- 1School of Pharmacy, Institute of Hepatology and Metabolic Diseases, Department of Hepatology, the Affiliated Hospital of Hangzhou Normal University, Hangzhou Normal University, Hangzhou, China
- 2Jinan Microecological Biomedicine Shandong Laboratory, Jinan, China
- 3The First Affiliated Hospital, Key Laboratory of Combined Multi-Organ Transplantation, Ministry of Public Health, Key Laboratory of Organ Transplantation of Zhejiang Province, School of Medicine, Zhejiang University, Hangzhou, China
Bacteria are important symbionts for humans, which sustain substantial influences on our health. Interestingly, some bastrains have been identified to have therapeutic applications, notably for antitumor activity. Thereby, oncologists have developed various therapeutic models and investigated the potential antitumor mechanisms for bacteria-mediated cancer therapy (BCT). Even though BCT has a long history and exhibits remarkable therapeutic efficacy in pre-clinical animal models, its clinical translation still lags and requires further breakthroughs. This review aims to focus on the established strains of therapeutic bacteria and their antitumor mechanisms, including the stimulation of host immune responses, direct cytotoxicity, the interference on cellular signal transduction, extracellular matrix remodeling, neoangiogenesis, and metabolism, as well as vehicles for drug delivery and gene therapy. Moreover, a brief discussion is proposed regarding the important future directions for this fantastic research field of BCT at the end of this review.
Therapeutic bacteria for cancer treatment
Humans and bacteria have a symbiotic relationship. In average, one human being contains approximately 30 trillion human cells and 39 trillion bacteria (1). Diverse microbial community flourish in our epidermal tissues, mucosal tissues and digestive system, regulating our physiological behaviors in sophisticated ways. For example, the notorious Helicobacter pylori has been proved to increase the risk of developing gastric cancer. With the advances in detection methods based on genome sequencing, residential bacteria are also identified in tissues which were conventionally considered “sterile”, including brains, placentas, kidneys and cancerous tissues of breast (2). Even though our knowledge about their roles in human health is still superficial, pioneering scientists and physicians have made great effort to utilize these microorganisms to fight against human cancer.
The modern concept of bacteria-mediated cancer therapy (BCT) originated from more than a century ago. A surgical oncologist, William Coley, tentatively treated cancer patients with a mixture of inactivated Streptococcus pyogenes and Serratia marcescens (Coley’s Toxins) in 1983. From that attempt, isolated bacterial strains replaced deliberate infections by pathogenic bacteria in unsterile conditions during the mysterious natural therapies aiming at suppressing cancerous cell growth with infectious bacteria before the emergence of chemotherapies and radiotherapies. Even though BCT has never been a mainstream clinical treatment option for cancer, mechanistic and translational studies have been continuously devoted to elucidate the mechanisms by which bacteria could influence the growth of solid cancer. More bacteria strains have been chosen with rational consideration of their unique biological properties, and further engineered with modern genetic tools to achieve better therapeutic efficacy and safety profile. Here, we listed those strains which have gone through or in the middle of clinical trials registered under the guideline of U.S. Food and Drug Administration (Table 1).
This review would mainly discuss about the versatility of BCT, the underlying biological mechanisms in the triangular relationship among bacteria, cancer cells and host immune system, as well as problems ungently requiring thorough investigations in this field (Figure 1).
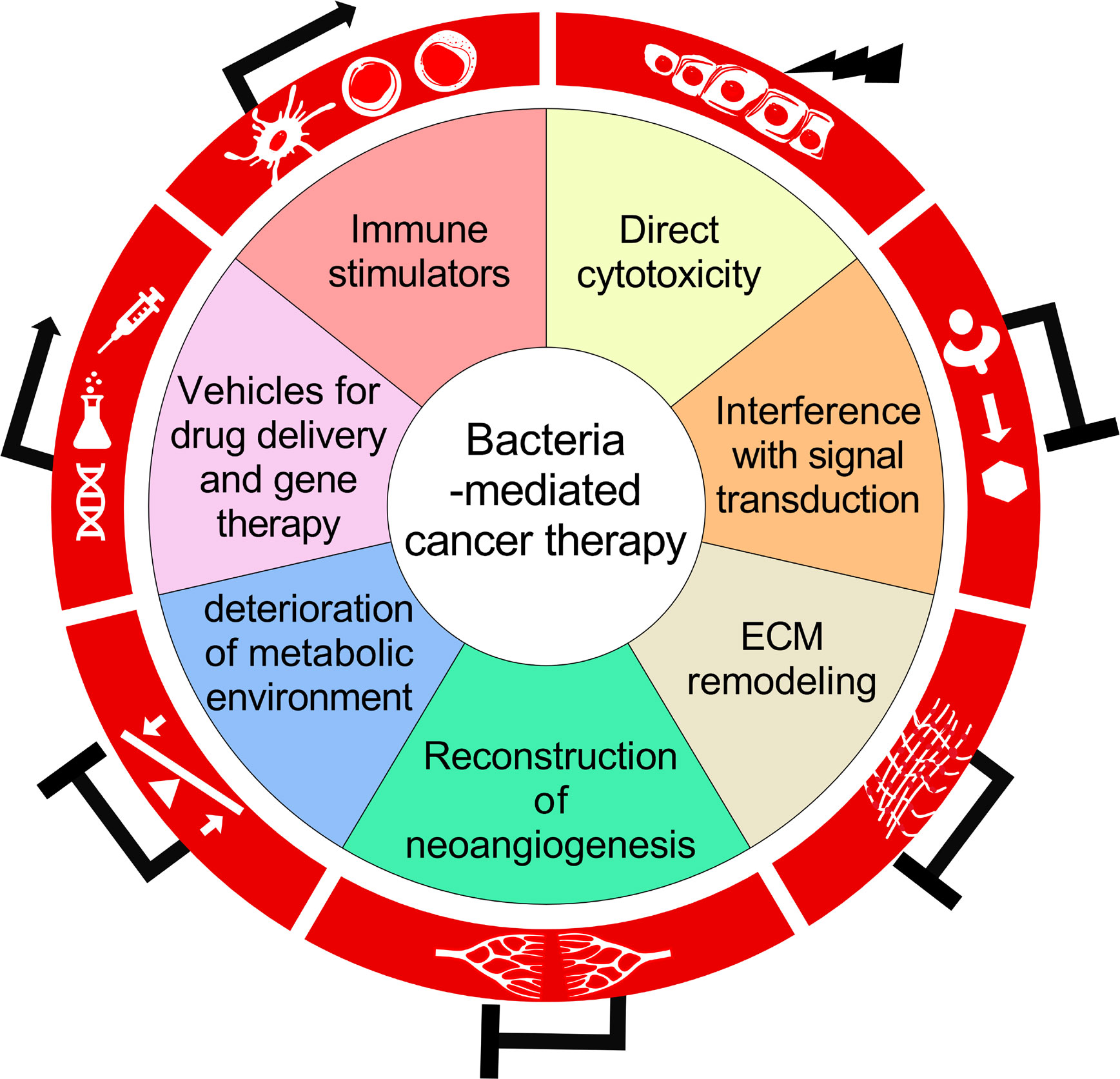
Figure 1 A schematic diagram summarizing the biological mechanisms for the antitumor activity of bacteria-mediated therapy.
The mechanisms of BCT
Therapeutic bacteria function as immune stimulators
Many bacterial components, such as lipopolysaccharide (LPS), flagella, and bacterial DNA, exhibits substantial and specific influences on the immunostimulatory responses induced by the administration of either live or inactivated bacteriological preparations (16). These pathogen-associated molecular patterns (PAMPs) immediately initiate innate immune responses, marked by the accumulation of granulocytes and macrophages into the sties of infection, as well as a coordinated elevation of proinflammatory cytokines and chemokines (17–19). During BCT in which aerobic or facultative anaerobic bacteria are used, a large titer of bacteria penetrates and colonizes in the hypoxic tumor microenvironment (TME) simultaneously. Such an intense infection, as well as consequent innate immune responses, concomitantly lead to the lysis of neighboring tumor cells and releasement of cellular content, including tumor-associated antigens (TAAs) and tumor-specific antigens (TSAs) (20). Antigen-presenting cells (APCs) engulf both bacteria and debris of cells infected by bacteria, and process them into antigens coupled with major histocompatibility complex (MHC) (21, 22). Eventually, antigen-specific T cells in draining lymph nodes are activated by APCs, marking the initiation of acquired immune responses. During the phase of acquired immune responses, the present of bacterial PAMPs in TME is still beneficial to maintain the proinflammatory status of T cells and macrophages (23, 24). Therefore, bacterial PAMPs play key functions for the antitumor effects of therapeutic bacteria (Figure 2).
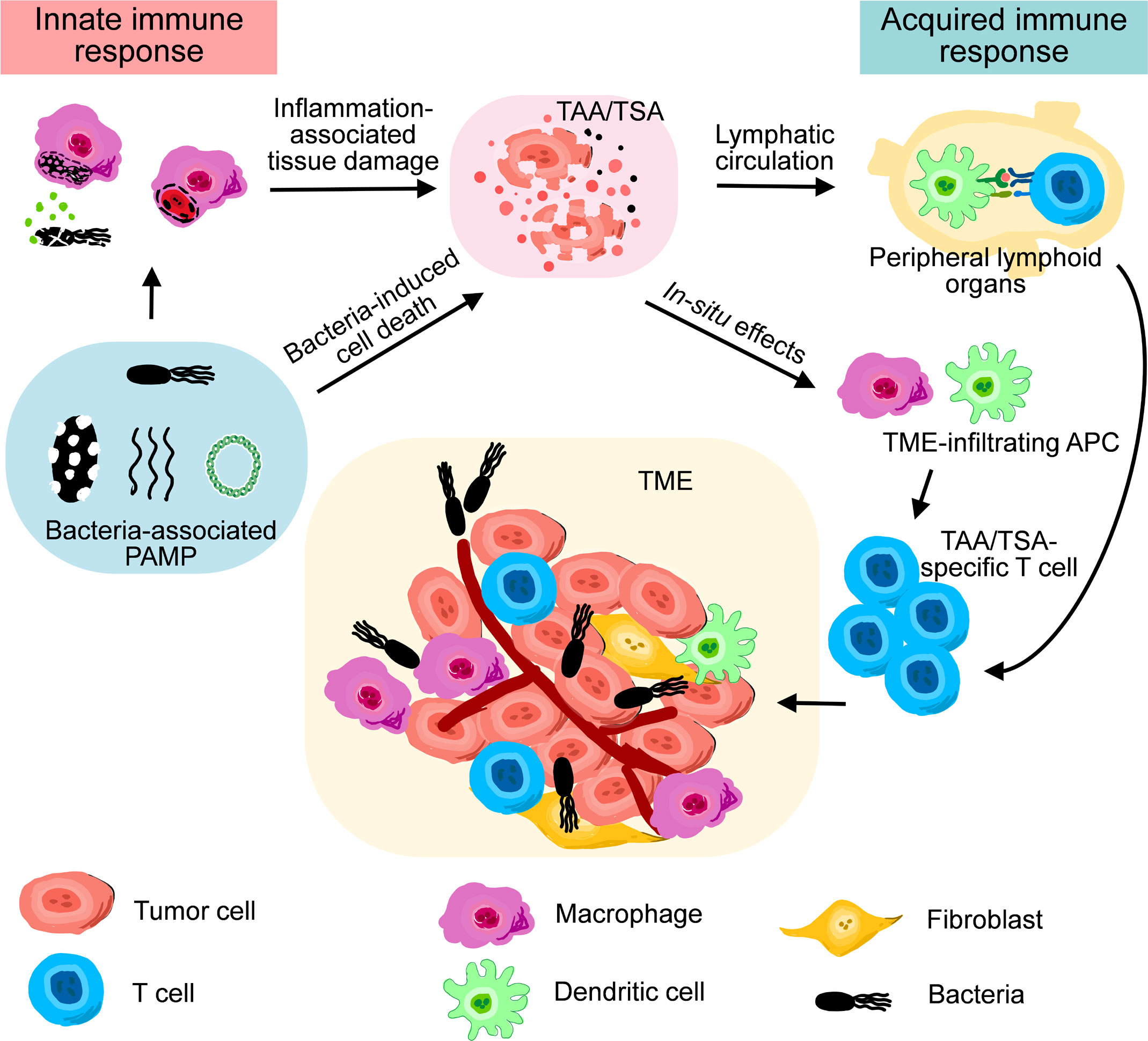
Figure 2 A schematic diagram illustrating the roles of innate immune response and acquired immune response during bacteria-mediated therapy.
LPS is an important structural component of the outer wall of Gram-negative bacteria. Structure of LPS consists of lipid A, the core oligosaccharide and the O-antigen. LPS forms a dense network of hydrophobic compounds through ionic and covalent bonds, providing barrier protection for bacterial outer membrane and protecting bacteria from the lethal effects of the complement system and macrophages (25). In addition to its role in bacterial membrane, LPS, especially its lipid A portion, is also an important signaling molecule for the innate immune system (26). LPS specifically activates Toll-like receptor 4 (TLR4) and CD14, which are widely expressed by CD8+ T cells, macrophages, natural killer (NK) cells and Dendritic cells (DCs), and initiates tumor-suppressive downstream signaling cascades mainly through myeloid differentiation primary response 88 (MYD88), such as the secretion of proinflammatory cytokines and cytotoxic factors, as well as the formation of inflammasome. For example, bacterial lipopolysaccharide (LPS) enhances prostate cancer metastasis potentially through NF-κB activation (27). Salmonella LPS could induce tumor-specific CD8+ T cell responses and the elevation of tumor necrosis factor-α (TNFα) in both TME and peripheral blood (28, 29). Moreover, Thuy Xuan Phan et al. demonstrated that Salmonella typhimurium enhanced inflammasome activation in tumor-infiltrating macrophages following their phagocytosis of Salmonella-infected tumor cells in a TLR4-dependent manner, and thus increased their level of interleukin-1β (IL-1β) secretion (30–32).
Flagellin is a subunit protein at the tip of bacterial flagella, the locomotive organ of bacteria, and it is recognized by TLR5 as a PAMP (33). Flagellin has been shown to induce perforin-dependent NK cell-mediated antitumor responses (34). Flagellin also activates NK cells through a TLR-independent pathway involving IL-18 and MYD88 to produce interferon-γ (IFNγ), a key cytokine in innate and adaptive immunity. Moreover, Francesc Coll et al. demonstrated that flagellin could significantly suppress tumor cell proliferation by decreasing the frequency of CD4+CD25+ regulatory T (Treg) cells (35). Chung Truong Nguyen et al. showed that flagellin also enhances a CD8+ T cell-dependent antitumor response in a peptide vaccine-based immunotherapeutic procedure (36).
Bacterial DNA is the genetic material shared by Gram-positive and Gram-negative bacteria, and it has broad-spectrum immune-stimulating effects. CpG motifs are the structural basis for its immune activity, which are recognized by TLR9 (37–40). Bacterial DNA directly activates mouse macrophages, B cells, and DCs in a TLR9-dependent manner, upregulates the expression of immunostimulatory molecules, modulates immune responses, and induces the secretion of various cytokines, including IL-12, IL-1, and TNFα (39, 41–44). Moreover, bacterial DNA indirectly activates Th1 and CTL through cytokines, DCs, and other monocytic cells (43–45). For example, KJ Stacey et al. showed that bacterial DNA directly activated APCs and upregulated the expression of MHC-class II molecules and costimulatory molecules such as CD86 and CD40, thus activating antigen-specific T cells to stimulate acquired immune responses (46, 47).
Apart from PAMPs, the production of TAAs/TSAs (tumor-associated antigens/tumor-specific antigens) is the key step for the successful shift from innate immune to acquired immune responses, which have the merit of high specificity and long-lasting memory (48). However, the efficacy of the concomitant lysis of tumor cells by bacteria-induced immune response is largely affected by the health conditions of recipients, such as the competency of immune system and the vasculature status of TME. Therefore, bacteria-mediated antigen delivery system is developed to bypass these limitations, in which bacteria are modified to carry TAA/TSAs by themselves (49, 50). This strategy of delivering TAAs/TSAs could effectively shape the host antitumor immune response and significantly suppress the growth of tumor. For example, Yu Mei et al. utilized the attenuated Salmonella typhimurium SL7207 to deliver a murine melanoma vaccine via the transformation of prokaryotic expression plasmids expressing the AIDA-1 autotransporter and DNA vaccine elements (51). Similarly, Xiong et al. constructed a novel SPI2-based oral Salmonella vaccine expressing mutant human Survivin in combination with NKT ligands, and achieved significantly improved generation of antigen-specific effector-memory CD8+ and CD4+ T cells, as well as increased antitumor efficacy, in murine models of colon cancer and glioblastoma (52). Apart from live bacteria-mediated antigen delivery system, bacteria components such as bacterial membrane could be directly used as vaccine adjuvant. For example, Ravi B Patel et al. used bacterial membrane-coated nanoparticles composed of immune activating PC7A/CpG polyplex core to in situ capture cancer neoantigens following radiation therapy, which facilitated their up-taken by DC cells to stimulate a strong antitumor T cell response (53).
Therapeutic effects of BCT independent of immune responses
In addition to the induction of host immune responses, therapeutic bacteria exhibit direct cytotoxicity to cancer cells. An in vitro cell killing experiment showed that Salmonella typhimurium A1-R, an attenuated Salmonella strain, could cause cell death for prostate cancer cell lines via mixed cytotoxic mechanisms independent of immune responses (54). For example, Salmonella invasion induced rapid necrosis due to cytoplasmic swelling and membrane disruption (54). Extensive intracellular Salmonella duplication also led to the bursting of invaded cells (54). Moreover, Clostridium novyi-NT could secret phospholipases, hemolysins and lipases to disrupt the structure of lipid bilayers of cancer cells, thereby causing direct cytotoxicity (55, 56).
Apart from direct cytotoxicity, bacterial infection could suppress the growth of cancer cells through interfering with intracellular signal transduction. For example, Salmonella typhimurium flagellin could suppress the proliferation of breast cancer cells by activating the membrane-anchored TLR5 of cancer cells (57). Salmonella infection would also suppress AKT/mTOR pathway, thus leading to the induction of apoptotic and autophagic pathways (58). Listeria monocytogenes could kill tumor cells by enhancing cytosolic reactive oxygen species (ROS) levels through the activation of nicotinamide adenine dinucleotide phosphate oxidase and intracellular calcium mobilization, and such immunogenic tumor cell death would further activate CD8+ T cells to eliminate both primary tumors and metastases (59). Other than the proliferation and death of cancer cells, other cellular behaviors such as drug sensitivity would be influenced by therapeutic bacteria. For example, Salmonella choleraesuis induced a significant increase in the protein level of connexin 43 which mediated gap intercellular communication between cancer cells, thus sensitizing them to cisplatin (60).
The extracellular matrix (ECM) of tumor tissues would also be changed after the infection of therapeutic bacteria. ECM is an important component that maintains tissue integrity, and regulates cell behaviors through transmembrane signaling transduction (61). Solid tumors generally contain a high abundance of collagens and hyaluronic acids (HA), making them stiffer than normal tissues (62, 63). Malignant cells proliferate and migrate faster in such mechanical environment (64, 65). Meanwhile, accumulated ECM also creates structural obstacles physically for various cancer therapies, including chemotherapies and immunotherapies (66, 67). For example, collagen-laminin network forms a firm barrier for the diffusion of large molecules, while excessive HA dampens the passive release of drugs from blood vessels via increased osmotic pressure and viscosity of tissue fluid (68). Therapeutic bacteria could reduce the stiffness of ECM by multiple mechanisms. For example, Salmonella typhimurium could convert plasminogen to plasmin, a broad-spectrum serine protease, by inactivating Plasminogen activator inhibitor 1 (PAI-1), thus leading to the degradation of laminin as well as the activation of matrix metalloproteinases (MMPs) precursors to degrade collagens (69). Listeria monocytogenes are also capable to secrete MMPs (70). Some Gram-positive bacteria such as Clostridium perfringens (Mu toxin), Clostridium difficile, Clostridium septicum (γ toxin) and Clostridium chauvoei produces hyaluronidase that degrades HA (71–73). Other mechanisms by which bacteria lead to ECM disruption have been systematically reviewed by Lennert Steukers et al. (74). Taking advantages of these bacterial enzymes targeting intertumoral ECM, therapeutic bacteria have been utilized to combat solid tumors which are difficult to target by conventional chemotherapies. For example, Nancy D. Ebelt et al. recently reported that genetically modified attenuated Salmonella typhimurium expressing exogenously bacterial hyaluronidase could invaded into the desmoplastic tumors and degraded HA with a significantly enhanced efficacy in the orthotopic human pancreatic ductal adenocarcinoma mice models (75).
Besides cytotoxicity, signal transduction interference and ECM remodeling, the reconstruction of neoangiogenesis and metabolism landscape are also important features for bacteria-mediated tumor therapy. For example, Salmonella typhimurium VNP20009 treatment could reduce the formation of neovascularization, therefore leading to retarded diffusion of nutrients in TME (76, 77). Salmonella choleraesuis has been demonstrated as both tumoricidal and antiangiogenic for the treatment of hepatocellular carcinoma (78). Moreover, both Salmonella typhimurium and Listeria monocytogenes are glucose-consumers, which would increase nutritional stress for tumor cells and deteriorate the metabolic microenvironment in TME (79, 80).
Therapeutic bacteria as tumor-targeting vehicles for drug delivery
Bacteria own several merits as pharmaceutical carriers for cancer therapies. First, anaerobic and facultative anaerobic bacteria have a natural tendency of penetrating and colonizing the hypoxic areas in tumor due to intrinsic locomotion, which exceeds the limits of vascularization. Second, bacteria own high surface-to-volume ratio, which provides suitable interface for particle absorption. Third, bacterial surface molecules such as polysaccharides and glycoproteins are potential subjects for chemical modifications. Last but not least, the cytotoxic effects of the delivered compounds and bacteria-induced immune activation might achieve synergistic effects during cancer therapy.
Therapeutic bacteria can be used as live vehicles (similar to “mules”). For example, Shuangqian Yan et al. reported a bacteria@MOFs system in which the flagellum of Escherichia coli MG1655 were modified with metal-organic framework (MOF) encapsulating chemical drugs through a one-step in situ method, could maintain viability and tumor selectivity to achieve effective delivery of chemical drugs to the poorly-vascularized areas inside the tumors (81). Moreover, additional guiding motifs could be incorporated. For example, Byung-Wook Park et al. linked Escherichia coli with drug-loaded polyelectrolyte multilayer microparticles with embedded magnetic nanoparticles at an approximately 1:1 ratio, to which system the authors referred as “microswimmers”, and such drug carriers with a diameter of 1 μm could directionally move at a mean speed of up to 22.5 μm/s under the orientation of an electromagnetic field, which would benefit the bacteria-mediated therapies for those highly vascularized tumors showing weak hypoxia (82). Packaging materials of different sizes and functions have been applied to decorate therapeutic bacteria (83–86), including polymeric particles (82, 87), polymer tubes (88), red blood cells (89–91), liposomes (92, 93), and nanoparticles (94–97). For example, Mukrime Birgul Akolpoglu et al. reported a biohybrid microrobotic platform by combining nanoliposomes and E. coli MG1655 (98). Recently, Fenton-like reactions which eliminate cancer cells by generating toxic ROS have developed rapidly as chemodynamic therapies, and therapeutic bacteria improve such therapies by biocatalyticly producing H2O2, a key reactant for Fenton-like reactions. For example, magnetic Fe3O4 nanoparticle-decorated Escherichia coli MG1655 overexpressing respiratory chain enzyme II (NDH-2) could support Fenton-like reaction by continuously producing H2O2, which triggered abundant tumor cell apoptosis by the excessive cytotoxic hydroxyl radicals (94).
However, frequent and repetitive administration is not suitable for live bacteria-mediated therapy, since its slow whole-body clearance might lead to accumulated systemic toxicity. Thus, drugs to be delivered by live bacteria should be chosen with great caution. Specifically, the ideal drugs to be delivered should be able to achieve sustained effects with one single dose.
Vesicles made with bacterial membranes, instead of live bacteria, are promising strategies to improve the safety profile of bacteria-mediated drug delivery. Small bacterial outer membrane vesicles (< 200 nm) are naturally released from Gram-negative bacteria during proliferation (99), and particles of such size often exhibit strong accumulation in TME through enhanced permeability and retention (EPR) effect during blood circulation. Recently Qi Chen et al. used DSPE-PEG-RGD-decorated bacterial outer membrane vesicles to encapsulate F127 mesoporous nanoparticles containing cytotoxic Tegafur. The obtained nanodrug could be preferentially accumulated at the site of tumors, and simultaneously induced the killing of tumor cells and the activation of immune cells such as macrophages and T cells. Eventually, the tumor antigens released by dead tumor cells initiated potent systemic immune responses eliminating both primary tumors and metastatic lesions (20).
Larger membrane vesicles maintaining the entire bacterial morphology can be produced via membrane perforation mediated by lysis gene E from bacteriophage øX174 (100), and they are often referred to as “bacterial ghost (BG)” by many researchers. BGs can be used immune adjuvants (101), and they have good structure integrity upon lyophilization, which is beneficial for massive production (102). Water-soluble drugs can be loaded into BGs through membrane poles (103), and these poles can be sealed by fusion with small membrane vesicles in the presence of Ca2+ ions to prevent drug leakage (104). In vitro evaluation showed that such drug-loaded bacterial ghosts could be up-taken by both macrophages and cancer cells, with a preference for macrophages (104). Considering the size of entire bacteria has exceed the range for strong EPR effect, BGs might not be the best choice for targeting tumor cells, but they are highly efficient for targeting APCs which preferentially engulf bacteria. For example, N.Dobrovolskienė et al. prepared BGs filled with tumor lysate containing numerous tumor antigens, and such antigen-loaded BGs induced a stronger dendritic cell activation compared to LPS (105).
The combination of BCT and photothermal/photodynamic therapy
Photothermal therapy (PTT) and photodynamic therapy (PDT) are new strategies for cancer therapy. PTT uses photosensitizers (PSs) with specific light absorption to convert light energy into heat energy to eliminate cancer cells, while PDT uses PSs to produce excessive ROS in the present of specific wavelengths of light. PTT, as a non‐invasive cancer treatment, can cause strong tumor ablation and simultaneously induce heat shock proteins produced by tumor cells, which is a family of proteins with moderate immunostimulant function (106). In principle, PTT could also provide cancer treatment that causes relatively little damage to surrounding healthy tissue, since thermal effects only occur when near-infrared light is applied and only in the presence of PTT reagents (107). PDT takes advantage of the active metabolism of tumor tissue; whereby non-toxic photosensitizers accumulate in tumor tissue after injection. When the tumor tissue is irradiated with harmless visible light, the activated photosensitizer transfers its energy to surrounding intracellular oxygen that forms reactive oxygen species (ROS), which specifically destroy the tumor cells and neovascularization (108). PSs for PTT/PDT include organic dye molecules, organic nanoparticles, noble metal materials, carbon-based materials, quantum dots, and metal oxide nanoparticles (109–111). The in vivo distribution pattern and local drug concentration of PSs are critical factors determining the antitumor efficacy and systemic toxicity for PTT/PDT.
In bacteria-mediated PTT/PDT therapy, PSs are attached to the surface of bacteria to construct a bacteria-driven PSs delivery system, which significantly improves the tumor-targeting efficacy of PSs. For example, Chen et al. attached the INPs (PSs-containing indocyanine green (ICG)) to the surface of YB1 (an attenuated therapeutic Salmonella Typhimurium strain) via amide bonds, and the YB1-INPs complex achieved effective targeting hypoxic areas in tumor, thus eliminating bulk tumor tissues in mice after NIR laser irradiation. Meanwhile, YB1-INPs also exhibited satisfying fluorescence (FL) imaging ability (112). Moreover, many bacteria could immobilize environmental metal ions on cell surface through biomineralization process (113). Inspired by this phenomenon, Zhang’s group conjugated palladium nanoparticles (Pd NPs) on the surface of the facultative anaerobic bacterium Shewanella oneidensis MR-1 by biomineralization, and such bacteria-based photothermal therapeutical platform significantly increased photothermal capacity under near-infrared (NIR) laser irradiation (114). In addition to biomineralization, Cheng-Hung Luo et al. utilized cargo-carrying method and antibody-directed method to decorate anaerobic Bifidobacterium breve and Clostridium difficile with upconversion nanorods for tumor imaging and Au nanorods for photothermal ablation upon NIR excitation (115).
The combination of BCT and gene therapy
In addition to chemotherapies, bacteria are effective carriers for gene therapies in the form of DNA and RNA. Plasmid is frequently used for bacteria-mediated gene therapies, due to its versatility for the engineering of genetic components, accessibility for massive production, high stability in physiology environment, as well as chemical activeness for further modifications. The gene products delivered by plasmids could be peptides, proteins, short hairpin RNAs and long non-coding RNAs. Both prokaryotic plasmids and eukaryotic plasmids can be delivered by bacteria, but their applications are different for cancer therapies.
Prokaryotic plasmids produce recombinant peptides and proteins within the bacterial protoplasm, and then these products are allocated to cytoplasm, membrane or extracellular space according to their sequence signature. Generally, the recombinant proteins produced by prokaryotic plasmids are released to TME either by secretion, bacterial lysis or endocytosis by multiple types of cells such as cancer cells, macrophages and granule cells (116, 117). For example, Loeffler et al. introduced the gene LIGHT, which encoded a human cytokine mediating tissue rejection, into attenuated Salmonella typhimurium by plasmid pGEN206, and the transformed Salmonella exhibited significantly improved antitumour activity without additional in vitro toxicity in murine carcinoma cell lines (118). Additionally, Escherichia coli stably transformed with a plasmid constitutively expressing nanobody antagonist against CD47 could stimulate systemic tumor antigen-specific immune responses, and induced durable tumor regression and long-term survival in a syngeneic tumor model (119). Such constitutive expressing systems are more suitable for the delivery of immune modulators compared to cytotoxin, since the short time window of uncontrolled bacterial distribution before they are restricted in TME could augment immune responses in favor of tumor elimination, while unintended delivery of cytotoxin to healthy organs would lead to systemic toxicity.
The incorporation of promoters in response to environmental signals, such as hypoxia, low pH and exogenous compounds, allows spatial and temporal regulation on the production of therapeutic molecules in TME. For example, hypoxia-inducible promoters, such as HIP-1 and NirB, are utilized to restrict gene expression within hypoxic TME, thus reducing toxicity to normal tissues (120–124). Meanwhile, chemical-inducible promoters (e.g., pBAD, pTet, and Pm) can activate gene expression through systemic administration of transcriptional inducers (e.g., L-arabinose, tetracyclines, and acetyl salicylic acid, respectively) in vivo (125–129).
In most cases, therapeutic proteins produced by bacteria need to be released into TME to convey biological effects (116). Therefore, the bacteria strains can be engineered to induce autolysis within TME, thus releasing therapeutic payloads in a controlled manner. For example, Eva María Camacho et al. developed an inducible autolysis system in response to anhydrotetracycline, in combination with a salicylate cascade system that allowed efficient production of therapeutic molecules in response to aspirin and a sifA mutation that liberated bacteria from the vacuoles to a cytosolic location for therapeutic Salmonella (130). Moreover, M. Omar Din et al. engineered Salmonella Typhimurium to lyse synchronously at a threshold population density and to release genetically encoded cargo in a pulsatile manner (131). Such engineering strategies may inspire development of therapeutic bio-communities within in vivo environments, where population dynamics are driven by interacting viruses, bacteria, and host immune cells (132).
Eukaryotic plasmids utilize the transcription and translation machinery of mammalian cells. Compared to prokaryotic systems, eukaryotic systems have the following advantages: firstly, protein products of eukaryotic systems are more biologically active due to the integrity of post-transcriptional modifications and higher structure, especially for those proteins with large molecular weight (133–135). In a study using Salmonella Typhimurium as a delivery vehicle, β-galactosidase expressed from a eukaryotic cassette induced substantially stronger immune responses than that expressed from a prokaryotic cassette (136). Secondly, the choice of therapeutic genes is more flexible, regardless of their subcellular locations of action. For example, RNA-based gene therapies often adopt eukaryotic plasmids to directly produce functional RNAs within target cells, thus bypassing the restrictions of RNA uptake efficacy in vivo. Huijie Jia et al. reported that attenuated Salmonella Typhimurium harboring eukaryotic plasmids expressing endostatin and Stat3-specific small interfering RNA (siRNA) conferred significant tumor-suppression effects in mouse tumor models (137). Moreover, therapeutic bacteria carrying eukaryotic plasmids encoding cytokines such as IL-4 and IL-18, and angiogenesis inhibitors such as endostatin and thrombospondin all resulted in retardation of tumor growth and prolonged survival of tumor-bearing mice (134, 138–140). Last but not least, eukaryotic plasmids could be loaded either within the protoplasm or on the surface of bacteria. Therefore, a considerable amount of plasmids can be loaded to a single bacterium due to surface-to-volume ratio (127).
However, the efficacy of plasmid endocytosis is the bottleneck for the eukaryotic plasmid-based therapies. Macrophages, the most important phagocytes in TME, are the ideal target for bacteria-mediated gene therapies. G Dietrich et al. reported that eukaryotic expression vectors can be delivered to macrophages using attenuated self-destructing Listeria monocytogenes (141). Similarly, Igentschev et al. also developed a plasmid-vector system targeting APCs with Listeria monocytogenes, which expressed the heterologous antigens under the control of an eukaryotic promoter in a similar fashion as in plasmids commonly used for vaccination with naked DNA (142). In addition to live bacteria, inactivated bacteria are also capable as vehicles of gene therapies targeting macrophages. For example, M.R.Miri et al. showed that BGs loaded with pEGFP-C1 plasmids were efficiently captured by murine macrophages (102, 143).
There are also some non-plasmid-dependent gene delivery methods. For example, Shuya Lu et al. reported that Chloroquine combined with PD-1 siRNA delivered with attenuated Salmonella could significantly enhance the tumor growth inhibition through upregulation of the number and activity of immune cells in tumor tissues (144). Qin Guo et al. developed a system in which bacterial outer membrane vesicles were coloaded with PTX and Redd1-siRNA (siRNA@M-/PTX-CA-OMVs) to regulate the tumor metabolic microenvironment and suppress tumor growth. Upon reaching the tumor site, the system was first triggered by tumor pH (pH 6.8) to release PTX. Then, the rest of the system would be taken up by M2 macrophages to increase their level of glycolysis (145).
Bacteria-mediated gene delivery is not only a therapeutic strategy, but also a useful diagnostic method. Genomically engineered bacterial can be detected non-invasively by fluoroscopy (146), magnetic resonance imaging (MRI) (147) and positron emission tomography (PET) (148) scan, which could be used to show the location of tumors. For example, Escherichia coli carrying pMW211-dsred plasmid could mark cancerous cells in their exact localization by luminescent signals (149). Studies have shown that there are many microorganisms differentially enriched in healthy population and cancer patients, such as gastric cancer, colorectal cancer, prostate cancer and oral cancer. Among them, many studies are focused on colorectal cancer and intestinal microorganisms, such as Fusobacterium nucleatum, Peptostreptococcus stomatis and Streptococcus salivarius, etc. All of these mentioned bacterial species are positively correlated with the occurrence of colorectal cancer and are expected to be potential diagnostic markers (150–152).
Conclusion and perspective
In contrast with traditional cancer therapies such as chemotherapy and radiotherapy (153–156), which are less effective for the hypoxic tumors, therapeutic bacteria preferentially penetrate and colonize in hypoxic areas in the tumors. Therefore, it is beneficial to combine BCT with traditional cancer therapies. For example, the combination of Salmonella typhimurium with 5-fluorouracil inhibited MC26-LucF tumor growth and prolonged survival in mice (131). The combination of Salmonella typhimurium VNP20009 and Triptolide, a traditional Chinese medicine, showed significantly enhanced antitumor activity by modulating tumor angiogenesis and host immune response (77). Moreover, preclinical studies demonstrated that combined administration of Cytolysin A-expressing Salmonella typhimurium and radiation therapy could reduce tumor growth to a greater extent than bacterial monotherapy (157).
Apart from chemotherapy, radiation therapy is also commonly used for the treatment of solid tumors. While radiation therapy shows good efficacy for many types of cancer, its damage to surrounding normal tissues remains a difficult problem. A few studies regarding E. coli, Salmonella Typhimurium, Salmonella, Clostridium and other strains (158–161) have demonstrated that bacterial therapy combined with radiotherapy can reduce radiation-associated damage, improve the therapeutic effect in radiotherapy, and reduce metastasis (162). However, the mechanistic study on the combination of bacteria-mediated cancer therapy and radiotherapy is still superficial, and the behavior of cancer cells, bacteria, and especially immune cells during radiotherapy required more in-depth investigation.
However, the following questions remains to be solved for the research field of bacteria-mediated cancer therapy:
The choice and optimization of bacterial strains
The clinical development of BCT faces substantial obstacles, mainly due to potential infection-related toxicity. For example, the Phase II clinical trial of ADXS11-001, an attenuated Listeria monocytogenes therapeutic vaccine, in patients with oropharyngeal cancer (NCT01598792) were suspended after a patient developed systemic listeriosis following vaccination (163).
The ideal strain should meet the following criteria: ① High tumor specificity. For example, a Salmonella strain displaying an integrin-binding RGD peptide on its outer membrane protein A (OmpA) showed a >1000-fold enrichment in the αvβ3 integrin-expressing U87MG and M21 xenografts compared to the control strain and an impressively enhanced antitumor activity in the MDA-MB-231 and MDA-MB-435 xenograft tumor models (164). ② High tumor-killing effect. Bacteria can be engineered to obtain enhanced anti-tumor activity by means such as gene elements coding for cytotoxic agents, as discuss in previous chapters in this review. ③ No/low systemic toxicity. LPS is one of the most potent TNFα stimulators and thus responsible for Gram-negative sepsis (165). Deletion of the msbB gene from Salmonella results in loss of myristoylation of lipid A, a critical component of LPS, and reduces its toxicity of by 10,000-fold. For example, VNP20009 with deletions of purI and msbB, two genes necessary for adenine and lipid A synthesis, respectively, have been safely administered to patients with metastatic melanoma and renal cell carcinoma in a phase I clinical study (3, 4). However, no antitumor effect was observed in patients treated with VNP20009, which might due be to the over-attenuation of bacteria (3, 166). Therefore, it should be noted that some of the virulence factors may also be responsible for the intrinsic antitumor activity of live bacteria. Whenever possible, attenuation should be achieved without substantially compromising the antitumor activity, unless the bacterial strain is used for the purpose of vaccination only.
The choice of patients suitable for BCT
The standards for participant recruitments may greatly affect the outcomes of clinical trials (23). The risks and potential benefits must be considered carefully for first-in-human (FIH) trials. In general, only patients without any response to conventional therapies should be enrolled in clinical trials. Even though the bacteria are highly attenuated, the administration of live bacteria still poses a serious risk of infection. Therefore, the immune status and prior/concomitant therapies of patients should be evaluated sufficiently during the design of clinical trials (167). For example, immunocompromised patients who receive other immunotherapies simultaneously should be excluded (168). In addition, patients with foreign transplants such as artificial heart valves should be excluded, since foreign transplants may provide refuges for oncolytic bacteria to escape immune clearance and cause serious adverse reactions (168).
The interaction between therapeutic bacteria and host bacterial flora
The long-term interaction of bacterial therapy on the normal flora of patients have not been investigated. It is not known yet whether therapeutic bacteria would hibernate in the locations of immune exemption, which might lead to unexpected health crisis in the long term. Moreover, the influence of host bacterial flora, as well as the antibiotic usage habits might substantially influence the effect of bacteria-mediated cancer therapies, which have not been studied comprehensively. Therefore, successful bacteria-mediated cancer therapies require interdisciplinary expertise, including oncologists, infectious disease specialists, immunologists and microbiologists.
Although many published studies on bacteria-based biotherapies have shown promising therapeutic effect in experimental models, its drawbacks are equally evident. Firstly, safety is the major concern due to the infectious nature of the bacteria. Secondly, limited drug loading efficiency is another challenge dampening the anticancer effect of bacteria. Thirdly, the manufacturing process of live bacteria is more complex than that of the small molecule anticancer drugs. Last but not least, when live bacteria could be used in a clinical settings, the potential impact on the environment would be also a concern that should be properly addressed (169, 170).
In conclusion, BCT is an emerging category of experimental cancer treatment, and what we’ve discovered might be the tip of an iceberg. From the first attempt of Coley’s strategy until today, great progress has been achieved. Thus, with more understanding of its mechanism, the bacteria, as well as bacteria-related therapeutics would become powerful weapons in the battle against cancers in the near future.
Author contributions
ML, XC, HG and FY are responsible for the collection, collation, and writing of the manuscript. JC and YQ are responsible for the concept development, revision, and review of the manuscript. All authors contributed to the article and approved the submitted version.
Funding
This work was supported by funding from Natural Science Foundation of Zhejiang Province (LR21H160001, LY21H160025), the Research Project of Jinan Microecological Biomedicine Shandong Laboratory (JNL-2022029C), “Pioneer” and “Leading Goose” R&D Program of Zhejiang Province (2021C03G2153004), National Natural Science Foundation of China (82072646, 81903143), and Start-up Grant of HZNU (4125C5021820470), Zhejiang Xinmiao (New-Shoot) Talent Project of China (2021R426071).
Conflict of interest
The authors declare that the research was conducted in the absence of any commercial or financial relationships that could be construed as a potential conflict of interest.
Publisher’s note
All claims expressed in this article are solely those of the authors and do not necessarily represent those of their affiliated organizations, or those of the publisher, the editors and the reviewers. Any product that may be evaluated in this article, or claim that may be made by its manufacturer, is not guaranteed or endorsed by the publisher.
References
1. Schwabe RF, Jobin C. The microbiome and cancer. Nat Rev Cancer (2013) 13(11):800–12. doi: 10.1038/nrc3610
2. Nejman D, Livyatan I, Fuks G, Gavert N, Zwang Y, Geller LT, et al. The human tumor microbiome is composed of tumor type-specific intracellular bacteria. Science (2020) 368(6494):973–80. doi: 10.1126/science.aay9189
3. Toso JF, Gill VJ, Hwu P, Marincola FM, Restifo NP, Schwartzentruber DJ, et al. Phase I study of the intravenous administration of attenuated salmonella typhimurium to patients with metastatic melanoma. J Clin Oncol (2002) 20(1):142–52. doi: 10.1200/JCO.2002.20.1.142
4. Heimann DM, Rosenberg SA. Continuous intravenous administration of live genetically modified salmonella typhimurium in patients with metastatic melanoma. J Immunother (2003) 26(2):179–80. doi: 10.1097/00002371-200303000-00011
5. Nemunaitis J, Cunningham C, Senzer N, Kuhn J, Cramm J, Litz C, et al. Pilot trial of genetically modified, attenuated salmonella expressing the e. Coli Cytosine Deaminase Gene Refractory Cancer Patients Cancer Gene Ther (2003) 10(10):737–44. doi: 10.1038/sj.cgt.7700634
6. Schmitz-Winnenthal FH, Hohmann N, Niethammer AG, Friedrich T, Lubenau H, Springer M, et al. Anti-angiogenic activity of Vxm01, an oral T-cell vaccine against vegf receptor 2, in patients with advanced pancreatic cancer: A randomized, placebo-controlled, phase 1 trial. Oncoimmunology (2015) 4(4):e1001217. doi: 10.1080/2162402X.2014.1001217
7. Janku F, Zhang HH, Pezeshki A, Goel S, Murthy R, Wang-Gillam A, et al. Intratumoral injection of clostridium novyi-nt spores in patients with treatment-refractory advanced solid tumors. Clin Cancer Res (2021) 27(1):96–106. doi: 10.1158/1078-0432.CCR-20-2065
8. Roberts NJ, Zhang L, Janku F, Collins A, Bai RY, Staedtke V, et al. Intratumoral injection of clostridium novyi-nt spores induces antitumor responses. Sci Transl Med (2014) 6(249):249ra111. doi: 10.1126/scitranslmed.3008982
9. Le DT, Brockstedt DG, Nir-Paz R, Hampl J, Mathur S, Nemunaitis J, et al. A live-attenuated listeria vaccine (Anz-100) and a live-attenuated listeria vaccine expressing mesothelin (Crs-207) for advanced cancers: Phase I studies of safety and immune induction. Clin Cancer Res (2012) 18(3):858–68. doi: 10.1158/1078-0432.CCR-11-2121
10. Le DT, Wang-Gillam A, Picozzi V, Greten TF, Crocenzi T, Springett G, et al. Safety and survival with gvax pancreas prime and listeria monocytogenes-expressing mesothelin (Crs-207) boost vaccines for metastatic pancreatic cancer. J Clin Oncol (2015) 33(12):1325–33. doi: 10.1200/JCO.2014.57.4244
11. Hassan R, Alley E, Kindler H, Antonia S, Jahan T, Honarmand S, et al. Clinical response of live-attenuated, listeria monocytogenes expressing mesothelin (Crs-207) with chemotherapy in patients with malignant pleural mesothelioma. Clin Cancer Res (2019) 25(19):5787–98. doi: 10.1158/1078-0432.CCR-19-0070
12. Le DT, Picozzi VJ, Ko AH, Wainberg ZA, Kindler H, Wang-Gillam A, et al. Results from a phase iib, randomized, multicenter study of gvax pancreas and crs-207 compared with chemotherapy in adults with previously treated metastatic pancreatic adenocarcinoma (Eclipse study). Clin Cancer Res (2019) 25(18):5493–502. doi: 10.1158/1078-0432.CCR-18-2992
13. Huh WK, Brady WE, Fracasso PM, Dizon DS, Powell MA, Monk BJ, et al. Phase ii study of axalimogene filolisbac (Adxs-hpv) for platinum-refractory cervical carcinoma: An nrg Oncology/Gynecologic oncology group study. Gynecol Oncol (2020) 158(3):562–9. doi: 10.1016/j.ygyno.2020.06.493
14. Maciag PC, Radulovic S, Rothman J. The first clinical use of a live-attenuated listeria monocytogenes vaccine: A phase I safety study of lm-Llo-E7 in patients with advanced carcinoma of the cervix. Vaccine (2009) 27(30):3975–83. doi: 10.1016/j.vaccine.2009.04.041
15. Dizman N, Meza L, Bergerot P, Alcantara M, Dorff T, Lyou Y, et al. Nivolumab plus ipilimumab with or without live bacterial supplementation in metastatic renal cell carcinoma: A randomized phase 1 trial. Nat Med (2022) 28(4):704–12. doi: 10.1038/s41591-022-01694-6
16. Sfondrini L, Rossini A, Besusso D, Merlo A, Tagliabue E, Menard S, et al. Antitumor activity of the tlr-5 ligand flagellin in mouse models of cancer. J Immunol (2006) 176(11):6624–30. doi: 10.4049/jimmunol.176.11.6624
17. Avogadri F, Martinoli C, Petrovska L, Chiodoni C, Transidico P, Bronte V, et al. Cancer immunotherapy based on killing of salmonella-infected tumor cells. Cancer Res (2005) 65(9):3920–7. doi: 10.1158/0008-5472.CAN-04-3002
18. Gu WY, Wang LH, Wu YH, Liu JP. Undo the brake of tumour immune tolerance with antibodies, peptide mimetics and small molecule compounds targeting pd-1/Pd-L1 checkpoint at different locations for acceleration of cytotoxic immunity to cancer cells. Clin Exp Pharmacol P (2019) 46(2):105–15. doi: 10.1111/1440-1681.13056
19. Fang YY, Kang YH, Zou H, Cheng XX, Xie T, Shi LY, et al. Beta-elemene attenuates macrophage activation and proinflammatory factor production Via crosstalk with Wnt/Beta-catenin signaling pathway. Fitoterapia (2018) 124:92–102. doi: 10.1016/j.fitote.2017.10.015
20. Chen Q, Bai H, Wu W, Huang G, Li Y, Wu M, et al. Bioengineering bacterial vesicle-coated polymeric nanomedicine for enhanced cancer immunotherapy and metastasis prevention. Nano Lett (2020) 20(1):11–21. doi: 10.1021/acs.nanolett.9b02182
21. Eberl G, Boneca IG. Bacteria and mamp-induced morphogenesis of the immune system. Curr Opin Immunol (2010) 22(4):448–54. doi: 10.1016/j.coi.2010.06.002
22. Drozdz M, Makuch S, Cieniuch G, Wozniak M, Ziolkowski P. Obligate and facultative anaerobic bacteria in targeted cancer therapy: Current strategies and clinical applications. Life Sci (2020) 261:118296. doi: 10.1016/j.lfs.2020.118296
23. Zhou S, Gravekamp C, Bermudes D, Liu K. Tumour-targeting bacteria engineered to fight cancer. Nat Rev Cancer (2018) 18(12):727–43. doi: 10.1038/s41568-018-0070-z
24. Liu XH, Wang J. The nucleosome remodeling and deacetylase complex has prognostic significance and associates with immune microenvironment in skin cutaneous melanoma. Int Immunopharmacol (2020) 88:106887. doi: 10.1016/j.intimp.2020.106887
25. Putker F, Bos MP, Tommassen J. Transport of lipopolysaccharide to the gram-negative bacterial cell surface. FEMS Microbiol Rev (2015) 39(6):985–1002. doi: 10.1093/femsre/fuv026
26. Park BS, Lee JO. Recognition of lipopolysaccharide pattern by Tlr4 complexes. Exp Mol Med (2013) 45:e66. doi: 10.1038/emm.2013.97
27. Jain S, Dash P, Minz AP, Satpathi S, Samal AG, Behera PK, et al. Lipopolysaccharide (Lps) enhances prostate cancer metastasis potentially through nf-kappab activation and recurrent dexamethasone administration fails to suppress it in vivo. Prostate (2019) 79(2):168–82. doi: 10.1002/pros.23722
28. Beutler B, Cerami A. The biology of Cachectin/Tnf–a primary mediator of the host response. Annu Rev Immunol (1989) 7:625–55. doi: 10.1146/annurev.iy.07.040189.003205
29. Huang X, Zhu J, Jiang Y, Xu C, Lv Q, Yu D, et al. Su5416 attenuated lipopolysaccharide-induced acute lung injury in mice by modulating properties of vascular endothelial cells. Drug Des Devel Ther (2019) 13:1763–72. doi: 10.2147/DDDT.S188858
30. Darji A, Guzman CA, Gerstel B, Wachholz P, Timmis KN, Wehland J, et al. Oral somatic transgene vaccination using attenuated s. typhimurium. Cell (1997) 91(6):765–75. doi: 10.1016/s0092-8674(00)80465-1
31. Cochlovius B, Stassar MJ, Schreurs MW, Benner A, Adema GJ. Oral DNA vaccination: Antigen uptake and presentation by dendritic cells elicits protective immunity. Immunol Lett (2002) 80(2):89–96. doi: 10.1016/s0165-2478(01)00313-3
32. Phan TX, Nguyen VH, Duong MT, Hong Y, Choy HE, Min JJ. Activation of inflammasome by attenuated salmonella typhimurium in bacteria-mediated cancer therapy. Microbiol Immunol (2015) 59(11):664–75. doi: 10.1111/1348-0421.12333
33. Chen J, Qiao Y, Chen G, Chang C, Dong H, Tang B, et al. Salmonella flagella confer anti-tumor immunological effect Via activating Flagellin/Tlr5 signalling within tumor microenvironment. Acta Pharm Sin B (2021) 11(10):3165–77. doi: 10.1016/j.apsb.2021.04.019
34. Leigh ND, Bian G, Ding X, Liu H, Aygun-Sunar S, Burdelya LG, et al. A flagellin-derived toll-like receptor 5 agonist stimulates cytotoxic lymphocyte-mediated tumor immunity. PloS One (2014) 9(1):e85587. doi: 10.1371/journal.pone.0085587
35. Coll F, Harrison EM, Toleman MS, Reuter S, Raven KE, Blane B, et al. Longitudinal genomic surveillance of mrsa in the uk reveals transmission patterns in hospitals and the community. Sci Transl Med (2017) 9(413):eaak9745. doi: 10.1126/scitranslmed.aak9745
36. Nguyen CT, Hong SH, Sin JI, Vu HV, Jeong K, Cho KO, et al. Flagellin enhances tumor-specific Cd8(+) T cell immune responses through Tlr5 stimulation in a therapeutic cancer vaccine model. Vaccine (2013) 31(37):3879–87. doi: 10.1016/j.vaccine.2013.06.054
37. Krieg AM. Cpg motifs in bacterial DNA and their immune effects. Annu Rev Immunol (2002) 20:709–60. doi: 10.1146/annurev.immunol.20.100301.064842
38. Bauer S, Kirschning CJ, Hacker H, Redecke V, Hausmann S, Akira S, et al. Human Tlr9 confers responsiveness to bacterial DNA Via species-specific cpg motif recognition. Proc Natl Acad Sci U.S.A. (2001) 98(16):9237–42. doi: 10.1073/pnas.161293498
39. Hemmi H, Takeuchi O, Kawai T, Kaisho T, Sato S, Sanjo H, et al. A toll-like receptor recognizes bacterial DNA. Nature (2000) 408(6813):740–5. doi: 10.1038/35047123
40. Takeshita F, Leifer CA, Gursel I, Ishii KJ, Takeshita S, Gursel M, et al. Cutting edge: Role of toll-like receptor 9 in cpg DNA-induced activation of human cells. J Immunol (2001) 167(7):3555–8. doi: 10.4049/jimmunol.167.7.3555
41. Weighardt H, Feterowski C, Veit M, Rump M, Wagner H, Holzmann B. Increased resistance against acute polymicrobial sepsis in mice challenged with immunostimulatory cpg oligodeoxynucleotides is related to an enhanced innate effector cell response. J Immunol (2000) 165(8):4537–43. doi: 10.4049/jimmunol.165.8.4537
42. Hartmann G, Krieg AM. Mechanism and function of a newly identified cpg DNA motif in human primary b cells. J Immunol (2000) 164(2):944–53. doi: 10.4049/jimmunol.164.2.944
43. Lipford GB, Bendigs S, Heeg K, Wagner H. Poly-guanosine motifs costimulate antigen-reactive Cd8 T cells while bacterial cpg-DNA affect T-cell activation Via antigen-presenting cell-derived cytokines. Immunology (2000) 101(1):46–52. doi: 10.1046/j.1365-2567.2000.00077.x
44. Bauer M, Redecke V, Ellwart JW, Scherer B, Kremer JP, Wagner H, et al. Bacterial cpg-DNA triggers activation and maturation of human Cd11c-, Cd123+ dendritic cells. J Immunol (2001) 166(8):5000–7. doi: 10.4049/jimmunol.166.8.5000
45. Liang H, Reich CF, Pisetsky DS, Lipsky PE. The role of cell surface receptors in the activation of human b cells by phosphorothioate oligonucleotides. J Immunol (2000) 165(3):1438–45. doi: 10.4049/jimmunol.165.3.1438
46. Stacey KJ, Sester DP, Sweet MJ, Hume DA. Macrophage activation by immunostimulatory DNA. Curr Top Microbiol Immunol (2000) 247:41–58. doi: 10.1007/978-3-642-59672-8_3
47. Bao YF, Chen QJ, Xie YS, Tao Z, Jin KH, Chen SL, et al. Ferulic acid attenuates oxidative DNA damage and inflammatory responses in microglia induced by Benzo(a)Pyrene. Int Immunopharmacol (2019) 77:105980. doi: 10.1016/j.intimp.2019.105980
48. Stern C, Kasnitz N, Kocijancic D, Trittel S, Riese P, Guzman CA, et al. Induction of Cd4(+) and Cd8(+) anti-tumor effector T cell responses by bacteria mediated tumor therapy. Int J Cancer (2015) 137(8):2019–28. doi: 10.1002/ijc.29567
49. Zhang Y, Miwa S, Zhang N, Hoffman RM, Zhao M. Tumor-targeting salmonella typhimurium A1-r arrests growth of breast-cancer brain metastasis. Oncotarget (2015) 6(5):2615–22. doi: 10.18632/oncotarget.2811
50. Alimoradi H, Matikonda SS, Gamble AB, Giles GI, Greish K. Hypoxia responsive drug delivery systems in tumor therapy. Curr Pharm Des (2016) 22(19):2808–20. doi: 10.2174/1381612822666160217130049
51. Mei Y, Zhao L, Liu Y, Gong H, Song Y, Lei L, et al. Combining DNA vaccine and Aida-1 in attenuated salmonella activates tumor-specific Cd4(+) and Cd8(+) T-cell responses. Cancer Immunol Res (2017) 5(6):503–14. doi: 10.1158/2326-6066.CIR-16-0240-T
52. Xiong G, Husseiny MI, Song L, Erdreich-Epstein A, Shackleford GM, Seeger RC, et al. Novel cancer vaccine based on genes of salmonella pathogenicity island 2. Int J Cancer (2010) 126(11):2622–34. doi: 10.1002/ijc.24957
53. Patel RB, Ye M, Carlson PM, Jaquish A, Zangl L, Ma B, et al. Development of an in situ cancer vaccine Via combinational radiation and bacterial-Membrane-Coated nanoparticles. Adv Mater (2019) 31(43):e1902626. doi: 10.1002/adma.201902626
54. Uchugonova A, Zhang Y, Salz R, Liu F, Suetsugu A, Zhang L, et al. Imaging the different mechanisms of prostate cancer cell-killing by tumor-targeting salmonella typhimurium A1-r. Anticancer Res (2015) 35(10):5225–9.
55. Bettegowda C, Huang X, Lin J, Cheong I, Kohli M, Szabo SA, et al. The genome and transcriptomes of the anti-tumor agent clostridium novyi-nt. Nat Biotechnol (2006) 24(12):1573–80. doi: 10.1038/nbt1256
56. Li L, You LS, Mao LP, Jin SH, Chen XH, Qian WB. Combing oncolytic adenovirus expressing beclin-1 with chemotherapy agent doxorubicin synergistically enhances cytotoxicity in human cml cells in vitro. Acta Pharmacol Sin (2018) 39(2):251–60. doi: 10.1038/aps.2017.100
57. Cai Z, Sanchez A, Shi Z, Zhang T, Liu M, Zhang D. Activation of toll-like receptor 5 on breast cancer cells by flagellin suppresses cell proliferation and tumor growth. Cancer Res (2011) 71(7):2466–75. doi: 10.1158/0008-5472.CAN-10-1993
58. Lee CH, Lin ST, Liu JJ, Chang WW, Hsieh JL, Wang WK. Salmonella induce autophagy in melanoma by the downregulation of Akt/Mtor pathway. Gene Ther (2014) 21(3):309–16. doi: 10.1038/gt.2013.86
59. Kim SH, Castro F, Paterson Y, Gravekamp C. High efficacy of a listeria-based vaccine against metastatic breast cancer reveals a dual mode of action. Cancer Res (2009) 69(14):5860–6. doi: 10.1158/0008-5472.CAN-08-4855
60. Chang WW, Lai CH, Chen MC, Liu CF, Kuan YD, Lin ST, et al. Salmonella enhance chemosensitivity in tumor through connexin 43 upregulation. Int J Cancer (2013) 133(8):1926–35. doi: 10.1002/ijc.28155
61. Huang J, Zhang L, Wan D, Zhou L, Zheng S, Lin S, et al. Extracellular matrix and its therapeutic potential for cancer treatment. Signal Transduct Target Ther (2021) 6(1):153. doi: 10.1038/s41392-021-00544-0
62. Kalluri R. Basement membranes: Structure, assembly and role in tumour angiogenesis. Nat Rev Cancer (2003) 3(6):422–33. doi: 10.1038/nrc1094
63. Insua-Rodriguez J, Oskarsson T. The extracellular matrix in breast cancer. Adv Drug Delivery Rev (2016) 97:41–55. doi: 10.1016/j.addr.2015.12.017
64. Paszek MJ, Zahir N, Johnson KR, Lakins JN, Rozenberg GI, Gefen A, et al. Tensional homeostasis and the malignant phenotype. Cancer Cell (2005) 8(3):241–54. doi: 10.1016/j.ccr.2005.08.010
65. Alonso-Nocelo M, Raimondo TM, Vining KH, Lopez-Lopez R, de la Fuente M, Mooney DJ. Matrix stiffness and tumor-associated macrophages modulate epithelial to mesenchymal transition of human adenocarcinoma cells. Biofabrication (2018) 10(3):035004. doi: 10.1088/1758-5090/aaafbc
66. Netti PA, Berk DA, Swartz MA, Grodzinsky AJ, Jain RK. Role of extracellular matrix assembly in interstitial transport in solid tumors. Cancer Res (2000) 60(9):2497–503.
67. Rahbari NN, Kedrin D, Incio J, Liu H, Ho WW, Nia HT, et al. Anti-vegf therapy induces ecm remodeling and mechanical barriers to therapy in colorectal cancer liver metastases. Sci Transl Med (2016) 8(360):360ra135. doi: 10.1126/scitranslmed.aaf5219
68. Fan YF, Shang WT, Lu GH, Guo KX, Deng H, Zhu XH, et al. Decreasing hyaluronic acid combined with drug-loaded nanoprobes improve the delivery and efficacy of chemotherapeutic drugs for pancreatic cancer. Cancer Lett (2021) 523:1–9. doi: 10.1016/j.canlet.2021.09.016
69. Haiko J, Laakkonen L, Juuti K, Kalkkinen N, Korhonen TK. The omptins of yersinia pestis and salmonella enterica cleave the reactive center loop of plasminogen activator inhibitor 1. J Bacteriol (2010) 192(18):4553–61. doi: 10.1128/JB.00458-10
70. Bitar AP, Cao M, Marquis H. The metalloprotease of listeria monocytogenes is activated by intramolecular autocatalysis. J Bacteriol (2008) 190(1):107–11. doi: 10.1128/JB.00852-07
71. Canard B, Garnier T, Saint-Joanis B, Cole ST. Molecular genetic analysis of the nagh gene encoding a hyaluronidase of clostridium perfringens. Mol Gen Genet (1994) 243(2):215–24. doi: 10.1007/BF00280319
72. Hafiz S, Oakley CL. Clostridium difficile: Isolation and characteristics. J Med Microbiol (1976) 9(2):129–36. doi: 10.1099/00222615-9-2-129
73. Princewill TJ, Oakley CL. Deoxyribonucleases and hyaluronidases of clostridium septicum and clostridium chauvoei. iii. relationship between the two organisms. Med Lab Sci (1976) 33(2):10–118.
74. Steukers L, Glorieux S, Vandekerckhove AP, Favoreel HW, Nauwynck HJ. Diverse microbial interactions with the basement membrane barrier. Trends Microbiol (2012) 20(3):147–55. doi: 10.1016/j.tim.2012.01.001
75. Ebelt ND, Zuniga E, Passi KB, Sobocinski LJ, Manuel ER. Hyaluronidase-expressing salmonella effectively targets tumor-associated hyaluronic acid in pancreatic ductal adenocarcinoma. Mol Cancer Ther (2020) 19(2):706–16. doi: 10.1158/1535-7163.MCT-19-0556
76. Jia LJ, Wei DP, Sun QM, Jin GH, Li SF, Huang Y, et al. Tumor-targeting salmonella typhimurium improves cyclophosphamide chemotherapy at maximum tolerated dose and low-dose metronomic regimens in a murine melanoma model. Int J Cancer (2007) 121(3):666–74. doi: 10.1002/ijc.22688
77. Chen J, Qiao Y, Tang B, Chen G, Liu X, Yang B, et al. Modulation of salmonella tumor-colonization and intratumoral anti-angiogenesis by triptolide and its mechanism. Theranostics (2017) 7(8):2250–60. doi: 10.7150/thno.18816
78. Lee CH, Wu CL, Shiau AL. Salmonella choleraesuis as an anticancer agent in a syngeneic model of orthotopic hepatocellular carcinoma. Int J Cancer (2008) 122(4):930–5. doi: 10.1002/ijc.23047
79. Bowden SD, Rowley G, Hinton JC, Thompson A. Glucose and glycolysis are required for the successful infection of macrophages and mice by salmonella enterica serovar typhimurium. Infect Immun (2009) 77(7):3117–26. doi: 10.1128/IAI.00093-09
80. Grubmuller S, Schauer K, Goebel W, Fuchs TM, Eisenreich W. Analysis of carbon substrates used by listeria monocytogenes during growth in J774a.1 macrophages suggests a bipartite intracellular metabolism. Front Cell Infect Microbiol (2014) 4:156. doi: 10.3389/fcimb.2014.00156
81. Yan S, Zeng X, Wang Y, Liu BF. Biomineralization of bacteria by a metal-organic framework for therapeutic delivery. Adv Healthc Mater (2020) 9(12):e2000046. doi: 10.1002/adhm.202000046
82. Park BW, Zhuang J, Yasa O, Sitti M. Multifunctional bacteria-driven microswimmers for targeted active drug delivery. ACS Nano (2017) 11(9):8910–23. doi: 10.1021/acsnano.7b03207
83. Li J, Ying S, Ren H, Dai J, Zhang L, Liang L, et al. Molecular dynamics study on the encapsulation and release of anti-cancer drug doxorubicin by chitosan. Int J Pharm (2020) 580:119241. doi: 10.1016/j.ijpharm.2020.119241
84. Feng C, Ouyang J, Tang ZM, Kong N, Liu Y, Fu LY, et al. Germanene-based theranostic materials for surgical adjuvant treatment: Inhibiting tumor recurrence and wound infection. Matter-Us (2020) 3(1):127–44. doi: 10.1016/j.matt.2020.04.022
85. Kong N, Zhang RN, Wu GW, Sui XB, Wang JQ, Kim NY, et al. Intravesical delivery of Kdm6a-mrna Via mucoadhesive nanoparticles inhibits the metastasis of bladder cancer. P Natl Acad Sci USA (2022) 119(7):e2112696119. doi: 10.1073/pnas.2112696119
86. Ma HY, Jiang ZM, Xu JY, Liu JQ, Guo ZN. Targeted nano-delivery strategies for facilitating thrombolysis treatment in ischemic stroke. Drug Delivery (2021) 28(1):357–71. doi: 10.1080/10717544.2021.1879315
87. Akin D, Sturgis J, Ragheb K, Sherman D, Burkholder K, Robinson JP, et al. Bacteria-mediated delivery of nanoparticles and cargo into cells. Nat Nanotechnol (2007) 2(7):441–9. doi: 10.1038/nnano.2007.149
88. Stanton MM, Park BW, Vilela D, Bente K, Faivre D, Sitti M, et al. Magnetotactic bacteria powered biohybrids target e. coli biofilms. ACS Nano (2017) 11(10):9968–78. doi: 10.1021/acsnano.7b04128
89. Alapan Y, Yasa O, Schauer O, Giltinan J, Tabak AF, Sourjik V, et al. Soft erythrocyte-based bacterial microswimmers for cargo delivery. Sci Robot (2018) 3(17):eaar4423. doi: 10.1126/scirobotics.aar4423
90. Buss N, Yasa O, Alapan Y, Akolpoglu MB, Sitti M. Nanoerythrosome-functionalized biohybrid microswimmers. APL Bioeng (2020) 4(2):026103. doi: 10.1063/1.5130670
91. Cao Z, Cheng S, Wang X, Pang Y, Liu J. Camouflaging bacteria by wrapping with cell membranes. Nat Commun (2019) 10(1):3452. doi: 10.1038/s41467-019-11390-8
92. Felfoul O, Mohammadi M, Taherkhani S, de Lanauze D, Zhong Xu Y, Loghin D, et al. Magneto-aerotactic bacteria deliver drug-containing nanoliposomes to tumour hypoxic regions. Nat Nanotechnol (2016) 11(11):941–7. doi: 10.1038/nnano.2016.137
93. Naciute M, Kiwitt T, Kemp RA, Hook S. Bacteria biohybrid oral vaccines for colorectal cancer treatment reduce tumor growth and increase immune infiltration. Vaccine (2021) 39(39):5589–99. doi: 10.1016/j.vaccine.2021.08.028
94. Fan JX, Peng MY, Wang H, Zheng HR, Liu ZL, Li CX, et al. Engineered bacterial bioreactor for tumor therapy Via fenton-like reaction with localized H2 O2 generation. Adv Mater (2019) 31(16):e1808278. doi: 10.1002/adma.201808278
95. Zheng DW, Chen Y, Li ZH, Xu L, Li CX, Li B, et al. Optically-controlled bacterial metabolite for cancer therapy. Nat Commun (2018) 9(1):1680. doi: 10.1038/s41467-018-03233-9
96. Suh S, Jo A, Traore MA, Zhan Y, Coutermarsh-Ott SL, Ringel-Scaia VM, et al. Nanoscale bacteria-enabled autonomous drug delivery system (Nanobeads) enhances intratumoral transport of nanomedicine. Adv Sci (Weinh) (2019) 6(3):1801309. doi: 10.1002/advs.201801309
97. Huang Z, Sun X, Liu X, Shen Y, Wang K. Macrophages as an active tumour-targeting carrier of Sn38-nanoparticles for cancer therapy. J Drug Target (2018) 26(5-6):458–65. doi: 10.1080/1061186X.2017.1419359
98. Akolpoglu MB, Alapan Y, Dogan NO, Baltaci SF, Yasa O, Aybar Tural G, et al. Magnetically steerable bacterial microrobots moving in 3d biological matrices for stimuli-responsive cargo delivery. Sci Adv (2022) 8(28):eabo6163. doi: 10.1126/sciadv.abo6163
99. Deatherage BL, Cookson BT. Membrane vesicle release in bacteria, eukaryotes, and archaea: A conserved yet underappreciated aspect of microbial life. Infect Immun (2012) 80(6):1948–57. doi: 10.1128/IAI.06014-11
100. Montanaro J, Inic-Kanada A, Ladurner A, Stein E, Belij S, Bintner N, et al. Escherichia coli nissle 1917 bacterial ghosts retain crucial surface properties and express chlamydial antigen: An imaging study of a delivery system for the ocular surface. Drug Des Devel Ther (2015) 9:3741–54. doi: 10.2147/DDDT.S84370
101. Riedmann EM, Kyd JM, Cripps AW, Lubitz W. Bacterial ghosts as adjuvant particles. Expert Rev Vaccines (2007) 6(2):241–53. doi: 10.1586/14760584.6.2.241
102. Paukner S, Kudela P, Kohl G, Schlapp T, Friedrichs S, Lubitz W. DNA-Loaded bacterial ghosts efficiently mediate reporter gene transfer and expression in macrophages. Mol Ther (2005) 11(2):215–23. doi: 10.1016/j.ymthe.2004.09.024
103. Rabea S, Alanazi FK, Ashour AE, Salem-Bekhit MM, Yassin AS, Moneib NA, et al. Salmonella-innovative targeting carrier: Loading with doxorubicin for cancer treatment. Saudi Pharm J (2020) 28(10):1253–62. doi: 10.1016/j.jsps.2020.08.016
104. Paukner S, Kohl G, Jalava K, Lubitz W. Sealed bacterial ghosts–novel targeting vehicles for advanced drug delivery of water-soluble substances. J Drug Target (2003) 11(3):151–61. doi: 10.1080/10611860310001593366
105. Dobrovolskiene N, Pasukoniene V, Darinskas A, Krasko JA, Zilionyte K, Mlynska A, et al. Tumor lysate-loaded bacterial ghosts as a tool for optimized production of therapeutic dendritic cell-based cancer vaccines. Vaccine (2018) 36(29):4171–80. doi: 10.1016/j.vaccine.2018.06.016
106. Huang X, Pan J, Xu F, Shao B, Wang Y, Guo X, et al. Bacteria-based cancer immunotherapy. Adv Sci (Weinh) (2021) 8(7):2003572. doi: 10.1002/advs.202003572
107. Jung HS, Verwilst P, Sharma A, Shin J, Sessler JL, Kim JS. Organic molecule-based photothermal agents: An expanding photothermal therapy universe. Chem Soc Rev (2018) 47(7):2280–97. doi: 10.1039/c7cs00522a
108. Fan Z, Zhuang C, Wang S, Zhang Y. Photodynamic and photothermal therapy of hepatocellular carcinoma. Front Oncol (2021) 11:787780. doi: 10.3389/fonc.2021.787780
109. Li X, Lovell JF, Yoon J, Chen X. Clinical development and potential of photothermal and photodynamic therapies for cancer. Nat Rev Clin Oncol (2020) 17(11):657–74. doi: 10.1038/s41571-020-0410-2
110. Li J, Zhao J, Tan T, Liu M, Zeng Z, Zeng Y, et al. Nanoparticle drug delivery system for glioma and its efficacy improvement strategies: A comprehensive review. Int J Nanomedicine (2020) 15:2563–82. doi: 10.2147/IJN.S243223
111. Kong N, Deng M, Sun XN, Chen YD, Sui XB. Polydopamine-functionalized Ca-(Pcl-Ran-Pla) nanoparticles for target delivery of docetaxel and chemo-photothermal therapy of breast cancer. Front Pharmacol (2018) 9:125. doi: 10.3389/fphar.2018.00125
112. Chen F, Zang Z, Chen Z, Cui L, Chang Z, Ma A, et al. Nanophotosensitizer-engineered salmonella bacteria with hypoxia targeting and photothermal-assisted mutual bioaccumulation for solid tumor therapy. Biomaterials (2019) 214:119226. doi: 10.1016/j.biomaterials.2019.119226
113. Huang J, Lin L, Sun D, Chen H, Yang D, Li Q. Bio-inspired synthesis of metal nanomaterials and applications. Chem Soc Rev (2015) 44(17):6330–74. doi: 10.1039/c5cs00133a
114. Chen QW, Liu XH, Fan JX, Peng SY, Wang JW, Wang XN, et al. Self-mineralized photothermal bacteria hybridizing with mitochondria-targeted metal–organic frameworks for augmenting photothermal tumor therapy. Advanced Funct Materials (2020) 30(14):1909806. doi: 10.1002/adfm.201909806
115. Luo CH, Huang CT, Su CH, Yeh CS. Bacteria-mediated hypoxia-specific delivery of nanoparticles for tumors imaging and therapy. Nano Lett (2016) 16(6):3493–9. doi: 10.1021/acs.nanolett.6b00262
116. Russmann H, Shams H, Poblete F, Fu Y, Galan JE, Donis RO. Delivery of epitopes by the salmonella type iii secretion system for vaccine development. Science (1998) 281(5376):565–8. doi: 10.1126/science.281.5376.565
117. Walker BJ, Stan GV, Polizzi KM. Intracellular delivery of biologic therapeutics by bacterial secretion systems. Expert Rev Mol Med (2017) 19:e6. doi: 10.1017/erm.2017.7
118. Loeffler M, Le'Negrate G, Krajewska M, Reed JC. Attenuated salmonella engineered to produce human cytokine light inhibit tumor growth. Proc Natl Acad Sci U.S.A. (2007) 104(31):12879–83. doi: 10.1073/pnas.0701959104
119. Chowdhury S, Castro S, Coker C, Hinchliffe TE, Arpaia N, Danino T. Programmable bacteria induce durable tumor regression and systemic antitumor immunity. Nat Med (2019) 25(7):1057–63. doi: 10.1038/s41591-019-0498-z
120. Mengesha A, Dubois L, Lambin P, Landuyt W, Chiu RK, Wouters BG, et al. Development of a flexible and potent hypoxia-inducible promoter for tumor-targeted gene expression in attenuated salmonella. Cancer Biol Ther (2006) 5(9):1120–8. doi: 10.4161/cbt.5.9.2951
121. Yang YW, Zhang CM, Huang XJ, Zhang XX, Zhang LK, Li JH, et al. Tumor-targeted delivery of a c-terminally truncated fadd (N-fadd) significantly suppresses the B16f10 melanoma Via enhancing apoptosis. Sci Rep (2016) 6:34178. doi: 10.1038/srep34178
122. del Castillo FJ, Leal SC, Moreno F, del Castillo I. The escherichia coli K-12 Shea gene encodes a 34-kda secreted haemolysin. Mol Microbiol (1997) 25(1):107–15. doi: 10.1046/j.1365-2958.1997.4391813.x
123. Ludwig A, Tengel C, Bauer S, Bubert A, Benz R, Mollenkopf HJ, et al. Slya, a regulatory protein from salmonella typhimurium, induces a haemolytic and pore-forming protein in escherichia coli. Mol Gen Genet (1995) 249(5):474–86. doi: 10.1007/BF00290573
124. Oscarsson J, Mizunoe Y, Uhlin BE, Haydon DJ. Induction of haemolytic activity in escherichia coli by the slya gene product. Mol Microbiol (1996) 20(1):191–9. doi: 10.1111/j.1365-2958.1996.tb02500.x
125. Jiang SN, Park SH, Lee HJ, Zheng JH, Kim HS, Bom HS, et al. Engineering of bacteria for the visualization of targeted delivery of a cytolytic anticancer agent. Mol Ther (2013) 21(11):1985–95. doi: 10.1038/mt.2013.183
126. Kim K, Jeong JH, Lim D, Hong Y, Lim HJ, Kim GJ, et al. L-asparaginase delivered by salmonella typhimurium suppresses solid tumors. Mol Ther Oncolytics (2015) 2:15007. doi: 10.1038/mto.2015.7
127. Royo JL, Becker PD, Camacho EM, Cebolla A, Link C, Santero E, et al. In vivo gene regulation in salmonella spp. by a salicylate-dependent control circuit. Nat Methods (2007) 4(11):937–42. doi: 10.1038/nmeth1107
128. Cheng W, Miao L, Zhang H, Yang O, Ge H, Li Y, et al. Induction of interleukin 2 expression in the liver for the treatment of H22 hepatoma in mice. Dig Liver Dis (2013) 45(1):50–7. doi: 10.1016/j.dld.2012.08.014
129. Loessner H, Endmann A, Leschner S, Westphal K, Rohde M, Miloud T, et al. Remote control of tumour-targeted salmonella enterica serovar typhimurium by the use of l-arabinose as inducer of bacterial gene expression in vivo. Cell Microbiol (2007) 9(6):1529–37. doi: 10.1111/j.1462-5822.2007.00890.x
130. Camacho EM, Mesa-Pereira B, Medina C, Flores A, Santero E. Engineering salmonella as intracellular factory for effective killing of tumour cells. Sci Rep (2016) 6:30591. doi: 10.1038/srep30591
131. Din MO, Danino T, Prindle A, Skalak M, Selimkhanov J, Allen K, et al. Synchronized cycles of bacterial lysis for in vivo delivery. Nature (2016) 536(7614):81–5. doi: 10.1038/nature18930
132. Cheong I, Huang X, Bettegowda C, Diaz LA Jr., Kinzler KW, Zhou S, et al. A bacterial protein enhances the release and efficacy of liposomal cancer drugs. Science (2006) 314(5803):1308–11. doi: 10.1126/science.1130651
133. Hense M, Domann E, Krusch S, Wachholz P, Dittmar KE, Rohde M, et al. Eukaryotic expression plasmid transfer from the intracellular bacterium listeria monocytogenes to host cells. Cell Microbiol (2001) 3(9):599–609. doi: 10.1046/j.1462-5822.2001.00138.x
134. Lee CH, Wu CL, Shiau AL. Systemic administration of attenuated salmonella choleraesuis carrying thrombospondin-1 gene leads to tumor-specific transgene expression, delayed tumor growth and prolonged survival in the murine melanoma model. Cancer Gene Ther (2005) 12(2):175–84. doi: 10.1038/sj.cgt.7700777
135. Fu W, Lan H, Li S, Han X, Gao T, Ren D. Synergistic antitumor efficacy of Suicide/Epnp gene and 6-methylpurine 2'-deoxyriboside Via salmonella against murine tumors. Cancer Gene Ther (2008) 15(7):474–84. doi: 10.1038/cgt.2008.19
136. Darji A, zur Lage S, Garbe AI, Chakraborty T, Weiss S. Oral delivery of DNA vaccines using attenuated salmonella typhimurium as carrier. FEMS Immunol Med Microbiol (2000) 27(4):341–9. doi: 10.1111/j.1574-695X.2000.tb01448.x
137. Jia H, Li Y, Zhao T, Li X, Hu J, Yin D, et al. Antitumor effects of Stat3-sirna and endostatin combined therapies, delivered by attenuated salmonella, on orthotopically implanted hepatocarcinoma. Cancer Immunol Immunother (2012) 61(11):1977–87. doi: 10.1007/s00262-012-1256-y
138. Agorio C, Schreiber F, Sheppard M, Mastroeni P, Fernandez M, Martinez MA, et al. Live attenuated salmonella as a vector for oral cytokine gene therapy in melanoma. J Gene Med (2007) 9(5):416–23. doi: 10.1002/jgm.1023
139. Liang K, Liu Q, Li P, Han Y, Bian X, Tang Y, et al. Endostatin gene therapy delivered by attenuated salmonella typhimurium in murine tumor models. Cancer Gene Ther (2018) 25(7-8):167–83. doi: 10.1038/s41417-018-0021-6
140. Lee CH, Wu CL, Shiau AL. Endostatin gene therapy delivered by salmonella choleraesuis in murine tumor models. J Gene Med (2004) 6(12):1382–93. doi: 10.1002/jgm.626
141. Dietrich G, Bubert A, Gentschev I, Sokolovic Z, Simm A, Catic A, et al. Delivery of antigen-encoding plasmid DNA into the cytosol of macrophages by attenuated suicide listeria monocytogenes. Nat Biotechnol (1998) 16(2):181–5. doi: 10.1038/nbt0298-181
142. Gentschev I, Dietrich G, Spreng S, Kolb-Maurer A, Daniels J, Hess J, et al. Delivery of protein antigens and DNA by virulence-attenuated strains of salmonella typhimurium and listeria monocytogenes. J Biotechnol (2000) 83(1-2):19–26. doi: 10.1016/s0168-1656(00)00293-5
143. Miri MR, Behzad-Behbahani A, Fardaei M, Farhadi A, Talebkhan Y, Mohammadi M, et al. Construction of bacterial ghosts for transfer and expression of a chimeric hepatitis c virus gene in macrophages. J Microbiol Methods (2015) 119:228–32. doi: 10.1016/j.mimet.2015.11.009
144. Lu S, Gao J, Jia H, Li Y, Duan Y, Song F, et al. Pd-1-Sirna delivered by attenuated salmonella enhances the antitumor effect of chloroquine in colon cancer. Front Immunol (2021) 12:707991. doi: 10.3389/fimmu.2021.707991
145. Guo Q, Li X, Zhou W, Chu Y, Chen Q, Zhang Y, et al. Sequentially triggered bacterial outer membrane vesicles for macrophage metabolism modulation and tumor metastasis suppression. ACS Nano (2021) 15(8):13826–38. doi: 10.1021/acsnano.1c05613
146. Yu YA, Weibel S, Szalay AA. Real-time imaging of tumors using replication-competent light-emitting microorganisms. Methods Mol Biol (2012) 872:159–75. doi: 10.1007/978-1-61779-797-2_11
147. Alphandery E. Applications of magnetosomes synthesized by magnetotactic bacteria in medicine. Front Bioeng Biotechnol (2014) 2:5. doi: 10.3389/fbioe.2014.00005
148. Brader P, Stritzker J, Riedl CC, Zanzonico P, Cai S, Burnazi EM, et al. Escherichia coli nissle 1917 facilitates tumor detection by positron emission tomography and optical imaging. Clin Cancer Res (2008) 14(8):2295–302. doi: 10.1158/1078-0432.CCR-07-4254
149. Sorensen M, Lippuner C, Kaiser T, Misslitz A, Aebischer T, Bumann D. Rapidly maturing red fluorescent protein variants with strongly enhanced brightness in bacteria. FEBS Lett (2003) 552(2-3):110–4. doi: 10.1016/s0014-5793(03)00856-1
150. Zeller G, Tap J, Voigt AY, Sunagawa S, Kultima JR, Costea PI, et al. Potential of fecal microbiota for early-stage detection of colorectal cancer. Mol Syst Biol (2014) 10:766. doi: 10.15252/msb.20145645
151. Eklof V, Lofgren-Burstrom A, Zingmark C, Edin S, Larsson P, Karling P, et al. Cancer-associated fecal microbial markers in colorectal cancer detection. Int J Cancer (2017) 141(12):2528–36. doi: 10.1002/ijc.31011
152. Mima K, Nishihara R, Qian ZR, Cao Y, Sukawa Y, Nowak JA, et al. Fusobacterium nucleatum in colorectal carcinoma tissue and patient prognosis. Gut (2016) 65(12):1973–80. doi: 10.1136/gutjnl-2015-310101
153. Wang XW, Liu ZT, Sui XB, Wu QB, Wang J, Xu C. Elemene injection as adjunctive treatment to platinum-based chemotherapy in patients with stage Iii/Iv non-small cell lung cancer: A meta-analysis following the prisma guidelines. Phytomedicine (2019) 59:152787. doi: 10.1016/j.phymed.2018.12.010
154. Lou JS, Yao P, Tsim KWK. Cancer treatment by using traditional Chinese medicine probing active compounds in anti-multidrug resistance during drug therapy. Curr Med Chem (2018) 25(38):5128–41. doi: 10.2174/0929867324666170920161922
155. Cheng HB, Ge XY, Zhuo SQ, Gao YA, Zhu B, Zhang JF, et al. Beta-elemene synergizes with gefitinib to inhibit stem-like phenotypes and progression of lung cancer Via down-regulating Ezh2. Front Pharmacol (2018) 9:1413. doi: 10.3389/fphar.2018.01413
156. Li YC, Sui XB, Su ZQ, Yu CY, Shi XG, Johnson NL, et al. Meta-analysis of paclitaxel-based chemotherapy combined with traditional Chinese medicines for gastric cancer treatment. Front Pharmacol (2020) 11:132. doi: 10.3389/fphar.2020.00132
157. Liu X, Jiang S, Piao L, Yuan F. Radiotherapy combined with an engineered salmonella typhimurium inhibits tumor growth in a mouse model of colon cancer. Exp Anim (2016) 65(4):413–8. doi: 10.1538/expanim.16-0033
158. Burdelya LG, Krivokrysenko VI, Tallant TC, Strom E, Gleiberman AS, Gupta D, et al. An agonist of toll-like receptor 5 has radioprotective activity in mouse and primate models. Science (2008) 320(5873):226–30. doi: 10.1126/science.1154986
159. Platt J, Sodi S, Kelley M, Rockwell S, Bermudes D, Low KB, et al. Antitumour effects of genetically engineered salmonella in combination with radiation. Eur J Cancer (2000) 36(18):2397–402. doi: 10.1016/s0959-8049(00)00336-1
160. Wai SN, Lindmark B, Soderblom T, Takade A, Westermark M, Oscarsson J, et al. Vesicle-mediated export and assembly of pore-forming oligomers of the enterobacterial clya cytotoxin. Cell (2003) 115(1):25–35. doi: 10.1016/s0092-8674(03)00754-2
161. Abdollahi H. Beneficial effects of cellular autofluorescence following ionization radiation: Hypothetical approaches for radiation protection and enhancing radiotherapy effectiveness. Med Hypotheses (2015) 84(3):194–8. doi: 10.1016/j.mehy.2014.12.021
162. Bettegowda C, Dang LH, Abrams R, Huso DL, Dillehay L, Cheong I, et al. Overcoming the hypoxic barrier to radiation therapy with anaerobic bacteria. Proc Natl Acad Sci U.S.A. (2003) 100(25):15083–8. doi: 10.1073/pnas.2036598100
163. Sacco JJ, Evans M, Harrington KJ, Man S, Powell N, Shaw RJ, et al. Systemic listeriosis following vaccination with the attenuated listeria monocytogenes therapeutic vaccine, Adxs11-001. Hum Vaccin Immunother (2016) 12(4):1085–6. doi: 10.1080/21645515.2015.1121338
164. Park SH, Zheng JH, Nguyen VH, Jiang SN, Kim DY, Szardenings M, et al. Rgd peptide cell-surface display enhances the targeting and therapeutic efficacy of attenuated salmonella-mediated cancer therapy. Theranostics (2016) 6(10):1672–82. doi: 10.7150/thno.16135
165. Beutler B, Rietschel ET. Innate immune sensing and its roots: The story of endotoxin. Nat Rev Immunol (2003) 3(2):169–76. doi: 10.1038/nri1004
166. Chorobik P, Czaplicki D, Ossysek K, Bereta J. Salmonella and cancer: From pathogens to therapeutics. Acta Biochim Pol (2013) 60(3):285–97.
167. Li XX, Yin JY, Tang J, Li YH, Yang QX, Xiao ZY, et al. Determining the balance between drug efficacy and safety by the network and biological system profile of its therapeutic target. Front Pharmacol (2018) 9:1245. doi: 10.3389/fphar.2018.01245
168. Chen Y, Liu X, Guo Y, Wang J, Zhang D, Mei Y, et al. Genetically engineered oncolytic bacteria as drug delivery systems for targeted cancer theranostics. Acta Biomater (2021) 124:72–87. doi: 10.1016/j.actbio.2021.02.006
169. Lou X, Chen Z, He Z, Sun M, Sun J. Bacteria-mediated synergistic cancer therapy: Small microbiome has a big hope. Nanomicro Lett (2021) 13(1):37. doi: 10.1007/s40820-020-00560-9
Keywords: bacteria-mediated cancer therapy, pathogen-associated molecular pattern (PAMP), drug delivery, cancer immunotherapy, bacterial cytotoxicity
Citation: Luo M, Chen X, Gao H, Yang F, Chen J and Qiao Y (2022) Bacteria-mediated cancer therapy: A versatile bio-sapper with translational potential. Front. Oncol. 12:980111. doi: 10.3389/fonc.2022.980111
Received: 28 June 2022; Accepted: 23 September 2022;
Published: 07 October 2022.
Edited by:
Giandomenico Roviello, University of Firenze, ItalyCopyright © 2022 Luo, Chen, Gao, Yang, Chen and Qiao. This is an open-access article distributed under the terms of the Creative Commons Attribution License (CC BY). The use, distribution or reproduction in other forums is permitted, provided the original author(s) and the copyright owner(s) are credited and that the original publication in this journal is cited, in accordance with accepted academic practice. No use, distribution or reproduction is permitted which does not comply with these terms.
*Correspondence: Yiting Qiao, eWl0aW5ncWlhb0B6anUuZWR1LmNu; Jianxiang Chen, Y2hlbmp4QGh6bnUuZWR1LmNu