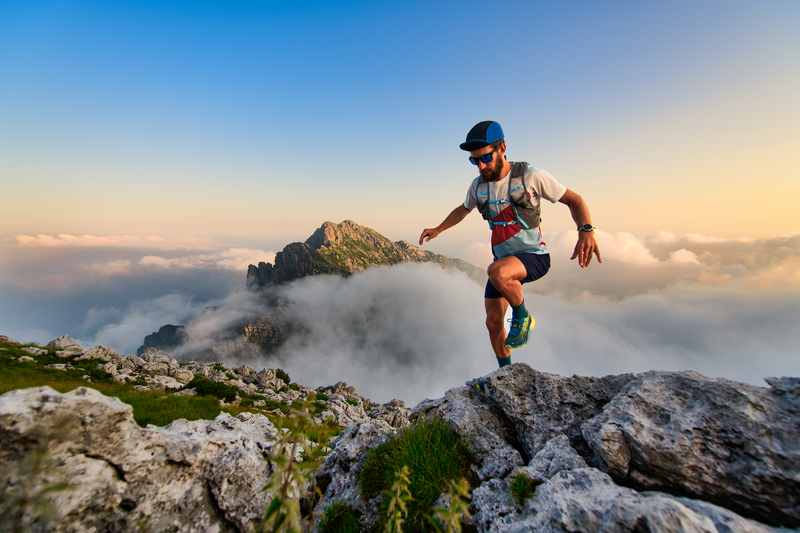
95% of researchers rate our articles as excellent or good
Learn more about the work of our research integrity team to safeguard the quality of each article we publish.
Find out more
REVIEW article
Front. Oncol. , 27 July 2022
Sec. Cancer Metabolism
Volume 12 - 2022 | https://doi.org/10.3389/fonc.2022.941643
This article is part of the Research Topic Sphingolipid Metabolism and Cancer View all 5 articles
Biologically active sphingolipids are closely related to the growth, differentiation, aging, and apoptosis of cancer cells. Some sphingolipids, such as ceramides, are favorable metabolites in the sphingolipid metabolic pathway, usually mediating antiproliferative responses, through inhibiting cancer cell growth and migration, as well as inducing autophagy and apoptosis. However, other sphingolipids, such as S1P, play the opposite role, which induces cancer cell transformation, migration and growth and promotes drug resistance. There are also other sphingolipids, as well as enzymes, played potentially critical roles in cancer physiology and therapeutics. This review aimed to explore the important roles of sphingolipid metabolism in cancer. In this article, we summarized the role and value of sphingolipid metabolism in cancer, including the distribution of sphingolipids, the functions, and their relevance to cancer diagnosis and prognosis. We also summarized the known and potential antitumor targets present in sphingolipid metabolism, analyzed the correlation between sphingolipid metabolism and tumor immunity, and summarize the antitumor effects of natural compounds based on sphingolipids. Through the analysis and summary of sphingolipid antitumor therapeutic targets and immune correlation, we aim to provide ideas for the development of new antitumor drugs, exploration of new therapeutic means for tumors, and study of immunotherapy resistance mechanisms.
Over the last 2–3 decades, we have gained a great understanding of the structural biodiversity, cell biology, metabolisms, and pathology of sphingolipids. Various molecules from this lipid family participate in a variety of cellular functions in health and disease (1). As shown in Figure 1, ceramides constitute the hub of sphingolipid metabolism and participate in the synthesis and catabolic metabolism of sphingolipids. Ceramides can be generated through several different mechanisms. Endogenous ceramides can be synthesized via de novo pathway following the action of a range of enzymes, such as serine palmitoyltransferase (SPT), ceramide synthase (CerS), and dihydroceramide desaturase (DES). Ceramides may also be produced through the decomposition of membrane sphingomyelins (SMs) or the degradation of complex sphingolipids under the action of sphingomyelinases (SMase) and glucosylceramidases, respectively. In addition, sphingosine, a product of sphingolipid catabolism, can be reacylated by CerS, resulting in the production of ceramides. Once produced, ceramides can briefly accumulate or be converted into different, sphingolipids, such as ceramide 1-phosphate (C1P), sphingosine 1-phosphate (S1P), and glucosylceramide (GlcCer). Ceramide can also be reversed into SMs, that is, phosphorylcholine and ceramide generate SMs under the action of sphingomyelin synthetase (SMS). In addition, ceramides can be glycosylated by different enzymes of the Golgi Apparatus into glycosphingolipids (GSLs). Moreover, sphingolipids are also glycosylated in the lysosomes (2). The phosphorylation of ceramide also occurs in Golgi Apparatus by CerK (3) (Figure 1).
The mortality rate caused by malignant tumors has nearly doubled in the last two decades (4), and cancer remains the highest cause of mortality worldwide. Sphingolipids and tumors are closely related. The correlation between them has received increasing attention from researchers. Sphingolipids, among all the reasons for the pathogenesis of cancer researched thus far, play a critical role in the initial and developmental stages of cancer. Sphingolipids serve as bioeffectors that coordinate all aspects of cancer biology, including apoptosis, cell proliferation, and cell migration. Ceramide and S1P are important sphingolipids but have opposite effects. Ceramide promotes cancer cells apoptosis, blocks cell growth; S1P promotes cancer cells survival, enhancing cell proliferation, angiogenesis. The dynamic balance of these two opposing sphingolipids is called the “Sphingolipid rheostat” (5). The sphingolipid-regulated processes are critical for the development, progression, metastasis, and drug resistance of different types of cancers (1).
Sphingolipids are also expressed differently in various tumor subtypes. Recent studies have shown that some classes of ceramides may be elevated in certain human malignancies. For example, C16:0 and C24:1 ceramides are up-regulated in node-positive pancreatic tissues (6). Similar observations on up-regulated sphingolipids have been found for B16 melanoma, murine sarcoma 180, and Lewis lung carcinoma (7); human head and neck squamous cell carcinoma (HNSCC) (8); rat nephroma-RA (9); and breast cancer (10). By contrast, colon cancer (11), ovarian carcinoma (12), and human astrocytoma (13) have lower ceramide levels than their corresponding healthy organizations. in certain human malignancies, since in some works depending on the species, their levels are decreased. Another published report found that C18-Cer is selectively down-regulated in HNSCC relative to in nonsquamous tumor tissues (14).
Then, in the downstream of sphingolipid pathways, the activity of SPHK1/S1P plays an important role in many cancers, and SPHK1 has been proven to be highly expressed in a variety of cancers (15–17). An investigation has been applied to test the tissue samples from prostate cancer patients and healthy individuals. The results proved that SPHK1 expression is 2-fold higher in tissues from patients than in healthy individuals (18). This phenomenon had also been observed in breast cancer. For example, a previous work performed microarray analysis on various subtypes in 1269 breast cancer samples and found increased SPHK1 expression in the tissues of patients with cancer (19). Another study showed that, in breast cancer, SPHK1 and ceramide galactosyltransferase (UGT8) are highly expressed in ER-negative tumors, and dihydroceramide synthases (LASS4 and LASS 6), acid ceramidase (ACDase), and glucosylceramide synthase (GCS) are highly expressed in ER-positive samples (19). Moreover, numerous other cancers have high SPHK1 expression (20–23). These cancers include oral squamous cell carcinoma (24), gastric cancer (15), hepatocellular carcinoma (HCC) (16, 17), glioblastoma multiforme (20), prostate cancer (21), esophageal cancer (22), and HNSCC (23) (Table 1). All the above pieces of information show that the cell-transformation-related alterations in sphingolipid metabolism are dependent on cancer types. We have summarized the abnormal sphingolipid metabolism of different cancers in Table 1. Changes in sphingolipid metabolism contribute to the progress of cancers and provide useful targets for the development of new targeted therapies. Moreover, some sphingolipids could be used as biomarkers in cancer diagnosis. The latest data have shown that in patients with breast cancer, the increase in C16-Cer is associated with metastatic lymph node (LN) status (10), suggesting that C16-Cer has metastatic potential in the clinic. Interestingly, an investigation on colorectal cancer (CRC) tissue has suggested that CRC shows increased amounts of S1P, Sph, and C14-Cer and markedly reduced amounts of C18-Cer and C20-Cer. The circulating contents of C16:0-Cer, C18:1-Cer, C20-Cer, and C24:1-Cer in more advanced CRC were higher than those in early lesions (32). These results suggest that early lesions can be distinguished from advanced CRC through the combined determination of the plasma concentrations of several ceramides. In addition to being the diagnostic biomarkers, sphingolipids could also be used as biomarkers in cancer prognosis. Clinical studies have reported that the expression levels of SPHK and S1P are associated with patient survival and cancer metastasis (22, 23). An online database of gene expression and clinical data from 1928 patients with NSCLC has showed that although the high expression of SPHK1 mRNA is significantly correlated with worsened overall survival (OS), the high expression of SPHK2 mRNA is correlated with improved OS (23). Interestingly, in esophageal cancer, the high expression of SPHK1 is associated with poor 5-year OS and increased SPHK1 levels are significantly correlated with LN metastasis; therefore, SPHK1 is a potential biomarker for outcome prediction in clinical practice (22). A meta-analysis has shown that increased SPHK1 expression is associated with poor prognosis in human cancers and could be a promising prognostic marker and therapeutic target in patients with malignancies (33). Despite SPHK1 and SPHK2 are highly homologous, different subsets of SPHK execute different physiological roles in cancers. However, both subtypes have important roles in inducing cancer cell survival (34, 35).
An increasing number of studies have explored the correlations between sphingolipids and tumors. The dynamic balance among sphingolipids maintains normal biological functions. In cancer, however, this balance can be disrupted. A growing body of evidence shows that in cancer, the levels of bioactive sphingolipids are altered. Thus, bioactive sphingolipids harbor the potential to act as important cancer biomarkers for the determination of disease progression (36). Given the different expression levels of metabolites in different cancers, the same sphingolipids could execute opposite roles in different cancers.
Ceramides are one of the most studied sphingolipids in cancers due to their role in cell differentiation and death. Diverse ceramides produce different lengths of ceramide chains that have unique and important biological functions in vivo. Ceramides can induce apoptosis through the mitochondrial pathway by changing the mitochondrial ultrastructure and reducing mitochondrial function and membrane potential, thus ultimately inducing apoptosis (37). For example, C18-Cer could modulate telomerase activity and mitochondrial dysfunction-induced apoptotic cell death in HNSCC (14), and C16-Cer could induce ER stress-mediated apoptosis in lung cancer (38). Ceramides can also cause cell death via autophagy. In liver and nasopharyngeal cancers, ceramides could induce autophagy through the regulation of Beclin-1 (39). Dihydroceramide could also activate the unfolded protein response that leads to cytotoxic autophagy in cancer cells (40). In colon cancer, ceramide inhibits cell proliferation and migration by down-regulating IL-10, STAT3, and NF-kB expression (41). Another published report has shown that in breast cancer, the overexpression of C16-Cer reduces the phosphorylation of Akt/mTOR and ERK (42). Ceramides have numerous mechanisms for cell death induction. Instead of inhibiting cell growth as mentioned above (42), C16-Cer protects against ER stress-induced apoptosis and enhances tumor development and growth in HNSCC (43). Similarly, ceramide has an opposite effect in colon cancer that is distinct from their effect in gallbladder cancer (44). C24-Cer induces cell proliferation and migration by binding to PIP4K2C to facilitate mTOR complex formation and activation (45) (Table 2).
In contrast to ceramides, S1P can modulate multifunctional biological activities in cancer, such as proliferation, migration, inflammation, and angiogenesis. As proposed by Hanahan and Weinberg, S1P is involved in all features of cancer, including sustained proliferative signaling, the evasion of growth inhibitors, resistance to apoptosis, the achievement of uncontrolled replication, angiogenesis, the activation of invasion and metastasis, the reprogramming of energy metabolism, and the evasion of the immune response (78). The generation of S1P from sphingosine is catalyzed by two SPHK isoforms, namely, SPHK1 and SPHK2. S1P receptors are called S1PR. S1P binds to five high-affinity G-coupled receptors with different tissue distribution patterns: S1PR 1–5. The specific role of S1P is dominated by the expressed S1PR subtype (44).
Similar to vascular endothelial growth factor, S1P has been reported to increase vascular permeability. The mechanism of activation involves the activation of the S1PR2 subtype and requires Rho/ROCK/PTEN signal transduction (79). In lymphatic cancer, S1P could prevent apoptosis by inhibiting the translocation of cytochrome c and Smac/DIABLO from the mitochondria to the cytoplasm (53). S1P has also been shown to influence the metastasis and migration of cancer cells. For example, S1P could induce HCC metastasis via establishing the MMP-7/syndecan-1/TGF-β1 autocrine loop (55). Moreover, it could stimulate the chemotaxis and invasion of ovarian cancer cells in a receptor-dependent manner that involves the activation of ERK, AKT, and p38 (43).
The abundance of sphingolipids is controlled by metabolic enzymes, which have a crucial role in regulating cancer cell death or survival as summarized in Table 2. Thus, we next focus on the enzymes participating in cancer physiology. Some enzymes have been proven to be capable of enhancing ceramide synthesis and break down S1P to promote cancer cell death. These enzymes include SMase, SPT, CerS, DES, and S1P lyase (SPL), which need therapeutic activators (1). On the other hand, some enzymes that could eliminate and metabolize ceramides, such as CerK, CDase, SPHK, and SMS, which need therapeutic inhibitors.
SMase is a sphingolipid enzyme that has been widely reported. It can be divided into three types in accordance with its optimal pH-dependent activity: acid SMase (ASMase), neutral SMase (NSMase), and alkaline SMase (alk-SMase) (45). Many pieces of evidence had suggested that the activation of ASMase is a necessary step for the initiation of apoptosis in stress-induced ceramide elevation (62). NSMase has also been reported to cause apoptosis in cancer (63, 64). In addition, the lack of alk-SMase has been shown to be capable of significantly increasing colon tumorigenesis (80).
Another one, SPHK1, can promote proliferation and migration by promoting JAK/STAT activation and up-regulating S1PR1 expression (81). SPHK1 promotes triple negative breast cancer (TNBC) translocation through the S1P/S1PR3/Notch signaling pathway (29). Convincing evidence indicates that the activation of SPHK1 contributes to cancer progression and tumor growth and impairs cancer cell apoptosis via suppressing STAT1 expression (72), as well as activating the ERK1/2 and PI3K/AKT pathways (29) Many studies have demonstrated that the activation of SPHK1 can induce cancer cell migration, and that the SPHK1/S1P axis enhances the metastatic potential of cancer cells. Mechanism studies have reported that SPHK1 increases the expression of Slug, vimentin, N-cadherin, and FAK in colon cancer (82) Notably, the active interaction of SPHK1 or S1P with ERK pathway promotes autophagy in colon cancer (68).
CerK is a calcium ion-dependent enzyme that catalyzes the production of C1P in cells. Intracellular C1P can perform a variety of biological functions, including the stimulation of tumor cell growth, the stimulation of VEGF release, the inhibition of tumor cell apoptosis, the promotion of tumor inflammation, the regulation of angiogenesis, and the stimulation of tumor cell migration (83–85). C1P regulates the invasion and migration of different types of cancer cells, including breast, lung, prostate, pancreas or leukemia cells (83). CerK phosphorylates cell-killing ceramides into C1P. The metabolic conversion of ceramide into SM is catalyzed by SMS. CerK and SMS can induce migration and invasion (61, 65). Some sphingolipid enzymes also have dual roles in different cancers as illustrated by CerK. Although CerK can regulate Rac1 activity and inhibit lamellipodium formation in A549 cells (60), it can promote migration in breast cancer (61).
Sphingolipids are currently recognized as multifaceted mediators in the biological and therapeutic fields of cancer. Therefore, they may become new targets for therapeutic application. Sphingolipid metabolism is becoming increasingly well studied in this field of cancer treatment. Several types of cytotoxic chemotherapeutic agents that cause ceramide accumulation, including doxorubicin, irinotecan, vincristine, and paclitaxel, have been clinically applied or undergoing clinical trials (43).
Although sphingolipids have been demonstrated to have an effective antitumor action, their poor solubility, uptake, bioavailability, and metabolic transformation have hindered the efforts to utilize their antitumor effect. Therefore, determining a way to improve efficacy is necessary for the development of ceramide-based therapy (Figure 2). For example, a previous work showed that in cultured TNBC cells, nanoliposomal tamoxifen enhances nanoliposomal C6-ceramide cytotoxicity. This reaction is accompanied by the induction of cell cycle arrest in G1 and G2 by caspase-induced DNA fragmentation. The permeability of the mitochondria and lysosomal membrane was enhanced at 18 and 2 h, respectively (86). The combination of C6-ceramide nanoliposomes with PPMP has been shown to lead to an increase in endogenous long-chain ceramide species, resulting in the apoptosis of leukemic natural killer (NK) cells via the mitochondrial endogenous cell death pathway (87). Moreover, in mice with hepatic tumors, the injection of nanoliposome-loaded LipC6 reduces the amount of tumor-associated macrophages and the ability of TAMs to inhibit the antitumor immune response. LipC6 also enhances the antitumor activity of tumor antigen-specific CD8+ T cells (88). A novel method for loading the short-chain C6 ceramide onto polyethylene glycol and polyethyleneimine co-conjugated ultra-small nano-GO can also result in high cancer-cell-killing potential in HCC (89).
In addition to sphingolipids, sphingolipid enzymes have also become important targets for anti-tumor selection. SMase could be activated after stimulation by radiation and chemotherapeutic drugs, such as leukemia and glioma. The activated SMase catalyzes the hydrolysis of SM to produce ceramide. The produced ceramide would continue to activate different protein kinases and protein phosphatases (JNK and PKC), thus initiating the cascade signaling pathway, transmitting extracellular signals to cells, and then causing apoptosis. Therefore, the inhibition of enzymes that break down ceramide can alleviate chemotherapy resistance via sphingolipid regulation. The inhibitors of sphingolipid-metabolizing enzymes can be used as chemical sensitizers (37). Interestingly, SPHK1 has also been reported to promote the oxaliplatin resistance of HCC cells via the modulation of the Akt/GSK3β signaling pathway (16). So, the SPHK1 inhibitor maybe reverses oxaliplatin resistance.
Given that the dysregulation of sphingolipid enzymes can lead to the occurrence of cancer, the discovery of the optimal characterized inhibitors and activators targeting sphingolipid enzymes is important. At present, many inhibitors for different types of sphingolipid enzymes, such as Acid ceramidase (ASAH1), ACDase, CerK, SPHK, and GCS, have been discovered (90). B13 is a ceramide analogue that has been shown to be an effective in vitro ACDase inhibitor (30). B13 can accumulate cellular ceramides in vivo. It has been modified with a series of lysosomotrophic molecules, such as LCL521 (30), to address its difficult accumulation in lysosomes. NVP-231 is a CerK inhibitor. NVP-231 decreases cell viability, DNA synthesis and colony formation in a concentration-dependent manner. It can increase DNA fragmentation and induce apoptosis (91). Dimethylsphingosine is a methylated form of sphingosine and is the first SPHK inhibitor (90). The SphK inhibitor is called SK I-II. SPHK I-II simultaneously inhibits SPHK1 and SPHK2, as well as other targets, and SPHK inhibition with SPHK I-II decreases MDR breast cancer proliferation and viability (92). FTY720, a sphingosine analogue that induces S1P receptor down-regulation, is another SPHK1 inhibitor (fingolimod). It inhibits SPHK1 and blocks the activation of multiple targets of this enzyme. It directly or indirectly inhibits multiple intracellular targets responsible for cell proliferation, migration, and angiogenesis (93). Moreover, preclinical data support its possible use as an antineoplastic drug (94). The GCS inhibitors D-threo-1-phenyl-2palmitoylamino-3-morpholino-l propanol (PPMP) and D-threo-1-phenyl-2-decanoylamino-3-morpholino-propanol (PDMP) are ceramide analogues. A study on leukemic cell lines has shown that by blocking GCS with PDMP and PPMP simultaneously, cells become desensitized to daunomycin because of the antiapoptotic action of galactoside ceramide accumulation (95). Thus, the effect of sphingolipid enzymes and their inhibitors on cancer cells is an interesting topic that needs further studies.
Drug resistance is a serious barrier to the successful therapy of patients with cancer. A large number of experiments have reported the mechanisms through which sphingolipids and their enzymes cause cancer drug resistance. Sphingolipid expression is also applied as a biomarker of drug resistance. The most widely known sphingolipid enzyme is GCS. This enzyme catalyzes the combination of glucose and ceramide to produce noncytotoxic GlcCer, which results in the decrement in cytotoxic Ceramide levels. The overexpression of GCS, which is particularly prominent in chemotherapy resistance, has been found in various cancers (96). In breast cancer, MDR1 overexpression is accompanied by other changes in genes, including GCS. MDR1 encodes P-glycoprotein, which could extrude anticancer drugs (97). Then, in breast cancer, high GCS expression is correlated with multidrug resistance (MDR), the poor prognosis of estrogen receptor (ER)+ tumors (30), and metastatic ER+ and human epidermal growth factor receptor 2 (HER2)+ tumors (Luminal B) (91). In ovcar-8 cancer cells, GCS transfection induces MDR1 overexpression and increases P-glycoprotein excretion in a dose-dependent manner. GSLs mediate MDR1 gene expression via cSrc and β-catenin signaling (97). In prostate cancer, SPHK1 inhibitors enhance the anticancer efficacy of enzalutamide (92). In addition, ceramide levels are lower in patients with chemoresistant leukemia than in patients with chemosensitive leukemia, whereas the activities of SMS and GCS in chemoresistant leukemia cells are higher than those in chemosensitive leukemia cells (98). Other studies have shown that sphingolipid enzymes, such as CDase and SPHK (99), are closely related to chemotherapy resistance.
A growing number of studies have demonstrated that metabolites in sphingolipid metabolism are closely associated with tumor immunity and that sphingolipids are involved in the interactions between cancer cells and the immune system. Sphingolipids are important components of the plasma membrane and can influence the function of receptors on the surfaces of immune cells. Metabolites are involved in the release of bioactive mediators, S1P, and ceramides, which influence the efflux and migration of lymphocytes into the tumor environment and regulate the critical pathways required for the activation of immune cells (100). The release of bioactive mediators, S1P, and ceramides can alter the function of immune cells, including Tregs lymphocytes, and macrophages, in the tumor microenvironment (TME), as well as alter the intracellular signaling pathways associated with immune cell activation or survival; such an alteration, in turn, may modulate the efficacy of antitumor immunotherapy (100). In a mouse model, ceramides inhibit the function of myeloid-derived suppressor cells (MDSCs) by activating lysosomal histone B and histone D; this effect leads to diminished autophagy and ER stress induction, thereby enhancing cytotoxic T lymphocyte function and inhibiting the growth of CMS4-met-derived soft tissue sarcoma tumors (77).
Other studies have reported that the metabolite C2-ceramide triggers apoptosis in melanoma cells by increasing PKCζ, as well as proinflammatory cytokines and signaling factors (101). C2-ceramide regulates immune cells in the TME and activates host protective immune responses through the up-regulation of PKCζ. In a PKCζ-dependent pathway, C2-ceramide repolarizes tumor-associated macrophages (TAMs) toward the M1 phenotype, thereby generating antitumor responses in vitro and in vivo (101). Ceramide-mediated alterations in host-protective angiogenic factors and alterations in T helper 1 cell (Th1) and T helper 2 cell (Th2) cytokine levels lead to improvements in the TME. C2-ceramide tilts the immune component within the TME toward a proinflammatory state and differentially regulates immune cell–cancer cell interactions to limit tumor growth. At the same time, C2-ceramide can increase the proportion of activated CD8+ T-cells in the TME and up-regulate perforin and granzyme B, which kill tumors in response (101).
Ceramides modified with nanomaterials can further modulate the efficacy of tumor immunotherapy. Nanoliposomal C6-ceramide (LipC6) not only induces apoptosis and prevents HCC-induced immune tolerance in HCC cells, it also significantly decelerates the growth of liver cancer cells, enhances tumor-infiltrating CD8+ T cells, and inhibits tumor-resident CD4+ CD25+ forkhead box protein P3 (FoxP3+) Treg cells in combination with anti-CTLA4 Ab (102). Molecular studies have shown that combination therapy inhibits the transcription factors Krüppel-like factor 2, FoxP3 and CTLA4 (102). The dysregulation of sphingolipid metabolism is also frequently associated with cancer stem cells (103, 104) and consequently generates a series of tumor immune-related effects; this situation suggests that ceramide is a key player in cancer immunotherapy (104). Elevated levels of ceramides induce cancer cell death (105), enhance the host protective immune response, and induce cancer regression (101, 106). Elevated levels of S1P induce an increase in the antiapoptotic proteins Bcl-2 and Bcl-XL in macrophages and the M2-type polarization of macrophages, which can also recruit immune cells to immune tissues for immune action (107, 108). S1P released from apoptotic or tumor cells, in combination with its specific receptor (S1PR1), induces the recruitment of circulating monocytes to the TME (109). α-Galactose ceramide (α-GalCer) has been noted for its role in activating invariant natural killer T (iNKT) lymphocytes and has been dicscovered to have a beneficial effect on the immune system. It has been noticed for its role in activating iNKT lymphocytes and has been found to exert oncogenic effects in a variety of tumors by inducing effective iNKT cells. The addition of PD-1 blockers to α-GalCer treatment prevents iNKT cell loss, resulting in the skewing of CD4+ cells toward Th1. This situation suggests that the combination of α-GalCer and immune checkpoint blockers may be a promising approach for improving the efficacy of tumor immunotherapy (110). In conclusion, sphingolipids are closely related to tumor immunity, and altered levels of sphingolipid metabolism are important factors influencing tumor immunotherapy (Figure 3).
Now that we understand that sphingolipids are closely associated with tumor immunotherapy, we are eager to learn the exact mechanisms underlying the important roles of SMases in association with tumor immunotherapy. In different T cell subpopulations in mice and humans, sphingolipids are metabolized by varying degrees into ceramides that, in turn, participate in immune regulation and influence T cell activation, differentiation, and effector functions (111). ASMase (mouse: Asm; human: ASM) is a key mediator of sphingolipid catabolism. SMase deficiency impairs T-cell function, whereas increasing SMase activity increases T-cell function, thereby improving the efficacy of anticancer immunotherapy. These phenomena suggest that increasing ASM activity in T cells through drugs may enhance T cell-mediated immunity and may thus improve tumor killing (111).
Ceramide synthase 5 is one of six enzymes that catalyze the production of ceramides from sphingosine. Ceramides are an essential component of cell membranes and act as signaling molecules. CerS5 knockout mice have significantly reduced CD8+ T cells in the colonic epithelium. This decrement is accompanied by the reduced expression of IL-1β, IFNγ, and IL-4. The mice show increased susceptibility to colon cancer. In vitro studies have found that the knockdown of ceramide synthase 5 in T cells impairs T cell activation (112). Moreover, SPHK1 produced S1P is directly linked to the activity of the lipid transcription factor peroxisome proliferator-activated receptor γ, which subsequently regulates lipolysis in T cells. The inhibition of SPHK1 increases the antitumor activity of T cells against murine melanoma (113). Alkaline ceramidase 3 (Acer3) mediates the immune response by regulating C18:1-ceramide levels in the cells of the innate immune system, and Acer3 deficiency increases the levels of proinflammatory cytokines in colonic epithelial cells; this effect may subsequently regulate the development and progression of intestinal cancer (114). Then, neutral SMase 2 (nSMase2) is encoded by SMPD3 and catalyzes the breakdown of SM to produce ceramide. In mouse models of melanoma and breast cancer, the overexpression of wild-type nSMase2 increases anti-PD-1 efficacy. This increase is associated with an enhanced Th1 response. In wild-type mice, nSMase2-overexpressing tumors accumulate ceramide and CD8+ tumor-infiltrating lymphocytes, which are associated with increased levels of transcripts encoding IFNγ and CXCL9. In vitro, small extracellular vesicles from melanoma cells overexpressing wild-type nSMase2 enhance the expression of IL12, CXCL9, and CCL19 in bone marrow-derived dendritic cells, suggesting that melanoma nSMase2 triggers Th1 polarization at the earliest stages of the immune response (115). This situation suggests that the modulation of sphingolipid metabolism-related enzymes may alter the efficacy of tumor immunotherapy effectively (Figure 4). Hope for the discovery of additional effective targets for modulating tumor immunotherapy has been found with the progression of the research on sphingolipid metabolism-related enzymes in relation to tumor immunity.
Natural products medicine has been practiced for thousands of years all over the world and has played an critical role in the treatment and prevention of different diseases throughout history (116). Given their safety and low side effects, natural products are used as alternative cancer therapies. Numerous natural products have various biological activities and low toxicity at the same time and are currently widely accepted as an excellent source of anticancer drugs (117). Previous studies have shown that some herbal compounds can treat and prevent cancer from recurring and metastasizing and can thus prolong the survival of postoperative patients with cancer. When applied in the early stage of cancer, active ingredients can cure pre-cancerous conditions and decrease the rate of cancer occurrence (118). With the transformation of modern medical concepts from treatment to strengthening the physique, the advantages of natural products have become more apparent than before. Thus, as indicated in Table 3, natural products can be used as platforms for the design of sphingolipid-related anticancer drugs.
Many natural compounds isolated from bacteria and fungi possess SPHK inhibition activity. B-5354c, which is produced by a marine bacterium, inhibits SPHK1 and SPHK2 with similar efficiencies (131) and acts on sphingosine in a noncompetitive manner. B-5354c can reduce the size of PC-3 tumors in vivo when combined with the chemotherapy drug irinotecan and can sensitize PC-3 and LNCaP prostate cancer cells to chemotherapy drugs, such as docetaxel and camptothecin (132). B-5354c has been further demonstrated to act as a chemosensitizer of PC-3 cells when used in conjunction with docetaxel (133). F-12509A (125, 126), extracted from Trichopeziella barbata, and S-15183a/b (126), extracted from Zopfifiella inermis, are also natural products that have been found to inhibit SPHK. Jaspine B is an anhydrophytosphingosine, extracted from marine sponge Jaspis sp, with potential antineoplastic property. It can increase the concentration of ceramides within cells by inhibiting SMS. Jaspine B can also induce apoptosis in human SK-Mel28 melanoma and murine B16 cells (127). Myriocin, extracted from Myriococcum ablomyces, specifically inhibits SPT, leading to the suppression of cell growth by triggering the cell cycle in melanoma cells (124) and significantly inhibiting tumor formation in melanoma mice (123). Myriocin combined with docetaxel and cisplatin can also inhibit the growth of cancer cells (120). In prostate cancer, sanguinarine, extracted from Sanguinaria canadensis, Chelidonium majus and Macleaya cordata, inhibits ACDase and GCS enzymes, subsequently resulting in ceramide generation and apoptosis (121).Tricin, a component extracted from rutabaga, exerts proliferation-inhibiting, proapoptotic, and migration-inhibiting effects on Lewis lung cancer cells. It inhibits tumor growth mainly by inhibiting PRKCA/SPHK/S1P signaling and antiapoptotic signaling (73). Resveratrol, extracted from Polygonum cuspidatum Sieb.et Zucc., induces autophagy in gastric cancer cells (HGC-27) by inhibiting dihydroceramide desaturase and increasing dihydroceramide (122). Moreover, resveratrol also triggers anti-proliferative and apoptotic effects in FLT3-ITD-positive acute myeloid leukemia cells through inhibition of ceramide catabolic enzymes (119, 134). Vincristine is a vinca alkaloid that can be obtained from the Madagascar periwinkle Catharanthus roseus (135, 136). The combination of vincristine and SPHK2 inhibitor ABC294640 significantly enhanced the inhibition of SPHK2 and further inhibited the survival and proliferation of T-cell acute lymphoblastic leukemia (T-ALL) cells (129).
Overall, targeting sphingolipid enzymes and altering sphingolipid metabolism can lead to promising anticancer effects. In addition, natural products are a primary source of potential anticancer agents and can thus improve and increase the effectiveness of cancer therapies. The discovery and clarification of their mechanisms of action are urgently needed.
Sphingolipids have a broad scope of biological functions. Numerous discoveries have elucidated the different roles and mechanisms of sphingolipids in cancers. The unique anticancer or oncogenic functions of sphingolipids in cancers rely on microenvironmental conditions, cell types, and immune status. The rapid metabolism and signaling of sphingolipids within biological membranes have a strong influence on the regulation of cell death and survival. Further studies are needed to determine how sphingolipid enzymes regulate proliferation in cancer systems. Therefore, additional frontier molecular and analytical tools and technologies should be developed and applied in sphingolipid-related anticancer investigations. Although a large number of preclinical trials have shown good results, FDA-approved drugs targeting sphingolipids in cancer treatment remain lacking. Natural drugs, such as TCMs, have made many contributions to the study of sphingolipid metabolism. In the future, strengthening the cooperation in the research and clinical practice of sphingolipids through the integration of Chinese and Western medicine will be necessary and is expected to increase the effectiveness of tumor diagnosis and treatment.
Endogenous ceramides are synthesized through the de novo pathway, which begins with palmitoyl-CoA and the condensation of the amino acid serine to form 3-ketosphinganine via SPT, leading to the synthesis of dihydroceramide by CerS. Dihydroceramides are then converted into ceramides by DES. Ceramides may also be produced through the breakdown of SMs or the degradation of complex sphingolipids under the action of SMase and GCase. In addition, the salvage pathway can generate endogenous ceramides. Sph, a product of sphingolipid breakdown metabolism, can be reanylated by CerS, resulting in the production of ceramides. Once produced, ceramides can briefly accumulate or be converted into different sphingolipids. Ceramides are phosphorylated by CerK to produce C1P and utilized as a substrate by CDase to liberate Sph, which is phosphorylated to generate S1P. Ceramides can also be reversibly metabolized into SM, that is, phosphorylcholine and ceramide generate SM under the action of SMS. GCS can remove ceramides by catalyzing the conversion of ceramides into glycosheath ester. Ceramides can also be converted into GalCer by CGT. (SPT, serine palmitoyl transferase; CerS, ceramide synthases; DES, dihydroceramide desaturase; SMs, sphingomyelins; SMase sphingomyelinases; GCase, glucosylceramidases; Sph, Sphingosine; CerK, ceramide kinase; C1P, ceramide CDase, 1-phosphate; ceramidase; S1P, sphingosine-1-phosphate; SM, sphingomyelin; SMS, phingomyelin synthetase; GCS, Glucoceramide synthetase; GalCer, galactosylceramide; CGT, galactosylceramide transferase.)
Ceramides have antitumor effects but have poor solubility, absorption, bioavailability, and metabolic conversion. Nanoliposomes can help address these disadvantages of ceramides. First, nanoliposomal tamoxifen can enhance the cytotoxicity of nanoliposomal C6-ceramide in TNBC cells, induce cell cycle arrest in the G1 and G2 phases, and increase the permeability of mitochondrial and lysosomal membranes, thus killing TNBC cells. Nanoliposomal C6-ceramide, when combined with PPMP, increases the amount of endogenous long-chain ceramides and increases the apoptosis of leukemic NK cells via the endogenous mitochondrial cell death pathway. Nanoliposomal C6-ceramide reduces the number of TAMs, induces the differentiation of TAMs into the M1 phenotype, increases CD8+ T cell activity, and suppresses the proliferation of hepatocellular tumors. (TNBC, Triple-Negative Breast Cancer; PPMP, [2]1-phenyl-2-palmitoylamino-3-morpholino-1-propanol; TAM, tumor-associated macrophage).
Ceramides activate lysosomal histone B and histone D, leading to the reduction in autophagy and the induction of endoplasmic reticulum stress. These effects further inhibit the function of MDSCs and enhance the activity of cytotoxic T lymphocytes. C2-ceramide up-regulates PKCζ, repolarizes TAM toward the M1 phenotype, increases the activation ratio of CD8+ T cells, and up-regulates the secretion of perforin and granzyme B to kill tumors. S1P induces the conversion of TAM into M2 and promotes the secretion of IL-4, IL-10, and TGFb, thus leading to tumor evasion. (Cer, ceramide; S1P, sphingosine 1 phosphate; MDSCs, myeloid-derived suppressor cells; TAM, tumor-associated macrophage).
Several enzymes are closely related to the effects of tumor immunotherapies. SMase catalyzes the production of ceramide from SM, triggering Th1 polarization, and enhancing anti-PD-1 efficacy. SMase induces ceramide accumulation, thus enhancing the activity of CD8+ tumor-infiltrating lymphocytes and increasing the expression levels of IFNγ, IL12, CXCL9, and CCL19. S1P is catalyzed by SphK directly in relation to the activity of the lipid transcription factor peroxisome proliferator-activated receptor γ, which promotes the lipolysis of T cells. Cer (red) indicates promoted immune killing of tumors, and S1P (green) indicates immunity inhibition, allowing tumors to escape. (Cer, ceramide; S1P, sphingosine 1 phosphate; SMase, sphingomyelinase; Th1, T helper 1; Cers, ceramide synthase; SphK, sphingosine kinase; PPAR γ, peroxisome proliferator-activated receptor γ).
(I) Conception and design: EL-HL, R-ZL, P-YY. (II) Administrative support: LL, EL-HL; (III) Manuscript writing: All authors; (IV) Final approval of manuscript: All authors.
This work is supported by the 2020 Guangdong Provincial Science and Technology Innovation Strategy Special Fund (Guangdong-Hong Kong-Macau Joint Lab) (No: 2020B1212030006). This work is also supported by Macao Science and Technology Development Fund (Project no: 0096/2018/A3, 0056/2020/AMJ), and from Dr. Neher's Biophysics Laboratory for Innovative Drug Discovery (001/2020/ALC). This work is also supported by 2020 Young Qihuang scholar granted to Prof. Elaine Leung from the National Administration of Traditional Chinese Medicine.
The authors declare that the research was conducted in the absence of any commercial or financial relationships that could be construed as a potential conflict of interest.
All claims expressed in this article are solely those of the authors and do not necessarily represent those of their affiliated organizations, or those of the publisher, the editors and the reviewers. Any product that may be evaluated in this article, or claim that may be made by its manufacturer, is not guaranteed or endorsed by the publisher.
α-GalCer, α-Galactose ceramide; ACDase, acid ceramidase; ASMase, acid sphingomyelinase; Acer3, Alkaline ceramidase 3; alk-SMase, alkaline sphingomyelinase; CDase, ceramidase; Cer, ceramide; C1P,ceramide 1-phosphate; UGT8, ceramide galactosyltransferase; CerK, ceramide kinase; CerS, ceramide synthase; DES, dihydroceramide desaturase; LASS, dihydroceramidsynthases; PDMP, D-threo-1-phenyl-2-decanoylamino-3-morpholino-propanol; PPMP, D-threo-1-phenyl-2palmitoylamino-3-morpholino-l propanol; FoxP3+, forkhead box protein P3; GlcCer, glucosylceramide; GSLs, glycosphingolipids; GCS, glucosylceramide synthase; LipC6, Nanoliposomal C6-ceramide; NSMase, neutral sphingomyelinase; NSMase2, neutral sphingomyelinase 2; PPAR γ, peroxisome proliferator-activated receptor γ; SPT, serine palmitoyl transferase; SMase, sphingomyelinases; SM, sphingomyelin; SMS, sphingomyelin synthetase; S1P, sphingosine 1-phosphate; SPL, sphingosine 1-phosphate lyase; SphK/SPHK, sphingosine kinase; SPHK1, sphingosine kinase 1; CRC, colorectal cancer; HCC, hepatocellular carcinoma; HNSCC, human head and neck squamous cell carcinoma; iNKT, invariant natural killer T; MDSCs, myeloid-derived suppressor cells; NK, natural killer; Th1, T helper 1 cell; Th2, T helper 2 cell; TNBC, triple negative breast cancer; TAM, tumor-associated macrophage. ER, estrogen receptor; HER2, human epidermal growth factor receptor 2; LN, lymph node; MDR, multidrug resistance; TME, tumor microenvironment; OS, overall survival.
1. Ogretmen B. Sphingolipid metabolism in cancer signalling and therapy. Nat Rev Cancer (2018) 18(1):33–50. doi: 10.1038/nrc.2017.96
2. Sandhoff K. Neuronal sphingolipidoses: Membrane lipids and sphingolipid activator proteins regulate lysosomal sphingolipid catabolism. Biochimie (2016) 130:146–51. doi: 10.1016/j.biochi.2016.05.004
3. Boath A, Graf C, Lidome E, Ullrich T, Nussbaumer P, Bornancin F. Regulation and traffic of ceramide 1-phosphate produced by ceramide kinase: Comparative analysis to glucosylceramide and sphingomyelin. J Biol Chem (2008) 283(13):8517–26. doi: 10.1074/jbc.M707107200
4. Sung H, Ferlay J, Siegel RL, Laversanne M, Soerjomataram I, Jemal A, et al. Global cancer statistics 2020: Globocan estimates of incidence and mortality worldwide for 36 cancers in 185 countries. CA: Cancer J Clin (2021) 71(3):209–49. doi: 10.3322/caac.21660
5. Gupta P, Taiyab A, Hussain A, Alajmi MF, Islam A, Hassan MI. Targeting the sphingosine Kinase/Sphingosine-1-Phosphate signaling axis in drug discovery for cancer therapy. Cancers (Basel) (2021) 13(8):1898. doi: 10.3390/cancers13081898
6. Jiang Y, DiVittore NA, Young MM, Jia Z, Xie K, Ritty TM, et al. Altered sphingolipid metabolism in patients with metastatic pancreatic cancer. Biomolecules (2013) 3(3):435–48. doi: 10.3390/biom3030435
7. Koyanagi S, Kuga M, Soeda S, Hosoda Y, Yokomatsu T, Takechi H, et al. Elevation of De novo ceramide synthesis in tumor masses and the role of microsomal dihydroceramide synthase. Int J Cancer (2003) 105(1):1–6. doi: 10.1002/ijc.11024
8. Karahatay S, Thomas K, Koybasi S, Senkal CE, ElOjeimy S, Liu X, et al. Clinical relevance of ceramide metabolism in the pathogenesis of human head and neck squamous cell carcinoma (Hnscc): Attenuation of C18-ceramide in hnscc tumors correlates with lymphovascular invasion and nodal metastasis. Cancer Lett (2007) 256(1):101–11. doi: 10.1016/j.canlet.2007.06.003
9. Kandyba A, Somova O, Kozlov A, Zubova E, Dudnik L, Alessenko A, et al. Sphingolipids of transplantable rat nephroma-Ra. Biochem Biokhimiia (2000) 65(6):703–6. doi: 10.1016/S0305-1978(99)00080-0
10. Schiffmann S, Sandner J, Birod K, Wobst I, Angioni C, Ruckhäberle E, et al. Ceramide synthases and ceramide levels are increased in breast cancer tissue. Carcinogenesis (2009) 30(5):745–52. doi: 10.1093/carcin/bgp061
11. Selzner M, Bielawska A, Morse MA, Rüdiger HA, Sindram D, Hannun YA, et al. Induction of apoptotic cell death and prevention of tumor growth by ceramide analogues in metastatic human colon cancer. Cancer Res (2001) 61(3):1233–40. doi: 10.1097/00002820-200102000-00011
12. Knapp P, Bodnar L, Blachnio-Zabielska A, Swiderska M, Chabowski A. A. Plasma and ovarian tissue sphingolipids profiling in patients with advanced ovarian cancer. Gynecol Oncol (2017) 147:139–144. doi: 10.1016/j.ygyno.2017.07.143
13. Riboni L, Campanella R, Bassi R, Villani R, Gaini SM, Martinelli-Boneschi F, et al. Ceramide levels are inversely associated with malignant progression of human glial tumors. Glia (2002) 39(2):105–13. doi: 10.1002/glia.10087
14. Koybasi S, Senkal CE, Sundararaj K, Spassieva S, Bielawski J, Osta W, et al. Defects in cell growth regulation by C18: 0-ceramide and longevity assurance gene 1 in human head and neck squamous cell carcinomas. J Biol Chem (2004) 279(43):44311–9. doi: 10.1074/jbc.M406920200
15. Li W, Yu C-P, J-t X, Zhang L, Weng G-X, Zheng H-q, et al. Sphingosine kinase 1 is associated with gastric cancer progression and poor survival of patients. Clin Cancer Res (2009) 15(4):1393–9. doi: 10.1158/1078-0432.CCR-08-1158
16. Wang F, Wu Z. Sphingosine kinase 1 overexpression is associated with poor prognosis and oxaliplatin resistance in hepatocellular carcinoma. Exp Ther Med (2018) 15(6):5371–6. doi: 10.3892/etm.2018.6086
17. Cai H, Xie X, Ji L, Ruan X, Zheng Z. Sphingosine kinase 1: A novel independent prognosis biomarker in hepatocellular carcinoma. Oncol Lett (2017) 13(4):2316–22. doi: 10.3892/ol.2017.5732
18. Malavaud B, Pchejetski D, Mazerolles C, de Paiva GR, Calvet C, Doumerc N, et al. Sphingosine kinase-1 activity and expression in human prostate cancer resection specimens. Eur J Cancer (2010) 46(18):3417–24. doi: 10.1016/j.ejca.2010.07.053
19. Ruckhäberle E, Rody A, Engels K, Gaetje R, von Minckwitz G, Schiffmann S, et al. Microarray analysis of altered sphingolipid metabolism reveals prognostic significance of sphingosine kinase 1 in breast cancer. Breast Cancer Res Treat (2008) 112(1):41–52. doi: 10.1007/s10549-007-9836-9
20. Kapitonov D, Allegood JC, Mitchell C, Hait NC, Almenara JA, Adams JK, et al. Targeting sphingosine kinase 1 inhibits akt signaling, induces apoptosis, and suppresses growth of human glioblastoma cells and xenografts. Cancer Res (2009) 69(17):6915–23. doi: 10.1158/0008-5472.CAN-09-0664
21. Nunes J, Naymark M, Sauer L, Muhammad A, Keun H, Sturge J, et al. Circulating sphingosine-1-Phosphate and erythrocyte sphingosine kinase-1 activity as novel biomarkers for early prostate cancer detection. Br J Cancer (2012) 106(5):909–15. doi: 10.1038/bjc.2012.14
22. Kawakita Y, Motoyama S, Sato Y, Koyota S, Wakita A, Liu J, et al. Sphingosine-1-Phosphate/Sphingosine kinase 1-dependent lymph node metastasis in esophageal squamous cell carcinoma. Surg Today (2017) 47(11):1312–20. doi: 10.1007/s00595-017-1514-x
23. Wang Y, Shen Y, Sun X, Hong TL, Huang LS, Zhong M. Prognostic roles of the expression of sphingosine-1-Phosphate metabolism enzymes in non-small cell lung cancer. Trans Lung Cancer Res (2019) 8(5):674. doi: 10.21037/tlcr.2019.10.04
24. Kato K, Shimasaki M, Kato T, Segami N, Ueda Y. Expression of sphingosine kinase-1 is associated with invasiveness and poor prognosis of oral squamous cell carcinoma. Anticancer Res (2018) 38(3):1361–8. doi: 10.21873/anticanres.12359
25. Deng Y, Hu JC, He SH, Lou B, Ding TB, Yang JT, et al. Sphingomyelin synthase 2 facilitates M2-like macrophage polarization and tumor progression in a mouse model of triple-negative breast cancer. Acta Pharmacol Sin (2021) 42(1):149–59. doi: 10.1038/s41401-020-0419-1
26. Payne AW, Pant DK, Pan T-C, Chodosh LA. Ceramide kinase promotes tumor cell survival and mammary tumor recurrence. Cancer Res (2014) 74(21):6352–63. doi: 10.1158/0008-5472.CAN-14-1292
27. Tsuchida J, Nagahashi M, Nakajima M, Moro K, Tatsuda K, Ramanathan R, et al. Breast cancer sphingosine-1-Phosphate is associated with phospho-sphingosine kinase 1 and lymphatic metastasis. J Surg Res (2016) 205(1):85–94. doi: 10.1016/j.jss.2016.06.022
28. Nagahashi M, Yamada A, Miyazaki H, Allegood JC, Tsuchida J, Aoyagi T, et al. Interstitial fluid sphingosine-1-Phosphate in murine mammary gland and cancer and human breast tissue and cancer determined by novel methods. J Mammary Gland Biol Neoplasia (2016) 21(1-2):9–17. doi: 10.1007/s10911-016-9354-7
29. Wang S, Liang Y, Chang W, Hu B, Zhang Y. Triple negative breast cancer depends on sphingosine kinase 1 (Sphk1)/Sphingosine-1-Phosphate (S1p)/Sphingosine 1-phosphate receptor 3 (S1pr3)/Notch signaling for metastasis. Med Sci monitor: Int Med J Exp Clin Res (2018) 24:1912. doi: 10.12659/msm.905833
30. Ruckhäberle E, Karn T, Hanker L, Gätje R, Metzler D, Holtrich U, et al. Prognostic relevance of glucosylceramide synthase (Gcs) expression in breast cancer. J Cancer Res Clin Oncol (2009) 135(1):81–90. doi: 10.1007/s00432-008-0436-9
31. Yuza K, Nagahashi M, Shimada Y, Nakano M, Tajima Y, Kameyama H, et al. Upregulation of phosphorylated sphingosine kinase 1 expression in colitis-associated cancer. J Surg Res (2018) 231:323–30. doi: 10.1016/j.jss.2018.05.085
32. Markowski AR, Błachnio-Zabielska AU, Guzińska-Ustymowicz K, Markowska A, Pogodzińska K, Roszczyc K, et al. Ceramides profile identifies patients with more advanced stages of colorectal cancer. Biomolecules (2020) 10(4):632. doi: 10.3390/biom10040632
33. Zhang C, Zhou C, Xu J, Xue S. Increased sphingosine kinase 1 expression is associated with poor prognosis in human solid tumors: A meta-analysis. Dis Markers (2022) 2022:8443932. doi: 10.1155/2022/8443932
34. Kohno M, Momoi M, Oo ML, Paik J-H, Lee Y-M, Venkataraman K, et al. Intracellular role for sphingosine kinase 1 in intestinal adenoma cell proliferation. Mol Cell Biol (2006) 26(19):7211–23. doi: 10.1128/MCB.02341-05
35. Pettus BJ, Bielawska A, Subramanian P, Wijesinghe DS, Maceyka M, Leslie CC, et al. Ceramide 1-phosphate is a direct activator of cytosolic phospholipase A2. J Biol Chem (2004) 279(12):11320–6. doi: 10.1074/jbc.M309262200
36. Morigny P, Zuber J, Haid M, Kaltenecker D, Riols F, Lima JDC, et al. High levels of modified ceramides are a defining feature of murine and human cancer cachexia. J Cachexia Sarcopenia Muscle (2020) 11(6):1459–75. doi: 10.1002/jcsm.12626
37. Ogretmen B, Hannun YA. Biologically active sphingolipids in cancer pathogenesis and treatment. Nat Rev Cancer (2004) 4(8):604–16. doi: 10.1038/nrc1411
38. Senkal CE, Ponnusamy S, Manevich Y, Meyers-Needham M, Saddoughi SA, Mukhopadyay A, et al. Alteration of ceramide synthase 6/C16-ceramide induces activating transcription factor 6-mediated endoplasmic reticulum (Er) stress and apoptosis Via perturbation of cellular Ca2+ and Er/Golgi membrane network. J Biol Chem (2011) 286(49):42446–58. doi: 10.1074/jbc.M111.287383
39. Li D, Wang L, Deng R, Tang J, Shen Y, Guo J, et al. The pivotal role of c-jun Nh2-terminal kinase-mediated beclin 1 expression during anticancer agents-induced autophagy in cancer cells. Oncogene (2009) 28(6):886–98. doi: 10.1038/onc.2008.441
40. Muñoz-Guardiola P, Casas J, Megías-Roda E, Solé S, Perez-Montoyo H, Yeste-Velasco M, et al. The anti-cancer drug Abtl0812 induces er stress-mediated cytotoxic autophagy by increasing dihydroceramide levels in cancer cells. Autophagy (2021) 17(6):1349–66. doi: 10.1080/15548627.2020.1761651
41. de Araujo Junior RF, Eich C, Jorquera C, Schomann T, Baldazzi F, Chan AB, et al. Ceramide and palmitic acid inhibit macrophage-mediated epithelial–mesenchymal transition in colorectal cancer. Mol Cell Biochem (2020) 468(1):153–68. doi: 10.1007/s11010-020-03719-5
42. Kim MH, Park JW, Lee EJ, Kim S, Shin SH, Ahn JH, et al. C16−Ceramide and sphingosine 1−Phosphate/S1pr2 have opposite effects on cell growth through mtor signaling pathway regulation. Oncol Rep (2018) 40(5):2977–87. doi: 10.3892/or.2018.6689
43. Park KS, Kim M-K, Lee HY, Kim SD, Lee SY, Kim JM, et al. S1p stimulates chemotactic migration and invasion in Ovcar3 ovarian cancer cells. Biochem Biophys Res Commun (2007) 356(1):239–44. doi: 10.1016/j.bbrc.2007.02.112
44. Truman J-P, García-Barros M, Obeid LM, Hannun YA. Evolving concepts in cancer therapy through targeting sphingolipid metabolism. Biochim Biophys Acta (BBA)-Molecular Cell Biol Lipids (2014) 1841(8):1174–88. doi: 10.1016/j.bbalip.2013.12.013
45. Ryland LK, Fox TE, Liu X, Loughran TP, Kester M. Dysregulation of sphingolipid metabolism in cancer. Cancer Biol Ther (2011) 11(2):138–49. doi: 10.4161/cbt.11.2.14624
46. Chang W-T, Wu C-Y, Lin Y-C, Wu M-T, Su K-L, Yuan S-S, et al. C2-Ceramide-Induced Rb-dominant senescence-like phenotype leads to human breast cancer mcf-7 escape from P53-dependent cell death. Int J Mol Sci (2019) 20(17):4292. doi: 10.3390/ijms20174292
47. Spassieva SD, Mullen TD, Townsend DM, Obeid LM. Disruption of ceramide synthesis by Cers2 down-regulation leads to autophagy and the unfolded protein response. Biochem J (2009) 424(2):273–83. doi: 10.1042/bj20090699
48. Senkal CE, Ponnusamy S, Bielawski J, Hannun YA, Ogretmen B. Antiapoptotic roles of ceramide-Synthase-6-Generated C16-ceramide Via selective regulation of the Atf6/Chop arm of er-Stress-Response pathways. FASEB J (2010) 24(1):296–308. doi: 10.1096/fj.09-135087
49. Zhang Y, Wang H, Chen T, Wang H, Liang X, Zhang Y, et al. C24-ceramide drives gallbladder cancer progression through directly targeting phosphatidylinositol 5-phosphate 4-kinase type-2 gamma to facilitate mammalian target of rapamycin signaling activation. Hepatology (2021) 73(2):692–712. doi: 10.1002/hep.31304
50. Barceló-Coblijn G, Martin ML, de Almeida RF, Noguera-Salvà MA, Marcilla-Etxenike A, Guardiola-Serrano F, et al. Sphingomyelin and sphingomyelin synthase (Sms) in the malignant transformation of glioma cells and in 2-hydroxyoleic acid therapy. Proc Natl Acad Sci (2011) 108(49):19569–74. doi: 10.1073/pnas.1115484108
51. Mitra P, Maceyka M, Payne SG, Lamour N, Milstien S, Chalfant CE, et al. Ceramide kinase regulates growth and survival of A549 human lung adenocarcinoma cells. FEBS Lett (2007) 581(4):735–40. doi: 10.1016/j.febslet.2007.01.041
52. Rivera IG, Ordoñez M, Presa N, Gangoiti P, Gomez-Larrauri A, Trueba M, et al. Ceramide 1-phosphate regulates cell migration and invasion of human pancreatic cancer cells. Biochem Pharmacol (2016) 102:107–19. doi: 10.1016/j.bcp.2015.12.009
53. Cuvillier O, Levade T. Sphingosine 1-phosphate antagonizes apoptosis of human leukemia cells by inhibiting release of cytochrome c and Smac/Diablo from mitochondria. Blood J Am Soc Hematol (2001) 98(9):2828–36. doi: 10.1182/blood.v98.9.2828
54. García-Bernal D, Redondo-Muñoz J, Dios-Esponera A, Chèvre R, Bailón E, Garayoa M, et al. Sphingosine-1-Phosphate activates chemokine-promoted myeloma cell adhesion and migration involving A4β1 integrin function. J Pathol (2013) 229(1):36–48. doi: 10.1002/path.4066
55. Zeng Y, Yao X, Chen L, Yan Z, Liu J, Zhang Y, et al. Sphingosine-1-Phosphate induced epithelial-mesenchymal transition of hepatocellular carcinoma Via an mmp-7/Syndecan-1/Tgf-B autocrine loop. Oncotarget (2016) 7(39):63324. doi: 10.18632/oncotarget.11450
56. Wang W, Hind T, Lam BWS, Herr DR. Sphingosine 1-phosphate signaling induces Snai2 expression to promote cell invasion in breast cancer cells. FASEB J (2019) 33(6):7180–91. doi: 10.1096/fj.201801635R
57. Kim DS, Hwang ES, Lee JE, Kim SY, Kwon SB, Park KC. Sphingosine-1-Phosphate decreases melanin synthesis via sustained erk activation and subsequent mitf degradation. J Cell Sci (2003) 116(Pt 9):1699–706. doi: 10.1242/jcs.00366
58. Shida D, Kitayama J, Yamaguchi H, Yamashita H, Mori K, Watanabe T, et al. Sphingosine 1-phosphate transactivates c-met as well as epidermal growth factor receptor (Egfr) in human gastric cancer cells. FEBS Lett (2004) 577(3):333–8. doi: 10.1016/j.febslet.2004.10.024
59. Zeng Y, Liu X, Yan Z, Xie L. Sphingosine 1-phosphate regulates proliferation, cell cycle and apoptosis of hepatocellular carcinoma cells Via syndecan-1. Prog biophysics Mol Biol (2019) 148:32–8. doi: 10.1016/j.pbiomolbio.2017.11.006
60. Tomizawa S, Tamori M, Tanaka A, Utsumi N, Sato H, Hatakeyama H, et al. Inhibitory effects of ceramide kinase on Rac1 activation, lamellipodium formation, cell migration, and metastasis of A549 lung cancer cells. Biochim Biophys Acta (BBA)-Molecular Cell Biol Lipids (2020) 1865(6):158675. doi: 10.1016/j.bbalip.2020.158675
61. Schwalm S, Erhardt M, Römer I, Pfeilschifter J, Zangemeister-Wittke U, Huwiler A. Ceramide kinase is upregulated in metastatic breast cancer cells and contributes to migration and invasion by activation of pi 3-kinase and akt. Int J Mol Sci (2020) 21(4):1396. doi: 10.3390/ijms21041396
62. Grassmé H, Schwarz H, Gulbins E. Molecular mechanisms of ceramide-mediated Cd95 clustering. Biochem Biophys Res Commun (2001) 284(4):1016–30. doi: 10.1006/bbrc.2001.5045
63. Soans E, Evans SC, Cipolla C, Fernandes E. Characterizing the sphingomyelinase pathway triggered by prima-1 derivatives in lung cancer cells with differing P53 status. Anticancer Res (2014) 34(7):3271–83. doi: 10.1186/1477-7819-12-198
64. Birbes H, Luberto C, Hsu Y-T, El Bawab S, Hannun YA, Obeid LM. A mitochondrial pool of sphingomyelin is involved in tnfα-induced bax translocation to mitochondria. Biochem J (2005) 386(3):445–51. doi: 10.1042/BJ20041627
65. Zheng K, Chen Z, Feng H, Chen Y, Zhang C, Yu J, et al. Sphingomyelin synthase 2 promotes an aggressive breast cancer phenotype by disrupting the homoeostasis of ceramide and sphingomyelin. Cell Death Dis (2019) 10(3):1–11. doi: 10.1038/s41419-019-1303-0
66. Lafont E, Milhas D, Carpentier S, Garcia V, Jin ZX, Umehara H, et al. Caspase-mediated inhibition of sphingomyelin synthesis is involved in fasl-triggered cell death. Cell Death Differ (2010) 17(4):642–54. doi: 10.1038/cdd.2009.130
67. Oskouian B, Sooriyakumaran P, Borowsky AD, Crans A, Dillard-Telm L, Tam YY, et al. Sphingosine-1-Phosphate lyase potentiates apoptosis Via P53-and P38-dependent pathways and is down-regulated in colon cancer. Proc Natl Acad Sci (2006) 103(46):17384–9. doi: 10.1073/pnas.0600050103
68. Xu C, Zhang W, Liu S, Wu W, Qin M, Huang J. Activation of the Sphk1/Erk/P−Erk pathway promotes autophagy in colon cancer cells. Oncol Lett (2018) 15(6):9719–24. doi: 10.3892/ol.2018.8588
69. Pan J, Tao YF, Zhou Z, Cao BR, Wu SY, Zhang YL, et al. An novel role of sphingosine kinase-1 (Sphk1) in the invasion and metastasis of esophageal carcinoma. J Transl Med (2011) 9:157. doi: 10.1186/1479-5876-9-157
70. Liu H, Ma Y, He HW, Zhao WL, Shao RG. Sphk1 (Sphingosine kinase 1) induces epithelial-mesenchymal transition by promoting the autophagy-linked lysosomal degradation of Cdh1/E-cadherin in hepatoma cells. Autophagy (2017) 13(5):900–13. doi: 10.1080/15548627.2017.1291479
71. Lee CF, Dang A, Hernandez E, Pong RC, Chen B, Sonavane R, et al. Activation of sphingosine kinase by lipopolysaccharide promotes prostate cancer cell invasion and metastasis Via Sphk1/S1pr4/Matriptase. Oncogene (2019) 38(28):5580–98. doi: 10.1038/s41388-019-0833-3
72. Hii L-W, Chung FF-L, Mai CW, Yee ZY, Chan HH, Raja VJ, et al. Sphingosine kinase 1 regulates the survival of breast cancer stem cells and non-stem breast cancer cells by suppression of Stat1. Cells (2020) 9(4):886. doi: 10.3390/cells9040886
73. Lee Y-S, Choi K-M, Lee S, Sin D-M, Yoo K-S, Lim Y, et al. Myriocin, a serine palmitoyltransferase inhibitor, suppresses tumor growth in a murine melanoma model by inhibiting De novo sphingolipid synthesis. Cancer Biol Ther (2012) 13(2):92–100. doi: 10.4161/cbt.13.2.18870
74. Liu SQ, Xu CY, Wu WH, Fu ZH, He SW, Qin MB, et al. Sphingosine kinase 1 promotes the metastasis of colorectal cancer by inducing the Epithelial−Mesenchymal transition mediated by the Fak/Akt/Mmps axis. Int J Oncol (2019) 54(1):41–52. doi: 10.3892/ijo.2018.4607
75. Nagahashi M, Yamada A, Katsuta E, Aoyagi T, Huang WC, Terracina KP, et al. Targeting the Sphk1/S1p/S1pr1 axis that links obesity, chronic inflammation, and breast cancer metastasis. Cancer Res (2018) 78(7):1713–25. doi: 10.1158/0008-5472.Can-17-1423
76. LeBlanc FR, Pearson JM, Tan SF, Cheon H, Xing JC, Dunton W, et al. Sphingosine kinase-2 is overexpressed in Large granular lymphocyte leukaemia and promotes survival through mcl-1. Br J Haematol (2020) 190(3):405–17. doi: 10.1111/bjh.16530
77. Liu Y-Y, Gupta V, Patwardhan GA, Bhinge K, Zhao Y, Bao J, et al. Glucosylceramide synthase upregulates Mdr1 expression in the regulation of cancer drug resistance through csrc and B-catenin signaling. Mol Cancer (2010) 9(1):1–15. doi: 10.1186/1476-4598-9-145
78. Sattar RSA, Sumi MP, Nimisha, Apurva, Kumar A, Sharma AK, et al. S1p signaling, its interactions and cross-talks with other partners and therapeutic importance in colorectal cancer. Cell Signal (2021) 86:110080. doi: 10.1016/j.cellsig.2021.110080
79. Sanchez T, Skoura A, Wu MT, Casserly B, Harrington EO, Hla T. Induction of vascular permeability by the sphingosine-1-Phosphate receptor–2 (S1p2r) and its downstream effectors rock and pten. Arteriosclerosis thrombosis Vasc Biol (2007) 27(6):1312–8. doi: 10.1161/ATVBAHA.107.143735
80. Chen Y, Zhang P, Xu S-C, Yang L, Voss U, Ekblad E, et al. Enhanced colonic tumorigenesis in alkaline sphingomyelinase (Npp7) knockout mice. Mol Cancer Ther (2015) 14(1):259–67. doi: 10.1158/1535-7163.MCT-14-0468-T
81. Long J, Yao Z, Sui Y, Fang S. Sphk1 promotes cancer progression through activating Jak/Stat pathway and up-regulating S1pr1 expression in colon cancer cells. Anticancer Agents Med Chem (2022) 22(2):254–60. doi: 10.2174/1871520621666210401105344
82. Xu C-Y, Liu S-Q, Qin M-B, Zhuge C-F, Qin L, Qin N, et al. Sphk1 modulates cell migration and emt-related marker expression by regulating the expression of p-fak in colorectal cancer cells. Int J Mol Med (2017) 39(5):1277–84. doi: 10.3892/ijmm.2017.2921
83. Camacho L, Ouro A, Gomez-Larrauri A, Carracedo A, Gomez-Muñoz A. Implication of ceramide Kinase/C1p in cancer development and progression. Cancers (Basel) (2022) 14(1):227. doi: 10.3390/cancers14010227
84. Ouro A, Arana L, Riazy M, Zhang P, Gomez-Larrauri A, Steinbrecher U, et al. Vascular endothelial growth factor mediates ceramide 1-Phosphate-Stimulated macrophage proliferation. Exp Cell Res (2017) 361(2):277–83. doi: 10.1016/j.yexcr.2017.10.027
85. Pascuali N, Scotti L, Di Pietro M, Oubiña G, Bas D, May M, et al. Ceramide-1-Phosphate has protective properties against cyclophosphamide-induced ovarian damage in a mice model of premature ovarian failure. Hum Reprod (2018) 33(5):844–59. doi: 10.1093/humrep/dey045
86. Morad SA, Levin JC, Shanmugavelandy SS, Kester M, Fabrias G, Bedia C, et al. Ceramide–antiestrogen nanoliposomal combinations–novel impact of hormonal therapy in hormone-insensitive breast cancer. Mol Cancer Ther (2012) 11(11):2352–61. doi: 10.1158/1535-7163.MCT-12-0594
87. Watters RJ, Fox TE, Tan S-F, Shanmugavelandy S, Choby JE, Broeg K, et al. Targeting glucosylceramide synthase synergizes with C6-ceramide nanoliposomes to induce apoptosis in natural killer cell leukemia. Leukemia lymphoma (2013) 54(6):1288–96. doi: 10.3109/10428194.2012.752485
88. Li G, Liu D, Kimchi ET, Kaifi JT, Qi X, Manjunath Y, et al. Nanoliposome C6-ceramide increases the anti-tumor immune response and slows growth of liver tumors in mice. Gastroenterology (2018) 154(4):1024–36.e9. doi: 10.1053/j.gastro.2017.10.050
89. Wang S-B, Ma Y-Y, Chen X-Y, Zhao Y-Y, Mou X-Z. Ceramide-graphene oxide nanoparticles enhance cytotoxicity and decrease hcc xenograft development: A novel approach for targeted cancer therapy. Front Pharmacol (2019) 10:69. doi: 10.3389/fphar.2019.00069
90. Itoh M, Kitano T, Watanabe M, Kondo T, Yabu T, Taguchi Y, et al. Possible role of ceramide as an indicator of chemoresistance: Decrease of the ceramide content Via activation of glucosylceramide synthase and sphingomyelin synthase in chemoresistant leukemia. Clin Cancer Res (2003) 9(1):415–23. doi: 10.1109/APSEC.2005.12
91. Liu Y-Y, Patwardhan GA, Xie P, Gu X, Giuliano AE, Cabot MC. Glucosylceramide synthase, a factor in modulating drug resistance, is overexpressed in metastatic breast carcinoma. Int J Oncol (2011) 39(2):425–31. doi: 10.3892/ijo.2011.1052
92. Lin HM, Mak B, Yeung N, Huynh K, Meikle TG, Mellett NA, et al. Overcoming enzalutamide resistance in metastatic prostate cancer by targeting sphingosine kinase. EBioMedicine (2021) 72:103625. doi: 10.1016/j.ebiom.2021.103625
93. Shaw J, Costa-Pinheiro P, Patterson L, Drews K, Spiegel S, Kester M. Novel sphingolipid-based cancer therapeutics in the personalized medicine era. Adv Cancer Res (2018) 140:327–66. doi: 10.1016/bs.acr.2018.04.016
94. Bai A, Szulc ZM, Bielawski J, Mayroo N, Liu X, Norris J, et al. Synthesis and bioevaluation of Ω-N-Amino analogs of B13. Bioorganic medicinal Chem (2009) 17(5):1840–8. doi: 10.1016/j.bmc.2009.01.057
95. Pastukhov O, Schwalm S, Zangemeister-Wittke U, Fabbro D, Bornancin F, Japtok L, et al. The ceramide kinase inhibitor nvp-231 inhibits breast and lung cancer cell proliferation by inducing m phase arrest and subsequent cell death. Br J Pharmacol (2014) 171(24):5829–44. doi: 10.1111/bph.12886
96. Antoon JW, White MD, Burow ME, Beckman BS. Dual inhibition of sphingosine kinase isoforms ablates tnf-induced drug resistance. Oncol Rep (2012) 27(6):1779–86. doi: 10.3892/or.2012.1743
97. White C, Alshaker H, Cooper C, Winkler M, Pchejetski D. The emerging role of Fty720 (Fingolimod) in cancer treatment. Oncotarget (2016) 7(17):23106–27. doi: 10.18632/oncotarget.7145
98. Wallington-Beddoe CT, Hewson J, Bradstock KF, Bendall LJ. Fty720 produces caspase-independent cell death of acute lymphoblastic leukemia cells. Autophagy (2011) 7(7):707–15. doi: 10.4161/auto.7.7.15154
99. Grazide S, Terrisse A-D, Lerouge S, Laurent G, Jaffrézou J-P. Cytoprotective effect of glucosylceramide synthase inhibition against daunorubicin-induced apoptosis in human leukemic cell lines. J Biol Chem (2004) 279(18):18256–61. doi: 10.1074/jbc.M314105200
100. Liu Y-Y, Hill RA, Li Y-T. Ceramide glycosylation catalyzed by glucosylceramide synthase and cancer drug resistance. Adv Cancer Res (2013) 117:59–89. doi: 10.1016/B978-0-12-394274-6.00003-0
101. Morad SAF, Cabot MC. The onus of sphingolipid enzymes in cancer drug resistance. Adv Cancer Res (2018) 140:235–63. doi: 10.1016/bs.acr.2018.04.013
102. Giussani P, Prinetti A, Tringali C. The role of sphingolipids in cancer immunotherapy. Int J Mol Sci (2021) 22(12):6492. doi: 10.3390/ijms22126492
103. Liu F, Li X, Lu C, Bai A, Bielawski J, Bielawska A, et al. Ceramide activates lysosomal cathepsin b and cathepsin d to attenuate autophagy and induces er stress to suppress myeloid-derived suppressor cells. Oncotarget (2016) 7(51):83907–25. doi: 10.18632/oncotarget.13438
104. Ghosh S, Juin SK, Nandi P, Majumdar SB, Bose A, Baral R, et al. Pkcζ mediated anti-proliferative effect of C2 ceramide on neutralization of the tumor microenvironment and melanoma regression. Cancer Immunol Immunother (2020) 69(4):611–27. doi: 10.1007/s00262-020-02492-0
105. Qi X, Wu F, Kim SH, Kaifi JT, Kimchi ET, Snyder H, et al. Nanoliposome C6-ceramide in combination with anti-Ctla4 antibody improves anti-tumor immunity in hepatocellular cancer. FASEB J (2022) 36(4):e22250. doi: 10.1096/fj.202101707R
106. Riccitelli E, Giussani P, Di Vito C, Condomitti G, Tringali C, Caroli M, et al. Extracellular sphingosine-1-Phosphate: A novel actor in human glioblastoma stem cell survival. PLoS One (2013) 8(6):e68229. doi: 10.1371/journal.pone.0068229
107. Ghosh S, Juin SK, Majumdar S. Cancer stem cells and ceramide signaling: The cutting edges of immunotherapy. Mol Biol Rep (2020) 47(10):8101–11. doi: 10.1007/s11033-020-05790-z
108. Oskouian B, Saba JD. Cancer treatment strategies targeting sphingolipid metabolism. Adv Exp Med Biol (2010) 688:185–205. doi: 10.1007/978-1-4419-6741-1_13
109. Ghosh S, Jawed JJ, Halder K, Banerjee S, Chowdhury BP, Saha A, et al. Tnfα mediated ceramide generation triggers cisplatin induced apoptosis in B16f10 melanoma in a pkcδ independent manner. Oncotarget (2018) 9(102):37627–46. doi: 10.18632/oncotarget.26478
110. von Wenckstern H, Zimmermann K, Kleuser B. The role of the lysophospholipid sphingosine 1-phosphate in immune cell biology. Archivum immunologiae therapiae experimentalis (2006) 54(4):239–51. doi: 10.1007/s00005-006-0028-9
111. Weigert A, Johann AM, von Knethen A, Schmidt H, Geisslinger G, Brüne B. Apoptotic cells promote macrophage survival by releasing the antiapoptotic mediator sphingosine-1-Phosphate. Blood (2006) 108(5):1635–42. doi: 10.1182/blood-2006-04-014852
112. Rodriguez YI, Campos LE, Castro MG, Aladhami A, Oskeritzian CA, Alvarez SE. Sphingosine-1 phosphate: A new modulator of immune plasticity in the tumor microenvironment. Front Oncol (2016) 6:218. doi: 10.3389/fonc.2016.00218
113. Wang Y, Bhave MS, Yagita H, Cardell SL. Natural killer T-cell agonist A-galactosylceramide and pd-1 blockade synergize to reduce tumor development in a preclinical model of colon cancer. Front Immunol (2020) 11:581301. doi: 10.3389/fimmu.2020.581301
114. Hollmann C, Wiese T, Dennstädt F, Fink J, Schneider-Schaulies J, Beyersdorf N. Translational approaches targeting ceramide generation from sphingomyelin in T cells to modulate immunity in humans. Front Immunol (2019) 10:2363. doi: 10.3389/fimmu.2019.02363
115. El-Hindi K, Brachtendorf S, Hartel JC, Oertel S, Birod K, Trautmann S, et al. Ceramide synthase 5 deficiency aggravates dextran sodium sulfate-induced colitis and colon carcinogenesis and impairs T-cell activation. Cancers (Basel) (2020) 12(7):1753. doi: 10.3390/cancers12071753
116. Chakraborty P, Vaena SG, Thyagarajan K, Chatterjee S, Al-Khami A, Selvam SP, et al. Pro-survival lipid sphingosine-1-Phosphate metabolically programs T cells to limit anti-tumor activity. Cell Rep (2019) 28(7):1879–93.e7. doi: 10.1016/j.celrep.2019.07.044
117. Wang K, Xu R, Snider AJ, Schrandt J, Li Y, Bialkowska AB, et al. Alkaline ceramidase 3 deficiency aggravates colitis and colitis-associated tumorigenesis in mice by hyperactivating the innate immune system. Cell Death Dis (2016) 7(3):e2124. doi: 10.1038/cddis.2016.36
118. Montfort A, Bertrand F, Rochotte J, Gilhodes J, Filleron T, Milhès J, et al. Neutral sphingomyelinase 2 heightens anti-melanoma immune responses and anti-Pd-1 therapy efficacy. Cancer Immunol Res (2021) 9(5):568–82. doi: 10.1158/2326-6066.Cir-20-0342
119. Rahman A, Pallichankandy S, Thayyullathil F, Galadari S. Critical role of H2o2 in mediating sanguinarine-induced apoptosis in prostate cancer cells Via facilitating ceramide generation, Erk1/2 phosphorylation, and par-4 cleavage. Free Radical Biol Med (2019) 134:527–44. doi: 10.1016/j.freeradbiomed.2019.01.039
120. Salma Y, Lafont E, Therville N, Carpentier S, Bonnafé M-J, Levade T, et al. The natural marine anhydrophytosphingosine, jaspine b, induces apoptosis in melanoma cells by interfering with ceramide metabolism. Biochem Pharmacol (2009) 78(5):477–85. doi: 10.1016/j.bcp.2009.05.002
121. Park T-S, Rosebury W, Kindt EK, Kowala MC, Panek RL. Serine palmitoyltransferase inhibitor myriocin induces the regression of atherosclerotic plaques in hyperlipidemic apoe-deficient mice. Pharmacol Res (2008) 58(1):45–51. doi: 10.1016/j.phrs.2008.06.005
122. Choi KE, Jung YS, Kim DH, Song JK, Kim JY, Jung YY, et al. Myriocin induces apoptotic lung cancer cell death Via activation of Dr4 pathway. Arch pharmacal Res (2014) 37(4):501–11. doi: 10.1007/s12272-013-0315-z
123. Kono K, Tanaka M, Ono Y, Hosoya T, Ogita T, Kohama T. S-15183a and b, new sphingosine kinase inhibitors, produced by a fungus. J antibiotics (2001) 54(5):415–20. doi: 10.7164/antibiotics.54.415
124. Kono K, Tanaka M, Ogita T, Hosoya T, Kohama T. F-12509a, a new sphingosine kinase inhibitor, produced by a discomycete. J Antibiotics (2000) 53(5):459–66. doi: 10.7164/antibiotics.53.459
125. Kono K, Tanaka M, Ogita T, Kohama T. Characterization of b-5354c, a new sphingosine kinase inhibitor, produced by a marine bacterium. J antibiotics (2000) 53(8):759–64. doi: 10.7164/antibiotics.53.759
126. Pchejetski D, Doumerc N, Golzio M, Naymark M, Teissié J, Kohama T, et al. Chemosensitizing effects of sphingosine kinase-1 inhibition in prostate cancer cell and animal models. Mol Cancer Ther (2008) 7(7):1836–45. doi: 10.1158/1535-7163.MCT-07-2322
127. Sauer L, Nunes J, Salunkhe V, Skalska L, Kohama T, Cuvillier O, et al. Sphingosine kinase 1 inhibition sensitizes hormone-resistant prostate cancer to docetaxel. Int J Cancer (2009) 125(11):2728–36. doi: 10.1002/ijc.24640
128. Batova A, Altomare D, Creek KE, Naviaux RK, Wang L, Li K, et al. Englerin a induces an acute inflammatory response and reveals lipid metabolism and er stress as targetable vulnerabilities in renal cell carcinoma. PLoS One (2017) 12(3):e0172632. doi: 10.1371/journal.pone.0172632
129. Li JX, Li RZ, Sun A, Zhou H, Neher E, Yang JS, et al. Metabolomics and integrated network pharmacology analysis reveal tricin as the active anti-cancer component of weijing decoction by suppression of prkca and sphingolipid signaling. Pharmacol Res (2021) 171:105574. doi: 10.1016/j.phrs.2021.105574
130. Signorelli P, Munoz-Olaya JM, Gagliostro V, Casas J, Ghidoni R, Fabriàs G. Dihydroceramide intracellular increase in response to resveratrol treatment mediates autophagy in gastric cancer cells. Cancer Lett (2009) 282(2):238–43. doi: 10.1016/j.canlet.2009.03.020
131. Xiang Y, Guo Z, Zhu P, Chen J, Huang Y. Traditional Chinese medicine as a cancer treatment: Modern perspectives of ancient but advanced science. Cancer Med (2019) 8(5):1958–75. doi: 10.1002/cam4.2108
132. Guo W, Tan H-Y, Wang N, Feng Y. Chinese Medicines for cancer treatment from the metabolomics perspective. Metabolomics-New Insights into Biol Med IntechOpen (2019) . 8(5):1958–75. doi: 10.1002/cam4.2108
133. Liu J, Wang S, Zhang Y, Fan Ht, Lin Hs. Traditional c hinese medicine and cancer: History, present situation, and development. Thorac Cancer (2015) 6(5):561–9. doi: 10.1111/1759-7714.12270
134. Ersöz N, Adan A. Resveratrol triggers anti-proliferative and apoptotic effects in Flt3-Itd-Positive acute myeloid leukemia cells Via inhibiting ceramide catabolism enzymes. Med Oncol (2022) 39(3):35. doi: 10.1007/s12032-021-01627-2
135. Wu H, Chen Y, Li Q, Gao Y, Zhang X, Tong J, et al. Intervention effect of qi-Yu-San-Long decoction on Lewis lung carcinoma in C57bl/6 mice: Insights from uplc–Qtof/Ms-Based metabolic profiling. J Chromatogr B (2018) 1102:23–33. doi: 10.1016/j.jchromb.2018.10.013
Keywords: sphingolipid metabolism, enzymes, cancer, immunotherapy, anticancer
Citation: Li R-Z, Wang X-R, Wang J, Xie C, Wang X-X, Pan H-D, Meng W-Y, Liang T-L, Li J-X, Yan P-Y, Wu Q-B, Liu L, Yao X-J and Leung EL-H (2022) The key role of sphingolipid metabolism in cancer: New therapeutic targets, diagnostic and prognostic values, and anti-tumor immunotherapy resistance. Front. Oncol. 12:941643. doi: 10.3389/fonc.2022.941643
Received: 11 May 2022; Accepted: 24 June 2022;
Published: 27 July 2022.
Edited by:
Margarita Ivanova, Lysosomal and Rare Disorders Research and Treatment Center, United StatesReviewed by:
Alberto Ouro, Health Research Institute of Santiago de Compostela (IDIS), SpainCopyright © 2022 Li, Wang, Wang, Xie, Wang, Pan, Meng, Liang, Li, Yan, Wu, Liu, Yao and Leung. This is an open-access article distributed under the terms of the Creative Commons Attribution License (CC BY). The use, distribution or reproduction in other forums is permitted, provided the original author(s) and the copyright owner(s) are credited and that the original publication in this journal is cited, in accordance with accepted academic practice. No use, distribution or reproduction is permitted which does not comply with these terms.
*Correspondence: Xiao-Jun Yao, eGp5YW9AbXVzdC5lZHUubW8=; Liang Liu, bGxpdUBndWNtLmVkdS5jbg==; Elaine Lai-Han Leung, bGhsZXVuZ0B1bS5lZHUubW8=
†These authors have contributed equally to this work
Disclaimer: All claims expressed in this article are solely those of the authors and do not necessarily represent those of their affiliated organizations, or those of the publisher, the editors and the reviewers. Any product that may be evaluated in this article or claim that may be made by its manufacturer is not guaranteed or endorsed by the publisher.
Research integrity at Frontiers
Learn more about the work of our research integrity team to safeguard the quality of each article we publish.