- 1Department of Implantology, Hospital of Stomatology, Jilin University, Changchun, China
- 2University of Science and Technology of China, Hefei, China
- 3Changchun Institute of Applied Chemistry, Chinese Academy of Sciences, Changchun, China
The aggressive growth of cancer cells brings extreme challenges to cancer therapy while triggering the exploration of the application of multimodal therapy methods. Multimodal tumor therapy based on photothermal nanomaterials is a new technology to realize tumor cell thermal ablation through near-infrared light irradiation with a specific wavelength, which has the advantages of high efficiency, less adverse reactions, and effective inhibition of tumor metastasis compared with traditional treatment methods such as surgical resection, chemotherapy, and radiotherapy. Photothermal nanomaterials have gained increasing interest due to their potential applications, remarkable properties, and advantages for tumor therapy. In this review, recent advances and the common applications of photothermal nanomaterials in multimodal tumor therapy are summarized, with a focus on the different types of photothermal nanomaterials and their application in multimodal tumor therapy. Moreover, the challenges and future applications have also been speculated.
1 Introduction
Cancer poses a serious threat to human health worldwide, despite the developments in modern medical technology. Cancer is difficult or impossible to cure because it involves various genetic changes and cell abnormalities. Moreover, its complexity and heterogeneity promote the aggressive growth of cancer cells, resulting in significant incidence and mortality rates (1–3). The three traditional methods of tumor treatment include surgery, radiotherapy, and chemotherapy. However, due to severe surgical trauma, nonspecific and excessive radiation, and the irreplaceable defects of these therapies in targeting, bio-compatibility, multidrug resistance, and drug accumulation, patients may suffer from serious physiological side effects, resulting in poor quality of life and difficulty in achieving the target treatment effect (4–6).
These treatment deficiencies have inspired the development of new, precise, and more effective treatment strategies for tumors. For example, several emerging treatment methods, such as photodynamic therapy (PDT) (7, 8), photothermal therapy (PTT) (9, 10), and photoacoustic therapy (11, 12) have improved or can potentially improve therapeutic outcomes. Among them, PTT is a new type of minimally invasive tumor light therapy that has developed rapidly in recent years. It mainly uses photothermal conversion nanomaterials with strong absorption in the near-infrared light region (wavelength range 700–1300 nm) (13–15) to convert the absorbed light energy into heat energy effectively under the irradiation of the near-infrared laser, resulting in an increase in the temperature of local tumor tissues up to 40–45°C (hyperthermia) or above 45°C (thermal ablation) (14, 16). This results in degeneration and necrosis of tumor cells to achieve the goal of tumor therapy. The destruction of tumor tissue by PTT mainly occurs through killing tumor cells and destroying tumor blood vessels. The ability of tumor cells to tolerate high temperatures is much lower than that of normal cells. Specifically, the thermal lethal temperature of most tumor cells is between 42 and 43°C, while normal cells can tolerate such temperatures for a prolonged period. Therefore, the local hyperthermia produced by PTT can selectively kill tumor cells and cause irreversible damage, while normal cells are not damaged. Regarding blood vessels, compared with the blood vessels of normal tissue, those of tumor tissue have abnormal morphological growth, imperfect tissue and function, and are prone to rupture when the temperature and pressure increase. This causes tumor tissue to be more prone to damage by hyperthermia. Thus, PTT can effectively destroy tumor blood vessels, killing tumor cells without damaging normal tissues or causing systemic toxic reactions. Because PTT has the advantages of rapid targeted killing, being minimally invasive, and minimal toxic side effects, it is also known as “green therapy,” which carries significant potential in the field of alternative surgical resection (14, 17, 18).
It has been reported that PTT requires a temperature above 50°C to achieve tumor thermal ablation. In addition, cancer cells treated at low temperatures (around 43°C) may survive through self-repair of their heat shock proteins (HSPs), which could lead to treatment resistance and reduce treatment efficiency (19, 20). In recent years, the great progress in nanomaterials, medicine, and biology has promoted the application of nanomaterials in tumor therapy (21, 22). Moreover, great progress has been made in the construction of multifunctional photothermal nanomaterials, which can integrate a variety of treatment modes into a single nano platform. Compared with monotherapy, the combination of multiple therapies usually shows superiority in therapeutic effect. This advanced synergistic therapy can not only maintain the advantages of non-invasive, low toxicity, and convenient administration of PTT, but also relieve the problems of non-selectivity and multidrug resistance of traditional chemotherapy, and has achieved good therapeutic results (20).
This review will focus on the research progress of photothermal nanomaterials in multimodal tumor therapy and consists of a brief introduction to the classification of photothermal nanomaterials and their relative merits. Subsequently, multimodal treatments of tumors based on photothermal nanomaterials are clarified in detail. Finally, an outlook is provided to address recent challenges and suggest better treatment applications and research focuses to pursue new opportunities ahead.
2 Classification of Photothermal Nanomaterials
The goal of PTT is to make use of the hyperthermic effect of photothermal agents (PTAs), which can absorb light energy and convert it into heat energy, raising the temperature of the lesion site and ultimately causing the death of tumor cells (23, 24). To reduce the influence of localized high temperatures on normal tissue, near-infrared (NIR) light is usually selected for PTT because it has less tissue absorption and scattering and is able to penetrate deep tissue (15, 25). In addition, the ideal photothermal material should have higher photothermal conversion efficiency (PCE) and be accumulated effectively in tumor tissue (26). With the progress of PTT research and the rapid development of nanomaterials, photothermal nanomaterials have been more widely used than other photothermal materials because of their higher PCE and ability to be used in a multimodal tumor therapy platform (27–29). This review summarizes the common photothermal nanomaterials, which are divided into inorganic, organic, and composite photothermal nanomaterials (Table 1).
2.1 Inorganic Photothermal Nanomaterials
2.1.1 Precious Metal Nanomaterials
Precious metal nanomaterials, including gold, silver, palladium, and platinum, are considered to be simple and effective PTAs (23, 67, 68) due to their strong surface plasmon resonance (SPR), synthetic tunability, biological imaging potential, and excellent photothermal properties, such as high PCE in the high-absorption cross-section and NIR region.
Studies have shown that gold is one of the most popular nanomaterials for mediating PTT (69, 70), as it has good biocompatibility and low cytotoxicity (71). The photothermal conversion phenomenon in gold nanoparticles (GNPs) is based on the collective oscillation of free electrons on the surface of GNPs under electromagnetic radiation. The local area around GNPs is heated by electronic excitation and relaxation, which leads to the destruction of tumor tissue (72). At present, several gold nanomaterials with unique size and morphology have been developed, including nanorods, nanospheres, nanostars, nanocages, and nanoshells, among others. Choe et al. (30) loaded high concentration gold nanoparticles into mesoporous silica nanocapsules to form yolk-shell-structured gold nanospheres (aAuYSs) to study their photothermal effect on drug-resistant ovarian cancer cells. Under 808-nm laser irradiation, the cultured cancer cells were eliminated when the concentration of aAuYSs was 300 μg/mL. Moreover, in vivo experiments showed that after the combined treatment of aAuYSs and doxorubicin (Dox), the tumor volume and size were significantly reduced, and the number of Ki-67-positive proliferating cancer cells sharply decreased, indicating that aAuYSs can be used as a multifunctional photothermal nanoplatform for PTT and combined therapy.
Silver nanoparticles (SNPs), another type of precious metal nanomaterial, have been widely used due to their unique properties, such as controllable size and shape, easy modification, and excellent optoelectronic properties. Similar to GNPs, the SPR of SNPs can be adjusted to the infrared region by changing their size and shape (73). Additionally, Kim et al. (31) prepared SNPs coated with bovine serum albumin (BSA) (BSA-SNPs), which could internalize and kill melanoma cells by inducing ROS through cell analysis. These nanoparticles were also found to play a potential role in inhibiting angiogenesis. In addition, BSA-SNPs showed a significant increase in the temperature of a suspension under the irradiation of a laser at 690 nm and had a strong photothermal conversion capability, which could be used for photothermal cancer therapy.
Palladium-based nanomaterials, such as palladium nanosheets (74), porous/hollow palladium nanoparticles (75), and palladium@M (M=Ag, Au, Pt, SiO2, ZIF-8) (76–80) nanocomposites, also show strong absorption in the NIR region, as well as ideal PCE, excellent photothermal stability, and good biocompatibility (81). Therefore, palladium-based photothermal nanomaterials have become an option for cancer imaging contrast agents and therapeutic agents. Chen et al. (32) designed palladium nanosheets with a thickness of 1.8 nm and a diameter of 5–80 nm to evaluate the effect of size on the biological behavior of these nanosheets through cell and animal model experiments. The experimental results showed that compared with the large palladium nanowires, the smaller nanowires demonstrated a better photothermal effect under ultra-low laser irradiation. In addition, in vivo experiments revealed that 5-nm palladium nanosheets could escape the reticuloendothelial system with a longer blood half-life and be excreted from the kidneys, while the large nanosheets accumulated in the liver and spleen.
As a photothermal nanomaterial, platinum nanoparticles (PtNPs) slowly and continuously increase the temperature with light irradiation, not exceeding 46°C, which can effectively avoid normal cell damage (82, 83). Apart from good optical and photothermal stability, PtNPs can also be involved in the design of multimodal tumor treatment platforms, which can be used in combination with chemotherapy or radiotherapy (84, 85). Zhou et al. synthesized dendritic platinum-copper alloy nanoparticles (DPCNs) as a multimodal, therapeutic, tumor imaging platform (33). The PTT in vitro assay revealed that DPCNs ingested by PC-9 cells could effectively kill cancer cells under NIR irradiation. In addition, compared with the control group treated with DPCNs/NIR or Dox alone, the killing rate of cancer cells treated with DPCNs/Dox and irradiated with NIR laser was higher, indicating that DPCNs have potential for photothermal and chemotherapy.
2.1.2 Transition Metal Dichalcogenide Nanomaterials
Transition metal dichalcogenides (TMDCs) are usually composed of one layer of transition metal atoms and two layers of chalcogenide atoms, and their generalized formula is MX2. M refers to the transition metals of groups 4–10, such as copper, molybdenum, tungsten, titanium, etc., while X refers to a chalcogen (86). It has been found that monolayer TMDCs exhibit strong NIR absorption, good PCE, and excellent photothermal stability (87, 88), giving TMDCs the potential to be used as PTAs (89).
In recent years, copper nanomaterials have been widely used in cancer therapy (90). Among those used in PTT for cancer, such as copper selenide, copper telluride, and copper oxide, copper sulfide is the most explored (91). It has been found that as a P-type semiconductor, copper chalcogenide nanomaterials have composition-dependent localized SPR and ideal PCE in the NIR region (92, 93). Moreover, Huang et al. (39) combined monoterpenoid sensitizer, borneol, and NIR-II PTA copper sulfide to make thermo-responsive vehicle NB/CuS@PCMNPs. Under the irradiation of a 1060-nm laser, the high temperature produced by copper sulfide nanoparticles can be used in PTT. The results of animal experiments showed that NB/CuS@PCMNPs could aggregate in the tumor site and significantly inhibit tumor growth.
Titanium disulfide is another common material for TMDCs with excellent stability, electrical conductivity, and strong absorption in the NIR window (41). In addition, due to the local SPR effect, the absorption peak of these nanosheets can be shifted from red to the range of 1000–1350 nm by adjusting the thickness and width of the nanowires (94). Fu et al. (41) made a multifunctional NIR-II nano-preparation based on titanium disulfide, which can be used in magnetic targeted NIR-II photoacoustic/magnetic resonance imaging-guided synergistic photothermal-immune combination therapy. The results of in vivo experiments showed that the primary tumors in the group that underwent PTT combined with immunotherapy disappeared without recurrence after 16 days of treatment. This significantly inhibited the tumor growth rate, indicating that titanium disulfide has great potential in the field of PTT combined with immunotherapy and imaging.
In addition, the crystal structure of molybdenum disulfide is a honeycomb, similar to graphene, which can be obtained through stripping or synthesis and has a variety of forms, such as nanosheets and quantum dots, among others (95–98). It has been found that molybdenum disulfide nanoparticles have become commonly used PTAs in cancer treatment due to their good biocompatibility, strong SPR, excellent PCE, and low production cost (99). Liu et al. (40) synthesized a mesoporous core-shell structure with molybdenum disulfide as the core and manganese dioxide as the shell. This structure was used to wrap the chemotherapeutic drug, Dox, and then modified with mPEG-NH2 to prepare MoS2@Dox/MnO2-PEG (MDMP) composite antitumor nanocomposites. The in vivo and in vitro experiments showed that MDMP had excellent antitumor activity (tumor survival rate: 11.8%) and good PCE (33.7%).
2.1.3 Metal Oxide Nanomaterials
In addition to TMDC nanomaterials, nanomaterials containing transition metal oxides have also received extensive attention in the field of PTT (100) due to their excellent PCE good biocompatibility, excellent chemical stability, adjustable band gap, and low cost. Iron oxide, molybdenum oxide, tungsten oxide, zinc oxide, and manganese oxide are used as common metal oxide nanomaterials.
Magnetic nanoparticles, mainly including magnetite (Fe3O4), maghemite (γ-Fe2O3), or a combination of the two (101) show great potential in cancer therapy in the form of magnetic resonance imaging-guided chemotherapy (102, 103), PDT (104, 105), and PTT (106, 107) due to their unique superparamagnetic iron oxide nanoparticles. In addition, iron oxide nanoparticles show excellent PCE in a biological environment and have good chemical stability and low cytotoxicity (108). Moreover, the US Food and Drug Administration has approved its application in the human body (109). Liu et al. (34) synthesized USPIO-PEG-sLex, which consists of nanocomposites of ultrasmall superparamagnetic iron oxide nanoparticles coated with polyethylene glycol (PEG) coupled with Sialyl Lewis X. The USPIO-PEG-sLex nanoparticles have good photothermal conversion properties, and the temperature and concentration of the solution are positively correlated with the power density of NIR on 808-nm wavelengths. The results of PTT in vitro showed that as the nanoparticle concentration increased, the survival rate of 5-8F cells significantly decreased, which could effectively inhibit the development of tumors (Figure 1).
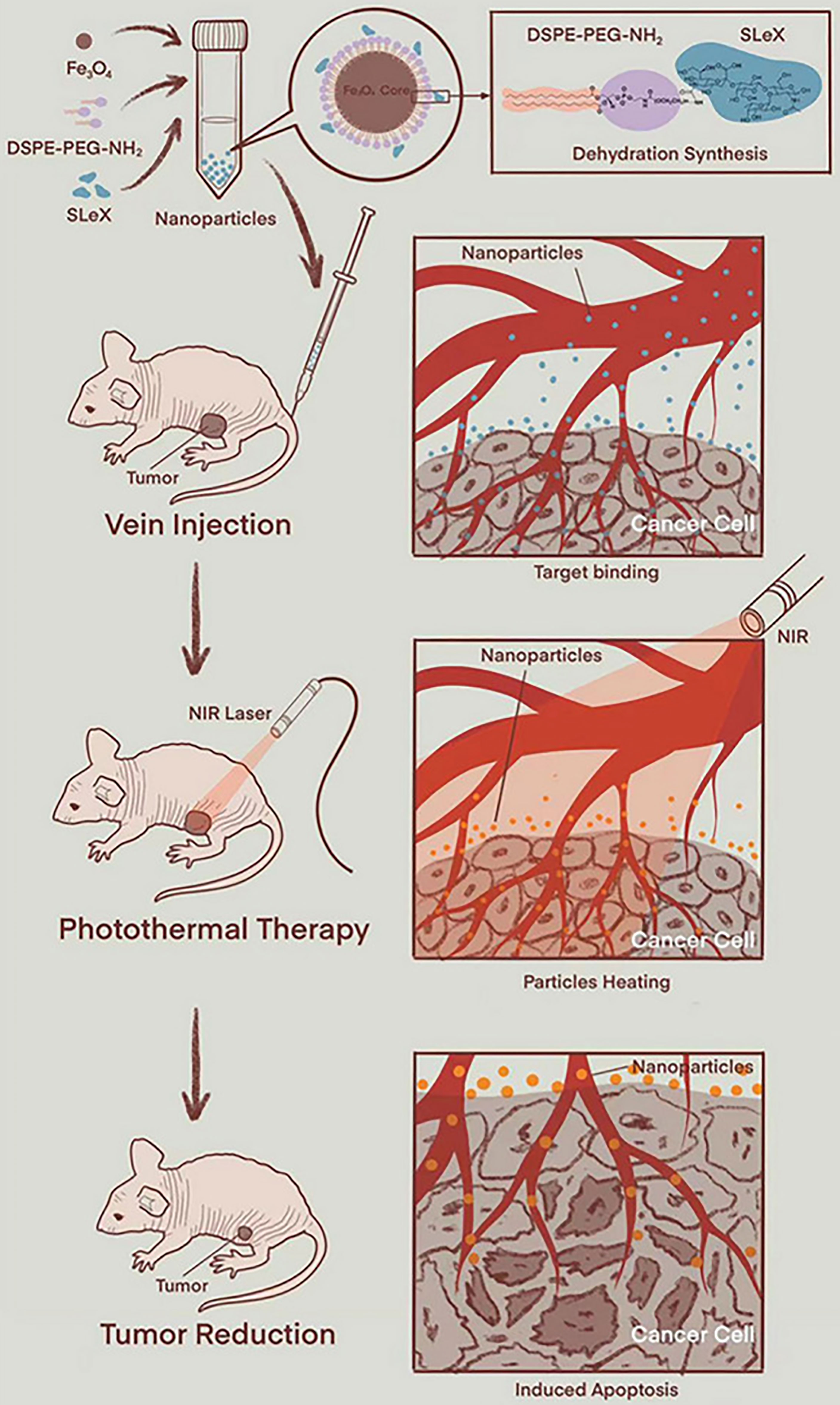
Figure 1 Schematic diagram of PTT (using animal experimental research as a model) (34). Reproduced with permission from Liu et al., 2021.
Molybdenum-based materials can be divided into two categories: transition metal oxides composed of molybdenum dioxide and molybdenum trioxide, and TMDCs composed of molybdenum disulfide. Transition metal molybdenum oxide has a similar, adjustable, local SPR effect to precious metal nanomaterials (110). Molybdenum trioxide nanoparticles have been reported to have excellent light absorption ability in the NIR region and can produce singlet oxygen under the irradiation of NIR light. Thus, molybdenum oxide nanomaterials can be used in PDT or PTT for tumors (111, 112). Qiu et al. (35) combined folic acid and α-lipoic acid-conjugated mPEG-NH2 (LA-PEG) and modified BSA with molybdenum oxide nanosheets to prepare multi-functional degradable FA-BSA-PEG/MoOx nanosheets (Figure 2). The results of in vivo and in vitro anti-tumor experiments showed that FA-BSA-PEG/MoOx nanosheets significantly increased the temperature of the tumor site, inducing immunogenic cell death, which triggered an immune response in vivo through the combination of PTT and chemotherapy, inhibiting primary tumor growth (inhibition rate: 51.7%) and lung metastasis (inhibition rate: 93.6%). This novel nanosheet is a promising avenue for combination therapy for breast tumors.
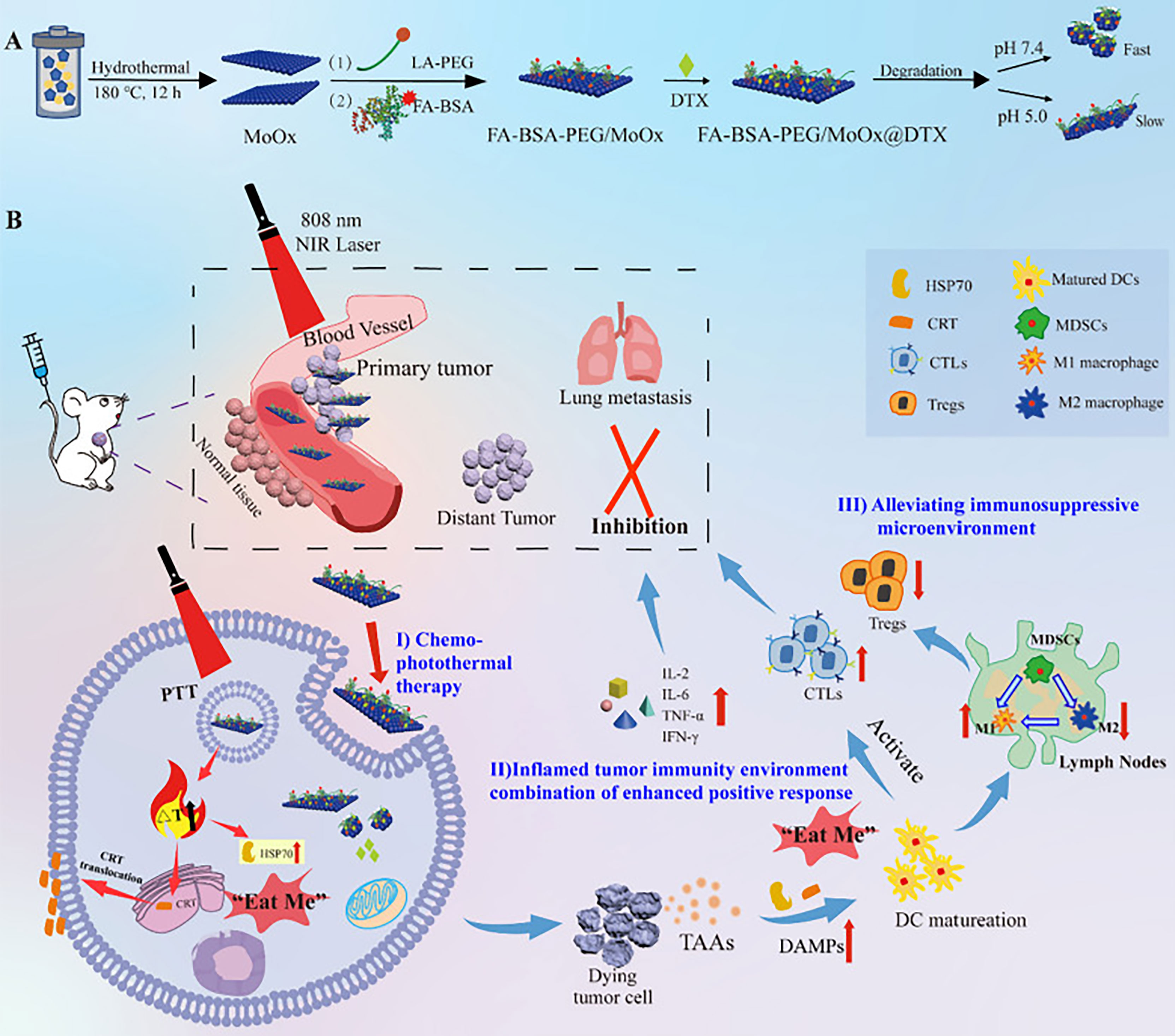
Figure 2 The schematic illustration of the multi-strategy for cancer treatment. (A) The preparation route of the FA-BSA-PEG/MoOx@DTX nanosheet and the in vitro antitumor and degradation experimental design; (B) the elucidation of the mechanism of FA-BSA-PEG/MoOx@DTX + NIR combination therapy for meliorating tumor immunosuppression, inhibiting distant tumor and lung metastasis (35). Reproduced with permission from (35).
Tungsten nanomaterials, similar to precious metal nanomaterials, exhibit a local SPR effect because of their outer-d valence electrons, which can be used to produce a photothermal effect (113). The size and shape of the nanoparticles also play a role in the SPR effect of tungsten nanomaterials (114, 115). Among tungsten nanomaterials with different stoichiometry, tungsten oxide nanomaterials are among the most widely used materials in the biomedical field (116, 117). In particular, W20O58, W18O49, and W24O68 are more common PTAs because of their excellent optical absorption capacity in the NIR region (118). Liang et al. (36) introduced oxygen vacancy (OV) tuning into oxygen-deficient tungsten trioxide nanosheets to optimize the chemical and electrical properties. The experimental results show that under the irradiation of single-wavelength NIR (808 nm), tungsten trioxide-OVs exhibited good PCE (41.6%) and an effective tumor inhibition rate (96.8%).
Zinc oxide is a multi-functional material with unique physical and chemical properties, such as high chemical stability, high electrochemical coupling coefficient, wide radiation absorption range, and high light stability (119, 120). Zinc oxide nanomaterials can appear in one-dimensional (121), two-dimensional (122), and three-dimensional (123) structures, providing one of the greatest assortments of particle structures among all known materials (124). Thus, zinc oxide is a potential alternative for PTT (72). Deng et al. (37) prepared multifunctional nanoparticles (Z@CD/P) using ZnO@CuS as the carrier, as well as β-cyclodextrin (β-CD) modified by 2,3-dimethyl maleic anhydride (DMA) (β-CD-DMA), and mPEG-NH2 modified by DMA (PEG-DMA) to increase stability. They were loaded with Dox and pirfenidone (PFD). Zinc oxide and copper sulfide were found to promote tumor cell death by regulating the pathway of ROS production as well as that of GSH-GPX4, and their photothermal conversion ability further promotes the anti-tumor effect.
Manganese oxide nanomaterials have great potential as PTAs and signal contrast agents for traditional PTT because of their excellent T1-weighted contrast signals, low cytotoxicity, and high PCE (72, 125). Liu et al. (126) proposed for the first time that ultra-thin manganese dioxide nanosheets have pH and redox responses as well as T1-weighted magnetic resonance imaging capabilities. Moreover, photothermal in vivo and in vitro experiments showed that these nanosheets also had good photothermal conversion ability (η: 21.4%) and a high inhibition rate on tumor growth (Figure 3). Xu et al. (38) designed bismuth/manganese oxide nanoparticles (mBMNI NPs) for targeting triple-negative breast cancer, which were encapsulated in the tumor cell membrane and loaded with indocyanine green. The result of the photothermal experiment showed that mBMNI NPs absorbed NIR laser efficiently and generated a large amount of heat for PTT. Apart from high-efficiency PTT, mBMNI NPs also performed chemodynamic therapy (CDT) and PDT synergistically through the generated singlet oxygen and ICG, offering great potential for targeted triple-negative breast cancer therapy.
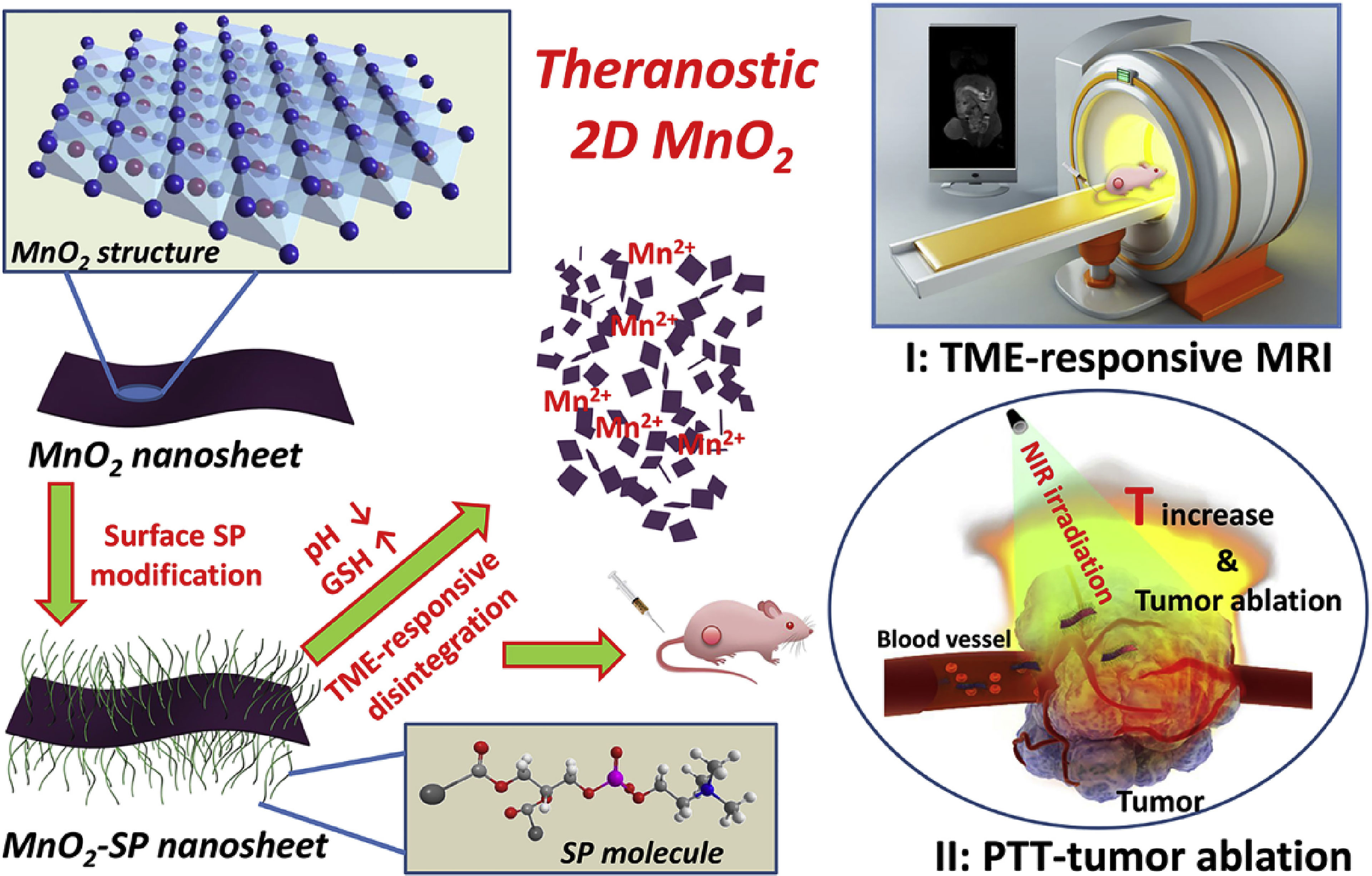
Figure 3 Schematic illustration of synthetic procedure for MnO2-SPs nanosheets and their specific functions for tumor theranostics with TME sensitivity, including the acidic/reducing condition-triggered T1-weighted MR imaging and efficient PTT against tumor (126). Reproduced with permission from (126).
2.1.4 Carbon-Based Nanomaterials
In recent years, carbon-based nanomaterials have been widely studied as inorganic materials for PTT for tumors (100). Many carbon-based nanostructures have been developed for biomedical applications, such as carbon dots, quantum dots, graphene, and carbon nanotubes, among others. The graphitic structure of carbon-based materials endows them with strong absorption in the NIR region and good PCE (127, 128). In addition, the ultra-high surface area of carbon-based materials enables them to build multifunctional nanoplatforms, which have optimistic application prospects in tumor therapy (129).
2.1.4.1 Carbon Dots
As a new type of 0 dimensionality material, carbon dots (CDs) not only inherit the advantages of small molecules (such as fluorophores) and traditional semiconductors (such as inorganic quantum dots), but they also have additional properties (130). For example, CDs have excellent photostability, good biocompatibility, permeability, low toxicity, low cost, and are easy to prepare (131). However, most CDs usually absorb light in the short wavelength region due to the π-π* transition of the C=C bond; therefore, other nanomaterials, such as metal nanoparticles (132) or semiconducting polymers (133) are needed as NIR-assisted PTAs (131). Lu et al. (42) assembled polydopamine (PDA) and carbon points on hollow mesoporous carbon (HMC) to construct a photothermal enhanced multi-functional system (HMC-SS-PDA@CDs). The results of in vivo experiments showed that under low-power, 808-nm laser radiation of 0.75 W/cm2, the antitumor drug-loaded HMC-SS-PDA@CDs inhibited tumor growth by 92.6% and significantly reduced the toxicity of Dox to cells, indicating that Dox/HMC-SS-PDA@CD nanoparticles have good photothermal chemotherapeutic synergism and ideal biocompatibility.
2.1.4.2 Quantum Dots
Compared with traditional fluorescent dyes and proteins, quantum dots (QDs) have significant advantages, such as broad luminescence excitation spectra and narrow symmetrical emission spectra with large Stokes shifts (134, 135). Different types of quantum dots have varying chemical compositions and properties, which can affect their potential applications. The new generation of quantum dots, including non-cadmium and NIR-II window quantum dots, have excellent optical properties and biocompatibility required for in vivo applications and good prospects in the field of tumor therapy and imaging (136–138). Wang et al. (43) have developed cadmium tellurium selenium/zinc sulfide core-shell quantum dots with excellent biocompatibility for PTT and fluorescence imaging of tumors. After being irradiated with blue light (80 mW/cm2 blue laser) for 20 min, the quantum dots were heated rapidly. Due to their photothermal and photodynamic effects, the quantum dots induce complete apoptosis of the Huh7 hepatoma cell line, providing a new avenue for tumor therapy.
2.1.4.3 Carbon Nanotubes
Carbon nanotubes (CNTs), originally proposed by Iijima (139), are currently the most widely used carbon-based nanomaterials in the biomedical field (140, 141). CNTs are divided into two types according to the number of layers in their structure: single-walled CNTs (SCNTs), which consist of a single graphene sheet, and multi-walled CNTs (MCNTs), which consist of several sheets forming concentric cylinders (142). CNTs have been reported to have broad NIR absorption and are affected by the size and shape of the nanomaterials (143). CNTs exposed to NIR laser absorbs light energy and converts it into thermal energy, which can be used to ablate cancer cells (144). Zhao et al. (44) coated SCNTs and MCNTs with peptide lipid and sucrose laurate, respectively and loaded anti-survivin siRNA to synthesize a nano-delivery system (denoted SCNT-PS and MCNT-PS, respectively) with good temperature sensitivity and photothermal properties for tumor immunity and combination PTT. The results showed that CNT/siRNA inhibited tumor growth by silencing the expression of survivin and exhibiting a photothermal effect under NIR laser. SCNT-PS/siRNA showed high antitumor activity and had a complete inhibitory effect on some tumors. Neither SCNT-PS nor MCNT-PS nanoparticles had obvious cytotoxicity at a concentration of up to 60 μg/mL.
2.1.4.4 Mesoporous Carbon Nanoparticles
Mesoporous carbon nanoparticles (MCNs) or hollow carbon nanospheres (HCNs) are mesoporous nanomaterials with high pore volume and specific surface area, which have attracted attention in recent years (145, 146). It has been found that MCNs have a unique structure that can load a large number of hydrophobic drugs as well as excellent biocompatibility, which makes them an effective drug carrier (147, 148). In addition, MCNs have high efficiency in converting NIR laser energy into thermal energy and can be used in tumor PTT (72, 149). Xu et al. (45) designed polyethylene glycol-graft-polyethylenimine (HPP)-modified HCNs as NIR-II responsive PTAs. The experimental results showed that HPP-HCNs have a PCE of 45.1% under 1064nm laser irradiation. The in vivo and in vitro experiments showed that HPP had limited cytotoxicity to mice and good photothermal activity towards killing cancer cells in the xenograft 4T1 tumor-bearing mice model, which significantly inhibited tumor growth.
2.1.4.5 Graphene-Based Nanomaterials
Graphene, as a common carbon-based nanomaterial, has a wide range of applications as a biosensor, drug carrier, and tumor PTA because of its strong NIR absorption (150, 151). However, graphene has poor dispersibility in physiological fluid and is considered to have certain biological toxicity (152). To overcome these limitations, graphene requires surface modification with specific materials (153). Therefore, graphene-based nanomaterials (GBNs) have received increasing attention (154–156). For example, GBNs have been found to have a large surface area and can be used as drug carriers (157). GBNs are also widely used as PTAs in tumor therapy because of their good photothermal conversion ability in the NIR region (158, 159). Generally, GBNs can be divided into several types, including graphene with varied layers, graphene oxide (GO), and reduced graphene oxide (rGO) (160).
GO and rGO have great potential in the field of biomedicine, especially in drug delivery, biosensors, and targeted tumor therapy because of their tunable physicochemical properties, excellent biocompatibility, and outstanding photothermal properties (160–163). Dash et al. (46) modified rGO with citrate-coated magnetic nanoparticles, coupled with gastrin-releasing peptide receptor-binding peptide, and loaded Dox through the π-π bond to synthesize an rGO-based magnetic nanocomposite (mGOG). The results of the in vitro experiments showed that after being combined with 808-nm laser irradiation, the 50% inhibiting concentration and apoptosis rate of tumor cells were 0.19 μg/mL and 76.8%, respectively. At the same time, the increased expression of heat shock protein HSP70 confirmed the magnitude of the photothermal effect of mGOG. In addition, the mouse model experiment showed that, after 5 min of NIR laser irradiation, the tumor volumes in the mice in the experimental group were significantly reduced, the survival time was significantly prolonged, and the antitumor effect was significant.
With the continuous study of GBNs, graphene quantum dots (GQDs) first discovered by Ponomarenko and Geim (164), have undergone vigorous development in the biomedical field. GQDs exhibit inherent fluorescence properties, low cytotoxicity, stable photoluminescence, good biocompatibility, and superior resistance to photobleaching (165). After NIR light irradiation, GQDs also show excellent photothermal conversion ability (166, 167). These unique physicochemical properties endow GQDs with excellent potential in tumor therapy. Chen et al. (47) combined aptamer-modified GQDs with magnetic chitosan to form novel photothermal-chemotherapy drug delivery nanosystems (DOX-Fe3O4@CGA). The results of an in vivo antitumor experiment showed that under NIR laser irradiation, the temperature of the tumor site in mice increased rapidly to 43–45°C, and the tumor volume and weight significantly decreased over time. Thus, DOX-Fe3O4@CGA significantly inhibited tumor growth and prolonged survival time in mice, demonstrating excellent synergistic therapeutic ability.
2.1.5 Other Inorganic Photothermal Nanomaterials
MXenes refer to a series of carbides, nitrides, and carbonitrides containing transition metals (mainly from groups 3 and 4), with unique structure and excellent physicochemical properties (168–170) (Figure 4). The typical molecular formula is expressed as Mn+1XnTx (e.g, Ti3C2Tx). Notably, MXenes have good optical properties for bioimaging and biosensors, and their excellent PCE and biocompatibility make them ideal candidates as efficient PTAs (172, 173). Lu et al. (48) coated a layer of red blood cell membrane on polydopamine-modified niobium carbide nanosheets coated with immunoadjuvant R837 to synthesize a new type of multifunctional niobium carbide nanoparticle (Nb2C@PDA-R837@RBCNP) for NIR-II PTT combined with immunotherapy. Nb2C@PDA-R837@RBCNPs exhibited high PCE under 1064-nm laser irradiation. Additionally, the circulation time in vivo was significantly prolonged, and the primary tumors were completely cleared in mice. Finally, the secondary tumor growth inhibition rate was as high as 89.8% due to the enhanced immune response.
Compared with other two-dimensional nanomaterials, black phosphorus nanosheets (BPNSs) have a larger extinction coefficient and higher PCE and are often used as PTAs for PTT (174–176). In addition, BPNSs have been widely used in biomedicine because of their large specific surface area, good biocompatibility, and biodegradability (177). Peng et al. (49) prepared BPNS-based multifunctional nanocomposites (BPNS-PAMAM@DOX-HA) by modifying BPNSs with hyaluronic acid and poly-amidoamine dendrimer and loading them with Dox. The results of the in vivo and in vitro experiments showed that BPNS-PAMAM@DOX-HA exhibited excellent tumor cytotoxicity and cellular uptake efficiency under 808-nm laser irradiation, significantly inhibited the growth of tumors in mice, and showed a more significant antitumor effect than chemotherapy or PTT alone.
Metal phosphorus-based nanomaterials (metal-PNMs) mainly include metal phosphide nanomaterials (e.g., ferrous phosphide) (178), metal phosphate nanomaterials (e.g., calcium phosphate) (179), and metal-black phosphorous nanocomposites (180). Among PNMs, metal-PNMs have been widely studied for tumor diagnosis and treatment due to their unique advantages such as excellent light absorption, inherent magnetism, and biodegradability (181). Jin et al. (50) created a novel anticancer nanoplatform (Co-P@mSiO2@DOX-MnO2) for the synergistic treatment of tumor chemotherapy and PTT, which used cobalt phosphide nanocomposite as the core and mesoporous silica as the shell, loaded with Dox, and combined with manganese dioxide nanosheets. The results showed that under the irradiation of 808-nm NIR laser, Co-P@mSio2@DOX-MnO2 rapidly increased the temperature of the tumor, reflecting the excellent photothermal conversion ability. Compared with the control group, the tumor growth inhibition of the Co-P@mSio2@DOX-MnO2 group was greater and the antitumor effect was significantly improved.
In recent years, upconversion nanoparticles (UCNPs) have attracted attention for their ability to convert NIR light into visible light or ultraviolet light with a shorter wavelength (182). UCNPs have the advantages of minimizing light damage, deep tissue penetration, low light bleaching, and good chemical stability (183–185), which give them great potential for application in tumor therapy (186). Zhang et al. (51) designed a lanthanide-doped UCNP nanotherapy platform (UCNPs@mSiO2FePc-MC540) coated with mesoporous silica for synergistic PDT and PTT, which included NaYF4:Yb, Er@NaLuF4:Nd@NaLuF4 UCNPs, and dual photosensitizing agents (merocyanine 540 and iron phthalocyanine). The results of the antitumor experiment showed that the survival rate of A549 cells in the UCNPs@mSiO2FePc-MC540 group decreased significantly under 808-nm light, while the tumor volume decreased to approximately 10% of the original volume, showing a significant antitumor effect.
Prussian blue (PB) is an iron-centered compound (Fe4[Fe(CN)6]3-xH2O, where x is the number of water molecules), which has been widely studied as a coordination compound (187, 188). PBNPs are widely used in immunosensors, biological imaging, drug release, and tumor therapy due to their large inner pore volume, adjustable size, easy synthesis, surface modification, good thermal stability, and biocompatibility (189–193). Liu et al. (52) mixed paclitaxel (PTX) and phase change materials (PCM) and loaded them onto polyethylene glycol-modified mesoporous PBNPs (mPBs) to construct a biocompatible nano-drug delivery system (PCM+PTX@mPBs/PEG). The in vitro cell experiment showed that the cellular uptake rate of PCM+PTX@mPBs/PEG increased significantly after 808-nm NIR laser irradiation. The in vivo antitumor experiment showed that PCM+PTX@mPBs/PEG could accumulate in the tumor site of mice by passive transport and significantly inhibit tumor growth by delivering chemotherapeutic drugs and a photothermal effect.
2.2 Organic Photothermal Nanomaterials
Inorganic photothermal nanomaterials are easy to prepare and highly modifiable (141), and tend to have higher PCE and better photothermal stability than organic nanomaterials (26, 194). However, the potential cytotoxicity caused by the poor biodegradability of inorganic materials hinders their clinical application (195). In contrast, organic photothermal nanomaterials are more biodegradable and biocompatible (26, 196) and mainly include organic small-molecule nanomaterials and conjugated polymer nanomaterials (14, 197). These two types of PTAs have shown good therapeutic effects and are frequently used for tumor imaging and treatment (198, 199). The most common organic photothermal nanomaterials are introduced below.
2.2.1 Organic Small-Molecule Nanomaterials
Common organic small-molecule photothermal materials include cyanine dyes, porphyrins, phthalocyanines, boron dipyrromethene, and diketopyrrolopyrrole (DPP). Although these small molecules have excellent photothermal conversion ability and biosafety, they also have limitations, such as poor water solubility and limited tumor accumulation. Through functional modification, nanocarriers can be designed to improve the solubilization and pharmacokinetics of small organic molecules and enhance the penetration and retention of therapeutic agents in tumor tissue, enhancing the therapeutic effect (26).
After modification to improve the photophysical properties, cyanine dyes are widely used in tumor PTT, imaging, and sensing because of their excellent biocompatibility and strong NIR absorption (200, 201). Cyanine molecules such as ICG, IR825, IR780, and cypate, are common PTAs that show potential for widespread application in fluorescence imaging and tumor therapy (26, 202). Guo etal. (53) synthesized zeolitic imidazolate framework-8 (ZIF-8) composite nanoparticles (Cy5.5&ICG@ZIF-8-Dex) using dimethyl sulfoxide/water solvent mixtures and loaded ICG and cyanine-5.5 (Cy5.5) for tumor imaging and PTT. The results of PTT showed that the A549 cells in the Cy5.5&ICG@ZIF-8-Dex group died in large numbers, and the tumor growth rate in mice was significantly inhibited, achieving an excellent therapeutic effect.
Porphyrin-based nanomaterials, with good photophysical properties and biocompatibility, have gained extensive attention in clinical tumor therapy and diagnostic imaging (203, 204). Studies have found that assembling porphyrin monomers with supramolecular nanostructures not only improves their physical and chemical properties and strengthens tumor accumulation, but also greatly enhances the range of application of porphyrin in the biomedical field (205, 206). Cao et al. (54) synthesized amphiphilic porphyrin (PPor) through conjugation with two PEG chains, and integrated perylene diimide into the porphyrin skeleton to form a D-A structure. The in vivo and in vitro antitumor experiments showed that under 808-nm laser irradiation, PPor nanoparticles completely disappeared from the primary tumor in mice and stimulated robust systemic antitumor immunity by releasing a large number of damage-associated molecular patterns and tumor-associated antigens, which significantly inhibited tumor metastasis.
Phthalocyanines (PCs) are regarded as second-generation photosensitizers in PDT because of their high molar absorption and excellent photostability (202, 207). With further research on PCs, it was found that PC nanomaterials also exhibit high PCE after irradiation with NIR light, giving them great potential in the application of PTT (208, 209). Feng et al. (55) designed T-MP nanoplatforms based on HER2 and targeted micellular PC. The results of in vivo and in vitro antitumor experiments showed that after 808-nm laser irradiation, the killing rate of HT-29 cells in the T-MP group was much higher than that in the control group. Additionally, primary tumor growth was significantly suppressed, and tumor lymph node metastasis was effectively overcome, greatly prolonging the survival time of mice.
Compared with other organic photothermal nanomaterials, boron dipyrromethene (BODIPY) has gained interest because of its strong absorption of long wavelengths, good photostability, excellent water solubility, and biocompatibility (210–214). Through chemical modification with a conjugated system, the nano-photosensitizer based on BODIPY has a higher absorption coefficient in the NIR region, which gives it the potential to be used as a PTA (215–217). Yu et al. (56) fabricated an NIR BODIPY dye with an upper phenyl-fused segment (BBDP). The photothermal experimental results demonstrated the PCE of BBDP nanoparticles to be as high as 54.2%, suggesting excellent photothermal capability. Moreover, in vitro antitumor experiments showed that under 690-nm laser irradiation, as the concentration of BBDP-NPs increased, the survival rate of tumor cells gradually decreased, indicating that BBDP-NPs have a good phototherapeutic effect.
DPP and its derivatives are widely used in fluorescence imaging and tumor therapy because of their easy modification, high molar extinction coefficient, and good photothermal stability (218, 219). In recent years, nanomaterials with a D-A-D structure based on DPP derivatives have received increasing attention (220, 221). Zheng et al. (57) synthesized three self-assembled nanoparticles with PEG as the side chain using three amphiphilic DPP derivatives (TPADPP, DTPADPP, and TPADDPP). The experimental results showed that these three nanoparticles can not only effectively gather in the tumor site, but also have good biological safety and low cytotoxicity in dark environments. Under the irradiation of a 635-nm laser, DTPADPP and TPADDPP nanoparticles showed an efficient photothermal effect, and tumor growth in mice was significantly inhibited, suggesting that they have a tumor ablation effect.
2.2.2 Conjugated Polymer Nanomaterials
Conjugated polymers with a large π-conjugated backbone and high electron delocalized structure have been widely used in tumor therapy because of their high extinction coefficient and good biocompatibility (222, 223). Moreover, because of their π-electrons, which can easily cause delocalization and transition, conjugated polymers can effectively convert absorbed light energy into heat, making it a suitable PTA (224). At present, the conjugated polymer nanomaterials mainly include polypyrrole (PPy), polyaniline (PANI), and donor-acceptor (D-A)-conjugated polymers, as well as poly-(3,4-ethylenedioxythiophene): poly(4-styrene sulfonate) (PEDOT : PSS).
As a potential PTA, PPy has good biocompatibility, excellent photothermal properties, photostability, and accessible synthesis characteristics (225, 226). Wang et al. (58) combined CS and carboxymethyl cellulose (CMC) through electrostatic interactions and loaded PPy and 5-fluorouracil (5-Fu) to prepare a novel composite nanoparticle: CMC/CS@PPy+5FuNP. In vitro biological studies showed that CMC/CS@PPy+5FuNPs can be effectively internalized by HepG2 cancer cells. The combination of the photothermal effect of PPy and toxicity of 5-FU can significantly improve the therapeutic efficiency on tumors, indicating that CMC/CS@PPy+5FuNPs have great potential in synergistic chemotherapy and PTT.
PANI has been reported as a type of organic photothermal nanomaterial with good stability, biocompatibility, and strong NIR absorptivity. It has been widely used in photoacoustic imaging (PAI) and PTT for tumors (227–229). Wu et al. (59) synthesized a glucose oxidase (GOx)-conjugated PANI nanoplatform (PANITG) for PTT for tumors. Under the irradiation of NIR laser, PANITG activates PTT in slightly acidic tumor microenvironments. The released GOx reacts with excess glucose in the tumor tissue, resulting in cancer starvation. The in vitro and in vivo antitumor experiments showed that glutamate produced by GOx-mediated catalytic reactions enhances the photothermal effect. Meanwhile, PTT also plays a role in promoting the catalytic reaction, indicating that the two synergistically exhibit a significant antitumor effect.
D-A-conjugated polymers have been widely used in PTT for tumors because of their extended light absorption ability and good PCE (230, 231). Liu et al. (60) synthesized D-A-conjugated nanoparticles using thiophene and triphenylamine (TPA) as donors and benzo[1,2-c:4,5-c′]bis([1,2,5]thiadiazole) (BBTD) as the acceptor. The molecular rotors and bulky alkyl chains were then introduced into the center Dmura core to reduce intermolecular interaction. NIRb14 nanoparticles (NIRb14NPs) with long alkyl chains exhibit better photothermal properties. Additionally, the in vivo and in vitro antitumor experiments showed that NIRb14NPs had a longer circulation time in vivo, demonstrating significant tumor growth inhibition and biosafety.
Poly(3,4-ethylenedioxythiophene): poly(styrene-sulfonate) (PEDOT : PSS) is a complex of conjugated polymer PEDOT and negatively charged polymer PSS (232–234). PEDOT : PSS is an aqueous-based conductive polymer nanoparticle with strong NIR absorption that has become a popular NIR PTA for its water-dispersibility, high PCE, excellent light stability, and good biocompatibility (235–237). Ko et al. (61) synthesized a kind of magneto-conjugated polymer core-shell nanoparticle (MNP@PEDOT : PSSNP) based on PEDOT : PSS by in situ surface polymerization. PTT experiments showed that after laser irradiation, compared with the control group, the tumor volume of tumor-bearing mice did not significantly change, while the tumors in the MNP@PEDOT : PSSNP group were completely cleared. Additionally, these nanoparticles did not affect other organs, showing an effective and safe anti-tumor effect.
PDA has a similar chemical structure to eumelanin and may have similar properties, including NIR-responsiveness, chelation, and drug-binding capability (238). Liu et al. (239) first discovered that PDA has remarkable photothermal conversion ability and applied PDA as PTA to tumors. Xu et al. (62) designed biodegradable folic acid-modified Cu2+-chelated PDA nanoparticles (Cu-PDA-FANPs) as an immunogenic cell death (ICD) inducer and multimodal tumor therapy technique (Figure 5). Experimental results showed that under the irradiation of 808-nm NIR light, Cu-PDA-FANPs could effectively convert light into heat and cooperate with Cu2+-mediated chemical dynamic therapy, promoting a systemic antitumor immune response, which can eliminate tumors in vivo and significantly inhibit tumor metastasis.
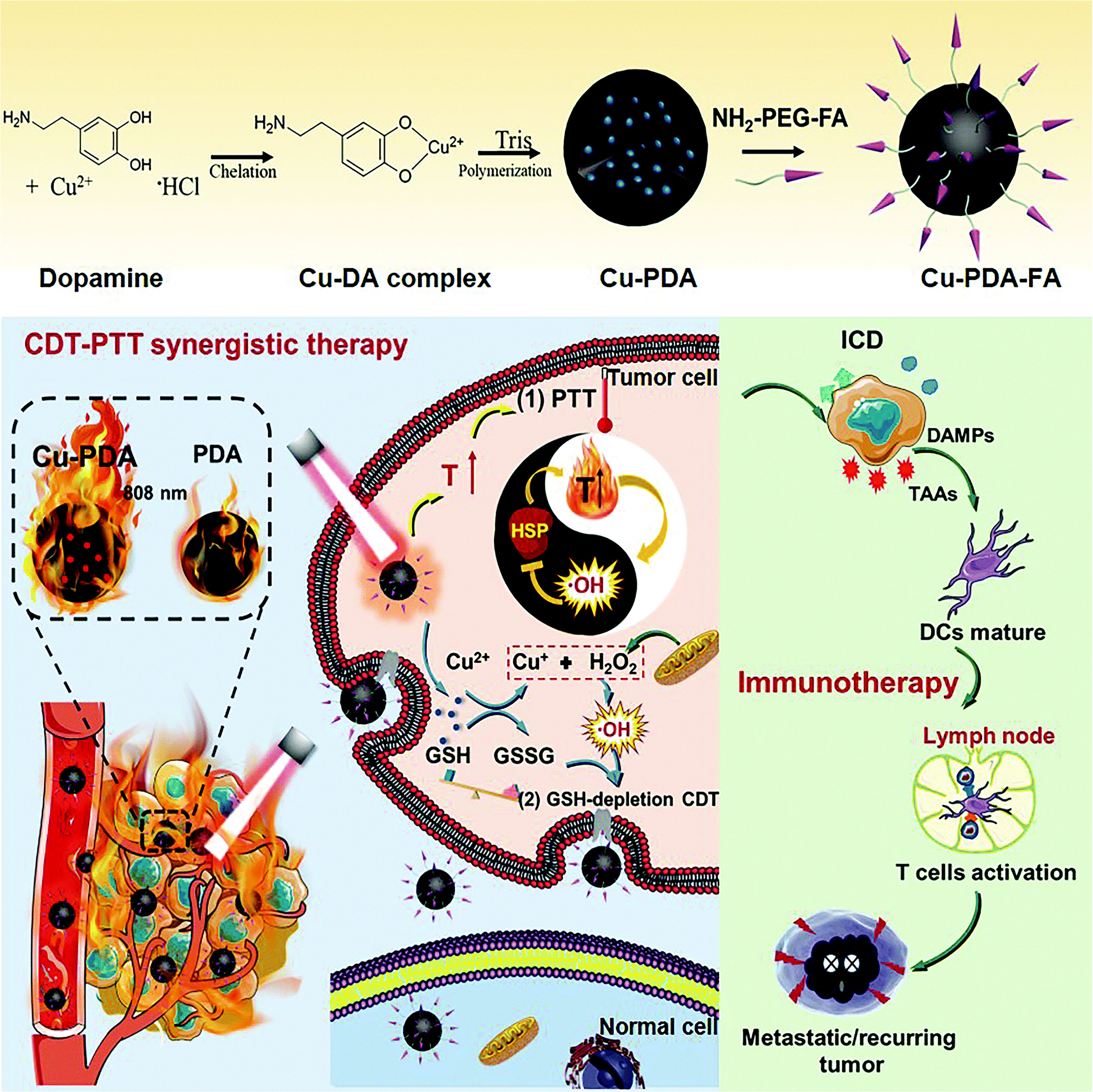
Figure 5 Schematic illustration of Cu–PDA–FA NP synthesis and Cu–PDA–FA NP-mediated PTT/CDT synergistic effect and antitumor immune activation (62). Reproduced with permission from Xu et al., 2022 (62).
2.2.3 Other Organic Photothermal Nanomaterials
Melanin is a natural polyphenol substance that can be divided into eumelanin and pheomelanin (240), according to the precursor molecular. Apart from their chelating function and drug-binding ability, natural melanin-based nanomaterials have many beneficial physical and chemical properties, including UV-Vis absorption and excellent PCE. Therefore, the application of natural and artificial melanin-based nanomaterials or melanin-like nanoparticles has achieved remarkable results in the field of biomedicine, especially as antitumor PTT (241, 242). For example, Xie et al. (63) prepared melanin and cellulose nanosheets (OMCNs) using black sesame hell as raw material by facile liquid-phase exfoliation. They then tested the photothermal properties and ability to kill cells of the OMCNs. The extinction coefficient of OMCNs at 808 nm was 3.1 L/g/cm, the PCE was approximately 37.3%, and the OMCNS demonstrated good light stability. The results of cell experiments showed that almost all SMCC-7721 and B16 cells were killed after NIR laser irradiation, indicating that OMCNs have a significant photothermal killing effect on cancer cells and great potential in antitumor therapy in vivo. In addition, Kang etal. (64) prepared dual laser-responsive multifunctional melanin-like nanoparticles (MNPs) for PDT, PTT, and chemotherapy, based on the KMnO4-oxidative polymerization of L-3,4-dihydroxyphenylalanine (L-DOPA), pheophorbide a, and Dox, and modified by FA. The results of antitumor experiments showed that after 670-nm and 808-nm laser irradiation, the MNP group showed more extensive damage and apoptosis than the control group, showing great potential for antitumor therapy.
2.3 Organic-Inorganic Hybrid Photothermal Nanomaterials
Inorganic photothermal nanomaterials have unique physicochemical properties, such as high molar extinction coefficients, good photothermal conversion rate, excellent photothermal stability, and easy modification; however, their poor biodegradability and potential cytotoxicity limit their use in clinical treatment (91, 243). In contrast, organic photothermal nanomaterials have ideal biodegradability and biocompatibility; however, the photothermal properties of most organic photothermal nanomaterials often require further modification to be used in the treatment of tumors in vivo (244, 245). Due to the unsatisfactory effect of inorganic or organic photothermal nanomaterials alone, the application of organic-inorganic composite nanomaterials in PTT has attracted attention. Organic-inorganic composites not only integrate their respective advantages and improve their physical and chemical properties, but also exhibit synergistm (246–248).
Common organic-inorganic composite nanomaterials include core-shell nanoparticles and metal-organic frameworks (MOFs) (249, 250). Odda etal. (65) synthesized surface-engineered iron oxide nanoparticles (α-Fe2O3NPs) and PEDOT into a novel core-shell photothermal nanoparticle (Fe2O3@PEDOT-siRNANP), which was loaded with siRNA for synergistic tumor gene therapy and PTT. The experimental results of photothermal conversion performance showed that Fe2O3@PEDOT-siRNANPs not only had good biocompatibility and water dispersibility but also demonstrated a high PCE (η = 54.3%) in the NIR region. In vitro and in vivo experiments showed that Fe2O3@PEDOT-siRNANPs induced greater cancer cell apoptosis and more pronounced tumor suppression after laser irradiation compared with GT or PTT alone. Bai etal. (66) were the first to prepare NIR emission carbon dots (RCDs) based on glutathione (GSH). They then synthesized a novel metal-organic framework nano-platform (RCDS@MIL-100) using RCDs, FeCl3, and trimesic acid solutions. In the tumor microenvironment, RCDS@MIL-100 NPs consumed GSH and released Fe2+, which could react with hydrogen peroxide to produce hydroxyl radicals. Under the irradiation of 660-nm laser, RCDs showed excellent photothermal conversion ability, promoted a Fenton reaction, and enhanced the therapeutic effect of CDT. The results of antitumor experiments indicated that tumors in the mice of the RCDS@MIL-100 group were completely removed, showing a highly effective antitumor effect.
3 Multimodal Therapy for Tumors Based on Photothermal Nanomaterials
Currently, chemotherapy (251), radiotherapy (252), and high-intensity focused ultrasound therapy (253) are widely used and successfully inhibit the growth or spread of tumors and prolong the survival time of patients. PDT (254) has also been shown to have significant advantages in the treatment of non-small cell lung cancer and esophageal cancer. Other treatments, such as PTT (194), immunotherapy (255), gene therapy (256), and magnetothermal therapy (257), have undergone significant research, though most are still in the preliminary clinical stage of research. These emerging tumor treatments have been shown to have ideal anticancer effects in many laboratory and preclinical studies and have broad applications for clinical treatment in the future. For example, ICG, a hydrophobic photosensitizer, is the only NIR imaging reagent approved by the USFDA and has been widely used in the biomedical field, especially for tumor therapy (258, 259). However, ICG has not achieved the eradication of all tumors or the prediction and prevention of metastasis, which is the limitation of single-mode immunotherapy. For example, some cancer cell subsets in heterogeneous tumor tissues may achieve mono-drug resistance to antineoplastic drugs (260). Moreover, long-term use of anticancer drugs often induces multidrug resistance in tumor tissues, which leads to reduced efficacy of chemotherapy (261). Additionally, because of the insensitivity of anoxic cancer cells to ionizing radiation, radiotherapy alone is often unable to achieve an ideal therapeutic effect in a hypoxic tumor environment (262).
Similarly, although PTT has unique advantages, its inherent limitations affect its clinical application. Because the temperature of the tumor site rises to 41–47°C during PTT, necrosis may also occur in the surrounding normal tissue. This leads to the infiltration of pro-inflammatory and immune suppressor cells, triggers a chronic inflammatory response, and promotes immunosuppression through the activation of checkpoint pathways that inhibit T cell responses (263–265). In addition, due to the limited depth of NIR light penetration into the tissue, the tumor cells outside the irradiation area are not completely removed, resulting in possible tumor recurrence and metastasis (263). These limitations inhibit the clinical applications of PTT.
To overcome these barriers of single-mode therapy, combination therapy by integrating two or more treatment modalities has been proposed as a solution (266). Combination therapy is based on synergistic and enhanced interactions between two or more treatments, which tends to produce super additive effects, known as “1+1>2” (267). Therefore, multi-functional nanomaterials are constructed by combining different types of therapeutic agents in a single nanostructure through physical adsorption or chemical binding, which can be used to create multimodal, collaborative therapy for tumors (268–270).
3.1 Dual-Modal Therapy Based on PTT
PTT facilitates other tumor treatment methods by its ability to increase the temperature of the tumor site and change the microenvironment. The heat generated during PTT can also promote the intracellular transmission and release of drugs, genes, and immune adjuvants and the disintegration of thermosensitive nanocarriers to enhance the therapeutic effects of chemotherapy, gene therapy, and immunotherapy. It can also accelerate the production of physical/chemical injury factors, such as ROS synthesis, to enhance the efficacy of PDT, sonodynamic therapy (SDT), and CDT based on the principle of oxygen injury. Additionally, due to the increase in temperature from PTT, the vascular permeability of tumor tissue increases, which promotes hemoperfusion, increases oxygen saturation, improves the hypoxic tumor environment, and enhances the efficacy of radiotherapy limited by hypoxia. At the same time, light induces ICD and upregulates tumor immunogenicity, which improves lymphocyte permeability and enhances antitumor immunity (263, 271). In this section, representative studies will be introduced to explain the synergy between PTT and additional therapies (Figure 6).
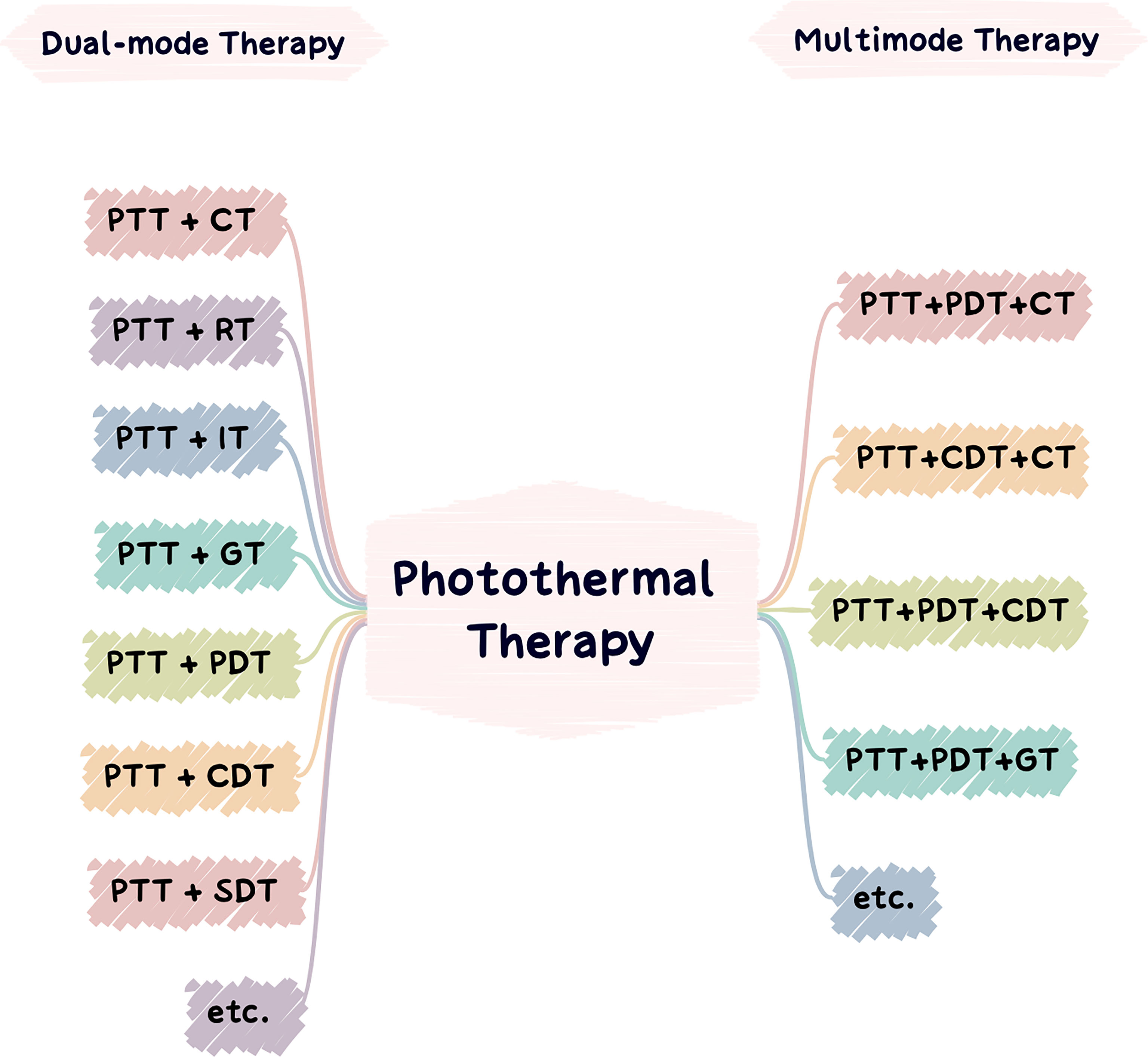
Figure 6 Applications of photothermal nanomaterials in tumor dual-mode therapy (left) and applications of photothermal nanomaterials in tumor multimode therapy (right).
3.1.1 PTT Acts Synergistically by Promoting the Uptake of Therapeutic Agents
Studies have found that the high temperatures produced by PTT can promote the uptake of nanocarriers by tumor cells and accelerate the decomposition of nanocarriers to enhance intracellular drug concentration and cytotoxicity (272). In addition, some anticancer drugs (e.g., cisplatin) exhibit a significantly enhanced ability to kill tumor cells under the high temperature produced by PTT (273). However, due to the thermal expansion of PTT, chemotherapeutic drugs can be more evenly distributed in tumor tissues, enhance heterogeneity, and inhibit tumor drug resistance and metastasis. Wang et al. (274) prepared an intelligent polymer-drug vehicle (MPPD@IR825/DTX) for chemo-photothermal combination therapy, which used poly(ethyleneimine)-poly (ϵ-caprolactone) block polymers as the core and dimethylmaleic anhydride-modified PEG as the shell, encapsulating docetaxel (DTX) and IR825. Compared with the free drug, IR825, MPPD@IR825/DTX nanoparticles exhibited higher temperatures under 808-nm NIR laser irradiation, which increased cytotoxicity and promoted apoptosis of tumor cells more effectively. Furthermore, the results of an in vivo antitumor experiment showed that the combination of chemotherapy and PTT has a better effect on tumor eradication, while chemotherapy or PTT alone cannot eliminate the tumor completely.
Similar to combination chemotherapy, PTT can enhance immunotherapy by promoting the uptake of immune adjuvants and the disintegration of nanocarriers by tumor cells. Some PTAs can also be used as immune adjuvants to promote the maturation of dendritic cells and the production of antitumor cytokines (275). After PTT, tumor tissue responds to high-temperature stress and promotes ICD of tumor cells, thereby enhancing antitumor immune responses (276, 277). Wang et al. (278) loaded immune adjuvants, imiquimod (IMQ) and ICG onto amorphous iron oxide nanoparticles (IONs) to design a tumor microenvironment-responsive nanoplatform (IMQ@IONs/ICG). The results of antitumor experiments in vivo and in vitro showed that IMQ@IONs/ICG had good photothermal conversion ability under 805-nm laser irradiation, induced in situ ICD, and cooperated with released IMQ to enhance the antitumor immune response and significantly inhibit tumor metastasis. Compared with the ICG alone group, the primary tumors in the IMQ@IONs/ICG group were completely eradicated after treatment, mesenteric metastasis was significantly reduced, and the survival time of mice was significantly prolonged.
Similarly, PTT can enhance the efficiency of tumor cell uptake by loosening the cell membrane and promoting the release of genes from nanocarriers to enhance gene therapy effects (279). Gene therapy can improve the efficacy of PTT by inhibiting the expression of specific heat shock proteins and overcoming the resistance of cancer cells to thermal damage (280). Xu et al. (281) synthesized a polypyrrole-poly(ethyleneimine)-siILK nanocomplex (PPRILK) gene PTT nanosystem based on the siRNA of integrin-linked kinase (ILK). The results of in vivo and in vitro experiments showed that after 808-nm laser irradiation, the tumor growth of the PPRILK treatment group was significantly slower and the damage to normal tissue was minimized compared to the laser and gene therapy groups, indicating that the combination of gene therapy and PTT can effectively ablate tumors and inhibit tumor recurrence.
3.1.2 PTT Acts Synergistically by Promoting the Production of Damaging Factors
As an innovative ROS-based cancer treatment, CDT mainly relies on in situ Fenton or Fenton-like reactions to generate hydroxyl radical and trigger oxidative damage (282, 283). A kinetic study found that when the temperature increased from 20°C to 50°C, the rate of Fenton reaction was significantly accelerated, giving PTT an unparalleled position in promoting CDT (284). A limitation of PTT is that the expression of HSPs inhibits heat-induced apoptosis (285). HSPs include redress misfolded proteins, such as HSP90 and HSP70, which can alleviate tumor ablation mediated by PTT. Interestingly, studies have found that ROS can effectively inhibit the expression of HSP70, suggesting that CDT, which can generate hydroxyl radicals, is suitable for inhibiting HSP activity and enhancing the efficacy of PTT (286). Huang etal. (287) mixed Ag2S nanodot-conjugated Fe-doped bioactive glass nanoparticles (BGN-Fe-Ag2S) with PEG double acrylates (PEGDA) and 2,2′-azobis[2-(2-imidazolin-2-yl)propane]-dihydrochloride solution to form a novel light-activated injectable nano-hydrogel (PBFA). The results of in vitro experiments showed that under the irradiation of an 808-nm laser, the solution temperature of the PBFA group increased significantly, the concentration of intracellular ROS increased, and the survival rate of tumor cells was much lower than that of the control groups at 33%. Additionally, compared with the control group, the PBFA group could inhibit tumor growth more effectively and showed better biological safety.
The principle of PDT is that photosensitizers are selectively activated and produce cytotoxic ROS through a specific wavelength of light induction, thus inducing tumor cell death (288). It has been reported that PTT produces mildly high-temperatures, can enhance cell membrane permeability to enhance tumor cell uptake of photosensitizer-loaded nanocarriers, and increases intracellular photosensitizer concentration to promote ROS synthesis, enhancing the therapeutic effect of PDT (289, 290). Sun et al. (291) prepared a novel target nanoprobe (Fe/ICG@HA) with porous Fe3O4 nanoparticles modified by HA and loaded with ICG. The results of antitumor experiments showed that the temperature of the tumor site in the Fe/ICG@HA group increased rapidly to 42.3°C after 808-nm NIR laser irradiation. After 14 days of treatment, the tumor volume of the Fe3O4 and ICG groups increased slightly, while that of the Fe/ICG@HA group decreased significantly. At the same time, histological examination showed that a large amount of singlet oxygen was produced between tumor cells, indicating that Fe/ICG@HA nanoprobe is a promising nanoplatform for combination PDT/PTT.
In recent years, SDT has been widely revered as a non-invasive tumor treatment method, whose action is to promote acoustic cavitation in tumor cells through the impact of ultrasound on sonosensitizers, thus producing an antitumor effect (292). Additionally, the energy generated by ultrasound can be converted into ROS in the presence of ultrasonic sensitizers (293). Because the lipid arrangement in the biofilm is affected by temperature and membrane permeability increases with temperature, PTT can enhance the SDT cavitation effect (263). Moreover, the ROS and oxygen environment on which SDT depends may enhance PTT/SDT synergism (294). Soratijahromi et al. (295) designed gold/manganese dioxide nanocomposite (Au/MnO2 NC) for combination therapy of SDT/PTT. The experimental results showed that under the irradiation of an 808-nm laser and ultrasound, Au/MnO2 NC shows excellent photothermal and acoustodynamic conversion ability. Compared with the control group, the production of ROS in the phototherapy/sonotherapy group was significantly increased, which was the most effective in inhibiting melanoma and showed good synergism.
3.1.3 PTT Acts Synergistically by Improving Tumor Hypoxic Environment
PTT can not only promote the generation of ROS to enhance the therapeutic effect of PDT but the mildly high-temperature can also accelerate blood flow to increase the saturated oxygen concentration of blood vessels, which improves the tumor hypoxia environment to promote oxygen production in oxygen-dependent PDT (296, 297). In addition, difficulty in distinguishing normal cells from tumor cells as well as hypoxia-limited ROS production are common pitfalls of radiotherapy (298). Currently, PTT-induced hyperthermia has been observed to accelerate intratumoral blood flow to improve tumor oxygenation (299), thereby reducing hypoxia-induced radioresistance to enhance radiotherapy efficacy (300). In addition, PTT can effectively inhibit the repair of DNA damage caused by X-ray radiation, increase the radiosensitivity of tumor cells, and improve synergism (301, 302). Ni et al. (303) assembled UCNPs coated with manganese dioxide and copper sulfide to create a multifunctional nanoplatform (UCCM) for combined radiotherapy and PTT. The results showed that the manganese dioxide coating produced a large amount of oxygen by interacting with hydrogen peroxide, which can improve the anoxic microenvironment and enhance the efficacy of radiotherapy. Meanwhile, under NIR laser irradiation, the dispersed copper sulfide nanoparticles absorbed light energy and converted it into thermal energy, which significantly inhibited tumor growth. Compared with the radiotherapy or PTT groups, the tumor-bearing mice in the UCCM group had lower cancer cell activity levels and more significant antitumor effects.
3.1.4 PTT Acts Synergistically With Other Methods
In addition to the synergistic effects of PTT with the methods described above, PTT can also promote synergism in other ways. For example, Yang et al. (304) combined superparamagnetic iron oxide nanoparticles and luminescent lead sulfide/cadmium sulfide quantum dots (Pb-based QDs) to create supernanoparticles (SASNs), which verified the feasibility of magnetothermal and photothermal dual-modal hyperthermia. Dual-modal heating with SASN as the heating agent showed an efficient heating output, which was better than magnetothermal and photothermal heating alone. Lu et al. (305) explored the synergism between gas therapy and PTT by designing sulfur dioxide prodrug-doped nanorattles. The experimental results showed that sulfur dioxide had goodPCE, while sulfur dioxide gas had certain cytotoxicity, which could effectively induce tumor cell apoptosis through pH-precise targeting. Compared with the control group without laser irradiation, the expression of pro-inflammatory proteins (Bax, P53, caspase-3) was significantly upregulated in superficial and deep tumors in the combined treatment group after 808-nm laser irradiation, while that of the anti-inflammatory protein, Bcl2, was significantly downregulated, and the apoptosis rate of tumor cells was higher.
3.2 Multimodal Therapy Based on PTT
Since the 1960s, the combination of two or more treatment strategies has shown a strong synergistic effect and reduced side effects. Hence, dual-modal or multimodal treatments have skillfully integrated the advantages of a single treatment into one system (282). In contrast to the limited therapeutic effects and possible side effects of single-modal immunotherapy, multimodal synergistic therapy may have the overall advantages of a variety of single-modal immunotherapies and produce higher anticancer effects at lower doses, avoiding high-dose side effects (306). Although dual-modal therapy shows better therapeutic effects than single-modal therapy, multimodal therapies (three or more based on PTT treatments), can further overcome the shortcomings and improve anticancer effectiveness (282). The potential synergistic effect of different treatments has been largely ignored in previous literature because of the complexity of the synergy among treatments. When multiple treatment modes are superimposed, whether contradictions or adverse effects will occur requires further systematic research and analysis. For example, compared with PTT or PDT alone, although their combination can provide a simpler treatment process and more ideal result, it requires higher laser power and irradiation time to initiate synergism. Whether this results in adverse effects on normal human tissue has not been reported (12). Based on the research experience of several groups (307, 308), a variety of therapeutic agents can be assembled in nanocarriers for combination treatment of multiple therapies with higher efficacy and almost no side effects. Below, we provide examples to introduce the research of several multimodal tumor therapy methods.
3.2.1 PTT Combined With PDT and Chemotherapy
Previous studies have shown that drugs, photosensitizers, and PTAs can be integrated into one nanostructure, thus enabling the combination of chemotherapy, PDT, and PTT (309). Because ROS produced during PDT can promote intracellular drug delivery by avoiding uptake of nuclear endosomes, PDT can effectively enhance chemotherapy. Therefore, the combination of PTT/PDT/chemotherapy may be more effective than their dual-modal combinations. Chen et al. (310) synthesized a new multimodal therapy system based on BP nanotablets using Dox as a model drug, which has pH/light-responsive drug release properties. In other words, drug release is further promoted under 808-nm illumination. The results of antitumor therapy in vivo showed that, compared with other control groups, the tumor growth inhibition of mice in the three-mode immunotherapy group was the most significant (inhibition rate as high as 95.5%), and the therapeutic effect was significantly enhanced, indicating that the multi-modal combination of PTT/PDT/chemotherapy is feasible.
3.2.2 PTT Combined With Chemotherapy and CDT
The synergistic effect between CDT and PTT has been widely studied and verified (219, 311, 312), but tumor tissues adapt stronger ROS defensive systems at high ROS levels, resulting in poor therapeutic effects from CDT (219, 311, 312). A study (313) found that when PTT andCDT are combined with chemotherapy, this multimodal method can not only overcome the limitation of PTT penetration depth and avoid drug resistance, but also improve the sensitivity of tumor tissue to ROS, achieving significant synergism. Wang et al. (314) designed a nanoparticle based on redox and light-responsiveness (RLR), which consists of ultrasmall iron oxide nanoparticles embedded in an amorphous hollow carbon framework as the core and stacked manganese dioxide flower-like nanosheet structures as the shell. RLR nanoparticles were synergistically treated by manganese dioxide consumption of GSH, iron ion-induced Fenton reaction, PTT, and chemotherapy (Dox). The in vivo and in vitro results showed that the RLR nanoparticles successfully achieved 99.4% and 99.0% tumor-killing rates, respectively through the synergistic action of CDT, photochemotherapy, and anticancer drugs on a single platform. These results show the potential of the RLR nanoparticle-based platform in multimodal tumor therapy.
3.2.3 PTT Combined With PDT and CDT
PDT and CDT are mainly ROS-mediated tumor therapy methods. Thus, combining them to construct an antitumor nanoplatform is a promising strategy to improve the antitumor effect (315, 316). Because PTT can improve the hypoxia of PDT, and CDT can inhibit the expression of HSP from PTT, the establishment of a PTT/PDT/CDT multimodal therapeutic platform has attracted attention. For example, Liu et al. (317) prepared biocompatible copper ferrite nanospheres (CFNs) (Figure 7). Under 650-nm laser irradiation, the Fenton reaction mediated by copper and iron ions was significant. Meanwhile, CFNs regulated the tumor microenvironment to enhance the therapeutic effect of PDT by promoting the production and consumption of GSH by oxygen. Under the irradiation of 808-nm laser, CFNs exhibited excellent photothermal conversion ability. The experimental results in vivo and in vitro also showed that when the two wavelengths of laser were irradiated at the same time, almost all tumor cells were killed and the tumors in tumor-bearing mice were eliminated, demonstrating an excellent synergistic antitumor effect.
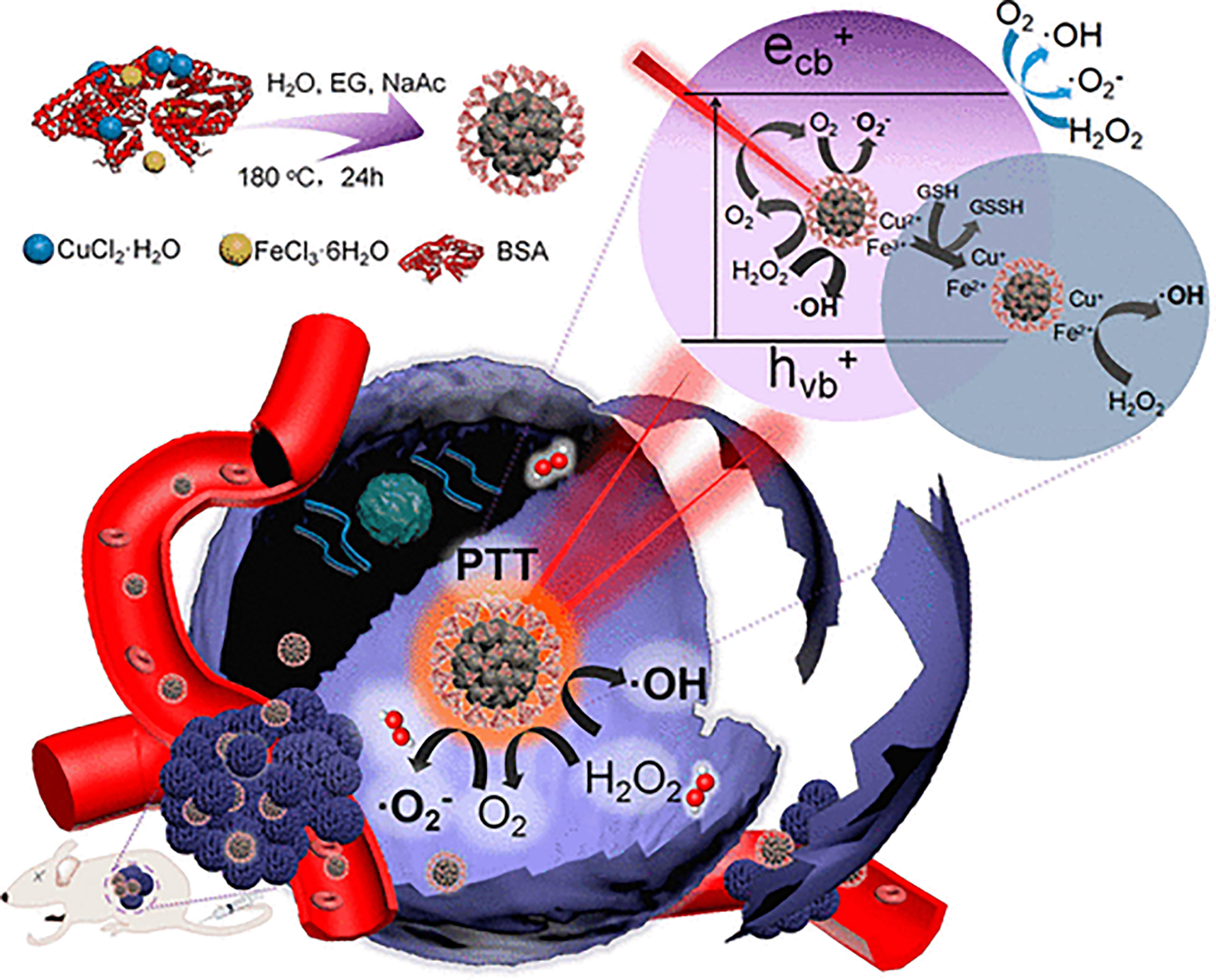
Figure 7 Schematic illustration of synthetic process and therapeutic mechanism of CFNs (317). Reproduced with permission from Liu et al., 2018.
3.2.4 PTT Combined With PDT and Gene Therapy
Studies have found that some specific types of siRNA (e.g., BAG3-siRNA) used in gene therapy can inhibit the expression of HSPs in cancer cells, which provides the possibility of gene therapy and PTT synergism (318). Among the nanomaterials that have been used to construct multifunctional platforms for tumor therapy and imaging, ultrathin BP nanosheets can not only act as photosensitizers for PDT by effectively promoting the production of large amounts of singlet oxygen (310), but also be widely used in PTT because of their excellent extinction coefficient and PCE (319). Therefore, the construction of a PTT/PDT/gene multimodal therapy nanosystem, based on a BP multifunctional nanoplatform, has aroused interest among researchers (320). Chen et al. (321) used PEG and polyethyleneimine-modified ultrathin BP nanoparticles (PPBP) as a human telomerase reverse transcriptase (hTERT) siRNA delivery system. When irradiated by different wavelengths of laser, PPBP nanoparticles showed excellent PDT and PTT activities, which further promoted the specific release of siRNA for gene silencing antitumor therapy. Experimental in vivo and in vitro results showed that compared with single-wavelength irradiation, when 660-nm and 808-nm lasers were irradiated together, the expression of hTERT mRNA in mice was significantly reduced and tumor growth was significantly inhibited under the action of PPBP-siRNA nanosheets. After 42 days of treatment, no obvious lung metastases were found. This study demonstrates that the PPBP-siRNA nanoplatform effectively inhibits tumor growth and metastasis through PTT/PDT/gene therapy synergism and verifies the feasibility of this multimodal therapy.
4 Conclusion and Outlook
Relying on the rapid development of nanoscience and polymer material technology, the research of photothermal nanomaterials in tumor treatment has made significant progress (322). Although the common inorganic and organic photothermal nanomaterials have great differences in their structures, they all have high PCE and tumor ablation capabilities. Nano phototherapy can not only directly kill tumor cells and reverse drug resistance, but also enhance immune responses (323). In addition, photothermal nanomaterials are becoming increasingly multifunctional through the modification of nanomaterials. Moreover, tumor treatment is becoming increasingly multimodal through the combination of various treatment methods, such as PTT and chemotherapy or PTT and PDT, which have achieved improved therapeutic effects. However, most of these methods are still in the laboratory stage, and these nanomaterials may have defects that limit their clinical application. Thus, the application of photothermal nanomaterials faces many challenges, such as:
1) Due to the different locations of tumor growth and tumor distances from the body surface, the tissue penetrated by laser irradiation has absorbs light, resulting in light weakening or extinction during PTT. In the future, more efforts should be focused on the research and development of PTAs with greater extinction coefficients. In addition, the application method of penetrating the body should also be developed to avoid laser attenuation, such as the administration of PTT at the same time as surgery.
2) The targeting of photothermal nanomaterials in the treatment of tumors, especially in temperature targeting, and avoidance of HSP should be improved. In the future, more attention should be paid to the modification of photothermal nanomaterials to achieve multifunctional uses. For example, treatment based on temperature sensitivity in combination with the application of pH-responsiveness can increase targeting capablities while realizing the multimodality of tumor treatment.
3)Although nanocarriers can co-assemble various therapeutic agents into a single system to build a multifunctional nanoplatform for tumor diagnosis, imaging, and multimodal therapy, the compatibility and composition of therapeutic agents in this technique require further exploration. For example, while PTT and PDT or CDT exert significant synergism, whether the proportion of PTAs and photosensitizer or chemodynamic drugs will inhibit the curative effect of synergistic therapy, as well as the optimal constituent ratio to achieve the best outcome have yet to be determined.
4)PTT is convenient for other treatment modes by virtue of its unique treatment principle; however, the deeper mechanism of the synergy between multimodal treatments remains to be explored. For example, PTT and PDT have an obvious synergistic effect, but the dominant modality has yet to be determined. In addition to the composition ratio of nanodrugs, whether promoting ROS production or alleviating tumor hypoxia is more significant, and how to design drug carriers to best exploit this synergism require further investigation.
5)Finally, the most important aspect is the biosafety of photothermal nanomaterials that remain in the body, which can administer toxicity to the human body. In addition, most solvents and chemicals in these nanomaterials are also harmful to the human body. Although nanomaterials may show low short-term cytotoxicity to cells, tissues, or organs, their long-term cytotoxicity and related immune reactions should be carefully evaluated. One possible method to reduce toxicity is to design biodegradable and cleanable PTAs. However, improving the biodegradability and clearance of PTAs may sacrifice their stability and retention time in the blood, resulting in reduced tumor uptake. Therefore, a balance must be achieved.
In all, photothermal nanomaterials in multimodal tumor therapy present great potential and will become increasingly beneficial with further research.
Author Contributions
FG contributed to the research retrieval and outline drafting. SC, XS and YT contributed to the research retrieval and drafting and critically revised the manuscript. YL, ZX and SZ critically revised the manuscript. Every author gave final approval and agreed to be accountable for all aspects of the work. All authors have read and agreed to the published version of the manuscript. All authors contributed to the article and approved the submitted version.
Funding
Financial support from the Projects directly under Jilin Provincial Department of Finance, China (JCSZ2019378-23), and the Scientific Research Project of Jilin Provincial Department of Education, China (JJKH20211215KJ).
Conflict of Interest
The authors declare that the research was conducted in the absence of any commercial or financial relationships that could be construed as a potential conflict of interest.
Publisher’s Note
All claims expressed in this article are solely those of the authors and do not necessarily represent those of their affiliated organizations, or those of the publisher, the editors and the reviewers. Any product that may be evaluated in this article, or claim that may be made by its manufacturer, is not guaranteed or endorsed by the publisher.
Acknowledgments
Authors are thankful to their respective departments/institutes/universities for providing space and other necessary facilities, which helped to draft this manuscript.
References
1. Sun XK. Construction of Multifunctional Composite Nanomaterials and Multi-Mode Tumor Therapy. [Dissertation Thesis]. China (JL): Jilin University (2019).
2. Das M, Mohanty C, Sahoo SK. Ligand-Based Targeted Therapy for Cancer Tissue. Expert Opin Drug Deliv (2009) 6(3):285–304. doi: 10.1517/17425240902780166
3. Mohanty C, Das M, Kanwar JR, Sahoo SK. Receptor Mediated Tumor Targeting: An Emerging Approach for Cancer Therapy. Curr Drug Deliv (2011) 8(1):45–58. doi: 10.2174/156720111793663606
4. Baumann M, Krause M, Hill R. Exploring the Role of Cancer Stem Cells in Radioresistance. Nat Rev Cancer (2008) 8(7):545–54. doi: 10.1038/nrc2419
5. Huang P, Wang D, Su Y, Huang W, Zhou Y, Cui D, et al. Combination of Small Molecule Prodrug and Nanodrug Delivery: Amphiphilic Drug-Drug Conjugate for Cancer Therapy. J Am Chem Soc (2014) 136(33):11748–56. doi: 10.1021/ja505212y
6. Kalbasi A, Komar C, Tooker GM, Liu M, Lee JW, Gladney WL, et al. Tumor-Derived CCL2 Mediates Resistance to Radiotherapy in Pancreatic Ductal Adenocarcinoma. Clin Cancer Res (2017) 23(1):137–48. doi: 10.1158/1078-0432.CCR-16-0870
7. Alzeibak R, Mishchenko TA, Shilyagina NY, Balalaeva IV, Vedunova MV, Krysko DV. Targeting Immunogenic Cancer Cell Death by Photodynamic Therapy: Past, Present and Future. J Immunother Cancer (2021) 9(1):e001926. doi: 10.1136/jitc-2020-001926
8. Kirillin M, Kurakina D, Khilov A, Orlova A, Shakhova M, Orlinskaya N, et al. Red and Blue Light in Antitumor Photodynamic Therapy With Chlorin-Based Photosensitizers: A Comparative Animal Study Assisted by Optical Imaging Modalities. BioMed Opt Express (2021) 12(2):872–92. doi: 10.1364/BOE.411518
9. Yim W, Borum RM, Zhou J, Mantri Y, Wu Z, Zhou J, et al. Ultrasmall Gold Nanorod-Polydopamine Hybrids for Enhanced Photoacoustic Imaging and Photothermal Therapy in Second Near-Infrared Window. Nanotheranostics (2022) 6(1):79–90. doi: 10.7150/ntno.63634
10. Li CL, Fisher CJ, Wilson BC, Weersink RA. Preclinical Evaluation of a Clinical Prototype Transrectal Diffuse Optical Tomography System for Monitoring Photothermal Therapy of Focal Prostate Cancer. J Biomed Opt (2022) 27(2):026001. doi:–10.1117/1.JBO.27.2.026001
11. Sun IC, Jo S, Dumani D, Yun WS, Yoon HY, Lim DK, et al. Theragnostic Glycol Chitosan-Conjugated Gold Nanoparticles for Photoacoustic Imaging of Regional Lymph Nodes and Delivering Tumor Antigen to Lymph Nodes. Nanomater (Basel) (2021) 11(7):1700. doi: 10.3390/nano11071700
12. Younis MR, Wang C, An R, Wang S, Younis MA, Li ZQ, et al. Low Power Single Laser Activated Synergistic Cancer Phototherapy Using Photosensitizer Functionalized Dual Plasmonic Photothermal Nanoagents. ACS Nano (2019) 13(2):2544–57. doi: 10.1021/acsnano.8b09552
13. Jain PK, Huang X, El-Sayed IH, El-Sayed MA. Noble Metals on the Nanoscale: Optical and Photothermal Properties and Some Applications in Imaging, Sensing, Biology, and Medicine. Acc Chem Res (2008) 41(12):1578–86. doi: 10.1021/ar7002804
14. Li J, Rao J, Pu K. Recent Progress on Semiconducting Polymer Nanoparticles for Molecular Imaging and Cancer Phototherapy. Biomaterials (2018) 155:217–35. doi: 10.1016/j.biomaterials.2017.11.025
15. Weissleder R. A Clearer Vision for In Vivo Imaging. Nat Biotechnol (2001) 19(4):316–7. doi: 10.1038/86684
16. Huang X, Zhang W, Guan G, Song G, Zou R, Hu J. Design and Functionalization of the NIR-Responsive Photothermal Semiconductor Nanomaterials for Cancer Theranostics. Acc Chem Res (2017) 50(10):2529–38. doi: 10.1021/acs.accounts.7b00294
17. Fiedler VU, Schwarzmaier HJ, Eickmeyer F, Müller FP, Schoepp C, Verreet PR. Laser-Induced Interstitial Thermotherapy of Liver Metastases in an Interventional 0.5 Tesla MRI System: Technique and First Clinical Experiences. J Magn Reson Imaging (2001) 13(5):729–37. doi: 10.1002/jmri.1101
18. Tian Q, Tang M, Sun Y, Zou R, Chen Z, Zhu M, et al. Hydrophilic Flower-Like CuS Superstructures as an Efficient 980 Nm Laser-Driven Photothermal Agent for Ablation of Cancer Cells. Adv Mater (2011) 23(31):3542–7. doi: 10.1002/adma.201101295
19. Li GC, Mivechi NF, Weitzel G. Heat Shock Proteins, Thermotolerance, and Their Relevance to Clinical Hyperthermia. Int J Hyperthermia (1995) 11(4):459–88. doi: 10.3109/02656739509022483
20. Luo Z. Construction of Nanocarriers for Photothermal/Chemotherapy Combined Cancer Treatment and Study on Their Anti-Tumor Mechanism. [Master’s Thesis]. China (TJ): Tianjin Univ Technol (2021)
21. Zhu Y, Zhao TJ, Liu M, Wang SW, Liu SL, Ai KL. Rheumatoid Arthritis Microenvironment Insights Into Treatment Effect of Nanomaterials. Nano Today (2022) 42:101358. doi: 10.1016/j.nantod.2021.101358
22. Zhao TJ, Wu W, Sui LH, Huang Q, Nan YY, Ai KL. Reactive Oxygen Species-Based Nanomaterials for the Treatment of Myocardial Ischemia Reperfusion Injuries. Bioactive Mater (2022) 7:42–72. doi: 10.1016/j.bioactmat.2021.06.006
23. Huang X, Jain PK, El-Sayed IH, El-Sayed MA. Plasmonic Photothermal Therapy (PPTT) Using Gold Nanoparticles. Lasers Med Sci (2008) 23(3):217–28. doi: 10.1007/s10103-007-0470-x
24. Doughty ACV, Hoover AR, Layton E, Murray CK, Howard EW, Chen WR. Nanomaterial Applications in Photothermal Therapy for Cancer. Mater (Basel) (2019) 12(5):779. doi: 10.3390/ma12050779
25. He YL, Cao YY, Wang YP. Progress on Photothermal Conversion in the Second NIR Window Based on Conjugated Polymers. Asian J Organic Chem (2018) 7(11):2201–12. doi: 10.1002/ajoc.201800450
26. Liu Y, Bhattarai P, Dai Z, Chen X. Photothermal Therapy and Photoacoustic Imaging via Nanotheranostics in Fighting Cancer. Chem Soc Rev (2019) 48(7):2053–108. doi: 10.1039/c8cs00618k
27. Xu Y, Liang X, Bhattarai P, Sun Y, Zhou Y, Wang S, et al. Enhancing Therapeutic Efficacy of Combined Cancer Phototherapy by Ultrasound-Mediated In Situ Conversion of Near-Infrared Cyanine/Porphyrin Microbubbles Into Nanoparticles. Adv Funct Mater (2017) 27(48):1704096. doi: 10.1002/adfm.201704096
28. Lin LS, Yang X, Zhou Z, Yang Z, Jacobson O, Liu Y, et al. Yolk-Shell Nanostructure: An Ideal Architecture to Achieve Harmonious Integration of Magnetic-Plasmonic Hybrid Theranostic Platform. Adv Mater (2017) 29(21):1606681. doi: 10.1002/adma.201606681
29. Wang Z, Huang P, Jacobson O, Wang Z, Liu Y, Lin L, et al. Biomineralization-Inspired Synthesis of Copper Sulfide-Ferritin Nanocages as Cancer Theranostics. ACS Nano (2016) 10(3):3453–60. doi: 10.1021/acsnano.5b07521
30. Choe HS, Shin MJ, Kwon SG, Lee H, Kim DK, Choi KU, et al. Yolk-Shell-Type Gold Nanoaggregates for Chemo- and Photothermal Combination Therapy for Drug-Resistant Cancers. ACS Appl Mater Interfaces (2021) 13(45):53519–29. doi: 10.1021/acsami.1c10036
31. Kim D, Amatya R, Hwang S, Lee S, Min KA, Shin MC. BSA-Silver Nanoparticles: A Potential Multimodal Therapeutics for Conventional and Photothermal Treatment of Skin Cancer. Pharmaceutics (2021) 13(4):575. doi: 10.3390/pharmaceutics13040575
32. Chen M, Chen S, He C, Mo S, Wang X, Liu G, et al. Safety Profile of Two-Dimensional Pd Nanosheets for Photothermal Therapy and Photoacoustic Imaging. Nano Res (2017) 10(4):1234–48. doi: 10.1007/s12274-016-1349-6
33. Zhou ZJ, Hu KW, Ma R, Yan Y, Ni B, Zhang YJ, et al. Dendritic Platinum-Copper Alloy Nanoparticles as Theranostic Agents for Multimodal Imaging and Combined Chemophotothermal Therapy. Adv Funct Mater (2016) 26(33):5971–8. doi: 10.1002/adfm.201601754
34. Liu Q, Liu L, Mo C, Zhou X, Chen D, He Y, et al. Polyethylene Glycol-Coated Ultrasmall Superparamagnetic Iron Oxide Nanoparticles-Coupled Sialyl Lewis X Nanotheranostic Platform for Nasopharyngeal Carcinoma Imaging and Photothermal Therapy. J Nanobiotech (2021) 19(1):171. doi: 10.1186/s12951-021-00918-0
35. Qiu N, Yang X, Zhang Y, Zhang J, Ji J, Zhang Y, et al. A Molybdenum Oxide-Based Degradable Nanosheet for Combined Chemo-Photothermal Therapy to Improve Tumor Immunosuppression and Suppress Distant Tumors and Lung Metastases. J Nanobiotech (2021) 19(1):428. doi: 10.1186/s12951-021-01162-2
36. Liang H, Xi H, Liu S, Zhang X, Liu H. Modulation of Oxygen Vacancy in Tungsten Oxide Nanosheets for Vis-NIR Light-Enhanced Electrocatalytic Hydrogen Production and Anticancer Photothermal Therapy. Nanoscale (2019) 11(39):18183–90. doi: 10.1039/c9nr06222j
37. Deng H, Yang Y, Zuo T, Fang T, Xu Y, Yang J, et al. Multifunctional ZnO@CuS Nanoparticles Cluster Synergize Chemotherapy and Photothermal Therapy for Tumor Metastasis. Nanomedicine (2021) 34:102399. doi: 10.1016/j.nano.2021.102399
38. Xu X, Zhang R, Yang X, Lu Y, Yang Z, Peng M, et al. A Honeycomb-Like Bismuth/Manganese Oxide Nanoparticle With Mutual Reinforcement of Internal and External Response for Triple-Negative Breast Cancer Targeted Therapy. Adv Healthc Mater (2021) 10(18):e2100518. doi: 10.1002/adhm.202100518
39. Huang B, Huang Y, Han H, Ge Q, Yang D, Hu Y, et al. An NIR-II Responsive Nanoplatform for Cancer Photothermal and Oxidative Stress Therapy. Front Bioeng Biotechnol (2021) 9:751757. doi: 10.3389/fbioe.2021.751757
40. Liu Q, Song P, Zhang W, Wang Z, Yang K, Luo J, et al. Acid-Sensitive Nanoparticles Based on Molybdenum Disulfide for Photothermal-Chemo Therapy. ACS Biomater Sci Eng (2022) 8(4):1706–16. doi: 10.1021/acsbiomaterials.1c01390
41. Fu Q, Li Z, Ye J, Li Z, Fu F, Lin SL, et al. Magnetic Targeted Near-Infrared II PA/MR Imaging Guided Photothermal Therapy to Trigger Cancer Immunotherapy. Theranostics (2020) 10(11):4997–5010. doi: 10.7150/thno.43604
42. Lu J, Wang K, Lei W, Mao Y, Di D, Zhao Q, et al. Polydopamine-Carbon Dots Functionalized Hollow Carbon Nanoplatform for Fluorescence-Imaging and Photothermal-Enhanced Thermochemotherapy. Mater Sci Eng C Mater Biol Appl (2021) 122:111908. doi: 10.1016/j.msec.2021.111908
43. Wang J, Su X, Zhao P, Gao D, Chen R, Wang L. Cancer Photothermal Therapy Based on Near Infrared Fluorescent CdSeTe/ZnS Quantum Dots. Analytical Methods (2021) 13(45):5509–15. doi: 10.1039/D1AY01635K
44. Zhao YN, Zhao TY, Cao YN, Sun J, Zhou Q, Chen HY, et al. Temperature-Sensitive Lipid-Coated Carbon Nanotubes for Synergistic Photothermal Therapy and Gene Therapy. ACS Nano (2021) 15(4):6517–29. doi: 10.1021/acsnano.0c08790
45. Xu ZR, Zhang YL, Zhou WX, Wang LJ, Xu GX, Ma MZ, et al. NIR-II-Activated Biocompatible Hollow Nanocarbons for Cancer Photothermal Therapy. J Nanobiotech (2021) 19(1):137. doi: 10.1186/s12951-021-00884-7
46. Dash BS, Lu Y-J, Chen H-A, Chuang C-C, Chen J-P. Magnetic and GRPR-Targeted Reduced Graphene Oxide/Doxorubicin Nanocomposite for Dual-Targeted Chemo-Photothermal Cancer Therapy. Mater Sci Eng: C (2021) 128:112311. doi: 10.1016/j.msec.2021.112311
47. Chen LL, Hong WZ, Duan SL, Li YP, Wang J, Zhu JM. Graphene Quantum Dots Mediated Magnetic Chitosan Drug Delivery Nanosystems for Targeting Synergistic Photothermal-Chemotherapy of Hepatocellular Carcinoma. Cancer Biol Ther (2022) 23(1):281–93. doi: 10.1080/15384047.2022.2054249
48. Lu Y, Zhang X, Hou X, Feng M, Cao Z, Liu J. Functionalized 2d Nb2C Nanosheets for Primary and Recurrent Cancer Photothermal/Immune-Therapy in the NIR-II Biowindow. Nanoscale (2021) 13(42):17822–36. doi: 10.1039/D1NR05126A
49. Peng FF, Zhao FX, Shan LW, Li RR, Jiang SS, Zhang P. Black Phosphorus Nanosheets-Based Platform for Targeted Chemo-Photothermal Synergistic Cancer Therapy. Colloids Surfaces B-Biointerfaces (2021) 198:111467. doi: 10.1016/j.colsurfb.2020.111467
50. Jin L, Liu J, Tang Y, Cao L, Zhang T, Yuan Q, et al. MnO2-Functionalized Co–P Nanocomposite: A New Theranostic Agent for pH-Triggered T1/T2 Dual-Modality Magnetic Resonance Imaging-Guided Chemo-Photothermal Synergistic Therapy. ACS Appl Mater Interfaces (2017) 9(48):41648–58. doi: 10.1021/acsami.7b10608
51. Zhang YH, Zhu XH, Zhang J, Wu YH, Liu JL, Zhang Y. Synergistic Upconversion Photodynamic and Photothermal Therapy Under Cold Near-Infrared Excitation. J Colloid Interface Sci (2021) 600:513–29. doi: 10.1016/j.jcis.2021.05.017
52. Liu R, Sang LH, Wang TY, Liu YH, Wang ZR, Li J, et al. Phase-Change Mesoporous Prussian Blue Nanoparticles for Loading Paclitaxel and Chemo-Photothermal Therapy of Cancer. Colloids Surfaces B-Biointerfaces (2021) 207:112018. doi: 10.1016/j.colsurfb.2021.112018
53. Guo H, Liu L, Hu Q, Dou H. Monodisperse ZIF-8@Dextran Nanoparticles Co-Loaded With Hydrophilic and Hydrophobic Functional Cargos for Combined Near-Infrared Fluorescence Imaging and Photothermal Therapy. Acta Biomater (2022) 137:290–304. doi: 10.1016/j.actbio.2021.10.006
54. Cao Y, Wei D, Yang L, Luo Z, Yu P, Li H, et al. Nanoplatform Self-Assembly From Small Molecules of Porphyrin Derivatives for NIR-II Fluorescence Imaging Guided Photothermal-Immunotherapy. Adv Healthc Mater (2022), e2102526. doi: 10.1002/adhm.202102526
55. Feng HY, Yuan Y, Zhang Y, Liu HJ, Dong X, Yang SC, et al. Targeted Micellar Phthalocyanine for Lymph Node Metastasis Homing and Photothermal Therapy in an Orthotopic Colorectal Tumor Model. Nanomicro Lett (2021) 13(1):145. doi: 10.1007/s40820-021-00666-8
56. Yu T, Zhang D, Wang J, Sun C, Cui T, Xu Z, et al. Near-Infrared Upper Phenyl-Fused BODIPY as a Photosensitizer for Photothermal-Photodynamic Therapy. J Mater Chem B (2022) 10(16):3048–54. doi: 10.1039/d2tb00012a
57. Zheng X, Bian S, Liu W, Zhang C, Wu J, Ren H, et al. Amphiphilic Diketopyrrolopyrrole Derivatives for Efficient Near-Infrared Fluorescence Imaging and Photothermal Therapy. ACS Omega (2021) 6(40):26575–82. doi: 10.1021/acsomega.1c03947
58. Wang F, Li J, Chen C, Qi H, Huang K, Hu S. Preparation and Synergistic Chemo-Photothermal Therapy of Redox-Responsive Carboxymethyl Cellulose/Chitosan Complex Nanoparticles. Carbohydr Polym (2022) 275:118714. doi: 10.1016/j.carbpol.2021.118714
59. Wu J, Zhang Y, Jiang K, Wang X, Blum NT, Zhang J, et al. Enzyme-Engineered Conjugated Polymer Nanoplatform for Activatable Companion Diagnostics and Multistage Augmented Synergistic Therapy. Adv Mater (2022) 34(18):e2200062. doi: 10.1002/adma.202200062
60. Liu S, Zhou X, Zhang H, Ou H, Lam JWY, Liu Y, et al. Molecular Motion in Aggregates: Manipulating TICT for Boosting Photothermal Theranostics. J Am Chem Soc (2019) 141(13):5359–68. doi: 10.1021/jacs.8b13889
61. Ko Y, Kim J, Jeong HY, Kwon G, Kim D, Ku M, et al. Antibacterial Poly (3,4-Ethylenedioxythiophene):Poly(Styrene-Sulfonate)/Agarose Nanocomposite Hydrogels With Thermo-Processability and Self-Healing. Carbohydr Polym (2019) 203:26–34. doi: 10.1016/j.carbpol.2018.09.026
62. Xu N, Hu A, Pu X, Wang J, Liao X, Huang Z, et al. Cu-Chelated Polydopamine Nanoparticles as a Photothermal Medium and "Immunogenic Cell Death" Inducer for Combined Tumor Therapy. J Mater Chem B (2022) 10(16):3104–18. doi: 10.1039/d2tb00025c
63. Xie Z, Lu R, Zhu Y, Peng M, Fan T, Ren P, et al. Liquid-Phase Exfoliation of Black Sesame to Create a Nanoplatform for In Vitro Photoluminescence and Photothermal Therapy. Nanomed (Lond) (2020) 15(21):2041–52. doi: 10.2217/nnm-2020-0151
64. Kang S, Baskaran R, Ozlu B, Davaa E, Kim JJ, Shim BS, et al. T1-Positive Mn2+-Doped Multi-Stimuli Responsive Poly(L-DOPA) Nanoparticles for Photothermal and Photodynamic Combination Cancer Therapy. Biomedicines (2020) 8(10):417. doi: 10.3390/biomedicines8100417
65. Odda AH, Cheang TY, Alesary HF, Liu L, Qian X, Ullah N, et al. A Multifunctional α-Fe2O3@PEDOT Core-Shell Nanoplatform for Gene and Photothermal Combination Anticancer Therapy. J Mater Chem B (2022) 10(9):1453–62. doi: 10.1039/d1tb02625a
66. Bai Y, Zhao J, Zhang L, Wang S, Hua J, Zhao S, et al. A Smart Near-Infrared Carbon Dot-Metal Organic Framework Assemblies for Tumor Microenvironment-Activated Cancer Imaging and Chemodynamic-Photothermal Combined Therapy. Adv Healthc Mater (2022), e2102759. doi: 10.1002/adhm.202102759
67. Lv Z, He S, Wang Y, Zhu X. Noble Metal Nanomaterials for NIR-Triggered Photothermal Therapy in Cancer. Adv Healthc Mater (2021) 10(6):e2001806. doi: 10.1002/adhm.202001806
68. Kim M, Lee JH, Nam JM. Plasmonic Photothermal Nanoparticles for Biomedical Applications. Adv Sci (Weinh) (2019) 6(17):1900471. doi: 10.1002/advs.201900471
69. Yao CP, Zhang LW, Wang J, He YL, Xin J, Wang SJ, et al. Gold Nanoparticle Mediated Phototherapy for Cancer. J Nanomater (2016) 2016:5497136. doi: 10.1155/2016/5497136
70. Amendoeira A, Garcia LR, Fernandes AR, Baptista PV. Light Irradiation of Gold Nanoparticles Toward Advanced Cancer Therapeutics. Advanced Ther (2020) 3(1):1900153. doi: 10.1002/adtp.201900153
71. Hu M, Chen J, Li ZY, Au L, Hartland GV, Li X, et al. Gold Nanostructures: Engineering Their Plasmonic Properties for Biomedical Applications. Chem Soc Rev (2006) 35(11):1084–94. doi: 10.1039/b517615h
72. Pivetta TP, Botteon CEA, Ribeiro PA, Marcato PD, Raposo M. Nanoparticle Systems for Cancer Phototherapy: An Overview. Nanomater (Basel) (2021) 11(11):3132. doi: 10.3390/nano11113132
73. Thompson EA, Graham E, MacNeill CM, Young M, Donati G, Wailes EM, et al. Differential Response of MCF7, MDA-MB-231, and MCF 10A Cells to Hyperthermia, Silver Nanoparticles and Silver Nanoparticle-Induced Photothermal Therapy. Int J Hyperthermia (2014) 30(5):312–23. doi: 10.3109/02656736.2014.936051
74. Huang X, Tang S, Mu X, Dai Y, Chen G, Zhou Z, et al. Freestanding Palladium Nanosheets With Plasmonic and Catalytic Properties. Nat Nanotechnol (2011) 6(1):28–32. doi: 10.1038/nnano.2010.235
75. Xiao JW, Fan SX, Wang F, Sun LD, Zheng XY, Yan CH. Porous Pd Nanoparticles With High Photothermal Conversion Efficiency for Efficient Ablation of Cancer Cells. Nanoscale (2014) 6(8):4345–51. doi: 10.1039/c3nr06843a
76. Huang X, Tang S, Liu B, Ren B, Zheng N. Enhancing the Photothermal Stability of Plasmonic Metal Nanoplates by a Core-Shell Architecture. Adv Mater (2011) 23(30):3420–5. doi: 10.1002/adma.201100905
77. Chen M, Tang S, Guo Z, Wang X, Mo S, Huang X, et al. Core-Shell Pd@Au Nanoplates as Theranostic Agents for in-Vivo Photoacoustic Imaging, CT Imaging, and Photothermal Therapy. Adv Mater (2014) 26(48):8210–6. doi: 10.1002/adma.201404013
78. Wei J, Li J, Sun D, Li Q, Ma J, Chen X, et al. A Novel Theranostic Nanoplatform Based on Pd@Pt-PEG-Ce6 for Enhanced Photodynamic Therapy by Modulating Tumor Hypoxia Microenvironment. Adv Funct Mater (2018) 28(17):1706310. doi: 10.1002/adfm.201706310
79. Tang S, Huang X, Zheng N. Silica Coating Improves the Efficacy of Pd Nanosheets for Photothermal Therapy of Cancer Cells Using Near Infrared Laser. Chem Commun (2011) 47(13):3948–50. doi: 10.1039/c1cc10451a
80. Zhu W, Chen M, Liu Y, Tian Y, Song Z, Song G, et al. A Dual Factor Activated Metal-Organic Framework Hybrid Nanoplatform for Photoacoustic Imaging and Synergetic Photo-Chemotherapy. Nanoscale (2019) 11(43):20630–7. doi: 10.1039/c9nr06349h
81. Liu Y, Li J, Chen M, Chen X, Zheng N. Palladium-Based Nanomaterials for Cancer Imaging and Therapy. Theranostics (2020) 10(22):10057–74. doi: 10.7150/thno.45990
82. Zhu YY, Li W, Zhao X, Zhou ZJ, Wang YT, Cheng YY, et al. Hyaluronic Acid-Encapsulated Platinum Nanoparticles for Targeted Photothermal Therapy of Breast Cancer. J BioMed Nanotechnol (2017) 13(11):1457–67. doi: 10.1166/jbn.2017.2446
83. Chen DH, Gao SP, Ge W, Li QW, Jiang H, Wang XM. One-Step Rapid Synthesis of Fluorescent Platinum Nanoclusters for Cellular Imaging and Photothermal Treatment. RSC Adv (2014) 4(76):40141–5. doi: 10.1039/c4ra07121b
84. Deng Y, Li E, Cheng X, Zhu J, Lu S, Ge C, et al. Facile Preparation of Hybrid Core-Shell Nanorods for Photothermal and Radiation Combined Therapy. Nanoscale (2016) 8(7):3895–9. doi: 10.1039/c5nr09102k
85. Zhao H, Xu J, Huang W, Zhan G, Zhao Y, Chen H, et al. Spatiotemporally Light-Activatable Platinum Nanocomplexes for Selective and Cooperative Cancer Therapy. ACS Nano (2019) 13(6):6647–61. doi: 10.1021/acsnano.9b00972
86. Chhowalla M, Shin HS, Eda G, Li LJ, Loh KP, Zhang H. The Chemistry of Two-Dimensional Layered Transition Metal Dichalcogenide Nanosheets. Nat Chem (2013) 5(4):263–75. doi: 10.1038/Nchem.1589
87. Wang C, Bai J, Liu YW, Jia XD, Jiang X. Polydopamine Coated Selenide Molybdenum: A New Photothermal Nanocarrier for Highly Effective Chemo-Photothermal Synergistic Therapy. ACS Biomater-Sci Eng (2016) 2(11):2011–7. doi: 10.1021/acsbiomaterials.6b00416
88. Zhu HJ, Lai ZC, Fang Y, Zhen X, Tan CL, Qi XY, et al. Ternary Chalcogenide Nanosheets With Ultrahigh Photothermal Conversion Efficiency for Photoacoustic Theranostics. Small (2017) 13(16):1604139. doi: 10.1002/smll.201604139
89. Li Z, Chen Y, Yang Y, Yu Y, Zhang Y, Zhu D, et al. Recent Advances in Nanomaterials-Based Chemo-Photothermal Combination Therapy for Improving Cancer Treatment. Front Bioeng Biotechnol (2019) 7:293. doi: 10.3389/fbioe.2019.00293
90. Gawande MB, Goswami A, Felpin FX, Asefa T, Huang X, Silva R, et al. Cu and Cu-Based Nanoparticles: Synthesis and Applications in Catalysis. Chem Rev (2016) 116(6):3722–811. doi: 10.1021/acs.chemrev.5b00482
91. Fernandes N, Rodrigues CF, Moreira AF, Correia IJ. Overview of the Application of Inorganic Nanomaterials in Cancer Photothermal Therapy. Biomater Sci (2020) 8(11):2990–3020. doi: 10.1039/d0bm00222d
92. Hessel CM, Pattani VP, Rasch M, Panthani MG, Koo B, Tunnell JW, et al. Copper Selenide Nanocrystals for Photothermal Therapy. Nano Lett (2011) 11(6):2560–6. doi: 10.1021/nl201400z
93. Lie SQ, Wang DM, Gao MX, Huang CZ. Controllable Copper Deficiency in Cu2-xSe Nanocrystals With Tunable Localized Surface Plasmon Resonance and Enhanced Chemiluminescence. Nanoscale (2014) 6(17):10289–96. doi: 10.1039/c4nr02294g
94. Zhu Z, Zou Y, Hu W, Li Y, Gu Y, Cao B, et al. Near-Infrared Plasmonic 2D Semimetals for Applications in Communication and Biology. Adv Funct Mater (2016) 26(11):1793–802. doi: 10.1002/adfm.201504884
95. Yuwen L, Zhou J, Zhang Y, Zhang Q, Shan J, Luo Z, et al. Aqueous Phase Preparation of Ultrasmall MoSe2 Nanodots for Efficient Photothermal Therapy of Cancer Cells. Nanoscale (2016) 8(5):2720–6. doi: 10.1039/c5nr08166a
96. Ding D, Guo W, Guo C, Sun J, Zheng N, Wang F, et al. MoO3-X Quantum Dots for Photoacoustic Imaging Guided Photothermal/Photodynamic Cancer Treatment. Nanoscale (2017) 9(5):2020–9. doi: 10.1039/c6nr09046j
97. Song G, Hao J, Liang C, Liu T, Gao M, Cheng L, et al. Degradable Molybdenum Oxide Nanosheets With Rapid Clearance and Efficient Tumor Homing Capabilities as a Therapeutic Nanoplatform. Angew Chem Int Ed Engl (2016) 55(6):2122–6. doi: 10.1002/anie.201510597
98. Wang JL, Sui LH, Huang J, Miao L, Nie YB, Ai KL. MoS2-Based Nanocomposites for Cancer Diagnosis and Therapy. Bioactive Mater (2021) 6(11):4209–42. doi: 10.1016/j.bioactmat.2021.04.021
99. Li S, Tan L, Xu W, Liu C, Wu Q, Fu C, et al. Doxorubicin-Loaded Layered MoS2 Hollow Spheres and Its Photothermo-Chemotherapy on Hepatocellular Carcinoma. J BioMed Nanotechnol (2017) 13(11):1557–64. doi: 10.1166/jbn.2017.2461
100. Jeong H, Park W, Kim DH, Na K. Dynamic Nanoassemblies of Nanomaterials for Cancer Photomedicine. Adv Drug Delivery Rev (2021) 177:113954. doi: 10.1016/j.addr.2021.113954
101. Estelrich J, Antònia Busquets M. Iron Oxide Nanoparticles in Photothermal Therapy. Molecules (2018) 23(7):1567. doi: 10.3390/molecules23071567
102. Maeng JH, Lee DH, Jung KH, Bae YH, Park IS, Jeong S, et al. Multifunctional Doxorubicin Loaded Superparamagnetic Iron Oxide Nanoparticles for Chemotherapy and Magnetic Resonance Imaging in Liver Cancer. Biomaterials (2010) 31(18):4995–5006. doi: 10.1016/j.biomaterials.2010.02.068
103. Hu J, Qian Y, Wang X, Liu T, Liu S. Drug-Loaded and Superparamagnetic Iron Oxide Nanoparticle Surface-Embedded Amphiphilic Block Copolymer Micelles for Integrated Chemotherapeutic Drug Delivery and MR Imaging. Langmuir (2012) 28(4):2073–82. doi: 10.1021/la203992q
104. Lai CW, Wang YH, Lai CH, Yang MJ, Chen CY, Chou PT, et al. Iridium-Complex-Functionalized Fe3O4/SiO2 Core/Shell Nanoparticles: A Facile Three-in-One System in Magnetic Resonance Imaging, Luminescence Imaging, and Photodynamic Therapy. Small (2008) 4(2):218–24. doi: 10.1002/smll.200700283
105. Ding J, Zhao J, Cheng K, Liu G, Xiu D. In Vivo Photodynamic Therapy and Magnetic Resonance Imaging of Cancer by TSPP-Coated Fe3O4 Nanoconjugates. J BioMed Nanotechnol (2010) 6(6):683–6. doi: 10.1166/jbn.2010.1165
106. Ma X, Tao H, Yang K, Feng L, Cheng L, Shi X, et al. A Functionalized Graphene Oxide-Iron Oxide Nanocomposite for Magnetically Targeted Drug Delivery, Photothermal Therapy, and Magnetic Resonance Imaging. Nano Res (2012) 5(3):199–212. doi: 10.1007/s12274-012-0200-y
107. Dong W, Li Y, Niu D, Ma Z, Gu J, Chen Y, et al. Facile Synthesis of Monodisperse Superparamagnetic Fe3O4 Core@Hybrid@Au Shell Nanocomposite for Bimodal Imaging and Photothermal Therapy. Adv Mater (2011) 23(45):5392–7. doi: 10.1002/adma.201103521
108. Zhu L, Zhou Z, Mao H, Yang L. Magnetic Nanoparticles for Precision Oncology: Theranostic Magnetic Iron Oxide Nanoparticles for Image-Guided and Targeted Cancer Therapy. Nanomed (Lond) (2017) 12(1):73–87. doi: 10.2217/nnm-2016-0316
109. Soetaert F, Korangath P, Serantes D, Fiering S, Ivkov R. Cancer Therapy With Iron Oxide Nanoparticles: Agents of Thermal and Immune Therapies. Adv Drug Delivery Rev (2020) 163-164:65–83. doi: 10.1016/j.addr.2020.06.025
110. Dhas N, Kudarha R, Garkal A, Ghate V, Sharma S, Panzade P, et al. Molybdenum-Based Hetero-Nanocomposites for Cancer Therapy, Diagnosis and Biosensing Application: Current Advancement and Future Breakthroughs. J Control Release (2021) 330:257–83. doi: 10.1016/j.jconrel.2020.12.015
111. Odda AH, Xu Y, Lin J, Wang G, Ullah N, Zeb A, et al. Plasmonic MoO3-X Nanoparticles Incorporated in Prussian Blue Frameworks Exhibit Highly Efficient Dual Photothermal/Photodynamic Therapy. J Mater Chem B (2019) 7(12):2032–42. doi: 10.1039/c8tb03148g
112. Bazaka K, Levchenko I, Lim JWM, Baranov O, Corbella C, Xu S, et al. MoS2-Based Nanostructures: Synthesis and Applications in Medicine. J Phys D. Appl Phys (2019) 52(18):183001. doi: 10.1088/1361-6463/ab03b3
113. Manthiram K, Alivisatos AP. Tunable Localized Surface Plasmon Resonances in Tungsten Oxide Nanocrystals. J Am Chem Soc (2012) 134(9):3995–8. doi: 10.1021/ja211363w
114. Guo W, Guo C, Zheng N, Sun T, Liu S. ). Csx WO3 Nanorods Coated With Polyelectrolyte Multilayers as a Multifunctional Nanomaterial for Bimodal Imaging-Guided Photothermal/Photodynamic Cancer Treatment. Adv Mater (2017) 29(4):1604157. doi: 10.1002/adma.201604157
115. Ghosh S, Khamarui S, Saha M, De SK. Fabrication of Tungsten Nanocrystals and Silver–Tungsten Nanonets: A Potent Reductive Catalyst. RSC Advances (2015) 5(49):38971–6. doi: 10.1039/c4ra16567e
116. Zuo H, Tao J, Shi H, He J, Zhou Z, Zhang C. Platelet-Mimicking Nanoparticles Co-Loaded With W18O49 and Metformin Alleviate Tumor Hypoxia for Enhanced Photodynamic Therapy and Photothermal Therapy. Acta Biomater (2018) 80:296–307. doi: 10.1016/j.actbio.2018.09.017
117. Yang ZY, Wang JF, Liu S, Sun F, Miao J, Xu E, et al. Tumor-Targeting W18O49 Nanoparticles for Dual-Modality Imaging and Guided Heat-Shock-Response-Inhibited Photothermal Therapy in Gastric Cancer. Particle Particle Syst Characterization (2019) 36(7):1900124. doi: 10.1002/ppsc.201900124
118. Zheng HD, Ou JZ, Strano MS, Kaner RB, Mitchell A, Kalantar-Zadeh K. Nanostructured Tungsten Oxide–Properties, Synthesis, and Applications. Advanced Funct Mater (2011) 21(12):2175–96. doi: 10.1002/adfm.201002477
119. Segets D, Gradl J, Taylor RK, Vassilev V, Peukert W. Analysis of Optical Absorbance Spectra for the Determination of ZnO Nanoparticle Size Distribution, Solubility, and Surface Energy. ACS Nano (2009) 3(7):1703–10. doi: 10.1021/nn900223b
120. Lou X, Shen H, Shen Y. Development of ZnO Series Ceramic Semiconductor Gas Sensors. J Sens Trans Technol (1991) 03:1–5. doi: 10.13873/j.1000-97871991.03.001
121. Nikoobakht B, Wang X, Herzing A, Shi J. Scalable Synthesis and Device Integration of Self-Registered One-Dimensional Zinc Oxide Nanostructures and Related Materials. Chem Soc Rev (2013) 42(1):342–65. doi: 10.1039/c2cs35164a
122. Chiua WS, Khiew PS, Clokea M, Isaa D, Tana TK, Radimanb S, et al. Photocatalytic Study of Two-Dimensional ZnO Nanopellets in the Decomposition of Methylene Blue. Chem Eng J (2010) 158(2):345−352. doi: 10.1016/j.cej.2010.01.052
123. Bitenc M, Orel ZC. Synthesis and Characterization of Crystalline Hexagonal Bipods of Zinc Oxide. Mater Res Bull (2009) 44(2):381−387. doi: 10.1016/j.materresbull.2008.05.005
124. Kołodziejczak-Radzimska A, Jesionowski T. Zinc Oxide-From Synthesis to Application: A Review. Mater (Basel) (2014) 7(4):2833–81. doi: 10.3390/ma7042833
125. Cai X, Zhu Q, Zeng Y, Zeng Q, Chen X, Zhan Y. Manganese Oxide Nanoparticles as Mri Contrast Agents in Tumor Multimodal Imaging and Therapy. Int J Nanomed (2019) 14:8321–44. doi: 10.2147/IJN.S218085
126. Liu Z, Zhang S, Lin H, Zhao M, Yao H, Zhang L, et al. Theranostic 2D Ultrathin MnO2 Nanosheets With Fast Responsibility to Endogenous Tumor Microenvironment and Exogenous NIR Irradiation. Biomaterials (2018) 155:54–63. doi: 10.1016/j.biomaterials.2017.11.015
127. Han B, Zhang YL, Chen QD, Sun HB. Carbon-Based Photothermal Actuators. Advanced Funct Mater (2018) 28(40):1802235. doi: 10.1002/adfm.201802235
128. Yu J, Lin YH, Yang L, Huang CC, Chen L, Wang WC, et al. Improved Anticancer Photothermal Therapy Using the Bystander Effect Enhanced by Antiarrhythmic Peptide Conjugated Dopamine-Modified Reduced Graphene Oxide Nanocomposite. Adv Healthc Mater (2017) 6(2):1600804. doi: 10.1002/adhm.201600804
129. Lim DJ, Sim M, Oh L, Lim K, Park H. Carbon-Based Drug Delivery Carriers for Cancer Therapy. Arch Pharm Res (2014) 37(1):43–52. doi: 10.1007/s12272-013-0277-1
130. Ding CQ, Zhu AW, Tian Y. Functional Surface Engineering of C-Dots for Fluorescent Biosensing and in Vivo Bioimaging. Acc Chem Res (2014) 47(1):20–30. doi: 10.1021/ar400023s
131. Du J, Xu N, Fan J, Sun W, Peng X. Carbon Dots for In Vivo Bioimaging and Theranostics. Small (2019) 15(32):e1805087. doi: 10.1002/smll.201805087
132. Zhang YJ, Sha R, Zhang L, Zhang WB, Jin PP, Xu WG, et al. Harnessing Copper-Palladium Alloy Tetrapod Nanoparticle-Induced Pro-Survival Autophagy for Optimized Photothermal Therapy of Drug-Resistant Cancer. Nat Commun (2018) 9:4236. doi: 10.1038/s41467-018-06529-y
133. Li DD, Zhang GB, Xu WG, Wang JX, Wang YC, Qiu LZ, et al. Investigating the Effect of Chemical Structure of Semiconducting Polymer Nanoparticle on Photothermal Therapy and Photoacoustic Imaging. Theranostics (2017) 7(16):4029–40. doi: 10.7150/thno.19538
134. Bilan R, Nabiev I, Sukhanova A. Quantum Dot-Based Nanotools for Bioimaging, Diagnostics, and Drug Delivery. Chembiochem (2016) 17(22):2103–14. doi: 10.1002/cbic.201600357
135. Yao J, Li L, Li P, Yang M. Quantum Dots: From Fluorescence to Chemiluminescence, Bioluminescence, Electrochemiluminescence, and Electrochemistry. Nanoscale (2017) 9(36):13364–83. doi: 10.1039/c7nr05233b
136. Yao J, Li PF, Li L, Yang M. Biochemistry and Biomedicine of Quantum Dots: From Biodetection to Bioimaging, Drug Discovery, Diagnostics, and Therapy. Acta Biomaterialia (2018) 74:36–55. doi: 10.1016/j.actbio.2018.05.004
137. Li LP, Zhang RP, Lu CX, Sun JH, Wang LJ, Qu BT, et al. In Situ Synthesis of NIR-Light Emitting Carbon Dots Derived From Spinach for Bio-Imaging Applications. J Mater Chem B (2017) 5(35):7328–34. doi: 10.1039/c7tb00634a
138. Zhang J, Hao GY, Yao CF, Yu JN, Wang J, Yang WT, et al. Albumin-Mediated Biomineralization of Paramagnetic NIR Ag2S QDs for Tiny Tumor Bimodal Targeted Imaging in Vivo. ACS Appl Mater Interfaces (2016) 8(26):16612–21. doi: 10.1021/acsami.6b04738
139. Iijima S. Helical Microtubules of Graphitic Carbon. Nature (1991) 354(6348):56–8. doi: 10.1038/354056a0
140. Augustine S, Singh J, Srivastava M, Sharma M, Das A, Malhotra BD. Recent Advances in Carbon Based Nanosystems for Cancer Theranostics. Biomater Sci (2017) 5(5):901–52. doi: 10.1039/c7bm00008a
141. Jaque D, Martínez Maestro L, del Rosal B, Haro-Gonzalez P, Benayas A, Plaza JL, et al. Nanoparticles for Photothermal Therapies. Nanoscale (2014) 6(16):9494–530. doi: 10.1039/C4NR00708E
142. He H, Pham-Huy LA, Dramou P, Xiao DL, Zuo PL, Pham-Huy C. Carbon Nanotubes: Applications in Pharmacy and Medicine. BioMed Res Int (2013) 2013:578290. doi: 10.1155/2013/578290
143. Iancu C, Mocan L. Advances in Cancer Therapy Through the Use of Carbon Nanotube-Mediated Targeted Hyperthermia. Int J Nanomed (2011) 6:1675–84. doi: 10.2147/ijn.S23588
144. Kam NWS, O'Connell M, Wisdom JA, Dai HJ. Carbon Nanotubes as Multifunctional Biological Transporters and Near-Infrared Agents for Selective Cancer Cell Destruction. Proc Natl Acad Sci United States America (2005) 102(33):11600–5. doi: 10.1073/pnas.0502680102
145. Wang LM, Sun Q, Wang X, Wen T, Yin JJ, Wang PY, et al. Using Hollow Carbon Nanospheres as a Light-Induced Free Radical Generator To Overcome Chemotherapy Resistance. J Am Chem Soc (2015) 137(5):1947–55. doi: 10.1021/ja511560b
146. Fang Y, Gu D, Zou Y, Wu ZX, Li FY, Che RC, et al. A Low-Concentration Hydrothermal Synthesis of Biocompatible Ordered Mesoporous Carbon Nanospheres With Tunable and Uniform Size. Angewandte Chemie-International Edition (2010) 49(43):7987–91. doi: 10.1002/anie.201002849
147. Li X, Liu C, Wang SY, Jiao J, Di DH, Jiang TY, et al. Poly(acrylic Acid) Conjugated Hollow Mesoporous Carbon as a Dual-Stimuli Triggered Drug Delivery System for Chemo-Photothermal Synergistic Therapy. Mater Sci Eng C-Materials Biol Appl (2017) 71:594–603. doi: 10.1016/j.msec.2016.10.037
148. Karavasili C, Amanatiadou EP, Sygellou L, Giasafaki DK, Steriotis TA, Charalambopoulou GC, et al. Development of New Drug Delivery System Based on Ordered Mesoporous Carbons: Characterisation and Cytocompatibility Studies. J Mater Chem B (2013) 1(25):3167–74. doi: 10.1039/c3tb20304b
149. Wang XD, Li X, Mao YL, Wang D, Zhao QF, Wang SL. Multi-Stimuli Responsive Nanosystem Modified by Tumor-Targeted Carbon Dots for Chemophototherapy Synergistic Therapy. J Colloid Interface Sci (2019) 552:639–50. doi: 10.1016/j.jcis.2019.05.085
150. Jin Y, Wang J, Ke H, Wang S, Dai Z. Graphene Oxide Modified PLA Microcapsules Containing Gold Nanoparticles for Ultrasonic/CT Bimodal Imaging Guided Photothermal Tumor Therapy. Biomaterials (2013) 34(20):4794–802. doi: 10.1016/j.biomaterials.2013.03.027
151. Chen YW, Chen PJ, Hu SH, Chen IW, Chen SY. NIR-Triggered Synergic Photo-Chemothermal Therapy Delivered by Reduced Graphene Oxide/Carbon/Mesoporous Silica Nanocookies. Adv Func Mater (2014) 24(4):451–9. doi: 10.1002/adfm.201301763
152. Hussein EA, Zagho MM, Nasrallah GK, Elzatahry AA. Recent Advances in Functional Nanostructures as Cancer Photothermal Therapy. Int J Nanomedicine (2018) 17(13):2897–906 doi: 10.2147/IJN.S161031
153. Kostarelos K, Novoselov KS. Exploring the Interface of Graphene and Biology. Science (2014) 344(6181):261–3. doi: 10.1126/science.1246736
154. Cheng C, Li S, Thomas A, Kotov NA, Haag R. Functional Graphene Nanomaterials Based Architectures: Biointeractions, Fabrications, and Emerging Biological Applications. Chem Rev (2017) 117(3):1826–914. doi: 10.1021/acs.chemrev.6b00520
155. Orecchioni M, Cabizza R, Bianco A, Delogu LG. Graphene as Cancer Theranostic Tool: Progress and Future Challenges. Theranostics (2015) 5(7):710–23. doi: 10.7150/thno.11387
156. de Melo-Diogo D, Costa EC, Alves CG, Lima-Sousa R, Ferreira P, Louro RO, et al. POxylated Graphene Oxide Nanomaterials for Combination Chemophototherapy of Breast Cancer Cells. Eur J Pharmaceutics Biopharmaceutics (2018) 131:162–9. doi: 10.1016/j.ejpb.2018.08.008
157. Moore TL, Podilakrishna R, Rao A, Alexis F. Systemic Administration of Polymer-Coated Nano-Graphene to Deliver Drugs to Glioblastoma. Particle Particle Syst Characterization (2014) 31(8):886–94. doi: 10.1002/ppsc.201300379
158. de Melo-Diogo D, Lima-Sousa R, Alves CG, Costa EC, Louro RO, Correia IJ. Functionalization of Graphene Family Nanomaterials for Application in Cancer Therapy. Colloids Surfaces B-Biointerfaces (2018) 171:260–75. doi: 10.1016/j.colsurfb.2018.07.030
159. de Melo-Diogo D, Pais-Silva C, Dias DR, Moreira AF, Correia IJ. Strategies to Improve Cancer Photothermal Therapy Mediated by Nanomaterials. Advanced Healthcare Mater (2017) 6(10):1700073. doi: 10.1002/adhm.201700073
160. Yao J, Sun Y, Yang M, Duan YX. Chemistry, Physics and Biology of Graphene-Based Nanomaterials: New Horizons for Sensing, Imaging and Medicine. J Mater Chem (2012) 22(29):14313–29. doi: 10.1039/c2jm31632c
161. Lin J, Chen XY, Huang P. Graphene-Based Nanomaterials for Bioimaging. Advanced Drug Delivery Rev (2016) 105:242–54. doi: 10.1016/j.addr.2016.05.013
162. Shim G, Kim MG, Park JY, Oh YK. Graphene-Based Nanosheets for Delivery of Chemotherapeutics and Biological Drugs. Advanced Drug Delivery Rev (2016) 105:205–27. doi: 10.1016/j.addr.2016.04.004
163. Jaleel JA, Sruthi S, Pramod K. Reinforcing Nanomedicine Using Graphene Family Nanomaterials. J Controlled Release (2017) 255:218–30. doi: 10.1016/j.jconrel.2017.04.041
164. Ponomarenko LA, Schedin F, Katsnelson MI, Yang R, Hill EW, Novoselov KS, et al. Chaotic Dirac Billiard in Graphene Quantum Dots. Science (2008) 320(5874):356–8. doi: 10.1126/science.1154663
165. Tade RS, Patil PO. Theranostic Prospects of Graphene Quantum Dots in Breast Cancer. ACS Biomater Sci Eng (2020) 6(11):5987–6008. doi: 10.1021/acsbiomaterials.0c01045
166. Fan HY, Yu XH, Wang K, Yin YJ, Tang YJ, Tang YL, et al. Graphene Quantum Dots (GQDs)-Based Nanomaterials for Improving Photodynamic Therapy in Cancer Treatment. Eur J Medicinal Chem (2019) 182:111620. doi: 10.1016/j.ejmech.2019.111620
167. Yao XX, Tian ZF, Liu JX, Zhu YF, Hanagata N. Mesoporous Silica Nanoparticles Capped With Graphene Quantum Dots for Potential Chemo-Photothermal Synergistic Cancer Therapy. Langmuir (2017) 33(2):591–9. doi: 10.1021/acs.langmuir.6b04189
168. Szuplewska A, Kulpinska D, Dybko A, Chudy M, Jastrzebska AM, Olszyna A, et al. Future Applications of MXenes in Biotechnology, Nanomedicine, and Sensors. Trends Biotechnol (2020) 38(3):264–79. doi: 10.1016/j.tibtech.2019.09.001
169. Wojciechowski T, Rozmysłowska-Wojciechowska A, Matyszczak G, Wrzecionek M, Olszyna A, Peter A, et al. Ti2C MXene Modified With Ceramic Oxide and Noble Metal Nanoparticles: Synthesis, Morphostructural Properties, and High Photocatalytic Activity. Inorganic Chem (2019) 58(11):7602–14. doi: 10.1021/acs.inorgchem.9b01015
170. Kalambate PK, Gadhari NS, Li X, Rao ZX, Navale ST, Shen Y, et al. Recent Advances in MXene-Based Electrochemical Sensors and Biosensors. Trac-Trends Analytical Chem (2019) 120:115643. doi: 10.1016/j.trac.2019.115643
171. Ronchi RM, Arantes JT, Santos SF. Synthesis, Structure, Properties and Applications of MXenes: Current Status and Perspectives. Ceramics Int (2019) 45(15):18167–88. doi: 10.1016/j.ceramint.2019.06.114
172. Iravani S, Varma RS. MXenes for Cancer Therapy and Diagnosis: Recent Advances and Current Challenges. ACS Biomater Sci Eng (2021) 7(6):1900–13. doi: 10.1021/acsbiomaterials.0c01763
173. Zhang JB, Fu Y, Mo AC. Multilayered Titanium Carbide MXene Film for Guided Bone Regeneration. Int J Nanomed (2019) 14:10091–102. doi: 10.2147/ijn.S227830
174. Shao JD, Xie HH, Huang H, Li ZB, Sun ZB, Xu YH, et al. Biodegradable Black Phosphorus-Based Nanospheres for In Vivo Photothermal Cancer Therapy. Nat Commun (2016) 7:12967. doi: 10.1038/ncomms12967
175. Chen L, Zhou GM, Liu ZB, Ma XM, Chen J, Zhang ZY, et al. Scalable Clean Exfoliation of High-Quality Few-Layer Black Phosphorus for a Flexible Lithium Ion Battery. Advanced Mater (2016) 28(3):510–+. doi: 10.1002/adma.201503678
176. Cheng L, Liu J, Gu X, Gong H, Shi X, Liu T, et al. PEGylated WS2 Nanosheets as a Multifunctional Theranostic Agent for In Vivo Dual-Modal CT/Photoacoustic Imaging Guided Photothermal Therapy. Adv Mater (2014) 26(12):1886–93. doi: 10.1002/adma.201304497
177. Qin L, Jiang SS, He HY, Ling GX, Zhang P. Functional Black Phosphorus Nanosheets for Cancer Therapy. J Controlled Release (2020) 318:50–66. doi: 10.1016/j.jconrel.2019.12.013
178. Liu Y, Zhen WY, Wang YH, Liu JH, Jin LH, Zhang TQ, et al. One-Dimensional Fe2P Acts as a Fenton Agent in Response to NIR II Light and Ultrasound for Deep Tumor Synergetic Theranostics. Angewandte Chemie-International Edition (2019) 58(8):2407–12. doi: 10.1002/anie.201813702
179. Huang KW, Hsu FF, Qiu JTT, Chern GJ, Lee YA, Chang CC, et al. Highly Efficient and Tumor-Selective Nanoparticles for Dual-Targeted Immunogene Therapy Against Cancer. Sci Adv (2020) 6(3):eaax5032. doi: 10.1126/sciadv.aax5032
180. Yao X, Yang B, Wang S, Dai Z, Zhang D, Zheng X, et al. A Novel Multifunctional FePt/BP Nanoplatform for Synergistic Photothermal/Photodynamic/Chemodynamic Cancer Therapies and Photothermally-Enhanced Immunotherapy. J Mater Chem B (2020) 8(35):8010–21. doi: 10.1039/D0TB00411A
181. Liu Y, Wang YH, Song SY, Zhang HJ. Tumor Diagnosis and Therapy Mediated by Metal Phosphorus-Based Nanomaterials. Advanced Mater (2021) 33(49):e2103936. doi: 10.1002/adma.202103936
182. Chen GY, Qiu HL, Prasad PN, Chen XY. Upconversion Nanoparticles: Design, Nanochemistry, and Applications in Theranostics. Chem Rev (2014) 114(10):5161–214. doi: 10.1021/cr400425h
183. Chatterjee DK, Rufaihah AJ, Zhang Y. Upconversion Fluorescence Imaging of Cells and Small Animals Using Lanthanide Doped Nanocrystals. Biomaterials (2008) 29(7):937–43. doi: 10.1016/j.biomaterials.2007.10.051
184. Idris NM, Li ZQ, Ye L, Sim EKW, Mahendran R, Ho PCL, et al. Tracking Transplanted Cells in Live Animal Using Upconversion Fluorescent Nanoparticles. Biomaterials (2009) 30(28):5104–13. doi: 10.1016/j.biomaterials.2009.05.062
185. Zhang F, Shi QH, Zhang YC, Shi YF, Ding KL, Zhao DY, et al. Fluorescence Upconversion Microbarcodes for Multiplexed Biological Detection: Nucleic Acid Encoding. Advanced Mater (2011) 23(33):3775–+. doi: 10.1002/adma.201101868
186. Liu YQ, Qin LY, Li HJ, Wang YX, Zhang R, Shi JM, et al. Application of Lanthanide-Doped Upconversion Nanoparticles for Cancer Treatment: A Review. Nanomedicine (2021) 16(24):2207–42. doi: 10.2217/nnm-2021-0214
187. Griffith WP. Cyanide Complexes of the Transition Metals. Q Rev Chem Soc (1962) 16(2):188–207. doi: 10.1039/QR9621600188
188. Adak S, Daemen LL, Hartl M, Williams D, Summerhill J, Nakotte H. Thermal Expansion in 3d-Metal Prussian Blue Analogs-A Survey Study. J Solid State Chem (2011) 184(11):2854–61. doi: 10.1016/j.jssc.2011.08.030
189. Long J, Guari Y, Guerin C, Larionova J. Prussian Blue Type Nanoparticles for Biomedical Applications. Dalton Trans (2016) 45(44):17581–7. doi: 10.1039/c6dt01299j
190. Wang D, Zhou J, Chen R, Shi R, Zhao G, Xia G, et al. Controllable Synthesis of Dual-MOFs Nanostructures for pH-Responsive Artemisinin Delivery, Magnetic Resonance and Optical Dual-Model Imaging-Guided Chemo/Photothermal Combinational Cancer Therapy. Biomaterials (2016) 100:27–40. doi: 10.1016/j.biomaterials.2016.05.027
191. Xiao F, Fan JL, Tond CY, Xiao C, Wang Z, Liu B, et al. An Erythrocyte Membrane Coated Mimetic Nano-Platform for Chemo-Phototherapy and Multimodal Imaging. Rsc Adv (2019) 9(48):27911–26. doi: 10.1039/c9ra05867b
192. Gautam M, Poudel K, Yong CS, Kim JO. Prussian Blue Nanoparticles: Synthesis, Surface Modification, and Application in Cancer Treatment. Int J Pharmaceutics (2018) 549(1-2):31–49. doi: 10.1016/j.ijpharm.2018.07.055
193. Busquets MA, Novella-Xicoy A, Guzman V, Estelrich J. Facile Synthesis of Novel Prussian Blue-Lipid Nanocomplexes. Molecules (2019) 24(22):4137. doi: 10.3390/molecules24224137
194. Cheng L, Wang C, Feng LZ, Yang K, Liu Z. Functional Nanomaterials for Phototherapies of Cancer. Chem Rev (2014) 114(21):10869–939. doi: 10.1021/cr400532z
195. Kowada T, Maeda H, Kikuchi K. BODIPY-Based Probes for the Fluorescence Imaging of Biomolecules in Living Cells. Chem Soc Rev (2015) 44(14):4953–72. doi: 10.1039/c5cs00030k
196. Guha S, Shaw SK, Spence GT, Roland FM, Smith BD. Clean Photothermal Heating and Controlled Release From Near-Infrared Dye Doped Nanoparticles Without Oxygen Photosensitization. Langmuir (2015) 31(28):7826–34. doi: 10.1021/acs.langmuir.5b01878
197. Song XJ, Chen Q, Liu Z. Recent Advances in the Development of Organic Photothermal Nano-Agents. Nano Res (2015) 8(2):340–54. doi: 10.1007/s12274-014-0620-y
198. Rajora MA, Lou JWH, Zheng G. Advancing Porphyrin's Biomedical Utility via Supramolecular Chemistry. Chem Soc Rev (2017) 46(21):6433–69. doi: 10.1039/c7cs00525c
199. Bhattarai P, Dai Z. Cyanine Based Nanoprobes for Cancer Theranostics. Adv Healthc Mater (2017) 6(14):1700262. doi: 10.1002/adhm.201700262
200. Sun W, Guo S, Hu C, Fan J, Peng X. Recent Development of Chemosensors Based on Cyanine Platforms. Chem Rev (2016) 116(14):7768–817. doi: 10.1021/acs.chemrev.6b00001
201. Luo S, Zhang E, Su Y, Cheng T, Shi C. A Review of NIR Dyes in Cancer Targeting and Imaging. Biomaterials (2011) 32(29):7127–38. doi: 10.1016/j.biomaterials.2011.06.024
202. Lan M, Zhao S, Liu W, Lee CS, Zhang W, Wang P. Photosensitizers for Photodynamic Therapy. Adv Healthc Mater (2019) 8(13):e1900132. doi: 10.1002/adhm.201900132
203. Zhou Y, Liang X, Dai Z. Porphyrin-Loaded Nanoparticles for Cancer Theranostics. Nanoscale (2016) 8(25):12394–405. doi: 10.1039/c5nr07849k
204. Lu K, He C, Lin W. Nanoscale Metal-Organic Framework for Highly Effective Photodynamic Therapy of Resistant Head and Neck Cancer. J Am Chem Soc (2014) 136(48):16712–5. doi: 10.1021/ja508679h
205. Liu K, Xing R, Zou Q, Ma G, Möhwald H, Yan X. Simple Peptide-Tuned Self-Assembly of Photosensitizers Towards Anticancer Photodynamic Therapy. Angew Chem Int Ed Engl (2016) 55(9):3036–9. doi: 10.1002/anie.201509810
206. Lu K, He C, Lin W. A Chlorin-Based Nanoscale Metal-Organic Framework for Photodynamic Therapy of Colon Cancers. J Am Chem Soc (2015) 137(24):7600–3. doi: 10.1021/jacs.5b04069
207. Lim CK, Shin J, Lee YD, Kim J, Oh KS, Yuk SH, et al. Phthalocyanine-Aggregated Polymeric Nanoparticles as Tumor-Homing Near-Infrared Absorbers for Photothermal Therapy of Cancer. Theranostics (2012) 2(9):871–9. doi: 10.7150/thno.4133
208. Zhao YY, Zhang L, Chen Z, Zheng BY, Ke M, Li X, et al. Nanostructured Phthalocyanine Assemblies With Efficient Synergistic Effect of Type I Photoreaction and Photothermal Action to Overcome Tumor Hypoxia in Photodynamic Therapy. J Am Chem Soc (2021) 143(34):13980–9. doi: 10.1021/jacs.1c07479
209. Mei L, Shi Y, Cao F, Liu X, Li XM, Xu Z, et al. PEGylated Phthalocyanine-Functionalized Graphene Oxide With Ultrahigh-Efficient Photothermal Performance for Triple-Mode Antibacterial Therapy. ACS Biomater Sci Eng (2021) 7(6):2638–48. doi: 10.1021/acsbiomaterials.1c00178
210. Turan IS, Yildiz D, Turksoy A, Gunaydin G, Akkaya EU. A Bifunctional Photosensitizer for Enhanced Fractional Photodynamic Therapy: Singlet Oxygen Generation in the Presence and Absence of Light. Angew Chem Int Ed Engl (2016) 55(8):2875–8. doi: 10.1002/anie.201511345
211. Kolemen S, Akkaya EU. Reaction-Based BODIPY Probes for Selective Bio-Imaging. Coordination Chem Rev (2018) 354:121–34. doi: 10.1016/j.ccr.2017.06.021
212. He L, Huang Y, Chang Y, You Y, Hu H, Leong KW, et al. A Highly Selective Dual-Therapeutic Nanosystem for Simultaneous Anticancer and Antiangiogenesis Therapy. J Mater Chem B (2017) 5(41):8228–37. doi: 10.1039/c7tb02163a
213. Chen HM, Zhang WZ, Zhu GZ, Xie J, Chen XY. Rethinking Cancer Nanotheranostics. Nat Rev Mater (2017) 2(7):17024. doi: 10.1038/natrevmats.2017.24
214. Shen G, Xing R, Zhang N, Chen C, Ma G, Yan X. Interfacial Cohesion and Assembly of Bioadhesive Molecules for Design of Long-Term Stable Hydrophobic Nanodrugs Toward Effective Anticancer Therapy. ACS Nano (2016) 10(6):5720–9. doi: 10.1021/acsnano.5b07276
215. Xu Y, Feng T, Yang T, Wei H, Yang H, Li G, et al. Utilizing Intramolecular Photoinduced Electron Transfer to Enhance Photothermal Tumor Treatment of Aza-BODIPY-Based Near-Infrared Nanoparticles. ACS Appl Mater Interfaces (2018) 10(19):16299–307. doi: 10.1021/acsami.8b03568
216. Sun W, Zhao X, Fan J, Du J, Peng X. Boron Dipyrromethene Nano-Photosensitizers for Anticancer Phototherapies. Small (2019) 15(32):e1804927. doi: 10.1002/smll.201804927
217. Ma C, Zhang T, Xie Z. Leveraging BODIPY Nanomaterials for Enhanced Tumor Photothermal Therapy. J Mater Chem B (2021) 9(36):7318–27. doi: 10.1039/d1tb00855b
218. Yang Z, Xu W, Wang J, Liu L, Chu Y, Wang Y, et al. Diketopyrrolopyrrole-Based Multifunctional Ratiometric Fluorescent Probe Andγ-Glutamyltranspeptidase-Triggered Activatable Photosensitizer for Tumor Therapy. J Mater Chem C. (2020) 8(24):8183–90. doi: 10.1039/d0tc01836h
219. Tang A, Zhan C, Yao J, Zhou E. Design of Diketopyrrolopyrrole (DPP)-Based Small Molecules for Organic-Solar-Cell Applications. Adv Mater (2017) 29(2):1600013. doi: 10.1002/adma.201600013
220. Cai Y, Liang P, Tang Q, Si W, Chen P, Zhang Q, et al. Diketopyrrolopyrrole-Based Photosensitizers Conjugated With Chemotherapeutic Agents for Multimodal Tumor Therapy. ACS Appl Mater Interfaces (2017) 9(36):30398–405. doi: 10.1021/acsami.7b09025
221. Deng W, Wu Q, Sun P, Yuan P, Lu X, Fan Q, et al. Zwitterionic Diketopyrrolopyrrole for Fluorescence/Photoacoustic Imaging Guided Photodynamic/Photothermal Therapy. Polym Chem (2018) 9(20):2805–12. doi: 10.1039/c8py00244d
222. Qian CG, Chen YL, Feng PJ, Xiao XZ, Dong M, Yu JC, et al. Conjugated Polymer Nanomaterials for Theranostics. Acta Pharmacol Sin (2017) 38(6):764–81. doi: 10.1038/aps.2017.42
223. Zhen X, Pu K, Jiang X. Photoacoustic Imaging and Photothermal Therapy of Semiconducting Polymer Nanoparticles: Signal Amplification and Second Near-Infrared Construction. Small (2021) 17(6):e2004723. doi: 10.1002/smll.202004723
224. Feng G, Zhang GQ, Ding D. Design of Superior Phototheranostic Agents Guided by Jablonski Diagrams. Chem Soc Rev (2020) 49(22):8179–234. doi: 10.1039/d0cs00671h
225. Xiao Z, Peng C, Jiang X, Peng Y, Huang X, Guan G, et al. Polypyrrole-Encapsulated Iron Tungstate Nanocomposites: A Versatile Platform for Multimodal Tumor Imaging and Photothermal Therapy. Nanoscale (2016) 8(26):12917–28. doi: 10.1039/c6nr03336a
226. Lu TY, Chiang CY, Fan YJ, Jheng PR, Quiñones ED, Liu KT, et al. Dual-Targeting Glycol Chitosan/Heparin-Decorated Polypyrrole Nanoparticle for Augmented Photothermal Thrombolytic Therapy. ACS Appl Mater Interfaces (2021) 13(8):10287–300. doi: 10.1021/acsami.0c20940
227. Zhou Y, Hu Y, Sun W, Zhou B, Zhu J, Peng C, et al. Polyaniline-Loaded γ-Polyglutamic Acid Nanogels as a Platform for Photoacoustic Imaging-Guided Tumor Photothermal Therapy. Nanoscale (2017) 9(34):12746–54. doi: 10.1039/c7nr04241h
228. Wang W, Wang L, Li Y, Liu S, Xie Z, Jing X. Nanoscale Polymer Metal-Organic Framework Hybrids for Effective Photothermal Therapy of Colon Cancers. Adv Mater (2016) 28(42):9320–5. doi: 10.1002/adma.201602997
229. Zhou J, Lu Z, Zhu X, Wang X, Liao Y, Ma Z, et al. NIR Photothermal Therapy Using Polyaniline Nanoparticles. Biomaterials (2013) 34(37):9584–92. doi: 10.1016/j.biomaterials.2013.08.075
230. Men X, Wang F, Chen HB, Liu YB, Men XX, Yuan Y, et al. Ultrasmall Semiconducting Polymer Dots with Rapid Clearance for Second Near-Infrared Photoacoustic Imaging and Photothermal Cancer Therapy. Advanced Funct Mater (2020) 30(24):Article 1909673. doi: 10.1002/adfm.201909673
231. Wei Z, Xue F, Xin F, Wu M, Wang B, Zhang X, et al. A Thieno-Isoindigo Derivative-Based Conjugated Polymer Nanoparticle for Photothermal Therapy in the NIR-II Bio-Window. Nanoscale (2020) 12(38):19665–72. doi: 10.1039/d0nr03771k
232. Khodagholy D, Doublet T, Gurfinkel MG, Quilichini P, Ismailova E, Leleux P, et al. Highly Conformable Conducting Polymer Electrodes for in Vivo Recordings. Adv Mater (2011) 23(36):H268–72. doi: 10.1002/adma.201102378
233. Vosgueritchian M, Lipomi DJ, Bao Z. Highly Conductive and Transparent PEDOT:PSS Films With a Fluorosurfactant for Stretchable and Flexible Transparent Electrodes. Adv Funct Mater (2011) 22(2):421–8. doi: 10.1002/adfm.201101775
234. Yun JM, Yeo JS, Kim J, Jeong HG, Kim DY, Noh YJ, et al. Solution-Processable Reduced Graphene Oxide as a Novel Alternative to PEDOT:PSS Hole Transport Layers for Highly Efficient and Stable Polymer Solar Cells. Adv Mater (2011) 23(42):4923–8. doi: 10.1002/adma.201102207
235. Jeong CJ, Sharker SM, In I, Park SY. Iron Oxide@PEDOT-Based Recyclable Photothermal Nanoparticles With Poly(Vinylpyrrolidone) Sulfobetaines for Rapid and Effective Antibacterial Activity. ACS Appl Mater Interfaces (2015) 7(18):9469–78. doi: 10.1021/acsami.5b02737
236. Khan S, Narula AK. Bio-Hybrid Blended Transparent and Conductive Films PEDOT: PSS:Chitosan Exhibiting Electro-Active and Antibacterial Properties. Eur Polymer J (2016) 81:161–72. doi: 10.1016/j.eurpolymj.2016.06.005
237. Ko Y, Kim D, Kim UJ, You J. Vacuum-Assisted Bilayer PEDOT:PSS/cellulose Nanofiber Compositefilm for Self-Standing,Flexible, Conductive Electrodes. Carbohydr Polymers (2017) 173:383–91. doi: 10.1016/j.carbpol.2017.05.096
238. Hong ZY, Feng HY, Bu LH. Melanin-Based Nanomaterials: The Promising Nanoplatforms for Cancer Diagnosis and Therapy. Nanomedicine (2020) 28:102211. doi: 10.1016/j.nano.2020.102211
239. Liu Y, Ai K, Liu J, Deng M, He Y, Lu L. Dopamine-Melanin Colloidal Nanospheres: An Efficient Near-Infrared Photothermal Therapeutic Agent for In Vivo Cancer Therapy. Adv Mater (2013) 25(9):1353–9. doi: 10.1002/adma.201204683
240. Liu Y, Ai K, Lu L. Polydopamine and its Derivative Materials: Synthesis and Promising Applications in Energy, Environmental, and Biomedical Fields. Chem Rev (2014) 114(9):5057–115. doi: 10.1021/cr400407a
241. Liu H, Yang Y, Liu Y, Pan J, Wang J, Man F, et al. Melanin-Like Nanomaterials for Advanced Biomedical Applications: A Versatile Platform With Extraordinary Promise. Adv Sci (2020) 7(7):1903129. doi: 10.1002/advs.201903129
242. Yue Y, Zhao X. Melanin-Like Nanomedicine in Photothermal Therapy Applications. Int J Mol Sci (2021) 22(1):399. doi: 10.3390/ijms22010399
243. Xiong J, Bian Q, Lei S, Deng Y, Zhao K, Sun S, et al. Bi19S27I3 Nanorods: A New Candidate for Photothermal Therapy in the First and Second Biological Near-Infrared Windows. Nanoscale (2021) 13(10):5369–82. doi: 10.1039/d0nr09137e
244. Zhou B, Li Y, Niu G, Lan M, Jia Q, Liang Q. Near-Infrared Organic Dye-Based Nanoagent for the Photothermal Therapy of Cancer. ACS Appl Mater Interfaces (2016) 8(44):29899–905. doi: 10.1021/acsami.6b07838
245. Yu CC, Xu LJ, Zhang YY, Timashev PS, Huang YY, Liang XJ. Polymer-Based Nanomaterials for Noninvasive Cancer Photothermal Therapy. ACS Appl Polym Mater (2020) 2(10):4289–305. doi: 10.1021/acsapm.0c00704
246. Hao MQ, Chen BB, Zhao XY, Zhao NN, Xu FJ. Organic/inorganic Nanocomposites for Cancer Immunotherapy. Mater Chem Front (2020) 4(9):2571–609. doi: 10.1039/d0qm00323a
247. Yu H, Chen M, Rice PM, Wang SX, White RL, Sun S. Dumbbell-Like Bifunctional Au–Fe3O4 Nanoparticles. Nano Lett (2005) 5(2):379–82. doi: 10.1021/nl047955q
248. Mezni A, Balti I, Mlayah A, Jouini N, Smiri LS. Hybrid Au-Fe3O4 Nanoparticles: Plasmonic, Surface Enhanced Raman Scattering, and Phase Transition Properties. J Phys Chem C. (2013) 117(31):16166–74. doi: 10.1021/jp4040826
249. Seaberg J, Montazerian H, Hossen MN, Bhattacharya R, Khademhosseini A, Mukherjee P. Hybrid Nanosystems for Biomedical Applications. ACS Nano (2021) 15(2):2099–142. doi: 10.1021/acsnano.0c09382
250. Park W, Shin H, Choi B, Rhim WK, Na K, Han DK. Advanced Hybrid Nanomaterials for Biomedical Applications. Prog Mater Sci (2020) 114:100686. doi: 10.1016/j.pmatsci.2020.100686
251. Ulbrich K, Hola K, Subr V, Bakandritsos A, Tucek J, Zboril R. Targeted Drug Delivery With Polymers and Magnetic Nanoparticles: Covalent and Noncovalent Approaches, Release Control, and Clinical Studies. Chem Rev (2016) 116(9):5338–431. doi: 10.1021/acs.chemrev.5b00589
252. Hogle WP. The State of the Art in Radiation Therapy. Semin Oncol Nurs (2006) 22(4):212–20. doi: 10.1016/j.soncn.2006.07.004
253. Zhang K, Chen HR, Li FQ, Wang Q, Zheng SG, Xu HX, et al. A Continuous Tri-Phase Transition Effect for HIFU-Mediated Intravenous Drug Delivery. Biomaterials (2014) 35(22):5875–85. doi: 10.1016/j.biomaterials.2014.03.043
254. Gao H, Liu R, Gao FP, Wang YL, Jiang XL, Gao XY. Plasmon-Mediated Generation of Reactive Oxygen Species From Near-Infrared Light Excited Gold Nanocages for Photodynamic Therapy in Vitro. ACS Nano (2014) 8(7):7260–71. doi: 10.1021/nn502325j
255. Wang C, Sun WJ, Wright G, Wang AZ, Gu Z. Inflammation-Triggered Cancer Immunotherapy by Programmed Delivery of CpG and Anti-PD1 Antibody. Advanced Mater (2016) 28(40):8912–20. doi: 10.1002/adma.201506312
256. Jayakumar MKG, Bansal A, Huang K, Yao R, Li BN, Zhang Y. Near-Infrared-Light-Based Nano-Platform Boosts Endosomal Escape and Controls Gene Knockdown in Vivo. ACS Nano (2014) 8(5):4848–58. doi: 10.1021/nn500777n
257. Canfarotta F, Piletsky SA. Engineered Magnetic Nanoparticles for Biomedical Applications. Adv Healthcare Mater (2014) 3(2):160–75. doi: 10.1002/adhm.201300141
258. Xue X, Fang T, Yin L, Jiang J, He Y, Dai Y, et al. Multistage Delivery of CDs-DOX/ICG-Loaded Liposome for Highly Penetration and Effective Chemo-Photothermal Combination Therapy. Drug Deliv (2018) 25(1):1826–39. doi: 10.1080/10717544.2018.1482975
259. Deng G, Zhu T, Zhou L, Zhang J, Li S, Sun Z, et al. Bovine Serum Albumin-Loaded Nano-Selenium/ICG Nanoparticles for Highly Effective Chemo-Photothermal Combination Therapy. RSC Adv (2017) 7(49):30717–24. doi: 10.1039/c7ra02384g
260. Valastyan S, Weinberg RA. Tumor Metastasis: Molecular Insights and Evolving Paradigms. Cell (2011) 147(2):275–92. doi: 10.1016/j.cell.2011.09.024
261. Dean M, Fojo T, Bates S. Tumour Stem Cells and Drug Resistance. Nat Rev Cancer (2005) 5(4):275–84. doi: 10.1038/nrc1590
262. Brown JM. Exploiting the Hypoxic Cancer Cell: Mechanisms and Therapeutic Strategies. Mol Med Today (2000) 6(4):157–62. doi: 10.1016/S1357-4310(00)01677-4
263. Xu C, Pu K. Second Near-Infrared Photothermal Materials for Combinational Nanotheranostics. Chem Soc Rev (2021) 50(2):1111–37. doi: 10.1039/d0cs00664e
264. Dong Q, Wang XW, Hu XX, Xiao LQ, Zhang L, Song LJ, et al. Simultaneous Application of Photothermal Therapy and an Anti-Inflammatory Prodrug Using Pyrene-Aspirin-Loaded Gold Nanorod Graphitic Nanocapsules. Angewandte Chemie-International Edition (2018) 57(1):177–81. doi: 10.1002/anie.201709648
265. Aggarwal BB, Vijayalekshmi RV, Sung B. Targeting Inflammatory Pathways for Prevention and Therapy of Cancer: Short-Term Friend, Long-Term Foe. Clin Cancer Res (2009) 15(2):425–30. doi: 10.1158/1078-0432.Ccr-08-0149
266. Piccolo MT, Menale C, Crispi S. Combined Anticancer Therapies: An Overview of the Latest Applications. Anti-Cancer Agents Medicinal Chem (2015) 15(4):408–22. doi: 10.2174/1871520615666150113123039
267. Grau C, Prakash Agarwal J, Jabeen K, Rab Khan A, Abeyakoon S, Hadjieva T, et al. Radiotherapy With or Without Mitomycin C in the Treatment of Locally Advanced Head and Neck Cancer: Results of the IAEA Multicentre Randomised Trial. Radiother Oncol (2003) 67(1):17–26. doi: 10.1016/S0167-8140(03)00020-3
268. Smith BR, Gambhir SS. Nanomaterials for In Vivo Imaging. Chem Rev (2017) 117(3):901–86. doi: 10.1021/acs.chemrev.6b00073
269. Brodin NP, Guha C, Tome WA. Photodynamic Therapy and Its Role in Combined Modality Anticancer Treatment. Technol Cancer Res Treat (2015) 14(4):355–68. doi: 10.1177/1533034614556192
270. Tian G, Zhang X, Gu ZJ, Zhao YL. Recent Advances in Upconversion Nanoparticles-Based Multifunctional Nanocomposites for Combined Cancer Therapy. Advanced Mater (2015) 27(47):7692–712. doi: 10.1002/adma.201503280
271. Sweeney EE, Cano-Mejia J, Fernandes R. Photothermal Therapy Generates a Thermal Window of Immunogenic Cell Death in Neuroblastoma. Small (2018) 14(20):e1800678. doi: 10.1002/smll.201800678
272. Zhang YX, Hou ZY, Ge YK, Deng KR, Liu B, Li XJ, et al. DNA-Hybrid-Gated Photothermal Mesoporous Silica Nanoparticles for MR-Responsive and Aptamer-Targeted Drug Delivery. ACS Appl Mater Interfaces (2015) 7(37):20696–706. doi: 10.1021/acsami.5b05522
273. Huang CC, Liu TM. Controlled Au-Polymer Nanostructures for Multiphoton Imaging, Prodrug Delivery, and Chemo-Photothermal Therapy Platforms. ACS Appl Mater Interfaces (2015) 7(45):25259–69. doi: 10.1021/acsami.5b07110
274. Wang XW, Gu YX, Li Q, Xu YP, Shi YF, Wang ZR, et al. Synergistic Chemo-Photothermal Cancer Therapy of pH-Responsive Polymeric Nanoparticles Loaded IR825 and DTX With Charge-Reversal Property. Colloids Surfaces B-Biointerfaces (2022) 209(Pt 2):112164. doi: 10.1016/j.colsurfb.2021.112164
275. Zhou FF, Wu S, Song S, Chen WR, Resasco DE, Xing D. Antitumor Immunologically Modified Carbon Nanotubes for Photothermal Therapy. Biomaterials (2012) 33(11):3235–42. doi: 10.1016/j.biomaterials.2011.12.029
276. Galluzzi L, Buqué A, Kepp O, Zitvogel L, Kroemer G. Immunogenic Cell Death in Cancer and Infectious Disease. Nat Rev Immunol (2017) 17(2):97–111. doi: 10.1038/nri.2016.107
277. Li J, Luo Y, Pu K. Electromagnetic Nanomedicines for Combinational Cancer Immunotherapy. Angew Chem Int Ed Engl (2020) 60(23):12682–705. doi: 10.1002/anie.202008386
278. Wang M, Li Y, Wang M, Liu KL, Hoover AR, Li M, et al. Synergistic Interventional Photothermal Therapy and Immunotherapy Using an Iron Oxide Nanoplatform for the Treatment of Pancreatic Cancer. Acta Biomaterialia (2022) 138:453–62. doi: 10.1016/j.actbio.2021.10.048
279. Kim J, Kim J, Jeong C, Kim WJ. Synergistic Nanomedicine by Combined Gene and Photothermal Therapy. Advanced Drug Delivery Rev (2016) 98:99–112. doi: 10.1016/j.addr.2015.12.018
280. Jung BK, Lee YK, Hong J, Ghandehari H, Yun CO. Mild Hyperthermia Induced by Gold Nanorod-Mediated Plasmonic Photothermal Therapy Enhances Transduction and Replication of Oncolytic Adenoviral Gene Delivery. ACS Nano (2016) 10(11):10533–43. doi: 10.1021/acsnano.6b06530
281. Xu M, Zhao D, Chen Y, Chen C, Zhang L, Sun L, et al. Charge Reversal Polypyrrole Nanocomplex-Mediated Gene Delivery and Photothermal Therapy for Effectively Treating Papillary Thyroid Cancer and Inhibiting Lymphatic Metastasis. ACS Appl Mater Interfaces (2022) 14(12):14072–86. doi: 10.1021/acsami.1c25179
282. Yao J, Zheng F, Yao C, Xu X, Akakuru OU, Chen T, et al. Rational Design of Nanomedicine for Photothermal-Chemodynamic Bimodal Cancer Therapy. Wiley Interdiscip Rev Nanomed Nanobiotechnol (2021) 13(3):e1682. doi: 10.1002/wnan.1682
283. Zhang C, Bu W, Ni D, Zhang S, Li Q, Yao Z, et al. Synthesis of Iron Nanometallic Glasses and Their Application in Cancer Therapy by a Localized Fenton Reaction. Angew Chem Int Ed Engl (2016) 55(6):2101–6. doi: 10.1002/anie.201510031
284. Sun SP, Li CJ, Sun JH, Shi SH, Fan MH, Zhou Q. Decolorization of an Azo Dye Orange G in Aqueous Solution by Fenton Oxidation Process: Effect of System Parameters and Kinetic Study. J Hazard Mater (2009) 161(2-3):1052–7. doi: 10.1016/j.jhazmat.2008.04.080
285. Yang Y, Zhu W, Dong Z, Chao Y, Xu L, Chen M, et al. 1d Coordination Polymer Nanofibers for Low-Temperature Photothermal Therapy. Adv Mater (2017) 29(40):1703588. doi: 10.1002/adma.201703588
286. Wu H, Liu L, Song L, Ma M, Gu N, Zhang Y. Enhanced Tumor Synergistic Therapy by Injectable Magnetic Hydrogel Mediated Generation of Hyperthermia and Highly Toxic Reactive Oxygen Species. ACS Nano (2019) 13(12):14013–23. doi: 10.1021/acsnano.9b06134
287. Huang H, Wang X, Wang W, Qu X, Song X, Zhang Y, et al. Injectable Hydrogel for Postoperative Synergistic Photothermal-Chemodynamic Tumor and Anti-Infection Therapy. Biomaterials (2022) 280:121289. doi: 10.1016/j.biomaterials.2021.121289
288. Castano AP, Demidova TN, Hamblin MR. Mechanisms in Photodynamic Therapy: Part Three-Photosensitizer Pharmacokinetics, Biodistribution, Tumor Localization and Modes of Tumor Destruction. Photodiagnosis Photodyn Ther (2005) 2(2):91–106. doi: 10.1016/S1572-1000(05)00060-8
289. Bhana S, Lin G, Wang L, Starring H, Mishra SR, Liu G, et al. Near-Infrared-Absorbing Gold Nanopopcorns With Iron Oxide Cluster Core for Magnetically Amplified Photothermal and Photodynamic Cancer Therapy. ACS Appl Mater Interfaces (2015) 7(21):11637–47. doi: 10.1021/acsami.5b02741
290. Melancon MP, Elliott AM, Shetty A, Huang Q, Stafford RJ, Li C. Near-Infrared Light Modulated Photothermal Effect Increases Vascular Perfusion and Enhances Polymeric Drug Delivery. J Control Release (2011) 156(2):265–72. doi: 10.1016/j.jconrel.2011.06.030
291. Sun X, Xu Y, Guo Q, Wang N, Wu B, Zhu C, et al. A Novel Nanoprobe for Targeted Imaging and Photothermal/Photodynamic Therapy of Lung Cancer. Langmuir (2022) 38(4):1360–7. doi: 10.1021/acs.langmuir.1c02434
292. Tachibana K, Feril L, Ikeda-Dantsuji Y. Sonodynamic Therapy. Ultrasonics (2008) 48(4):253–9. doi: 10.1016/j.ultras.2008.02.003
293. Son S, Kim JH, Wang X, Zhang C, Yoon SA, Shin J, et al. Multifunctional Sonosensitizers in Sonodynamic Cancer Therapy. Chem Soc Rev (2020) 49(11):3244–61. doi: 10.1039/c9cs00648f
294. Qian X, Zheng Y, Chen Y. Micro/Nanoparticle-Augmented Sonodynamic Therapy (SDT): Breaking the Depth Shallow of Photoactivation. Adv Mater (2016) 28(37):8097–129. doi: 10.1002/adma.201602012
295. Soratijahromi E, Mohammadi S, Dehdari Vais R, Azarpira N, Sattarahmady N. Photothermal/sonodynamic Therapy of Melanoma Tumor by a Gold/Manganese Dioxide Nanocomposite: In Vitro and In Vivo Studies. Photodiagnosis Photodyn Ther (2020) 31:101846. doi: 10.1016/j.pdpdt.2020.101846
296. Vijayaraghavan P, Liu CH, Vankayala R, Chiang CS, Hwang KC. Designing Multi-Branched Gold Nanoechinus for NIR Light Activated Dual Modal Photodynamic and Photothermal Therapy in the Second Biological Window. Adv Mater (2014) 26(39):6689–95. doi: 10.1002/adma.201400703
297. Zhao R, Han X, Li Y, Wang H, Ji T, Zhao Y, et al. Photothermal Effect Enhanced Cascade-Targeting Strategy for Improved Pancreatic Cancer Therapy by Gold Nanoshell@Mesoporous Silica Nanorod. ACS Nano (2017) 11(8):8103–13. doi: 10.1021/acsnano.7b02918
298. Baskar R, Lee KA, Yeo R, Yeoh KW. Cancer and Radiation Therapy: Current Advances and Future Directions. Int J Med Sci (2012) 9(3):193–9. doi: 10.7150/ijms.3635
299. Cheng L, Yuan C, Shen SD, Yi X, Gong H, Yang K, et al. Bottom-Up Synthesis of Metal-Ion-Doped WS2 Nanoflakes for Cancer Theranostics. ACS Nano (2015) 9(11):11090–101. doi: 10.1021/acsnano.5b04606
300. Chao Y, Wang GL, Liang C, Yi X, Zhong XY, Liu JJ, et al. Rhenium-188 Labeled Tungsten Disulfide Nanoflakes for Self-Sensitized, Near-Infrared Enhanced Radioisotope Therapy. Small (2016) 12(29):3967–75. doi: 10.1002/smll.201601375
301. Mao FX, Wen L, Sun CX, Zhang SH, Wang GL, Zeng JF, et al. Ultrasmall Biocompatible Bi2Se3 Nanodots for Multimodal Imaging-Guided Synergistic Radiophotothermal Therapy Against Cancer. ACS Nano (2016) 10(12):11145–55. doi: 10.1021/acsnano.6b06067
302. Li Q, Hang LF, Jiang W, Dou JX, Xiao L, Tang XF, et al. Pre- and Post-Irradiation Mild Hyperthermia Enabled by NIR-II for Sensitizing Radiotherapy. Biomaterials (2020) 257:120235. doi: 10.1016/j.biomaterials.2020.120235
303. Ni J, Xu H, Zhong Y, Zhou Y, Hu S. Activatable UCL/CT/MR-Enhanced In Vivo Imaging-Guided Radiotherapy and Photothermal Therapy. J Mater Chem B (2022) 10(4):549–61. doi: 10.1039/D1TB02006D
304. Yang F, Skripka A, Tabatabaei MS, Hong SH, Ren F, Benayas A, et al. Multifunctional Self-Assembled Supernanoparticles for Deep-Tissue Bimodal Imaging and Amplified Dual-Mode Heating Treatment. ACS Nano (2019) 13(1):408–20. doi: 10.1021/acsnano.8b06563
305. Lu Q, Lu T, Xu M, Yang L, Song Y, Li N. SO(2) Prodrug Doped Nanorattles With Extra-High Drug Payload for "Collusion Inside and Outside" Photothermal/pH Triggered - Gas Therapy. Biomaterials (2020) 257:120236. doi: 10.1016/j.biomaterials.2020.120236
306. Fan W, Shen B, Bu W, Chen F, Zhao K, Zhang S, et al. Rattle-Structured Multifunctional Nanotheranostics for Synergetic Chemo-/Radiotherapy and Simultaneous Magnetic/Luminescent Dual-Mode Imaging. J Am Chem Soc (2013) 135(17):6494–503. doi: 10.1021/ja312225b
307. Wang X, Chen H, Zhang K, Ma M, Li F, Zeng D, et al. An Intelligent Nanotheranostic Agent for Targeting, Redox-Responsive Ultrasound Imaging, and Imaging-Guided High-Intensity Focused Ultrasound Synergistic Therapy. Small (2014) 10(7):1403–11. doi: 10.1002/smll.201302846
308. Xie J, Lee S, Chen X. Nanoparticle-Based Theranostic Agents. Adv Drug Delivery Rev (2010) 62(11):1064–79. doi: 10.1016/j.addr.2010.07.009
309. Gong H, Cheng L, Xiang J, Xu H, Feng LZ, Shi XZ, et al. Near-Infrared Absorbing Polymeric Nanoparticles as a Versatile Drug Carrier for Cancer Combination Therapy. Advanced Funct Mater (2013) 23(48):6059–67. doi: 10.1002/adfm.201301555
310. Chen W, Ouyang J, Liu H, Chen M, Zeng K, Sheng J, et al. Black Phosphorus Nanosheet-Based Drug Delivery System for Synergistic Photodynamic/Photothermal/Chemotherapy of Cancer. Adv Mater (2017) 29(5):1603864. doi: 10.1002/adma.201603864
311. Tang ZM, Zhao PR, Ni DL, Liu YY, Zhang M, Wang H, et al. Pyroelectric Nanoplatform for NIR-II-Triggered Photothermal Therapy With Simultaneous Pyroelectric Dynamic Therapy. Mater Horizons (2018) 5(5):946–52. doi: 10.1039/c8mh00627j
312. Hu P, Wu T, Fan W, Chen L, Liu Y, Ni D, et al. Near Infrared-Assisted Fenton Reaction for Tumor-Specific and Mitochondrial DNA-Targeted Photochemotherapy. Biomaterials (2017) 141:86–95. doi: 10.1016/j.biomaterials.2017.06.035
313. He C, Liu D, Lin W. Self-Assembled Core-Shell Nanoparticles for Combined Chemotherapy and Photodynamic Therapy of Resistant Head and Neck Cancers. ACS Nano (2015) 9(1):991–1003. doi: 10.1038/s41565-019-0373-6
314. Wang S, Yang L, Cho HY, Dean Chueng ST, Zhang H, Zhang Q, et al. Programmed Degradation of a Hierarchical Nanoparticle With Redox and Light Responsivity for Self-Activated Photo-Chemical Enhanced Chemodynamic Therapy. Biomaterials (2019) 224:119498. doi: 10.1016/j.biomaterials.2019.119498
315. Zhuang X, Ma X, Xue X, Jiang Q, Song L, Dai L, et al. A Photosensitizer-Loaded DNA Origami Nanosystem for Photodynamic Therapy. ACS Nano (2016) 10(3):3486–95. doi: 10.1021/acsnano.5b07671
316. Shen Z, Song J, Yung BC, Zhou Z, Wu A, Chen X. Emerging Strategies of Cancer Therapy Based on Ferroptosis. Adv Mater (2018) 30(12):e1704007. doi: 10.1002/adma.201704007
317. Liu Y, Zhen W, Jin L, Zhang S, Sun G, Zhang T, et al. All-In-One Theranostic Nanoagent With Enhanced Reactive Oxygen Species Generation and Modulating Tumor Microenvironment Ability for Effective Tumor Eradication. ACS Nano (2018) 12(5):4886–93. doi: 10.1021/acsnano.8b01893
318. Du X, Zhao C, Zhou M, Ma T, Huang H, Jaroniec M, et al. Hollow Carbon Nanospheres With Tunable Hierarchical Pores for Drug, Gene, and Photothermal Synergistic Treatment. Small (2017) 13(6):1602592. doi: 10.1002/smll.201602592
319. Tao W, Zhu X, Yu X, Zeng X, Xiao Q, Zhang X, et al. Black Phosphorus Nanosheets as a Robust Delivery Platform for Cancer Theranostics. Adv Mater (2017) 29(1):1603276. doi: 10.1002/adma.201603276
320. Lei W, Liu G, Zhang J, Liu M. Black Phosphorus Nanostructures: Recent Advances in Hybridization, Doping and Functionalization. Chem Soc Rev (2017) 46(12):3492–509. doi: 10.1039/c7cs00021a
321. Chen L, Chen C, Chen W, Li K, Chen X, Tang X, et al. Biodegradable Black Phosphorus Nanosheets Mediate Specific Delivery of hTERT siRNA for Synergistic Cancer Therapy. ACS Appl Mater Interfaces (2018) 10(25):21137–48. doi: 10.1021/acsami.8b04807
322. Cheng K, Zhou J, Chen Y, Chen DZ. Progress of Stimulation Re-sponse Strategy Based on Nanomaterials in Tumor Therapy. Cancer Res Prev Treat (2019) 46(9):841–46 doi: 10.3971/j.issn.1000-8578.2019.19.0285
Keywords: photothermal therapy, nanomaterial, tumor, multimodal therapy, photothermal agents, synergistic effect
Citation: Shi X, Tian Y, Liu Y, Xiong Z, Zhai S, Chu S and Gao F (2022) Research Progress of Photothermal Nanomaterials in Multimodal Tumor Therapy. Front. Oncol. 12:939365. doi: 10.3389/fonc.2022.939365
Received: 09 May 2022; Accepted: 26 May 2022;
Published: 06 July 2022.
Edited by:
Kelong Ai, Central South University, ChinaReviewed by:
Peng Zhang, Nanjing Stomatological Hospital Medical School of Nanjing University, ChinaZheng Yang Li, Tianjin Stomatological Hospital, China
Copyright © 2022 Shi, Tian, Liu, Xiong, Zhai, Chu and Gao. This is an open-access article distributed under the terms of the Creative Commons Attribution License (CC BY). The use, distribution or reproduction in other forums is permitted, provided the original author(s) and the copyright owner(s) are credited and that the original publication in this journal is cited, in accordance with accepted academic practice. No use, distribution or reproduction is permitted which does not comply with these terms.
*Correspondence: Shunli Chu, Y2h1c2xAamx1LmVkdS5jbg==; Fengxiang Gao, Z2Z4MjZAY2lhYy5hYy5jbg==
†These authors have contributed equally to this work and share first authorship