- 1Department of Clinical Laboratory Diagnostics, Binzhou Medical University, Binzhou, China
- 2Department of Clinical Laboratory, Dazhou Women and Children’s Hospital, Dazhou, China
- 3Key Laboratory of RNA Biology, Center for Big Data Research in Health, Institute of Biophysics, Chinese Academy of Sciences, Beijing, China
- 4Biomedical Pioneering Innovation Center (BIOPIC), Beijing Advanced Innovation Center for Genomics, Peking-Tsinghua Center for Life Sciences, Peking University Genome Editing Research Center, State Key Laboratory of Protein and Plant Gene Research, School of Life Sciences, Peking University, Beijing, China
Since tyrosine phosphorylation is reversible and dynamic in vivo, the phosphorylation state of proteins is controlled by the opposing roles of protein tyrosine kinases (PTKs) and protein tyrosine phosphatase (PTPs), both of which perform critical roles in signal transduction. Of these, intracellular non-receptor PTPs (PTPNs), which belong to the largest class I cysteine PTP family, are essential for the regulation of a variety of biological processes, including but not limited to hematopoiesis, inflammatory response, immune system, and glucose homeostasis. Additionally, a substantial amount of PTPNs have been identified to hold crucial roles in tumorigenesis, progression, metastasis, and drug resistance, and inhibitors of PTPNs have promising applications due to striking efficacy in antitumor therapy. Hence, the aim of this review is to summarize the role played by PTPNs, including PTPN1/PTP1B, PTPN2/TC-PTP, PTPN3/PTP-H1, PTPN4/PTPMEG, PTPN6/SHP-1, PTPN9/PTPMEG2, PTPN11/SHP-2, PTPN12/PTP-PEST, PTPN13/PTPL1, PTPN14/PEZ, PTPN18/PTP-HSCF, PTPN22/LYP, and PTPN23/HD-PTP, in human cancer and immunotherapy and to comprehensively describe the molecular pathways in which they are implicated. Given the specific roles of PTPNs, identifying potential regulators of PTPNs is significant for understanding the mechanisms of antitumor therapy. Consequently, this work also provides a review on the role of non-coding RNAs (ncRNAs) in regulating PTPNs in tumorigenesis and progression, which may help us to find effective therapeutic agents for tumor therapy.
Introduction
Protein tyrosine phosphatases (PTPs) are a wide class of enzymes that oppose protein tyrosine kinases (PTKs) (1). PTPs can be categorized into four families based on the amino acid sequence of its catalytic structural domain, each with different substrate specificities (2). Of these, 17 intracellular non-receptor PTPs, belonging to the largest class I cysteine PTP family, are designated as PTPNs (2). Extensive evidence suggests that PTPNs are involved in a range of physiological and pathological processes, including but not limited to hematopoiesis, inflammatory response, immune system, cell proliferation and differentiation, and glucose homeostasis (3–6). Furthermore, PTPNs hold a critical role in tumor progression by dephosphorylating various substrate proteins to activate or inhibit oncogenic pathways (7, 8). More notably, several PTPNs are implicated in resistance to chemotherapy and radiotherapy, and numerous studies have demonstrated that targeting certain PTPNs can boost anti-tumor immunity and efficacy, which stimulates the immune system to attack tumors (9). Therefore, PTPNs are remarkably promising therapeutic targets to combat tumors.
Non-coding RNAs (ncRNAs) are a type of RNA transcript found in many eukaryotic genomes that function as a regulator of cellular processes, including chromatin remodeling, transcription, post-transcriptional modifications and signal transduction (10, 11). In recent years, ncRNAs have been linked to the development and progression of cancer, particularly microRNAs (miRNAs), long-stranded non-coding RNAs (lncRNAs), and circular RNAs (circRNAs) (11, 12). Of these, miRNAs are defined as short ncRNAs of approximately 22 nt, lncRNAs are ncRNAs with transcripts longer than 200 nt, and circRNAs are closed continuous loop structures lacking a terminal 5’ cap and a 3’ polyadenylated tail (11). Interestingly, lncRNAs and circRNAs can perform as miRNA sponges, binding to miRNAs and altering their function. Here, ncRNAs act as tumor promoters and suppressors, depending on targeting PTPNs.
In this review, we elaborate on the roles played by PTPN family members and provide a comprehensive summary of the molecular pathways in which PTPNs are involved in various human cancers. Subsequently, the essential position occupied by PTPNs in the immune system and cancer immunotherapy is further described. Furthermore, we characterize how ncRNAs modulate PTPNs in tumorigenesis and hypothesize that ncRNA regulation in combination with immunotherapy may lead to more precise and effective efficacy.
The physiological role of PTPNs
PTPNs, belonging to the PTP family, share the common property of possessing phosphatase activity that dephosphorylates a series of proteins, thereby governing cellular signal transduction and biological processes. Several studies have shown that PTPN2 and PTPN6 are highly expressed in hematopoietic cells and act as negative signaling regulators (13, 14). For instance, PTPN2 dephosphorylates and inactivates signal transducer and activator of transcription (STAT) protein, which is required to maintain cellular homeostasis in the hematopoietic system (15). Furthermore, PTPN2 also holds an essential role in glucose homeostasis. For example, PTPN2 negatively regulates the insulin receptor (INSR) signaling pathway through dephosphorylation of INSR and controls gluconeogenesis and hepatic glucose production through negative regulation of the interleukin-6 (IL-6) signaling pathway (16, 17). PTPN14 is required for the regulation of lymphangiogenesis (18). In addition, PTPNs perform crucial roles in the regulation of immune cell development and inflammatory responses, which will be described in later sections. Overall, the PTPN family members act as a “brake” and are essential for the maintenance of homeostasis in the body.
Role of PTPNs in the context of cancer
Members of the PTPN family hold crucial roles in cancer genesis, progression, metastasis, and drug resistance by dephosphorylating a variety of substrate proteins to execute oncogenic or tumor suppressive functions in various cancers (Figure 1).
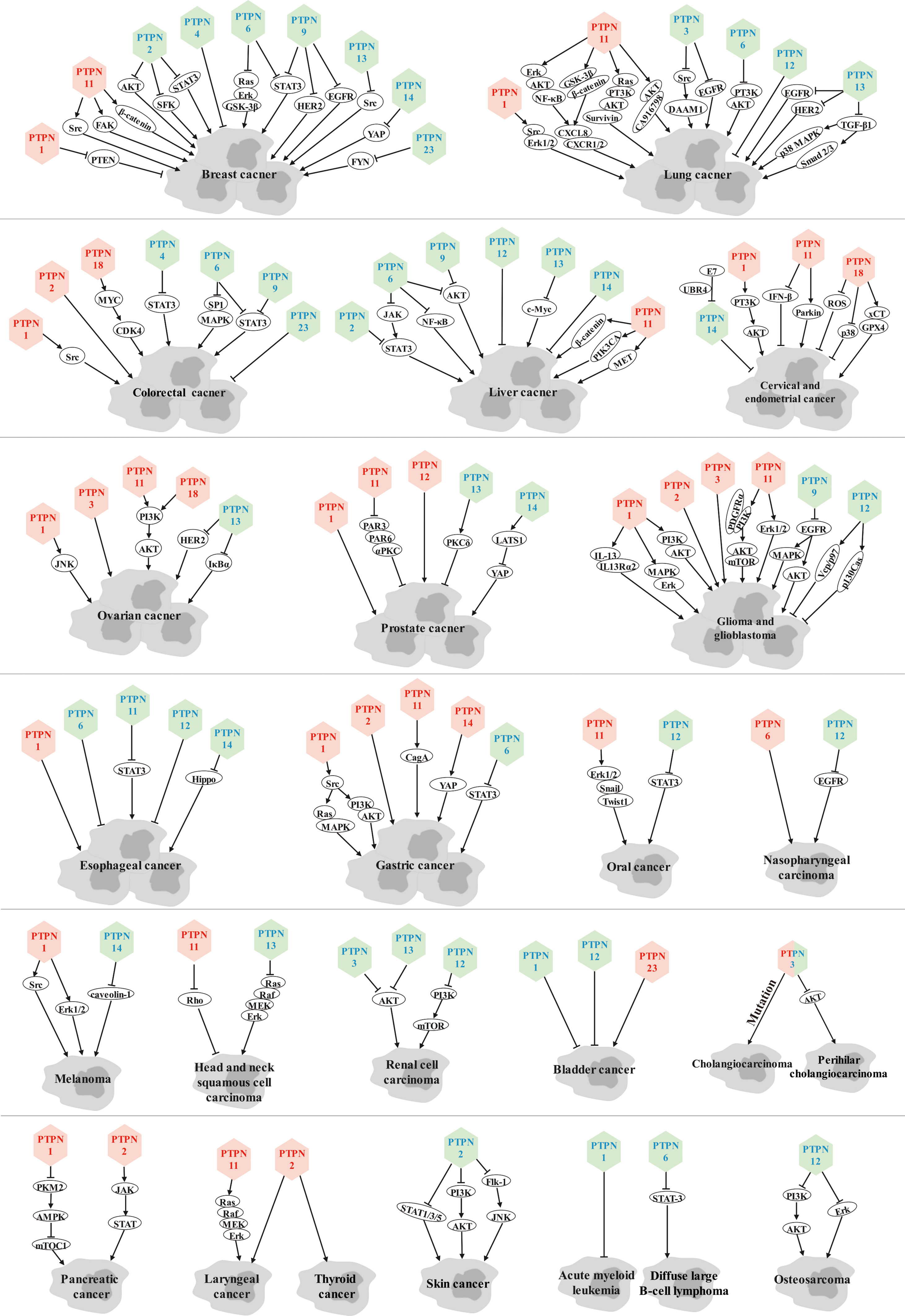
Figure 1 Role of PTPN family members in various cancers. Red font represents tumor promoters and blue font represents tumor suppressors.
Breast cancer
PTPN family members have been extensively investigated in breast cancer, with PTPN1 and PTPN11 driving the progression of breast cancer. PTPN1, which is required for invadopodia formation (19), promotes invasiveness of breast cancer cells by negatively regulating PTEN and facilitates human epidermal growth factor receptor 2 (HER2)-induced breast tumorigenesis with lung metastasis (20, 21). PTPN11 is essential for HER2, IL-6, and platelet-derived growth factor-B (PDGF-B)-induced tumorigenesis and epithelial-to-mesenchymal transition (EMT) (22–24). Mechanistically, PTPN11 enhances the oncogenic activity of β-catenin and activates Src family kinases, as well as regulates focal adhesion kinase (FAK) to promote epidermal growth factor (EGF)-induced lamellipodia persistence and migration of triple-negative breast cancer (TNBC) cells (25–27).
However, most PTPN family members function as tumor suppressors in breast cancer, including PTPN2, PTPN4, PTPN6, PTPN9, PTPN13, PTPN14, and PTPN23. Specifically, PTPN2 is implicated in the subtype specificity of breast cancer, and low expression in patients with Luminal A and HER2-positive tumors is linked to a higher recurrence rate, but not in patients with triple-negative tumors (28). More importantly, loss of PTPN2 is coupled with activation of oncogenic signaling pathways, such as protein kinase B (AKT), Src family kinase (SFK) and STAT3 signaling pathways, and also with resistance to tamoxifen (29, 30). PTPN6 dampens the oncogenic characteristics of breast cancer by dephosphorylating STAT3 and inactivating the Ras/extracellular signal-regulated kinase (Erk)/glycogen synthase kinase-3beta (GSK-3β) signaling pathway (31, 32). PTPN9 suppresses the growth and invasion of breast cancer cells by negatively regulating HER2 and epidermal growth factor receptor (EGFR) and suppressing STAT3 activation (33, 34). Furthermore, PTPN4 and PTPN13 are favorable prognostic biomarkers for breast cancer patients (35, 36), in which PTPN13 induces apoptosis of breast cancer cells and inhibits breast tumor aggressiveness by directly inactivating Src kinase and stabilizing intercellular adhesion and promoting desmosome formation (37–39). PTPN14 negatively regulates the oncogenic function of yes-associated protein (YAP) by modulating the subcellular localization of YAP and suppressing its transcriptional co-activator activity (40), and also inhibits breast cancer metastasis by altering protein transport (41). PTPN23 is identified as a suppressor of cell motility and invasion in breast cancer cells by inhibiting FYN kinase (42).
Lung cancer
As with breast cancer, PTPN1 and PTPN11 also serve as oncogenic factors in lung cancer. PTPN1 contributes to the proliferation and metastasis of non-small cell lung cancer (NSCLC) cells by enhancing the Erk1/2 signaling pathway and diminishing the expression of p-Src (Tyr527), which activates Src (43). Of note, PTPN11 is not only essential for lung cancer cell growth, but also confers chemotherapy resistance (44). Mechanistically, overexpression of PTPN11 increases resistance to tyrosine kinase inhibitors (TKI) in either EGFR mutant or EGFR wild-type NSCLC cells through Erk-AKT- nuclear factor kappa B (NF-κB) and GSK3β-β-Catenin signaling pathway-mediated C-X-C motif chemokine ligand 8 (CXCL8)-chemokine receptor 1/2 (CXCR1/2) feedback loop that promotes stemness and tumorigenesis (45, 46). Moreover, PTPN11 confers cisplatin resistance to lung cancer cells through activation of the AKT-CA916798 pathway and the Ras/phosphoinositide 3-kinase (PI3K)/AKT1/survivin pathway, respectively (47, 48). Consequently, PTPN11 inhibitors in combination with chemotherapy may be a promising therapy strategy for patients with lung cancer.
Furthermore, PTPN3, PTPN6, PTPN12 and PTPN13 play tumor suppressor roles in lung cancer. PTPN3 suppresses lung cancer cell proliferation and migration by counteracting Src-mediated disheveled-associated activator of morphogenesis 1 (DAAM1) activation and actin polymerization and by promoting EGFR endocytic degradation (49, 50). Similarly, PTPN6 inactivates PI3K/AKT signaling pathway (51). PTPN12 is a valuable prognostic biomarker for patients with NSCLC (52). PTPN13 negatively regulates the growth and migration of lung cancer cells in vitro and inhibits tumorigenicity in vivo by controlling tyrosine phosphorylation of EGFR and HER2 and by inhibiting transforming growth factor beta1 (TGF-β1)-induced activation of p38 mitogen-activated protein kinase (MAPK) and Smad 2/3 pathways, respectively (53, 54).
Colorectal cancer
In colorectal cancer, PTPN1, PTPN2 and PTPN18 undoubtedly drive its progression. Among them, PTPN1 expression is related to poor prognosis in colorectal cancer patients through dephosphorylation of the Tyr530 site of Src, which activates the Src signaling pathway and enhances the oncogenicity of colon cancer (55, 56). Similarly, high expression of PTPN2 is implicated in the incidence of colorectal cancer (57), and specific deletion of PTPN2 in bone marrow cells and macrophages prevents the development of colorectal cancer, although it promotes inflammation in the intestine (58). PTPN18 activates myelocytomatosis oncogene (MYC) signaling pathway and further potentiates cyclin-dependent kinase 4 (CDK4) expression to promote colorectal cancer progression (59).
PTPN4, PTPN6 and PTPN9, as tumor suppressors, suppress the progression of colorectal cancer by dephosphorylating pSTAT3 at the Tyr705 residue and restraining the transcriptional activity of STAT3 (60–62). Moreover, PTPN6 also facilitates chemosensitivity of colorectal cancer cells by inhibiting specificity protein 1 (SP1)/MAPK signaling pathway (63). Additionally, PTPN23 also suppresses the proliferation and EMT of human intestinal cancer cells (64).
Liver cancer
The vast majority of the reported PTPN family members hold an inhibitory role in hepatocellular carcinoma (HCC), with the exception of PTPN11, which drives HCC progression by potentiating oncogenic proteins such as β-Catenin, PIK3CA and MET, and is associated with chemoresistance in HCC patients (65, 66).
PTPN2 can prevent hepatocyte progression to HCC by inactivating STAT3 signaling and suppressing T-cell recruitment in obese C57BL/6 mice (67). Consistently, PTPN6 overexpression inhibits the proliferation, migration, invasion and tumorigenicity of HCC cells by suppressing multiple oncogenic pathways, including janus kinase (JAK)/STAT, NF-κB and AKT signaling pathways (68). Likewise, PTPN9 contributes to the inhibition of HCC growth and metastasis by repressing the AKT pathway (69). In addition, PTPN12 and PTPN13 are associated with a favorable prognosis in HCC patients. Mechanistically, PTPN13 suppresses HCC progression by directly and competitively binding insulin-like growth factor 2 mRNA-binding protein 1 (IGF2BP1) to diminish the intracellular concentration of functional IGF2BP1, thereby promoting c-Myc mRNA degradation (70). Furthermore, PTPN14 significantly represses the proliferation, migration, and invasion of HCC cells in vitro and tumor growth and metastasis in vivo (71).
Cervical and endometrial cancer
Currently, most PTPN family members perform oncogenic roles in cervical and endometrial cancers, with the exception of PTPN14. In detail, PTPN1 expression is linked to poor prognosis in cervical cancer possibly through activation of the PI3K/AKT pathway (72). Importantly, PTPN11 contributes to the growth and migration of cervical cancer cells and decreases the sensitivity of cells to cisplatin (73). Specifically, PTPN11 facilitates cervical cancer cell proliferation by suppressing interferon-β (IFN-β) production and restricts chemotherapeutic drug-induced apoptosis of cervical cancer cells through Parkin-dependent autophagy (74, 75). Likewise, PTPN18 promotes proliferation and metastasis and restrains apoptosis in endometrial cancer (76). Mechanistically, silencing of PTPN18 induced ferroptosis in endometrial cancer cells by increasing intracellular reactive oxygen species (ROS) levels and p-p38 expression as well as decreasing the expression of glutathione peroxidase 4 (GPX4) and system xc(-) cystine/glutamate antiporter (xCT) (72).
What’s more, the deletion of PTPN14 contributes to human papillomavirus (HPV)-mediated cervical carcinogenesis, while the major transforming activity of high-risk HPV is linked to the E7 oncoprotein. Mechanistically, the crystal structure of the terminal structural domain of E7 C binds to the catalytic structural domain of PTPN14 and induces proteasome-mediated degradation of PTPN14 via the ubiquitin ligase UBR4 (77, 78), thereby restricting keratin-forming cell differentiation and contributing to HPV-mediated tumorigenesis (79).
Ovarian cancer
PTPN family members almost contribute to the progression of ovarian cancer, except for PTPN13. PTPN1 and PTPN6 are highly expressed in ovarian cancer cell lines (80, 81), in which PTPN1 accelerates ovarian cancer progression in a c-Jun N-terminal kinase (JNK)-dependent mechanism (80). Strikingly, PTPN3 confers chemoresistance and tumor stem cell-like characteristics to ovarian cancer cells (82). Furthermore, PTPN11 and PTPN18 potentiate ovarian cancer invasion and metastasis through activation of PI3K/AKT axis (83, 84).
However, high PTPN13 expression in patients with high-grade plasma ovarian cancer is related to better prognosis (85). Mechanistically, PTPN13 dephosphorylates the signaling domain of HER2 and the phosphorylation of tyrosine 42 on IκBα (IκBα-pY42), respectively, thereby attenuating the invasiveness and metastasis of ovarian cancer (86, 87).
Prostate cancer
In prostate cancer, PTPN1 and PTPN12 are linked to poor prognosis in patients (88, 89). Importantly, PTPN11 promotes prostate cancer metastasis by attenuating the PAR3/PAR6/atypical protein kinase C (aPKC) polarity protein complex, resulting in disruption of cell polarity, dysregulation of cell-cell junctions, and increased EMT (90).
In contrast, PTPN13 and PTPN14 function as tumor suppressors in prostate cancer. Specifically, PTPN13 suppresses the proliferation and migration of prostate cancer cells and stimulates apoptosis mediated by PKCδ (91). Furthermore, PTPN14 restrains cell proliferation and invasion by enhancing phosphorylation of YAP through activation of large tumor suppressor 1 (LATS1), an effect that leads to a significant decrease in YAP-mediated transcriptional activity (92).
Glioma and glioblastoma
PTPN1 and PTPN2 can be used as predictors of poor prognosis in glioma patients (93, 94). Mechanistically, PTPN1 promotes glioma progression through activation of MAPK/Erk and PI3K/AKT pathways as well as IL-13-mediated adhesion, migration and invasion of IL13Rα2-expressing cancer cells (93, 95). Up-regulation of PTPN2 expression induced by inflammatory response and oxidative stress contributes to glioma progression (96). Furthermore, PTPN11 regulates proliferation and tumorigenicity of glioma stem cells (97).
Likewise, PTPN2 and PTPN3 are correlated with poor patient prognosis in glioblastoma (GBM) (94, 98). In Ink4a/Arf-deficient glioblastomas, PTPN11 regulates the interaction of PI3K with PDGFRα and activates the downstream AKT/mTOR pathway, ultimately promoting tumorigenesis (99). In addition, the multivariate signaling regulatory function of PTPN11 holds a crucial role in GBM cellular decision-making. PTPN11-driven Erk1/2 activity is dominant in driving cellular proliferation and PTPN11-mediated antagonism of STAT3 phosphorylation prevails in the promotion of GBM cell death in response to EGFR and c-MET co-inhibition (100).
But for PTPN9, which appears to be a tumor suppressor, leads to decreased glioma cell viability by reducing the phosphorylation of EGFR and cooperating with BRAF (V600E) inhibitors to restrain MAPK and AKT signaling (101). Furthermore, PTPN12 controls GBM cell growth and invasion by interacting with the ATP-dependent ubiquitin segregase valosin-containing protein (Vcp)/p97 and regulating phosphorylation and stability of the focal adhesion protein p130Cas (Crk-associated substrate) (102).
Esophageal cancer
Interestingly, PTPN1 expression is implicated in the incidence of esophageal cancer (57), while PTPN6 is down-regulated in esophageal cancer and PTPN12 is a favorable prognostic biomarker for patients with esophageal squamous cell carcinoma (103, 104). What’s more, PTPN11 and PTPN14 suppress malignant progression and chemoresistance in esophageal cancer through dephosphorylation of STAT3 and negative regulation of the Hippo signaling pathway, respectively (105, 106).
Gastric cancer
PTPN1 significantly promotes gastric cancer (GC) cell proliferation in vitro and tumor growth in vivo by regulating Src-related signaling pathways, such as the Src/Ras/MAPK and Src/PI3K/AKT pathways (107–109). Furthermore, PTPN1 is implicated in the poor prognosis of gastric cancer, and PTPN2 is linked to the incidence of gastric cancer (57, 109). It is well known that Helicobacter pylori is a high risk factor for gastric cancer. Jing Jiang et al. reveals that PTPN11 expression is elevated in gastric cancer with H. pylori infection (110). In the early stages of gastric carcinogenesis, CagA from H. pylori translocates into gastric epithelial cells, undergoes tyrosine phosphorylation, and binds to PTPN11 in the human gastric mucosa in vivo to form a complex which is thought to contribute to the development of gastric cancer (111). SHIP2, similar to PTPN11, also binds to CagA in a tyrosine phosphorylation-dependent manner and increases CagA delivery into gastric epithelial cells (112). Of note, PTPN14 enhances the proliferation and migration of GC cells by promoting YAP phosphorylation in the Hippo signaling pathway (113). On the contrary, PTPN6 attenuates the invasion and migration of GC cells by dephosphorylating STAT3 (114).
Other cancers
In oral cancer, PTPN11 is significantly up-regulated and promotes cell invasion and metastasis through the Erk1/2-Snail/Twist1 pathway (115). In contrast, PTPN12 suppresses oral cancer cell proliferation and invasion through induction of STAT3 dephosphorylation (116). In nasopharyngeal carcinoma (NPC), PTPN6 potentiates radioresistance and restrains cellular senescence (117). However, PTPN12, a favorable prognostic biomarker for NPC patients, suppresses the proliferation and migration of NPC cells through negative regulation of EGFR (118). In melanoma, PTPN1 promotes melanoma progression by activating the Src signaling pathway through dephosphorylating the Tyr530 site of Src as well as by enhancing the Erk1/2 signaling pathway, respectively (119, 120). Moreover, PTPN14 blocks caveolin-1-induced cancer cell metastasis by decreasing phosphorylation at the Tyr14 site of caveolin-1 (121). In the head and neck squamous cell carcinoma, PTPN11 promotes invadopodia formation through suppression of Rho signaling, leading to cancer metastasis (122). Yet, PTPN13 controls the progression of spontaneous or HPV-induced squamous cell carcinoma by inhibiting Ras/Raf/MEK/Erk signaling (123). In clear cell renal cell carcinoma, PTPN3 and PTPN13 act as tumor suppressors by inactivating the AKT signaling pathway (124, 125), whereas PTPN12 restrains the proliferation of renal cell carcinoma by inhibiting PI3K/mechanistic target of rapamycin (mTOR) pathway activity (126). In bladder cancer, PTPN1 and PTPN12 function as tumor suppressors to attenuate the growth, invasion and migration of cancer cells (127, 128). However, PTPN23 can regulate the motility of bladder cancer cells (129). In cholangiocarcinoma more than 40% of PTPN3 somatic mutations, activation of PTPN3 mutations promotes cancer cell proliferation and migration and is linked to cancer recurrence (130). Strikingly, PTPN3 suppresses proliferation through inhibition of AKT phosphorylation and is correlated with a favorable prognosis in patients with perihilar cholangiocarcinoma (131). In pancreatic cancer, PTPN1 and PTPN2 are highly expressed and associated with poor survival. Specifically, PTPN1 directly decreases pyruvate kinase M2 (PKM2) Tyr105 phosphorylation, which further leads to AMP-activated protein kinase (AMPK) inactivation, thereby increasing mTOC1 activity. PTPN2 activates the JAK-STAT signaling pathway to promote cancer progression (132, 133). Furthermore, inflammatory response and oxidative stress induce up-regulation of PTPN2, which accelerates the progression of laryngeal and thyroid cancers (134, 135). PTPN11 also promotes laryngeal cancer growth through the Ras/Raf/Mek/Erk pathway and serves as a prognostic indicator for laryngeal cancer (136). Several studies have shown that PTPN2, which exerts tumor suppressive effects in skin carcinogenesis, suppresses proliferation and induces apoptosis by negatively regulating multiple oncogenic signaling pathways, including STAT1, STAT3, STAT5, PI3K/AKT, and fetal liver kinase 1 (Flk-1)/JNK signaling pathways (137–139). In hematologic tumors, specific deficiency of PTPN1 in mouse bone marrow accelerates the development of acute myeloid leukemia (140), and PTPN6 inhibits the progression of diffuse large B-cell lymphoma by dephosphorylating STAT3 (141). Finally, PTPN12 inhibits tumor progression in osteosarcoma cells probably by inactivating PI3K/AKT and Erk pathways (142).
In summary, we have comprehensively described the role of PTPN family members in human cancers and observed that various PTPN family members are implicated in almost all oncogenic phenotypes, such as tumor proliferation, metastasis, and drug resistance, through unique molecular pathways. Interestingly, the majority of PTPN family members perform oncogenic or tumor suppressive functions depending on the tumor in which they are located. Nevertheless, a small number of PTPN family members exert more specific functions, for instance, PTPN11 as a tumor promoter whereas PTPN13 as a tumor suppressor in almost all cancers.
Dual role of PTPNs in specific cancers
Strikingly, we observed that a portion of the PTPN family members have dual roles in the same cancer. For instance, PTPN1 in hepatocellular carcinoma, PTPN11 in colon cancer and PTPN12 in breast cancer can be both tumor promoters and tumor suppressors based on different molecular pathways (Figure 2). Elucidating the dual roles of certain PTPN may lead to better understanding of its exact functions in tumorigenesis.
Dual-sidedness of PTPN1 in hepatocellular carcinoma
Leukocyte-derived chemotoxin 2 (LECT2), a tumor suppressor in HCC, contributes to blocking vascular invasion and metastasis in HCC by recruiting PTPN1 to antagonize MET receptor activation (143). However, Wei-Tien Tai et al. and Fang Yuan et al. proposed that PTPN1 exerts a carcinogenic role in HCC. Specifically, Pituitary homeobox 1 (PITX1) exerts a tumor suppressive effect in hepatocarcinogenesis through regulation of Ras guanosine triphosphatase-activating protein (p120RasGAP) expression levels, but PTPN1 attenuates the protein stability of PITX by directly dephosphorylating PITX1 at residues Y160, Y175 and Y179 (144). Furthermore, down-regulation of PTPN1 expression inhibits HCC progression possibly by inactivating the PI3K/AKT pathway and activating the AMPK pathway (145).
Two faces of PTPN11 in colon cancer
Controversially, Wang Y et al. (146) and Yu M et al. (147) demonstrated that PTPN11 promotes vascular growth and proliferation of colon cancer cells as well as resistance to oxaliplatin through AKT and Erk signaling pathways. However, Yuan H et al. (148) and Wei B et al. (149) argued that PTPN11 possesses anticancer activity in colon cancer. Mechanistically, the anticancer effects of PTPN11 are achieved by interacting with CD81 and inhibiting its expression and by inhibiting DNA repair and enhancing stimulator of interferon genes (STING) pathway-mediated antitumor immunity, respectively.
Dual role of PTPN12 in breast cancer
In addition, several studies have shown that PTPN12 is linked to a favorable prognosis in TNBC patients by suppressing multiple oncogenic tyrosine kinases, including HER2 and EGFR, thereby dampening breast cancer cell survival, migration and EMT (150–152). However, Harris IS et al. noted that PTPN12 is highly expressed in TNBC and promotes resistance to oxidative stress and supports tumorigenesis by regulating forkhead box O (FOXO) signaling (153).
Regarding the controversy of the three PTPN members mentioned above in specific cancers, we have speculated the following three reasons. First, there may be some differences in various experimental settings and tumor cell lines. Second, there are also differences in molecular mutation profiles in tumor cell lines. If mutations occur in the regulator of PTPN, it might lead to a change in the function of PTPN so that the exact opposite function appears in the same cancer. Furthermore, mutations in PTPN itself can result in opposite functions, as previously described, where PTPN3 inhibits the progression of cholangiocarcinoma by suppressing the AKT signaling pathway, whereas mutated PTPN3 promotes the oncogenic properties of cholangiocarcinoma (130, 131). Likewise, mutations occurring in PTPN11 significantly potentiate its function, producing oncogenes that facilitate the proliferation of leukemic cells (154). Third, PTPNs possess phosphatase active structural domains that regulate the dephosphorylation of many substrate proteins, including tumor promoters or suppressors, so focusing on only one molecular pathway may introduce bias for the overall role of PTPNs in a particular cancer.
Therefore, the study of PTPNs in combination with cell lines in vitro and mouse genetic studies in vivo, by knocking out specific regulators and effectors, will contribute to a better understanding of the specific signaling pathways regulated by PTPNs.
PTPNs are crucial targets for regulating immune cells development and cancer immunotherapy
Given that PTPNs occupy important roles in human tumors, in addition to achieving pro- or anti-tumor effects by modulating multiple oncogenic pathways, perhaps they also reshape the tumor microenvironment by inducing tumor cells to secrete various cytokines and chemokines. It is well known that immune cells are an essential component of the tumor microenvironment (TME) (155). There is growing evidence that some PTPN family members can negatively regulate the development and differentiation of immune cells to achieve an anti-inflammatory response and prevent the onset of autoimmune diseases, but on the other hand provide an opportunity for tumors to evade surveillance by the immune system. Since the immunosuppressive tumor microenvironment creates the appropriate conditions for tumor proliferation and metastasis, an accumulating number of studies indicate that targeting PTPNs could activate the body’s immune system, thereby enhancing the efficacy of anti-tumor immunity (Figure 3).
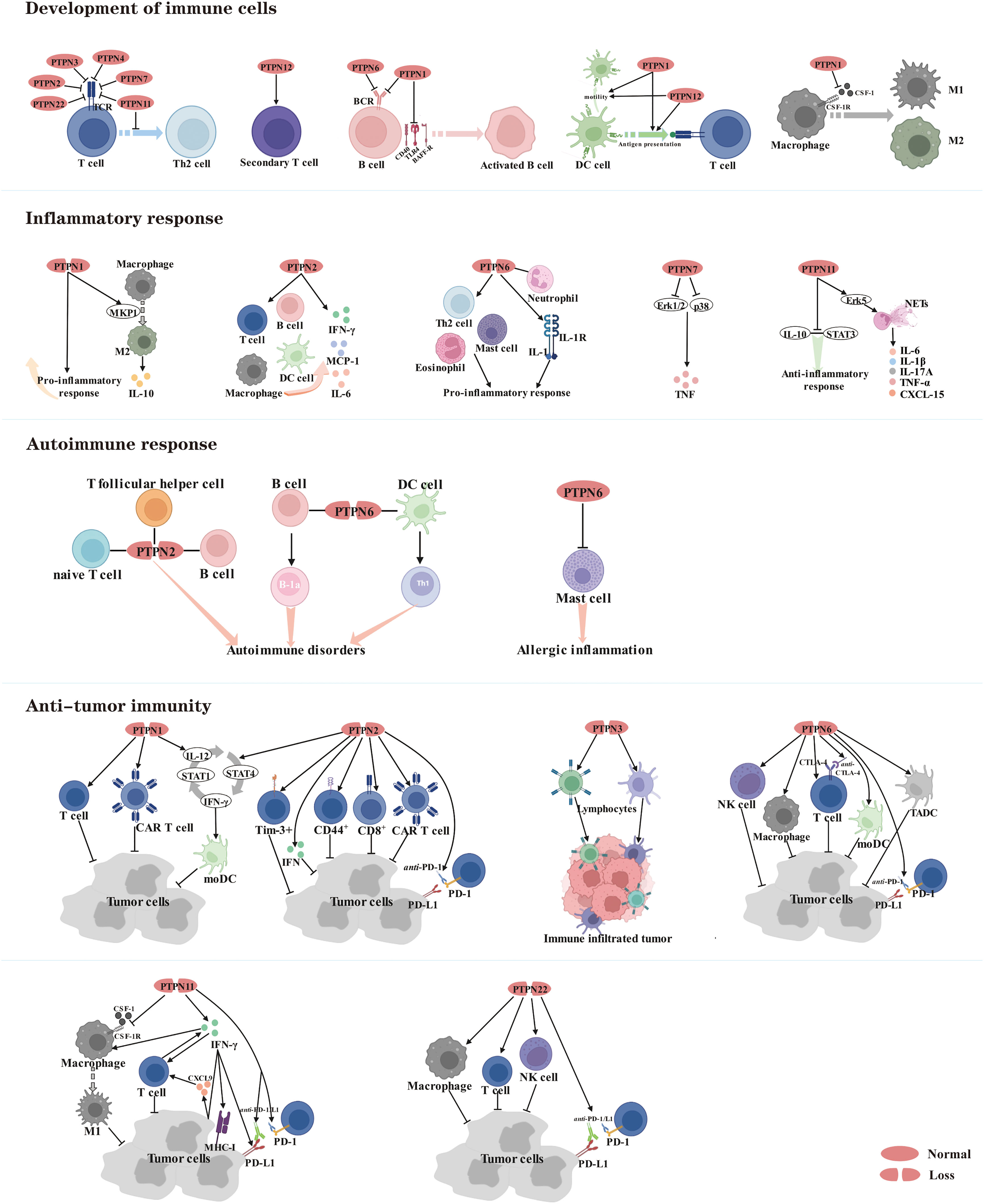
Figure 3 Members of the PTPN family are involved in regulating the development of immune cells and serve as therapeutic targets for inflammatory diseases, autoimmune diseases and cancer.
PTPNs mediate the physiological functions of immune cells
During development and maturation, various inhibitory receptors linked with phosphatases are expressed by subsets of T cells. Phosphatases, in turn, dephosphorylate key players in receptor signaling pathways. Several studies have shown that PTPN family members are crucial for suppressing T cell activation, with PTPN2, PTPN3, PTPN4, PTPN7, PTPN11 and PTPN22 negatively regulating T-cell receptor (TCR) signaling (156–163). Specifically, PTPN2 dephosphorylates and inactivates Src family kinases to regulate T cell responses (162). PTPN22 functions in a synergistic manner when forming a complex with C-terminal Src kinase (CSK), and dissociation of this complex is necessary to recruit PTPN22 to the plasma membrane, where it down-regulates TCR signaling and inhibits T cell activation (164). However, end-binding protein 1 (EB1) specifically binds to the P1 structural domain of PTPN22 by competing with CSK, which contributes to the regulation of TCR signaling (165). Another essential purpose of PTPN11 is to prevent T cells from differentiating into the T-helper 2 (Th2) phenotype (166). However, phosphatase activities of PTPN3 and PTPN4 are now dispensable for T cell development and T cell effector function (156, 157, 167). PTPN12, although not required for T cell development or primary responses, promotes secondary T cell responses by dephosphorylating the protein tyrosine kinase Pyk2 (168).
Likewise, PTPN1 and PTPN6 are also negative regulators of B-cell receptor (BCR) signaling and hold a vital role in modulating B cell activation and immunological tolerance (169, 170). Mechanistically, PTPN1 restricts B cell activation via negatively regulating CD40, B cell activating factor receptor (BAFF-R), and toll-like Receptor 4 (TLR4) signaling in B cells (169).
In Dendritic cells (DCs), ablation of PTPN1 and PTPN12 impairs motility in vivo and prevents efficient antigen presentation to T cells (171, 172). These results indicate that PTPN1 and PTPN12 hold a significant regulatory role in modulating a central DC function of initiating adaptive immune responses in response to innate immune cell activation.
Pro-inflammatory macrophages M1 and tolerance-inducible macrophages M2 are the two major subpopulations of macrophages, the former mediating host defense and the latter undertaking homeostatic and tissue regeneration functions (173). PTPN1 negatively regulates macrophage development through macrophage-colony stimulating factor 1 (CSF-1) signaling. Mechanistically, PTPN1 deficiency tends to make cells more sensitive to CSF-1, resulting in increased phosphorylation of the CSF-1 receptor (CSF-1R) in bone marrow-derived macrophages and increased inflammatory activity, implying that PTPN1 is a critical regulator of bone marrow differentiation and macrophage activation in vivo (174, 175).
PTPNs perform pivotal roles in the regulation of inflammatory and autoimmune responses
Currently, several studies place PTPN family members as a double-edged sword in the regulation of inflammatory responses. PTPN1 deletion displays enhanced inflammatory activity in vitro and in vivo through constitutive overexpression of activation markers as well as greater sensitivity to endotoxins (174). However, PTPN1 deletion increases Mitogen-activated protein kinase phosphatase-1 (MKP1) expression in mouse macrophages, facilitating M2 macrophage polarization, which promotes the production of anti-inflammatory cytokine (IL-10) according to another study (176).
PTPN2 is a negative regulator of cytokine signaling, and its loss-of-function carriers have increased susceptibility to the development of inflammatory diseases (173). For instance, PTPN2-/- mice develop progressive systemic inflammatory diseases with dermatitis, liver inflammation, chronic myocarditis, gastritis, nephritis, and salpingitis, as well as elevated serum interferon-γ (IFN-γ) (177). Mechanistically, PTPN2 deficiency promotes increased infiltration of B and T lymphocytes, macrophages and DCs (178, 179), and up-regulated IFN-γ induces STAT signaling and secretion of IL-6 and monocyte chemoattractant protein-1 (MCP-1) (180).
PTPN6 is a key regulatory protein in the control of inflammatory cell signaling, and its knockdown not only increases systemic inflammation in mice, but more importantly, is also implicated in human inflammatory diseases (181). For instance, Th2 cell production and mast cell-specific cytokine production are potentiated in Motheaten mice with a natural mutation in PTPN6. In an OVA-induced model of allergic airway inflammation, eosinophil inflammation, mucus hypersecretion, and airway hyperresponsiveness are enhanced in Motheaten mice, all of which contribute to the development of allergic disease (182). Furthermore, conditional deletion of PTPN6 in neutrophils is sufficient to initiate IL-1 receptor-dependent inflammatory skin diseases. Mechanistically, PTPN6 prevents caspase-8- and Ripk3-Mlkl-dependent cell death and concomitant IL-1α/β release (183).
PTPN7 exerts an anti-inflammatory function by negatively regulating Erk1/2 and p38, which enhance pro-inflammatory tumor necrosis factor (TNF)-production (184).
Intriguingly, PTPN11 holds many functions in distinct cells. PTPN11 protects mice from intestinal inflammation in epithelial cells (185), while promotes colitis and colitis-driven colon cancer in macrophages (186). Mechanistically, PTPN11 impairs IL-10-STAT3 signaling and its dependent anti-inflammatory response in human and mouse macrophages (186). Additionally, PTPN11 promotes the formation of neutrophil extracellular traps (NETs) through the Erk5 pathway, leading to pro-inflammatory cytokines such as TNF-α, IL-1β, IL-6, IL-17A, and CXCL-15, which exacerbate the inflammatory response (187).
Currently, despite extensive research on the mechanisms underlying autoimmune diseases, the fundamental causes remain unknown. Here, the main focus is on the regulatory mechanisms by which PTPN2 and PTPN6 can be involved in autoimmunity. Autoimmunity is characterized by a significant increase associated with antinuclear antibodies, inflammatory cytokines and immunoglobulins. Autoimmunity is exacerbated by PTPN2 deficits in numerous immune lineages, including naive T cells, T follicular helper cells (Tfh), and B cells (188). PTPN6 deficiency in B cells and DC cells promotes B-1a cell development and Th1 cell differentiation, respectively, leading to autoimmune disorders (189, 190). In addition, PTPN6 acts as a key negative regulator in allergic inflammation and allergen-induced anaphylaxis by regulating the function of mast cells (191).
PTPNs are emerging targets for cancer immunotherapy
In recent years, significant progress has been made in the immunotherapy of cancer. With the intensive exploration of immune checkpoints, several PTPN family members have been revealed to possess essential roles in anti-tumor immunity and are promising therapeutic targets.
PTPN1 is elevated in intra-tumor T cells, and knocking it out promotes T cell antitumor activity and chimeric antigen receptor (CAR) T cell efficacy against solid tumors (192). Furthermore, deletion of PTPN1 and PTPN2 in DCs stimulated the growth of IL-12 and IFN-γ, which amplified the IL-12/STAT4/IFN-γ/STAT1/IL-12 positive autocrine loop, boosting the therapeutic potential of mature monocyte-derived dendritic cells (moDCs) in tumor-bearing mice (193).
In several studies, PTPN2 has been proven to be a negative regulator of interferon signaling (194, 195). Lack of PTPN2 in tumor cells enhances immunotherapy efficacy through augmenting interferon-mediated antigen presentation and growth inhibition (196). What’s more, PTPN2 deficiency in T cells boosts the generation of Tim-3+ cells, CD44+ effector/memory T cells, and CD8+ T cell infiltration and cytotoxicity in tumors, as well as the efficacy of anti- programmed cell death protein 1 (PD-1) and CAR T cells in solid tumors by promoting activation of Src family kinase LCK and cytokine-induced STAT-5 signaling (195, 197–199), which can actually facilitate tumor control and improve immunotherapy potency.
Furthermore, inhibition of PTPN3 in lymphocytes expands the proportion of tumor-infiltrating lymphocytes and activated lymphocyte cytotoxicity, as well as the anticancer effect on small cell lung cancer (SCLC) and large cell neuroendocrine carcinoma (LCNEC) (200, 201).
PTPN6 shows a negative regulatory effect on the activation of T cells, natural killer (NK) cells and macrophages. However, deletion of PTPN6 significantly strengthens the capacity of these immune cells for tumor killing and promotes anti-tumor immunity (202–204). Of note, a considerable and durable T cell-mediated suppression of tumor growth was observed when PTPN6 knockdown of OT-I T cells was combined with anti-PD-1 and cytotoxic T-lymphocyte-associated protein 4 (CTLA-4) immunotherapy (205). And the ability of tumor-associated DCs (TADCs) and MoDCs to take up and process immune complexes (IC) containing tumor antigens bound to antitumor antibody, ultimately inducing anti-tumor immunity in vivo, was augmented by simultaneous inhibition of PTPN6 and phosphatases regulating AKT activation (206).
PTPN11, involved in the regulation of tumor and immune cell signaling, is a critical modulator of PD-1 and B and T lymphocyte attenuator (BTLA) immune checkpoint pathways and promising drug target in tumor immunotherapy (207). Inhibition of PTPN11 activity enhances tumor-intrinsic IFN-γ signaling, resulting in increased chemoattractant cytokine release and cytotoxic T cell recruitment, as well as increased expression of major histocompatibility complex (MHC) class I and programmed cell death ligand 1 (PD-L1) on the surface of cancer cells, along with decreased differentiation and suppression of immunosuppressive myeloid cells in the tumor microenvironment (208, 209). In highly aggressive mouse models of breast cancer and melanoma, simultaneous suppression of CSF-1R and PTPN11 to activate macrophages and promote phagocytosis may be an effective strategy for macrophage-based immunotherapy (210). Mechanistically, PTPN11 deletion attenuates CSF-1 receptor signaling, which depletes pro-tumor M2 macrophages while increasing anti-tumor M1 macrophages (211). On the other hand, deletion of PTPN11 enhances macrophage response to IFN-γ and increases production of the tumor cell-derived cytokine CXCL9, thereby promoting tumor infiltration of IFN-γ-producing T cells (212). More importantly, PTPN11 inhibitors combined with immunotherapies, such as anti-PD-1/L1, would reverse immunosuppression in the tumor microenvironment (TME) and potentiate the systemic antitumor effect in NSCLC cancer (213, 214).
Currently, research on PTPN22 is not only limited to autoimmune diseases, but more evidence indicates a considerable importance in tumor immunity. Implantation of syngeneic tumors in PTPN22-/- mice resulted in greater infiltration and activation of macrophages, NK cells and T cells, which in turn led to spontaneous tumor regression. More importantly, the combination with anti-PD-1/L1 therapy in the presence of PTPN22 inhibition significantly enhanced the antitumor efficacy (215, 216).
Based on the above findings, PTPN family members still serve as negative regulators in the immune system by restricting the development and differentiation of immune cells to achieve anti-inflammatory and anti-autoimmune responses. But on the other hand, PTPNs could make tumor cells evade immune surveillance.
Non-coding RNAs regulate the role of PTPNs in cancers
A substantial body of evidence has demonstrated that inhibition of some PTPN family members can considerably improve the efficacy of antitumor immunity, and the availability of small molecule inhibitors has given hope. However, drug discovery is an extremely long process, so the search for new therapeutic tools is urgent. The presence of a large number of non-protein-coding RNAs in the human genome and the potential for these non-coding RNAs to affect normal gene expression and disease progression make them a new class of targets for drug discovery (217). Therefore, we present here a comprehensive summary of ncRNAs, including miRNAs, lncRNAs and circRNAs, involved in the regulation of PTPNs in tumors and other diseases (Figure 4).
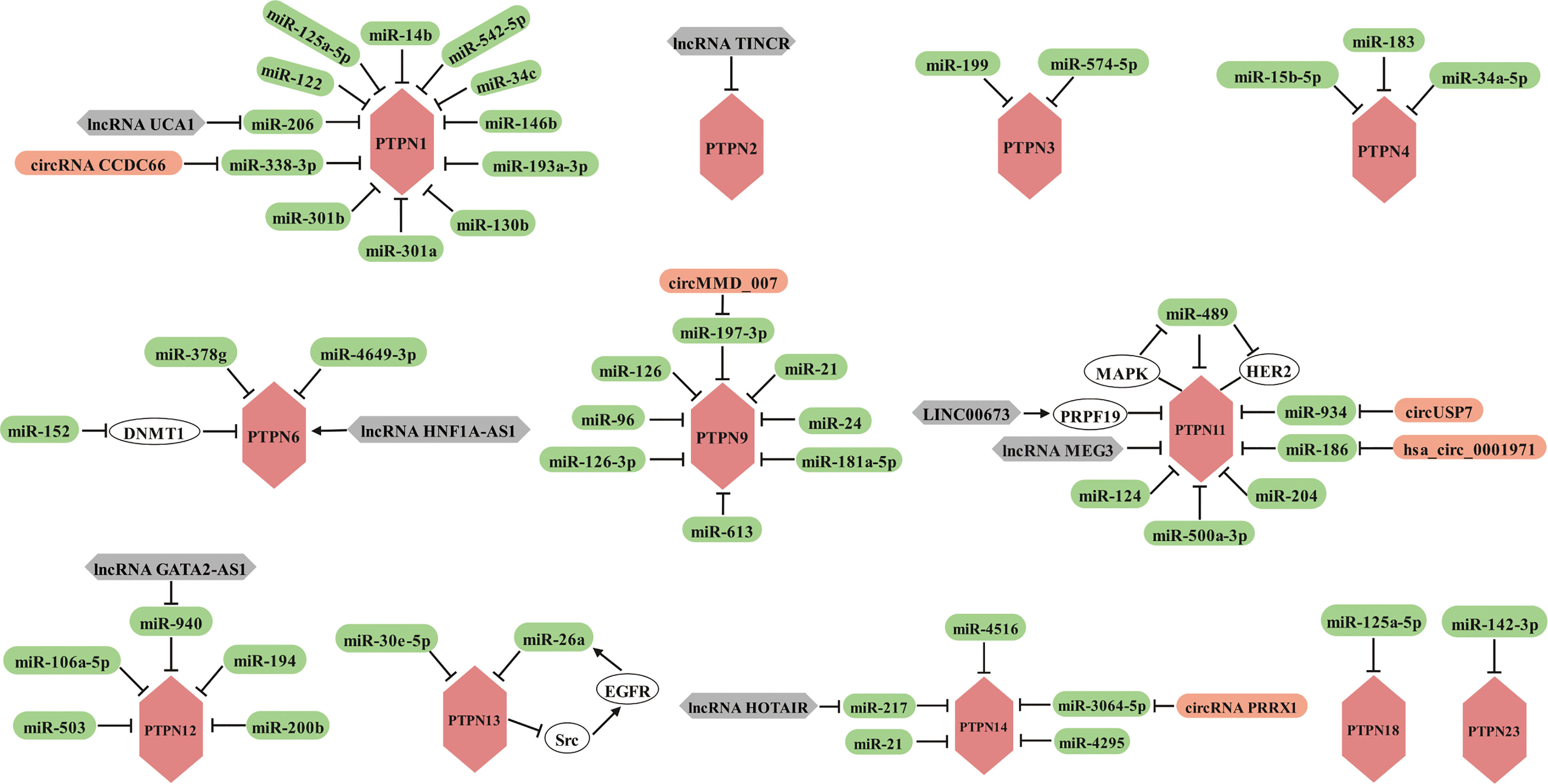
Figure 4 Members of the PTPN family are regulated by miRNAs, lncRNAs and circRNAs in various cancers.
MicroRNAs are implicated in the regulation of tumorigenesis, progression, metastasis, and drug resistance by targeting PTPNs
Currently, it is widely reported that miRNAs can influence the disease process, especially in cancer, by regulating the expression of PTPN1. In hepatocellular carcinoma, miR-122 and miR-206 target the 3’ untranslated region (3’ UTR) of PTPN1 mRNA and induce its degradation (218, 219), while miR-125a-5p suppresses PTPN1 expression via the MAPK signaling pathway (220), both of which ultimately alleviated the progression of hepatocellular carcinoma. Interestingly, miR-14b reverses the EMT phenotype of cisplatin-resistant lung adenocarcinoma cells by targeting PTPN1 (221). PTPN1 has also been discovered to be a target of numerous miRNAs in various malignancies. PTPN1 is targeted by miR-542-5p and miR-34c in glioma (222, 223), miR-146b and miR-338-3p in gastric cancer (224, 225), and miR-193a-3p in breast cancer (226). By regulating PTPN1, the miRNAs described above serve as tumor suppressors in cancers. Conversely, by targeting PTPN1, a tumor suppressor in bladder cancer, the miR-130 family (miR-130b, miR-301a, and miR-301b) contributes to cancer development (127).
The previously mentioned PTPN3 can confer chemotherapy resistance and tumor stem cell-like characteristics to ovarian cancer cells, but its expression is regulated by miR-199 (82). What’s more, miR-574-5p facilitates phosphorylation of p44/42 MAPKs by targeting PTPN3, thereby promoting angiogenesis in gastric cancer (227).
PTPN4 appears to function as a tumor suppressor in cancer, miR-15b-5p activates STAT3 signaling by targeting PTPN4 to promote oral squamous cell carcinoma progression (228). And miR-183 promotes migration and invasion of CD133+/CD326+ lung adenocarcinoma initiating cells by inhibiting PTPN4 (229). Furthermore, miR-34a-5p inhibits ROS-mediated apoptosis in papillary thyroid cancer cells through down-regulation of PTPN4 (230).
The regulation of PTPN6 is primarily mediated by a tiny proportion of miRNAs. Among them, miR-152 indirectly promotes PTPN6 expression to suppress lymphoma growth through down-regulation of DNA methyltransferase 1 (DNMT1) (231). But in nasopharyngeal carcinoma, miR-4649-3p inhibits cell proliferation and miR-378g partially enhances the radiosensitivity of NPC cells both by targeting PTPN6 (232, 233).
PTPN9 exhibits various functions depending on the type of tumor. PTPN9 promotes proliferation and invasion of esophageal cancer cells and non-small cell lung cancer and is negatively regulated by miR-126 and miR-126-3p, respectively (234, 235). But in cervical, breast, colorectal and gastric cancers, PTPN9 functions as a tumor suppressor with an essential role in suppressing tumor proliferation, invasion and migration. However, PTPN9 is targeted by miR-613 and miR-96 in cervical cancer (236–238), miR-96 and miR-24 in breast cancer (239, 240), miR-21 in colorectal cancer (241), and miR-181a-5p in gastric cancer (242).
PTPN11, which is considered an oncogenic factor and a key target for cancer immunotherapy according to several studies, is mediated by several miRNAs. MiR-124 and miR-489 inhibited the progression of renal cell carcinoma and hypopharyngeal squamous cell carcinoma by suppressing the expression of PTPN11, respectively (243, 244). In cutaneous squamous cell carcinoma, miR-204 inhibits STAT3 and facilitates the MAPK signaling pathway, possibly through PTPN11, a direct target of miR-204 (245). In oral squamous cell carcinoma, miR-186 directly binds to 3’ UTR of PTPN11 mRNA and inhibits the expression, which suppresses the signaling activity of Erk and AKT that is required for cancer cell growth (246). In hepatocellular carcinoma, miR-186 inhibits self-renewal of hepatocellular carcinoma stem cells and is more sensitive to cisplatin treatment by binding to 3’-UTR of PTPN11 mRNA and reducing its expression (247). However, miR-500a-3p promotes HCC cancer stem cell properties by targeting PTPN11, a negative regulator of the JAK/STAT3 signaling pathway (248). Moreover, HER2 is a direct target of miR-489, overexpression of miR-489 suppresses breast cancer invasion by attenuating HER2-PTPN11-MAPK signaling, which in turn inhibits miR-489, producing a mutually inhibitory loop (249).
PTPN12 suppresses progression of multiple cancers and is negatively controlled by miR-106a-5p in hepatocellular carcinoma (250), miR-503 in retinoblastoma (251), miR-194 in ovarian cancer (252), and miR-200b in colon cancer (253).
MiR-30e-5p promotes lung adenocarcinoma cell growth by targeting PTPN13 and is detrimental to the survival of LUAD patients (254). What’s more, miR-26a desensitizes NSCLC cells to tyrosine kinase inhibitors by targeting PTPN13. Mechanistically, miR-26a, which is downstream of EGFR signaling, directly targets and silences PTPN13 to maintain activation of Src, the dephosphorylated substrate of PTPN13, thereby enhancing the EGFR pathway in regulatory circuits (255).
By targeting PTPN14, miR-21 promotes intrahepatic cholangiocarcinoma proliferation and growth in vitro and in vivo (256), miR-4295 and miR-4516 contribute to the progression of osteosarcoma and glioblastoma, respectively (257, 258). But miR-217 inhibits EMT in gastric cancer (259).
In addition, miR-125a-5p is implicated in imatinib resistance in gastrointestinal stromal tumor (GIST). Mechanistically, overexpression of miR-125a-5p suppresses PTPN18 expression and subsequently enhances phosphorylated FAK (pFAK) expression in GIST cells, which contributes to imatinib resistance in GIST (260, 261).
PTPN23, which features a tumor suppressor function in testicular germ cell tumors, is regulated by miR-142-3p (262).
In light of the fact that almost all miRNAs target and suppress the expression of PTPNs, miRNA modulation combined with immunotherapy may be a novel therapeutic strategy.
LncRNAs and circRNAs regulate the role of PTPNs in cancers in part by sponging miRNAs
In recent years, a growing review of the literature revealed that lncRNA and circRNA appear to play a pivotal role in cancer and hold considerable promise as novel biomarkers and therapeutic targets (263). Next, we illustrate the mechanisms by which lncRNAs and circRNAs modulate PTPN family members, respectively.
PTPN family members have been shown to be regulated by different lncRNAs, including but not limited to lncRNA UCA1, TINCR, HNF1A-AS1, LINC00673, MEG3, GATA2-AS1, and HOTAIR. Specifically, lncRNA UCA1 accelerates the proliferation of breast cancer cells based on the miR-206/PTPN1 axis (264). In hepatocellular carcinoma, lncRNA TINCR interacts directly with and inhibits PTPN2 to promote the proliferation and invasion through activating STAT3 signaling (265). However, lncRNA HNF1A-AS1 reverses the malignancy of hepatocellular carcinoma by enhancing the phosphatase activity of PTPN6 (266). Furthermore, LINC00673 can strengthen the interaction of PTPN11 with PRPF19, an E3 ubiquitin ligase, and promote PTPN11 degradation through ubiquitination, resulting in reduced Src-Erk oncogenic signaling and enhanced STAT1-dependent antitumor response activation (267). PTPN11 can be targeted by lncRNA MEG3, thereby suppressing the proliferation and metastasis of renal cell carcinoma (243). On the basis of the miR-940/PTPN12 axis, lncRNA GATA2-AS1 restrains esophageal squamous cell carcinoma progression (268). In contrast, ectopic expression of lncRNA HOTAIR promotes drug resistance and augments GC cell proliferation and migration by inhibiting miR-217 expression and enhancing PTPN14 expression (269).
CircRNAs with similar regulatory mechanisms to lncRNAs are implicated in the regulation of PTPN family members, namely circRNA CCDC66, circMMD 007, circUSP7, has_circ_0001971, and circPRRX1. CircRNA CCDC66, in particular, promotes osteosarcoma proliferation and metastasis by sponging miR-338-3p to increase PTPN1 expression (270). Likewise, circMMD_007 promotes oncogenic effects in lung adenocarcinoma progression via the miR-197-3p/PTPN9 axis (271). CircUSP7 renders NSCLC patients resistant to anti-PD1 immunotherapy. Mechanistically, circUSP7 suppresses CD8+ T cell function by sponging miR-934 to up-regulate PTPN11 expression (272). Moreover, hsa_circ_0001971 promotes the proliferation of oral squamous carcinoma cells via miR-186/PTPN11 axis (273). Finally, circPRRX1 strengthens doxorubicin resistance in gastric cancer by regulating miR-3064-5p/PTPN14 signaling (274).
Taken together, lncRNAs and circRNAs serve as oncogenic or tumor suppressors and regulate the expression of PTPNs overwhelmingly through sponging miRNA, thereby dominating cancer progression.
Conclusion and perspective
Over the last three decades, a growing series of investigations have been able to conclude that PTPNs perform an essential role in almost all phenotypes of tumor cells. As described previously, there are numerous members of the PTPN family that all exert distinct functions in various malignancies, although they share the same catalytic structural domain. Some PTPNs exploit their structural domains with phosphatase activity to dephosphorylate a variety of oncogenic substrate proteins to achieve activation or inactivation, thereby participating in the regulation of cancer progression. Importantly, some members confer stem cell-like and EMT characteristics to tumor cells. The functions and signaling pathways regulated by PTPN family members are tissue- and cell-specific, so most PTPNs exert their functions still depend on the type of tumor in which they are located, but a small proportion of members still serve more specific functions, for instance, PTPN11 exerts oncogenic effects, while PTPN13 holds a tumor suppressive role in almost all cancers. Currently, although part of the mechanisms underlying the engagement of PTPN family members in tumors have been elucidated, the understanding of their operational mechanisms is even more challenging and urgent. In the future, a better understanding of the multifunctional and sophisticated regulatory mechanisms of PTPNs may be significant for the development of more specific targeted therapeutic strategies.
What’s more, PTPNs also hold critical role in cancer treatment, growing evidence suggested that PTPNs are implicated in chemotherapy and radiotherapy resistance. For instance, PTPN11 confers cisplatin resistance in small cell lung cancer, while PTPN6 promotes radiation resistance in nasopharyngeal carcinoma. Furthermore, inhibiting PTPNs, regardless of immune checkpoint inhibitors or CAR T therapies, can significantly improve the efficacy of immunotherapy. Currently, PTPN11 is the most reported to be associated with tumor immunotherapy and has spawned multiple inhibitors, including but not limited to SHP099 and TN0155, which in combination with anti-PD-1 and anti-PD-L1 therapies can significantly improve the malignant characteristics of tumors (209, 213, 275). Accordingly, further development of novel PTPNs inhibitors and investigation of the safety of these compounds is urgently need for conquering cancer in future.
Given the special role of PTPNs in cancer progression and immunotherapy, the question about how PTPNs inhibitors can move from the laboratory to rational clinical application should be the next thing to consider. As previously described, PTPNs perform a negative regulatory role in the immune system. Inhibition of PTPNs can activate a variety of immune cells involved in the killing of tumor cells, and also significantly potentiate the efficacy of cancer immunotherapy. In brief, PTPN inhibitors are positive modulators of antitumor immunotherapy. Then regarding the rational use of PTPNs inhibitors there are three situations: first, if PTPN plays a carcinogenic role, the usage of PTPN inhibitors will dramatically enhance the antitumor efficacy by suppressing the carcinogenic properties of PTPN while activating the immune system to combat tumors. Second, if PTPN exerts a tumor suppressive function, the use of PTPN inhibitors needs to be more cautious because it is not clear whether the anti-cancer effect of PTPN itself or the anti-tumor immune effect of PTPN inhibition is stronger, which needs to be further studied in mouse models. Third, there is the issue of the dual-sidedness of PTPN in specific cancers that we mentioned earlier, which requires even more in vivo experiments to further explore. Therefore, the rational and accurate clinical use of these inhibitors depends on the individual situation.
As we all know, the inhibitors applied in the clinic must be with high specificity. For the development of PTPN inhibitors, there is still a thorny issue of specificity. Furthermore, designing drug-like inhibitors for PTPNs is challenging due to their highly conserved and positively charged active site structures (276). Hence, the elucidation of the mechanisms regulating PTPNs may become a new therapeutic strategy. Here, we systematically summarize the ncRNAs involved in the regulation of PTPNs as an alternative to developing new inhibitors and propose that ncRNAs, especially miRNAs, in combination with immunotherapy, which may be a promising therapeutic approach to combat tumors.
Author Contributions
XT and CQ participated in consolidation of information and writing. HZ and YL guided and supervised this study. All authors contributed to the article and approved the submitted version.
Funding
This work was supported by National Natural Science Foundations of China (81802400, 81902519) and China Postdoctoral Science Foundation (2020M670053). This work was also supported in part by the Postdoctoral Fellowship of Peking-Tsinghua Center for Life Sciences.
Conflict of Interest
The authors declare that the research was conducted in the absence of any commercial or financial relationships that could be construed as a potential conflict of interest.
Publisher’s Note
All claims expressed in this article are solely those of the authors and do not necessarily represent those of their affiliated organizations, or those of the publisher, the editors and the reviewers. Any product that may be evaluated in this article, or claim that may be made by its manufacturer, is not guaranteed or endorsed by the publisher.
References
1. Gurzov EN, Stanley WJ, Brodnicki TC, Thomas HE. Protein tyrosine phosphatases: molecular switches in metabolism and diabetes. Trends Endocrinol Metab (2015) 26:30–9. doi: 10.1016/j.tem.2014.10.004
2. Alonso A, Sasin J, Bottini N, Friedberg I, Friedberg I, Osterman A, et al. Protein tyrosine phosphatases in the human genome. CELL (2004) 117:699–711. doi: 10.1016/j.cell.2004.05.018
3. Hao F, Wang C, Sholy C, Cao M, Kang X. Strategy for leukemia treatment targeting SHP-1,2 and SHIP. Front Cell Dev Biol (2021) 9:730400. doi: 10.3389/fcell.2021.730400
4. Spalinger MR, Schwarzfischer M, Scharl M. The role of protein tyrosine phosphatases in inflammasome activation. Int J Mol Sci (2020) 21(15):5481. doi: 10.3390/ijms21155481
5. Abdelsalam SS, Korashy HM, Zeidan A, Agouni A. The role of protein tyrosine phosphatase (PTP)-1B in cardiovascular disease and its interplay with insulin resistance. Biomolecules (2019) 9(7):286. doi: 10.3390/biom9070286
6. Mustelin T, Vang T, Bottini N. Protein tyrosine phosphatases and the immune response. Nat Rev Immunol (2005) 5:43–57. doi: 10.1038/nri1530
7. Pike KA, Tremblay ML. TC-PTP and PTP1B: Regulating JAK-STAT signaling, controlling lymphoid malignancies. CYTOKINE (2016) 82:52–7. doi: 10.1016/j.cyto.2015.12.025
8. Roskoski RJ. Src kinase regulation by phosphorylation and dephosphorylation. Biochem Biophys Res Commun (2005) 331:1–14. doi: 10.1016/j.bbrc.2005.03.012
9. Kerr DL, Haderk F, Bivona TG. Allosteric SHP2 inhibitors in cancer: Targeting the intersection of RAS, resistance, and the immune microenvironment. Curr Opin Chem Biol (2021) 62:1–12. doi: 10.1016/j.cbpa.2020.11.007
10. Anastasiadou E, Jacob LS, Slack FJ. Non-coding RNA networks in cancer. Nat Rev Cancer (2018) 18:5–18. doi: 10.1038/nrc.2017.99
11. Wang J, Zhu S, Meng N, He Y, Lu R, Yan GR. ncRNA-encoded peptides or proteins and cancer. Mol Ther (2019) 27:1718–25. doi: 10.1016/j.ymthe.2019.09.001
12. Li Y, Li G, Guo X, Yao H, Wang G, Li C. Non-coding RNA in bladder cancer. Cancer Lett (2020) 485:38–44. doi: 10.1016/j.canlet.2020.04.023
13. Duval R, Bui LC, Mathieu C, Nian Q, Berthelet J, Xu X, et al. Benzoquinone, a leukemogenic metabolite of benzene, catalytically inhibits the protein tyrosine phosphatase PTPN2 and alters STAT1 signaling. J Biol Chem (2019) 294:12483–94. doi: 10.1074/jbc.RA119.008666
14. Yuan T, Ma H, Du Z, Xiong X, Gao H, Fang Z, et al. Shp1 positively regulates EGFR signaling by controlling EGFR protein expression in mammary epithelial cells. Biochem Biophys Res Commun (2017) 488:439–44. doi: 10.1016/j.bbrc.2017.04.072
15. Baker SJ, Rane SG, Reddy EP. Hematopoietic cytokine receptor signaling. ONCOGENE (2007) 26:6724–37. doi: 10.1038/sj.onc.1210757
16. Dodd GT, Lee-Young RS, Bruning JC, Tiganis T. TCPTP regulates insulin signaling in AgRP neurons to coordinate glucose metabolism with feeding. DIABETES (2018) 67:1246–57. doi: 10.2337/db17-1485
17. Fukushima A, Loh K, Galic S, Fam B, Shields B, Wiede F, et al. T-Cell protein tyrosine phosphatase attenuates STAT3 and insulin signaling in the liver to regulate gluconeogenesis. DIABETES (2010) 59:1906–14. doi: 10.2337/db09-1365
18. Au AC, Hernandez PA, Lieber E, Nadroo AM, Shen YM, Kelley KA, et al. Protein tyrosine phosphatase PTPN14 is a regulator of lymphatic function and choanal development in humans. Am J Hum Genet (2010) 87:436–44. doi: 10.1016/j.ajhg.2010.08.008
19. Cortesio CL, Chan KT, Perrin BJ, Burton NO, Zhang S, Zhang ZY, et al. Calpain 2 and PTP1B function in a novel pathway with src to regulate invadopodia dynamics and breast cancer cell invasion. J Cell Biol (2008) 180:957–71. doi: 10.1083/jcb.200708048
20. Liu X, Chen Q, Hu XG, Zhang XC, Fu TW, Liu Q, et al. PTP1B promotes aggressiveness of breast cancer cells by regulating PTEN but not EMT. Tumour Biol (2016) 37:13479–87. doi: 10.1007/s13277-016-5245-1
21. Julien SG, Dube N, Read M, Penney J, Paquet M, Han Y, et al. Protein tyrosine phosphatase 1B deficiency or inhibition delays ErbB2-induced mammary tumorigenesis and protects from lung metastasis. Nat Genet (2007) 39:338–46. doi: 10.1038/ng1963
22. Zhao H, Martin E, Matalkah F, Shah N, Ivanov A, Ruppert JM, et al. Conditional knockout of SHP2 in ErbB2 transgenic mice or inhibition in HER2-amplified breast cancer cell lines blocks oncogene expression and tumorigenesis. ONCOGENE (2019) 38:2275–90. doi: 10.1038/s41388-018-0574-8
23. Sun X, Zhang J, Wang Z, Ji W, Tian R, Zhang F, et al. Shp2 plays a critical role in IL-6-Induced EMT in breast cancer cells. Int J Mol Sci (2017) 18(2):395. doi: 10.3390/ijms18020395
24. Zhang L, Yuan C, Peng J, Zhou L, Jiang Y, Lin Y, et al. SHP-2-Mediated upregulation of ZEB1 is important for PDGF-B-Induced cell proliferation and metastatic phenotype in triple negative breast cancer. Front Oncol (2020) 10:1230. doi: 10.3389/fonc.2020.01230
25. Martin E, Agazie YM. SHP2 potentiates the oncogenic activity of beta-catenin to promote triple-negative breast cancer. Mol Cancer Res (2021) 19:1946–56. doi: 10.1158/1541-7786.MCR-21-0060
26. Sausgruber N, Coissieux MM, Britschgi A, Wyckoff J, Aceto N, Leroy C, et al. Tyrosine phosphatase SHP2 increases cell motility in triple-negative breast cancer through the activation of SRC-family kinases. ONCOGENE (2015) 34:2272–8. doi: 10.1038/onc.2014.170
27. Hartman ZR, Schaller MD, Agazie YM. The tyrosine phosphatase SHP2 regulates focal adhesion kinase to promote EGF-induced lamellipodia persistence and cell migration. Mol Cancer Res (2013) 11:651–64. doi: 10.1158/1541-7786.MCR-12-0578
28. Veenstra C, Karlsson E, Mirwani SM, Nordenskjold B, Fornander T, Perez-Tenorio G, et al. The effects of PTPN2 loss on cell signalling and clinical outcome in relation to breast cancer subtype. J Cancer Res Clin Oncol (2019) 145:1845–56. doi: 10.1007/s00432-019-02918-y
29. Karlsson E, Veenstra C, Emin S, Dutta C, Perez-Tenorio G, Nordenskjold B, et al. Loss of protein tyrosine phosphatase, non-receptor type 2 is associated with activation of AKT and tamoxifen resistance in breast cancer. Breast Cancer Res Treat (2015) 153:31–40. doi: 10.1007/s10549-015-3516-y
30. Shields BJ, Wiede F, Gurzov EN, Wee K, Hauser C, Zhu HJ, et al. TCPTP regulates SFK and STAT3 signaling and is lost in triple-negative breast cancers. Mol Cell Biol (2013) 33:557–70. doi: 10.1128/MCB.01016-12
31. Liu CY, Chen KF, Chao TI, Chu PY, Huang CT, Huang TT, et al. Sequential combination of docetaxel with a SHP-1 agonist enhanced suppression of p-STAT3 signaling and apoptosis in triple negative breast cancer cells. J Mol Med (Berl) (2017) 95:965–75. doi: 10.1007/s00109-017-1549-x
32. Geng Q, Xian R, Yu Y, Chen F, Li R. SHP-1 acts as a tumor suppressor by interacting with EGFR and predicts the prognosis of human breast cancer. Cancer Biol Med (2021) 19(4):468–485. doi: 10.20892/j.issn.2095-3941.2020.0501
33. Yuan T, Wang Y, Zhao ZJ, Gu H. Protein-tyrosine phosphatase PTPN9 negatively regulates ErbB2 and epidermal growth factor receptor signaling in breast cancer cells. J Biol Chem (2010) 285:14861–70. doi: 10.1074/jbc.M109.099879
34. Su F, Ren F, Rong Y, Wang Y, Geng Y, Wang Y, et al. Protein tyrosine phosphatase Meg2 dephosphorylates signal transducer and activator of transcription 3 and suppresses tumor growth in breast cancer. Breast Cancer Res (2012) 14:R38. doi: 10.1186/bcr3134
35. Wang D, Wang S, Chen L, He D, Han S, Huang B, et al. The correlation of PTPN4 expression with prognosis in breast cancer. Int J Clin Exp Pathol (2018) 11:4845–53.
36. Revillion F, Puech C, Rabenoelina F, Chalbos D, Peyrat JP, Freiss G. Expression of the putative tumor suppressor gene PTPN13/PTPL1 is an independent prognostic marker for overall survival in breast cancer. Int J Cancer (2009) 124:638–43. doi: 10.1002/ijc.23989
37. Bompard G, Puech C, Prebois C, Vignon F, Freiss G. Protein-tyrosine phosphatase PTPL1/FAP-1 triggers apoptosis in human breast cancer cells. J Biol Chem (2002) 277:47861–9. doi: 10.1074/jbc.M208950200
38. Glondu-Lassis M, Dromard M, Lacroix-Triki M, Nirde P, Puech C, Knani D, et al. PTPL1/PTPN13 regulates breast cancer cell aggressiveness through direct inactivation of src kinase. Cancer Res (2010) 70:5116–26. doi: 10.1158/0008-5472.CAN-09-4368
39. Hamyeh M, Bernex F, Larive RM, Naldi A, Urbach S, Simony-Lafontaine J, et al. PTPN13 induces cell junction stabilization and inhibits mammary tumor invasiveness. THERANOSTICS (2020) 10:1016–32. doi: 10.7150/thno.38537
40. Liu X, Yang N, Figel SA, Wilson KE, Morrison CD, Gelman IH, et al. PTPN14 interacts with and negatively regulates the oncogenic function of YAP. ONCOGENE (2013) 32:1266–73. doi: 10.1038/onc.2012.147
41. Belle L, Ali N, Lonic A, Li X, Paltridge JL, Roslan S, et al. The tyrosine phosphatase PTPN14 (Pez) inhibits metastasis by altering protein trafficking. Sci Signal (2015) 8:a18. doi: 10.1126/scisignal.2005547
42. Zhang S, Fan G, Hao Y, Hammell M, Wilkinson JE, Tonks NK. Suppression of protein tyrosine phosphatase N23 predisposes to breast tumorigenesis via activation of FYN kinase. Genes Dev (2017) 31:1939–57. doi: 10.1101/gad.304261.117
43. Liu H, Wu Y, Zhu S, Liang W, Wang Z, Wang Y, et al. PTP1B promotes cell proliferation and metastasis through activating src and ERK1/2 in non-small cell lung cancer. Cancer Lett (2015) 359:218–25. doi: 10.1016/j.canlet.2015.01.020
44. Mainardi S, Mulero-Sanchez A, Prahallad A, Germano G, Bosma A, Krimpenfort P, et al. SHP2 is required for growth of KRAS-mutant non-small-cell lung cancer in vivo. Nat Med (2018) 24:961–7. doi: 10.1038/s41591-018-0023-9
45. Sun YJ, Zhuo ZL, Xian HP, Chen KZ, Yang F, Zhao XT. Shp2 regulates migratory behavior and response to EGFR-TKIs through ERK1/2 pathway activation in non-small cell lung cancer cells. Oncotarget (2017) 8:91123–33. doi: 10.18632/oncotarget.20249
46. Xia L, Yang F, Wu X, Li S, Kan C, Zheng H, et al. SHP2 inhibition enhances the anticancer effect of osimertinib in EGFR T790M mutant lung adenocarcinoma by blocking CXCL8 loop mediated stemness. Cancer Cell Int (2021) 21:337. doi: 10.1186/s12935-021-02056-x
47. Yang X, Tang C, Luo H, Wang H, Zhou X. Shp2 confers cisplatin resistance in small cell lung cancer via an AKT-mediated increase in CA916798. Oncotarget (2017) 8:23664–74. doi: 10.18632/oncotarget.15641
48. Tang C, Luo H, Luo D, Yang H, Zhou X. Src homology phosphotyrosyl phosphatase 2 mediates cisplatin-related drug resistance by inhibiting apoptosis and activating the Ras/PI3K/Akt1/survivin pathway in lung cancer cells. Oncol Rep (2018) 39:611–8. doi: 10.3892/or.2017.6109
49. Li MY, Peng WH, Wu CH, Chang YM, Lin YL, Chang GD, et al. PTPN3 suppresses lung cancer cell invasiveness by counteracting src-mediated DAAM1 activation and actin polymerization. ONCOGENE (2019) 38:7002–16. doi: 10.1038/s41388-019-0948-6
50. Li MY, Lai PL, Chou YT, Chi AP, Mi YZ, Khoo KH, et al. Protein tyrosine phosphatase PTPN3 inhibits lung cancer cell proliferation and migration by promoting EGFR endocytic degradation. ONCOGENE (2015) 34:3791–803. doi: 10.1038/onc.2014.312
51. Chi D, Wang D, Zhang M, Ma H, Chen F, Sun Y. CLEC12B suppresses lung cancer progression by inducing SHP-1 expression and inactivating the PI3K/AKT signaling pathway. Exp Cell Res (2021) 409:112914. doi: 10.1016/j.yexcr.2021.112914
52. Cao X, Chen YZ, Luo RZ, Zhang L, Zhang SL, Zeng J, et al. Tyrosine-protein phosphatase non-receptor type 12 expression is a good prognostic factor in resectable non-small cell lung cancer. Oncotarget (2015) 6:11704–13. doi: 10.18632/oncotarget.3588
53. Scrima M, De Marco C, De Vita F, Fabiani F, Franco R, Pirozzi G, et al. The nonreceptor-type tyrosine phosphatase PTPN13 is a tumor suppressor gene in non-small cell lung cancer. Am J Pathol (2012) 180:1202–14. doi: 10.1016/j.ajpath.2011.11.038
54. Zhu N, Zhang XJ, Zou H, Zhang YY, Xia JW, Zhang P, et al. PTPL1 suppresses lung cancer cell migration via inhibiting TGF-beta1-induced activation of p38 MAPK and smad 2/3 pathways and EMT. Acta Pharmacol Sin (2021) 42:1280–7. doi: 10.1038/s41401-020-00596-y
55. Hoekstra E, Das AM, Swets M, Cao W, van der Woude CJ, Bruno MJ, et al. Increased PTP1B expression and phosphatase activity in colorectal cancer results in a more invasive phenotype and worse patient outcome. Oncotarget (2016) 7:21922–38. doi: 10.18632/oncotarget.7829
56. Zhu S, Bjorge JD, Fujita DJ. PTP1B contributes to the oncogenic properties of colon cancer cells through src activation. Cancer Res (2007) 67:10129–37. doi: 10.1158/0008-5472.CAN-06-4338
57. Chen J, Zhao X, Yuan Y, Jing JJ. The expression patterns and the diagnostic/prognostic roles of PTPN family members in digestive tract cancers. Cancer Cell Int (2020) 20:238. doi: 10.1186/s12935-020-01315-7
58. Spalinger MR, Manzini R, Hering L, Riggs JB, Gottier C, Lang S, et al. PTPN2 regulates inflammasome activation and controls onset of intestinal inflammation and colon cancer. Cell Rep (2018) 22:1835–48. doi: 10.1016/j.celrep.2018.01.052
59. Li C, Li SZ, Huang XC, Chen J, Liu W, Zhang XD, et al. PTPN18 promotes colorectal cancer progression by regulating the c-MYC-CDK4 axis. Genes Dis (2021) 8:838–48. doi: 10.1016/j.gendis.2020.08.001
60. Zhang BD, Li YR, Ding LD, Wang YY, Liu HY, Jia BQ. Loss of PTPN4 activates STAT3 to promote the tumor growth in rectal cancer. Cancer Sci (2019) 110:2258–72. doi: 10.1111/cas.14031
61. Fan LC, Teng HW, Shiau CW, Lin H, Hung MH, Chen YL, et al. SHP-1 is a target of regorafenib in colorectal cancer. Oncotarget (2014) 5:6243–51. doi: 10.18632/oncotarget.2191
62. Wang D, Cheng Z, Zhao M, Jiao C, Meng Q, Pan H, et al. PTPN9 induces cell apoptosis by mitigating the activation of Stat3 and acts as a tumor suppressor in colorectal cancer. Cancer MANAG Res (2019) 11:1309–19. doi: 10.2147/CMAR.S187001
63. Fang H, Ma W, Guo X, Wang J. PTPN6 promotes chemosensitivity of colorectal cancer cells via inhibiting the SP1/MAPK signalling pathway. Cell Biochem Funct (2021) 39:392–400. doi: 10.1002/cbf.3604
64. van der Lely L, Hafliger J, Montalban-Arques A, Babler K, Schwarzfischer M, Sabev M, et al. Loss of PTPN23 promotes proliferation and epithelial-to-Mesenchymal transition in human intestinal cancer cells. Inflammation Intest Dis (2019) 4:161–73. doi: 10.1159/000502861
65. Xiang D, Cheng Z, Liu H, Wang X, Han T, Sun W, et al. Shp2 promotes liver cancer stem cell expansion by augmenting beta-catenin signaling and predicts chemotherapeutic response of patients. HEPATOLOGY (2017) 65:1566–80. doi: 10.1002/hep.28919
66. Liu JJ, Li Y, Chen WS, Liang Y, Wang G, Zong M, et al. Shp2 deletion in hepatocytes suppresses hepatocarcinogenesis driven by oncogenic beta-catenin, PIK3CA and MET. J Hepatol (2018) 69:79–88. doi: 10.1016/j.jhep.2018.02.014
68. Wen LZ, Ding K, Wang ZR, Ding CH, Lei SJ, Liu JP, et al. SHP-1 acts as a tumor suppressor in hepatocarcinogenesis and HCC progression. Cancer Res (2018) 78:4680–91. doi: 10.1158/0008-5472.CAN-17-3896
69. Ying D, Ruan Y, Zhou X. MEG2 inhibits the growth and metastasis of hepatocellular carcinoma by inhibiting AKT pathway. GENE (2019) 687:1–8. doi: 10.1016/j.gene.2018.11.003
70. Yan Y, Huang P, Mao K, He C, Xu Q, Zhang M, et al. Anti-oncogene PTPN13 inactivation by hepatitis b virus X protein counteracts IGF2BP1 to promote hepatocellular carcinoma progression. ONCOGENE (2021) 40:28–45. doi: 10.1038/s41388-020-01498-3
71. Zhang D, Wu F, Song J, Meng M, Fan X, Lu C, et al. A role for the NPM1/PTPN14/YAP axis in mediating hypoxia-induced chemoresistance to sorafenib in hepatocellular carcinoma. Cancer Cell Int (2022) 22:65. doi: 10.1186/s12935-022-02479-0
72. Wang H, Peng S, Cai J, Bao S. Silencing of PTPN18 induced ferroptosis in endometrial cancer cells through p-P38-Mediated GPX4/xCT down-regulation. Cancer MANAG Res (2021) 13:1757–65. doi: 10.2147/CMAR.S278728
73. Cao M, Gao D, Zhang N, Duan Y, Wang Y, Mujtaba H, et al. Shp2 expression is upregulated in cervical cancer, and Shp2 contributes to cell growth and migration and reduces sensitivity to cisplatin in cervical cancer cells. Pathol Res Pract (2019) 215:152621. doi: 10.1016/j.prp.2019.152621
74. Meng F, Zhao X, Zhang S. SHP-2 phosphatase promotes cervical cancer cell proliferation through inhibiting interferon-beta production. J Obstet Gynaecol Res (2013) 39:272–9. doi: 10.1111/j.1447-0756.2012.01952.x
75. Yan D, Zhu D, Zhao X, Su J. SHP-2 restricts apoptosis induced by chemotherapeutic agents via parkin-dependent autophagy in cervical cancer. Cancer Cell Int (2018) 18:8. doi: 10.1186/s12935-018-0505-3
76. Cai J, Huang S, Yi Y, Bao S. Downregulation of PTPN18 can inhibit proliferation and metastasis and promote apoptosis of endometrial cancer. Clin Exp Pharmacol Physiol (2019) 46:734–42. doi: 10.1111/1440-1681.13098
77. Yun HY, Kim MW, Lee HS, Kim W, Shin JH, Kim H, et al. Structural basis for recognition of the tumor suppressor protein PTPN14 by the oncoprotein E7 of human papillomavirus. PloS Biol (2019) 17:e3000367. doi: 10.1371/journal.pbio.3000367
78. White EA, Munger K, Howley PM. High-risk human papillomavirus E7 proteins target PTPN14 for degradation. MBIO (2016) 7(5):e01530-16. doi: 10.1128/mBio.01530-16
79. Hatterschide J, Bohidar AE, Grace M, Nulton TJ, Kim HW, Windle B, et al. PTPN14 degradation by high-risk human papillomavirus E7 limits keratinocyte differentiation and contributes to HPV-mediated oncogenesis. Proc Natl Acad Sci U.S.A. (2019) 116:7033–42. doi: 10.1073/pnas.1819534116
80. Wang W, Cao Y, Zhou X, Wei B, Zhang Y, Liu X. PTP1B promotes the malignancy of ovarian cancer cells in a JNK-dependent mechanism. Biochem Biophys Res Commun (2018) 503:903–9. doi: 10.1016/j.bbrc.2018.06.094
81. Mok SC, Kwok TT, Berkowitz RS, Barrett AJ, Tsui FW. Overexpression of the protein tyrosine phosphatase, nonreceptor type 6 (PTPN6), in human epithelial ovarian cancer. GYNECOL Oncol (1995) 57:299–303. doi: 10.1006/gyno.1995.1146
82. Li S, Cao J, Zhang W, Zhang F, Ni G, Luo Q, et al. Protein tyrosine phosphatase PTPN3 promotes drug resistance and stem cell-like characteristics in ovarian cancer. Sci Rep (2016) 6:36873. doi: 10.1038/srep36873
83. Hu Z, Li J, Gao Q, Wei S, Yang B. SHP2 overexpression enhances the invasion and metastasis of ovarian cancer in vitro and in vivo. Onco Targets Ther (2017) 10:3881–91. doi: 10.2147/OTT.S138833
84. Mao N, Li H, Yang H, Su J, Liang Z, He G. PTPN18 stimulates the development of ovarian cancer by activating the PI3K/AKT signaling. Evid Based Complement Alternat Med (2022) 2022:1091042. doi: 10.1155/2022/1091042
85. D’Hondt V, Lacroix-Triki M, Jarlier M, Boissiere-Michot F, Puech C, Coopman P, et al. High PTPN13 expression in high grade serous ovarian carcinoma is associated with a better patient outcome. Oncotarget (2017) 8:95662–73. doi: 10.18632/oncotarget.21175
86. Zhu JH, Chen R, Yi W, Cantin GT, Fearns C, Yang Y, et al. Protein tyrosine phosphatase PTPN13 negatively regulates Her2/ErbB2 malignant signaling. ONCOGENE (2008) 27:2525–31. doi: 10.1038/sj.onc.1210922
87. Wang Y, Li M, Huang T, Li J. Protein tyrosine phosphatase L1 inhibits high-grade serous ovarian carcinoma progression by targeting IkappaBalpha. Onco Targets Ther (2018) 11:7603–12. doi: 10.2147/OTT.S167106
88. Lessard L, Labbe DP, Deblois G, Begin LR, Hardy S, Mes-Masson AM, et al. PTP1B is an androgen receptor-regulated phosphatase that promotes the progression of prostate cancer. Cancer Res (2012) 72:1529–37. doi: 10.1158/0008-5472.CAN-11-2602
89. Weidemann SA, Sauer C, Luebke AM, Moller-Koop C, Steurer S, Hube-Magg C, et al. High-level expression of protein tyrosine phosphatase non-receptor 12 is a strong and independent predictor of poor prognosis in prostate cancer. BMC Cancer (2019) 19:944. doi: 10.1186/s12885-019-6182-3
90. Zhang K, Zhao H, Ji Z, Zhang C, Zhou P, Wang L, et al. Shp2 promotes metastasis of prostate cancer by attenuating the PAR3/PAR6/aPKC polarity protein complex and enhancing epithelial-to-mesenchymal transition. ONCOGENE (2016) 35:1271–82. doi: 10.1038/onc.2015.184
91. Castilla C, Chinchon D, Medina R, Torrubia FJ, Japon MA, Saez C. PTPL1 and PKCdelta contribute to proapoptotic signalling in prostate cancer cells. Cell Death Dis (2013) 4:e576. doi: 10.1038/cddis.2013.90
92. Wang R, Du Y, Shang J, Dang X, Niu G. PTPN14 acts as a candidate tumor suppressor in prostate cancer and inhibits cell proliferation and invasion through modulating LATS1/YAP signaling. Mol Cell Probes (2020) 53:101642. doi: 10.1016/j.mcp.2020.101642
93. Jin T, Li D, Yang T, Liu F, Kong J, Zhou Y. PTPN1 promotes the progression of glioma by activating the MAPK/ERK and PI3K/AKT pathways and is associated with poor patient survival. Oncol Rep (2019) 42:717–25. doi: 10.3892/or.2019.7180
94. Wang PF, Cai HQ, Zhang CB, Li YM, Liu X, Wan JH, et al. Molecular and clinical characterization of PTPN2 expression from RNA-seq data of 996 brain gliomas. J Neuroinflamm (2018) 15:145. doi: 10.1186/s12974-018-1187-4
95. Bartolome RA, Martin-Regalado A, Jaen M, Zannikou M, Zhang P, de Los RV, et al. Protein tyrosine phosphatase-1B inhibition disrupts IL13Ralpha2-promoted invasion and metastasis in cancer cells. Cancers (Basel) (2020) 12(2):500. doi: 10.3390/cancers12020500
96. Wu L, Wang F, Xu J, Chen Z. PTPN2 induced by inflammatory response and oxidative stress contributed to glioma progression. J Cell Biochem (2019) 120:19044–51. doi: 10.1002/jcb.29227
97. Roccograndi L, Binder ZA, Zhang L, Aceto N, Zhang Z, Bentires-Alj M, et al. SHP2 regulates proliferation and tumorigenicity of glioma stem cells. J Neurooncol (2017) 135:487–96. doi: 10.1007/s11060-017-2610-x
98. Wang Y, Su Y, Ji Z, Lv Z. High expression of PTPN3 predicts progression and unfavorable prognosis of glioblastoma. Med Sci Monit (2018) 24:7556–62. doi: 10.12659/MSM.911531
99. Liu KW, Feng H, Bachoo R, Kazlauskas A, Smith EM, Symes K, et al. SHP-2/PTPN11 mediates gliomagenesis driven by PDGFRA and INK4A/ARF aberrations in mice and humans. J Clin Invest (2011) 121:905–17. doi: 10.1172/JCI43690
100. Furcht CM, Buonato JM, Skuli N, Mathew LK, Munoz RA, Simon MC, et al. Multivariate signaling regulation by SHP2 differentially controls proliferation and therapeutic response in glioma cells. J Cell Sci (2014) 127:3555–67. doi: 10.1242/jcs.150862
101. Yao TW, Zhang J, Prados M, Weiss WA, James CD, Nicolaides T. EGFR blockade prevents glioma escape from BRAFV600E targeted therapy. Oncotarget (2015) 6:21993–2005. doi: 10.18632/oncotarget.4014
102. Chen Z, Morales JE, Guerrero PA, Sun H, McCarty JH. PTPN12/PTP-PEST regulates phosphorylation-dependent ubiquitination and stability of focal adhesion substrates in invasive glioblastoma cells. Cancer Res (2018) 78:3809–22. doi: 10.1158/0008-5472.CAN-18-0085
103. Liu L, Zhang S, Liu X, Liu J. Aberrant promoter 2 methylationmediated downregulation of protein tyrosine phosphatase, nonreceptor type 6, is associated with progression of esophageal squamous cell carcinoma. Mol Med Rep (2019) 19:3273–82. doi: 10.3892/mmr.2019.9971
104. Cao X, Li Y, Luo RZ, He LR, Yang J, Zeng MS, et al. Tyrosine-protein phosphatase nonreceptor type 12 is a novel prognostic biomarker for esophageal squamous cell carcinoma. Ann Thorac Surg (2012) 93:1674–80. doi: 10.1016/j.athoracsur.2011.12.056
105. Qi C, Han T, Tang H, Huang K, Min J, Li J, et al. Shp2 inhibits proliferation of esophageal squamous cell cancer via dephosphorylation of Stat3. Int J Mol Sci (2017) 18(1):134. doi: 10.3390/ijms18010134
106. Lu Y, Wang Z, Zhou L, Ma Z, Zhang J, Wu Y, et al. FAT1 and PTPN14 regulate the malignant progression and chemotherapy resistance of esophageal cancer through the hippo signaling pathway. Anal Cell Pathol (Amst) (2021) 2021:9290372. doi: 10.1155/2021/9290372
107. Wang J, Chen X, Liu B, Zhu Z. Suppression of PTP1B in gastric cancer cells in vitro induces a change in the genome-wide expression profile and inhibits gastric cancer cell growth. Cell Biol Int (2010) 34:747–53. doi: 10.1042/CBI20090447
108. Wang J, Liu B, Chen X, Su L, Wu P, Wu J, et al. PTP1B expression contributes to gastric cancer progression. Med Oncol (2012) 29:948–56. doi: 10.1007/s12032-011-9911-2
109. Wang N, She J, Liu W, Shi J, Yang Q, Shi B, et al. Frequent amplification of PTP1B is associated with poor survival of gastric cancer patients. Cell Cycle (2015) 14:732–43. doi: 10.1080/15384101.2014.998047
110. Jiang J, Jin MS, Kong F, Wang YP, Jia ZF, Cao DH, et al. Increased expression of tyrosine phosphatase SHP-2 in helicobacter pylori-infected gastric cancer. World J Gastroenterol (2013) 19:575–80. doi: 10.3748/wjg.v19.i4.575
111. Yamazaki S, Yamakawa A, Ito Y, Ohtani M, Higashi H, Hatakeyama M, et al. The CagA protein of helicobacter pylori is translocated into epithelial cells and binds to SHP-2 in human gastric mucosa. J Infect Dis (2003) 187:334–7. doi: 10.1086/367807
112. Fujii Y, Murata-Kamiya N, Hatakeyama M. Helicobacter pylori CagA oncoprotein interacts with SHIP2 to increase its delivery into gastric epithelial cells. Cancer Sci (2020) 111:1596–606. doi: 10.1111/cas.14391
113. Han X, Sun T, Hong J, Wei R, Dong Y, Huang D, et al. Nonreceptor tyrosine phosphatase 14 promotes proliferation and migration through regulating phosphorylation of YAP of hippo signaling pathway in gastric cancer cells. J Cell Biochem (2019) 120:17723–30. doi: 10.1002/jcb.29038
114. Kim SH, Yoo HS, Joo MK, Kim T, Park JJ, Lee BJ, et al. Arsenic trioxide attenuates STAT-3 activity and epithelial-mesenchymal transition through induction of SHP-1 in gastric cancer cells. BMC Cancer (2018) 18:150. doi: 10.1186/s12885-018-4071-9
115. Wang HC, Chiang WF, Huang HH, Shen YY, Chiang HC. Src-homology 2 domain-containing tyrosine phosphatase 2 promotes oral cancer invasion and metastasis. BMC Cancer (2014) 14:442. doi: 10.1186/1471-2407-14-442
116. Su Z, Tian H, Song HQ, Zhang R, Deng AM, Liu HW. PTPN12 inhibits oral squamous epithelial carcinoma cell proliferation and invasion and can be used as a prognostic marker. Med Oncol (2013) 30:618. doi: 10.1007/s12032-013-0618-4
117. Sun Z, Pan X, Zou Z, Ding Q, Wu G, Peng G. Increased SHP-1 expression results in radioresistance, inhibition of cellular senescence, and cell cycle redistribution in nasopharyngeal carcinoma cells. Radiat Oncol (2015) 10:152. doi: 10.1186/s13014-015-0445-1
118. Lin Q, Wang H, Lin X, Zhang W, Huang S, Zheng Y. PTPN12 affects nasopharyngeal carcinoma cell proliferation and migration through regulating EGFR. Cancer Biother Radiopharm (2018) 33:60–4. doi: 10.1089/cbr.2017.2254
119. Wang Q, Pan Y, Zhao L, Qi F, Liu J. Protein tyrosine phosphatase 1B(PTP1B) promotes melanoma cells progression through src activation. Bioengineered (2021) 12:8396–406. doi: 10.1080/21655979.2021.1988376
120. Liu J, Luan W, Zhang Y, Gu J, Shi Y, Yang Y, et al. HDAC6 interacts with PTPN1 to enhance melanoma cells progression. Biochem Biophys Res Commun (2018) 495:2630–6. doi: 10.1016/j.bbrc.2017.12.145
121. Diaz-Valdivia NI, Diaz J, Contreras P, Campos A, Rojas-Celis V, Burgos-Ravanal RA, et al. The non-receptor tyrosine phosphatase type 14 blocks caveolin-1-enhanced cancer cell metastasis. Oncogene (2020) 39:3693–709. doi: 10.1038/s41388-020-1242-3
122. Tsai WC, Chen CL, Chen HC. Protein tyrosine phosphatase SHP2 promotes invadopodia formation through suppression of rho signaling. Oncotarget (2015) 6:23845–56. doi: 10.18632/oncotarget.4313
123. Hoover AC, Strand GL, Nowicki PN, Anderson ME, Vermeer PD, Klingelhutz AJ, et al. Impaired PTPN13 phosphatase activity in spontaneous or HPV-induced squamous cell carcinomas potentiates oncogene signaling through the MAP kinase pathway. Oncogene (2009) 28:3960–70. doi: 10.1038/onc.2009.251
124. Peng XS, Yang JP, Qiang YY, Sun R, Cao Y, Zheng LS, et al. PTPN3 inhibits the growth and metastasis of clear cell renal cell carcinoma via inhibition of PI3K/AKT signaling. Mol Cancer Res (2020) 18:903–12. doi: 10.1158/1541-7786.MCR-19-1142
125. Long Q, Sun J, Lv J, Liang Y, Li H, Li X. PTPN13 acts as a tumor suppressor in clear cell renal cell carcinoma by inactivating akt signaling. Exp Cell Res (2020) 396:112286. doi: 10.1016/j.yexcr.2020.112286
126. Piao YR, Jin ZH. Protein tyrosine phosphatase nonreceptor type 12 suppresses the proliferation of renal cell carcinoma by inhibiting the activity of the PI3K/mTOR pathway. J Buon (2015) 20:1258–66.
127. Monoe Y, Jingushi K, Kawase A, Hirono T, Hirose R, Nakatsuji Y, et al. Pharmacological inhibition of miR-130 family suppresses bladder tumor growth by targeting various oncogenic pathways via PTPN1. Int J Mol Sci (2021) 22(9):4751. doi: 10.3390/ijms22094751
128. Piao Y, Liu X, Lin Z, Jin Z, Jin X, Yuan K, et al. Decreased expression of protein tyrosine phosphatase non-receptor type 12 is involved in the proliferation and recurrence of bladder transitional cell carcinoma. Oncol Lett (2015) 10:1620–6. doi: 10.3892/ol.2015.3454
129. Mariotti M, Castiglioni S, Maier JA. Inhibition of T24 human bladder carcinoma cell migration by RNA interference suppressing the expression of HD-PTP. Cancer Lett (2009) 273:155–63. doi: 10.1016/j.canlet.2008.08.017
130. Gao Q, Zhao YJ, Wang XY, Guo WJ, Gao S, Wei L, et al. Activating mutations in PTPN3 promote cholangiocarcinoma cell proliferation and migration and are associated with tumor recurrence in patients. Gastroenterology (2014) 146:1397–407. doi: 10.1053/j.gastro.2014.01.062
131. Sun R, Chen T, Li M, Liu Z, Qiu B, Li Z, et al. PTPN3 suppresses the proliferation and correlates with favorable prognosis of perihilar cholangiocarcinoma by inhibiting AKT phosphorylation. BioMed Pharmacother (2020) 121:109583. doi: 10.1016/j.biopha.2019.109583
132. Xu Q, Wu N, Li X, Guo C, Li C, Jiang B, et al. Inhibition of PTP1B blocks pancreatic cancer progression by targeting the PKM2/AMPK/mTOC1 pathway. Cell Death Dis (2019) 10:874. doi: 10.1038/s41419-019-2073-4
133. Kuang W, Wang X, Ding J, Li J, Ji M, Chen W, et al. PTPN2, a key predictor of prognosis for pancreatic adenocarcinoma, significantly regulates cell cycles, apoptosis, and metastasis. Front Immunol (2022) 13:805311. doi: 10.3389/fimmu.2022.805311
134. Yang HJ, Yu G, Wang Y, Guo X. Inflammatory response or oxidative stress induces upregulation of PTPN2 and thus promotes the progression of laryngocarcinoma. Eur Rev Med Pharmacol Sci (2020) 24:4314–9. doi: 10.26355/eurrev_202004_21012
135. Zhang Z, Xu T, Qin W, Huang B, Chen W, Li S, et al. Upregulated PTPN2 induced by inflammatory response or oxidative stress stimulates the progression of thyroid cancer. Biochem Biophys Res Commun (2020) 522:21–5. doi: 10.1016/j.bbrc.2019.11.047
136. Gu J, Han T, Ma RH, Zhu YL, Jia YN, Du JJ, et al. SHP2 promotes laryngeal cancer growth through the Ras/Raf/Mek/Erk pathway and serves as a prognostic indicator for laryngeal cancer. Int J Oncol (2014) 44:481–90. doi: 10.3892/ijo.2013.2191
137. Kim M, Morales LD, Lee CJ, Olivarez SA, Kim WJ, Hernandez J, et al. Overexpression of TC-PTP in murine epidermis attenuates skin tumor formation. Oncogene (2020) 39:4241–56. doi: 10.1038/s41388-020-1282-8
138. Baek M, Kim M, Lim JS, Morales LD, Hernandez J, Mummidi S, et al. Epidermal-specific deletion of TC-PTP promotes UVB-induced epidermal cell survival through the regulation of flk-1/JNK signaling. Cell Death Dis (2018) 9:730. doi: 10.1038/s41419-018-0781-9
139. Lee H, Kim M, Baek M, Morales LD, Jang IS, Slaga TJ, et al. Targeted disruption of TC-PTP in the proliferative compartment augments STAT3 and AKT signaling and skin tumor development. Sci Rep (2017) 7:45077. doi: 10.1038/srep45077
140. Le Sommer S, Morrice N, Pesaresi M, Thompson D, Vickers MA, Murray GI, et al. Deficiency in protein tyrosine phosphatase PTP1B shortens lifespan and leads to development of acute leukemia. Cancer Res (2018) 78:75–87. doi: 10.1158/0008-5472.CAN-17-0946
141. Demosthenous C, Han JJ, Hu G, Stenson M, Gupta M. Loss of function mutations in PTPN6 promote STAT3 deregulation via JAK3 kinase in diffuse large b-cell lymphoma. Oncotarget (2015) 6:44703–13. doi: 10.18632/oncotarget.6300
142. Wang X, Wang X, Lai J, Xu W, Zhu W, Chen G. Protein tyrosine phosphatase non-receptor type 12 suppresses tumor progression in osteosarcoma cells. J Orthop Sci (2022) S0949-2658(22)00007-0. doi: 10.1016/j.jos.2021.12.018
143. Chen CK, Yang CY, Hua KT, Ho MC, Johansson G, Jeng YM, et al. Leukocyte cell-derived chemotaxin 2 antagonizes MET receptor activation to suppress hepatocellular carcinoma vascular invasion by protein tyrosine phosphatase 1B recruitment. Hepatology (2014) 59:974–85. doi: 10.1002/hep.26738
144. Tai WT, Chen YL, Chu PY, Chen LJ, Hung MH, Shiau CW, et al. Protein tyrosine phosphatase 1B dephosphorylates PITX1 and regulates p120RasGAP in hepatocellular carcinoma. Hepatology (2016) 63:1528–43. doi: 10.1002/hep.28478
145. Yuan F, Gao Q, Tang H, Shi J, Zhou Y. OphiopogoninB targets PTP1B to inhibit the malignant progression of hepatocellular carcinoma by regulating the PI3K/AKT and AMPK signaling pathways. Mol Med Rep (2022) 25(4):122. doi: 10.3892/mmr.2022.12638
146. Wang Y, Salvucci O, Ohnuki H, Tran AD, Ha T, Feng JX, et al. Targeting the SHP2 phosphatase promotes vascular damage and inhibition of tumor growth. EMBO Mol Med (2021) 13:e14089. doi: 10.15252/emmm.202114089
147. Yu M, Xu C, Zhang H, Lun J, Wang L, Zhang G, et al. The tyrosine phosphatase SHP2 promotes proliferation and oxaliplatin resistance of colon cancer cells through AKT and ERK. Biochem Biophys Res Commun (2021) 563:1–7. doi: 10.1016/j.bbrc.2021.05.068
148. Yuan H, Zhao J, Yang Y, Wei R, Zhu L, Wang J, et al. SHP-2 interacts with CD81 and regulates the malignant evolution of colorectal cancer by inhibiting epithelial-mesenchymal transition. Cancer Manag Res (2020) 12:13273–84. doi: 10.2147/CMAR.S270813
149. Wei B, Xu L, Guo W, Wang Y, Wu J, Li X, et al. SHP2-mediated inhibition of DNA repair contributes to cGAS-STING activation and chemotherapeutic sensitivity in colon cancer. Cancer Res (2021) 81:3215–28. doi: 10.1158/0008-5472.CAN-20-3738
150. Wu MQ, Hu P, Gao J, Wei WD, Xiao XS, Tang HL, et al. Low expression of tyrosine-protein phosphatase nonreceptor type 12 is associated with lymph node metastasis and poor prognosis in operable triple-negative breast cancer. Asian Pac J Cancer Prev (2013) 14:287–92. doi: 10.7314/APJCP.2013.14.1.287
151. Sun T, Aceto N, Meerbrey KL, Kessler JD, Zhou C, Migliaccio I, et al. Activation of multiple proto-oncogenic tyrosine kinases in breast cancer via loss of the PTPN12 phosphatase. Cell (2011) 144:703–18. doi: 10.1016/j.cell.2011.02.003
152. Li J, Davidson D, Martins SC, Zhong MC, Wu N, Park M, et al. Loss of PTPN12 stimulates progression of ErbB2-dependent breast cancer by enhancing cell survival, migration, and epithelial-to-Mesenchymal transition. Mol Cell Biol (2015) 35:4069–82. doi: 10.1128/MCB.00741-15
153. Harris IS, Blaser H, Moreno J, Treloar AE, Gorrini C, Sasaki M, et al. PTPN12 promotes resistance to oxidative stress and supports tumorigenesis by regulating FOXO signaling. Oncogene (2014) 33:1047–54. doi: 10.1038/onc.2013.24
154. Chan RJ, Feng GS. PTPN11 is the first identified proto-oncogene that encodes a tyrosine phosphatase. Blood (2007) 109:862–7. doi: 10.1182/blood-2006-07-028829
155. Hinshaw DC, Shevde LA. The tumor microenvironment innately modulates cancer progression. Cancer Res (2019) 79:4557–66. doi: 10.1158/0008-5472.CAN-18-3962
156. Bauler TJ, Hughes ED, Arimura Y, Mustelin T, Saunders TL, King PD. Normal TCR signal transduction in mice that lack catalytically active PTPN3 protein tyrosine phosphatase. J Immunol (2007) 178:3680–7. doi: 10.4049/jimmunol.178.6.3680
157. Bauler TJ, Hendriks WJ, King PD. The FERM and PDZ domain-containing protein tyrosine phosphatases, PTPN4 and PTPN3, are both dispensable for T cell receptor signal transduction. PloS One (2008) 3:e4014. doi: 10.1371/journal.pone.0004014
158. Oh-hora M, Ogata M, Mori Y, Adachi M, Imai K, Kosugi A, et al. Direct suppression of TCR-mediated activation of extracellular signal-regulated kinase by leukocyte protein tyrosine phosphatase, a tyrosine-specific phosphatase. J Immunol (1999) 163:1282–8.
159. Wu Y, Deng W, McGinley EC, Klinke DN. Melanoma exosomes deliver a complex biological payload that upregulates PTPN11 to suppress T lymphocyte function. Pigment Cell Melanoma Res (2017) 30:203–18. doi: 10.1111/pcmr.12564
160. Saito T. Molecular dynamics of Co-signal molecules in T-cell activation. Adv Exp Med Biol (2019) 1189:135–52. doi: 10.1007/978-981-32-9717-3_5
161. Takehara T, Wakamatsu E, Machiyama H, Nishi W, Emoto K, Azuma M, et al. PD-L2 suppresses T cell signaling via coinhibitory microcluster formation and SHP2 phosphatase recruitment. Commun Biol (2021) 4:581. doi: 10.1038/s42003-021-02111-3
162. Wiede F, Shields BJ, Chew SH, Kyparissoudis K, van Vliet C, Galic S, et al. T Cell protein tyrosine phosphatase attenuates T cell signaling to maintain tolerance in mice. J Clin Invest (2011) 121:4758–74. doi: 10.1172/JCI59492
163. Salmond RJ, Brownlie RJ, Zamoyska R. Multifunctional roles of the autoimmune disease-associated tyrosine phosphatase PTPN22 in regulating T cell homeostasis. Cell Cycle (2015) 14:705–11. doi: 10.1080/15384101.2015.1007018
164. Vang T, Liu WH, Delacroix L, Wu S, Vasile S, Dahl R, et al. LYP inhibits T-cell activation when dissociated from CSK. Nat Chem Biol (2012) 8:437–46. doi: 10.1038/nchembio.916
165. Zhang X, Yu Y, Bai B, Wang T, Zhao J, Zhang N, et al. PTPN22 interacts with EB1 to regulate T-cell receptor signaling. FASEB J (2020) 34:8959–74. doi: 10.1096/fj.201902811RR
166. Salmond RJ, Huyer G, Kotsoni A, Clements L, Alexander DR. The src homology 2 domain-containing tyrosine phosphatase 2 regulates primary T-dependent immune responses and Th cell differentiation. J Immunol (2005) 175:6498–508. doi: 10.4049/jimmunol.175.10.6498
167. Young JA, Becker AM, Medeiros JJ, Shapiro VS, Wang A, Farrar JD, et al. The protein tyrosine phosphatase PTPN4/PTP-MEG1, an enzyme capable of dephosphorylating the TCR ITAMs and regulating NF-kappaB, is dispensable for T cell development and/or T cell effector functions. Mol Immunol (2008) 45:3756–66. doi: 10.1016/j.molimm.2008.05.023
168. Davidson D, Shi X, Zhong MC, Rhee I, Veillette A. The phosphatase PTP-PEST promotes secondary T cell responses by dephosphorylating the protein tyrosine kinase Pyk2. Immunity (2010) 33:167–80. doi: 10.1016/j.immuni.2010.08.001
169. Medgyesi D, Hobeika E, Biesen R, Kollert F, Taddeo A, Voll RE, et al. The protein tyrosine phosphatase PTP1B is a negative regulator of CD40 and BAFF-r signaling and controls b cell autoimmunity. J Exp Med (2014) 211:427–40. doi: 10.1084/jem.20131196
170. Yam-Puc JC, Zhang L, Maqueda-Alfaro RA, Garcia-Ibanez L, Zhang Y, Davies J, et al. Enhanced BCR signaling inflicts early plasmablast and germinal center b cell death. iScience (2021) 24:102038. doi: 10.1016/j.isci.2021.102038
171. Martin-Granados C, Prescott AR, Le Sommer S, Klaska IP, Yu T, Muckersie E, et al. A key role for PTP1B in dendritic cell maturation, migration, and T cell activation. J Mol Cell Biol (2015) 7:517–28. doi: 10.1093/jmcb/mjv032
172. Rhee I, Zhong MC, Reizis B, Cheong C, Veillette A. Control of dendritic cell migration, T cell-dependent immunity, and autoimmunity by protein tyrosine phosphatase PTPN12 expressed in dendritic cells. Mol Cell Biol (2014) 34:888–99. doi: 10.1128/MCB.01369-13
173. Spalinger MR, Crawford M, Bobardt SD, Li J, Sayoc-Becerra A, Santos AN, et al. Loss of protein tyrosine phosphatase non-receptor type 2 reduces IL-4-driven alternative macrophage activation. Mucosal Immunol (2022) 15:74–83. doi: 10.1038/s41385-021-00441-3
174. Heinonen KM, Dube N, Bourdeau A, Lapp WS, Tremblay ML. Protein tyrosine phosphatase 1B negatively regulates macrophage development through CSF-1 signaling. Proc Natl Acad Sci U.S.A. (2006) 103:2776–81. doi: 10.1073/pnas.0508563103
175. Heinonen KM, Tremblay ML. Protein tyrosine phosphatase 1B in hematopoiesis. Cell Cycle (2006) 5:1053–6. doi: 10.4161/cc.5.10.2735
176. Xu X, Wang X, Guo Y, Bai Y, He S, Wang N, et al. Inhibition of PTP1B promotes M2 polarization via MicroRNA-26a/MKP1 signaling pathway in murine macrophages. Front Immunol (2019) 10:1930. doi: 10.3389/fimmu.2019.01930
177. Heinonen KM, Nestel FP, Newell EW, Charette G, Seemayer TA, Tremblay ML, et al. T-Cell protein tyrosine phosphatase deletion results in progressive systemic inflammatory disease. Blood (2004) 103:3457–64. doi: 10.1182/blood-2003-09-3153
178. Hering L, Katkeviciute E, Schwarzfischer M, Niechcial A, Riggs JB, Wawrzyniak M, et al. Macrophages compensate for loss of protein tyrosine phosphatase N2 in dendritic cells to protect from elevated colitis. Int J Mol Sci (2021) 22(13):6820. doi: 10.3390/ijms22136820
179. Hering L, Katkeviciute E, Schwarzfischer M, Busenhart P, Gottier C, Mrdjen D, et al. Protein tyrosine phosphatase non-receptor type 2 function in dendritic cells is crucial to maintain tissue tolerance. Front Immunol (2020) 11:1856. doi: 10.3389/fimmu.2020.01856
180. Scharl M, Hruz P, McCole DF. Protein tyrosine phosphatase non-receptor type 2 regulates IFN-gamma-induced cytokine signaling in THP-1 monocytes. Inflammation Bowel Dis (2010) 16:2055–64. doi: 10.1002/ibd.21325
181. Kiratikanon S, Chattipakorn SC, Chattipakorn N, Kumfu S. The regulatory effects of PTPN6 on inflammatory process: Reports from mice to men. Arch Biochem Biophys (2022) 721:109189. doi: 10.1016/j.abb.2022.109189
182. Kamata T, Yamashita M, Kimura M, Murata K, Inami M, Shimizu C, et al. Src homology 2 domain-containing tyrosine phosphatase SHP-1 controls the development of allergic airway inflammation. J Clin Invest (2003) 111:109–19. doi: 10.1172/JCI15719
183. Speir M, Nowell CJ, Chen AA, O’Donnell JA, Shamie IS, Lakin PR, et al. Ptpn6 inhibits caspase-8- and Ripk3/Mlkl-dependent inflammation. Nat Immunol (2020) 21:54–64. doi: 10.1038/s41590-019-0550-7
184. Seo H, Lee IS, Park JE, Park SG, Lee DH, Park BC, et al. Role of protein tyrosine phosphatase non-receptor type 7 in the regulation of TNF-alpha production in RAW 264.7 macrophages. PloS One (2013) 8:e78776. doi: 10.1371/journal.pone.0078776
185. Coulombe G, Leblanc C, Cagnol S, Maloum F, Lemieux E, Perreault N, et al. Epithelial tyrosine phosphatase SHP-2 protects against intestinal inflammation in mice. Mol Cell Biol (2013) 33:2275–84. doi: 10.1128/MCB.00043-13
186. Xiao P, Zhang H, Zhang Y, Zheng M, Liu R, Zhao Y, et al. Phosphatase Shp2 exacerbates intestinal inflammation by disrupting macrophage responsiveness to interleukin-10. J Exp Med (2019) 216:337–49. doi: 10.1084/jem.20181198
187. Ding Y, Ouyang Z, Zhang C, Zhu Y, Xu Q, Sun H, et al. Tyrosine phosphatase SHP2 exacerbates psoriasis-like skin inflammation in mice via ERK5-dependent NETosis. MedComm (2020) 2022) 3:e120. doi: 10.1002/mco2.120
188. Wiede F, Sacirbegovic F, Leong YA, Yu D, Tiganis T. PTPN2-deficiency exacerbates T follicular helper cell and b cell responses and promotes the development of autoimmunity. J Autoimmun (2017) 76:85–100. doi: 10.1016/j.jaut.2016.09.004
189. Pao LI, Lam KP, Henderson JM, Kutok JL, Alimzhanov M, Nitschke L, et al. B cell-specific deletion of protein-tyrosine phosphatase Shp1 promotes b-1a cell development and causes systemic autoimmunity. Immunity (2007) 27:35–48. doi: 10.1016/j.immuni.2007.04.016
190. Kaneko T, Saito Y, Kotani T, Okazawa H, Iwamura H, Sato-Hashimoto M, et al. Dendritic cell-specific ablation of the protein tyrosine phosphatase Shp1 promotes Th1 cell differentiation and induces autoimmunity. J Immunol (2012) 188:5397–407. doi: 10.4049/jimmunol.1103210
191. Zhou L, Oh SY, Zhou Y, Yuan B, Wu F, Oh MH, et al. SHP-1 regulation of mast cell function in allergic inflammation and anaphylaxis. PloS One (2013) 8:e55763. doi: 10.1371/journal.pone.0055763
192. Wiede F, Lu KH, Du X, Zeissig MN, Xu R, Goh PK, et al. PTP1B is an intracellular checkpoint that limits T-cell and CAR T-cell antitumor immunity. Cancer Discovery (2022) 12:752–73. doi: 10.1158/2159-8290.CD-21-0694
193. Penafuerte C, Feldhammer M, Mills JR, Vinette V, Pike KA, Hall A, et al. Downregulation of PTP1B and TC-PTP phosphatases potentiate dendritic cell-based immunotherapy through IL-12/IFNgamma signaling. Oncoimmunology (2017) 6:e1321185. doi: 10.1080/2162402X.2017.1321185
194. Lawson KA, Sousa CM, Zhang X, Kim E, Akthar R, Caumanns JJ, et al. Functional genomic landscape of cancer-intrinsic evasion of killing by T cells. Nature (2020) 586:120–6. doi: 10.1038/s41586-020-2746-2
195. LaFleur MW, Nguyen TH, Coxe MA, Miller BC, Yates KB, Gillis JE, et al. PTPN2 regulates the generation of exhausted CD8(+) T cell subpopulations and restrains tumor immunity. Nat Immunol (2019) 20:1335–47. doi: 10.1038/s41590-019-0480-4
196. Manguso RT, Pope HW, Zimmer MD, Brown FD, Yates KB, Miller BC, et al. In vivo CRISPR screening identifies Ptpn2 as a cancer immunotherapy target. Nature (2017) 547:413–8. doi: 10.1038/nature23270
197. Katkeviciute E, Hering L, Montalban-Arques A, Busenhart P, Schwarzfischer M, Manzini R, et al. Protein tyrosine phosphatase nonreceptor type 2 controls colorectal cancer development. J Clin Invest (2021) 131(1):e140281. doi: 10.1172/JCI140281
198. Wiede F, Lu KH, Du X, Liang S, Hochheiser K, Dodd GT, et al. PTPN2 phosphatase deletion in T cells promotes anti-tumour immunity and CAR T-cell efficacy in solid tumours. EMBO J (2020) 39:e103637. doi: 10.15252/embj.2019103637
199. Goh PK, Wiede F, Zeissig MN, Britt KL, Liang S, Molloy T, et al. PTPN2 elicits cell autonomous and non-cell autonomous effects on antitumor immunity in triple-negative breast cancer. Sci Adv (2022) 8:k3338. doi: 10.1126/sciadv.abk3338
200. Koga S, Onishi H, Masuda S, Fujimura A, Ichimiya S, Nakayama K, et al. PTPN3 is a potential target for a new cancer immunotherapy that has a dual effect of T cell activation and direct cancer inhibition in lung neuroendocrine tumor. Transl Oncol (2021) 14:101152. doi: 10.1016/j.tranon.2021.101152
201. Fujimura A, Nakayama K, Imaizumi A, Kawamoto M, Oyama Y, Ichimiya S, et al. PTPN3 expressed in activated T lymphocytes is a candidate for a non-antibody-type immune checkpoint inhibitor. Cancer Immunol Immunother (2019) 68:1649–60. doi: 10.1007/s00262-019-02403-y
202. Watson HA, Dolton G, Ohme J, Ladell K, Vigar M, Wehenkel S, et al. Purity of transferred CD8(+) T cells is crucial for safety and efficacy of combinatorial tumor immunotherapy in the absence of SHP-1. Immunol Cell Biol (2016) 94:802–8. doi: 10.1038/icb.2016.45
203. Ben-Shmuel A, Sabag B, Puthenveetil A, Biber G, Levy M, Jubany T, et al. Inhibition of SHP-1 activity by PKC-theta regulates NK cell activation threshold and cytotoxicity. Elife (2022) 11:e73282. doi: 10.7554/eLife.73282
204. Myers DR, Abram CL, Wildes D, Belwafa A, Welsh A, Schulze CJ, et al. Shp1 loss enhances macrophage effector function and promotes anti-tumor immunity. Front Immunol (2020) 11:576310. doi: 10.3389/fimmu.2020.576310
205. Snook JP, Soedel AJ, Ekiz HA, O’Connell RM, Williams MA. Inhibition of SHP-1 expands the repertoire of antitumor T cells available to respond to immune checkpoint blockade. Cancer Immunol Res (2020) 8:506–17. doi: 10.1158/2326-6066.CIR-19-0690
206. Carmi Y, Prestwood TR, Spitzer MH, Linde IL, Chabon J, Reticker-Flynn NE, et al. Akt and SHP-1 are DC-intrinsic checkpoints for tumor immunity. JCI Insight (2016) 1:e89020. doi: 10.1172/jci.insight.89020
207. Chen YN, LaMarche MJ, Chan HM, Fekkes P, Garcia-Fortanet J, Acker MG, et al. Allosteric inhibition of SHP2 phosphatase inhibits cancers driven by receptor tyrosine kinases. Nature (2016) 535:148–52. doi: 10.1038/nature18621
208. Wang Y, Mohseni M, Grauel A, Diez JE, Guan W, Liang S, et al. SHP2 blockade enhances anti-tumor immunity via tumor cell intrinsic and extrinsic mechanisms. Sci Rep (2021) 11:1399. doi: 10.1038/s41598-021-80999-x
209. Zhao M, Guo W, Wu Y, Yang C, Zhong L, Deng G, et al. SHP2 inhibition triggers anti-tumor immunity and synergizes with PD-1 blockade. Acta Pharm Sin B (2019) 9:304–15. doi: 10.1016/j.apsb.2018.08.009
210. Ramesh A, Kumar S, Nandi D, Kulkarni A. CSF1R- and SHP2-Inhibitor-Loaded nanoparticles enhance cytotoxic activity and phagocytosis in tumor-associated macrophages. Adv Mater (2019) 31:e1904364. doi: 10.1002/adma.201904364
211. Quintana E, Schulze CJ, Myers DR, Choy TJ, Mordec K, Wildes D, et al. Allosteric inhibition of SHP2 stimulates antitumor immunity by transforming the immunosuppressive environment. Cancer Res (2020) 80:2889–902. doi: 10.1158/0008-5472.CAN-19-3038
212. Xiao P, Guo Y, Zhang H, Zhang X, Cheng H, Cao Q, et al. Myeloid-restricted ablation of Shp2 restrains melanoma growth by amplifying the reciprocal promotion of CXCL9 and IFN-gamma production in tumor microenvironment. Oncogene (2018) 37:5088–100. doi: 10.1038/s41388-018-0337-6
213. Chen D, Barsoumian HB, Yang L, Younes AI, Verma V, Hu Y, et al. SHP-2 and PD-L1 inhibition combined with radiotherapy enhances systemic antitumor effects in an anti-PD-1-Resistant model of non-small cell lung cancer. Cancer Immunol Res (2020) 8:883–94. doi: 10.1158/2326-6066.CIR-19-0744
214. Li J, Jie HB, Lei Y, Gildener-Leapman N, Trivedi S, Green T, et al. PD-1/SHP-2 inhibits Tc1/Th1 phenotypic responses and the activation of T cells in the tumor microenvironment. Cancer Res (2015) 75:508–18. doi: 10.1158/0008-5472.CAN-14-1215
215. Cubas R, Khan Z, Gong Q, Moskalenko M, Xiong H, Ou Q, et al. Autoimmunity linked protein phosphatase PTPN22 as a target for cancer immunotherapy. J Immunother Cancer (2020) 8(2):e001439. doi: 10.1136/jitc-2020-001439
216. Ho WJ, Croessmann S, Lin J, Phyo ZH, Charmsaz S, Danilova L, et al. Systemic inhibition of PTPN22 augments anticancer immunity. J Clin Invest (2021) 131(17):e146950. doi: 10.1172/JCI146950
217. Matsui M, Corey DR. Non-coding RNAs as drug targets. Nat Rev Drug Discovery (2017) 16:167–79. doi: 10.1038/nrd.2016.117
219. Yang Q, Zhang L, Zhong Y, Lai L, Li X. miR-206 inhibits cell proliferation, invasion, and migration by down-regulating PTP1B in hepatocellular carcinoma. Biosci Rep (2019) 39(5):BSR20181823. doi: 10.1042/BSR20181823
220. Xu X, Tao Y, Niu Y, Wang Z, Zhang C, Yu Y, et al. miR-125a-5p inhibits tumorigenesis in hepatocellular carcinoma. Aging (Albany NY) (2019) 11:7639–62. doi: 10.18632/aging.102276
221. Han Q, Cheng P, Yang H, Liang H, Lin F. miR-146b reverses epithelial-mesenchymal transition via targeting PTP1B in cisplatin-resistance human lung adenocarcinoma cells. J Cell Biochem (2019) 10.1002/jcb.29554. doi: 10.1002/jcb.29554
222. Li Z, Hu C, Zhen Y, Pang B, Yi H, Chen X. Pristimerin inhibits glioma progression by targeting AGO2 and PTPN1 expression via miR-542-5p. Biosci Rep (2019) 39(5):BSR20182389. doi: 10.1042/BSR20182389
223. Shu Y, Yao S, Cai S, Li J, He L, Zou J, et al. miR-34c inhibits proliferation of glioma by targeting PTP1B. Acta Biochim Biophys Sin (Shanghai) (2021) 53:325–32. doi: 10.1093/abbs/gmaa178
224. Xu J, Zhang Z, Chen Q, Yang L, Yin J. miR-146b regulates cell proliferation and apoptosis in gastric cancer by targeting PTP1B. Dig Dis Sci (2020) 65:457–63. doi: 10.1007/s10620-019-05771-8
225. Sun F, Yu M, Yu J, Liu Z, Zhou X, Liu Y, et al. miR-338-3p functions as a tumor suppressor in gastric cancer by targeting PTP1B. Cell Death Dis (2018) 9:522. doi: 10.1038/s41419-018-0611-0
226. Yu M, Liu Z, Liu Y, Zhou X, Sun F, Liu Y, et al. PTP1B markedly promotes breast cancer progression and is regulated by miR-193a-3p. FEBS J (2019) 286:1136–53. doi: 10.1111/febs.14724
227. Zhang S, Zhang R, Xu R, Shang J, He H, Yang Q. MicroRNA-574-5p in gastric cancer cells promotes angiogenesis by targeting protein tyrosine phosphatase non-receptor type 3 (PTPN3). Gene (2020) 733:144383. doi: 10.1016/j.gene.2020.144383
228. Liu X, Dong Y, Song D. Inhibition of microRNA-15b-5p attenuates the progression of oral squamous cell carcinoma via modulating the PTPN4/STAT3 axis. Cancer Manag Res (2020) 12:10559–72. doi: 10.2147/CMAR.S272498
229. Zhu C, Deng X, Wu J, Zhang J, Yang H, Fu S, et al. MicroRNA-183 promotes migration and invasion of CD133(+)/CD326(+) lung adenocarcinoma initiating cells via PTPN4 inhibition. Tumour Biol (2016) 37:11289–97. doi: 10.1007/s13277-016-4955-8
230. Tang Y, Yang H, Yu J, Li Z, Xu Q, Ding B, et al. Crocin induces ROS-mediated papillary thyroid cancer cell apoptosis by modulating the miR-34a-5p/PTPN4 axis in vitro. Toxicol Appl Pharmacol (2022) 437:115892. doi: 10.1016/j.taap.2022.115892
231. Wang QM, Lian GY, Song Y, Peng ZD, Xu SH, Gong Y. Downregulation of miR-152 contributes to DNMT1-mediated silencing of SOCS3/SHP-1 in non-Hodgkin lymphoma. Cancer Gene Ther (2019) 26:195–207. doi: 10.1038/s41417-018-0057-7
232. Pan X, Peng G, Liu S, Sun Z, Zou Z, Wu G. MicroRNA-4649-3p inhibits cell proliferation by targeting protein tyrosine phosphatase SHP-1 in nasopharyngeal carcinoma cells. Int J Mol Med (2015) 36:559–64. doi: 10.3892/ijmm.2015.2245
233. Lin T, Zhou F, Zhou H, Pan X, Sun Z, Peng G. MicroRNA-378g enhanced radiosensitivity of NPC cells partially by targeting protein tyrosine phosphatase SHP-1. Int J Radiat Biol (2015) 91:859–66. doi: 10.3109/09553002.2015.1096028
234. Zhu J, Li H, Ma J, Huang H, Qin J, Li Y. PTPN9 promotes cell proliferation and invasion in Eca109 cells and is negatively regulated by microRNA-126. Oncol Lett (2017) 14:1419–26. doi: 10.3892/ol.2017.6315
235. Chen J, Ding C, Yang X, Zhao J. BMSCs-derived exosomal MiR-126-3p inhibits the viability of NSCLC cells by targeting PTPN9. J BUON (2021) 26:1832–41.
236. Li WT, Wang BL, Yang CS, Lang BC, Lin YZ. MiR-613 promotes cell proliferation and invasion in cervical cancer via targeting PTPN9. Eur Rev Med Pharmacol Sci (2018) 22:4107–14. doi: 10.26355/eurrev_201807_15402
237. Ma X, Shi W, Peng L, Qin X, Hui Y. MiR-96 enhances cellular proliferation and tumorigenicity of human cervical carcinoma cells through PTPN9. Saudi J Biol Sci (2018) 25:863–7. doi: 10.1016/j.sjbs.2017.10.020
238. Chen X, Liu W, Guo X, Huang S, Song X. Ropivacaine inhibits cervical cancer cell growth via suppression of the miR96/MEG2/pSTAT3 axis. Oncol Rep (2020) 43:1659–68. doi: 10.3892/or.2020.7521
239. Hong Y, Liang H, Uzair-Ur- R, Wang Y, Zhang W, Zhou Y, et al. miR-96 promotes cell proliferation, migration and invasion by targeting PTPN9 in breast cancer. Sci Rep (2016) 6:37421. doi: 10.1038/srep37421
240. Du WW, Fang L, Li M, Yang X, Liang Y, Peng C, et al. MicroRNA miR-24 enhances tumor invasion and metastasis by targeting PTPN9 and PTPRF to promote EGF signaling. J Cell Sci (2013) 126:1440–53. doi: 10.1242/jcs.118299
241. Bao Z, Gao S, Zhang B, Shi W, Li A, Tian Q. The critical role of the miR-21-MEG2 axis in colorectal cancer. Crit Rev Eukaryot Gene Expr (2020) 30:509–18. doi: 10.1615/CritRevEukaryotGeneExpr.2020036484
242. Liu Z, Sun F, Hong Y, Liu Y, Fen M, Yin K, et al. MEG2 is regulated by miR-181a-5p and functions as a tumour suppressor gene to suppress the proliferation and migration of gastric cancer cells. Mol Cancer (2017) 16:133. doi: 10.1186/s12943-017-0695-7
243. Zhou H, Tang K, Liu H, Zeng J, Li H, Yan L, et al. Regulatory network of two tumor-suppressive noncoding RNAs interferes with the growth and metastasis of renal cell carcinoma. Mol Ther Nucleic Acids (2019) 16:554–65. doi: 10.1016/j.omtn.2019.04.005
244. Kikkawa N, Hanazawa T, Fujimura L, Nohata N, Suzuki H, Chazono H, et al. miR-489 is a tumour-suppressive miRNA target PTPN11 in hypopharyngeal squamous cell carcinoma (HSCC). Br J Cancer (2010) 103:877–84. doi: 10.1038/sj.bjc.6605811
245. Toll A, Salgado R, Espinet B, Diaz-Lagares A, Hernandez-Ruiz E, Andrades E, et al. MiR-204 silencing in intraepithelial to invasive cutaneous squamous cell carcinoma progression. Mol Cancer (2016) 15:53. doi: 10.1186/s12943-016-0537-z
246. Cai Z, Hao XY, Liu FX. MicroRNA-186 serves as a tumor suppressor in oral squamous cell carcinoma by negatively regulating the protein tyrosine phosphatase SHP2 expression. Arch Oral Biol (2018) 89:20–5. doi: 10.1016/j.archoralbio.2018.01.016
247. Yao H, Yang Z, Lou Y, Huang J, Yang P, Jiang W, et al. miR-186 inhibits liver cancer stem cells expansion via targeting PTPN11. Front Oncol (2021) 11:632976. doi: 10.3389/fonc.2021.632976
248. Jiang C, Long J, Liu B, Xu M, Wang W, Xie X, et al. miR-500a-3p promotes cancer stem cells properties via STAT3 pathway in human hepatocellular carcinoma. J Exp Clin Cancer Res (2017) 36:99. doi: 10.1186/s13046-017-0568-3
249. Patel Y, Shah N, Lee JS, Markoutsa E, Jie C, Liu S, et al. A novel double-negative feedback loop between miR-489 and the HER2-SHP2-MAPK signaling axis regulates breast cancer cell proliferation and tumor growth. Oncotarget (2016) 7:18295–308. doi: 10.18632/oncotarget.7577
250. Liang Z, Li X, Duan F, Song L, Wang Z, Li X, et al. Protein tyrosine phosphatase non-receptor type 12 (PTPN12), negatively regulated by miR-106a-5p, suppresses the progression of hepatocellular carcinoma. Hum Cell (2022) 35:299–309. doi: 10.1007/s13577-021-00627-8
251. Cheng Y, Liu W. MicroRNA-503 serves an oncogenic role in retinoblastoma progression by directly targeting PTPN12. Exp Ther Med (2019) 18:2285–92. doi: 10.3892/etm.2019.7795
252. Liang T, Li L, Cheng Y, Ren C, Zhang G. MicroRNA-194 promotes the growth, migration, and invasion of ovarian carcinoma cells by targeting protein tyrosine phosphatase nonreceptor type 12. Onco Targets Ther (2016) 9:4307–15. doi: 10.2147/OTT.S90976
253. Rossi L, Bonmassar E, Faraoni I. Modification of miR gene expression pattern in human colon cancer cells following exposure to 5-fluorouracil in vitro. Pharmacol Res (2007) 56:248–53. doi: 10.1016/j.phrs.2007.07.001
254. Zhuang L, Shou T, Li K, Gao CL, Duan LC, Fang LZ, et al. MicroRNA-30e-5p promotes cell growth by targeting PTPN13 and indicates poor survival and recurrence in lung adenocarcinoma. J Cell Mol Med (2017) 21:2852–62. doi: 10.1111/jcmm.13198
255. Xu S, Wang T, Yang Z, Li Y, Li W, Wang T, et al. miR-26a desensitizes non-small cell lung cancer cells to tyrosine kinase inhibitors by targeting PTPN13. Oncotarget (2016) 7:45687–701. doi: 10.18632/oncotarget.9920
256. Wang LJ, He CC, Sui X, Cai MJ, Zhou CY, Ma JL, et al. MiR-21 promotes intrahepatic cholangiocarcinoma proliferation and growth in vitro and in vivo by targeting PTPN14 and PTEN. Oncotarget (2015) 6:5932–46. doi: 10.18632/oncotarget.3465
257. Liang G, Duan C, He J, Ma W, Dai X. PTPN14, a target gene of miR-4295, restricts the growth and invasion of osteosarcoma cells through inactivation of YAP1 signalling. Clin Exp Pharmacol Physiol (2020) 47:1301–10. doi: 10.1111/1440-1681.13296
258. Cui T, Bell EH, McElroy J, Becker AP, Gulati PM, Geurts M, et al. miR-4516 predicts poor prognosis and functions as a novel oncogene via targeting PTPN14 in human glioblastoma. ONCOGENE (2019) 38:2923–36. doi: 10.1038/s41388-018-0601-9
259. Liu YP, Sun XH, Cao XL, Jiang WW, Wang XX, Zhang YF, et al. MicroRNA-217 suppressed epithelial-to-mesenchymal transition in gastric cancer metastasis through targeting PTPN14. Eur Rev Med Pharmacol Sci (2017) 21:1759–67.
260. Huang WK, Akcakaya P, Gangaev A, Lee L, Zeljic K, Hajeri P, et al. miR-125a-5p regulation increases phosphorylation of FAK that contributes to imatinib resistance in gastrointestinal stromal tumors. Exp Cell Res (2018) 371:287–96. doi: 10.1016/j.yexcr.2018.08.028
261. Akcakaya P, Caramuta S, Ahlen J, Ghaderi M, Berglund E, Ostman A, et al. microRNA expression signatures of gastrointestinal stromal tumours: associations with imatinib resistance and patient outcome. Br J Cancer (2014) 111:2091–102. doi: 10.1038/bjc.2014.548
262. Tanaka K, Kondo K, Kitajima K, Muraoka M, Nozawa A, Hara T. Tumor-suppressive function of protein-tyrosine phosphatase non-receptor type 23 in testicular germ cell tumors is lost upon overexpression of miR142-3p microRNA. J Biol Chem (2013) 288:23990–9. doi: 10.1074/jbc.M113.478891
263. Bhan A, Soleimani M, Mandal SS. Long noncoding RNA and cancer: A new paradigm. Cancer Res (2017) 77:3965–81. doi: 10.1158/0008-5472.CAN-16-2634
264. Li Y, Zeng Q, Qiu J, Pang T, Xian J, Zhang X. Long non-coding RNA UCA1 promotes breast cancer by upregulating PTP1B expression via inhibiting miR-206. Cancer Cell Int (2019) 19:275. doi: 10.1186/s12935-019-0958-z
265. Tang C, Feng W, Bao Y, Du H. Long non-coding RNA TINCR promotes hepatocellular carcinoma proliferation and invasion via STAT3 signaling by direct interacting with T-cell protein tyrosine phosphatase (TCPTP). Bioengineered (2021) 12:2119–31. doi: 10.1080/21655979.2021.1930336
266. Ding CH, Yin C, Chen SJ, Wen LZ, Ding K, Lei SJ, et al. The HNF1alpha-regulated lncRNA HNF1A-AS1 reverses the malignancy of hepatocellular carcinoma by enhancing the phosphatase activity of SHP-1. Mol Cancer (2018) 17:63. doi: 10.1186/s12943-018-0813-1
267. Zheng J, Huang X, Tan W, Yu D, Du Z, Chang J, et al. Pancreatic cancer risk variant in LINC00673 creates a miR-1231 binding site and interferes with PTPN11 degradation. Nat Genet (2016) 48:747–57. doi: 10.1038/ng.3568
268. Niu Y, Guo Y, Li Y, Shen S, Liang J, Guo W, et al. LncRNA GATA2-AS1 suppresses esophageal squamous cell carcinoma progression via the mir-940/PTPN12 axis. Exp Cell Res (2022) 416(2):113130. doi: 10.1016/j.yexcr.2022.113130
269. Wang H, Qin R, Guan A, Yao Y, Huang Y, Jia H, et al. HOTAIR enhanced paclitaxel and doxorubicin resistance in gastric cancer cells partly through inhibiting miR-217 expression. J Cell Biochem (2018) 119:7226–34. doi: 10.1002/jcb.26901
270. Xiang D, Li Y, Lin Y. Circular RNA circCCDC66 contributes to malignant phenotype of osteosarcoma by sponging miR-338-3p to upregulate the expression of PTP1B. BioMed Res Int (2020) 2020:4637109. doi: 10.1155/2020/4637109
271. Zhu L, Guo T, Chen W, Lin Z, Ye M, Pan X. CircMMD_007 promotes oncogenic effects in the progression of lung adenocarcinoma through microRNA-197-3p/protein tyrosine phosphatase non-receptor type 9 axis. Bioengineered (2022) 13:4991–5004. doi: 10.1080/21655979.2022.2037956
272. Chen SW, Zhu SQ, Pei X, Qiu BQ, Xiong D, Long X, et al. Cancer cell-derived exosomal circUSP7 induces CD8(+) T cell dysfunction and anti-PD1 resistance by regulating the miR-934/SHP2 axis in NSCLC. Mol Cancer (2021) 20:144. doi: 10.1186/s12943-021-01448-x
273. Jun W, Shaobo O, Xianhua Z, Siyu Z, Mingyang C, Xin F, et al. Deregulation of hsa_circ_0001971/miR-186 and hsa_circ_0001874/miR-296 signaling pathways promotes the proliferation of oral squamous carcinoma cells by synergistically activating SHP2/PLK1 signals. Sci Rep (2021) 11:20561. doi: 10.1038/s41598-021-99488-2
274. Wang S, Ping M, Song B, Guo Y, Li Y, Jia J. Exosomal CircPRRX1 enhances doxorubicin resistance in gastric cancer by regulating MiR-3064-5p/PTPN14 signaling. Yonsei Med J (2020) 61:750–61. doi: 10.3349/ymj.2020.61.9.750
275. Liu C, Lu H, Wang H, Loo A, Zhang X, Yang G, et al. Combinations with allosteric SHP2 inhibitor TNO155 to block receptor tyrosine kinase signaling. Clin Cancer Res (2021) 27:342–54. doi: 10.1158/1078-0432.CCR-20-2718
Keywords: PTPNs, cancer, immunotherapy, miRNAs, lncRNAs, circRNAs
Citation: Tang X, Qi C, Zhou H and Liu Y (2022) Critical roles of PTPN family members regulated by non-coding RNAs in tumorigenesis and immunotherapy. Front. Oncol. 12:972906. doi: 10.3389/fonc.2022.972906
Received: 19 June 2022; Accepted: 04 July 2022;
Published: 26 July 2022.
Edited by:
João Pessoa, University of Coimbra, PortugalReviewed by:
Ulrike Lorenz, University of Virginia, United StatesBonsu Ku, Korea Research Institute of Bioscience and Biotechnology (KRIBB), South Korea
Copyright © 2022 Tang, Qi, Zhou and Liu. This is an open-access article distributed under the terms of the Creative Commons Attribution License (CC BY). The use, distribution or reproduction in other forums is permitted, provided the original author(s) and the copyright owner(s) are credited and that the original publication in this journal is cited, in accordance with accepted academic practice. No use, distribution or reproduction is permitted which does not comply with these terms.
*Correspondence: Honghong Zhou, emhvdWhvbmdob25nQGlicC5hYy5jbg==; Yongshuo Liu, bGl1eW9uZ3NodW9AcGt1LmVkdS5jbg==
†These authors have contributed equally to this work