vitamin c-induced cancer cell death. React Oxygen Species (2020) 10:180–96. doi: 10.20455/ros.2020.833
82. Du J, Martin SM, Levine M, Wagner BA, Buettner GR, Wang SH, et al. Mechanisms of ascorbate-induced cytotoxicity in pancreatic cancer. Clin Cancer Res (2010) 16:509–20. doi: 10.1158/1078-0432.CCR-09-1713
83. Kuiper C, Dachs GU, Munn D, Currie MJ, Robinson BA, Pearson JF, et al. Increased tumor ascorbate is associated with extended disease-free survival and decreased hypoxia-inducible factor-1 activation in human colorectal cancer. Front Oncol (2014) 4:10. doi: 10.3389/fonc.2014.00010
84. Dang DT, Chen F, Gardner LB, Cummins JM, Rago C, Bunz F, et al. Hypoxia-inducible factor-1A promotes nonhypoxia-mediated proliferation in colon cancer cells and xenografts. Cancer Res (2006) 66:1684–693. doi: 10.1158/0008-5472.CAN-05-2887
85. Shukla SK, Purohit V, Mehla K, Gunda V, Chaika NV, Vernucci E, et al. MUC1 and HIF-1alpha signaling crosstalk induces anabolic glucose metabolism to impart gemcitabine resistance to pancreatic cancer. Cancer Cell (2017) 32:71–87.e7. doi: 10.1016/j.ccell.2017.06.004
86. Giatromanolaki A, Koukourakis MI, Sivridis E, Turley H, Talks K, Pezzella F, et al. Relation of hypoxia inducible factor 1 alpha and 2 alpha in operable non-small cell lung cancer to angiogenic/molecular profile of tumours and survival. Br J Cancer (2001) 85:881–90. doi: 10.1054/bjoc.2001.2018
87. Semenza GL. Defining the role of hypoxia-inducible factor 1 in cancer biology and therapeutics. Oncogene (2010) 29:625–34. doi: 10.1038/onc.2009.441
88. Jin X, Dai L, Ma Y, Wang J, Liu Z. Implications of HIF-1α in the tumorigenesis and progression of pancreatic cancer. Cancer Cell Int (2020) 20:273. doi: 10.1186/s12935-020-01370-0
89. Semenza GL. HIF-1 mediates metabolic responses to intratumoral hypoxia and oncogenic mutations. J Clin Invest (2013) 123:3664–71. doi: 10.1172/JCI67230
90. Kuiper C, Molenaar IG, Dachs GU, Currie MJ, Sykes PH, Vissers MC. Low ascorbate levels are associated with increased hypoxia-inducible factor-1 activity and an aggressive tumor phenotype in endometrial cancer. Cancer Res (2010) 70:5749–58. doi: 10.1158/0008-5472.CAN-10-0263
91. Campbell EJ, Vissers MCM, Wohlrab C, Hicks KO, Strother RM, Bozonet SM, et al. Pharmacokinetic and anticancer properties of high dose ascorbate in solid tumours of ascorbate-dependent mice. Free Radic Biol Med (2016) 99:451–62. doi: 10.1016/j.freeradbiomed.2016.08.027
92. Gao P, Zhang H, Dinavahi R, Li F, Xiang Y, Raman V, et al. HIF-dependent antitumorigenic effect of antioxidants in vivo. Cancer Cell (2007) 12:230–8. doi: 10.1016/j.ccr.2007.08.004
93. Lee Chong T, Ahearn EL, Cimmino L. Reprogramming the epigenome with vitamin c. Front Cell Dev Biol (2019) 7:128. doi: 10.3389/fcell.2019.00128
94. Kroeze LI, van der Reijden BA, Jansen JH. 5-hydroxymethylcytosine: An epigenetic mark frequently deregulated in cancer. Biochim Biophys Acta (2015) 1855:144–54. doi: 10.1016/j.bbcan.2015.01.001
95. Franci G, Ciotta A, Altucci L. The jumonji family: past, present and future of histone demethylases in cancer. Biomol Concepts (2014) 5:209–24. doi: 10.1515/bmc-2014-0010
96. Turcan S, Rohle D, Goenka A, Walsh LA, Fang F, Yilmaz E, et al. IDH1 mutation is sufficient to establish the glioma hypermethylator phenotype. Nature (2012) 483:479–83. doi: 10.1038/nature10866
97. Tsukada Y, Fang J, Erdjument-Bromage H, Warren ME, Borchers CH, Tempst P, et al. Histone demethylation by a family of JmjC domain-containing proteins. Nature (2006) 439:811–6. doi: 10.1038/nature04433
98. Polireddy K, Dong R, Reed G, Yu J, Chen P, Williamson S, et al. High dose parenteral ascorbate inhibited pancreatic cancer growth and metastasis: mechanisms and a phase I/IIa study. Sci Rep (2017) 7:17188. doi: 10.1038/s41598-017-17568-8
99. Wang K, Jiang H, Li W, Qiang M, Dong T, Li H. Role of vitamin c in skin diseases. Front Physiol (2018) 9:819. doi: 10.3389/fphys.2018.00819
100. Boyera N, Galey I, Bernard BA. Effect of vitamin c and its derivatives on collagen synthesis and cross-linking by normal human fibroblasts. Int J Cosmet Sci (1998) 20:151–8. doi: 10.1046/j.1467-2494.1998.171747.x
101. Vivcharenko V, Wojcik M, Palka K, Przekora A. Highly porous and superabsorbent biomaterial made of marine-derived polysaccharides and ascorbic acid as an optimal dressing for exuding wound management. Mater (Basel) (2021) 14:1211. doi: 10.3390/ma14051211
102. Stewart MS, Cameron GS, Pence BC. Antioxidant nutrients protect against UVB-induced oxidative damage to DNA of mouse keratinocytes in culture. J Invest Dermatol (1996) 106:1086–9. doi: 10.1111/1523-1747.ep12339344
103. Hakozaki T, Date A, Yoshii T, Toyokuni S, Yasui H, Sakurai H. Visualization and characterization of UVB-induced reactive oxygen species in a human skin equivalent model. Arch Dermatol Res (2008) 300:S51–6. doi: 10.1007/s00403-007-0804-3
104. Cárcamo JM, Pedraza A, Bórquez-Ojeda O, Golde DW. Vitamin c suppresses TNF alpha-induced NF-kappaB activation by inhibiting I kappa b alpha. Biochemistry (2002) 41:12995–3002. doi: 10.1021/bi0263210
105. Farris PK. Topical vitamin c: a useful agent for treating photoaging and other dermatologic conditions. Dermatol Surg (2005) 31:814–8. doi: 10.1111/j.1524-4725.2005.31725
106. Peterkofsky B, Udenfriend S. Enzymatic hydroxylation of proline in microsomal polypeptide leading to formation of collagen. Proc Natl Acad Sci USA (1965) 53:335–42. doi: 10.1073/pnas.53.2.335
107. Pinnell SR. Regulation of collagen biosynthesis by ascorbic acid: a review. Yale J Biol Med (1985) 58:553–9.
108. Shaw G, Lee-Barthel A, Ross ML, Wang B, Baar K. Vitamin c-enriched gelatin supplementation before intermittent activity augments collagen synthesis. Am J Clin Nutr (2017) 105:136–43. doi: 10.3945/ajcn.116.138594
109. Piersma B, Wouters OY, de Rond S, Boersema M, Gjaltema RAF, Bank RA. Ascorbic acid promotes a TGFβ1-induced myofibroblast phenotype switch. Physiol Rep (2017) 5:e13324. doi: 10.14814/phy2.13324
110. Cha J, Roomi MW, Ivanov V, Kalinovsky T, Niedzwiecki A, Rath M. Ascorbate supplementation inhibits growth and metastasis of B1 6FO melanoma and 4T1. Int J Oncol (2013) 42:55–64. doi: 10.3892/ijo.2012.1712
111. Su M, Chao G, Liang M, Song J, Wu K. Anticytoproliferative effect of vitamin c on rat hepatic stellate cells. Am J Transl Res (2016) 8:2820–5.
112. Rodrigues da Silva M, Schapochnik A, Peres Leal M, Esteves J, Bichels Hebeda C, Sandri S, et al. Beneficial effects of ascorbic acid to treat lung fibrosis induced by paraquat. PLoS One (2018) 13:e0205535. doi: 10.1371/journal.pone.0205535
113. Cameron E, Pauling L. Supplemental ascorbate in the supportive treatment of cancer: Prolongation of survival times in terminal human cancer. Proc Natl Acad Sci USA (1976) 73:3685–9. doi: 10.1073/pnas.73.10.3685
114. Cameron E, Pauling L. Supplemental ascorbate in the supportive treatment of cancer: reevaluation of prolongation of survival times in terminal human cancer. Proc Natl Acad Sci USA (1978) 75:4538–42. doi: 10.1073/pnas.75.9.4538
115. Creagan ET, Moertel CG, O’Fallon JR, Schutt AJ, O’Connell MJ, Rubin J, et al. Failure of high-dose vitamin c (ascorbic acid) therapy to benefit patients with advanced cancer. a controlled trial. N Engl J Med (1979) 301:687–90. doi: 10.1056/NEJM197909273011303
116. Moertel CG, Fleming TR, Creagan ET, Rubin J, O’Connell MJ, Ames MM. High-dose vitamin c versus placebo in the treatment of patients with advanced cancer who have had no prior chemotherapy. a randomized double-blind comparison. N Engl J Med (1985) 312:137–41. doi: 10.1056/NEJM198501173120301
117. Carr AC, Spencer E, Das A, Meijer N, Lauren C, MacPherson S, et al. Patients undergoing myeloablative chemotherapy and hematopoietic stem cell transplantation exhibit depleted vitamin c status in association with febrile neutropenia. Nutrients (2020) 12:1879. doi: 10.3390/nu12061879
118. Mayland CR, Bennett MI, Allan K. Vitamin c deficiency in cancer patients. Palliat Med (2005) 19:17–20. doi: 10.1191/0269216305pm970oa
119. ClinicalTrials.gov Identifier: NCT00954525. Intravenous vitamin c in combination with standard chemotherapy for pancreatic cancer. Available at: https://www.clinicaltrials.gov/ct2/show/NCT00954525 [Accessed August 15, 2022].
120. ClinicalTrials.gov Identifier: NCT00006021. Arsenic trioxide plus vitamin c in treating patients with recurrent or refractory multiple myeloma. (2003) Available at: https://clinicaltrials.gov/ct2/show/NCT00006021 [Accessed August 15, 2022]
121. Bahlis NJ, McCafferty-Grad J, Jordan-McMurry I, Neil J, Reis I, Kharfan-Dabaja M, et al. Feasibility and correlates of arsenic trioxide combined with ascorbic acid-mediated depletion of intracellular glutathione for the treatment of relapsed/refractory multiple myeloma. Clin Cancer Res (2002) 8:3658–68.
122. ClinicalTrials.gov Identifier: NCT00317811. Bortezomib, ascorbic acid, and melphalan in treating patients with newly diagnosed multiple myeloma. (2006). Available at: https://clinicaltrials.gov/ct2/show/NCT00317811 [Accessed August 15, 2022].
123. Berenson JR, Yellin O, Woytowitz D, Flam MS, Cartmell A, Patel R, et al. Bortezomib, ascorbic acid and melphalan (BAM) therapy for patients with newly diagnosed multiple myeloma: an effective and well-tolerated frontline regimen. Eur J Haematol (2009) 82:433–9. doi: 10.1111/j.1600-0609.2009.01244.x
124. Welsh JL, Wagner BA, van’t Erve TJ, Zehr PS, Berg DJ, Halfdanarson TR, et al. Pharmacological ascorbate with gemcitabine for the control of metastatic and node-positive pancreatic cancer (PACMAN): results from a phase I clinical trial. Cancer Chemother Pharmacol (2013) 71:765–75. doi: 10.1007/s00280-013-2070-8
125. ClinicalTrials.gov Identifier: NCT01049880. A research trial of high dose vitamin c and chemotherapy for metastatic pancreatic cancer. (2015). Available at: https://clinicaltrials.gov/ct2/show/NCT01049880 [Accessed August 15, 2022].
126. ClinicalTrials.gov Identifier: NCT01050621. Trial of chemotherapy plus intravenous vitamin c in patients with advanced cancer for whom chemotherapy alone is only marginally effective. (2010). Available at: https://clinicaltrials.gov/ct2/show/NCT01050621 [Accessed August 15, 2022].
127. ClinicalTrials.gov Identifier: NCT01080352. Vitamin c as an anti-cancer drug. (2010). Available at: https://clinicaltrials.gov/ct2/show/NCT01080352 [Accessed August 15, 2022].
128. Nielsen TK, Højgaard M, Andersen JT, Jørgensen NR, Zerahn B, Kristensen B, et al. Weekly ascorbic acid infusion in castration-resistant prostate cancer patients: a single-arm phase II trial. Transl Androl Urol (2017) 6:517–28. doi: 10.21037/tau.2017.04.42
129. ClinicalTrials.gov Identifier: NCT01364805. New treatment option for pancreatic cancer. (2011). Available at: https://clinicaltrials.gov/ct2/show/NCT01364805 [Accessed August 15, 2022].
130. ClinicalTrials.gov Identifier: NCT00228319. Treatment of newly diagnosed ovarian cancer with antioxidants. (2005). Available at: https://clinicaltrials.gov/ct2/show/NCT00228319 [Accessed August 15, 2022].
131. Ma Y, Chapman J, Levine M, Polireddy K, Drisko J, Chen Q. High-dose parenteral ascorbate enhanced chemosensitivity of ovarian cancer and reduced toxicity of chemotherapy. Sci Transl Med (2014) 6:222ra18. doi: 10.1126/scitranslmed.3007154
132. ClinicalTrials.gov Identifier: NCT02655913. Safety and efficacy of vitamin c infusion in combination with local mEHT to treat non small cell lung cancer. (2016). Available at: https://clinicaltrials.gov/ct2/show/NCT02655913 [Accessed August 15, 2022].
133. Ou J, Zhu X, Chen P, Du Y, Lu Y, Peng X, et al. A randomized phase II trial of best supportive care with or without hyperthermia and vitamin c for heavily pretreated, advanced, refractory non-small-cell lung cancer. J Adv Res (2020) 24:175–82. doi: 10.1016/j.jare.2020.03.004
134. ClinicalTrials.gov Identifier: NCT01905150. Ph 2 trial of vitamin c & G-FLIP (Low doses gemcitabine, 5FU, leucovorin, irinotecan, oxaliplatin) for pancreatic cancer. (2013). Available at: https://clinicaltrials.gov/ct2/show/NCT01905150 [Accessed August 15, 2022].
135. Bruckner H, Hirschfeld A, Gurell D, Lee K. Broad safety impact of high-dose ascorbic acid and induction chemotherapy for high-risk pancreatic cancer. J Clin Oncol (2017) 35(15_suppl):e15711. doi: 10.1200/JCO.2017.35.15_suppl.e15711
136. ClinicalTrials.gov Identifier: NCT01754987. A study of vitamin c in the treatment of liver cancer to determine if it is safe and effective. (2012). Available at: https://clinicaltrials.gov/ct2/show/NCT01754987 [Accessed August 15, 2022].
137. ClinicalTrials.gov Identifier: NCT03410030. Trial of ascorbic acid (AA) + nanoparticle paclitaxel protein bound + cisplatin + gemcitabine (AA NABPLAGEM). (2018). Available at: https://clinicaltrials.gov/ct2/show/NCT03410030 [Accessed August 15, 2022].
138. ClinicalTrials.gov Identifier: NCT03964688. Effect of vitamin c in autologous stem cell transplantations. (2019). Available at: https://clinicaltrials.gov/ct2/show/NCT03964688 [Accessed August 15, 2022].
139. ClinicalTrials.gov Identifier: NCT02905578. A phase 2 trial of high-dose ascorbate for pancreatic cancer (PACMAN 2.1). (2016). Available at: https://clinicaltrials.gov/ct2/show/NCT02905578 [Accessed August 15, 2022].
140. ClinicalTrials.gov Identifier: NCT03146962. High dose vitamin c intravenous infusion in patients with resectable or metastatic solid tumor malignancies. (2017). Available at: https://clinicaltrials.gov/ct2/show/NCT03146962 [Accessed August 15, 2022].
141. ClinicalTrials.gov Identifier. Ascorbic acid and combination chemotherapy for the treatment of relapsed or refractory lymphoma or clonal cytopenia of undetermined significance. (2018). Available at: https://clinicaltrials.gov/ct2/show/NCT03418038 [Accessed August 15, 2022].
142. ClinicalTrials.gov Identifier: NCT03433781. A phase Ib/IIa study evaluating the safety and tolerability of vitamin c in patients with intermediate or high risk myelodysplastic syndrome with TET2 mutations. (2018). Available at: https://clinicaltrials.gov/ct2/show/NCT03433781 [Accessed August 15, 2022].
143. ClinicalTrials.gov Identifier: NCT03508726. High dose ascorbate with preoperative radiation in patients with locally advanced soft tissue sarcomas. (2018). Available at: https://clinicaltrials.gov/ct2/show/NCT03508726 [Accessed August 15, 2022].
144. ClinicalTrials.gov Identifier: NCT03682029. Epigenetics, vitamin c, and abnormal blood cell formation - vitamin c in patients with low-risk myeloid malignancies. (2018). Available at: https://clinicaltrials.gov/ct2/show/NCT03682029 [Accessed August 15, 2022].
145. ClinicalTrials.gov Identifier: NCT03799094. Vitamin c and tyrosine kinase inhibitor in lung cancer patients with epidermal growth factor receptor mutations. (2019). Available at: https://clinicaltrials.gov/ct2/show/NCT03799094 [Accessed August 15, 2022].
146. ClinicalTrials.gov Identifier: NCT03999723. Combining active and passive DNA hypomethylation. (2019). Available at: https://clinicaltrials.gov/ct2/show/NCT03999723 [Accessed August 15, 2022].
147. ClinicalTrials.gov Identifier: NCT04033107. High dose vitamin c combined with metformin in the treatment of malignant tumors. (2019). Available at: https://clinicaltrials.gov/ct2/show/NCT04033107 [Accessed August 15, 2022].
148. ClinicalTrials.gov Identifier: NCT04046094. Intravenous (IV) vitamin c with chemotherapy for cisplatin ineligible bladder cancer patients. (2019). Available at: https://clinicaltrials.gov/ct2/show/NCT04046094 [Accessed August 15, 2022].
149. ClinicalTrials.gov Identifier: NCT04516681. IV ascorbic acid in peritoneal metastatic colorectal cancer. (2020). Available at: https://clinicaltrials.gov/ct2/show/NCT04516681 [Accessed August 15, 2022].
150. ClinicalTrials.gov Identifier: NCT04634227. Gemcitabine plus ascorbate for sarcoma in adults (Pilot). (2020). Available at: https://clinicaltrials.gov/ct2/show/NCT04634227 [Accessed August 15, 2022].
151. ClinicalTrials.gov Identifier: NCT04801511. Preoperative IMRT with concurrent high-dose vitamin c and mFOLFOX6 in locally advanced rectal cancer. (2021). Available at: https://clinicaltrials.gov/ct2/show/NCT04801511 [Accessed August 15, 2022].
152. ClinicalTrials.gov Identifier: NCT02516670. Docetaxel with or without ascorbic acid in treating patients with metastatic prostate cancer. (2022). Available at: https://clinicaltrials.gov/ct2/show/NCT02516670 [Accessed August 15, 2022].
153. Drisko JA, Chapman J, Hunter VJ. The use of antioxidants with first-line chemotherapy in two cases of ovarian cancer. J Am Coll Nutr (2003) 22:118–23. doi: 10.1080/07315724.2003.10719284
154. González MJ, Berdiel MJ, Miranda-Massari JR, López D, Duconge J, Rodriguez JL, et al. High dose intravenous vitamin c and metastatic pancreatic cancer: two cases. Integr Cancer Sci Ther (2016) 3:1–2. doi: 10.15761/ICST.1000219
155. Padayatty SJ, Riordan HD, Hewitt SM, Katz A, Hoffer LJ, Levine M. Intravenously administered vitamin c as cancer therapy: three cases. CMAJ (2006) 174:937–42. doi: 10.1503/cmaj.050346
156. Riordan HD, Riordan NH, Jackson JA, Casciari JJ, Hunninghake R, González MJ, et al. Intravenous vitamin c as a chemotherapy agent: a report on clinical cases. P R Health Sci J (2004) 23:115–8.
157. Seo MS, Kim JK, Shim JY. High-dose vitamin c promotes regression of multiple pulmonary metastases originating from hepatocellular carcinoma. Yonsei Med J (2015) 56:1449–52. doi: 10.3349/ymj.2015.56.5.1449
158. Drisko JA, Serrano OK, Spruce LR, Chen Q, Levine M. Treatment of pancreatic cancer with intravenous vitamin c: a case report. Anticancer Drugs (2018) 29:373–9. doi: 10.1097/CAD.0000000000000603
159. Schoenfeld JD, Sibenaller ZA, Mapuskar KA, Wagner BA, Cramer-Morales KL, Furqan M, et al. O2·- and H2O2-mediated disruption of fe metabolism causes the differential susceptibility of NSCLC and GBM cancer cells to pharmacological ascorbate. Cancer Cell (2017) 31:487–500. doi: 10.1016/j.ccell.2017.02.018
160. Zhao H, Zhu H, Huang J, Zhu Y, Hong M, Zhu H, et al. The synergy of vitamin c with decitabine activates TET2 in leukemic cells and significantly improves overall survival in elderly patients with acute myeloid leukemia. Leuk Res (2018) 66:1–7. doi: 10.1016/j.leukres.2017.12.009
161. Vollbracht C, Schneider B, Leendert V, Weiss G, Auerbach L, Beuth J. Intravenous vitamin c administration improves quality of life in breast cancer patients during chemo-/radiotherapy and aftercare: results of a retrospective, multicentre, epidemiological cohort study in Germany. In Vivo (2011) 25:983–90.
162. Takahashi H, Mizuno H, Yanagisawa A. High-dose intravenous vitamin c improves quality of life in cancer patients. Pers Med Univ (2012) 1:49–53. doi: 10.1016/j.pmu.2012.05.008
163. Jacobs C, Hutton B, Ng T, Shorr R, Clemons M. Is there a role for oral or intravenous ascorbate (vitamin c) in treating patients with cancer? a systematic review. Oncologist (2015) 20:210–23. doi: 10.1634/theoncologist.2014-0381
164. Cockfield JA, Schafer ZT. Antioxidant defenses: A context-specific vulnerability of cancer cells. Cancers (Basel) (2019) 11:1208. doi: 10.3390/cancers11081208
165. Buechler MB, Pradhan RN, Krishnamurty AT, Cox C, Calviello AK, Wang AW, et al. Cross-tissue organization of the fibroblast lineage. Nature (2021) 593:575–9. doi: 10.1038/s41586-021-03549-5
166. Gaggioli C, Hooper S, Hidalgo-Carcedo C, Grosse R, Marshall JF, Harrington K, et al. Fibroblast-led collective invasion of carcinoma cells with differing roles for RhoGTPases in leading and following cells. Nat Cell Biol (2007) 9:1392–400. doi: 10.1038/ncb1658
167. Albrengues J, Bertero T, Grasset E, Bonan S, Maiel M, Bourget I, et al. Epigenetic switch drives the conversion of fibroblasts into proinvasive cancer-associated fibroblasts. Nat Commun (2015) 6:10204. doi: 10.1038/ncomms10204
168. Provenzano PP, Cuevas C, Chang AE, Goel VK, Von Hoff DD, Hingorani SR. Enzymatic targeting of the stroma ablates physical barriers to treatment of pancreatic ductal adenocarcinoma. Cancer Cell (2012) 21:418–29. doi: 10.1016/j.ccr.2012.01.007
169. Nathanson SD, Nelson L. Interstitial fluid pressure in breast cancer, benign breast conditions, and breast parenchyma. Ann Surg Oncol (1994) 1:333–8. doi: 10.1007/BF03187139
170. Liotta LA. Tumor invasion and metastases–role of the extracellular matrix: Rhoads memorial award lecture. Cancer Res (1986) 46:1–7.
171. Egeblad M, Rasch MG, Weaver VM. Dynamic interplay between the collagen scaffold and tumor evolution. Curr Opin Cell Biol (2010) 22:697–706. doi: 10.1016/j.ceb.2010.08.015
172. Özdemir BC, Pentcheva-Hoang T, Carstens JL, Zheng X, Wu CC, Simpson TR, et al. Depletion of carcinoma-associated fibroblasts and fibrosis induces immunosuppression and accelerates pancreas cancer with reduced survival. Cancer Cell (2014) 25:719–34. doi: 10.1016/j.ccr.2014.04.005
173. Yoshida GJ, Azuma A, Miura Y, Orimo A. Activated fibroblast program orchestrates tumor initiation and progression; molecular mechanisms and the associated therapeutic strategies. Int J Mol Sci (2019) 20:2256. doi: 10.3390/ijms20092256
174. Yoshida GJ. Metabolic reprogramming: the emerging concept and associated therapeutic strategies. J Exp Clin Cancer Res (2015) 34:111. doi: 10.1186/s13046-015-0221-y
175. Zhang Y, Bian Y, Wang Y, Wang Y, Duan X, Han Y, et al. HIF-1α is necessary for activation and tumour-promotion effect of cancer-associated fibroblasts in lung cancer. J Cell Mol Med (2021) 25:5457–69. doi: 10.1111/jcmm.16556
176. Zhang D, Wang Y, Shi Z, Liu J, Sun P, Hou X, et al. Metabolic reprogramming of cancer-associated fibroblasts by IDH3α downregulation. Cell Rep (2015) 10:1335–48. doi: 10.1016/j.celrep.2015.02.006
177. Sanz-Moreno V, Gaggioli C, Yeo M, Albrengues J, Wallberg F, Viros A, et al. ROCK and JAK1 signaling cooperate to control actomyosin contractility in tumor cells and stroma. Cancer Cell (2011) 20:229–45. doi: 10.1016/j.ccr.2011.06.018
Keywords: ascorbic acid, antitumor effect, cancer, cancer-associated fibroblast, antioxidant, intravenous administration
Citation: Maekawa T, Miyake T, Tani M and Uemoto S (2022) Diverse antitumor effects of ascorbic acid on cancer cells and the tumor microenvironment. Front. Oncol. 12:981547. doi: 10.3389/fonc.2022.981547
Received: 29 June 2022; Accepted: 25 August 2022;
Published: 20 September 2022.
Edited by:
Nadia Judith Jacobo-Herrera, Instituto Nacional de Ciencias Médicas y Nutrición Salvador Zubirán (INCMNSZ), MexicoReviewed by:
Linli Zhou, University of Cincinnati, United States;Maja M. Grabacka, University of Agriculture in Krakow, PolandCopyright © 2022 Maekawa, Miyake, Tani and Uemoto. This is an open-access article distributed under the terms of the Creative Commons Attribution License (CC BY). The use, distribution or reproduction in other forums is permitted, provided the original author(s) and the copyright owner(s) are credited and that the original publication in this journal is cited, in accordance with accepted academic practice. No use, distribution or reproduction is permitted which does not comply with these terms.
*Correspondence: Toru Miyake, bXlrQGJlbGxlLnNoaWdhLW1lZC5hYy5qcA==