- 1Molecular Pharmacology Unit, Department of Applied Research and Technological Development, Fondazione Istituto di Ricovero e Cura a Carattere Scientifico (IRCCS) Istituto Nazionale dei Tumori, Milan, Italy
- 2Sarcoma Service, Department of Surgery, Fondazione Istituto di Ricovero e Cura a Carattere Scientifico (IRCCS) Istituto Nazionale dei Tumori, Milan, Italy
Well differentiated and dedifferentiated liposarcomas (WDLPS and DDLPS) are tumors of the adipose tissue poorly responsive to conventional cytotoxic chemotherapy which currently remains the standard-of-care. The dismal prognosis of the DDLPS subtype indicates an urgent need to identify new therapeutic targets to improve the patient outcome. The amplification of the two driver genes MDM2 and CDK4, shared by WDLPD and DDLPS, has provided the rationale to explore targeting the encoded ubiquitin-protein ligase and cell cycle regulating kinase as a therapeutic approach. Investigation of the genomic landscape of WD/DDLPS and preclinical studies have revealed additional potential targets such as receptor tyrosine kinases, the cell cycle kinase Aurora A, and the nuclear exporter XPO1. While the therapeutic significance of these targets is being investigated in clinical trials, insights into the molecular characteristics associated with dedifferentiation and progression from WDLPS to DDLPS highlighted additional genetic alterations including fusion transcripts generated by chromosomal rearrangements potentially providing new druggable targets (e.g. NTRK, MAP2K6). Recent years have witnessed the increasing use of patient-derived cell and tumor xenograft models which offer valuable tools to accelerate drug repurposing and combination studies. Implementation of integrated “multi-omics” investigations applied to models recapitulating WD/DDLPS genetics, histologic differentiation and biology, will hopefully lead to a better understanding of molecular alterations driving liposarcomagenesis and DDLPS progression, as well as to the identification of new therapies tailored on tumor histology and molecular profile.
Introduction
Well differentiated (WD) and dedifferentiated (DD) liposarcomas (LPS) represent the most frequent adipose tissue tumors occurring preferably in adults, particularly in the retroperitoneum and extremities (1). WDLPS and DDLPS exhibit different aggressive potential reflecting their morphologic diversity. WDLPS, a low-grade tumor characterized by malignant adipocytes, may recur locally after surgery, a condition potentially lethal when presenting in the retroperitoneum. DDLPS is a high-grade non-lipogenic malignancy with propensity to both recur locally and metastasize, particularly when located in the retroperitoneum compared to extremity and trunk wall (2–4). WD and DD components often coexist, suggesting that WDLPS and DDLPS represent evolution states of one disease. Indeed, they share peculiar supernumerary ring and giant marker chromosomes including amplified sequences of 12q13-15 (5), which cause overexpression of genes that act as oncogenic drivers (i.e. MDM2 and CDK4) and represent adipocytic differentiation/diagnostic markers (i.e. HMGA2 and CPM) (6–8). In comparison to WDLPS, DDLPS generally harbor additional epigenetic and genetic alterations (6–8). DDLPS may undergo heterologous differentiation associated with a more aggressive phenotype mainly evident when rhabdomyoblastic traits are acquired (9–12).
Surgery represents the curative treatment for localized WDLPS/DDLPS. Neoadjuvant radiation therapy has been suggested to reduce the risk of local recurrence (13) occurring in about one in three-four patients (2, 3). LPS-specific therapeutic options for patients who develop local recurrence and distant metastasis are lacking and both WDLPS and DDLPS are poorly responsive to either conventional cytotoxic chemotherapy or clinically tested targeted therapies (14, 15). These issues underline the need to identify new actionable targets and biology-driven LPS-specific therapeutic approaches to impact patient outcomes (8, 16, 17).
Herein, after briefly summarizing the current standard-of-care and recent findings from clinical investigations on new therapeutic strategies for WD/DDLPS, we focus on the rationale behind emerging treatment options exploiting potential vulnerabilities based on LPS biology. We do not address here immunotherapeutic approaches, which are summarized in other reviews (8, 18).
Conventional systemic and histotype-specific therapies
Therapies used in most soft tissue sarcomas (STS) derive from studies that investigated a general STS patient population. Mirroring STS, anthracycline-based therapies remain the first-line treatment for advanced DDLPS (19). However, Phase II/III trials showed that the low tumor response to doxorubicin (<10%) was enhanced when combined with high-dose ifosfamide (22%) (15). High-dose ifosfamide has shown effectiveness in recurrent WD/DDLPS even after previous treatment with anthracyclines plus ifosfamide. Gemcitabine or docetaxel monotherapies are also commonly used second-line treatments (14, 20, 21). The addition of docetaxel to gemcitabine improved treatment efficacy compared to gemcitabine alone but, due to the increased toxicity, this option needs a careful patient selection (22). An increasing knowledge about drug sensitivities of STS histologies and results from several retrospective and prospective clinical trials have prompted histology-driven treatments (19, 23). Two marine-derived cytotoxic drugs, trabectedin and eribulin, have been approved by FDA and EMA for the treatment of metastatic WDLPS/DDLPS following phase II studies and further comparative phase III trials showing improved benefit over dacarbazine (24, 25). However, trabectedin impact on progression-free survival did not translate into a survival benefit (26), while eribulin produced only a limited improvement of overall survival without improving progression-free survival (27). Retrospective evidence suggested that low grade DDLPS may benefit from trabectedin while ifosfamide may be more active for high grade DDLPS (28). Nevertheless, clinical, histological and molecular features, as well as predictive biomarkers guiding the selection of patients for these treatments and improving their therapeutic index are lacking (29).
Overexpressed biomarkers as therapeutic targets: MDM2 AND CDK4
MDM2 and CDK4 amplification in over 90% of WDLPS and DDLPS, besides representing a diagnostic tool, provides the rationale for evaluating novel therapies (6, 16, 30, 31).
MDM2 encodes an E3 ubiquitin-protein ligase that binds p53 promoting its proteasome-mediated degradation thus negatively regulating its tumor suppressor function (32, 33). MDM2 knockdown heavily impacted DDLPS cell proliferation (34, 35). Likewise, DDLPS cells underwent cell cycle arrest and apoptosis after exposure to nutlins (e.g. nutlin 3a, idasanutlin) (36–38), a prototypical class of MDM2 antagonists specifically designed to block the interaction between MDM2 and p53 (32, 33) (Table 1). DDLPS cells harboring wt p53 exhibited a higher responsiveness to MDM2 antagonists belonging to different chemical classes (i.e. nutlin3a, idasanutlin, siremadlin) (Table 1) with respect to p53-mutant cell lines (35, 48). A comparative study demonstrated that the higher effectiveness of the MDM2 inhibitor SAR405838 (Table 1), compared to its analogue MI-219 and nutlin 3a in inhibiting DDLPS cell growth and inducing apoptosis, relied on the presence of both wt p53 and MDM2 amplification (49). Gene expression analyses implicated restoration of the p53 pathway and reactivation of pro-apoptotic genes in the potent antitumor activity of SAR405838 against a DDLPS xenograft. The MDM2-p53 inhibitor BI-907828 (Table 1) induced tumor regression in two DDLPS PDX models with long lasting effect and a complete pathological response in one of them (50).
Inhibition of histone deacetylases can affect MDM2 expression/function by acting at both transcriptional and post-translational levels (51, 52). Indeed, in DDLPS models, MDM2 downmodulation induced by histone deacetylase inhibitors such as vorinostat and romidepsin has been associated with antitumor activity in vitro and in vivo (35, 53). Interestingly, the antitumor activity of the proteasome inhibitor bortezomib against a DDLPS PDX was also associated with MDM2 downmodulation (54).
Preclinical studies suggested rationale-based drug combinations to improve the antitumor efficacy of MDM2-targeting agents. Given the aberrant activation of the PI3K/AKT/mTOR pro-survival pathway observed in DDLPS (55), idasanutlin was tested in combination with the PI3K/mTOR inhibitor NVP-BEZ235 resulting in enhanced cell growth inhibition, apoptotic cell death, and reduction of tumor growth rate (38). Roy et al. (48) described a p53-dependent paradoxical activation of ERK pathway as a mechanism of resistance triggered by idasanutlin. Mechanistically, reactive oxygen species production, due to drug-induced mitochondrial translocation of p53, promotes the activation of receptor tyrosine kinases (RTKs) (e.g. IGF1R, PDGFRβ) and the downstream MEK-1/ERK pathway. The combination of MDM2 antagonists with the MEK1/2 inhibitor GSK112021B synergistically inhibited cell growth and potentiated apoptotic cell death. In mice bearing wt p53 DDLPS xenografts, this drug combination decreased tumor growth and increased survival.
CDK4 encodes cyclin-dependent kinase 4, which allows G1/S cell cycle progression by phosphorylating Rb. The clinical availability of CDK4/6 inhibitors fostered exploration of CDK4 as therapeutic target in DDLPS (39–41). Both genetic and pharmacological downmodulation of CDK4 inhibited DDLPS cell proliferation inducing cell cycle arrest, apoptosis, and antitumor activity in vivo (34, 38, 56, 57). The CDK4 inhibitor ribociclib significantly delayed DDLPS xenograft growth, an effect associated with decreased Rb phosphorylation, BrdU incorporation and 18F-Fluorodeoxy-D-glucose uptake (56). CDK4/6 inhibitors can induce a senescent-like cell state which could variably impact tumor growth/progression (58). In WD/DDLPS cell lines, CDK4 inhibitors (i.e. palbociclib, ribociclib, abemaciclib) (Table 1) induced senescence associated with accumulation of unphosphorylated Rb and post-translational downregulation of MDM2 (57). MDM2 loss was directly implicated in the senescent program triggered by treatment with CDK4 inhibitors. Notably, a lower expression of MDM2 in tumor biopsies after treatment with palbociclib was associated with tumor response. These findings suggested a favorable interaction between CDK4 inhibitors and agents affecting MDM2 expression/function. Indeed, palbociclib sensitized DDLPS cells to apoptosis induced by idasanutlin through promoting a full activation of the p53 pathway (38). Moreover, this combination reduced DDLPS xenografts growth and improved mice survival. The enhanced antitumor activity observed upon co-treatment with suboptimal doses of ribociclib and siremadlin prompted the clinical evaluation of this combination (59) (Table 2).
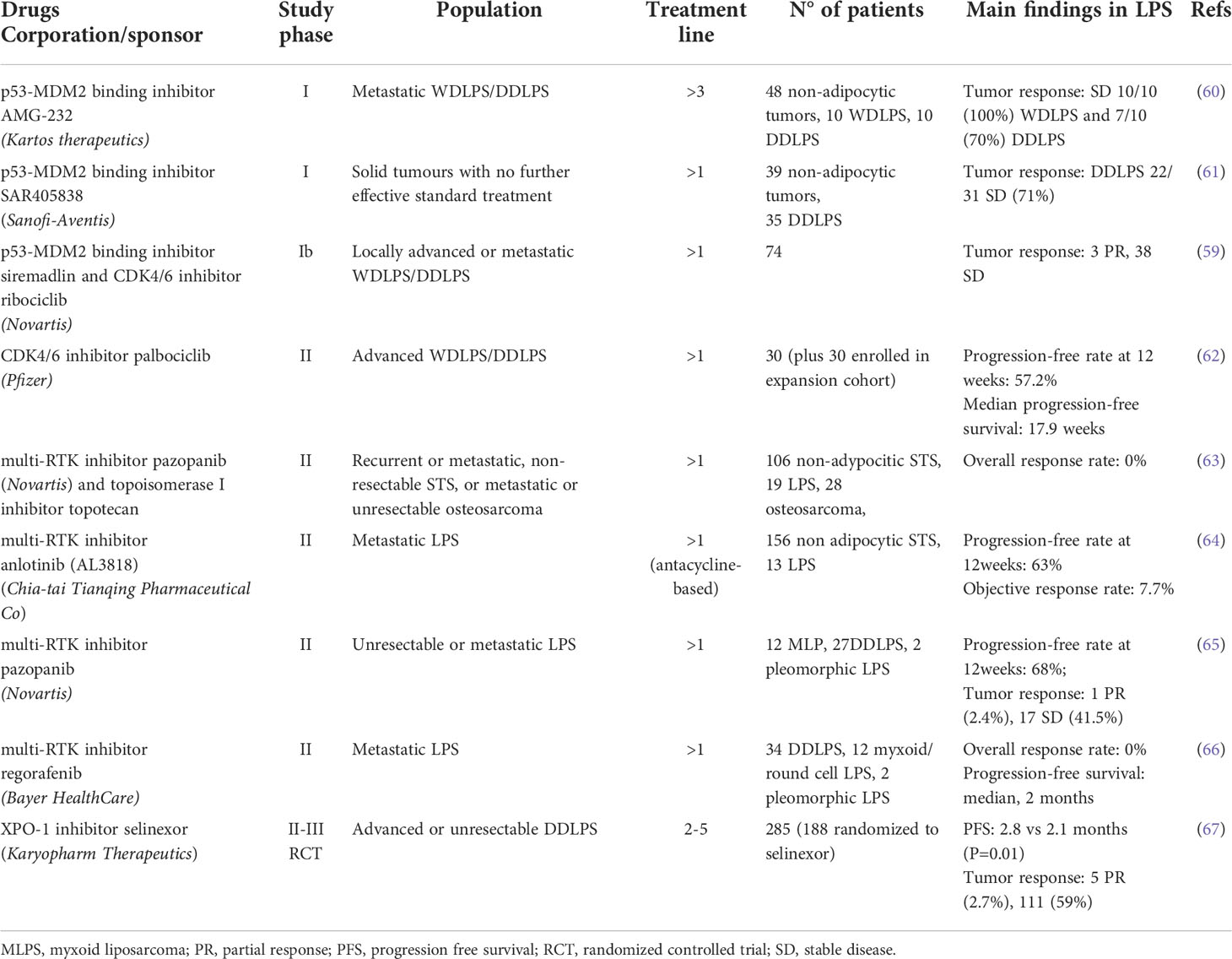
Table 2 Summary of results from clinical trials that tested innovative treatment options for patients with metastatic WDLPS and DDLPS.
Additional preclinical studies suggested combination strategies including palbociclib and anti-IGF1R agents (e.g. R1507 or NVP-AEW541) which resulted in enhanced inhibition of cell cycle progression and metabolic activity (68). The combination of palbociclib with recombinant methionase heavily impacted tumor cell dependence on methionine in an orthotopic DDLPS PDX model resistant to doxorubicin (69).
Published findings of early clinical trials investigating MDM2 and CDK4 inhibitors in WD/DDLPS patients are summarized in Table 2 (59–62).
Potential targets and investigational therapies
Receptor tyrosine kinases
Growth factor/RTKs drive and coordinate adipocytic differentiation by transducing stimulatory or inhibitory signals depending on multiple endogenous and environmental factors (70–72). The deregulated expression/function in LPS development and progression suggested some RTK axes as potential actionable targets. MET, AXL, KIT, and IGF1R were found overexpressed in WD/DDLPS cells versus normal adipocytes and pre-adipocytes (73) and genetic analyses evidenced common amplifications of IGF2, IGF1R, ERBB3 together with FGFR1, FGFR3 and PDGFR in WD/DDLPS (74–76). The more extensive amplification of genes encoding RTKs in DD than in WD components corroborated their role in promoting disease progression and the therapeutic potential of their targeting.
Overexpression and rare mutations of FGFRs, along with amplification of the adaptor protein FRS2 and the autocrine production of FGFs, contribute to the aberrant activation of the FGFR pathway in DDLPS (31, 74–82). Consistently, FGFR1 and/or FGFR4 overexpression has been associated with shorter disease-free survival and overall survival in WD/DDLPS patients (79). Treatment of DDPLS cells with the FGFR inhibitor erdafitinib (42, 43) (Table 1) reduced cell viability and induced apoptosis. Moreover, combination of erdafitinib with idasanutlin resulted in a synergistic antiproliferative effect, enhanced apoptosis and reduced DDLPS xenograft growth rate. In a case report, a patient with metastatic DDLPS refractory to multiple lines of conventional and targeted therapies experienced disease stabilization after erdafitinib treatment. These findings suggested FGFR1/FGFR4 expression as predictive biomarker in clinical trials investigating FGFR inhibitors.
The FGFR inhibitors infigratinib and LY2874455 (43, 44) exhibited in vitro and in vivo antitumor activity in a FRS2-amplified DDLPS experimental model originated from a high-grade metastatic tumor unresponsive to several conventional chemotherapeutics as well as to the MDM2 inhibitor RG7112 and palbociclib (Table 1) (81, 82). Differently from FRS2 amplification, expression of FGFR signaling components (e.g. FGFs, FGFRL1) was suggested to modulate cell response to FGFR inhibitors. Moreover, activation of FGF/FGFR signaling is markedly affected by the co-accessory molecules heparan sulfate proteoglycans (HSPGs) (83, 84). In particular, the HSPG syndecan-1 was demonstrated to promote proliferation and inhibit differentiation of adipocyte progenitors (85, 86). Syndecan-1 was found overexpressed in DDPLS compared with normal adipose tissue and lipomas and its expression was controlled by FGF, a circuit that the FGFR inhibitor PD173074 could interrupt (86). FGF2 exerts a biphasic effect on adipogenesis with low concentrations enhancing adipogenesis (87). Moreover, the activity of adipokines such as FGF21 could be affected by the expression of the coreceptor Klotho which was found significantly reduced in DDLPS compared to healthy adipose tissue (88, 89). Notably, high levels of Klotho were associated with better survival in LPS patients (89). Klotho also modulates the insulin/IGF1 signaling (90), another positive regulator of adipocytic diffentiation (70, 71). Klotho overexpression reduced IGF1R signaling, decreased DDLPS cell proliferation and promoted gemcitabine-induced apoptosis (89). Analogously, the IGF1R inhibitor BMS-754807 increased gemcitabine-induced cell death confirming the implication of the RTK in DDLPS drug resistance. A combinatorial drug screening identified several synergistic target pairs in a DDLPS cell line including EGFR and IGF1R, IGF1R and CDK4, IGF1R and EGFR, IGF1R and STAT3. The combination of anti-IGF1R agents (e.g. R1507 or NVP-AEW541) with CDK4 inhibitors cooperatively suppressed the activation of proteins within the crucial AKT pathway (68).
The constitutive or HGF-induced Met activation enhanced DDLPS cell proliferation, migration and invasion. Accordingly, MET knockdown or pharmacological targeting by the Met inhibitors SU11274 and tepotinib (91) (Table 1) reduced DDPLS tumorigenic potential in vitro and in vivo. A high-throughput drug screening evidenced the antiproliferative activity of the Met inhibitors foretinib and crizotinib (45) (Table 1) on a panel of PDX-derived DDLPS cell lines (54). Interestingly, death receptor upregulation induced by Met inhibitor PHA-665752 pretreatment enhanced the antiproliferative and pro-apoptotic activity of TRAIL in DDLPS cell lines (92).
Inhibition of RTKs present on tumor and microenvironment cells was suggested to partecipate in the antitumor activity of multi-targeting RTK inhibitors (i.e. ponatinib, apatinib pazopanib) in DDPLS models (Table 1). Angiogenesis inhibition contributed to DDLPS PDX growth delay induced by pazopanib alone and in combination with doxorubicin (43, 45, 93–95). Clinical trials of anlotinib and pazopanib have been recently reported (Table 2) (63–65).
Additional investigational therapies
WD/DDLPS are characterized by MDM2 overexpression associated with wild-type p53. MDM2-mediated ubiquitination downmodulates the tumor suppression function of p53 by promoting its nuclear export through exportin-1 (XPO1) and degradation (96). XPO1 overexpression, observed in LPS samples and cell lines (97), was recently confirmed in samples comprising the WD and DD components of primary tumors as well as the normal adipose tissue from DDLPS patients (98). These findings support targeting the nuclear export as a rational therapeutic approach for WD/DDLPS. The selective XPO1 inhibitor selinexor (46) (Table 1) decreased DDLPS cell growth by inducing cell cycle arrest and apoptosis (97, 98). Mechanistic studies showed that selinexor inhibited the IGF1R/AKT pathway activation by upregulating the expression of IGF binding protein 5 which acts as a tumor suppressor in DDLPS cells (97). Cell response to this drug was associated with a decrease of the survivin anti-apoptotic cytoplasmic pool (98). Selinexor significantly reduced the growth of a tumor xenograft from an established DDLPS cell line (97) and showed a moderate activity, anyway higher than doxorubicin, in three DDLPS PDXs displaying myogenic and rhabdomyoblastic heterologous differentiation (98). A phase III trials showed a small, though statistically significant, benefit for selinexor compared with placebo, suggesting also absence of the calcium and MDM2 binding protein CALB1 as a predictive biomarker for longer progression-free survival (67) (Table 2).
Recent reports highlighted the potential of the mitotic serine-threonine kinase Aurora A (AURKA) as therapeutic target. AURKA was found significantly upregulated in DDLPS compared to WDLPS and patients with high AURKA expression in tumors showed shorter recurrence-free survival (99). AURKA knockout and enzyme blockade by the inhibitors alisertib and AMG900 induced DDLPS cell cycle arrest and apoptosis (47) (Table 1). Alisertib also efficiently suppressed tumor xenograft growth (100, 101). Of note, DDLPS cell lines displayed heterogeneous sensitivity to AURKA/B inhibitors either alone or in combination with cytotoxic chemotherapeutics likely related to different tumor cell molecular characteristics (99–101).
Discussion
Therapeutic options for WD/DDLPS are limited and patient outcomes remain unsatisfactory. The pathognomonic amplification of genes implicated in cell cycle and growth control has provided the rational bases for clinical evaluation of targeting agents. Despite high expectations, first reports recorded modest benefit from MDM2 and CDK4 inhibitors as single agents. MDM2 inhibitors evaluated in phase I/II trials showed disease stabilization in WDLPS and DDLPS (Table 2) (60, 61). The CDK4 inhibitor palbociclib resulted in favorable PFS and occasional tumor responses in a phase II trial for advanced WD/DDLPS (62). However, a real world experience in retroperitoneal diseases showed very limited clinical activity of single-agent palbociclib (102). Recently, trials are combining MDM2 and CDK4 inhibitors. A phase Ib study testing the combination of siremadlin and ribociclib recorded stable disease and manageable treatment-related toxicity supporting the feasibility of this approach (Table 2) (59).
Other recent trials have reported on pazopanib and other TKI inhibitors for liposarcomas (63–66) (Table 2). Extensive genomic analyses of WD/DDLPS and functional studies are currently actively exploring additional targets.
Among the recurrently amplified genes in WD/DDLPS, HMGA2 deserves deeper investigation. In addition to its role as an oncoprotein associated with aberrant expression in several tumor types, HMGA2 promotes a cancer stem cell phenotype, chemoresistance, and is involved in adipogenesis at the clonal expansion step from preadipocytes to adipocytes (103–107). HMGA2 transcript is overexpressed, or implicated in gene fusions, in DDLPS significantly more frequently than in their paired WDLPS samples (7). Efforts to identify HMGA2 inhibitors are ongoing (108, 109). Nonetheless, high levels of MDM2 concurrent with low HMGA2 amplification did correlate with low overall survival (110). The severe DDLPS rhabdomioblastic variant may harbor low HMGA2 amplification, which may have therapeutic implications as a lower expression of HMGA2 may result in higher drug sensitivity (98). In-depth investigation on the role of genetic alterations of HMGA2, and its relation with other players in DDLPS oncogenesis/progression, is needed to decipher its therapeutic relevance.
Recent insights into the WD/DDLPS molecular characteristics revealed an intrinsic heterogeneity reflecting early dedifferentiation and genomic instability. Although the mechanisms underlying dedifferentiation are not fully understood, additional genetic alterations, beyond already knew gene amplifications, may provide potential targets. Changes associated with progression from WDLPS to DDLPS include rearrangements of several chromosomal regions. Fusion transcripts appear more frequent in DDLPS than WDLPS (7) although few information exists about their association with histologic characteristics. Importantly, some of them are potentially druggable, such as fusions involving NTRK or MAP2K6 genes described in case reports (111, 112).
Differentiation therapy may provide new therapeutic opportunities for DDLPS. Agonists of PPARγ, a key effector of adipogenesis, were shown to be effective inducers of re-differentiation in LPS preclinical models and clinical samples (113–115). Currently, while early clinical trials provided mixed results (116, 117), other trials of PPARγ ligands are underway in LPS patients. PPARγ is under the repressive control of the FUS-CHOP fusion oncogene in mixoid liposarcoma. Interestingly, the ability of trabectedin to displace FUS-CHOP from its targets promoters provided the rationale for the clinical evaluation of the PPARγ agonist pioglitazone (115). Although such differentiating approach appears of particular interest for mixoid liposarcoma, the maintenance of the adipocytic program could be pharmacologically exploited in at least subsets of DDLPS (73, 118).
Therapeutic progresses in LPS have been hampered by the disease rarity and the consequent challenge in designing prospective clinical trials and establishing predictive experimental models. In recent years, PDX models of WD/DDLPS, recapitulating tumor histology, biology and genetics, have been developed providing the opportunity to explore novel therapeutic approaches and relevant biomarkers (54, 98, 119). Patient-derived cell and xenograft models represent valuable tools to assess the therapeutic relevance of molecular alterations that, besides chromosomal amplifications shared by WDLPS and DDLPS, drive liposarcomagenesis and disease progression. Integrated “multi-omics” investigation will contribute to identify novel druggable vulnerabilities and synthetic lethal drug combinations eventually enhancing the development of innovative, biology-driven, effective treatments for WD/DDLPS patients.
Author contributions
GC, SP and CL contributed to conception and design of the review. All authors contributed to the article and approved the submitted version.
Funding
The authors acknowledge the support by “5x1000 Founds”, 2016 Italian Ministry of Health; Institutional grant BRI2017, GR-2019-12369175; FRRB-584-797,60; International Accelerator Award funded by AIRC [ID #24297]/Cancer Research UK [C56167/A29363]/Fundacion Científica, Asociacion Espanola Contra el Cancer [Foundation AECC-GEACC19007MA].
Conflict of interest
The authors declare that the research was conducted in the absence of any commercial or financial relationships that could be construed as a potential conflict of interest.
Publisher’s note
All claims expressed in this article are solely those of the authors and do not necessarily represent those of their affiliated organizations, or those of the publisher, the editors and the reviewers. Any product that may be evaluated in this article, or claim that may be made by its manufacturer, is not guaranteed or endorsed by the publisher.
References
1. The WHO classification of tumours editorial board. “Solid Tissue Tumors”. In: WHO classification of tumours 5th edition - soft tissue and bone tumours, (2020) International Agency for Research on Cancer (IARC) Press, Lyon, France, 3:2–12.
2. Gronchi A, Strauss DC, Miceli R, Bonvalot S, Swallow CJ, Hohenberger P, et al. Variability in patterns of recurrence after resection of primary retroperitoneal sarcoma (RPS): A report on 1007 patients from the multi-institutional collaborative RPS working group. Ann Surg (2016) 263(5):1002–9. doi: 10.1097/SLA.0000000000001447
3. Tan MC, Brennan MF, Kuk D, Agaram NP, Antonescu CR, Qin LX, et al. Histology-based classification predicts pattern of recurrence and improves risk stratification in primary retroperitoneal sarcoma. Ann Surg (2016) 263(3):593–600. doi: 10.1097/SLA.0000000000001149
4. Gootee J, Aurit S, Curtin C, Silberstein P. Primary anatomical site, adjuvant therapy, and other prognostic variables for dedifferentiated liposarcoma. J Cancer Res Clin Oncol (2019) 145(1):181–92. doi: 10.1007/s00432-018-2777-3
5. Dei Tos AP. Liposarcoma: New entities and evolving concepts. Ann Diagn Pathol (2000) 4(4):252–66. doi: 10.1053/adpa.2000.8133
6. Conyers R, Young S, Thomas DM. Liposarcoma: Molecular genetics and therapeutics. Sarcoma (2011) 2011:483154. doi: 10.1155/2011/483154
7. Beird HC, Wu CC, Ingram DR, Wang WL, Alimohamed A, Gumbs C, et al. Genomic profiling of dedifferentiated liposarcoma compared to matched well-differentiated liposarcoma reveals higher genomic complexity and a common origin. Cold Spring Harb Mol Case Stud (2018) 4(2):a002386. doi: 10.1101/mcs.a002386
8. Nishio J, Nakayama S, Nabeshima K, Yamamoto T. Biology and management of dedifferentiated liposarcoma: State of the art and perspectives. J Clin Med (2021) 10(15):3230. doi: 10.3390/jcm10153230
9. Gronchi A, Collini P, Miceli R, Valeri B, Renne SL, Dagrada G, et al. Myogenic differentiation and histologic grading are major prognostic determinants in retroperitoneal liposarcoma. Am J Surg Pathol (2015) 39(3):383–93. doi: 10.1097/PAS.0000000000000366
10. Kurzawa P, Mullen JT, Chen YL, Johnstone SE, Deshpande V, Chebib I, et al. Prognostic value of myogenic differentiation in dedifferentiated liposarcoma. Am J Surg Pathol (2020) 44(6):799–804. doi: 10.1097/PAS.0000000000001436
11. Dorian Yarih GO, Claudia Hs CS, Alethia AC, Mario AB, Emmanuel ME, Ernesto RA. Myogenic dedifferentiation is associated with poor outcomes in retroperitoneal dedifferentiated liposarcomas. Rare Tumors (2021) 13:1–6. doi: 10.1177/2036361320986655
12. Makise N, Yoshida A, Komiyama M, Nakatani F, Yonemori K, Kawai A, et al. Dedifferentiated liposarcoma with Epithelioid/Epithelial features. Am J Surg Pathol (2017) 41(11):1523–31. doi: 10.1097/PAS.0000000000000910
13. Bonvalot S, Gronchi A, Le Péchoux C, Swallow CJ, Strauss D, Meeus P, et al. Preoperative radiotherapy plus surgery versus surgery Alone for patients with primary retroperitoneal sarcoma (EORTC-62092: STRASS): a multicentre, open-label, randomised, phase 3 trial. Lancet Oncol (2020) 21(10):1366–77. doi: 10.1016/S1470-2045(20)30446-0
14. Gahvari Z, Parkes A. Dedifferentiated liposarcoma: Systemic therapy options. Curr Treat Options Oncol (2020) 21(2):15. doi: 10.1007/s11864-020-0705-7
15. Stacchiotti S, van der Graaf WTA, Sanfilippo RG, Marreaud SI, Van Houdt WJ, Judson IR, et al. First-line chemotherapy in advanced intrabdominal well-differentiated/dedifferentiated liposarcoma: An EORTC soft tissue and bone sarcoma group retrospective analysis. Cancer (2022) 128(15):2932–8. doi: 10.1002/cncr.34264
16. Crago AM, Singer S. Clinical and molecular approaches to well differentiated and dedifferentiated liposarcoma. Curr Opin Oncol (2011) 23(4):373–8. doi: 10.1097/CCO.0b013e32834796e6
17. Thway K. Well-differentiated liposarcoma and dedifferentiated liposarcoma: An updated review. Semin Diagn Pathol (2019) 36(2):112–21. doi: 10.1053/j.semdp.2019.02.006
18. Casadei L, de Faria FCC, Lopez-Aguiar A, Pollock RE, Grignol V. Targetable pathways in the treatment of retroperitoneal liposarcoma. Cancers (2022) 14(6):1362. doi: 10.3390/cancers14061362
19. Gamboa AC, Gronchi A, Cardona K. Soft-tissue sarcoma in adults: An update on the current state of histiotype-specific management in an era of personalized medicine. CA Cancer J Clin (2020) 70(3):200–29. doi: 10.3322/caac.21605
20. McGovern Y, Zhou CD, Jones RL. Systemic therapy in metastatic or unresectable well-Differentiated/Dedifferentiated liposarcoma. Front Oncol (2017) 7:292. doi: 10.3389/fonc.2017.00292
21. Sanfilippo R, Bertulli R, Marrari A, Fumagalli E, Pilotti S, Morosi C, et al. High-dose continuous-infusion ifosfamide in advanced well-differentiated/dedifferentiated liposarcoma. Clin Sarcoma Res (2014) 4(1):16. doi: 10.1186/2045-3329-4-16
22. Maki RG, Wathen JK, Patel SR, Priebat DA, Okuno SH, Samuels B, et al. Randomized phase II study of gemcitabine and docetaxel compared with gemcitabine alone in patients with metastatic soft tissue sarcomas: Results of sarcoma alliance for research through collaboration study 002. J Clin Oncol (2007) 25(19):2755–63. doi: 10.1200/JCO.2006.10.4117
23. Frezza AM, Stacchiotti S, Gronchi A. Systemic treatment in advanced soft tissue sarcoma: What is standard, what is new. BMC Med (2017) 15(1):109. doi: 10.1186/s12916-017-0872-y
24. Demetri GD, von Mehren M, Jones RL, Hensley ML, Schuetze SM, Staddon A, et al. Efficacy and safety of trabectedin or dacarbazine for metastatic liposarcoma or leiomyosarcoma after failure of conventional chemotherapy: Results of a phase III randomized multicenter clinical trial. J Clin Oncol (2016) 34(8):786–93. doi: 10.1200/JCO.2015.62.4734
25. Schöffski P, Chawla S, Maki RG, Italiano A, Gelderblom H, Choy E, et al. Eribulin versus dacarbazine in previously treated patients with advanced liposarcoma or leiomyosarcoma: A randomised, open-label, multicentre, phase 3 trial. Lancet (2016) 387(10028):1629–37. doi: 10.1016/S0140-6736(15)01283-0
26. Patel S, von Mehren M, Reed DR, Kaiser P, Charlson J, Ryan CW, et al. Overall survival and histology-specific subgroup analyses from a phase 3, randomized controlled study of trabectedin or dacarbazine in patients with advanced liposarcoma or leiomyosarcoma. Cancer (2019) 125(15):2610–20. doi: 10.1002/cncr.32117
27. Demetri GD, Schöffski P, Grignani G, Blay JY, Maki RG, Van Tine BA, et al. Activity of eribulin in patients with advanced liposarcoma demonstrated in a subgroup analysis from a randomized phase III study of eribulin versus dacarbazine. J Clin Oncol (2017) 35(30):3433–9. doi: 10.1200/JCO.2016.71.6605
28. Fabbroni C, Fucà G, Ligorio F, Fumagalli E, Barisella M, Collini P, et al. Impact of pathological stratification on the clinical outcomes of advanced well-Differentiated/Dedifferentiated liposarcoma treated with trabectedin. Cancers (2021) 13(6):1453. doi: 10.3390/cancers13061453
29. Phillips E, Jones RL, Huang P, Digklia A. Efficacy of eribulin in soft tissue sarcomas. Front Pharmacol (2022) 13:869754. doi: 10.3389/fphar.2022.869754
30. Pilotti S, Della Torre G, Lavarino C, Sozzi G, Minoletti F, Vergani B, et al. Molecular abnormalities in liposarcoma: Role of MDM2 and CDK4-containing amplicons at 12q13-22. J Pathol (1998) 185(2):188–90. doi: 10.1002/(SICI)1096-9896(199806)185:2<188::AID-PATH53>3.0.CO;2-2
31. Cancer Genome Atlas Research Network, Cancer Genome Atlas Research Network. Comprehensive and integrated genomic characterization of adult soft tissue sarcomas. Cell (2017) 171(4):950–65.e28. doi: 10.1016/j.cell.2017.10.014
32. Munisamy M, Mukherjee N, Thomas L, Pham AT, Shakeri A, Zhao Y, et al. Therapeutic opportunities in cancer therapy: Targeting the p53-MDM2/MDMX interactions. Am J Cancer Res (2021) 11(12):5762–81.
33. Konopleva M, Martinelli G, Daver N, Papayannidis C, Wei A, Higgins B, et al. MDM2 inhibition: An important step forward in cancer therapy. Leukemia (2020) 34(11):2858–74. doi: 10.1038/s41375-020-0949-z
34. Barretina J, Taylor BS, Banerji S, Ramos AH, Lagos-Quintana M, Decarolis PL, et al. Subtype-specific genomic alterations define new targets for soft-tissue sarcoma therapy. Nat Genet (2010) 42(8):715–21. doi: 10.1038/ng.619
35. Ou WB, Zhu J, Eilers G, Li X, Kuang Y, Liu L, et al. HDACi inhibits liposarcoma via targeting of the MDM2-p53 signaling axis and PTEN, irrespective of p53 mutational status. Oncotarget (2015) 6(12):10510–20. doi: 10.18632/oncotarget.3230
36. Müller CR, Paulsen EB, Noordhuis P, Pedeutour F, Saeter G, Myklebost O. Potential for treatment of liposarcomas with the MDM2 antagonist nutlin-3A. Int J Cancer (2007) 121(1):199–205. doi: 10.1002/ijc.22643
37. Singer S, Socci ND, Ambrosini G, Sambol E, Decarolis P, Wu Y, et al. Gene expression profiling of liposarcoma identifies distinct biological types/subtypes and potential therapeutic targets in well-differentiated and dedifferentiated liposarcoma. Cancer Res (2007) 67(14):6626–36. doi: 10.1158/0008-5472.CAN-07-0584
38. Laroche-Clary A, Chaire V, Algeo MP, Derieppe MA, Loarer FL, Italiano A. Combined targeting of MDM2 and CDK4 is synergistic in dedifferentiated liposarcomas. J Hematol Oncol (2017) 10(1):123. doi: 10.1186/s13045-017-0482-3
39. Schettini F, De Santo I, Rea CG, De Placido P, Formisano L, Giuliano M, et al. CDK 4/6 inhibitors as single agent in advanced solid tumors. Front Oncol (2018) 8:608. doi: 10.3389/fonc.2018.00608
40. Goel S, Bergholz JS, Zhao JJ. Targeting CDK4 and CDK6 in cancer. Nat Rev Cancer (2022) 22(6):356–72. doi: 10.1038/s41568-022-00456-3
41. Qi J, Ouyang Z. Targeting CDK4/6 for anticancer therapy. Biomedicines (2022) 10(3):685. doi: 10.3390/biomedicines10030685
42. Katoh M. Fibroblast growth factor receptors as treatment targets in clinical oncology. Nat Rev Clin Oncol (2019) 16(2):105–22. doi: 10.1038/s41571-018-0115-y
43. Roskoski R Jr. The role of fibroblast growth factor receptor (FGFR) protein-tyrosine kinase inhibitors in the treatment of cancers including those of the urinary bladder. Pharmacol Res (2020) 151:104567. doi: 10.1016/j.phrs.2019.104567
44. Ornitz DM, Itoh N. New developments in the biology of fibroblast growth factors. WIREs Mech Dis (2022) 14(4):e1549. doi: 10.1002/wsbm.1549
45. Zhong L, Li Y, Xiong L, Wang W, Wu M, Yuan T, et al. Small molecules in targeted cancer therapy: Advances, challenges, and future perspectives. Signal Transduct Target Ther (2021) 6(1):201. doi: 10.1038/s41392-021-00572-w
46. Azmi AS, Uddin MH, Mohammad RM. The nuclear export protein XPO1 - from biology to targeted therapy. Nat Rev Clin Oncol (2021) 18(3):152–69. doi: 10.1038/s41571-020-00442-4
47. Novais P, Silva PMA, Amorim I, Bousbaa H. Second-generation antimitotics in cancer clinical trials. Pharmaceutics (2021) 13:1011. doi: 10.3390/pharmaceutics13071011
48. Roy S, Laroche-Clary A, Verbeke S, Derieppe MA, Italiano A. MDM2 antagonists induce a paradoxical activation of Erk1/2 through a P53-dependent mechanism in dedifferentiated liposarcomas: Implications for combinatorial strategies. Cancers (2020) 12(8):2253. doi: 10.3390/cancers12082253
49. Bill KL, Garnett J, Meaux I, Ma X, Creighton CJ, Bolshakov S, et al. SAR405838: A novel and potent inhibitor of the MDM2:p53 axis for the treatment of dedifferentiated liposarcoma. Clin Cancer Res (2016) 22(5):1150–60. doi: 10.1158/1078-0432.CCR-15-1522
50. Cornillie J, Wozniak A, Li H, Gebreyohannes YK, Wellens J, Hompes D, et al. Anti-tumor activity of the MDM2-TP53 inhibitor BI-907828 in dedifferentiated liposarcoma patient-derived xenograft models harboring MDM2 amplification. Clin Transl Oncol (2020) 22(4):546–54. doi: 10.1007/s12094-019-02158-z
51. Mrakovcic M, Kleinheinz J, Fröhlich LF. p53 at the crossroads between different types of HDAC inhibitor-mediated cancer cell death. Int J Mol Sci (2019) 20(10):2415. doi: 10.3390/ijms20102415
52. Wienken M, Moll UM, Dobbelstein M. Mdm2 as a chromatin modifier. J Mol Cell Biol (2017) 9(1):74–80. doi: 10.1093/jmcb/mjw046
53. Seligson ND, Stets CW, Demoret BW, Awasthi A, Grosenbacher N, Shakya R, et al. Inhibition of histone deacetylase 2 reduces MDM2 expression and reduces tumor growth in dedifferentiated liposarcoma. Oncotarget (2019) 10(55):5671–9. doi: 10.18632/oncotarget.27144
54. Jo EB, Hong D, Lee YS, Lee H, Park JB, Kim SJ. Establishment of a novel PDX mouse model and evaluation of the tumor suppression efficacy of bortezomib against liposarcoma. Transl Oncol (2019) 12(2):269–81. doi: 10.1016/j.tranon.2018.09.015
55. Smith KB, Tran LM, Tam BM, Shurell EM, Li Y, Braas D, et al. Novel dedifferentiated liposarcoma xenograft models reveal PTEN down-regulation as a malignant signature and response to PI3K pathway inhibition. Am J Pathol (2013) 182(4):1400–11. doi: 10.1016/j.ajpath.2013.01.002
56. Zhang YX, Sicinska E, Czaplinski JT, Remillard SP, Moss S, Wang Y, et al. Antiproliferative effects of CDK4/6 inhibition in CDK4-amplified human liposarcoma in vitro and in vivo. Mol Cancer Ther (2014) 13(9):2184–93. doi: 10.1158/1535-7163.MCT-14-0387
57. Kovatcheva M, Liu DD, Dickson MA, Klein ME, O'Connor R, Wilder FO, et al. MDM2 turnover and expression of ATRX determine the choice between quiescence and senescence in response to CDK4 inhibition. Oncotarget (2015) 6(10):8226–43. doi: 10.18632/oncotarget.3364
58. Barr AR, McClelland SE. Cells on lockdown: long-term consequences of CDK4/6 inhibition. EMBO J (2022) 41(6):e110764. doi: 10.15252/embj.2022110764
59. Abdul Razak AR, Bauer S, Suarez C, Lin CC, Quek R, Hütter-Krönke ML, et al. Co-Targeting of MDM2 and CDK4/6 with siremadlin and ribociclib for the treatment of patients with well-differentiated or dedifferentiated liposarcoma: Results from a proof-of-Concept, phase ib study. Clin Cancer Res (2022) 28(6):1087–97. doi: 10.1158/1078-0432.CCR-21-1291
60. Gluck WL, Gounder MM, Frank R, Eskens F, Blay JY, Cassier PA, et al. Phase 1 study of the MDM2 inhibitor AMG 232 in patients with advanced P53 wild-type solid tumors or multiple myeloma. Invest N Drugs (2020) 38(3):831–43. doi: 10.1007/s10637-019-00840-1
61. de Jonge M, de Weger VA, Dickson MA, Langenberg M, Le Cesne A, Wagner AJ, et al. A phase I study of SAR405838, a novel human double minute 2 (HDM2) antagonist, in patients with solid tumours. Eur J Cancer (2017) 76:144–51. doi: 10.1016/j.ejca.2017.02.005
62. Dickson MA, Schwartz GK, Keohan ML, D'Angelo SP, Gounder MM, Chi P, et al. Progression-free survival among patients with well-differentiated or dedifferentiated liposarcoma treated with CDK4 inhibitor palbociclib: A phase 2 clinical trial. JAMA Oncol (2016) 2(7):937–40. doi: 10.1001/jamaoncol.2016.0264
63. Schulte B, Mohindra N, Milhem M, Attia S, Robinson S, Monga V, et al. Phase II study of pazopanib with oral topotecan in patients with metastatic and non-resectable soft tissue and bone sarcomas. Br J Cancer (2021) 125(4):528–33. doi: 10.1038/s41416-021-01448-0
64. Chi Y, Fang Z, Hong X, Yao Y, Sun P, Wang G, et al. Safety and efficacy of anlotinib, a multikinase angiogenesis inhibitor, in patients with refractory metastatic soft-tissue sarcoma. Clin Cancer Res (2018) 24(21):5233–8. doi: 10.1158/1078-0432.CCR-17-3766
65. Samuels BL, Chawla SP, Somaiah N, Staddon AP, Skubitz KM, Milhem MM, et al. Results of a prospective phase 2 study of pazopanib in patients with advanced intermediate-grade or high-grade liposarcoma. Cancer (2017) 123(23):4640–7. doi: 10.1002/cncr.30926
66. Riedel RF, Ballman KV, Lu Y, Attia S, Loggers ET, Ganjoo KN, et al. A randomized, double-blind, placebo-controlled, phase II study of regorafenib versus placebo in Advanced/Metastatic, treatment-refractory liposarcoma: Results from the SARC024 study. Oncologist (2020) 25(11):e1655–62. doi: 10.1634/theoncologist.2020-0679
67. Gounder MM, Razak AA, Somaiah N, Chawla S, Martin-Broto J, Grignani G, et al. Selinexor in advanced, metastatic dedifferentiated liposarcoma: A multinational, randomized, double-blind, placebo-controlled trial. J Clin Oncol (2022) 40(22):2479–90. doi: 10.1200/JCO.21.01829
68. Miller ML, Molinelli EJ, Nair JS, Sheikh T, Samy R, Jing X, et al. Drug synergy screen and network modeling in dedifferentiated liposarcoma identifies CDK4 and IGF1R as synergistic drug targets. Sci Signal (2013) 6(294):ra85. doi: 10.1126/scisignal.2004014
69. Igarashi K, Kawaguchi K, Kiyuna T, Miyake K, Miyaki M, Yamamoto N, et al. Metabolic targeting with recombinant methioninase combined with palbociclib regresses a doxorubicin-resistant dedifferentiated liposarcoma. Biochem Biophys Res Commun (2018) 506(4):912–7. doi: 10.1016/j.bbrc.2018.10.119
70. Gregoire FM, Smas CM, Sook Sul H. Understanding adypocyte differentiation. . Physiol Rev (1998) 78(3):783–809. doi: 10.1152/physrev.1998.78.3.783
71. Niemelä S, Sarkanen JR, Ashammaki N. “Adipose tissue and adypocyte differentiation: molecular and cellular aspects and tissue engineering applications”. In: Ashammakhi N, Reis R, & Chiellini F, editors. Topics in tissue engineering, (2008) University of Oulu 4(4).
72. Ghaben AL, Scherer PE. Adipogenesis and metabolic health. Nat Rev Mol Cell Biol (2019) 20(4):242–58. doi: 10.1038/s41580-018-0093-z
73. Peng T, Zhang P, Liu J, Nguyen T, Bolshakov S, Belousov R, et al. An experimental model for the study of well-differentiated and dedifferentiated liposarcoma; Deregulation of targetable tyrosine kinase receptors. Lab Invest (2011) 91(3):392–403. doi: 10.1038/labinvest.2010.185
74. Kanojia D, Nagata Y, Garg M, Lee DH, Sato A, Yoshida K, et al. Genomic landscape of liposarcoma. Oncotarget (2015) 6(40):42429–44. doi: 10.18632/oncotarget.6464
75. Xu L, Xie X, Shi X, Zhang P, Liu A, Wang J, et al. Potential application of genomic profiling for the diagnosis and treatment of patients with sarcoma. Oncol Lett (2021) 21(5):353. doi: 10.3892/ol.2021.12614
76. Asano N, Yoshida A, Mitani S, Kobayashi E, Shiotani B, Komiyama M, et al. Frequent amplification of receptor tyrosine kinase genes in welldifferentiated/ dedifferentiated liposarcoma. Oncotarget (2017) 8(8):12941–52. doi: 10.18632/oncotarget.14652
77. Wang X, Asmann YW, Erickson-Johnson MR, Oliveira JL, Zhang H, Moura RD, et al. High-resolution genomic mapping reveals consistent amplification of the fibroblast growth factor receptor substrate 2 gene in well-differentiated and dedifferentiated liposarcoma. Genes Chromosomes Cancer (2011) 50(11):849–58. doi: 10.1002/gcc.20906
78. Zhang K, Chu K, Wu X, Gao H, Wang J, Yuan YC, et al. Amplification of FRS2 and activation of FGFR/FRS2 signaling pathway in high-grade liposarcoma. Cancer Res (2013) 73(4):1298–307. doi: 10.1158/0008-5472.CAN-12-2086
79. Dadone-Montaudié B, Laroche-Clary A, Mongis A, Chamorey E, Mauro ID, Chaire V, et al. Novel therapeutic insights in dedifferentiated liposarcoma: A role for FGFR and MDM2 dual targeting. Cancers (2020) 12(10):3058. doi: 10.3390/cancers12103058
80. Li C, Shen Y, Ren Y, Liu W, Li M, Liang W, et al. Oncogene mutation profiling reveals poor prognosis associated with FGFR1/3 mutation in liposarcoma. Hum Pathol (2016) 55:143–50. doi: 10.1016/j.humpath.2016.05.006
81. Hanes R, Grad I, Lorenz S, Stratford EW, Munthe E, Reddy CC, et al. Preclinical evaluation of potential therapeutic targets in dedifferentiated liposarcoma. Oncotarget (2016) 7(34):54583–95. doi: 10.18632/oncotarget.10518
82. Hanes R, Munthe E, Grad I, Han J, Karlsen I, McCormack E, et al. Preclinical evaluation of the pan-FGFR inhibitor LY2874455 in FRS2-amplified liposarcoma. Cells (2019) 8(2):189. doi: 10.3390/cells8020189
83. Belov AA, Mohammadi M. Molecular mechanisms of fibroblast growth factor signaling in physiology and pathology. Cold Spring Harb Perspect Biol (2013) 5(6):a015958. doi: 10.1101/cshperspect.a015958
84. Lanzi C, Cassinelli G. Receptor tyrosine kinases and heparan sulfate proteoglycans: Interplay providing anticancer targeting strategies and new therapeutic opportunities. Biochem Pharmacol (2020) 178:114084. doi: 10.1016/j.bcp.2020.114084
85. Yu C, Peall IW, Pham SH, Okolicsanyi RK, Griffiths LR, Haupt LM. Syndecan-1 facilitates the human mesenchymal stem cell osteo-adipogenic balance. Int J Mol Sci (2020) 21(11):3884. doi: 10.3390/ijms21113884
86. Zaragosi LE, Dadone B, Michiels JF, Marty M, Pedeutour F, Dani C, et al. Syndecan-1 regulates adipogenesis: New insights in dedifferentiated liposarcoma tumorigenesis. Carcinogenesis (2015) 36(1):32–40. doi: 10.1093/carcin/bgu222
87. Kim S, Ahn C, Bong N, Choe S, Lee DK. Biphasic effects of FGF2 on adipogenesis. PLoS One (2015) 10(3):e0120073. doi: 10.1371/journal.pone.0120073
88. Fan J, Sun Z. The antiaging gene klotho regulates proliferation and differentiation of adipose-derived stem cells. Stem Cells (2016) 34(6):1615–25. doi: 10.1002/stem.2305
89. Delcroix V, Mauduit O, Tessier N, Montillaud A, Lesluyes T, Ducret T, et al. The role of the anti-aging protein klotho in IGF-1 signaling and reticular calcium leak: Impact on the chemosensitivity of dedifferentiated liposarcomas. Cancers (2018) 10(11):439. doi: 10.3390/cancers10110439
90. Kurosu H, Yamamoto M, Clark JD, Pastor JV, Nandi A, Gurnani P, et al. Suppression of aging in mice by the hormone klotho. Science (2005) 309(5742):1829–33. doi: 10.1126/science.1112766
91. Bill KL, Garnett J, Ma X, May CD, Bolshakov S, Lazar AJ, et al. The hepatocyte growth factor receptor as a potential therapeutic target for dedifferentiated liposarcoma. Lab Invest (2015) 95(8):951–61. doi: 10.1038/labinvest.2015.62
92. Jo EB, Lee YS, Lee H, Park JB, Park H, Choi YL, et al. Combination therapy with c-met inhibitor and TRAIL enhances apoptosis in dedifferentiated liposarcoma patient-derived cells. BMC Cancer (2019) 19(1):496. doi: 10.1186/s12885-019-5713-2
93. Kanojia D, Garg M, Martinez J, Anand MT, Luty SB, Doan NB, et al. Kinase profiling of liposarcomas using RNAi and drug screening assays identified druggable targets. J Hematol Oncol (2017) 10(1):173. doi: 10.1186/s13045-017-0540-x
94. Cui L, Yan L, Guan X, Dong B, Zhao M, Lv A, et al. Anti-tumor effect of apatinib and relevant mechanisms in liposarcoma. Front Oncol (2021) 11:739139. doi: 10.3389/fonc.2021.739139
95. Li H, Wozniak A, Sciot R, Cornillie J, Wellens J, Van Looy T, et al. Pazopanib, a receptor tyrosine kinase inhibitor, suppresses tumor growth through angiogenesis in dedifferentiated liposarcoma xenograft models. Transl Oncol (2014) 7(6):665–71. doi: 10.1016/j.tranon.2014.09.007
96. Freedman DA, Levine AJ. Nuclear export is required for degradation of endogenous p53 by MDM2 and human papillomavirus E6. Mol Cell Biol (1998) 18(12):7288–93. doi: 10.1128/MCB.18.12.7288
97. Garg M, Kanojia D, Mayakonda A, Said JW, Doan NB, Chien W, et al. Molecular mechanism and therapeutic implications of selinexor (KPT-330) in liposarcoma. Oncotarget (2017) 8(5):7521–32. doi: 10.18632/oncotarget.13485
98. Zuco V, Pasquali S, Tortoreto M, Brich S, Percio S, Dagrada GP, et al. Selinexor versus doxorubicin in dedifferentiated liposarcoma PDXs: Evidence of greater activity and apoptotic response dependent on p53 nuclear accumulation and survivin down-regulation. J Exp Clin Cancer Res (2021) 40(1):83. doi: 10.1186/s13046-021-01886-x
99. Yen CC, Chen SC, Hung GY, Wu PK, Chua WY, Lin YC, et al. Expression profile−driven discovery of AURKA as a treatment target for liposarcoma. Int J Oncol (2019) 55(4):938–48. doi: 10.3892/ijo.2019.4861
100. Nair JS, Schwartz GK. MLN-8237: A dual inhibitor of aurora a and b in soft tissue sarcomas. Oncotarget (2016) 7(11):12893–903. doi: 10.18632/oncotarget.7335
101. Mattei JC, Bouvier-Labit C, Barets D, Macagno N, Chocry M, Chibon F, et al. Pan aurora kinase inhibitor: A promising targeted-therapy in dedifferentiated liposarcomas with differential efficiency depending on sarcoma molecular profile. Cancers (2020) 12(3):583. doi: 10.3390/cancers12030583
102. Nassif EF, Cope B, Traweek R, Witt RG, Erstad DJ, Scally CP, et al. Real-world use of palbociclib monotherapy in retroperitoneal liposarcomas at a large volume sarcoma center. Int J Cancer (2022) 150(12):2012–24. doi: 10.1002/ijc.33956
103. Zhang S, Mo Q, Wang X. Oncological role of HMGA2. Int J Oncol (2019) 55(4):775–88. doi: 10.3892/ijo.2019.4856
104. Mansoori B, Mohammadi A, Ditzel HJ, Duijf PHG, Khaze V, Gjerstorff MF, et al. HMGA2 as a critical regulator in cancer development. Genes (2021) 12(2):269. doi: 10.3390/genes12020269
105. Stacchiotti S, Zuco V, Tortoreto M, Cominetti D, Frezza AM, Percio S, et al. Comparative assessment of antitumor effects and autophagy induction as a resistance mechanism by cytotoxics and EZH2 inhibition in INI1-negative epithelioid sarcoma patient-derived xenograft. Cancers (2019) 11(7):1015. doi: 10.3390/cancers11071015
106. Natarajan S, Hombach-Klonisch S, Dröge P, Klonisch T. HMGA2 inhibits apoptosis through interaction with ATR-CHK1 signaling complex in human cancer cells. Neoplasia (2013) 15(3):263–80. doi: 10.1593/neo.121988
107. Palmieri D, Valentino T, D'Angelo D, De Martino I, Postiglione I, Pacelli R, et al. HMGA proteins promote ATM expression and enhance cancer cell resistance to genotoxic agents. Oncogene (2011) 30(27):3024–35. doi: 10.1038/onc.2011.21
108. Su L, Deng Z, Leng F. The mammalian high mobility group protein AT-hook 2 (HMGA2): Biochemical and biophysical properties, and its association with adipogenesis. Int J Mol Sci (2020) 21(10):3710. doi: 10.3390/ijms21103710
109. Su L, Bryan N, Battista S, Freitas J, Garabedian A, D'Alessio F, et al. Identification of HMGA2 inhibitors by AlphaScreen-based ultra-high-throughput screening assays. Sci Rep (2020) 10(1):18850. doi: 10.1038/s41598-020-75890-0
110. Yamashita K, Kohashi K, Yamada Y, Akatsuka S, Ikuta K, Nishida Y, et al. Prognostic significance of the MDM2/HMGA2 ratio and histological tumor grade in dedifferentiated liposarcoma. Genes Chromosomes Cancer (2021) J60(1):26–37. doi: 10.1002/gcc.22899
111. Brahmi M, Dufresne A, Verret B, Tirode F, Blay JY. NTRK fusion in soft tissue sarcomas harboring MDM2/CDK4 amplification: Three case reports. Ann Oncol (2021) 32(6):813–4. doi: 10.1016/j.annonc.2021.02.019
112. Olson N, Gularte-Mérida R, Selenica P, Da Cruz Paula A, Alemar B, Weigelt B, et al. Molecular characterization of a rare dedifferentiated liposarcoma with rhabdomyosarcomatous differentiation in a 24 year old. Int J Surg Pathol (2020) 28(4):454–63. doi: 10.1177/1066896919890401
113. Tontonoz P, Singer S, Forman BM, Sarraf P, Fletcher JA, Fletcher CD, et al. Terminal differentiation of human liposarcoma cells induced by ligands for peroxisome proliferator-activated receptor gamma and the retinoid X receptor. Proc Natl Acad Sci U.S.A. (1997) 94(1):237–41. doi: 10.1073/pnas.94.1.237
114. Demetri GD, Fletcher CD, Mueller E, Sarraf P, Naujoks R, Campbell N, et al. Induction of solid tumor differentiation by the peroxisome proliferator-activated receptor-gamma ligand troglitazone in patients with liposarcoma. Proc Natl Acad Sci U.S.A. (1999) 96(7):3951–6. doi: 10.1073/pnas.96.7.3951
115. Frapolli R, Bello E, Ponzo M, Craparotta I, Mannarino L, Ballabio S, et al. Combination of PPARγ agonist pioglitazone and trabectedin induce adipocyte differentiation to overcome trabectedin resistance in myxoid liposarcomas. Clin Cancer Res (2019) 25(24):7565–75. doi: 10.1158/1078-0432.CCR-19-0976
116. Debrock G, Vanhentenrijk V, Sciot R, Debiec-Rychter M, Oyen R, Van Oosterom A. A phase II trial with rosiglitazone in liposarcoma patients. Br J Cancer (2003) 89(8):1409–12. doi: 10.1038/sj.bjc.6601306
117. Pishvaian MJ, Marshall JL, Wagner AJ, Hwang JJ, Malik S, Cotarla I, et al. A phase 1 study of efatutazone, an oral peroxisome proliferator-activated receptor gamma agonist, administered to patients with advanced malignancies. Cancer (2012) 118(21):5403–13. doi: 10.1002/cncr.27526
118. Kim YJ, Yu DB, Kim M, Choi YL. Adipogenesis induces growth inhibition of dedifferentiated liposarcoma. Cancer Sci (2019) 110(8):2676–83. doi: 10.1111/cas.14036
Keywords: well differentiated liposarcoma, dedifferentiated liposarcoma, soft tissue sarcoma, MDM2, CDK4, XPO1, receptor tyrosine kinase, investigational therapy
Citation: Cassinelli G, Pasquali S and Lanzi C (2022) Beyond targeting amplified MDM2 and CDK4 in well differentiated and dedifferentiated liposarcomas: From promise and clinical applications towards identification of progression drivers. Front. Oncol. 12:965261. doi: 10.3389/fonc.2022.965261
Received: 09 June 2022; Accepted: 12 August 2022;
Published: 02 September 2022.
Edited by:
Bekir Cinar, Clark Atlanta University, United StatesReviewed by:
Ming Zhao, Zhejiang Provincial People’s Hospital, ChinaCopyright © 2022 Cassinelli, Pasquali and Lanzi. This is an open-access article distributed under the terms of the Creative Commons Attribution License (CC BY). The use, distribution or reproduction in other forums is permitted, provided the original author(s) and the copyright owner(s) are credited and that the original publication in this journal is cited, in accordance with accepted academic practice. No use, distribution or reproduction is permitted which does not comply with these terms.
*Correspondence: Cinzia Lanzi, Y2luemlhLmxhbnppQGlzdGl0dXRvdHVtb3JpLm1pLml0